- Department of Mechanical and Materials Engineering, Worcester Polytechnic Institute, Worcester, MA, United States
Liquid marbles (LMs) are versatile soft matter systems comprising a liquid core encapsulated by a shell of hydrophobic particles. LMs exhibit non-wetting properties and hence have enhanced mobility compared with droplets on a solid substrate. Recent advances have expanded their fabrication methods, material choices, and applications, particularly in microfluidics. This mini-review highlights the progress in LM research over the past 2 years, focusing on novel fabrication techniques, unique materials, multi-physical control schemes, and emerging applications including solar energy harvesting, cell culture, controlled substance delivery, gas sensing, and pollutant decontamination. We provide opinions on the unaddressed needs in LM research and on the trend of LM developments, specifically for the applications of LMs in lab-on-a-chip applications.
1 Introduction
Liquid marbles (LMs) are soft matter systems that have recently garnered significant attention across scientific disciplines. An LM consists of a liquid droplet encapsulated by a layer of hydrophobic particles, forming a core-shell structure (Bormashenko, 2011; Aussillous and Quéré, 2006). The particle shell creates a barrier that prevents direct contact between the liquid core and the substrate, enabling liquid marbles to exhibit non-wetting properties and to assume spherical or puddle-like shapes (Li, 2019). Typically, LMs are millimetric water droplets covered with nano- to micrometer-sized particles (Tenjimbayashi et al., 2023a), exhibiting enhanced mobility on surfaces and responding to external stimuli such as magnetic or electric fields. More generally, the liquid core can be water (Gallo et al., 2021), organic liquids (Xue et al., 2010), or a colloidal solution (Tian et al., 2013), while the particles in the shell can be made of polymers (Fernandes et al., 2015), metal oxides (Feng et al., 2023), and carbon-based substances (Sivan et al., 2013), each conferring different properties and functionalities to the LMs. Notably, the shell can be made of more than one material, providing unique functions and properties. For example, Janus LMs (Lekshmi and Varanakkottu, 2023), characterized by having two different types of particles in their shells, exhibit asymmetric properties useful in targeted delivery systems and directional sensing applications. Composite LMs (Roy et al., 2020) have a shell composed of a thin layer of silicone oil with solid particles embedded, which enhances the LM stability and functionality particularly for controlled drug release and catalyst supports. The dual nature of LMs, combining fluid dynamics with solid surface interactions, facilitates efficient mixing and mass transfer, avoids boundary effects, and provides large-scale quantitative response platforms for high-throughput analysis (Song et al., 2023). This makes LMs promising candidates for wide applications such as biosensing, chemical reactions, microreactors, bioreactors, and advanced manufacturing techniques.
Current research on LMs is increasingly focused on achieving controllability, repeatability, and practical applications, particularly for in-the-field uses. To realize this, technological advancements are needed in the development of new materials, fabrication methods, and control mechanisms. Although there has been substantial effort in these areas, as reflected in previously published review papers (Tenjimbayashi et al., 2023a; Ooi et al., 2021; Sun et al., 2022; Huang et al., 2023), more recent studies in the past 1–2 years have not been thoroughly reviewed, especially those with new materials as well as demonstrations of novel applications. These advancements are crucial for advancing both the fundamental understanding and practical applications of LMs. This paper aims to address this gap by reviewing recent progress in LMs over the past year or so, categorizing these advancements by fabrication methods, the use of unique materials or structures, control schemes, and emerging applications. Moreover, the paper highlights key innovations and offers perspectives on future research directions in LMs, with a particular emphasis on their potential for lab-on-a-chip systems.
2 Fabrication
Fabrication of LMs requires coating droplets with particles. Traditional techniques rely on either surface tension by rolling the droplet off an inclined particle bed (Whitby et al., 2012) or electrostatic attraction forces by applying a voltage difference between the droplet and the particle bed (Ireland et al., 2019). However, precise control of the LM size has been difficult for these techniques, despite its importance in achieving repeatability and reliability in lab-on-a-chip systems. To tackle this challenge, Sreejith et al. (Sreejith et al., 2019) introduced an automated LM generator that allowed for precise control of the size and volume of each LM. This method utilizes electrohydrodynamic forces to push the liquid through a needle tip to form a droplet, which is subsequently coated with hydrophobic particles. By tuning the applied voltage, the droplet volume and hence the LM size can be well controlled. Alternatively, Tenjimbayashi (Tenjimbayashi, 2023) employed a superhydrophobic mesh to produce uniformly sized droplets that were subsequently stabilized with a powder coating, with a precise control of the droplet size by the mesh pore size, making it suitable for microfluidic applications requiring high throughput and precision control. Besides the initial fabrication stage, the LM size continues to change spontaneously due to unavoidable evaporation (Dandan and Erbil, 2009; Tosun and Erbil, 2009; Fullarton et al., 2018). This can be addressed by noninvasive liquid refilling of LMs, which was demonstrated by leveraging vapor diffusion through porous materials followed by condensation (Sreejith et al., 2022). This method enhances the longevity and practicality of LMs to facilitate long-term microfluidic applications.
3 Materials and structures of LMs
Various materials have been explored for LM cores and shells, each offering unique advantages and hence leading to different LM characteristics and applications. LMs in early research were made with generic materials, such as polymers, metal oxides, and carbon-based substances, which are not optimized for specific applications. By comparison, recent studies have expanded the horizon by incorporating materials with new properties optimized for specific functions or applications, such as biocompatibility for drug delivery, magnetic materials for easy modulation scheme, and responsivity to environmental changes for sensing. Review on LMs with unique materials and structures, which is a category rarely summarized by previous published reviews, will help to inspire the research community to expand the applications by providing previously unavailable LM characteristics.
3.1 Hydrogel-based LMs
Hydrogels are versatile materials with wide applications in biology, chemistry, and engineering. Their biocompatibility and customizable properties make them ideal LM core materials for environmental applications like biosensing. One example is the spinnable hydrogel LMs that leverage the intrinsic properties of hydrogels for advanced water decontamination and colorimetric detection (Pereira et al., 2024). These LMs are composed of a network of polymer chains that can retain a significant amount of water, allowing them to dynamically concentrate pollutants and hence to efficiently removing pollutants from the environment. The integration of pH-sensitive dyes provided a cost-effective solution for water quality assessment. Another work utilized the natural properties of cellulose to create a durable and robust cellulose-based LMs for gas sensing (Li et al., 2023). The cellulose in these hydrogel marbles provides a highly porous structure, facilitating efficient diffusion of environmental gases into the LMs. The porosity and high mechanical stability of cellulose allow the marbles to maintain integrity under challenging conditions, such as withstanding a fracture height of 10.5 m, 420 times greater than that of a water marble. The hydrogel LM bounce-back distance was 25.5 mm after a 60-mm free fall, 881% higher than that of non-hydrogel LMs (Li et al., 2023).
Enhanced position control is another benefit offered by hydrogel-based LMs. For example, LMs with a hydrogel core containing magnetic nanoparticles from magnetotactic bacteria Magnetospirillum magneticum sp. AMB-1, were not only magnetically responsive, allowing position control via external magnetic fields, but also exhibited high biocompatibility, which is critical for biomedical applications such as drug delivery. The protective hydrogel core could be used to enclose antibiotics, opening up new possibilities for delivering hydrophobic substances to biological environments (Bielas et al., 2023a).
3.2 Magnetic LMs
Magnetic liquid marbles (MLMs) are the LMs containing magnetic materials and have drawn attention for their potential applications in microfluidics, microactuators, drug delivery, and sensing, thanks to their readily controllable dynamics by magnetic fields. Besides the abovementioned work (Bielas et al., 2023a), MLMs can be readily created by coating water droplets with superhydrophobic Fe3O4 nanoparticles, and this magnetic shell allows LM position manipulation by magnetic fields (Zhao et al., 2012). In the meantime, the detection of light reflection from the MLM’s core allowed real-time observation of chemical reactions inside (Zhao et al., 2015).
Ferrofluid LMs (FLMs) are a special type of MLMs with magnetic liquid cores. As an example, the FLMs, where the cores contained magnetic iron oxide nanoparticles in water, were manipulated by coil-generated DC and pulse-width-modulated (PWM) magnetic fields (Mohammadrashidi et al., 2023a). The FLM dynamic responses to the fields were parametrically studied on the FLM volume, distance from the coil, and coil current. Compared with DC, the PWM field allowed for more precise manipulation of FLMs and moved FLMs at a distance farther away from the coil. The same research group explored both the vibration and jumping of FLMs in magnetic fields (Mohammadrashidi et al., 2023b). In both cases, magnetic fields deformed these marbles and, when turned off, transformed the surface energy into marble kinetic energy to realize the motions. Further, the authors developed new theoretical models to estimate damping characteristics and calculate the jump height. These advances enhance the understanding of FLM dynamics and reveal their potential for microfluidic applications like sensing. The responses of FLM core and various shell particles to dynamic magnetic fields are not limited to position control (Bielas et al., 2023b). The magnetic actuation not only heated the marbles but mechanically strengthened their structures. The heat evaporated the core liquid, leaving a robust but empty shell ready to be refilled with functional materials like antibiotics. Such FLMs also allow complex structures, such as patchy and Janus marbles, to be created under magnetic fields, demonstrating the potential application in drug delivery.
Compared with the recent experimental research on MLMs, less work has been done on the simulation and theoretical studies. The most notable theoretical progress was made by Poorreza et al. (Poorreza et al., 2023). They proposed that adding a permanent magnet could counteract the marble’s weight, preventing it from flattening into a puddle shape and helping it retain a near-spherical form, thereby enhancing the sensitivity of an MLM-based accelerometer.
3.3 Polymer-based LMs
Polymers also have customizable properties and hence provide unique opportunities when used in LMs. A typical work created the LM shell using micrometer-sized fatty acid (FA) particles, which is a phase-change material sensitive to light and temperature changes, coated by polypyrrole (PPy) (Tsumura et al., 2023). When exposed to light, the FA underwent a phase change from solid to liquid, leading to LM disruption and hence controlled release of encapsulated substances. By tuning the light intensity and FA materials, the disruption time was adjustable from being almost instantaneous to approximately 10 seconds. These LMs can offer versatile lab-on-a-chip functions such as sensing and controlled drug delivery.
3.4 Superhydrophobic LMs
Superhydrophobic materials have also been used for LM construction. In one study (Wang et al., 2023), researchers proposed using a superhydrophobic organotin precursor for LM construction. They synthesized a chalcogenidometalate cluster, identified as pseudopentasupertetrahedron-1 (PPS-1). This cluster was composed of [(BuSn)3SnCd4S13(OH)] units, which were assembled into two distinct tetrahedral patterns that were interconnected and formed a robust diamond network. PPS-1 is superhydrophobic and stable in aqueous solutions across a broad pH range from one to 13, making it ideal for LM construction and hydrophobicity-based sensing applications.
4 Control schemes of LMs
One of the most appealing aspects of LMs is their dynamic responsivity to external stimuli, such as the light, magnetic fields, and pH changes. These responses include changes of shapes, structures, mechanical stability, and movements, as shown in Figure 1, all of which open up a wide array of potential applications.
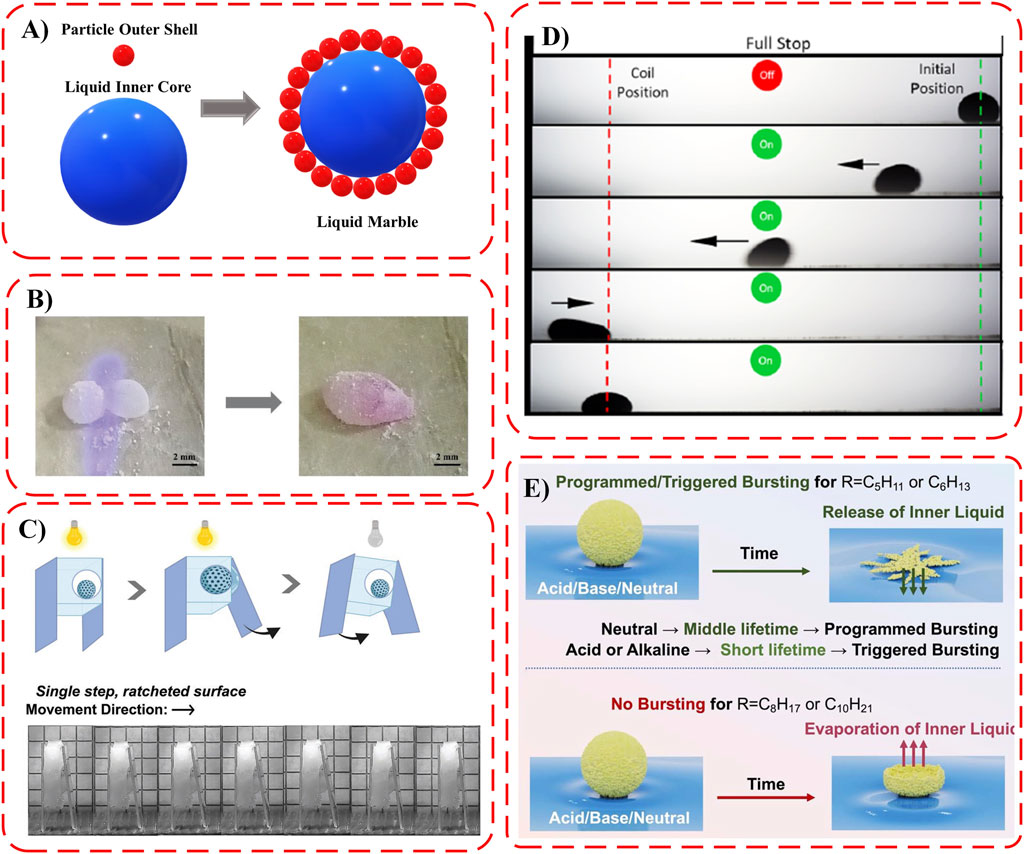
Figure 1. (A) Schematic representation of a generic liquid marble (LM), showing its structure, (B) Photo of ultraviolet light-triggered coalescence of two contacting liquid marbles(left: before and right: after coalescence, respectively) (Lv et al., 2023), (C) (top) Schematic of a soft actuator in a walking robot illuminated by a lamp. The thin top layer expands as the encased liquid marble pushes the robot’s front leg forward. When the lamp is turned off, the back leg retracts, completing a step. (bottom) Experimental image of the walking robot. (Gomez et al., 2023), (D) Sequential images showing the manipulation of a LM under a magnetic field. The coil is activated (“On”) to move the liquid marble from its initial position towards the coil position. Arrows indicate the direction of movement. (Mohammadrashidi et al., 2023a), and (E) Top) Illustration of LMs with both programmed (moderate lifetime) and triggered release mechanisms for the inner liquid in a water pool, sensitive to pH. Bottom) LMs that fail to release their inner liquid exhibit evaporation of encapsulated water from the marble. (Kumar et al., 2024). All figures in (B–E) are reprinted with permission.
4.1 Magnetic control
As described in Section 3.2, extensive studies have recently developed magnetic techniques to modulate the MLM movements and positions (Zhao et al., 2012), initiate jumping (Mohammadrashidi et al., 2023b), and control the vibrations (Mohammadrashidi et al., 2023a), promising high potential in micro-actuators, drug delivery, and sensing. Such control is typically done by external magnetic fields without physical contacts, offering a versatile alternative to conventional fluid dynamic control.
Friction is a crucial factor for motion control of LMs. MLMs floating on water surfaces slide, instead of rolling on solid substrates, with lower friction when magnetically moved (Sarkhosh et al., 2023; Dayyani et al., 2024). A previous review (Huang et al., 2023) has a thorough discussion on the friction of LMs, not limited to MLMs. We note that a new study (Sun et al., 2023), not included in the previous review, extended the knowledge by studying the impact of the LM deformation on rolling resistance, with parametric study of marble size, surface roughness, and contact angle on various surfaces. This work provides valuable insights on optimizing LM friction in different applications.
4.2 Optical and thermal control
LMs made with light- and thermo-responsive materials allow for optical and thermal manipulation, respectively, providing unique functionalities in sensing and actuation, in addition to drug delivery. As discussed in Section 3.3, the optical and thermal responsiveness of LMs with FA/PPy shells promises controlled substance delivery, release, and sensing in microfluidics (Tsumura et al., 2023). Alternatively, LMs developed with photothermal graphene nano-colloids expand in response to optical illumination (Gomez et al., 2023). Such LMs served as a soft actuator in a soft walker robot, underscoring their potential in locomotion generation of robots, especially those incorporated with lab-on-a-chip systems. Additionally, ultraviolet (UV) light has also been used to control merging and bursting of LMs (Lv et al., 2023). This UV-triggered LM coalescence allows for controlled mixing of substances previously contained in two separate LMs and sequential micro-reactions in situ, opening new avenues for precise chemical reaction manipulation in microfluidics.
4.3 pH control
The disintegration of LMs can be controlled by altering the environmental pH levels (Kumar et al., 2024). The authors demonstrated precisely control the moment of bursting, facilitating targeted release and mixing of contents. This study highlights how pH adjustments can enhance the functionality and efficiency of LM-based lab-on-a-chip devices. Besides experimental demonstrations, a fundamental understanding of the rupture kinetics of LMs is crucial for expanding their applications. Guo et al. (2023) provided substantial insights into this area by using the Digital Image Correlation (DIC) algorithm, which allowed for precise tracking and analysis of particle movements on the surface of a bursting LM. The study of such movements offers a granular view of the structural changes during the burst, enhancing both the knowledge of the burst and the design of LM control mechanisms to inspire future advances on controlled release through rupture.
5 Applications
In their early stages, LMs were used in lab-on-a-chip systems mainly as droplet-based microreactors, since each LM is a liquid container with non-wetting behaviors and mobility. They have also been utilized in chemical and biological sensing for analyte detection. These early applications laid the groundwork for the more advanced and specialized uses of liquid marbles seen today. The applications of LMs have expanded significantly in the past few years, bringing the scientifically intriguing LMs closer to practical usages. Figure 2 provides an overview of some of the emerging applications of LMs, which will be detailed below.
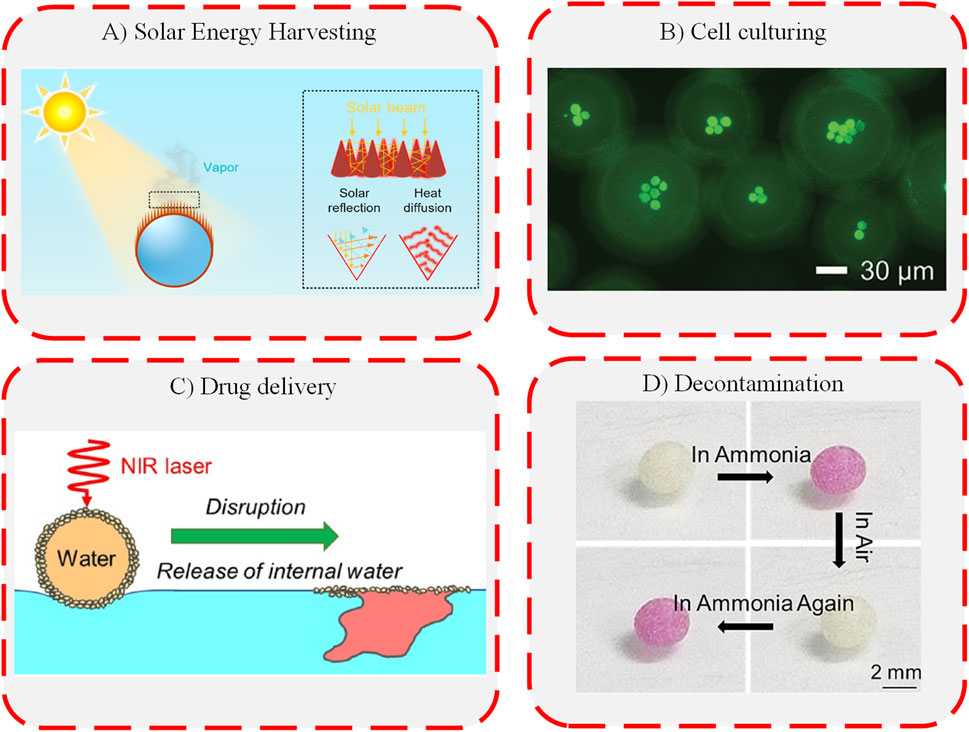
Figure 2. Overview of applications of liquid marbles in various fields, including (A) Solar energy harvesting (LMs with an upward nanowire array enhance light absorption through multiple reflections) (Liu et al., 2024), (B) Cell culturing, showing individual cells inside LMs (Tenjimbayashi et al., 2023b), (C) Drug delivery (release of the inner liquid core by an external stimulus) (Tsumura et al., 2023) and (D) Decontamination (colorimetric sensing of ammonia gas; the LM changes color upon contact with ammonia) (Li et al., 2023). All figures are reprinted with permission.
5.1 Solar energy harvesting
LMs have been gaining attention in the past year or so for their potential to enhance solar evaporation processes, a key area of innovation within renewable energy technologies. LMs embedded with tunable nanowires optimize solar energy harvesting for water evaporation, as shown in Figure 2A (Liu et al., 2024). Adjustments of such nanowire arrays maximized solar absorption through multiple reflections, consequently increasing photon absorption and boosting photothermal conversion, thereby increasing the efficiency of water evaporation within the marbles. By leveraging the LMs’ ability of concentrating solar energy, this method can benefit applications like desalination and wastewater treatment. Further expanding on this concept, rougher LM surfaces were found to enhance solar energy capture, thus accelerating the evaporation (Feng et al., 2023). This finding underscores the importance of surface engineering of LMs, including the design, materials, and fabrication of LMs, in boosting solar energy harvesting efficiency.
5.2 Cell culture
The porous shells of LMs facilitate gas exchanges with the environment, which has been proven valuable for maintaining a naturalistic microenvironment for cell culturing. As an example, a small number of cells were isolated by using LMs (Tenjimbayashi et al., 2023b), as shown in Figure 2B, allowing detailed examination of individual cells. This work proves the benefits of LMs in single-cell-level cellular analysis. In reproductive biotechnology, LMs were found to better support bovine oocyte development, when compared with traditional culture systems, by providing a more conducive environment for cell maturation, thanks to the easier and efficient mass transfer through the shells (Fernández-Montoro et al., 2023). Furthermore, the application of LMs has extended to micro-photo-bioreactors (Nguyen et al., 2023). LMs were used as dynamic bioreactors for cultivating microalgae, demonstrating their potential of optimizing the light exposure and nutrient distribution. The abovementioned studies exemplify the adaptability and efficacy of LMs in enhancing cell growth and productivity in cell culturing.
5.3 Drug delivery
With their cores serving as cargos and shells as carriers, LMs are intrinsically suitable for controlled and targeted release of therapeutic agents (Figure 2C). Although drug delivery is one of the early-stage applications of LMs, it was still one of the most actively researched applications in the past year or so. The new progress focused on enhanced precision of delivery control and novel control mechanisms. As discussed in Section 3.1, LMs encapsulating magnetosomes within biocompatible hydrogel shells allow magnetic-field-controlled drug release (Bielas et al., 2023a). Such LMs hold promise for targeted drug delivery to specific tissues or organs in cancer therapy and localized treatment of inflammatory diseases. As another example, LMs served as floating drug reservoirs, and the floating time for drug delivery systems was preprogrammed by the LM surface properties (Barman et al., 2023). By coating LMs with hydrophobic shell, the floating time can be adjusted to meet specific drug release requirements, allowing LMs find applications in drug delivery with prolonged release formulations. Additionally, biodegradable LMs offer a novel approach for controlled delivery and release of fertilizer in soil (McQuillan et al., 2023). By encapsulating fertilizer particles within biodegradable polymer shells, the LMs provide a protective barrier that controls the release of nutrients into the targeted soil, leading to enhanced crop yields and reduced fertilizer runoff.
5.4 Pollutant sensing and decontamination
LMs have shown promising applications in environmental sciences and engineering, such as pollutant sensing and decontamination. The hydrogel-based LMs discussed in Section 3.1 can selectively absorb demonstrating high adsorption capacity and rapid pollutant removal, in addition to real-time colorimetric sensing of pollutants (Pereira et al., 2024). Moreover, cellulose-based LMs can selectively adsorb target gases such as ammonia, which causes color changes in LMs and facilitates pollutant sensing (Li et al., 2023). The LMs can also release the absorbed gasses in a controlled manner, as shown in Figure 2D, ensuring repeated pollutant removal from the environment. The mechanical robustness and gas absorption capabilities bestow such cellulose-based LMs potential for long-term sensing and decontamination in harsh conditions (Li et al., 2023).
6 Conclusion and outlook
There have been significant advancements in LMs in the past 1–2 years. By showcasing the innovations in the formation methods, material variations and control mechanisms, we emphasize their novel and diverse applications, ranging from pollutant removal to solar energy harvesting. In addition to continuing these advancements, future research is still needed on the LM stability, functionalities, and maneuverability, detailed below. The nature of the structures of LMs makes them mechanically weak and their lifetime susceptible to environmental challenges. Developing environmentally and mechanically robust LMs like cellulose-based LMs (Li et al., 2023) will be crucial for realizing practical in-the-field applications. Multi-physical functions enabled by novel materials and designs of LMs will boost their potential for high-impact applications. Magnetic, optical, biological, chemical, mechanical, and environmental functions have been studied at their infant stages, but electrical and electrochemical functions have yet to be demonstrated, despite their accessibility in LMs and adaptation in traditional microfluidic systems. The combination of multi-physical functions in these small marbles has not been widely seen in the literature but will create a unique platform to realize well-controlled complex functions that are not available in other platforms. Additionally, further enhancements of the LM responsiveness to external multi-physical changes will create new interaction modalities between LMs and their environments, improve the reliability and temporospatial resolution of the functionality control, and expand their applications. With the current momentum of research progress, LMs possess a high potential to become a unique and versatile lab-on-a-chip platform to meet the demands of diverse scientific and engineering fields.
Author contributions
HG: Formal analysis, Investigation, Methodology, Visualization, Writing–original draft. YL: Conceptualization, Funding acquisition, Methodology, Project administration, Resources, Supervision, Writing–review and editing.
Funding
The author(s) declare that financial support was received for the research, authorship, and/or publication of this article. This work was supported in part with funding from the Gapontsev Family Collaborative Venture Fund.
Conflict of interest
The authors declare that the research was conducted in the absence of any commercial or financial relationships that could be construed as a potential conflict of interest.
Publisher’s note
All claims expressed in this article are solely those of the authors and do not necessarily represent those of their affiliated organizations, or those of the publisher, the editors and the reviewers. Any product that may be evaluated in this article, or claim that may be made by its manufacturer, is not guaranteed or endorsed by the publisher.
References
Aussillous, P., and Quéré, D. (2006). Properties of liquid marbles. Proc. R. Soc. A Math. Phys. Eng. Sci. 462, 973–999. doi:10.1098/rspa.2005.1581
Barman, N., Shome, A., Kumar, S., Mondal, P., Jain, K., Tenjimbayashi, M., et al. (2023). Preprogramming floating time of liquid marble. Adv. Funct. Mater. 33. doi:10.1002/adfm.202214840
Bielas, R., Kubiak, T., Kopčanský, P., Šafařík, I., and Józefczak, A. (2023b). Tunable particle shells of thermo-responsive liquid marbles under alternating magnetic field. J. Mol. Liq. 391, 123283. doi:10.1016/j.molliq.2023.123283
Bielas, R., Kubiak, T., Molcan, M., Dobosz, B., Rajnak, M., and Józefczak, A. (2023a). Biocompatible hydrogel-based liquid marbles with magnetosomes. Materials 17, 99. doi:10.3390/ma17010099
Bormashenko, E. (2011). Liquid marbles: properties and applications. Curr. Opin. Colloid and Interface Sci. 16, 266–271. doi:10.1016/j.cocis.2010.12.002
Dandan, M., and Erbil, H. Y. (2009). Evaporation rate of graphite liquid marbles: comparison with water droplets. Langmuir 25, 8362–8367. doi:10.1021/la900729d
Dayyani, H., Mohseni, A., and Bijarchi, M. A. (2024). Dynamic behavior of floating magnetic liquid marbles under steady and pulse-width-modulated magnetic fields. Lab. Chip 24, 2005–2016. doi:10.1039/d3lc00578j
Feng, Y., Yao, G., Xu, J., Wang, L., and Liu, G. (2023). Effect of surface roughness on the solar evaporation of liquid marbles. J. Colloid Interface Sci. 629, 644–653. doi:10.1016/j.jcis.2022.09.116
Fernandes, A. M., Mantione, D., Gracia, R., Leiza, J. R., Paulis, M., and Mecerreyes, D. (2015). From polymer latexes to multifunctional liquid marbles. ACS Appl. Mater Interfaces 7, 4433–4441. doi:10.1021/am509040x
Fernández-Montoro, A., Angel-Velez, D., Benedetti, C., Azari-Dolatabad, N., Pascottini, O. B., Van Soom, A., et al. (2023). Alternative culture systems for bovine oocyte in vitro maturation: liquid marbles and differentially shaped 96-well plates. Animals 13, 1635. doi:10.3390/ani13101635
Fullarton, C., Draper, T. C., Phillips, N., Mayne, R., De Lacy Costello, B. P. J., and Adamatzky, A. (2018). Evaporation, lifetime, and robustness studies of liquid marbles for collision-based computing. Langmuir 34, 2573–2580. doi:10.1021/acs.langmuir.7b04196
Gallo, A., Tavares, F., Das, R., and Mishra, H. (2021). How particle-particle and liquid-particle interactions govern the fate of evaporating liquid marbles. Soft Matter 17, 7628–7644. doi:10.1039/d1sm00750e
Gomez, J. C., Vishnosky, N. S., Kim, S. T., Dinca, S. A., Finkelstein, E. B., and Steinhardt, R. C. (2023). Robotic locomotion and Piezo1 activity controlled with novel liquid marble-based soft actuators. Adv. Funct. Mater. 33. doi:10.1002/adfm.202214893
Guo, J., Sun, Y., Tee, C. A. T., Liu, C., Bai, S., Huang, Y., et al. (2023). The movement pattern of particles on the surface of liquid marble during rupture based on the DIC algorithm. Colloids Surfaces A Physicochem. Eng. Aspects 679, 132546. doi:10.1016/j.colsurfa.2023.132546
Huang, Z., Xie, Y., Chen, H., Yu, Z., Shi, L., and Jin, J. (2023). Fundamentals and manipulation of bare droplets and liquid marbles as open microfluidic platforms. Processes 11, 983. doi:10.3390/pr11040983
Ireland, P. M., Thomas, C. A., Lobel, B. T., Webber, G. B., Fujii, S., and Wanless, E. J. (2019) “Electrostatic formation of liquid marbles - statistical model,” in Journal of physics: conference series, 1322.
Kumar, S., Barman, N., Borbora, A., Mondal, P., Tenjimbayashi, M., and Manna, U. (2024). pH-triggered adjustable bursting of liquid marbles in water pools. J. Mater. Chem. A 12, 3362–3372. doi:10.1039/d3ta06238d
Lekshmi, B. S., and Varanakkottu, S. N. (2023) “Janus liquid marbles: fabrication techniques, recent developments, and applications,” in Droplet 2.
Li, N., Wanyan, H., Lu, S., Xiao, H., Zhang, M., Liu, K., et al. (2023). Robust cellulose-based hydrogel marbles with excellent stability for gas sensing. Carbohydr. Polym. 306, 120617. doi:10.1016/j.carbpol.2023.120617
Li, X. (2019). Liquid marbles and liquid plasticines with nanoparticle monolayers. Adv. Colloid Interface Sci. 271, 101988. doi:10.1016/j.cis.2019.101988
Liu, Q., Wang, L., Liu, Z., and Liu, G. (2024). Solar evaporation of liquid marbles with tunable nanowire array. Sol. Energy Mater. Sol. Cells 264, 112626. doi:10.1016/j.solmat.2023.112626
Lv, Q., Li, J., Wang, R., and Zhang, L. (2023). Ultraviolet-light–triggered coalescence of liquid marbles for multistep microreactions. Part. and Part. Syst. Charact. 40. doi:10.1002/ppsc.202300076
McQuillan, R. V., Stevens, G. W., and Mumford, K. A. (2023). Implementation of biodegradable liquid marbles as a novel controlled release fertilizer. ACS Sustain. Chem. and Eng. 11, 122–132. doi:10.1021/acssuschemeng.2c04605
Mohammadrashidi, M., Azizian, P., Bijarchi, M. A., and Shafii, M. B. (2023b). Vibration and jumping of ferrofluid marbles under an initial magnetic perturbation. Langmuir 39, 9406–9417. doi:10.1021/acs.langmuir.3c00894
Mohammadrashidi, M., Bijarchi, M. A., Shafii, M. B., and Taghipoor, M. (2023a). Experimental and theoretical investigation on the dynamic response of ferrofluid liquid marbles to steady and pulsating magnetic fields. Langmuir 39, 2246–2259. doi:10.1021/acs.langmuir.2c02811
Nguyen, N. K., Tran, D. T., Chuang, A., Singha, P., Kijanka, G., Burford, M., et al. (2023). Liquid marble - a high-yield micro-photobioreactor platform. React. Chem. Eng. 8, 2710–2716. doi:10.1039/d3re00221g
Ooi, C. H., Vadivelu, R., Jin, J., Sreejith, K. R., Singha, P., Nguyen, N. K., et al. (2021). Liquid marble-based digital microfluidics-fundamentals and applications. Lab. Chip 21, 1199–1216. doi:10.1039/d0lc01290d
Pereira, V., Ang, Z. Z., Chong, C., Li, H., and Lee, H. K. (2024). Spinnable hydrogel marbles: a dynamic miniature molecule concentrator for efficient water decontamination and colorimetric detection. Chem. Eng. J. 480, 148132. doi:10.1016/j.cej.2023.148132
Poorreza, E., Hadjiaghaie Vafaie, R., Mehdipoor, M., and Ghavifekr, H. B. (2023). An experimental study of a magnetic liquid marble and a novel scheme for improving the sensitivity of a liquid marble-based accelerometer. Colloid J. 85, 629–649. doi:10.1134/s1061933x23600124
Roy, P. K., Binks, B. P., Bormashenko, E., Legchenkova, I., Fujii, S., and Shoval, S. (2020). Manufacture and properties of composite liquid marbles. J. Colloid Interface Sci. 575, 35–41. doi:10.1016/j.jcis.2020.04.066
Sarkhosh, M. H., Yousefi, M., Bijarchi, M. A., Nejat Pishkenari, H., and Forghani, K. (2023). Manipulation of ferrofluid marbles and droplets using repulsive force in magnetic digital microfluidics. Sensors Actuators A Phys. 363, 114733. doi:10.1016/j.sna.2023.114733
Sivan, V., Tang, S. Y., O’Mullane, A. P., Petersen, P., Eshtiaghi, N., Kalantar-Zadeh, K., et al. (2013). Liquid metal marbles. Adv. Funct. Mater. 23, 144–152. doi:10.1002/adfm.201200837
Song, Y., Wang, L., Xu, T., Zhang, G., and Zhang, X. (2023). Emerging open-channel droplet arrays for biosensing. Natl. Sci. Rev. 10, nwad106. doi:10.1093/nsr/nwad106
Sreejith, K. R., Ooi, C. H., Jin, J., Dao, D. V., and Nguyen, N. T. (2019). An automated on-demand liquid marble generator based on electrohydrodynamic pulling. Rev. Sci. Instrum. 90, 055102. doi:10.1063/1.5094522
Sreejith, K. R., Singha, P., Nguyen, N. K., Ooi, C. H., Dao, D. V., and Nguyen, N. T. (2022). Noninvasive refilling of liquid marbles with water for microfluidic applications. Appl. Phys. Lett. 120. doi:10.1063/5.0074887
Sun, Y., Zhao, M., Th Tee, C. A., Song, L., Guo, J., Pan, J., et al. (2023). Exploring the effects of liquid marbles’ deformation on their rolling resistance. Langmuir 39, 16618–16627. doi:10.1021/acs.langmuir.3c02617
Sun, Y., Zheng, Y., Liu, C., Zhang, Y., Wen, S., Song, L., et al. (2022). Liquid marbles, floating droplets: preparations, properties, operations and applications. RSC Adv. 12, 15296–15315. doi:10.1039/d2ra00735e
Tenjimbayashi, M. (2023). Production of small powder-stabilized droplets using superhydrophobic mesh. Appl. Phys. Lett. 122. doi:10.1063/5.0155219
Tenjimbayashi, M., Mouterde, T., Roy, P. K., and Uto, K. (2023a). Liquid marbles: review of recent progress in physical properties, formation techniques, and lab-in-a-marble applications in microreactors and biosensors. Nanoscale 15, 18980–18998. doi:10.1039/d3nr04966c
Tenjimbayashi, M., Yamamoto, S., and Uto, K. (2023b). Drycells: cell-suspension micro liquid marbles for single-cell picking. Adv. Mater. 35, e2300486. doi:10.1002/adma.202300486
Tian, J., Fu, N., Chen, X. D., and Shen, W. (2013). Respirable liquid marble for the cultivation of microorganisms. Colloids Surfaces B Biointerfaces 106, 187–190. doi:10.1016/j.colsurfb.2013.01.016
Tosun, A., and Erbil, H. Y. (2009). Evaporation rate of PTFE liquid marbles. Appl. Surf. Sci. 256, 1278–1283. doi:10.1016/j.apsusc.2009.10.035
Tsumura, Y., Fameau, A. L., Matsui, K., Hirai, T., Nakamura, Y., and Fujii, S. (2023). Photo- and thermoresponsive liquid marbles based on fatty acid as phase change material coated by polypyrrole: from design to applications. Langmuir 39, 878–889. doi:10.1021/acs.langmuir.2c03086
Wang, B., Yan, G. X., Cheng, Y. J., Chen, E. X., He, L., Zhou, X., et al. (2023). Superhydrophobic pseudosupertetrahedral sulfido metalate clusters for constructing liquid marbles. Inorg. Chem. 62, 10054–10058. doi:10.1021/acs.inorgchem.3c01501
Whitby, C. P., Bian, X., and Sedev, R. (2012). Spontaneous liquid marble formation on packed porous beds. Soft Matter 8, 11336. doi:10.1039/c2sm26529j
Xue, Y., Wang, H., Zhao, Y., Dai, L., Feng, L., Wang, X., et al. (2010). Magnetic liquid marbles: a “precise” miniature reactor. Adv. Mater. 22, 4814–4818. doi:10.1002/adma.201001898
Zhao, Y., Xu, Z., Niu, H., Wang, X., and Lin, T. (2015). Magnetic liquid marbles: toward “lab in a droplet,”. Adv. Funct. Mater. 25, 437–444. doi:10.1002/adfm.201403051
Keywords: liquid marbles, hydrophobic particles shells, microfluidics, nonwetting phenomena, solar energy harvesting, microbioreactors
Citation: Ghavami H and Liu Y (2024) Recent advancements in liquid marbles: fabrication, materials, control mechanisms, and applications. Front. Lab. Chip. Technol. 3:1451278. doi: 10.3389/frlct.2024.1451278
Received: 18 June 2024; Accepted: 27 August 2024;
Published: 11 September 2024.
Edited by:
Xiangchun Xuan, Clemson University, United StatesReviewed by:
Sajad Razavi Bazaz, Harvard Medical School, United StatesChenguang Zhang, Sanofi Genzyme, United States
Copyright © 2024 Ghavami and Liu. This is an open-access article distributed under the terms of the Creative Commons Attribution License (CC BY). The use, distribution or reproduction in other forums is permitted, provided the original author(s) and the copyright owner(s) are credited and that the original publication in this journal is cited, in accordance with accepted academic practice. No use, distribution or reproduction is permitted which does not comply with these terms.
*Correspondence: Yuxiang Liu, eWxpdTExQHdwaS5lZHU=