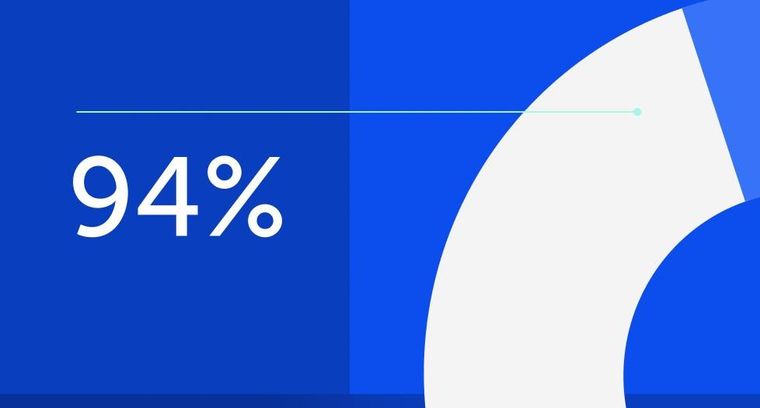
94% of researchers rate our articles as excellent or good
Learn more about the work of our research integrity team to safeguard the quality of each article we publish.
Find out more
REVIEW article
Front. Lab Chip Technol., 30 April 2024
Sec. Organ on a Chip
Volume 3 - 2024 | https://doi.org/10.3389/frlct.2024.1383783
This article is part of the Research TopicReviews in Organ on a ChipView all 4 articles
For patients with end stage organ failure, organ transplant is frequently the only curative option available. However, organs available for transplant are in critically short supply around the world, which has led to lengthy wait times and increased mortality. Increased global life expectancy, coupled with raised age thresholds for recipients, has heightened demand and further compounded the need for alternative strategies. Bioengineering substitutes including organ-on-a-chip and 3D bioprinting technologies have made considerable strides toward whole organ generation. Skin is the organ where the most advances have been made thus far, due to the relatively less complex spatial architecture and industry interest in the development of sophisticated models for pharmaceutical and cosmetics testing. Here, we discuss the challenges of recapitulating the complexity of native skin, including a stratified structure, vascularization, and inclusion of skin appendages, such as hair follicles and sweat glands. We discuss current technological and biological progress in the field of tissue and organ bioengineering as well as highlight future challenges to generate de novo tissue for skin grafting.
For many patients with end-stage organ dysfunction, organ transplantation offers the only realistic chance for survival or substantial improvement of quality of life (Black et al., 2018). Although there has been a large increase in transplants performed over the past 30 years, unfortunately, organ demand currently far outstrips supply (Shacham et al., 2018) as there has been an even greater increase in the number of patients added to waiting lists.
Therefore, researchers have discussed the idea of lab-grown tissue and organs as an alternative pathway to investigate the multiple challenges associated with organ transplant. Although the scientific community has not yet generated fully functional, lab-grown organs, strides in microfabrication, iPSC technologies, and in vitro techniques are generating advanced and innovative bioengineered in vitro tissue constructs that may bring us closer to achieving this ambitious milestone. In this context, the skin is the most accessible tissue, and researchers are exploring a variety of approaches and techniques to generate skin tissue in the lab for grafting purposes.
Other reviews have discussed in detail the cells or scaffolds available to generate skin tissue constructs (Catalano et al., 2013; Chaudhari et al., 2016; Vig et al., 2017; Dearman et al., 2021a; Weng et al., 2021a; Sierra-Sánchez et al., 2021; Wei et al., 2022). This review focuses on reviewing the wide range of techniques available as well as discussing the biological milestones achieved in recent years. Thus, in the first section of this review, we discuss the key structural and functional parameters inherent to native skin, along with guiding factors for generating gold-standard skin constructs. We then move to review the use of bioengineering technologies, including skin-on-a-chip platforms (section 2), electrodynamic methods (section 3), and 3D bioprinting for dermatology research (section 4). Finally, in section 5, we offer a roadmap for clinical translation, identifying current challenges and future directions in the field. Through synthesizing these discussions our aim is to provide a comprehensive overview of tissue engineering for skin tissue generation, highlighting key advancements and outlining future directions and challenges.
Due to its role as the first line of defense against injury, skin remains vulnerable to damage with lacerations and burns arising from thermal, friction, radiation, or chemical agents (Zhou et al., 2020). Some of this damage can be severe, such as third-degree burns or diabetic ulcers, which are difficult to heal and can be life-threatening (Chen et al., 2023). Chronic wounds, such as diabetic ulcers, often fail to heal properly whilst large burns are problematic to repair due to the massive loss of tissue or necrosis-induced inability to develop a provisional ECM (Bhardwaj et al., 2017). Genetic and chronic dermatological conditions such as epidermolysis bullosa can also lead to an increase need for skin grafts. Thus, skin grafts are among the most common surgical procedures with more than 160,000 procedures performed per year for severe burn victims in the US alone (Serebrakian et al., 2018).
Arguably, the role of any engineered skin construct is to restore barrier function in patients with severe skin damage since any full thickness wound with a diameter greater than 4 cm is unlikely to heal adequately on its own (MacNeil, 2007; Jorgensen et al., 2023) Skin grafts also play a fundamental role in accelerating healing processes in chronic ulcers or pressure sores, reducing pain in superficial burns, or ameliorating suboptimally healed wounds (MacNeil, 2007). The current gold standard approach is autologous grafting, which relies on using the patient’s own skin. Autologous grafts can be either split thickness where all of the epidermis and part of the dermis is taken from a healthy area of skin elsewhere on the patient’s body, or full thickness where the epidermis and dermis are harvested from the donor site. While autologous grafts decrease the chances of rejection, the harvesting process generates a secondary wound site, that is subject to infection and eventual bacterial sepsis (MacNeil, 2007; Goodarzi et al., 2018). Moreover, autologous grafting can only be performed in small areas of the body and the procedure often can result in considerable pain and long-term hospitalization (Goodarzi et al., 2018; Przekora, 2020). Another option is allogeneic grafts from deceased donors or porcine xenogeneic grafts. However, these only offer temporary coverage due to rejection by the recipient’s immune system (Yamamoto et al., 2018) and there are uncertainties surrounding long-term clinical safety (Goodarzi et al., 2018). Therefore, bioengineered skin constructs are a promising solution to the limitations of conventional grafting methods and could potentially reduce morbidity and enhance quality of life (Przekora, 2020). Accordingly, as the human body lacks the ability to close deep wounds or heal extensive burns and chronic lesions without surgical assistance, there is an unmet need for more effective, low-cost, and scalable interventions (Jorgensen et al., 2023). However, successful development of skin tissue demands a comprehensive understanding of skin structure and cellular composition in order to maximize their therapeutic potential.
Skin represents the largest, fastest growing organ in the body, and it maintains homeostatic balance, reduces water loss, and serves as an essential barrier against physical, chemical, and biological hazards. Anatomically, the skin is traditionally divided into three layers (Figure 1). The epidermis represents the outermost skin layer and encompasses a 0.1–0.2 mm squamous cell epithelium predominantly composed of multiple stratified layers of keratinocytes, with the uppermost cornified layer, the stratum corneum, exposed to air. This layer also hosts other cell types such as melanocytes (i.e., melanin-producing cells responsible for skin pigmentation), immune cells (e.g., Langerhans cells), and other mechanosensory structures (e.g., Merkel cells) (Risueño et al., 2021). The dermis lies beneath the epidermis and is a 2–6 mm connective tissue layer with fibroblasts as the primary cellular component, along with macrophages, mast cells, adipocytes, lymphocytes, and Schwann cells (Risueño et al., 2021). The dermis also includes blood and lymphatic vessels, contributing to cell trafficking in and out of the skin and providing nutrients for the epidermis. This layer contributes towards both tensile strength and elasticity, remodels ECM components including collagen and fibronectin, and hosts the vascular network, sensory receptors, sweat glands and hair follicles (Risueño et al., 2021). The hypodermis is the innermost layer of the skin, and is predominantly composed of adipocytes and endothelial cells, playing numerous roles in native skin including vascularization, immune activity, cushioning, and separating the skin from the muscle beneath (Oualla-Bachiri et al., 2020).
Figure 1. Structure of the skin. (A) Illustration of human skin layers, depicting structures from epidermis to subcutaneous fat layer. (B) Detailed diagram highlighting the five layers comprising the epidermis: Stratum Corneum, Stratum Lucidum, Stratum Granulosum, Stratum Spinosum, and Stratum Basale.
Overall, skin grafts used in the clinic are classified into several categories according to their complexity. The most employed skin grafts are Class I skin substitutes, which are temporary wound dressings without cellular components; these can be single layered constructs that are either natural or synthetic, or bilayered engineered substitutes. Class II surrogates are permanent constructs; however, these are single layered and are either epidermal or dermal. Class III structures are composite constructs with increased complexity. Class III can be subdivided into xenografts, autografts, allografts or can be bioengineered skin surrogates (Vecin and Kirsner, 2023). In addition to providing a provisional ECM, skin constructs can further accelerate wound healing by inducing crucial aspects of natural healing and functional tissue regeneration through the addition of bioactive molecules, such as growth factors, cell binding peptides, antimicrobial molecules, or liposomes, can foster chemical signaling during each of the four stages (Chouhan et al., 2018).
Numerous skin substitutes are commercially available, yet none recapitulate the structural and functional complexity of human skin (Mansbridge, 2020) and have been plagued by integration issues, immune rejection, and material bio-incompatibility (Phua et al., 2021). Skin substitutes currently on the market can be acellular or cellularized with allogeneic or xenogenic (i.e., animal origin) sources. Acellular dermal products, such as Alloderm, Biobrane, and Dermacell, are comprised of ECM components and are used to treat burns or chronic ulcers; these can promote the formation of new ECM growth and the influx of fibroblasts and endothelial cells (Urciuolo et al., 2019). However, acellular dermal products often constitute a temporal wound dressing rather than an integrated skin substitute (Oualla-Bachiri et al., 2020). Cellular products are seeded with either keratinocytes, fibroblasts, or both. These cellularized constructs can include an epidermal layer (Epicel); dermal (Transcyte); or have double layer such as Apligraf. Apligraf was FDA-approved in 1998 and it has been extensively used to treat chronic ulcers as it mimics aspects of human wound healing, such as the production of cytokines and growth factors (Oualla-Bachiri et al., 2020); however, there are problems surrounding the short shelf-life, fragility, and response to the allogeneic components (Urciuolo et al., 2019).
The ideal skin construct recapitulates the structure and function of native skin (Figure 2). Specifically, from the outside in, the layers of the skin are the epidermis, dermis, and hypodermis. The hypodermis is frequently omitted from the majority of skin constructs, despite playing a crucial role in human skin functionality (Zimoch et al., 2021). This absence is perhaps due to the mechanical fragility of this tissue and inconsistent viability of mature adipocytes when seeded into 3D matrices (Zimoch et al., 2021). Additionally, since the role of the hypodermis in the skin barrier function is limited, most of the early skin dressings have focused upon generating epi-dermal layers. However, recent evidence is highlighting the role of hypodermis in multiple functions including immune response or hormone regulation. Other relevant characteristics of skin grafts are the ability to promote vascularization, resist infection, and tolerate mechanical shear forces. Ideally, skin constructs should also include the presence of skin appendages, such as sweat glands and hair follicles, which are present in native skin and play relevant functions (Dearman et al., 2021b).
Figure 2. The figure illustrates crucial considerations in skin tissue engineering. (A) The extracellular matrix (ECM) in the dermis, consisting of collagens, glycosaminoglycans, and elastins, contributes to the mechanical properties essential for skin functionality. (B) Addressing minimal antigenicity is vital, as allogeneic skin surrogates pose rejection risks, especially in compromised immune systems. (C) Ensuring vascularization is crucial for graft success, (D) while the inclusion of skin appendages, such as hair follicles and glands, enhances skin functionality. (E) Adhesive properties are essential for both temporary wound dressings and permanent grafts, promoting integration with host tissue.
In vivo models have been the gold standard in preclinical research and have significantly advanced our understanding of complex interactions within an entire living system and elucidated the pathogenesis of the immune response, tissue rejection, and immunosuppressive protocols (Weinhart et al., 2019). Numerous species, such as rodents and swine have been indispensable in the pursuit of understanding complex biochemical processes involved in burns, lacerations, surgical site infections, abrasions, acute and chronic wounds, and an array of pathogenic microorganisms involved in skin infections (Ding et al., 2022).
To mitigate some of the issues inherent in using animal models, researchers have widened the pool of species for dermatology research to include rabbits, non-human primates, and pigs among others (Avci et al., 2013). Nonetheless, fundamental differences exist between animal and human skin. For instance, mechanisms involved in wound closure exhibit critical differences between murine and human skin, thus limiting clinical translation (Bang et al., 2022). Structurally, human skin presents relevant differences such as thicker epidermal layer (i.e., few dozens of cells) as opposed to mouse skin (i.e., few cells). Thus, only certain species are suitable for xenografts, predominantly wild type pigs, which offer a larger supply of skin tissue at a lower cost. However, the risk of vascularization failure, zoonoses, vascular disease, and even cancer are key considerations (Yamamoto et al., 2018).
Therefore, whilst animal models can be utilized to evaluate the safety and efficacy of novel approaches, such studies may not deliver translational results. Additionally, animal models can be costly, low throughput, and there are ethical concerns that have led to the development of the 3Rs (Refinement, Reduction, and Replacement), guiding principles to minimize the number of animals used (Saglam-Metiner et al., 2019). An in-depth discussion regarding the current models for organ transplantation is beyond the scope of the present study and has been covered in (Wenzel et al., 2021).
2D cell studies have a long history in preclinical dermatology research and make use of adherent monolayers of human keratinocytes or fibroblasts as a reductionist skin construct. 2D in vitro models (i.e., culture flasks and well-plates) are inexpensive, highly reproducible, and high throughput. However, they lack the complexity of 3D environments and tissue organization, which profoundly affect cell function. In this context, most 2D systems are characterized by the absence of cell-matrix interactions, overly stiff substrates (e.g., polystyrene Young’s modulus is orders of magnitude larger than human skin), and forced apical-basal polarity, which alters cell morphology and function. These environmental cues also induce alterations in gene expression, cell proliferation, differentiation, and even apoptosis (Fernandez-Carro et al., 2022). Furthermore, 2D static culture conditions cannot adequately capture the mechanical stresses (e.g., shear stress) that cells find in in vivo skin (Li and Kilian, 2015; Jensen and Teng, 2020; Rama Varma and Fathi, 2023). Consequently, although conventional 2D cell culture has undoubtedly provided useful scientific insight into cell behavior and response to stimuli, they struggle to generate structurally accurate and fully functional skin constructs for tissue transplantation.
Representing an advance on basic 2D co-cultures, 3D models permit the generation of layers that better resemble the in vivo structure and are thus a better representation of human skin. In terms of skin models, Transwells have been extensively used to generate stratified epitheliums that include the same strata than in vivo skin. These models often use Transwells plates as co-culture systems where dermal fibroblasts are cultured on the bottom of the well and keratinocytes are cultured on top of the porous insert. This prevents direct contact between cell types but enables exchange of soluble factors that promote keratinocyte differentiation into a stratified epithelium (Hofmann et al., 2023). Researchers have also combined 3D hydrogels and Transwells to generate more sophisticated models. In these models, fibroblasts mixed with a 3D collagen matrix are cast within the Transwell insert and after collagen polymerization keratinocytes are seeded on top of the collagen matrix. The Transwell is initially submerged in media to allow cell attachment and after a few days in culture the insert is lifted to generate an air-liquid interface. This approach exposes the apical side of keratinocytes to air to induce differentiation into a stratified epithelium while the basolateral side receives nutrients through the hydrogel and culture media underneath (Hofmann et al., 2023).
3D models permit the generation of multiple stacked layers of varying cellular and molecular complexity, enabling the inclusion of the extracellular matrix (ECM) and cell-ECM interactions. Despite these significant improvements, Transwell models still lack some of the structures found in vivo, including vascularization (Sun et al., 2023) and an absence of immune cells, which play a critical role in inflammation, wound healing, and microbiome tolerance (Hofmann et al., 2023). Consequently, these factors limit opportunities for tissue transplantation.
Other classic 3D models include spheroids and organoids, which are 3D self-organizing mass of cells. Multiple studies have shown the capacity of these organoids to generate structures that resemble those found in vivo (e.g., brain organoids). However, in the case of skin, organoids and spheroids struggle to induce keratinocyte differentiation into a stratified epithelium due to challenges associated with maintaining and air-liquid interface (Randall et al., 2018). Therefore, they have been used to generate other skin structures such as appendages. Bang et al. (2022) described a protocol to generate human induced pluripotent stem cell (hiPSC)-derived hair-bearing, intricately structured organoids, akin to 18-week-old fetal tissue, which possess stratified epidermal and dermal layers, pigmented hair follicles, sebaceous glands, Merkel cells, and even sensory neurons to mimic the neural circuitry required for touch.
Nonetheless, there are significant limitations which hinder their utility in clinical practice as they are also difficult to scale in part due the absence of a perfused vascular system, which causes hypoxia, nutrient starvation, and waste product accumulation at the core of the organoid (Achilli et al., 2012). Overall, the majority of traditional models suffer from a lack of structural complexity, as they cannot mimic the dynamic 3D environment, depth, or permeability of native skin, and thus are unsuitable for transplantation. Consequently, there is an urgent unmet need for skin surrogates, which can recapitulate the complexity of native skin.
Advances in microtechnologies and microfabrication during the last 2 decades have led to the development of advanced microphysiological systems, which include organ-on-a-chip platforms, a versatile alternative to traditional methods to generate tissue and organ-like constructs. Microphysiological systems are based on 3D in vitro platforms that mimic relevant features of the tissue or organ architecture (e.g., liver-on-a-chip). These platforms leverage the predictable behavior of fluids at the microscale (e.g., laminar flow) to control cell organization, thus allowing the user to generate spatially controlled structures (e.g., liver-sinusoid). These technologies allow the generation of microtissues/organs with specific configurations, biocompatibility, flexible manipulation, and micro/nanoscale integration (Ma et al., 2018). Such techniques enable the investigation of additional variables compared to traditional in vitro platforms or animal models (Richard et al., 2020). Microtechnologies also offer the opportunity to study the effects of so-called lifestyle factors, such as environmental pollution; xenotransplantations; rejection and patient-specific precision models; drug toxicity, immunosuppressive protocols; and secondary tumor development in a more physiologically relevant manner.
Organ-on-a-chip platforms, also known as tissue-chips, are in vitro devices that include one or more microchambers often connected by a series of microchannels designed to mimic the in vivo organization (Figure 3). Organ-on-a-chip devices can be manufactured in several materials, including thermoplastics, elastomers, or even glass, with polydimethylsiloxane (PDMS) being one of the most common ones due to its transparency, ability to support pneumatic valves, and permeability to oxygen and carbon dioxide. Most organ-on-a-chip rely on culturing cells in microchambers that mimic the tin vivo organization while they use a network of microchannels to perfuse media, drugs, or cells to study cell biology. Porous membranes are often used to separate multiple cell types in a way that resembles the spatial organization observed in vivo (e.g., in lung-on-a-chip devices). The potential for multi-organ-on-a-chip platforms to be used as investigative tools for secondary drug toxicity or systemic toxicity are further applications as these systems, which may recapitulate one or more organ functions and in vivo patho/physiological responses in real-time. This adaptability enables investigation of pharmaceutical interventions that are not feasible in isolated 2D or 3D in vitro settings and may mitigate animal model limitations by offering a standardized 3D culture platform that replicates the human cell-microenvironment. Moreover, the compatibility of immunosuppressive medications and the immune system can also be tested, such as instant blood-mediated inflammatory reaction and auto-and allo-immunity (Abadpour et al., 2020).
Figure 3. Techniques used in organ-on-a-chip fabrication. (A) Photolithography: This process involves exposing a light-sensitive photoresist on the substrate to UV light through a photomask, forming a pattern. After development, this pattern serves as a template for subsequent steps, like etching or bonding, enabling the precise creation of microfluidic structures for applications such as lab-on-a-chip devices and biomedical sensors. Figure adapted from Scott and Ali 2021 (Scott and Ali, 2021). (B) Soft Lithography: Microfluidic devices begin with the fabrication of an SU-8 master mold. Polydimethylsiloxane (PDMS) is then cast on the mold, plasma treated, and contact pressure bonded. Figure adapted from Scott and Ali 2021 (Scott and Ali, 2021). (C) Micromilling: A subtractive manufacturing process utilizing rotating cutting tools to precisely remove material from a workpiece, typically consisting of a worktable, cutting tool (commonly an endmill), and overhead spindle; this technique has evolved with modern computer numerical control (CNC) systems for enhanced automation, repeatability, and precision in fabricating microfluidic devices. (D) Stereolithography: A liquid photopolymer resin is selectively cured by exposure to ultraviolet (UV) light in a pattern defined by a computer-aided design (CAD) model. The cured layers gradually stack on top of each other, forming intricate microfluidic structures. (E) Hot Embossing: The process involves heating a thermoplastic material, often a polymer sheet, above its glass transition temperature and pressing it against a mold with the desired microfluidic features. The mold contains a negative relief of the microfluidic pattern. Upon cooling, the material solidifies, retaining the replicated microstructures. (F) Injection Molding: A thermoplastic material is melted and injected into a mold cavity that contains the negative geometry of the desired microfluidic structure. The material solidifies as it cools, and the mold is opened to release the finished microfluidic device.
Organ-on-a-chip platforms offer multiple advantages, including high throughput, low costs, serial arrangement, and integration with other technologies such as electrochemical sensors. Microfluidic systems leverage controlled laminar flow that occurs in the microscale to permit particle separation; controlled mixing; accelerated biochemical reactions; biomarker-identification; drug screening; and real-time imaging. Real time monitoring is also possible due to sensor integration. Microfluidic-systems can be used to control stem cell microenvironment and differentiation - with the potential for large-scale expansion and broader applications. Stem cells have therapeutic potential, due to their ability to differentiate into multiple cell lineages. However, controlling proliferation, maintaining undifferentiated pluripotency, and directing differentiation using traditional in vitro culturing processes such as Tanswells and Petri dishes remains challenging (Zhang et al., 2017). As microfluidic technologies allow precise control over the microenvironment, their use in conjunction with stem cells has the potential to elevate organ-on-a-chip platforms.
In the clinical setting, organs from older or obese donors are often deemed as having ‘marginal’ quality are often not used for transplantation (Ashammakhi et al., 2018). Organ-on-a-chip platforms offer opportunities to support organ function and elongate the time window for transplantation by leveraging their capacity to manipulate and control fluid flow. For example, in the future, donor livers affected by steatosis (i.e., an excessive buildup of fat) could be salvaged using arrays of organ-on-a-chip devices to maintain blood perfusion and use kidney-on-a-chip platforms to filter and remove undesired substances from the organ Ashammakhi et al offer an in-depth discussion on applications of organ-on-chip platforms for organ salvage (Ashammakhi et al., 2018). In the context of skin transplantation and skin grafting, skin-on-a-chip (SoC) technologies offer greater potential to recapitulate the many structural and functional features that are necessary to generate biomimetic skin alternatives that are suitable for successful transplantation.
SoC platforms allow precise control over the microenvironment, recapitulate mechanical cues, and permit the incorporation of sensors, and even skin appendages such as eccrine sweat glands and hair follicles (Sutterby et al., 2020). SoC platforms rely on in vitro devices that have a series of culture chambers and microchannels that mimic the spatial structure of the in vivo tissue, including those that feature in the epidermis, dermis, hypodermis, blood vessels, sweat glands, etc. Several considerations are key in the development of biomimetic SoC platforms, including cell source (i.e., autologous, or allogeneic cells), the scaffold required to mimic the extracellular matrix in a physiologically relevant manner and with mechanical stability, the incorporation of perfused vasculature (Sutterby et al., 2020). Arguably, the first consideration to address is whether the SoC platform will rely on intact tissue samples (Ex vivo SoC) or cell suspensions (bioengineered SoC) to generate the tissue construct.
Ex vivo skin-on-a-chip (SoC) models frequently involve the direct culture of skin tissue into the platform (Risueño et al., 2021). Ex vivo SoC devices often include tissue holding compartments for the tissue explant as well as a network of microchannels for nutrient perfusion (Risueño et al., 2021). O’Neill et al. cultured skin biopsies in an ex vivo SoC to evaluate the effect of nutrient perfusion on tissue viability over time (O’Neill et al., 2008). Their results showed that perfusing culture medium led to superior keratinocyte viability compared to traditional static methods. Kim et al. also cultured skin biopsies in a SoC platform to characterize neutrophil response to infections (i.e., S. aureus) (Kim et al., 2019). They exposed human skin biopsies to Staphylococcus aureus and then cultured them in the SoC platform to monitor neutrophil migration into the skin biopsy. Wagner et al. also used tissue biopsies from skin and liver to establish a skin-liver co-culture for a double organ-on-a-chip model that enables the generation of an air liquid interface in the skin compartment (Wagner et al., 2013). This work was extended by Maschmeyer et al. as a four organ-on-a-chip platform that includes skin, liver, intestine and kidney with spatial-temporal separation of two microfluidic flows by tubule epithelia (Maschmeyer et al., 2015). Whilst there are advantages, such as the ability to use both healthy and diseased human skin, and thus capture the full complexity of native skin and the microenvironment, these models rely on culturing tissue samples obtained from donors, which decreases their potential as systems to generate tissue de novo for skin grafts (Fernandez-Carro et al., 2022). Thus, ex vivo SoC models may provide interesting tools for research, but they will struggle to meet the demand of tissue needed for skin grafts, which has encouraged researchers to explore other techniques to generate de novo skin tissue.
Other SoC technologies rely on cell suspensions and 3D hydrogels in the microfluidic chambers to generate the tissue construct while they use the microfluidic channels for nutrient perfusion and waste product removal (Risueño et al., 2021) via gravity-driven flow, capillary forces, or pump systems to simulate in vivo blood flow (Sun et al., 2023). Using cell suspensions and letting them expand in the SoC offers greater potential to generate a scalable tissue source for skin grafting. Lee et al. used gravity driven flow through a SoC which incorporated microfluidic channels that allow for vascular endothelial cell culture with a chamber for 3D skin cell culture that permits interaction between different tissue compartments, such as the epidermis and dermis (Lee et al., 2017). The authors demonstrated that the device successfully supported cell growth and keratinocyte differentiation into a stratified epithelium, while culture medium flow was essential to maintain fibroblast viability. However, the authors also noted that the flow rate and/or channel dimensions could be optimized to provide fluidic shear stress more similar to in vivo in dermal vasculature. The model also underperformed in terms of consistent epidermal stratification in comparison to their Transwell-based model, potentially due to heterogeneous nutrient and growth factor diffusion through the platform. Although various materials are used as ECM scaffolds, Type I collagen is the most commonly used scaffold for skin constructs (Sun et al., 2023) and Song et al. used a pumpless, gravity driven SoC platform seeded with primary keratinocytes and fibroblasts to establish that rat tail collagen promotes cell differentiation and skin maturation better than collagen derived from either porcine skin or duck feet (Song et al., 2018). This group also developed an iteration of the platform which incorporates both a gravity-driven flow system to control cell culture media flow rates through the microchannel network to ensure efficient transportation of nutrients to dermal and epidermal layers, while also using a shaking protocol to recirculate culture medium. However, despite observing reduced collagen contraction in the dynamic chip and robust proliferation and differentiation, the expression of key proteins, such as fibronectin, collagen IV, and keratin 10, was similar, or poorer, to the Transwell model. The authors thus acknowledge the chip culture conditions require improvement to better recapitulate native skin (Song et al., 2018). Abaci et al. (Abaci et al., 2015) devised a pumpless full thickness skin equivalent with both epidermal and dermal compartments that was based on blood residence times in native skin tissue, and which could be maintained long term in their microfluidic platform. The model permitted the generation of an air-liquid interface and enabled the flow of culture medium at desired rates without external pumps or tubes. This pumpless SoC model that mimics the epidermis and dermis, using a gravity flow system to rotate the device at 15° on both sides, was used by Jeon et al. (Jeon et al., 2020) to test the effects of the drug sorafenib on dermal cells. Kim et al. (Kim et al., 2020) used the platform to investigate effects of Curcuma longa leaf extract of skin formation and maturation. Lim extended this concept by developing a wrinkled SoC using cyclic uniaxial stretching. This was achieved by applying mechanical stimuli to the skin construct using an electromagnet within the platform structure and applying a magnetic field to the tissue to produce skin wrinkling (Lim et al., 2018). Mori et al. used similar techniques to demonstrate that stretching led to thicker, more differentiated epidermal layers (Mori et al., 2017).
Traditionally, many SoC devices have relied upon soft lithography for microdevice fabrication. Materials such as PDMS are inexpensive, gas permeable, optically transparent, and compatible with intricate designs. However, they suffer from lengthy fabrication processes precluding scale-up deformability and leakage under high flow rates (Raj M and Chakraborty, 2020). In recent years, the portfolio of fabrication techniques available for SoC has diversified. Micro-machining techniques (e.g., CNC milling) allow for more flexibility fabrication and broader material selection options, which contributes to overcoming some of the PDMS limitations. Risueno, Valencia et al. generated a micromachined SoC model with a fibrin gel and undifferentiated keratinocyte layer (Risueño et al., 2021). Using a two-chamber vinyl device with parallel flow that allows fluids of different viscosities through a single channel without mixing; this technique enables in situ generation of epidermal and dermal compartments. This represents the first SoC that generated a 3D structure directly within the device channel and the authors argued that this platform could eventually translate to the generation of other complex tissues.
Sriram et al. developed a full thickness skin equivalent with a cellular and matrix architecture that recapitulates human skin (Sriram et al., 2018). The model was constructed using five microstructured poly (methyl methacrylate) (PMMA) layers to avoid some of the chemical and biocompatibility concerns surrounding PDMS and a polycarbonate microporous membrane was used to separate the apical and basal microfluidic compartments. Circumventing some of the issues pertaining to the generation of complex 3D structures using channels, the membrane served as a support structure for the dermal compartment, whilst also permitting nutrients, metabolites, and compounds to diffuse easily. Sriram et al. evaluated multiple design and flow parameters to optimize keratinocyte differentiation into a stratified epithelium. They observed thicker epidermal layer with improved polarized columnar basal keratinocytes, keratohyalin granules in the stratum granulosum, and keratinized stratum corneum. The addition of dynamic perfusion and precise control over the microenvironment enhanced morphogenesis and differentiation along with improving barrier function and reducing permeability. Consequently, their findings represent a considerable improvement over their standard tissue culture insert control and alleviates the concerns surrounding weak dermo-epidermal junctions, which affect many cultured epidermal autografts. The presence of mature dermo-epidermal junctions provides greater opportunities for clinical applications and, as the model uses thermoplastics with a one-step thermal bonding fabrication technique and micromilling of microfluidic features, the model is compatible with injection molding and hot embossing to enable mass scale-up, which is not possible with PDMS models. Nonetheless, the model still requires further optimization of media and air flow and lacks some structures found in native skin, such as appendages (e.g., hair follicles), immune components, and the hypodermis. Altogether, researchers have developed a variety of microfluidic and organ-on-a-chip platforms for skin research (Figure 4). Each of these platforms provide different advantages and present limitations that should be considered when deciding what should the system structure and organization.
Figure 4. Microfluidic Applications for Skin. (A) Pumpless skin on a chip model to mimic the architecture of vascular networks in skin. The model is constructed with a polydimethylsiloxane (PDMS) main frame, featuring two layers covered by polystyrene sheets. The bottom PDMS layer incorporates microfluidic channels, a two-reservoir chamber, and a section for cultured skin tissue. The microchannels prevent occlusion and facilitate even media distribution. The skin chip model maintains tissue viability without expensive growth factors under a renewing fed-batch perfusion flow regimen (Mohamadali et al., 2023). (B) Wrinkled skin on a chip model (WSOC) relies on PDMS-made well with two horizontal compartments separated by a porous membrane and an electromagnet. Human fibroblasts are embedded in a collagen layer within a cell chamber. The stratum corneum of the epidermis is formed by spraying human keratinocytes onto the collagen layer containing fibroblasts. After 4 days, the cells are exposed to air for differentiation, and a uniaxial stretch is applied to the cell-containing gel using an electromagnet, thereby stretching the model to create wrinkles. The model incorporates a perfusion mechanism to support fibroblast viability and functionality. This model can be used for studying skin tissue dynamics and responses under controlled mechanical stimuli (Lim et al., 2018). (C) Microfluidic model of an Innervated epidermal layer. PDMS-based four-channel microdevice comprising a chamber with three-dimensional nerve cells and an epidermal keratinocyte layer. This well-established technology uses pillars between the different channels to generate an air-liquid interfacing culture and spatial compartmentalization. This technology is ideally suited for studying cell-cell interactions and real-time microscopy inspection (Ahn et al., 2023a). (D) Gravity flow microfluidic skin model. This model comprises a structure with two PDMS layers assembled on a glass base. The bottom layer includes fluidic channels and a vascular cell chamber, while the top layer features a chamber for the dermis and epidermis. A gravity-induced flow system, controlled by a computer-controlled motor stage, regulates the flow rate, primarily through tilting adjustments. A porous membrane sheet between layers supports the 3D dermis matrix, with polycarbonate proving more stable than polyester (Lee et al., 2017).
The majority of the bioengineered SoC models discussed focused on establishing a dermal-epidermal structure, while the hypodermis has remained largely neglected. Thus, interest in recapitulating the trilayer composition of native skin (i.e., epidermis, dermis, and hypodermis) has prompted researchers to explore alternative tissue engineering techniques to develop skin surrogates that include a hypodermal layer and provide better suited skin constructs for grafting. Constructs which incorporate a hypodermal layer may be of particular relevance for patients where the subcutaneous layer has been lost due to deep wounds or extensive burns. Several models include a basement membrane as an additional layer.
In vivo, the basement membrane is mostly composed of type IV and VII collagens and laminin and sits at the epidermal-dermal junction to provide mechanical support for keratinocytes located at the bottom of the epidermis (Balavigneswaran et al., 2023a). The literature describes models that make use of biomimetic basement membrane substitutes, such as Huang et al trilayered nanofibrous scaffold and Lin et al. trilayered chitosan-based scaffold with nanofibers, yet these do not replicate the subcutaneous layer of the hypodermis (Lin et al., 2015; Huang et al., 2019). Conversely, Haldar et al. engineered a ‘smart’ regenerative trilayer skin substitute that featured a biodegradable polymer scaffold-based to foster growth and maturation of different cell types needed for optimal deep wound healing (Haldar et al., 2019). Single and bilayer substitutes frequently lack the range of physical and mechanical properties required for full-thickness wound healing and to minimize scarring. To address this concern, Haldar devised a model that incorporated a range of fabrication techniques to recapitulate the varied architecture, mechanical, and physical attributes of the epidermis, dermis, and hypodermis (Haldar et al., 2019). To mimic the microarchitecture of native skin, different techniques were used to fabricate the upper two layers. The epidermal layer consisted of polycaprolactone (PCL) cast in a mold, casting results in a compact, minimally porous structure with appropriate barrier function. The dermal layer was also fabricated using PCL that was electrospun. This layer simulated the structure of the dermis as electrospinning leads to nanofibrous structures with low individual tensile strength. Thus, despite being constructed of similar materials, using alternative fabrication techniques allowed the authors to capture important differences in structural morphology found in vivo across the skin layers. This approach ensured that keratinocytes and fibroblasts were restricted to epidermal and dermal layers that had similar mechanical properties than their in vivo counterparts. The hypodermal layer was fabricated using lyophilized gelatin, which facilitates attachment to the patient’s wound bed and promotes integration with host tissue after grafting. Overall, this configuration simulated the physiological tensile strength and water permeability necessary for robust barrier function. The resulting construct promoted wound closing in all the construct layers without an inflammatory response. Further studies should consider enriching the hypodermal layer construct with additional cellular components, such as adipocytes.
Other studies have explored the use of stromal or stem cells to generate hypodermis-like layers that would be later combined with the dermal and epidermal components. Vermette et al. (2007) produced an autologous engineered adipose hypodermis substitute using human stromal cells extracted from lipoaspirated or resected fat (VERMETTE et al., 2007). Trottier et al. reported the use of adipose-derived stem/stromal cells for inclusion in engineered skin and highlighted the greater availability of adipose tissue in comparison to the dermis as a cell source for rapid production of autologous skin substitutes for severely burned patients (Trottier et al., 2008). Building on this earlier work, Monfort et al. developed a human tissue-engineered skin that included a blood plasma-based hypodermis and determined that the inclusion of a subcutaneous layer enhanced epidermal differentiation and keratinocyte maturation inducing a pluri-stratified epithelium (Monfort et al., 2013). Overall, few models include all three layers of human skin, but the emergence of new biological and engineering techniques is helping to tackle this limitation.
Vascularization poses a critical challenge for lab-grown tissues since sustainable supply of oxygen and nutrients to the cells is essential to ensure permanent engraftment and integration with host tissue (Baltazar et al., 2020). Nutrient and oxygen diffusion often limits cell survival to the first 200 or 300 µm in human tissue, while distances >300 µm result in oxygen and nutrient starvation, which eventually can lead to necrosis (Salameh et al., 2021). Cutaneous vasculature is also involved in numerous pathologies, including melanoma metastasis, inflammatory diseases, tumor growth and wound healing; thus, including vasculature in microfluidic skin models not only enhances their translational value but increases their potential as a viable option for grafting (Zoio et al., 2022).
Microfluidic devices have shown significant progress in modeling vasculature and angiogenesis, offering advantages over conventional 2D cultures. The development of a physiological microvasculature involves various processes at both the cellular and molecular levels, including the recruitment of mural cells, basement membrane development, organ-specific differentiation, expression of adhesive molecules, and intercellular junction formation (Bergers and Song, 2005). Factors such as VEGF and angiopoietin play crucial roles in vessel generation and maturation (Jeon et al., 2014). While simplified 2D systems have provided insights into certain aspects of vascular biology, the presence of a 3D microenvironment is essential for generating fully perfused physiologically relevant tissue constructs (Clevers, 2016).
Various vascularized microfluidic models have been developed to investigate vascular biology, including angiogenesis, vasculogenesis, or anastomosis. These models range from microchannels line with endothelial cells to advanced systems that generate in vivo-like, fully perfusable microvasculature (Hu et al., 2019). They have demonstrated the generation of branching microvascular networks and have explored factors influencing vessel stability, diameter, and permeability (Amstad, 2017). Notably, some microfluidic models have successfully co-cultured endothelial cells with other cell types, such as fibroblasts or mesenchymal stem cells, to study heterotypic interactions and their impact on network development, maturation, and functionality (Brassard-Jollive et al., 2020). As discussed, integration of vasculature in tissue constructs is vital due to microvascular circulation’s role in maintaining tissue homeostasis and providing nutrients to tissue-resident cells. Recently, Herland et al. developed an eight-organ-on-a-chip (BBB, brain, skin, lung, heart, liver, intestine, and kidney) system with automated culture, perfusion, and control, utilizing a universal blood-like medium for the vascular compartment and specific mediums for individual organs (Herland et al., 2020). Zhang et al. has furthered this vascularized network technology by developing PDMS tubes that mimic diverse blood vessel types. These tubes were lined with endothelial cells and coupled to organ-on-a-chip platforms, revealing responsive endothelium formation upon exposure to drugs (Zhang et al., 2016). These advanced models hold promise for studying angiogenesis and other complex processes, such as cancer cell extravasation, in a more physiologically relevant context.
Wufuer et al. generated a SoC with epidermal, dermal and blood vessel components where each layer is separated by porous membranes that permitted interlayer communication (Wufuer et al., 2016). However, their model relied upon 2D porous membranes and critics argue that the presence of these membranes decreases the translatability of the model and will negatively impact its grafting potential (Sun et al., 2023). Nevertheless, this model represents an early attempt to create a vascular layer and the membranes facilitate interlayer communication that is observed in vivo. Marino et al. highlighted the possibilities of advancing engineered tissue constructs by generating a skin graft not only with patient cells, but which included ex vivo ‘pre vascularized’ lymph and blood capillaries that demonstrated branching post-transplantation into immunocompromised rodent wound models (Marino et al., 2014). Consequently, two broad strategies to address the lack of vasculature in engineered skin equivalents have been recently employed: the first approach involves the addition of growth factors, reactive oxygen-species-inducing nanoparticles, and stem cells with the goal of promoting angiogenesis in the host tissue toward the graft, and the second is the prevascularization of skin constructs for grafting, such as the inclusion of vessel-forming cells into scaffolds (Amirsadeghi et al., 2020).
Grober et al. developed a biological vascularized scaffold (BioVaSC) made from decellularized porcine jejunum that was cut and placed in a frame (Groeber et al., 2016). The construct was seeded with human fibroblasts, keratinocytes, and microvascular endothelial cells. This produced a skin equivalent with a perfused vascular system with stratified epidermal and dermal layers, along with a papillary-like architecture at the dermal-epidermal-junction. Although this technique has interesting potential, even for other tissues, the reliance on porcine jejunum raises some concerns about scalability of the approach (Linke et al., 2007).
Controlling medium or blood perfusion through the SoC vasculature would be a valuable tool to maintain these tissue constructs alive for prolonged periods of time (Salameh et al., 2021). Mori et al. generated a skin equivalent with perfusable vascular channels lined with endothelial cells connected to an external peristaltic pump and tubes, resulting in the development of tight junctions on the vascular channel wall (Mori et al., 2017). Salameh advanced this method to develop a fully vascularized, perfusable skin equivalent that including three endothelial cell-lined perfusable channels that formed angiogenic sprouts, and a layer of human umbilical vein endothelial cells (HUVECs) sandwiched between two fibroblast layers to form a network of capillaries organized by vasculogenesis in the dermal component (Salameh et al., 2021). This HUVEC microvascular network was then able to branch out and connect with the angiogenic sprouts to form a sophisticated model that was used to test skin permeability. Blood vessels under perfusion exhibited improved barrier function coefficients in comparison to static models with an absence of perfusion, thus demonstrating the importance of these factors in the development of accurate skin models. However, the permeability coefficient observed in these perfused vessels was still higher than that observed in porcine skin, which is typically the benchmark for testing chemical permeation (Rama Varma and Fathi, 2023). Salameh’s microvascularized platform enhanced substance delivery such as drugs and environmental pollutants via the circulatory system (Salameh et al., 2021). However, the authors acknowledge that their models display several limitations, such as the use of HUVECs isolated from large vessels rather than human dermal microvascular endothelial cells, the lack of arteries, veins, and lymphatic vessels.
Jones et al. developed a novel microvascularized SoC by integrating human cells in a full thickness skin surrogate within a microfluidic device with a previously established microvascular network (Jones et al., 2022). The inclusion of HUVEC-pericyte co-cultures represents an additional layer complexity in this model and it was determined that microvasculature integrity and barrier function was improved when pericytes are present. This model also displayed enhanced stratification and differentiation, including structure, thickness, and expression of terminal differentiation markers, such as involucrin and transglutaminase 1. Taken together, these findings indicate that vascularization plays a key role in the generation of more mature skin constructs within SoC platforms. Whilst Jones et al. did use an orbital shaker to encourage medium flow, they did not observe additional maturation of their constructs. Conversely, Rimal et al. reported an improved vascularized skin equivalent that utilized a dynamic flow culture system and a 3D bioreactor; this technique offered an improvement over static environments for intricate in vivo platforms and that dynamic flow aided wound healing, restored homeostasis, and facilitated superior skin barrier factors, epidermal differentiation, and modulation of the ECM (Rimal et al., 2021).
Zimoch et al. also bioengineered a sophisticated tri-layered human skin surrogate that contained a hypodermis and a prevascularized dermal layer which displayed neovascularization when transplanted into a host animal and resulted in rapid in vivo perfusion (Zimoch et al., 2021). Such models could potentially alleviate concerns surrounding integration and survival post-transplant; however, whether models that promote angiogenesis can attain the magnitude necessary for robust engraftment as a permanent therapeutic intervention remains the focal issue in the development of skin equivalents for clinical use (Phua et al., 2021).
Most of the prevascularized platforms discussed rely on endothelial cells self-assembling into functional blood vessels. However, the user has little control regarding the structure and properties of those vessels (e.g., network geometry). The inclusion of more sophisticated techniques, such as bioprinting, to generate vasculature networks with specific geometrical designs could enable the development of more complex, intricate vascular architectures.
Advances in microfluidic technology and their integration with human induced pluripotent stem cells (hiPSCs) also provides a path towards personalized screening protocols prior to organ transplantation. The vascular system relies on the coordinated functioning of various cellular components, including endothelial cells (ECs), pericytes, and vascular smooth muscle cells (VSMCs) (Cochrane et al., 2019). ECs form a selective barrier for nutrient and oxygen delivery, while pericytes and VSMCs play crucial roles in stabilizing EC tubes and regulating vascular tone (Cochrane et al., 2019). hiPSCs can be differentiated into ECs, pericytes, and VSMCs, providing a renewable and ethically acceptable source of these cells (Cochrane et al., 2019). Patient-specific hiPSC-derived vascular cells have been used for disease modeling and studying disease mechanisms (Peelen et al., 2021). In theory, a vasculature model could be assembled using host hiPSCs in a SoC device to generate autologous tissue for skin grafting, which would prevent risk of graft rejection and increase graft survival.
The majority of current skin surrogates lack an immune component, such as Langerhans cells, dendritic cells, and macrophages that contribute towards homeostasis and combating disease; the addition of these cells may help decrease post grafting infection and improve graft integration and tissue homeostasis (Hong et al., 2023).
Ramadan and Ting (2016), used an immune competent 3D SoC cell culture system with dynamic media perfusion that mimics the human skin microenvironment, improved tight junction formation, and extended cell viability (Ramadan and Ting, 2016). This model included two channels with a porous membrane between and the authors seeded immortalized human keratinocytes to form an epidermal-like monolayer on the membrane. The system included dynamic media perfusion that exposed the culture to shear stress, but which also promoted healthy barrier function due to continuous nutrient delivery and cellular waste removal, Following inoculation of a human leukemic monocyte lymphoma (U937) cell line in the lower channel to represent dendritic immune cells, keratinocytes were stimulated via application of lipopolysaccharides, nickel sulfate, and ultraviolet (UV) irradiation to test response to external stimuli and to determine posterior cytokine release. The authors used transepithelial resistance (TEER) as an epidermal barrier integrity measure Additionally, shear stress induced by dynamic media perfusion through the microfluidic system enhanced keratinocyte tight junction formation compared to static conditions. Although this model provided a relevant example on how to integrate immune cells in SoC platforms, transmigration of leukocytes could not be observed due to the lack of a dermal compartment and a vascular network (Sutterby et al., 2020). Moreover, the authors concluded that the reliance on immortalized cell lines may have affected the findings as these cells may exhibit different behaviors when compared to primary cell cultures. They also point out that in vitro skin models are more permeable that native skin, which can lead to over predictions of skin sensitivity due to higher penetration of topical substances. In this context, studies that include comparisons of in vitro and in vivo data are necessary.
To address some of these limitations, Kwak et al. developed a microfluidic SoC with vasculature to mimic the immune response in a more physiologically relevant manner (Kwak et al., 2020). The authors co-cultured fibroblasts and keratinocytes with vascular endothelial cells to create a model with an epidermis, dermis, and endothelium. The authors included leukocytes in circulating media as the vascular endothelium is involved in leukocyte migration towards areas of inflammation. Exposing the model to ultraviolet irradiation gave rise to an immune response and increased neutrophil migration and cytokine secretion in comparison to controls.
Skin is exposed to a vast array of microorganisms including bacteria, fungi, and viral components. While some of them live symbiotically on human skin (they constitute the microbiome) others may cause severe tissue damage (e.g., infections such as necrotizing fasciitis may lead to life-threatening situations) (Fernandez-Carro et al., 2022). Dysbiosis, an imbalance in the microbiome, is believed to underlie numerous skin pathologies along with the systemic immune response involved in chronic inflammation (Park and Lee, 2017); therefore, elucidating mediators involved in local changes between microorganisms and the skin barrier is a key area, which most current models fail to address (Byrd et al., 2018). There have been some attempts to include skin microbiota: Holland et al. explored microbial colonization of a Transwell-based engineered tissue equivalent (Holland et al., 2008); Shepherd et al. and Haisma et al. looked at wound healing and S. aureus - a pathogenic skin colonizer (Shepherd et al., 2009; Haisma et al., 2014); and Bojar et al. used a commercial product, Labskin, to examine the microbiome ecosystem (Bojar, 2015). However, such models tend to lack the broad range of microorganisms found in vivo which could provide a more complete picture of dysbiosis-related skin pathophysiology (Fernandez-Carro et al., 2022).
Melanocytes are responsible for melanogenesis, the production of melanin pigments, that determines the color phenotype of the skin. Melanocytes are not routinely added to engineered skin constructs, despite conferring both protection against ultraviolet (UV) radiation and enhancing the aesthetic properties of grafted skin surrogates, which can profoundly reduce patient distress (Dai et al., 2018). Constructs which lack melanocytes take on a vitiligo-like appearance (i.e., lack of pigmentation results on pink-colored skin); consequently, as the first study to investigate whether the inclusion of autologous melanocytes within engineered skin can produce tunable pigmentation, Böttcher-Haberzeth et al. generated a range of dermo-epidermal skin surrogates that included melanocytes with the aim of matching the color of the presumptive transplant site as far as possible (Biedermann et al., 2015). This was achieved by obtaining a range of human foreskin samples with light and dark pigmentation types from which they isolated keratinocytes, dermal fibroblasts, and melanocytes. The fibroblasts were combined with collagen and placed in cell culture inserts and then keratinocytes and melanocytes were seeded on top of the dermal and were later transplanted onto full thickness wounds on rats to determine whether the original skin color of a patient can be replicated. The authors explored the generation of light and dark pigmentation by changing the ratios of keratinocytes, fibroblasts, and melanocytes, in the platform. Their results indicated that skin construct matching could be successfully quantified subjectively, but also objectively using chromameter evaluation. A key point is that all 3 cell types are fundamental in producing the desired results as there are considerable cell-cell and cell-secreted factor interactions that are tightly involved in regulating phenotype and function. Moreover, using all 3 cell types from the same donor is important due to their interactions with one another to regulate the skin phenotype. Using keratinocytes and melanocytes, but fibroblasts from an alternative donor, led to different pigmentation types and suggests that autologous cell populations are preferred for clinical use. Whilst this is undoubtably useful knowledge, the study did not provide longitudinal data as it is possible that hypo and/or hyperpigmentation may arise over time due to melanocyte instability. Moreover, the authors did not address whether melanocyte presence impacted UV protection or whether this resulted in increased risk of malignant melanoma. However, Boyce et al. determined in a subsequent study that dermo-epidermal skin constructs seeded with melanocytes not only restored pigmentation, but also conferred UV photoprotection (Boyce et al., 2017). Supp et al. also concluded that skin constructs that included both light skin-derived and dark skin-derived human melanocytes exhibited similar mean cell density at 8 weeks post-transplant and also shielded against UV-induced DNA damage (Supp et al., 2020). Consequently, the risk of skin cancer in graft recipients may be reduced if melanocytes are integrated into engineered skin surrogates.
A further limitation of many SoC platforms is the absence of appendages such as eccrine and apocrine sweat glands, sensory neurons, and hair follicles; these appendages arise from a layer of multipotent progenitor cells during skin morphogenesis and are intrinsically involved with the regulation of body temperature and fluid retention, along with touch and pain sensation (Wang et al., 2016; Lee et al., 2020).
Hair follicles are located in the dermis, consist of hair papillae, hair matrix, root sheath and hair bulges, and contribute towards sensory function, display antibacterial properties, and inhibit scarring (Weng et al., 2020). To this end and as an early iteration, Ataç et al. developed a dynamically perfused SoC platform that permitted extended culture of hair follicles, thus demonstrating the importance of medium perfusion to maintain dermal cells and structures in vitro (Ataç et al., 2013). Unfortunately, culturing human hair papillae in vitro is extremely challenging and relies upon the internal microenvironment to control gene expression which typically declines in culture along with hair follicle induction capacity. There have been some attempts to resolve the issue; for instance, Abaci et al. made use of 3D printing to microfabricate plastic molds that could be used to simulate the 3D microenvironment of human hair papillae and successfully formed vascularized follicles (Abaci et al., 2018). Augustine also used 3D bioprinting as a scaffold for mouse fibroblasts and epidermal cells and did observe in vitro hair follicle regeneration (Augustine, 2018); however human and mouse hair follicles differ, and human hair follicles have proven more challenging to regenerate (Weng et al., 2020).
Sebaceous glands secrete sebum, lubricate the skin, and, as a component of the integumentary system, play a substantial role in the formation of the skin’s acid mantle which serves as a protective barrier against bacteria and viruses (Weng et al., 2020). Sebaceous glands are generally observed between the hair follicle and arrector pili muscle and are formed in association with hair follicles during morphogenesis to make up the pilosebaceous unit (Wang et al., 2016; Hosseini et al., 2022). Wang et al. investigated whether epidermal stem cells (Epi-SCs) and skin-derived precursors (SKPs) could offer an appropriate source for generating bioengineered skin substitutes with hair follicles and sebaceous glands (Wang et al., 2016). Interestingly, the authors isolated Epi-SCs from adult human foreskin, glabrous tissue without hair follicles, which expressed typical markers of epidermal cells, CD29, CD49f, K15, and K19. These were labeled with BrdU and transplanted, along with murine SKPs, topically or through subcutaneous injection to excisional wounds. Hair growth was detectable 15 days post-transplant and histological analysis revealed the formation of de novo hair follicles and sebaceous glands. Furthermore, numerous cells expressed Ki67, indicative of active proliferation, and human Epi-SCs were present in the epidermal basal layer, which suggests that these cells had formed renewable structures. Culturing neonatal mouse Epi-SCs with dexamethasone/insulin/rosiglitazone/XAV939 (DIRX) induced differentiation into sebocytes, which were positive for Lrig1, a sebocyte marker, after 3 days. In vivo, the transplantation of Epi-SCs and SKPs generated sebaceous glands with hair follicles, whereas Epi-SCs and fibroblasts gave rise to a limited number of sebaceous gland-like structures without hair follicles. As epidermal stem cells are readily available, the authors concluded that these offer an attractive candidate for the generation of appendages within engineered skin. Conversely, the isolation, purification, and expansion of stem cells derived from appendages can be challenging and these cells can display in vitro senescence; moreover, reprogramming to generate skin progenitors can lead to appendages that are not identical to those found in native skin and, thus, further investigation is necessary to elucidate the optimal biochemical environment (Hosseini et al., 2022).
Eccrine sweat glands maintain homeostatic body temperature and protect against bacterial and viral agents (Li et al., 2013). They are tubular structures that cross the dermis and epidermis are open to the skin surface and thermoregulatory function is compromised in patients with serious injuries, such as extensive burns; however, they are absent in the majority of skin constructs. Li et al. harvested human eccrine sweat glands and cultured them in 3D Matrigel matrices (Li et al., 2013). In Matrigel, epithelial cells did produce tubular-like and coiled spherical structures that resembled in vivo eccrine glands. Histology confirmed the presence of a central lumen within each tubular structure with either single layers of epithelial cells or stratified epithelium which are observed in the secretory and duct regions, respectively. However, despite these interesting results, Matrigel is significantly softer (100 pa-3 kPa) than many skin tissues (0.00109 MPa–169.1 MPa) and the viscoelastic properties are potentially problematic at room temperature, which limits clinical translation (Hosseini et al., 2022). Additionally, Matrigel contains xenogeneic proteins and other factors which may interact with the immune system, which raises concerns regarding therapeutic use (Hosseini et al., 2022). Collagen type I matrices are widely used and are less expensive than Matrigel. For instance, Huang et al. investigated wither sweat glands could be incorporated into tissue constructs (Huang et al., 2010). The authors cultured sweat glands on gelatin microspheres containing epidermal growth factor (EGF) and integrated them into their model which was composed of a fibroblast and collagen-based matrix with a layer of human keratinocytes on top. The construct was then transplanted onto full thickness murine wounds. The sweat gland-microsphere complexes differentiated into a sweat gland-like structure and promoted wound healing, which indicates that it may be feasible to include sweat glands in engineered tissue constructs; however, collagen is also frequently derived from animal tissues and has similar concerns regarding antigenicity.
The skin is innervated with an array of nerve endings which permit discrimination between temperature, pain, pressure, or touch (Blais et al., 2013). However, these become damaged following deep wounds and lead to neuropathy (Laverdet et al., 2017). Some, albeit imperfect, recovery of cutaneous nerve function occurs as new nerve fibers migrate from the wound bed or sprout from adjacent healthy regions, but many patients experience altered sensations, sensory deficits, and lasting pain (Laverdet et al., 2017). The risk of these symptoms is exacerbated in those who have received skin grafts (Blais et al., 2013). Post-graft persistent itching, acute or chronic pain, and compromised tactile perception are side-effects that have deleterious effects on quality of life; these debilitating phenomena are associated with aberrant or insufficient sensory nerve fiber regeneration and an absence of sensory units, including hair follicles and sensory corpuscles, after the skin has become damaged and some level healing has occurred (Girard et al., 2017).
Neuronal subtypes innervate cutaneous layers and anatomical location is highly specific. Heavily myelinated Aβ fibers innervate the dermis and carry information related to touch. Thinly myelinated Aδ fibers that detect cold, pressure and acute pain innervate both dermal and epidermal layers. They respond to fast pain that is located to one specific area of the body and can initiate the withdrawal reflex, for instance, when touching a hot surface (Ahn et al., 2023b). Unmyelinated C-fibers also innervate the epidermal and dermal layers and detect touch, temperature and pain. Rather than responding to acute pain, C-fibers react relatively slowly and are associated with lasting, diffuse pain within the body (Ahn et al., 2023b). Perhaps unsurprisingly, the epidermis is fundamental to sensory function, with keratinocytes and Merkel cells playing pivotal roles (Martorina et al., 2017; Ahn et al., 2023b). Many epidermal cells express neuropeptides, sensorial proteins, and receptors that interact with sensory nerves and influence axon growth and nerve fiber density and morphology (Ahn et al., 2023b). Additionally, free nerve endings are widely distributed within the skin and surround hair follicles to detect temperature, mechanical stimuli and dangerous stimuli (Weng et al., 2020); free nerve endings of C-fibers are known to be located close to epidermal keratinocytes and provide the basis for synaptic-like junctions (Ahn et al., 2023b).
Biedermann et al. generated dermo-epidermal skin constructs using a Transwell system with human dermal fibroblasts and collagen type and either keratinocytes, or keratinocytes plus melanocytes, or sweat glands, which were subsequently transplanted onto full thickness lesions on rats (Biedermann et al., 2013). The data indicated that host-sponsored sensory innervation was evident within 6–8 weeks post-transplantation; however, nerve fibers were only observed within the dermal, and not the epidermal compartment and the composition of the epidermal layer did not influence nerve fiber growth dynamics. This suggests that the host nerve fibers did not exhibit preferential ingrowth towards particular cell types within the epidermis. Whilst the study is an early example of the potential for skin constructs to either attract neuronal innervation or passively facilitate host ingrowth into the graft within 8 weeks, it was not possible for the authors to draw meaningful conclusions regarding quantitative skin sensibility in terms of threshold determination or qualitative functionality, such as thermal or mechanical nociception.
Graft-site neuroregeneration is essential for restoring sensory function and stem cell differentiation and healthy axon extension are known to aid skin nerve repair (Przekora, 2020; Weng et al., 2020). Blais et al. developed a skin construct composed of human keratinocytes seeded on a collagen sponge with fibroblasts to determine whether post-transplant innervation was feasible (Blais et al., 2013). They also seeded dorsal root ganglia-derived mouse sensory neurons at the bottom of the construct to investigate whether collagen permits axonal migration. Following transplant in an immunodeficient murine model, the authors detected migration after 2 months and Schwann cells, which formed a longitudinally aligned band of Büngner-like structure to guide axonal migration. The authors also concluded that laminin, which can also be secreted by Schwann cells, plays a pivotal role in enhancing peripheral nerve cell regeneration.
Adding large molecules, such as laminin, to a carefully selected ECM offers a straightforward approach to aid axonal migration and, therefore, more general nerve regeneration (Blais et al., 2013). However, this strategy does not enable specifically guided regeneration. Nonetheless, despite the advantages of including a range of cells into engineered tissues to increase innervation, the lack of availability of Schwann cells, which are instrumental in axon myelination and stabilization, remains a significant limitation as these must be obtained from peripheral nerve biopsy which carries significant risk to the donor (Blais et al., 2013). Whilst pre-seeding skin constructs with adult stem or Schwann cells has a strong rationale, attempts have been limited. Wang et al. seeded iPSC-derived neural crest cells into tubular scaffolds to generate tissue engineered nerve conduits (Wang et al., 2015). The authors observed that the stem cells were able to differentiate into Schwann cells and promoted axon myelination along with accelerated post-transplantation regeneration of sciatic nerves–thus suggesting the possibility of translation to the clinic. Unfortunately, whilst this approach can help restore pain and temperature perception, deficits in touch cannot be alleviated in this manner; to tackle this problem, the inclusion of tactile sensors and hair follicles is necessary, but it remains a significant challenge in engineering skin surrogates (Blais et al., 2013).
A further difficulty in restoring nerve function are the issues surrounding adequate vascularization in skin grafts (Przekora, 2020). Successful neuro-regeneration appears to depend heavily on there being a robust vascular network at the grafting location as angiogenesis not only supplies nutrients and neurotrophic factors to nerve cells, but also prevents excessive and detrimental fibroblast proliferation after tissue damage (Przekora, 2020).
Establishment of fully innervated skin constructs with functional neural networks has proven elusive and much research has focused upon neurite growth and interactions with various cell types rather than functionality (Martorina et al., 2017). For instance, Gingras et al. investigated neurite outgrowth and the influence of epidermal and endothelial cells of peripheral nerve regeneration and Cadau et al. used the same model to examine the effects of glycation, which crosslinks ECM reducing sugars and proteins, on engineered skin constructs and on capillary and sensory nerve-like networks (Gingras et al., 2003; Cadau et al., 2015). Conversely, Martorina et al. reported that they successfully recapitulated sensory function in response to an external stimulus in their sponge-based, co-culture engineered skin tissue (Martorina et al., 2017). The data demonstrated that the construct sensed a topical application of capsaicin and propagated calcium waves through the neuronal network; however, although the model exhibited an appropriately differentiated epidermal equivalent, the distribution of intra-epidermal free nerve endings and axon patterning does not resemble that present in native skin (Ahn et al., 2023b).
Ahn et al. developed a microfluidic platform to analyze 3D interactions between keratinocytes and sensory neurons (Ahn et al., 2023b). They used a slope-air liquid interface to induce improved epidermal differentiation and barrier function compared to planar culturing techniques and developed a hydrogel-based system with multiple channels to mimic cellular and subcellular compartmentalization along with cell-cell and cell-ECM interactions. The device contained four channels, including a soma compartment with neurons and an epidermal channel for keratinocytes that were connected by two axon-guiding microchannels. The model did enable innervation of the epidermal keratinocyte layer and spatial distribution of sensory neurons and intra-epidermal free nerve endings in the acellular dermal ECM and epidermal-like layers, respectively, resembled the physiological patterning observed in human skin. Nonetheless, there were limitations, such as the use of rodent sensory neurons, due to difficulties in obtaining primary adult human sensory neurons. Human iPSCs or hiNSC-derived sensory neurons may remedy the translational challenges arising from interspecies differences, although these cell types were not used due to concerns surrounding inconsistencies in function in comparison to human or rodent cells. Despite the advantages of the model, there is still a lack of integration of sensory appendages, innervation, and vasculature in current engineered skin models.
Ultimately, there is a need for innovative models and potentially transplantable grafts that are an improvement over traditional models; SoC and 3D bioprinting technologies are feasible advances that may bridge the translational gap from laboratory to clinical applications (Baltazar et al., 2020).
Electrospraying and electrospinning are techniques that draw a jet of polymer solution through high voltage electric fields to produce either droplets or ultrafine nanofibers with high spatial resolution. These polymers may be natural, synthetic or polymeric composites such as collagen, poly (glycolic acid) (PGA), or a combination of both natural and synthetic materials, for instance gelatin and poly (caprolactone) (PCL) to overcome limitations in mechanical properties and biocompatibility that may be observed in single-component polymer types (Qian et al., 2014). Electrospinning offers the advantage of generating fibers with an ECM-like structure, including the fibrillar morphology of native skin (Hernández-Rangel and Martin-Martinez, 2021), and the range of compatible polymeric materials permits the generation of scaffolds with a wide variety of attributes (i.e., porosity, stiffness), which contribute to cell proliferation and migration (Lanno et al., 2020).
However, although these techniques show promise in tissue engineering incorporation of cells can be challenging due to small pore sizes and difficulties in seeding electrospun scaffolds. Building upon these techniques, bio-electrospraying and cell-electrospinning benefit from the direct addition of living cells or growth factors via microencapsulation to generate scaffolds that for a variety of applications in wound healing (Gao et al., 2021a; Chen et al., 2021; Luo et al., 2021).
Developed in the mid-2000s, bio-electrospraying (Gaskell, 1997; Jayasinghe et al., 2011; Wilm, 2011; Konermann et al., 2013; Parhizkar et al., 2017) has been posited as an alternative technology to produce de novo 3D structures minimizing shear stress due to the large inner bore needles used (Jayarajan et al., 2024). This technique involves a hollow needle to which a high voltage is applied and a grounding element below the exit point of the needle. As cells suspended in a pre-polymer compound flow through the needle, the suspension becomes charged and, upon exit, is transformed into a spray of cell-containing droplets. In an early study (Jayasinghe et al., 2011), used primary mouse lung fibroblasts that were biosprayed using a Matrigel biopolymer to produce matrices which were implanted subcutaneously. The authors did not identify any aberrant cellular behaviors in the biosprayed matrices compared to controls and noted that the ability for migration and microenvironment remodeling was not compromised. Moreover, in vitro findings did not induce adverse effects in the murine model and the bio-electrospray protocol did not impair engraftment in living tissue. The authors concluded that this technique is compatible with gene therapy delivery and holds promise for the repair, replacement, or regeneration of damaged tissue.
Jayarajan et al. used bio-electrospraying to generate 3D organotypic skin cultures by seeding a human fibroblast-laden hydrogel into a 6-well plate with six grounding electrodes and then biospraying keratinocytes as a second layer (Jayarajan et al., 2024). The authors initially determined that the epidermal layer was not uniform and that the impact of spraying larger keratinocyte droplets had produced troughs in the hydrogel. This problem was addressed by incubating the fibroblast layer for 72 h to increase stiffness, and also by reducing the cell density within the keratinocyte droplets to generate a uniform layer. Although the study did not aim to generate stratified epidermal layers, the results demonstrate the potential for using bio-spraying as a technique compatible with fibroblasts and keratinocytes in collagen and media, respectively. The authors indicate that this approach will subsequently permit the recapitulation of full-thickness skin tissues, particularly with the addition of modified hydrogels.
Cell electrospinning is a high-resolution technique that relies on expelling a stream of electrically charged, liquid biomaterial that form continuous, randomly oriented fibers (<50 nm) as they exit the apex of the cone (Gaskell, 1997). The technology relies on a nozzle tip that is connected to a high voltage direct- or alternating current, a flow controller, and a capture device (Keirouz et al., 2023). Cell electrospinning represents an advancement on regular electrospinning, since it allows the user to include live cells in the fibrous ECM. Moreover, cell electrospinning achieves a more uniform distribution of cells in comparison to traditional electrospinning as it negates the need for post-fabrication cell seeding (Keirouz et al., 2023; Nosoudi et al., 2023). Demonstrating the feasibility of the technique, Townsend-Nicholson and Jayasinghe (2006) first embedded astrocytoma cells in electrospun fibers and, following refinement of the technique, the resulting cell-laden scaffold can then be used for an array of tissue engineering applications (Eddaoudi et al., 2010). However, various parameters, including electric field intensity, the nozzle tip and capture device distance from one another, bioink concentration, viscosity, and speed of propulsion, and temperature and humidity of the environment have a fundamental impact on scaffold morphology (Si et al., 2023).
A key advantage of this method is the ability to generate cell-laden scaffolds with small and larger cell numbers, the capacity to capture complex spatial architecture in the z-axes, and the ability to cross-stitch the architecture to control the level of stiffness to withstand internal and external forces following transplantation; for instance, Ehler et al. developed a cardiac patch which demonstrated the potential to function under mechanical and biological stress (Ehler and Jayasinghe, 2014). However, there are several limitations, including difficulties in controlling cell density. Moreover, fibers placement accuracy may be reduced by the whipping phenomenon that arises during fiber generation as a result of instabilities in the expelled jet of cell suspension (Nosoudi et al., 2023; Si et al., 2023).
Electrospun nanofiber scaffolds hold interest for wound healing application as they can promote cell proliferation and differentiation due to the large, interconnected porous surface area which facilitates gas and nutrient exchange (Xu L. et al., 2022; Xu H. et al., 2022). Since cell-electrospinning allows the inclusion of live cells in the fibrous matrix, an array of cell types have been included in electrospun scaffolds including macrophages, endothelial cells, neutrophils, bone marrow mesenchymal stem cells, neural stem cells, fibroblasts and keratinocytes (Hong et al., 2019; Maurmann et al., 2023; Nosoudi et al., 2023). Crosslinked, rather than axial, nanofibers may promote superior fibroblast and keratinocyte migration within a 3D environment (Wang and Cui, 2021). These considerations can be accommodated by this technology; however, there are limitations such as loss of mechanical strength and maintenance of cellular viability over time (Hong et al., 2019; Wang and Cui, 2021; Maurmann et al., 2023).
To ameliorate limitations due to bulkiness of the device and the need for a power supply, portable, battery-operated electrospinning devices have been developed. These portable devices directly deposit nanofibers in the affected skin (in situ bioelectrospinning) and can be personalized to the target location and used beyond optimal operating theater conditions, such as conflict zones or low-resource settings (Xu S. et al., 2022). Although the feasibility of deploying portable devices for electrospinning wound dressings has previously been studied (Yan et al., 2019; Zhao et al., 2020), Xu et al. reported the first in situ cell electrospun dressing using a handheld device to apply bone marrow-derived stem cell (BMSCs)/polyvinyl alcohol (PVA) scaffolds directly to full-thickness injury sites (Xu S. et al., 2022). Cell survival immediately after cell electrospinning increased from 90.15% to 99.21% after 7 days in culture, which indicated that that cell proliferation was not impaired by the presence of the electrospun scaffold. The authors also determined that post-transplant, the wound closure time was significantly shorter in the cell/PVA group compared to either PVA alone or control groups. Interestingly, after 7 days, the granulation tissue was thicker, epithelial tissue had migrated towards the wound center, and skin appendage regeneration was evident in the cell/PVA condition. After 14 days, the wound was closed and covered by epithelized epithelial tissue, and an increase in subcutaneous fibroblasts and collagen fibers were detected. The authors noted that fiber deposition was not always accurate and that the repair mechanism remains unclear. Thus, there is a need for additional studies evaluating the cellular and molecular mechanisms driving wound healing in these scaffolds.
Organ-on-a-chip platforms offer great versatility to generate spatially organized tissue-constructs in the microscale. However, they struggle to generate the large 3D organ architectures that would be needed for skin or tissue transplantation (Rothbauer et al., 2022; Wu et al., 2023). State-of-the-art technologies, such as 3D bioprinting, may be able to fulfill this need. Specifically, bioprinting allows the fabrication of scaffold layers including living cells and ECM components that can be precisely stacked to mimic in vivo organization (Dey and Ozbolat, 2020; Matai et al., 2020). Various subcategories of 3D bioprinting, including extrusion, droplet and laser, have been developed with the aim of fabricating multicellular tissues and, eventually, living organs for transplantation.
Over the past decade, due to its thin and stratified nature, skin emerged as an early test bed for 3D bioprinting aimed for tissue repair and regeneration (Murphy and Atala, 2014). This idealized workflow would first use computer scanning imaging to design a graft perfectly matching the size and architecture needed in the wound site. Subsequently, bioprinting would be used to fabricate structures layer by layer according to a spatial geometry of choice to be transplanted. Alternatively, the skin surrogate could be directly printed onto the wound site using in situ bioprinting. Ideally, the scaffold would slowly degrade or integrate with host tissue, gradually leading to tissue regeneration (Weng et al., 2021b).
Bioink selection is one of the central considerations of 3D bioprinting. Bioink refers to a hydrogel or solution that is composed of a mixture of living cells, biologically active factors (e.g., cytokines and chemokines), and biological scaffolding materials.
Bioink development poses considerable challenges as pre- and post-printing requirements differ: bioink must remain in a dynamic state during the printing process, yet must rapidly solidify, retain its shape over time, and provide a suitable environment for cell and tissue development (Freeman et al., 2022). Therefore, gelation kinetics, rheological properties, resolution potential, and the ability to mimic the ECM are key considerations (Weng et al., 2021b). A major consideration of any substance to be implanted into the human body is that it is biocompatible, biodegradable, and bioinert to ensure cell survival and minimize host immune response (Balavigneswaran et al., 2023b). As bioink is in direct contact with cells, it is essential that the bioink is conducive to maintaining cell viability and mimics the in vivo microenvironment. Further, printed bioink should recapitulate biophysical properties of the tissue such as porosity, stiffness, architecture, to ensure both engraftment and printability of complex skin phenotypes (Javaid and Haleem, 2021).
Choices of scaffolding materials range from natural biopolymers, including proteins like collagen, fibrinogen, gelatin or albumin, to polysaccharides like cellulose, chitin, and chitosan, or synthetic polymers such as polycaprolactone (PCL) and poly (lactic-co-glycolic) acid (PLGA) (Tarassoli et al., 2018; Wang and Liu, 2018). These materials should be printable, which depends upon overall mechanical properties, including viscosity and shear thinning, and capacity for cross-linking, whether using physical, chemical, ionic or stereocomplex mechanisms (Tarassoli et al., 2018).
Bioink formulations can be further refined to address particular concerns, such as bacterial infection and severe inflammation which can impede or prevent wound healing (Wang et al., 2024). For example, Zeng et al. determined that functionalizing a silk fibroin/kappa-carrageenan (kCA)-based hydrogel dressing with the polyphenol found in green tea, epigallocatechin-3-gallate (EGCG), and Cu2+ enhanced the sustained release of their antibacterial and antioxidant properties (Zeng et al., 2022). This formulation also improved scavenging of intracellular reactive oxygen species, small molecules, such as hydrogen peroxide and hydroxyl radical that play a fundamental role in inflammation severity and slow tissue regeneration (Zhao et al., 2017; Wang et al., 2024). The EGCG compound alone demonstrated a 43% killing rate of S. aureus and E. coli; however, submerging the hydrogel in the Cu2+ for 30 min significantly increased the kill ratio to 93%. Zhao et al. developed the first series of chitosan-based, antibacterial, autonomously self-healing hydrogels which promoted wound healing (Zhao et al., 2017). While dressings with antibacterial agents can reduce infection risk, wound coverings and skin constructs may result in more prolonged beneficial activity and reduced cytotoxicity. The use of elastic hydrogels means they are able to self-repair several times in a row after bearing external mechanical forces, as demonstrated by the authors. The capacity to recover in this manner lengthens the lifespan of the skin construct and reduces inflammatory responses. Self-healing hydrogels are also believed to improve vascularization.
Other authors have reported using bioinks to improve vascularization; for instance, Ouyang et al., used gelatin as a sacrificial material to achieve structural fidelity and enhanced endothelialization (Ouyang et al., 2020). To ensure scaffold robustness, these authors used two bioinks, a gelatin templating and a photo-crosslinkable matrix bioink, printed side-by-side with embedded fibroblasts and HUVEC cells. This workflow allowed the authors to photo-crosslink the matrix and then incubate the structure at 37°C to liquify the gelatin to create a channel network. This technique demonstrated improved, uniform tubular structures, robust viability of human dermal fibroblasts, and enhanced in situ endothelialization. These HUVEC-lined channels successfully recapitulated complex vascular networks in 3D structures.
Due to the shortcomings inherent to many bioinks, such as suboptimal mechanical properties, Kim et al. developed a decellularized porcine skin as novel skin-derived extracellular matrix bioink (Kim et al., 2018). The bioink contained cytokines, growth factors, and other ECM components and exhibited excellent wound healing properties in vivo. However, most ECM formulations are unsuitable for bioprinting by themselves due to their low viscosity and mechanical strength. Other biomaterials would need to be added to complement this formulation, such as gelatin (Zhang et al., 2023). In the following sections, we will discuss all other components to be considered to optimize bioprinting of a transplantable construct.
Along with the choice of biomaterial, cell selection is a crucial determinant of whether a bioprinted tissue is functional in vivo. Cells for bioprinting must be non-immunogenic and should be amenable to in vitro expansion; furthermore, whilst keratinocytes and fibroblasts are frequently used in bioengineered skin constructs, additional cell types such as melanocytes, Langerhans cells, hair follicle papillae, adipocytes and epidermal stem cells have been integrated into more complex models (Gao et al., 2021b). Additionally, non-skin derived cells may also be included. A further consideration for clinical translation is that extremely large numbers of cells in the range of billions are required to mimic human skin. At present, cell expansion methods can only achieve cell expansion in terms of millions of cells, thus novel technologies are necessary to propel industrial scale-up (Varkey et al., 2019).
Growth factors can be defined as biologically active proteins released by cells, and they promote cell proliferation and differentiation. Well established examples include epidermal growth factor (EGF), which plays a role during wound healing and the production of collagen-I fibers; basic fibroblast growth factors (bFGF) and fibroblast growth factor-2 (FGF-2) which are essential during neovascularization, epithelial morphogenesis, and limit wound contraction; and vascular endothelial growth factor (VEGF) which is fundamental to angiogenesis.
3D bioprinting has great potential in recapitulating live microenvironments and tissues. Moreover, 3D bioprinting is high throughput, reproducible and has a shorter production cycle compared to other methods (Gao et al., 2021b). To achieve these goals, various techniques have been employed (Figure 5), including inkjet, microextrusion, and laser-assisted printing:
Figure 5. Bioprinting Techniques. (A) Laser-induced Forward Transfer (LIFT) involves utilizing a pulsed laser beam on a donor slide or ribbon coated with a laser-energy-absorbing layer such as gold or titanium. This layer contains the material of interest, like cells, hydrogels, or growth factors. The laser’s energy leads to the material’s evaporation, generating a high-pressure bubble that propels the material towards a receiving substrate positioned below the donor slide. (B) Inkjet bioprinting is a contactless method that involves depositing droplets of cells or biomaterials onto specific substrates. The prevalent inkjet bioprinters operate on a drop-on-demand principle, employing thermal, piezoelectric, and electrostatic inkjet nozzles as the primary. (C) Extrusion-based bioprinting involves a controlled dispensing system using pneumatic pressure or mechanical pistons to manage biomaterials at controlled temperatures. This technique produces continuous biomaterial filaments rather than droplets, depositing them in two dimensions along the x- and z-axes before layering in the y-axis, creating a 3D structure. It enables the deposition of high cell densities, hydrogels, and biocompatible copolymers. (D) Cell electrospinning leverages electrical forces to produce fibrous 3D scaffolds with cells embedded in the 3D matrix. The application of an electric field between the nozzle tip and the grounded collector causes the droplet at the tip of the nozzle to elongate and a liquid jet from the cone. The liquid jet is rapidly whipping, creating a random orientation of the micro/nanofibers. These micro/nanofibers provide a large surface area-to-volume ratio, which can be relevant for a variety of cellular processes. (E) Cell electrospray is based on electrically charging a liquid medium and force it to move through a large-bore needle, which forces the liquid to split into numerous microdroplets that contain the cells of interest.
This method is based on the principles of traditional inkjet printing and uses thermal, electromagnetic, or piezoelectric forces to deposit bioink droplets onto a substrate. Inkjet bioprinting offers excellent compatibility with commonly used biomaterials, is adaptable, and highly accurate impact construct viability (Davoodi et al., 2020). This method has been used to generate vascular system, bone, and cartilage constructs; for instance, Lee et al created a functional vasculature by using simultaneous printing of cells and ECM around the vascular channel (Lee et al., 2014a; Lee et al., 2014b). Gao et al. developed a method that condensed four to five steps into a one-step bioprinting protocol that demonstrated superior mechanical properties, enhanced viability, and the acrylated poly (ethylene glycol) (PEG)-peptide scaffold significantly inhibited bone marrow-derived human mesenchymal cell hypertrophy during chondrogenic differentiation and also enhanced osteogenic differentiation (Lee et al., 2014c; Gao et al., 2015).
Lee et al. used inkjet-based 3D bioprinting to generate a preliminary biomimetic skin construct using two keratinocyte, three fibroblast, and eight collagen layers (Lee V. et al., 2014). The model was able to successfully maintain the shape, structure, and function during the desired culture period. Extending this approach, Park et al. created self-organized 3D collagen microstructures using drop-on-demand inkjet printing that incorporated papillary microstructures at the dermal-epidermal junction, which enabled fibroblasts to be patterned at a higher density (Park et al., 2020). Ng et al. described a two-step approach to generate pigmented skin substitutes by printing keratinocytes and melanocytes into the surface of their collagen-fibroblast dermis, which displayed superior distribution and a greater number of biomarkers in comparison to skin surrogates generated using traditional pipetting techniques (Ng et al., 2018; Gao et al., 2021b).
Inkjet bioprinting has been used to generate more sophisticated skin surrogates; however, vascularization remains challenging. To promote neovascularization, Yanez et al. constructed a skin substitute that contained keratinocytes, fibroblasts, and HUVECs seeded in a collagen type I and fibrinogen matrix (Yanez et al., 2015). These were transplanted onto thymus-free nude mice and exhibited the formation of new microvessels and accelerated wound healing in comparison to a commercially available wound dressing (Apligraf) with the goal of creating customized skin surrogates, Jin et al. used a gelatin bioink with fibrinogen and alginate to harden the tissue post-printing and the cultured tissue maintained a survival rate of 90% after 14 days (Jin et al., 2022). The authors focused on calcium ions due to their role in keratinization. The results indicated that the addition of calcium ions and an air-liquid interface increased expression of loricin, a component of the conformed cellular envelope, which serves as a terminally differentiated stage marker for keratinocytes. Although they observed epidermal cell differentiation and migration to the upper layers of the epidermis, the keratinocytes failed to form a binding monolayer and instead exhibited a scattered distribution. The authors suggest that extending the time spent in culture or applying a thin layer of gelatin to the surface of the construct may ameliorate some of these drawbacks.
Extrusion printing uses a pneumatic, solenoid, or mechanical dispensing system that deposit continuous filaments of bioink through a nozzle (Zhang et al., 2019) This technique can accommodate higher viscosity substances, including bioinks with cell densities that are physiologically relevant, and has been used for tissue and organ generation applications. One of the main drawbacks of this technique are the shear forces used during the extrusion process, which if not carefully balanced, can reduce cell viability (Murphy and Atala, 2014).
Pourchet et al. developed a bioink that was composed of alginate, gelatin, and fibrinogen to generate a scaffold-free epidermis-dermis system in the shape of an adult human ear that permitted spatial control over complex structures (Pourchet et al., 2017). Daikuara et al. also used extrusion-based printing, in this case with a composite bioink of platelet lysate, a source of growth factors involved in wound healing, and gelatin methacryloyl to generate a skin construct which attained appropriate rigidity, cell adhesion, viability, and proliferation (Daikuara et al., 2022).
Quilez et al. generated a bilayer skin construct via continuous extrusion bioprinter and bioinks using a human plasma-derived fibrin matrix seeded with primary human fibroblasts with primary human keratinocytes on top of the scaffold (Quílez et al., 2020). The model demonstrated that large areas of skin construct could be rapidly printed. By analyzing markers such as an anti-K10 antibody, which labels differentiated epidermal keratinocytes, the authors concluded that the construct possessed structural and functional similarities to native skin and skin constructs that were manually produced by their group. Cubo et al. used a modified extrusion bioprinter with four syringes to print a human bilayered skin equivalent using bioinks containing primary human fibroblasts and keratinocytes along with human plasma (Cubo et al., 2016). Authors were able to print 100 cm2 of skin in less than 35 min, a clear feat in the field. Using histological and immunological analysis the authors determined that the in vitro skin construct was similar to native skin with a stratum basale, spinosum, granulosum, and a mature stratum corneum indicative of terminal differentiation, indicating complete differentiation of the skin surrogate. Further analysis using established skin markers (e.g., Collagen VII), revealed that dermo-epidermal junctions were correctly formed. This is essential for mechanical stability and its absence results in the extreme blistering observed in Dystrophic Epidermolysis Bullosa patients (i.e., genetic skin disease caused by mutations in Collagen VII gene).
As previously discussed, the ideal skin surrogate requires an air-liquid interface; however, this is problematic for extrusion-based bioprinting as the cells are encapsulated within the bioink. Consequently, Ahn et al. utilized a sacrificial gelatin-assisted extrusion bioprinting technique which displayed improved epidermal differentiation and stratification in comparison to other extrusion bioprinting technologies (Ahn M. et al., 2023).
These findings suggest that extrusion-based 3D bioprinting, in conjunction with tailored bioinks, can facilitate the generation of complex, physiologically relevant wound repair. Nevertheless, extrusion-based bioprinting is slower compared to other types of bioprinting and has a lower resolution that can limit clinical applications.’ However, there are numerous advantages in comparison to jet and laser-based methods, such as faster speed, wider array of suitable bioinks, greater mechanical strength, and the ability to bioprint porous grid structures, which can aid nutrient and metabolite circulation (Weng et al., 2021b).
Initially developed as a high-resolution metal patterning technique for the computer chip industry, this technique is nozzle- and scaffold-free (Zennifer et al., 2022). A laser beam pulses onto a donor ‘ribbon’ structure that has an energy absorbing layer with bioink. The heat generated by this process allows the deposited bioink to rapidly evaporate and the resultant liquid droplets to be sprayed onto a substrate; this method is non-contact and, as it avoids mechanical stress, cell viability is preserved (Xie et al., 2020).
Specifically, Koch et al. determined that laser-assisted bioprinting did not have deleterious effects on cells, such as increased apoptosis or fragmented DNA (Koch et al., 2010; Koch et al., 2012). They used laser-induced forward transfer, a process involving a laser pulse to deposit cells onto a film, which are propelled forward onto a Matriderm scaffold. Human fibroblast, keratinocyte cell lines and mesenchymal stem cells were included in their constructs with cell viability of 90%–98%, and retained proliferation capacity. Building upon their own work, Michael et al. used laser-assisted bioprinting to generate a fully cellularized skin construct with fibroblasts and keratinocytes on top of a stabilizing matrix (e.g., Matriderm) (Michael et al., 2013). The authors determined that the technique does not negatively affect cellular phenotype or function and could be applied to the generation of other tissue types. After transplant, the Matriderm carrier became populated by both the printed and host fibroblasts, which resulted in integration of the construct into the wound. Although there was a lack of full keratinocyte differentiation and vascularization, the authors proposed that printing ridges and a thicker epidermis will resolve these issues. While the potential of this technique is high and preserves cell viability, high throughput printing is limited due to the lack of a rapid gelation mechanism (Weng et al., 2021b). In the last years, there has been a rapid increase of studies leveraging bioprinting techniques to generate sophisticated bioengineered skin systems (Figure 6).
Figure 6. Bioprinting Applications for Skin. (A) Laser assisted bioprinting of endothelial cells (HPMECs, GFP-labeled) printed in grid patterns or as lines on Matrigel showed vessel formation within 24 h (blue=Hoechst stain). The printed cells in patterns demonstrated tubular structures with lumens. This technique could be used to create the microvasculature present in the skin. Scale bars are 1 mm (I), 200 µm (ii left and iii left), 50 µm (ii right and iii center), and 10 µm (iii right) (Koch et al., 2021). (B) Cell electrospinning to generate aligned nano or microfibers with mouse myoblast cells with an electric field of 0.075-kV/mm and flow rate of 0.25 mL/h (Wang Y. et al., 2022). (C) Bio-electrospraying 3D organotypic skin cultures. (i) Manual and bio-electrospray seeding process. (ii) Digital high speed images showing electrospraying of hydrogels containing fibroblasts and keratinocytes. (iii) Bright field microscope images of the cryosectioned bio-electrosprayed organotypic skin tissues (Jayarajan et al., 2024). (D) A 3D skin model is produced through a microextrusion-inkjet hybrid printing process, involving the dispensing of a dermal layer containing human dermal fibroblasts and collagen, followed by inkjet printing of human keratinocytes onto the cultured dermis, concluding with short-term epidermal and extended air–liquid interface cultures to construct the epidermal layer (Lee et al., 2021).
Bioprinting has several limitations in respect to clinical translation, including relative fragility of constructs due to initial weak mechanical strengths, risks of contamination during transport, and maintaining viability of the graft in culture (Singh et al., 2020). This can negatively impact structural fidelity and increase contamination and subsequent infection risks. In situ bioprinting, (i.e., in vivo), has emerged as a promising alternative. In situ bioprinting involves the graft being directly printed onto the target location in the living host’s body, which is then essentially used as a bioreactor (Singh et al., 2020). Early attempts by Binder et al. made use of inkjet printing and collagen-fibrinogen hydrogel precursors with embedded human fibroblasts and keratinocytes (Binder et al., 2010). Grafts were printed in a layer-by-layer fashion directly onto the injury site. Enhanced wound recovery was observed with complete closure at 3 weeks with a dermal and epidermal structure present.
Some other examples have been reported since. Sofokleous et al. developed a portable electro-hydrodynamic multi-needle spray gun to allow delivery of artificial skin, along with drugs and wound dressings to injured skin (Sofokleous et al., 2013). Later, Hakimi et al. produced planar dermal and epidermal tissue sheets from a microfluidic cartridge that were tested in murine and porcine models as proof-of-concept (Hakimi et al., 2018). Another example was reported by Albanna et al., who developed a mobile printing system consisting of a portable wound scanner and printer head with XYZ movement capacity within a small frame for on-site treatment of extensive cutaneous injury (Albanna et al., 2019). This mobile inkjet printer delivers a hydrogel with either autologous or allogeneic dermal fibroblasts and epidermal keratinocytes for rapid wound closure with only <15% of the original injury size observed at 14 days and full closure after 3 weeks versus collagen and fibrin matrix alone or untreated controls. Reduced wound contraction, and a dermal structure similar to that of healthy skin was also noted.
In situ bioprinting can be used in conjunction with other innovative technologies, including machine learning, as the increased geometrical accuracy afforded by artificial intelligence helps to compensate for movement and the irregular topography of wound sites (Freeman et al., 2022). The generation of grafts for posterior implantation is done away from to the wound surface and grafts are printed on a planar substrate before grafting to the target site. This can lead to geometric mismatches between the construct and target surface (Zhu et al., 2020; Freeman et al., 2022). In contrast, in situ bioprinting is directly targeted to the graft site and artificial intelligence (AI) can be optimized to sense and predict the printing environment including moving freeform surfaces, for example, printing cell-laden hydrogels onto a free-moving hand or live mice (Zhu et al., 2018). 3D bioprinting can involve AI at different levels and be divided into broad categories (Zhu et al., 2020):
1) Without AI - this is conventional 3D bioprinting where computer-aided design models are used to fabricate the model in a deterministic manner using a pre-computed toolpath. Albeit offering the least flexibility of all the techniques, this is the most common approach.
2) Open-loop AI - this approach uses pre-acquired, offline data, such as laser scans of multi-view images, and complex algorithms to reconstruct the precise 3D geometry of the graft site (Mahmoudi et al., 2023). This information is used by the printing platform to determine optimal tool path that considers varied geometrical parameters, such as skin deformation to maximize fidelity to a dynamically changing host site (Zhu et al., 2020). This workflow has the potential to accommodate shape-morphing properties of materials, induced by mechanical load and thermal expansion, to print a 3D structure that complies with a changeable graft site. Open-loop techniques permit high structural fidelity, thus allowing an improved match between the printed construct and the unique surface of the target defect. However, once the construct is printed the system cannot adapt to changes, including recipient movement during print operations (Zhu et al., 2020; Mahmoudi et al., 2023).
3) Closed-loop AI - sensing and adapting to dynamic changes are key benefits of this technology. Sensory data generated by integrated cameras and strain gauges is processed by AI in real-time to recognize both low- and high-level properties of the graft site. Moreover, the toolpath can be adjusted in real-time via a feedback-control system that can assess and correct for a vast array of factors. These factors include nozzle clogging, drift of the print head, temperature changes, topography of the graft site and human error (Zhu et al., 2018; Pugliese and Regondi, 2022; Mahmoudi et al., 2023). Closed-loop systems use online data to permit real-time adjustments to enhance printing quality, even where the recipient is moving, due to online tracking. Consequently, rapid online updates to guide the tool path is clearly advantageous when considering clinical translation (Zhu et al., 2020; Pugliese and Regondi, 2022).
4) Predictive AI - this technology has the potential to mitigate some of the limitations of closed-loop systems in which movement of the target location is assumed to be quasi-static. When the host site undergoes rapid changes, closed-loop approaches cannot provide real-time feedback quickly enough to avoid latency in corrective adjustments. Predictive AI uses previous data to sense current conditions, for example, the configuration of the wound site, and anticipate future states, such as deformation, to reduce error. Therefore, it is particularly useful for printing on moving targets and on organs, such as a beating heart, which exhibit quasi-repetitive movement. This technology is inherently complicated and still under development. However, it is believed that such sophisticated systems are essential to advance other innovative tools, including 3D printer/‘robotic surgeon’ hybrids, to facilitate safe in situ printing on the fragile tissues of living recipients (Zhu et al., 2020).
Handheld printing devices are convenient and permit the physician to directly print onto the wound site. However, their application is limited to simple structures and there is the risk of human error, especially if the device is heavy or uncomfortable to operate (MacAdam et al., 2022; Xie et al., 2022). Conversely, robotic technology can perform accurate, repetitive movements without human fatigue. Robotic-based systems are now routinely used in minimally invasive surgical settings to assist the surgeon with the precise operation of instruments (Xie et al., 2022). This occurs via remote, computer-aided control over robotic arms and has laid the foundations for their use in in situ bioprinting (Wang X. et al., 2022). Robotic assisted in situ printing (i.e., bioprinting robots controlled by computers) exhibit improved accuracy and flexibility, particularly when printing curved surfaces and complex geometries (Dong et al., 2022). Robotic systems have been used to enhance spatial control and consistency in comparison to manual, handheld tools which are limited in terms of their ability to print non-fixed structures or to adapt to anatomical differences. However, delays in responding to tissue topography can lead to poor printing quality or tissue damage. Combining predictive AI with robotic ‘surgeons’ can alleviate some of these concerns and reduce printing errors. Nevertheless, the use of robots to assist in clinical settings has not currently progressed beyond prototyping phases and the majority of machine-controlled platforms are only suitable for epidermal applications (Zhu et al., 2018) as they use a gantry that can move in the X, Y, and Z-axes enclosed in large frames (O’Neill et al., 2017; Zhu et al., 2018).
Nonetheless, whilst the incorporation of AI into in situ bioprinting is intriguing and holds the promise of alleviating numerous difficulties, the technology is costly and requires further development to determine the parameters of control between AI and the human user, and to overcome technological limitations to avoid errors and to allow switching between autonomous and semi-autonomous control (Zhu et al., 2020). Further considerations for clinical translation include the challenge of operating a printing that is integrated with the operative site in the body, whilst simultaneously maintaining a sterile surgical field and navigating confidentiality regulations such as HIPAA as patient information may be disclosed not only to medical staff, but engineers and other related staff (Matai et al., 2020).
Whilst both microfluidic organ-on-a-chip and 3D bioprinting represent cutting edge technologies in the fields of tissue and/or organ engineering, each method displays several limitations, which impede clinical application. 3D bioprinting permits the rapid generation of complex biomimetic architectures at high resolution. However, the resultant engineered tissues differ considerably from that of native tissue with most printing techniques focusing upon geometry rather than function (Ma et al., 2018). Conversely, microfluidic technologies recapitulate physiological responses via the precise control over fluids that carry cells, biomolecules or cells and laminar flow permits the input of multiple materials without mixing (Richard et al., 2020); however, operating at the microscale, this approach faces immense difficulties in scaling up to sizes suitable for tissue and organ transplantation (Ma et al., 2018). However, the notion of hybrid bioprinting-microfluidic platforms is gaining traction and there have been a number of attempts to generate tissues that are both structurally and functionally competent.
Human tissue exists at the micro, meso, and macro scales and this hierarchical cellular organization underpins proper tissue and/or organ function (Richard et al., 2020). However, 3D bioprinting operates at the macroscale level and is currently limited by the inability to achieve the microscale resolution necessary for appropriate microenvironmental cell signaling for cell differentiation and spontaneous morphogenesis (Richard et al., 2020). Therefore, the addition of microfluidic technologies, which utilize fluids and physical parameters at the microscale, can be employed to enhance microstructural control over zone-specific heterogeneity in multi-material and multi-cell bioprinted tissues (Richard et al., 2020). Seol et al. engineered a customized “BioMask” skin substitute combined with a wound dressing to address the complications inherent to treating severe facial injuries (Seol et al., 2018). The strategy used integrated tissue-organ printing that combines extrusion bioprinting and a multi cartridge element to print different material in a controlled manner to produce a trilayered structure that precisely matches the contours of a patient’s face - an advance over constructs engineered as a flat tissue.
With the popularity of extrusion bioprinting much of the focus of integrating 3D bioprinting with microfluidic technology has been on this technique (Davoodi et al., 2020). This has led to the development of instruments that offer improved manipulation of small (<10–9 L) bioink volumes through small microchannels allowing the simultaneous extrusion of multiple bioinks to fabricate heterogeneous structures (Davoodi et al., 2020).
Despite many improvements in skin construct development, there are still limitations as native skin does not exist as a planar patch, as seen in most models. Where geometry is more complex, such as the face or the hand, there is an unmet need for grafts able to fully enclose irregularly shaped regions, rather than a patchwork of smaller grafts. To address this concern, Seol et al. engineered a customized “BioMask” skin substitute combined with a wound dressing to treat severe facial injuries (Seol et al., 2018). The strategy used integrated tissue-organ printing that combines extrusion bioprinting and a multi cartridge element to print different material in a controlled manner to produce a trilayered structure that precisely matches the contours of a patient’s face - an advance over constructs engineered as a flat tissue.
An unmet need is to generate grafts capable of mimicking the mechanical and structural properties of continuous skin (Pappalardo et al., 2023). Using a 3D printed permeable hand-shaped scaffold, the authors seeded cells in layers, beginning with fibroblasts and collagen I using a PDMS negative mold to form the dermis. After 14 days, the space between the dermal layer and the mold was injected with primary human keratinocytes in culture medium to generate an epidermal layer. They subsequently exposed one side of the construct to medium and the outer side to air to ensure cornification of the glove-like skin equivalent. The model was vascularized with human dermal blood endothelial cells to aid graft viability and demonstrated that the construct could be successfully transplanted onto a mouse hindlimb, thus illustrating the potential for personalized care for challenging geometries. The edgeless construct displayed superior resistance to rupture stress compared to conventional hydrogel-based models, which can withstand more robust handling and grafting. However, although this technology offers numerous benefits, including the potential to grow constructs from small autologous biopsies, the engineered tissue currently lacks the appendages, pigmentation, and immune function present in native skin.
Overall, 3D bioprinted cell constructs are a promising alternative to traditional methods and can achieve high levels of reproducibility, is a high throughput technology, and can generate on-demand, spatially complex structures that include multiple cell types. However, maintaining precision can be problematic, particularly at the corners of printed structures, due to the fluctuating flow and pressure in the device; nonetheless, the use of PDMS-based microfluidics chips to ameliorate some of the issues with bioprinting as a stand-alone technique requires time consuming multistep photolithography protocols, and the inherent risks of nozzle clogging remains. Combining extrusion and microfluidic-based bioprinting systems may offer higher resolution results than extrusion printing alone and spatial gradients are possible; conversely, speed and resolution remains lower than inkjet techniques, which also present superior cell viability (Davoodi et al., 2020). Consequently, further optimization of both bioinks and printing hardware is required (Davoodi et al., 2020). Nonetheless, if the goal is to transition beyond lab-based construct generation for basic research to fully functional, biomimetic tissues, then combining technologies offers novel opportunities for regenerative medicine.
The emergence of innovative technologies to generate sophisticated skin surrogates offers a promising route toward lab-grown skin and other tissues. However, this enthusiasm must be tempered, as there are a number of regulatory steps that must be overcome to enable safe and cost-effective deployment of these technologies into the clinic. To comply with legal requirements and to ensure patient safety, manufacturers of medical devices and therapeutic interventions are obligated to comply with several rigorous hurdles before a product can be brought to market. There are regional monitoring agencies, including the European Medicines Agency (EMA), Health Canada, and the Australia Therapeutic Goods Administration (TGA), which evaluate risk and maintain safety standards (Beheshtizadeh et al., 2022). In the United States, the US Food and Drug Administration (FDA) oversees approval of drugs and medical devices, and the regulatory pathway depends upon factors such as whether the product uses human cells or tissues, animal-derived or synthetic materials and the intended application (Belsky and Smiell, 2021). Skin surrogates are a heterogenous product that may be cellular or acellular; can vary in terms of human, animal, or synthetically derived sources; and may feature either the epidermis or dermis alone or can be composite structures. These factors influence which FDA pathway is most appropriate and the amount of supporting data required regulatory complexity increases from Class I devices (low risk) through to Class III (high risk) (Belsky and Smiell, 2021).
Commercially available tissue engineered, and regenerative medicine products have only been available for the past 2 decades and determining the most appropriate pathway to evaluate safety and efficacy has been fraught with difficulties (Mansbridge, 2020). Engineered skin substitutes are frequently considered combination products (Varkey et al., 2019) that do not readily fit into FDA the regulatory centers, which are the Center Drug Evaluation and Research (CDER) (Varkey et al., 2019); the Center for Devices and Radiological Health (CDRH); and the Center for Biologics Evaluation and Research (CBER) (Mansbridge, 2020). Historically, skin replacements were classified as devices; however, it became clear that the rapidly evolving field of tissue engineering could also fit into the category of biologics due to the cellular component. The regulatory trajectory seems to follow this direction with constructs containing keratinocytes and fibroblasts (e.g., GINTUIT), which are often comprised of both device and biologic components, are being approved by the CBER (Falanga, 2020).
Engineered skin substitutes must meet the requirements of the FDA Quality System Regulation (QSR)/Medical Device Good Manufacturing practices (GMP) and the use of novel manufacturing processes, such as microfluidics and 3D printing, can incur additional quality evaluations to confirm reliability and repeatability of graft fabrication and performance (Savoji et al., 2018). The inclusion of cells also requires the CBER premarket approval requirement (PMA) (Savoji, 2018), making the process lengthy and complex (Savoji et al., 2018). There has been concerted efforts to collaborate with academic and industry bodies to balance safety with timely access to novel interventions. However, considerable opportunities for improvement remain, which would then expedite treatment for patients with life threatening medical concerns (Savoji et al., 2018).
Significant progress has been made in the field of tissue engineering, yet many challenges remain in the pursuit of generating biocompatible, scalable, and commercially viable transplantable constructs for clinical application. Even in the arena of skin substitutes which has witnessed the greatest headway, commercially available options are, arguably, rudimentary approximations of native skin often lacking full stratification, vascularization, sensory function, or appendages. Regulatory approval for engineered tissues has been further impeded by the reliance on animal models as transplant recipients, raising concerns surrounding translatability to humans due to immunological inter-species differences (Zamboni et al., 2018).
However, bioengineering techniques continue to evolve and improve, for instance 3D printing is now moving in the direction of 4D ‘smart’ printing, which essentially refers to constructs that respond to environmental cues and modify their structure and/or function in a preprogrammed manner to external stimuli such as temperature or ultraviolet light (Arif et al., 2022). Nonetheless, generation of complex multilayer architecture with numerous cell types that can also regenerate specialized skin appendages, such as sweat glands and hair follicles, remains elusive. Overall, there are still some challenges that need to be addressed to consistently reproducible skin constructs on a commercially feasible scale which are also cost-effective and easily transportable without compromising the delicately balanced 3D microenvironment of human skin.
Nevertheless, advances in the development of biomaterials, cutting-edge fabrication techniques, and improved understanding of the mechanical and biochemical properties of specific human tissue types allows for cautious optimism in the move from bench to bedside. Additionally, recent emphasis on connecting complicated manufactured tissue constructs and host tissues, promoting vascularization and sensory innervation, represents a considerable step forward. However, as our understanding of the complex interactions between scaffolds, cells, ECM, and the injury location increases, additional questions are raised–thus highlighting importance collaboration between researchers and clinicians. Thus, we believe an interdisciplinary approach offers the potential to accelerate the development of truly biomimetic transplantable precision-engineered tissues.
Due to the chronic global undersupply of donor organs, it is imperative that alternative strategies are developed to mitigate the shortfall and reduce patient mortality and morbidity. Moreover, even when patients are fortunate enough to undergo organ transplantation, the risks of graft rejection and the need for long-term immunosuppression present serious risks. Consequently, there is an urgent need for innovative solutions to ameliorate these challenges. To this end, bioengineered tissues and organs have emerged as an interesting concept; however, to date, these constructs display limited utility in clinical practice as they frequently lack spatial complexity, complete vascularization, immune components, and a fully functional phenotype.
Organ-on-a-chip and 3D bioprinting platforms represent an advance over traditional methods and progress has been made in generating skin constructs, but they also present several limitations and a fully functional skin substitute that captures all properties present in native tissue has yet to be realized (Figure 7). Whilst bioprinting offers excellent opportunities to produce intricately layered structures, maintaining cell viability and preserving tissue function can be challenging. Conversely, microfluidic technologies permit precise control over extremely small volumes of fluid, which recapitulates physiologically relevant functionality, but, due to the limited size of such devices, the potential to generate tissues and/or organs suitable for transplantation remains limited. Nonetheless, with further research, combining microfluidic techniques with 3D bioprinting may offer a powerful, integrative approach to mitigate the challenges inherent to each platform when utilized individually.
Figure 7. Models for organ transplant. Table summarizes the main models used in organ transplant research: (A) animals, (B) 2D culture, (C) organoids, (D) organ on a chip, (E) Cell electrospray, (F) Bioelectrospinning, and (G) 3D bioprinting. Advantages and limitations of each model type are also discussed.
Nonetheless, despite considerable advances in bioengineering and regenerative medicine, the gap from ‘bench to bedside’ for even state-of-the-art engineered tissues is yet to be bridged. The generation of implantable tissues and whole organs that exhibit structural complexity, long term survival, and a functional phenotype has proven elusive; however, increased understanding of tissue genesis, the dynamic microenvironment, vascularization, and the immunological milieu has potential to expand the supply of transplantable tissues and to ameliorate suffering.
Overall, whilst the feasibility of using lab generated constructs for transplantation is currently speculative, over time, it is conceivable that engineered tissues and whole organs will augment the critically limited pool of available donor organs. Many bioengineering approaches exist and, as no single platform excels at every application, it is possible that a chosen fabrication technique - or combinations thereof - may be precisely tailored to suit the specific parameters of the target tissue, organ, or clinical need. However, further optimization is required to translate engineered organs from bench to bedside, and to transform science fiction into clinical reality.
CR-M: Conceptualization, Writing–original draft, Writing–review and editing. JP: Conceptualization, Writing–original draft, Writing–review and editing. FR-M: Conceptualization, Writing–original draft, Writing–review and editing. DA-A: Conceptualization, Writing–original draft, Writing–review and editing. MV-M: Conceptualization, Writing–original draft, Writing–review and editing, Supervision. JA: Conceptualization, Writing–original draft, Writing–review and editing, Supervision.
The author(s) declare that financial support was received for the research, authorship, and/or publication of this article. This work was supported by EB Research Partnership, Inc. (“EB Charities”).
The authors declare that the research was conducted in the absence of any commercial or financial relationships that could be construed as a potential conflict of interest.
All claims expressed in this article are solely those of the authors and do not necessarily represent those of their affiliated organizations, or those of the publisher, the editors and the reviewers. Any product that may be evaluated in this article, or claim that may be made by its manufacturer, is not guaranteed or endorsed by the publisher.
Abaci, H. E., Coffman, A., Doucet, Y., Chen, J., Jacków, J., Wang, E., et al. (2018). Tissue engineering of human hair follicles using a biomimetic developmental approach. Nat. Commun. 9 (1), 5301–5311. doi:10.1038/s41467-018-07579-y
Abaci, H. E., Gledhill, K., Guo, Z., Christiano, A. M., and Shuler, M. L. (2015). Pumpless microfluidic platform for drug testing on human skin equivalents. Lab. Chip 15, 882–888. doi:10.1039/c4lc00999a
Abadpour, S., Aizenshtadt, A., Olsen, P. A., Shoji, K., Wilson, S. R., Krauss, S., et al. (2020). Pancreas-on-a-Chip technology for transplantation applications. Curr. Diab Rep. 20, 72. doi:10.1007/s11892-020-01357-1
Achilli, T. M., Meyer, J., and Morgan, J. R. (2012). Advances in the formation, use and understanding of multi-cellular spheroids. Expert Opin. Biol. Ther. 12, 1347–1360. doi:10.1517/14712598.2012.707181
Ahn, J., Ohk, K., Won, J., Choi, D. H., Jung, Y. H., Yang, J. H., et al. (2023a). Modeling of three-dimensional innervated epidermal like-layer in a microfluidic chip-based coculture system. Nat. Commun. 14, 1488. doi:10.1038/s41467-023-37187-4
Ahn, J., Ohk, K., Won, J., Choi, D. H., Jung, Y. H., Yang, J. H., et al. (2023b). Modeling of three-dimensional innervated epidermal like-layer in a microfluidic chip-based coculture system. Nat. Commun. 14 (1), 1488. doi:10.1038/s41467-023-37187-4
Ahn, M., Cho, W., Lee, H., Park, W., Lee, S., Back, J. W., et al. (2023c). Engineering of uniform epidermal layers via sacrificial gelatin bioink-assisted 3D extrusion bioprinting of skin (adv. Healthcare mater. 27/2023). Adv. Healthc. Mater. 12, 2370171. doi:10.1002/adhm.202370171
Albanna, M., Binder, K. W., Murphy, S. V., Kim, J., Qasem, S. A., Zhao, W., et al. (2019). In situ bioprinting of autologous skin cells accelerates wound healing of extensive excisional full-thickness wounds. Sci. Rep. 9 (1), 1856. doi:10.1038/s41598-018-38366-w
Amirsadeghi, A., Jafari, A., Eggermont, L. J., Hashemi, S. S., Bencherif, S. A., and Khorram, M. (2020). Vascularization strategies for skin tissue engineering. Biomater. Sci. 8, 4052. doi:10.1039/D0BM00266F
Amstad, E. (2017). Microfluidics: a tool to control the size and composition of particles. Chim. (Aarau) 71, 334. doi:10.2533/chimia.2017.334
Arif, Z. U., Khalid, M. Y., Ahmed, W., and Arshad, H. (2022). A review on four-dimensional (4D) bioprinting in pursuit of advanced tissue engineering applications. Bioprinting 27, e00203. doi:10.1016/j.bprint.2022.e00203
Ashammakhi, N., Wesseling-Perry, K., Hasan, A., Elkhammas, E., and Zhang, Y. S. (2018). Kidney-on-a-chip: untapped opportunities. Kidney Int. 94, 1073–1086. doi:10.1016/j.kint.2018.06.034
Ataç, B., Wagner, I., Horland, R., Lauster, R., Marx, U., Tonevitsky, A. G., et al. (2013). Skin and hair on-a-chip: in vitro skin models versus ex vivo tissue maintenance with dynamic perfusion. Lab. Chip 13, 3555. doi:10.1039/c3lc50227a
Augustine, R. (2018). Skin bioprinting: a novel approach for creating artificial skin from synthetic and natural building blocks. Prog. Biomater. 7, 77–92. doi:10.1007/s40204-018-0087-0
Avci, P., Sadasivam, M., Gupta, A., De Melo, W. C., Huang, Y. Y., Yin, R., et al. (2013). Animal models of skin disease for drug discovery. Expert Opin. Drug Discov. 8, 331–355. doi:10.1517/17460441.2013.761202
Balavigneswaran, C. K., Selvaraj, S., Vasudha, T. K., Iniyan, S., and Muthuvijayan, V. (2023a). Tissue engineered skin substitutes: a comprehensive review of basic design, fabrication using 3D printing, recent advances and challenges. Biomater. Adv. 153, 213570. doi:10.1016/j.bioadv.2023.213570
Balavigneswaran, C. K., Selvaraj, S., Vasudha, T. K., Iniyan, S., and Muthuvijayan, V. (2023b). Tissue engineered skin substitutes: a comprehensive review of basic design, fabrication using 3D printing, recent advances and challenges. Biomater. Adv. 153, 213570. doi:10.1016/j.bioadv.2023.213570
Baltazar, T., Merola, J., Catarino, C., Xie, C. B., Kirkiles-Smith, N. C., Lee, V., et al. (2020). Three dimensional bioprinting of a vascularized and perfusable skin graft using human keratinocytes, fibroblasts, pericytes, and endothelial cells. Tissue Eng. Part A 26, 227–238. doi:10.1089/ten.tea.2019.0201
Bang, S., Tahk, D., Choi, Y. H., Lee, S., Lim, J., Lee, S., et al. (2022). 3D microphysiological system-inspired scalable vascularized tissue constructs for regenerative medicine. Adv. Funct. Mater. 32, 2105475. doi:10.1002/adfm.202105475
Beheshtizadeh, N., Gharibshahian, M., Pazhouhnia, Z., Rostami, M., Zangi, A. R., Maleki, R., et al. (2022). Commercialization and regulation of regenerative medicine products: promises, advances and challenges. Biomed. Pharmacother. 153, 113431. doi:10.1016/j.biopha.2022.113431
Belsky, K., and Smiell, J. (2021). Navigating the regulatory pathways and requirements for tissue-engineered products in the treatment of burns in the United States. J. Burn Care and Res. 42, 774–784. doi:10.1093/jbcr/iraa210
Bergers, G., and Song, S. (2005). The role of pericytes in blood-vessel formation and maintenance. Neuro-Oncology 7, 452–464. doi:10.1215/s1152851705000232
Bhardwaj, N., Chouhan, D., and Mandal, B. B. (2017). Tissue engineered skin and wound healing: current strategies and future directions. Curr. Pharm. Des. 23, 3455–3482. doi:10.2174/1381612823666170526094606
Biedermann, T., Boettcher-Haberzeth, S., and Reichmann, E. (2013). Tissue engineering of skin for wound coverage. Eur. J. Pediatr. Surg. 23, 375–382. doi:10.1055/s-0033-1352529
Biedermann, T., Böttcher-Haberzeth, S., Klar, A. S., Widmer, D. S., Pontiggia, L., Weber, A. D., et al. (2015). The influence of stromal cells on the pigmentation of tissue-engineered dermo-epidermal skin grafts. Tissue Eng. Part A 21, 960–969. doi:10.1089/ten.tea.2014.0327
Binder, K. W., Zhao, W., Aboushwareb, T., Dice, D., Atala, A., and Yoo, J. J. (2010). In situ bioprinting of the skin for burns. J. Am. Coll. Surg. 211, S76. doi:10.1016/j.jamcollsurg.2010.06.198
Black, C. K., Termanini, K. M., Aguirre, O., Hawksworth, J. S., and Sosin, M. (2018). Solid organ transplantation in the 21st century. Ann. Transl. Med. 6, 409. doi:10.21037/atm.2018.09.68
Blais, M., Parenteau-Bareil, R., Cadau, S., and Berthod, F. (2013). Concise review: tissue-engineered skin and nerve regeneration in burn treatment. Stem Cells Transl. Med. 2, 545–551. doi:10.5966/sctm.2012-0181
Bojar, R. A. (2015). Studying the human skin microbiome using 3D in vitro skin models. Appl. Vitro Toxicol. 1, 165–171. doi:10.1089/aivt.2015.0002
Boyce, S. T., Lloyd, C. M., Kleiner, M. C., Swope, V. B., Abdel-Malek, Z., and Supp, D. M. (2017). Restoration of cutaneous pigmentation by transplantation to mice of isogeneic human melanocytes in dermal–epidermal engineered skin substitutes. Pigment. Cell Melanoma Res. 30, 531–540. doi:10.1111/pcmr.12609
Brassard-Jollive, N., Monnot, C., Muller, L., and Germain, S. (2020). In vitro 3D systems to model tumor angiogenesis and interactions with stromal cells. Front. Cell Dev. Biol. 8, 594903. doi:10.3389/fcell.2020.594903
Byrd, A. L., Belkaid, Y., and Segre, J. A. (2018). The human skin microbiome. Nat. Rev. Microbiol. 16 (3), 143–155. doi:10.1038/nrmicro.2017.157
Cadau, S., Leoty-Okombi, S., Pain, S., Bechetoille, N., André-Frei, V., and Berthod, F. (2015). In vitro glycation of an endothelialized and innervated tissue-engineered skin to screen anti-AGE molecules. Biomaterials 51, 216–225. doi:10.1016/j.biomaterials.2015.01.066
Catalano, E., Cochis, A., Varoni, E., Rimondini, L., and Azzimonti, B. (2013). Tissue-engineered skin substitutes: an overview. J. Artif. Organs 16, 397–403. doi:10.1007/s10047-013-0734-0
Chaudhari, A. A., Vig, K., Baganizi, D., Sahu, R., Dixit, S., Dennis, V., et al. (2016). Future prospects for scaffolding methods and biomaterials in skin tissue engineering: a review. Int. J. Mol. Sci. 17, 1974. doi:10.3390/ijms17121974
Chen, C., Wang, Y., Zhang, D., Wu, X., Zhao, Y., Shang, L., et al. (2021). Natural polysaccharide based complex drug delivery system from microfluidic electrospray for wound healing. Appl. Mater. Today 23, 101000. doi:10.1016/j.apmt.2021.101000
Chen, J., Fan, Y., Dong, G., Zhou, H., Du, R., Tang, X., et al. (2023). Designing biomimetic scaffolds for skin tissue engineering. Biomater. Sci. 11, 3051–3076. doi:10.1039/d3bm00046j
Chouhan, D., Lohe, T. u., Samudrala, P. K., and Mandal, B. B. (2018). In situ forming injectable silk fibroin hydrogel promotes skin regeneration in full thickness burn wounds. Adv. Healthc. Mater. 7, 1801092. doi:10.1002/adhm.201801092
Clevers, H. (2016). Modeling development and disease with organoids. Cell 165, 1586–1597. doi:10.1016/j.cell.2016.05.082
Cochrane, A., Albers, H. J., Passier, R., Mummery, C. L., van den Berg, A., Orlova, V. V., et al. (2019). Advanced in vitro models of vascular biology: human induced pluripotent stem cells and organ-on-chip technology. Adv. Drug Deliv. Rev. 140, 68–77. doi:10.1016/j.addr.2018.06.007
Cubo, N., Garcia, M., Del Cañizo, J. F., Velasco, D., and Jorcano, J. L. (2016). 3D bioprinting of functional human skin: production and in vivo analysis. Biofabrication 9, 015006. doi:10.1088/1758-5090/9/1/015006
Dai, R., Hua, W., Xie, H., Chen, W., Xiong, L., and Li, L. (2018). The human skin-derived precursors for regenerative medicine: current state, challenges, and perspectives. Stem Cells Int. 2018, 1–11. doi:10.1155/2018/8637812
Daikuara, L. Y., Chen, X., Yue, Z., Skropeta, D., Wood, F. M., Fear, M. W., et al. (2022). 3D bioprinting constructs to facilitate skin regeneration. Adv. Funct. Mater. 32, 2105080. doi:10.1002/adfm.202105080
Davoodi, E., Sarikhani, E., Montazerian, H., Ahadian, S., Costantini, M., Swieszkowski, W., et al. (2020). Extrusion and microfluidic-based bioprinting to fabricate biomimetic tissues and organs. Adv. Mater. Technol. 5, 1901044. doi:10.1002/admt.201901044
Dearman, B. L., Boyce, S. T., and Greenwood, J. E. (2021a). Advances in skin tissue bioengineering and the challenges of clinical translation. Front. Surg. 8, 640879. doi:10.3389/fsurg.2021.640879
Dearman, B. L., Boyce, S. T., and Greenwood, J. E. (2021b). Advances in skin tissue bioengineering and the challenges of clinical translation. Front. Surg. 8, 640879. doi:10.3389/fsurg.2021.640879
Dey, M., and Ozbolat, I. T. (2020). 3D bioprinting of cells, tissues and organs. Sci. Rep. 10, 14023. doi:10.1038/s41598-020-70086-y
Ding, X., Tang, Q., Xu, Z., Xu, Y., Zhang, H., Zheng, D., et al. (2022). Challenges and innovations in treating chronic and acute wound infections: from basic science to clinical practice. Burns Trauma 10, 14. doi:10.1093/burnst/tkac014
Dong, H., Hu, B., Zhang, W., Xie, W., Mo, J., Sun, H., et al. (2022). Robotic-assisted automated in situ bioprinting. Int. J. Bioprinting 9 (1), 629. doi:10.18063/ijb.v9i1.629
Eddaoudi, A., Townsend-Nicholson, A., Timms, J. F., Schorge, S., and Jayasinghe, S. N. (2010). Molecular characterisation of post-bio-electrosprayed human brain astrocytoma cells. Analyst 135, 2600. doi:10.1039/c0an00213e
Ehler, E., and Jayasinghe, S. N. (2014). Cell electrospinning cardiac patches for tissue engineering the heart. Analyst 139, 4449–4452. doi:10.1039/c4an00766b
Falanga, V. (2020). “Bioengineered skin constructs,” in Principles of tissue engineering (Elsevier), 1331–1352. doi:10.1016/B978-0-12-818422-6.00073-3
Fernandez-Carro, E., Angenent, M., Gracia-Cazaña, T., Gilaberte, Y., Alcaine, C., and Ciriza, J. (2022). Modeling an optimal 3D skin-on-chip within microfluidic devices for pharmacological studies. Pharmaceutics 14, 1417. doi:10.3390/pharmaceutics14071417
Freeman, S., Calabro, S., Williams, R., Jin, S., and Ye, K. (2022). Bioink formulation and machine learning-empowered bioprinting optimization. Front. Bioeng. Biotechnol. 10, 913579. doi:10.3389/fbioe.2022.913579
Gao, C., Lu, C., Jian, Z., Zhang, T., Chen, Z., Zhu, Q., et al. (2021b). 3D bioprinting for fabricating artificial skin tissue. Colloids Surfaces B Biointerfaces 208, 112041. doi:10.1016/j.colsurfb.2021.112041
Gao, C., Zhang, L., Wang, J., Jin, M., Tang, Q., Chen, Z., et al. (2021a). Electrospun nanofibers promote wound healing: theories, techniques, and perspectives. J. Mater. Chem. B 9, 3106–3130. doi:10.1039/d1tb00067e
Gao, G., Yonezawa, T., Hubbell, K., Dai, G., and Cui, X. (2015). Inkjet-bioprinted acrylated peptides and PEG hydrogel with human mesenchymal stem cells promote robust bone and cartilage formation with minimal printhead clogging. Biotechnol. J. 10, 1568–1577. doi:10.1002/biot.201400635
Gaskell, S. J. (1997). Electrospray: principles and practice. J. Mass Spectrom. 32, 677–688. doi:10.1002/(SICI)1096-9888(199707)32:7<677::AID-JMS536>3.0.CO;2-G
Gingras, M., Bergeron, J., Déry, J., Durham, H. D., and Berthod, F. (2003). In vitro development of a tissue-engineered model of peripheral nerve regeneration to study neurite growth. FASEB J. 17, 1–16. doi:10.1096/fj.02-1180fje
Girard, D., Laverdet, B., Buhé, V., Trouillas, M., Ghazi, K., Alexaline, M. M., et al. (2017). Biotechnological management of skin burn injuries: challenges and perspectives in wound healing and sensory recovery. Tissue Eng. Part B Rev. 23, 59–82. doi:10.1089/ten.teb.2016.0195
Goodarzi, P., Falahzadeh, K., Nematizadeh, M., Farazandeh, P., Payab, M., Larijani, B., et al. (2018). Tissue engineered skin substitutes. Adv. Exp. Med. Biol. 1107, 143–188. doi:10.1007/5584_2018_226
Groeber, F., Engelhardt, L., Lange, J., Kurdyn, S., Schmid, F. F., Rücker, C., et al. (2016). A first vascularized skin equivalent as an alternative to animal experimentation. ALTEX 33, 415–422. doi:10.14573/altex.1604041
Haisma, E. M., de Breij, A., Chan, H., van Dissel, J. T., Drijfhout, J. W., Hiemstra, P. S., et al. (2014). LL-37-derived peptides eradicate multidrug-resistant Staphylococcus aureus from thermally wounded human skin equivalents. Antimicrob. Agents Chemother. 58, 4411–4419. doi:10.1128/aac.02554-14
Hakimi, N., Cheng, R., Leng, L., Sotoudehfar, M., Ba, P. Q., Bakhtyar, N., et al. (2018). Handheld skin printer: in-situ formation of planar biomaterials and tissues. Lab. Chip 18, 1440–1451. doi:10.1039/c7lc01236e
Haldar, S., Sharma, A., Gupta, S., Chauhan, S., Roy, P., and Lahiri, D. (2019). Bioengineered smart trilayer skin tissue substitute for efficient deep wound healing. Mater. Sci. Eng. C 105, 110140. doi:10.1016/j.msec.2019.110140
Herland, A., Maoz, B. M., Das, D., Somayaji, M. R., Prantil-Baun, R., Novak, R., et al. (2020). Quantitative prediction of human pharmacokinetic responses to drugs via fluidically coupled vascularized organ chips. Nat. Biomed. Eng. 4, 421–436. doi:10.1038/s41551-019-0498-9
Hernández-Rangel, A., and Martin-Martinez, E. S. (2021). Collagen based electrospun materials for skin wounds treatment. J. Biomed. Mater. Res. - Part A 109, 1751–1764. doi:10.1002/jbm.a.37154
Hofmann, E., Schwarz, A., Fink, J., Kamolz, L. P., and Kotzbeck, P. (2023). Modelling the complexity of human skin in vitro. Biomedicines 11, 794. doi:10.3390/biomedicines11030794
Holland, D. B., Bojar, R. A., Jeremy, A. H. T., Ingham, E., and Holland, K. T. (2008). Microbial colonization of an in vitro model of a tissue engineered human skin equivalent – a novel approach. FEMS Microbiol. Lett. 279, 110–115. doi:10.1111/j.1574-6968.2007.01021.x
Hong, J., Yeo, M., Yang, G. H., and Kim, G. (2019). Cell-electrospinning and its application for tissue engineering. Int. J. Mol. Sci. 20, 6208. doi:10.3390/ijms20246208
Hong, Z.-X., Zhu, S. T., Li, H., Luo, J. Z., Yang, Y., An, Y., et al. (2023). Bioengineered skin organoids: from development to applications. Mil. Med. Res. 10, 40. doi:10.1186/s40779-023-00475-7
Hosseini, M., Koehler, K. R., and Shafiee, A. (2022). Biofabrication of human skin with its appendages. Adv. Healthc. Mater. 11, e2201626. doi:10.1002/adhm.202201626
Hu, C., Chen, Y., Tan, M. J. A., Ren, K., and Wu, H. (2019). Microfluidic technologies for vasculature biomimicry. Analyst 144, 4461–4471. doi:10.1039/c9an00421a
Huang, R., He, Z., Bian, Y., Lei, Z., Wang, H., Long, Y., et al. (2019). A biomimetic basement membrane substitute based on tri-layered nanofibrous scaffold for skin reconstruction. J. Biomed. Nanotechnol. 15, 2332–2350. doi:10.1166/jbn.2019.2865
Huang, S., Xu, Y., Wu, C., Sha, D., and Fu, X. (2010). In vitro constitution and in vivo implantation of engineered skin constructs with sweat glands. Biomaterials 31, 5520–5525. doi:10.1016/j.biomaterials.2010.03.060
Javaid, M., and Haleem, A. (2021). 3D bioprinting applications for the printing of skin: a brief study. Sensors Int. 2, 100123. doi:10.1016/j.sintl.2021.100123
Jayarajan, V., Auguste, J. O., Gene, K. A., Auguste, L., Nunez, C., Marcinowski, B., et al. (2024). Bio-electrospraying 3-D organotypic human skin cultures. Small 20, 2304940. doi:10.1002/smll.202304940
Jayasinghe, S. N., Warnes, G., and Scotton, C. J. (2011). Bio-electrosprayed living composite matrix implanted into mouse models. Macromol. Biosci. 11, 1364–1369. doi:10.1002/mabi.201100131
Jensen, C., and Teng, Y. (2020). Is it time to start transitioning from 2D to 3D cell culture? Front. Mol. Biosci. 7, 33. doi:10.3389/fmolb.2020.00033
Jeon, H. M., Kim, K., Choi, K. C., and Sung, G. Y. (2020). Side-effect test of sorafenib using 3-D skin equivalent based on microfluidic skin-on-a-chip. J. Industrial Eng. Chem. 82, 71–80. doi:10.1016/j.jiec.2019.09.044
Jeon, J. S., Bersini, S., Whisler, J. A., Chen, M. B., Dubini, G., Charest, J. L., et al. (2014). Generation of 3D functional microvascular networks with human mesenchymal stem cells in microfluidic systems. Integr. Biol. 6, 555–563. doi:10.1039/c3ib40267c
Jin, S., Oh, Y. N., Son, Y. R., Kwon, B., Park, J. H., Gang, M. J., et al. (2022). Three-dimensional skin tissue printing with human skin cell lines and mouse skin-derived epidermal and dermal cells. J. Microbiol. Biotechnol. 32, 238–247. doi:10.4014/jmb.2111.11042
Jones, C. F. E., Di Cio, S., Connelly, J. T., and Gautrot, J. E. (2022). Design of an integrated microvascularized human skin-on-a-chip tissue equivalent model. Front. Bioeng. Biotechnol. 10, 915702. doi:10.3389/fbioe.2022.915702
Jorgensen, A. M., Gorkun, A., Mahajan, N., Willson, K., Clouse, C., Jeong, C. G., et al. (2023). Multicellular bioprinted skin facilitates human-like skin architecture in vivo. Sci. Transl. Med. 15, eadf7547. doi:10.1126/scitranslmed.adf7547
Keirouz, A., Wang, Z., Reddy, V. S., Nagy, Z. K., Vass, P., Buzgo, M., et al. (2023). The history of electrospinning: past, present, and future developments. Adv. Mater. Technol. 8. doi:10.1002/admt.202201723
Kim, B. S., Kwon, Y. W., Kong, J. S., Park, G. T., Gao, G., Han, W., et al. (2018). 3D cell printing of in vitro stabilized skin model and in vivo pre-vascularized skin patch using tissue-specific extracellular matrix bioink: a step towards advanced skin tissue engineering. Biomaterials 168, 38–53. doi:10.1016/j.biomaterials.2018.03.040
Kim, J. J., Ellett, F., Thomas, C. N., Jalali, F., Anderson, R. R., Irimia, D., et al. (2019). A microscale, full-thickness, human skin on a chip assay simulating neutrophil responses to skin infection and antibiotic treatments. Lab. Chip 19, 3094–3103. doi:10.1039/c9lc00399a
Kim, K., Jeon, H. M., Choi, K. C., and Sung, G. Y. (2020). Testing the effectiveness of Curcuma longa leaf extract on a skin equivalent using a pumpless skin-on-a-chip model. Int. J. Mol. Sci. 21, 3898. doi:10.3390/ijms21113898
Koch, L., Deiwick, A., and Chichkov, B. (2021). Capillary-like formations of endothelial cells in defined patterns generated by laser bioprinting. Micromachines (Basel) 12, 1538. doi:10.3390/mi12121538
Koch, L., Deiwick, A., Schlie, S., Michael, S., Gruene, M., Coger, V., et al. (2012). Skin tissue generation by laser cell printing. Biotechnol. Bioeng. 109, 1855–1863. doi:10.1002/bit.24455
Koch, L., Kuhn, S., Sorg, H., Gruene, M., Schlie, S., Gaebel, R., et al. (2010). Laser printing of skin cells and human stem cells. Tissue Eng. Part C. Methods 16, 847–854. doi:10.1089/ten.tec.2009.0397
Konermann, L., Ahadi, E., Rodriguez, A. D., and Vahidi, S. (2013). Unraveling the mechanism of electrospray ionization. Anal. Chem. 85, 2–9. doi:10.1021/ac302789c
Kwak, B. S., Jin, S., Kim, S. J., Kim, E. J., Chung, J. H., and Sung, J. H. (2020). Microfluidic skin chip with vasculature for recapitulating the immune response of the skin tissue. Biotechnol. Bioeng. 117, 1853–1863. doi:10.1002/bit.27320
Lanno, G. M., Ramos, C., Preem, L., Putrinš, M., Laidmäe, I., Tenson, T., et al. (2020). Antibacterial porous electrospun fibers as skin scaffolds for wound healing applications. ACS Omega 5, 30011–30022. doi:10.1021/acsomega.0c04402
Laverdet, B., Girard, D., Bayout, A., Bordeau, N., Demiot, C., and Desmoulière, A. (2017). Effects of small-fiber neuropathy induced by resiniferatoxin on skin healing and axonal regrowth after burn. Burns 43, 562–572. doi:10.1016/j.burns.2016.09.016
Lee, H.-R., Park, J. A., Kim, S., Jo, Y., Kang, D., and Jung, S. (2021). 3D microextrusion-inkjet hybrid printing of structured human skin equivalents. Bioprinting 22, e00143. doi:10.1016/j.bprint.2021.e00143
Lee, J., Rabbani, C. C., Gao, H., Steinhart, M. R., Woodruff, B. M., Pflum, Z. E., et al. (2020). Hair-bearing human skin generated entirely from pluripotent stem cells. Nature 582 (7812), 399–404. doi:10.1038/s41586-020-2352-3
Lee, S., Jin, S. P., Kim, Y. K., Sung, G. Y., Chung, J. H., and Sung, J. H. (2017). Construction of 3D multicellular microfluidic chip for an in vitro skin model. Biomed. Microdevices 19, 22. doi:10.1007/s10544-017-0156-5
Lee, V., Singh, G., Trasatti, J. P., Bjornsson, C., Xu, X., Tran, T. N., et al. (2014d). Design and fabrication of human skin by three-dimensional bioprinting. Tissue Eng. Part C. Methods 20, 473–484. doi:10.1089/ten.tec.2013.0335
Lee, V. K., Kim, D. Y., Ngo, H., Lee, Y., Seo, L., Yoo, S. S., et al. (2014a). Creating perfused functional vascular channels using 3D bio-printing technology. Biomaterials 35, 8092–8102. doi:10.1016/j.biomaterials.2014.05.083
Lee, V. K., Kim, D. Y., Ngo, H., Lee, Y., Seo, L., Yoo, S. S., et al. (2014c). Creating perfused functional vascular channels using 3D bio-printing technology. Biomaterials 35, 8092–8102. doi:10.1016/j.biomaterials.2014.05.083
Lee, V. K., Lanzi, A. M., Ngo, H., Yoo, S. S., Vincent, P. A., and Dai, G. (2014b). Generation of multi-scale vascular network system within 3D hydrogel using 3D bio-printing technology. Cell. Mol. Bioeng. 7, 460–472. doi:10.1007/s12195-014-0340-0
Li, H., Chen, L., Zhang, M., Tang, S., and Fu, X. (2013). Three-dimensional culture and identification of human eccrine sweat glands in matrigel basement membrane matrix. Cell Tissue Res. 354, 897–902. doi:10.1007/s00441-013-1718-3
Li, Y., and Kilian, K. A. (2015). Bridging the gap: from 2D cell culture to 3D microengineered extracellular matrices. Adv. Healthc. Mater. 4, 2780–2796. doi:10.1002/adhm.201500427
Lim, H. Y., Kim, J., Song, H. J., Kim, K., Choi, K. C., Park, S., et al. (2018). Development of wrinkled skin-on-a-chip (WSOC) by cyclic uniaxial stretching. J. Industrial Eng. Chem. 68, 238–245. doi:10.1016/j.jiec.2018.07.050
Lin, H. Y., Chen, S. H., Chang, S. H., and Huang, S. T. (2015). Tri-layered chitosan scaffold as a potential skin substitute. J. Biomaterials Sci. Polym. Ed. 26, 855–867. doi:10.1080/09205063.2015.1061350
Linke, K., Schanz, J., Hansmann, J., Walles, T., Brunner, H., and Mertsching, H. (2007). Engineered liver-like tissue on a capillarized matrix for applied research. Tissue Eng. 13, 2699–2707. doi:10.1089/ten.2006.0388
Luo, Z., Che, J., Sun, L., Yang, L., Zu, Y., Wang, H., et al. (2021). Microfluidic electrospray photo-crosslinkable κ-Carrageenan microparticles for wound healing. Eng. Regen. 2, 257–262. doi:10.1016/j.engreg.2021.10.002
Ma, J., Wang, Y., and Liu, J. (2018). Bioprinting of 3D tissues/organs combined with microfluidics. RSC Adv. 8, 21712–21727. doi:10.1039/c8ra03022g
MacAdam, A., Chaudry, E., McTiernan, C. D., Cortes, D., Suuronen, E. J., and Alarcon, E. I. (2022). Development of in situ bioprinting: a mini review. Front. Bioeng. Biotechnol. 10, 940896. doi:10.3389/fbioe.2022.940896
MacNeil, S. (2007). Progress and opportunities for tissue-engineered skin. Nature 445 (7130), 874–880. doi:10.1038/nature05664
Mahmoudi, Z., Sedighi, M., Jafari, A., Naghieh, S., Stefanek, E., Akbari, M., et al. (2023). In situ 3D bioprinting: a promising technique in advanced biofabrication strategies. Bioprinting 31, e00260. doi:10.1016/j.bprint.2023.e00260
Mansbridge, J. (2020). Tissue-engineered skin products. Princ. Tissue Eng., 1483–1497. doi:10.1016/B978-0-12-818422-6.00081-2
Marino, D., Luginbühl, J., Scola, S., Meuli, M., and Reichmann, E. (2014). Bioengineering dermo-epidermal skin grafts with blood and lymphatic capillaries. Sci. Transl. Med. 6, 221ra14. doi:10.1126/scitranslmed.3006894
Martorina, F., Casale, C., Urciuolo, F., Netti, P. A., and Imparato, G. (2017). In vitro activation of the neuro-transduction mechanism in sensitive organotypic human skin model. Biomaterials 113, 217–229. doi:10.1016/j.biomaterials.2016.10.051
Maschmeyer, I., Lorenz, A. K., Schimek, K., Hasenberg, T., Ramme, A. P., Hübner, J., et al. (2015). A four-organ-chip for interconnected long-term co-culture of human intestine, liver, skin and kidney equivalents. Lab. Chip 15, 2688–2699. doi:10.1039/c5lc00392j
Matai, I., Kaur, G., Seyedsalehi, A., McClinton, A., and Laurencin, C. T. (2020). Progress in 3D bioprinting technology for tissue/organ regenerative engineering. Biomaterials 226, 119536. doi:10.1016/j.biomaterials.2019.119536
Maurmann, N., França, F. S., Girón, J., and Pranke, P. (2023). Cell electrospinning: a review of materials and methodologies for biofabrication. Adv. Biol. 7, e2300058. doi:10.1002/adbi.202300058
Michael, S., Sorg, H., Peck, C. T., Koch, L., Deiwick, A., Chichkov, B., et al. (2013). Tissue engineered skin substitutes created by laser-assisted bioprinting form skin-like structures in the dorsal skin fold chamber in mice. PLoS One 8, e57741. doi:10.1371/journal.pone.0057741
Mohamadali, M., Ghiaseddin, A., Irani, S., Amirkhani, M. A., and Dahmardehei, M. (2023). Design and evaluation of a skin-on-a-chip pumpless microfluidic device. Sci. Rep. 13, 8861. doi:10.1038/s41598-023-34796-3
Monfort, A., Soriano-Navarro, M., García-Verdugo, J. M., and Izeta, A. (2013). Production of human tissue-engineered skin trilayer on a plasma-based hypodermis. J. Tissue Eng. Regen. Med. 7, 479–490. doi:10.1002/term.548
Mori, N., Morimoto, Y., and Takeuchi, S. (2017). Skin integrated with perfusable vascular channels on a chip. Biomaterials 116, 48–56. doi:10.1016/j.biomaterials.2016.11.031
Murphy, S. V., and Atala, A. (2014). 3D bioprinting of tissues and organs. Nat. Biotechnol. 32 (8), 773–785. doi:10.1038/nbt.2958
Ng, W. L., Qi, J. T. Z., Yeong, W. Y., and Naing, M. W. (2018). Proof-of-concept: 3D bioprinting of pigmented human skin constructs. Biofabrication 10, 025005. doi:10.1088/1758-5090/aa9e1e
Nosoudi, N., Hasanzadeh, A., Hart, M., and Weaver, B. (2023). Advancements and future perspectives in cell electrospinning and bio-electrospraying. Adv. Biol. 7, e2300213. doi:10.1002/adbi.202300213
O’Neill, A. T., Monteiro-Riviere, N. A., and Walker, G. M. (2008). Characterization of microfluidic human epidermal keratinocyte culture. Cytotechnology 56, 197–207. doi:10.1007/s10616-008-9149-9
O’Neill, J. J., Johnson, R. A., Dockter, R. L., and Kowalewski, T. M. (2017). “3D bioprinting directly onto moving human anatomy,” in IEEE International Conference on Intelligent Robots and Systems, Vancouver, BC, Canada, 24-28 September 2017, 934–940.
Oualla-Bachiri, W., Fernández-González, A., Quiñones-Vico, M. I., and Arias-Santiago, S. (2020). From grafts to human bioengineered vascularized skin substitutes. Int. J. Mol. Sci. 21, 8197. doi:10.3390/ijms21218197
Ouyang, L., Armstrong, J. P. K., Chen, Q., Lin, Y., and Stevens, M. M. (2020). Void-free 3D bioprinting for in situ endothelialization and microfluidic perfusion. Adv. Funct. Mater. 30, 1908349. doi:10.1002/adfm.201908349
Pappalardo, A., Alvarez Cespedes, D., Fang, S., Herschman, A. R., Jeon, E. Y., Myers, K. M., et al. (2023). Engineering edgeless human skin with enhanced biomechanical properties. Sci. Adv. 9, eade2514. doi:10.1126/sciadv.ade2514
Parhizkar, M., Reardon, P., Knowles, J., Browning, R., Stride, E., Pedley, R., et al. (2017). Performance of novel high throughput multi electrospray systems for forming of polymeric micro/nanoparticles. Mater. Des. 126, 73–84. doi:10.1016/j.matdes.2017.04.029
Park, J. A., Lee, H. R., Park, S. Y., and Jung, S. (2020). Self-organization of fibroblast-laden 3D collagen microstructures from inkjet-printed cell patterns. Adv. Biosyst. 4, 1900280. doi:10.1002/adbi.201900280
Park, Y. J., and Lee, H. K. (2017). The role of skin and orogenital microbiota in protective immunity and chronic immune-mediated inflammatory disease. Front. Immunol. 8, 1955. doi:10.3389/fimmu.2017.01955
Peelen, D. M., Hoogduijn, M. J., Hesselink, D. A., and Baan, C. C. (2021). Advanced in vitro research models to study the role of endothelial cells in solid organ transplantation. Front. Immunol. 12, 607953. doi:10.3389/fimmu.2021.607953
Phua, Q. H., Han, H. A., and Soh, B. S. (2021). Translational stem cell therapy: vascularized skin grafts in skin repair and regeneration. J. Transl. Med. 19, 83–11. doi:10.1186/s12967-021-02752-2
Pourchet, L. J., Thepot, A., Albouy, M., Courtial, E. J., Boher, A., Blum, L. J., et al. (2017). Human skin 3D bioprinting using scaffold-free approach. Adv. Healthc. Mater. 6. doi:10.1002/adhm.201601101
Przekora, A. (2020). A concise review on tissue engineered artificial skin grafts for chronic wound treatment: can we reconstruct functional skin tissue in vitro? Cells 9, 1622. doi:10.3390/cells9071622
Pugliese, R., and Regondi, S. (2022). Artificial intelligence-empowered 3D and 4D printing technologies toward smarter biomedical materials and approaches. Polymers 14, 2794. doi:10.3390/polym14142794
Qian, Y., Zhang, Z., Zheng, L., Song, R., and Zhao, Y. (2014). Fabrication and characterization of electrospun polycaprolactone blended with chitosan-gelatin complex nanofibrous mats. J. Nanomater. 2014, 1–7. doi:10.1155/2014/964621
Quílez, C., de Aranda Izuzquiza, G., García, M., López, V., Montero, A., Valencia, L., et al. (2020). Bioprinting for skin. Methods Mol. Biol. 2140, 217–228. doi:10.1007/978-1-0716-0520-2_14
Raj M, K., and Chakraborty, S. (2020). PDMS microfluidics: a mini review. J. Appl. Polym. Sci. 137, 48958. doi:10.1002/app.48958
Ramadan, Q., and Ting, F. C. W. (2016). In vitro micro-physiological immune-competent model of the human skin. Lab. Chip 16, 1899–1908. doi:10.1039/c6lc00229c
Rama Varma, A., and Fathi, P. (2023). Vascularized microfluidic models of major organ structures and cancerous tissues. Biomicrofluidics 17, 061502. doi:10.1063/5.0159800
Randall, M. J., Jüngel, A., Rimann, M., and Wuertz-Kozak, K. (2018). Advances in the biofabrication of 3D skin in vitro: healthy and pathological models. Front. Bioeng. Biotechnol. 6, 154. doi:10.3389/fbioe.2018.00154
Richard, C., Neild, A., Cadarso, V. J., Cadarso, V. J., and Cadarso, V. J. (2020). The emerging role of microfluidics in multi-material 3D bioprinting. Lab. Chip 20, 2044–2056. doi:10.1039/c9lc01184f
Rimal, R., Marquardt, Y., Nevolianis, T., Djeljadini, S., Marquez, A. B., Huth, S., et al. (2021). Dynamic flow enables long-term maintenance of 3-D vascularized human skin models. Appl. Mater. Today 25, 101213. doi:10.1016/j.apmt.2021.101213
Risueño, I., Valencia, L., Jorcano, J. L., and Velasco, D. (2021). Skin-on-a-chip models: general overview and future perspectives. Apl. Bioeng. 5, 030901. doi:10.1063/5.0046376
Rothbauer, M., Eilenberger, C., Spitz, S., Bachmann, B. E. M., Kratz, S. R. A., Reihs, E. I., et al. (2022). Recent advances in additive manufacturing and 3D bioprinting for organs-on-A-chip and microphysiological systems. Front. Bioeng. Biotechnol. 10, 837087. doi:10.3389/fbioe.2022.837087
Saglam-Metiner, P., Gulce-Iz, S., and Biray-Avci, C. (2019). Bioengineering-inspired three-dimensional culture systems: organoids to create tumor microenvironment. Gene 686, 203–212. doi:10.1016/j.gene.2018.11.058
Salameh, S., Tissot, N., Cache, K., Lima, J., Suzuki, I., Marinho, P. A., et al. (2021). A perfusable vascularized full-thickness skin model for potential topical and systemic applications. Biofabrication 13, 035042. doi:10.1088/1758-5090/abfca8
Savoji, H., Godau, B., Hassani, M. S., and Akbari, M. (2018). Skin tissue substitutes and biomaterial risk assessment and testing. Front. Bioeng. Biotechnol. 6, 86. doi:10.3389/fbioe.2018.00086
Scott, S., and Ali, Z. (2021). Fabrication methods for microfluidic devices: an overview. Micromachines (Basel) 12, 319. doi:10.3390/mi12030319
Seol, Y. J., Lee, H., Copus, J. S., Kang, H. W., Cho, D. W., Atala, A., et al. (2018). 3D bioprinted biomask for facial skin reconstruction. Bioprinting 10, e00028. doi:10.1016/j.bprint.2018.e00028
Serebrakian, A. T., Pickrell, B. B., Varon, D. E., Mohamadi, A., Grinstaff, M. W., Rodriguez, E. K., et al. (2018). Meta-Analysis and systematic review of skin graft donor-site dressings with future guidelines. Plastic Reconstr. Surg. - Glob. Open 6, e1928. doi:10.1097/GOX.0000000000001928
Shacham, E., Loux, T., Barnidge, E. K., Lew, D., and Pappaterra, L. (2018). Determinants of organ donation registration. Am. J. Transplant. 18, 2798–2803. doi:10.1111/ajt.15025
Shepherd, J., Douglas, I., Rimmer, S., Swanson, L., and MacNeil, S. (2009). Development of three-dimensional tissue-engineered models of bacterial infected human skin wounds. Tissue Eng. Part C. Methods 15, 475–484. doi:10.1089/ten.tec.2008.0614
Si, Y., Shi, S., and Hu, J. (2023). Applications of electrospinning in human health: from detection, protection, regulation to reconstruction. Nano Today 48, 101723. doi:10.1016/j.nantod.2022.101723
Sierra-Sánchez, Á., Kim, K. H., Blasco-Morente, G., and Arias-Santiago, S. (2021). Cellular human tissue-engineered skin substitutes investigated for deep and difficult to heal injuries. npj Regen. Med. 6, 35. doi:10.1038/s41536-021-00144-0
Singh, S., Choudhury, D., Yu, F., Mironov, V., and Naing, M. W. (2020). In situ bioprinting – bioprinting from benchside to bedside? Acta Biomater. 101, 14–25. doi:10.1016/j.actbio.2019.08.045
Sofokleous, P., Stride, E., Bonfield, W., and Edirisinghe, M. (2013). Design, construction and performance of a portable handheld electrohydrodynamic multi-needle spray gun for biomedical applications. Mater. Sci. Eng. C 33, 213–223. doi:10.1016/j.msec.2012.08.033
Song, H. J., Lim, H. Y., Chun, W., Choi, K. C., Lee, T. Y., Sung, J. H., et al. (2018). Development of 3D skin-equivalent in a pump-less microfluidic chip. J. Industrial Eng. Chem. 60, 355–359. doi:10.1016/j.jiec.2017.11.022
Sriram, G., Alberti, M., Dancik, Y., Wu, B., Wu, R., Feng, Z., et al. (2018). Full-thickness human skin-on-chip with enhanced epidermal morphogenesis and barrier function. Mater. Today 21, 326–340. doi:10.1016/j.mattod.2017.11.002
Sun, W., Liu, Z., Xu, J., Cheng, Y., Yin, R., Ma, L., et al. (2023). 3D skin models along with skin-on-a-chip systems: a critical review. Chin. Chem. Lett. 34, 107819. doi:10.1016/j.cclet.2022.107819
Supp, D. M., Hahn, J. M., Lloyd, C. M., Combs, K. A., Swope, V. B., Abdel-Malek, Z., et al. (2020). Light or dark pigmentation of engineered skin substitutes containing melanocytes protects against ultraviolet light-induced DNA damage in vivo. J. Burn Care and Res. 41, 751–760. doi:10.1093/jbcr/iraa029
Sutterby, E., Thurgood, P., Baratchi, S., Khoshmanesh, K., and Pirogova, E. (2020). Microfluidic skin-on-a-chip models: toward biomimetic artificial skin. Small 16, e2002515. doi:10.1002/smll.202002515
Tarassoli, S. P., Jessop, Z. M., Al-Sabah, A., Gao, N., Whitaker, S., Doak, S., et al. (2018). Skin tissue engineering using 3D bioprinting: an evolving research field. J. Plastic, Reconstr. Aesthetic Surg. 71, 615–623. doi:10.1016/j.bjps.2017.12.006
Trottier, V., Marceau-Fortier, G., Germain, L., Vincent, C., and Fradette, J. (2008). IFATS collection: using human adipose-derived stem/stromal cells for the production of new skin substitutes. Stem Cells 26, 2713–2723. doi:10.1634/stemcells.2008-0031
Urciuolo, F., Casale, C., Imparato, G., and Netti, P. A. (2019). Bioengineered skin substitutes: the role of extracellular matrix and vascularization in the healing of deep wounds. J. Clin. Med. 8, 2083. doi:10.3390/jcm8122083
Varkey, M., Visscher, D. O., Van Zuijlen, P. P. M., Atala, A., and Yoo, J. J. (2019). Skin bioprinting: the future of burn wound reconstruction? Burns Trauma 7, 4. doi:10.1186/s41038-019-0142-7
Vecin, N. M., and Kirsner, R. S. (2023). Skin substitutes as treatment for chronic wounds: current and future directions. Front. Med. (Lausanne) 10, 1154567. doi:10.3389/fmed.2023.1154567
Vermette, M., Trottier, V., Menard, V., Saintpierre, L., Roy, A., and Fradette, J. (2007). Production of a new tissue-engineered adipose substitute from human adipose-derived stromal cells. Biomaterials 28, 2850–2860. doi:10.1016/j.biomaterials.2007.02.030
Vig, K., Chaudhari, A., Tripathi, S., Dixit, S., Sahu, R., Pillai, S., et al. (2017). Advances in skin regeneration using tissue engineering. Int. J. Mol. Sci. 18, 789. doi:10.3390/ijms18040789
Wagner, I., Materne, E. M., Brincker, S., Süßbier, U., Frädrich, C., Busek, M., et al. (2013). A dynamic multi-organ-chip for long-term cultivation and substance testing proven by 3D human liver and skin tissue co-culture. Lab. Chip 13, 3538. doi:10.1039/c3lc50234a
Wang, C., Li, W., Zhang, Z., Lei, D., Che, G., Gou, C., et al. (2024). A novel iron sulfide phase with remarkable hydroxyl radical generation capability for contaminants degradation. Water Res. 251, 121166. doi:10.1016/j.watres.2024.121166
Wang, L., Meece, K., Williams, D. J., Lo, K. A., Zimmer, M., Heinrich, G., et al. (2015). Differentiation of hypothalamic-like neurons from human pluripotent stem cells. J. Clin. Invest. 125, 796–808. doi:10.1172/jci79220
Wang, X., and Liu, C. (2018). 3D bioprinting of adipose-derived stem cells for organ manufacturing. Adv. Exp. Med. Biol. 1078, 3–14. doi:10.1007/978-981-13-0950-2_1
Wang, X., Wang, X., Liu, J., Cai, T., Guo, L., Wang, S., et al. (2016). Hair follicle and sebaceous gland de novo regeneration with cultured epidermal stem cells and skin-derived precursors. Stem Cells Transl. Med. 5, 1695–1706. doi:10.5966/sctm.2015-0397
Wang, X., Yang, C., Yu, Y., and Zhao, Y. (2022b). In situ 3D bioprinting living photosynthetic scaffolds for autotrophic wound healing. Research 2022, 9794745. doi:10.34133/2022/9794745
Wang, Y., Yuan, X., Yao, B., Zhu, S., Zhu, P., and Huang, S. (2022a). Tailoring bioinks of extrusion-based bioprinting for cutaneous wound healing. Bioact. Mater. 17, 178–194. doi:10.1016/j.bioactmat.2022.01.024
Wang, Z., and Cui, W. (2021). Two sides of electrospun fiber in promoting and inhibiting biomedical processes. Adv. Ther. 4. doi:10.1002/adtp.202000096
Wei, C., Feng, Y., Che, D., Zhang, J., Zhou, X., Shi, Y., et al. (2022). Biomaterials in skin tissue engineering. Int. J. Polym. Mater. Polym. Biomaterials 71, 993–1011. doi:10.1080/00914037.2021.1933977
Weinhart, M., Hocke, A., Hippenstiel, S., Kurreck, J., and Hedtrich, S. (2019). 3D organ models—revolution in pharmacological research? Pharmacol. Res. 139, 446–451. doi:10.1016/j.phrs.2018.11.002
Weng, T., Wu, P., Zhang, W., Zheng, Y., Li, Q., Jin, R., et al. (2020). Regeneration of skin appendages and nerves: current status and further challenges. J. Transl. Med. 18 (1), 53–17. doi:10.1186/s12967-020-02248-5
Weng, T., Zhang, W., Xia, Y., Wu, P., Yang, M., Jin, R., et al. (2021a). 3D bioprinting for skin tissue engineering: current status and perspectives. J. Tissue Eng. 12, 204173142110285. doi:10.1177/20417314211028574
Weng, T., Zhang, W., Xia, Y., Wu, P., Yang, M., Jin, R., et al. (2021b). 3D bioprinting for skin tissue engineering: current status and perspectives. J. Tissue Eng. 12, 204173142110285. doi:10.1177/20417314211028574
Wenzel, N., Blasczyk, R., and Figueiredo, C. (2021). Animal models in allogenic solid organ transplantation. Transplantology 2, 412–424. doi:10.3390/transplantology2040039
Wilm, M. (2011). Principles of electrospray ionization. Mol. Cell. Proteomics 10, M111.009407. doi:10.1074/mcp.M111.009407
Wu, Y., Qin, M., and Yang, X. (2023). Organ bioprinting: progress, challenges and outlook. J. Mat. Chem. B 11, 10263–10287. doi:10.1039/d3tb01630g
Wufuer, M., Lee, G., Hur, W., Jeon, B., Kim, B. J., Choi, T. H., et al. (2016). Skin-on-a-chip model simulating inflammation, edema and drug-based treatment. Sci. Rep. 6 (1), 37471–37512. doi:10.1038/srep37471
Xie, N., Shi, G., Shen, Y., Xu, Y., Wang, H., Feng, H., et al. (2022). Research progress of robot technology in in situ 3D bioprinting. Int. J. Bioprinting 8, 614. doi:10.18063/ijb.v8i4.614
Xie, Z., Gao, M., Lobo, A. O., and Webster, T. J. (2020). 3D bioprinting in tissue engineering for medical applications: the classic and the hybrid. Polymers 12, 1717. doi:10.3390/polym12081717
Xu, H., Zhang, F., Wang, M., Lv, H., Yu, D. G., Liu, X., et al. (2022b). Electrospun hierarchical structural films for effective wound healing. Biomater. Adv. 136, 212795. doi:10.1016/j.bioadv.2022.212795
Xu, L., Liu, Y., Zhou, W., and Yu, D. (2022a). Electrospun medical sutures for wound healing: a review. Polymers 14, 1637. doi:10.3390/polym14091637
Xu, S., Lu, T., Yang, L., Luo, S., Wang, Z., and Ye, C. (2022c). In situ cell electrospun using a portable handheld electrospinning apparatus for the repair of wound healing in rats. Int. Wound J. 19, 1693–1704. doi:10.1111/iwj.13769
Yamamoto, T., Iwase, H., King, T. W., Hara, H., and Cooper, D. K. C. (2018). Skin xenotransplantation: historical review and clinical potential. Burns 44, 1738–1749. doi:10.1016/j.burns.2018.02.029
Yan, X., Yu, M., Ramakrishna, S., Russell, S. J., and Long, Y. Z. (2019). Advances in portable electrospinning devices for: in situ delivery of personalized wound care. Nanoscale 11, 19166–19178. doi:10.1039/c9nr02802a
Yanez, M., Rincon, J., Dones, A., De Maria, C., Gonzales, R., and Boland, T. (2015). In vivo assessment of printed microvasculature in a bilayer skin graft to treat full-thickness wounds. Tissue Eng. Part A 21, 224–233. doi:10.1089/ten.tea.2013.0561
Zamboni, W. C., Szebeni, J., Kozlov, S. V., Lucas, A. T., Piscitelli, J. A., and Dobrovolskaia, M. A. (2018). Animal models for analysis of immunological responses to nanomaterials: challenges and considerations. Adv. Drug Deliv. Rev. 136-137, 82–96. doi:10.1016/j.addr.2018.09.012
Zeng, Z., Guo, C., Lu, D., Geng, Z., Pei, D., and Yu, S. (2022). Polyphenol–metal functionalized hydrogel dressing with sustained release, antibacterial, and antioxidant properties for the potential treatment of chronic wounds. Macromol. Mater. Eng. 307, 2200262. doi:10.1002/mame.202200262
Zennifer, A., Subramanian, A., and Sethuraman, S. (2022). Design considerations of bioinks for laser bioprinting technique towards tissue regenerative applications. Bioprinting 27, e00205. doi:10.1016/j.bprint.2022.e00205
Zhang, B., Gao, L., Ma, L., Luo, Y., Yang, H., and Cui, Z. (2019). 3D bioprinting: a novel Avenue for manufacturing tissues and organs. Engineering 5, 777–794. doi:10.1016/j.eng.2019.03.009
Zhang, B., Montgomery, M., Chamberlain, M., Ogawa, S., Korolj, A., Pahnke, A., et al. (2016). Biodegradable scaffold with built-in vasculature for organ-on-a-chip engineering and direct surgical anastomosis. Nat. Mater. 15, 669–678. doi:10.1038/nmat4570
Zhang, H., Wang, Y., Zheng, Z., Wei, X., Chen, L., Wu, Y., et al. (2023). Strategies for improving the 3D printability of decellularized extracellular matrix bioink. Theranostics 13, 2562–2587. doi:10.7150/thno.81785
Zhang, J., Wei, X., Zeng, R., Xu, F., and Li, X. J. (2017). Stem cell culture and differentiation in microfluidic devices toward organ-on-a-chip. Future Sci. OA 3, 187–2056. doi:10.4155/fsoa-2016-0091
Zhao, L., Niu, L., Liang, H., Tan, H., Liu, C., and Zhu, F. (2017). PH and glucose dual-responsive injectable hydrogels with insulin and fibroblasts as bioactive dressings for diabetic wound healing. ACS Appl. Mater Interfaces 9, 37563–37574. doi:10.1021/acsami.7b09395
Zhao, Y. T., Zhang, J., Gao, Y., Liu, X. F., Liu, J. J., Wang, X. X., et al. (2020). Self-powered portable melt electrospinning for in situ wound dressing. J. Nanobiotechnology 18, 111. doi:10.1186/s12951-020-00671-w
Zhou, F., Hong, Y., Liang, R., Zhang, X., Liao, Y., Jiang, D., et al. (2020). Rapid printing of bio-inspired 3D tissue constructs for skin regeneration. Biomaterials 258, 120287. doi:10.1016/j.biomaterials.2020.120287
Zhu, Z., Guo, S., Hirdler, T., Eide, C., Fan, X., Tolar, J., et al. (2018). 3D printed functional and biological materials on moving freeform surfaces. Adv. Mater. 30, 1707495. doi:10.1002/adma.201707495
Zhu, Z., Ng, D. W. H., Park, H. S., and McAlpine, M. C. (2020). 3D-printed multifunctional materials enabled by artificial-intelligence-assisted fabrication technologies. Nat. Rev. Mater. 6 (1), 27–47. doi:10.1038/s41578-020-00235-2
Zimoch, J., Zielinska, D., Michalak-Micka, K., Rütsche, D., Böni, R., Biedermann, T., et al. (2021). Bio-engineering a prevascularized human tri-layered skin substitute containing a hypodermis. Acta Biomater. 134, 215–227. doi:10.1016/j.actbio.2021.07.033
Keywords: tissue engineering, 3D bioprinting, microfluidics, bioengineering, microphysiological culture systems, microphysiological device design, skin, transplantation
Citation: Reed-McBain CA, Patel JD, Reed-McBain FLK, Al-Adra D, Virumbrales-Muñoz M and Ayuso JM (2024) Moving lab-grown tissues into the clinic: organ-on-a-chip and bioengineered skin systems. Front. Lab. Chip. Technol. 3:1383783. doi: 10.3389/frlct.2024.1383783
Received: 08 February 2024; Accepted: 08 April 2024;
Published: 30 April 2024.
Edited by:
Josep Samitier Martí, Institute for Bioengineering of Catalonia (IBEC), SpainReviewed by:
Suwan Jayasinghe, University College London, United KingdomCopyright © 2024 Reed-McBain, Patel, Reed-McBain, Al-Adra, Virumbrales-Muñoz and Ayuso. This is an open-access article distributed under the terms of the Creative Commons Attribution License (CC BY). The use, distribution or reproduction in other forums is permitted, provided the original author(s) and the copyright owner(s) are credited and that the original publication in this journal is cited, in accordance with accepted academic practice. No use, distribution or reproduction is permitted which does not comply with these terms.
*Correspondence: Jose M. Ayuso, YXl1c29kb21pbmd1QHdpc2MuZWR1
Disclaimer: All claims expressed in this article are solely those of the authors and do not necessarily represent those of their affiliated organizations, or those of the publisher, the editors and the reviewers. Any product that may be evaluated in this article or claim that may be made by its manufacturer is not guaranteed or endorsed by the publisher.
Research integrity at Frontiers
Learn more about the work of our research integrity team to safeguard the quality of each article we publish.