- 1Department of Chemistry, Colorado State University, Fort Collins, CO, United States
- 2Department of Chemical and Biological Engineering, Colorado State University, Fort Collins, CO, United States
- 3School of Biomedical Engineering, Colorado State University, Fort Collins, CO, United States
- 4Metallurgy and Materials Science Research Institute, Chulalongkorn University, Bangkok, Thailand
Microfluidic paper-based analytical devices (µPADs) have gained significant attention in analytical science and technology due to their numerous advantages over traditional analytical techniques, including cost-effectiveness, miniaturization, and sustainability. µPADs are widely used in point-of-care diagnostics, health monitoring, environmental detection, food safety, forensics, and security. Since the first report in 2007, there have been substantial advancements in their fabrication techniques, detection methods, and applications. Over the years, significant efforts and advancements have been made to improve the cost-effectiveness, manufacturing scalability, user-friendliness, and sustainability of µPADs. In this review, we explore the general trends and advancements in the fabrication, sensing methods, and applications of µPADs, highlighting key improvements and innovations. We also examine the eco-friendliness of µPADs and present real-world success stories from field studies and citizen science initiatives. Additionally, we address the challenges associated with transitioning µPADs from the lab to the field. Finally, we examine the outlook for µPADs and propose strategies to improve their usefulness in various fields.
1 Introduction
For centuries, paper has been a workhorse in the world of analysis, serving primarily as a writing surface. However, its unique properties extend far beyond this familiar role. Recent advancements have revealed paper as a powerful tool for scientific detection due to its versatility and accessibility (Ozer et al., 2020). Paper is inexpensive and available all over the world, and transports aqueous fluids by capillary forces, offering a passive means of fluid transport without the need for active pumping mechanisms. Paper’s composition allows for a wide range of thicknesses, from the thin and lightweight sheets to the thicker and more robust cardboard. This versatility, combined with its stackability, storability, and transportability, makes it ideal for various applications. Due to its cellulose or cellulose-polymer composition, the paper has a natural advantage in working with biological samples, offering excellent absorbency and biocompatibility. Moreover, its chemical modifiability allows for versatile functionalization, facilitating covalent binding to proteins, nucleic acids, or small molecules. This adaptability broadens the scope of paper-based analytical systems (PADS) in diagnostics, environmental monitoring, and beyond (Noviana et al., 2021; Martinez et al., 2010). As shown in Figure 1, the recent decade has seen a surge in the popularity of paper-based analytical systems following the introduction of the first μPAD in 2007 (Martinez et al., 2007). This simple innovation showcased the potential of paper as a platform for low-cost and portable analysis, sparking its application across various fields.
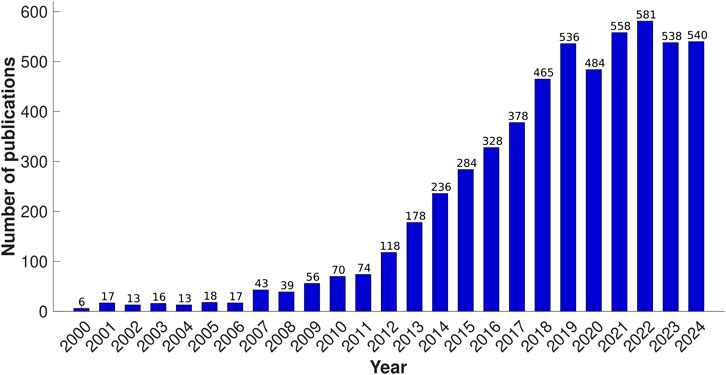
Figure 1. Number of publications involving paper-based microfluidics since 2000, derived from Web of Science search results using search criteria: “paper-based*” OR “patterned paper” OR “paper device*” AND “microfluidic*” (Title), as of 2 December 2024.
Many researchers have previously reviewed advancements in microfluidic systems, their commercialization, and their application in various diagnostic, health monitoring, food safety, environmental detection, forensics, etc. (Silva-Neto et al., 2023; Trinh et al., 2022; Han et al., 2020; Hao et al., 2021; Boobphahom et al., 2020; Aryal et al., 2024b; Kummari et al., 2023; Chen et al., 2024; Li and Feng, 2020; Pereira de Oliveira et al., 2018; Qi et al., 2020). Additionally, researchers have explored innovative fabrication techniques for μPADs, extending their use to non-conventional applications (Yamada et al., 2017a; Xia et al., 2016; Noviana et al., 2020a). Building on these recent advancements explored in prior works, this review offers a deeper analysis of overarching trends in microfluidic fabrication techniques, detection methodologies, and their practical applications. We provide a critical assessment of their benefits, drawbacks, and essential implementation considerations. Furthermore, we introduce a novel section exploring the sustainability of μPADs through the lens of the 12 Principles of Green Chemistry. To bridge the gap between research and real-world application, we recognize the challenges and their solutions in transitioning μPADs to practical use, highlighting successful outcomes from field studies and citizen science initiatives. Finally, we provide a future-oriented perspective on μPAD development, proposing methods to enhance their applicability and widespread adoption.
2 Advancements in fabrication techniques
The general principle behind designing µPADs involves creating a hydrophobic barrier to guide aqueous/hydrophilic liquids through capillary-driven cellulose microchannels. These channels contain a cellulose network with numerous -OH groups that interact strongly with aqueous or polar samples to facilitate the flow (Martinez et al., 2010). The resulting microchannels can be left open or sealed using transparent films, tapes, or thin polymer sheets. The mechanism behind fluid movement in paper-based devices generally adheres to the Washburn equation (Equation 1).
where l is the distance, γ is the surface tension, θ is the contact angle, η denotes the fluid viscosity, r is the average pore radius, and t is the time (Washburn, 1921). The rate of capillary wicking depends on the channel thickness and the purity of the cellulose. The thickness of the paper substrate determines the height of the microchannels, while the resolution and consistency of the hydrophobic barrier depend on the fabrication method (Martinez et al., 2010). The method chosen for patterning requires the best balance between cost, user-friendliness, and desired resolution.
Once the pattern is created, reagents specific to the assay are applied to the sensing region, and necessary transducers are attached. The sample is then added to the sample inlet, and the analyte travels to the sensing region through the hydrophilic microchannels. Here, it reacts with reagents to produce signals based on the detection technique. Additionally, the paper can be patterned to include pre-treatment steps such as masking and pH adjustments (Figure 2).
Over the years, significant advancements have occurred in the fabrication of paper-based analytical systems. In 1700s the paper was used as litmus paper tests for pH measurements, marking the early use of paper for analytical purposes (Banks and Watt, 1784). The 1900s saw the introduction of urine dipsticks, spot tests for colorimetric detection, chromatographic methods, and lateral flow assays, further expanding the applications of paper in diagnostics (West, 1945; Comer, 1956; Toennies and Kolb, 1951; Mark et al., 2010; Hawkes et al., 1982). In the late 2000s, groundbreaking fabrication techniques such as photolithography, inkjet printing, wax printing, and plasma treatment (Martinez et al., 2007; Abe et al., 2008; Li et al., 2008; Lu et al., 2009; Carrilho et al., 2009) were developed. These techniques significantly enhanced the precision and capabilities of µPADs.
In the early 2010s, techniques such as screen printing (Dungchai et al., 2011), flexographic printing (Olkkonen et al., 2010), lacquer spraying (Nurak et al., 2013), and laser treatment (Chitnis et al., 2011) advanced the field of paper-based microfluidics, significantly improving the accuracy and speed of these systems. From 2014 to 2016, innovations in digital paper microfluidics (Fobel et al., 2014), wet etching (Cai et al., 2014), flash foam stamp lithography (He et al., 2014), movable-type wax printing (Zhang et al., 2014), rubber stamping (Dornelas et al., 2015), and 3D-printed μPADs (He et al., 2016) marked a transformative shift towards more sophisticated, customizable, and versatile paper-based devices. Between 2017 and 2020, the introduction of laser printed µPADs (LP-µPADs) (Ghosh et al., 2019), correction pens (Mani et al., 2019), and hybrid photo paper-based microfluidic device (hPPMD) (Zargaryan et al., 2020) enabled the creation of more precise and defined patterns on paper substrates, enhancing the complexity of microchannels. More recently (2021-2024), innovations such as two-sided patterning (Monju et al., 2023), DIY dual μPADS (Sousa et al., 2023), acid-free paper-based analytical devices (Af-PADs) (Rawat et al., 2023), and superhydrophobic eggshells (Thangjitsirisin et al., 2024) appear to be promising avenues worth exploring for the development of μPADs.
Among these, particularly noteworthy platforms with significant potential include the incorporation of 3D printing technologies, the development of 3D layered paper-based systems, and the creation of laminate capillary-driven microfluidic systems (Park et al., 2018; Jang et al., 2021; de Oliveira et al., 2017). Additionally, processes like digital light processing have further refined the fabrication and functionality of µPADs (Amini et al., 2023). Looking forward, the field is poised for continued advancements, with an emphasis on even more sensitive, specific, and user-friendly systems. Figure 3 illustrates the timeline of fabrication techniques used for developing paper-based microfluidics. In this section, we will explore the most common fabrication methods in research, prototyping, and commercialization that have been predominantly used in the field.
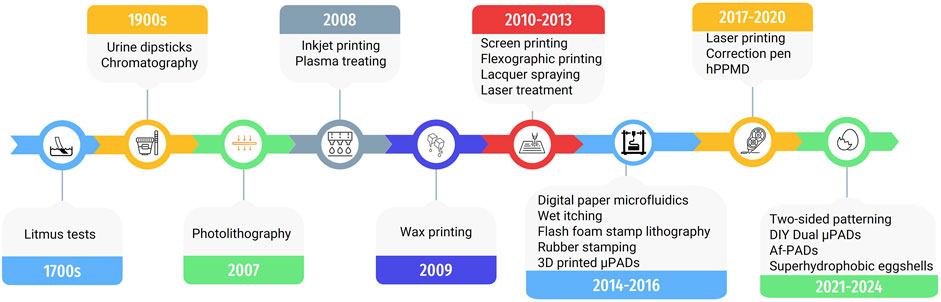
Figure 3. Timeline illustrating the development and advancements in fabrication methods for paper-based analytical devices.
2.1 Photolithography
Photolithography is a complex technique that works by transferring structural geometry from a photomask and was the first method used for patterning paper-based microfluidic systems (Martinez et al., 2007). This process begins by coating the substrate with photoresist material. Next, a pattern is designed on the substrate (Figure 4E). The substrate is then cured, and the uncured photoresist is removed using a solvent, leaving behind a cured photoresist barrier (He et al., 2015c).
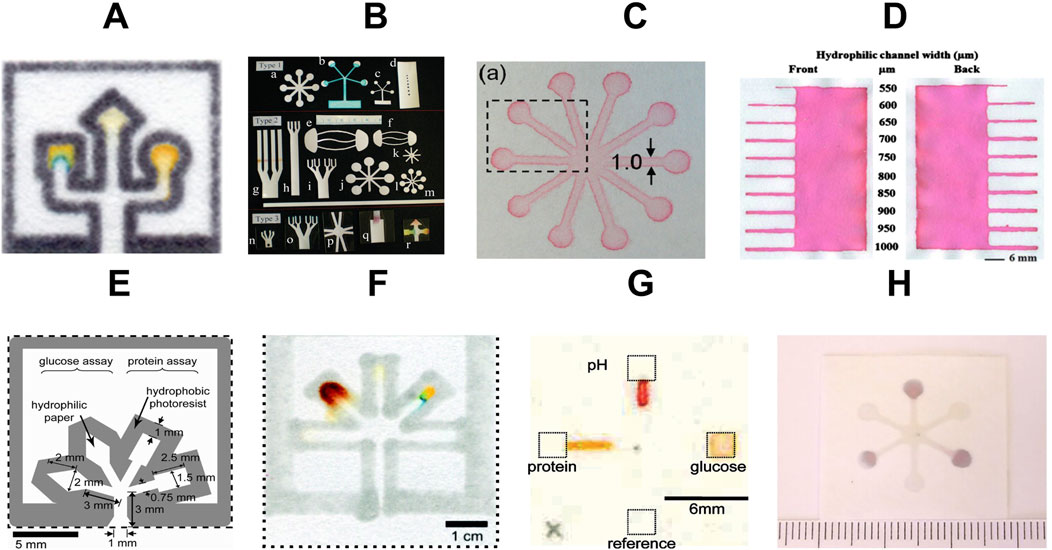
Figure 4. Common fabrication methods for paper-based microfluidc devices. (A) wax printing (adapted from (Carrilho et al., 2009) with permission, copyright 2009, American Chemical Society). (B) knife cutting (adapted from (Fenton et al., 2009) with permission, copyright 2009, American Chemical Society). (C) Flexographic printing (adapted from (Olkkonen et al., 2010) with permission, copyright 2009, American Chemical Society). (D) wax screen printing (Reproduced from (Dungchai et al., 2011) with permission from the Royal Society of Chemistry). (E) photolithography (adapted from (Martinez et al., 2008a) with permission, copyright 2008, American Chemical Society). (F) plotting (adapted from (Bruzewicz et al., 2008) with permission, copyright 2008, American Chemical Society). (G) inkjet printing (adapted from (Abe et al., 2008) with permission, copyright 2008, American Chemical Society). (H) plasma treatment (adapted from (Li et al., 2008) with permission, copyright 2008, American Chemical Society).
This technique can achieve the resolution with features as tiny as 100 μm or smaller (Gallatin and Liddle, 2024). This makes it excellent for designing complex devices. However, the process is time-consuming, involving several steps that can take hours to complete. Moreover, the requirement for extra instruments like a UV lamp, photomasks, spin coater, mask aligners, cleanroom facilities, hot plate, etc. can be problematic in terms of cost-effectiveness. While modern tools can speed up production for large-scale manufacturing, the cost is very high (up to millions) (Gallatin and Liddle, 2024). Furthermore, residue from the photoresist can cause colorimetric reactions and unwanted background (Dungchai et al., 2011). These expenses make photolithography less practical for small-scale projects or research with limited budgets. Despite these challenges, its precision and ability to handle large-scale production make it valuable for industries like semiconductor manufacturing. For μPADs, the high cost and complexity often outweigh its benefits in smaller projects.
Over the years, this technology has evolved from manual UV-lithography to faster alternatives such as FLASH (fast lithographic activation of sheets) (Martinez et al., 2008b), DMPC (dynamic mask photocuring) (He et al., 2015b), laser-based direct writing approaches (Songok et al., 2014), and utilizing the photocatalytic properties of TiO2 to fabricate µPADs (He et al., 2015a). These advancements have simplified the technique further while saving time and money, making it more suitable for high-frequency prototyping and manufacturing.
Despite the advancements in photolithography for µPADs, some key considerations remain crucial for successful µPAD development using this method. The first step is selecting a photoresist compatible with both the paper substrate and the intended chemical or biological assays. Next, it is essential to ensure the photomask design accurately reflects the desired microfluidic patterns, as high-resolution masks are vital for precise patterning. Finally, choosing solvents that effectively remove uncured photoresists without compromising the paper or the cured patterns is critical for achieving reliable and high-quality µPADs.
2.2 Plasma treatment
Plasma treatment is another commonly used technique for creating microchannel patterns on paper substrates. Initially, paper is hydrophobicized using materials such as octadecyl trichlorosilane (OTS), hexamethyldisilane, or fluorosilanes (Li et al., 2021; Lim et al., 2019). Following this, plasma treatment is performed under vacuum conditions. During plasma treatment, specific regions of the paper become hydrophilic, making channels for fluid flow (Figure 4H). The most common plasma-treated µPADs are created using a sizing agent that makes the paper more water-resistant (like AKD-heptane) (Li et al., 2008).
This technique achieves moderate to high resolution in creating hydrophobic barriers and hydrophilic channels on paper substrates, enabling the production of well-defined microfluidic patterns. One of its key strengths lies in the rapid treatment times, typically requiring only a few minutes to complete the process, which enhances efficiency in device fabrication. However, the adoption of plasma treatment faces significant barriers due to its high initial equipment costs. The specialized plasma generators and vacuum chambers necessary for this process often come with a substantial price tag, ranging from several thousand to tens of thousands of dollars (Ozer et al., 2020). This high upfront investment poses a considerable challenge, particularly for smaller laboratories or startups with limited resources. Additionally, these systems are not compatible with organic solvents. Since many biological and chemical assays require reagents that involve organic solvents, this limitation might pose an issue (He et al., 2015c). Consequently, while plasma treatment offers excellent control over surface properties and produces thermally and chemically stable barriers, its commercial potential remains limited. The requirement for expensive, specialized equipment and the need for vacuum conditions during processing makes it less accessible for widespread adoption, especially in resource-constrained settings or mass production scenarios. As a result, plasma treatment is more likely to find its niche in specialized research environments or high-end applications where the precision and quality of surface modification justify the high equipment costs. Nevertheless, efforts have been underway to develop portable systems that can create microplasmas, offering cheaper and more flexible alternatives that can be used under ambient conditions (Kao and Hsu, 2014).
When patterning paper with plasma treatment, several critical factors must be considered to ensure optimal device performance. The intensity and duration of plasma exposure influence the depth and degree of surface change, while the presence of sizing agents or other chemicals in the paper can affect treatment efficiency. Manufacturing challenges may arise from excessively intricate channel designs, potentially leading to issues like clogging or uneven flow. It's essential to use mask materials resistant to plasma and compatible with clean patterning to maintain channel clarity. Moreover, the surface chemistry post-plasma treatment should be compatible with subsequent treatments, such as biomolecule immobilization for additional device functionalities.
2.3 Inkjet printing
The fusion of paper-based microfluidics with printing technologies represents an important step in µPADs fabrication (Li et al., 2012b; Xia et al., 2016; Tortorich et al., 2018). In the inkjet printing method, hydrophobic barriers are applied onto paper using specialized hydrophobic inks such as Alkyl Ketene Dimer (AKD), Methylsilsesquioxane (MSQ), and Hexadecenyl succinic anhydride (HSA) (Akyazi et al., 2018). Following printing, these inks are cured either through UV exposure or heat treatment, creating high-resolution hydrophobic barriers (Yamada et al., 2015) as shown in Figure 4G. Inkjet printing has numerous advantages beyond channel creation. It serves as a versatile tool for depositing a range of substances, including colorimetric indicators, metal precursors, enzymes, ionophores, pH buffers, masking reagents, and blocking reagents, into both sensing and pretreatment regions (Tenda et al., 2016). This method ensures better accuracy in reagent volumes than manual pipetting techniques. Moreover, it facilitates accurate positioning and spatial confinement of deposited reagents, which is crucial for optimizing device performance. The continuous evolution of inkjet systems, such as Piezoelectric inkjet printing (e.g., Epson) and Thermal inkjet printing (e.g., Canon, Hewlett Packard), has further enhanced the capabilities and efficiency of this fabrication approach (Maejima et al., 2013; Yamada et al., 2015).
Inkjet printing is one of the most effective methods for developing μPADs, offering high resolution in the range of 50–200 μm. This level of precision enables the creation of detailed channel designs and intricate patterns, making it ideal for a variety of analytical applications. In terms of speed, inkjet printing is particularly efficient for small-scale production. Budget inkjet printers can achieve output rates of up to 15–25 pages per minute, making them well-suited for rapid prototyping and small-batch manufacturing. The technique is also cost-effective, striking a balance between affordability and functionality. Entry-level inkjet printers suitable for μPAD fabrication are available for as little as $30 to $100, while more advanced models for specialized tasks can cost up to $250. Operational expenses are reasonable as well, with replacement ink cartridges typically priced between $10 to $35. This combination of precision, speed, and cost-efficiency makes inkjet printing a versatile and accessible option for μPAD production.
When fabricating µPADs via inkjet printing, some factors must be considered. One concern is the potential formation of crystals or solid deposits at the printer nozzle, which can restrict the range of reagent concentrations feasible for use. Additionally, volatile reagents may evaporate too quickly, necessitating the use of less volatile alternatives (e.g., substituting cyclohexane for tetrahydrofuran). Another issue is the slow curing process, which can lead to low pattern resolution due to the spreading of reagents or hydrophobic ink. A potential solution for µPADs that require UV curing after printing is to immediately freeze them post-printing.
2.4 Wax printing/patterning
Since 2009, wax-patterned μPADs have been a popular choice. In these devices, the paper substrate is first patterned with wax to create channels that guide fluids. This initial patterning is followed by a heating step (using an oven, hot plate, or other hot surface) that melts the wax and allows it to penetrate the paper fibers, creating hydrophobic barriers that define hydrophilic channels (Dungchai et al., 2011). After cooling, the solidified wax creates the final design as shown in Figures 4A,D.
A key advantage of this method is the elimination of organic solvents during fabrication. Furthermore, wax patterning offers versatility, with techniques ranging from simple wax pens to commercial wax printers, screen printing, wax dipping, and even 3D printing of wax onto the paper surface (He et al., 2015c). The resolution achievable with wax printing ranges from hundreds of micrometers to centimeters, making it suitable for many analytical applications. This technique is highly efficient, offering fast production speeds ideal for mass manufacturing. Wax printing requires relatively low-cost wax materials, making it a cheaper alternative for resource-limited settings. Its simplicity and scalability provide high commercial potential, particularly for producing disposable μPADs for environmental or healthcare monitoring. However, commercial wax printers have been discontinued, so researchers are encouraged to explore alternative options such as thermal transfer printing, laser cutting, screen printing, photolithography, and laminate capillary-driven microfluidics (Ruiz et al., 2022; Le et al., 2017; Chiang et al., 2019).
2.5 Laser treatment
Many researchers use laser treatment in the making of µPADs (He et al., 2015c). The technique uses a CO₂ laser or such laser to create or remove material from the paper substrate. Using a laser’s power, speed, and focus, channel depth and width can be controlled. The laser creates these channels by vaporizing the material off the paper. As a result, the unetched areas stay hydrophilic, allowing liquids to flow through and giving the device its microfluidic properties (Ghosh et al., 2019; Sousa et al., 2024). In 2011, laser treatment was first adapted for fabricating μPADs by using a CO2 laser cutter to treat the surface of parchment paper (Chitnis et al., 2011).
Laser treatment technique achieves high resolution, capable of producing features smaller than 100 μm, which is particularly advantageous for applications requiring intricate channel designs or fine control over fluid flow. The process is relatively fast, especially for small-scale production, allowing for rapid prototyping and iteration of device designs. While the initial investment in laser equipment can be moderate to high, the operational costs are generally low, and the method offers good repeatability. The commercial potential of laser treatment is particularly notable for specialized applications that demand high-resolution features or complex geometries. Its non-contact nature and ability to process various paper substrates make it adaptable to different μPAD designs.
Recent advances in fabricating microfluidic paper-based analytical devices using a laser printer (LP-µPAD) have harnessed the precision of xerography-based commercial laser printers to create high-resolution microfluidic devices on paper (Ghosh et al., 2019). This direct printing procedure automates the deposition of hydrophobic toner ink, eliminating human interference and fabrication irregularities (Ghosh et al., 2019). Moreover, utilizing silver nanowire (AgNW) ink patterned with a solvent-free transfer method is also a promising alternative (Huang et al., 2016).
2.6 Other methods
In addition to these popular methods, various techniques have evolved over the years for the fabrication of µPADs. One commonly used technique is flexographic printing (Figure 4C), where hydrophobic barriers or inks are printed on paper using volatile organic compounds or polystyrene ink (Olkkonen et al., 2010). This is one of the fastest µPAD fabrication techniques but struggles with design flexibility. It also requires multiple printing optimization methods to achieve higher resolution, making it less suitable for lab prototyping but holding potential for commercial manufacturing.
Cutting and shaping to create 2D and 3D µPADs have also been adopted to develop instrument-free fabrications (Figure 4B). This technique involves using handheld blades, biopsy punches, knife cutting, laser cutting, etc. (Zhong et al., 2024). It is economical and advantageous because it does not require any chemical-laden instrumentation. Since no wax, organic solvents, or inks are used, there is a better chance of achieving higher resolution. However, the technique is limited to specific designs because boundaries cannot be defined as precisely as with wax or solvent lining.
Wet etching is another method, where hydrophobic paper is etched with a specific pattern (Cai et al., 2014). It starts with preparing the substrate, which includes cleaning and putting a protective mask or pattern (e.g., trimethyloctadecylsilane (TMOS)) on it. This mask defines the areas that will be unaffected by etching. The substrate is then immersed in an etchant solution (e.g., NaOH), which selectively reacts with the exposed portions based on the mask design. The etchant dissolves or changes the substance in these locations while leaving the masked sections unaffected, creating microfluidic channels. After the desired etching time, the mask is normally removed, and the substrate is cleaned to eliminate any remaining chemicals.
Stacking and origami techniques are also useful methods for developing µPADs (Kaewarsa et al., 2021; Das et al., 2019). These techniques enable multi-step chemical and biological assays. Folding, sliding, and rotating can be used to automate multiple steps in these systems (Carrell et al., 2019; Yakoh et al., 2022; Verma et al., 2018). Furthermore, chemical vapor-phase deposition, corona treatment, chemical modifications, stamping, plotting (Figure 4F), and lacquer spraying methods have also been employed to develop µPADs (Demirel and Babur, 2014; Jiang et al., 2016; Curto et al., 2013; Liu et al., 2017; Shakeri et al., 2019). Figure 4 shows the common fabrication methods for creating patterns on paper-based microfluidic devices.
Table 1 shows the summary of fabrication methods for µPADs. This table compares various techniques based on their suitability for mass production, ease of use, and resource requirements. Among these methods, wax printing, inkjet printing, and flexographic printing show the most promise for commercial applications due to their balance of resolution, speed, and cost-effectiveness. Laser treatment is emerging as a versatile method for high-resolution fabrication. Photolithography, while offering high resolution, faces challenges in large-scale adoption due to its complexity and cost. Screen printing remains relevant for certain applications due to its simplicity and moderate resolution. Plasma treatment, plotting, and wet etching have more limited commercial potential due to various constraints in scalability or process complexity. Therefore, for large-scale production, techniques such as inkjet and flexographic printing are recommended, while more accessible methods like wax printing and plotting are ideal for beginners with limited resources.
In addition to the aforementioned factors, the choice of recognition elements for μPADs is crucial, as their compatibility with the fabrication method directly affects the device’s sensitivity and specificity. For wax printing, thermally stable and polar recognition elements are ideal. Reagents requiring organic solvents like DMSO or chloroform should be avoided, as they can dissolve the wax barrier. Photolithography benefits from UV-resistant and stable materials. For screen printing, recognition elements that integrate well with conductive inks are ideal. Recognition elements that are heat-tolerant and stable under high-energy laser processing are essential for laser-treated μPADs. For inkjet printing, recognition elements compatible with low-viscosity ink formulations are essential. Additionally, the elements should be stable in liquid form and maintain their functional properties after deposition. For plotting, elements that can interact effectively with hydrophilic surfaces are ideal. For wet etching, recognition elements must be compatible with chemical solvents and porous substrates, remaining stable in the presence of acids or bases and retaining their functionality throughout the etching process. Most of these physical properties of elements are considered if they are pretreated on substrates before the fabrication process. On the other hand, chemical properties such as solvent compatibility, stability, and potential interactions with the hydrophobic barrier are crucial factors that need to be carefully studied before using a fabrication technique, regardless of pre- or post-treatment.
3 Advancements in sensing methods
In addition to the fabrication technique, when building paper-based microfluidic devices, it is critical to choose the suitable detection technique for the intended application. Colorimetry, fluorescence, electrochemical techniques, and chemiluminescence are some of the most used detection methods. Each method differs in terms of sensitivity and the complexity of the required detection system, which affects its applicability for diverse applications (Yamada et al., 2017a). In this section, we will explore the most frequently used detection techniques, and their common practices, and delve into their distinct advantages and limitations.
3.1 Colorimetry
Colorimetry is a commonly used method to generate signals in µPADs because the analyte concentration can be directly or indirectly correlated with the color intensity. Additionally, the color can be quantified using various portable platforms. There are numerous established colorimetric chemistries, including metal-ligand complexation, enzyme-inhibition assays, acid-base indicators, and redox indicators (Yamada et al., 2017a). Often, these signals are distinguishable enough to allow for semi-quantitative and qualitative observations, as seen in pregnancy tests, pH strips, and home water test kits (Morbioli et al., 2017).
Advancements in colorimetric detection have led to innovations ranging from simple spot tests to distance-based detection, where the distance of the colored signal is proportional to the analyte concentration, making it a valuable tool for semi-quantitative analysis (Cate et al., 2015; Cate et al., 2013). Furthermore, other methods such as time-based quantitative color readouts, counting-based readouts, barcode-like readouts, text-displaying readouts (Figure 5A), and direct result readouts have been developed over the years (Karita and Kaneta, 2014; Guan et al., 2014; Li and Macdonald, 2016; Lewis et al., 2012; Lewis et al., 2013; Zhang et al., 2015; Li et al., 2012a). These innovative techniques enable calibration-free assays, enhancing the utility and accuracy of colorimetric detection in paper-based microfluidics.
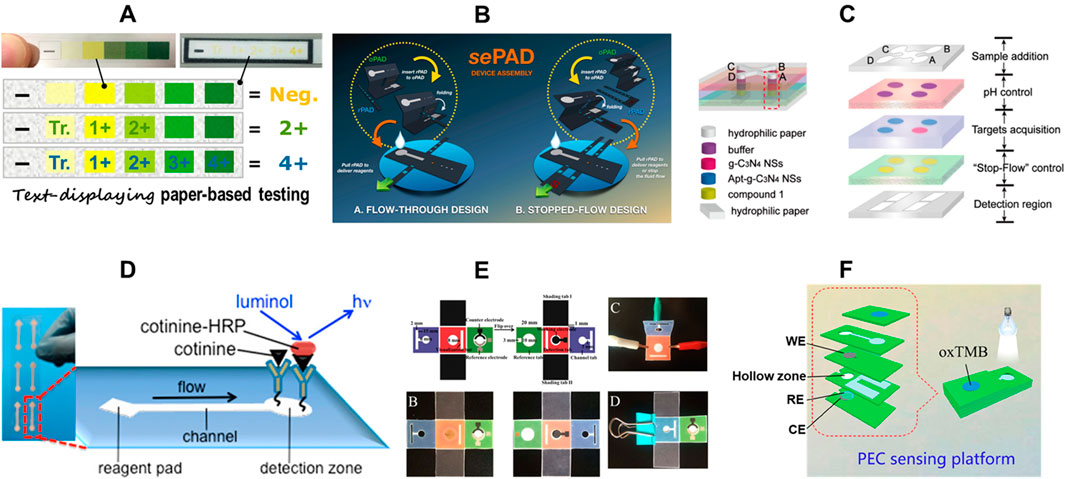
Figure 5. Common sensing methods for paper-based microfluidc devices. (A) text-displaying colorimetric paper-based sensor (adapted from (Yamada et al., 2017b) with permission, copyright 2017, American Chemical Society). (B) 3D Capillary-Driven Paper-Based Sequential Microfluidic Device (adapted from (Yakoh et al., 2019) with permission, copyright 2019, American Chemical Society). (C) Ratiometric paper-based device with digital readout via fluorescence detection (adapted from (Wang et al., 2019) with permission, copyright 2019, American Chemical Society). (D) Chemiluminescence based µPAD for cotinine detection in mouse serum (adapted from (Liu et al., 2013) with permission, copyright 2013, American Chemical Society). (E) Colorimetric and electrochemiluminescence dual-mode sensing of lead ion on µPAD (adapted from (Xu et al., 2018) with permission, copyright 2018, American Chemical Society). (F) Photoelectrochemical/Visual Lab-on-Paper Sensing via Signal Amplification of CdS Quantum Dots@Leaf-Shape ZnO (adapted from (Kong et al., 2018) with permission, copyright 2018, American Chemical Society).
While colorimetric systems are simple and effective, they do have drawbacks. A significant issue in quantitative colorimetric systems is the non-homogeneity of color (Morbioli et al., 2017; Yan-Qi and Liang, 2020). In µPADs, colored indicators used for detection can migrate unevenly, leading to patchy color development. This happens because highly soluble signaling molecules spread outwards during the sample flow, accumulating at the edges of the designated detection zone. This issue can be tackled by employing attachments, either covalent or non-covalent, directly onto the paper itself (Tan et al., 2022). Another major hurdle is the inconsistency of lighting. Initially, colorimetric systems were imaged using scanners, which provided higher resolution and consistent lighting. However, for Point-of-Need (PoN) or Point-of-Care (PoC) devices, it is ideal to have systems that are instrument-free or can be quantified using easily accessible tools like smartphones. Constructing a lightbox for imaging is one solution to this issue, allowing the use of a smartphone camera. Introducing a background region of interest (ROI) is another way to mitigate this problem, as it helps normalize intensities under varying lighting conditions (Tan et al., 2022; Aryal et al., 2024d; Richardson et al., 2021). Recently, third-party apps for color quantification have become available in app stores that have an onsite calibration feature built into them. Another challenge arises if the analyte itself is colored. In such cases, using a color control can help mitigate this issue. While distance-based semi-quantitative signal readouts allow for calibration-free assays, they are susceptible to observer-dependent readout errors. Counting-based semi-quantitative signal readouts are less prone to observer-dependent errors and can achieve calibration-free assays, but they have relatively limited resolution and are often time-dependent. Similarly, timing-based signal readout systems face the same limitations. Text-based detection platforms are often restricted by the target analytes that can be used for detection, as they may not be applicable for every assay (Yamada et al., 2017a).
3.2 Electrochemical detection
Electrochemical paper-based analytical devices (ePADs) are among the most used µPADs after colorimetric µPADs. This technology offers several advantages. Firstly, ePADs leverage external detectors like potentiostats, enabling them to achieve significantly lower detection limits, reaching the picomolar range (Aryal et al., 2024b; Adkins et al., 2015; Oh and Chow, 2015; Mettakoonpitak et al., 2016). This surpasses the capabilities of techniques like fluorescence and colorimetry (Pohanka and Skládal, 2008; Dungchai et al., 2009; Liu et al., 2014a). Additionally, ePAD results are unaffected by color variations within the sample itself, unlike colorimetric assays which can be prone to misinterpretations due to background color (Dortez et al., 2024). ePADs exhibit minimal impact from variations in sample volume as long as the designated electrode area is adequately covered (Yamada et al., 2017a). This provides greater flexibility compared to colorimetric methods where precise volume control is often critical. Moreover, electrochemical measurements can be performed relatively quickly, taking only seconds to minutes. This speed is especially advantageous when handling many samples (Noviana et al., 2020b). The conventional configuration for an electrochemical system consists of three electrodes: a working electrode, a counter electrode, and a reference electrode (Adkins et al., 2015; Dungchai et al., 2009). Since the development of the first ePAD in 2009 by screen printing carbon ink on paper (Dungchai et al., 2009), many other methods have been developed over the years. These methods include stencil printing, inkjet printing, microwire placement, laser scribing, using carbon tape, pencil drawing, spray and spin coating, laser scribing, and sputtering (Noviana et al., 2020b; Ataide et al., 2020; de Araujo et al., 2017). Figure 5B shows an example of innovative electrochemical sensing on paper using 3D capillary-driven sequential microfluidics.
While electrochemical µPADs outperform colorimetric paper devices in terms of analytical performance owing to the usage of external detectors (e.g., potentiostats), due to their high cost (usually > $1000) and operating complexity, laboratory-type electrochemistry stations are inaccessible to end consumers. The most important problem for the real-world deployment of ePADs is to build user-friendly signal detection interfaces. User-friendly signal detection interfaces allow for real-time data analysis and easy-to-understand data displays, helping users make quick decisions. To bridge the gap, many handheld potentiostats have been developed that allow for onsite/remote sensing. Some notable examples of portable potentiostats integrated with μPADs include inkjet-printed paper-based potentiostats (Bezuidenhout et al., 2018), USB-controlled potentiostats (Chhorn and Teeramongkonrasmee, 2018), battery-less NFC potentiostats (Chaiyo et al., 2024), and Wi-Fi cloud-based portable potentiostats (Bianchi et al., 2020). Among these portable systems, the wireless NFC potentiostats hold great promise. These potentiostats utilize near-field communication (NFC) for wireless power and data exchange with a user-friendly smartphone app (Krorakai et al., 2021). These portable and cost-effective systems offer analytical capabilities on par with traditional benchtop potentiostats (Beck et al., 2023). These potentiostats have been applied in μPADs-based electrochemical sensing of analytes like cholesterol and glucose and in performing various electrochemical lateral flow assays (Chaiyo et al., 2024; Gonzalez-Macia et al., 2024; Promsuwan et al., 2023; Lazaro et al., 2023). However, compared to benchtop potentiostats, portable potentiostats usually have higher noise levels, a smaller voltage range, and lower accuracy. Because of these drawbacks, portable potentiostats may not be as ideal for uses needing a broad voltage range or high precision and require further research and development (Aryal et al., 2024b).
Researchers using ePADs may face challenges such as restricted mass transfer, electrode fouling, a limited dynamic range, and inconsistent performance with disposable electrodes. To address these issues, smaller channels and increased flow rates can be employed to enhance mass transfer and allow analyte molecules more time to interact with electrode surfaces. To mitigate electrode fouling and improve electron transfer in electrochemical sensors, various strategies can be explored. These include employing nanoengineered surfaces like nanoporous metals and nanocarbons, applying antifouling layers such as PEG, zwitterionic polymers, and biopolymers, utilizing nanoporous membranes, and integrating hydrogels (Russo et al., 2021). In certain instances, the limited dynamic range issue can be resolved by expanding the electrode surface area (Arroyo-Currás et al., 2017). The use of ePADs coupled with wireless technology has been increasing and holds promising potential for the future (Solhi et al., 2020; Ruecha et al., 2017; Deroco et al., 2023; Fan et al., 2017). This method enables the wireless acquisition of data, which can be utilized for continuous monitoring in various fields such as sports, healthcare, agriculture, and defense. It allows users to receive alerts in case of abnormal situations and to be warned before these situations worsen. However, one challenge that wireless ePADs face in terms of sensing is the lifespan of the signals (Solhi et al., 2020). Researchers are encouraged to work on integrating wireless and remote sensing technologies to make this system more robust and reliable.
3.3 Chemiluminescence
Chemiluminescence (CL)-based paper microfluidics (μ-PCLDs) have also gained popularity, particularly in CL-immunoassays. CL works on the principle of generating photons through a chemical reaction, and these photons are typically quantified in the dark to avoid non-specific and background signals (Calabretta et al., 2021). Figure 5D shows an example of a chemiluminescence based detection of cotinine in serum using a μPAD. With advancements in noise control modifications, this method has become more sensitive and specific, allowing for more accurate routine biomedical and health monitoring applications (Ge et al., 2014). μ-PCLDs can be tailored to achieve a broad dynamic range, depending on the detection target. They can detect analytes like uric acid and glucose at millimolar concentrations while also being capable of detecting DNA at molar levels (Wang et al., 2013c; Yu et al., 2011b; Yu et al., 2011a). CL on paper is more efficient than typical CL tests because it consumes fewer samples and reagents, shortens analysis time, and uses automated, compact, and integrated detection techniques (Aryal et al., 2024b). Additionally, the development of antibody-based probes has enabled these systems to detect antigens at trace levels, even in complex matrices (Calabretta et al., 2021). Over time, CL-based microfluidics have been employed for detecting tumor markers, DNA, antibodies, and other biomolecules (Yamada et al., 2017a).
However, there remains a significant gap in translating μ-PCLDs from lab to practical use. This gap is primarily due to the need for expensive instruments, such as luminescence readers. While μ-PCLDs offer incremental gains in sensitivity compared to colorimetric µPADs, these gains are often overshadowed by the exponential increase in operational costs. Despite this, the development of CMOS sensors, which are less complex and less expensive than conventional instruments, offers a promising solution (Punjiya et al., 2014; Hu et al., 2020; Khan et al., 2018).
3.4 Fluorescence
Fluorescence based methods are also used with µPADs. The unique properties of fluorescent materials enable the creation of these assays. Tiny fluorescent particles, such as rhodamine, pyrene, or anthracenes, are deposited onto the paper (Nawaz et al., 2021). These particles then interact with the target molecule of interest. When ultraviolet light of a specific wavelength shines on the paper, any bound analyte triggers the fluorescent particles to emit light (Patel et al., 2021; Lian et al., 2020). The intensity of this emitted light, captured with a smartphone or scanner, can be analyzed using software like ImageJ to quantify the amount of target analyte present (Patel et al., 2021). Figure 5C shows an example of ratiometric sensing with fluorescence detection and digital readout.
µPADs using fluorescence measurements face more limitations compared to other detection methods. First, the paper itself can introduce noise by reflecting the excitation light, leading to a lower signal-to-noise ratio compared to techniques like electrochemiluminescence (Yamada et al., 2017a). Additionally, when using non-pure cellulose paper for convenience, the presence of optical brightening agents can further disrupt the assay by causing unwanted background fluorescence (Kwon et al., 2012). Some fluorescent molecules are unstable on paper across time and varying assay conditions, especially in fluctuating temperature and humidity environments. Use of stabilizing buffers or chelating agents can be used to enhance their stability.
3.5 Electrochemiluminescence
Electrochemiluminescence (ECL) is a light emission process that occurs when chemical species generated through oxidation-reduction reactions undergo exothermic reactions, emitting light energy. Depending on the source of radicals, there are two main ways electrochemiluminescence (ECL) can occur. In one method, light is produced when a single molecule undergoes a reaction that creates energetic particles. In the other method, light is generated by the interaction between a special molecule and another molecule in the solution (Chinnadayyala et al., 2019).
ECL-µPADs have gained popularity in healthcare applications, particularly for PoC diagnosis and disease monitoring. However, due to the electrochemical nature of the process, most luminophores in ECL are less soluble in water, making it challenging to create binding sites without affecting their chemical properties. This presents a significant hurdle in designing µPADs with ECL readouts, often resulting in compromised sensitivity compared to electrochemical methods. To address these challenges, various signal amplification strategies have been developed over the years. One promising advancement is the incorporation of nanomaterials, which can act as electrocatalysts in ECL reactions. These nanomaterials promote electron transfer at the electrode interface and increase the loading capacity of ECL labels. Common labels include carbon dots, inorganic complexes, semiconductor nanocrystals, graphene quantum dots, and photoluminescent carbon nanocrystals (Chinnadayyala et al., 2019). While paper-based ECL biosensors have seen progress in research labs, their commercialization also remains a challenge. A crucial step towards practical application involves a comprehensive understanding of key design aspects for ECL paper devices. This includes factors like detection limits, specificity, assay speed, analysis format, compatibility with various sample types, packaging considerations, labeling requirements, shelf life needs, and target production costs (Chinnadayyala et al., 2019).
3.6 Other sensing methods
Other methods for signal detection in μPADs include photoelectrochemical detection (Figure 5F), surface-enhanced Raman scattering (SERS), digital microfluidics on paper, calorimetry, dual mode detection (Figure 5E), and light-reflectance (Davaji and Lee, 2014; Abbas et al., 2013; Liu et al., 2014b; Fang et al., 2014; Fobel et al., 2014). In photoelectrochemical (PEC) detection, the process is reversed compared to electrochemiluminescence. Instead of light triggering a chemical reaction, chemical light emission from an internal system, such as luminol-hydrogen peroxide, is converted into an electrical current (Zhao et al., 2016). This current is then detected by a semiconductor material, such as quantum dots. Researchers have used PEC as a labeling technique for various immunoassays, including those targeting tumor marker proteins, ATP (adenosine triphosphate), and DNA (Wang et al., 2013a; Wang et al., 2013b; Ge et al., 2013). Digital microfluidics (DMF) technology offers a powerful tool for handling tiny liquid droplets, ranging from nanoliters to microliters in volume (Choi et al., 2012). This technique uses an array of electrodes and electrical fields to manipulate these droplets. By controlling the electrical fields applied to the electrodes, functionalities such as merging separate droplets, splitting a single droplet into smaller ones, mixing different droplets, and directing the flow of droplets across the surface can be achieved (Choi et al., 2012). This technique is an exciting addition to sensing on μPADs. Table 2 compares analytical methods for detection on μPADs, showcasing their strengths and weaknesses. Figure 5 depicts common sensing methods for μPADs.
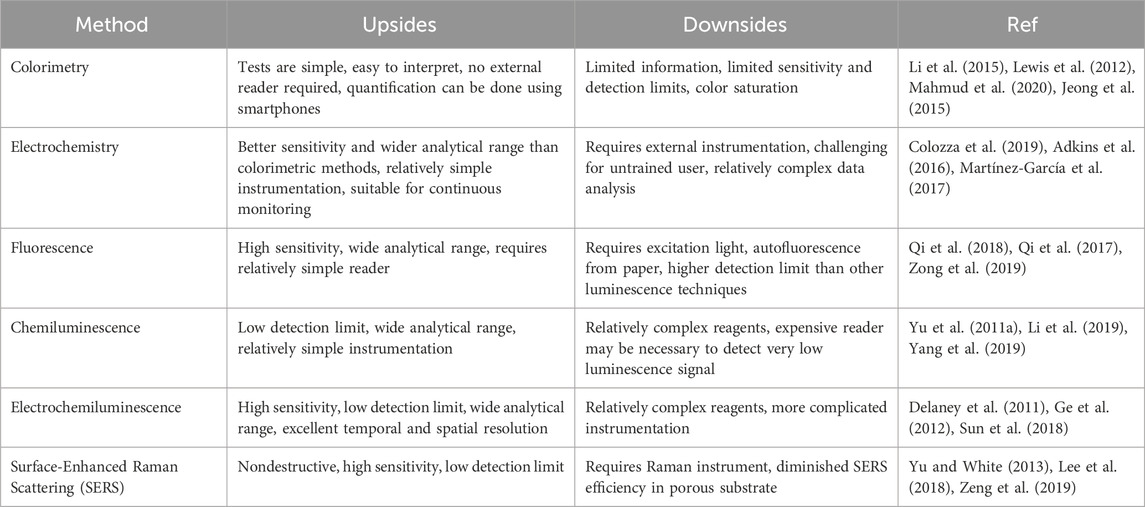
Table 2. Comparison of analytical methods for detection on μPADs. (Adapted from (Noviana et al., 2021) with permissions, copyright 2021, American Chemical Society).
The integration of smartphones with microfluidic paper-based analytical devices (μPADs) has significantly advanced their capabilities, transforming them into portable, user-friendly diagnostic tools ideal for point-of-care applications and resource-limited settings. Lately, colorimetric paper-based platforms have seen a significant advancement. A few notable examples include the following: a novel smartphone-based solution with a color correction algorithm to achieve accurate and absolute color measurements (Zhang et al., 2023), the development of smartphone applications for color quantification (Shen et al., 2012; Wu et al., 2024), and the development of a custom app to analyze specific μPAD regions to provide accurate concentration data (Liu et al., 2024). For electrochemical μPADs, miniaturized potentiostats connect to smartphones via audio jacks or data ports, enabling real-time control, data processing, and signal analysis directly on the smartphone screen (Beck et al., 2023). Fluorescence-based μPADs have also seen notable advancements, A notable advancement is the smartphone-integrated colorimetric sensor array and fluorometric detection system, which uses image processing algorithms to quantify fluorescence intensity (Chellasamy et al., 2021). Together, these innovations make μPADs increasingly sophisticated and accessible for on-site, real-time diagnostics without the need for complex laboratory equipment.
4 Applications
One of the primary goals of μPADs is to offer users easy-to-use, inexpensive, and portable testing/monitoring tools. This translates to real-world benefits across a wide range of applications where fast results and user-friendliness are critical. The significance of μPADs lies in their ability to empower anyone, regardless of technical expertise, to conduct tests or monitor samples on-site, at the point of care, need, or incident. This opens doors for applications in various fields, including diagnostics, disease monitoring, environmental checks, food safety analysis, and even forensics and defense.
4.1 PoC and disease monitoring
While urbanization is increasing globally, a sizable proportion of the population in emerging countries still lives in rural areas (Desa, 2014). Even though poverty is a difficult concept to define, many people in developing countries rely on subsistence activities for a living, which significantly impacts their health (Yetisen et al., 2013; Sociales, 2009). These resource-limited environments frequently lack the technical skills and staff available in urban areas, making access to healthcare difficult. Affordable technologies are critical for empowering these local populations and improving their healthcare access. This is where μPAD technologies can be particularly beneficial. While these technologies are more urgently needed in developing nations, where even basic illness or disease diagnosis can be challenging, recent pandemics have also underscored the importance of PoC sensors in developed countries as well. This demonstrates the broad applications and usefulness of μPAD technologies across various global contexts. A prime example of the importance of these devices emerged during the COVID-19 pandemic. On 30 January 2020, the World Health Organization (WHO) declared the COVID-19 pandemic a global health crisis (Ravi et al., 2020; World Health Organization, 2020). Since the onset of the pandemic, a significant number of paper-based systems for COVID-19 diagnostics have been developed and published (Pinheiro et al., 2021; Kim and Lee, 2022; de Araujo et al., 2024). Long before and throughout the COVID-19 pandemic, researchers have actively explored using paper-based platforms to create biosensors for diagnosing various diseases and infections (Silva-Neto et al., 2023). These devices offer a promising and versatile approach to healthcare diagnostics. Here are some examples of μPADs for diagnostics and health monitoring:
4.1.1 DNA extraction and detection
μPADs can be used to extract and detect DNA from biological samples. These instruments can be designed to extract DNA from a variety of biological samples by employing integrated buffers to degrade cells and specialized molecules to extract DNA (Shetty and Shetty, 2020; Ye and Lei, 2023; Chong et al., 2021). Moreover, they amplify DNA directly on paper using methods like recombinase polymerase amplification (RPA) (Jauset-Rubio et al., 2016; Lobato and O'Sullivan, 2018; Bai et al., 2022) and loop-mediated isothermal amplification (LAMP) (Seok et al., 2017; Das et al., 2023; Atceken et al., 2023), which enable accurate identification of certain DNA targets.
4.1.2 RNA extraction and detection
μPADs are also useful for RNA extraction and detection by employing techniques like solid-phase or magnetic bead-based capture (He et al., 2017; Liu et al., 2009; Malpartida-Cardenas et al., 2023; Bender et al., 2021). These methods use RNA-specific binding agents, enabling direct capture and purification of RNA on the paper itself, promoting selectivity and simplifying the process. Following extraction, the RNA can be utilized for downstream processes like sequencing or reverse transcription-polymerase chain reaction (RT-PCR), which can help with RNA-based assays, including gene expression analysis and RNA virus identification and characterization (Oruganti et al., 2023; Huber et al., 2004; Basiri et al., 2021).
4.1.3 Antigen detection
These paper-based sensors can identify antigens through immunoassays. Antibodies specific to the target antigen are usually immobilized onto the paper substrate in immunoassays conducted on paper-based sensors. If the antigen is present in the sample, it attaches to the immobilized antibodies and forms a complex. Then, using signal amplification techniques such as colorimetric changes, fluorescence, or electrochemical signals, this interaction is observed (Liang et al., 2012; Jaewjaroenwattana et al., 2023; Hristov et al., 2021; Baldo et al., 2024). This offers a rapid and user-friendly method for diagnosing active infections.
4.1.4 Antibody detection
These devices can detect antibodies produced by the immune system in response to a specific infection, such as SARS-CoV-2 (Kasetsirikul et al., 2021; Bao et al., 2022; Antiochia, 2021). First viral antigens or specific recombinant proteins are immobilized onto the paper substrate. When a sample like blood, serum, or plasma is applied, any antibodies present in the sample that recognize the immobilized antigens bind strongly. This binding is visualized and/or quantified using various detection methods (Tenda et al., 2018; Kasetsirikul et al., 2020; Zhao and Liu, 2016). This helps determine past exposure and an individual’s immune status (Silva-Neto et al., 2023; Solhi et al., 2020). Figure 6A shows a pulling-force spinning top used for serum separation, integrated with paper-based microfluidic devices for COVID-19 diagnosis. the paper-based microfluidic ELISA detects antibodies targeting the receptor-binding domain (RBD) of the SARS-CoV-2 spike protein, confirming it is designed to assess the body’s immune response
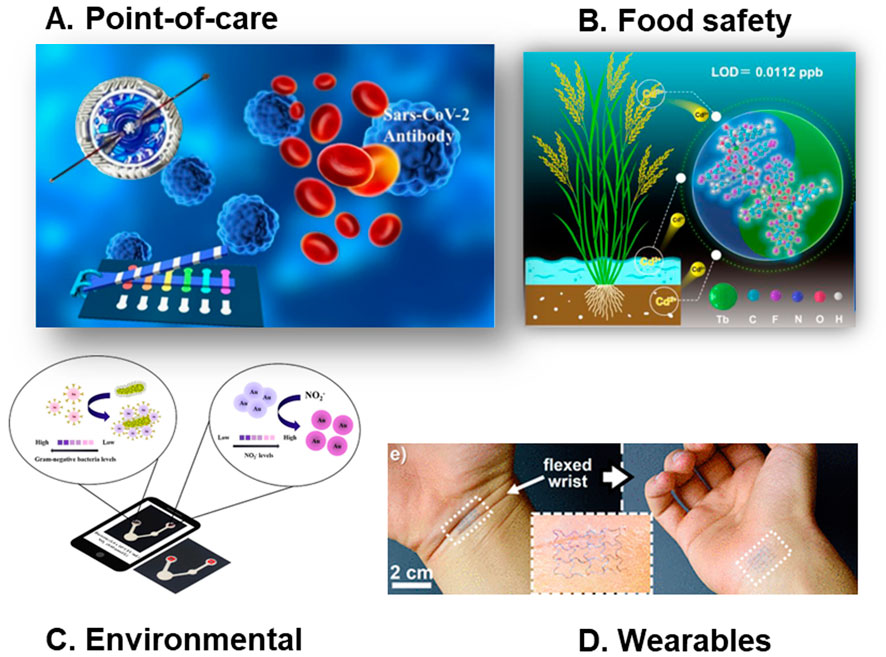
Figure 6. Common applications of paper-based microfluidc devices. (A) A pulling-force spinning top used for serum separation, integrated with paper-based microfluidic devices for COVID-19 diagnosis (adapted from (Gong et al., 2021) with permission, copyright 2021, American Chemical Society). (B) Visual and real-time monitoring of Cd2⁺ in rice using [2+2] lanthanide clusters (adapted from (Yu et al., 2023) with permission, copyright 2023, American Chemical Society). (C) A microfluidic paper analytical device utilizing gold nanomaterials for the simultaneous quantification of Gram-negative bacteria and nitrite ions in water samples (adapted from (Khachornsakkul et al., 2023) with permission, copyright 2023, American Chemical Society). (D) Wearable and Implantable Epidermal Paper-Based Electronics (adapted from (Sadri et al., 2018) with permission, copyright 2018, American Chemical Society).
4.1.5 Wearables
The field of non-invasive health monitoring is witnessing a surge in the use of wearable sensors that incorporate μPADs. Most of these tools analyze sweat, a readily accessible biofluid, to detect a range of biomarkers like glucose, lactate, pH, and chloride (Noviana et al., 2021; Deroco et al., 2023; Baldo et al., 2020). This enables real-time monitoring of factors like physical activity and cardiovascular health. Furthermore, μPADs are being integrated into systems designed for continuous screening of biochemical markers in other body fluids like tears and saliva as well (Noviana et al., 2021). This eliminates the need for complex procedures like pre-treatment or sample manipulation, significantly improving user experience. Additionally, the integration of Internet of Things (IoT) technology with these wearable sensors is gaining traction (Deroco et al., 2023). This marriage of technologies allows for real-time data collection and management, even in remote or resource-limited settings, making personalized healthcare more accessible. Figure 6D shows a very creative wearable and implantable epidermal paper-based electronics.
There have been numerous works in μPAD-based sensing techniques, particularly in the PoC field. These innovations have significantly enhanced diagnostic capabilities and disease monitoring. Blood typing (Jarujamrus et al., 2012), coagulation monitoring (Hegener et al., 2017), inflammatory biomarkers testing (Kamakoti et al., 2023), glucose monitoring (Liu et al., 2016), cancer biomarkers testing (Kaewarsa et al., 2021), cardiac biomarker testing (Boonkaew et al., 2021), salivary iron testing (Prakobdi et al., 2024), urinary tract infection (UTI) diagnosis, (Hasandka et al., 2022), and sexually transmitted infection (STI) Screening (Gaydos and Hardick, 2014) are some examples of promising advancements by μPADs for PoC diagnosis.
4.2 Environmental monitoring
Pollution is a major issue worldwide, affecting the health of people in developed and developing countries. Pollution can be of greater concern in developing countries, where large populations suffer from industrial waste, poor hygiene, unsafe drinking water, and unclean indoor air caused by burning organic matter due to the lack of established and enforced regulatory policies. The problem worsens in these areas because they lack affordable and fast ways to test for pollution/toxins. The progress of industry in developed countries has also created new types of pollution that can spread quickly and overwhelm the environment’s natural ability to clean itself. While traditional methods for monitoring environmental contaminants are reliable, they suffer from being time-consuming, expensive, and require specialized personnel. These limitations render them unsuitable for on-site testing. Therefore, researchers have increasingly focused on μPADs as a promising alternative for the detection of a wide range of contaminants, including heavy metals, pesticides, nutrients, microorganisms, and emerging threats like PFAS (per- and poly-fluoroalkyl substances) and particulate matter. We recently published a critical review on microfluidics for environmental monitoring, encompassing all the latest advances in the field (Aryal et al., 2024b). In this section, we will only focus on the general trends and gaps observed in μPADs for environmental monitoring.
The use of μPADs in environmental analysis spans various matrices, such as water, soil, air, agriculture products, etc. The most targeted contaminants are heavy metals, pesticides, nutrients, and per- and polyfluoroalkyl substances (PFAs) (Aryal et al., 2024b). Among the detection techniques employed, colorimetric methods are the most prevalent due to their simplicity and cost-effectiveness as well as their ability to be used onsite (Patel et al., 2021; Aryal et al., 2024a). Most applications have also focused on water samples. This is because water’s natural fluidic properties, such as low viscosity and surface tension, enable consistent flow and efficient mixing within the microchannels, ensuring precise sample processing (Saez et al., 2021; Atencia and Beebe, 2005). In contrast, for soil or air matrices, the samples need to be pretreated to get them into fluids, or complicated detection methods must be used to accommodate the differences in sample properties. In soil matrices, μPADs are primarily used for detecting nutrients, microorganisms, and other inorganic contaminants. The detection techniques used in soil analysis are more varied compared to those in water-based systems where colorimetric detection is very common. This diversity arises because soil samples require pretreatment and clarification before colorimetric analysis, as the natural color of the soil can interfere with the results. In addition to detecting contaminants, μPADs in soil are also used to monitor the behaviors of microorganisms, including their growth patterns, metabolic activities, and interactions with soil components. For air matrices, the primary focus is on detecting particulate metals and airborne pathogens. The most common techniques in air analysis are electrochemical and colorimetric methods. Figure 6C shows an innovative use of μPAD utilizing gold nanomaterials for the simultaneous quantification of Gram-negative bacteria and nitrite ions in water samples.
Detecting contaminants using μPADs in complex matrices like soil, seawater, and air remains challenging. Factors such as topography, temperature, and humidity can further complicate the measurement in these matrices. Therefore, when designing μPADs for complex environmental matrices, it is not possible to create a perfect miniaturized copy of a real soil or air environment within a microfluidic chip. Instead, these devices should focus on mimicking specific aspects of soil or air under controlled conditions. Moreover, when designing these tools, scientists need to clearly define which soil or air features they aim to replicate, and their findings should reflect the specific conditions they mimicked. μPAD-based PoN sensors are a promising development for environmental monitoring; however, many of these quick tests often rely on qualitative colorimetric methods to indicate results. Such methods can be subjective to the reader/user. Alternatively, techniques like EChem or fluorescence require external power and additional equipment. Therefore, there’s a critical need to develop quantitative/semi-quantitative colorimetric methods specifically for PoN applications. This is important because the majority of contamination occurs in resource-limited settings where sophisticated quantification resources may not be readily available.
4.3 Food safety
Ensuring the safety of food is a top priority for both the food industry and those involved in agriculture. This means in the food industry, thorough checking for biological hazards, chemical contaminants, and allergic substances is essential to ensure food safety. Similarly in agriculture, regular monitoring of pesticide content, nutritional content, and potential health risks from cultivation to harvest is crucial for producing safe and healthy food. μPADs are increasingly being used to identify various contaminants in foods and beverages due to their portability and simplicity (Nilghaz et al., 2021). These devices provide a quick and easy solution for food safety and quality control in a variety of situations, including food processing plants and residences (Mitrogiannopoulou et al., 2023). They are used to identify foodborne pathogens like E. coli and Salmonella and pollutants like pesticides and heavy metals (Ji et al., 2020). They are also used to detect food spoilage, evaluate food quality indicators, and even analyze nutritional content (Hua et al., 2018; Soman et al., 2024; Wang et al., 2022). Figure 6B shows an innovative μPAD-based method of visual and real-time monitoring of Cd2⁺ in rice using [2+2] lanthanide clusters.
Detection of contaminants and microorganisms in food and beverages using μPADs involves several sensing methodologies depending upon the detection limit required and complexity of sample pretreatment. For bacterial detection, enzymatic activity or immunoassays are frequently employed (Nadar et al., 2021). These methods rely on colorimetric detection based on enzyme reactions with chromogenic substrates or electrochemical detection based on redox reactions involving the transfer of electrons between the bacterial metabolites or immunological markers and the electrode. To detect heavy metal contaminants like lead (Pb) and cadmium (Cd), electrochemical methods are commonly used (Busa et al., 2016). The most commonly used method for pesticide detection involves enzymatic inhibition by the pesticide, with color or an electrochemical signal used as the readout (Nadar et al., 2021; Hefner et al., 2024). Additionally, for detecting some other pesticide contaminants, methods like electrochemiluminescence (ECL) and chemiluminescence are employed (Busa et al., 2016). Even after years of advances, the promise of μPADs for food quality and safety analysis remains largely unfulfilled due to limitations in user-friendliness and sensitivity. The majority of food samples need to be pretreated before using them in μPADs. Therefore, more research on integrating on-chip sample pretreatment is needed.
4.4 Security and forensics
In critical security concerns such as terrorist attacks, chemical spills, biological warfare incidents, radiological emergencies, hazardous material accidents, fire, and explosion accidents, swift detection of contaminants is crucial. In the aftermath of an incident, it's essential to quickly screen numerous people and surfaces for harmful substances before sending samples to a lab for confirmation. Similarly, at high-traffic checkpoints, rapid tests are necessary to detect harmful chemicals and prohibited drugs that might be smuggled. This is where μPADs can provide prompt results that save time and enable security officials to make timely decisions. Paper-based microfluidics have the potential to be utilized in international security systems for the detection of various prohibited chemicals, warfare agents, and explosives. They are employed in screening luggage, packages, and suspicious materials at checkpoints. Common targeted chemicals include peroxide explosives like triacetone triperoxide (TATP) and diacetone diperoxide (DADP), as well as nitroaromatic explosives such as trinitrotoluene (TNT), picric acid (PA), and dinitrotoluene (DNT) (Gökdere et al., 2019; Tian et al., 2017; Pramanik et al., 2019). μPADs are also used to detect illegal drugs in the field, thereby supporting law enforcement efforts (Noviana et al., 2020a).
In forensic analysis, presumptive tests serve as rapid initial screenings that help investigators detect potential substances. However, these tests have significant drawbacks: they yield a lot of false positives or negatives, potentially damage valuable DNA evidence, are not label-free, and can contaminate samples with chemical reagents (Bazyar, 2023). To overcome these challenges, μPADs can offer a compact size, portability, reduced risk of contamination, and safe sample storage for subsequent analysis (Bazyar, 2023; Harshey et al., 2023). Forensic investigations involve a wide array of diagnostic tasks, ranging from identifying and analyzing body fluids to detecting drugs of abuse and explosive residues. Common toxins detected include cyanide, ethanol, nitrite, and arsenic (Musile et al., 2023). They are also used to identify drugs and drugs of abuse, such as cathinones, 3,4-methylenedioxymethamphetamine (MDMA), xylazine, morphine, synthetic cannabinoids, benzodiazepines, cocaine, fentanyl, tetrahydrocannabinol, and ketamine (Musile et al., 2023). Moreover, µPADs are employed to detect psychoactive substances commonly used in drug-facilitated crimes, such as gamma-hydroxybutyric acid (GHB), metamizole, midazolam, flunitrazepam, and scopolamine (Musile et al., 2023). Additionally, μPADs can be employed for the preliminary analysis of gunshot residue, counterfeit pharmaceuticals, and evidence at crime scenes (Bazyar, 2023; Harshey et al., 2023; de Oliveira et al., 2017). While μPADs hold promise for security and forensic analysis, additional research is necessary to integrate them with portable and cost-effective platforms. This integration is crucial for ensuring that the developed sensors are both portable and quantifiable at the point of incident. Figure 6 shows the common applications of paper-based microfluidics in different sectors.
5 Sustainability and eco-friendliness
Many existing analytical methods consume a disproportionate amount of energy to detect small amounts of analytes. The trade-off between incremental gains in detection sensitivity and substantial energy consumption raises questions about the overall sustainability and cost-effectiveness of such approaches. Fundamentally, the environmental cost of employing these energy-intensive methods may outweigh the benefits gained from the increased precision, especially when detecting small contaminations. This highlights the need for a more balanced and sustainable approach to sensing technologies. In this context, µPADs emerge as a convincing alternative, offering effective detection capabilities without the excessive energy demands of traditional methods. µPADs ability to operate with low to negligible energy consumption challenges the assumption that advanced functionality must be achieved at the cost of sustainability. In this prospect, paper is an easily available, renewable resource compared to plastics and silicon, which are normally used in microfluidic devices. This reduces dependence on non-renewable resources and promotes the use of sustainable materials. Manufacturing µPADs typically involves simpler techniques that use less energy and fewer harsh chemicals compared to making conventional microfluidic chips.
Given the variability in assay components within µPADs, it's challenging to make sweeping generalizations and assign an average greenness score. However, herein we analyze the µPADs' compliance with the twelve main principles of green chemistry by Anastas and Warner (Anastas and Warner, 2000; Gałuszka et al., 2013). Using the criteria for each principle by the AGREE Greenness matrix (Pena-Pereira et al., 2020), these characteristics are evaluated to assess the environmental friendliness of the µPADs (Table 2).
Figure 7 depicts the spectrum of greenness comparing paper-based microfluidics to traditional heavy instrumentation-based measurements. Traditional analytical methods, situated at the lower (red) spectrum of greenness and sustainability, are characterized by high energy consumption, greater sample requirements, longer analysis times, and numerous procedural steps. In contrast, the majority of microfluidic μPADs adhere to the principles of green chemistry, placing them at the higher (green) end of the spectrum. Within μPADs themselves, systems at the lower end often require off-chip pretreatment steps and may involve the use of toxic reagents applied on paper. Furthermore, these systems depend on energy-consuming instruments for analysis. Conversely, at the higher end of the spectrum, there are qualitative or semi-quantitative green sensors. These sensors utilize natural reagents and offer the advantage of not needing any external readout instruments. Table 3 presents the assessment of μPADs in relation to the 12 principles of green chemistry, comparing their alignment with these principles.
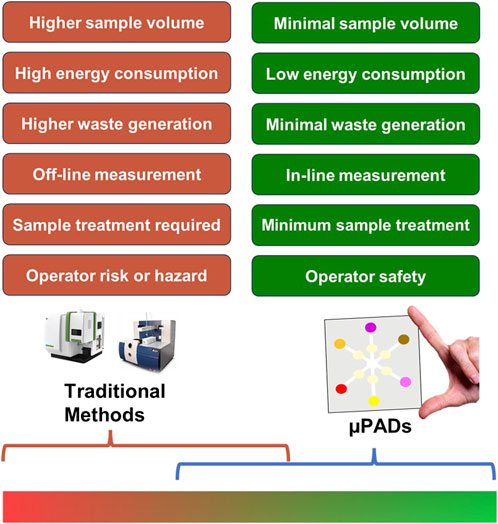
Figure 7. Spectrum of greenness analysis in μPADs compared with traditional analytical methods. The color gradient indicates the greenness spanning from low (red) to high (green) sustainability levels.
6 Bench-to-field challenges
In academia, there’s often a prevailing notion that researchers should focus solely on developing methods or making discoveries, leaving the task of implementation to industry (Kumar et al., 2015). While there is some truth to this perspective, it can result in a plethora of research findings languishing in academic journals, merely serving as academic achievements rather than making tangible impacts. This phenomenon isn't confined to theoretical research alone; even practical innovations, like μPADs and sensors, can suffer a similar fate. Many of μPADs inventions, including point-of-care (PoC) or point-of-need (PoN) sensors, are thoroughly crafted in academic settings but fail to transition into practical use outside the lab.
While academia is increasingly investigating the potential of μPADs for PoC diagnostics and PoN testing, putting them into practice proves more challenging than expected. Initial field evaluations of μPADs for PoC diagnostics and PoN testing encounter several challenges. These include sample preparation, limited accuracy, batch variability, short shelf life, reliance on specific readout systems, usability issues, significant production costs, and regulatory approval. While addressing these challenges can yield considerable benefits, such as enhancing technology, cutting development costs, and attracting additional funding, a central obstacle remains. The skillset necessary for successful field testing often diverges from the expertise typical within academic departments like chemistry or biology. As indicated by Kumar et al., for every 60 papers published on laboratory tests aimed at PoC diagnostics, only one paper focuses on field testing or clinical evaluation (Kumar et al., 2015). Public health professionals or scientists skilled in fieldwork pose a distinct advantage here. Conversely, the academics may lack relevant experience. To bridge this gap, increased collaboration with public health experts and greater integration of field evaluation training into relevant academic programs are essential.
PoC testing refers to medical diagnostic tests conducted directly where a patient receives care. While many of these paper-based tests have made strides in transitioning from proof-of-concept to PoC, researchers continue to develop tools to further facilitate this shift. Whiteside’s group used μPADs to design a liver function test, which they then trialed in a hospital setting in Vietnam (Vella et al., 2012; Mace et al., 2012; Kumar et al., 2014). Additionally, they pioneered self-forming step-gradients in density to create a test for sickle cell disease, which underwent evaluation in a clinical setting in Zambia (Kumar et al., 2015). Diagnostics for All (DFA) has successfully commercialized paper-based liver function tests, which are now used in resource-limited settings (Pollock et al., 2013). Fio Corporation’s Deki Reader, a mobile device-based platform for reading and interpreting paper-based rapid diagnostic tests, has been deployed in multiple countries for malaria and HIV testing (Noble et al., 2020). Biosense Technologies’ uChek, a smartphone-based urinalysis system using paper test strips, has gained traction in India and other developing countries (Flaucher et al., 2022). Abbott’s BinaxNOW COVID-19 Ag Card, a paper-based rapid antigen test, received FDA Emergency Use Authorization and has been widely used for point-of-care COVID-19 testing (Pollreis et al., 2021; Genomeweb, 2020; Valera et al., 2021). Nima Sensor, a portable gluten and peanut detector using paper-based microfluidics, has been commercialized and is helping individuals with food allergies (Taylor et al., 2018; Burt et al., 2018). Spot-On Sciences has commercialized HemaSpot, a paper-based blood collection device that simplifies remote sampling for various diagnostic applications (Prosperi et al., 2021). Paperfuge, a low-cost paper-based centrifuge developed by researchers at Stanford University, has been successfully deployed for malaria diagnosis in Uganda (Bhamla et al., 2017; Bhamla et al., 2016). The SARS-CoV-2 Rapid Antigen Test developed by SD Biosensor and Roche has been widely adopted globally, demonstrating the scalability of paper-based diagnostics (Flinck et al., 2022). Foldscope, an ultra-low-cost paper microscope, has been distributed to over 150 countries, enabling field diagnostics and science education (Moreno-Roman and Bobick, 2022). These works showcase the potential of μPADs in collaborating with communities in various regions. These examples illustrate the promising transition of μPADs from research to real-world use, demonstrating their commercial viability and impact. Their success, particularly in medical diagnostics, highlights their potential to address global health challenges in resource-limited settings.
In the realm of environmental applications, most developed systems are designed for point-of-need scenarios, where contamination or issues arise. Unfortunately, many μPADs developed for environmental monitoring have yet to bridge the gap from proof-of-concept to user-friendly, non-expert-based monitoring. Affordable and user-friendly devices are key to implementing high-frequency measurements, enabling non-experts and citizens to actively monitor their surroundings. There are some examples of where μPADs have been used by citizens to gather their crucial environmental data without the scientist visits. Pamme’s team developed a paper microfluidic device for monitoring phosphate levels in freshwater, leveraging citizen science involvement (Richardson et al., 2021). We recently developed a citizen-led global phosphate monitoring sensor and mapped the phosphate levels of countries like Nepal, Thailand, Germany, Chile, Brazil, and the USA (Aryal et al., 2024c). Sameenoi’s team developed an on-site chloride test in tap water, empowering untrained local community staff to conduct the tests (Tasangtong et al., 2024). These achievements and developments are promising examples of translating μPADs into the hands of citizens, though such examples are very few.
7 Conclusion and future perspectives
While advancements have been made in paper-based sensor performance through material selection, design optimization, advanced manufacturing, and functional material integration, achieving ideal performance across all metrics remains a challenge. The following key aspects need to be considered to realize the commercial success of μPADs.
7.1 Enhanced sensitivity and multiplexing
To improve the sensitivity and multiplexing capabilities of μPADs, researchers must focus on developing advanced microfluidic networks while incorporating signal amplification techniques. Interconnected designs with multiple channels and reaction zones enable the simultaneous detection of various targets, enhancing diagnostic efficiency. Incorporating signal amplification techniques, such as CRISPR/Cas biosensing systems combined with novel nanomaterials, can significantly boost detection sensitivity and signal strength, making μPADs more effective for complex analytical applications.
7.2 Integration with smart technologies
By leveraging artificial intelligence, machine learning, and the Internet of Things, data analysis and interpretation can become more efficient and accurate. Furthermore, the development of universal data processing applications compatible with various smartphone models would simplify result interpretation, making these platforms more user-friendly and widely applicable.
7.3 Improved fabrication and reproducibility
Optimizing patterning techniques can reduce batch-to-batch variations and enable stable mass production. Standardizing fabrication protocols and promoting the complete disclosure of methodologies in published works will enhance reproducibility, fostering greater confidence in μPAD-based technologies among researchers and users alike.
7.4 Automation and portability
Automated test readouts are essential for improving detection reproducibility and minimizing user error. Integrating μPADs with portable detectors can enable real-time, on-site diagnostics and testing, making them invaluable tools in various real-world applications. These systems include smartphone-based detection systems, portable transmittance colorimeters, paired-emitter-detector diode (PEDD) systems, miniaturized electrochemical detectors, and automated lateral flow devices.
7.5 Materials and manufacturing consistency
Achieving scalability in μPADs requires a focus on material consistency, user-centric development, and manufacturing precision. It is essential to use consistent materials for both prototyping and mass production, reducing the risk of costly redesigns. Additionally, prioritizing user needs is crucial by considering safety for non-professional use and designing devices with user-friendly dimensions that cater to the target audience. Finally, manufacturing precision must be assessed by evaluating parameters like volumes, setup costs, and batch sizes to ensure cost-effective and high-quality production of μPADs.
7.6 Real-world testing and validation
The primary scalability issues arise from the lack of field trials within the research. This gap is evident in many μPADs platforms, where researchers often neglect the importance of testing sensors with actual real-world samples. Consequently, there is a significant misuse of the term “proof-of-concept,” with published μPADs results often being difficult or impossible to reproduce. Many published works fail to properly mention the full protocols or describe them very poorly. To ensure μPADs perform effectively outside the laboratory, extensive field trials with real-world samples are critical. These trials help validate the performance of μPADs in diverse environmental conditions. Efforts should also focus on integrating sample collection, pretreatment, analysis, and data interpretation into single, user-friendly platforms tailored for resource-limited settings, ensuring practical usability.
Author contributions
PA: Writing–original draft, Writing–review and editing. CH: Funding acquisition, Writing–review and editing, Writing–original draft.
Funding
The author(s) declare that financial support was received for the research, authorship, and/or publication of this article. We acknowledge financial support from the US Army DEVCOM Center (W911QY2120003).
Conflict of interest
The author(s) declared that they were an editorial board member of Frontiers, at the time of submission. This had no impact on the peer review process and the final decision
Publisher’s note
All claims expressed in this article are solely those of the authors and do not necessarily represent those of their affiliated organizations, or those of the publisher, the editors and the reviewers. Any product that may be evaluated in this article, or claim that may be made by its manufacturer, is not guaranteed or endorsed by the publisher.
References
Abbas, A., Brimer, A., Slocik, J. M., Tian, L., Naik, R. R., and Singamaneni, S. (2013). Multifunctional analytical platform on a paper strip: separation, preconcentration, and subattomolar detection. Anal. Chem. 85, 3977–3983. doi:10.1021/ac303567g
Abe, K., Suzuki, K., and Citterio, D. (2008). Inkjet-printed microfluidic multianalyte chemical sensing paper. Anal. Chem. 80, 6928–6934. doi:10.1021/ac800604v
Adkins, J. A., Noviana, E., and Henry, C. S. (2016). Development of a quasi-steady flow electrochemical paper-based analytical device. Anal. Chem. 88, 10639–10647. doi:10.1021/acs.analchem.6b03010
Adkins, J., Boehle, K., and Henry, C. (2015). Electrochemical paper-based microfluidic devices. Electrophoresis 36, 1811–1824. doi:10.1002/elps.201500084
Akyazi, T., Basabe-Desmonts, L., and Benito-Lopez, F. (2018). Review on microfluidic paper-based analytical devices towards commercialisation. Anal. Chim. acta 1001, 1–17. doi:10.1016/j.aca.2017.11.010
Amini, A., Guijt, R. M., Themelis, T., De Vos, J., and Eeltink, S. (2023). Recent developments in digital light processing 3D-printing techniques for microfluidic analytical devices. J. Chromatogr. A 1692, 463842. doi:10.1016/j.chroma.2023.463842
Anastas, P. T., and Warner, J. C. (2000). Green chemistry: theory and practice. Oxford, England: Oxford University Press.
Antiochia, R. (2021). Paper-based biosensors: Frontiers in point-of-care detection of COVID-19 disease. Biosensors 11, 110. doi:10.3390/bios11040110
Arroyo-Currás, N., Scida, K., Ploense, K. L., Kippin, T. E., and Plaxco, K. W. (2017). High surface area electrodes generated via electrochemical roughening improve the signaling of electrochemical aptamer-based biosensors. Anal. Chem. 89, 12185–12191. doi:10.1021/acs.analchem.7b02830
Aryal, P., Boes, J., Brack, E., Alexander, T., and Henry, C. S. (2024a). Fill, fold, photo: preconcentration and multiplex detection of trace level heavy metals in water. ACS sensors 9, 5479–5488. doi:10.1021/acssensors.4c01708
Aryal, P., Hefner, C. E., Martinez, B., Brack, E., and Henry, C. S. (2024c). Citizen-based water quality monitoring: field testing a user-friendly sensor for phosphate detection in global surface waters. Anal. Chem. 96, 18369–18376. doi:10.1021/acs.analchem.4c02123
Aryal, P., Hefner, C., Martinez, B., and Henry, C. S. (2024b). Microfluidics in environmental analysis: advancements, challenges, and future prospects for rapid and efficient monitoring. Lab a Chip 24, 1175–1206. doi:10.1039/d3lc00871a
Aryal, P., Indrianingsih, A. W., and Henry, C. S. (2024d). Smartphone-enabled green anthocyanin sensor for Fe (III) sensing on paper using capillary-driven microfluidics. Green Anal. Chem. 8, 100091. doi:10.1016/j.greeac.2023.100091
Ataide, V. N., Mendes, L. F., Gama, L. I., De Araujo, W. R., and Paixão, T. R. (2020). Electrochemical paper-based analytical devices: ten years of development. Anal. Methods 12, 1030–1054. doi:10.1039/c9ay02350j
Atceken, N., Munzer Alseed, M., Dabbagh, S. R., Yetisen, A. K., and Tasoglu, S. (2023). Point-of-Care diagnostic platforms for loop-mediated isothermal amplification. Adv. Eng. Mater. 25, 2201174. doi:10.1002/adem.202201174
Atencia, J., and Beebe, D. J. (2005). Controlled microfluidic interfaces. Nature 437, 648–655. doi:10.1038/nature04163
Bai, Y., Ji, J., Ji, F., Wu, S., Tian, Y., Jin, B., et al. (2022). Recombinase polymerase amplification integrated with microfluidics for nucleic acid testing at point of care. Talanta 240, 123209. doi:10.1016/j.talanta.2022.123209
Baldo, T. A., Ataide, V. N., Park, J., Panraksa, Y., Martinez, B., Anderson, L. B., et al. (2024). Automated enzyme-linked immunosorbent assay for point-of-care COVID-19 testing. Electrochimica Acta 144525. doi:10.1016/j.electacta.2024.144525
Baldo, T. A., De Lima, L. F., Mendes, L. F., De Araujo, W. R., Paixao, T. R., and Coltro, W. K. (2020). Wearable and biodegradable sensors for clinical and environmental applications. ACS Appl. Electron. Mater. 3, 68–100. doi:10.1021/acsaelm.0c00735
Banks, J., and Watt, J. (1784). On a new method of preparing a test liquor to shew the presence of acids and alkalies in chemical mixtures. By Mr. James Watt, Engineer; Communicated by Sir Joseph Banks, Bart. PRS. Philosophical Trans. R. Soc. Lond. 74, 419–422.
Bao, L., Park, J., Qin, B., and Kim, B. (2022). Anti-SARS-CoV-2 IgM/IgG antibodies detection using a patch sensor containing porous microneedles and a paper-based immunoassay. Sci. Rep. 12, 10693. doi:10.1038/s41598-022-14725-6
Basiri, A., Heidari, A., Nadi, M. F., Fallahy, M. T. P., Nezamabadi, S. S., Sedighi, M., et al. (2021). Microfluidic devices for detection of RNA viruses. Rev. Med. virology 31, 1–11. doi:10.1002/rmv.2154
Bazyar, H. (2023). On the application of microfluidic-based technologies in forensics: a review. Sensors 23, 5856. doi:10.3390/s23135856
Beck, J. J., Alenicheva, V., Rahn, K. L., Russo, M. J., Baldo, T. A., and Henry, C. S. (2023). Evaluating the performance of an inexpensive, commercially available, NFC-powered and smartphone controlled potentiostat for electrochemical sensing. Electroanalysis 35, e202200552. doi:10.1002/elan.202200552
Bender, A. T., Sullivan, B. P., Zhang, J. Y., Juergens, D. C., Lillis, L., Boyle, D. S., et al. (2021). HIV detection from human serum with paper-based isotachophoretic RNA extraction and reverse transcription recombinase polymerase amplification. Analyst 146, 2851–2861. doi:10.1039/d0an02483j
Bezuidenhout, P., Smith, S., and Joubert, T.-H. (2018). A low-cost inkjet-printed paper-based potentiostat. Appl. Sci. 8, 968. doi:10.3390/app8060968
Bhamla, M. S., Benson, B., Chai, C., Katsikis, G., Johri, A., and Prakash, M. (2016). Paperfuge: an ultra-low cost, hand-powered centrifuge inspired by the mechanics of a whirligig toy. bioRxiv, 072207. doi:10.1101/072207
Bhamla, M. S., Benson, B., Chai, C., Katsikis, G., Johri, A., and Prakash, M. (2017). Hand-powered ultralow-cost paper centrifuge. Nat. Biomed. Eng. 1, 0009. doi:10.1038/s41551-016-0009
Bianchi, V., Boni, A., Fortunati, S., Giannetto, M., Careri, M., and De Munari, I. (2020). A Wi-Fi cloud-based portable potentiostat for electrochemical biosensors. IEEE Trans. Instrum. Meas. 69, 3232–3240. doi:10.1109/tim.2019.2928533
Boobphahom, S., Nguyet Ly, M., Soum, V., Pyun, N., Kwon, O.-S., Rodthongkum, N., et al. (2020). Recent advances in microfluidic paper-based analytical devices toward high-throughput screening. Molecules 25, 2970. doi:10.3390/molecules25132970
Boonkaew, S., Jang, I., Noviana, E., Siangproh, W., Chailapakul, O., and Henry, C. S. (2021). Electrochemical paper-based analytical device for multiplexed, point-of-care detection of cardiovascular disease biomarkers. Sensors Actuators B Chem. 330, 129336. doi:10.1016/j.snb.2020.129336
Bruzewicz, D. A., Reches, M., and Whitesides, G. M. (2008). Low-cost printing of poly (dimethylsiloxane) barriers to define microchannels in paper. Anal. Chem. 80, 3387–3392. doi:10.1021/ac702605a
Burt, A., Artiuch, J., Chun, E., Cipriani, M., Leung, A., Scutari, A., et al. (2018). Development and preliminary validation of the Nima peanut sensor: a consumer device for food testing. Ann. Allergy, Asthma and Immunol. 121, S12. doi:10.1016/j.anai.2018.09.034
Busa, L. S. A., Mohammadi, S., Maeki, M., Ishida, A., Tani, H., and Tokeshi, M. (2016). Advances in microfluidic paper-based analytical devices for food and water analysis. Micromachines 7, 86. doi:10.3390/mi7050086
Cai, L., Xu, C., Lin, S., Luo, J., Wu, M., and Yang, F. (2014). A simple paper-based sensor fabricated by selective wet etching of silanized filter paper using a paper mask. Biomicrofluidics 8, 056504. doi:10.1063/1.4898096
Calabretta, M. M., Zangheri, M., Calabria, D., Lopreside, A., Montali, L., Marchegiani, E., et al. (2021). Paper-based immunosensors with bio-chemiluminescence detection. Sensors 21, 4309. doi:10.3390/s21134309
Carrell, C. S., Wydallis, R. M., Bontha, M., Boehle, K. E., Beveridge, J. R., Geiss, B. J., et al. (2019). Rotary manifold for automating a paper-based Salmonella immunoassay. RSC Adv. 9, 29078–29086. doi:10.1039/c9ra07106g
Carrilho, E., Martinez, A. W., and Whitesides, G. M. (2009). Understanding wax printing: a simple micropatterning process for paper-based microfluidics. Anal. Chem. 81, 7091–7095. doi:10.1021/ac901071p
Cate, D. M., Dungchai, W., Cunningham, J. C., Volckens, J., and Henry, C. S. (2013). Simple, distance-based measurement for paper analytical devices. Lab a Chip 13, 2397–2404. doi:10.1039/c3lc50072a
Cate, D. M., Noblitt, S. D., Volckens, J., and Henry, C. S. (2015). Multiplexed paper analytical device for quantification of metals using distance-based detection. Lab a Chip 15, 2808–2818. doi:10.1039/c5lc00364d
Chaiyo, S., Kunpatee, K., Kalcher, K., Yakoh, A., and Pungjunun, K. (2024). 3D paper-based device integrated with a battery-less NFC potentiostat for nonenzymatic detection of cholesterol. ACS Meas. Sci. Au 4, 432–441. doi:10.1021/acsmeasuresciau.4c00012
Chellasamy, G., Ankireddy, S. R., Lee, K.-N., Govindaraju, S., and Yun, K. (2021). Smartphone-integrated colorimetric sensor array-based reader system and fluorometric detection of dopamine in male and female geriatric plasma by bluish-green fluorescent carbon quantum dots. Mater. Today Bio 12, 100168. doi:10.1016/j.mtbio.2021.100168
Chen, J. L., Njoku, D. I., Tang, C., Gao, Y., Chen, J., Peng, Y. K., et al. (2024). Advances in microfluidic paper-based analytical devices (µPADs): design, fabrication, and applications. Small Methods 8, 2400155. doi:10.1002/smtd.202400155
Chhorn, S., and Teeramongkonrasmee, A. (2018) “A portable USB-controlled potentiostat for paper-based electrochemical applications,” in 2018 15th international conference on electrical engineering/electronics, computer, telecommunications and information technology (ECTI-CON), 2018. IEEE, 321–324.
Chiang, C.-K., Kurniawan, A., Kao, C.-Y., and Wang, M.-J. (2019). Single step and mask-free 3D wax printing of microfluidic paper-based analytical devices for glucose and nitrite assays. Talanta 194, 837–845. doi:10.1016/j.talanta.2018.10.104
Chinnadayyala, S. R., Park, J., Le, H. T. N., Santhosh, M., Kadam, A. N., and Cho, S. (2019). Recent advances in microfluidic paper-based electrochemiluminescence analytical devices for point-of-care testing applications. Biosens. Bioelectron. 126, 68–81. doi:10.1016/j.bios.2018.10.038
Chitnis, G., Ding, Z., Chang, C.-L., Savran, C. A., and Ziaie, B. (2011). Laser-treated hydrophobic paper: an inexpensive microfluidic platform. Lab a Chip 11, 1161–1165. doi:10.1039/c0lc00512f
Choi, K., Ng, A. H., Fobel, R., and Wheeler, A. R. (2012). Digital microfluidics. Annu. Rev. Anal. Chem. 5, 413–440. doi:10.1146/annurev-anchem-062011-143028
Chong, K. W. Y., Thong, Z., and Syn, C. K. C. (2021) Recent trends and developments in forensic DNA extraction, 3. Wiley Interdisciplinary Reviews: Forensic Science.e1395
Colozza, N., Kehe, K., Dionisi, G., Popp, T., Tsoutsoulopoulos, A., Steinritz, D., et al. (2019). A wearable origami-like paper-based electrochemical biosensor for sulfur mustard detection. Biosens. Bioelectron. 129, 15–23. doi:10.1016/j.bios.2019.01.002
Comer, J. (1956). Semiquantitative specific test paper for glucose in urine. Anal. Chem. 28, 1748–1750. doi:10.1021/ac60119a030
Curto, V. F., Lopez-Ruiz, N., Capitan-Vallvey, L. F., Palma, A. J., Benito-Lopez, F., and Diamond, D. (2013). Fast prototyping of paper-based microfluidic devices by contact stamping using indelible ink. RSC Adv. 3, 18811–18816. doi:10.1039/c3ra43825b
Das, D., Dsouza, A., Kaur, N., Soni, S., and Toley, B. J. (2019). Paper stacks for uniform rehydration of dried reagents in paper microfluidic devices. Sci. Rep. 9, 15755. doi:10.1038/s41598-019-52202-9
Das, D., Masetty, M., and Priye, A. (2023). Paper-based loop mediated isothermal amplification (LAMP) platforms: integrating the versatility of paper microfluidics with accuracy of nucleic acid amplification tests. Chemosensors 11, 163. doi:10.3390/chemosensors11030163
Davaji, B., and Lee, C. H. (2014). A paper-based calorimetric microfluidics platform for bio-chemical sensing. Biosens. Bioelectron. 59, 120–126. doi:10.1016/j.bios.2014.03.022
De Araujo, W. R., Lukas, H., Torres, M. D., Gao, W., and De La Fuente-Nunez, C. (2024). Low-cost biosensor technologies for rapid detection of COVID-19 and future pandemics. ACS Nano 18, 1757–1777. doi:10.1021/acsnano.3c01629
Delaney, J. L., Hogan, C. F., Tian, J., and Shen, W. (2011). Electrogenerated chemiluminescence detection in paper-based microfluidic sensors. Anal. Chem. 83, 1300–1306. doi:10.1021/ac102392t
Demirel, G., and Babur, E. (2014). Vapor-phase deposition of polymers as a simple and versatile technique to generate paper-based microfluidic platforms for bioassay applications. Analyst 139, 2326–2331. doi:10.1039/c4an00022f
De Oliveira, R. A., Camargo, F., Pesquero, N. C., and Faria, R. C. (2017). A simple method to produce 2D and 3D microfluidic paper-based analytical devices for clinical analysis. Anal. Chim. acta 957, 40–46. doi:10.1016/j.aca.2017.01.002
Deroco, P. B., Wachholz Junior, D., and Kubota, L. T. (2023). Paper-based wearable electrochemical sensors: a new generation of analytical devices. Electroanalysis 35, e202200177. doi:10.1002/elan.202200177
Desa, U. (2014) “World urbanization prospects, the 2011 revision,” in Population division. United Nations Secretariat: department of economic and social affairs.
de Araujo, W. R., Frasson, C. M., Ameku, W. A., Silva, J. R., Angnes, L., and Paixão, T. R. (2017). Single-step reagentless laser scribing fabrication of electrochemical paper-based analytical devices. Angew. Chem. 129, 15309–15313. doi:10.1002/ange.201708527
Dornelas, K. L., Dossi, N., and Piccin, E. (2015). A simple method for patterning poly (dimethylsiloxane) barriers in paper using contact-printing with low-cost rubber stamps. Anal. Chim. acta 858, 82–90. doi:10.1016/j.aca.2014.11.025
Dortez, S., Crevillen, A. G., Escarpa, A., and Cinti, S. (2024). Electroanalytical paper-based device for reliable detection and quantification of sugars in milk. Sensors Actuators B Chem. 398, 134704. doi:10.1016/j.snb.2023.134704
Dungchai, W., Chailapakul, O., and Henry, C. S. (2009). Electrochemical detection for paper-based microfluidics. Anal. Chem. 81, 5821–5826. doi:10.1021/ac9007573
Dungchai, W., Chailapakul, O., and Henry, C. S. (2011). A low-cost, simple, and rapid fabrication method for paper-based microfluidics using wax screen-printing. Analyst 136, 77–82. doi:10.1039/c0an00406e
Fang, L., Jiang, J., Wang, J., and Deng, C. (2014). Non-uniform capillary model for unidirectional fiber bundles considering pore size distribution. J. Reinf. Plastics Compos. 33, 1430–1440. doi:10.1177/0731684414533739
Fan, Y., Liu, J., Wang, Y., Luo, J., Xu, H., Xu, S., et al. (2017). A wireless point-of-care testing system for the detection of neuron-specific enolase with microfluidic paper-based analytical devices. Biosens. Bioelectron. 95, 60–66. doi:10.1016/j.bios.2017.04.003
Fenton, E. M., Mascarenas, M. R., López, G. P., and Sibbett, S. S. (2009). Multiplex lateral-flow test strips fabricated by two-dimensional shaping. ACS Appl. Mater. and interfaces 1, 124–129. doi:10.1021/am800043z
Flaucher, M., Nissen, M., Jaeger, K. M., Titzmann, A., Pontones, C., Huebner, H., et al. (2022). Smartphone-based colorimetric analysis of urine test strips for at-home prenatal care. IEEE J. Transl. Eng. Health Med. 10, 1–9. doi:10.1109/jtehm.2022.3179147
Flinck, H., Kerimov, D., Luukinen, B., Seiskari, T., and Aittoniemi, J. (2022). Evaluation of the Roche-SD Biosensor rapid antigen test: antigen is not reliable in detecting SARS-CoV-2 at the early stage of infection with respiratory symptoms. Diagnostic Microbiol. Infect. Dis. 102, 115628. doi:10.1016/j.diagmicrobio.2021.115628
Fobel, R., Kirby, A. E., Ng, A. H., Farnood, R. R., and Wheeler, A. R. (2014). Paper microfluidics goes digital. Adv. Mater. 26, 2838–2843. doi:10.1002/adma.201305168
Gałuszka, A., Migaszewski, Z., and Namieśnik, J. (2013). The 12 principles of green analytical chemistry and the SIGNIFICANCE mnemonic of green analytical practices. TrAC Trends Anal. Chem. 50, 78–84. doi:10.1016/j.trac.2013.04.010
Gaydos, C., and Hardick, J. (2014). Point of care diagnostics for sexually transmitted infections: perspectives and advances. Expert Rev. anti-infective Ther. 12, 657–672. doi:10.1586/14787210.2014.880651
Ge, L., Wang, P., Ge, S., Li, N., Yu, J., Yan, M., et al. (2013). Photoelectrochemical lab-on-paper device based on an integrated paper supercapacitor and internal light source. Anal. Chem. 85, 3961–3970. doi:10.1021/ac4001496
Ge, L., Yan, J., Song, X., Yan, M., Ge, S., and Yu, J. (2012). Three-dimensional paper-based electrochemiluminescence immunodevice for multiplexed measurement of biomarkers and point-of-care testing. Biomaterials 33, 1024–1031. doi:10.1016/j.biomaterials.2011.10.065
Ge, L., Yu, J., Ge, S., and Yan, M. (2014). Lab-on-paper-based devices using chemiluminescence and electrogenerated chemiluminescence detection. Anal. Bioanal. Chem. 406, 5613–5630. doi:10.1007/s00216-014-7756-1
Ghosh, R., Gopalakrishnan, S., Savitha, R., Renganathan, T., and Pushpavanam, S. (2019). Fabrication of laser printed microfluidic paper-based analytical devices (LP-µPADs) for point-of-care applications. Sci. Rep. 9, 7896. doi:10.1038/s41598-019-44455-1
Gökdere, B., Üzer, A., Durmazel, S., Erçağ, E., and Apak, R. (2019). Titanium dioxide nanoparticles–based colorimetric sensors for determination of hydrogen peroxide and triacetone triperoxide (TATP). Talanta 202, 402–410. doi:10.1016/j.talanta.2019.04.071
Gong, F., Wei, H.-X., Qi, J., Ma, H., Liu, L., Weng, J., et al. (2021). Pulling-force spinning top for serum separation combined with paper-based microfluidic devices in COVID-19 ELISA diagnosis. ACS sensors 6, 2709–2719. doi:10.1021/acssensors.1c00773
Gonzalez-Macia, L., Li, Y., Zhang, K., Nunez-Bajo, E., Barandun, G., Cotur, Y., et al. (2024). NFC-enabled potentiostat and nitrocellulose-based metal electrodes for electrochemical lateral flow assay. Biosens. Bioelectron. 251, 116124. doi:10.1016/j.bios.2024.116124
Guan, L., Tian, J., Cao, R., Li, M., Cai, Z., and Shen, W. (2014). Barcode-like paper sensor for smartphone diagnostics: an application of blood typing. Anal. Chem. 86, 11362–11367. doi:10.1021/ac503300y
Han, T., Jin, Y., Geng, C., Aziz, A. U. R., Zhang, Y., Deng, S., et al. (2020). Microfluidic paper-based analytical devices in clinical applications. Chromatographia 83, 693–701. doi:10.1007/s10337-020-03892-1
Hao, Z., Chen, H., Shi, X., Tan, W., and Zhu, G. (2021). Fabrication for paper-based microfluidic analytical devices and saliva analysis application. Microfluid. Nanofluidics 25, 80. doi:10.1007/s10404-021-02476-1
Harshey, A., Kumar, A., Kumar, A., Das, T., Nigam, K., and Srivastava, A. (2023). Focusing the intervention of paper-based microfluidic devices for the forensic investigative purposes. Microfluid. Nanofluidics 27, 65. doi:10.1007/s10404-023-02674-z
Hasandka, A., Singh, A. R., Prabhu, A., Singhal, H. R., Nandagopal, M. S. G., and Mani, N. K. (2022). Paper and thread as media for the frugal detection of urinary tract infections (UTIs). Anal. Bioanal. Chem. 414, 847–865. doi:10.1007/s00216-021-03671-3
Hawkes, R., Niday, E., and Gordon, J. (1982). A dot-immunobinding assay for monoclonal and other antibodies. Anal. Biochem. 119, 142–147. doi:10.1016/0003-2697(82)90677-7
He, P. J., Katis, I. N., Eason, R. W., and Sones, C. L. (2015a). Laser-based patterning for fluidic devices in nitrocellulose. Biomicrofluidics 9, 026503. doi:10.1063/1.4919629
Hefner, C. E., Aryal, P., Brack, E., Alexander, T., and Henry, C. S. (2024). Capillary-flow driven microfluidic sensor based on tyrosinase for fast user-friendly assessment of pesticide exposures. Analyst 149, 5684–5692. doi:10.1039/d4an01203h
Hegener, M. A., Li, H., Han, D., Steckl, A. J., and Pauletti, G. M. (2017). Point-of-care coagulation monitoring: first clinical experience using a paper-based lateral flow diagnostic device. Biomed. microdevices 19, 64–69. doi:10.1007/s10544-017-0206-z
He, H., Li, R., Chen, Y., Pan, P., Tong, W., Dong, X., et al. (2017). Integrated DNA and RNA extraction using magnetic beads from viral pathogens causing acute respiratory infections. Sci. Rep. 7, 45199. doi:10.1038/srep45199
He, Y., Gao, Q., Wu, W.-B., Nie, J., and Fu, J.-Z. (2016). 3D printed paper-based microfluidic analytical devices. Micromachines 7, 108. doi:10.3390/mi7070108
He, Y., Wu, W.-B., and Fu, J.-Z. (2015b). Rapid fabrication of paper-based microfluidic analytical devices with desktop stereolithography 3D printer. RSC Adv. 5, 2694–2701. doi:10.1039/c4ra12165a
He, Y., Wu, Y., Fu, J.-Z., and Wu, W.-B. (2015c). Fabrication of paper-based microfluidic analysis devices: a review. Rsc Adv. 5, 78109–78127. doi:10.1039/c5ra09188h
He, Y., Wu, Y., Xiao, X., Fu, J., and Xue, G. (2014). A low-cost and rapid microfluidic paper-based analytical device fabrication method: flash foam stamp lithography. RSC Adv. 4, 63860–63865. doi:10.1039/c4ra11150h
Hristov, D., Rijal, H., Gomez-Marquez, J., and Hamad-Schifferli, K. (2021). Developing a paper-based antigen assay to differentiate between coronaviruses and SARS-CoV-2 spike variants. Anal. Chem. 93, 7825–7832. doi:10.1021/acs.analchem.0c05438
Hua, M. Z., Li, S., Wang, S., and Lu, X. (2018). Detecting chemical hazards in foods using microfluidic paper-based analytical devices (μPADs): the real-world application. Micromachines 9, 32. doi:10.3390/mi9010032
Huang, G.-W., Feng, Q.-P., Xiao, H.-M., Li, N., and Fu, S.-Y. (2016). Rapid laser printing of paper-based multilayer circuits. ACS Nano 10, 8895–8903. doi:10.1021/acsnano.6b04830
Huber, M., Wei, T.-F., Müller, U. R., Lefebvre, P. A., Marla, S. S., and Bao, Y. P. (2004). Gold nanoparticle probe-based gene expression analysis with unamplified total human RNA. Nucleic Acids Res. 32, e137. doi:10.1093/nar/gnh133
Hu, C., Annese, V. F., Velugotla, S., Al-Rawhani, M., Cheah, B. C., Grant, J., et al. (2020). Disposable paper-on-CMOS platform for real-time simultaneous detection of metabolites. IEEE Trans. Biomed. Eng. 67, 2417–2426. doi:10.1109/tbme.2019.2962239
Jaewjaroenwattana, J., Phoolcharoen, W., Pasomsub, E., Teengam, P., and Chailapakul, O. (2023). Electrochemical paper-based antigen sensing platform using plant-derived monoclonal antibody for detecting SARS-CoV-2. Talanta 251, 123783. doi:10.1016/j.talanta.2022.123783
Jang, I., Kang, H., Song, S., Dandy, D. S., Geiss, B. J., and Henry, C. S. (2021). Flow control in a laminate capillary-driven microfluidic device. Analyst 146, 1932–1939. doi:10.1039/d0an02279a
Jarujamrus, P., Tian, J., Li, X., Siripinyanond, A., Shiowatana, J., and Shen, W. (2012). Mechanisms of red blood cells agglutination in antibody-treated paper. Analyst 137, 2205–2210. doi:10.1039/c2an15798e
Jauset-Rubio, M., Svobodová, M., Mairal, T., Mcneil, C., Keegan, N., Saeed, A., et al. (2016). Ultrasensitive, rapid and inexpensive detection of DNA using paper based lateral flow assay. Sci. Rep. 6, 37732. doi:10.1038/srep37732
Jeong, S.-G., Lee, S.-H., Choi, C.-H., Kim, J., and Lee, C.-S. (2015). Toward instrument-free digital measurements: a three-dimensional microfluidic device fabricated in a single sheet of paper by double-sided printing and lamination. Lab a Chip 15, 1188–1194. doi:10.1039/c4lc01382d
Jiang, Y., Hao, Z., He, Q., and Chen, H. (2016). A simple method for fabrication of microfluidic paper-based analytical devices and on-device fluid control with a portable corona generator. RSC Adv. 6, 2888–2894. doi:10.1039/c5ra23470k
Shetty, P. J., and Shetty, P. J. (2020). The evolution of DNA extraction methods. Am. J. Biomed. Sci. and Res. 8, 39–45. doi:10.34297/ajbsr.2020.08.001234
Kaewarsa, P., Vilaivan, T., and Laiwattanapaisal, W. (2021). An origami paper-based peptide nucleic acid device coupled with label-free DNAzyme probe hybridization chain reaction for prostate cancer molecular screening test. Anal. Chim. Acta 1186, 339130. doi:10.1016/j.aca.2021.339130
Kamakoti, V., Kinnamon, D., Choi, K. H., Jagannath, B., Ramasamy, M., and Prasad, S. (2023) “Fully electronic urine dipstick probe for combinatorial detection of inflammatory biomarkers,” in Advances in medical imaging, detection, and diagnosis. Singapore: Jenny Stanford Publishing.
Kao, P.-K., and Hsu, C.-C. (2014). Battery-operated, portable, and flexible air microplasma generation device for fabrication of microfluidic paper-based analytical devices on demand. Anal. Chem. 86, 8757–8762. doi:10.1021/ac501945q
Karita, S., and Kaneta, T. (2014). Acid–base titrations using microfluidic paper-based analytical devices. Anal. Chem. 86, 12108–12114. doi:10.1021/ac5039384
Kasetsirikul, S., Umer, M., Soda, N., Sreejith, K. R., Shiddiky, M. J., and Nguyen, N.-T. (2020). Detection of the SARS-CoV-2 humanized antibody with paper-based ELISA. Analyst 145, 7680–7686. doi:10.1039/d0an01609h
Kasetsirikul, S., Umer, M., Soda, N., Sreejith, K. R., Shiddiky, M. J., and Nguyen, N.-T. (2021). A paper-based immunofluorescent device for the detection of SARS-CoV-2 humanized antibody.
Khachornsakkul, K., Del-Rio-Ruiz, R., Creasey, H., Widmer, G., and Sonkusale, S. R. (2023). Gold nanomaterial-based microfluidic paper analytical device for simultaneous quantification of gram-negative bacteria and nitrite ions in water samples. ACS sensors 8, 4364–4373. doi:10.1021/acssensors.3c01769
Khan, S. M., Gumus, A., Nassar, J. M., and Hussain, M. M. (2018). CMOS enabled microfluidic systems for healthcare based applications. Adv. Mater. 30, 1705759. doi:10.1002/adma.201705759
Kim, S., and Lee, J.-H. (2022). Current advances in paper-based biosensor technologies for rapid COVID-19 diagnosis. Biochip J. 16, 376–396. doi:10.1007/s13206-022-00078-9
Kong, Q., Cui, K., Zhang, L., Wang, Y., Sun, J., Ge, S., et al. (2018). “On–Off–On” Photoelectrochemical/visual lab-on-paper sensing via signal amplification of CdS quantum dots@ leaf-shape ZnO and quenching of Au-modified prism-anchored octahedral CeO2 nanoparticles. Anal. Chem. 90, 11297–11304. doi:10.1021/acs.analchem.8b01844
Krorakai, K., Klangphukhiew, S., Kulchat, S., and Patramanon, R. (2021). Smartphone-based NFC potentiostat for wireless electrochemical sensing. Appl. Sci. 11, 392. doi:10.3390/app11010392
Kumar, A. A., Hennek, J. W., Smith, B. S., Kumar, S., Beattie, P., Jain, S., et al. (2015). From the bench to the field in low-cost diagnostics: two case studies. Angew. Chem. Int. Ed. 54, 5836–5853. doi:10.1002/anie.201411741
Kumar, A. A., Patton, M. R., Hennek, J. W., Lee, S. Y. R., D’Alesio-Spina, G., Yang, X., et al. 2014. Density-based separation in multiphase systems provides a simple method to identify sickle cell disease, Proc. Natl. Acad. Sci. U. S. A. Density-based Sep. Multiph. Syst. provides a simple method identify Sick. Cell. Dis. 111, 14864–14869. doi:10.1073/pnas.1414739111
Kummari, S., Panicker, L. R., Rao Bommi, J., Karingula, S., Sunil Kumar, V., Mahato, K., et al. (2023). Trends in paper-based sensing devices for clinical and environmental monitoring. Biosensors 13, 420. doi:10.3390/bios13040420
Kwon, H., Samain, F., and Kool, E. T. (2012). Fluorescent DNAs printed on paper: sensing food spoilage and ripening in the vapor phase. Chem. Sci. 3, 2542–2549. doi:10.1039/c2sc20461d
Lazaro, A., Villarino, R., Lazaro, M., Canellas, N., Prieto-Simon, B., and Girbau, D. (2023). Recent advances in batteryless NFC sensors for chemical sensing and biosensing. Biosensors 13, 775. doi:10.3390/bios13080775
Lee, M., Oh, K., Choi, H.-K., Lee, S. G., Youn, H. J., Lee, H. L., et al. (2018). Subnanomolar sensitivity of filter paper-based SERS sensor for pesticide detection by hydrophobicity change of paper surface. ACS sensors 3, 151–159. doi:10.1021/acssensors.7b00782
Le, S., Zhou, H., Nie, J., Cao, C., Yang, J., Pan, H., et al. (2017). Fabrication of paper devices via laser-heating-wax-printing for high-tech enzyme-linked immunosorbent assays with low-tech pen-type pH meter readout. Analyst 142, 511–516. doi:10.1039/c6an02422j
Lewis, G. G., Ditucci, M. J., and Phillips, S. T. (2012). Quantifying analytes in paper-based microfluidic devices without using external electronic readers. Angew. Chem. Int. Ed. 51, 12707–12710. doi:10.1002/anie.201207239
Lewis, G. G., Robbins, J. S., and Phillips, S. T. (2013). Point-of-care assay platform for quantifying active enzymes to femtomolar levels using measurements of time as the readout. Anal. Chem. 85, 10432–10439. doi:10.1021/ac402415v
Li, Y. Q., and Feng, L. (2020). Progress in paper-based colorimetric sensor array. Chin. J. Anal. Chem. 48, 1448–1457. doi:10.1016/s1872-2040(20)60057-3
Li, A., Wang, G., Zhang, Y., Zhang, J., He, W., Ren, S., et al. (2021). Preparation methods and research progress of superhydrophobic paper. Coord. Chem. Rev. 449, 214207. doi:10.1016/j.ccr.2021.214207
Liang, J., Wang, Y., and Liu, B. (2012). Paper-based fluoroimmunoassay for rapid and sensitive detection of antigen. RSC Adv. 2, 3878–3884. doi:10.1039/c2ra20156a
Lian, J., Xu, Q., Wang, Y., and Meng, F. (2020). Recent developments in fluorescent materials for heavy metal ions analysis from the perspective of forensic chemistry. Front. Chem. 8, 593291. doi:10.3389/fchem.2020.593291
Li, F., Liu, J., Guo, L., Wang, J., Zhang, K., He, J., et al. (2019). High-resolution temporally resolved chemiluminescence based on double-layered 3D microfluidic paper-based device for multiplexed analysis. Biosens. Bioelectron. 141, 111472. doi:10.1016/j.bios.2019.111472
Li, J., and Macdonald, J. (2016). Multiplex lateral flow detection and binary encoding enables a molecular colorimetric 7-segment display. Lab a Chip 16, 242–245. doi:10.1039/c5lc01323b
Li, M., Cao, R., Nilghaz, A., Guan, L., Zhang, X., and Shen, W. (2015). “Periodic-table-style” paper device for monitoring heavy metals in water. Anal. Chem. 87, 2555–2559. doi:10.1021/acs.analchem.5b00040
Li, M., Tian, J., Al-Tamimi, M., and Shen, W. (2012a). Paper-Based blood typing device that reports patient’s blood type “in writing”. Angew. Chem. 124, 5593–5597. doi:10.1002/ange.201201822
Lim, H., Jafry, A. T., and Lee, J. (2019). Fabrication, flow control, and applications of microfluidic paper-based analytical devices. Molecules 24, 2869. doi:10.3390/molecules24162869
Liu, C.-J., Lien, K.-Y., Weng, C.-Y., Shin, J.-W., Chang, T.-Y., and Lee, G.-B. (2009). Magnetic-bead-based microfluidic system for ribonucleic acid extraction and reverse transcription processes. Biomed. microdevices 11, 339–350. doi:10.1007/s10544-008-9240-1
Liu, B., Du, D., Hua, X., Yu, X. Y., and Lin, Y. (2014a). Paper-based electrochemical biosensors: from test strips to paper-based microfluidics. Electroanalysis 26, 1214–1223. doi:10.1002/elan.201400036
Liu, J., Geng, Q., and Geng, Z. (2024). A route to the colorimetric detection of alpha-fetoprotein based on a smartphone. Micromachines 15, 1116. doi:10.3390/mi15091116
Liu, N., Xu, J., An, H.-J., Phan, D.-T., Hashimoto, M., and Lew, W. S. (2017). Direct spraying method for fabrication of paper-based microfluidic devices. J. Micromechanics Microengineering 27, 104001. doi:10.1088/1361-6439/aa82ce
Liu, Q., Wang, J., Wang, B., Li, Z., Huang, H., Li, C., et al. (2014b). Paper-based plasmonic platform for sensitive, noninvasive, and rapid cancer screening. Biosens. Bioelectron. 54, 128–134. doi:10.1016/j.bios.2013.10.067
Liu, S., Su, W., and Ding, X. (2016). A review on microfluidic paper-based analytical devices for glucose detection. Sensors 16, 2086. doi:10.3390/s16122086
Liu, W., Cassano, C. L., Xu, X., and Fan, Z. H. (2013). Laminated paper-based analytical devices (LPAD) with origami-enabled chemiluminescence immunoassay for cotinine detection in mouse serum. Anal. Chem. 85, 10270–10276. doi:10.1021/ac402055n
Li, X., Ballerini, D. R., and Shen, W. (2012b). A perspective on paper-based microfluidics: current status and future trends. Biomicrofluidics 6, 011301–1130113. doi:10.1063/1.3687398
Li, X., Tian, J., Nguyen, T., and Shen, W. (2008). Paper-based microfluidic devices by plasma treatment. Anal. Chem. 80, 9131–9134. doi:10.1021/ac801729t
Lobato, I. M., and O'Sullivan, C. K. (2018). Recombinase polymerase amplification: basics, applications and recent advances. Trac Trends Anal. Chem. 98, 19–35. doi:10.1016/j.trac.2017.10.015
Lu, Y., Shi, W., Jiang, L., Qin, J., and Lin, B. (2009). Rapid prototyping of paper-based microfluidics with wax for low-cost, portable bioassay. Electrophoresis 30, 1497–1500. doi:10.1002/elps.200800563
Mace, C. R., Akbulut, O., Kumar, A. A., Shapiro, N. D., Derda, R., Patton, M. R., et al. (2012). Aqueous multiphase systems of polymers and surfactants provide self-assembling step-gradients in density. J. Am. Chem. Soc. 134, 9094–9097. doi:10.1021/ja303183z
Maejima, K., Tomikawa, S., Suzuki, K., and Citterio, D. (2013). Inkjet printing: an integrated and green chemical approach to microfluidic paper-based analytical devices. RSC Adv. 3, 9258–9263. doi:10.1039/c3ra40828k
Mahmud, M. A., Blondeel, E. J., and Macdonald, B. D. (2020). Counting-based microfluidic paper-based devices capable of analyzing submicroliter sample volumes. Biomicrofluidics 14, 014107. doi:10.1063/1.5131751
Malpartida-Cardenas, K., Baum, J., Cunnington, A., Georgiou, P., and Rodriguez-Manzano, J. (2023). A dual paper-based nucleic acid extraction method from blood in under ten minutes for point-of-care diagnostics. Analyst 148, 3036–3044. doi:10.1039/d3an00296a
Mani, N. K., Prabhu, A., Biswas, S. K., and Chakraborty, S. (2019). Fabricating paper based devices using correction pens. Sci. Rep. 9, 1752. doi:10.1038/s41598-018-38308-6
Mark, D., Haeberle, S., Roth, G., Von Stetten, F., and Zengerle, R. (2010). Microfluidic lab-on-a-chip platforms: requirements, characteristics and applications. NATO Sci. Peace Secur. Ser. A Chem. Biol., 305–376. doi:10.1007/978-90-481-9029-4_17
Martinez, A. W., Phillips, S. T., Butte, M. J., and Whitesides, G. M. (2007). Patterned paper as a platform for inexpensive, low-volume, portable bioassays. Angew. Chem. - Int. Ed. 46, 1318–1320. doi:10.1002/anie.200603817
Martinez, A. W., Phillips, S. T., Carrilho, E., Thomas, S. W., Sindi, H., and Whitesides, G. M. (2008a). Simple telemedicine for developing regions: camera phones and paper-based microfluidic devices for real-time, off-site diagnosis. Anal. Chem. 80, 3699–3707. doi:10.1021/ac800112r
Martinez, A. W., Phillips, S. T., Whitesides, G. M., and Carrilho, E. (2010). Diagnostics for the developing world: microfluidic paper-based analytical devices. Anal. Chem. 82, 3–10. doi:10.1021/ac9013989
Martinez, A. W., Phillips, S. T., Wiley, B. J., Gupta, M., and Whitesides, G. M. (2008b). FLASH: a rapid method for prototyping paper-based microfluidic devices. Lab a Chip 8, 2146–2150. doi:10.1039/b811135a
Martínez-García, G., Pérez-Julián, E., Agüí, L., Cabré, N., Joven, J., Yáñez-Sedeño, P., et al. (2017). An electrochemical enzyme biosensor for 3-hydroxybutyrate detection using screen-printed electrodes modified by reduced graphene oxide and thionine. Biosensors 7, 50. doi:10.3390/bios7040050
Mettakoonpitak, J., Boehle, K., Nantaphol, S., Teengam, P., Adkins, J. A., Srisa-Art, M., et al. (2016). Electrochemistry on paper-based analytical devices: a review. Electroanalysis 28, 1420–1436. doi:10.1002/elan.201501143
Mitrogiannopoulou, A.-M., Tselepi, V., and Ellinas, K. (2023). Polymeric and paper-based lab-on-a-chip devices in food safety: a review. Micromachines 14, 986. doi:10.3390/mi14050986
Monju, T., Hirakawa, M., Kuboyama, S., Saiki, R., and Ishida, A. (2023). A fabrication technique for paper-based analytical devices via two-sided patterning with thermal-transfer printer and laminator. Sensors Actuators B Chem. 375, 132886. doi:10.1016/j.snb.2022.132886
Morbioli, G. G., Mazzu-Nascimento, T., Stockton, A. M., and Carrilho, E. (2017). Technical aspects and challenges of colorimetric detection with microfluidic paper-based analytical devices (μPADs)-A review. Anal. Chim. acta 970, 1–22. doi:10.1016/j.aca.2017.03.037
Moreno-Roman, P., and Bobick, K. (2022). Foldscope: increasing science accessibility worldwide. Microsc. Today 30, 42–45. doi:10.1017/s1551929522000633
Musile, G., Grazioli, C., Fornasaro, S., Dossi, N., De Palo, E. F., Tagliaro, F., et al. (2023). Application of paper-based microfluidic analytical devices (µPAD) in forensic and clinical toxicology: a review. Biosensors 13, 743. doi:10.3390/bios13070743
Nadar, S. S., Patil, P. D., Tiwari, M. S., and Ahirrao, D. J. (2021). Enzyme embedded microfluidic paper-based analytic device (μPAD): a comprehensive review. Crit. Rev. Biotechnol. 41, 1046–1080. doi:10.1080/07388551.2021.1898327
Nawaz, H., Zhang, X., Chen, S., You, T., and Xu, F. (2021). Recent studies on cellulose-based fluorescent smart materials and their applications: a comprehensive review. Carbohydr. Polym. 267, 118135. doi:10.1016/j.carbpol.2021.118135
Nilghaz, A., Mousavi, S. M., Li, M., Tian, J., Cao, R., and Wang, X. (2021). Paper-based microfluidics for food safety and quality analysis. Trends Food Sci. and Technol. 118, 273–284. doi:10.1016/j.tifs.2021.08.029
Noble, L., Scott, L., Stewart-Isherwood, L., Molifi, S. J., Sanne, I., Da Silva, P., et al. (2020). Continuous quality monitoring in the field: an evaluation of the performance of the Fio Deki Reader™ for rapid HIV testing in South Africa. BMC Infect. Dis. 20, 320. doi:10.1186/s12879-020-4932-0
Noviana, E., Carrão, D. B., Pratiwi, R., and Henry, C. S. (2020a). Emerging applications of paper-based analytical devices for drug analysis: a review. Anal. Chim. Acta 1116, 70–90. doi:10.1016/j.aca.2020.03.013
Noviana, E., Mccord, C. P., Clark, K. M., Jang, I., and Henry, C. S. (2020b). Electrochemical paper-based devices: sensing approaches and progress toward practical applications. Lab a Chip 20, 9–34. doi:10.1039/c9lc00903e
Noviana, E., Ozer, T., Carrell, C. S., Link, J. S., Mcmahon, C., Jang, I., et al. (2021). Microfluidic paper-based analytical devices: from design to applications. Chem. Rev. 121, 11835–11885. doi:10.1021/acs.chemrev.0c01335
Nurak, T., Praphairaksit, N., and Chailapakul, O. (2013). Fabrication of paper-based devices by lacquer spraying method for the determination of nickel (II) ion in waste water. Talanta 114, 291–296. doi:10.1016/j.talanta.2013.05.037
Oh, J.-M., and Chow, K.-F. (2015). Recent developments in electrochemical paper-based analytical devices. Anal. Methods 7, 7951–7960. doi:10.1039/c5ay01724f
Olkkonen, J., Lehtinen, K., and Erho, T. (2010). Flexographically printed fluidic structures in paper. Anal. Chem. 82, 10246–10250. doi:10.1021/ac1027066
Oruganti, S., Gundimeda, S. L., Buddolla, V., Lakshmi, B. A., and Kim, Y.-J. (2023). Based diagnostic chips for viral detection. Clin. Chim. Acta 117413. doi:10.1016/j.cca.2023.117413
Ozer, T., Mcmahon, C., and Henry, C. S. (2020). Advances in paper-based analytical devices. Annu. Rev. Anal. Chem. 13, 85–109. doi:10.1146/annurev-anchem-061318-114845
Park, C., Han, Y. D., Kim, H. V., Lee, J., Yoon, H. C., and Park, S. (2018). Double-sided 3D printing on paper towards mass production of three-dimensional paper-based microfluidic analytical devices (3D-μPADs). Lab a Chip 18, 1533–1538. doi:10.1039/c8lc00367j
Patel, S., Jamunkar, R., Sinha, D., Patle, T. K., Patle, T. K., Kant, T., et al. (2021). Recent development in nanomaterials fabricated paper-based colorimetric and fluorescent sensors: a review. Trends Environ. Anal. Chem. 31, e00136. doi:10.1016/j.teac.2021.e00136
Pena-Pereira, F., Wojnowski, W., and Tobiszewski, M. (2020). AGREE—analytical GREEnness metric approach and software. Anal. Chem. 92, 10076–10082. doi:10.1021/acs.analchem.0c01887
Pereira de Oliveira, L., Rocha, D. P., Reis de Araujo, W., Abarza Muñoz, R. A., Longo Cesar Paixão, T. R., and Oliveira Salles, M. (2018). Forensics in hand: new trends in forensic devices (2013–2017). Anal. methods 10, 5135–5163. doi:10.1039/c8ay01389f
Pinheiro, T., Cardoso, A. R., Sousa, C. E., Marques, A. C., Tavares, A. P., Matos, A. M., et al. (2021). Paper-based biosensors for COVID-19: a review of innovative tools for controlling the pandemic. ACS omega 6, 29268–29290. doi:10.1021/acsomega.1c04012
Pohanka, M., and Skládal, P. (2008). Electrochemical biosensors-principles and applications. J. Appl. Biomed. 6, 57–64. doi:10.32725/jab.2008.008
Pollock, N. R., Mcgray, S., Colby, D. J., Noubary, F., Nguyen, H., Nguyen, T. A., et al. (2013). Field evaluation of a prototype paper-based point-of-care fingerstick transaminase test. PloS one 8, e75616. doi:10.1371/journal.pone.0075616
Pollreis, R. E., Roscoe, C., Phinney, R. J., Malesha, S. S., Burns, M. C., Ceniseros, A., et al. (2021). Evaluation of the Abbott BinaxNOW COVID-19 Test Ag Card for rapid detection of SARS-CoV-2 infection by a local public health district with a rural population. Plos one 16, e0260862. doi:10.1371/journal.pone.0260862
Prakobdi, C., Baldo, T. A., Aryal, P., Link, J., Saetear, P., and Henry, C. S. (2024). Non-invasive iron deficiency diagnosis: a saliva-based approach using capillary flow microfluidics. Anal. Methods 16, 2489–2495. doi:10.1039/d3ay01933k
Pramanik, B., Singha, N., and Das, D. (2019). Sol-gel-and paper-based detection of picric acid at femtogram level by a short peptide gelator. ACS Appl. Polym. Mater. 1, 833–843. doi:10.1021/acsapm.9b00071
Promsuwan, K., Soleh, A., Samoson, K., Saisahas, K., Wangchuk, S., Saichanapan, J., et al. (2023). Novel biosensor platform for glucose monitoring via smartphone based on battery-less NFC potentiostat. Talanta 256, 124266. doi:10.1016/j.talanta.2023.124266
Prosperi, C., Kaduskar, O., Bhatt, V., Hasan, A. Z., Vivian Thangaraj, J. W., Kumar, M. S., et al. (2021). Diagnostic accuracy of dried blood spots collected on HemaSpot HF devices compared to venous blood specimens to estimate measles and rubella seroprevalence. Msphere 6, e0133020. doi:10.1128/mSphere.01330-2001330-20
Punjiya, M., Mostafalu, P., and Sonkusale, S. (2014) “Low-cost paper-based electrochemical sensors with CMOS readout IC,” in 2014 IEEE biomedical circuits and systems conference (BioCAS) proceedings. IEEE, 324–327.
Qi, J., Fan, X. X., Deng, D. M., He, H. B., and Luo, L. Q. (2020). Progress in rapid detection techniques using paper-based platforms for food safety. Chin. J. Anal. Chem. 48, 1616–1624. doi:10.1016/s1872-2040(20)60064-0
Qi, J., Li, B., Wang, X., Fu, L., Luo, L., and Chen, L. (2018). Rotational paper-based microfluidic-chip device for multiplexed and simultaneous fluorescence detection of phenolic pollutants based on a molecular-imprinting technique. Anal. Chem. 90, 11827–11834. doi:10.1021/acs.analchem.8b01291
Qi, J., Li, B., Wang, X., Zhang, Z., Wang, Z., Han, J., et al. (2017). Three-dimensional paper-based microfluidic chip device for multiplexed fluorescence detection of Cu2+ and Hg2+ ions based on ion imprinting technology. Sensors Actuators B Chem. 251, 224–233. doi:10.1016/j.snb.2017.05.052
Ravi, N., Cortade, D. L., Ng, E., and Wang, S. X. (2020). Diagnostics for SARS-CoV-2 detection: a comprehensive review of the FDA-EUA COVID-19 testing landscape. Biosens. Bioelectron. 165, 112454. doi:10.1016/j.bios.2020.112454
Rawat, S., Misra, N., Shelkar, S. A., and Kumar, V. (2023). Tailoring Acid Free-Paper based Analytical Devices (Af-PADs) via radiation assisted modification of cellulose paper. Carbohydr. Polym. 317, 121116. doi:10.1016/j.carbpol.2023.121116
Richardson, S., Iles, A., Rotchell, J. M., Charlson, T., Hanson, A., Lorch, M., et al. (2021). Citizen-led sampling to monitor phosphate levels in freshwater environments using a simple paper microfluidic device. Plos one 16, e0260102. doi:10.1371/journal.pone.0260102
Ruecha, N., Lee, J., Chae, H., Cheong, H., Soum, V., Preechakasedkit, P., et al. (2017). Paper-based digital microfluidic chip for multiple electrochemical assay operated by a wireless portable control system. Adv. Mater. Technol. 2, 1600267. doi:10.1002/admt.201600267
Ruiz, R. A., Gonzalez, J. L., Vazquez-Alvarado, M., Martinez, N. W., and Martinez, A. W. (2022). Beyond wax printing: fabrication of paper-based microfluidic devices using a thermal transfer printer. Anal. Chem. 94, 8833–8837. doi:10.1021/acs.analchem.2c01534
Russo, M. J., Han, M., Desroches, P. E., Manasa, C. S., Dennaoui, J., Quigley, A. F., et al. (2021). Antifouling strategies for electrochemical biosensing: mechanisms and performance toward point of care based diagnostic applications. ACS sensors 6, 1482–1507. doi:10.1021/acssensors.1c00390
Sadri, B., Goswami, D., Sala De Medeiros, M., Pal, A., Castro, B., Kuang, S., et al. (2018). Wearable and implantable epidermal paper-based electronics. ACS Appl. Mater. and interfaces 10, 31061–31068. doi:10.1021/acsami.8b11020
Saez, J., Catalan-Carrio, R., Owens, R. M., Basabe-Desmonts, L., and Benito-Lopez, F. (2021). Microfluidics and materials for smart water monitoring: a review. Anal. Chim. Acta 1186, 338392. doi:10.1016/j.aca.2021.338392
Seok, Y., Joung, H.-A., Byun, J.-Y., Jeon, H.-S., Shin, S. J., Kim, S., et al. (2017). A paper-based device for performing loop-mediated isothermal amplification with real-time simultaneous detection of multiple DNA targets. Theranostics 7, 2220–2230. doi:10.7150/thno.18675
Shakeri, A., Jarad, N. A., Leung, A., Soleymani, L., and Didar, T. F. (2019). Biofunctionalization of glass-and paper-based microfluidic devices: a review. Adv. Mater. Interfaces 6, 1900940. doi:10.1002/admi.201900940
Shen, L., Hagen, J. A., and Papautsky, I. (2012). Point-of-care colorimetric detection with a smartphone. Lab a Chip 12, 4240–4243. doi:10.1039/c2lc40741h
Silva-Neto, H. A., Arantes, I. V., Ferreira, A. L., Do Nascimento, G. H., Meloni, G. N., De Araujo, W. R., et al. (2023). Recent advances on paper-based microfluidic devices for bioanalysis. TrAC Trends Anal. Chem. 158, 116893. doi:10.1016/j.trac.2022.116893
Sociales, N. U. D. D. A. E. Y. (2009). Rethinking poverty: report on the world social situation 2010. New York, NY: United Nations, Department of Economic and Social Affairs.
Solhi, E., Hasanzadeh, M., and Babaie, P. (2020). Electrochemical paper-based analytical devices (ePADs) toward biosensing: recent advances and challenges in bioanalysis. Anal. methods 12, 1398–1414. doi:10.1039/d0ay00117a
Soman, S. S., Samad, S. A., Venugopalan, P., Kumawat, N., and Kumar, S. (2024). Microfluidic paper analytic device (μPAD) technology for food safety applications. Biomicrofluidics 18, 031501. doi:10.1063/5.0192295
Songok, J., Tuominen, M., Teisala, H., Haapanen, J., Mäkelä, J., Kuusipalo, J., et al. (2014). Paper-based microfluidics: fabrication technique and dynamics of capillary-driven surface flow. ACS Appl. Mater. and Interfaces 6, 20060–20066. doi:10.1021/am5055806
Sousa, L. R., Guinati, B. G., Maciel, L. I., Baldo, T. A., Duarte, L. C., Takeuchi, R. M., et al. (2024). Office paper and laser printing: a versatile and affordable approach for fabricating paper-based analytical devices with multimodal detection capabilities. Lab a Chip 24, 467–479. doi:10.1039/d3lc00840a
Sousa, L. R., Silva-Neto, H. A., Castro, L. F., Oliveira, K. A., Figueredo, F., Cortón, E., et al. (2023). “Do it yourself” protocol to fabricate dual-detection paper-based analytical device for salivary biomarker analysis. Anal. Bioanal. Chem. 415, 4391–4400. doi:10.1007/s00216-023-04581-2
Sun, X., Li, B., Tian, C., Yu, F., Zhou, N., Zhan, Y., et al. (2018). Rotational paper-based electrochemiluminescence immunodevices for sensitive and multiplexed detection of cancer biomarkers. Anal. Chim. acta 1007, 33–39. doi:10.1016/j.aca.2017.12.005
Tan, W., Zhang, L., Jarujamrus, P., Doery, J. C., and Shen, W. (2022). Improvement strategies on colorimetric performance and practical applications of Paper-based analytical devices. Microchem. J. 180, 107562. doi:10.1016/j.microc.2022.107562
Tasangtong, B., Pholsaptanakorn, T., Tapsawut, T., Wiwekwin, N., Mettakoonpitak, J., Na Nongkhai, P., et al. (2024). User-friendly diameter-based measurement paper sensor for chloride detection in water. Talanta Open 9, 100305. doi:10.1016/j.talo.2024.100305
Taylor, S. L., Nordlee, J. A., Jayasena, S., and Baumert, J. L. (2018). Evaluation of a handheld gluten detection device. J. food Prot. 81, 1723–1728. doi:10.4315/0362-028x.jfp-18-184
Tenda, K., Ota, R., Yamada, K., Henares, T. G., Suzuki, K., and Citterio, D. (2016). High-resolution microfluidic paper-based analytical devices for sub-microliter sample analysis. Micromachines 7, 80. doi:10.3390/mi7050080
Tenda, K., van Gerven, B., Arts, R., Hiruta, Y., Merkx, M., and Citterio, D. (2018). Paper-based antibody detection devices using bioluminescent BRET-switching sensor proteins. Angew. Chem. Int. Ed. 57, 15369–15373. doi:10.1002/anie.201808070
Thangjitsirisin, K., Seeharaj, P., and Choengchan, N. (2024). Superhydrophobic eggshell for fabrication of hydrophobic barrier of paper-based analytical device for colorimetric determination of ammonium ion in water. Microchem. J. 200, 110464. doi:10.1016/j.microc.2024.110464
Tian, X., Peng, H., Li, Y., Yang, C., Zhou, Z., and Wang, Y. (2017). Highly sensitive and selective paper sensor based on carbon quantum dots for visual detection of TNT residues in groundwater. Sensors Actuators B Chem. 243, 1002–1009. doi:10.1016/j.snb.2016.12.079
Toennies, G., and Kolb, J. J. (1951). Techniques and reagents for paper chromatography. Anal. Chem. 23, 823–826. doi:10.1021/ac60054a002
Tortorich, R. P., Shamkhalichenar, H., and Choi, J.-W. (2018). Inkjet-printed and paper-based electrochemical sensors. Appl. Sci. 8, 288. doi:10.3390/app8020288
Trinh, K. T. L., Chae, W. R., and Lee, N. Y. (2022). Recent advances in the fabrication strategies of paper-based microfluidic devices for rapid detection of bacteria and viruses. Microchem. J. 180, 107548. doi:10.1016/j.microc.2022.107548
Valera, E., Jankelow, A., Lim, J., Kindratenko, V., Ganguli, A., White, K., et al. (2021). COVID-19 point-of-care diagnostics: present and future. ACS Nano 15, 7899–7906. doi:10.1021/acsnano.1c02981
Vella, S. J., Beattie, P., Cademartiri, R., Laromaine, A., Martinez, A. W., Phillips, S. T., et al. (2012). Measuring markers of liver function using a micropatterned paper device designed for blood from a fingerstick. Anal. Chem. 84, 2883–2891. doi:10.1021/ac203434x
Verma, M. S., Tsaloglou, M.-N., Sisley, T., Christodouleas, D., Chen, A., Milette, J., et al. (2018). Sliding-strip microfluidic device enables ELISA on paper. Biosens. Bioelectron. 99, 77–84. doi:10.1016/j.bios.2017.07.034
Wang, L., Zhu, F., Zhu, Y., Xie, S., Chen, M., Xiong, Y., et al. (2019). Intelligent platform for simultaneous detection of multiple aminoglycosides based on a ratiometric paper-based device with digital fluorescence detector readout. ACS sensors 4, 3283–3290. doi:10.1021/acssensors.9b01845
Wang, M., Cui, J., Wang, Y., Yang, L., Jia, Z., Gao, C., et al. (2022). Microfluidic paper-based analytical devices for the determination of food contaminants: developments and applications. J. Agric. Food Chem. 70, 8188–8206. doi:10.1021/acs.jafc.2c02366
Wang, P., Ge, L., Ge, S., Yu, J., Yan, M., and Huang, J. (2013a). A paper-based photoelectrochemical immunoassay for low-cost and multiplexed point-of-care testing. Chem. Commun. 49, 3294–3296. doi:10.1039/c3cc00149k
Wang, Y., Ge, L., Wang, P., Yan, M., Ge, S., Li, N., et al. (2013b). Photoelectrochemical lab-on-paper device equipped with a porous Au-paper electrode and fluidic delay-switch for sensitive detection of DNA hybridization. Lab a Chip 13, 3945–3955. doi:10.1039/c3lc50430a
Wang, Y., Wang, S., Ge, S., Wang, S., Yan, M., Zang, D., et al. (2013c). Facile and sensitive paper-based chemiluminescence DNA biosensor using carbon dots dotted nanoporous gold signal amplification label. Anal. Methods 5, 1328–1336. doi:10.1039/c2ay26485d
Washburn, E. W. (1921). The dynamics of capillary flow. Phys. Rev. 17, 273–283. doi:10.1103/physrev.17.273
West, P. W. (1945). Selective spot test for copper. Industrial and Eng. Chem. Anal. Ed. 17, 740–741. doi:10.1021/i560147a024
World Health Organization (2020). WHO Director-General’s remarks at the media briefing on 2019-nCoV on 11 February 2020.
Wu, K.-H., Huang, W.-C., Wang, J.-C., and Wang, S.-H. (2024). Paper-based colorimetric sensor using Photoshop and a smartphone app for the quantitative detection of carbofuran. Anal. Methods 16, 1043–1049. doi:10.1039/d3ay02211k
Xia, Y., Si, J., and Li, Z. (2016). Fabrication techniques for microfluidic paper-based analytical devices and their applications for biological testing: a review. Biosens. Bioelectron. 77, 774–789. doi:10.1016/j.bios.2015.10.032
Xu, J., Zhang, Y., Li, L., Kong, Q., Zhang, L., Ge, S., et al. (2018). Colorimetric and electrochemiluminescence dual-mode sensing of lead ion based on integrated lab-on-paper device. ACS Appl. Mater. and interfaces 10, 3431–3440. doi:10.1021/acsami.7b18542
Yakoh, A., Chaiyo, S., Siangproh, W., and Chailapakul, O. (2019). 3D capillary-driven paper-based sequential microfluidic device for electrochemical sensing applications. ACS sensors 4, 1211–1221. doi:10.1021/acssensors.8b01574
Yakoh, A., Mehmeti, E., Kalcher, K., and Chaiyo, S. (2022). Hand-operated, paper-based rotational vertical-flow immunosensor for the impedimetric detection of α-fetoprotein. Anal. Chem. 94, 5893–5900. doi:10.1021/acs.analchem.2c00079
Yamada, K., Henares, T. G., Suzuki, K., and Citterio, D. (2015). Paper-based inkjet-printed microfluidic analytical devices. Angew. Chem. Int. Ed. 54, 5294–5310. doi:10.1002/anie.201411508
Yamada, K., Shibata, H., Suzuki, K., and Citterio, D. (2017a). Toward practical application of paper-based microfluidics for medical diagnostics: state-of-the-art and challenges. Lab a Chip 17, 1206–1249. doi:10.1039/c6lc01577h
Yamada, K., Suzuki, K., and Citterio, D. (2017b). Text-displaying colorimetric paper-based analytical device. Acs Sensors 2, 1247–1254. doi:10.1021/acssensors.7b00464
Yang, R., Li, F., Zhang, W., Shen, W., Yang, D., Bian, Z., et al. (2019). Chemiluminescence immunoassays for simultaneous detection of three heart disease biomarkers using magnetic carbon composites and three-dimensional microfluidic paper-based device. Anal. Chem. 91, 13006–13013. doi:10.1021/acs.analchem.9b03066
Yetisen, A. K., Akram, M. S., and Lowe, C. R. (2013). Paper-based microfluidic point-of-care diagnostic devices. Lab a Chip 13, 2210–2251. doi:10.1039/c3lc50169h
Ye, X., and Lei, B. (2023). The current status and trends of DNA extraction. Bioessays 45, 2200242. doi:10.1002/bies.202200242
Yu, W. W., and White, I. M. (2013). Inkjet-printed paper-based SERS dipsticks and swabs for trace chemical detection. Analyst 138, 1020–1025. doi:10.1039/c2an36116g
Yu, J., Ge, L., Huang, J., Wang, S., and Ge, S. (2011a). Microfluidic paper-based chemiluminescence biosensor for simultaneous determination of glucose and uric acid. Lab a Chip 11, 1286–1291. doi:10.1039/c0lc00524j
Yu, J., Wang, S., Ge, L., and Ge, S. (2011b). A novel chemiluminescence paper microfluidic biosensor based on enzymatic reaction for uric acid determination. Biosens. Bioelectron. 26, 3284–3289. doi:10.1016/j.bios.2010.12.044
Yu, X., Chang, W., Zhang, H., Cai, Z., Yang, Y., and Zeng, C. (2023). Visual and real-time monitoring of Cd2+ in water, rice, and rice soil with test paper based on [2+ 2] lanthanide clusters. Inorg. Chem. 62, 6387–6396. doi:10.1021/acs.inorgchem.3c00255
Zargaryan, A., Farhoudi, N., Haworth, G., Ashby, J. F., and Au, S. H. (2020). Hybrid 3D printed-paper microfluidics. Sci. Rep. 10, 18379. doi:10.1038/s41598-020-75489-5
Zeng, F., Mou, T., Zhang, C., Huang, X., Wang, B., Ma, X., et al. (2019). Paper-based SERS analysis with smartphones as Raman spectral analyzers. Analyst 144, 137–142. doi:10.1039/c8an01901k
Zhang, G., Song, S., Panescu, J., Shapiro, N., Dannemiller, K. C., and Qin, R. (2023). A novel systems solution for accurate colorimetric measurement through smartphone-based augmented reality. PloS one 18, e0287099. doi:10.1371/journal.pone.0287099
Zhang, Y., Fan, J., Nie, J., Le, S., Zhu, W., Gao, D., et al. (2015). Timing readout in paper device for quantitative point-of-use hemin/G-quadruplex DNAzyme-based bioassays. Biosens. Bioelectron. 73, 13–18. doi:10.1016/j.bios.2015.04.081
Zhang, Y., Zhou, C., Nie, J., Le, S., Qin, Q., Liu, F., et al. (2014). Equipment-free quantitative measurement for microfluidic paper-based analytical devices fabricated using the principles of movable-type printing. Anal. Chem. 86, 2005–2012. doi:10.1021/ac403026c
Zhao, W.-W., Xu, J.-J., and Chen, H.-Y. (2016). Photoelectrochemical detection of metal ions. Analyst 141, 4262–4271. doi:10.1039/c6an01123c
Zhao, C., and Liu, X. (2016). A portable paper-based microfluidic platform for multiplexed electrochemical detection of human immunodeficiency virus and hepatitis C virus antibodies in serum. Biomicrofluidics 10, 024119. doi:10.1063/1.4945311
Zhong, S., Xue, L., Wang, Y., Zhang, C., Liu, N., Li, L., et al. (2024). Paper-based microfluidic chips for wide time range fluid control based on knife crafting and laser cutting. Sensors Actuators B Chem. 415, 135956. doi:10.1016/j.snb.2024.135956
Keywords: paper-based microfluidics, fabrication methods, detection techniques, applications, point-of-care, point-of-need
Citation: Aryal P and Henry CS (2024) Advancements and challenges in microfluidic paper-based analytical devices: design, manufacturing, sustainability, and field applications. Front. Lab. Chip. Technol. 3:1467423. doi: 10.3389/frlct.2024.1467423
Received: 19 July 2024; Accepted: 04 December 2024;
Published: 20 December 2024.
Edited by:
Hamed Golmohammadi, Chemistry and Chemical Engineering Research Center, IranReviewed by:
Zeinab Bagheri, Shahid Beheshti University, IranForough Ghasemi, Agricultural Biotechnology Research Institute of Iran, Iran
Copyright © 2024 Aryal and Henry. This is an open-access article distributed under the terms of the Creative Commons Attribution License (CC BY). The use, distribution or reproduction in other forums is permitted, provided the original author(s) and the copyright owner(s) are credited and that the original publication in this journal is cited, in accordance with accepted academic practice. No use, distribution or reproduction is permitted which does not comply with these terms.
*Correspondence: Charles S. Henry, Y2h1Y2suaGVucnlAY29sb3N0YXRlLmVkdQ==