- Department of Anatomy and Cell Biology, Schulich School of Medicine & Dentistry, University of Western Ontario, London, ON, Canada
Altered sensory information processing, and auditory processing, in particular, is a common impairment in individuals with autism spectrum disorder (ASD). One prominent hypothesis for the etiology of ASD is an imbalance between neuronal excitation and inhibition. The selective GABAB receptor agonist R-Baclofen has been shown previously to improve social deficits and repetitive behaviors in several mouse models for neurodevelopmental disorders including ASD, and its formulation Arbaclofen has been shown to ameliorate social avoidance symptoms in some individuals with ASD. The present study investigated whether R-Baclofen can remediate ASD-related altered sensory processing reliant on excitation/inhibition imbalance in the auditory brainstem. To assess a possible excitation/inhibition imbalance in the startle-mediating brainstem underlying ASD-like auditory-evoked behaviors, we detected and quantified brain amino acid levels in the nucleus reticularis pontis caudalis (PnC) of rats with a homozygous loss-of-function mutation in the ASD-linked gene Contactin-associated protein-like 2 (Cntnap2) and their wildtype (WT) littermates using Matrix-Assisted Laser Desorption Ionization Mass Spectrometry (MALDI MS). Abnormal behavioral read-outs of brainstem auditory signaling in Cntnap2 KO rats were accompanied by increased levels of GABA, glutamate, and glutamine in the PnC. We then compared the effect of R-Baclofen on behavioral read-outs of brainstem auditory signaling in Cntnap2 KO and WT rats. Auditory reactivity, sensory filtering, and sensorimotor gating were tested in form of acoustic startle response input-output functions, short-term habituation, and prepulse inhibition before and after acute administration of R-Baclofen (0.75, 1.5, and 3 mg/kg). Systemic R-Baclofen treatment improved disruptions in sensory filtering in Cntnap2 KO rats and suppressed exaggerated auditory startle responses, in particular to moderately loud sounds. Lower ASR thresholds in Cntnap2 KO rats were increased in a dose-dependent fashion, with the two higher doses bringing thresholds close to controls, whereas shorter ASR peak latencies at the threshold were further exacerbated. Impaired prepulse inhibition increased across various acoustic prepulse conditions after administration of R-Baclofen in Cntnap2 KO rats, whereas R-Baclofen did not affect prepulse inhibition in WT rats. Our findings suggest that GABAB receptor agonists may be useful for pharmacologically targeting multiple aspects of sensory processing disruptions involving neuronal excitation/inhibition imbalances in ASD.
Introduction
Autism spectrum disorders (ASD) are neurodevelopmental disorders characterized by behavioral deficits in social interaction and unusual social communication as well as stereotyped, repetitive behaviors with restricted interests including sensory issues (DSM-5, 2013). Sensory abnormalities are present in over 90% of children with autism and can lead to great distress in everyday life settings (O’Neill and Jones, 1997; Leekam et al., 2007). Impairments in pre-attentive filtering of inundating sensory information, for example in noisy environments (Ornitz et al., 1993; Braff et al., 2001; Perry et al., 2007; Stevenson et al., 2017), are often accompanied by increased loudness perception (Khalfa et al., 2004; Danesh et al., 2015) and exaggerated reflexive responses to sudden sounds (Chamberlain et al., 2013; Kohl et al., 2014; Takahashi et al., 2016). The neural basis underlying ASD-related differences in sensory and other symptomatic behaviors has been hypothesized to be an imbalance in excitation and inhibition (E/I; Rubenstein and Merzenich, 2003). Indeed, alterations in biomarkers for GABA and glutamate (Glu) abundance and neurotransmission have been described in humans with ASD as well as in a multitude of rodent models with targeted mutations in risk genes for ASD (e.g., Yip et al., 2008; Blatt and Fatemi, 2011; Harada et al., 2011; Coghlan et al., 2012; Sgadò et al., 2013; Gaetz et al., 2014; Bridi et al., 2017; Horder et al., 2018b). Treatment options for ASD are currently limited, although pharmacological agents that modulate E/I balance showed promising preliminary results in clinical trials (for review, see Oberman, 2012; Port et al., 2019). As such, the selective GABAB receptor agonist Arbaclofen or its formulation R-Baclofen has been shown to ameliorate social avoidance symptoms in some individuals with ASD or the related genetic disorder Fragile X Syndrome (Berry-Kravis et al., 2012, 2017; Erickson et al., 2014; Veenstra-VanderWeele et al., 2017) and to improve social behavior deficits and repetitive behaviors in several corresponding genetic mouse models (Henderson et al., 2012; Silverman et al., 2015; Sinclair et al., 2017a; Stoppel et al., 2018). However, to the best of our knowledge, to date, no preclinical or clinical study has thoroughly investigated the potential of R-Baclofen for the treatment of behavioral outcomes of sensory abnormalities associated with ASD.
Homozygous loss-of-function mutations in the Contactin Associated Protein-like 2 (Cntnap2) gene have been identified as one of the rare single gene causes for ASD (Strauss et al., 2006; Poot, 2017). The protein encoded by Cntnap2, the neurexin CASPR2, shows enriched expression in sensory pathways of the brain (Gordon et al., 2016). CASPR2 is involved in neurotransmitter release and excitability through its clustering of voltage-gated potassium channels located at the juxtaparanodes of myelinated axons, at axonal segments, and synaptic terminals (Poliak et al., 2003; Scott et al., 2017). Rats and mice with knockout of the Cntnap2 gene display alterations in sensory processing, both on the neuronal and behavioral level (Peñagarikano et al., 2011; Truong et al., 2015; Scott et al., 2017, 2020; Townsend and Smith, 2017; Dawes et al., 2018). In particular, alterations in brainstem auditory processing and auditory reactivity (Scott et al., 2018, 2020) reflect those reported in individuals with ASD (for review, see Sinclair et al., 2017b).
In the present study, we investigated if selective activation of GABAB receptors can remediate ASD-related altered sensory processing reliant on auditory brainstem function. We compared deficits in behavioral measures of auditory brainstem function from adult female and male Cntnap2 knockout (KO) and wildtype (WT) controls after acute administration of vehicle (saline) or three different doses of R-Baclofen (0.75, 1.5, 3 mg/kg). Reflexive responses to startle-eliciting sounds were used to determine the efficacy of R-Baclofen to normalize acoustic reactivity, sensory filtering (i.e., short-term habituation), and sensorimotor gating (i.e., prepulse inhibition, PPI) in Cntnap2 KO rats. The implicit (reflexive) reactivity to acoustic stimuli is mediated by a short primary pathway in the lower brainstem that activates spinal motor neurons to produce the startle response (Figure 1). An important element of the startle pathway is the nucleus reticularis pontis caudalis (PnC), the sensorimotor interface where cochlear root neurons (CRN) synapse on premotor neurons. Importantly, the transition of sensory input into the motor output can be directly influenced in the PnC by excitatory or inhibitory afferents (for review, see Koch, 1999; Simons-Weidenmaier et al., 2006). To further determine how R-Baclofen affects the transduction of sensory input into motor output within the brainstem startle circuit, we determined the threshold, effective stimulus (ES50), saturation, and slope of the startle input-output (ASR I-O) functions, as well as startle peak latencies. Finally, we quantified GABA, Glu, and glutamine (Gln) levels in the startle mediating PnC from Cntnap2 KO and WT rats using Matrix-Assisted Laser Desorption Ionization Mass Spectrometry (MALDI MS) to determine if E/I imbalance underlies ASD-like deficits in brainstem auditory processing and behavior.
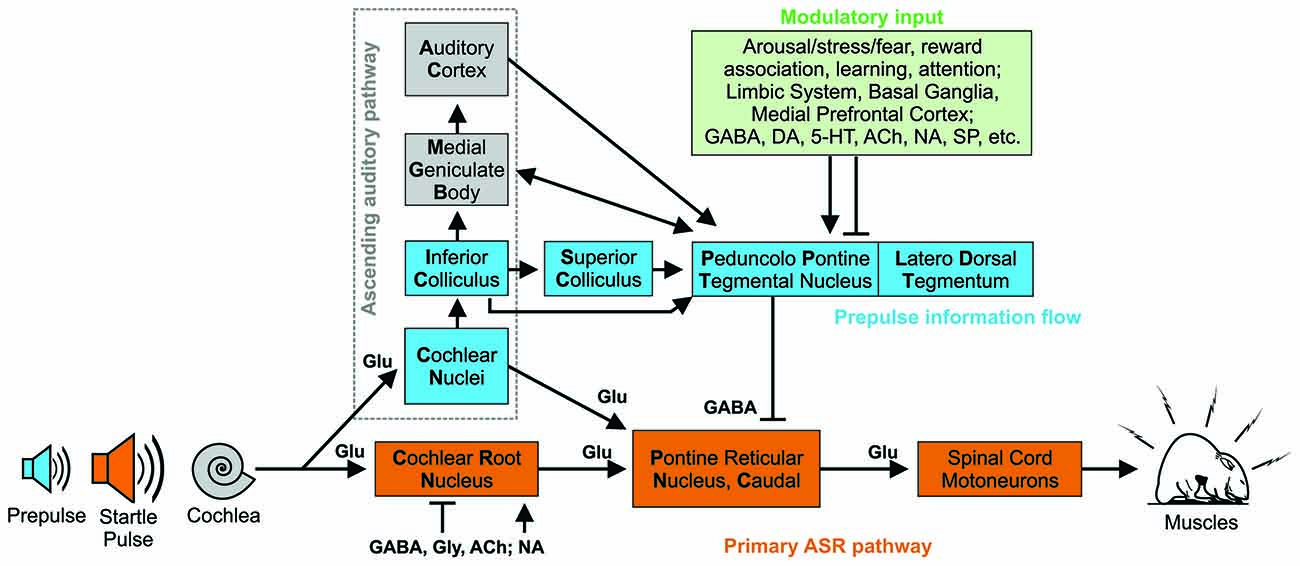
Figure 1. Hypothetical simplified primary and modulatory neural circuitry underlying acoustic startle response and prepulse inhibition with presumed or confirmed neurotransmitters. In the primary ASR pathway (orange), the startle pulse sound information is transmitted via the auditory nerve to cochlear root neurons (CRN) in rodents which give short-latency input to the PnC (Pontine Reticular Nucleus, Caudal). These neurons project directly to cranial and spinal cord motoneurons to produce the motor response. Multiple pathways have been identified for sensorimotor gating modulating ASR. The acoustic prepulse is processed through the ascending auditory pathway (gray dotted frame) via the cochlear nucleus (CN) and the inferior colliculus. From there the prepulse information is transmitted directly or indirectly to the pedunculopontine tegmental nucleus (PPT) and neighboring region laterodorsal tegmental nucleus. Projections from the PPT to the PnC mediate PPI as a form of feed-forward inhibition. The PPT receives modulatory input (green pathway) from a variety of brain structures that can influence PPI e.g., linked with fear and arousal. Abbreviations: ACh, Acetyl choline; GABA, gamma aminobutyric acid; Glu, Glutamate; Gly, Glycine; DA, Dopamine; 5-HT, Serotonine; NA, Noradrenaline, SP, Substance P. Based on data and information from Lauer et al. (2017), Fulcher et al. (2020), and Gómez-Nieto et al. (2020).
Overall, the present study provides preclinical evidence that acute, systemic R-Baclofen treatment reverses many disruptions in brainstem-mediated auditory processing and behavior associated with mutations in the autism-linked gene Cntnap2. These findings support further investigations of GABAB receptor agonists as promising pharmacological targets for the rescue of sensory processing deficits seen in neurodevelopmental disorders including ASD.
Materials and Methods
Animals
Male (M) and female (F) adult Sprague–Dawley wildtype (Cntnap2 WT) and homozygous knockout (Cntnap2 KO) rats were used in this study. Heterozygous breeders were obtained from Horizon Discovery (Boyertown, PA, USA), and all experimental animals were obtained from heterozygous crossings. Rats were housed in a temperature-controlled room on a 12 h light/dark cycle with ad libitum food and water. Behavioral testing was performed during the light phase of the cycle (lights on at 07:00 h). All procedures were approved by the University of Western Ontario Animal Care Committee and were in accordance with the guidelines established by the Canadian Council on Animal Care.
Acoustic Startle Responses (ASRs)
To investigate the effects of R-Baclofen on ASRs to startle-eliciting sounds, rats of the two genotypes (WT: 6 F, 5 M; KO: 6 F, 5 M) were tested after injection of 0.75, 1.5, and 3 mg/kg i.p. R-Baclofen at 8- to 11-months of age. The assessment of acoustic reactivity, sensory filtering, and sensorimotor gating was conducted in sound-attenuating startle boxes (LE116; Panlab) using the StartFear system (Panlab) and STARTLE software module (PACKWIN-CSST, PACKWIN version 2.0; Panlab) as described (Scott et al., 2018, 2020). In brief, using a pressure-sensitive platform, the rat’s acoustic reactivity was measured as the magnitude of its startle response to acoustic stimuli (ASR I-O function) at varying intensities [pulse: 20 ms, 65–115 dB SPL in 5 dB steps, 10 stimuli of each in randomized order, inter-trial interval (ITI): 15, 17.5, or 20 s during a continuous background noise 60 dB SPL white noise]. To determine the startle threshold, effective stimulus ES50, and saturation (Figure 2) of each animal, we first scaled the ASR I-O function of a given animal and treatment between 0 and 1, then fit the scaled function with a GraphPad Prism 8.4.3 in-built model (Model: Standard curves to interpolate—Sigmoidal, 4PL, X is concentration; Method: Prism’s default parameters; Compare: “Do the best-fit values of selected unshared parameters differ between data sets?,” Comparison method: Extra sum-of-squares F test, Parameters: Bottom, Top, ES50, HillSlope; Constrain: constrain bottom to 0, top to 1; Initial values: choose automatically) with the following equation:
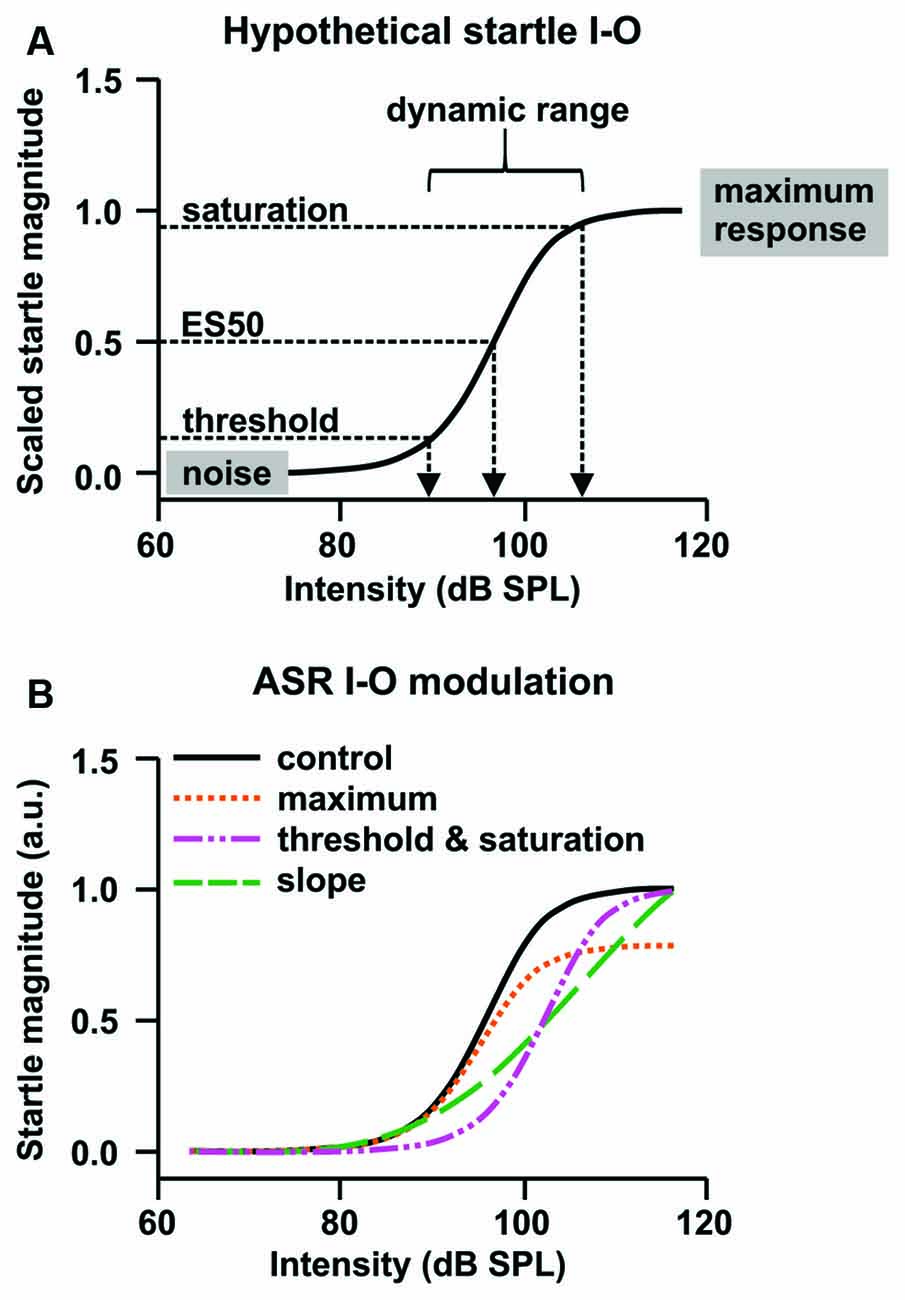
Figure 2. Hypothetical plasticity of ASR input-output (I-O) functions. (A) The sigmoid function describing the relationship between startle stimulus (input) and startle response magnitude (output). The ASR threshold is a putative measure for ASR excitability, the efficient stimulus (ES) for the sound pulse potency, and the ASR maximum for motor capacity. Within the dynamic range of the I-O function, a small stimulus change can produce a large response change. The slope of the dynamic range can be used as metric for the reflex efficiency. (B) The control ASR I-O function (black solid line) could be altered through an increase or decrease in the maximum response to the loudest startle pulse (orange), a left- or right-shift of the curve, thereby increasing or decreasing ASR threshold, ES50, and saturation (pink), a steepening or flattening of the slope of the dynamic range of the function (green), or a combination of these effects (based on data and information from Hince and Martin-Iverson, 2005; Martin-Iverson and Stevenson, 2005). Normalization of the ASR I-O function to the individual startle magnitude at the loudest startle pulse allows analysis of threshold and slope without confounding effects of altered maximum response.
Y is the ASR magnitude
Bottom is the lower plateau of the startle pulse intensity (dB SPL) on the Y axis
Top is the upper plateau of the startle pulse intensity (dB SPL) on the Y axis
HillSlope is the steepness of the slope
ES50 is the startle pulse intensity that gives an ASR magnitude halfway between Bottom and Top.
We then solved the above equation for X and calculated the ASR threshold and saturation for each animal from their individual curve fit parameters using MATLAB R2019a:
The ASR threshold X was defined at Y equal to 25% and the ASR saturation at Y equal to 90% of the span between the Top and Bottom plateau. We chose these values because the estimated ASR threshold and saturation after saline injection matched the between startle pulse intensity comparisons within genotype. ASR peak latencies were extracted within the I-O dynamic range (i.e., we rounded up threshold and rounded down saturation estimates to extract latencies of responses to actually measured sound pulse intensities).
Sensorimotor gating (expressed as the percentage of prepulse inhibition, %PPI) was determined by the extent that the rat’s startle response to the 105 dB SPL pulse was attenuated when a brief prepulse was presented 30 or 100 ms prior to the startle stimulus (prepulse: 10 ms, 65, 75, or 85 dB SPL). Because startle reactivity can affect sensorimotor gating (Csomor et al., 2008), differences in baseline startle magnitude were calculated using the startle-only trials during PPI blocks and analyzed. The control startle stimulus (105 dB SPL) without prepulse and each combination of prepulse lead time and intensity was presented 10 times. The relative percentage of PPI was calculated using the maximum startle amplitudes as follows:
The latency of the startle response was also measured in trials with/without the prepulse, as an increase in startle latency in PPI trials is typical for sensorimotor gating (Ison et al., 1973; Hoffman and Ison, 1980). Relative changes in latency were calculated as the time to reach the maximum startle magnitude on startle pulse-alone trials subtracted from that during prepulse trials, i.e., positive values represented a latency increase (Lyall et al., 2009).
To determine the impact of R-Baclofen on sensory filtering, Cntnap2 WT and KO rats were repeatedly presented a startle-eliciting stimulus (20 ms white noise at 105 dB SPL; 5 ms rise/fall time, ITI: 15, 17.5, or 20 s during a continuous background noise 60 dB SPL white noise) and the degree that their startle response habituated was compared across the genotypes and treatments. Habituation was assessed from the first eight trials with the startle magnitudes normalized to the magnitude at the first trial. A habituation score was calculated for each animal using the following formula (Scott et al., 2018):
A sensitization score was calculated for each animal using the following formula (Meincke et al., 2004):
Before the behavioral procedures associated with the ASR (i.e., acoustic reactivity, sensory filtering, and sensorimotor gating), animals were handled and acclimated to the startle boxes over three 10 min sessions. During these acclimation sessions, only background noise (60 dB SPL, white noise) was presented to the animals.
Drug Application
R-Baclofen (provided by Simons Foundation Autism Research Initiative, SFARI through Clinical Research Assoc., LLC) was dissolved in 0.9% saline freshly on each experimental day and administered intraperitoneally (i.p., injection volume: 1 ml/kg) 1 h before the start of the test session in doses of 0.75, 1.5, and 3 mg/kg. Doses and injection time before testing were chosen in accordance with the literature (Henderson et al., 2012; Silverman et al., 2015; Lorrai et al., 2016). The vehicle condition was represented by the administration of an equal volume of saline. In the first experimental block (Supplementary Figure 1), each treatment was administered on two consecutive days in the following order: saline (Day 1&2), R-Baclofen at 0.75 mg/kg (Day 3&4), 1.5 mg/kg (Day 5&6), and 3 mg/kg (Day 7&8). We chose to inject R-Baclofen in increasing doses to avoid the need for week-long washout times between treatments (Henderson et al., 2012) and to keep behavioral testing as concise as possible. On the first day of each dose, injections of saline or R-Baclofen were followed by behavioral tests for sensory filtering (habituation) and acoustic reactivity (ASR I-O), and on the second day by the behavioral test for sensorimotor gating (PPI). After the first experimental block and a 1-week washout period, we repeated the same sequence of behavioral procedures over 8 days with saline injection. This second experimental block was used to control for effects of repeated testing. Over 2 days preceding either experimental block, rats were habituated to handling, behavioral testing, and intraperitoneal injections; specifically, rats received one injection of 1 ml/kg saline 1 h prior to testing on both days (not shown in Supplementary Figure 1). No statistical differences were found for either genotype between saline treatments across the two experimental blocks for habituation and acoustic reactivity and data were pooled across days for the most accurate genotype comparisons after saline. For PPI of ASR, genotype comparisons after saline were made based on Day 2 (Supplementary Figure 1) because there was a significant difference between the PPI of the two experimental blocks. Repeated testing within the second experimental block did not alter the PPI in Cntnap2 WT and KO rats (Supplementary Figure 2) and we assumed that effects of repeated testing within the first experimental block were also negligible. For most consistent comparisons of R-Baclofen treatment effects within or between genotypes, data were compared within the first treatment block.
MALDI
In order to analyze if altered brain amino acid abundances underlie the Cntnap2-linked changes in auditory-evoked behaviors, 8 Cntnap2 WT (4 F, 4 M) and 8 Cntnap2 KO (4 F, 4 M) rats were deeply anesthetized with carbon dioxide and decapitated at 4- to 5-months-old. Brains were extracted and fresh frozen, and stored at −80°C, until cryosectioned at 10μm (Thermo-Fisher Scientific CryoStar NX50), and mounted on Indium tin oxide (ITO)-coated glass slides (Hudson Surface Technology Inc., Old Tappan, NJ, USA). Zinc oxide (Sigma-Aldrich, St. Louis, MO, USA) was selected as the MALDI matrix and prepared to 1 mg/ml in 50% ACN and 0.1% TFA (Fisher Scientific, Waltham, MA, USA) and applied onto the slides with TM-SprayerTM (HTX Technologies, LLC, Chapel Hill, NC, USA). Afterward, α-cyano-4-hydroxycinnamic acid standards were spotted onto the slides for internal mass calibration. ZnO matrix deposition using the TM Sprayer and MS data analysis of neurotransmitters were performed as previously described (Chen et al., 2021). MALDI-MS sample analyses were performed on a Sciex TOF/TOF 5800 MALDI mass spectrometer (Sciex, Framingham, MA, USA). Images were acquired in the positive ion reflectron mode at a mass range of 50–300 m/z using the TOF-TOF Series Explorer and Data Explorer were used for data acquisition and processing, respectively (Sciex). MS images were acquired at 70 μm raster with 50 shots/spectrum, and the laser energy was optimized based on the signal intensity, peak resolution and signal-to-noise ratio. MALDI MS images were visualized and analyzed through an experimentally blinded observer using Tissue ViewTM Software IDLVM (Sciex). PnC and superior olivary complex (SOC) region of interest in the brainstem were manually selected to generate the average mass spectra. Mass peaks corresponding to neurotransmitters and metabolites ([GABA+K]+: 142m/z, [Glu+K]+: 186m/z, [Gln+K]+: 185m/z, [Choline+K]+: 143m/z, [Norepinephrine+K]+: 208m/z) were acquired from each mass spectra (Chen et al., 2021). Comparative analysis was performed based on the area under the curve (AUC) ratio (ratio between the peak of interest AUC to total AUC from 100 to 250m/z; Caughlin et al., 2017). Intensity values corresponding to the mass peaks were compared between respective WT-KO pairs on the slides. One female WT-KO pair had to be excluded due to significant tearing in the tissue that left the PnC region of interest unusable, resulting in three pairs of female and four pairs of male Cntnap2 WT-KO rats. No significant differences between data from females and males were observed and data were pooled.
Statistical Analysis
Unless otherwise stated, data that followed normal distribution are presented as group mean with standard deviation (SD), and not normally distributed data as group median with interquartile range (IQR). Depending on the experimental design and distribution of the data, differences of the means were compared for statistical significance either by student’s t-test, paired t-test, Welch t-test, one sample t-test, one sample Wilcoxon test, Mann–Whitney test, 2-way ANOVA, repeated measures (RM) ANOVA, Mixed-effect analysis, or Friedmann tests using GraphPad Prism 8.4.3 (La Jolla, USA). For 2-way ANOVA comparisons we did not assume sphericity because R-Baclofen was administered in consecutive, increasing doses (Supplementary Figure 1) and we used Greenhouse-Geisser correction where applicable. Two-way ANOVA, RM ANOVA, Mixed-effect analysis, or Friedmann tests were followed by multiple comparison tests with correction for type 1 error after Sidak’s, Dunnett’s, or Dunns’s multiple comparisons test. The relative amount of prepulse inhibition was additionally analyzed by random permutation tests in consideration of small sample sizes to estimate the population mean from samples (resampling by bootstrapping, property mean, 10,000 random samples without replacement). Statistical significance level was α = 0.05, and resulting p values are reported in the legends using: (*)p < 0.1, *p ≤ 0.05; **p ≤ 0.01; ***p ≤ 0.001; n.s., not significant.
Results
Cntnap2 KO Rats Display Deficient Short-Term Habituation, Exaggerated Sensitization, and Increased Acoustic Reactivity
In order to investigate whether selective activation of GABAB receptors can remediate ASD-related altered sensory processing reliant on auditory brainstem function, we analyzed auditory reactivity, filtering, and sensorimotor gating in adult Cntnap2 KO rats (n = 6 F, n = 5 M) in comparison to WT littermates (n = 6 F, n = 5 M) after acute administration of R-Baclofen (0.75, 1.5, and 3 mg/kg) or vehicle (saline).
To most accurately assess genotype-related differences between Cntnap2 WT and KO rats in sensory filtering and acoustic reactivity (Figure 3), we first averaged the respective data from the five experimental days where short-term habituation and ASR I-O trials were measured 1 h after saline injection (Day # 1, 16, 18, 20, 22, see timeline in Supplementary Figure 1). To assess sensory filtering, short-term habituation of the startle response was measured across the first eight startle trials of the test day. Short-term habituation across the first eight startle trials of the test day revealed significantly less declined startle responses in Cntnap2 KO compared with WT rats, in particular at trial number eight (Cntnap2 WT: n = 11, Cntnap2 KO: n = 11, two-way RM ANOVA, trial × genotype p = 0.0225, F(7,140) = 2.425, trial p = 0.0012, F(4.714, 94.28) = 4.528, genotype p = 0.0053, F(1,20) = 9.792, Sidak’s multiple comparisons test, p = 0.0151, Figure 3B), indicating that KO rats do not habituate across trials. Habituation scores calculated from trial 7 and 8 in relation to trial 1 were significantly increased in Cntnap2 KO rats compared with WT rats (Figure 3C Left, Cntnap2 WT: 0.78 ± 0.11, KO: 0.97 ± 0.18 dB SPL, two-sided student’s t-test p = 0.0082), confirming perturbed habituation across trials and indicating impaired sensory filtering in Cntnap2 KO rats. Furthermore, Cntnap2 KO rats displayed greater sensitization scores calculated from trial 2–4 in relation to trial 1 than Cntnap2 WT rats (Figure 3C Right, Cntnap2 WT:0.82 ± 0.15, KO:0.99 ± 0.16, two-sided student’s t-test p = 0.0150).
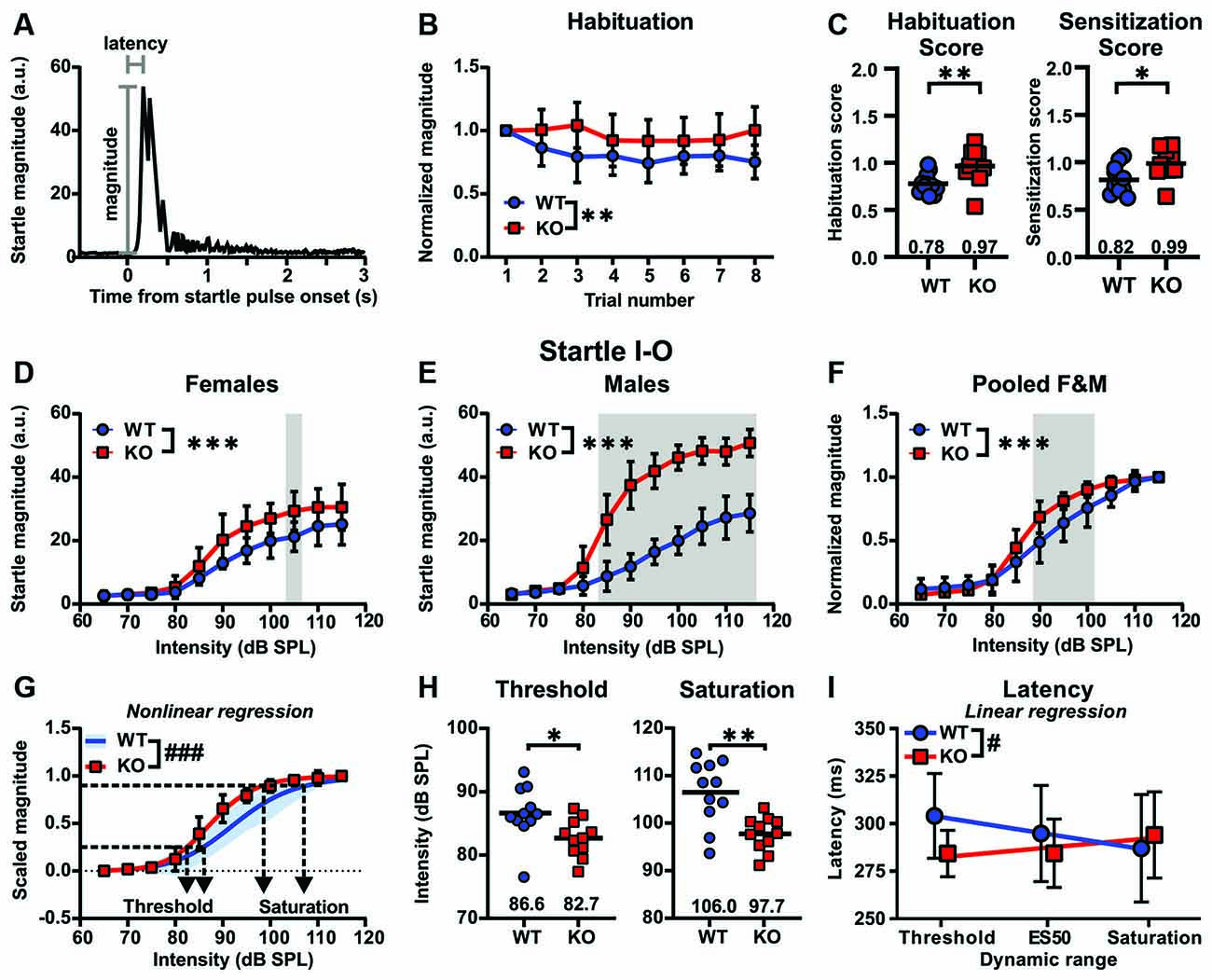
Figure 3. Impaired short-term habituation and increased acoustic reactivity in Cntnap2 KO compared with wildtype (WT) rats. (A) Schematic raw acoustic startle trace to a 115 dB SPL startle pulse. ASR peak magnitude and latency is determined within the recording window of 500 ms from startle pulse onset. (B,C) Sensory filtering as measured by short-term habituation is perturbed in Cntnap2 KO rats. (B) Mean ± standard deviation (SD) startle response magnitudes across eight subsequent trials normalized to the first trial in Cntnap2 WT and KO rats. Values <1 indicate habituation of the startle response. Cntnap2 KO rats showed less declined startle responses than WT rats, indicating perturbed habituation, in particular at trial number eight (Cntnap2 WT: n = 11, Cntnap2 KO: n = 11, two-way repeated measures (RM) ANOVA, trial × genotype p = 0.0225, F(7,140) = 2.425, trial p = 0.0012, F(4.714, 94.28) = 4.528, genotype p = 0.0053, F(1,20) = 9.792, Sidak’s multiple comparisons test, p = 0.0151). (C) Left: Individual habituation scores calculated from the average of the last two trials divided by that of the first trial (WT: blue circles, KO: red squares, mean: horizontal black line). Values <1 indicate habituation of the startle response. Cntnap2 KO rats had significantly greater habituation scores compared with WT rats (two-sided student’s t-test p = 0.0082). Right: Individual sensitization scores calculated from the average of trials 2–4 divided by that of the first trial (WT: blue circles, KO: red squares, mean: horizontal black line). Cntnap2 KO rats showed greater sensitization scores than WT rats (two-sided student’s t-test p = 0.0150). (D–F) Mean ± SD startle responses in female (F) (D), male (M) (E), and pooled male and female (C) Cntnap2 WT (blue circles) and KO (red squares) rats to startle pulses with sound intensities from 65 to 115 dB SPL in 5 dB steps. (D) Acoustic startle magnitudes were significantly increased in female Cntnap2 KO (WT: n = 6, KO: n = 6, two-way ANOVA, intensity × genotype p = 0.1598, F(10,110) = 1.471, intensity p < 0.0001, F(10,110) = 61.70, genotype p < 0.0001, F(1,110) = 28.83) and (E) male Cntnap2 KO rats (WT: n = 5, KO: n = 5, two-way ANOVA, intensity × genotype p < 0.0001, F(10,88) = 14.97, intensity p < 0.0001, F(10,88) = 100.1, genotype p < 0.0001, F(1,88) = 301.7) compared with WT littermates when collapsing over intensities, indicated by a leftward shift of the I-O ASR function. In particular, startle magnitudes were elevated in female Cntnap2 KO at 105 dB SPL (Sidak’s multiple comparisons test, p = 0.0303) and in male Cntnap2 KO rats at 85–115 dB SPL (Sidak’s multiple comparisons test, all p < 0.0001). (F) Normalized ASR I-O functions pooled for male and female Cntnap2 WT and KO rats were significantly different (Cntnap2 WT: n = 11, Cntnap2 KO: n = 11, two-way ANOVA intensity × genotype p < 0.0001, F(10,220) = 4.750, intensity p < 0.0001, F(10,220) = 313.5, genotype p < 0.0001, F(1,220) = 20.63). Normalized startle magnitudes in Cntnap2 KO rats were particularly increased in comparison to Cntnap2 WT rats at 90–100 dB SPL (Sidak’s multiple comparisons test, 90 dB SPL: p < 0.0001, 95 dB SPL: p = 0.0005, 100 dB SPL: p = 0.0069). (G) Sigmoidal curves (lines) fitted to the startle magnitudes scaled between 0 and 1 were significantly different in Cntnap2 WT (SD, blue area) and Cntnap2 KO rats (mean ± SD, red squares and error bars; p < 0.0001, curve fit values see Table 1). Dotted horizontal line at 0.25 determined as ASR threshold and at 0.9 as ASR saturation. (H) Individual ASR thresholds (Left) and saturation (Right) extracted from individual sigmoidal curve fits were significantly lower in Cntnap2 KO rats (blue squares, horizontal black lines: mean) compared with WT controls (red circles, horizontal black lines: mean; two-sided student’s t-test, threshold: p = 0.0210, saturation: p = 0.0012). (I) Linear regression of ASR peak latencies across the dynamic range of Cntnap2 WT (mean ± SD, blue circles and error bars, Y = −8.537 * X + 312.4, r2 = 0.9979, blue line) and KO rats (mean ± SD, red squares and error bars, KO: Y = 4.910 * X + 277.8, r2 = 0.7576, red line). The slopes of the regression lines were significantly different (p = 0.0408). ES50, acoustic startle pulse intensity that gives a startle magnitude at 50%. *p < 0.05; **p < 0.01; ***p < 0.001; #p < 0.05 (comparison of regression lines); ###p < 0.001 (comparison of regression lines).
To assess acoustic reactivity in Cntnap2 WT and KO rats, startle response magnitudes to a series of startle pulses of increasing intensity (65–115 dB in 5 dB SPL increments) were measured and analyzed. Deficient short-term habituation and exaggerated sensitization in Cntnap2 KO rats described above were accompanied by increased ASR magnitudes of the startle I-O growth function in both female (Figure 3D) and, even more so, in male (Figure 3E) Cntnap2 KO rats compared with respective WT controls (F WT: n = 6, F KO: n = 6, two-way ANOVA, intensity × genotype p = 0.1598, F(10,110) = 1.471, intensity p < 0.0001, F(10,110) = 61.70, genotype p < 0.0001, F(1,110) = 28.83; M WT: n = 5, M KO: n = 5, two-way ANOVA, intensity × genotype p < 0.0001, F(10,88) = 14.97, intensity p < 0.0001, F(10,88) = 100.1, genotype p < 0.0001, F(1,88) = 301.7). ASR magnitudes were particularly increased at 105 dB SPL, and at 85–115 dB SPL startle pulse intensity in female and male Cntnap2 KO rats, respectively (Figure 3D, WT F vs. KO F, Sidak’s multiple comparisons test, 105 dB SPL: p = 0.0303, Figure 3E, WT M vs. KO M, Sidak’s multiple comparisons test, 85–115 dB SPL: all p < 0.0001). While ASR magnitudes were similar in female and male Cntnap2 WT rats (F WT: n = 6, M WT: n = 5, two-way ANOVA, intensity × sex p < 0.9551, F(10,99) = 0.3743, intensity p < 0.0001, F(10,99) = 59.17, sex p < 0.1054, F(1,99) = 2.670), exaggerated ASR magnitudes were more pronounced in male than female Cntnap2 KO rats (note the higher startle magnitudes in Cntnap2 KO males compared with KO females in Figures 3E,D, respectively, F: n = 6, M: n = 5, two-way ANOVA, intensity × sex p < 0.0001, F(10,99) = 6.864, intensity p < 0.0001, F(10,99) = 103.9, sex p < 0.0001, F(1,99) = 167.3).
To optimize the comparison of ASR magnitudes especially to moderate startle pulse intensities between animals (see hypothetical plasticity of ASR I-O in Figure 2 and Supplementary Figure 3), ASR magnitudes of all animals were normalized to their individual magnitude at the loudest startle pulse intensity (115 dB SPL). Normalization of ASR I-O magnitudes eliminated sex differences and the data were pooled for male and female Cntnap2 WT or KO rats, respectively (Figure 3F, Cntnap2 WT F: n = 6, M: n = 5, Cntnap2 KO F: n = 6, M: n = 6, three-way ANOVA, intensity × genotype × sex p = 0.4238, F(10,198) = 1.025, genotype × sex p = 0.1392, F(1,198) = 2.205, intensity × sex p = 0.6617, F(10,198) = 0.7657, intensity × genotype p < 0.0001, F(10,198) = 5.021, sex p = 0.8462, F(1,198) = 0.03771, genotype p < 0.0001, F(1,198) = 21.53, intensity p < 0.0001, F(10,198) = 307.1). Normalized startle magnitudes in Cntnap2 KO rats were increased in comparison to Cntnap2 WT rats, particularly at 90–100 dB SPL (Figure 3F, Cntnap2 WT: n = 11, Cntnap2 KO: n = 11, two-way ANOVA, intensity × genotype p < 0.0001, F(10,220) = 4.750, intensity p < 0.0001, F(10,220) = 313.5, genotype p < 0.0001, F(1,220) = 20.63, Sidak’s multiple comparisons test, 90 dB SPL: p < 0.0001, 95 dB SPL: p = 0.0005, 100 dB SPL: p = 0.0069).
Besides the change in maximum startle response obtainable (ASR capacity, Figures 3D,E), the relationship between the startle pulse intensity and response magnitude could be altered in Cntnap2 KO rats through several underlying mechanisms (Figure 2). To extract dynamic range characteristics including startle threshold and saturation from the startle I-O growth functions of individual animals, sigmoidal curves were fitted to the experimental data (scaled between 0 and 1). The ASR threshold was defined as 25%, and the ASR saturation as 90% of the scaled magnitude. The average fitted curves were significantly different between Cntnap2 WT and KO rats, with fitted curves from KO rats showing both a steeper slope and a leftward shift of ES50 (startle pulse intensity that gives the half-maximal response, Figure 3G and Table 1, Cntnap2 WT: n = 11 rats, Cntnap2 KO: n = 11 rats, p < 0.0001). Increased startle magnitudes and altered dynamic range in Cntnap2 KO rats (Figures 3D–G) were paralleled by a significantly lower startle threshold (Figure 3H Left, Cntnap2 WT: 86.6 ± 4.29 dB SPL, KO: 82.7 ± 2.97 dB SPL, two-sided student’s t-test: p = 0.0210) and saturation (Figure 3H Right, Cntnap2 WT: 106.5 ± 6.8 dB SPL, KO: 97.8.7 ± 3.6 dB SPL, two-sided student’s t-test: p = 0.0012). This means that, on average, Cntnap2 KO rats reach the 25% and 90% criterion at lower startle pulse intensities than WT rats—further indicators for the left-shift of the ASR I-O function and increased acoustic reactivity in Cntnap2 KO rats. Taken together, the ASR I-O functions and their parameters extracted from the sigmoidal curve fits demonstrated increased ASR capacity (maximal response possible), stimulus potency (ES50), ASR excitability (ASR threshold), dynamic range top plateau (ASR saturation), and ASR efficiency (slope) in Cntnap2 KO rats.
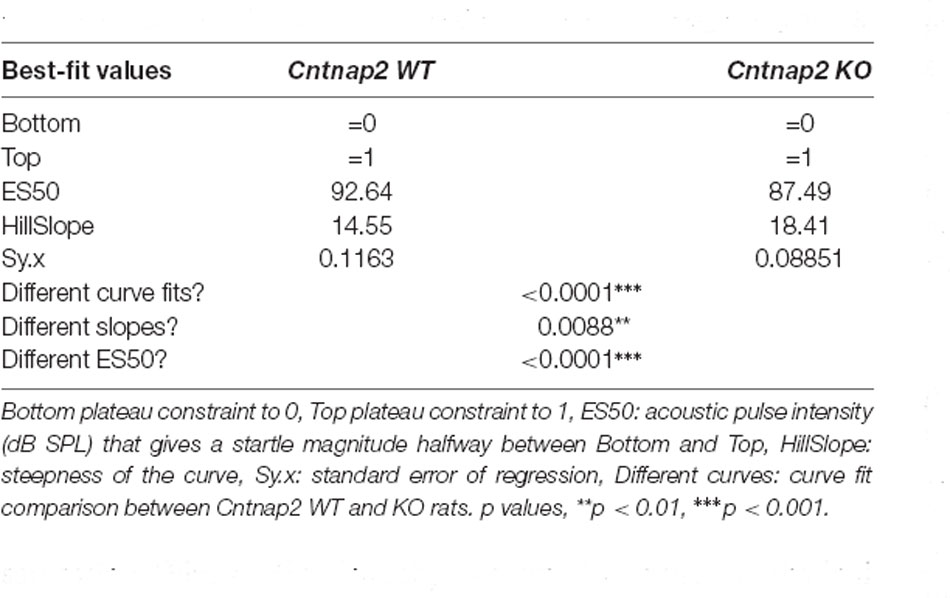
Table 1. Comparison of sigmoidal curve fit of ASR I-O function with magnitude scaled between 0 and 1 in Cntnap2 WT and KO rats corresponding to Figure 3G.
ASR magnitude and latency are in general negatively correlated (i.e., the higher the magnitude, the shorter the latency; Hoffman and Searle, 1968). Peak latencies in Cntnap2 WT and KO rats were investigated across the ASR dynamic range, in particular at near startle I-O threshold, at ES50, and saturation (Figure 3I). Thereby, we could compare individual latencies at startle pulse intensities that yielded similar ASR magnitudes in Cntnap2 WT and KO rats relative to their dynamic range. As expected, Cntnap2 WT rats showed a negative relationship between startle pulse intensities across the dynamic range and peak startle latency (Figure 3I, slope m = −8.537 ms/increment, deviation from zero p = 0.0291, F(1,1) = 479.3), indicating shortening of latency with increasing ASR magnitudes across the dynamic range. In Cntnap2 KO rats, however, no such negative relationship was found (Figure 3I, slope m = 4.910 ms/increment, deviation from zero p = 0.3277, F(1,1) = 3.126, WT vs. KO p = 0.0408). In contrast to the WT controls, Cntnap2 KO rats showed significantly shorter latencies near startle I-O threshold (Cntnap2 WT: 304.1 ± 22.3 ms, KO: 284.31 ± 12.2 ms, two-sided student’s t-test, p = 0.0181) and their startle peak latencies did not further decrease across the dynamic range (Figure 3I, deviation from zero p = 0.3277, F(1,1) = 3.126). The shorter peak latencies near the threshold and the lack of further shortening of latency across the dynamic range are indicators for an overall increased response strength in Cntnap2 KO rats. Taken together, our results show that Cntnap2 KO rats have increased auditory reactivity and impaired habituation.
Excitatory and Inhibitory Neurotransmitter Levels Are Altered in the Startle-Mediating Brainstem From Cntnap2 KO Rats
In order to assess possible alterations in neuronal excitation/inhibition within the startle-mediating brainstem circuitry that might underlie ASD-related sensory processing deficits (for review, see Sinclair et al., 2017b), we quantified GABA, glutamate, and glutamine amino acid levels ([GABA+K]+: 142m/z, [Glu+K]+: 186m/z, [Gln+K]+: 185m/z) in the PnC (nucleus reticularis pontis caudalis) of fresh frozen coronal brain tissue sections from adult Cntnap2 WT and KO rats using MALDI MS (Figure 4, Table 2). Visual inspection of the intensity map images showed an increase in signal intensity of all three amino acids in the brainstem and middle cerebellar peduncle region of Cntnap2 KO (Figure 4B) compared with WT rats (Figure 4A). The AUC analysis of individual amino acid peaks in the mass spectra of the PnC region (Figure 4C) showed a significant increase in AUC ratio for GABA (Figure 4D Left, WT n = 7, KO n = 7, paired t-test p = 0.0242). AUC ratios of glutamate (Figure 4D Middle, paired t-test p = 0.0858) and glutamine (Figure 4D Right, paired t-test p = 0.0703) were slightly increased by statistical tendency. Comparative analysis (Table 2) showed a 2-fold increase in GABA in the PnC region from Cntnap2 KO rats (one sample t-test p = 0.0222) and by tendency a 1.4-fold increase in both glutamate (one sample t-test p = 0.0935) and glutamine (one sample t-test p = 0.0814). Consequently, the ratio between Glu/Gln was similar in Cntnap2 KO and WT rats (one sample t-test p = 0.4051), whereas GABA/Gln was significantly enhanced (one sample t-test p = 0.0335, Table 2). Finally, GABA was more enhanced than Glu, as evidenced by significantly decreased Glu/GABA ratio (one sample t-test p = 0.0349) and a slight, yet statistically insignificant, increase in GABA/Glu ratio (one sample t-test p = 0.0853). Importantly, the comparative analysis showed no differences in two other metabolite levels in the PnC region (Table 2), i.e., [Choline+K]+: 143m/z (one sample t-test p = 0.3336) and [Norepinephrine+K]+: 208m/z (one sample t-test p = 0.1383), indicating that the increases in Glu, GABA, and Gln levels were not based on a general impairment in metabolism or neurotransmission in Cntnap2 KO rats. Furthermore, GABA, Glu, and Gln levels were not altered in the SOC within the auditory brainstem of Cntnap2 KO rats (Supplementary Figure 4). This indicated that the amino acid level increases in the PnC in Cntnap2 KO rats were not ubiquitous throughout the brain. Taken together, our findings indicate aberrant levels of GABA, Glu, and Gln in the PnC of Cntnap2 KO rats. This suggests that altered implicit auditory-evoked behaviors linked with functional deletion of Cntnap2 are associated with an imbalance of excitation and inhibition, particularly affecting the GABA neurotransmitter system.
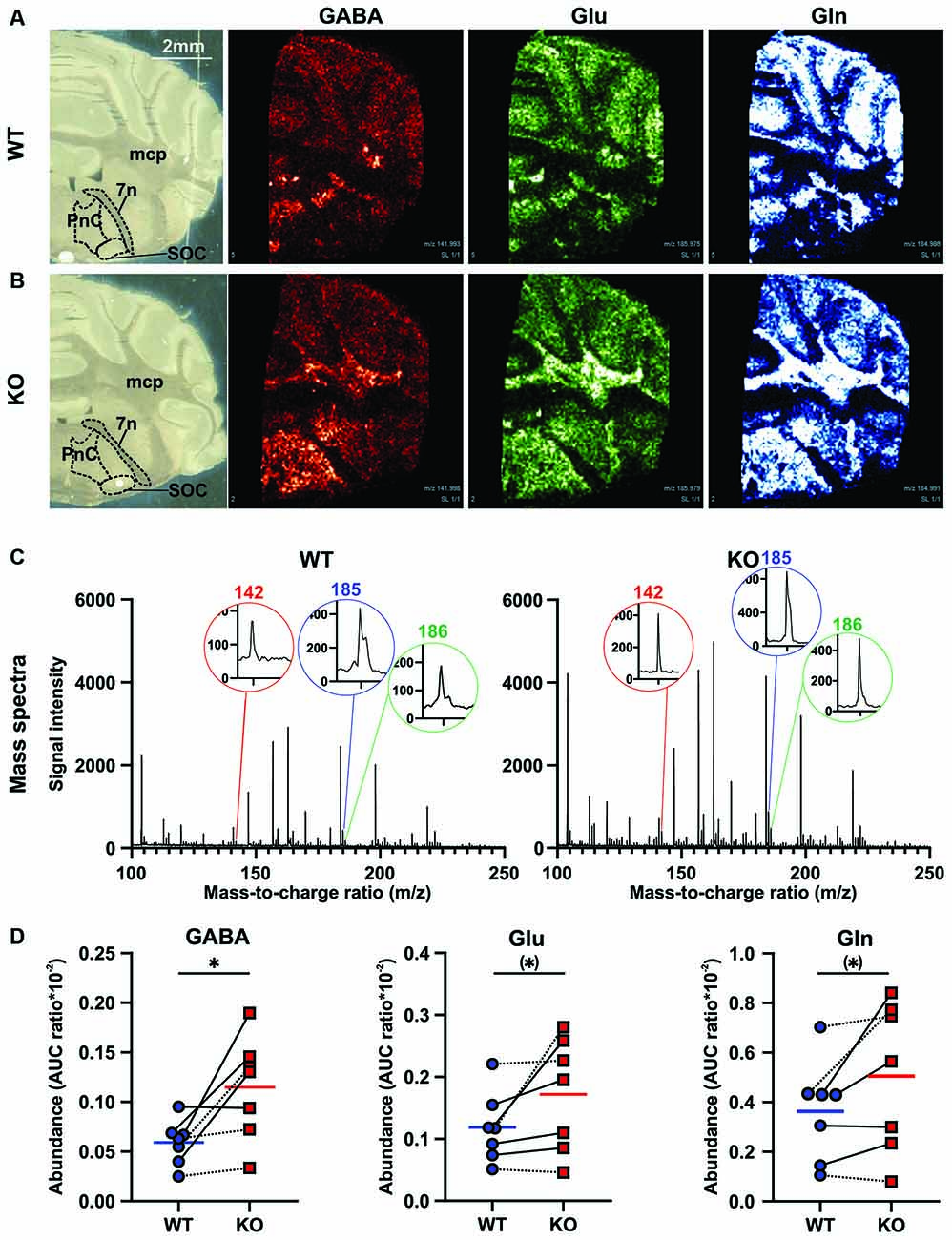
Figure 4. Effect of Cntnap2 knockout on the MALDI MS signals of amino acids in fresh frozen rat brain tissue. MALDI-MS generated intensity maps of GABA ([GABA+K]+: 142m/z), glutamate ([Glu+K]+: 186 m/z), and glutamine ([Gln+ K]+: 185m/z) from a (A) Cntnap2 WT and (B) KO rat. The signal of GABA, Glu, and Gln appears to be enhanced in the brainstem including and surrounding the PnC and in the mcp region in Cntnap2 KO rats. (C) Mass spectra from the PnC region of interest acquired on a Cntnap2 WT (Left) and KO (Right) rat coronal brain slice with ZnO in the mass region 100–250. Mass peaks corresponding to neurotransmitters ([GABA+K]+: 142m/z, [Glu+K]+: 186m/z, [Gln+ K]+: 185m/z) were acquired from each mass spectra. (D) The degree of signal enhancement in the PnC region can be seen through pairwise comparative under the curve analysis for Cntnap2 KO rats (n = 7, red squares and horizontal line) compared with WT controls (n = 7, blue circles and horizontal line). The area under the curve (AUC) ratio was significantly enhanced for GABA (Left, paired t-test p = 0.0242), and by tendency for Glu (Middle, paired t-test p = 0.0858) and Gln (Right, paired t-test p = 0.0703). Dotted lines denote female, solid lines male WT-KO pairs. Abbreviations: PnC, nucleus reticularis pontis caudalis; mcp, middle cerebellar peduncle; 7n, facial nerve. Scale bar: 2mm. (*)p < 0.1, *p < 0.05.

Table 2. Statistical comparison of MALDI MS AUC ratio (AUC ratio*10−2, paired t-test) and fold changes (one sample t test) for GABA, Glu, Gln, Choline, and Norepinephrine in the PnC region of experimentally naïve Cntnap2 WT and KO rats.
R-Baclofen Treatment Improves Disruptions in Habituation in Cntnap2 KO Rats
We first investigated the potential of R-Baclofen to remediate perturbed short-term habituation in Cntnap2 KO rats. Short-term habituation of the startle response was measured across the first eight startle trials of the test day 1 h after systemic injection of 0.75, 1.5, or 3 mg/kg R-Baclofen (Figure 5). In both Cntnap2 WT (Figure 5A) and KO rats (Figure 5B), the highest dose of R-Baclofen at 3 mg/kg led to a greater decline of startle magnitudes across the first eight trials in comparison with saline administration (Figure 5A, Cntnap2 WT: n = 11, Two-way RM ANOVA, trial p < 0.0001, F(7,80) = 8.884, treatment p = 0.0071, F(2.647, 211.7) = 4.397, trial × treatment p = 0.8352, F(21,240) = 0.6965, Dunnett’s multiple comparisons test, saline vs. 0.75 mg/kg p = 0.6549, saline vs. 1.5 mg/kg p = 0.1267, saline vs. 3 mg/kg p = 0.0084, Figure 5B, Cntnap2 KO: n = 11, Two-way RM ANOVA, trial p = 0.6752, F(7,80) = 0.6960, treatment p < 0.0001, F(2.864, 229.1) = 10.16, trial × treatment p = 0.7925, F(21,240) = 0.7925, Dunnett’s multiple comparisons test, saline vs. 0.75 mg/kg p = 0.7085, saline vs. 1.5 mg/kg p = 0.0606, saline vs. 3 mg/kg p < 0.0001). Habituation scores (Figure 5C) and sensitization scores (Figure 5D) were calculated and compared across R-Baclofen doses within genotype, and between equally treated Cntnap2 WT and KO rats. Mixed-effects analysis showed significantly reduced habituation scores with 3 mg/kg R-Baclofen in comparison to saline in both genotypes, thereby confirming enhanced short-term habituation through R-Baclofen in Cntnap2 WT and KO rats (Figure 5C, Mixed-effects analysis, Cntnap2 WT: n = 9–11 rats, Cntnap2 KO: n = 11 rats, genotype p = 0.0034, F(1,20) = 11.03, treatment p = 0.0005, F(2.785, 53.84) = 7.327, treatment × genotype p = 0.9632, F(3,58) = 0.09379, Dunnett’s multiple comparison’s test, WT: saline vs. 0.75 mg/kg p = 0.8601, saline vs. 1.5 mg/kg p = 0.2785, saline vs. 3 mg/kg: p = 0.0118; KO: saline vs. 0.75 mg/kg p = 0.9861, saline vs. 1.5 mg/kg p = 0.8595, saline vs. 3 mg/kg: p = 0.0205). To further analyze the effects of the three doses of R-Baclofen on short-term habituation between Cntnap2 WT and KO rats, we performed straight-line regressions of the habituation scores depending on the treatment, and compared the slopes and elevations of the two regression lines (Figure 5C). The elevations of the regression lines were significantly different in Cntnap2 KO compared with WT rats, resulting from the overall greater habituation scores across treatments in Cntnap2 KO rats (Figure 5C, Cntnap2 WT: n = 9–11 rats, elevation: c = 0.8004, Cntnap2 KO: n = 11 rats, elevation: c = 1.073, p = 0.0093). The slopes were similar in Cntnap2 WT and KO rats, showing a negative relationship between the R-Baclofen dose and habituation score in both genotypes (i.e., the higher the dose, the lower the habituation score, Figure 5C, Cntnap2 WT: n = 9–11 rats, slope: m = −0.1273, Cntnap2 KO: n = 11 rats, slope: m = −0.1514, p = 0.6940). This indicates that the selective activation of GABAB receptors by R-Baclofen had a similar suppressive mode of action on habituation scores in Cntnap2 WT and KO rats. In contrast to short-term habituation, R-Baclofen did not induce a statistically significant reduction in sensitization scores, neither within nor between Cntnap2 WT and KO rats (Figure 5D, two-way RM-ANOVA, Cntnap2 WT: n = 11 rats, Cntnap2 KO: n = 11 rats, genotype p = 0.0966, F(1,20) = 3.040, treatment p = 0.1430, F(2.628, 52.56) = 1.930, treatment × genotype p = 0.5867, F(3,60) = 0.6490; Linear regression, Cntnap2 WT: Y = −0.04851 * X + 0.8589, Sy.x = 0.05019, Cntnap2 KO: Y = −0.08989 * X + 1.014, Sy.x = 0.05991, WT vs. KO: slopes p = 0.3053, elevation p = 0.0548). Taken together, our results suggest that higher doses of R-Baclofen have the potential to improve deficient sensory filtering in Cntnap2 KO rats by enhancing short-term habituation. Sensitization of the ASR, however, appeared insensitive to the influence of R-Baclofen. This indicates that the cellular mechanisms or neural circuits controlling short-term habituation and sensitization are not affected the same way by selective activation of GABAB receptors though systemic administration of R-Baclofen.
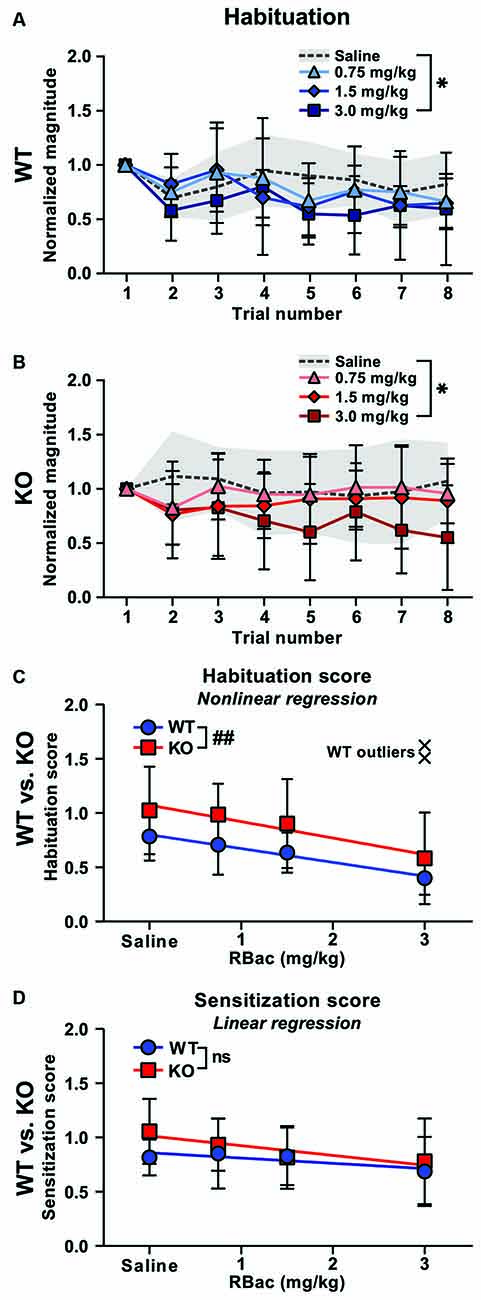
Figure 5. Higher doses of R-Baclofen (RBac) normalize habituation in Cntnap2 KO rats. (A,B) Mean ± SD startle response magnitudes across eight subsequent trials normalized to the first trial in Cntnap2 WT (A, blue symbols) and KO rats (B, red symbols) 1 h after injection of 0.75 mg/kg (triangles and error bars), 1.5 mg/kg (diamonds and error bars), or 3.0 mg/kg (squares and error bars) R-Baclofen compared with vehicle (saline, dotted line and gray area). Values <1 indicate habituation of the startle response. 3 mg/kg R-Baclofen led to a greater decline of startle magnitudes across the first eigth trials in comparison with saline administration in both Cntnap2 WT (A, n = 11, Two-way RM ANOVA, trial p < 0.0001, F(7,80) = 8.884, treatment p = 0.0071, F(2.647, 211.7) = 4.397, trial × treatment p = 0.8352, F(21,240) = 0.6965, Dunnett’s multiple comparisons test, saline vs. 0.75 mg/kg p = 0.6549, saline vs. 1.5 mg/kg p = 0.1267, saline vs. 3 mg/kg p = 0.0084) and Cntnap2 KO rats (B, n = 11, Two-way RM ANOVA, trial p = 0.6752, F(7,80) = 0.6960, treatment p < 0.0001, F(2.864, 229.1) = 10.16, trial × treatment p = 0.7925, F(21,240) = 0.7925, Dunnett’s multiple comparisons test, saline vs. 0.75 mg/kg p = 0.7085, saline vs. 1.5 mg/kg p = 0.0606, saline vs. 3 mg/kg p < 0.0001). (C) Straight-line regression of the habituation scores in Cntnap2 WT (blue circles and error bars, mean ± SD) and KO rats (red squares and error bars, mean ± SD). Mixed-effects analysis showed significantly reduced habituation scores with 3 mg/kg R-Baclofen in comparison to saline in both genotypes (Cntnap2 WT: n = 9–11 rats, Cntnap2 KO: n = 11 rats, genotype p = 0.0034, F(1,20) = 11.03, treatment p = 0.0005, F(2.785, 53.84) = 7.327, treatment × genotype p = 0.9632, F(3,58) = 0.09379, Dunnett’s multiple comparison’s test, WT: saline vs. 0.75 mg/kg p = 0.8601, saline vs. 1.5 mg/kg p = 0.2785, saline vs. 3 mg/kg: p = 0.0118; KO: saline vs. 0.75 mg/kg p = 0.9861, saline vs. 1.5 mg/kg p = 0.8595, saline vs. 3 mg/kg: p = 0.0205). The slopes of the regression lines showed no R-Baclofen dose-dependent differences in Cntnap2 WT (n = 9–11 rats, Y = −0.1273 * X + 0.8004, Sy.x = 0.2131) and KO rats (n = 11 rats, Y = −0.1514* X + 1.073, Sy.x = 0.3777), whereas the elevations of the regression lines were significantly different (p = 0.0093). Two Cntnap2 WT outliers at 3 mg/kg R-Baclofen (black crosses) were excluded from the straight-line regression and mixed-effects analysis using Prism GraphPad’s “Detect and eliminate outliers” method. (D) R-Baclofen treatment did not induce a statistically significant reduction in sensitization scores, neither in Cntnap2 WT nor KO rats (two-way RM-ANOVA, Cntnap2 WT: n = 11 rats, Cntnap2 KO: n = 11 rats, genotype p = 0.0966, F(1,20) = 3.040, treatment p = 0.1430, F(2.628, 52.56) = 1.930, treatment × genotype p = 0.5867, F(3,60) = 0.6490). Regression lines were similar in both genotypes (Cntnap2 WT: Y = −0.04851 * X + 0.8589, Sy.x = 0.05019, Cntnap2 KO: Y = −0.08989 * X + 1.014, Sy.x = 0.05991, WT vs. KO: slopes p = 0.3053, elevation p = 0.0548). *p < 0.05; ##p < 0.01 (comparison of regression lines); n.s.: not significant.
R-Baclofen Ameliorates Exaggerated ASRs in Cntnap2 KO Rats to Moderate, but Not to High Startle Pulse Intensities
The relationship between startle pulse intensities and ASR magnitudes can be altered through a number of variables, such as the genotype and pharmaceuticals (Figure 2; for review, see Koch, 1999). We next aimed to test if R-Baclofen could decrease the enhanced ASR magnitudes and ASR capacity in Cntnap2 KO rats. We first compared the effects of R-Baclofen on the ASR I-O function and maximal response magnitudes within genotype and sex (Figure 6). In Cntnap2 WT rats, all three doses of R-Baclofen (0.75, 1.5, 3 mg/kg) significantly decreased the ASR magnitudes to startle pulses of increasing intensity compared with saline in both females and males (Figure 6A Left, Cntnap2 WT F: n = 6, Two-way RM ANOVA, intensity p < 0.0001, F(10,55) = 44.28, treatment p < 0.0001, F(2.453, 134.9) = 86.07, intensity × treatment p < 0.0001, F(30,165) = 4.390, Dunnett’s multiple comparisons test, saline vs. 0.75 mg/kg p < 0.0001, saline vs. 1.5 mg/kg p < 0.0001, saline vs. 3 mg/kg: p < 0.0001; Right, Cntnap2 WT M: n = 5, Two-way RM ANOVA, intensity p < 0.0001, F(10,44) = 21.39, treatment p < 0.0001, F(2.511, 110.5) = 63.83, intensity × treatment p < 0.0001, F(30,132) = 2.847, Dunnett’s multiple comparisons test, saline vs. 0.75 mg/kg p = 0.0002, saline vs. 1.5 mg/kg p < 0.0001, saline vs. 3 mg/kg: p < 0.0001). This decrease in ASR magnitudes was evident across a wide range of startle pulse intensities after injection of 1.5 and 3 mg/kg R-Baclofen in female and male Cntnap2 WT rats (post hoc comparisons matched for startle pulse intensities see Supplementary Table 1). In Cntnap2 KO rats, 1.5 and 3 mg/kg R-Baclofen significantly reduced ASR magnitudes in both females and males, whereas the lowest dose of R-Baclofen (0.75 mg/kg) did not (Figure 6B Left, Cntnap2 KO F: n = 6, Two-way RM ANOVA, intensity p < 0.0001, F(10,55) = 17.99, treatment p < 0.0001, F(1.911, 105.1) = 29.81, intensity × treatment p = 0.0405, F(30,165) = 1.568, Dunnett’s multiple comparisons test, saline vs. 0.75 mg/kg p > 0.9999, saline vs. 1.5 mg/kg p = 0.0309, saline vs. 3 mg/kg: p < 0.0001; Right, Cntnap2 KO M: n = 5, Two-way RM ANOVA, intensity p < 0.0001, F(10,44) = 77.47, treatment p < 0.0001, F(2.137, 94.04) = 20.74, intensity × treatment p = 0.1091, F(30,132) = 1.384, Dunnett’s multiple comparisons test, saline vs. 0.75 mg/kg p = 0.9802, saline vs. 1.5 mg/kg p = 0.0003, saline vs. 3 mg/kg: p < 0.0001). Interestingly, in both female and male Cntnap2 KO rats, the reduction in ASR magnitude was only present in response to weaker, but not to higher startle pulse intensities (post hoc comparisons matched for startle pulse intensities see Supplementary Table 1). To further investigate the effect of R-Baclofen on maximum ASR capacity, we compared the ASR magnitudes at the loudest startle pulse (115 dB SPL) relative to respective saline controls (Figures 6C,D). In female and male Cntnap2 WT rats, R-Baclofen induced a dose-dependent reduction in maximal ASR magnitude [Figure 6C, median (IQR), WT F: n = 6 rats, 0.75 mg/kg re saline: 0.79 (0.49–1.06), 1.5 mg/kg re saline: 0.70 (0.60–0.88), 3 mg/kg re saline: 0.38 (0.30–0.52), Friedman test, p = 0.0085, Dunn’s multiple comparisons test, 0.75 mg/kg vs. saline: p > 0.9999, 1.5 mg/kg vs. saline: p = 0.5391, 3 mg/kg vs. saline: p = 0.0052; WT M: n = 5 rats, 0.75 mg/kg re saline: 0.71 (0.64–0.99), 1.5 mg/kg re saline: 0.66 (0.39–0.74), 3 mg/kg re saline: 0.52 (0.35–0.68), Friedman test, p = 0.0120, Dunn’s multiple comparisons test, 0.75 mg/kg vs. saline: p = 0.6620, 1.5 mg/kg vs. saline: p = 0.0825, 3.0 mg/kg vs. saline: p = 0.0099]. In contrast, maximal ASR magnitudes were similar irrespective of treatment in both female and male Cntnap2 KO rats (Figure 6D, median (IQR), KO F: n = 6 rats, 0.75 mg/kg re saline: 1.04 (0.85–1.26), 1.5 mg/kg re saline: 1.19 (0.91–1.42), 3 mg/kg re saline: 0.59 (0.42–0.79), Friedman test, p = 0.1268; KO M: n = 5 rats, 0.75 mg/kg re saline: 1.00 (0.94–1.17), 1.5 mg/kg re saline: 1.02 (0.88–1.09), 3 mg/kg re saline: 0.98 (0.83–1.02), Friedman test, p = 0.3720). In summary, as expected, R-Baclofen decreased magnitudes in the ASR I-O growth functions. In doing so, Cntnap2 KO rats showed a higher minimal effective dose (1.5 mg/kg) than WT rats (0.75 mg/kg). The reduction in ASR magnitudes in Cntnap2 KO rats was restricted to lower startle pulse intensities, whereas the increased maximal ASR capacity was not ameliorated by R-Baclofen. This notion was further corroborated by between genotype comparisons, in particular, ASR magnitudes in Cntnap2 KO males after R-Baclofen treatment compared to saline-injected WT males (Supplementary Figures 5D–F). R-Baclofen dose-dependently reduced ASR magnitudes in Cntnap2 KO males and brought them closer to WT control levels. However, ASR magnitudes were most notably downregulated for low to medium startle pulse intensities, but not for the highest startle pulse intensities tested (Supplementary Figures 5D–F). Furthermore, post hoc testing of normalized ASR I-O functions matched for startle pulse intensities did not find statistically significant differences between treatments in Cntnap2 WT rats, whereas in Cntnap2 KO rats ASR magnitudes were reduced in particular at 85 and 90 dB SPL after 1.5 mg/kg R-Baclofen and at 90 dB SPL after 3 mg/kg R-Baclofen administration (Dunnett’s multiple comparisons test, saline vs. 1.5 mg/kg: 85 dB SPL: p = 0.0105, 90 dB SPL: p = 0.0322; saline vs. 3 mg/kg: 90 dB SPL: p = 0.0375, Supplementary Figure 6). The minimal effective dose of R-Baclofen in Cntnap2 KO rats determined from their normalized ASR magnitudes after treatment with R-Baclofen compared to those in WT rats after saline injection was 1.5 mg/kg (Supplementary Figures 6C–E). These differences between Cntnap2 WT and KO rats after normalizing magnitudes to the individual ASR capacities further emphasize the differential effect of R-Baclofen on ASR I-O growth functions in the two genotypes. It suggests that the R-Baclofen effect was distinctly suppressive on ASRs to weaker startle pulse intensities in KO rats. In contrast, lack of such a suppression indicated that in WT rats R-Baclofen particularly impacted their ASRs to higher startle pulse intensities.
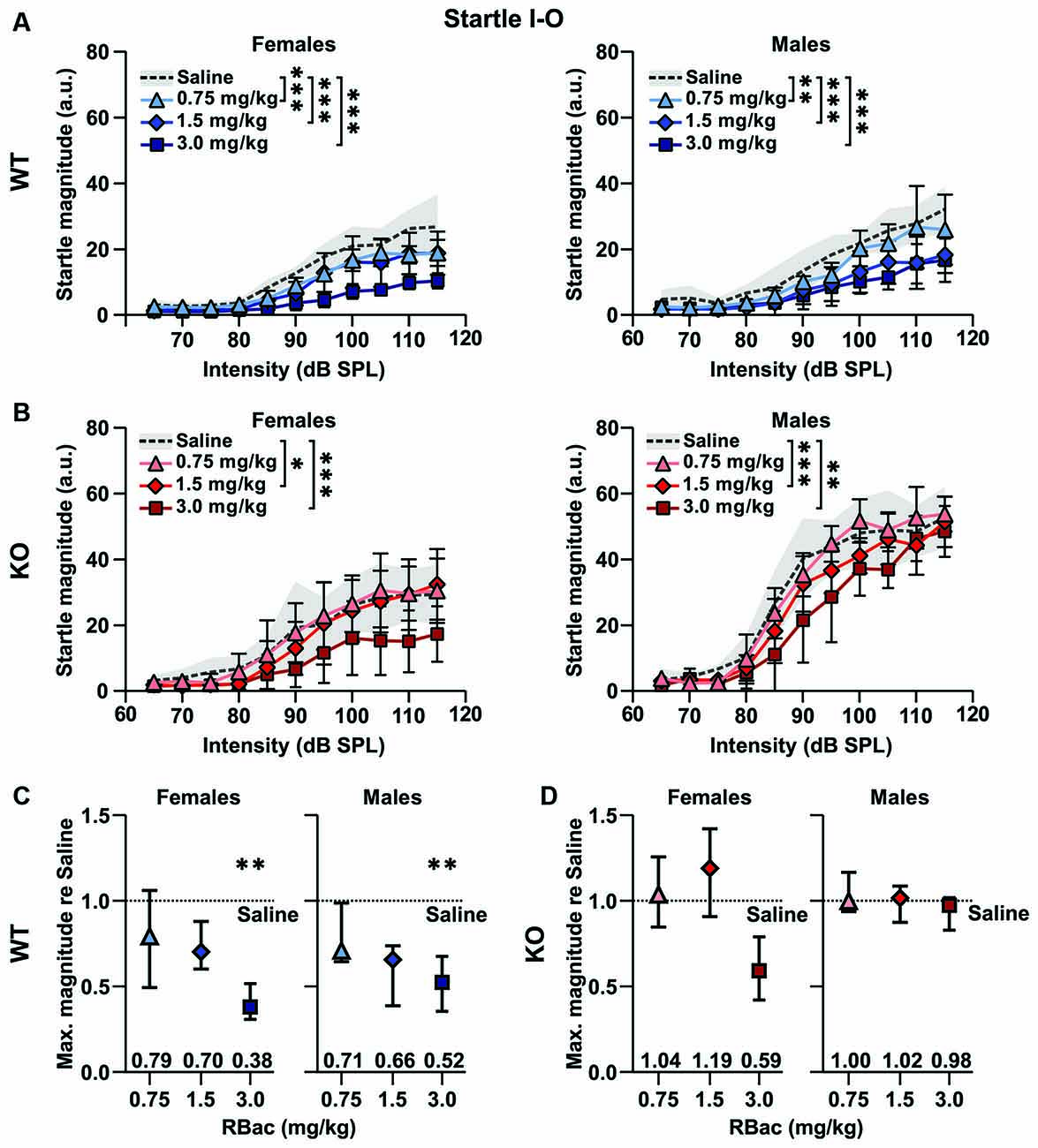
Figure 6. Medium and high doses of R-Baclofen decrease ASR magnitudes in Cntnap2 KO rats to control levels. (A,B) Mean ± SD startle response magnitudes to increasing startle pulse intensities after injection of saline (dotted line and gray area), 0.75 mg/kg (triangles), 1.5 mg/kg (diamonds), 3 mg/kg R-Baclofen (squares) in Cntnap2 WT rats (A, blue symbols) and Cntnap2 KO rats (B, red symbols). Startle magnitudes were significantly reduced after injection of 0.75, 1.5, or 3 mg/kg R-Baclofen in comparison with injection of saline in female (A, Left) and male (A, Right) Cntnap2 WT rats (A, Left, Cntnap2 WT F: n = 6, two-way RM ANOVA, intensity p < 0.0001, F(10,55) = 44.28, treatment p < 0.0001, F(2.453, 134.9) = 86.07, intensity × treatment p < 0.0001, F(30,165) = 4.390, Dunnett’s multiple comparisons test, saline vs. 0.75 mg/kg p < 0.0001, saline vs. 1.5 mg/kg p < 0.0001, saline vs. 3 mg/kg: p < 0.0001; A, Right, Cntnap2 WT M: n = 5, two-way RM ANOVA, intensity p < 0.0001, F(10,44) = 21.39, treatment p < 0.0001, F(2.511, 110.5) = 63.83, intensity × treatment p < 0.0001, F(30,132) = 2.847, Dunnett’s multiple comparisons test, saline vs. 0.75 mg/kg p = 0.0002, saline vs. 1.5 mg/kg p < 0.0001, saline vs. 3 mg/kg: p < 0.0001). (B) Startle magnitudes in female (B, Left) and male (B, Right) Cntnap2 KO rats were significantly reduced after injection of 1.5, or 3 mg/kg, but not with 0.75 mg/kg, R-Baclofen in comparison with saline injection (B, Left, Cntnap2 KO F: n = 6, Two-way RM ANOVA, intensity p < 0.0001, F(10,55) = 17.99, treatment p < 0.0001, F(1.911, 105.1) = 29.81, intensity × treatment p = 0.0405, F(30,165) = 1.568, Dunnett’s multiple comparisons test, saline vs. 0.75 mg/kg p > 0.9999, saline vs. 1.5 mg/kg p = 0.0309, saline vs. 3 mg/kg: p < 0.0001; B, Right, Cntnap2 KO M: n = 5, Two-way RM ANOVA, intensity p < 0.0001, F(10,44) = 77.47, treatment p < 0.0001, F(2.137, 94.04) = 20.74, intensity × treatment p = 0.1091, F(30,132) = 1.384, Dunnett’s multiple comparisons test, saline vs. 0.75 mg/kg p = 0.9802, saline vs. 1.5 mg/kg p = 0.0003, saline vs. 3 mg/kg: p < 0.0001). (C,D) Comparison of ASR maximum response. (C) In both female (Left) and male (Right) Cntnap2 WT rats, R-Baclofen induced a significant decrease in the maximum ASR capacity at 115 dB SPL (WT F: Friedman test, p = 0.0085, Dunn’s multiple comparisons test, 0.75 mg/kg p > 0.9999, 1.5 mg/kg p = 0.5391, 3.0 mg/kg p = 0.0052; WT M: Friedman test, p = 0.0120, Dunn’s multiple comparisons test, 0.75 mg/kg p = 0.6620, 1.5 mg/kg p = 0.0825, 3.0 mg/kg p = 0.0099). (D) R-Baclofen did not induce a decrease in maximum ASR capacity from female (Left) nor male (Right) Cntnap2 KO rats (KO F: Friedman test, p = 0.1268; KO M: Friedman test, p = 0.3720). *p < 0.05; **p < 0.01; ***p < 0.001.
R-Baclofen Treatment Normalizes ASR I-O Threshold and Saturation in Cntnap2 KO Rats, but Exacerbates Shorter ASR Peak Latencies
Sigmoidal curves were fitted to the ASR I-O data scaled between 0 and 1 for individual animals of both genotypes and all treatments. Average curve fits were significantly different between Cntnap2 KO rats treated with 0.75 mg/kg R-Baclofen and Cntnap2 WT rats after saline injection (Figure 7A and Table 3, p < 0.0001, F(2,238) = 12.12). In contrast to this, average curve fits were similar between Cntnap2 KO rats treated with 1.5 mg/kg (Figure 7B and Table 3, p = 0.6048, F(2,238) = 0.5039) or 3 mg/kg R-Baclofen (Figure 7C and Table 3, p = 0.7751, F(2,238) = 0.2550) compared with Cntnap2 WT rats after saline injection. ASR thresholds extracted at the 25% scaled magnitude generally increased with the dose of R-Baclofen (Figure 7D and Supplementary Table 2). This increase was significant in Cntnap2 KO rats in particular with 1.5 mg/kg R-Baclofen in comparison with saline, but not in WT rats (Figure 7D Left, Cntnap2 WT rats (n = 11), mean ± SD saline: 87.9 ± 5.1 dB SPL, 0.75 mg/kg: 88.1 ± 5.0 dB SPL, 1.5 mg/kg: 89.4 ± 4.3 dB SPL, 3 mg/kg: 92.2 ± 5.8 dB SPL, RM ANOVA, p = 0.0685, Figure 7D Middle, Cntnap2 KO rats (n = 11), saline: 82.8 ± 4.7 dB SPL, 0.75 mg/kg: 84.3 ± 3.4 dB SPL, 1.5 mg/kg: 86.5 ± 3.9 dB SPL, 3 mg/kg: 88.8 ± 5.4 dB SPL, RM ANOVA, p = 0.0291, Dunnett’s multiple comparisons test, saline vs. 0.75 mg/kg p = 0.5583, saline vs. 1.5 mg/kg p = 0.0315, saline vs. 3 mg/kg: p = 0.0784). Comparison of ASR thresholds in R-Baclofen-treated Cntnap2 KO rats with saline-treated WT rats showed that thresholds were increased to control level after injection of 1.5 and 3 mg/kg R-Baclofen, while they were by tendency still lower than in controls with 0.75 mg/kg R-Baclofen (Figure 7D Right, two-sided student’s t-test, WT—Saline vs. KO–0.75 mg/kg: p = 0.0690, WT—Saline vs. KO–1.5 mg/kg: p = 0.4839, WT—Saline vs. KO–3 mg/kg: p = 0.6819). Saturation of the ASR I-O function extracted at the 90% scaled magnitude was significantly altered through R-Baclofen in Cntnap2 KO rats, but not in WT rats (Figure 7E and Supplementary Table 2). In particular, 3 mg/kg R-Baclofen increased ASR I-O saturation in Cntnap2 KO rats compared with saline [Figure 7E Left, Cntnap2 WT rats (n = 11), median (IQR), saline: 109.3 (97.4-115.0) dB SPL, 0.75 mg/kg: 100.7 (99.1-106.7) dB SPL, 1.5 mg/kg: 104.3 (98.5-112.8) dB SPL, 3 mg/kg: 111.3 (97.7-112.2) dB SPL, Friedman test, p = 0.5915, Figure 7E Middle, Cntnap2 KO rats (n = 11), saline: 100.8 (94.2-102.3) dB SPL, 0.75 mg/kg: 97.2 (95.3-103.1) dB SPL, 1.5 mg/kg: 109.3 (99.4-110.2) dB SPL, 3 mg/kg: 104.0 (97.9-115.5) dB SPL, Friedman test, p = 0.0240, Dunn’s multiple comparisons test, saline vs. 0.75 mg/kg: p > 0.9999, saline vs. 1.5 mg/kg: p = 0.2959, saline vs. 3 mg/kg: p = 0.0150]. Comparison between genotypes showed that ASR I-O saturation in Cntnap2 KO rats with 1.5 and 3 mg/kg R-Baclofen was similar to saturation in WT rats after saline injection, while there was a slight, yet not quite significant, difference with 0.75 mg/kg (Figure 7E Right, Mann–Whitney test, WT—Saline vs. KO–0.75 mg/kg: p = 0.0879, WT—Saline vs. KO–1.5 mg/kg: p = 0.7477, WT—Saline vs. KO–3 mg/kg: p = 0.8470). Taken together, our results suggest that selective activation of GABAB receptors by 1.5 mg/kg and 3 mg/kg R-Baclofen can normalize acoustic reactivity in Cntnap2 KO rats through a right-shift in ASR I-O function and an increase in ASR threshold and saturation sound levels to control levels.
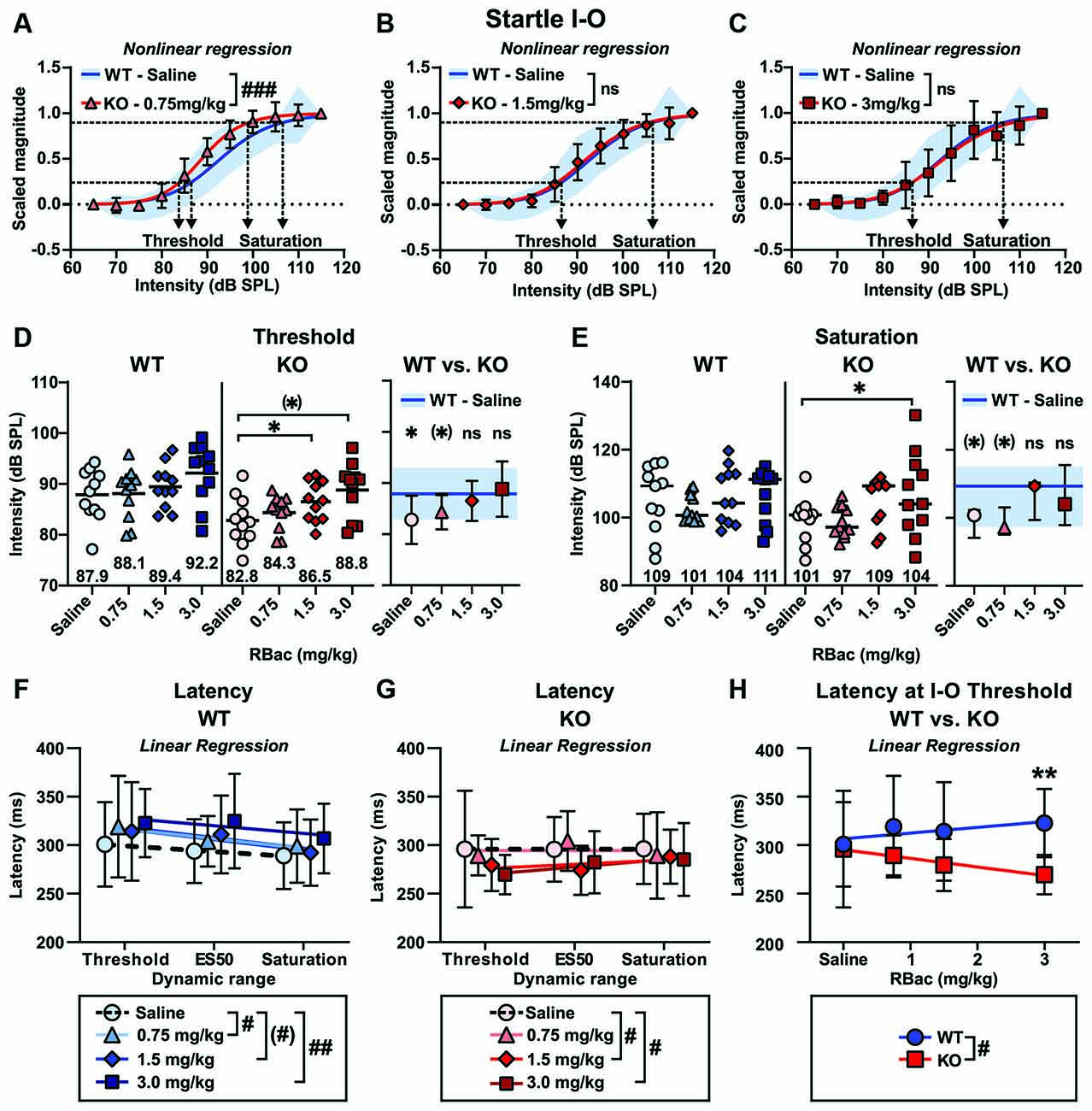
Figure 7. Medium and high doses of R-Baclofen increase ASR I-O threshold and saturation in Cntnap2 KO rats close to controls but shorten ASR peak latencies. (A–C) Sigmoidal curves (lines) fitted to the startle magnitudes scaled between 0 and 1 in Cntnap2 WT rats with saline (SD, blue area) and Cntnap2 KO rats (mean ± SD) with 0.75 mg/kg (A, red triangles and error bars), 1.5 mg/kg (B, red diamonds and error bars), and 3 mg/kg R-Baclofen (C, red squares and error bars). Dotted horizontal line at 0.25 determined as ASR threshold and at 0.9 as ASR saturation (curve fit values see Table 3). The average curve fit was significantly different from controls in Cntnap2 KO rats after administration of 0.75 mg/kg (A, p < 0.0001, F(2,238) = 12.12), but similar to controls with 1.5 mg/kg (B, p = 0.6048, F(2,238) = 0.5039), and 3 mg/kg R-Baclofen (C, p = 0.7751, F(2,238) = 0.2550). (D) Individual ASR thresholds in Cntnap2 WT (Left), KO (Middle), and WT vs. KO rats (Right) extracted from individual sigmoidal curve fits (ASR threshold values see Supplementary Table 2). (D, Left) Mean ASR thresholds were not significantly increased in Cntnap2 WT rats with R-Baclofen (0.75 mg/kg: light blue triangles and horizontal black line, 1.5 mg/kg: blue diamonds and horizontal black line, 3 mg/kg: dark blue squares and horizontal black line) compared to saline (circles and horizontal black line, RM ANOVA, p = 0.0685). (D, Middle) Mean ASR thresholds were significantly increased in Cntnap2 KO rats with R-Baclofen compared to saline (saline: circles and horizontal black line, 0.75 mg/kg: light red triangles and horizontal black line, 1.5 mg/kg: red diamonds and horizontal black line, 3 mg/kg: dark red squares and horizontal black line, RM ANOVA, p = 0.0291, Dunnett’s multiple comparisons test, saline vs. 0.75 mg/kg: p = 0.5583, saline vs. 1.5 mg/kg: p = 0.0315, saline vs. 3 mg/kg: p = 0.0784). (D, Right) Mean ± SD ASR thresholds in Cntnap2 KO rats were significantly different from WT controls (after saline, blue line and area) with saline (two-sided student’s t-test, p = 0.0244), not quite significantly different with 0.75 mg/kg (two-sided student’s t-test, p = 0.0690), and similar to controls with 1.5 mg/kg (two-sided student’s t-test, p = 0.4839) and 3 mg/kg R-Baclofen (two-sided student’s t-test, p = 0.6819). (E) Individual ASR saturation levels in Cntnap2 WT (Left), KO (Middle), and WT vs. KO rats (Right) extracted from individual sigmoidal curve fits (ASR saturation values see Supplementary Table 2). (E, Left) Median ASR saturation levels were not significantly altered in Cntnap2 WT rats with R-Baclofen (0.75 mg/kg: light blue triangles and horizontal black line, 1.5 mg/kg: blue diamonds and horizontal black line, 3 mg/kg: dark blue squares and horizontal black line) compared to saline (circles and horizontal black line, Friedman test, p = 0.5915). (E, Middle) Median ASR saturation levels were significantly increased in Cntnap2 KO rats with R-Baclofen compared to saline (saline: circles and horizontal black line, 0.75 mg/kg: light red triangles and horizontal black line, 1.5 mg/kg: red diamonds and horizontal black line, 3 mg/kg: dark red squares and horizontal black line, Friedman test, p = 0.0240, Dunn’s multiple comparisons test, saline vs. 0.75 mg/kg: p > 0.9999, saline vs. 1.5 mg/kg: p = 0.2959, saline vs. 3 mg/kg: p = 0.0150). (E, Right) Median ± interquartile range (IQR) ASR saturation levels in Cntnap2 KO rats were by tendency different from WT controls (after saline, blue line and area) with saline (Mann–Whitney test, p = 0.0879) and 0.75 mg/kg (Mann–Whitney test, p = 0.0879), and similar to controls with 1.5 mg/kg (Mann–Whitney test, p = 0.7477) and 3 mg/kg R-Baclofen (Mann–Whitney test, p = 0.8470). (F,G) Linear regression of ASR peak latencies across the dynamic range of Cntnap2 WT (F) and KO (G) rats with saline or R-Baclofen (mean ± SD, linear regression fits see Table 4). Elevations of the regression lines were significantly different in Cntnap2 WT (F, p = 0.0034, F(3,7) = 12.46; saline vs. 0.75 mg/kg: p = 0.0202, F(1,3) = 20.50; saline vs. 1.5 mg/kg: p = 0.0620, F(1,3) = 8.468; saline vs. 3 mg/kg: p = 0.0099, F(1,3) = 34.45) and KO rats (G; p = 0.0336, F(3,7) = 5.192; saline vs. 0.75 mg/kg: p = 0.8075, F(1,3) = 0.07070; saline vs. 1.5 mg/kg: p = 0.0375, F(1,3) = 12.76; saline vs. 3 mg/kg: p = 0.0272, F(1,3) = 16.37). (H) Linear regression of ASR peak latencies near threshold across treatment in Cntnap2 WT (blue circles and error bars, mean ± SD) and KO rats (red squares and error bars, mean ± SD). Slopes of the regression lines were significantly different (p = 0.0116, F(1,4) = 19.41; WT: blue line, Y = 5.893 * X + 306.6, Sy.x = 7.147; KO: red line, Y = −8.876 * X + 295.3, Sy.x = 2.057). (*)p < 0.1; *p < 0.05; **p < 0.01; comparison of regression lines: (#)p < 0.1; #p < 0.05; ##p < 0.01; ###p < 0.001, n.s., not significant.
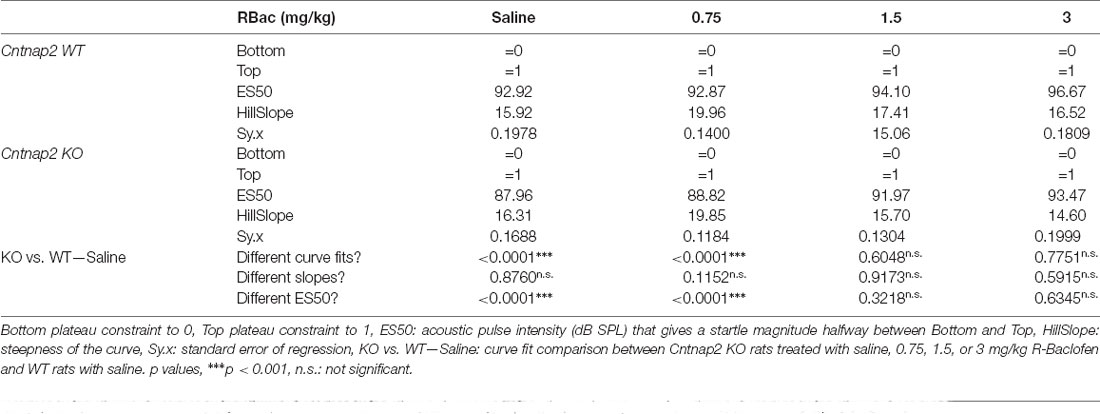
Table 3. Comparison of sigmoidal regression fit of ASR I-O function with magnitude scaled between 0 and 1 in Cntnap2 WT and KO rats.
We next investigated R-Baclofen-related changes in ASR peak latencies in Cntnap2 WT and KO rats across the ASR dynamic range (Figures 7F–H and Table 4). In Cntnap2 WT rats, R-Baclofen increased the ASR peak latencies across the dynamic range by means of greater regression line elevations in comparison with saline (most notably at 0.75 and 3 mg/kg, Figure 7F and Table 4, elevation: p = 0.0034). In contrast to this, R-Baclofen decreased the ASR peak latencies across the dynamic range by means of smaller regression line elevations in Cntnap2 KO rats compared with saline (most notably at 1.5 and 3 mg/kg, Figure 7G and Table 4, elevation: p = 0.0336). Slopes of the peak latency regression lines across the ASR dynamic range were not altered through R-Baclofen in comparison with saline within genotypes, neither in Cntnap2 WT nor KO rats (Figures 7F–G and Table 4, slopes: WT p = 0.8086, KO p = 0.7055).
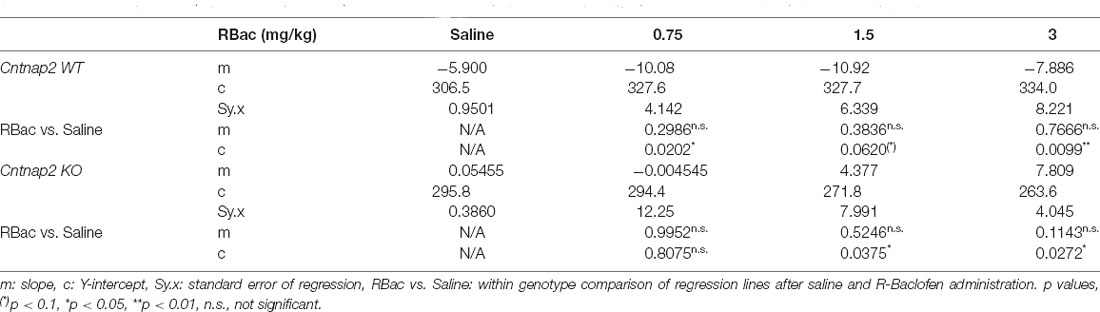
Table 4. Linear regression of ASR peak latencies in Cntnap2 WT and KO rats after treatment with saline or R-Baclofen (0.75, 1.5, 3 mg/kg).
As shown in Figure 7 and Supplementary Figures 5, 6, R-Baclofen decreased ASR magnitudes in Cntnap2 KO rats particularly at lower startle pulse intensities near ASR I-O threshold. To further analyze the effects of the three doses of R-Baclofen on peak latencies near ASR threshold, we performed linear regressions of the peak latencies at ASR I-O threshold across treatments (Figure 7H). Comparison of the two regression lines from Cntnap2 WT and KO rats showed that the slopes were significantly different (Figure 7H, WT: Y = 5.893 * X + 306.6, Sy.x = 7.147; KO: Y = −8.876 * X + 295.3, Sy.x = 2.057, slopes p = 0.0116). In Cntnap2 KO rats, the ASR peak latency at I-O threshold was negatively related to the R-Baclofen dose (i.e., the higher the dose, the shorter the latency, Figure 7H, slope m = −8.876 ms/increment, deviation from zero p = 0.0107, F(1,2) = 91.68). No such relationship between latency and R-Baclofen dose was found in Cntnap2 WT rats (Figure 7H, slope m = 5.893 ms/increment, deviation from zero p = 0.2088, F(1,2) = 3.347). The differential effects of R-Baclofen on startle peak latencies at threshold in Cntnap2 WT and KO rats became especially prominent at 3 mg/kg (Figure 7H, two-way RM ANOVA, treatment p = 0.8260, F(2.290, 45.79) = 0.2271, genotype p = 0.0152, F(1,20) = 7.050, treatment × genotype p = 0.1859, F(3,60) = 1.657, Sidak’s multiple comparisons test, WT vs. KO: Saline p = 0.9991, 0.75 mg/kg p = 0.3525, 1.5 mg/kg p = 0.2288, 3 mg/kg p = 0.0021). This indicates that the cellular mechanisms or neural circuits controlling ASR peak latencies near ASR thresholds are affected differently by selective activation of GABAB receptors through systemic administration of R-Baclofen in Cntnap2 WT and KO rats.
R-Baclofen Improves Sensorimotor Gating in Cntnap2 KO Rats by Means of Increasing the Relative Amount and Relative Latencies of Startle in PPI Trials
The effect of R-Baclofen on sensorimotor gating in Cntnap2 WT and KO rats was assessed using the PPI of the startle. The relative amount of PPI (%PPI) elicited by three prepulse stimulus levels (65, 75, and 85 dB SPL) at two different ISIs (30 and 100 ms) was first compared between Cntnap2 WT and KO rats after injection of saline. Cntnap2 KO rats had robust, but statistically nonsignificant, lower %PPI than WT rats for all prepulse conditions (Figure 8A and Table 5). Random permutation tests of %PPI for prepulses with 75 dB SPL, 100 ms, as well as 85 dB SPL, 30 ms between Cntnap2 WT and KO rats gave estimated p values of p = 0.0017 and p = 0.0163 (40 repetitions of 10,000 random samples without replacement, see Table 5), indicating a significant PPI deficit in Cntnap2 KO rats for these two prepulse types (Figure 8A and Table 5). In Cntnap2 WT rats, R-Baclofen showed no significant effect on %PPI elicited by any of the six prepulse types (Figure 8B, statistical comparisons see Table 6). In contrast, KO rats showed a significant increase in %PPI in four of the six prepulse conditions through R-Baclofen (intensity, ISI: 75 dB SPL, 30 ms, 75 dB SPL, 100 ms, 85 dB SPL, 30 ms; 85 dB SPL, 100 ms, Figure 8C, for statistical comparisons see Table 6). In particular, %PPI in Cntnap2 KO rats was increased with 1.5 mg/kg (prepulse 85 dB SPL, 100 ms) or 3 mg/kg R-Baclofen (prepulse 75 dB SPL, 30 ms, 75 dB SPL, 100 ms, 85 dB SPL, 30 ms; Figure 8C, for statistical comparisons see Table 6). Taken together, our results suggest that GABAB receptor agonist R-Baclofen can improve deficient sensorimotor gating in Cntnap2 KO rats by increasing the relative amount of PPI.
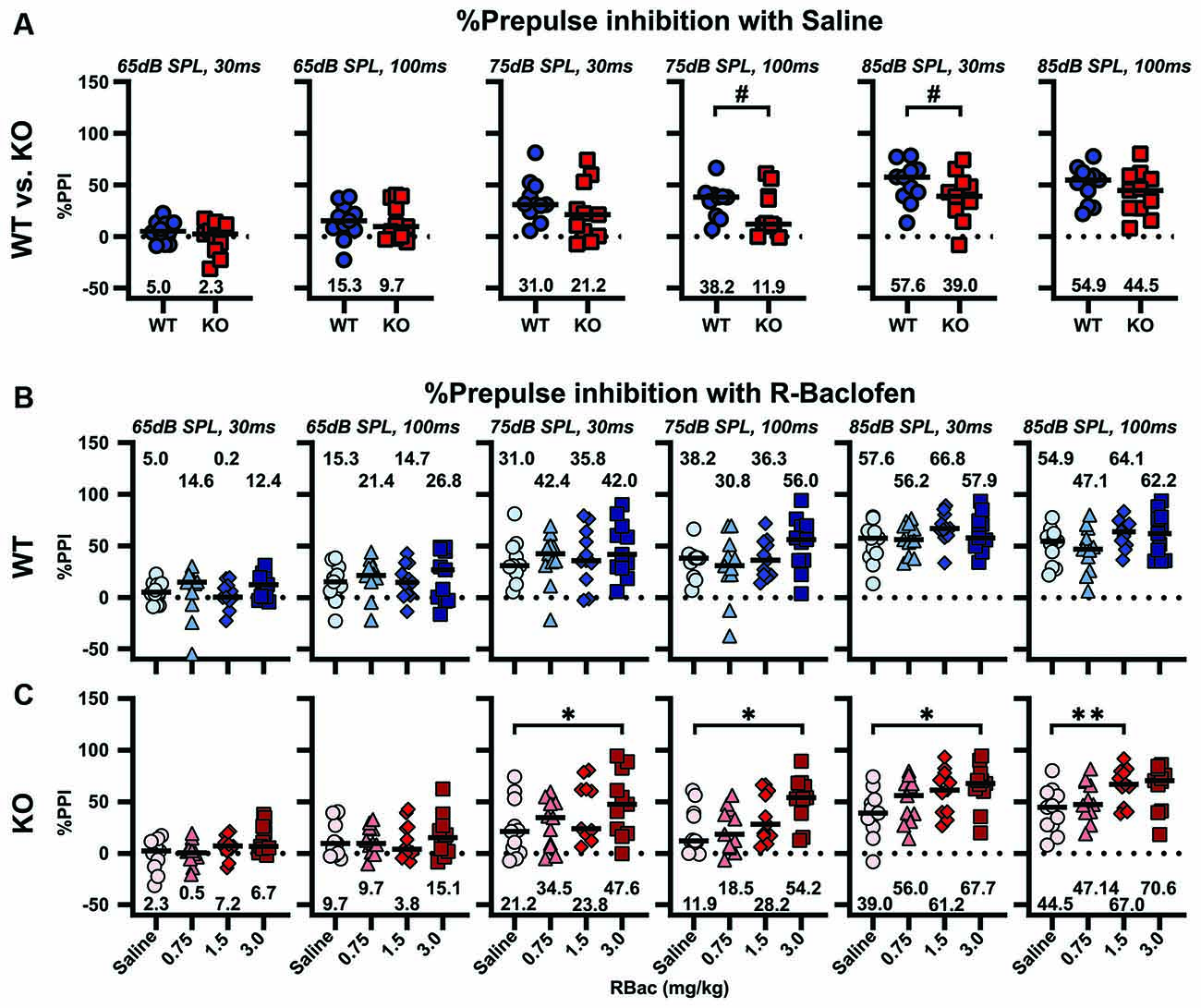
Figure 8. R-Baclofen increases the relative amount of PPI (%PPI) in Cntnap2 KO rats. (A–C) %PPI was elicited by six different prepulse conditions with three stimulus levels at two different ISIs (from left to right: 65 dB SPL, 30 ms; 65 dB SPL, 100 ms; 75 dB SPL, 30 ms; 75 dB SPL, 100 ms; 85 dB SPL, 30 ms; 85 dB SPL, 100 ms). Scatter plots depict individual %PPI for each prepulse condition and black horizontal lines represent the median %PPI. (A) %PPI for each prepulse condition in Cntnap2 WT (blue circles) and KO rats (red squares) after saline injection. Cntnap2 KO rats had consistently, but statistically nonsignificant, lower %PPI than WT rats. Estimated p values of p = 0.0017 and p = 0.0163 indicate a significant PPI deficit in Cntnap2 KO rats for prepulses with 75 dB SPL, 100 ms, and 85 dB SPL, 30 ms (for statistical comparisons and estimated p values through resampling see Table 5). (B) There were no significant differences in %PPI in Cntnap2 WT rats between saline (circles), and 0.75 mg/kg (light blue triangles), 1.5 mg/kg (blue diamonds), and 3 mg/kg R-Baclofen (dark blue squares) for any of the six prepulse conditions (for statistical comparisons see Table 6). (C) In Cntnap2 KO rats, %PPI was significantly increased through R-Baclofen (0.75 mg/kg: light red triangles, 1.5 mg/kg: red diamonds, 3 mg/kg R-Baclofen: dark red squares) compared with saline (circles), in particular with prepulses of 75 dB SPL, 30 ms; 75 dB SPL, 100 ms; 85 dB SPL, 30 ms (3 mg/kg); and 85 dB SPL, 100 ms (1.5 mg/kg). For statistical comparisons see Table 6. Dotted horizontal lines at 0%PPI represent no PPI of the startle. *p < 0.05; **p < 0.01; #p < 0.05 estimated p-value through resampling.

Table 5. Statistical comparison and estimated p values through resampling of %PPI elicited by six prepulse conditions in Cntnap2 WT and KO rats after injection of saline.
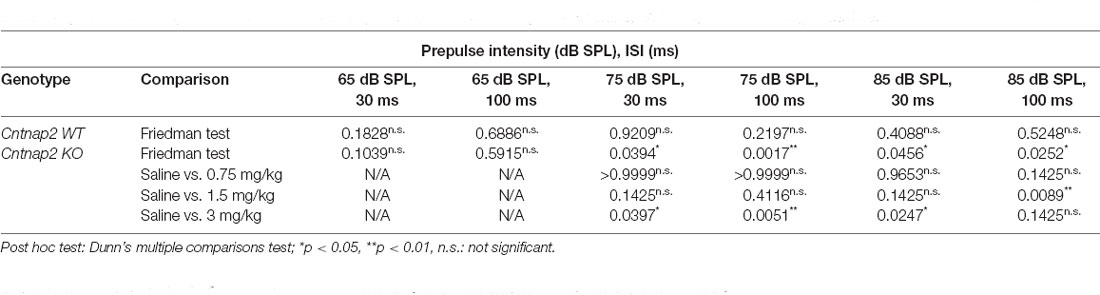
Table 6. Statistical comparison of %PPI within Cntnap2 WT or KO rats after injection of saline, and 0.75, 1.5, and 3 g/kg R-Baclofen.
To analyze the influence of R-Baclofen on temporal properties of sensorimotor gating, we compared the change in latency to the maximum startle response in trials with and without a prepulse between Cntnap2 WT and KO rats (Figure 9). After injection of saline, Cntnap2 KO rats showed generally shorter relative latencies than WT rats. The difference was significant for relative latencies to the prepulse type with 85 dB SPL, 30 ms (Figure 9A, two-sided student’s t-test p = 0.0195). The shorter relative latencies in trials that included a prepulse indicated impaired temporal characteristics of sensorimotor gating in Cntnap2 KO rats compared to WT rats. Within genotype, comparisons showed that R-Baclofen did not significantly increase the relative latencies in either Cntnap2 WT or KO rats for any of the six prepulse types, even though there appeared to be a slight increase in relative latency for some prepulse conditions in Cntnap2 KO rats (shown for prepulse condition 85 dB SPL, 30 ms in Figure 9B, Left: WT, RM ANOVA, p = 0.9282, F = 0.1226; Right: KO, RM ANOVA, p = 0.5611, F = 0.6374; for statistical results of all six prepulse conditions see Supplementary Figure 8). Therefore, we aimed to analyze if subtle changes in relative latency through R-Baclofen had the potential to increase latencies in Cntnap2 KO rats to WT control levels after saline injection. Indeed, all three doses of R-Baclofen increased the relative latency in Cntnap2 KO rats to levels similar to WT controls for prepulse type 85 dB SPL, 30 ms (Figure 9C, two-sided student’s t-test, WT—Saline vs. KO–0.75 mg/kg: p = 0.4381, WT—Saline vs. KO–1.5 mg/kg: p = 0.2627, WT—Saline vs. KO–3 mg/kg: p = 0.3069). This indicates that GABAB receptor agonist R-Baclofen can improve deficient sensorimotor gating in Cntnap2 KO rats by subtle increases of the relative latency of startle in PPI trials with a minimal dose of 0.75 mg/kg R-Baclofen.
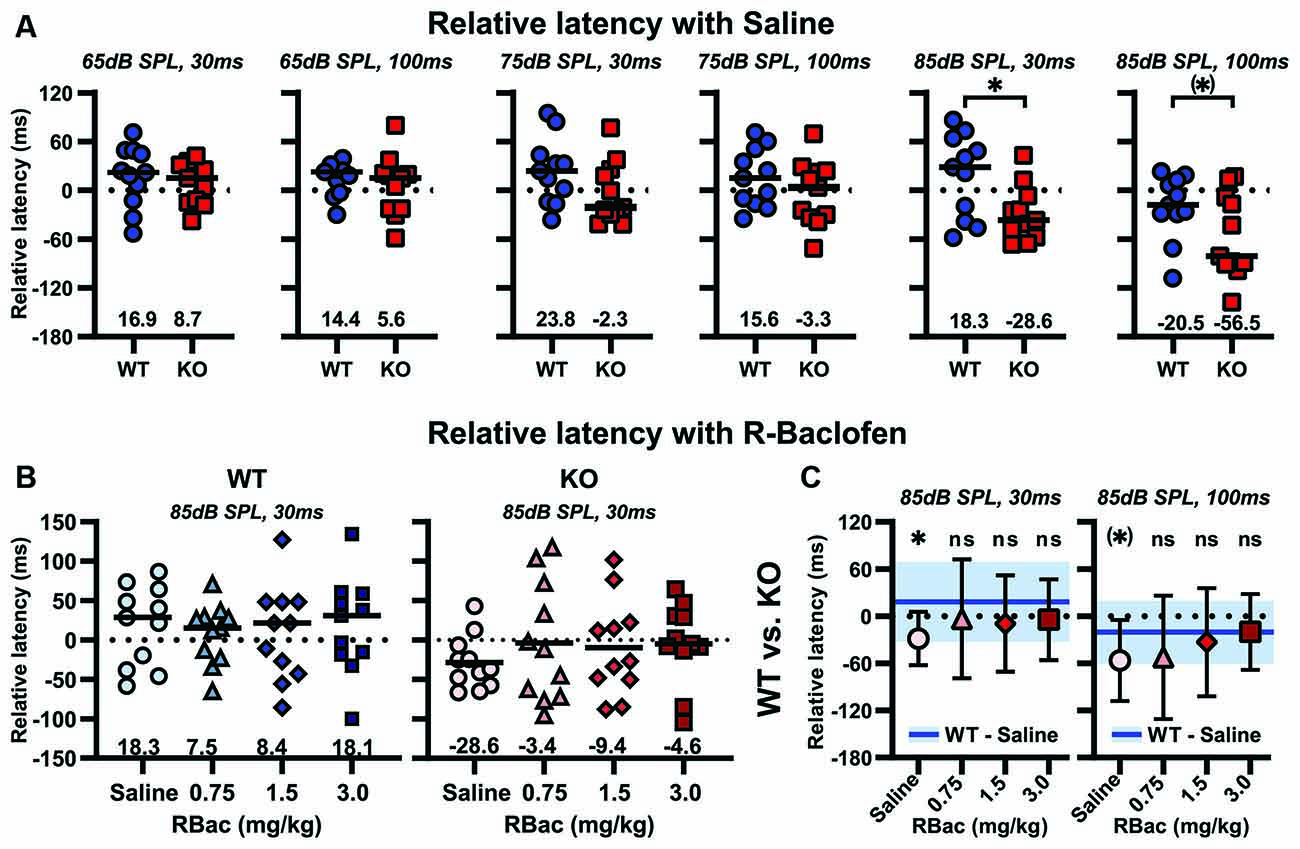
Figure 9. R-Baclofen increases the relative latencies of startle in PPI trials (ms) in Cntnap2 KO rats compared with WT controls. (A) Relative latencies of startle in PPI trials for six different prepulse conditions with three stimulus levels at two different ISIs. Scatter plots depict individual relative latencies of startle in PPI trials for each prepulse condition and black horizontal lines represent the mean relative latency of startle. After saline injection, Cntnap2 KO rats (red squares) had consistently shorter relative latencies of startle in PPI trials than WT rats (blue circles), with a significant difference for the prepulse condition 85 dB SPL, 30 ms and a tendency for the prepulse condition 85 dB SPL, 100 ms (two-sided student’s t-test, from left to right: 65 dB SPL, 30 ms; p = 0.5569; 65 dB SPL, 100 ms; p = 0.5043; 75 dB SPL, 30 ms; p = 0.1335; 75 dB SPL, 100 ms; p = 0.2566; 85 dB SPL, 30 ms; p = 0.0195; 85 dB SPL, 100 ms; p = 0.0819). (B) Scatter plots depicting individual (symbols) and mean relative latencies of startle in PPI trials (black horizontal lines) for prepulse condition 85 dB SPL, 30 ms in Cntnap2 WT (Left, blue) and KO rats (Right, red) after injection of saline (circles), 0.75 mg/kg (triangles), 1.5 mg/kg (diamonds), and 3 mg/kg R-Baclofen (squares). R-Baclofen did not significantly increase relative latencies in Cntnap2 WT (Left: WT, RM ANOVA, p = 0.9282, F = 0.1226). There was a slight, yet statistically not significant, increase in KO rats (Right: KO, RM ANOVA, p = 0.5611, F = 0.6374). (C, Left) Mean ± SD relative latency to prepulse condition 85 dB SPL, 30 ms in Cntnap2 KO rats was significantly different from WT controls (after saline, blue line and area) with saline (two-sided student’s t-test, p = 0.0195), and similar to controls with 0.75 mg/kg (two-sided student’s t-test, p = 0.4381), 1.5 mg/kg (two-sided student’s t-test, p = 0.2627) and 3 mg/kg R-Baclofen (two-sided student’s t-test, p = 0.3069). (C, Right) Mean ± SD relative latency to prepulse condition 85 dB SPL, 100 ms in Cntnap2 KO rats was by tendency different from WT controls (after saline, blue line and area) with saline (two-sided student’s t-test, p = 0.0819), and similar to controls with 0.75 mg/kg (Welch’s t-test, p = 0.2479), 1.5 mg/kg (two-sided student’s t-test, p = 0.6028) and 3 mg/kg R-Baclofen (two-sided student’s t-test, p = 0.9833). Dotted horizontal lines at 0 Relative Latency (ms) represent similar latency to the maximum startle response in trials with vs. without a prepulse. (*)p < 0.1, *p < 0.05; n.s.: not significant.
Discussion
The present study sought to investigate whether selective activation of GABAB receptors can remediate ASD-related altered sensory processing reliant on auditory brainstem function. We, therefore, compared behavioral read-outs of brainstem auditory signaling from rats with the homozygous knockout of Cntnap2 to their WT littermates, with and without administration of R-Baclofen. Homozygous loss-of-function of Cntnap2 leads to characteristic changes in brainstem-mediated auditory processing and behavior (Scott et al., 2018, 2020). Here, we demonstrate that these functional changes are accompanied by increased levels of excitatory and inhibitory neurotransmitters in the startle-mediating PnC and that they can largely be remediated by selective activation of GABAB receptors through R-Baclofen. In the present study, R-Baclofen: (1) improved deficient sensory filtering by enhancing short-term habituation; (2) suppressed exaggerated responses to moderately loud startling sounds; (3) rectified dynamic range response characteristics including ASR threshold, half-maximal response, and saturation; (4) improved sensorimotor gating by means of the relative amount of PPI and latency of startle in PPI trials; (5) but did not improve startle sensitization and peak response latency at ASR threshold in Cntnap2 KO rats. Therefore, our results provide evidence that GABAB receptor agonists may be useful for pharmacologically targeting multiple aspects of sensory processing disruptions in ASD.
E/I Imbalance in Cntnap2 KO Rats
Perturbed balance in neuronal excitation and inhibition is commonly assumed a possible final shared mechanism in autism (for review, see Rubenstein and Merzenich, 2003) that might underlie altered auditory processing in ASD (for review, see Sinclair et al., 2017b). Cntnap2 is suggested to be involved in the regulation of neuronal circuit E/I balance, evidenced by decreased dendritic arborization and spine development after Cntnap2 knockdown in cortical neurons (Anderson et al., 2012), and by increased excitatory synaptic input (Scott et al., 2017) and disrupted maturation of GABAergic inhibitory transmission in the cortex of Cntnap2 KO mice (Bridi et al., 2017). Given the expression of Cntnap2 along the ascending auditory and startle-mediating pathways (Figure 1)—including auditory nerve, dorsal, ventral, and granular layers of the cochlear nucleus (CN), SOC, dorsal nucleus of the lateral lemniscus, inferior colliculus, medial geniculate body, CRN, PnC, and pedunculopontine tegmental nucleus (PPT; Gordon et al., 2016; Scott et al., 2018)—it is plausible to assume that an irregular E/I balance in the auditory brainstem from Cntnap2 KO rats is underlying the ASD-like altered implicit auditory-evoked behaviors observed in the present study (Figures 3, 8A, 9A). Indeed, quantification of amino acid levels through MALDI MS imaging demonstrated an increase in glutamine, glutamate, and GABA in the PnC from Cntnap2 KO rats (Figure 4). Herein, GABA appeared to be disproportionally elevated, evidenced by lower Glu/GABA, increased GABA/Glu, but similar Glu/Gln ratio compared with WT controls. Due to the limited spatial resolution of MALDI imaging (80 μm), we can neither draw conclusions about the (sub-) cellular localization (extra- or intracellular, neuronal or glial, vesicular or cytoplasmic) of the detected amino acids, nor about the availability of the neurotransmitters for synaptic signaling (Waagepetersen et al., 2001; for reviews see Choudhury et al., 2011; Coghlan et al., 2012). Astrocytic-derived glutamine is the precursor of both glutamate and GABA. Normally, more glutamine is transferred from astrocytes to glutamatergic neurons, since GABAergic neurons have a greater capability of re-utilizing their neurotransmitter by re-uptake (for review, see Walls et al., 2015). The perturbed relations between Gln, Glu, and GABA in the PnC from Cntnap2 KO rats indicate a dysregulation in the glutamine-glutamate/GABA re-uptake and/or synthetization cycle that might result in disturbance of the functional E/I homeostasis and underlie ASD pathogenesis (Van Elst et al., 2014). These alterations could be the result of a compensatory upregulation of neurotransmitter levels in response to decreased postsynaptic receptor availability, as has been observed in form of decreased glutamate receptor expression in the PFC from Cntnap2 KO mice (Kim et al., 2019), impaired glutamate receptor trafficking to the cell surface of hippocampal Cntnap2 KO neurons (Varea et al., 2015), and reduced GABA receptor subunit expression in autistic human brain samples (Fatemi et al., 2009; Blatt and Fatemi, 2011). A compensatory upregulation of GAD67, one of the isoforms of the key synthesizing enzymes for GABA, has been observed in ASD brains, possibly to provide increased GABAergic feed-forward inhibition to compensate for the loss of cerebellar neurons (Yip et al., 2008). Interestingly, reduced numbers of cortical GABAergic interneurons have also been observed in Cntnap2 KO mice (Peñagarikano et al., 2011) and reduced numbers of neurons in the auditory brainstem of humans with ASD (Kulesza et al., 2011). It remains to be elucidated whether Cntnap2 KO rats have reduced numbers of neurons in the PnC and whether the elevated GABA level observed in our study is correlated with (insufficient) compensatory upregulation (Antoine et al., 2019) of GABAergic feedforward inhibition from the PPT to the PnC (Yeomans et al., 2010; Fulcher et al., 2020). It should be noted that abnormalities in glutamine, glutamate, and GABA levels appear to be highly age-, species-, strain-, and brain region/circuit-specific (Horder et al., 2013, 2018a,b; Van Elst et al., 2014). Interestingly, GABA, Glu, and Gln levels were not altered in the SOC within the auditory brainstem of Cntnap2 KO rats (Supplementary Figure 4). This indicates that Cntnap2 might not generally interact with the Glu-Gln/GABA system throughout the brain. It rather suggests that Glu-Gln/GABA system dysregulation might be a secondary effect of functional Cntnap2 deletion that is confined to certain brain regions or neural circuits. Analogous to our findings in the PnC from Cntnap2 KO rats, BTBR T+tf/J mice show increases in all three amino acids particularly in the striatum, but not in the PFC (Horder et al., 2018b). Several other rodent models of ASD presented with other distinct Glu, Gln, and GABA concentration profiles, three of them recapitulating the reduction in striatal Glu from the adult autistic human cohort (Horder et al., 2018b). Higher absolute concentrations of GABA and glutamate, as well as lower Glu/GABA ratios, on the other hand, have been described in blood plasma from pediatric and adolescent autistic patients (El-Ansary and Al-Ayadhi, 2014; Al-Otaish et al., 2018). Increased combined Gln and Glu signals in the anterior cingulate from children and adolescents with ASD have been interpreted as an indicator of neuronal overexcitation (Bejjani et al., 2012; Van Elst et al., 2014), and increased GABA—a product of glutamate metabolism—as a consequence of significantly elevated glutamate and/or decreased breakdown of GABA into glutamate (El-Ansary and Al-Ayadhi, 2014; for review, see Walls et al., 2015; Zheng et al., 2019). Given that baseline ASRs rely on the glutamatergic excitation of PnC giant neurons (Ebert and Koch, 1992), and GABA receptors on PnC giant neurons mediate a substantial part of PPI (Yeomans et al., 2010), the dysregulation of the Glu-Gln/GABA system likely perturbs acoustic startle circuitry and behavior in Cntnap2 KO rats. Even though future immunohistochemical and electrophysiological studies are needed to investigate the anatomical distribution and functional correlation of amino acid levels in the startle-mediating pathway in closer detail, our results strongly indicate that altered implicit auditory-evoked behaviors commonly observed in ASD (Chamberlain et al., 2013; Kohl et al., 2014; Takahashi et al., 2016) might result from disturbed E/I balance within the neuronal startle circuit.
R-Baclofen Mechanism of Action
At this point, we can only speculate how R-Baclofen treatment improves the behavioral read-outs of sensory processing in Cntnap2 KO rats. The efficacy of R-Baclofen could result from its ability to dampen hyperexcitability via pre- and postsynaptic mechanisms. Baclofen stimulates metabotropic GABAB receptors which function as presynaptic auto- or heteroreceptors to inhibit the vesicular release of GABA or glutamate, respectively (Waldmeier et al., 2008; Delaney et al., 2018). Postsynaptically, R-Baclofen activates inward-rectifying potassium channels that cause neuronal hyperpolarization. Together, these mechanisms serve to tonically hyperpolarize neurons, decrease resting membrane potential, and reduce cell firing (Gandal et al., 2012; for review, see Wu and Sun, 2015). R-Baclofen may be beneficial in Cntnap2 KO animals by counteracting the reported reduction in the number of GABAergic interneurons and asynchronous neuronal firing (Peñagarikano et al., 2011; Vogt et al., 2017), decreased GABAergic phasic and tonic inhibition (Bridi et al., 2017), increased neurotransmitter release and increased postsynaptic excitatory responses in Cntnap2 KO animals (Scott et al., 2017), and the dysregulated glutamine-glutamate/GABA cycle indicated by the lacking rebalance of Glu/GABA ratios in the present study.
In the auditory system, Baclofen has been shown to have large effects on overall excitability (Szczepaniak and Møller, 1995), including the suppression of sound-evoked activity and/or hyperexcitability in the CN (Martin, 1982; Caspary et al., 1984), inferior colliculus (Szczepaniak and Møller, 1996; Sun et al., 2006) and auditory cortex (Lu et al., 2011). In a genetic mouse model of E/I dysfunction, Baclofen dose-dependently normalized auditory-evoked potentials, elevated ASRs, and deficient PPI of ASRs. This was linked to the improvement of several elements of E/I homeostasis such as circuit excitability, neural synchrony, and signal-to-noise ratio (Gandal et al., 2012).
ASR amplitudes are the sum of habituation and the parallel independent process of sensitization, with habituation being the decrease and sensitization the initial increase in magnitude to a series of sound pulses (Payne and Anderson, 1967; Groves and Thompson, 1970; Geyer and Braff, 1982; Pilz and Schnitzler, 1996; Rankin et al., 2009). Impaired habituation and increased sensitization apparent in our study in Cntnap2 KO rats (Figures 3B,C) are also associated with ASD in humans (Perry et al., 2007; Chamberlain et al., 2013; Madsen et al., 2014). Short-term habituation relies on synaptic depression at the axon terminals of the CRN sensory afferents in the PnC (Figure 1), likely mediated by activation of voltage- and calcium-dependent potassium channels (Ebert and Koch, 1992; Weber et al., 2002; Simons-Weidenmaier et al., 2006; Zaman et al., 2017). Lack of Cntnap2 in KO rats might interfere directly with startle habituation through its function in clustering of voltage-gated potassium channels (Poliak et al., 2003; Dawes et al., 2018; Scott et al., 2018). At an auditory glutamatergic synapse featuring strong synaptic depression, Baclofen modulated transmitter release in an activity-dependent manner (Brenowitz et al., 1998) which might explain the improvement of short-term habituation in Cntnap2 WT and KO rats with R-Baclofen (Figures 5A–C). Sensitization, on the other hand, is caused by extrinsic modulation of the startle pathway (Figure 1) by structures including the periaqueductal gray, the amygdala, and the bed nucleus of the stria terminalis (Leaton and Supple, 1986; Fendt et al., 1994a, b; Davis et al., 1997)—structures that all express Cntnap2 (Alarcón et al., 2008; Gordon et al., 2016). The ineffectiveness of R-Baclofen to suppress increased ASR sensitization in Cntnap2 KO rats or sensitization in WT controls (Figure 5D) might be due to the fact that the modulatory input from these structures altering sensitization includes several neurotransmitters other than GABA or Glu, such as noradrenaline (Fendt et al., 1994a), substance P (Krase et al., 1994), glycine (Plappert et al., 2001), or dopamine (Halberstadt and Geyer, 2009). In support of this notion, Baclofen was also unable to reverse dopamine receptor agonist apomorphine-induced disruptions in sensorimotor gating, while it did reverse NMDA receptor antagonist effects (Bortolato et al., 2004).
Baclofen and its formulations R- and S-Baclofen are well known to suppress ASRs in controls and in animals with proposed E/I dysfunction, either genetically or pharmacologically induced (e.g., Bortolato et al., 2004; Lu et al., 2011; Gandal et al., 2012). In our hands, R-Baclofen was more potent in Cntnap2 WT rats (effective dose of 0.75 mg/kg) than in Cntnap2 KO rats (effective dose of 1.5 mg/kg, Figure 6) and more effective in female than in male KO rats (Supplementary Figure 5). This sex-dependent effect of R-Baclofen on the ASR I-O function is probably due to the fact that Cntnap2 KO males had higher ASR magnitudes than females to begin with (i.e., without R-Baclofen, Figures 3D,E). Sex effects on ASR I-O function in untreated rats from the Cntnap2 model have been described before (Scott et al., 2018). In humans, the male prevalence of ASD symptoms has been attributed to sex-differential factors such as reduced susceptibility in females, or lower mutational burden threshold in males. In this regard, mutations affecting GABA signaling appear to be particularly pervasive in males (for reviews, see Werling and Geschwind, 2013; Rylaarsdam and Guemez-Gamboa, 2019), and Cntnap2 mutations affect functional responses of cortical circuitry more strongly in male than in female mice (Townsend and Smith, 2017). Interestingly, the Cntnap2 gene is differentially expressed in sexually dimorphic song nuclei essential for vocal learning in songbirds (Panaitof et al., 2010) in accordance with the sexual dimorphism of neural circuitry in vocal control areas (Nottebohm and Arnold, 1976); and genetic variants in the CNTNAP2 gene are associated with gender differences among dyslexic children (Gu et al., 2018). Exploring in more detail the neurobiological basis of sex-dependent differences in startle responses and efficacy of R-Baclofen found in Cntnap2 KO rats should be considered in future studies.
In addition to differences in the effective dose, R-Baclofen suppressed ASR magnitudes across a wide range of startle pulse intensities in Cntnap2 WT rats, whereas in KO rats the maximum ASR capacity was unaltered (Figure 6). A similar phenomenon has been described in rats after treatment with S-Baclofen to suppress salicylate-induced enhancement of ASRs (Lu et al., 2011). The robustly increased ASRs to high sound intensities in Cntnap2 KO rats might be due to increased excitatory input from the CN to the PnC (Figure 1). Behavioral studies showed that electrolytic lesions of the CN reduced ASRs particularly to loud sound intensities of 110 and 115 dB SPL (Meloni and Davis, 1998). In contrast, chemical lesions of CRNs or the PnC blocked ASRs at all intensities (Lee et al., 1996). Interestingly, Flores et al. (2015) identified an alternative pathway from the cochlea to the CN for the detection of loud, potentially tissue-damaging, auditory stimuli. One might speculate if this form of sensation (termed “auditory nociception”) is increased in Cntnap2 KO rats and contributes to their exaggerated ASRs (Figure 3) as well as greater active sound avoidance (Scott et al., 2020). “Auditory nociception” would have similarities to C-fiber nociception (Flores et al., 2015) which is indeed enhanced in Cntnap2 KO animals (Dawes et al., 2018). Taken together, the dramatically reduced ASRs including maximum capacity in Cntnap2 WT rats by R-Baclofen (Figure 6) might be predominantly due to reduced excitability in CRNs and/or the PnC. In contrast, in Cntnap2 KO rats, the R-Baclofen-induced suppression of exaggerated responses to moderate startling sounds might be the behavioral outcome of an interaction between reduction in CRNs and/or PnC hyperexcitability, and unproportionally high excitatory input from the CN to PnC.
The decrease of ASRs to moderate startle pulse intensities through R-Baclofen in Cntnap2 KO rats was accompanied by the normalization of their ASR thresholds to control levels, indicated by an increase of the minimum sound intensity required to elicit a response (from about 83–89 dB SPL at the 25% response magnitude, Figure 7, Supplementary Table 2). The high acoustic input required to reach the ASR threshold and elicit a motor response is likely determined by a high firing threshold in the CRNs. In contrast, electrophysiological data have shown that PnC neurons, that receive rapid input from the CRNs, have a relatively low firing threshold (Wagner and Mack, 1998; Brosda et al., 2011). Given the expression of Cntnap2 in CRNs from WT rats (Scott et al., 2018), its lack in Cntnap2 KO rats may result in neuronal hyperexcitability in the CRNs, leading to lower ASR thresholds (Figure 3H). CRN neurons receive inhibitory GABAergic input that modulates their neuronal responses and consequently the ASR output (for review, see Osen et al., 1991; Gómez-Nieto et al., 2008). Therefore, R-Baclofen might attenuate intrinsic excitability and increase firing thresholds of CRNs, and thereby normalize ASR thresholds in Cntnap2 KO rats. Alternatively, R-Baclofen might take effect by blocking the glutamate release from the auditory nerve fibers (Martin, 1982) synapsing onto CRNs (Gómez-Nieto et al., 2014).
The normalization of the ASR threshold in Cntnap2 KO rats through R-Baclofen was correlated with a parallel rightward shift of the I-O function, determined by an increase in the half-maximal response (ES50) and ASR saturation (90% response magnitude, Figure 7, Table 3). This means that—while the extent of the I-O dynamic range remained similar—the I-O dynamic range was shifted to higher startle pulse intensities. Conversely, this indicates that the acoustic stimulus potency was decreased by R-Baclofen. In the dynamic range of the I-O function, a small stimulus change can produce a large response change (Stoddart et al., 2008) and the slope is an important aspect of the ASR I-O function as it directly reflects the sensorimotor integration process (Hince and Martin-Iverson, 2005). R-Baclofen did not induce a change in ASR I-O slope within the genotype (Supplementary Figure 7 and Supplementary Table 3). Therefore, it can be assumed that in Cntnap2 KO rats the ASR efficiency, i.e., the transduction of sensory information into motor output, remained at a similar rate (Figure 2). This speaks against a generalized increase in inhibition of the ASR system through R-Baclofen, as this would also predict a change in slope (Hince and Martin-Iverson, 2005). Interestingly, Martin-Iverson and Stevenson (2005) found a change in ASR I-O slope through emotional modulatory input such as fear, modified by dopaminergic signaling (Figure 1). It should be pointed out that R-Baclofen normalized the ASR I-O fitted curves from Cntnap2 KO rats to control levels, despite the unaltered slope in the within genotype comparisons (Figures 7A–C, Supplementary Figure 7).
ASR magnitude and latency are in general negatively correlated (i.e., the higher the magnitude, the shorter the latency; Hoffman and Searle, 1968). In a unique approach, we analyzed ASR peak latencies from individual animals relative to their dynamic range characteristics (i.e., threshold, ES50, saturation, Figures 7F–H). This allowed us to investigate the processing speed between sensory (acoustic) input and maximum ASR motor output without the confounding effect of genotype-related differences in startle magnitudes. Peak latencies were significantly shorter in Cntnap2 KO than in WT rats, specifically at the ASR threshold. Surprisingly, R-Baclofen led to even shorter, rather than longer, ASR peak latencies in Cntnap2 KO rats. As outlined above, motor responses to low, near-threshold, acoustic inputs are likely gated by CRN activity (Wagner and Mack, 1998; Brosda et al., 2011). It might be possible that the shift in threshold to higher sound intensities in Cntnap2 KO rats with R-Baclofen goes along with more synchronous short-latency inputs to the PnC, thereby speeding up temporal processing (Gandal et al., 2012; Harris and Dubno, 2017). In contrast to GABAB receptor activation through R-Baclofen, pharmacological modulation of other neurotransmitter receptors targeting ASR sensitization might have shown normalizing effects on ASR latency, since the course of response latency is dominated by ASR sensitization (Pilz and Schnitzler, 1996).
In addition to increased acoustic reactivity, Cntnap2 KO rats consistently presented with disrupted sensorimotor gating in two of our previous studies, despite differences in the acoustic prepulse conditions (Scott et al., 2020) or breeding scheme (Scott et al., 2018). In line with these previous results, Cntnap2 KO rats in the present study also displayed robustly lower PPI of ASRs than WT controls (Figure 8A). These differences were statistically significant for two prepulse conditions (75 dB SPL, 100 ms and 85 dB SPL, 30 ms ISI) with a random permutation test for small sample sizes. R-Baclofen improved sensorimotor gating in Cntnap2 KO rats as shown by a dose-dependent increase in PPI for four prepulse conditions (75 dB SPL, 30 ms; 75 dB SPL, 100 ms; 85 dB SPL, 30 ms; 85 dB SPL, 100 ms, Figure 8C). Likewise, enhancing GABAergic inhibition through Baclofen previously rescued PPI disrupted by pharmacological NMDA receptor blockade (Bortolato et al., 2004; Arai et al., 2008; Fejgin et al., 2009) or hypofunction (Gandal et al., 2012). In control animals, Baclofen per se produced no significant changes in PPI at any given dose in these previous studies (Bortolato et al., 2004), similar to Cntnap2 WT rats in our study (Figure 8B). This was due to the uniform suppression of response magnitudes in trials with and without a prepulse (Supplementary Figure 9A). In contrast, in Cntnap2 KO rats the response magnitudes to the prepulse + startle pulse condition were suppressed more strongly by R-Baclofen than the ones to the startle pulse alone condition (Supplementary Figure 9B). Previous studies have demonstrated the involvement of GABAB receptors in prepulse processing and sensorimotor gating (Koch et al., 2000; Takahashi et al., 2007; Yeomans et al., 2010). R-Baclofen might improve the behavioral salience of weak acoustic prepulses through increased feedforward inhibition onto the PnC (Carlson and Willott, 1996; Price et al., 2008; Antoine et al., 2019) achieved by decreased spontaneous firing (“neuronal noise”) and improved neural synchrony in response to the prepulse (Gandal et al., 2012) within the PPI circuitry (Figure 1). It is unlikely that the improved sensorimotor gating was due to changes in detectability of the prepulse in the auditory periphery (i.e., hearing thresholds) since Baclofen does not affect the sound-evoked cochlear output and summed auditory nerve potentials (Martin, 1982). In addition, the prepulse elicited response (at 100 ms, Supplementary Figure 10) was not increased with R-Baclofen, which is different from a pharmacologically induced rodent model of schizophrenia-like sensorimotor gating deficits (Yee et al., 2004).
In line with our previous results (Scott et al., 2018), Cntnap2 KO rats did not only show disrupted PPI in terms of amplitudes but also a lack of the typical increase in startle latency in PPI trials (Figure 9A; Ison et al., 1973; Hoffman and Ison, 1980). However, in contrast to ASR peak latencies (at the threshold, Figures 7F–H), R-Baclofen prolonged and normalized the startle latency in PPI trials from Cntnap2 KO rats (Figure 9C). This might underscore that the changes in neuronal transmission rectifying not only PPI amplitudes but also latencies mainly lie within the circuit branch processing prepulse information and take effect downstream of the CRN (i.e., GABAergic PPT projections onto PnC).
Model Validity and Clinical Implications
Even though we cannot fully exclude dose-dependent myorelaxant properties of R-Baclofen (Davidoff, 1985; Nevins et al., 1993), it is reasonable to assume that the changes we observed in Cntnap2 KO rats were mostly due to the brainstem processing involved in ASR generation. This is because the maximum ASR as a putative index for motor capacity (Hince and Martin-Iverson, 2005) was not altered in Cntnap2 KO rats even with 3 mg/kg R-Baclofen, and ASR peak latencies at the threshold were shortened, not prolonged. Importantly, intrathecal administration of Baclofen reversed enhanced ASRs and restored reduced PPI of the blink reflex in patients with spinal cord injury, strongly suggesting a muscle tone regulating effect of Baclofen at the brainstem level (Kumru et al., 2009; Kumru and Kofler, 2012). Future studies should address in more detail the sites and mechanisms of R-Baclofen action. The most promising target of R-Baclofen action is the PnC as it is the sensorimotor interface of the startle circuit (Figure 1), where the transition of sensory input into the motor output can be directly influenced (for review, see Koch, 1999). Using cannulated microelectrodes, R-Baclofen infusions into the PnC and simultaneous electrophysiological recordings in behaving Cntnap2 WT and KO rats would allow to assess changes in startle responses correlated to changes in PnC neuronal activity without possible systemic effects of R-Baclofen. Suppression of the speculated PnC hyperexcitability in Cntnap2 KO rats through local application of R-Baclofen might attenuate their exaggerated startle responses, in particular to moderate startling sounds. Furthermore, microinfusions of R-Baclofen to the cochlear round window membrane might be a useful tool to dissect the contribution of the sensory (as opposed to motor) branch in the ASR pathway to effects observed in our study. The round window membrane delivery approach of R-Baclofen to the inner ear might reduce glutamate release from the auditory nerve fibers synapsing onto CRNs (Gómez-Nieto et al., 2014), resulting in less sound-evoked PnC activity, and possibly a shift in ASR thresholds as well as reduced startle response magnitudes. Lastly, R-Baclofen-induced alterations in modulatory input to the PnC might be identified through local application to the PPT. Simultaneous electrophysiological recordings of sound-evoked activity in PnC neurons to a prepulse+startle pulse sound paradigm would help scrutinize R-Baclofen-induced changes in GABAergic feedforward inhibition from the PPT to the PnC that might underlie altered PPI of startle in Cntnap2 KO rats in the present study. On a cellular level, R-Baclofen actions on excitatory and inhibitory transmission (mediated by presynaptic GABAB heteroreceptors or autoreceptors, respectively) could be addressed by examining its effects on excitatory (glutamatergic) and inhibitory (GABAergic) postsynaptic currents using whole-cell voltage clamp recordings in PnC giant neurons from Cntnap2 WT and KO rats.
Rats with homozygous, and to a lesser extent heterozygous, functional deletion of the Cntnap2 gene display behavioral alterations that parallel core symptoms of ASD, including deficits in sociability, repetitive stereotypy, and sensory abnormalities (Scott et al., 2018, 2020). Therefore, the Cntnap2 rat model for autism does not only have a high construct but also face validity. This is particularly important considering that ASD diagnosis and consequently validation of treatments rely on evaluating behavioral traits both in clinical testing and in preclinical models that seek to recapitulate those behavioral traits from humans (for reviews, see Servadio et al., 2015; Kazdoba et al., 2016; Möhrle et al., 2020; Scott et al., 2021). One limitation of our study might be that single gene mutations such as Cntnap2 account for no more than 1% of ASD cases (for review, see Yoo, 2015). However, the majority of ASD susceptibility genes seem to converge in shared or interacting biological pathways that are typically involved in synapse formation and function, transcriptional control, and chromatin-remodeling (De Rubeis et al., 2014; Iossifov et al., 2014; Pinto et al., 2014). Therefore, monogenic rodent models including Cntnap2 are useful tools in the search of standardized objective biomarkers for the neurological basis, and the utility of diagnosis and treatment of ASD.
Exaggerated acoustic reactivity and impaired sensorimotor gating have been described in individuals with autism (Perry et al., 2007; Chamberlain et al., 2013; Kohl et al., 2014; Takahashi et al., 2016) along with other sensory alterations affecting the auditory, visual, touch, smell/taste and pain domain. Exploring the usefulness of therapeutic approaches to rectify sensory alterations might be of particular importance considering that atypical low-level sensory processing might exacerbate or interact with other, higher-level, symptoms in individuals with ASD (O’Neill and Jones, 1997; Leekam et al., 2007). For example, regarding the auditory system, timing deficits within the brainstem negatively impact rapid acoustic processing, predictive of a higher risk for developing speech processing issues and language disorders (Benasich et al., 2002; Wible et al., 2004; Abrams et al., 2006), representing core symptoms of ASD (for review, see Alarcón et al., 2008; Mody and Belliveau, 2013; Rodenas-Cuadrado et al., 2016). Interestingly, rodent models with mutations in Cntnap2 parallel slowed neurotransmission along the ascending auditory brainstem reported in ASD (Rosenhall et al., 2003; Kwon et al., 2007; Miron et al., 2016; Scott et al., 2018), and deficient language-relevant rapid auditory processing seen in infants carrying variants of Cntnap2 (Truong et al., 2015; Riva et al., 2018). Targeting E/I balance to modulate more spectrotemporally complex auditory processes such as brainstem representation and higher-level perception of speech-like sounds in Cntnap2 KO rats is an exciting consideration for future studies.
Conclusion
In conclusion, this study demonstrated a relationship between Cntnap2 gene deletion, disrupted excitatory/inhibitory homeostasis, auditory brainstem-mediated sensory processing, and symptoms of ASD. Increasing GABAergic signaling via the GABAB receptor agonist R-Baclofen improved many aspects of acoustic reactivity, sensory filtering, and sensorimotor gating in Cntnap2 KO rats. These findings encourage further efforts to establish translatable paradigms based on auditory-evoked behaviors for preclinical and clinical therapeutic screening for neurodevelopmental disorders. Our results support the hypothesis that enhancing inhibitory transmission improves ASD relevant deficits and that GABAB receptors are a promising therapeutic target for restoring neural circuit and behavioral abnormalities in disorders characterized by E/I imbalance.
Data Availability Statement
The raw data supporting the conclusions of this article will be made available by the authors, without undue reservation.
Ethics Statement
The animal study was reviewed and approved by the University of Western Ontario Animal Care Committee, and all procedures were in accordance with the guidelines established by the Canadian Council on Animal Care.
Author Contributions
DM, SW, and SS: participated in research design. DM and WW: conducted experiments. DM: performed data analysis. DM, WW, SW, and SS: wrote or contributed to the writing of the manuscript. All authors contributed to the article and approved the submitted version.
Funding
This research was supported by Canadian Institutes of Health Research (CIHR), Natural Sciences and Engineering Research Council of Canada (NSERC), BrainsCAN (Rat Behavioral Facility), and the Deutsche Forschungsgemeinschaft (DFG, German Research Foundation)—project number 442662585.
Conflict of Interest
The authors declare that the research was conducted in the absence of any commercial or financial relationships that could be construed as a potential conflict of interest.
Publisher’s Note
All claims expressed in this article are solely those of the authors and do not necessarily represent those of their affiliated organizations, or those of the publisher, the editors and the reviewers. Any product that may be evaluated in this article, or claim that may be made by its manufacturer, is not guaranteed or endorsed by the publisher.
Acknowledgments
We thank SFARI for providing R-Baclofen.
Supplementary Material
The Supplementary Material for this article can be found online at: https://www.frontiersin.org/articles/10.3389/fnint.2021.710593/full#supplementary-material.
References
Abrams, D. A., Nicol, T., Zecker, S. G., and Kraus, N. (2006). Auditory brainstem timing predicts cerebral asymmetry for speech. J. Neurosci. 26, 11131–11137. doi: 10.1523/JNEUROSCI.2744-06.2006
Alarcón, M., Abrahams, B. S., Stone, J. L., Duvall, J. A., Perederiy, J. V., Bomar, J. M., et al. (2008). Linkage, association and gene-expression analyses identify Cntnap2 as an autism-susceptibility gene. Am. J. Hum. Genet. 82, 150–159. doi: 10.1016/j.ajhg.2007.09.005
Al-Otaish, H., Al-Ayadhi, L., Bjørklund, G., Chirumbolo, S., Urbina, M. A., El-Ansary, A., et al. (2018). Relationship between absolute and relative ratios of glutamate, glutamine and GABA and severity of autism spectrum disorder. Metab. Brain Dis. 33, 843–854. doi: 10.1007/s11011-018-0186-6
Anderson, G. R., Galfin, T., Xu, W., Aoto, J., Malenka, R. C., Südhof, T. C., et al. (2012). Candidate autism gene screen identifies critical role for cell-adhesion molecule CASPR2 in dendritic arborization and spine development. Proc. Natl. Acad. Sci. U S A 109, 18120–18125. doi: 10.1073/pnas.1216398109
Antoine, M. W., Langberg, T., Schnepel, P., and Feldman, D. E. (2019). Increased excitation-inhibition ratio stabilizes synapse and circuit excitability in four autism mouse models. Neuron 101, 648–661.e644. doi: 10.1016/j.neuron.2018.12.026
Arai, S., Takuma, K., Mizoguchi, H., Ibi, D., Nagai, T., Takahashi, K., et al. (2008). Involvement of pallidotegmental neurons in methamphetamine- and MK-801-induced impairment of prepulse inhibition of the acoustic startle reflex in mice: reversal by GABAB receptor agonist baclofen. Neuropsychopharmacology 33, 3164–3175. doi: 10.1038/npp.2008.41
Bejjani, A., O’Neill, J., Kim, J. A., Frew, A. J., Yee, V. W., Ly, R., et al. (2012). Elevated glutamatergic compounds in pregenual anterior cingulate in pediatric autism spectrum disorder demonstrated by 1H MRS and 1H MRSI. PLoS One 7:e38786. doi: 10.1371/journal.pone.0038786
Benasich, A. A., Thomas, J. J., Choudhury, N., and Leppänen, P. H. T. (2002). The importance of rapid auditory processing abilities to early language development: evidence from converging methodologies. Dev. Psychobiol. 40, 278–292. doi: 10.1002/dev.10032
Berry-Kravis, E., Hagerman, R., Visootsak, J., Budimirovic, D., Kaufmann, W. E., Cherubini, M., et al. (2017). Arbaclofen in fragile X syndrome: results of phase 3 trials. J. Neurodev. Disord. 9:3. doi: 10.1186/s11689-016-9181-6
Berry-Kravis, E. M., Hessl, D., Rathmell, B., Zarevics, P., Cherubini, M., Walton-Bowen, K., et al. (2012). Effects of STX209 (arbaclofen) on neurobehavioral function in children and adults with fragile X syndrome: a randomized, controlled, phase 2 trial. Sci. Transl. Med. 4:152ra127. doi: 10.1126/scitranslmed.3004214
Blatt, G. J., and Fatemi, S. H. (2011). Alterations in GABAergic biomarkers in the autism brain: research findings and clinical implications. Anat. Rec. (Hoboken) 294, 1646–1652. doi: 10.1002/ar.21252
Bortolato, M., Frau, R., Aru, G. N., Orrù, M., and Gessa, G. L. (2004). Baclofen reverses the reduction in prepulse inhibition of the acoustic startle response induced by dizocilpine, but not by apomorphine. Psychopharmacology 171, 322–330. doi: 10.1007/s00213-003-1589-5
Braff, D. L., Geyer, M. A., and Swerdlow, N. R. (2001). Human studies of prepulse inhibition of startle: normal subjects, patient groups and pharmacological studies. Psychopharmacology (Berl) 156, 234–258. doi: 10.1007/s002130100810
Brenowitz, S., David, J., and Trussell, L. (1998). Enhancement of synaptic efficacy by presynaptic GABAB receptors. Neuron 20, 135–141. doi: 10.1016/s0896-6273(00)80441-9
Bridi, M. S., Park, S. M., and Huang, S. (2017). Developmental diisruption of GABAA R-meditated inhibition in Cntnap2 KO mice. eNeuro 4:ENEURO.0162–0117.2017. doi: 10.1523/ENEURO.0162-17.2017
Brosda, J., Hayn, L., Klein, C., Koch, M., Meyer, C., Schallhorn, R., et al. (2011). Pharmacological and parametrical investigation of prepulse inhibition of startle and prepulse elicited reactions in wistar rats. Pharmacol. Biochem. Behav. 99, 22–28. doi: 10.1016/j.pbb.2011.03.017
Carlson, S., and Willott, J. F. (1996). The behavioral salience of tones as indicated by prepulse inhibition of the startle response: relationship to hearing loss and central neural plasticity in C57BL/6J mice. Hear. Res. 99, 168–175. doi: 10.1016/s0378-5955(96)00098-6
Caspary, D. M., Rybak, L. P., and Faingold, C. L. (1984). Baclofen reduces tone-evoked activity of cochlear nucleus neurons. Hear. Res. 13, 113–122. doi: 10.1016/0378-5955(84)90102-3
Caughlin, S., Park, D. H., Yeung, K. K., Cechetto, D. F., and Whitehead, S. N. (2017). Sublimation of DAN matrix for the detection and visualization of gangliosides in rat brain tissue for MALDI imaging mass spectrometry. J. Vis. Exp. 121:55254. doi: 10.3791/55254
Chamberlain, P. D., Rodgers, J., Crowley, M. J., White, S. E., Freeston, M. H., South, M., et al. (2013). A potentiated startle study of uncertainty and contextual anxiety in adolescents diagnosed with autism spectrum disorder. Mol. Autism 4:31. doi: 10.1186/2040-2392-4-31
Chen, C., Laviolette, S. R., Whitehead, S. N., Renaud, J. B., and Yeung, K. K. (2021). Imaging of neurotransmitters and small molecules in brain tissues using laser desorption/ionization mass spectrometry assisted with zinc oxide nanoparticles. J. Am. Soc. Mass Spectrom. 32, 1065–1079. doi: 10.1021/jasms.1c00021
Choudhury, P., Lahiri, S., and Rajamma, U. (2011). Glutamate mediated signaling in the pathophysiology of autism spectrum disorders. Pharmacol. Biochem. Behav. 100, 841–849. doi: 10.1016/j.pbb.2011.06.023
Coghlan, S., Horder, J., Inkster, B., Mendez, M. A., Murphy, D. G., Nutt, D. J., et al. (2012). GABA system dysfunction in autism and related disorders: from synapse to symptoms. Neurosci. Biobehav. Rev. 36, 2044–2055. doi: 10.1016/j.neubiorev.2012.07.005
Csomor, P. A., Yee, B. K., Vollenweider, F. X., Feldon, J., Nicolet, T., and Quednow, B. B. (2008). On the influence of baseline startle reactivity on the indexation of prepulse inhibition. Behav. Neurosci. 122, 885–900. doi: 10.1037/0735-7044.122.4.885
Danesh, A. A., Lang, D., Kaf, W., Andreassen, W. D., Scott, J., Eshraghi, A. A., et al. (2015). Tinnitus and hyperacusis in autism spectrum disorders with emphasis on high functioning individuals diagnosed with Asperger’s Syndrome. Int. J. Pediatr. Otorhinolaryngol. 79, 1683–1688. doi: 10.1016/j.ijporl.2015.07.024
Davidoff, R. A. (1985). Antispasticity drugs: mechanisms of action. Ann. Neurol. 17, 107–116. doi: 10.1002/ana.410170202
Davis, M., Walker, D. L., and Lee, Y. (1997). Roles of the amygdala and bed nucleus of the stria terminalis in fear and anxiety measured with the acoustic startle reflex. Ann. N.Y. Acad. Sci. 821, 305–331. doi: 10.1111/j.1749-6632.1997.tb48289.x
Dawes, J. M., Weir, G. A., Middleton, S. J., Patel, R., Chisholm, K. I., Pettingill, P., et al. (2018). Immune or genetic-mediated disruption of CASPR2 causes pain hypersensitivity due to enhanced primary afferent excitability. Neuron 97, 806–822.e810. doi: 10.1016/j.neuron.2018.01.033
De Rubeis, S., He, X., Goldberg, A. P., Poultney, C. S., Samocha, K., Ercument Cicek, A., et al. (2014). Synaptic, transcriptional and chromatin genes disrupted in autism. Nature 515, 209–215. doi: 10.1038/nature13772
Delaney, A. J., Crane, J. W., Holmes, N. M., Fam, J., and Westbrook, R. F. (2018). Baclofen acts in the central amygdala to reduce synaptic transmission and impair context fear conditioning. Sci. Rep. 8:9908. doi: 10.1038/s41598-018-28321-0
DSM-5, American Psychiatric Association. (2013). Diagnostic and Statistical Manual of Mental Disorders (DSM-5), 5th Edn. Washington, DC: American Psychiatric Publishing.
Ebert, U., and Koch, M. (1992). Glutamate receptors mediate acoustic input to the reticular brain stem. NeuroReport 3, 429–432. doi: 10.1097/00001756-199205000-00013
El-Ansary, A., and Al-Ayadhi, L. (2014). GABAergic/glutamatergic imbalance relative to excessive neuroinflammation in autism spectrum disorders. J. Neuroinflammation 11:189. doi: 10.1186/s12974-014-0189-0
Erickson, C. A., Veenstra-Vanderweele, J. M., Melmed, R. D., McCracken, J. T., Ginsberg, L. D., Sikich, L., et al. (2014). STX209 (arbaclofen) for autism spectrum disorders: an 8-week open-label study. J. Autism Dev. Disord. 44, 958–964. doi: 10.1007/s10803-013-1963-z
Fatemi, S. H., Folsom, T. D., Reutiman, T. J., and Thuras, P. D. (2009). Expression of GABAB receptors is altered in brains of subjects with autism. Cerebellum (London, England) 8, 64–69. doi: 10.1007/s12311-008-0075-3
Fejgin, K., Pålsson, E., Wass, C., Finnerty, N., Lowry, J., Klamer, D., et al. (2009). Prefrontal GABAB receptor activation attenuates phencyclidine-induced impairments of prepulse inhibition: involvement of nitric oxide. Neuropsychopharmacology 34, 1673–1684. doi: 10.1038/npp.2008.225
Fendt, M., Koch, M., and Schnitzler, H.-U. (1994a). Amygdaloid noradrenaline is involved in the sensitization of the acoustic startle response in rats. Pharmacol. Biochem. Behav. 48, 307–314. doi: 10.1016/0091-3057(94)90532-0
Fendt, M., Koch, M., and Schnitzler, H.-U. (1994b). Lesions of the central gray block the sensitization of the acoustic startle response in rats. Brain Res. 661, 163–173. doi: 10.1016/0006-8993(94)91193-2
Flores, E. N., Duggan, A., Madathany, T., Hogan, A. K., Márquez, F. G., Kumar, G., et al. (2015). A non-canonical pathway from cochlea to brain signals tissue-damaging noise. Curr. Biol. 25, 606–612. doi: 10.1016/j.cub.2015.01.009
Fulcher, N., Azzopardi, E., De Oliveira, C., Hudson, R., Schormans, A. L., Zaman, T., et al. (2020). Deciphering midbrain mechanisms underlying prepulse inhibition of startle. Prog. Neurobiol. 185:101734. doi: 10.1016/j.pneurobio.2019.101734
Gaetz, W., Bloy, L., Wang, D. J., Port, R. G., Blaskey, L., Levy, S. E., et al. (2014). GABA estimation in the brains of children on the autism spectrum: measurement precision and regional cortical variation. NeuroImage 86, 1–9. doi: 10.1016/j.neuroimage.2013.05.068
Gandal, M. J., Sisti, J., Klook, K., Ortinski, P. I., Leitman, V., Liang, Y., et al. (2012). GABAB-mediated rescue of altered excitatory-inhibitory balance, gamma synchrony and behavioral deficits following constitutive NMDAR-hypofunction. Transl. Psychiatry 2:e142. doi: 10.1038/tp.2012.69
Geyer, M. A., and Braff, D. L. (1982). Habituation of the blink reflex in normals and schizophrenic patients. Psychophysiology 19, 1–6. doi: 10.1111/j.1469-8986.1982.tb02589.x
Gómez-Nieto, R., Hormigo, S., and López, D. (2020). Prepulse inhibition of the auditory startle reflex assessment as a hallmark of brainstem sensorimotor gating mechanisms. Brain Res. 10:639. doi: 10.3390/brainsci10090639
Gómez-Nieto, R., Horta-Junior, J. A. C., Castellano, O., Herrero-Turrión, M. J., Rubio, M. E., López, D. E., et al. (2008). Neurochemistry of the afferents to the rat cochlear root nucleus: Possible synaptic modulation of the acoustic startle. Neuroscience 154, 51–64. doi: 10.1016/j.neuroscience.2008.01.079
Gómez-Nieto, R., Horta-Júnior, J. D. A. C., Castellano, O., Millian-Morell, L., Rubio, M. E., López, D. E., et al. (2014). Origin and function of short-latency inputs to the neural substrates underlying the acoustic startle reflex. Front. Neurosci. 8:216. doi: 10.3389/fnins.2014.00216
Gordon, A., Salomon, D., Barak, N., Pen, Y., Tsoory, M., Kimchi, T., et al. (2016). Expression of Cntnap2 (Caspr2) in multiple levels of sensory systems. Mol. Cell. Neurosci. 70, 42–53. doi: 10.1016/j.mcn.2015.11.012
Groves, P. M., and Thompson, R. F. (1970). Habituation: a dual-process theory. Psychol. Rev. 77, 419–450. doi: 10.1037/h0029810
Gu, H., Hou, F., Liu, L., Luo, X., Nkomola, P. D., Xie, X., et al. (2018). Genetic variants in the Cntnap2 gene are associated with gender differences among dyslexic children in China. EBioMedicine 34, 165–170. doi: 10.1016/j.ebiom.2018.07.007
Halberstadt, A. L., and Geyer, M. A. (2009). Habituation and sensitization of acoustic startle: opposite influences of dopamine D1 and D2-family receptors. Neurobiol. Learn Mem. 92, 243–248. doi: 10.1016/j.nlm.2008.05.015
Harada, M., Taki, M. M., Nose, A., Kubo, H., Mori, K., Nishitani, H., et al. (2011). Non-invasive evaluation of the GABAergic/glutamatergic system in autistic patients observed by MEGA-editing proton MR spectroscopy using a clinical 3 Tesla instrument. J. Autism Dev. Disord. 41, 447–454. doi: 10.1007/s10803-010-1065-0
Harris, K. C., and Dubno, J. R. (2017). Age-related deficits in auditory temporal processing: unique contributions of neural dyssynchrony and slowed neuronal processing. Neurobiol. Aging 53, 150–158. doi: 10.1016/j.neurobiolaging.2017.01.008
Henderson, C., Wijetunge, L., Kinoshita, M. N., Shumway, M., Hammond, R. S., Postma, F. R., et al. (2012). Reversal of disease-related pathologies in the fragile X mouse model by selective activation of GABAB receptors with Arbaclofen. Sci. Transl. Med. 4:152ra128. doi: 10.1126/scitranslmed.3004218
Hince, D. A., and Martin-Iverson, M. T. (2005). Differences in prepulse inhibition (PPI) between wistar and sprague-dawley rats clarified by a new method of PPI standardization. Behav. Neurosci. 119, 66–77. doi: 10.1037/0735-7044.119.1.66
Hoffman, H. S., and Ison, J. R. (1980). Reflex modification in the domain of startle: I. Some empirical findings and their implications for how the nervous system processes sensory input. Psychol. Rev. 87, 175–189.
Hoffman, H. S., and Searle, J. L. (1968). Acoustic and temporal factors in the evocation of startle. J. Acoust. Soc. Am. 43, 269–282. doi: 10.1121/1.1910776
Horder, J., Andersson, M., Mendez, M. A., Singh, N., Tangen, Ä., Lundberg, J., et al. (2018a). GABAA receptor availability is not altered in adults with autism spectrum disorder or in mouse models. Sci. Transl. Med. 10:eaam8434. doi: 10.1126/scitranslmed.aam8434
Horder, J., Lavender, T., Mendez, M., O’gorman, R., Daly, E., Craig, M., et al. (2013). Reduced subcortical glutamate/glutamine in adults with autism spectrum disorders: a [1 H] MRS study. Transl. Psychiatry 3:e279. doi: 10.1038/tp.2013.53
Horder, J., Petrinovic, M. M., Mendez, M. A., Bruns, A., Takumi, T., Spooren, W., et al. (2018b). Glutamate and GABA in autism spectrum disorder-a translational magnetic resonance spectroscopy study in man and rodent models. Transl. Psychiatry 8:106. doi: 10.1038/s41398-018-0155-1
Iossifov, I., O’Roak, B. J., Sanders, S. J., Ronemus, M., Krumm, N., Levy, D., et al. (2014). The contribution of de novo coding mutations to autism spectrum disorder. Nature 515:216. doi: 10.1038/nature13908
Ison, J. R., McAdam, D. W., and Hammond, G. R. (1973). Latency and amplitude changes in the acoustic startle reflex of the rat produced by variation in auditory prestimulation. Physiol. Behav. 10, 1035–1039. doi: 10.1016/0031-9384(73)90185-6
Kazdoba, T. M., Leach, P. T., and Crawley, J. N. (2016). Behavioral phenotypes of genetic mouse models of autism. Genes Brain Behav. 15, 7–26. doi: 10.1111/gbb.12256
Khalfa, S., Bruneau, N., Rogé, B., Georgieff, N., Veuillet, E., Adrien, J. L., et al. (2004). Increased perception of loudness in autism. Hear. Res. 198, 87–92. doi: 10.1016/j.heares.2004.07.006
Kim, J.-W., Park, K., Kang, R. J., Gonzales, E. L. T., Kim, D. G., Oh, H. A., et al. (2019). Pharmacological modulation of AMPA receptor rescues social impairments in animal models of autism. Neuropsychopharmacology 44, 314–323. doi: 10.1038/s41386-018-0098-5
Koch, M. (1999). The neurobiology of startle. Prog. Neurobiol. 59, 107–128. doi: 10.1016/s0301-0082(98)00098-7
Koch, M., Fendt, M., and Kretschmer, B. D. (2000). Role of the substantia nigra pars reticulata in sensorimotor gating, measured by prepulse inhibition of startle in rats. Behav. Brain Res. 117, 153–162. doi: 10.1016/s0166-4328(00)00299-0
Kohl, S., Wolters, C., Gruendler, T. O. J., Vogeley, K., Klosterkötter, J., Kuhn, J., et al. (2014). Prepulse inhibition of the acoustic startle reflex in high functioning autism. PLoS One 9:e92372. doi: 10.1371/journal.pone.0092372
Krase, W., Koch, M., and Schnitzler, H.-U. (1994). Substance P is involved in the sensitization of the acoustic startle response by footshocks in rats. Behav. Brain Res. 63, 81–88. doi: 10.1016/0166-4328(94)90053-1
Kulesza, R. J. Jr., Lukose, R., and Stevens, L. V. (2011). Malformation of the human superior olive in autistic spectrum disorders. Brain Res. 1367, 360–371. doi: 10.1016/j.brainres.2010.10.015
Kumru, H., and Kofler, M. (2012). Effect of spinal cord injury and of intrathecal baclofen on brainstem reflexes. Clin. Neurophysiol. 123, 45–53. doi: 10.1016/j.clinph.2011.06.036
Kumru, H., Kofler, M., Valls-Solé, J., Portell, E., and Vidal, J. (2009). Brainstem reflexes are enhanced following severe spinal cord injury and reduced by continuous intrathecal baclofen. Neurorehabil. Neural Repair 23, 921–927. doi: 10.1177/1545968309335979
Kwon, S., Kim, J., Choe, B. H., Ko, C., and Park, S. (2007). Electrophysiologic assessment of central auditory processing by auditory brainstem responses in children with autism spectrum disorders. J. Korean Med. Sci. 22, 656–659. doi: 10.3346/jkms.2007.22.4.656
Lauer, A. M., Behrens, D., and Klump, G. (2017). Acoustic startle modification as a tool for evaluating auditory function of the mouse: Progress, pitfalls and potential. Neurosci. Biobehav. Rev. 77, 194–208. doi: 10.1016/j.neubiorev.2017.03.009
Leaton, R. N., and Supple, W. F. Jr. (1986). Cerebellar vermis: essential for long-term habituation of the acoustic startle response. Science 232, 513–515. doi: 10.1126/science.3961494
Lee, Y., López, D. E., Meloni, E. G., and Davis, M. (1996). A primary acoustic startle pathway: obligatory role of cochlear root neurons and the nucleus reticularis pontis caudalis. J. Neurosci. 16, 3775–3789. doi: 10.1523/JNEUROSCI.16-11-03775.1996
Leekam, S. R., Nieto, C., Libby, S. J., Wing, L., and Gould, J. (2007). Describing the sensory abnormalities of children and adults with autism. J. Autism Dev. Disord. 37, 894–910. doi: 10.1007/s10803-006-0218-7
Lorrai, I., Maccioni, P., Gessa, G. L., and Colombo, G. (2016). R(+)-Baclofen, but not S(−)-Baclofen, alters alcohol self-administration in alcohol-preferring rats. Front. Psychiatry 7:68. doi: 10.3389/fpsyt.2016.00068
Lu, J., Lobarinas, E., Deng, A., Goodey, R., Stolzberg, D., Salvi, R. J., et al. (2011). GABAergic neural activity involved in salicylate-induced auditory cortex gain enhancement. Neuroscience 189, 187–198. doi: 10.1016/j.neuroscience.2011.04.073
Lyall, A., Swanson, J., Liu, C., Blumenthal, T. D., and Turner, C. P. (2009). Neonatal exposure to MK801 promotes prepulse-induced delay in startle response time in adult rats. Exp. Brain Res. 197, 215–222. doi: 10.1007/s00221-009-1906-2
Madsen, G. F., Bilenberg, N., Cantio, C., and Oranje, B. (2014). Increased prepulse inhibition and sensitization of the startle reflex in autistic children. Autism Res. 7, 94–103. doi: 10.1002/aur.1337
Martin, M. R. (1982). Baclofen and the brain stem auditory evoked potential. Exp. Neurol. 76, 675–680. doi: 10.1016/0014-4886(82)90135-2
Martin-Iverson, M. T., and Stevenson, K. N. (2005). Apomorphine effects on emotional modulation of the startle reflex in rats. Psychopharmacology (Berl) 181, 60–70. doi: 10.1007/s00213-005-2217-3
Meincke, U., Light, G. A., Geyer, M. A., Braff, D. L., and Gouzoulis-Mayfrank, E. (2004). Sensitization and habituation of the acoustic startle reflex in patients with schizophrenia. Psychiatry Res. 126, 51–61. doi: 10.1016/j.psychres.2004.01.003
Meloni, E. G., and Davis, M. (1998). The dorsal cochlear nucleus contributes to a high intensity component of the acoustic startle reflex in rats. Hear. Res. 119, 69–80. doi: 10.1016/s0378-5955(98)00040-9
Miron, O., Ari-Even Roth, D., Gabis, L. V., Henkin, Y., Shefer, S., Dinstein, I., et al. (2016). Prolonged auditory brainstem responses in infants with autism. Autism Res. 9, 689–695. doi: 10.1002/aur.1561
Mody, M., and Belliveau, J. W. (2013). Speech and language impairments in autism: insights from behavior and neuroimaging. N. Am. J. Med. Sci. (Boston) 5, 157–161. doi: 10.7156/v5i3p157
Möhrle, D., Fernández, M., Peñagarikano, O., Frick, A., Allman, B., Schmid, S., et al. (2020). What we can learn from a genetic rodent model about autism. Neurosci. Biobehav. Rev. 109, 29–53. doi: 10.1016/j.neubiorev.2019.12.015
Nevins, M. E., Nash, S. A., and Beardsley, P. M. (1993). Quantitative grip strength assessment as a means of evaluating muscle relaxation in mice. Psychopharmacology 110, 92–96. doi: 10.1007/BF02246955
Nottebohm, F., and Arnold, A. P. (1976). Sexual dimorphism in vocal control areas of the songbird brain. Science 194, 211–213. doi: 10.1126/science.959852
Oberman, L. M. (2012). mGluR antagonists and GABA agonists as novel pharmacological agents for the treatment of autism spectrum disorders. Expert Opin. Investig. Drugs 21, 1819–1825. doi: 10.1517/13543784.2012.729819
O’Neill, M., and Jones, R. S. (1997). Sensory-perceptual abnormalities in autism: a case for more research? J. Autism Dev. Disord. 27, 283–293. doi: 10.1023/a:1025850431170
Ornitz, E. M., Lane, S. J., Sugiyama, T., and de Traversay, J. (1993). Startle modulation studies in autism. J. Autism Dev. Disord. 23, 619–637. doi: 10.1007/BF01046105
Osen, K., López, D., Slyngstad, T., Ottersen, O., and Storm-Mathisen, J. (1991). GABA-like and glycine-like immunoreactivities of the cochlear root nucleus in rat. J. Neurocytol. 20, 17–25. doi: 10.1007/BF01187131
Panaitof, S. C., Abrahams, B. S., Dong, H., Geschwind, D. H., and White, S. A. (2010). Language-related Cntnap2 gene is differentially expressed in sexually dimorphic song nuclei essential for vocal learning in songbirds. J. Comp. Neurol. 518, 1995–2018. doi: 10.1002/cne.22318
Payne, R., and Anderson, D. C. (1967). Scopolamine-produced changes in activity and in the startle response: implications for behavioral activation. Psychopharmacologia 12, 83–90. doi: 10.1007/BF00402758
Peñagarikano, O., Abrahams, B. S., Herman, E. I., Winden, K. D., Gdalyahu, A., Dong, H., et al. (2011). Absence of Cntnap2 leads to epilepsy, neuronal migration abnormalities and core autism-related deficits. Cell 147, 235–246. doi: 10.1016/j.cell.2011.08.040
Perry, W., Minassian, A., Lopez, B., Maron, L., and Lincoln, A. (2007). Sensorimotor gating deficits in adults with autism. Biol. Psychiatry 61, 482–486. doi: 10.1016/j.biopsych.2005.09.025
Pilz, P. K. D., and Schnitzler, H.-U. (1996). Habituation and sensitization of the acoustic startle response in rats: Amplitude, threshold and latency measures. Neurobiol. Learn. Mem. 66, 67–79. doi: 10.1006/nlme.1996.0044
Pinto, D., Delaby, E., Merico, D., Barbosa, M., Merikangas, A., Klei, L., et al. (2014). Convergence of genes and cellular pathways dysregulated in autism spectrum disorders. Am. J. Hum. Genet. 94, 677–694. doi: 10.1016/j.ajhg.2014.03.018
Plappert, C. F., Pilz, P. K., Becker, K., Becker, C. M., and Schnitzler, H. U. (2001). Increased sensitization of acoustic startle response in spasmodic mice with a mutation of the glycine receptor alpha1-subunit gene. Behav. Brain Res. 121, 57–67. doi: 10.1016/s0166-4328(00)00385-5
Poliak, S., Salomon, D., Elhanany, H., Sabanay, H., Kiernan, B., Pevny, L., et al. (2003). Juxtaparanodal clustering of Shaker-like K+ channels in myelinated axons depends on Caspr2 and TAG-1. J. Cell. Biol. 162, 1149–1160. doi: 10.1083/jcb.200305018
Poot, M. (2017). Intragenic Cntnap2 deletions: a bridge too far? Mol. Syndromol. 8, 118–130. doi: 10.1159/000456021
Port, R. G., Oberman, L. M., and Roberts, T. P. (2019). Revisiting the excitation/inhibition imbalance hypothesis of ASD through a clinical lens. Br. J. Radiol. 92:20180944. doi: 10.1259/bjr.20180944
Price, C. J., Scott, R., Rusakov, D. A., and Capogna, M. (2008). GABAB receptor modulation of feedforward inhibition through hippocampal neurogliaform cells. J. Neurosci. 28, 6974–6982. doi: 10.1523/JNEUROSCI.4673-07.2008
Rankin, C. H., Abrams, T., Barry, R. J., Bhatnagar, S., Clayton, D. F., Colombo, J., et al. (2009). Habituation revisited: an updated and revised description of the behavioral characteristics of habituation. Neurobiol. Learn. Mem. 92, 135–138. doi: 10.1016/j.nlm.2008.09.012
Riva, V., Cantiani, C., Benasich, A. A., Molteni, M., Piazza, C., Giorda, R., et al. (2018). From Cntnap2 to early expressive language in infancy: The mediation role of rapid auditory processing. Cereb. Cortex 28, 2100–2108. doi: 10.1093/cercor/bhx115
Rodenas-Cuadrado, P., Pietrafusa, N., Francavilla, T., La Neve, A., Striano, P., Vernes, S.C., et al. (2016). Characterisation of CASPR2 deficiency disorder—a syndrome involving autism, epilepsy and language impairment. BMC Med. Genet. 17:8. doi: 10.1186/s12881-016-0272-8
Rosenhall, U., Nordin, V., Brantberg, K., and Gillberg, C. (2003). Autism and auditory brain stem responses. Ear Hear. 24, 206–214. doi: 10.1097/01.AUD.0000069326.11466.7E
Rubenstein, J. L., and Merzenich, M. M. (2003). Model of autism: increased ratio of excitation/inhibition in key neural systems. Genes Brain Behav. 2, 255–267. doi: 10.1034/j.1601-183x.2003.00037.x
Rylaarsdam, L., and Guemez-Gamboa, A. (2019). Genetic causes and modifiers of autism spectrum disorder. Front. Cell. Neurosci. 13:385. doi: 10.3389/fncel.2019.00385
Scott, K. E., Kazazian, K., Mann, R. S., Möhrle, D., Schormans, A. L., Schmid, S., et al. (2020). Loss of Cntnap2 in the rat causes autism-related alterations in social interactions, stereotypic behavior and sensory processing. Autism Res. 13, 1698–1717. doi: 10.1002/aur.2364
Scott, K. E., Schormans, A. L., Pacoli, K. Y., De Oliveira, C., Allman, B. L., Schmid, S., et al. (2018). Altered auditory processing, filtering and reactivity in the Cntnap2 knock-out rat model for neurodevelopmental disorders. J. Neurosci. 38, 8588–8604. doi: 10.1523/JNEUROSCI.0759-18.2018
Scott, K. E., Schulz, S. E., Moehrle, D., Allman, B. L., Oram Cardy, J. E., Stevenson, R. A., et al. (2021). Closing the species gap: translational approaches to studying sensory processing differences relevant for autism spectrum disorder. Autism Res. 14, 1322–1331. doi: 10.1002/aur.2533
Scott, R., Sánchez-Aguilera, A., van Elst, K., Lim, L., Dehorter, N., Bae, S. E., et al. (2017). Loss of Cntnap2 causes axonal excitability deficits, developmental delay in cortical myelination and abnormal stereotyped motor behavior. Cereb. Cortex 29, 586–597. doi: 10.1093/cercor/bhx341
Servadio, M., Vanderschuren, L. J., and Trezza, V. (2015). Modeling autism-relevant behavioral phenotypes in rats and mice: Do ’autistic’ rodents exist? Behav. Pharmacol. 26, 522–540. doi: 10.1097/FBP.0000000000000163
Sgadò, P., Genovesi, S., Kalinovsky, A., Zunino, G., Macchi, F., Allegra, M., et al. (2013). Loss of GABAergic neurons in the hippocampus and cerebral cortex of Engrailed-2 null mutant mice: implications for autism spectrum disorders. Exp. Neurol. 247, 496–505. doi: 10.1016/j.expneurol.2013.01.021
Silverman, J. L., Pride, M. C., Hayes, J. E., Puhger, K. R., Butler-Struben, H. M., Baker, S., et al. (2015). GABAB receptor agonist R-Baclofen reverses social deficits and reduces repetitive behavior in two mouse models of autism. Neuropsychopharmacology 40, 2228–2239. doi: 10.1038/npp.2015.66
Simons-Weidenmaier, N. S., Weber, M., Plappert, C. F., Pilz, P. K., and Schmid, S. (2006). Synaptic depression and short-term habituation are located in the sensory part of the mammalian startle pathway. BMC Neurosci. 7:38. doi: 10.1186/1471-2202-7-38
Sinclair, D., Featherstone, R., Naschek, M., Nam, J., Du, A., Wright, S., et al. (2017a). GABAB agonist Baclofen normalizes auditory-evoked neural oscillations and behavioral deficits in the Fmr1 knockout mouse model of fragile X syndrome. eNeuro 4:ENEURO.0380–0316.2017. doi: 10.1523/ENEURO.0380-16.2017
Sinclair, D., Oranje, B., Razak, K. A., Siegel, S. J., and Schmid, S. (2017b). Sensory processing in autism spectrum disorders and Fragile X syndrome-From the clinic to animal models. Neurosci. Biobehav. Rev. 76, 235–253. doi: 10.1016/j.neubiorev.2016.05.029
Stevenson, R. A., Baum, S. H., Segers, M., Ferber, S., Barense, M. D., Wallace, M. T., et al. (2017). Multisensory speech perception in autism spectrum disorder: from phoneme to whole-word perception. Autism Res. 10, 1280–1290. doi: 10.1002/aur.1776
Stoddart, C. W., Noonan, J., and Martin-Iverson, M. T. (2008). Stimulus quality affects expression of the acoustic startle response and prepulse inhibition in mice. Behav. Neurosci. 122, 516–526. doi: 10.1037/0735-7044.122.3.516
Stoppel, L. J., Kazdoba, T. M., Schaffler, M. D., Preza, A. R., Heynen, A., Crawley, J. N., et al. (2018). R-Baclofen reverses cognitive deficits and improves social interactions in two lines of 16p11.2 deletion mice. Neuropsychopharmacology 43, 513–524. doi: 10.1038/npp.2017.236
Strauss, K. A., Puffenberger, E. G., Huentelman, M. J., Gottlieb, S., Dobrin, S. E., Parod, J. M., et al. (2006). Recessive symptomatic focal epilepsy and mutant contactin-associated protein-like 2. N. Engl. J. Med. 354, 1370–1377. doi: 10.1056/NEJMoa052773
Sun, H., Ma, C. L., Kelly, J. B., and Wu, S. H. (2006). GABAB receptor-mediated presynaptic inhibition of glutamatergic transmission in the inferior colliculus. Neurosci. Lett. 399, 151–156. doi: 10.1016/j.neulet.2006.01.049
Szczepaniak, W. S., and Møller, A. (1995). Effects of L-Baclofen and D-Baclofen on the auditory system: A study of click-evoked potentials from the inferior colliculus in the rat. Ann. Otol. Rhinol. Laryngol. 104, 399–404. doi: 10.1177/000348949510400511
Szczepaniak, W. S., and Møller, A. R. (1996). Effects of (−)-baclofen, clonazepam and diazepam on tone exposure-induced hyperexcitability of the inferior colliculus in the rat: possible therapeutic implications for pharmacological management of tinnitus and hyperacusis. Hear. Res. 97, 46–53. doi: 10.1016/s0378-5955(96)80006-2
Takahashi, H., Komatsu, S., Nakahachi, T., Ogino, K., and Kamio, Y. (2016). Relationship of the acoustic startle Rresponse and its modulation to emotional and behavioral problems in typical development children and those with autism spectrum disorders. J. Autism Dev. Disord. 46, 534–543. doi: 10.1007/s10803-015-2593-4
Takahashi, K., Nagai, T., Kamei, H., Maeda, K., Matsuya, T., Arai, S., et al. (2007). Neural circuits containing pallidotegmental GABAergic neurons are involved in the prepulse inhibition of the startle reflex in mice. Biol. Psychiatry 62, 148–157. doi: 10.1016/j.biopsych.2006.06.035
Townsend, L. B., and Smith, S. L. (2017). Genotype- and sex-dependent effects of altered Cntnap2 expression on the function of visual cortical areas. J. Neurodev. Disord. 9:2. doi: 10.1186/s11689-016-9182-5
Truong, D. T., Rendall, A. R., Castelluccio, B. C., Eigsti, I. M., and Fitch, R. H. (2015). Auditory processing and morphological anomalies in medial geniculate nucleus of Cntnap2 mutant mice. Behav. Neurosci. 129, 731–743. doi: 10.1037/bne0000096
Van Elst, L. T., Maier, S., Fangmeier, T., Endres, D., Mueller, G., Nickel, K., et al. (2014). Disturbed cingulate glutamate metabolism in adults with high-functioning autism spectrum disorder: evidence in support of the excitatory/inhibitory imbalance hypothesis. Mol. Psychiatry 19, 1314–1325. doi: 10.1038/mp.2014.62
Varea, O., Martin-de-Saavedra, M. D., Kopeikina, K. J., Schürmann, B., Fleming, H. J., Fawcett-Patel, J. M., et al. (2015). Synaptic abnormalities and cytoplasmic glutamate receptor aggregates in contactin associated protein-like 2/Caspr2 knockout neurons. Proc. Natl. Acad. Sci. U S A 112, 6176–6181. doi: 10.1073/pnas.1423205112
Veenstra-VanderWeele, J., Cook, E. H., King, B. H., Zarevics, P., Cherubini, M., Walton-Bowen, K., et al. (2017). Arbaclofen in children and Aadolescents with autism spectrum disorder: a randomized, controlled, phase 2 trial. Neuropsychopharmacology 42, 1390–1398. doi: 10.1038/npp.2016.237
Vogt, D., Cho, K. K. A., Shelton, S. M., Paul, A., Huang, Z. J., Sohal, V. S., et al. (2017). Mouse Cntnap2 and human Cntnap2 ASD alleles cell autonomously regulate PV+ cortical interneurons. Cereb. Cortex 28, 3868–3879. doi: 10.1093/cercor/bhx248
Waagepetersen, H. S., Sonnewald, U., Gegelashvili, G., Larsson, O. M., and Schousboe, A. (2001). Metabolic distinction between vesicular and cytosolic GABA in cultured GABAergic neurons using 13C magnetic resonance spectroscopy. J. Neurosci. Res. 63, 347–355. doi: 10.1002/1097-4547(20010215)63:4<347::AID-JNR1029>3.0.CO;2-G
Wagner, T., and Mack, A. (1998). Membrane properties of giant neurons in the caudal pontine reticular formation in vitro. NeuroReport 9, 1211–1215. doi: 10.1097/00001756-199804200-00046
Waldmeier, P. C., Kaupmann, K., and Urwyler, S. (2008). Roles of GABAB receptor subtypes in presynaptic auto- and heteroreceptor function regulating GABA and glutamate release. J. Neural Transm. (Vienna) 115, 1401–1411. doi: 10.1007/s00702-008-0095-7
Walls, A. B., Waagepetersen, H. S., Bak, L. K., Schousboe, A., and Sonnewald, U. (2015). The glutamine-glutamate/GABA cycle: function, regional differences in glutamate and GABA production and effects of interference with GABA metabolism. Neurochem. Res. 40, 402–409. doi: 10.1007/s11064-014-1473-1
Weber, M., Schnitzler, H. U., and Schmid, S. (2002). Synaptic plasticity in the acoustic startle pathway: the neuronal basis for short-term habituation? Eur. J. Neurosci. 16, 1325–1332. doi: 10.1046/j.1460-9568.2002.02194.x
Werling, D. M., and Geschwind, D. H. (2013). Sex differences in autism spectrum disorders. Curr. Opin. Neurol. 26, 146–153. doi: 10.1097/WCO.0b013e32835ee548
Wible, B., Nicol, T., and Kraus, N. (2004). Atypical brainstem representation of onset and formant structure of speech sounds in children with language-based learning problems. Biol. Psychol. 67, 299–317. doi: 10.1016/j.biopsycho.2004.02.002
Wu, C., and Sun, D. (2015). GABA receptors in brain development, function and injury. Metab. Brain Dis. 30, 367–379. doi: 10.1007/s11011-014-9560-1
Yee, B. K., Chang, D. T., and Feldon, J. (2004). The effects of dizocilpine and phencyclidine on prepulse inhibition of the acoustic startle reflex and on prepulse-elicited reactivity in C57BL6 mice. Neuropsychopharmacology 29, 1865–1877. doi: 10.1038/sj.npp.1300480
Yeomans, J. S., Bosch, D., Alves, N., Daros, A., Ure, R. J., Schmid, S., et al. (2010). GABA receptors and prepulse inhibition of acoustic startle in mice and rats. Eur. J. Neurosci. 31, 2053–2061. doi: 10.1111/j.1460-9568.2010.07236.x
Yip, J., Soghomonian, J. J., and Blatt, G. J. (2008). Increased GAD67 mRNA expression in cerebellar interneurons in autism: implications for Purkinje cell dysfunction. J. Neurosci. Res. 86, 525–530. doi: 10.1002/jnr.21520
Yoo, H. (2015). Genetics of autism spectrum disorder: Current status and possible clinical applications. Exp. Neurobiol. 24, 257–272. doi: 10.5607/en.2015.24.4.257
Zaman, T., De Oliveira, C., Smoka, M., Narla, C., Poulter, M. O., Schmid, S., et al. (2017). BK channels mediate synaptic plasticity underlying habituation in rats. J. Neurosci. 37, 4540–4551. doi: 10.1523/JNEUROSCI.3699-16.2017
Keywords: autism spectrum disorders, sensory processing, startle, GABA, R-Baclofen, CNTNAP2, animal model
Citation: Möhrle D, Wang W, Whitehead SN and Schmid S (2021) GABAB Receptor Agonist R-Baclofen Reverses Altered Auditory Reactivity and Filtering in the Cntnap2 Knock-Out Rat. Front. Integr. Neurosci. 15:710593. doi: 10.3389/fnint.2021.710593
Received: 16 May 2021; Accepted: 27 July 2021;
Published: 20 August 2021.
Edited by:
Randy J. Kulesza, Lake Erie College of Osteopathic Medicine, United StatesReviewed by:
Angel Nunez, Autonomous University of Madrid, SpainJoseph A. McQuail, University of South Carolina, United States
Copyright © 2021 Möhrle, Wang, Whitehead and Schmid. This is an open-access article distributed under the terms of the Creative Commons Attribution License (CC BY). The use, distribution or reproduction in other forums is permitted, provided the original author(s) and the copyright owner(s) are credited and that the original publication in this journal is cited, in accordance with accepted academic practice. No use, distribution or reproduction is permitted which does not comply with these terms.
*Correspondence: Dorit Möhrle, ZG9yaXQubW9laHJsZUBnb29nbGVtYWlsLmNvbQ==