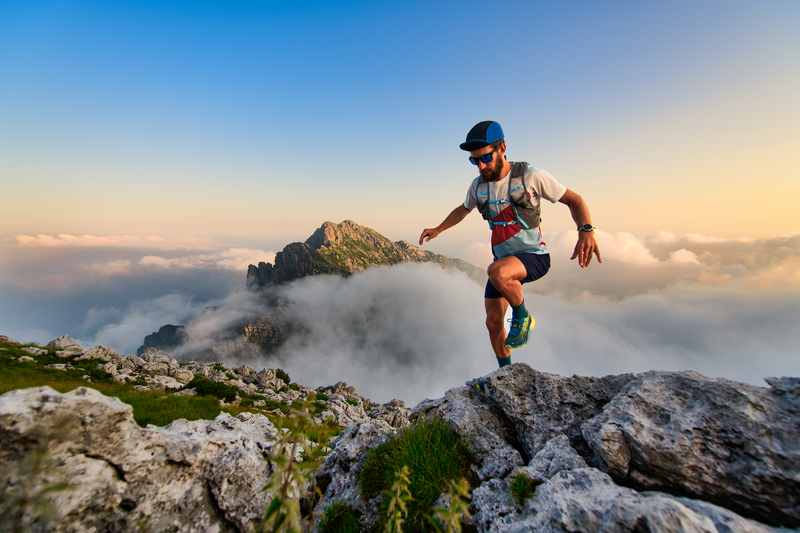
95% of researchers rate our articles as excellent or good
Learn more about the work of our research integrity team to safeguard the quality of each article we publish.
Find out more
REVIEW article
Front. Integr. Neurosci. , 30 October 2019
Volume 13 - 2019 | https://doi.org/10.3389/fnint.2019.00057
This article is part of the Research Topic Biomarkers to Enable Therapeutics Development in Neurodevelopmental Disorders View all 20 articles
Epilepsy is associated with numerous neurodevelopmental disorders. Transcranial magnetic stimulation (TMS) of the motor cortex coupled with electromyography (EMG) enables biomarkers that provide measures of cortical excitation and inhibition that are particularly relevant to epilepsy and related disorders. The motor threshold (MT), cortical silent period (CSP), short interval intracortical inhibition (SICI), intracortical facilitation (ICF), and long interval intracortical inhibition (LICI) are among TMS-derived metrics that are modulated by antiepileptic drugs. TMS may have a practical role in optimization of antiepileptic medication regimens, as studies demonstrate dose-dependent relationships between TMS metrics and acute medication administration. A close association between seizure freedom and normalization of cortical excitability with long-term antiepileptic drug use highlights a plausible utility of TMS in measures of anti-epileptic drug efficacy. Finally, TMS-derived biomarkers distinguish patients with various epilepsies from healthy controls and thus may enable development of disorder-specific biomarkers and therapies both within and outside of the epilepsy realm.
Epilepsy is among the most common neurologic disorders in childhood, and accompanies numerous neurodevelopmental disorders, particularly the autism spectrum disorders (ASDs; Levisohn, 2007; Tuchman and Rapin, 2002; Danielsson et al., 2005). For patient populations with epilepsy, biomarkers that reflect magnitudes of cortical excitation and inhibition are highly desirable as metrics of disease severity and target engagement by therapeutics. Transcranial magnetic stimulation (TMS) is a 30-year-old protocol for focal, noninvasive, electrical cortical stimulation that enables such measures across ages (Barker et al., 1985). In TMS, powerful fluctuating extracranial magnetic fields induce intracranial electrical current. When placed at the scalp, TMS induces electric current in the nearby cerebral cortex and allows for the operator to either measure or modulate focal cortical excitability.
The TMS “dose” per experiment is defined by hardware factors that affect the electromagnetic field. These include coil shape, size, electrical properties, and its placement relative to cortical structures. The stimulation parameter space also includes individual stimulus components such as pulse shape (rectangular, sinusoidal, exponential) and amplitude. The physiologic response to TMS is further determined by stimulus train parameters such as frequency, duration, inter-train interval, and the number of trains per unit time. The electric field generated by TMS is not measured in vivo but can be effectively modeled and represented in volts per meter (V/m). Alternatively, TMS-induced current density can be approximated in ampere per meter2 (A/m2; Peterchev et al., 2012).
Stimulation focality in TMS is in part governed by coil geometry. With a common type of TMS coil termed figure-of-eight, the volume of depolarized cortex with a single stimulus can be as small as 1 cm3. When positioned over the motor cortex, TMS by a figure-of-eight coil enables selective activation of intrinsic hand muscles in the limb contralateral to the stimulated hemisphere, without co-activation of more proximal muscle groups. Such motor cortex activation can be quantified with skin surface electromyography (EMG) that records a per-stimulus motor evoked potential (MEP) which predictably reflects the magnitude of stimulation and is the main outcome measure in TMS studies of the cortical excitation:inhibition (E:I) ratio (Figure 1; reviewed in Kobayashi and Pascual-Leone, 2003; Frye et al., 2008).
Figure 1. Representative transcranial magnetic stimulation (TMS) motor cortex activation. (A) An approximation of stimulating electric field (e-field) induced by a single TMS pulse is displayed on a 3D reconstruction of an individual’s anatomic magnetic resonance imaging (MRI), where field center is indicated by the junction between the red and blue arrows, indicating the direction of induced current, with corresponding e-field strength at the stimulation site (V/m) shown in red in the bottom left. The composite map of left hemispheric stimulation sites evoking motor evoked potentials (MEPs) of the right abductor pollicis brevis (APB) muscle, where intensity of response is color coded (heat map) from lowest (gray) to highest (white), are displayed on the cortical surface rendering. (B) Representative right APB MEP sample (green deflection) showing right APB resultant from left hemisphere stimulation, where the vertical line (white) corresponds to stimulus time. MEP amplitude and latency are indicated on the right.
TMS is unique among brain stimulation protocols in that it has both diagnostic and therapeutic potential. Three TMS protocols, all combined with surface EMG to measure MEP amplitude, are commonly employed to measure the cortical E:I ratio in epilepsy: (1) single-pulse TMS (spTMS); (2) paired-pulse TMS (ppTMS); and (3) repetitive TMS (rTMS). While there is appreciable device-to-device output variability of focality and magnitude of stimulation via TMS, the overall pulse width, and pulse shape (monophasic and biphasic) are relatively consistent across devices. Experimental devices with variable pulse width and shape are emerging, but thus to date are not widely implemented (Peterchev et al., 2011). In the most common embodiment of spTMS, the motor cortex is stimulated while muscle activation in a contralateral limb is monitored by surface EMG. spTMS, when used to determine the resting motor threshold (rMT), guides stimulation intensity in therapeutic rTMS (Figure 2A; Theodore, 2003; Ziemann, 2004).
Figure 2. TMS-derived metrics of motor cortex excitation and inhibition. (A) Resting motor threshold (rMT) for the APB muscle is calculated by identifying the minimum stimulus strength, measured in percent machine output (% MO), that evokes an MEP of a fixed amplitude (typically ≥50 μV) in the APB at rest in a majority of trials. Stimulus strength is indicated in the left panel, with resulting MEPs shown in the right panel, where red arrows indicate the time of stimulation and percent stimulator output is proportionate to the arrow length. (B) ppTMS paradigms where a subthreshold conditioning stimulus (short red vertical line) followed by a supra-threshold test stimulus (longer red vertical line). At short inter-stimulus-intervals (ISIs) (1–5 ms) short interval intracortical inhibition (SICI) is seen with inhibition of the test MEP by the antecedent conditioning stimulus. At longer ISIs (10–20 ms), test MEP amplitude is enhanced relative to the control MEP, such that ICF is seen. (C) Still longer ISIs (50–300 ms) are applied with two suprathreshold stimuli in LICI protocols where the MEP resultant from the test stimulus is predictably lower in amplitude than the preceding MEP resulting from the conditioning stimulus. In (D) the cortical silent period (CSP), interruption of ongoing electromyography (EMG) activity for a voluntarily contracting target muscle, occurs following single-pulse TMS (spTMS).
spTMS coupled with surface EMG is also emerging as an important tool for functional topographic corticospinal tract mapping for purposes of presurgical planning (Lefaucheur and Picht, 2016; Hameed et al., 2017; Hannula and Ilmoniemi, 2017; Kaye et al., 2017b). ppTMS is an experimental technique, also delivered over the motor cortex, used to measure the cortical E:I ratio. In most common ppTMS protocols, two consecutive pulses are delivered to the hand motor region at a fixed inter-stimulus-interval (ISI) such that the MEP resultant from the second (test) stimulus is modulated by an antecedent (conditioning) stimulus. Depending on stimulus intensity and the ISI, ppTMS can reveal the magnitude of regional inhibitory or excitatory signaling strength (Figures 2B–D; Ziemann, 2003; Dhamne et al., 2015; Hsieh et al., 2017; Damar et al., 2018).
rTMS, delivered in trains lasting minutes, is most commonly used to modulate regional cortical excitability to suppress neuropsychiatric symptoms. In the motor cortex, rTMS is commonly administered in high-frequency (>10 Hz) or low-frequency (<1 Hz) protocols aimed to enhance or suppress, respectively the MEP amplitude to provide a metric of cortical plasticity. Notably, such suppression and facilitation varies among individuals (Maeda et al., 2002). The physiologic mechanisms by which rTMS modifies cortical excitability are not completely understood but resemble well-described phenomena of use dependent long-term potentiation (LTP) and long-term depression (LTD) of excitatory synaptic strength modulated by glutamatergic and gamma-aminobutyric acid (GABA)-ergic mediators (Fitzgerald et al., 2006; Pascual-Leone et al., 2011; Pilato et al., 2012; Muller et al., 2014; Yang et al., 2014).
Patterned rTMS protocols, such as theta burst stimulation (TBS) of the motor cortex, are also used to measure cortical plasticity, where the two principal patterns of TBS are continuous theta burst stimulation (cTBS) and intermittent theta burst stimulation (iTBS). Both consist of delivery of 50 Hz pulses in bursts of three with an inter-burst interval of 200 ms, which mimic endogenous theta rhythms. As with other TMS protocols intended to produce biomarkers, TBS relies on changes in MEP amplitude as the main outcome measure. cTBS paradigms involve continuous train of TBS over a given duration, which, in typically developing individuals, result in net MEP suppression or depression of corticospinal excitability in most instances. In iTBS, a 2-s train of TBS is repeatedly delivered every 10 s, —in healthy adults, this often (though not always) leads to MEP facilitation or corticospinal excitation (Jannati et al., 2017). Mechanistically, as with conventional rTMS, TBS protocols likely engage mechanisms of glutamatergic and GABA-ergic synaptic plasticity (Huang et al., 2005; Stagg et al., 2009; Oberman et al., 2011; Mix et al., 2015; Blumberger et al., 2018).
spTMS and ppTMS are well-tolerated by subjects at the extremes of age, with only rare and mild adverse events reported among infant, child and elderly populations (Liepert et al., 2001; Eyre, 2003; Hameed et al., 2017; Kaye et al., 2017a). Several TMS devices are now FDA-cleared for use in children and adults. TMS safety and tolerability in patients with epilepsy is underscored by the growing use of neuronavigated TMS (TMS is coupled with frameless stereotaxy; Figure 1) as a presurgical functional mapping tool in children with developmental delay and/or epilepsy who candidates for respective epilepsy surgery (Narayana et al., 2015; Kaye et al., 2017a,b).
Specifically among children, the subjective perception of TMS seems favorable. Children who undergo TMS generally rate the experience as positive with little adverse events occurring during the sessions. Some children have even reported TMS to be more enjoyable than watching TV or going to the dentist (Garvey et al., 2001). Notably, in patients with epilepsy, per-subject risk for seizure with rTMS, spTMS or ppTMS is higher, yet is less than 3% crude per-subject risk (Schrader et al., 2004; Bae et al., 2007; Rossi et al., 2009; Pereira et al., 2016). The favorable safety profile of TMS has allowed for its use for studying cortical excitability in elderly patients with neurodegenerative disorders (Liepert et al., 2001).
TMS protocols are also available in rats, which underscores the versatility of motor cortex TMS as a protocol that is available in both clinical and preclinical arenas (Rotenberg et al., 2008; Hsieh et al., 2011; Gersner et al., 2015; Tang et al., 2016; Damar et al., 2018; Hameed et al., 2018).
A range of cortical excitability measures that are affected by both epilepsy and antiepileptic drugs (AEDs) can be obtained by TMS coupled with surface EMG. Motor threshold (MT) is often defined as the minimum percentage of stimulator output (% MO) that evokes an MEP of a fixed amplitude (typically >50 μV) in a target muscle either at rest (rMT) or during voluntary contraction (active motor threshold, aMT) in a majority of trials (Theodore, 2003; Ziemann et al., 2015; Figure 2A). The cortical silent period (CSP) is a TMS-induced interruption of activity in the EMG of the voluntarily contracting target muscle. The early segment of the CSP is related to spinal inhibition while the later segment is hypothesized to be of motor cortical origin. Short-interval intracortical inhibition (SICI) results from inhibition of the test MEP by a conditioning stimulus. This ppTMS protocol involves the application of a subthreshold conditioning stimulus and supra-threshold test stimulus at short ISIs (1–5 ms). Stimulation using a similar protocol but with longer ISIs of 10–20 ms results in intracortical facilitation (ICF; Kobayashi and Pascual-Leone, 2003; Ziemann, 2004). Long-interval intracortical inhibition (LICI) is measured using ppTMS with two supra-threshold stimuli applied at long ISIs of 50–300 ms in which the conditioning stimulus inhibits the test MEP. Such TMS-EMG parameters are summarized in Table 1 (Rotenberg, 2018) and illustrated in Figures 2B–D.
rMT reflects the degree of cortical excitability which is affected by voltage-gated sodium channel blockers. Carbamazepine (CBZ), lacosamide (LCM), lamotrigine (LTG), and phenytoin (PHT), increase rMT compared to the rMT in drug-naïve patients with epilepsy and in patients without epilepsy; these changes are reversible with withdrawal of the given medication (Chen et al., 1997; Manganotti et al., 1999; Kimiskidis et al., 2005; Lee et al., 2005; Li et al., 2009; Lang et al., 2013; Ziemann et al., 2015). The effect of levetiracetam (LEV) on rMT remains uncertain, as Sohn et al. (2001) show no change in rMT with LEV administration, while Reis et al. (2004) report a significant increase in rMT in patients taking LEV (Sohn et al., 2001; Reis et al., 2004).
Notably, there is a dose-dependent relationship between rMT and certain AEDs. Lee et al. (2005) measured serial rMT and serum drug levels in healthy volunteers taking gradually increasing dosages of CBZ over 5 weeks followed by an abrupt cessation of the drug. rMT increased with increasing serum drug levels of total and free CBZ (Lee et al., 2005). In 7 of 10 patients, upon abrupt CBZ cessation, rMT remained elevated initially and then gradually returned to the baseline over several days despite the abrupt drop in serum CBZ levels. The sustained increase in rMT despite absent serum CBZ level indicates that the rMT (and perhaps other TMS-derived E:I metrics) may distinguish between drug pharmacodynamics and pharmacokinetics (Lee et al., 2005). As with CBZ, Lang et al. (2013) show a trend towards a dose-responsive effect on rMT with LCM dosages of 200 mg and 400 mg (Lang et al., 2013).
The effect a drug has on rMT can also provide information regarding its antiepileptic mechanism of action. For example, valproate (VPA) has no significant effect on rMT in healthy volunteers. VPA does increase rMT in focal epilepsies while its effect on rMT in patients with idiopathic generalized epilepsy (IGE) remains unclear as there are contradictory findings among studies (Reutens et al., 1993; Kazis et al., 2006; Li et al., 2009; Zunhammer et al., 2011; Badawy et al., 2014). Topiramate (TPM), like VPA has several mechanisms of action, including voltage gated sodium channel antagonism, but does not affect rMT while reducing ICF as its anti-epileptic properties stem primarily from inhibition of ligand-gated AMPA subtype glutamate receptors and agonist effects on some subtypes of the GABAA receptor (Angehagen et al., 2005). If VPA and TPM do not modulate the rMT, then while in vitro they may indeed have sodium channel blocking properties, these are not prominent in vivo, or in humans—thus the antiepileptic efficacy of these AEDs is less likely due to the sodium channel properties.
Antiepileptic medications also affect CSP duration, SICI, and LICI—all measures of components of GABA-ergic inhibition. CSP duration and SICI reflect motor cortical postsynaptic inhibition. ICF reflects glutamate receptor-mediated excitability that counters the inhibitory circuits reflected in SICI. GABAA receptor positive allosteric modulators such as benzodiazepines prolong short CSPs when low-intensity stimulation is used and shorten long CSPs when high-intensity stimulation is used. SICI is thought to represent fast inhibitory postsynaptic potentials (IPSPs) in corticospinal neurons mediated by α2- or α3-GABAA receptors. SICI is predictably enhanced by benzodiazepines and barbiturates. LICI reflects slow IPSPs mediated in part by GABAB receptors. LICI, where the long interval between pulses enables signals to propagate across multiple local and distal networks also likely reflects an aggregate inhibitory tone that is mediated by the GABAA receptor system (Hsieh et al., 2011). As expected, vigabatrin increases LICI, while there are conflicting reports on lorazepam’s effect on LICI (Ziemann et al., 1996; Teo et al., 2009). In animal models, LICI is also enhanced by pentobarbital and suppressed by the GABAA receptor blocker pentylenetetrazole (PTZ; Hsieh et al., 2011).
Notably, however, tiagabine has a more complex interaction between the GABAA and GABAB receptor subtypes. Increased extracellular GABA availability in this instance results in predictable CSP prolongation and increased LICI. However, tiagabine decreases SICI which is controlled by presynaptic GABAB receptor-mediated autoinhibition of inhibitory interneurons. This contributes to the net increase in excitatory response as illustrated by the increase in ICF with tiagabine administration.
N-methyl-D-aspartate (NMDA)-receptor antagonists such as dextrorphan, the active metabolite of the prodrug dextromethorphan, and memantine, and benzodiazepines such as diazepam decrease ICF while enhancing SICI (Schwenkreis et al., 1999). Table 2 summarizes the effects of various classes of drugs on these variables.
While changes in TMS parameters following acute drug administration aid in the identification of mechanisms of action of various drugs (or identify the mechanism of TMS-derived phenomena) at the receptor level, the effect of long-term administration of antiepileptic medications on these parameters may serve as a proxy for prognosticating efficacy of antiepileptic medications. A longitudinal study with 1-year follow-up illustrated a reduction in cortical excitability in patients with IGE or focal epilepsy who became seizure-free with anti-seizure medications (Badawy et al., 2010). In fact, while the rMTs were overall higher in these patients than in the control subjects without epilepsy, only the subset of patients with epilepsy who became seizure-free demonstrated an increase in rMT. These findings were independent of seizure type, seizure frequency, patient current age or age at seizure onset, and serum levels of the medication.
A subsequent study with a 3-year follow-up period compared measures of cortical inhibition and facilitation in patients with IGE or focal epilepsy, between those who remained refractory to antiepileptic drugs and those who achieved seizure freedom (Badawy et al., 2013). The mean rMT was higher in the affected hemisphere in patients with focal epilepsy compared to the unaffected hemisphere prior to initiation of anti-seizure medications. There was no difference in pre-drug treatment rMT between control patients without epilepsy and patients with IGE. Patients whose focal seizures remained refractory following initiation of one AED had an increase in rMT in the contralateral (unaffected) hemisphere such that there was no difference between the rMT in the two hemispheres. In patients who achieved seizure freedom on monotherapy, mean rMTs were higher in bilateral hemispheres in patients with IGE and patients with focal epilepsy compared to those patients who remained refractory. This pattern was maintained by the 30–36 months follow-up time-frame. Patients with refractory focal epilepsy developed a hyperexcitable contralateral hemisphere (at ISIs of 2 and 5 ms) at 30–36 months. A similar hyperexcitable response was also seen during a time of continued seizures in patients who would become seizure-free after the second medication. When those patients became seizure-free, however, there was subsequent normalization of all ISIs by the 30–36 months time frame. For the seizure-free patients in this cohort, rMT was higher than that measured in non-epilepsy controls, and SICI and LICI gradually increased to normal or near normal-values at most ISIs (Badawy et al., 2013).
These results suggest a close association between seizure freedom and normalization of TMS-derived cortical excitability metrics with prolonged AED use in patients with both focal and generalized epilepsy. Whether this effect is due to a change within the brain’s predisposition to generate seizures or attributable to the cessation of continued seizure activity is unknown. However, regardless of the drug(s) used, a common effect of successful AED treatment is the restoration of normal responses to TMS.
As SICI reflects the activity of intracortical inhibitory circuits, particularly that of GABAA receptor-mediated activity (Ziemann, 2004), serial SICI measurements over a given time course provide an index for GABA-mediated motor cortex inhibition (Maeda et al., 2002). Cantello et al. (2007) tested a range of TMS-derived metrics in healthy volunteers placed on the ketogenic diet (KD), to find that short-term KD (14-days) was followed by significant SICI enhancement. Notably, rMT was unchanged after KD initiation suggesting a prominent GABAA receptor contribution to the KD antiepileptic mechanism of action (Cantello et al., 2007).
Di Lazzaro et al. (2004) compared baseline TMS measures (rMT, SICI) for five patients with medically-refractory epilepsy who underwent vagus nerve stimulator (VNS) implantation. TMS measures were obtained in the stimulator-off and stimulator-on conditions. Patient rMT was higher than healthy age-matched controls but did not change with the VNS on. In contrast, SICI significantly increased when the VNS was on. As with KD, these results indicate a TMS-derived marker of target engagement, and a capacity for TMS-EMG to identify a GABAergic contributor to an antiepileptic intervention’s mechanism (Di Lazzaro et al., 2004).
Changes in cortical excitability detected by ppTMS can be used in both preclinical and clinical studies to develop and assess the efficacy of novel AEDs. Huperzine A (HupA), a traditional Chinese medicine administered for treatment of epilepsy, is a naturally occurring esquiterpene alkaloid compound found in the firmoss Huperzia serrata that is both an acetylcholinesterase inhibitor and N-methyl-D-aspartate receptor (NMDAR) antagonist. Preclinical trials show that HupA suppresses seizures in a range of rodent epilepsy models. By ppTMS and differential pharmacology, Gersner et al. (2015) identified a potent GABAergic effect of HupA that was reflected in preserved paired-pulse inhibition of the MEP when rats were co-administered HupA and PTZ (a convulsant and GABA-A receptor blocker), and augmented LICI when HupA was administered in isolation (Gersner et al., 2015). The group concluded that at least in part the anti-seizure HupA effects may result from the enhancement of cortical GABAergic tone. These initial preclinical results justify continued preclinical and clinical investigations of HupA as a potential new anti-seizure drug compound.
Retigabine (RTG) is a newer generation drug that acts as a positive allosteric opener of KCNQ2–5 potassium channels to increase potassium efflux resulting in neuronal hyperpolarization and a decrease in neuronal excitability. In a cross-over, double-blind, placebo-controlled, randomized control trial, Ossemann et al. (2016) used single-pulse TMS with a figure-of-eight coil to measure rMT and aMT, and intensity to obtain a 1 mV peak-to-peak amplitude potential (SI1mV), and ppTMS to measure SICI, LICI, and ICF (Ossemann et al., 2016). Baseline measurements and measurements 2 h following administration of an oral dose of 400 mg RTG or placebo were obtained. RTG increased rMT, aMT, and S1mV compared to placebo, suggesting that RTG decreases neuronal excitability by increasing the resting potential as hypothesized from in vitro studies. However, SICI/ICF, and LICI were not significantly different between the RTG and placebo groups, suggesting that RTG does not affect intracortical inhibition (Ossemann et al., 2016).
XEN1101 is a voltage-gated potassium channel opener in the early stages of development that has shown promising preliminary data as a new antiseizure drug through the use of TMS. In a Phase 1 open-label study, spTMS was used to measure rMT in healthy control subjects taking 10 mg, 15 mg, or 20 mg of XEN1101. Premoli et al. (2019) found that 20 mg of XEN1101 decreased cortical excitability compared to the lower dosages (Premoli et al., 2019). In a subsequent double-blind, randomized, two-period crossover study, XEN1101 elevated rMT in a plasma concentration-dependent fashion. These encouraging findings support that XEN1101 reduces corticospinal and cortical excitability in a plasma concentration-dependent manner and have prompted plans for Phase 2 clinical trials.
As expected, alterations in cortical inhibitory networks are also seen in various genetic and metabolic epilepsies (Table 3). SICI is decreased in patients with progressive myoclonic epilepsies (PME), including Unverricht-Lundborg disease (ULD), Lafora body disease (LBD), progressive myoclonic ataxia, sialidosis, and myoclonic epilepsy with ragged red fibers (MERRF). In patients with noncortical myoclonus, such as those with DYT-1 myoclonus-dystonia syndrome, SICI can be normal or mildly impaired. These findings help elucidate the pathophysiology of these diseases. For example, mutations in laforin or malin lead to formation and accumulation of neuronatin aggregates, typically found in parvalbumin-positive inhibitory interneurons (PVINs), resulting in significant reduction in and degeneration of PVINs on brain biopsy of patients with LBD. This reduction in cortical inhibition is reflected by the decreased SICI, and simultaneously illustrates the role of cortical PVINs on SICI (Rotenberg, 2018).
SICI is also reduced in SCN1A-related epilepsies such as Dravet syndrome (DS), which again reflects abnormal cortical inhibition networks, while the other TMS-derived markers of cortical excitability remain normal (Stern et al., 2017). These findings are consistent with preclinical data showing PVIN and somatostain-positive inhibitory interneuron dysfunction in murine DS models (Tai et al., 2014).
LICI abnormalities can also indicate cortical inhibitory network dysfunction, but, unlike SICI, reflect GABAB receptor activity. In patients with succinic semialdehyde dehydrogenase (SSADH) deficiency, LICI is reduced and CSP is shortened while SICI is preserved. These findings are supported by preclinical data showing GABAB receptor loss and/or dysfunction in a murine SSADH deficiency model (Rotenberg, 2018).
Additionally, rMT is increased in young patients with SSADH deficiency compared to their parents who are heterozygous for the causal pathogenic variant. However, this may be related to the age-dependent changes in rMT seen in healthy children and in patients with epilepsy (Hameed et al., 2017; Säisänen et al., 2018). Increase in rMT can also be found in several forms of cortical myoclonus, such as PME. In contrast to patients with chronic refractory IGE or those with chronic refractory FE, interictal cortical excitability is decreased in Lennox–Gastaut syndrome (LGS), where cortical excitability was lower in LGS patients. Cortical excitability was also lower in LGS when compared with healthy controls. This low cortical excitability across TMS measures thus distinguishes LGS from other medically refractory epilepsy syndromes (often showing measures of increased cortical excitability; Badawy et al., 2012).
Noninvasive stimulation of the motor cortex with TMS has practical and easily attainable implications for identification of biomarkers in epilepsy. TMS-derived metrics of E:I properties resultant from motor cortex stimulation paradigms elucidate mechanisms of action, pharmacodynamics, and pharmacokinetics of AEDs, and speak to the underlying pathophysiology of a range of epilepsy disorders. A range of established protocols and metrics are available in numerous laboratories, and can not only be deployed to measure disease severity, predict and measure response to existing treatments in epilepsy, but also aid in the identification and development of novel areas for target engagement in the treatment of an array of disorders.
All authors wrote and revised the manuscript, approved the final version, and agreed to be accountable for the content of the work.
AR is supported by the Boston Children’s Hospital Translational Research Program, National Institutes of Health (NIH, NINDS NS088583), Massachusetts Life Sciences, The Assimon Family, and the Sestito Family. The content is solely the responsibility of the authors and does not necessarily represent the official views of Harvard University and its affiliated academic health care centers, National Institutes of Health, or any of the other listed granting agencies.
TMS, transcranial magnetic stimulation; V/m, volts per meter; A/m2, ampere per meter2; T, tesla; spTMS, single-pulse TMS; ppTMS, paired-pulse TMS; rTMS, repetitive TMS; EMG, electromyography; rMT, resting motor threshold; E:I, excitation to inhibition ratio; APB, abductor pollicis brevis; ISI, inter-stimulus-interval; LTP, long-term potentiation; LTD, long-term depression; TBS, theta burst stimulation; cTBS, continuous theta burst stimulation; iTBS, intermittent theta burst stimulation; MEP, motor evoked potential; CS, corticospinal; AEDs, antiepileptic drugs; MT, motor threshold; % MO, percent machine output; aMT, active motor threshold; CSP, cortical silent period; LICI, long-interval intracortical inhibition; SICI, short-interval intracortical inhibition; CBZ, carbamazepine; LCM, lacosamide; LTG, lamotrigine; PHT, phenytoin; LEV, levetiracetam; VPA, valproate; IGE, idiopathic generalized epilepsy; TPM, topiramate; AMPA, alpha-amino-3-hydroxy-5-methy;-4-isoxazole propionic acid; KD, ketogenic diet; VNS, vagus nerve stimulator; HupA, Huperzine A; NMDAR, N-methyl-D-aspartate receptor; PTZ, pentylenetetrazole; RTG, Retigabine; PME, progressive myoclonic epilepsies; ULD, including Unverricht-Lundborg disease; LBD, Lafora body disease; MERRF, myoclonic epilepsy with ragged red fibers; PVINs, parvalbumin-positive inhibitory interneurons; DS, Dravet syndrome; (SSADH) deficiency, succinic semialdehyde dehydrogenase.
The authors declare that the research was conducted in the absence of any commercial or financial relationships that could be construed as a potential conflict of interest.
Angehagen, M., Rönnbäck, L., Hansson, E., and Ben-Menachem, E. (2005). Topiramate reduces AMPA-induced Ca2+ transients and inhibits GluR1 subunit phosphorylation in astrocytes from primary cultures. J. Neurochem. 94, 1124–1130. doi: 10.1111/j.1471-4159.2005.03259.x
Badawy, R. A. B., Jackson, G. D., Berkovic, S. F., and MacDonell, R. A. L. (2013). Cortical excitability and refractory epilepsy: a three-year longitudinal transcranial magnetic stimulation study. Int. J. Neural Syst. 23:1250030. doi: 10.1142/s012906571250030x
Badawy, R. A. B., MacDonell, R. A. L., Berkovic, S. F., Newton, M. R., and Jackson, G. D. (2010). Predicting seizure control: cortical excitability and antiepileptic medication. Ann. Neurol. 67, 64–73. doi: 10.1002/ana.21806
Badawy, R. A. B., MacDonell, R. A. L., Vogrin, S. J., Lai, A., and Cook, M. J. (2012). Cortical excitability decreases in Lennox-Gastaut syndrome. Epilepsia 53, 1546–1553. doi: 10.1111/j.1528-1167.2012.03599.x
Badawy, R. A. B., Strigaro, G., and Cantello, R. (2014). TMS, cortical excitability and epilepsy: the clinical impact. Epilepsy Res. 108, 153–161. doi: 10.1016/j.eplepsyres.2013.11.014
Bae, E. H., Schrader, L. M., Machii, K., Alonso-Alonso, M., Riviello, J. J. Jr., Pascual-Leone, A., et al. (2007). Safety and tolerability of repetitive transcranial magnetic stimulation in patients with epilepsy: a review of the literature. Epilepsy Behav. 10, 521–528. doi: 10.1016/j.yebeh.2007.03.004
Barker, A. T., Jalinous, R., and Freeston, I. L. (1985). Non-invasive magnetic stimulation of human motor cortex. Lancet 1, 1106–1107. doi: 10.1016/s0140-6736(85)92413-4
Blumberger, D. M., Vila-Rodriguez, F., Thorpe, K. E., Feffer, K., Noda, Y., Giacobbe, P., et al. (2018). Effectiveness of theta burst versus high-frequency repetitive transcranial magnetic stimulation in patients with depression (THREE-D): a randomised non-inferiority trial. Lancet 391, 1683–1692. doi: 10.1016/S0140-6736(18)30295-2
Cantello, R., Varrasi, C., Tarletti, R., Cecchin, M., D’Andrea, F., Veggiotti, P., et al. (2007). Ketogenic diet: electrophysiological effects on the normal human cortex. Epilepsia 48, 1756–1763. doi: 10.1111/j.1528-1167.2007.01156.x
Chen, R., Samii, A., Caños, M., Wassermann, E. M., and Hallett, M. (1997). Effects of phenytoin on cortical excitability in humans. Neurology 49, 881–883. doi: 10.1212/wnl.49.3.881
Damar, U., Gersner, R., Johnstone, J. T., Kapur, K., Collins, S., Schachter, S., et al. (2018). Alterations in the timing of huperzine A cerebral pharmacodynamics in the acute traumatic brain injury setting. J. Neurotrauma 35, 393–397. doi: 10.1089/neu.2017.5258
Danielsson, S., Gillberg, I. C., Billstedt, E., Gillberg, C., and Olsson, I. (2005). Epilepsy in young adults with autism: a prospective population-based follow-up study of 120 individuals diagnosed in childhood. Epilepsia 46, 918–923. doi: 10.1111/j.1528-1167.2005.57504.x
Dhamne, S. C., Ekstein, D., Zhuo, Z., Gersner, R., Zurakowski, D., Loddenkemper, T., et al. (2015). Acute seizure suppression by transcranial direct current stimulation in rats. Ann. Clin. Transl. Neurol. 2, 843–856. doi: 10.1002/acn3.226
Di Lazzaro, V., Oliviero, A., Pilato, F., Saturno, E., Dileone, M., Meglio, M., et al. (2004). Effects of vagus nerve stimulation on cortical excitability in epileptic patients. Neurology 62, 2310–2312. doi: 10.1212/01.wnl.0000131743.45131.ae
Eyre, J. A. (2003). Development and plasticity of the corticospinal system in man. Neural Plast. 10, 93–106. doi: 10.1155/np.2003.93
Fitzgerald, P. B., Fountain, S., and Daskalakis, Z. J. (2006). A comprehensive review of the effects of rTMS on motor cortical excitability and inhibition. Clin. Neurophysiol. 117, 2584–2596. doi: 10.1016/j.clinph.2006.06.712
Frye, R. E., Rotenberg, A., Ousley, M., and Pascual-Leone, A. (2008). Transcranial magnetic stimulation in child neurology: current and future directions. J. Child Neurol. 23, 79–96. doi: 10.1177/0883073807307972
Garvey, M. A., Kaczynski, K. J., Becker, D. A., and Bartko, J. J. (2001). Subjective reactions of children to single-pulse transcranial magnetic stimulation. J. Child Neurol. 16, 891–894. doi: 10.1177/088307380101601205
Gersner, R., Ekstein, D., Dhamne, S. C., Schachter, S. C., and Rotenberg, A. (2015). Huperzine A prophylaxis against pentylenetetrazole-induced seizures in rats is associated with increased cortical inhibition. Epilepsy Res. 117, 97–103. doi: 10.1016/j.eplepsyres.2015.08.012
Hameed, M. Q., Dhamne, S. C., Gersner, R., Kaye, H. L., Oberman, L. M., Pascual-Leone, A., et al. (2017). Transcranial magnetic and direct current stimulation in children. Curr. Neurol. Neurosci. Rep. 17:11. doi: 10.1007/s11910-017-0719-0
Hameed, M. Q., Hsieh, T.-H., Morales-Quezada, L., Lee, H. H. C., Damar, U., MacMullin, P. C., et al. (2018). Ceftriaxone treatment preserves cortical inhibitory interneuron function via transient salvage of GLT-1 in a rat traumatic brain injury model. Cereb. Cortex doi: 10.1093/cercor/bhy328 [Epub ahead of print].
Hannula, H., and Ilmoniemi, R. J. (2017). “Basic principles of navigated TMS,” in Navigated Transcranial Magnetic Stimulation in Neurosurgery, ed. S. M. Krieg (Cham: Springer), 3–29.
Hsieh, T.-H., Dhamne, S. C., Chen, J.-J. J., Pascual-Leone, A., Jensen, F. E., and Rotenberg, A. (2011). A new measure of cortical inhibition by mechanomyography and paired-pulse transcranial magnetic stimulation in unanesthetized rats. J. Neurophysiol. 107, 966–972. doi: 10.1152/jn.00690.2011
Hsieh, T. H., Lee, H. H. C., Hameed, M. Q., Pascual-Leone, A., Hensch, T. K., and Rotenberg, A. (2017). Trajectory of parvalbumin cell impairment and loss of cortical inhibition in traumatic brain injury. Cereb. Cortex 27, 5509–5524. doi: 10.1093/cercor/bhw318
Huang, Y.-Z., Edwards, M. J., Rounis, E., Bhatia, K. P., and Rothwell, J. C. (2005). Theta burst stimulation of the human motor cortex. Neuron 45, 201–206. doi: 10.1016/j.neuron.2004.12.033
Jannati, A., Block, G., Oberman, L. M., Rotenberg, A., and Pascual-Leone, A. (2017). Interindividual variability in response to continuous theta-burst stimulation in healthy adults. Clin. Neurophysiol. 128, 2268–2278. doi: 10.1016/j.clinph.2017.08.023
Kaye, H. L., Gersner, R., Boes, A. D., Pascual-Leone, A., and Rotenberg, A. (2017a). Persistent uncrossed corticospinal connections in patients with intractable focal epilepsy. Epilepsy Behav. 75, 66–71. doi: 10.1016/j.yebeh.2017.07.013
Kaye, H. L., Peters, J. M., Gersner, R., Chamberland, M., Sansevere, A., and Rotenberg, A. (2017b). Neurophysiological evidence of preserved connectivity in tuber tissue. Epilepsy Behav. Case Rep. 7, 64–68. doi: 10.1016/j.ebcr.2016.10.001
Kazis, D. A., Kimiskidis, V. K., Papagiannopoulos, S., Sotirakoglou, K., Divanoglou, D., Vlaikidis, N., et al. (2006). The effect of valproate on silent period and corticomotor excitability. Epileptic Disord. 8, 136–142.
Kimiskidis, V. K., Papagiannopoulos, S., Sotirakoglou, K., Kazis, D. A., Kazis, A., and Mills, K. R. (2005). Silent period to transcranial magnetic stimulation: construction and properties of stimulus-response curves in healthy volunteers. Exp. Brain Res. 163, 21–31. doi: 10.1007/s00221-004-2134-4
Kobayashi, M., and Pascual-Leone, A. (2003). Transcranial magnetic stimulation in neurology. Lancet Neurol. 2, 145–156. doi: 10.1016/S1474-4422(03)00321-1
Lang, N., Rothkegel, H., Peckolt, H., and Deuschl, G. (2013). Effects of lacosamide and carbamazepine on human motor cortex excitability: a double-blind, placebo-controlled transcranial magnetic stimulation study. Seizure 22, 726–730. doi: 10.1016/j.seizure.2013.05.010
Lee, H. W., Seo, H. J., Cohen, L. G., Bagic, A., and Theodore, W. H. (2005). Cortical excitability during prolonged antiepileptic drug treatment and drug withdrawal. Clin. Neurophysiol. 116, 1105–1112. doi: 10.1016/j.clinph.2004.12.004
Lefaucheur, J.-P., and Picht, T. (2016). The value of preoperative functional cortical mapping using navigated TMS. Neurophysiol. Clin. 46, 125–133. doi: 10.1016/j.neucli.2016.05.001
Levisohn, P. M. (2007). The autism-epilepsy connection. Epilepsia 48, 33–35. doi: 10.1111/j.1528-1167.2007.01399.x
Li, X., Ricci, R., Large, C. H., Anderson, B., Nahas, Z., and George, M. S. (2009). Lamotrigine and valproic acid have different effects on motorcortical neuronal excitability. J. Neural Transm. 116, 423–429. doi: 10.1007/s00702-009-0195-z
Liepert, J., Bär, K. J., Meske, U., and Weiller, C. (2001). Motor cortex disinhibition in Alzheimer’s disease. Clin. Neurophysiol. 112, 1436–1441. doi: 10.1016/s1388-2457(01)00554-5
Maeda, F., Gangitano, M., Thall, M., and Pascual-Leone, A. (2002). Inter-and intra-individual variability of paired-pulse curves with transcranial magnetic stimulation (TMS). Clin. Neurophysiol. 113, 376–382. doi: 10.1016/s1388-2457(02)00008-1
Manganotti, P., Bongiovanni, L. G., Zanette, G., Turazzini, M., and Fiaschi, A. (1999). Cortical excitability in patients after loading doses of lamotrigine: a study with magnetic brain stimulation. Epilepsia 40, 316–321. doi: 10.1111/j.1528-1157.1999.tb00711.x
Mix, A., Hoppenrath, K., and Funke, K. (2015). Reduction in cortical parvalbumin expression due to intermittent theta-burst stimulation correlates with maturation of the perineuronal nets in young rats. Dev. Neurobiol. 75, 1–11. doi: 10.1002/dneu.22205
Muller, P. A., Dhamne, S. C., Vahabzadeh-Hagh, A. M., Pascual-Leone, A., Jensen, F. E., and Rotenberg, A. (2014). Suppression of motor cortical excitability in anesthetized rats by low frequency repetitive transcranial magnetic stimulation. PLoS One 9:e91065. doi: 10.1371/journal.pone.0091065
Narayana, S., Rezaie, R., McAfee, S. S., Choudhri, A. F., Babajani-Feremi, A., Fulton, S., et al. (2015). Assessing motor function in young children with transcranial magnetic stimulation. Pediatr. Neurol. 52, 94–103. doi: 10.1016/j.pediatrneurol.2014.08.031
Oberman, L., Edwards, D., Eldaief, M., and Pascual-Leone, A. (2011). Safety of theta burst transcranial magnetic stimulation: a systematic review of the literature. J. Clin. Neurophysiol. 28, 67–74. doi: 10.1097/wnp.0b013e318205135f
Ossemann, M., de Fays, K., Bihin, B., and Vandermeeren, Y. (2016). Effect of a single dose of retigabine in cortical excitability parameters: a cross-over, double-blind placebo-controlled TMS study. Epilepsy Res. 126, 78–82. doi: 10.1016/j.eplepsyres.2016.06.004
Pascual-Leone, A., Freitas, C., Oberman, L., Horvath, J. C., Halko, M., Eldaief, M., et al. (2011). Characterizing brain cortical plasticity and network dynamics across the age-span in health and disease with TMS-EEG and TMS-fMRI. Brain Topogr. 24, 302–315. doi: 10.1007/s10548-011-0196-8
Pereira, L. S., Müller, V. T., da Mota Gomes, M., Rotenberg, A., and Fregni, F. J. (2016). Safety of repetitive transcranial magnetic stimulation in patients with epilepsy: a systematic review. Epilepsy Behav. 57, 167–176. doi: 10.1016/j.yebeh.2016.01.015
Peterchev, A. V., Murphy, D. L., and Lisanby, S. H. (2011). Repetitive transcranial magnetic stimulator with controllable pulse parameters. J. Neural. Eng. 8, 1–24. doi: 10.1088/1741-2560/8/3/036016
Peterchev, A. V., Wagner, T. A., Miranda, P. C., Nitsche, M. A., Paulus, W., Lisanby, S. H., et al. (2012). Fundamentals of transcranial electric and magnetic stimulation dose: definition, selection, and reporting practices. Brain Stimul. 5, 435–453. doi: 10.1016/j.brs.2011.10.001
Pilato, F., Profice, P., Ranieri, F., Capone, F., Di Iorio, R., Florio, L., et al. (2012). Synaptic plasticity in neurodegenerative diseases evaluated and modulated by in vivo neurophysiological techniques. Mol. Neurobiol. 46, 563–571. doi: 10.1007/s12035-012-8302-9
Premoli, I., Beatch, G., Rossini, P., Abela, E., Posadas, K., Green, L., et al. (2019). TMS-EEG and TMS-EMG to assess the pharmacodynamic profile of a novel potassium channel opener (XEN1101) on human cortical excitability. Brain Stimul. 12:459. doi: 10.1016/j.brs.2018.12.494
Reis, J., Wentrup, A., Hamer, H. M., Mueller, H.-H., Knake, S., Tergau, F., et al. (2004). Levetiracetam influences human motor cortex excitability mainly by modulation of ion channel function—a TMS study. Epilepsy Res. 62, 41–51. doi: 10.1016/j.eplepsyres.2004.08.001
Reutens, D. C., Berkovic, S. F., Macdonell, R. A. L., and Bladin, P. F. (1993). Magnetic stimulation of the brain in generalized epilepsy: reversal of cortical hyperexcitability by anticonvulsants. Ann. Neurol. 34, 351–355. doi: 10.1002/ana.410340308
Rossi, S., Hallett, M., Rossini, P. M., and Pascual-Leone, A. (2009). Safety, ethical considerations, and application guidelines for the use of transcranial magnetic stimulation in clinical practice and research. Clin. Neurophysiol. 120, 2008–2039. doi: 10.1016/j.clinph.2009.08.016
Rotenberg, A. (2018). “Measures of cortical excitability by transcranial magnetic stimulation,” in Inherited Metabolic Epilepsies, ed. P. L. Pearl (New York, NY: Demos), 201–206.
Rotenberg, A., Muller, P., Birnbaum, D., Harrington, M., Riviello, J. J., Pascual-Leone, A., et al. (2008). Seizure suppression by EEG-guided repetitive transcranial magnetic stimulation in the rat. Clin. Neurophysiol. 119, 2697–2702. doi: 10.1016/j.clinph.2008.09.003
Säisänen, L., Julkunen, P., Lakka, T., Lindi, V., Könönen, M., and Määttä, S. (2018). Development of corticospinal motor excitability and cortical silent period from mid-childhood to adulthood-a navigated TMS study. Neurophysiol. Clin. 48, 65–75. doi: 10.1016/j.neucli.2017.11.004
Schrader, L. M., Stern, J. M., Koski, L., Nuwer, M. R., and Engel, J. Jr. (2004). Seizure incidence during single-and paired-pulse transcranial magnetic stimulation (TMS) in individuals with epilepsy. Clin. Neurophysiol. 115, 2728–2737. doi: 10.1016/j.clinph.2004.06.018
Schwenkreis, P., Witscher, K., Janssen, F., Addo, A., Dertwinkel, R., Zenz, M., et al. (1999). Influence of the N-methyl-D-aspartate antagonist memantine on human motor cortex excitability. Neurosci. Lett. 270, 137–140. doi: 10.1016/s0304-3940(99)00492-9
Sohn, Y. H., Kaelin-Lang, A., Jung, H. Y., and Hallett, M. (2001). Effect of levetiracetam on human corticospinal excitability. Neurology 57, 858–863. doi: 10.1212/wnl.57.5.858
Stagg, C. J., Wylezinska, M., Matthews, P. M., Johansen-Berg, H., Jezzard, P., Rothwell, J. C., et al. (2009). Neurochemical effects of theta burst stimulation as assessed by magnetic resonance spectroscopy. J. Neurophysiol. 101, 2872–2877. doi: 10.1152/jn.91060.2008
Stern, W. M., Sander, J. W., Rothwell, J. C., and Sisodiya, S. M. (2017). Impaired intracortical inhibition demonstrated in vivo in people with Dravet syndrome. Neurology 88, 1659–1665. doi: 10.1212/wnl.0000000000003868
Tai, C., Abe, Y., Westenbroek, R. E., Scheuer, T., and Catterall, W. A. (2014). Impaired excitability of somatostatin-and parvalbumin-expressing cortical interneurons in a mouse model of Dravet syndrome. Proc. Natl. Acad. Sci. U S A 111, E3139–E3148. doi: 10.1073/pnas.1411131111
Tang, A. D., Lowe, A. S., Garrett, A. R., Woodward, R., Bennett, W., Canty, A. J., et al. (2016). Construction and evaluation of rodent-specific rTMS coils. Front. Neural Circuits 10:47. doi: 10.3389/fncir.2016.00047
Teo, J. T. H., Terranova, C., Swayne, O., Greenwood, R. J., and Rothwell, J. C. (2009). Differing effects of intracortical circuits on plasticity. Exp. Brain Res. 193, 555–563. doi: 10.1007/s00221-008-1658-4
Theodore, W. H. (2003). Transcranial magnetic stimulation in epilepsy. Epilepsy Curr. 3, 191–197. doi: 10.1046/j.1535-7597.2003.03607.x
Tuchman, R., and Rapin, I. (2002). Epilepsy in autism. Lancet Neurol. 1, 352–358. doi: 10.1016/S1474-4422(02)00160-6
Yang, X.-R., Kirton, A., Wilkes, T. C., Pradhan, S., Liu, I., Jaworska, N., et al. (2014). Glutamate alterations associated with transcranial magnetic stimulation in youth depression: a case series. J. ECT 30, 242–247. doi: 10.1097/YCT.0000000000000094
Ziemann, U. (2003). Pharmacology of TMS. Suppl. Clin. Neurophysiol. 56, 226–231. doi: 10.1016/S1567-424X(09)70226-0
Ziemann, U. (2004). TMS and drugs. Clin. Neurophysiol. 115, 1717–1729. doi: 10.1016/j.clinph.2004.03.006
Ziemann, U., Lönnecker, S., Steinhoff, B. J., and Paulus, W. (1996). The effect of lorazepam on the motor cortical excitability in man. Exp. Brain Res. 109, 127–135. doi: 10.1007/bf00228633
Ziemann, U., Reis, J., Schwenkreis, P., Rosanova, M., Strafella, A., Badawy, R., et al. (2015). TMS and drugs revisited 2014. Clin. Neurophysiol. 126, 1847–1868. doi: 10.1016/j.clinph.2014.08.028
Keywords: biomarker (development), transcranial magnetic stimulation (TMS), epilepsy—abnormalities, classification, drug therapy, drug development and application, neuromodulation, motor cortex excitability
Citation: Tsuboyama M, Kaye HL and Rotenberg A (2019) Biomarkers Obtained by Transcranial Magnetic Stimulation of the Motor Cortex in Epilepsy. Front. Integr. Neurosci. 13:57. doi: 10.3389/fnint.2019.00057
Received: 20 July 2019; Accepted: 23 September 2019;
Published: 30 October 2019.
Edited by:
Stephanie R. Jones, Brown University, United StatesReviewed by:
Philip Tseng, Taipei Medical University, TaiwanCopyright © 2019 Tsuboyama, Kaye and Rotenberg. This is an open-access article distributed under the terms of the Creative Commons Attribution License (CC BY). The use, distribution or reproduction in other forums is permitted, provided the original author(s) and the copyright owner(s) are credited and that the original publication in this journal is cited, in accordance with accepted academic practice. No use, distribution or reproduction is permitted which does not comply with these terms.
*Correspondence: Alexander Rotenberg, YWxleGFuZGVyLnJvdGVuYmVyZ0BjaGlsZHJlbnMuaGFydmFyZC5lZHU=
Disclaimer: All claims expressed in this article are solely those of the authors and do not necessarily represent those of their affiliated organizations, or those of the publisher, the editors and the reviewers. Any product that may be evaluated in this article or claim that may be made by its manufacturer is not guaranteed or endorsed by the publisher.
Research integrity at Frontiers
Learn more about the work of our research integrity team to safeguard the quality of each article we publish.