- 1Institute of Biological Chemistry, Biophysics and Bioengineering, School of Engineering and Physical Sciences, Heriot-Watt University, Edinburgh, United Kingdom
- 2Tissues, Cells and Advanced Therapeutics, Jack Copland Centre, Scottish National Blood Transfusion Service, Edinburgh, United Kingdom
Microfluidic devices are useful tools for a wide range of biomedical, industrial, and environmental applications. Hybrid microfluidic devices utilising more than two materials are increasingly being used for their capacity to produce unique structures and perform novel functions. However, an analysis of publications across the field shows that whilst hybrid microfluidic devices have been reported, there remains no system of classifying hybrid devices which could help future researchers in optimising material selection. To resolve this issue, we propose a system of classifying hybrid microfluidic devices primarily as containing either hybrid structural, chemical, or electrical components. This is expanded upon and developed into a hierarchy, with combinations of different primary components categorised into secondary or tertiary hybrid device groupings. This classification approach is useful as it describes materials that can be combined to create novel hybrid microfluidic devices.
Introduction
The term microfluidics describes the manipulation of tiny volumes of fluid within channels at the microscale (Akbari et al., 2023). Prior to the advent of microfluidics as a defined field, micro-sized channels were often contained within components of scientific and engineering instruments mostly unrelated to microfluidic applications (Ren et al., 2013; Convery and Gadegaard, 2019). Currently when compared with early microfluidics, microchannels with various geometries are used for many different applications and have huge potential for use within biomedical science (Ramachandraiah et al., 2017; David et al., 2019; Lu et al., 2019; Pritchard et al., 2019; Guzniczak et al., 2020; Xie et al., 2022), industrial processing (Estrada-Osorio et al., 2024; Jayan et al., 2024; Wang et al., 2024; Yi et al., 2024), environmental research (Hill et al., 2022; AlMashrea et al., 2024; Du and Yang, 2024; Sun et al., 2024) and increasingly reaching into other fields (Apoorva et al., 2024; Lei et al., 2024; Reyes et al., 2024). The manufacturing of these microchannels relies upon the use of appropriate techniques, equipment, and materials to produce microfluidic devices consistently and accurately (Ren et al., 2013; Gale et al., 2018; Scott and Ali, 2021; Akbari et al., 2023). New applications often require new channel geometries and the integration of novel components, and it is therefore not unreasonable to suggest that new manufacturing techniques and materials will be necessary to facilitate new microfluidic applications. Hybrid microfluidic devices, comprised of more than one type of material, can be used to meet these requirements. As part of the evaluation of the current state of microfluidic device manufacturing, this mini review focuses on hybrid microfluidic devices that utilise a combination of fabrication techniques and are comprised of at least two materials. Hybrid devices have been reviewed elsewhere but the reviews focus on a summary of the concepts of combining materials (Ren et al., 2013) or require substantial updating in line with current findings (Hou et al., 2017).
Materials and methods
This review expands upon two previous publications (Ren et al., 2013; Hou et al., 2017) that simply described material considerations for use in hybrid microfluidic devices, and for the first time provides a system of categorization of hybrid microfluidic devices. Between February and March 2024, literature searches of PubMed were performed using the following terms: “Hybrid microfluidic device,” “PDMS hybrid device,” “Composite microfluidic device,” “Glass hybrid device,” “PMMA hybrid device,” and “Polymer hybrid device” with a focus on relevant literature published since 2013.
Although this review aims to provide an update to previous hybrid device reviews, it is not an exhaustive list; examples of devices were chosen to demonstrate both the diversity in material choice and flexibility with which they can be combined for different applications. This was considered of particular importance as it is known that material selection impacts flow, biocompatibility and function of microfluidic devices (Nielsen et al., 2020). A range of papers with different applications were therefore included, and this review will aid researchers in material choice for future microfluidic device designs. The materials related to tubing and fluid connections were considered outside the scope of this review.
Outcomes
Hybrid microfluidic devices have previously been described in a variety of ways and do not have sub-categories to define related device compositions. We propose categorising hybrid microfluidic devices into three primary categories; composites as electrical components; composites as chemical components and composites as structural components.
When considering primary categories, hybrid devices can contain electrical components (comprehensively reviewed in (Tawade and Mastrangeli, 2024)) that are integrated and used as sensors or for performing functions such as organ-on-chip monitoring, or cell-matrix interactions within the microfluidic channel. Second, chemical components are integrated into hybrid devices in the form of surface coating materials (reviewed in (Tu et al., 2012)), or as catalysts (reviewed in (Solsona et al., 2019)) for chemical reactions and others. The third category is hybrid devices using composite materials as structural components. Here, two different materials are bonded together to form the walls of the microchannel or as one material acting as a filter within the microchannel, as well as for less functional reasons, such as one material simply acting as a support substrate. When the microfluidic device has a single function, they can be referred to as a primary hybrid device, but different combinations are possible and secondary (containing two types of hybrid components), and tertiary (containing all three types of hybrid components) are also possible to fabricate. This is presented schematically in Figure 1 where examples of microfluidic structures are depicted for each category. In the structural component bubble, an inertial focusing channel is shown and has been fabricated with two materials shown in yellow and red. The chemical components bubble shows a microchannel structure (red) with a functional coating (yellow) whereas the electrical component bubble depicts a microfluidic device with an integrated component (black circuit).
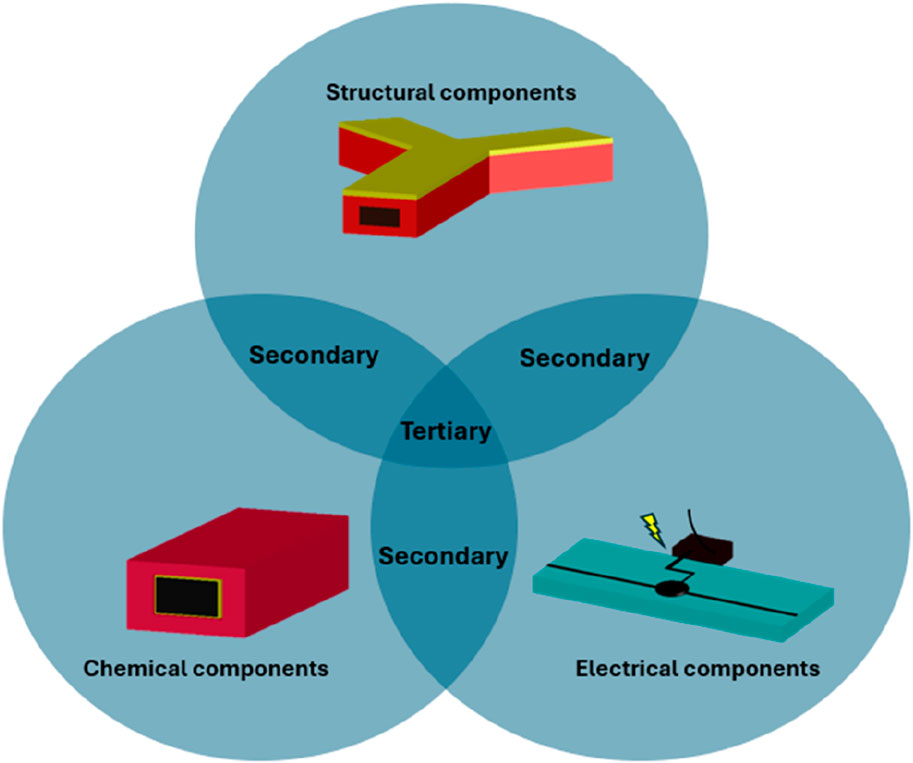
Figure 1. Categorisation of hybrid microfluidic devices. The three primary categories of chemical (surface coating in yellow, microchannel in red), structural (materials shown in yellow and red) and electrical (electrodes in black) can be combined to form secondary or tertiary categories of device.
At least two different materials need to be incorporated for a device to be categorised as a primary hybrid microfluidic device and must form the microchannel walls to be considered a primary structural device. Hybrid devices can be considered primary electrical component devices where an electrical component is integrated but the channel is comprised of only one material. A primary chemical component can be considered a hybrid device where the microchannel is coated with a chemical reagent or the device itself is comprised of a functionalised material to perform the intended application. For the purposes of this review, devices containing reservoirs or those that require the introduction of reagents to the fluidic system are not classified as part of the chemical component category. All other hybrid devices can be considered as devices characterised as having combinations of these primary components and are therefore defined as secondary or tertiary hybrid devices. An extensive, but not exhaustive, list of example hybrid devices across the hierarchy is presented in sections A, B and C of Table 1 with discussions of specific devices of interest.
Section A of Table 1 lists microfluidic devices that can be categorised as a primary hybrid device and can be further divided into devices containing structural, chemical, or electrical hybrid components. Polydimethylsiloxane (PDMS) and polymethyl methacrylate (PMMA) are two of the most commonly used substrates in hybrid microfluidic device manufacture owing to their biocompatibility, flexibility in application, capacity to bond to a wide range of materials and cost-effectiveness (Nielsen et al., 2020). PDMS substrates can be easily modified to generate relatively complex 3D structures employing the widely used technique of soft lithography. However, although PDMS has proven a useful material for many applications, microchannels constructed of PDMS have been shown to absorb small molecules and deform under fluid pressure (Raj M and Chakraborty, 2020). These two issues can impact device functionality where the correct microchannel architecture or the quantification of biomolecules are critical for the application (Hou et al., 2017; Nielsen et al., 2020; Raj M and Chakraborty, 2020). PMMA, on the other hand, does not deform under fluid pressure, can be fully optically transparent, and has higher chemical inertness and greater biocompatibility than PDMS (Gencturk et al., 2017). Despite being one of the most widely used materials in microfluidic devices for cell biology applications, PMMA is not as versatile as PDMS when it comes to generating complex microchannel architectures (Gencturk et al., 2017). Other substrate materials have been used for structural hybrid devices, but their use may be limited by cost, complexity of fabrication technique or issues relating to chemical or biological compatibility. Glass generally has higher costs than thermoplastics but retains excellent biocompatibility, optical transparency and robustness (Hou et al., 2017). Likewise, despite some advantages, silicon lacks optical transparency and photosensitive resins often have poor biocompatibility (Ren et al., 2013; Hou et al., 2017).
As listed in Table 1, hybrid devices utilising PDMS often use soft-lithography and hybrid devices utilising PMMA often use laser ablation for manufacture. Laser ablation is a low-cost, highly replicable fabrication technique and can be used to cut or, etch PMMA at a much higher throughput rate than the use of soft lithography with PDMS (Gencturk et al., 2017; Hou et al., 2017). Generally, where hybrid devices in section A of Table 1 are categorized as having primary electrical or chemical components, a chemically or biologically inert material was used for the microchannel structure to act as a scaffold material for the chemical or electrically compatible material in order to perform their function.
Section B of Table 1 lists secondary hybrid microfluidic devices where materials have been combined to produce a structural channel whilst also simultaneously providing an electrical or chemical functionality. Similarly to primary component hybrid devices, the majority of listed devices are comprised of polymer materials as a base substrate and are often combined with glass, but more recently a range of other materials such as paper, hydrogels and other inorganic materials have also been used. Hydrogels have been used as bioscaffolds (Trappmann et al., 2017) for cell attachment whereas paper has been used as a fluid carrier (Chen et al., 2023). The photoresist SU-8 has extremely high biocompatibility and has historically been utilised as a critical material in microelectronics (Nemani et al., 2013). In some secondary hybrid devices, the coating of microchannels is used to convey a chemical functionality to an otherwise relatively chemical inert microchannel (Hesari et al., 2016) or may contain bioink materials for cell culture (Richard et al., 2020).
The third category, tertiary hybrid microfluidic devices, contain all three of the components discussed previously and a range of examples are listed in section C of Table 1. As before, polymers and glass are the primary substrate materials used in fabrication. Many of these devices utilise the electronic components purely as biosensors although some are used to generate electrical fields to enable a specific function in the device. It is of note that tertiary hybrid devices often have greater complexity and by definition are comprised of more materials than those classified as primary or secondary hybrid devices.
Conclusion
Many microfluidic devices are comprised of at least two materials, but modern multifunctional devices often use more. This review provides an important, but not exhaustive, review of current hybrid material microfluidic devices and proposes a new categorisation system for devices made of multiple materials. Whilst hybrid devices have not previously been systemically categorised, this review aims to provide a new approach to describe intra- and intergroup commonalities, and an insight into why different materials were selected for combination. The development of devices using multiple, diverse materials to achieve complex functionality requires the use of a range of manufacturing techniques, and this review further aims to aid researchers in their selection of materials for future hybrid device fabrication. We acknowledge that as microfluidic device functions increase in complexity with the integration of more materials this review may require updating in the future.
Author contributions
TC: Conceptualization, Data curation, Methodology, Writing–original draft. PB: Supervision, Writing–review and editing. AF: Supervision, Writing–review and editing. HB: Conceptualization, Supervision, Writing–review and editing.
Funding
The author(s) declare that financial support was received for the research, authorship, and/or publication of this article. This work was supported by Medical Research Scotland (grant number 50167-2019) and internal research funding from NHS National Services Scotland.
Conflict of interest
The authors declare that the research was conducted in the absence of any commercial or financial relationships that could be construed as a potential conflict of interest.
Publisher’s note
All claims expressed in this article are solely those of the authors and do not necessarily represent those of their affiliated organizations, or those of the publisher, the editors and the reviewers. Any product that may be evaluated in this article, or claim that may be made by its manufacturer, is not guaranteed or endorsed by the publisher.
References
Abaci, H. E., Devendra, R., Smith, Q., Gerecht, S., and Drazer, G. (2012). Design and development of microbioreactors for long-term cell culture in controlled oxygen microenvironments. Biomed. Microdevices 14 (1), 145–152. doi:10.1007/s10544-011-9592-9
Abate, M. F., Ahmed, M. G., Li, X., Yang, C., and Zhu, Z. (2020). Distance-based paper/PMMA integrated ELISA-chip for quantitative detection of immunoglobulin G. Lab a Chip 20 (19), 3625–3632. doi:10.1039/d0lc00505c
Ahmadianyazdi, A., Miller, I. J., and Folch, A. (2023). Tunable resins with PDMS-like elastic modulus for stereolithographic 3D-printing of multimaterial microfluidic actuators. Lab a Chip 23 (18), 4019–4032. doi:10.1039/d3lc00529a
Akbari, Z., Raoufi, M. A., Mirjalali, S., and Aghajanloo, B. (2023). A review on inertial microfluidic fabrication methods. Biomicrofluidics 17 (5), 051504. doi:10.1063/5.0163970
AlMashrea, B. A., Almehdi, A. M., and Damiati, S. (2024). Simple microfluidic devices for in situ detection of water contamination: a state-of-art review. Front. Bioeng. Biotechnol. 12, 1355768. doi:10.3389/fbioe.2024.1355768
Annabi, N., Selimović, Š., Acevedo Cox, J. P., Ribas, J., Afshar Bakooshli, M., Heintze, D., et al. (2013). Hydrogel-coated microfluidic channels for cardiomyocyte culture. Lab a Chip 13 (18), 3569–3577. doi:10.1039/c3lc50252j
Apoorva, S., Nguyen, N. T., and Sreejith, K. R. (2024). Recent developments and future perspectives of microfluidics and smart technologies in wearable devices. Lab. Chip 24, 1833–1866. doi:10.1039/d4lc00089g
Bandara, G. C., Heist, C. A., and Remcho, V. T. (2018). Patterned polycaprolactone-filled glass microfiber microfluidic devices for total protein content analysis. Talanta 176, 589–594. doi:10.1016/j.talanta.2017.08.031
Beebe, D. J., Moore, J. S., Bauer, J. M., Yu, Q., Liu, R. H., Devadoss, C., et al. (2000). Functional hydrogel structures for autonomous flow control inside microfluidic channels. Nature 404 (6778), 588–590. doi:10.1038/35007047
Berry, S. B., Zhang, T., Day, J. H., Su, X., Wilson, I. Z., Berthier, E., et al. (2017). Upgrading well plates using open microfluidic patterning. Lab a Chip 17 (24), 4253–4264. doi:10.1039/c7lc00878c
Blazej, R. G., Kumaresan, P., and Mathies, R. A. (2006). Microfabricated bioprocessor for integrated nanoliter-scale Sanger DNA sequencing. Proc. Natl. Acad. Sci. 103 (19), 7240–7245. doi:10.1073/pnas.0602476103
Brassard, D., Geissler, M., Descarreaux, M., Tremblay, D., Daoud, J., Clime, L., et al. (2019). Extraction of nucleic acids from blood: unveiling the potential of active pneumatic pumping in centrifugal microfluidics for integration and automation of sample preparation processes. Lab a Chip 19 (11), 1941–1952. doi:10.1039/c9lc00276f
Broccoli, A., Vollertsen, A. R., Roels, P., van Vugt, A., van den Berg, A., and Odijk, M. (2023). Nanoparticle printing for microfluidic applications: bipolar electrochemistry and localized Raman sensing spots. Micromachines (Basel). 14 (2), 453. doi:10.3390/mi14020453
Bunge, F., van den Driesche, S., and Vellekoop, M. J. (2018). PDMS-free microfluidic cell culture with integrated gas supply through a porous membrane of anodized aluminum oxide. Biomed. Microdevices 20 (4), 98. doi:10.1007/s10544-018-0343-z
Busek, M., Nøvik, S., Aizenshtadt, A., Amirola-Martinez, M., Combriat, T., Grünzner, S., et al. (2021). Thermoplastic elastomer (TPE)-Poly(Methyl methacrylate) (PMMA) hybrid devices for active pumping PDMS-free organ-on-a-chip systems. Biosens. (Basel). 11 (5), 162. doi:10.3390/bios11050162
Capodacqua, F. M. C., Volpe, A., Gaudiuso, C., and Ancona, A. (2023). Bonding of PMMA to silicon by femtosecond laser pulses. Sci. Rep. 13 (1), 5062. doi:10.1038/s41598-023-31969-y
Carvell, T., Burgoyne, P., Milne, L., Campbell, J. D. M., Fraser, A. R., and Bridle, H. (2024). Human leucocytes processed by fast-rate inertial microfluidics retain conventional functional characteristics. J. R. Soc. Interface 21 (212), 20230572. doi:10.1098/rsif.2023.0572
Chang, C.-W., Cheng, Y.-J., Tu, M., Chen, Y.-H., Peng, C.-C., Liao, W.-H., et al. (2014). A polydimethylsiloxane–polycarbonate hybrid microfluidic device capable of generating perpendicular chemical and oxygen gradients for cell culture studies. Lab. Chip 14 (19), 3762–3772. doi:10.1039/c4lc00732h
Chartier, I., Bory, C., Fuchs, A., Freida, D., Manaresi, N., Ruty, M., et al. (2003) Fabrication of hybrid plastic-silicon microfluidic devices for individual cell manipulation by dielectrophoresis. Proceedings Volume 5345, Microfluidics, BioMEMS, and Medical Microsystems II. doi:10.1117/12.530705
Chen, Y., Hu, Y., and Lu, X. (2023). An integrated paper microfluidic device based on isothermal amplification for simple sample-to-answer detection of Campylobacter jejuni. Appl. Environ. Microbiol. 89 (7), e0069523. doi:10.1128/aem.00695-23
Coelho, B. J., Neto, J. P., Sieira, B., Moura, A. T., Fortunato, E., Martins, R., et al. (2023). Hybrid digital-droplet microfluidic chip for applications in droplet digital nucleic acid amplification: design, fabrication and characterization. Sensors (Basel) 23 (10), 4927. doi:10.3390/s23104927
Cong, X.-Z., Feng, J., Zhang, H.-J., Zhang, L.-Z., Lin, T.-Y., Chen, G., et al. (2024). Microfluidic device-based in vivo detection of PD-L1-positive small extracellular vesicles and its application for tumor monitoring. Anal. Chem. 96 (6), 2658–2665. doi:10.1021/acs.analchem.3c05418
Convery, N., and Gadegaard, N. (2019). 30 years of microfluidics. Micro Nano Eng. 2, 76–91. doi:10.1016/j.mne.2019.01.003
David, P., Peter, A., Barbara, M., Randy, A., Sanna, M., Meng, Z., et al. (2019). High-throughput microfluidic cell sorting platform (MICS). Protoc. Exch. doi:10.21203/rs.2.10282/v1
Do, T. D., Pham, U. T., Nguyen, L. P., Nguyen, T. M., Bui, C. N., Oliver, S., et al. (2023). Fabrication of a low-cost microfluidic device for high-throughput drug testing on static and dynamic cancer spheroid culture models. Diagn. (Basel) 13 (8), 1394. doi:10.3390/diagnostics13081394
Dou, M., Dominguez, D. C., Li, X., Sanchez, J., and Scott, G. (2014). A versatile PDMS/paper hybrid microfluidic platform for sensitive infectious disease diagnosis. Anal. Chem. 86 (15), 7978–7986. doi:10.1021/ac5021694
Du, X. Y., and Yang, J. Y. (2024). Biomimetic microfluidic chips for toxicity assessment of environmental pollutants. Sci. Total Environ. 919, 170745. doi:10.1016/j.scitotenv.2024.170745
Du, Y., Reitemeier, J., Jiang, Q., Bappy, M. O., Bohn, P. W., and Zhang, Y. (2024). Hybrid printing of fully integrated microfluidic devices for biosensing. Small 20 (5), 2304966. doi:10.1002/smll.202304966
Eades, J., Audiffred, J. F., Fincher, M., Choi, J. W., Soper, S. A., and Monroe, W. T. (2023). A simple micromilled microfluidic impedance cytometer with vertical parallel electrodes for cell viability analysis. Micromachines (Basel) 14 (2), 283. doi:10.3390/mi14020283
Enck, K., Rajan, S. P., Aleman, J., Castagno, S., Long, E., Khalil, F., et al. (2020). Design of an adhesive film-based microfluidic device for alginate hydrogel-based cell encapsulation. Ann. Biomed. Eng. 48 (3), 1103–1111. doi:10.1007/s10439-020-02453-9
Estrada-Osorio, D. V., Escalona-Villalpando, R. A., Gurrola, M. P., Chaparro-Sánchez, R., Rodríguez-Morales, J. A., Arriaga, L. G., et al. (2024). Abiotic, hybrid, and biological electrocatalytic materials applied in microfluidic fuel cells: a comprehensive review. ACS Meas. Sci. Au 4 (1), 25–41. doi:10.1021/acsmeasuresciau.3c00044
Fan, Y., Liu, S., and Zhang, Y. (2018). Direct bonding of polymer/glass-based microfluidic chips with dry film photoresist. Microsyst. Technol. 24 (3), 1659–1665. doi:10.1007/s00542-017-3541-3
Felici, E., Regiart, M. D., Pereira, S. V., Ortega, F. G., Angnes, L., Messina, G. A., et al. (2023). Microfluidic platform integrated with carbon nanofibers-decorated gold nanoporous sensing device for serum PSA quantification. Biosens. (Basel). 13 (3), 390. doi:10.3390/bios13030390
Gale, B. K., Jafek, A. R., Lambert, C. J., Goenner, B. L., Moghimifam, H., Nze, U. C., et al. (2018). A review of current methods in microfluidic device fabrication and future commercialization prospects. Inventions 3 (3), 60. doi:10.3390/inventions3030060
Gencturk, E., Mutlu, S., and Ulgen, K. O. (2017). Advances in microfluidic devices made from thermoplastics used in cell biology and analyses. Biomicrofluidics 11 (5), 051502. doi:10.1063/1.4998604
Guo, H., Zhao, P., Xiao, G., Zhang, Z., and Yao, J. (2010). Optical manipulation of microparticles in an SU-8/PDMS hybrid microfluidic chip incorporating a monolithically integrated on-chip lens set. IEEE J. Sel. Top. Quantum Electron. 16 (4), 919–926. doi:10.1109/jstqe.2009.2033212
Gupta, U., Kumar, N., Lata, A., Singh, P., and Arun, R. K. (2023). Bio-inspired self-pumping microfluidic device for cleaning of urea using reduced graphene oxide (rGO) modified polymeric nanohybrid membrane. Int. J. Biol. Macromol. 241, 124614. doi:10.1016/j.ijbiomac.2023.124614
Guzniczak, E., Otto, O., Whyte, G., Willoughby, N., Jimenez, M., and Bridle, H. (2020). Deformability-induced lift force in spiral microchannels for cell separation. Lab. Chip 20 (3), 614–625. doi:10.1039/c9lc01000a
Haque, M. E., Conde, A. J., MacPherson, W. N., Knight, S. R., Carter, R. M., and Kersaudy-Kerhoas, M. (2023). A microfluidic finger-actuated blood lysate preparation device enabled by rapid acoustofluidic mixing. Lab a Chip 23 (1), 62–71. doi:10.1039/d2lc00968d
Hassanpour-Tamrin, S., Sanati-Nezhad, A., and Sen, A. (2021). A simple and low-cost approach for irreversible bonding of polymethylmethacrylate and polydimethylsiloxane at room temperature for high-pressure hybrid microfluidics. Sci. Rep. 11 (1), 4821. doi:10.1038/s41598-021-83011-8
Hawkins, J., Browne, E. P., Arcaro, K. F., and Sun, Y. (2024). Rapid cell isolation in breastmilk in a non-clinical setting by a deterministic lateral displacement device and selective water and fat absorption. Lab a Chip 24 (3), 604–614. doi:10.1039/d3lc00899a
Hesari, Z., Soleimani, M., Atyabi, F., Sharifdini, M., Nadri, S., Warkiani, M. E., et al. (2016). A hybrid microfluidic system for regulation of neural differentiation in induced pluripotent stem cells. J. Biomed. Mater. Res. Part A 104 (6), 1534–1543. doi:10.1002/jbm.a.35689
Hill, C., Willoughby, N., and Bridle, H. (2022). Efficient high-concentration dewatering of Chlorella vulgaris utilising spiral inertial microfluidics. Bioresour. Technol. Rep. 18, 101014. doi:10.1016/j.biteb.2022.101014
Hou, X., Zhang, Y. S., Santiago, G. T.-d, Alvarez, M. M., Ribas, J., Jonas, S. J., et al. (2017). Interplay between materials and microfluidics. Nat. Rev. Mater. 2 (5), 17016. doi:10.1038/natrevmats.2017.16
Hua, X., Zhu, Q., Liu, Y., Zhou, S., Huang, P., Li, Q., et al. (2023). A double tangential flow filtration-based microfluidic device for highly efficient separation and enrichment of exosomes. Anal. Chim. Acta 1258, 341160. doi:10.1016/j.aca.2023.341160
Huh, D., Matthews, B. D., Mammoto, A., Montoya-Zavala, M., Hsin, H. Y., and Ingber, D. E. (2010). Reconstituting organ-level lung functions on a chip. Science 328 (5986), 1662–1668. doi:10.1126/science.1188302
Jayan, H., Yin, L., Xue, S., Zou, X., and Guo, Z. (2024). Raman spectroscopy-based microfluidic platforms: a promising tool for detection of foodborne pathogens in food products. Food Res. Int. 180, 114052. doi:10.1016/j.foodres.2024.114052
Kaigala, G. V., Hoang, V. N., Stickel, A., Lauzon, J., Manage, D., Pilarski, L. M., et al. (2008). An inexpensive and portable microchip-based platform for integrated RT–PCR and capillary electrophoresis. Analyst 133 (3), 331–338. doi:10.1039/b714308g
Kanabekova, P., Dauletkanov, B., Bekezhankyzy, Z., Toktarkan, S., Martin, A., Pham, T. T., et al. (2024). A hybrid fluorescent nanofiber membrane integrated with microfluidic chips towards lung-on-a-chip applications. Lab a Chip 24 (2), 224–233. doi:10.1039/d3lc00751k
Kim, H., Min, K.-I., Inoue, K., Im, D. J., Kim, D.-P., and Yoshida, J.-i. (2016). Submillisecond organic synthesis: outpacing Fries rearrangement through microfluidic rapid mixing. Science. 352 (6286), 691–694. doi:10.1126/science.aaf1389
Krakos, A., Cieślak, A., Hartel, E., Łabowska, M. B., Kulbacka, J., and Detyna, J. (2023). 3D bio-printed hydrogel inks promoting lung cancer cell growth in a lab-on-chip culturing platform. Microchim. Acta 190 (9), 349. doi:10.1007/s00604-023-05931-8
Ku, X., Zhuang, G., and Li, G. (2018). A universal approach for irreversible bonding of rigid substrate-based microfluidic devices at room temperature. Microfluid. Nanofluidics 22 (2), 17. doi:10.1007/s10404-018-2039-3
Kuo, J. S., Ng, L., Yen, G. S., Lorenz, R. M., Schiro, P. G., Edgar, J. S., et al. (2009). A new USP Class VI-compliant substrate for manufacturing disposable microfluidic devices. Lab. Chip 9 (7), 870–876. doi:10.1039/b818873d
Kuo, T.-C., Cannon, D. M., Shannon, M. A., Bohn, P. W., and Sweedler, J. V. (2003). Hybrid three-dimensional nanofluidic/microfluidic devices using molecular gates. Sensors Actuators A Phys. 102 (3), 223–233. doi:10.1016/s0924-4247(02)00394-1
Laurenciano, C. J. D., Tseng, C.-C., Chen, S.-J., Lu, S.-Y., Tayo, L. L., and Fu, L.-M. (2021). Microfluidic colorimetric detection platform with sliding hybrid PMMA/paper microchip for human urine and blood sample analysis. Talanta 231, 122362. doi:10.1016/j.talanta.2021.122362
Lei, X., Ye, W., Safdarin, F., and Baghaei, S. (2024). Microfluidics devices for sports: a review on technology for biomedical application used in fields such as biomedicine, drug encapsulation, preparation of nanoparticles, cell targeting, analysis, diagnosis, and cell culture. Tissue Cell 87, 102339. doi:10.1016/j.tice.2024.102339
Lei, Z., Xie, D., Mbogba, M. K., Chen, Z., Tian, C., Xu, L., et al. (2019). A microfluidic platform with cell-scale precise temperature control for simultaneous investigation of the osmotic responses of multiple oocytes. Lab a Chip 19 (11), 1929–1940. doi:10.1039/c9lc00107g
Leung, J. C., Taylor-Kamall, R. W., Hilliker, A. J., and Rezai, P. (2015). Agar-polydimethylsiloxane devices for quantitative investigation of oviposition behaviour of adult Drosophila melanogaster. Biomicrofluidics 9 (3), 034112. doi:10.1063/1.4922737
Li, O.-L., Tong, Y.-L., Chen, Z.-G., Liu, C., Zhao, S., and Mo, J.-Y. (2008). A glass/PDMS hybrid microfluidic chip embedded with integrated electrodes for contactless conductivity detection. Chromatographia 68 (11), 1039–1044. doi:10.1365/s10337-008-0808-y
Li, Y., Xu, F., liu, J., Zhang, Q., and Fan, Y. (2023). Rapid-release reversible bonding of PMMA-based microfluidic devices with PBMA coating. Biomed. Microdevices 26 (1), 6. doi:10.1007/s10544-023-00690-y
Liu, C.-C., Ko, C.-H., Fu, L.-M., and Jhou, Y.-L. (2023). Light-shading reaction microfluidic PMMA/paper detection system for detection of cyclamate concentration in foods. Food Chem. 400, 134063. doi:10.1016/j.foodchem.2022.134063
Lu, M., Lezzar, D. L., Vörös, E., and Shevkoplyas, S. S. (2019). Traditional and emerging technologies for washing and volume reducing blood products. J. Blood Med. 10, 37–46. doi:10.2147/jbm.s166316
Matsui, T., Franzke, J., Manz, A., and Janasek, D. (2007). Temperature gradient focusing in a PDMS/glass hybrid microfluidic chip. Electrophoresis 28 (24), 4606–4611. doi:10.1002/elps.200700272
Moraes, F. C., Lima, R. S., Segato, T. P., Cesarino, I., Cetino, J. L. M., Machado, S. A. S., et al. (2012). Glass/PDMS hybrid microfluidic device integrating vertically aligned SWCNTs to ultrasensitive electrochemical determinations. Lab a Chip 12 (11), 1959–1962. doi:10.1039/c2lc40141j
Mu, X., Liang, Q., Hu, P., Ren, K., Wang, Y., and Luo, G. (2009). Laminar flow used as "liquid etch mask" in wet chemical etching to generate glass microstructures with an improved aspect ratio. Lab. Chip 9 (14), 1994–1996. doi:10.1039/b904769g
Nakatani, M., Tanaka, Y., Okayama, S., and Hashimoto, M. (2020). A simplified PDMS microfluidic device with a built-in suction actuator for rapid production of monodisperse water-in-oil droplets. ELECTROPHORESIS 41 (24), 2114–2121. doi:10.1002/elps.202000105
Nemani, K. V., Moodie, K. L., Brennick, J. B., Su, A., and Gimi, B. (2013). In vitro and in vivo evaluation of SU-8 biocompatibility. Mater. Sci. Eng. C 33 (7), 4453–4459. doi:10.1016/j.msec.2013.07.001
Nguyen, T., Jung, S. H., Lee, M. S., Park, T.-E., Ahn, S.-k., and Kang, J. H. (2019). Robust chemical bonding of PMMA microfluidic devices to porous PETE membranes for reliable cytotoxicity testing of drugs. Lab a Chip 19 (21), 3706–3713. doi:10.1039/c9lc00338j
Nielsen, J. B., Hanson, R. L., Almughamsi, H. M., Pang, C., Fish, T. R., and Woolley, A. T. (2020). Microfluidics: innovations in materials and their fabrication and functionalization. Anal. Chem. 92 (1), 150–168. doi:10.1021/acs.analchem.9b04986
Ou, J., Glawdel, T., Ren, C. L., and Pawliszyn, J. (2009). Fabrication of a hybrid PDMS/SU-8/quartz microfluidic chip for enhancing UV absorption whole-channel imaging detection sensitivity and application for isoelectric focusing of proteins. Lab a Chip 9 (13), 1926–1932. doi:10.1039/b821438g
Palacio-Castañeda, V., Kooijman, L., Venzac, B., Verdurmen, W. P. R., and Le Gac, S. (2020). Metabolic switching of tumor cells under hypoxic conditions in a tumor-on-a-chip model. Micromachines (Basel) 11 (4), 382. doi:10.3390/mi11040382
Pérez-Sosa, C., Peñaherrera-Pazmiño, A. B., Rosero, G., Bourguignon, N., Aravelli, A., Bhansali, S., et al. (2022). Novel reproducible manufacturing and reversible sealing method for microfluidic devices. Micromachines (Basel) 13 (5), 650. doi:10.3390/mi13050650
Pramanik, S. K., and Suzuki, H. (2019). Microfluidic device with a push–pull sequential solution-exchange function for affinity sensing. Microfluid. Nanofluidics 23 (2), 19. doi:10.1007/s10404-019-2188-z
Pritchard, R. H., Zhukov, A. A., Fullerton, J. N., Want, A. J., Hussain, F., la Cour, M. F., et al. (2019). Cell sorting actuated by a microfluidic inertial vortex. Lab a Chip 19 (14), 2456–2465. doi:10.1039/c9lc00120d
Qiu, H., Yan, J., Sun, X., Liu, J., Cao, W., Yang, X., et al. (2003). Microchip capillary electrophoresis with an integrated indium tin oxide electrode-based electrochemiluminescence detector. Anal. Chem. 75 (20), 5435–5440. doi:10.1021/ac034500x
Qiu, J., Li, J., Guo, Z., Zhang, Y., Nie, B., Qi, G., et al. (2023). 3D printing of individualized microfluidic chips with DLP-based printer. Materials 16 (21), 6984. doi:10.3390/ma16216984
Raj M, K., and Chakraborty, S. (2020). PDMS microfluidics: a mini review. J. Appl. Polym. Sci. 137 (27), 48958. doi:10.1002/app.48958
Ramachandraiah, H., Svahn, H. A., and Russom, A. (2017). Inertial microfluidics combined with selective cell lysis for high throughput separation of nucleated cells from whole blood. RSC Adv. 7 (47), 29505–29514. doi:10.1039/c7ra02992f
Razavi Bazaz, S., Rouhi, O., Raoufi, M. A., Ejeian, F., Asadnia, M., Jin, D., et al. (2020). 3D printing of inertial microfluidic devices. Sci. Rep. 10 (1), 5929. doi:10.1038/s41598-020-62569-9
Ren, K., Zhou, J., and Wu, H. (2013). Materials for microfluidic chip fabrication. Accounts Chem. Res. 46 (11), 2396–2406. doi:10.1021/ar300314s
Reyes, D. R., Esch, M. B., Ewart, L., Nasiri, R., Herland, A., Sung, K., et al. (2024). From animal testing to in vitro systems: advancing standardization in microphysiological systems. Lab. Chip 24 (5), 1076–1087. doi:10.1039/d3lc00994g
Richard, C., Neild, A., and Cadarso, V. J. (2020). The emerging role of microfluidics in multi-material 3D bioprinting. Lab a Chip 20 (12), 2044–2056. doi:10.1039/c9lc01184f
Roychoudhury, A., Dear, J. W., Kersaudy-Kerhoas, M., and Bachmann, T. T. (2023). Amplification-free electrochemical biosensor detection of circulating microRNA to identify drug-induced liver injury. Biosens. Bioelectron. 231, 115298. doi:10.1016/j.bios.2023.115298
Sanjay, S. T., Li, M., Zhou, W., Li, X., and Li, X. (2020). A reusable PMMA/paper hybrid plug-and-play microfluidic device for an ultrasensitive immunoassay with a wide dynamic range. Microsyst. Nanoeng. 6, 28. doi:10.1038/s41378-020-0143-5
Santaniello, T., Yan, Y., Tocchio, A., Martello, F., Milani, P., and Lenardi, C. (2015). A room-temperature bonding technique for the packaging of hydrogel-based hybrid microfluidic devices. Microfluid. Nanofluidics 19 (1), 31–41. doi:10.1007/s10404-015-1544-x
Scott, S. M., and Ali, Z. (2021). Fabrication methods for microfluidic devices: an overview. Micromachines 12 (3), 319. doi:10.3390/mi12030319
Shameli, S. M., Glawdel, T., Liu, Z., and Ren, C. L. (2012). Bilinear temperature gradient focusing in a hybrid PDMS/glass microfluidic chip integrated with planar heaters for generating temperature gradients. Anal. Chem. 84 (6), 2968–2973. doi:10.1021/ac300188s
Simon, D., Obst, F., Haefner, S., Heroldt, T., Peiter, M., Simon, F., et al. (2019). Hydrogel/enzyme dots as adaptable tool for non-compartmentalized multi-enzymatic reactions in microfluidic devices. React. Chem. Eng. 4 (1), 67–77. doi:10.1039/c8re00180d
Sivakumar, R., Trinh, K. T. L., and Lee, N. Y. (2020). Heat and pressure-resistant room temperature irreversible sealing of hybrid PDMS-thermoplastic microfluidic devices via carbon-nitrogen covalent bonding and its application in a continuous-flow polymerase chain reaction. RSC Adv. 10 (28), 16502–16509. doi:10.1039/d0ra02332a
Solsona, M., Vollenbroek, J. C., Tregouet, C. B. M., Nieuwelink, A. E., Olthuis, W., van den Berg, A., et al. (2019). Microfluidics and catalyst particles. Lab a Chip 19 (21), 3575–3601. doi:10.1039/c9lc00318e
Sun, Z., Ma, C., Yu, C., and Li, Z. (2024). Microplastic separation and enrichment in microchannels under derivative electric field gradient by bipolar electrode reactions. Sci. Rep. 14 (1), 4626. doi:10.1038/s41598-024-54921-0
Suzuki, Y., Yamada, M., and Seki, M. (2010). Sol–gel based fabrication of hybrid microfluidic devices composed of PDMS and thermoplastic substrates. Sensors Actuators B Chem. 148 (1), 323–329. doi:10.1016/j.snb.2010.04.018
Tawade, P., and Mastrangeli, M. (2024). Integrated electrochemical and optical biosensing in organs-on-chip. ChemBioChem. 25 (3), e202300560. doi:10.1002/cbic.202300560
Thaweeskulchai, T., and Schulte, A. (2022). Diode laser and polyimide tape enables cheap and fast fabrication of flexible microfluidic sensing devices. Micromachines (Basel). 13 (12), 2214. doi:10.3390/mi13122214
Thaweeskulchai, T., and Schulte, A. (2023). Basic guide to multilayer microfluidic fabrication with polyimide tape and diode laser. Micromachines (Basel). 14 (2), 324. doi:10.3390/mi14020324
Trappmann, B., Baker, B. M., Polacheck, W. J., Choi, C. K., Burdick, J. A., and Chen, C. S. (2017). Matrix degradability controls multicellularity of 3D cell migration. Nat. Commun. 8 (1), 371. doi:10.1038/s41467-017-00418-6
Tseng, C.-C., Lu, S.-Y., Chen, S.-J., Wang, J.-M., Fu, L.-M., and Wu, Y.-H. (2022). Microfluidic aptasensor POC device for determination of whole blood potassium. Anal. Chim. Acta 1203, 339722. doi:10.1016/j.aca.2022.339722
Tu, Q., Wang, J.-C., Zhang, Y., Liu, R., Liu, W., Ren, L., et al. (2012). Surface modification of poly(dimethylsiloxane) and its applications in microfluidics-based biological analysis. Rev. Anal. Chem. 31 (3-4), 177–192. doi:10.1515/revac-2012-0016
Tzianni, Ε. I., Moutsios, I., Moschovas, D., Avgeropoulos, A., Govaris, K., Panagiotidis, L., et al. (2022). Smartphone paired SIM card-type integrated creatinine biosensor. Biosens. Bioelectron. 207, 114204. doi:10.1016/j.bios.2022.114204
Vedhanayagam, A., Golfetto, M., Ram, J. L., and Basu, A. S. (2023). Rapid micromolding of sub-100 µm microfluidic channels using an 8K stereolithographic resin 3D printer. Micromachines (Basel) 14 (8), 1519. doi:10.3390/mi14081519
Wang, C., Weng, G., Li, J., Zhu, J., and Zhao, J. (2024). A review of SERS coupled microfluidic platforms: from configurations to applications. Anal. Chim. Acta 1296, 342291. doi:10.1016/j.aca.2024.342291
Wang, F., and Burns, M. A. (2009). Performance of nanoliter-sized droplet-based microfluidic PCR. Biomed. Microdevices 11 (5), 1071–1080. doi:10.1007/s10544-009-9324-6
Washburn, A. L., Gunn, L. C., and Bailey, R. C. (2009). Label-Free quantitation of a cancer biomarker in complex media using silicon photonic microring resonators. Anal. Chem. 81 (22), 9499–9506. doi:10.1021/ac902006p
Wei, Y., Wang, T., Wang, Y., Zeng, S., Ho, Y.-P., and Ho, H.-P. (2023). Rapid prototyping of multi-functional and biocompatible parafilm®-based microfluidic devices by laser ablation and thermal bonding. Micromachines 14 (3), 656. doi:10.3390/mi14030656
Wong, I., Atsumi, S., Huang, W. C., Wu, T. Y., Hanai, T., Lam, M. L., et al. (2010). An agar gel membrane-PDMS hybrid microfluidic device for long term single cell dynamic study. Lab. Chip 10 (20), 2710–2719. doi:10.1039/c004719h
Wu, A., Wang, L., Jensen, E., Mathies, R., and Boser, B. (2010). Modular integration of electronics and microfluidic systems using flexible printed circuit boards. Lab. Chip 10 (4), 519–521. doi:10.1039/b922830f
Wu, H., Huang, B., and Zare, R. N. (2006). Generation of complex, static solution gradients in microfluidic channels. J. Am. Chem. Soc. 128 (13), 4194–4195. doi:10.1021/ja058530o
Wu, M.-H., Cai, H., Xu, X., Urban, J. P. G., Cui, Z.-F., and Cui, Z. (2005). A SU-8/PDMS hybrid microfluidic device with integrated optical fibers for online monitoring of lactate. Biomed. Microdevices 7 (4), 323–329. doi:10.1007/s10544-005-6074-y
Xiang, J., Qi, J., Hu, D., Wang, C., Wang, L., Wu, Y., et al. (2024). Molecularly imprinted metal-organic frameworks assisted cloth and paper hybrid microfluidic devices for visual detection of gonyautoxin. J. Hazard. Mater. 469, 133969. doi:10.1016/j.jhazmat.2024.133969
Xie, Y., Dai, L., and Yang, Y. (2022). Microfluidic technology and its application in the point-of-care testing field. Biosens. Bioelectron. X 10, 100109. doi:10.1016/j.biosx.2022.100109
Yang, S. H., Park, J., Youn, J. R., and Song, Y. S. (2018). Programmable microfluidic logic device fabricated with a shape memory polymer. Lab a Chip 18 (18), 2865–2872. doi:10.1039/c8lc00627j
Yi, H., Fu, T., Ma, D., Zhu, C., and Ma, Y. (2024). Spontaneous transfer of droplets across a microfluidic liquid-liquid interface. Langmuir 40, 5508–5517. doi:10.1021/acs.langmuir.4c00047
Yuan, B., Jin, Y., Sun, Y., Wang, D., Sun, J., Wang, Z., et al. (2012). A strategy for depositing different types of cells in three dimensions to mimic tubular structures in tissues. Adv. Mater. 24 (7), 890–896. doi:10.1002/adma.201104589
Zhou, J., Ren, K., Zheng, Y., Su, J., Zhao, Y., Ryan, D., et al. (2010). Fabrication of a microfluidic Ag/AgCl reference electrode and its application for portable and disposable electrochemical microchips. ELECTROPHORESIS 31 (18), 3083–3089. doi:10.1002/elps.201000113
Zhou, J., Yan, H., Zheng, Y., and Wu, H. (2009). Highly fluorescent poly(dimethylsiloxane) for on-chip temperature measurements. Adv. Funct. Mater. 19 (2), 324–329. doi:10.1002/adfm.200801064
Zhu, J., Wang, Y., Tang, S., Su, H., Wang, X., Du, W., et al. (2023). A PDMS–agar hybrid microfluidic device for the investigation of chemical–mechanical associative learning behavior of C. elegans. Micromachines 14 (8), 1576. doi:10.3390/mi14081576
Zhu, Z., Wu, D., Li, S., Han, Y., Xiang, N., Wang, C., et al. (2021). A polymer-film inertial microfluidic sorter fabricated by jigsaw puzzle method for precise size-based cell separation. Anal. Chim. Acta 1143, 306–314. doi:10.1016/j.aca.2020.11.001
Keywords: hybrid, microfluidic device, microfluidics, manufactoring, fabrication, materials
Citation: Carvell T, Burgoyne P, Fraser AR and Bridle H (2024) Categorising hybrid material microfluidic devices. Front. Lab. Chip. Technol. 3:1412290. doi: 10.3389/frlct.2024.1412290
Received: 04 April 2024; Accepted: 30 April 2024;
Published: 13 May 2024.
Edited by:
Nan Zhang, University College Dublin, IrelandReviewed by:
Yiqiang Fan, Beijing University of Chemical Technology, ChinaCopyright © 2024 Carvell, Burgoyne, Fraser and Bridle. This is an open-access article distributed under the terms of the Creative Commons Attribution License (CC BY). The use, distribution or reproduction in other forums is permitted, provided the original author(s) and the copyright owner(s) are credited and that the original publication in this journal is cited, in accordance with accepted academic practice. No use, distribution or reproduction is permitted which does not comply with these terms.
*Correspondence: Helen Bridle, aC5sLmJyaWRsZUBody5hYy51aw==