- 1Center for Mind/Brain Sciences, University of Trento, Rovereto, Italy
- 2Lleida’s Institute for Biomedical Research Dr. Pifarre Foundation (IRBLleida), Lleida, Spain
In recent years, the role of the dopaminergic system in the regulation of social behavior is being progressively outlined, and dysfunctions of the dopaminergic system are increasingly associated with neurodevelopmental disorders, including autism spectrum disorder (ASD). To study the role of the dopaminergic (DA) system in an animal model of ASD, we investigated the effects of embryonic exposure to valproic acid (VPA) on the postnatal development of the mesencephalic DA system in the domestic chick. We found that VPA affected the rostro-caudal distribution of DA neurons, without changing the expression levels of several dopaminergic markers in the mesencephalon. We also investigated a potential consequence of this altered DA neuronal distribution in the septum, a social brain area previously associated to social behavior in several vertebrate species, describing alterations in the expression of genes linked to DA neurotransmission. These findings support the emerging hypothesis of a role of DA dysfunction in ASD pathogenesis. Together with previous studies showing impairments of early social orienting behavior, these data also support the use of the domestic chick model to investigate the neurobiological mechanisms potentially involved in early ASD symptoms.
Introduction
Autism spectrum disorder (ASD) comprises a group of neurodevelopmental conditions strongly characterized by impairments in sociability and social communication. In recent years, the role of the dopaminergic (DA) system in the regulation of social behavior in animal models is being progressively outlined (Gunaydin and Deisseroth, 2014; Yamaguchi et al., 2017; Silva et al., 2020), often in association to motivational and reward mechanisms (Bariselli et al., 2018), while dysfunctions of the dopaminergic system in neurodevelopmental disorders and ASD are also emerging (Scott-Van Zeeland et al., 2010; Supekar et al., 2018; Zürcher et al., 2021).
Neuromodulatory systems, such as the those for the neurotransmitter dopamine, are evolutionarily very conserved (Yamamoto and Vernier, 2011), emerge in early embryonic development from ancient brain areas and are already mature at birth (Ferrari et al., 2012), innervating the neonatal brain. Thus, they represent an ideal target to modulate complex cognitive abilities originating in early development, such as social orienting behavior. Approximately 75% of the total number of DA cells in the brain are located in the ventral part of the mesencephalon, giving rise to two major ascending DA systems that project to specific brain areas (Björklund and Dunnett, 2007). DA neurons in the mammalian ventral tegmental area (VTA) of the mesencephalon mainly project to the ventral striatum, the septum, the amygdala and the medial prefrontal cortex and constitute the mesocorticolimbic (or mesolimbic) pathway. Neurons of this pathway belong to the reward system and are thought to play a major role in controlling social reward, social learning and affiliative behavior. On the other hand, neurons originating in the mammalian substantia nigra (SN) and innervating the dorsal striatum, form the mesostriatal pathway and are mainly involved in motor control. Despite the distinct categorization, neurons of the SN and the VTA have a complex organization and are intertwined in the mesencephalon, having also partially overlapping projections and sharing similar functions (Wise, 2009; Ilango et al., 2014). Furthermore, both DA mesencephalic nuclei are also blended with other type of neurons, as for example GABA and glutamate releasing neurons (Morales and Margolis, 2017) that contribute to their functional features (Bourdy et al., 2014).
In humans, the activation of the mesocorticolimbic pathway has been associated to processing of social stimuli (Spreckelmeyer et al., 2009), while a reduced activation of the same pathway has been described in children and adults with ASD (Scott-Van Zeeland et al., 2010; Supekar et al., 2018). Pharmacological studies in mice have shown that administration of DA mimetic and antagonizing drugs can modulate social interaction bidirectionally. In a seminal work Gunaydin and coworkers (Gunaydin et al., 2014) demonstrated a causal link between activation of VTA DA neurons and social interaction in mice using optogenetic tools. In addition, chemogenetic inhibition of VTA neurons projecting to Nucleus Accumbens (NAc) in mice has been associated to reduced social novelty seeking (Bariselli et al., 2018) and the molecular bases of such mechanisms in neurodevelopmental disorders models are starting to be elucidated (Bariselli et al., 2016, 2018). Recent studies have also highlighted the role of the lateral septum, a limbic structure strongly innervated by the VTA DA neurons, in sociability and social novelty seeking in mice (Mesic et al., 2015), emphasizing the role of DA neurotransmission in the regulation of social behavior (Hostetler et al., 2017; Shin et al., 2018). Overall, it appears that activation of the mesolimbic system, connecting the VTA to the NAc and to the LS, have a prosocial effect on social stimuli processing as well as on social interaction.
In this study, we harnessed the evolutionary conserved nature of the DA neuromodulatory system to investigate the neurobiological mechanisms affected by valproic acid (VPA) treatment in domestic chicks and potentially linked to the social behavioral deficits underlying ASD. VPA is an anticonvulsant known to interfere with development of the social brain, whose prenatal exposure is associated in humans with neural tube malformations, reduced cognitive function, and an increased risk for developing ASD (Christensen et al., 2013). VPA embryonic exposure has been extensively used to model ASD core symptoms in diverse animal species (see for a review Bambini-Junior et al., 2014) including the domestic chick, where it induces alterations of several aspects of social behavior (Nishigori et al., 2013; Sgadò et al., 2018; Lorenzi et al., 2019; Zachar et al., 2019; Adiletta et al., 2021). Nishigori et al. (2013) investigated the effect of different doses and time of administration and found that 35 μmole/egg of VPA injected at E14 induced alterations in social aggregation without affecting filial imprinting. We have expanded this study confirming a detrimental effect of VPA on early emerging social responses to different type of visual stimuli, either stationary (the face-like configuration visible in a stuffed hen, Sgadò et al., 2018; or in a schematic representation Adiletta et al., 2021) or dynamic (speed changes Lorenzi et al., 2019). An additional study by Zachar et al. (2019) analyzed the effect of a different VPA dose (35 μM) on several aspects of social behavior, passive avoidance learning and anxiety. The authors found reduced social exploration and sociability deficits, appearing at the third postnatal week, while no alterations in avoidance learning or social communication was reported (Zachar et al., 2019).
Here we examined the anatomical and molecular layout of the mesencephalic DA system in domestic chicks exposed to VPA during embryonic development. We found that VPA affected the rostro-caudal distribution of DA neurons, without changing the expression levels of several dopaminergic markers in the mesencephalon. We also investigated a potential consequence of this altered DA neuronal distribution in the septum, a social brain area previously associated to social behavior in several vertebrate species (Lorenzi et al., 2017; Mayer et al., 2017; Clemens et al., 2020) and we observed alterations in the expression of genes linked to DA neurotransmission.
Materials and Methods
Ethical Approval
All experiments were conducted according to the current Italian and European Community laws for the ethical treatment of animals. The experimental procedures were approved by the Ethical Committee of the University of Trento and licensed by the Italian Health Ministry (permit number 986/2016-PR).
Embryo Injections
Fertilized eggs of domestic chicks (Gallus gallus), of the Ross 308 (Aviagen) strain, were obtained from a local commercial hatchery [Agricola Berica, Montegalda (VI), Italy]. Upon arrival the eggs were incubated in the dark at 37.5°C and 60% relative humidity, with rocking. One week before the predicted date of hatching, on embryonic day 14 (E14), fertilized eggs were selected by a light test and injected. Chick embryo injection was performed according to previous reports (Nishigori et al., 2013; Sgadò et al., 2018). Briefly, a small hole was made on the flat end of the egg and either VPA (Sodium Valproate, Sigma Aldrich, 35 μmoles) or vehicle (double distilled injectable water; CTRL group) were administered by dropping 200 μl of solution into the air sac of each fertilized egg. Eggs were assigned to the treatment groups randomly. After sealing the hole with paper tape, eggs were placed back in the incubator until E18, when they were prepared for hatching by incubation at 37.7°C, with 72% humidity, and in complete darkness. The day of hatching was considered post-hatching day 0 (P0).
Immunohistochemistry
After 2 days of dark incubation, P2 chicks were overdosed by an intramuscular injection of 0.05 ml per 10 g of body weight of Ketamine/Xylazine Solution (1:1 Ketamine 10 mg/ml + Xylazine 2 mg/ml). After 5 min chicks were transcardially perfused with phosphate-buffered saline (PBS) and ice-cold paraformaldehyde (4% PFA in PBS). Before removing the brain from the skulls, a coronal plane cut was performed using a stereotaxic apparatus (Kuenzel and Masson, 1988) to ensure correct orientation following the stereotaxic coordinates (45°) for coronal sectioning. Brains were then embedded in 7% bovine gelatine in PBS (4.2 g Bovine gelatine in 60 ml PBS at 40°C), using the plane cut to position the brain on the coronal plane. After cooling, the brains were post-fixed and cryopreserved in 4% PFA/PBS/20% sucrose for approximately 6 h at room temperature, and then transferred to 30% Sucrose/0.4%PFA/PBS for further 72 h. Brains were then frozen in dry ice for 30 min before sectioning the entire brain in 60 μm coronal serial sections. For free-floating immunostaining, sections were washed 3 times in PBST (0.005% Triton/PBS) between each of the following steps. After incubation in 0.3% H2O2/PBS for 20 min, the sections were treated for 30 min with blocking solution (3% normal goat serum in PBS). Primary antibody reaction was carried out for 10 days at 4°C in blocking solution (1:1000 mouse monoclonal Tyrosine Hydroxylase antibody; T2928, Sigma-Aldrich). Sections were then incubated in biotinylated goat-anti-mouse antibody for 24 h at 4°C in blocking solution (1:200, BA-1000, Vector Laboratories). Color reaction was performed using the Vectastain Elite ABC Kit (PK-6100, Vector Laboratories) and the DAB kit (SK-4600, Vector Laboratories) following the manufacturer instructions. Finally, sections were rinsed and mounted on gelatin-coated slides, dehydrated and coverslipped.
Cell Counts
Counts of Tyrosine Hydroxylase (TH) positive cells in the substantia nigra and VTA were performed on 8 sets of serial sections per animal, sampled at 300 μm intervals (n = 3 CTRL and 3 VPA chicks). Counting of TH immunoreactive cells was performed blind to the experimental condition using ZEN imaging software (Zeiss, Germany). All the sections were aligned on the rostral to caudal axis using Plate 34 of the chick brain atlas as reference (Puelles et al., 2007). A rectangle of 150 × 250 pixels (pixel size = 0.43 μm) was placed over the samples and TH-positive cells within this area were manually counted. Cell densities were separately counted in the SN and the VTA as number of cells/area. For each analyzed brain slice, the sampling field was moved randomly through the area of interest at least four times for each dopaminergic subgroup.
Tissue Dissection
For microdissection of the SN and the VTA neurons, P2 chicks reared in complete darkness were euthanized via carbon dioxide gaseous asphyxiation, their brain extracted and then fast-frozen in dry-ice-cold isopentane solution. 100 μm coronal sections were cut using a Leica CM1850 UV Cryostat at −15°C, and stored at −20°C. To better localize the targeted areas, sections were stained for 15 min with a 0.01% cresyl violet solution dissolved in 100% ethanol, and then progressively dehydrated in 75, 90, and 100% ethanol (1 min/each). All solutions were prepared fresh and filter-sterilized to avoid RNases contaminations. Substantia nigra and VTA regions were finally dissected out using a 20G needle and immediately processed for total RNA extraction. For dissections of the septum, P2 chicks reared in darkness were euthanized by carbon dioxide gaseous asphyxiation, the brain extracted, and the area of interest directly dissected. Briefly, two coronal cuts were performed approximately 2 and 4 mm anterior to the bregma to isolate the septum in the anterior-posterior axis according to Puelles et al. (2007) and the septum was carefully removed with forceps, fresh frozen in dry ice and immediately processed for total RNA extraction.
Total RNA Extraction
Total RNA extraction from the septum was performed using the RNeasy Mini Kit (QIAGEN), while RNA from SN and VTA samples was extracted using the PicoPure™ RNA Isolation Kit (Applied Biosystems™, Thermo Fisher Scientific). Both extractions were performed according to the manufacturers’ instructions. Reverse transcription for both types of extracted materials was performed with the SuperScript™ VILO™ cDNA Synthesis Kit (Invitrogen, Thermo Fisher Scientific; Monza, Italy), following manufacturer’s instructions.
Reverse Transcription-Quantitative Polymerase Chain Reaction
Reverse transcription-quantitative polymerase chain reaction (RT-qPCR) was carried out with PowerUp™ SYBR™ Green Master Mix (2x) (Thermo Fisher Scientific; Monza, Italy) for the septum and with SsoAdvanced™ Universal SYBR® Green Supermix (Bio-Rad, Milan, Italy) for mesencephalic samples. Both reactions were performed using a CFX96™ Real-Time System (Bio-Rad, Milan, Italy). Commercially synthesized primers (Merck Life Science Srl, Milan, Italy) used in this work are listed in Table 1. Quantitation cycles (Cq) values were calculated using the second derivative maximum method. Data were normalized on the expression of TBP (TATA-Box Binding Protein) and HMBS (Hydroxymethylbilane Synthase) reference genes using the DeltaCt (dCt) method (Pfaffl, 2001).
Statistical Analysis
Statistical evaluation of the effect of treatment on the distribution and number of DA neurons of the different subgroups and of the log2 gene expression levels (dCt) was assessed using mixed-effect models using the nlme package in R.1 For Tukey pairwise comparison tests, we used the emmeans package in R.2
Results
Previous studies demonstrated a remarkable effect of exposure to VPA during embryonic development on the dopaminergic system (Schiavi et al., 2019; Ádám et al., 2020; Messina et al., 2020; Román et al., 2021). To evaluate the effect of VPA on the dopaminergic system of domestic chicks, we performed immunohistochemical analysis and quantification of DA cell number in the SN and the VTA of VPA- and vehicle injected chicks, 48 h after hatching (P2). Brain sections from VPA- and vehicle-injected domestic chicks were immunolabeled for Tyrosine Hydroxylase (TH), the rate limiting enzyme for DA synthesis. Representative images of TH immunohistochemically labeled cells in the SN and VTA are shown in Figure 1A and Supplementary Figure 1. TH-positive cells where then counted and DA cell densities (cells/area, see section “Materials and Methods”) were quantified in each of the serial sections encompassing the mesencephalon, separating neurons of the SN from those of the VTA, and outlining their respective rostro-caudal distribution. To assess the effect of treatment (VPA and CTRL), DA group (SN and VTA) and rostro-caudal distribution (a set of 8 sections, from the most rostral to the most caudal) on DA density, we used a linear mixed model (LMM), considering treatment, group and section as fixed factors. We compared a model with random-intercepts-only to one with random slope and intercepts, without covariance between intercepts and slope, and found that the second model fitted the data significantly better. We found that the overall density of DA cells was significantly different between the two DA groups [SN vs. VTA: F(1,60) = 40.2371, p < 0.0001] and in the different rostro-caudal positions [sections: F(7,60) = 10.5726, p < 0.0001] but no significant main effect of treatment was found in the overall number of DA cells (Figure 1B; mean cells/area in substantia nigra CTRL 5.574 [95% C.I. 4.232–6.915], VPA 5.574 [95% C.I. 2.451–8.696] in VTA CTRL 9.302 [95% C.I. 2.354–16.250], VPA 9.229 [95% C.I. 8.064–10.394; treatment: F(1,4) = 0.7096, p = 0.4470]. We also observed a significant interaction between treatment and section [treatment*section: F(7,60) = 4.2912, p = 0.0007], indicating an effect of treatment on the rostro-caudal distribution of DA neurons. More interestingly, a triple interaction between treatment, section and group was observed [treatment*section*group: F(7,60) = 2.2929, p = 0.0387], suggesting that the effect of treatment on the rostro-caudal distribution was different in the two DA subgroups (Figures 1C,D). No other significant interactions were found between the other factors [treatment*group: F(1,60) = 0.0039, p = 0.9503; section*group: F(7,60) = 2.1493, p = 0.0518]. Given the differences on the overall density of DA cells in the subgroups, we analyzed VTA and SN distribution separately. The pairwise comparison of the cell densities in VPA and vehicle-treated domestic chicks in each section of the separate groups (Figures 1C,D) revealed a change in the distribution of the DA cell densities toward the posterior part of the mesencephalon, and thus a caudal shift in the distribution of DA neurons in VPA-injected chick compared to controls, more prominent in the substantia nigra [Figure 1C; CTRL vs. VPA, section1 t(4) = 8.7974, p = 0.0009; section 2 t(4) = 3.1817, p = 0.0335; section 3 t(4) = 2.4302, p = 0.0720; section 4 t(4) = 1.9438, p = 0.1238; section 5 t(4) = 0.5757, p = 0.5957; section 6 t(4) = −3.5038, p = 0.0248, section 7 t(4) = −3.7756, p = 0.0195; section 8 t(4) = −5.0059, p = 0.0075] than in the VTA [Figure 1D; CTRL vs. VPA, section1 t(4) = 3.3448, p = 0.0287; section 2 t(4) = 1.0207, p = 0.3651; section 3 t(4) = 1.0597, p = 0.3490; section 4 t(4) = −1.6494, p = 0.1744; section 5 t(4) = −1.0495 p = 0.3532; section 6 t(4) = 0.4148, p = 0.6996, section 7 t(4) = −0.2410 p = 0.8214; section 8 t(4) = −3.8945 p = 0.0176]. Overall, our statistical analysis indicated a significant effect of VPA injection in the second embryonic week on the development of the mesencephalic dopaminergic neurons detectable at P2 as an alteration of the rostro-caudal distribution of DA cells.
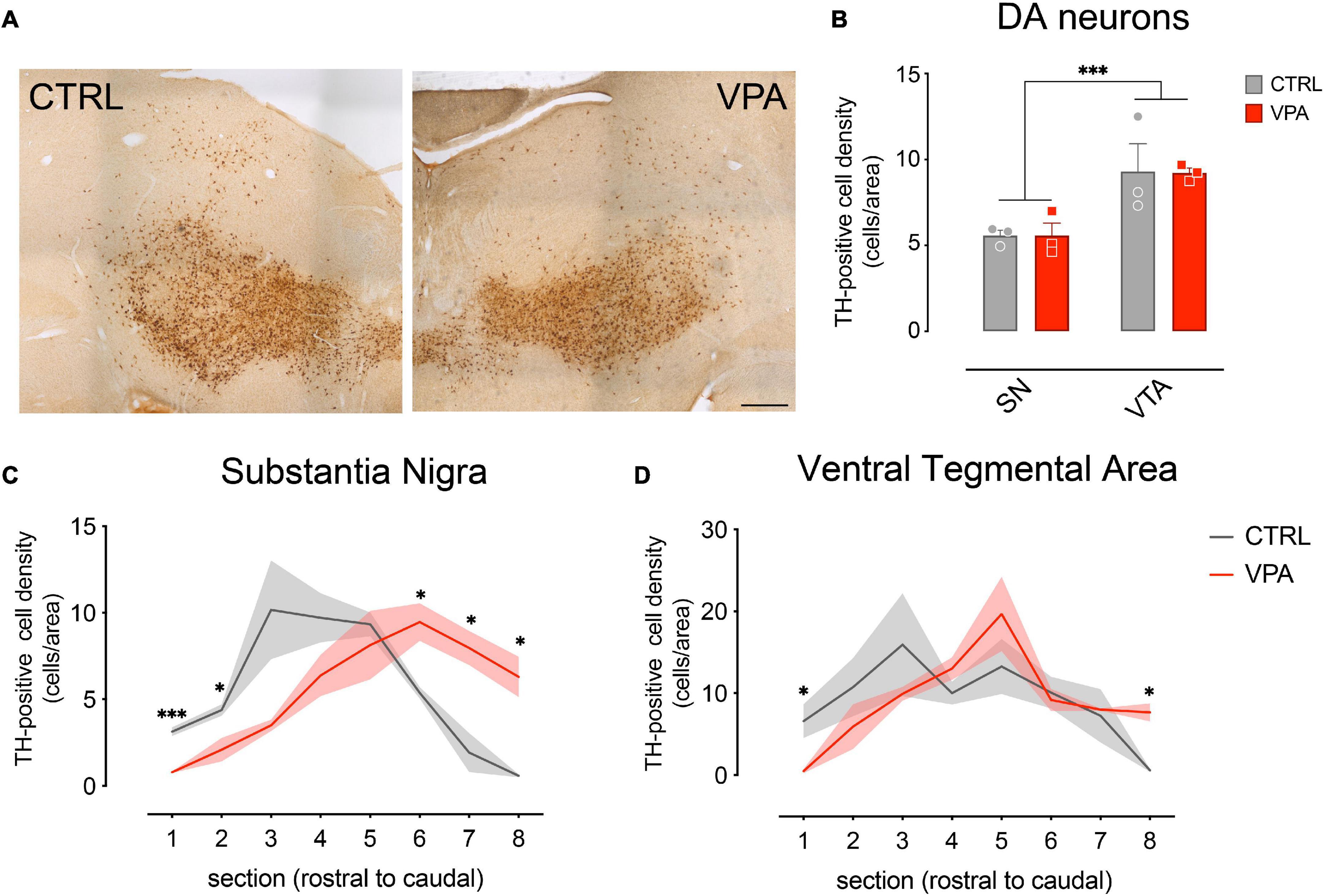
Figure 1. Immunohistochemical analysis and quantification of cell densities. (A) Brain sections from CTRL and VPA chicks immunolabeled for Tyrosine Hydroxylase (TH). (B) Number of TH-positive DA neurons in both SN and VTA. TH-positive cells count was performed on 8 sets of serial sections per animal, sampled at 300 μm intervals. Rostro-caudal alignment of the brain sections was based on atlas reference (Plate 34 from Puelles et al., 2007). (C) Substantia Nigra DA cell density measured in its rostro-caudal distribution. (D) Ventral Tegmental Area DA cell density measured in its rostro-caudal distribution. Scale bar 500 μm. ∗p < 0.05, ∗∗∗p < 0.001.
To assess the molecular changes induced by VPA on the dopaminergic system at P2, we micro-dissected DA neurons of the SN and VTA (Figure 2A; n = 6 animal per treatment group, two independent experiments) of the entire rostro-caudal distribution, and measured the expression levels of genes involved in development (En1, TH, TPH2, Gad1) and neurotransmission (DRD1, DRD2, 5HTR1A, 5HTR2A, GABRA1, GABBR2). To assess the effect of treatment, DA group and transcripts, we used a linear mixed model, considering treatment, group and transcript as fixed factors and the experimental unit (experiment) as random factor. We compared a model with random-intercepts-only to one with random slopes and intercepts and found that the random slopes and intercepts model fitted the data significantly better. The statistical analysis indicated a significant difference in the expression levels between the transcripts analyzed in the two dopaminergic subgroups [transcripts: F(8,152) = 406.6968, p < 0.0001; group: F(1,152) = 3.7786, p = 0.0538; gene*group: F(8,152) = 42.0532, p < 0.0001], however we could not detect any significant effect of VPA exposure at E14 on the expression of the genes at P2 [treatment: F(1,10) = 0.0372, p = 0.8509; treatment*gene: F(8,152) = 0.2526, p = 0.9795; treatment*group: F(1,152) = 0.1096, p = 0.7410; treatment*gene*group: F(8,152) = 0.7626, p = 0.6362]. Independent on the treatment, some of the genes showed differences in expression levels between the two dopaminergic subgroups as indicated by the Tukey pairwise comparison for the transcripts in each DA subgroup (Figure 2B; SN vs. VTA). DRD2 [t(152) = 4.8848, p < 0.0001], TH [t(152) = 6.2522, p < 0.0001], GAD1 [t(152) = 4.4819, p < 0.0001] and GABRA1 [t(152) = 2.8885, p = 0.0044] show increased levels in the SN compared to the VTA, while TPH2 [t(152) = −15.5918, p < 0.0001] was expressed at higher levels in the VTA.
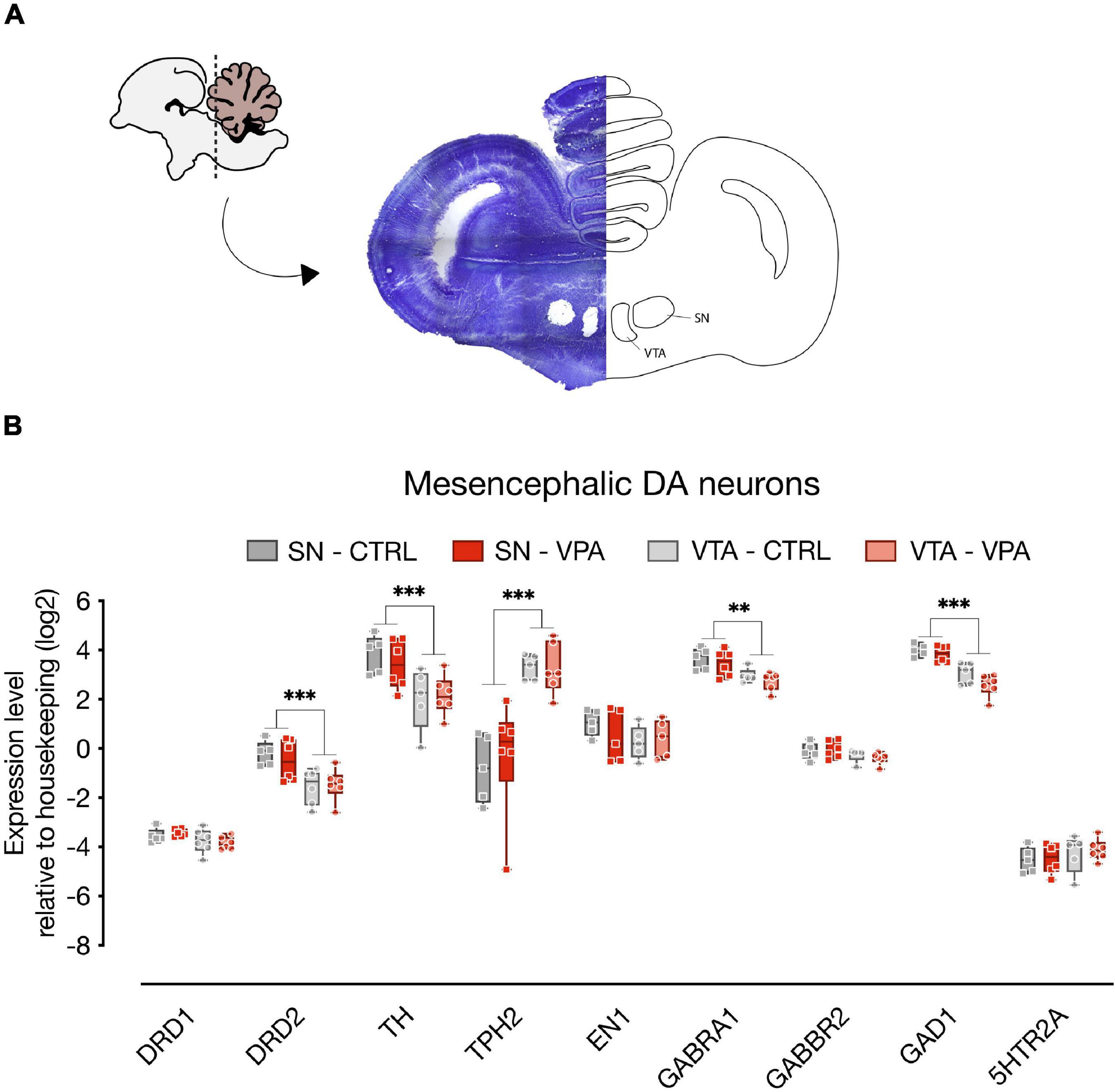
Figure 2. Gene expression levels in the SN and VTA. (A) Schematic representation of the microdissected areas of interest (SN, Substantia Nigra; VTA, Ventral Tegmental Area) from coronal sections (adapted from Plate 33 in Puelles et al., 2007). (B) Box and whisker plot (median, min to max) of relative expression (dCt, log2) values for each group. Changes in expression of DRD1, DRD2, TH, TPH2, EN1, GABRA1, GABBR2, GAD1, and 5HTR2A in both SN and VTA of VPA- and vehicle-injected chicks were analyzed at P2. ∗∗p < 0.01, ∗∗∗p < 0.0001.
We then examined gene expression changes in the septum, a brain area highly innervated by dopaminergic input coming from the SN and the VTA (Montagnese et al., 2008) and involved in social behavior (Hostetler et al., 2017; Shin et al., 2018), that has been shown to activate in domestic chicks in response to exposure to conspecifics (Lorenzi et al., 2017; Mayer et al., 2017). We assessed the expression of genes involved in neurotransmission (Figure 3; n = 8 animals per group, four independent experiments), such as the dopamine receptors (DRD1 and DRD2) and the DA responsive protein DARPP32, the serotonin receptors (5HTR1A and 5HTR2A) and the serotonin transporter 5HTT, and genes involved in synaptic plasticity coding for the NMDA subunits (GRIN2A and GRIN2B), and CREB1. We also measured the expression levels of the mesotocin receptor (OXTR), the avian homolog of oxytocin, since the lateral part of the septum is known to receive consistent oxytocinergic innervation (Loveland et al., 2019; Horiai et al., 2020). We again used linear mixed models to evaluate the effect of treatment (CTRL vs. VPA) and transcripts, and found that the best fitting model was a random slopes and intercepts model with the same parameters used for analysis of gene expression in the mesencephalon. We found a significant main effect of treatment [F(1,138) = 7.7599, p = 0.0061] and a significant differences in the levels of expression of the transcripts [F(9,138) = 39.1627, p < 0.0001]. We also observed a significant interaction between treatment and transcript [F(9,138) = 5.1513, p < 0.0001], indicating an effect of treatment on some of the transcripts. The Tukey pairwise comparison indicated that expression of DRD1 [t(138) = 2.0741, p = 0.0399], DARPP32 [t(138) = 5.6749, p < 0.0001], and GRIN2A [t(138) = 2.9705, p = 0.0035] was decreased in VPA-injected chicks (Figure 3), while 5HTT [t(138) = −2.0297, p = 0.0443] expression was increased by the treatment, and expression of the other transcripts did not change [5HTR1A: t(138) = 1.4917, p = 0.1381; 5HTR2A: t(138) = −0.5621, p = 0.5749; CREB1: t(138) = 0.7844, p = 0.4342; DRD2: t(138) = 1.5030, p = 0.1351; GRIN2B: t(138) = 1.6429, p = 0.1027; OXTR: t(138) = −0.2558, p = 0.7985].
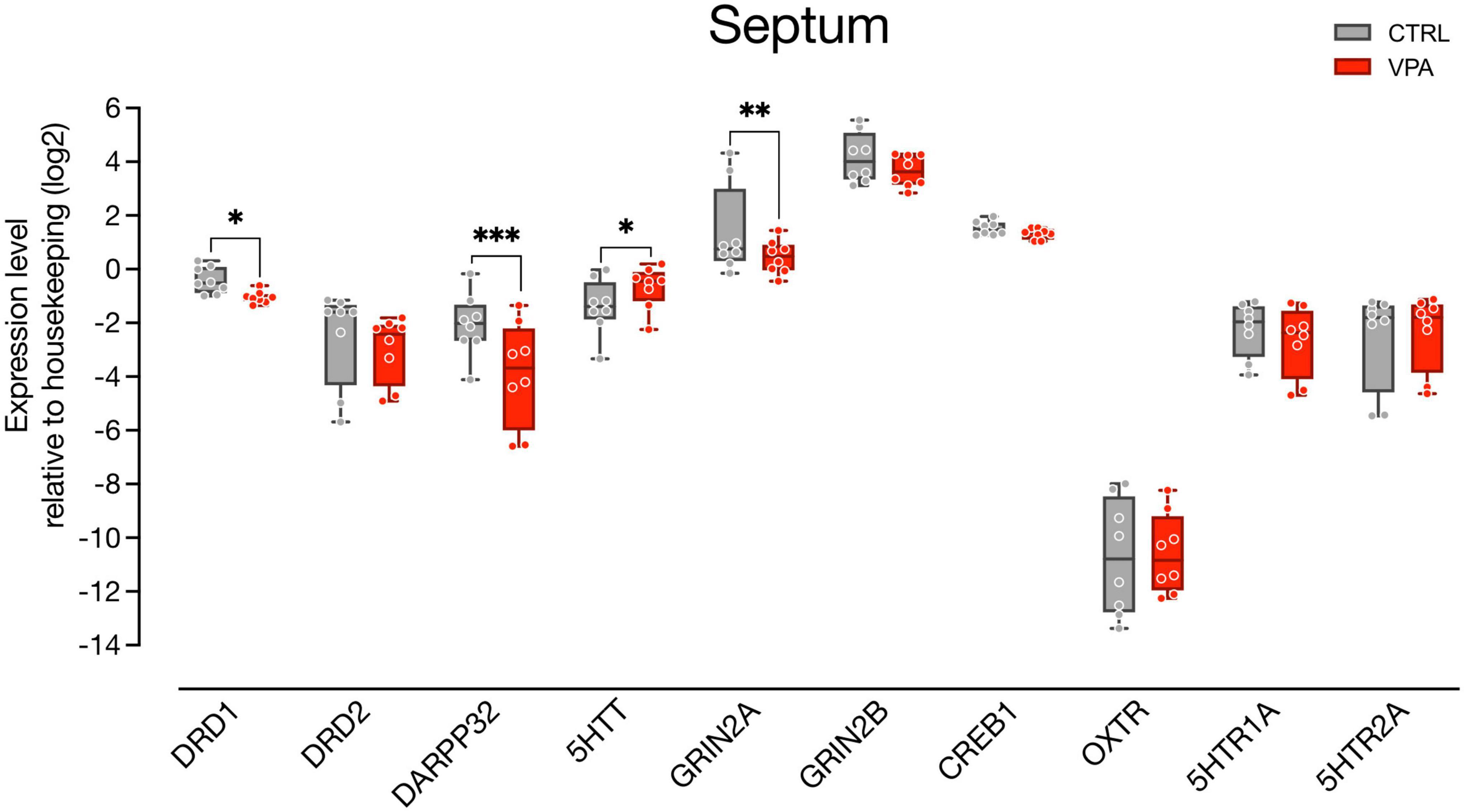
Figure 3. Gene expression levels in the septum. Box and whisker plot (median, min to max) of relative expression (dCt, log2) values for each group. Changes in expression of DRD1, DRD2, DARPP32, 5HTT, GRIN2A, GRIN2B, CREB1, OXTR, 5HTR1A, and 5HTR2A in septum samples collected from P2 chicks embryonically exposed to VPA. ∗p < 0.05, ∗∗p < 0.001, ∗∗∗p < 0.0001.
Discussion
The dopaminergic system has been shown to influence key aspects of social and affiliative behavior in humans and other vertebrates (Opendak et al., 2021; Solié et al., 2022), and to cooperate in modulating key components of the social brain network (Gunaydin and Deisseroth, 2014). In addition, accumulating evidence point to an involvement of DA neurotransmission in atypical social development in children with ASD (Scott-Van Zeeland et al., 2010; Supekar et al., 2018; Zürcher et al., 2021).
In the present study, we investigated developmental dysregulations of the DA system in a VPA model of ASD implemented in domestic chicks, that could potentially contribute to the behavioral deficits observed in the chicks’ spontaneous responses to social stimuli, including altered response to faces (Adiletta et al., 2021). More specifically, we analyzed the number, distribution, and the developmental gene expression of DA neurons in the postnatal mesencephalon of domestic chicks embryonically exposed to VPA, and then assessed gene expression changes in the septum, a region of the social brain network highly innervated by DA terminals (Lindvall and Stenevi, 1978; Gaspar et al., 1985) and involved in sociability and social novelty seeking (Mesic et al., 2015).
Consistent with our hypothesis of an effect of VPA on the DA system, when analyzing the rostro-caudal portions of the mesencephalon, we observed a caudal shift in the distribution of mesencephalic DA population at P2, 9 days after embryonic exposure to VPA. Neuroanatomical alterations of mesencephalic TH population was already described in mice exposed to VPA by Ádám et al. (2020), however, without analyses on the distribution of the DA population. Moreover, differently from the work of Ádám et al. (2020), here we found no difference in the density of DA neurons (measured as number of cells/area) between the two treatments groups, suggesting the preservation of the overall profile of the DA population in our model, as indicated also by our gene expression analysis in the mesencephalon. Interestingly, VPA exposure was performed at E14, long after embryonic dopaminergic proliferation and differentiation events have terminated (Andersson et al., 2006), deeming unlikely a direct influence of VPA on neurogenesis or differentiation of DA neurons. A recent report by Huang et al. (2019) has described developmental expansion in the DA and serotonin neurons in chicken mid-late embryogenesis, revealing an increase in TH expression in the mesencephalon between E12 and E14 and later, between E16 and E19, accompanied by increased levels of DA in the brainstem. Thus, waves of DA neuron expansions may also occur in mid-late embryogenesis. In the light of these data, VPA treatment could impinge on this naturally occurring late expansion of the DA population and then affect the distribution/migration of the newly born DA neurons, causing a shift in their rostro-caudal positioning. Our data on the expression of TH suggest that VPA does not regulate proliferation of the DA population, nor does it affect the number of DA neurons, as confirmed by the cell counts and by the expression of both TH and En1, that did not change upon treatment with VPA. The observed changes in DA neurons distribution may occur through different mechanisms. One possibility is through regulation of genes affecting migration, as for example the CXC motif chemokine receptor 4 (Cxcr4), a G-protein coupled receptor binding to the chemokine Cxcl12, and involved in cell migration (Li and Ransohoff, 2008). Cxcr4 controls the migration of hippocampal neurons and interneurons (Wang et al., 2011), and its expression is regulated by VPA prenatal administration in mice (Sakai et al., 2018). Embryonic exposure with VPA in mice induces a downregulation of Cxcr4 and an increase in the density of hippocampal granule cells that are aberrantly positioned, this phenotype is reversed by postnatal overexpression of Cxcr4 (Sakai et al., 2018). The mechanisms through which VPA acts on Cxcr4 in murine hippocampal granule cells is still not entirely clear. VPA could directly affect Cxcr4 expression or it could affect cell migration through its well-known effect on GABA, since GABA agonists have been shown to activate Cxcr4 (Guyon et al., 2013). Interestingly, CxCl12/Cxcr4 signaling also regulates the migration and orientation of DA neurons during development in mice (Yang et al., 2013). Future studies will clarify the mechanisms through which VPA exerts its effect on DA neurons and whether other brainstem populations, as for example the serotonergic neurons of the raphe nuclei, are also affected by VPA treatment.
To investigate the potential consequences of the observed rostro-caudal shift in the distribution of the mesencephalic DA neurons, we have also assessed changes in the expression of genes involved in DA neurotransmission in one of the target regions of the mesocorticolimbic pathway, the septum, known to modulate different aspects of social behavior in mice (see for a review Menon et al., 2022) as well as in domestic chicks (Lorenzi et al., 2017; Mayer et al., 2017). We found that DRD1, DARPP32 and GRIN2A were downregulated upon VPA exposure, suggesting deficits in dopaminergic signaling in this brain region. Previous studies have investigated the role of dopaminergic signaling in social behavior, demonstrating increased DARPP32 phophorylation mediated by DRD1 signaling in the nucleus accumbens of rats subjected to same-sex social interactions (Scheggi et al., 2020). Direct stimulation of DRD1 in the nucleus accumbens has also been shown to produce significant increase in same-sex social interaction in mice (Gunaydin et al., 2014). Interestingly, VPA embryonic exposure in rats abolishes the DRD1-mediated phosphorylation of DARPP32 (Scheggi et al., 2020) suggesting that a decrease in the DRD1-mediated signaling could produce detrimental effects on social behavior. It remains to be established how exactly VPA may act on DRD1 expression and whether a similar mechanism is also in place in the septum of domestic chicks in association to exposure to social partners.
We also found that expression of the serotonin transporter (5HTT) was increased in the septum, suggestive of alterations also in serotonergic neurotransmission. Several studies and meta-analysis have confirmed an increase in 5HT in the blood (hyperserotonemia) of autistic individuals, such that hyperserotonemia has become a reliable biomarker for these disorders (see for a review Lam et al., 2006; Gabriele et al., 2014). Epidemiological and animal model studies have suggested that perinatal alterations in 5HT, either above or below typical levels, may cause social behavioral deficits resembling ASD (Garbarino et al., 2019).
Previous studies have reported alterations in the number of DA neurons or in DA neurotransmission in several animal models of ASD (see for a recent review Kosillo and Bateup, 2021). A reduction in the number of DA neurons in the SN (but not the VTA) was found in adult mice lacking the Fmr1 gene (Fish et al., 2013). Further studies demonstrated reduced striatal DA transmission and striatal DA re-uptake without significant changes in the striatal tissue DA content in Fmr1 mutant mice (Fulks et al., 2010; Sørensen et al., 2015). Alterations in DA-mediated responses have also been reported in the BTBR mice (Squillace et al., 2014), a model for idiopathic autism, accompanied by decreased TH expression in several DA innervated brain regions (Chao et al., 2020). Interestingly, intranasal dopamine administration efficiently rescued the cognitive and social deficits of both BTBR and Fmr1 mutant ASD models (Chao et al., 2020), suggesting a causal role of DA deficiency in the behavioral phenotype of the mice.
Notably, in our study, we investigated for the first time DA-related deficits on an ASD model implemented in the domestic chicks. Differently from other common animal models, chicks are precocial species able to independently behave soon after hatching (Versace and Vallortigara, 2015), displaying remarkable early and spontaneous social responses, already shown to have similar features to the social orienting behavior observed in human newborns (Di Giorgio et al., 2017). Domestic chicks also enable researchers to study early neurodevelopmental mechanisms, without the interference of sophisticated, divergent, strategies of adaptive learning that emerge later. We thus believe that the domestic chick represents an ideal candidate model to study the causal relationship between social orienting behavior, emerging at early postnatal stages, and any underlying neurobiological alterations mediated by VPA or any other genetic manipulation associated to ASD. Further studies should thus investigate the potential causal relationship between DA signaling alterations and the early social orienting deficits observed in VPA exposed chicks, including impairments in face processing and affiliative behavior.
Data Availability Statement
The original contributions presented in the study are included in the article/Supplementary Material, further inquiries can be directed to the corresponding author.
Ethics Statement
The animal study was reviewed and approved by the Ethical Committee of the University of Trento and the Italian Health Ministry (permit number 986/2016-PR).
Author Contributions
PS conceived and designed the experiments. AA and AP conducted the experiments. NT provided technical support. PS and AA analyzed the data and wrote the manuscript. All authors read and approved the final manuscript.
Funding
This work was supported by the University of Trento (intramural funds to AA, NT, and PS).
Conflict of Interest
The authors declare that the research was conducted in the absence of any commercial or financial relationships that could be construed as a potential conflict of interest.
Publisher’s Note
All claims expressed in this article are solely those of the authors and do not necessarily represent those of their affiliated organizations, or those of the publisher, the editors and the reviewers. Any product that may be evaluated in this article, or claim that may be made by its manufacturer, is not guaranteed or endorsed by the publisher.
Acknowledgments
We thank Giorgio Vallortigara and Andrea Messina for their support. Dr. Tommaso Pecchia for help with the experimental apparatus, Grazia Gambardella and Roberta Guidolin for administrative help and Ciro Petrone for animal facility management.
Supplementary Material
The Supplementary Material for this article can be found online at: https://www.frontiersin.org/articles/10.3389/fnint.2022.804881/full#supplementary-material
Footnotes
- ^ https://cran.r-project.org/web/packages/nlme/index.html
- ^ https://cran.r-project.org/web/packages/emmeans/index.html
References
Ádám, Á, Kemecsei, R., Company, V., Murcia-Ramón, R., Juarez, I., Gerecsei, L., et al. (2020). Gestational exposure to sodium valproate disrupts fasciculation of the mesotelencephalic dopaminergic tract, with a selective reduction of dopaminergic output from the ventral tegmental area. Front. Neuroanat. 14:29. doi: 10.3389/fnana.2020.00029
Adiletta, A., Pedrana, S., Rosa-Salva, O., and Sgadò, P. (2021). Spontaneous visual preference for face-like stimuli is impaired in newly-hatched domestic chicks exposed to valproic acid during embryogenesis. Front. Behav. Neurosci. 15:733140. doi: 10.3389/fnbeh.2021.733140
Andersson, E., Tryggvason, U., Deng, Q., Friling, S., Alekseenko, Z., Robert, B., et al. (2006). Identification of intrinsic determinants of midbrain dopamine neurons. Cell 124, 393–405. doi: 10.1016/j.cell.2005.10.037
Bambini-Junior, V., Baronio, D., MacKenzie, J., Zanatta, G., Riesgo, R., dos, S., et al. (2014). “Prenatal exposure to valproate in animals and autism,” in Comprehensive Guide to Autism, eds V. Patel, V. Preedy, and C. Martin (New York, NY: Springer), 1779–1793.
Bariselli, S., Hörnberg, H., Prévost-Solié, C., Musardo, S., Hatstatt-Burklé, L., Scheiffele, P., et al. (2018). Role of VTA dopamine neurons and neuroligin 3 in sociability traits related to nonfamiliar conspecific interaction. Nat. Commun. 9:3173. doi: 10.1038/s41467-018-05382-3
Bariselli, S., Tzanoulinou, S., Glangetas, C., Prévost-Solié, C., Pucci, L., Viguié, J., et al. (2016). SHANK3 controls maturation of social reward circuits in the VTA. Nat. Neurosci. 19, 926–934. doi: 10.1038/nn.4319
Björklund, A., and Dunnett, S. (2007). Dopamine neuron systems in the brain: an update. Trends Neurosci. 30, 194–202. doi: 10.1016/j.tins.2007.03.006
Bourdy, R., Sánchez-Catalán, M.-J., Kaufling, J., Balcita-Pedicino, J. J., Freund-Mercier, M.-J., Veinante, P., et al. (2014). Control of the nigrostriatal dopamine neuron activity and motor function by the tail of the ventral tegmental area. Neuropsychopharmacology 39, 2788–2798. doi: 10.1038/npp.2014.129
Chao, O. Y., Pathak, S. S., Zhang, H., Dunaway, N., Li, J.-S., Mattern, C., et al. (2020). Altered dopaminergic pathways and therapeutic effects of intranasal dopamine in two distinct mouse models of autism. Mol. Brain 13:111. doi: 10.1186/s13041-020-00649-7
Christensen, J., Grønborg, T., Sørensen, M., Schendel, D., Parner, E., Pedersen, L., et al. (2013). Prenatal valproate exposure and risk of autism spectrum disorders and childhood autism. JAMA 309, 1696–1703.
Clemens, A. M., Wang, H., and Brecht, M. (2020). The lateral septum mediates kinship behavior in the rat. Nat. Commun. 11:3161. doi: 10.1038/s41467-020-16489-x
Di Giorgio, E., Loveland, J. L., Mayer, U., Rosa-Salva, O., Versace, E., and Vallortigara, G. (2017). Filial responses as predisposed and learned preferences: early attachment in chicks and babies. Behav. Brain Res. 325, 90–104. doi: 10.1016/j.bbr.2016.09.018
Ferrari, D., Mdzomba, B., Dehorter, N., Lopez, C., Michel, F., Libersat, F., et al. (2012). Midbrain dopaminergic neurons generate calcium and sodium currents and release dopamine in the striatum of pups. Front. Cell Neurosci. 6:7. doi: 10.3389/fncel.2012.00007
Fish, E. W., Krouse, M. C., Stringfield, S. J., Diberto, J. F., Robinson, J. E., and Malanga, C. J. (2013). Changes in sensitivity of reward and motor behavior to dopaminergic, glutamatergic, and cholinergic drugs in a mouse model of fragile X syndrome. PLoS One 8:e77896. doi: 10.1371/journal.pone.0077896
Fulks, J. L., O’Bryhim, B. E., Wenzel, S. K., Fowler, S. C., Vorontsova, E., Pinkston, J. W., et al. (2010). Dopamine release and uptake impairments and behavioral alterations observed in mice that model fragile x mental retardation syndrome. ACS Chem. Neurosci. 1, 679–690. doi: 10.1021/cn100032f
Gabriele, S., Sacco, R., and Persico, A. M. (2014). Blood serotonin levels in autism spectrum disorder: a systematic review and meta-analysis. Eur. Neuropsychopharmacol. 24, 919–929. doi: 10.1016/j.euroneuro.2014.02.004
Garbarino, V. R., Gilman, T. L., Daws, L. C., and Gould, G. G. (2019). Extreme enhancement or depletion of serotonin transporter function and serotonin availability in autism spectrum disorder. Pharmacol. Res. 140, 85–99. doi: 10.1016/j.phrs.2018.07.010
Gaspar, P., Berger, B., Alvarez, C., Vigny, A., and Henry, J. P. (1985). Catecholaminergic innervation of the septal area in man: immunocytochemical study using TH and DBH antibodies. J. Comp. Neurol. 241, 12–33. doi: 10.1002/cne.902410103
Gunaydin, L., Grosenick, L., Finkelstein, J., Kauvar, I., Fenno, L., Adhikari, A., et al. (2014). Natural neural projection dynamics underlying social behavior. Cell 157, 1535–1551. doi: 10.1016/j.cell.2014.05.017
Gunaydin, L. A., and Deisseroth, K. (2014). Dopaminergic dynamics contributing to social behavior. Cold Spring Harb. Symp. Quant. Biol. 79, 221–227. doi: 10.1101/SQB.2014.79.024711
Guyon, A., Kussrow, A., Olmsted, I., Sandoz, G., Bornhop, D., and Nahon, J. (2013). Baclofen and other GABAB receptor agents are allosteric modulators of the CXCL12 chemokine receptor CXCR4. J. Neurosci. 33, 11643–11654.
Horiai, M., Otsuka, A., Hidema, S., Hiraoka, Y., Hayashi, R., Miyazaki, S., et al. (2020). Targeting oxytocin receptor (Oxtr)-expressing neurons in the lateral septum to restore social novelty in autism spectrum disorder mouse models. Sci. Rep. 10:22173. doi: 10.1038/s41598-020-79109-0
Hostetler, C. M., Hinde, K., Maninger, N., Mendoza, S. P., Mason, W. A., Rowland, D. J., et al. (2017). Effects of pair bonding on dopamine D1 receptors in monogamous male titi monkeys (Callicebus cupreus). Am. J. Primatol. 79, 1–9. doi: 10.1002/ajp.22612
Huang, X., Kuang, S., Applegate, T., Lin, T., and Cheng, H. (2019). The development of the serotonergic and dopaminergic systems during chicken mid-late embryogenesis. Mol. Cell Endocrinol. 493:110472. doi: 10.1016/j.mce.2019.110472
Ilango, A., Kesner, A. J., Keller, K. L., Stuber, G. D., Bonci, A., and Ikemoto, S. (2014). Similar roles of substantia nigra and ventral tegmental dopamine neurons in reward and aversion. J. Neurosci. 34, 817–822. doi: 10.1523/JNEUROSCI.1703-13.2014
Kosillo, P., and Bateup, H. (2021). Dopaminergic dysregulation in syndromic autism spectrum disorders: insights from genetic mouse models. Front. Neural Circuits 15:700968. doi: 10.3389/fncir.2021.700968
Kuenzel, W. J., and Masson, M. (1988). A Stereotaxic Atlas of the Brain of the Chick (Gallus Domesticus). Baltimore, MD: Johns Hopkins University Press.
Lam, K. S. L., Aman, M. G., and Arnold, L. E. (2006). Neurochemical correlates of autistic disorder: a review of the literature. Res. Dev. Disabil. 27, 254–289. doi: 10.1016/j.ridd.2005.03.003
Li, M., and Ransohoff, R. (2008). Multiple roles of chemokine CXCL12 in the central nervous system: a migration from immunology to neurobiology. Prog. Neurobiol. 84, 116–131. doi: 10.1016/j.pneurobio.2007.11.003
Lindvall, O., and Stenevi, U. (1978). Dopamine and noradrenaline neurons projecting to the septal area in the rat. Cell Tissue Res. 190, 383–407. doi: 10.1007/BF00219554
Lorenzi, E., Mayer, U., Rosa-Salva, O., and Vallortigara, G. (2017). Dynamic features of animate motion activate septal and preoptic areas in visually naïve chicks (Gallus gallus). Neuroscience 354, 54–68. doi: 10.1016/j.neuroscience.2017.04.022
Lorenzi, E., Pross, A., Rosa-Salva, O., Versace, E., Sgadò, P., and Vallortigara, G. (2019). Embryonic exposure to valproic acid affects social predispositions for dynamic cues of animate motion in newly-hatched chicks. Front. Physiol. 10:501. doi: 10.3389/fphys.2019.00501
Loveland, J. L., Stewart, M. G., and Vallortigara, G. (2019). Effects of oxytocin-family peptides and substance P on locomotor activity and filial preferences in visually naïve chicks. Eur. J. Neurosci. 50, 3674–3687. doi: 10.1111/ejn.14520
Mayer, U., Rosa-Salva, O., and Vallortigara, G. (2017). First exposure to an alive conspecific activates septal and amygdaloid nuclei in visually-naïve domestic chicks (Gallus gallus). Behav. Brain Res. 317, 71–81. doi: 10.1016/j.bbr.2016.09.031
Menon, R., Süß, T., Oliveira, V., Neumann, I., and Bludau, A. (2022). Neurobiology of the lateral septum: regulation of social behavior. Trends Neurosci. 45, 27–40. doi: 10.1016/j.tins.2021.10.010
Mesic, I., Guzman, Y. F., Guedea, A. L., Jovasevic, V., Corcoran, K. A., Leaderbrand, K., et al. (2015). Double dissociation of the roles of metabotropic glutamate receptor 5 and oxytocin receptor in discrete social behaviors. Neuropsychopharmacology 40, 2337–2346. doi: 10.1038/npp.2015.81
Messina, A., Boiti, A., Sovrano, V., and Sgadò, P. (2020). Micromolar valproic acid doses preserve survival and induce molecular alterations in neurodevelopmental genes in two strains of zebrafish larvae. Biomolecules 10:1364. doi: 10.3390/biom10101364
Montagnese, C., Zachar, G., Bálint, E., and Csillag, A. (2008). Afferent connections of septal nuclei of the domestic chick (Gallus domesticus): a retrograde pathway tracing study. J. Comp. Neurol. 511, 109–150. doi: 10.1002/cne.21837
Morales, M., and Margolis, E. (2017). Ventral tegmental area: cellular heterogeneity, connectivity and behaviour. Nat. Rev. Neurosci. 18, 73–85. doi: 10.1038/nrn.2016.165
Nishigori, H., Kagami, K., Takahashi, A., Tezuka, Y., Sanbe, A., and Nishigori, H. (2013). Impaired social behavior in chicks exposed to sodium valproate during the last week of embryogenesis. Psychopharmacology 227, 393–402. doi: 10.1007/s00213-013-2979-y
Opendak, M., Raineki, C., Perry, R., Rincón-Cortés, M., Song, S., Zanca, R., et al. (2021). Bidirectional control of infant rat social behavior via dopaminergic innervation of the basolateral amygdala. Neuron 109, 4018–4035.e7. doi: 10.1016/j.neuron.2021.09.041
Pfaffl, M. W. (2001). A new mathematical model for relative quantification in real-time RT-PCR. Nucleic Acids Res. 29:e45. doi: 10.1093/nar/29.9.e45
Puelles, L., Martinez-de-la-Torre, M., Martinez, S., Watson, C., and Paxinos, G. (2007). The Chick Brain in Stereotaxic Coordinates: An Atlas featuring Neuromeric Subdivisions and Mammalian Homologies. Amsterdam: Elsevier Science.
Román, V., Adham, N., Foley, A., Hanratty, L., Farkas, B., Lendvai, B., et al. (2021). Cariprazine alleviates core behavioral deficits in the prenatal valproic acid exposure model of autism spectrum disorder. Psychopharmacology 238, 2381–2392. doi: 10.1007/s00213-021-05851-6
Sakai, A., Matsuda, T., Doi, H., Nagaishi, Y., Kato, K., and Nakashima, K. (2018). Ectopic neurogenesis induced by prenatal antiepileptic drug exposure augments seizure susceptibility in adult mice. Proc. Natl. Acad. Sci. U. S. A. 115, 4270–4275.
Scheggi, S., Guzzi, F., Braccagni, G., De Montis, M., Parenti, M., and Gambarana, C. (2020). Targeting PPARα in the rat valproic acid model of autism: focus on social motivational impairment and sex-related differences. Mol. Autism 11:62. doi: 10.1186/s13229-020-00358-x
Schiavi, S., Iezzi, D., Manduca, A., Leone, S., Melancia, F., Carbone, C., et al. (2019). Reward-related behavioral, neurochemical and electrophysiological changes in a rat model of autism based on prenatal exposure to valproic acid. Front. Cell Neurosci. 13:479. doi: 10.3389/fncel.2019.00479
Scott-Van Zeeland, A., Dapretto, M., Ghahremani, D., Poldrack, R., and Bookheimer, S. (2010). Reward processing in autism. Autism Res. 3, 53–67.
Sgadò, P., Rosa-Salva, O., Versace, E., and Vallortigara, G. (2018). Embryonic exposure to valproic acid impairs social predispositions of newly-hatched chicks. Sci. Rep. 8:5919. doi: 10.1038/s41598-018-24202-8
Shin, S., Pribiag, H., Lilascharoen, V., Knowland, D., Wang, X.-Y., and Lim, B. K. (2018). Drd3 signaling in the lateral septum mediates early life stress-induced social dysfunction. Neuron 97, 195–208.e6. doi: 10.1016/j.neuron.2017.11.040
Silva, P. A., Trigo, S., Marques, C. I., Cardoso, G. C., and Soares, M. C. (2020). Experimental evidence for a role of dopamine in avian personality traits. J. Exp. Biol. 223:jeb216499. doi: 10.1242/JEB.216499
Solié, C., Girard, B., Righetti, B., Tapparel, M., and Bellone, C. (2022). VTA dopamine neuron activity encodes social interaction and promotes reinforcement learning through social prediction error. Nat. Neurosci. 25, 86–97. doi: 10.1038/s41593-021-00972-9
Sørensen, E. M., Bertelsen, F., Weikop, P., Skovborg, M. M., Banke, T., Drasbek, K. R., et al. (2015). Hyperactivity and lack of social discrimination in the adolescent Fmr1 knockout mouse. Behav. Pharmacol. 26, 733–740. doi: 10.1097/FBP.0000000000000152
Spreckelmeyer, K., Krach, S., Kohls, G., Rademacher, L., Irmak, A., Konrad, K., et al. (2009). Anticipation of monetary and social reward differently activates mesolimbic brain structures in men and women. Soc. Cogn. Affect Neurosci. 4, 158–165. doi: 10.1093/scan/nsn051
Squillace, M., Dodero, L., Federici, M., Migliarini, S., Errico, F., Napolitano, F., et al. (2014). Dysfunctional dopaminergic neurotransmission in asocial BTBR mice. Transl. Psychiatry 4:e427. doi: 10.1038/tp.2014.69
Supekar, K., Kochalka, J., Schaer, M., Wakeman, H., Qin, S., Padmanabhan, A., et al. (2018). Deficits in mesolimbic reward pathway underlie social interaction impairments in children with autism. Brain 141, 2795–2805. doi: 10.1093/brain/awy191
Versace, E., and Vallortigara, G. (2015). Origins of knowledge: insights from precocial species. Front. Behav. Neurosci. 9:338. doi: 10.3389/fnbeh.2015.00338
Wang, Y., Li, G., Stanco, A., Long, J., Crawford, D., Potter, G., et al. (2011). CXCR4 and CXCR7 have distinct functions in regulating interneuron migration. Neuron 69, 61–76. doi: 10.1016/j.neuron.2010.12.005
Wise, R. (2009). Roles for nigrostriatal–not just mesocorticolimbic–dopamine in reward and addiction. Trends Neurosci. 32, 517–524. doi: 10.1016/j.tins.2009.06.004
Yamaguchi, Y., Lee, Y.-A., Kato, A., Jas, E., and Goto, Y. (2017). The roles of dopamine D2 receptor in the social hierarchy of rodents and primates. Sci. Rep. 7:43348. doi: 10.1038/srep43348
Yamamoto, K., and Vernier, P. (2011). The evolution of dopamine systems in chordates. Front. Neuroanat. 5:21. doi: 10.3389/fnana.2011.00021
Yang, S., Edman, L., Sánchez-Alcañiz, J., Fritz, N., Bonilla, S., Hecht, J., et al. (2013). Cxcl12/Cxcr4 signaling controls the migration and process orientation of A9-A10 dopaminergic neurons. Development 140, 4554–4564. doi: 10.1242/dev.098145
Zachar, G., Tóth, A., Gerecsei, L., Zsebõk, S., Ádám, Á, and Csillag, A. (2019). Valproate exposure in ovo attenuates the acquisition of social preferences of young post-hatch domestic chicks. Front. Physiol. 10:881. doi: 10.3389/fphys.2019.00881
Zürcher, N. R., Walsh, E. C., Phillips, R. D., Cernasov, P. M., Tseng, C.-E. J., Dharanikota, A., et al. (2021). A simultaneous [11C]raclopride positron emission tomography and functional magnetic resonance imaging investigation of striatal dopamine binding in autism. Transl. Psychiatry 11:33. doi: 10.1038/s41398-020-01170-0
Keywords: autism spectrum disorder, valproic acid, brain development, dopamine, midbrain, septum, domestic chicks
Citation: Adiletta A, Pross A, Taricco N and Sgadò P (2022) Embryonic Valproate Exposure Alters Mesencephalic Dopaminergic Neurons Distribution and Septal Dopaminergic Gene Expression in Domestic Chicks. Front. Integr. Neurosci. 16:804881. doi: 10.3389/fnint.2022.804881
Received: 29 October 2021; Accepted: 07 February 2022;
Published: 16 March 2022.
Edited by:
Patricia Gaspar, Institut National de la Santé et de la Recherche Médicale (INSERM), FranceReviewed by:
Laurence Goutebroze, Institut National de la Santé et de la Recherche Médicale (INSERM), FranceLesley J. Rogers, University of New England, Australia
Melissa Martin, US Environmental Protection Agency (EPA), United States
Copyright © 2022 Adiletta, Pross, Taricco and Sgadò. This is an open-access article distributed under the terms of the Creative Commons Attribution License (CC BY). The use, distribution or reproduction in other forums is permitted, provided the original author(s) and the copyright owner(s) are credited and that the original publication in this journal is cited, in accordance with accepted academic practice. No use, distribution or reproduction is permitted which does not comply with these terms.
*Correspondence: Paola Sgadò, cGFvbGEuc2dhZG9AdW5pdG4uaXQ=
†These authors have contributed equally to this work and share first authorship