- 1Area of Neurosciences, Biology of Reproduction Department, Ciencias Biológicas y de la Salud, Universidad Autónoma Metropolitana, Unidad Iztapalapa, Mexico City, Mexico
- 2Posgrado en Biología Experimental, Universidad Autónoma Metropolitana, Unidad Iztapalapa, Mexico City, Mexico
- 3Instituto de Investigaciones Biomédicas, Universidad Nacional Autónoma de México, Ciudad Universitaria, Mexico City, Mexico
- 4Departamento de Fisiología, Facultad de Medicina, Universidad Nacional Autónoma de México, Mexico City, Mexico
- 5Instituto Mexicano del Seguro Social, Centro Médico Nacional Siglo XXI, Hospital de Especialidades, Unidad de Investigación Médica en Bioquímica, Mexico City, Mexico
- 6Departamento de Anatomía, Facultad de Medicina, Universidad Nacional Autónoma de México, México City, Mexico
Sleep has a major role in learning, memory consolidation, and metabolic function. Although it is known that sleep restriction increases the accumulation of amyloid β peptide (Aβ) and the risk to develop Alzheimer’s disease (AD), the mechanism behind these effects remains unknown. In this review, we discuss how chronic sleep restriction induces metabolic and cognitive impairments that could result in the development of AD in late life. Here, we integrate evidence regarding mechanisms whereby metabolic signaling becomes disturbed after short or chronic sleep restriction in the context of cognitive impairment, particularly in the accumulation of Aβ in the brain. We also discuss the role of the blood-brain barrier in sleep restriction with an emphasis on the transport of metabolic signals into the brain and Aβ clearance. This review presents the unexplored possibility that the alteration of peripheral metabolic signals induced by sleep restriction, especially insulin resistance, is responsible for cognitive deficit and, subsequently, implicated in AD development.
Introduction
Sleep is a reversible physiological process characterized by the loss of consciousness, reduction in locomotor activity, and decreased response to external stimuli. It also includes important physiological changes that allow the organism to enter an energy-saving status (e.g., decreasing temperature, heart rate, and blood pressure) (Roffwarg et al., 1966; Glotzbach and Heller, 1976; Kräuchi et al., 2000).
The amount of sleep varies considerably from one person to another but on average most adults need about 7 to 8 h of sleep each night to feel well-rested (Hirshkowitz, 2004). Sleep is crucial for the regulation of endocrine functions (Alford et al., 1973; Spiegel et al., 1999; Leproult and Van Cauter, 2009), the immune response (Rico-Rosillo and Vega-Robledo, 2018), memory processing (Rasch and Born, 2013), brain plasticity (Abel et al., 2013), blood-brain barrier regulation (Gómez-González et al., 2013; He et al., 2014), and brain debris clearance (Xie et al., 2013).
Sleep consists of two distinct phases: rapid eye movement (REM) sleep and non(N)-REM sleep, which can be identified using polysomnography (Roffwarg et al., 1966; Hirshkowitz, 2004). NREM sleep is subdivided into three stages; Stages one and two are often considered “light sleep, ” whereas stage three, also called slow wave sleep (SWS), is the deepest stage. In humans, REM sleep and NREM sleep alternate throughout the sleep period (Roffwarg et al., 1966; Saper et al., 2001; Fuller et al., 2006; Irwin, 2015). Total sleep time and the length of each stage decrease throughout life. Particularly, the decrease of REM sleep and SWS is observed in aging (Roffwarg et al., 1966; Li et al., 2018). This fact is relevant in the context of cognitive functions because both REM sleep and SWS are essential for learning and memory consolidation (Ackermann and Rasch, 2014; Boyce et al., 2017).
The common approaches to investigate the physiological role of sleep are: total sleep deprivation (24 h or more without sleep), sleep fragmentation (multiple awaking during total sleep time), and sleep restriction, also known as partial sleep deprivation (sleeping less than the recommended time depending on the age) (Banks and Dinges, 2007; Reynolds and Banks, 2010).
Voluntary sleep restriction is a prevalent problem in modern society (Potter et al., 2016) that affects neurobehavioral and physiological functioning. It contributes to the development of a range of negative health outcomes, including cardiovascular diseases, type 2 diabetes, and neurodegenerative diseases (Kincheski et al., 2017; Chattu et al., 2018).
In this review, we discuss how sleep restriction is related to metabolic and cognitive impairment. Briefly, we propose that chronic sleep restriction may induce the most common neurodegenerative disease worldwide Alzheimer’s disease (AD), by impairing brain insulin signaling.
The Cognitive Cost of Sleep Restriction
Cognitive functioning refers to multiple mental abilities, including learning and memory, and it naturally declines with age. The term “mild cognitive impairment” (MCI) has been used to describe minor cognitive problems that could predispose to the development of neuropathological or psychiatric conditions including AD, vascular dementia, and another types of dementia (Golomb et al., 2004). In the following sections, we restrict the use of the term “cognitive impairment” to refer to the memory deficit associated with sleep restriction, as studied in humans or animal models.
One meta-analysis showed that people who present lower cognitive performance (i.e., low working memory or attention) sleep less than 7 h per night (Mohlenhoff et al., 2018).
In adults, sleep shorter than 7 h in acute or prolonged periods compromises cognitive processes and alertness (Cousins and Fernández, 2019; Zhang et al., 2019). Chronic sleep restriction to either 6 h or 4 h per day for 14 days results in cumulative cognitive performance deficits, including deficits in working memory (Van Dongen et al., 2003; Rhea et al., 2017) This suggests that even relatively moderate sleep restriction has an important neurobiological “cost”. Extending the time of chronic sleep restriction to 6 weeks also impairs cognitive function, particularly spatial orientation and alertness; but these functions may be recovered after 2 nights of proper sleep (Van Dongen et al., 2003; Hennecke et al., 2021).
Worryingly, sleep restriction also affects children and adolescents. In children, sleep restriction increases sleepiness and inattentive behaviors (Fallone et al., 2001). Also, a single night of sleep restriction (>5 h in bed) induced a deficit in verbal creativity and abstract thinking (Randazzo et al., 1998). Adolescents commonly get less than the recommended 8 to 10 h of sleep per night (Owens et al., 2014), which has been associated with a deficit in working memory (Short and Chee, 2019) and executive functions (Lo et al., 2016).
Short sleeping time is often associated with impaired performance in memory tasks (Mantua and Simonelli, 2019); in addition, the relationship between sleep, cognitive deficit, and the prevalence of AD has been confirmed by a meta-analysis (Bubu et al., 2017). A study of nearly 8,000 people found that those who consistently got 6 h of sleep or less per night during 25 years from their 50 s to 60 s were 30 percent more likely to develop dementia later in life, compared with those who slept 7 h per night (Sabia et al., 2021).
β-amyloid peptide (Aβ) aggregation is present in preclinical stages of AD; the excitotoxic and neuroinflammatory properties of Aβ seem to play an important role in the induction of neurodegeneration (Tatarnikova et al., 2015). Another characteristic of AD is the disturbance of the cytoskeleton in nerve cells, which is associated with the hyperphosphorylation of Tau-protein (Tatarnikova et al., 2015).
Sleep restriction worsens the memory impairment and Aβ deposition in a mouse model of AD (Rothman et al., 2013); it can also induce cognitive impairment and Aβ accumulation in animal models without a genetic predisposition to develop AD. Sleep restriction increases the levels of Aβ in the hippocampus (Brice et al., 2020), the expression of Aβ42 and Aβ1–40, and the levels of the enzyme β-secretase that cleaves the Amyloid Precursor Protein (APP) for the synthesis of Aβ (Chen et al., 2017). Recently, studies performed in young mice demonstrated that chronic sleep restriction for 12 months induces hippocampal neurodegeneration along with spatial memory deficits, neuroinflammation, gliosis, and an increase in Aβ42 and hyperphosphorylated tau protein (Owen et al., 2021). Chronic sleep restriction in C57BL/6J mice decreases the number of neurons and volume of the CA1 region of the hippocampus, resulting in lower performance in the conditioning test of place. In addition, lower performance in this test correlated with an increased concentration of Aβ (Owen et al., 2021). This evidence reinforces the idea that Aβ accumulation due to sleep restriction can induce cognitive impairment and neurodegeneration without a genetic predisposition to develop AD.
Several authors have discussed the association between sleep and the pathogenesis of AD via Aβ and other factors like Tau-protein; and some have proposed sleep interventions to reduce AD symptoms (Cordone et al., 2019, 2021; Wang and Holtzman, 2020; Özcan et al., 2020).
Age plays an important role in cognition since aging is characterized by a decrease in REM sleep, an increase in NREM sleep and, frequently, sleep fragmentation, which is related to cognitive decline (Berkley, 2021).
A clinical study in older participants without dementia (>60 years) reported that participants that slept less than 6 h had significantly higher levels of circulating Aβ42 oligomer than those that slept for more than 7 h (Liu et al., 2021).
Furthermore, another study using positron emission tomography (PET), performed in 22 healthy participants (age 22 to 72 years old), revealed that only one night of sleep loss increased the levels of Aβ (Shokri-Kojori et al., 2018). Interestingly, the CSF Aβ42 levels of the participants between the ages of 40 and 60 years old were the highest of all (Ooms et al., 2014), suggesting that sleep loss interferes with the clearance of Aβ (Mendelsohn and Larrick, 2013).
Inflammation is probably one of the mechanisms by which sleep restriction alters brain physiology. During sleep restriction, there is an increase in proinflammatory molecules in the hippocampus (Zielinski et al., 2014; Kincheski et al., 2017), although the origin of these molecules is not well understood. Access of circulating molecules into the hippocampus is limited by the BBB, but several circulating inflammatory molecules, and metabolic signals can cross it. In addition, metabolic signals such as insulin participate in the modulation of synaptic plasticity in the hippocampus (Banks, 2006; Peineau et al., 2018; Lyra e Silva et al., 2021).
Unlike neuroinflammation and neurodegeneration, which are detected after prolonged sleep restriction, systemic changes in metabolic and inflammatory mediators appear after a single night without sleep (Zielinski et al., 2014). Thus, chronic sleep loss could induce a sustained impairment in the signaling of these mediators, contributing to the development of cognitive detriments, and chronically increasing the risk to develop AD.
Sleep Restriction Alters Metabolic Regulation: The Link for Cognitive Impairment
Chronic sleep restriction induces metabolic alterations that lead to metabolic disorders, which in turn are known risk factors for developing AD (Ott et al., 1999; Whitmer et al., 2005, 2008). Acute and chronic sleep loss induces glucose intolerance and insulin resistance; decrease insulin-like growth factor (IGF)-1 and adiponectin levels (Van Leeuwen et al., 2010; Broussard et al., 2015, 2016); and induce dyslipidemia and systemic low-grade inflammation (Gangwisch et al., 2010; Broussard et al., 2015) in non-obese subjects (humans and animal models).
How could the metabolic impairment caused by sleep restriction be the origin of cognitive disturbances? In the next section, we will discuss the metabolic signals associated with sleep restriction that have a direct effect on brain function and cognitive performance.
Insulin: the Main Suspect
Insulin is a hormone secreted by the β cells of the pancreas (Thevis et al., 2009) that has a key role in maintaining normal blood glucose levels by facilitating cellular glucose uptake (Dimitriadis et al., 2011). Insulin resistance occurs when insulin-dependent tissues require increased concentrations of this hormone to achieve the biological effects (Wilcox, 2005).
Sleep loss reduces insulin sensitivity in healthy individuals (Spiegel et al., 2005; Buxton et al., 2010; McNeil et al., 2013; for review see Koren et al., 2015). In humans, a single night of less than 4 h of sleep significantly raises blood glucose levels and reduces insulin sensitivity (Donga et al., 2010). The same has been observed in rats subjected to 4 h of sleep restriction at the beginning or in the middle of the rest period (Jha et al., 2016). On the contrary, recovering normal sleep time is associated with an improvement in insulin sensitivity (Simon et al., 2019). This contributes to the proposal that avoiding sleep loss may help to prevent the development of metabolic syndrome (Leproult and Van Cauter, 2009).
Insulin is necessary for memory and learning (Zhao et al., 2004). Insulin receptors are expressed in brain areas such as the olfactory bulb, hypothalamus, and hippocampus (Kleinridders et al., 2014; Soto et al., 2019). Insulin crosses the BBB in the hippocampus (Spinelli et al., 2019) and regulates the recruitment of N-methyl-D-aspartate (NMDA) receptors in excitatory synapses (Skeberdis et al., 2001), contributing to hippocampal long-term potentiation (LTP) (Van Der Heide et al., 2005). At the same time, insulin is related to the regulation of α-amino-3-hydroxy-5-methyl-4-isoxazolepropionic acid (AMPA) receptors by modulating the endocytosis of these receptors through phosphatidylinositol 3-kinase/protein kinase C (PI3K-PKC; Huang et al., 2004).
Brain insulin resistance can be defined as a failure of brain cells to respond to insulin (Arnold et al., 2018). Memory and learning are impaired in animal models of insulin resistance (Park et al., 2013; Grünblatt et al., 2015; Pratchayasakul et al., 2015), but some studies indicate that intracerebral administration of insulin is able to restore cognitive function (Haj-ali et al., 2009). These studies indicate that insulin transport from the blood into the brain is crucial for cognition through neuronal signaling.
This hypothesis is supported by the fact that insulin receptor (IR), and IGF-1 receptor (IGF1R) specific knock-outs in the hippocampus and in the amygdala not only decrease the expression of AMPA receptors, but also develop into glucose intolerance, anxiety-like behaviors and impaired memory recognition, and spatial memory (Soto et al., 2019).
In AD patients, the activation of the IR is reduced, mainly in the hippocampus and hypothalamus, because of the phosphorylation of serine-residues in the IR substrate (IRS). This phosphorylation prevents the translation of the insulin signal upon binding to the IR (Steen et al., 2005). Furthermore, ex vivo insulin stimulation activated the canonical signaling pathways significantly less in post-mortem brain sections of AD patients as compared to those of healthy subjects, suggesting the presence of central insulin resistance in AD (Talbot et al., 2012).
Also, the inoculation of a viral vector containing short hairpin RNA (ShRNA) against human and rat IRS1 into the dorsal hippocampus of Wistar rats decreased their performance on the T-maze cognitive test and the novel object recognition test, in the absence of pro-inflammatory cytokines (Sánchez-Sarasúa et al., 2021).
These results suggest that brain insulin resistance might be a plausible mechanism that increases the incidence of AD after sleep restriction. Up until now, there is no evidence indicating if sleep restriction induces central insulin resistance; therefore, more studies are needed to explore this relationship. These studies should aim to test whether sleep restriction changes brain IR, modifies insulin transport through the BBB, or promotes any other change in insulin signaling.
Glucose, Hyperglycemia, and Advanced Glycation End Products
Chronic hyperglycemia associated with insulin resistance could influence cognitive performance. Hyperglycemia is associated with deterioration in mood and cognitive function in patients with type 2 diabetes (Greenwood et al., 2003; Sommerfield et al., 2004).
Chronic hyperglycemia leads to the accelerated formation of advanced glycation end products (AGEs), commonly observed in obesity, diabetes, and AD (Cai et al., 2016; Pugazhenthi et al., 2017). AGEs are a very large group of molecules formed from non-enzymatic glycation reactions that affect the structure and function of proteins, amino acids, and nucleic acids (Singh et al., 2001). Under inflammatory conditions such as acute sepsis, the interaction between AGEs, and their receptors (RAGE) in the brain results in the activation of pro-inflammatory genes in the blood, hippocampus, and prefrontal cortex. These changes are associated with neuroinflammation, increased Aβ immunodetection, and enhanced Tau phosphorylation in the hippocampus. In this model, blocking RAGE inhibits neuroinflammation and neurodegeneration markers (Gasparotto et al., 2018). Similar findings indicating that RAGE antagonist or RAGE knockout mice fail to present spatial memory deficits suggest that AGES play a role in cognitive impairment (Cai et al., 2016; Pugazhenthi et al., 2017; Momeni et al., 2021).
In the brain, exposure to high glucose levels for 24 h increases the expression of ionic cotransporters associated with edema formation in rodents (Klug et al., 2021). It also alters brain endothelial cell function and decreases GLUT1 expression, which results in a decrease of glucose uptake into the brain (for a review see Leão et al., 2020). Further, Aβ accumulation is associated with the decrease in membrane expression of the glucose transporter GLUT1 (Winkler et al., 2015), and microvascular endothelial cells are more susceptible to Aβ toxicity under hyperglycemic conditions, suggesting that hyperglycemia is also a risk factor for vascular damage associated with AD (Carvalho et al., 2014). Indeed, AD and type 2 diabetes patients with hyperglycemia have lower glucose uptake by the brain (Hendrix et al., 2021). This suggests that the brain’s glucose supply is altered during neurodegenerative events and that other mechanisms such as the action of AGEs could be involved in the cognitive impairment observed in these patients.
These mechanisms could also be involved in sleep restriction-associated cognitive impairment, because: (1) isolated brain endothelial cells from sleep-restricted mice have lower levels of GLUT1 and decreased 2-deoxy-glucose uptake in the brain (He et al., 2014); and (2) sleep restriction increases the plasmatic soluble RAGE in rats (Liu et al., 2020).
Low-Grade Inflammation
Sleep loss induces a chronic low-grade inflammatory state similar to those observed under obese conditions: it involves a subtle but sustained increase of interleukin 6 (IL-6), tumor necrosis factor (TNF)-α, interleukin 1 (IL-1), interleukin 17A (IL-17A), and C-reactive protein (CRP) (for review (Hurtado-Alvarado et al., 2013; Irwin et al., 2016). Increased IL-6 and TNF-α plasma levels have been associated with glucose intolerance and a reduction of IRS1 in the muscle of sleep-restricted rats (Venancio and Suchecki, 2015). Low-grade inflammation is related to the incidence of AD (Tao et al., 2018), diabetes, and cardiovascular diseases (Vinuesa et al., 2021). In a metabolic context, some pro-inflammatory cytokines such as TNF-α influence insulin sensitivity by activating pathways such as IKK, JNK, and MAPK in the adipose tissue and muscle of obese animals (Hotamisligil, 2006; Chan et al., 2019).
Proinflammatory cytokines in the brain increase after one day of sleep restriction (Zielinski et al., 2014). Those inflammatory factors correlate with Aβ42 deposition and a higher expression of RAGE in the hippocampus and cortex (Liu et al., 2020). IL-1β and TNF-α in the brain also correlate with the increase in β-site APP-cleaving enzyme 1 (BACE1) which is a key molecule that facilitates Aβ accumulation (Liu et al., 2020). Particularly, the high expression of TNF-α in the hippocampus of sleep-restricted animals decreases synaptic connections, as well as performance in the novel object recognition test (Kincheski et al., 2017).
In AD mouse models, TNF-α signaling in hippocampal neurons mediates synapse loss and memory impairment by disrupting insulin signaling (Lourenco et al., 2013). Moreover, IL-6 has been proposed as a key signal that links memory impairment and metabolic dysfunction in AD (Lyra e Silva et al., 2021).
Peripheral signals secreted after sleep restriction are necessary to initiate hippocampal inflammation since BALB/c mice, which have a predominant Th2 activation and fail to produce systemic proinflammatory cytokines after sleep restriction, do not overexpress neuroinflammatory markers in the hippocampus (Hurtado-Alvarado et al., 2018). Thus, the proinflammatory cytokines induced by sleep restriction (e.g., TNF-α and IL-6) could contribute to the development of cognitive impairment and participate in the development of neuroinflammation in the regions involved in memory and learning, probably by modifying insulin sensitivity in the brain.
Other Metabolic Signals Altered by Sleep Restriction
Free Fatty Acids
The sleeping period is accompanied by several hours of fasting, in which the main energy substrate is provided by free fatty acids (FFA) that are released into the circulation from the white adipose tissue (Shostak et al., 2013; Kumar Jha et al., 2015). Under sleep restriction, energy demands are increased due to the waking state of the individual (St-Onge, 2017); if food is not available, these demands could be met by an increased glucose production from the liver, or, alternatively, by increased FFA release and oxidation. Because insulin resistance occurs shortly after sleep deprivation, it is unlikely that glucose would serve as a fuel source in this condition. Indeed, endogenous glucose production does not increase during sleep deprivation (Knutson, 2007), thus, energy requirements must be met with an increase in FFA oxidation. One study found that four consecutive nights of sleep loss in healthy men are associated with an increase in circulating FFA (Broussard et al., 2015). Also, prolonged REM sleep restriction (21 days) in rats produces a substantial decrease in retroperitoneal adipose tissue mass (Venancio and Suchecki, 2015), indicating that lipid mobilization takes place under this condition.
In addition, sleep loss is also associated with an increase in food consumption and preference for highly energetic lipid-rich food (Knutson, 2007; Hogenkamp et al., 2013; Briançon-Marjollet et al., 2015). In this sense, FFA coming either from triglyceride storage or from a high-energy meal could contribute to elevated FFA levels during sleep restriction. In addition, the increase in FFA levels after sleep restriction correlates with insulin resistance (Broussard et al., 2015). In accordance with this, some authors have hypothesized that insulin resistance could be caused by high levels of FFA, which stimulate the production of cytokines in the periphery but also in the brain (Roden et al., 1996; Sears and Perry, 2015). FFA can bind to toll-like receptors in the brain, contributing to an increased inflammatory status (Könner and Brüning, 2011). These observations suggest that increased FFA levels after sleep deprivation could be related to insulin resistance and neuroinflammation, thus providing a possible explanation for how this cascade of events is initiated. More research is still necessary to evaluate if FFA indeed contributes to insulin resistance and the subsequent cognitive impairment observed after sleep restriction, as well as to dissect the contribution of each type of FFA (short chain/long chain, saturated/unsaturated) in these processes.
Insulin-Like Growth Factor-1
IGF-1 is part of the IGF signaling system (also composed of insulin, and insulin receptors) produced mainly in the liver (70%), and its receptor IGF-1R is found predominantly in the hippocampus (Adem et al., 1989; Doré et al., 1997). A single night of sleep loss caused a decrease in circulating IGF-1 levels in humans (Chennaoui et al., 2014) and rats (Everson et al., 2012). Interestingly, the metabolic disturbances induced by sleep loss in rats, such as hyperglycemia and elevated blood pressure can be prevented by a daily administration of systemic IGF-1 (Chen et al., 2014).
Several studies demonstrate that IGF-1 has a key role in synaptic excitability (Xing et al., 2007) and hippocampal neuron polarity (Sosa et al., 2006). It also promotes LTP in the prefrontal cortex and hippocampus, and a decrease in IGF-1 is considered to be an important marker in age-related memory decline (Lynch et al., 2001; Burgdorf et al., 2015).
Defective insulin and IGF-1 signaling in the brain are associated with neurological disorders such as AD (for review see Ferreira, 2021). Moreover, IGF-1/ IGF-1R signaling in the hippocampus is altered in a rat model of vascular dementia; its downregulation contributes to impaired learning and memory (Gong et al., 2012). More evidence is needed to elucidate the role of IGF-1 in the brain after sleep restriction.
Adiponectin
Adiponectin is an adipokine secreted by the adipose tissue in inverse proportion to the amount of fat. Only a handful of studies have described the effects of sleep restriction on adiponectin levels in humans, and the results are controversial. Kotani et al. (2007) found that the amount of systemic adiponectin was negatively correlated with hours of sleep in a study of 109 healthy men. Conversely, a study conducted on Caucasian and African American men and women who were only allowed 4 h of bedtime for 5 consecutive days found that only Caucasian women showed decreased levels of adiponectin (Simpson et al., 2010).
The effect of sleep restriction on adiponectin levels in children and adolescents has also been studied. Hitze et al. (2009) found that sleeping less than 10 h for children or 9 for adolescents correlated with lower levels of adiponectin. As far as we know, there is no evidence of adiponectin changes associated with sleep restriction in animal models. In fact, only one study in rats subjected to sleep deprivation for 96 h measured adiponectin levels and did not find changes (Rosa Neto et al., 2010).
Despite the lack of conclusive evidence, adiponectin is an important candidate for determining the molecules involved in the detrimental effects of sleep restriction in cognition since: (1) adiponectin increases insulin sensitivity (Fang and Judd, 2018); (2) it is considered an anti-inflammatory and protective agent of the vasculature (including BBB; Fang and Judd, 2018); (3) adiponectin receptors are expressed in the central nervous system (CNS; Bloemer et al., 2018); and (4) adiponectin knockout mice perform poorly on the new object recognition test and exhibit LTP deficiency (Bloemer et al., 2019). Further, in a rat model in which olfactory memory impairment and olfactory damage were caused by intracerebroventricular administration of Aβ41–42, intracerebroventricular (icv) adiponectin prevented olfactory memory impairments (Guzmán-Ruiz et al., 2021), which are common early symptoms in patients with MCI and AD.
The study of the central and peripheral alterations of this adipokine in animal models of sleep restriction could provide a potential target to improve insulin resistance and the associated cognitive impairments.
Leptin
Sleep restriction causes a decrease in systemic leptin levels (Spiegel et al., 2004; Taheri et al., 2004). However, the effect of decreased sleep time on systemic leptin levels in humans is controversial. Some reports indicate leptin increases after sleep restriction while systemic meta-analysis indicates that the evidence supporting leptin changes is not strong enough (Reynolds et al., 2012; Zhu et al., 2019).
Still, the role of leptin, an adipokine involved in energy, is worthy to be considered. In rats, sleep restriction decreases leptin levels concomitant to increasing proinflammatory cytokines and decreasing glucose tolerance (Leproult and Van Cauter, 2009; Venancio and Suchecki, 2015; Chen et al., 2017).
Leptin receptors are expressed in different brain regions, including the hippocampus (Håkansson et al., 1998), where leptin can promote spatial learning (Drel et al., 2006) probably via IL-1β receptors (Erion et al., 2014). Mice that do not express the leptin receptor (db/db) exhibit cognitive deficits (Dinel et al., 2011), and obese rodents, which are insensitive to leptin, also show cognitive deficits and have deteriorated LTP (Li et al., 2002; Winocur et al., 2005). The effects of leptin deficiency on cognitive function have been attributed to inflammatory events in obese models, but the role of leptin and the leptin receptor has also been studied in an AD model (Pratap and Holsinger, 2020).
Circulating molecules require access to the brain to display their central function. In this way, the BBB serves as an interface between peripheral signals and the neural environment that is dramatically altered under sleep restriction (Gómez-González et al., 2013; He et al., 2014; Hurtado-Alvarado et al., 2016a, 2018). Moreover, under pathological conditions such as diabetes and AD, there is a reduction in the access of metabolic signals into the brain concomitant to neuroinflammation and blood-brain barrier disruption (Banks, 2006, 2020; Rhea et al., 2017; Rhea and Banks, 2021). Hence, the role of the blood-brain barrier should be considered in the mechanisms by which sleep restriction alters cognitive function.
The Role of the Blood-Brain Barrier in Cognitive Impairment Associated With Sleep Restriction
The optimal function of the BBB is essential for brain function. The BBB limits non-specific transport between endothelial cells by tight junctions and low level of pinocytosis limiting paracellular diffusion of hydrophilic compounds (for review see Gómez-González et al., 2012; Daneman and Prat, 2015; Profaci et al., 2020). The general components of the BBB are microvascular brain endothelial cells, pericytes, astrocytes, and capillary adjacent microglia. The expression of transporters and receptors in brain endothelial cells is responsible for the active transport of circulating nutrients into the brain (e.g., glucose by GLUT1) or the efflux of metabolites from the brain to the circulation such as lipoprotein receptor-related protein 1 (LRP1) (for review see Gómez-González et al., 2012; Daneman and Prat, 2015; Profaci et al., 2020).
Sleep-induced changes in the BBB are related to neuroinflammation, oxidative stress, excitotoxicity, and subsequent neuronal loss (Engelhardt and Sorokin, 2009; Gómez-González et al., 2012). Sleep restriction changes BBB morphology and function. In rats, 10 days of sleep restriction (4 h of sleep per day) increases the BBB permeability to Evans blue, sodium fluorescein, and dextrans (10 and 70 kDa) by the disruption of tight junctions and the increase in pinocytosis (Gómez-González et al., 2013; Hurtado-Alvarado et al., 2016b, 2018). Also, mice that were restricted for 6 days (sleep period 6 h per day) increased their BBB permeability to sodium fluorescein by tight junction disentanglement (He et al., 2014).
Interestingly, the hippocampal BBB permeability of sleep-restricted rats remains higher after 2 h of sleep recovery, unlike other brain areas such as the cortex and basal nuclei that have a complete restoration of basal BBB in this period (Gómez-González et al., 2013; Hurtado-Alvarado et al., 2017). However, 24 h of sleep recovery after sleep restriction is enough to restore the normal permeability of the BBB (He et al., 2014).
Impaired BBB function also affects other components of the neurovascular unit. Sleep restriction induces overexpression of markers classically associated with gliosis such as iba-1 for microglia and glial fibrillar acidic protein (GFAP) for astrocytes. It also increases the expression of the A2A receptor and matrix metalloproteinase (MMP)-9 and induces detachment of pericytes from endothelial cells (Hurtado-Alvarado et al., 2016b; Medina-Flores et al., 2020).
Sleep restriction increases the Aβ deposition in the cortex and hippocampus of sleep-restricted animals (Zhao et al., 2019; Owen et al., 2021). A proposed mechanism that leads to Aβ accumulation is the decrease in LRP1 levels in the brain (Zhao et al., 2019). LRP1 is a major endothelial surface receptor that mediates clearance of Aβ across the BBB so that it may circulate towards the liver and kidneys for systemic clearance (for review see Cockerill et al., 2018). Sleep restriction also increases RAGE in the hippocampus and prefrontal cortex (Zhao et al., 2019). In normal conditions, RAGEs are responsible for the transport of Aβ from the circulation into the brain. Thus, increasing their expression during sleep restriction could further contribute to Aβ deposition in the brain (Deane et al., 2003).
Even though Aβ clearance mechanisms in the BBB remain to be explored under sleep restriction, it is known that Aβ levels in the brain fluctuate along the day, with lower levels during the resting period (Roh et al., 2012; Lucey et al., 2017). This agrees with the evidence that shows that Aβ is largely cleared via the brain’s glymphatic system (Xie et al., 2013).
Hypothetical Mechanism of AD Development Induced by Chronic Sleep Restriction
AD is considered by some authors as a CNS pathology caused by the dysfunction of the BBB. This is based on the hypothesis that Aβ decomposition in the CNS initiates a molecular cascade that causes neurodegeneration (Cockerill et al., 2018). Further, some authors have published reviews that integrate the role of insulin in Aβ transport in the brain and the consequences this may have on AD development (Vandal et al., 2015; Mullins et al., 2017; Rhea et al., 2020; Alves et al., 2021; Rhea and Banks, 2021). Based on the evidence presented, we propose that chronic sleep restriction induces peripheral low-grade inflammation and insulin resistance leading to hyperglycemia which together alter the BBB function (Figure 2). The BBB deterioration associated with sleep restriction can allow the entrance of exogenous molecules such as albumin, dyes, or toxic molecules (i.e., glutamate, urea) but probably impair the entrance of glucose via GLUT1 reduction and central insulin resistance. Since there is a tight relationship between LRP1 levels and IR signaling (Gali et al., 2019), it is possible that, in sleep-restricted rats, the decrease in LRP1 (Zhao et al., 2019) is associated with altered IR signaling, resulting in insulin resistance. Insulin resistance could be involved in the upregulation of RAGE, which would decrease the efflux of Aβ from the brain into the blood. Despite the lack of evidence regarding the relationship between insulin and RAGE in the BBB, mice administrated with streptozotocin (that causes insulin deficiency and type 1 diabetes) have an upregulation of RAGE which would contribute to Aβ deposition in this model (Liu et al., 2009). The decrease in circulating molecules such as adiponectin and IGF-1 during sleep restriction could contribute to the establishment of brain insulin resistance while FFAs could also worsen systemic insulin resistance.
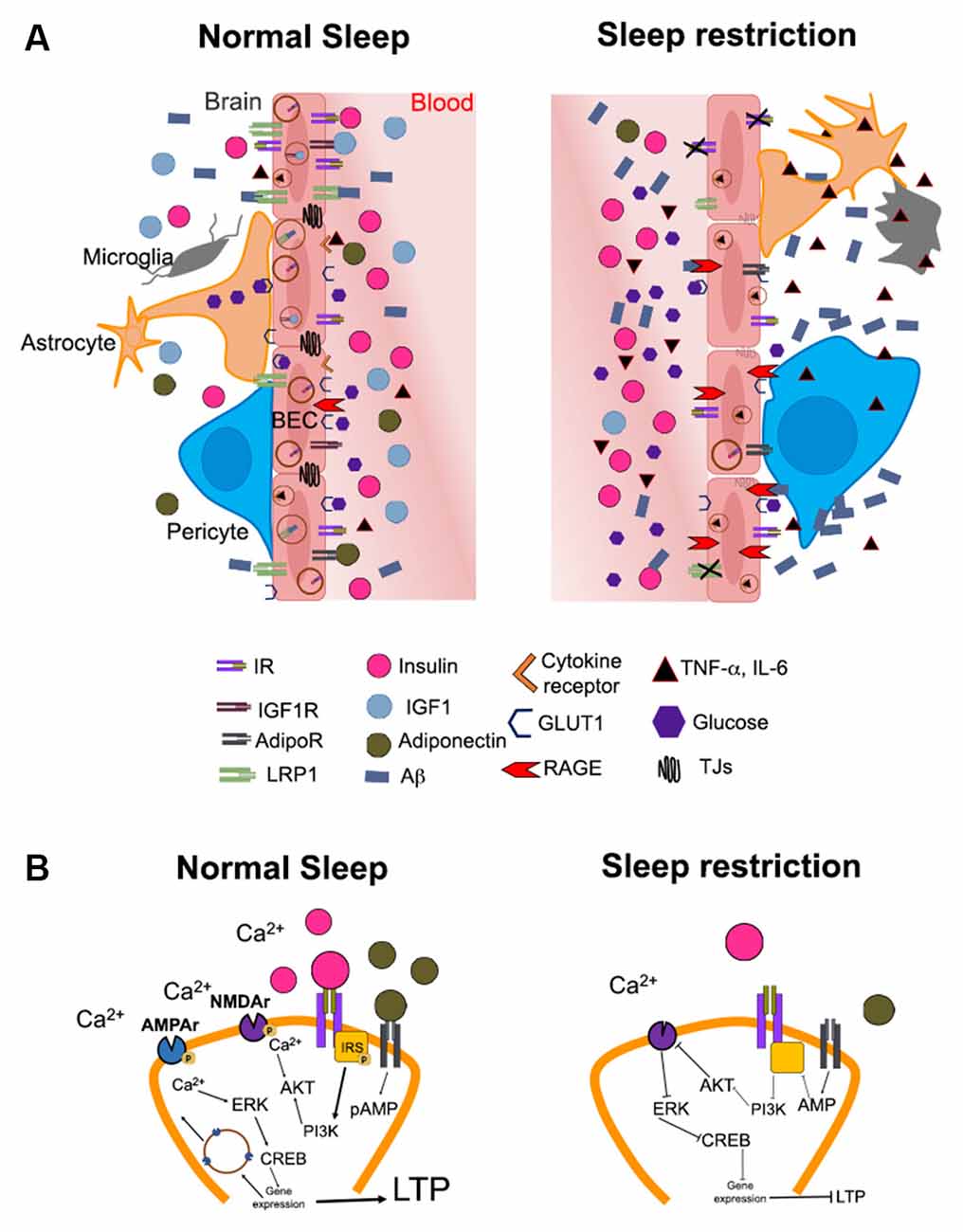
Figure 1. Hypothetical mechanisms by which altered brain insulin signaling induced by sleep restriction may promote the development of Alzheimer’s disease. (A) The illustration shows the possible changes in the access of metabolic signals into the brain (e.g., hippocampus) during normal sleep and sleep restriction. Substances in the periphery are limited in their passage to the brain due to the blood-brain barrier (BBB). The BBB is constituted by brain endothelial cells, pericytes, astrocytes, microglia, and neurons. In normal sleeping conditions (left), metabolic signals such as IGF-1 (blue circles), adiponectin (green circles), glucose (purple hexagons), and insulin (pink circles) circulate in the appropriate concentrations through blood vessels and may bind to their receptors in the brain. The functions of insulin signaling include the modulation of low-density lipoprotein receptor-related protein 1 (LRP1) expression, which mediates amyloid beta (Aβ, blue rectangles) efflux into the bloodstream. IGF-1 and adiponectin bind to their receptors on endothelial cells, possibly increasing insulin sensitivity and modulating the expression of LRP1 receptors. In addition, glucose enters the brain through glucose transporter 1 (GLUT1) for proper brain functioning. Conversely, sleep restriction (right) increases peripheral levels of pro-inflammatory cytokines such as TNF-α and IL-6 (black triangles). The components of the BBB show morphological changes, probably due to an increase in pro-inflammatory cytokines and a decrease in adiponectin and IGF-1. Pericytes detach from endothelial cells, in addition, both astrocytes and microglia change to an “active” morphology due to inflammation. There is also a decrease in the expression of LRP1 due to the impairment in insulin signaling and the decrease of IGF-1 and adiponectin. In this way, the efflux of Aβ from the brain to the blood is decreased, while the expression of receptors for advanced glycation end products (RAGE), which can recapture circulating AB, is increased. The decrease in glucose uptake mediated by GLUT1 during sleep restriction could further contribute to hyperglycemia and the formation of advanced glycation end products (AGEs). In addition, the increase or maintenance of peripheral insulin levels (peripheral insulin resistance) is associated with a decrease in the entrance of insulin into the brain (central insulin resistance), probably due to decreased expression of the insulin receptor (IR). AdipoR and IGF-1 could also decrease in these conditions, although this is not yet fully understood. (B) In normal conditions (left), the binding of the insulin receptor (IR) with its ligand and/or adiponectin with its receptor (AdipoR) causes phosphorylation of the insulin receptor substrate (IRS, yellow square) triggering a signaling cascade that involves the activation of phosphatidylinositol-3 kinase (PI3K) and protein kinase B (AKT). This promotes phosphorylation of the N-methyl-D-aspartate (NMDA) receptor (purple figure) and Ca2+ influx into the neuron. The increase in intracellular Ca2+ promotes the activation of cAMP response element-binding protein (CREB), which functions as a transcription factor for genes that promote the mobilization of α-amino-3-hydroxy-5-methyl-4-isoxazolepropionic acid (AMPA) receptors (blue) to the cell membrane. These events promote long-term potentiation (LTP). On the contrary, during sleep restriction (right), the decrease in insulin in the brain parenchyma combined with the decrease in the concentration of adiponectin, causes a decrease in the phosphorylation of the insulin receptor substrate; therefore, the activation of this signaling pathway is diminished. This decreases AMPA receptors and prevents activation of NMDAs, ultimately producing a decrease in the expression of LTP-related genes.
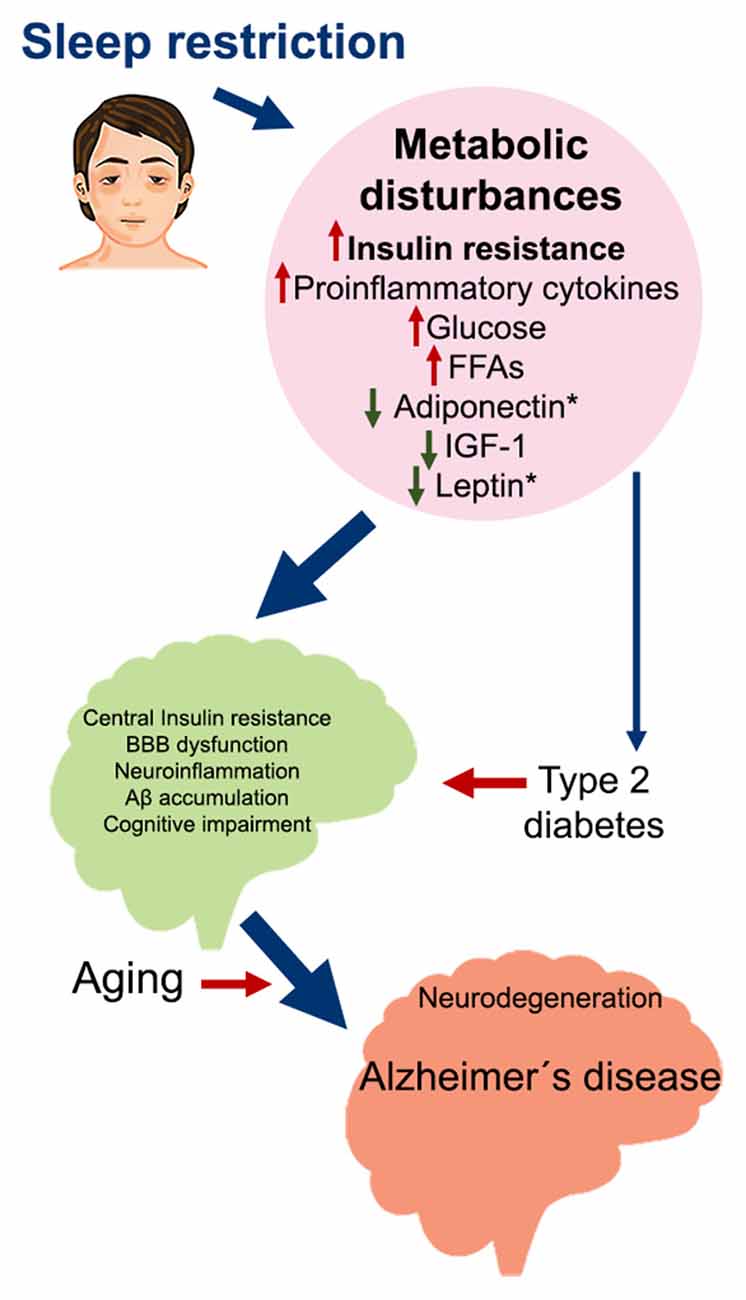
Figure 2. Metabolic impairment induced by sleep restriction in the development of Alzheimer’s disease. Proposed sequence of events that may trigger Alzheimer’s disease as a consequence of chronic sleep restriction. Sleep restriction induces several metabolic disturbances in the periphery, including insulin resistance; increased proinflammatory cytokines, glucose, and FFA; and decreased adiponectin, IGF-1, and leptin. These changes can promote several alterations in the brain, such as central insulin resistance, BBB dysfunction, neuroinflammation, Aβ accumulation, and cognitive impairment. At the same time, persistent metabolic disturbances may develop into Type 2 diabetes, which is also associated with the same brain alterations. On top of this, aging broadens the risk for developing Alzheimer’s disease and probably other neurodegenerative diseases. Abbreviations: FFA, free fatty acids; IGF-1, insulin-like growth factor 1; BBB, blood-brain barrier.
Insulin resistance induced by sleep restriction could also contribute to Aβ accumulation in the brain by the regulation of LRP1 and RAGE function (Figure 1), driving neuroinflammation, neurodegeneration, and cognitive impairment, all factors that contribute to AD development (Figure 2).
In humans, other conditions such as obesity (Simon et al., 2021), circadian disruption (Bolsius et al., 2021), changes in gut microbiota (Ekundayo et al., 2021), and sleep disturbances like obstructive apnea (Siachpazidou et al., 2020) coexist with sleep restriction, which makes it difficult to separate the effects of sleep restriction from the others. In addition, these conditions have a direct relationship with sleep quality, which further promotes the coexistence of these factors. In this sense, bad habits could establish a vicious cycle between metabolic impairments, cognitive deficit, and sleep deficiency, potentially increasing the risk of AD development.
Further Considerations and Concluding Remarks
Chronic sleep loss because of voluntary bedtime restriction is an endemic condition in modern society. Moreover, sleep restriction is a health problem observed since childhood. The fact that children and adolescents get less hours of sleep than recommended and that this has an impact on metabolism is worrying in an obese-prone society. As well as obesity prevention, interventions associated with sleep quality should be proposed as a strategy to reduce AD symptoms (Cordone et al., 2019, 2021).
Special interest has been placed in sleep hygiene to improve the quality of sleep. In this way, it is suggested to avoid the use of psychoactive substances at night (Cordone et al., 2021) such as caffeine (Roehrs and Roth, 2008), energy drinks (Sampasa-Kanyinga et al., 2018), and smoking (McNamara et al., 2014). Conversely, there are various drugs that are used to treat sleep deficiency in AD patients. One of them is melatonin, which improves the amount of sleep in these patients (Xu et al., 2015). Furthermore, cognitive improvement has been observed in patients undergoing long-term melatonin treatment (Wade et al., 2014). Another recommendation to improve sleep time is exercise. People with AD who perform regular physical activity are known to have fewer sleep-related problems when compared to patients who do not exercise regularly (Christofoletti et al., 2011). Exposure to light in the morning is also one of the strategies that, combined with exercise, increases sleep time, and decreases awakenings at night in AD patients (Memon et al., 2020). Girls between the ages of 9 to 11 years that sleep for enough hours for their age but bear social jetlag have a higher BMI. This denotes the importance of the circadian component of sleep and its relationship to metabolic problems that can occur before adulthood. Another important factor to consider is diet. Older adults under a Mediterranean diet which is high in vegetables, cereals, fruits, and seeds have a better quality of sleep (Mamalaki et al., 2018). The extension of sleep time has been proposed as an alternative to reduce metabolic problems and the risk to develop obesity (Hoddy et al., 2020). In other studies, regarding sleep extension, increasing participants’ bedtime has been found to reduce their intake of foods high in fat and carbohydrates (Al Khatib et al., 2018).
It is imperative to recognize the importance of sleep as an essential process for physiology and cognitive function, as well as to acknowledge that the cost of each night with insufficient sleep goes beyond poor concentration and bad mood, it becomes an important risk factor to develop serious conditions such as insulin resistance that, eventually, could make us even more susceptible to neurodegenerative diseases (Figure 2).
Author Contributions
JG-A: conceptualization, investigation, writing, review and editing, and figures designing. RM-H: investigation, writing, review and editing, validation, and language editing. MG-R and NG-V: writing, supervision, review and editing, and validation. MC and JV-M: supervision, review and editing, and validation. GH-A: conceptualization, supervision, writing, review, editing, and validation. All authors contributed to the article and approved the submitted version.
Conflict of Interest
The authors declare that the research was conducted in the absence of any commercial or financial relationships that could be construed as a potential conflict of interest.
Publisher’s Note
All claims expressed in this article are solely those of the authors and do not necessarily represent those of their affiliated organizations, or those of the publisher, the editors and the reviewers. Any product that may be evaluated in this article, or claim that may be made by its manufacturer, is not guaranteed or endorsed by the publisher.
Acknowledgments
We thank CONACyT for the fellowship assigned to JG-A, number 936131.
References
Abel, T., Havekes, R., Saletin, J. M., and Walker, M. P. (2013). Sleep, plasticity and memory from molecules to whole-brain networks. Curr. Biol. 23, R774–R788. doi: 10.1016/j.cub.2013.07.025
Ackermann, S., and Rasch, B. (2014). Differential effects of non-REM and REM sleep on memory consolidation? Curr. Neurol. Neurosci. Rep. 14:430. doi: 10.1007/s11910-013-0430-8
Adem, A., Jossan, S. S., d’Argy, R., Gillberg, P. G., Nordberg, A., Winblad, B., et al. (1989). Insulin-like growth factor 1 (IGF-1) receptors in the human brain: quantitative autoradiographic localization. Brain Res. 503, 299–303. doi: 10.1016/0006-8993(89)91678-8
Al Khatib, H. K., Hall, W. L., Creedon, A., Ooi, E., Masri, T., McGowan, L., et al. (2018). Sleep extension is a feasible lifestyle intervention in free-living adults who are habitually short sleepers: a potential strategy for decreasing intake of free sugars? A randomized controlled pilot study. Am. J. Clin. Nutr. 107, 43–53 doi: 10.1093/ajcn/nqx030
Alford, F. P., Baker, H. W. G., Burger, H. G., de Kretser, D. M., Hudson, B., Johns, M. W., et al. (1973). Temporal patterns of integrated plasma hormone levels during sleep and wakefulness. I. thyroid-stimulating hormone, growth hormone and cortisol. J. Clin. Endocrinol. Metab. 37, 841–847. doi: 10.1210/jcem-37-6-841
Alves, S. S., da Silva-Junior, R. M. P., Servilha-Menezes, G., Homolak, J., Šalković-Petrišić, M., and Garcia-Cairasco, N. (2021). Insulin Resistance as a common link between current Alzheimer’s disease hypotheses. J. Alzheimers Dis. 82, 71–105. doi: 10.3233/JAD-210234
Arnold, S. E., Arvanitakis, Z., Macauley-Rambach, S. L., Koenig, A. M., Wang, H. Y., Ahima, R. S., et al. (2018). Brain insulin resistance in type 2 diabetes and Alzheimer disease: concepts and conundrums. Nat. Rev. Neurol. 14, 168–181. doi: 10.1038/nrneurol.2017.185
Banks, W. A. (2006). Blood-brain barrier and energy balance. Obesity 14, 234S–237S. doi: 10.1038/oby.2006.315
Banks, W. A. (2020). The blood-brain barrier interface in diabetes mellitus: dysfunctions, mechanisms and approaches to treatment. Curr. Pharm. Des. 26, 1438–1447. doi: 10.2174/1381612826666200325110014
Banks, S., and Dinges, D. F. (2007). Behavioral and physiological consequences of sleep restriction. J. Clin. Sleep Med. 3, 519–528. doi: 10.5664/jcsm.26918
Berkley, A. S. (2021). Sleep, aging, and daily functioning. Nurs. Clin. North Am. 56, 287–298. doi: 10.1016/j.cnur.2021.02.007
Bloemer, J., Pinky, P. D., Govindarajulu, M., Hong, H., Judd, R., Amin, R. H., et al. (2018). Role of adiponectin in central nervous system disorders. Neural Plast. 2018:4593530. doi: 10.1155/2018/4593530
Bloemer, J., Pinky, P. D., Smith, W. D., Bhattacharya, D., Chauhan, A., Govindarajulu, M., et al. (2019). Adiponectin knockout mice display cognitive and synaptic deficits. Front. Endocrinol. 10:819. doi: 10.3389/fendo.2019.00819
Bolsius, Y. G., Zurbriggen, M. D., Kim, J. K., Kas, M. J., Meerlo, P., Aton, S. J., et al. (2021). The role of clock genes in sleep, stress and memory. Biochem. Pharmacol. 191:114493. doi: 10.1016/j.bcp.2021.114493
Boyce, R., Williams, S., and Adamantidis, A. (2017). REM sleep and memory. Curr. Opin. Neurobiol. 44, 167–177. doi: 10.1016/j.conb.2017.05.001
Briançon-Marjollet, A., Weiszenstein, M., Henri, M., Thomas, A., Godin-Ribuot, D., and Polak, J. (2015). The impact of sleep disorders on glucose metabolism: endocrine and molecular mechanisms. Diabetol. Metab. Syndr. 7:25. doi: 10.1186/s13098-015-0018-3
Brice, K. N., Hagen, C. W., Peterman, J. L., Figg, J. W., Braden, P. N., Chumley, M. J., et al. (2020). Chronic sleep restriction increases soluble hippocampal Aβ-42 and impairs cognitive performance. Physiol. Behav. 226:113128. doi: 10.1016/j.physbeh.2020.113128
Broussard, J. L., Chapotot, F., Abraham, V., Day, A., Delebecque, F., Whitmore, H. R., et al. (2015). Sleep restriction increases free fatty acids in healthy men. Diabetologia 58, 791–798. doi: 10.1007/s00125-015-3500-4
Broussard, J. L., Kilkus, J. M., Delebecque, F., Abraham, V., Day, A., Whitmore, H. R., et al. (2016). Elevated ghrelin predicts food intake during experimental sleep restriction. Obesity 24, 132–138. doi: 10.1002/oby.21321
Bubu, O., Brannick, M., Mortimer, J., Umasabor-Bubu, O., Sebastião, Y., Wen, Y., et al. (2017). Sleep, cognitive impairment, and Alzheimer’s disease: a systematic review and meta-analysis. Sleep 40:zsw032. doi: 10.1093/sleep/zsw032
Burgdorf, J., Zhang, X., Colechio, E. M., Ghoreishi-Haack, N., Gross, A., Kroes, R. A., et al. (2015). Insulin-like growth factor I produces an antidepressant-like effect and elicits N-methyl-D-aspartate receptor independent long-term potentiation of synaptic transmission in medial prefrontal cortex and hippocampus. Int. J. Neuropsychopharmacol. 19:pyv101. doi: 10.1093/ijnp/pyv101
Buxton, O. M., Pavlova, M., Reid, E. W., Wang, W., Simonson, D. C., and Adler, G. K. (2010). Sleep restriction for 1 week reduces insulin sensitivity in healthy men. Diabetes 59, 2126–2133. doi: 10.2337/db09-0699
Cai, Z., Liu, N., Wang, C., Q27n, B., Zhou, Y., Xiao, M., et al. (2016). Role of RAGE in Alzheimer’s disease. Cell. Mol. Neurobiol. 36, 483–495. doi: 10.1007/s10571-015-0233-3
Carvalho, C., Katz, P. S., Dutta, S., Katakam, P. V. G., Moreira, P. I., and Busija, D. W. (2014). Increased susceptibility to amyloid-β toxicity in rat brain microvascular endothelial cells under hyperglycemic conditions. J. Alzheimers Dis. 38, 75–83. doi: 10.3233/JAD-130464
Chan, K. L., Cathomas, F., and Russo, S. J. (2019). Central and peripheral inflammation link metabolic syndrome and major depressive disorder. Physiology 34, 123–133. doi: 10.1152/physiol.00047.2018
Chattu, V., Manzar, M. D., Kumary, S., Burman, D., Spence, D., and Pandi-Perumal, S. (2018). The global problem of insufficient sleep and its serious public health implications. Healthcare 7:1. doi: 10.3390/healthcare7010001
Chen, L., Huang, J., Yang, L., Zeng, X.-A., Zhang, Y., Wang, X., et al. (2017). Sleep deprivation accelerates the progression of alzheimer’s disease by influencing Aβ-related metabolism. Neurosci. Lett. 650, 146–152. doi: 10.1016/j.neulet.2017.04.047
Chen, H., Zhao, Z., Zheng, J., Chen, X., Zou, J., Shi, Y., et al. (2014). The effect of IGF-1 on symptoms of sleep deprivation in a rat model of inflammatory heart disease and metabolic syndrome. Biochem. Biophys. Res. Commun. 446, 843–849. doi: 10.1016/j.bbrc.2014.02.123
Chennaoui, M., Drogou, C., Sauvet, F., Gomez-Merino, D., Scofield, D. E., and Nindl, B. C. (2014). Effect of acute sleep deprivation and recovery on Insulin-like Growth Factor-I responses and inflammatory gene expression in healthy men. Eur. Cytokine Netw. 25, 52–57. doi: 10.1684/ecn.2014.0356
Christofoletti, G., Oliani, M. M., Bucken-Gobbi, L. T., Gobbi, S., Beinotti, F., and Stella, F. (2011). Physical activity attenuates neuropsychiatric disturbances and caregiver burden in patients with dementia. Clinics 66, 613–618. doi: 10.1590/s1807-59322011000400015
Cockerill, I., Oliver, J.-A., Xu, H., Fu, B. M., and Zhu, D. (2018). Blood-brain barrier integrity and clearance of amyloid-β from the BBB. Adv. Exp. Med. Biol. 1097, 261–278. doi: 10.1007/978-3-319-96445-4_14
Cordone, S., Annarumma, L., Rossini, P. M., and De Gennaro, L. (2019). Sleep and β-amyloid deposition in alzheimer disease: insights on mechanisms and possible innovative treatments. Front. Pharmacol. 10:695. doi: 10.3389/fphar.2019.00695
Cordone, S., Scarpelli, S., Alfonsi, V., De Gennaro, L., and Gorgoni, M. (2021). Sleep-based interventions in Alzheimer’s disease: promising approaches from prevention to treatment along the disease trajectory. Pharmaceuticals 14:383. doi: 10.3390/ph14040383
Cousins, J. N., and Fernández, G. (2019). The impact of sleep deprivation on declarative memory. Prog. Brain Res. 246, 27–53. doi: 10.1016/bs.pbr.2019.01.007
Daneman, R., and Prat, A. (2015). The blood-brain barrier. Cold Spring Harb. Perspect. Biol. 7:a020412. doi: 10.1101/cshperspect.a020412
Deane, R., Du Yan, S., Submamaryan, R. K., LaRue, B., Jovanovic, S., Hogg, E., et al. (2003). RAGE mediates amyloid-β peptide transport across the blood-brain barrier and accumulation in brain. Nat. Med. 9, 907–913. doi: 10.1038/nm890
Dimitriadis, G., Mitron, P., Lambadiari, V., Maratou, E., and Raptis, S. A. (2011). Insulin effects in muscle and adipose tissue. Diabetes Res. Clin. Pract. 93, S52–S59. doi: 10.1016/S0168-8227(11)70014-6
Dinel, A.-L., André, C., Aubert, A., Ferreira, G., Layé, S., and Castanon, N. (2011). Cognitive and emotional alterations are related to hippocampal inflammation in a mouse model of metabolic syndrome. PLoS One 6:e24325. doi: 10.1371/journal.pone.0024325
Donga, E., Van Dijk, M., Van Dijk, J. G., Biermasz, N. R., Lammers, G. J., Van Kralingen, K. W., et al. (2010). A single night of partial sleep deprivation induces insulin resistance in multiple metabolic pathways in healthy subjects. J. Clin. Endocrinol. Metab. 95, 2963–2968. doi: 10.1210/jc.2009-2430
Doré, S., Kar, S., Rowe, W., and Quirion, R. (1997). Distribution and levels of [125I]IGF-I, [125I]IGF-II and [125I]insulin receptor binding sites in the hippocampus of aged memory-unimpaired and -impaired rats. Neuroscience 80, 1033–1040. doi: 10.1016/s0306-4522(97)00154-1
Drel, V. R., Mashtalir, N., Ilnytska, O., Shin, J., Li, F., Lyzogubov, V. V., et al. (2006). The leptin-deficient (ob/ob) mouse: a new animal model of peripheral neuropathy of type 2 diabetes and obesity. Diabetes 55, 3335–3343. doi: 10.2337/db06-0885
Ekundayo, T. C., Olasehinde, T. A., Okaiyeto, K., and Okoh, A. I. (2021). Microbial pathogenesis and pathophysiology of Alzheimer’s disease: a systematic assessment of Microorganisms’ implications in the neurodegenerative disease. Front. Neurosci. 15:648484. doi: 10.3389/fnins.2021.648484
Engelhardt, B., and Sorokin, L. (2009). The blood-brain and the blood-cerebrospinal fluid barriers: function and dysfunction. Semin. Immunopathol. 31, 497–511. doi: 10.1007/s00281-009-0177-0
Erion, J. R., Wosiski-Kuhn, M., Dey, A., Hao, S., Davis, C. L., Pollock, N. K., et al. (2014). Obesity elicits interleukin 1-mediated deficits in hippocampal synaptic plasticity. J. Neurosci. 34, 2618–2631. doi: 10.1523/JNEUROSCI.4200-13.2014
Everson, C. A., Folley, A. E., and Toth, J. M. (2012). Chronically inadequate sleep results in abnormal bone formation and abnormal bone marrow in rats. Exp. Biol. Med. 237, 1101–1109. doi: 10.1258/ebm.2012.012043
Fallone, G., Acebo, C., Arnedt, J. T., Seifer, R., and Carskadon, M. A. (2001). Effects of acute sleep restriction on behavior, sustained attention and response inhibition in children. Percept. Mot. Skills 93, 213–229. doi: 10.2466/pms.2001.93.1.213
Fang, H., and Judd, R. L. (2018). Adiponectin regulation and function. Compr. Physiol. 8, 1031–1063. doi: 10.1002/cphy.c170046
Ferreira, S. T. (2021). Brain insulin, insulin-like growth factor 1 and glucagon-like peptide 1 signalling in Alzheimer’s disease. J. Neuroendocrinol. 33:e12959. doi: 10.1111/jne.12959
Fuller, P. M., Gooley, J. J., and Saper, C. B. (2006). Neurobiology of the sleep-wake cycle: sleep architecture, circadian regulation, and regulatory feedback. J. Biol. Rhythms 21, 482–493. doi: 10.1177/0748730406294627
Gali, C. C., Fanaee-Danesh, E., Zandl-Lang, M., Albrecher, N. M., Tam-Amersdorfer, C., Stracke, A., et al. (2019). Amyloid-β impairs insulin signaling by accelerating autophagy-lysosomal degradation of LRP-1 and IR-β in blood-brain barrier endothelial cells in vitro and in 3XTg-AD mice. Mol. Cell. Neurosci. 99:103390. doi: 10.1016/j.mcn.2019.103390
Gangwisch, J. E., Malaspina, D., Babiss, L. A., Opler, M. G., Posner, K., Shen, S., et al. (2010). Short sleep duration as a risk factor for hypercholesterolemia: analyses of the national longitudinal study of adolescent health. Sleep 33, 956–961. doi: 10.1093/sleep/33.7.956
Gasparotto, J., Girardi, C. S., Somensi, N., Ribeiro, C. T., Moreira, J. C. F., Michels, M., et al. (2018). Receptor for advanced glycation end products mediates sepsis-triggered amyloid-β accumulation, Tau phosphorylation, and cognitive impairment. J. Biol. Chem. 293, 226–244. doi: 10.1074/jbc.M117.786756
Glotzbach, S. F., and Heller, H. C. (1976). Central nervous regulation of body temperature during sleep. Science 194, 537–539. doi: 10.1126/science.973138
Golomb, J., Kluger, A., and Ferris, S. H. (2004). Mild cognitive impairment: historical development and summary of research. Dialogues Clin. Neurosci. 6, 351–367. doi: 10.31887/DCNS.2004.6.4/jgolomb
Gómez-González, B., Hurtado-Alvarado, G., Esqueda-León, E., Santana- Miranda, R., Rojas-Zamorano, J., and Velázquez-Moctezuma, J. (2013). REM sleep loss and recovery regulates blood-brain barrier function. Curr. Neurovasc. Res. 10, 197–207. doi: 10.2174/15672026113109990002
Gómez-González, B., Hurtado-Alvarado, G., and Velázquez-Moctezuma, J. (2012). Local and temporal regulation of the blood-brain barrier during normal and altered physiological states. The Blood-Brain Barrier New Research, 1–42.
Gong, X., Ma, M., Fan, X., Li, M., Liu, Q., Liu, X., et al. (2012). Down-regulation of IGF-1/IGF-1R in hippocampus of rats with vascular dementia. Neurosci. Lett. 513, 20–24. doi: 10.1016/j.neulet.2012.01.077
Greenwood, C. E., Kaplan, R. J., Hebblethwaite, S., and Jenkins, D. J. A. (2003). Carbohydrate-induced memory impairment in adults with type 2 diabetes. Diabetes Care 26, 1961–1966. doi: 10.2337/diacare.26.7.1961
Grünblatt, E., Bartl, J., Iuhos, D.-I., Knezovic, A., Trkulja, V., Riederer, P., et al. (2015). Characterization of cognitive deficits in spontaneously hypertensive rats, accompanied by brain insulin receptor dysfunction. J. Mol. Psychiatry 3:113325. doi: 10.1016/j.jenvman.2021.113325
Guzmán-Ruiz, M. A., Herrera-González, A., Jiménez, A., Candelas-Juárez, A., Quiroga-Lozano, C., Castillo-Díaz, C., et al. (2021). Protective effects of intracerebroventricular adiponectin against olfactory impairments in an amyloid β1–42 rat model. BMC Neurosci. 22:14. doi: 10.1186/s12868-021-00620-9
Haj-ali, V., Mohaddes, G., and Babri, S. H. (2009). Intracerebroventricular insulin improves spatial learning and memory in male wistar rats. Behav. Neurosci. 123, 1309–1314. doi: 10.1037/a0017722
Håkansson, M.-L., Brown, H., Ghilardi, N., Skoda, R. C., and Meister, B. (1998). Leptin receptor immunoreactivity in chemically defined target neurons of the hypothalamus. J. Neurosci. 18, 559–572. doi: 10.1523/JNEUROSCI.18-01-00559.1998
He, J., Hsuchou, H., He, Y., Kastin, A. J., Wang, Y., and Pan, W. (2014). Sleep restriction impairs blood-brain barrier function. J. Neurosci. 34, 14697–14706. doi: 10.1523/JNEUROSCI.2111-14.2014
Hendrix, R. D., Ou, Y., Davis, J. E., Odle, A. K., Groves, T. R., Allen, A. R., et al. (2021). Alzheimer amyloid-β- peptide disrupts membrane localization of glucose transporter 1 in astrocytes: implications for glucose levels in brain and blood. Neurobiol. Aging 97, 73–88. doi: 10.1016/j.neurobiolaging.2020.10.001
Hennecke, E., Lange, D., Steenbergen, F., Fronczek-Poncelet, J., Elmenhorst, D., Bauer, A., et al. (2021). Adverse interaction effects of chronic and acute sleep deficits on spatial working memory but not on verbal working memory or declarative memory. J. Sleep Res. 30:e13225. doi: 10.1111/jsr.13225
Hirshkowitz, M. (2004). Normal human sleep: an overview. Med. Clin. North Am. 88, 551–565. doi: 10.1016/j.mcna.2004.01.001
Hitze, B., Bosy-Westphal, A., Bielfeldt, F., Settler, U., Plachta-Danielzik, S., Pfeuffer, M., et al. (2009). Determinants and impact of sleep duration in children and adolescents: data of the Kiel Obesity Prevention Study. Eur. J. Clin. Nutr. 63, 739–746. doi: 10.1038/ejcn.2008.41
Hoddy, K. K., Potts, K. S., Bazzano, L. A., and Kirwan, J. P. (2020). Sleep extension: a potential target for obesity treatment. Curr. Diab. Rep. 20:81. doi: 10.1007/s11892-020-01360-6
Hogenkamp, P. S., Nilsson, E., Nilsson, V. C., Chapman, C. D., Vogel, H., Lundberg, L. S., et al. (2013). Acute sleep deprivation increases portion size and affects food choice in young men. Psychoneuroendocrinology 38, 1668–1674. doi: 10.1016/j.psyneuen.2013.01.012
Hotamisligil, G. S. (2006). Inflammation and metabolic disorders. Nature 444, 860–867. doi: 10.1038/nature05485
Huang, C.-C., Lee, C.-C., and Hsu, K.-S. (2004). An investigation into signal transduction mechanisms involved in insulin-induced long-term depression in the CA1 region of the hippocampus. J. Neurochem. 89, 217–231. doi: 10.1111/j.1471-4159.2003.02307.x
Hurtado-Alvarado, G., Becerril-Villanueva, E., Contis-Montes de Oca, A., Domínguez-Salazar, E., Salinas-Jazmín, N., Pérez-Tapia, S. M., et al. (2018). The yin/yang of inflammatory status: blood-brain barrier regulation during sleep. Brain Behav. Immun. 69, 154–166. doi: 10.1016/j.bbi.2017.11.009
Hurtado-Alvarado, G., Domínguez-Salazar, E., Pavon, L., Velázquez-Moctezuma, J., and Gómez-González, B. (2016a). Blood-brain barrier disruption induced by chronic sleep loss: low-grade inflammation may be the link. J. Immunol. Res. 2016:4576012. doi: 10.1155/2016/4576012
Hurtado-Alvarado, G., Domínguez-Salazar, E., Velázquez-Moctezuma, J., and Gómez-González, B. (2016b). A2A adenosine receptor antagonism reverts the blood-brain barrier dysfunction induced by sleep restriction. PLoS One 11:e0167236. doi: 10.1371/journal.pone.0167236
Hurtado-Alvarado, G., Pavón, L., Castillo-García, S., Hernández, M., Domínguez-Salazar, E., Velázquez-Moctezuma, J., et al. (2013). Sleep loss as a factor to induce cellular and molecular inflammatory variations. Clin. Dev. Immunol. 2013:801341. doi: 10.1155/2013/801341
Hurtado-Alvarado, G., Velázquez-Moctezuma, J., and Gómez-González, B. (2017). Chronic sleep restriction disrupts interendothelial junctions in the hippocampus and increases blood-brain barrier permeability. J. Microsc. 268, 28–38. doi: 10.1111/jmi.12583
Irwin, M. R. (2015). Why sleep is important for health: a psychoneuroimmunology perspective. Annu. Rev. Psychol. 66, 143–172. doi: 10.1146/annurev-psych-010213-115205
Irwin, M. R., Olmstead, R., and Carroll, J. E. (2016). Sleep disturbance, sleep duration, and inflammation: a systematic review and meta-analysis of cohort studies and experimental sleep deprivation. Biol. Psychiatry 80, 40–52. doi: 10.1016/j.biopsych.2015.05.014
Jha, P. K., Foppen, E., Kalsbeek, A., and Challet, E. (2016). Sleep restriction acutely impairs glucose tolerance in rats. Physiol. Rep. 4:e12839. doi: 10.14814/phy2.12839
Kincheski, G. C., Valentim, I. S., Clarke, J. R., Cozachenco, D., Castelo-Branco, M. T. L., Ramos-Lobo, A. M., et al. (2017). Chronic sleep restriction promotes brain inflammation and synapse loss and potentiates memory impairment induced by amyloid-β oligomers in mice. Brain Behav. Immun. 64, 140–151. doi: 10.1016/j.bbi.2017.04.007
Kleinridders, A., Ferris, H. A., Cai, W., and Kahn, C. R. (2014). Insulin action in brain regulates systemic metabolism and brain function. Diabetes 63, 2232–2243. doi: 10.2337/db14-0568
Klug, N. R., Chechneva, O. V., Hung, B. Y., and O’Donnell, M. E. (2021). High glucose-induced effects on Na+-K+-2Cl− cotransport and Na+/H+ exchange of blood-brain barrier endothelial cells: involvement of SGK1, PKCβII, and SPAK/OSR1. Am. J. Physiol. Cell Physiol. 320, C619–C634. doi: 10.1152/ajpcell.00177.2019
Knutson, K. L. (2007). Impact of sleep and sleep loss on glucose homeostasis and appetite regulation. Sleep Med. Clin. 2, 187–197. doi: 10.1016/j.jsmc.2007.03.004
Könner, A. C., and Brüning, J. C. (2011). Toll-like receptors: linking inflammation to metabolism. Trends Endocrinol. Metab. 22, 16–23. doi: 10.1016/j.tem.2010.08.007
Koren, D., O’Sullivan, K. L., and Mokhlesi, B. (2015). Metabolic and glycemic sequelae of sleep disturbances in children and adults. Curr. Diab. Rep. 15:562. doi: 10.1007/s11892-014-0562-5
Kotani, K., Sakane, N., Saiga, K., Kato, M., Ishida, K., Kato, Y., et al. (2007). Serum adiponectin levels and lifestyle factors in Japanese men. Heart Vessels 22, 291–296. doi: 10.1007/s00380-006-0969-2
Kräuchi, K., Cajochen, C., Werth, E., and Wirz-Justice, A. (2000). Functional link between distal vasodilation and sleep-onset latency? Am. J. Physiol. Regul. Integr. Comp. Physiol. 278, 741–748. doi: 10.1152/ajpregu.2000.278.3.R741
Kumar Jha, P., Challet, E., and Kalsbeek, A. (2015). Circadian rhythms in glucose and lipid metabolism in nocturnal and diurnal mammals. Mol. Cell. Endocrinol. 418, 74–88. doi: 10.1016/j.mce.2015.01.024
Leão, L. L., Tangen, G., Barca, M. L., Engedal, K., Santos, S. H. S., Machado, F. S. M., et al. (2020). Does hyperglycemia downregulate glucose transporters in the brain? Med. Hypotheses 139:109614. doi: 10.1016/j.mehy.2020.109614
Leproult, R., and Van Cauter, E. (2009). Role of sleep and sleep loss in hormonal release and metabolism. Endocr. Dev. 17, 11–21. doi: 10.1159/000262524
Li, X.-L., Aou, S., Oomura, Y., Hori, N., Fukunaga, K., and Hori, T. (2002). Impairment of long-term potentiation and spatial memory in leptin receptor-deficient rodents. Neuroscience 113, 607–615. doi: 10.1016/s0306-4522(02)00162-8
Li, J., Vitiello, M. V., and Gooneratne, N. S. (2018). Sleep in normal aging. Sleep Med. Clin. 13, 1–11. doi: 10.1016/j.jsmc.2017.09.001
Liu, Y., Chen, L., Huang, S., Lv, Z., Hu, L., Luo, J., et al. (2021). Sleep duration and efficiency are associated with plasma amyloid-β7 in non-demented older people. Neurol. Sci. doi: 10.1007/s10072-021-05271-6 [Epub ahead of print].
Liu, L. P., Hong, H., Liao, J. M., Wang, T. S., Wu, J., Chen, S. S., et al. (2009). Upregulation of RAGE at the blood-brain barrier in streptozotocin-induced diabetic mice. Synapse 63, 636–642. doi: 10.1002/syn.20644
Liu, P., Zhao, B., Wei, M., Li, Y., Liu, J., Ma, L., et al. (2020). Activation of inflammation is associated with amyloid-β accumulation induced by chronic sleep restriction in rats. J. Alzheimers Dis. 74, 759–773. doi: 10.3233/JAD-191317
Lo, J. C., Ong, J. L., Leong, R. L. F., Gooley, J. J., and Chee, M. W. L. (2016). Cognitive performance, sleepiness, and mood in partially sleep deprived adolescents: the need for sleep study. Sleep 39, 687–698. doi: 10.5665/sleep.5552
Lourenco, M. V., Clarke, J. R., Frozza, R. L., Bomfim, T. R., Forny-Germano, L., Batista, A. F., et al. (2013). TNF-α mediates PKR-dependent memory impairment and brain IRS-1 inhibition induced by Alzheimer’s β-amyloid oligomers in mice and monkeys. Cell Metab. 18, 831–843. doi: 10.1016/j.cmet.2013.11.002
Lucey, B. P., Fagan, A. M., Holtzman, D. M., Morris, J. C., and Bateman, R. J. (2017). Diurnal oscillation of CSF Aβ and other AD biomarkers. Mol. Neurodegener. 12:36. doi: 10.1186/s13024-017-0161-4
Lynch, C. D., Lyons, D., Khan, A., Bennett, S. A., and Sonntag, W. E. (2001). Insulin-like growth factor-1 selectively increases glucose utilization in brains of aged animals. Endocrinology 142, 506–509. doi: 10.1210/endo.142.1.8053
Lyra e Silva, N. M., Gonçalves, R. A., Pascoal, T. A., Lima-Filho, R. A. S., de Resende, E. P. F., Vieira, E. L. M., et al. (2021). Pro-inflammatory interleukin-6 signaling links cognitive impairments and peripheral metabolic alterations in Alzheimer’s disease. Transl. Psychiatry 11:251. doi: 10.1038/s41398-021-01349-z
Mamalaki, E., Anastasiou, C. A., Ntanasi, E., Tsapanou, A., Kosmidis, M. H., Dardiotis, E., et al. (2018). Associations between the mediterranean diet and sleep in older adults: results from the hellenic longitudinal investigation of aging and diet study. Geriatr. Gerontol. Int. 18, 1543–1548. doi: 10.1111/ggi.13521
Mantua, J., and Simonelli, G. (2019). Sleep duration and cognition: is there an ideal amount? Sleep 42:zsz010. doi: 10.1093/sleep/zsz010
McNamara, J. P., Wang, J., Holiday, D. B., Warren, J. Y., Paradoa, M., Balkhi, A. M., et al. (2014). Sleep disturbances associated with cigarette smoking. Psychol. Health Med. 19, 410–419. doi: 10.1080/13548506.2013.832782
McNeil, J., Doucet, É., and Chaput, J.-P. (2013). Inadequate sleep as a contributor to obesity and type 2 diabetes. Can. J. Diabetes 37, 103–108. doi: 10.1016/j.jcjd.2013.02.060
Medina-Flores, F., Hurtado-Alvarado, G., Contis-Montes de Oca, A., López-Cervantes, S. P., Konigsberg, M., Deli, M. A., et al. (2020). Sleep loss disrupts pericyte-brain endothelial cell interactions impairing blood-brain barrier function. Brain Behav. Immun. 89, 118–132. doi: 10.1016/j.bbi.2020.05.077
Memon, A. A., Coleman, J. J., and Amara, A. W. (2020). Effects of exercise on sleep in neurodegenerative disease. Neurobiol. Dis. 140:104859. doi: 10.1016/j.nbd.2020.104859
Mendelsohn, A. R., and Larrick, J. W. (2013). Sleep facilitates clearance of metabolites from the brain: glymphatic function in aging and neurodegenerative diseases. Rejuvenation Res. 16, 518–523. doi: 10.1089/rej.2013.1530
Mohlenhoff, B. S., Insel, P. S., Mackin, R. S., Neylan, T. C., Flenniken, D., Nosheny, R., et al. (2018). Total sleep time interacts with age to predict cognitive performance among adults. J. Clin. Sleep Med. 14, 1587–1594. doi: 10.5664/jcsm.7342
Momeni, Z., Neapetung, J., Pacholko, A., Kiir, T. A. B., Yamamoto, Y., Bekar, L. K., et al. (2021). Hyperglycemia induces RAGE-dependent hippocampal spatial memory impairments. Physiol. Behav. 229:113287. doi: 10.1016/j.physbeh.2020.113287
Mullins, R. J., Diehl, T. C., Chia, C. W., and Kapogiannis, D. (2017). Insulin resistance as a link between amyloid-β and tau pathologies in Alzheimer’s disease. Front. Aging Neurosci. 9:118. doi: 10.3389/fnagi.2017.00118
Ooms, S., Overeem, S., Besse, K., Rikkert, M. O., Verbeek, M., and Claassen, J. A. H. R. (2014). Effect of 1 night of total sleep deprivation on cerebrospinal fluid β-amyloid 42 in healthy middle-aged men a randomized clinical trial. JAMA Neurol. 71, 971–977. doi: 10.1001/jamaneurol.2014.1173
Ott, A., Stolk, R. P., Van Harskamp, F., Pols, H. A. P., Hofman, A., and Breteler, M. M. B. (1999). Diabetes mellitus and the risk of dementia: the Rotterdam study. Neurology 53, 1937–1942. doi: 10.1212/wnl.53.9.1937
Owens, J., Au, R., Carskadon, M., Millman, R., Wolfson, A., Braverman, P. K., et al. (2014). Insufficient sleep in adolescents and young adults: an update on causes and consequences. Pediatrics 134, e921–e932. doi: 10.1542/peds.2014-1696
Owen, J. E., Zhu, Y., Fenik, P., Zhan, G., Bell, P., Liu, C., et al. (2021). Late-in-life neurodegeneration after chronic sleep loss in young adult mice. Sleep doi: 10.1093/sleep/zsab057 [Epub ahead of Print].
Özcan, G. G., Lim, S., Leighton, P. L. A., Allison, W. T., and Rihel, J. (2020). Sleep is bi-directionally modified by amyloid β oligomers. eLife 9:e53995. doi: 10.7554/eLife.53995
Park, S., Kim, D. S., Kang, S., and Moon, N. R. (2013). β-amyloid-induced cognitive dysfunction impairs glucose homeostasis by increasing insulin resistance and decreasing β-cell mass in non-diabetic and diabetic rats. Metabolism 62, 1749–1760. doi: 10.1016/j.metabol.2013.08.007
Peineau, S., Rabiant, K., Pierrefiche, O., and Potier, B. (2018). Synaptic plasticity modulation by circulating peptides and metaplasticity: involvement in Alzheimer’s disease. Pharmacol. Res. 130, 385–401. doi: 10.1016/j.phrs.2018.01.018
Potter, G. D. M., Skene, D. J., Arendt, J., Cade, J. E., Grant, P. J., and Hardie, L. J. (2016). Circadian rhythm and sleep disruption: causes, metabolic consequences, and countermeasures. Endocr. Rev. 37, 584–608. doi: 10.1210/er.2016-1083
Pratap, A. A., and Holsinger, R. M. D. (2020). Altered brain leptin and leptin receptor expression in the 5xfad mouse model of alzheimer’s disease. Pharmaceuticals 13:401. doi: 10.3390/ph13110401
Pratchayasakul, W., Sa-nguanmoo, P., Sivasinprasasn, S., Pintana, H., Tawinvisan, R., Sripetchwandee, J., et al. (2015). Obesity accelerates cognitive decline by aggravating mitochondrial dysfunction, insulin resistance and synaptic dysfunction under estrogen-deprived conditions. Horm. Behav. 72, 68–77. doi: 10.1016/j.yhbeh.2015.04.023
Profaci, C. P., Munji, R. N., Pulido, R. S., and Daneman, R. (2020). The blood-brain barrier in health and disease: important unanswered questions. J. Exp. Med. 217:e20190062. doi: 10.1084/jem.20190062
Pugazhenthi, S., Qin, L., and Reddy, P. H. (2017). Common neurodegenerative pathways in obesity, diabetes, and Alzheimer’s disease. Bioch. Biophys. Acta 1863, 1037–1045. doi: 10.1016/j.bbadis.2016.04.017
Randazzo, A. C., Muehlbach, M. J., Schweitzer, P. K., and Walsh, J. K. (1998). Cognitive function following acute sleep restriction in children ages 10–14. Sleep 21, 861–868.
Rasch, B., and Born, J. (2013). About sleep’s role in memory. Physiol. Rev. 93, 681–766. doi: 10.1152/physrev.00032.2012
Reynolds, A. C., and Banks, S. (2010). Total sleep deprivation, chronic sleep restriction and sleep disruption. Prog. Brain Res. 185, 91–103. doi: 10.1016/B978-0-444-53702-7.00006-3
Reynolds, A. C., Dorrian, J., Liu, P. Y., van Dongen, H. P. A., Wittert, G. A., Harmer, L. J., et al. (2012). Impact of five nights of sleep restriction on glucose metabolism, leptin and testosterone in young adult men. PLoS One 7:e41218. doi: 10.1371/journal.pone.0041218
Rhea, E. M., and Banks, W. A. (2021). A historical perspective on the interactions of insulin at the blood-brain barrier. J. Neuroendocrinol. 33:e12929. doi: 10.1111/jne.12929
Rhea, E. M., Raber, J., and Banks, W. A. (2020). ApoE and cerebral insulin: trafficking, receptors, and resistance. Neurobiol. Dis. 137:104755. doi: 10.1016/j.nbd.2020.104755
Rhea, E. M., Salameh, T. S., Logsdon, A. F., Hanson, A. J., Erickson, M. A., and Banks, W. A. (2017). Blood-brain barriers in obesity. AAPS J. 19, 921–930. doi: 10.1208/s12248-017-0079-3
Rico-Rosillo, M. G., and Vega-Robledo, G. B. (2018). Sueño y sistema inmune. Rev. Alerg. Méx. 65, 160–170. doi: 10.29262/ram.v65i2.359
Roden, M., Price, T. B., Perseghin, G., Petersen, K. F., Rothman, D. L., Cline, G. W., et al. (1996). Mechanism of free fatty acid-induced insulin resistance in humans. J. Clin. Invest. 97, 2859–2865. doi: 10.1172/JCI118742
Roehrs, T., and Roth, T. (2008). Caffeine: sleep and daytime sleepiness. Sleep Med. Rev. 12, 153–162. doi: 10.1016/j.smrv.2007.07.004
Roffwarg, H. P., Muzio, J. N., and Dement, W. C. (1966). Ontogenetic development of the human sleep-dream cycle. Science 152, 604–619. doi: 10.1126/science.152.3722.604
Roh, J. H., Huang, Y., Bero, A. W., Kasten, T., Stewart, F. R., Bateman, R. J., et al. (2012). Disruption of the sleep-wake cycle and diurnal fluctuation of β-amyloid in mice with Alzheimer’s disease pathology. Sci. Transl. Med. 4:150ra122. doi: 10.1126/scitranslmed.3004291
Rosa Neto, J. C., Lira, F. S., Venancio, D. P., Cunha, C. A., Oyama, L. M., Pimentel, G. D., et al. (2010). Sleep deprivation affects inflammatory marker expression in adipose tissue. Lipids Health Dis. 9:125. doi: 10.1186/1476-511X-9-125
Rothman, S. M., Herdener, N., Frankola, K. A., Mughal, M. R., and Mattson, M. P. (2013). Chronic mild sleep restriction accentuates contextual memory impairments and accumulations of cortical Aβ and pTau in a mouse model of Alzheimer’s disease. Brain Res. 1529, 200–208. doi: 10.1016/j.brainres.2013.07.010
Sabia, S., Fayosse, A., Dumurgier, J., van Hees, V. T., Paquet, C., Sommerlad, A., et al. (2021). Association of sleep duration in middle and old age with incidence of dementia. Nat. Commun. 12:2289. doi: 10.1038/s41467-021-22354-2
Sampasa-Kanyinga, H., Hamilton, H. A., and Chaput, J.-P. (2018). Sleep duration and consumption of sugar-sweetened beverages and energy drinks among adolescents. Nutrition 48, 77–81. doi: 10.1016/j.nut.2017.11.013
Sánchez-Sarasúa, S., Ribes-Navarro, A., Beltrán-Bretones, M. T., and Sánchez-Pérez, A. M. (2021). AAV delivery of shRNA against IRS1 in GABAergic neurons in rat hippocampus impairs spatial memory in females and male rats. Brain Struct. Funct. 226, 163–178. doi: 10.1007/s00429-020-02155-x
Saper, C. B., Chou, T. C., and Scammell, T. E. (2001). The sleep switch: hypothalamic control of sleep and wakefulness. Trends Neurosci. 24, 726–731. doi: 10.1016/s0166-2236(00)02002-6
Sears, B., and Perry, M. (2015). The role of fatty acids in insulin resistance. Lipids Health Dis. 14:121. doi: 10.1186/s12944-015-0123-1
Shokri-Kojori, E., Wang, G.-J., Wiers, C. E., Demiral, S. B., Guo, M., Kim, S. W., et al. (2018). β-Amyloid accumulation in the human brain after one night of sleep deprivation. Proc. Natl. Acad. Sci. U S A 115, 4483–4488. doi: 10.1073/pnas.1721694115
Short, M. A., and Chee, M. W. L. (2019). Adolescent sleep restriction effects on cognition and mood. Prog. Brain Res. 115, 4483–4488. doi: 10.1016/bs.pbr.2019.02.008
Shostak, A., Husse, J., and Oster, H. (2013). Circadian regulation of adipose function. Adipocyte 2, 201–206. doi: 10.4161/adip.26007
Siachpazidou, D. I., Stavrou, V. T., Astara, K., Pastaka, C., Gogou, E., Hatzoglou, C., et al. (2020). Alzheimer’s disease in patients with obstructive sleep apnea syndrome. Tanaffos 19, 176–185.
Simon, S. L., Behn, C. D., Cree-Green, M., Kaar, J. L., Pyle, L., Hawkins, S. M. M., et al. (2019). Too late and not enough: school year sleep duration, timing, and circadian misalignment are associated with reduced insulin sensitivity in adolescents with overweight/obesity. J. Pediatr. 205, 257.e1–264.e1. doi: 10.1016/j.jpeds.2018.10.027
Simon, S. L., Higgins, J., Melanson, E., Wright, K. P. J., and Nadeau, K. J. (2021). A model of adolescent sleep health and risk for type 2 diabetes. Curr. Diab. Rep. 21:4. doi: 10.1007/s11892-020-01373-1
Simpson, N. S., Banks, S., Arroyo, S., and Dinges, D. F. (2010). Effects of sleep restriction on adiponectin levels in healthy men and women. Physiol. Behav. 101, 693–698. doi: 10.1016/j.physbeh.2010.08.006
Singh, R., Barden, A., Mori, T., and Beilin, L. (2001). Advanced glycation end-products: a review. Diabetologia 44, 129–146. doi: 10.1007/s001250051591
Skeberdis, V. A., Lan, J. Y., Zheng, X., Zukin, R. S., and Bennett, M. V. L. (2001). Insulin promotes rapid delivery of N-methyl-D-aspartate receptors to the cell surface by exocytosis. Proc. Natl. Acad. Sci. U S A 98, 3561–3566. doi: 10.1073/pnas.051634698
Sommerfield, A. J., Deary, I. J., and Frier, B. M. (2004). and impairs cognitive performance in people with type 2 diabetes. Diabetes Care 27, 2335–2340. doi: 10.2337/diacare.27.10.2335
Sosa, L., Dupraz, S., Laurino, L., Bollati, F., Bisbal, M., Cáceres, A., et al. (2006). IGF-1 receptor is essential for the establishment of hippocampal neuronal polarity. Nat. Neurosci. 9, 993–995. doi: 10.1038/nn1742
Soto, M., Cai, W., Konishi, M., and Kahn, C. R. (2019). Insulin signaling in the hippocampus and amygdala regulates metabolism and neurobehavior. Proc. Natl. Acad. Sci. U S A 116, 6379–6384. doi: 10.1073/pnas.1817391116
Spiegel, K., Knutson, K., Leproult, R., Tasali, E., and Van Cauter, E. (2005). Sleep loss: a novel risk factor for insulin resistance and Type 2 diabetes. J. Appl. Physiol. 99, 2008–2019. doi: 10.1152/japplphysiol.00660.2005
Spiegel, K., Leproult, R., and Van Cauter, E. (1999). Impact of sleep debt on metabolic and endocrine function. Lancet 354, 1435–1439. doi: 10.1016/S0140-6736(99)01376-8
Spiegel, K., Tasali, E., Penev, P., and Van Cauter, E. (2004). Brief communication: sleep curtailment in healthy young men is associated with decreased leptin levels, elevated ghrelin levels, and increased hunger and appetite. Ann. Intern. Med. 141, 846–850. doi: 10.7326/0003-4819-141-11-200412070-00008
Spinelli, M., Fusco, S., and Grassi, C. (2019). Brain insulin resistance and hippocampal plasticity: mechanisms and biomarkers of cognitive decline. Front. Neurosci. 13:788. doi: 10.3389/fnins.2019.00788
Steen, E., Terry, B. M., Rivera, E. J., Cannon, J. L., Neely, T. R., Tavares, R., et al. (2005). Impaired insulin and insulin-like growth factor expression and signaling mechanisms in Alzheimer’s disease—is this type 3 diabetes? J. Alzheimers Dis. 7, 63–80. doi: 10.3233/jad-2005-7107
St-Onge, M.-P. (2017). Sleep-obesity relation: underlying mechanisms and consequences for treatment. Obes. Rev. 18, 34–39. doi: 10.1111/obr.12499
Taheri, S., Lin, L., Austin, D., Young, T., and Mignot, E. (2004). Short sleep duration is associated with reduced leptin, elevated ghrelin, and increased body mass index. PLoS Med. 1:e62. doi: 10.1371/journal.pmed.0010062
Talbot, K., Wang, H.-Y., Kazi, H., Han, L.-Y., Bakshi, K., Stucky, A., et al. (2012). Demonstrated brain insulin resistance in Alzheimer’s disease patients is associated with IGF-1 resistance, IRS-1 dysregulation, and cognitive decline. J. Clin. Invest. 122, 1316–1338. doi: 10.1172/JCI59903
Tao, Q., Ang, T. F. A., DeCarli, C., Auerbach, S. H., Devine, S., Stein, T. D., et al. (2018). Association of chronic low-grade inflammation with risk of Alzheimer disease in ApoE4 carriers. JAMA Netw. Open 1:e183597. doi: 10.1001/jamanetworkopen.2018.3597
Tatarnikova, O. G., Orlov, M. A., and Bobkova, N. V. (2015). β-amyloid and tau-protein: structure, interaction and prion-like properties. Biochemistry 80, 1800–1819. doi: 10.1134/S000629791513012X
Thevis, M., Thomas, A., and Schänzer, W. (2009). “Insulin,” in, Doping in Sports: Biochemical Principles, Effects and Analysis. Handbook of Experimental Pharmacology, vol 195, eds D. Thieme and P. Hemmersbach (Berlin, Heidelberg: Springer), 209–226.
Van Der Heide, L. P., Kamal, A., Artola, A., Gispen, W. H., and Ramakers, G. M. J. (2005). Insulin modulates hippocampal activity-dependent synaptic plasticity in a N-methyl-D-aspartate receptor and phosphatidyl-inositol-3-kinase-dependent manner. J. Neurochem. 94, 1158–1166. doi: 10.1111/j.1471-4159.2005.03269.x
Van Dongen, H. P. A., Maislin, G., Mullington, J. M., and Dinges, D. F. (2003). The cumulative cost of additional wakefulness: dose-response effects on neurobehavioral functions and sleep physiology from chronic sleep restriction and total sleep deprivation. Sleep 26, 117–126. doi: 10.1093/sleep/26.2.117
Vandal, M., Bourassa, P., and Calon, F. (2015). Can insulin signaling pathways be targeted to transport Aβ out of the brain? Front. Aging Neurosci. 7:114. doi: 10.3389/fnagi.2015.00114
Venancio, D. P., and Suchecki, D. (2015). Prolonged REM sleep restriction induces metabolic syndrome-related changes: mediation by pro-inflammatory cytokines. Brain Behav. Immun. 47, 109–117. doi: 10.1016/j.bbi.2014.12.002
Van Leeuwen, W. M. A., Hublin, C., Sallinen, M., Härmä, M., Hirvonen, A., and Porkka-Heiskanen, T. (2010). Prolonged sleep restriction affects glucose metabolism in healthy young men. Int. J. Endocrinol. 2010:108641. doi: 10.1155/2010/108641
Vinuesa, A., Pomilio, C., Gregosa, A., and Bentivegna, M. (2021). Inflammation and insulin resistance as risk factors and potential therapeutic targets for Alzheimer’s disease. Front. Neurosci. 15:653651. doi: 10.3389/fnins.2021.653651
Wade, A. G., Farmer, M., Harari, G., Fund, N., Laudon, M., Nir, T., et al. (2014). Add-on prolonged-release melatonin for cognitive function and sleep in mild to moderate Alzheimer’s disease: a 6-month, randomized, placebo-controlled, multicenter trial. Clin. Interv. Aging 9, 947–961. doi: 10.2147/CIA.S65625
Wang, C., and Holtzman, D. M. (2020). Bidirectional relationship between sleep and Alzheimer’s disease: role of amyloid, tau, and other factors. Neuropsychopharmacology 45, 104–120. doi: 10.1038/s41386-019-0478-5
Whitmer, R. A., Gunderson, E. P., Barrett-Connor, E., Quesenberry, C. P., and Yaffe, K. (2005). Obesity in middle age and future risk of dementia: a 27 year longitudinal population based study. Br. Med. J. 330, 1360–1362. doi: 10.1136/bmj.38446.466238.E0
Whitmer, R. A., Gustafson, D. R., Barrett-Connor, E., Haan, M. N., Gunderson, E. P., and Yaffe, K. (2008). Central obesity and increased risk of dementia more than three decades later. Neurology 71, 1057–1064. doi: 10.1212/01.wnl.0000306313.89165.ef
Winkler, E. A., Nishida, Y., Sagare, A. P., Rege, S. V., Bell, R. D., Perlmutter, D., et al. (2015). GLUT1 reductions exacerbate Alzheimer’s disease vasculo-neuronal dysfunction and degeneration. Nat. Neurosci. 18, 521–530. doi: 10.1038/nn.3966
Winocur, G., Greenwood, C. E., Piroli, G. G., Grillo, C. A., Reznikov, L. R., Reagan, L. P., et al. (2005). Memory impairment in Obese Zucker rats: an investigation of cognitive function in an animal model of insulin resistance and obesity. Behav. Neurosci. 119, 1389–1395. doi: 10.1037/0735-7044.119.5.1389
Xie, L., Kang, H., Xu, Q., Chen, M. J., Liao, Y., Thiyagarajan, M., et al. (2013). Sleep drives metabolite clearance from the adult brain. Science 342, 373–377. doi: 10.1126/science.1241224
Xing, C., Yin, Y., Chang, R., Gong, X., He, X., and Xie, Z. (2007). Effects of insulin-like growth factor 1 on synaptic excitability in cultured rat hippocampal neurons. Exp. Neurol. 205, 222–229. doi: 10.1016/j.expneurol.2007.01.029
Xu, J., Wang, L.-L., Dammer, E. B., Li, C.-B., Xu, G., Chen, S.-D., et al. (2015). Melatonin for sleep disorders and cognition in dementia: a meta-analysis of randomized controlled trials. Am. J. Alzheimers Dis. Other Dement. 30, 439–447. doi: 10.1177/1533317514568005
Zhang, L., Shao, Y., Liu, Z., Li, C., Chen, Y., and Zhou, Q. (2019). Decreased information replacement of working memory after sleep deprivation: evidence from an event-related potential study. Front. Neurosci. 13:408. doi: 10.3389/fnins.2019.00408
Zhao, W.-Q., Chen, H., Quon, M. J., and Alkon, D. L. (2004). Insulin and the insulin receptor in experimental models of learning and memory. Eur. J. Pharmacol. 490, 71–81. doi: 10.1016/j.ejphar.2004.02.045
Zhao, B., Liu, P., Wei, M., Li, Y., Liu, J., Ma, L., et al. (2019). Chronic sleep restriction induces Aβ accumulation by disrupting the balance of Aβ production and clearance in rats. Neurochem. Res. 44, 859–873. doi: 10.1007/s11064-019-02719-2
Zhu, B., Shi, C., Park, C. G., Zhao, X., and Reutrakul, S. (2019). Effects of sleep restriction on metabolism-related parameters in healthy adults: a comprehensive review and meta-analysis of randomized controlled trials. Sleep Med. Rev. 45, 18–30. doi: 10.1016/j.smrv.2019.02.002
Zielinski, M. R., Kim, Y., Karpova, S. A., McCarley, R. W., Strecker, R. E., and Gerashchenko, D. (2014). Chronic sleep restriction elevates brain interleukin-1 β and tumor necrosis factor-α and attenuates brain-derived neurotrophic factor expression. Neurosci. Lett. 580, 27–31. doi: 10.1016/j.neulet.2014.07.043
Keywords: sleep restriction, cognitive function, Alzheimer’s disease, metabolism, insulin resistance
Citation: García-Aviles JE, Méndez-Hernández R, Guzmán-Ruiz MA, Cruz M, Guerrero-Vargas NN, Velázquez-Moctezuma J and Hurtado-Alvarado G (2021) Metabolic Disturbances Induced by Sleep Restriction as Potential Triggers for Alzheimer’s Disease. Front. Integr. Neurosci. 15:722523. doi: 10.3389/fnint.2021.722523
Received: 08 June 2021; Accepted: 26 July 2021;
Published: 03 September 2021.
Edited by:
Vinay V. Parikh, Temple University, United StatesReviewed by:
Adrian Rodriguez-Contreras, City College of New York (CUNY), United StatesAngel Nunez, Autonomous University of Madrid, Spain
Copyright © 2021 García-Aviles, Méndez-Hernández, Guzmán-Ruiz, Cruz, Guerrero-Vargas, Velázquez-Moctezuma and Hurtado-Alvarado. This is an open-access article distributed under the terms of the Creative Commons Attribution License (CC BY). The use, distribution or reproduction in other forums is permitted, provided the original author(s) and the copyright owner(s) are credited and that the original publication in this journal is cited, in accordance with accepted academic practice. No use, distribution or reproduction is permitted which does not comply with these terms.
*Correspondence: Gabriela Hurtado Alvarado, aHVhbC5nMzEzQGdtYWlsLmNvbQ==; Z2FicmllbGFodXJ0YWRvYWx2QGlpYmlvbWVkaWNhcy51bmFtLm14; Javier Velázquez-Moctezuma, anZlbGF6cXVlem1vY3RlenVtYUBnbWFpbC5jb20=; anZtQHhhbnVtLnVhbS5teA==