- Department of Biomedical Engineering, University of Connecticut, Storrs, CT, United States
Tissue chips have become one of the most potent research tools in the biomedical field. In contrast to conventional research methods, such as 2D cell culture and animal models, tissue chips more directly represent human physiological systems. This allows researchers to study therapeutic outcomes to a high degree of similarity to actual human subjects. Additionally, as rocket technology has advanced and become more accessible, researchers are using the unique properties offered by microgravity to meet specific challenges of modeling tissues on Earth; these include large organoids with sophisticated structures and models to better study aging and disease. This perspective explores the manufacturing and research applications of microgravity tissue chip technology, specifically investigating the musculoskeletal, cardiovascular, and nervous systems.
1 Introduction
Researchers have begun to transition from traditional biomedical research methods to tissue chips to better understand complexities of the human body. While conventional monolayer cell culture and animal models are effective in the initial study of a condition or cure, more intricate processes are required to truly predict how these factors will perform. Tissue chips house cells akin to their natural state, implementing three-dimensional (3D) culture, semipermeable membranes, and mechanical stimulation to precisely model their typical environment (Yau et al., 2021; Rice et al., 2022). This allows researchers to accurately mimic the body and its pathologies, to better understand both diseases and possible therapeutics (Low and Tagle, 2016). These chips can incorporate microfluidic environments as well as controllable software that alters fluid flow and pressure to meticulously recreate the desired tissue (Bi et al., 2006; Yau et al., 2023a; Yau et al., 2023b).
In the past century, space exploration has greatly advanced at a low cost, as reusable spacecrafts and rocket performance improve (Mu et al., 2022a). Accordingly, microgravity has become much more accessible.
Microgravity simulators do exist on Earth. Experimental platforms such as 2D clinostats, rotating wall vessels, and random positioning machines attempt to reproduce the effects observed in true microgravity (Herranz et al., 2013). Interpreting data from these simulators can at times prove complex and erroneous (Ferranti et al., 2020a; Silvani et al., 2022) Another alternative are aircrafts that fly in parabolic maneuvers, creating true zero gravity conditions, albeit for less than 30 s at a time (Zheng et al., 2017; Shelhamer, 2016; Wang et al., 2015). However, these on-Earth simulations greatly reduce the cost and time associated with microgravity studies in orbit. Overall, these simulated microgravity conditions appear to recreate similar conditions to what’s experienced in space (Wuest et al., 2015). More studies are necessary to identify possible differences in true and simulated microgravity.
This perspective addresses the advantages and limitations of microgravityand their impact on the ability of models to properly mimic in-vivo conditions. Current research on the musculoskeletal, cardiovascular, and nervous systems is discussed and insights are provided on how implementation in microgravity could provide improved results.
2 Unique properties of space
A lack of gravity causes cells to rapidly adapt, impacting a variety of characteristics; in-vitro tissues form more sophisticated and organized structures, similar to those seen in-vivo (Chang and Hughes-Fulford, 2009). 3D cell aggregates, known as spheroids, thus may benefit from utilization in space. Spheroids mimic the natural spatial architecture of certain tissues, increasing cell-cell interactions and the intricacy of structures compared to conventional 2D cultures (Juarez-Moreno et al., 2022; Kadletz et al., 2015). While spheroids have been proven as effective models, they are not simple to produce. To alleviate these difficulties, some have turned to microgravity. Various cells exposed to microgravity saw an increase in cellular aggregation, passively improving the formation of both tissue and tumor spheroids (Masiello et al., 2014; Grimm et al., 2018; Aleshcheva et al., 2016; Buken et al., 2019).
Microgravity expedites both the fabrication time and the modeled disease progression of tissue chips. One cause is the altered differentiation and proliferation of stem cells in microgravity. Human induced pluripotent stem cells cultured on the International Space Station (ISS) for 6 weeks showed a modified expression of 2,000 genes compared to their Earth-cultured counterparts (Giulianotti and Low, 2019). This modified differences can prove beneficial for researchers; Hagiwara et al. found that endothelial progenitor cells exposed to microgravity show greater angiogenic potential, conceivably quickening the vascularization of future tissue chips (Hagiwara et al., 2018). Various other groups observed that mesenchymal stem cells are guided towards an osteogenic phenotype in space without the need for cytokines, accelerating the fabrication of bone tissue chips (Gambacurta et al., 2019; Cazzaniga et al., 2016) Cardiac tissue modeling has also shown potential; in addition to enhanced angiogenesis, the proliferation and differentiation of cardiomyocytes has been observed in microgravity (Jha et al., 2016). Similarly, microgravity bolsters proliferation, survival, and shortened cell cycles in neural crest stem cells (Han et al., 2021) while stimulating neuroprotective effects in mesenchymal stem cells (Otsuka et al., 2018).
Microgravity additionally eliminates loading force. In orbit, astronauts lose cartilage, muscle, and bone mass due to the drastic decrease in mechanical stimuli (Ganse et al., 2022; Comfort et al., 2021; Mu et al., 2022a; Grimm et al., 2016; Willey et al., 2011). For instance, bone cells are mechanosensory, sensing and relaying mechanical signals. During load-induced strain, these cells release signaling molecules (Wang et al., 2021), inducing either formation or resorption (Juhl et al., 2021; Man et al., 2022). Without sufficient loading, bone tissue will be catabolized (NIAMS, 2017). These effects, along with the others listed, are summarized in Table 1:
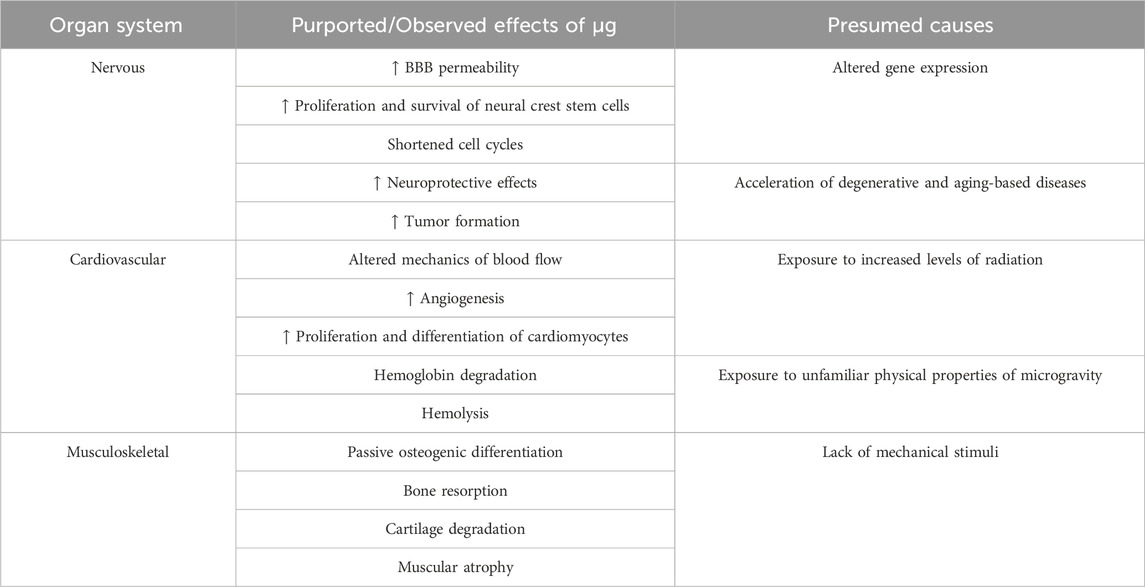
TABLE 1. This table showcases the multitude of effects that befall the human body in microgravity. The musculoskeletal system is exposed to a complete lack of mechanical stimuli, causing muscle atrophy, cartilage degradation, and bone resorption. Further, the blood brain barrier undergoes an increase in permeability, compromising the integrity of the brain’s primary protective system. Microgravity also accelerates tumor formation. In the heart, it provides modified mechanisms of blood flow in addition to hemoglobin degradation and hemolysis. Overall, these results are due to the altered gene expression, acceleration of disease, exposure to radiation, and exposure to the physical properties of microgravity in space.
While these loading-related diseases can be studied on Earth, the methods to cause these catabolic effects in models are typically limited to inducing the downstream chemical signaling seen in mechanotransduction. However, many of these signaling pathways are not well understood. A single stimulus may cause the release of a multitude of factors. Even then, the dose and frequency of downstream signaling molecules must be greatly scrutinized to determine their similarity to real disease conditions. Microgravity, however, offers the ability to directly alter the physical stimulus that causes these downstream effects. Instead of comprehensively mapping each pathway, one can directly cause the lack of loading that induces this broad signaling naturally. Thus, microgravity provides a rigorous testing environment for therapies designed to fight against these conditions, offering a streamlined ability to evaluate possible therapeutics.
To take full advantage of these benefits, tissue chips must be optimally designed prior to their journey into LEO. Due to the limited amount of current research done in space, anticipating design issues, and correcting them when they occur, is a challenging endeavor. Computer-aided design (CAD) has the potential to steer clear of these limitations; CAD allows researchers to increase the intricacies of their chip design, perform extremely accurate measurements, and diversify methods of fabrication (Tsur, 2020). These factors could be utilized to develop improved tissue models for microgravity-based research before they even have to get in a rocket. In a similar vein, machine learning models can also be trained to review each study, developing new and improved methods to fine tune previous ideas. As more information is gathered, more will be understood by these models, resulting in continuously optimized tissue chip designs for use in microgravity.
In addition to its plentiful biological applications, researchers can also take advantage of microgravity’s physical properties to improve the fabrication of tissue chips. Due to the lack of gravity, forces such as convection and sedimentation do not occur in LEO. This allows polymeric tissue scaffolds formed in solution, such as hydrogels, to develop a much more homogeneous structure. This enhanced architecture should, in theory, promote higher levels of cell adhesion and interaction.
3 Advantages of microgravity
This review will focus primarily on how space may improve musculoskeletal, cardiovascular, and nervous system tissue chips. As of now, models of these systems can take the most advantage of microgravity.
3.1 Musculoskeletal system
The musculoskeletal system is crucial to support our body and preserve mobility. It comprises bone, muscle, cartilage, along with other specialized connective tissues. Musculoskeletal diseases, like osteoporosis and osteoarthritis, can be especially burdensome, as they directly impact one’s ability to be active.
Bone is a dynamic and complex tissue, constantly adapting to external mechanical stimuli; moderate loading will strengthen bone, but inadequate loading will diminish it (Rowe et al., 2022). To study this phenomenon, Paek et al. developed an effective high-throughput bone-on-a-chip platform to mimic the structure of an osteon, a functional unit of bone, to act as a drug screening platform for osteoporosis (Paek et al., 2023). The presence of sclerostin, in which causes bone resorption, was used to assess drug efficacy. While the therapeutic did decrease sclerostin concentration, the chip itself did not truly model a diseased state. Thus, it is unclear if the drug would be therapeutically effective. If taken to space, microgravity could recapitulate the onset of osteoporosis; additionally, the physical properties could generate a more homogeneous osteon structure. Similarly, Galvan et al. developed a tissue chip to model partially developed bone tissue (Galván-Chacón et al., 2022). The passive osteogenic differentiation and increased cellular arrangement could vastly improve their model. These theoretical approaches have been verified by animal models in LEO. Coulombe et al. confirmed the acceleration of osteoporosis in space, even observing the intricacies of osteoporotic development, like varying levels of bone loss depending on the skeletal maturity of the animal (Coulombe et al., 2021).
Chondral breakdown in LEO has also been observed in mice (Kwok et al., 2021). Some scientists have already taken advantage of this with novel tissue models. Grodzinsky et al. utilized microgravity to study inflammation-related interactions in a cartilage-bone-synovium model (Dwivedi et al., 2022; Low and Giulianotti, 2019a). Shi et al. and van Loo et al., may benefit from the influenced cell aggregation in LEO to further enhance their spheroid cultures to study hypoxic chondrocytes and overall cartilage formation, respectively (Shi et al., 2015; van Loo et al., 2024).
Skeletal muscle has similar potential in microgravity. On Earth, Ortega et al. designed a muscle-on-a-chip to monitor two factors lead to muscle atrophy (Ortega et al., 2019). Muscle atrophy is a common consequence of aging (Altun et al., 2010), resulting from immobilization and disuse (Bodine, 2013). By employing Ortega et al.‘s tissue chip in microgravity, scientists could observe how a lack of loading affects cellular signaling in muscle tissue, with real-time monitoring. This would eradicate the current need for ethically ambiguous animal models while providing invaluable information that even on-Earth tissue models may implement to accurately mimic atrophic progression. The effects of microgravity on skeletal muscle atrophy have already been well documented in tissue chips taken to the ISS (Parafati et al., 2023), astronauts in orbit (Lee et al., 2022), and LEO-based animal models (Okada et al., 2021). Interestingly, these effects remain with cells after their return to Earth (Takahashi et al., 2021). These models, along with other musculoskeletal chips, have potential to see the improvements outlined in Figure 1:
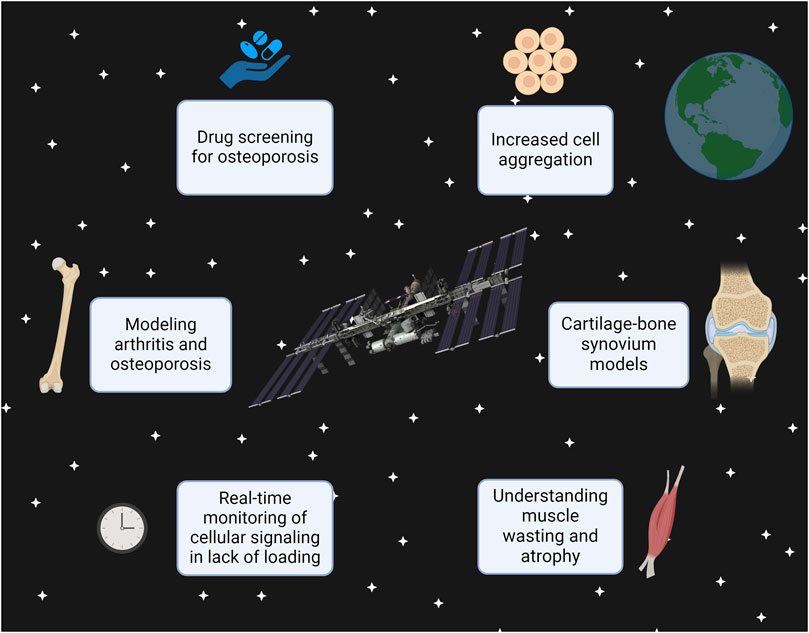
FIGURE 1. This figure demonstrates that future developments, such as using the 3D printer on the ISS, can allow scientists to manufacture tissue chips in space rather than Earth. The types of research that can be done in microgravity conditions are displayed, specifically using the musculoskeletal system as an example. This figure was created with Biorender.com.
3.2 Cardiovascular system
Cardiovascular diseases are a leading cause of mortality, affecting over 17 million people each year (Amini et al., 2021). As microgravity has promoted angiogenesis (Morbidelli et al., 2021; Shi et al., 2017), induced spheroid formation in endothelial cells (Dittrich et al., 2018), and expedited the differentiation of cardiac progenitors from pluripotent stem cells (Jha et al., 2016), it may provide an excellent environment for cardiac tissue modeling.
Figtree et al. (2017) cocultured cardiac myocytes, endothelial cells, and fibroblasts to study cardiac fibrosis in vascularized cardiac spheroids. While their model was successful, the increased cellular aggregation and angiogenic potential in microgravity would likely further strengthen their model. Additionally, adapting successful animal cardiac models (Liu et al., 2021; Chen et al., 2019) to microgravity-exposed tissue chips might provide a more repeatable, accurate method to study drug efficacy, especially as microgravity could accelerate disease progression (Lynch et al., 2007; Walls et al., 2020).
Vascular damage is another factor of cardiovascular disease that has been observed during zero gravity conditions. To understand these outcomes, large scale artery dynamics were modeled in simulated microgravity, providing key insights on their modified mechanics (Caddy et al., 2024).
Tang et al. created a heart-on-a-chip platform with induced pluripotent stem cells to evaluating therapeutics and study the importance of the endothelial layer. The aforementioned impacts on cell differentiation and organization observed in microgravity could assist this model even further in its accuracy (Tang et al., 2022). Collectively, cardiovascular tissue chips have not yet implemented microgravity as much as musculoskeletal models, yet they maintain a high degree of potential.
3.3 Nervous system
The nervous system grants us the ability to breathe, think, and move, making it uniquely devastating when this essential network fails. In 2019, 10 million died due to neurological disorders, with a burden of disease that continues to increase (Ding et al., 2022; Feigin et al., 2020). Thus, understanding its underlying processes are essential. To perform these investigations, tissue chips are employed as in-vitro blood-brain-barrier (BBB) models to develop permeable therapeutics and recreate malignant tumors.
Neurodegenerative diseases such as Alzheimer’s disease (AD), Parkinson’s disease (PD), and multiple sclerosis affect millions. These chronic conditions devastate both patients and their families (Praznikov Victor, 2022). Tissue chips offer an opportunity to better grasp the complexities of these diseases. Park et al. developed a microfluidic chip with 3D neurospheroids to precisely capture in-vivo conditions seen in AD, such as reduced viability and disrupted neural networks (Grimm et al., 2022). By promoting increased spheroid formation in microgravity (Grimm et al., 2020), this already effective model could be further perfected to model AD. Shin et al. similarly created a chip to model AD-associated BBB dysfunction, demonstrating how plaque deposition increases BBB permeability (Shin et al., 2019). In LEO, this model could experience increased cell-cell interaction, cell adhesion, and possible accelerated disease progression (Low and Giulianotti, 2019b; Yau et al., 2023b) to further improve their AD tissue chip design. The study of the BBB under microgravity has already been a topic of interest for scientists. In fact, Hinojosa et al. have sent their model of the BBB to the ISS to studying real-time cell-cell interactions (Reporter, 2020). In 2018, Leong et al. developed a microfluidic device for use in space to understand the relationship between the brain and vasculature, as well as the impact of neuropsychiatric drugs (Reporter, 2022).
Tsybko et al. studied how glial cell-derived neurotrophic factor (GDNF), which can promote the recovery of motor function in PD (Björklund et al., 1997), is affected in mouse brains exposed to simulated microgravity (Tsybko et al., 2015). This study also found that spaceflight had negative effects on the dopamine system, with dysregulation of genetic control over GDNF being a potential cause. This offers new insight to protect the health of astronauts, as well as new methods to create Parkinsons-on-a-chip models by using microgravity.
In addition to AD and PD, brain tumor models may benefit from microgravity. Glioblastomas are aggressive tumors with low life expectancies (Jovčevska, 2020). This level of importance makes them prime candidates for accelerated study in microgravity. To better model BBB permeability observed in glioblastomas, Silvani et al. used microgravity to decrease the expression of tight junction proteins, directly increasing permeability. This low gravity-induced permeability could also act therapeutically, temporarily allowing chemotherapeutics to cross the BBB (Silvani et al., 2021). Other brain tumor models are likely to benefit from the enhanced tumor formation and structural architecture seen in microgravity (Samiei et al., 2020).
Similar to Silvani et al.’s observations, microgravity has been seen to decrease the BBB’s tight junction proteins in mice. This acceleration of neurodegeneration is another concern for astronauts. A novel BBB-on-a-chip could be created to visualize how microgravity affects the brain via specific gene expression (Yan et al., 2021) and oxidative stress (Chen et al., 2009) previously observed in animal studies. Understanding the mechanism behind these spaceflight-induced physiological changes can allow scientists to develop possible solutions.
3.4 Physiological effects of microgravity
While microgravity has its benefits for furthering research, astronauts who spend significant amounts of time in LEO observe changes in their tissue functions. In this vein, tissue chips can be utilized to understand how prolonged exposure to microgravity impacts systems such as the musculoskeletal, cardiovascular, and nervous systems are impacted.
The aforementioned loss of musculoskeletal tissue likely arises from a loss of load, but it may be exacerbated by radiation (Hamilton et al., 2006).
This radiation has also been observed to increase oxidative stress and inflammation in the carotid arteries of astronauts (Lee et al., 2020) along with structural remodeling and fibrotic alterations of cardiac tissue (Xu et al., 2021). Tissue chips may be the ideal option to study these phenomena. This research may also prove beneficial on Earth, providing insights into the heart damage seen in thoracic cancer radiation therapy (Schaue and McBride, 2015).
Venous thrombosis (Auñón-Chancellor et al., 2020), anemia, and decreased hemoglobin concentration (Trudel et al., 2020; Nicogossian, 2003) have all been observed in astronauts on the ISS. Further haemodynamic studies, such as Caddy et al.’s, are necessary to resolve these issues (Caddy et al., 2024).Alternatively, the hemoglobin degradation and hemolysis (Trudel et al., 2022) can be utilized in tissue chips to evaluate mechanisms of hemolysis-causing diseases, such as malaria, or test possible therapies. Anemia also increases the susceptibility to infectious diseases like tuberculosis (Gelaw et al., 2021), providing a more rigorous testing apparatus if implemented.
Gray and white matter alterations, cognitive and motor ability reductions, and the development of neuro-optic syndrome have all been detected after spaceflight (Riascos et al., 2019; Marfia et al., 2022; Roberts et al., 2019). The study of brain organoids in microgravity is necessary to understand the impact of radiation on astrocytes and neural architectures, like the BBB, to avoid these outcomes (Roggan et al., 2023).
3.5 Limitations of research in zero gravity
While microgravity has the potential to further biomedical research in a new frontier, it must be scrutinized before it can achieve clinical relevance. The same changes in gene expression that benefit researchers, such as cell differentiation and proliferation, can be problematic when trying to apply results from drug studies to human subjects. A drug may be effective in microgravity when cells express certain genes, but when that same drug is implemented on Earth, it may not completely translate its efficacy. Perhaps a gene needed for the drug’s success was upregulated in microgravity but downregulated on Earth. Additionally, microgravity causes complex changes cytoskeleton organization (Wu et al., 2022). Some studies have shown that microgravity decreases expression of actin while others found the opposite to occur (Bradbury et al., 2020).
These observations also depend on cell type; in human neuroblastoma cells, microgravity showed microtubule bending but had no influence on actin dynamics (Rösner et al., 2006). In glioma cells, microgravity resulted in B-tubulin disorganization (Wang et al., 2016). However, cardiovascular progenitor cells cultured in space increased the expression of cytoskeleton genes (Baio et al., 2018).
Space research has striking potential in terms of tissue chip research, but the in-space changes of cells and disease progression must be investigated further before it can be accepted as a true replica of disease conditions on Earth. It is possible that exposure to microgravity itself could alter a disease’s mechanisms rather than only accelerating their progression. Also, in-space facilities have strict loading capacity and experimental limitations, perhaps leading to oversimplification, and therefore inaccuracy, of the tissue chip models on board (Mu et al., 2022b; Ferranti et al., 2020b). In addition, cosmic radiation may greatly impact the efficacy of these tissue chips (Li et al., 2018; Nwanaji-Enwerem et al., 2022; Soucy et al., 2011; Belli et al., 2002). Perhaps protective apparati could be developed to mitigate the exposure to this radiation, or at the very least attenuate it to a lesser degree. In all, these observations obtained from microgravity-exposed tissue chips require more time before they can be directly utilized in clinically relevant comparisons. They can, however, provide a foundational understanding, whose breadth will continue to expand over time.
4 Discussion
As tissue chip and space travel technologies advance, scientists can fully utilize the accelerated disease progression, cell aggregation, spheroid formation, and variation in genetic expression in microgravity to further biomedical research. These characteristics will lead to a deeper understanding of the mechanisms, signaling, and therapeutic possibilities for an array of conditions. This has begun for the musculoskeletal, cardiovascular, and nervous systems, and others will likely follow suit. In the future, it is likely microgravity will become ubiquitous in this field, providing researchers with passive methods to most accurately model disease and rigorously assess therapies. Through this investigation of accelerated disease conditions and unique properties, microgravity can aid us in creating effective and long-lasting therapies, using space to improve the quality of life for us here on Earth.
Data availability statement
The original contributions presented in the study are included in the article/supplementary material, further inquiries can be directed to the corresponding author.
Author contributions
AJ: Conceptualization, Investigation, Writing–original draft, Writing–review and editing. ML: Conceptualization, Investigation, Supervision, Writing–original draft, Writing–review and editing. YC: Conceptualization, Funding acquisition, Supervision, Validation, Visualization, Writing–review and editing.
Funding
The author(s) declare financial support was received for the research, authorship, and/or publication of this article. This study is supported by NIH 7R01AR072027, NIH 1R21AR079153-01A1, NSF 2025362, NSF 2234570, NASA 80JSC022CA006, DOD W81XWH2110274 and the University of Connecticut.
Acknowledgments
We acknowledge NIH, NSF, NASA, DOD, CASIS and the University of Connecticut for funding and support.
Conflict of interest
YC is a co-founder of Eascra Biotech, Inc.
The remaining authors declare that the research was conducted in the absence of any commercial or financial relationships that could be construed as a potential conflict of interest.
The author(s) declared that they were an editorial board member of Frontiers, at the time of submission. This had no impact on the peer review process and the final decision.
Publisher’s note
All claims expressed in this article are solely those of the authors and do not necessarily represent those of their affiliated organizations, or those of the publisher, the editors and the reviewers. Any product that may be evaluated in this article, or claim that may be made by its manufacturer, is not guaranteed or endorsed by the publisher.
References
Aleshcheva, G., Bauer, J., Hemmersbach, R., Slumstrup, L., Wehland, M., Infanger, M., et al. (2016). Scaffold-free tissue formation under real and simulated microgravity conditions. Basic and Clin. Pharmacol. Toxicol. 119 (Suppl. 3), 26–33. doi:10.1111/bcpt.12561
Altun, M., Besche, H. C., Overkleeft, H. S., Piccirillo, R., Edelmann, M. J., Kessler, B. M., et al. (2010). Muscle wasting in aged, sarcopenic rats is associated with enhanced activity of the ubiquitin proteasome pathway. J. Biol. Chem. 285, 39597–39608. doi:10.1074/jbc.M110.129718
Amini, M., Zayeri, F., and Salehi, M. (2021). Trend analysis of cardiovascular disease mortality, incidence, and mortality-to-incidence ratio: results from global burden of disease study 2017. BMC Public Health 21, 401. doi:10.1186/s12889-021-10429-0
Auñón-Chancellor, S. M., Pattarini, J. M., Moll, S., and Sargsyan, A. (2020). Venous thrombosis during spaceflight. N. Engl. J. Med. 382, 89–90. doi:10.1056/NEJMc1905875
Baio, J., Martinez, A. F., Silva, I., Hoehn, C. V., Countryman, S., Bailey, L., et al. (2018). Cardiovascular progenitor cells cultured aboard the International Space Station exhibit altered developmental and functional properties. npj Microgravity 4, 13. doi:10.1038/s41526-018-0048-x
Belli, M., Sapora, O., and Tabocchini, M. A. (2002). Molecular targets in cellular response to ionizing radiation and implications in space radiation protection. J. Radiat. Res. 43, S13–S19. doi:10.1269/jrr.43.S13
Bi, H., Meng, S., Li, Y., Guo, K., Chen, Y., Kong, J., et al. (2006). Deposition of PEG onto PMMA microchannel surface to minimize nonspecific adsorption. Lab. Chip 6, 769–775. doi:10.1039/B600326E
Björklund, A., Rosenblad, C., Winkler, C., and Kirik, D. (1997). Studies on neuroprotective and regenerative effects of GDNF in a partial lesion model of Parkinson's disease. Neurobiol. Dis. 4, 186–200. doi:10.1006/nbdi.1997.0151
Bodine, S. C. (2013). Disuse-induced muscle wasting. Int. J. Biochem. Cell Biol. 45, 2200–2208. doi:10.1016/j.biocel.2013.06.011
Bradbury, P., Wu, H., Choi, J. U., Rowan, A. E., Zhang, H., Poole, K., et al. (2020). Modeling the impact of microgravity at the cellular level: implications for human disease. Front. Cell Dev. Biol. 8, 96. doi:10.3389/fcell.2020.00096
Buken, C., Sahana, J., Corydon, T. J., Melnik, D., Bauer, J., Wehland, M., et al. (2019). Morphological and molecular changes in juvenile normal human fibroblasts exposed to simulated microgravity. Sci. Rep. 9, 11882. doi:10.1038/s41598-019-48378-9
Caddy, H. T., Kelsey, L. J., Parker, L. P., Green, D. J., and Doyle, B. J. (2024). Modelling large scale artery haemodynamics from the heart to the eye in response to simulated microgravity. npj Microgravity 10, 7. doi:10.1038/s41526-024-00348-w
Cazzaniga, A., Maier, J. A. M., and Castiglioni, S. (2016). Impact of simulated microgravity on human bone stem cells: new hints for space medicine. Biochem. Biophysical Res. Commun. 473, 181–186. doi:10.1016/j.bbrc.2016.03.075
Chang, T. T., and Hughes-Fulford, M. (2009). Monolayer and spheroid culture of human liver hepatocellular carcinoma cell line cells demonstrate distinct global gene expression patterns and functional phenotypes. Tissue Eng. Part A 15, 559–567. doi:10.1089/ten.tea.2007.0434
Chen, H., Qu, L., Li, Q., Bi, L., Huang, Z., and Li, Y. (2009). Simulated microgravity-induced oxidative stress in different areas of rat brain. Sheng li xue bao Acta Physiol. Sin. 61, 108–114.
Chen, P., Liu, J., Ruan, H., Zhang, M., Wu, P., Yimei, D., et al. (2019). Protective effects of Salidroside on cardiac function in mice with myocardial infarction. Sci. Rep. 9, 18127. doi:10.1038/s41598-019-54713-x
Comfort, P., McMahon, J. J., Jones, P. A., Cuthbert, M., Kendall, K., Lake, J. P., et al. (2021). Effects of spaceflight on musculoskeletal health: a systematic review and meta-analysis, considerations for interplanetary travel. Sports Med. 51, 2097–2114. doi:10.1007/s40279-021-01496-9
Coulombe, J. C., Sarazin, B. A., Mullen, Z., Ortega, A. M., Livingston, E. W., Bateman, T. A., et al. (2021). Microgravity-induced alterations of mouse bones are compartment- and site-specific and vary with age. Bone 151, 116021. doi:10.1016/j.bone.2021.116021
Ding, C., Wu, Y., Chen, X., Chen, Y., Wu, Z., Lin, Z., et al. (2022). Global, regional, and national burden and attributable risk factors of neurological disorders: the Global Burden of Disease study 1990–2019. Front. Public Health 10, 952161. doi:10.3389/fpubh.2022.952161
Dittrich, A., Grimm, D., Sahana, J., Bauer, J., Krüger, M., Infanger, M., et al. (2018). Key proteins involved in spheroid formation and angiogenesis in endothelial cells after long-term exposure to simulated microgravity. Cell. Physiology Biochem. 45, 429–445. doi:10.1159/000486920
Dwivedi, G., Flaman, L., Alaybeyoglu, B., Struglics, A., Frank, E. H., Chubinskya, S., et al. (2022). Inflammatory cytokines and mechanical injury induce post-traumatic osteoarthritis-like changes in a human cartilage-bone-synovium microphysiological system. Arthritis Res. Ther. 24, 198. doi:10.1186/s13075-022-02881-z
Feigin, V. L., Vos, T., Nichols, E., Owolabi, M. O., Carroll, W. M., Dichgans, M., et al. (2020). The global burden of neurological disorders: translating evidence into policy. Lancet Neurology 19, 255–265. doi:10.1016/S1474-4422(19)30411-9
Ferranti, F., Del Bianco, M., and Pacelli, C. (2020a). Advantages and limitations of current microgravity platforms for space biology research. Appl. Sci. 11, 68. doi:10.3390/app11010068
Ferranti, F., Del Bianco, M., and Pacelli, C. (2020b). Advantages and limitations of current microgravity platforms for space biology research. Appl. Sci. 11, 68. doi:10.3390/app11010068
Figtree, G. A., Bubb, K. J., Tang, O., Kizana, E., and Gentile, C. (2017). Vascularized cardiac spheroids as novel 3D in vitro models to study cardiac fibrosis. Cells Tissues Organs 204, 191–198. doi:10.1159/000477436
Galván-Chacón, V. P., Zampouka, A., Hesse, B., Bohner, M., Habibovic, P., and Barata, D. (2022). Bone-on-a-Chip: a microscale 3D biomimetic model to study bone regeneration. Adv. Eng. Mater 24, 2101467. doi:10.1002/adem.202101467
Gambacurta, A., Merlini, G., Ruggiero, C., Diedenhofen, G., Battista, N., Bari, M., et al. (2019). Human osteogenic differentiation in Space: proteomic and epigenetic clues to better understand osteoporosis. Sci. Rep. 9, 8343. doi:10.1038/s41598-019-44593-6
Ganse, B., Cucchiarini, M., and Madry, H. (2022). Joint cartilage in long-duration spaceflight. Biomedicines 10, 1356. doi:10.3390/biomedicines10061356
Gelaw, Y., Getaneh, Z., and Melku, M. (2021). Anemia as a risk factor for tuberculosis: a systematic review and meta-analysis. Environ. Health Prev. Med. 26, 13. doi:10.1186/s12199-020-00931-z
Giulianotti, M. A., and Low, L. A. (2019). Pharmaceutical research enabled through microgravity: perspectives on the use of the international space station U.S. National laboratory. Pharm. Res. 37, 1. doi:10.1007/s11095-019-2719-z
Grimm, D., Egli, M., Krüger, M., Riwaldt, S., Corydon, T. J., Kopp, S., et al. (2018). Tissue engineering under microgravity conditions-use of stem cells and specialized cells. Stem Cells Dev. 27, 787–804. doi:10.1089/scd.2017.0242
Grimm, D., Grosse, J., Wehland, M., Mann, V., Reseland, J. E., Sundaresan, A., et al. (2016). The impact of microgravity on bone in humans. Bone 87, 44–56. doi:10.1016/j.bone.2015.12.057
Grimm, D., Schulz, H., Krüger, M., Cortés-Sánchez, J. L., Egli, M., Kraus, A., et al. (2022). The fight against cancer by microgravity: the multicellular spheroid as a metastasis model. Int. J. Mol. Sci. 23, 3073. doi:10.3390/ijms23063073
Grimm, D., Wehland, M., Corydon, T. J., Richter, P., Prasad, B., Bauer, J., et al. (2020). The effects of microgravity on differentiation and cell growth in stem cells and cancer stem cells. Stem Cells Transl. Med. 9, 882–894. doi:10.1002/sctm.20-0084
Hagiwara, H., Higashibata, A., Ogawa, S., Kanazawa, S., Mizuno, H., and Tanaka, R. (2018). Effectiveness of endothelial progenitor cell culture under microgravity for improved angiogenic potential. Sci. Rep. 8, 14239–14242. doi:10.1038/s41598-018-32073-2
Hamilton, S. A., Pecaut, M. J., Gridley, D. S., Travis, N. D., Bandstra, E. R., Willey, J. S., et al. (2006). A murine model for bone loss from therapeutic and space-relevant sources of radiation. J. Appl. Physiology 101, 789–793. doi:10.1152/japplphysiol.01078.2005
Han, Y., Zeger, L., Tripathi, R., Egli, M., Ille, F., Lockowandt, C., et al. (2021). Molecular genetic analysis of neural stem cells after space flight and simulated microgravity on earth. Biotechnol. Bioeng. 118, 3832–3846. doi:10.1002/bit.27858
Herranz, R., Anken, R., Boonstra, J., Braun, M., Christianen, P. C. M., de Geest, M., et al. (2013). Ground-based facilities for simulation of microgravity: organism-specific recommendations for their use, and recommended terminology. Astrobiology 13, 1–17. doi:10.1089/ast.2012.0876
Jha, R., Wu, Q., Singh, M., Preininger, M. K., Han, P., Ding, G., et al. (2016). Simulated microgravity and 3D culture enhance induction, viability, proliferation and differentiation of cardiac progenitors from human pluripotent stem cells. Sci. Rep. 6, 30956. doi:10.1038/srep30956
Jovčevska, I. (2020). Next generation sequencing and machine learning technologies are painting the epigenetic portrait of glioblastoma. Front. Oncol. 10, 798. doi:10.3389/fonc.2020.00798
Juarez-Moreno, K., Chávez-García, D., Hirata, G., and Vazquez-Duhalt, R. (2022). Monolayer (2D) or spheroids (3D) cell cultures for nanotoxicological studies? Comparison of cytotoxicity and cell internalization of nanoparticles. Toxicol. Vitro 85, 105461. doi:10.1016/j.tiv.2022.105461
Juhl, O. J., Buettmann, E. G., Friedman, M. A., DeNapoli, R. C., Hoppock, G. A., and Donahue, H. J. (2021). Update on the effects of microgravity on the musculoskeletal system. npj Microgravity 7, 28. doi:10.1038/s41526-021-00158-4
Kadletz, L., Heiduschka, G., Domayer, J., Schmid, R., Enzenhofer, E., and Thurnher, D. (2015). Evaluation of spheroid head and neck squamous cell carcinoma cell models in comparison to monolayer cultures. Oncol. Lett. 10, 1281–1286. doi:10.3892/ol.2015.3487
Kwok, A. T., Mohamed, N. S., Plate, J. F., Yammani, R. R., Rosas, S., Bateman, T. A., et al. (2021). Spaceflight and hind limb unloading induces an arthritic phenotype in knee articular cartilage and menisci of rodents. Sci. Rep. 11, 10469. doi:10.1038/s41598-021-90010-2
Lee, P. H. U., Chung, M., Ren, Z., Mair, D. B., and Kim, D.-Ho (2022). Factors mediating spaceflight-induced skeletal muscle atrophy. Am. J. Physiology-Cell Physiology 322, C567–C580. doi:10.1152/ajpcell.00203.2021
Lee, S. M. C., Ribeiro, L. C., Martin, D. S., Zwart, S. R., Feiveson, A. H., Laurie, S. S., et al. (2020). Arterial structure and function during and after long-duration spaceflight. J. Appl. Physiology 129, 108–123. doi:10.1152/japplphysiol.00550.2019
Li, Z., Jella, K. K., Jaafar, L., Li, S., Park, S., Story, M. D., et al. (2018). Exposure to galactic cosmic radiation compromises DNA repair and increases the potential for oncogenic chromosomal rearrangement in bronchial epithelial cells. Sci. Rep. 8, 11038. doi:10.1038/s41598-018-29350-5
Liu, B., Li, L., Liu, G., Ding, W., Chang, W., Xu, T., et al. (2021). Baicalein attenuates cardiac hypertrophy in mice via suppressing oxidative stress and activating autophagy in cardiomyocytes. Acta Pharmacol. Sin. 42, 701–714. doi:10.1038/s41401-020-0496-1
Low, L. A., and Giulianotti, M. A. (2019a). Tissue chips in space: modeling human diseases in microgravity. Pharm. Res. 37, 8. doi:10.1007/s11095-019-2742-0
Low, L. A., and Giulianotti, M. A. (2019b). Tissue chips in space: modeling human diseases in microgravity. Pharm. Res. 37, 8. doi:10.1007/s11095-019-2742-0
Low, L. A., and Tagle, D. A. (2016). Tissue Chips to aid drug development and modeling for rare diseases. Expert Opin. Orphan Drugs 4, 1113–1121. doi:10.1080/21678707.2016.1244479
Lynch, G. S., Schertzer, J. D., and Ryall, J. G. (2007). Therapeutic approaches for muscle wasting disorders. Pharmacol. Ther. 113, 461–487. doi:10.1016/j.pharmthera.2006.11.004
Man, J., Graham, T., Squires-Donelly, G., and Laslett, A. L. (2022). The effects of microgravity on bone structure and function. npj Microgravity 8, 9. doi:10.1038/s41526-022-00194-8
Marfia, G., Navone, S. E., Guarnaccia, L., Campanella, R., Locatelli, M., Miozzo, M., et al. (2022). Space flight and central nervous system: friends or enemies? Challenges and opportunities for neuroscience and neuro-oncology. J. Neurosci. Res. 100, 1649–1663. doi:10.1002/jnr.25066
Masiello, M. G., Cucina, A., Proietti, S., Palombo, A., Coluccia, P., D’Anselmi, F., et al. (2014). Phenotypic switch induced by simulated microgravity on MDA-MB-231 breast cancer cells. BioMed Res. Int. 2014, 1–12. doi:10.1155/2014/652434
Morbidelli, L., Genah, S., and Cialdai, F. (2021). Effect of microgravity on endothelial cell function, angiogenesis, and vessel remodeling during wound healing. Front. Bioeng. Biotechnol. 9, 720091. doi:10.3389/fbioe.2021.720091
Mu, X., He, W., Rivera, V. A. M., De Alba, R. A. D., Newman, D. J., and Zhang, Y. S. (2022a). Small tissue chips with big opportunities for space medicine. Life Sci. Space Res. 35, 150–157. doi:10.1016/j.lssr.2022.09.002
Mu, X., He, W., Rivera, V. A. M., De Alba, R. A. D., Newman, D. J., and Zhang, Y. S. (2022b). Small tissue chips with big opportunities for space medicine. Life Sci. Space Res. 35, 150–157. doi:10.1016/j.lssr.2022.09.002
NIAMS (2017). Osteoporosis. Available from: https://www.niams.nih.gov/health-topics/osteoporosis.
Nicogossian, A. (2003). Medicine and space exploration. Lancet 362 (Suppl. l), 8–s9. doi:10.1016/s0140-6736(03)15055-6
Nwanaji-Enwerem, J., Boileau, P., Galazka, J. M., and Cardenas, A. (2022). In vitro relationships of galactic cosmic radiation and epigenetic clocks in human bronchial epithelial cells. Environ. Mol. Mutagen. 63, 184–189. doi:10.1002/em.22483
Okada, R., Fujita, S., Suzuki, R., Hayashi, T., Tsubouchi, H., Kato, C., et al. (2021). Transcriptome analysis of gravitational effects on mouse skeletal muscles under microgravity and artificial 1 g onboard environment. Sci. Rep. 11, 9168. doi:10.1038/s41598-021-88392-4
Ortega, M. A., Fernández-Garibay, X., Castaño, A. G., De Chiara, F., Hernández-Albors, A., Balaguer-Trias, J., et al. (2019). Muscle-on-a-chip with an on-site multiplexed biosensing system for in situ monitoring of secreted IL-6 and TNF-α. Lab. Chip 19, 2568–2580. doi:10.1039/C9LC00285E
Otsuka, T., Imura, T., Nakagawa, K., Shrestha, L., Takahashi, S., Kawahara, Y., et al. (2018). Simulated microgravity culture enhances the neuroprotective effects of human cranial bone-derived mesenchymal stem cells in traumatic brain injury. Stem Cells Dev. 27, 1287–1297. doi:10.1089/scd.2017.0299
Paek, K., Kim, S., Tak, S., Kim, M. K., Park, J., Chung, S., et al. (2023). A high-throughput biomimetic bone-on-a-chip platform with artificial intelligence-assisted image analysis for osteoporosis drug testing. Bioeng. Transl. Med. 8, e10313. doi:10.1002/btm2.10313
Parafati, M., Giza, S., Shenoy, T. S., Mojica-Santiago, J. A., Hopf, M., Malany, L. K., et al. (2023). Human skeletal muscle tissue chip autonomous payload reveals changes in fiber type and metabolic gene expression due to spaceflight. NPJ Microgravity 9, 77. doi:10.1038/s41526-023-00322-y
Praznikov Victor, M. D. (2022). Diagnosis and treatment of alzheimer's disease and Parkinson's disease with resonance medicine. J. ISSN 2766, 2276. doi:10.37871/jbres1544
Reporter (2020). Organs-on-Chips as a platform for studying effects of microgravity on human physiology: blood-brain barrier-chip in health and disease. Available from: https://reporter.nih.gov/project-details/9402972.
Reporter (2022). Integrated microphysiological system of cerebral organoid and blood vessel for disease modeling and neuropsychiatric drug screening. Available from: https://reporter.nih.gov/project-details/9401926.
Riascos, R. F., Kamali, A., Hakimelahi, R., Mwangi, B., Rabiei, P., Seidler, R. D., et al. (2019). Longitudinal analysis of quantitative brain MRI in astronauts following microgravity exposure. J. Neuroimaging 29, 323–330. doi:10.1111/jon.12609
Rice, O., Surian, A., and Chen, Y. (2022). Modeling the blood-brain barrier for treatment of central nervous system (CNS) diseases. J. Tissue Eng. 13, 204173142210959. doi:10.1177/20417314221095997
Roberts, D. R., Asemani, D., Nietert, P. J., Eckert, M. A., Inglesby, D. C., Bloomberg, J. J., et al. (2019). Prolonged microgravity affects human brain structure and function. AJNR. Am. J. Neuroradiol. 40, 1878–1885. doi:10.3174/ajnr.A6249
Roggan, M. D., Kronenberg, J., Wollert, E., Hoffmann, S., Nisar, H., Konda, B., et al. (2023). Unraveling astrocyte behavior in the space brain: radiation response of primary astrocytes. Front. Public Health 11, 1063250. doi:10.3389/fpubh.2023.1063250
Rösner, H., Wassermann, T., Möller, W., and Hanke, W. (2006). Effects of altered gravity on the actin and microtubule cytoskeleton of human SH-SY5Y neuroblastoma cells. Protoplasma 229, 225–234. doi:10.1007/s00709-006-0202-2
Rowe, P., Koller, A., and Sharma, S. (2022). Physiology, bone remodeling. Treasure Island, FL, USA: StatPearls Publishing.
Samiei, E., Seyfoori, A., Toyota, B., Ghavami, S., and Akbari, M. (2020). Investigating programmed cell death and tumor invasion in a three-dimensional (3D) microfluidic model of glioblastoma. Int. J. Mol. Sci. 21, 3162. doi:10.3390/ijms21093162
Schaue, D., and McBride, W. H. (2015). Opportunities and challenges of radiotherapy for treating cancer. Nat. Rev. Clin. Oncol. 12, 527–540. doi:10.1038/nrclinonc.2015.120
Shelhamer, M. (2016). Parabolic flight as a spaceflight analog. J. Appl. Physiology 120, 1442–1448. doi:10.1152/japplphysiol.01046.2015
Shi, F., Wang, Y., Hu, Z., Xu, H., Sun, J., Gao, Y., et al. (2017). Simulated microgravity promotes angiogenesis through RhoA-dependent rearrangement of the actin cytoskeleton. Cell. Physiology Biochem. 41, 227–238. doi:10.1159/000456060
Shi, Y., Ma, J., Zhang, X., Li, H., Jiang, L., and Qin, J. (2015). Hypoxia combined with spheroid culture improves cartilage specific function in chondrocytes. Integr. Biol. 7, 289–297. doi:10.1039/c4ib00273c
Shin, Y., Choi, S. H., Kim, E., Bylykbashi, E., Kim, J. A., Chung, S., et al. (2019). Blood-brain barrier dysfunction in a 3D in vitro model of alzheimer's disease. Adv. Sci. 6, 1900962. doi:10.1002/advs.201900962
Silvani, G., Basirun, C., Wu, H., Mehner, C., Poole, K., Bradbury, P., et al. (2021). A 3D-bioprinted vascularized glioblastoma-on-a-chip for studying the impact of simulated microgravity as a novel pre-clinical approach in brain tumor therapy. Adv. Ther. 4, 2100106. doi:10.1002/adtp.202100106
Silvani, G., Bradbury, P., Basirun, C., Mehner, C., Zalli, D., Poole, K., et al. (2022). Testing 3D printed biological platform for advancing simulated microgravity and space mechanobiology research. npj Microgravity 8, 19. doi:10.1038/s41526-022-00207-6
Soucy, K. G., Lim, H. K., Kim, J. H., Oh, Y., Attarzadeh, D. O., Sevinc, B., et al. (2011). HZE56Fe-Ion irradiation induces endothelial dysfunction in rat aorta: role of xanthine oxidase. Radiat. Res. 176, 474–485. doi:10.1667/rr2598.1
Takahashi, H., Nakamura, A., and Shimizu, T. (2021). Simulated microgravity accelerates aging of human skeletal muscle myoblasts at the single cell level. Biochem. Biophysical Res. Commun. 578, 115–121. doi:10.1016/j.bbrc.2021.09.037
Tang, Y., Tian, F., Miao, X., Wu, D., Wang, Y., Wang, H., et al. (2022). Heart-on-a-chip using human iPSC-derived cardiomyocytes with an integrated vascular endothelial layer based on a culture patch as a potential platform for drug evaluation. Biofabrication 15, 015010. doi:10.1088/1758-5090/ac975d
Trudel, G., Shafer, J., Laneuville, O., and Ramsay, T. (2020). Characterizing the effect of exposure to microgravity on anemia: more space is worse. Am. J. Hematol. 95, 267–273. doi:10.1002/ajh.25699
Trudel, G., Shahin, N., Ramsay, T., Laneuville, O., and Louati, H. (2022). Hemolysis contributes to anemia during long-duration space flight. Nat. Med. 28, 59–62. doi:10.1038/s41591-021-01637-7
Tsur, E. E. (2020). Computer-aided design of microfluidic circuits. Annu. Rev. Biomed. Eng. 22, 285–307. doi:10.1146/annurev-bioeng-082219-033358
Tsybko, A. S., Ilchibaeva, T. V., Kulikov, A. V., Kulikova, E. A., Krasnov, I. B., Sychev, V. N., et al. (2015). Effect of microgravity on glial cell line-derived neurotrophic factor and cerebral dopamine neurotrophic factor gene expression in the mouse brain. J. Neurosci. Res. 93, 1399–1404. doi:10.1002/jnr.23600
van Loo, B., Schot, M., Gurian, M., Kamperman, T., and Leijten, J. (2024). Single-step biofabrication of in situ spheroid-forming compartmentalized hydrogel for clinical-sized cartilage tissue formation. Adv. Healthc. Mater. 13, 2300095. doi:10.1002/adhm.202300095
Walls, S., Diop, S., Birse, R., Elmen, L., Gan, Z., Kalvakuri, S., et al. (2020). Prolonged exposure to microgravity reduces cardiac contractility and initiates remodeling in Drosophila. Cell Rep. 33, 108445. doi:10.1016/j.celrep.2020.108445
Wang, H., Liu, H., Wang, X., and Zhang, C. (2021). The lack of mass transfer in bone lacunar-canalicular system may be the decisive factor of osteoporosis under microgravity. Life Sci. Space Res. 31, 80–84. doi:10.1016/j.lssr.2021.09.002
Wang, P., Wang, Z., Wang, D., Tian, Y., Li, F., Zhang, S., et al. (2015). Altered gravity simulated by parabolic flight and water immersion leads to decreased trunk motion. PLoS One 10, e0133398. doi:10.1371/journal.pone.0133398
Wang, X., Du, J., Wang, D., Zeng, F., Wei, Y., Wang, F., et al. (2016). Effects of simulated microgravity on human brain nervous tissue. Neurosci. Lett. 627, 199–204. doi:10.1016/j.neulet.2016.06.004
Willey, J. S., Lloyd, S. A. J., Nelson, G. A., and Bateman, T. A. (2011). Space radiation and bone loss. Gravit. Space Biol. Bull. 25, 14–21.
Wu, X., Yang, X., Tian, R., Li, Y., Wang, C., Fan, Y., et al. (2022). Cells respond to space microgravity through cytoskeleton reorganization. FASEB J. official Publ. Fed. Am. Soc. Exp. Biol. 36, e22114. doi:10.1096/fj.202101140R
Wuest, S. L., Richard, S., Kopp, S., Grimm, D., and Egli, M. (2015). Simulated microgravity: critical review on the use of random positioning machines for mammalian cell culture. BioMed Res. Int. 2015, 1–8. doi:10.1155/2015/971474
Xu, P., Yi, Y., Luo, Y., Liu, Z., Xu, Y., Cai, J., et al. (2021). Radiation-induced dysfunction of energy metabolism in the heart results in the fibrosis of cardiac tissues. Mol. Med. Rep. 24, 842. doi:10.3892/mmr.2021.12482
Yan, R., Liu, H., Lv, F., Deng, Y., and Li, Y. (2021). Rac1/Wave2/Arp3 pathway mediates rat blood-brain barrier dysfunction under simulated microgravity based on proteomics strategy. Int. J. Mol. Sci. 22, 5165. doi:10.3390/ijms22105165
Yau, A., Jogdand, A., and Chen, Y. (2023a). Blood-brain-barrier modeling with tissue chips for research applications in space and on Earth. Front. Space Technol. 4, 4. doi:10.3389/frspt.2023.1176943
Yau, A., Wang, Z., Ponthempilly, N., Zhang, Y., Wang, X., and Chen, Y. (2023b). Biosensor integrated tissue chips and their applications on Earth and in space. Biosens. Bioelectron. 222, 114820. doi:10.1016/j.bios.2022.114820
Yau, A., Yeh, K., and Chen, Y. (2021). Musculoskeletal tissue engineering. Amsterdam, Netherlands: Elsevier.
Keywords: tissue chip, microgravity, biomimetic, spheroid, mechanotransduction, stem cell
Citation: Jogdand A, Landolina M and Chen Y (2024) Organs in orbit: how tissue chip technology benefits from microgravity, a perspective. Front. Lab. Chip. Technol. 3:1356688. doi: 10.3389/frlct.2024.1356688
Received: 18 December 2023; Accepted: 20 February 2024;
Published: 07 March 2024.
Edited by:
Maria Kitsara, Nanoplasmas P. C., GreeceReviewed by:
Elishai Ezra Tsur, Open University of Israel, IsraelMarco Campisi, Dana–Farber Cancer Institute, United States
Copyright © 2024 Jogdand, Landolina and Chen. This is an open-access article distributed under the terms of the Creative Commons Attribution License (CC BY). The use, distribution or reproduction in other forums is permitted, provided the original author(s) and the copyright owner(s) are credited and that the original publication in this journal is cited, in accordance with accepted academic practice. No use, distribution or reproduction is permitted which does not comply with these terms.
*Correspondence: Yupeng Chen, eXVwZW5nLmNoZW5AdWNvbm4uZWR1