- 1Department of Entomology, Martin-Gatton College of Agriculture, Food and Environment, University of Kentucky, Lexington, KY, United States
- 2Department of Genetics, Yale School of Medicine, New Haven, CT, United States
More than 100 RNA chemical modifications to cellular RNA have been identified. N6-methyladenosine (m6A) is the most prevalent modification of mRNA. RNA modifications have recently attracted significant attention due to their critical role in regulating mRNA processing and metabolism. tRNA and rRNA rank among the most heavily modified RNAs, and their modifications are essential for maintaining their structure and function. With our advanced understanding of RNA modifications, increasing evidence suggests RNA modifications are important in regulating various aspects of insect life. In this review, we will summarize recent studies investigating the impact of RNA modifications in insects, particularly highlighting the role of m6A in insect development, reproduction, and adaptation to the environment.
1 Introduction
RNA plays pivotal roles in various cellular processes, including transcription and translation. Unlike DNA, which always exists in a double-stranded structure, RNA molecules are typically single-stranded and self-fold into the functional conformation with a three‐dimensional structure (e.g., transfer RNA, tRNA, and ribosomal RNA, rRNA). Furthermore, RNA molecules are subject to significantly more chemical modifications of their bases compared to DNA, with over 100 RNA modifications identified to date (1). Among major classes of RNA, transfer RNA (tRNA) contains the highest proportion of RNA modifications, with approximately 17% of all tRNA nucleotides being modified (2). Ribosome RNA (rRNA) comes in second, with around 2% of nucleotides being modified (3). Many rRNA modifications are located at the functional sites and are critical for their function (3–5). Additionally, many tRNA and rRNA modifications exhibit conservation across different organisms (2, 6). Historically, the function of mRNA modifications was poorly understood. The rapid development in genome-scale detection methods enables the accurate mapping of several mRNA modifications such as N6-methyladenosine (m6A), 5-methylcytidine (m5C), pseudouridine (Ψ), and N4-acetylcytidine (ac4C), greatly enhancing our understanding of mRNA modifications (7–12). Among them, the m6A modification is the most prevalent mRNA modification and the most intensively studied so far, with many important functions of m6A in cellular and biological processes having been discovered (13–20).
Writers, readers and erasers are the three most important groups of proteins regulating the dynamics and function of RNA modifications. Writers are responsible for installing the RNA modifications. Some writers are functional when they form a complex with other writer proteins or RNAs. For example, rRNA 2’-O-methylation and pseudouridine (Ψ), the two most abundant modifications in rRNA, are installed by small ribonucleoproteins (sRNP) complexes. The mRNA m6A transmethylase complex, the best understood mRNA modification writer, comprises the heterodimeric enzymes Mettl3 (Methyltransferase 3) and Mettl14 (Methyltransferase 14), as well as other accessory proteins, including WTAP (Wilms tumor 1-associated protein), VIRMA (Vir Like M6A Methyltransferase Associated), RBM15 (RNA-binding motif protein 15), ZC3H13 (zinc finger CCCH-type containing 13), and Hakai (21–23). RNA modification writers can also be stand-alone enzymes, including those that are responsible for installing many rRNA modifications such as N1-methyladenosine (m1A), m5C, N7-methylguanosine (m7G), 3-methyluridine (m3U) and a few mRNA modifications such as m5C, Ψ, and ac4C (3, 24). Reader proteins can directly recognize the RNA modifications or indirectly bind to the RNAs that undergo structure change after modifications, execute the biological function and regulate RNA metabolisms. The study of RNA modification readers mainly focuses on mRNA modifications. Among mRNA modification readers, the function and mechanisms of m6A mRNA readers are the most well understood. The mRNA m6A readers are mainly YTH family proteins, distributed in both cell cytosol and nucleus (25). Readers for other mRNA modifications such as m1A, m5C and ac4C have also been reported, but their binding mechanisms and regulatory roles are not yet clear (24). Lastly, eraser proteins can remove RNA modifications and reverse them back to an unmodified state. The existence of erasers has important implications, as it suggests that RNA modifications can serve as an epigenetic pathway to regulate gene expression bidirectionally. Compared to tRNA and rRNA, the mRNA is more dynamic and serves as the mediator of gene expression. Thus, the regulation of mRNA through modifications is more straightforward and has greater potential for understanding development and disease. Like readers, mRNA erasers are the most studied and understood in the mRNA m6A pathway. Two m6A demethylases, FTO (fat mass and obesity-associated) and ALKBH5 (alkB homolog 5, RNA demethylase), have been identified in mammals (17, 22). One potential m6A eraser, ALKBH8, was recently identified in insects (26).
RNA modifications play critical roles in biological functions. Previous studies in Escherichia coli bacteria and Saccharomyces cerevisiae yeast have suggested that tRNA and rRNA modifications are important in the formation and maintenance of their structure, with some modifications occurring at the key nucleotide within functional active sites (2–4). In the past decade, our understanding of mRNA modifications, particularly m6A, has experienced explosive advancements. The m6A regulates nearly every step of mRNA maturation and activity, including splicing (27, 28), polyadenylation (29), nuclear export (30, 31), stabilization (32), degradation (33) and translation (34). Therefore, m6A plays a critical role in regulating gene expression and affecting animal physiological processes by directly acting on mRNAs. Several reviews have exquisitely addressed the molecular mechanisms and therapeutic potentials of m6A (22, 25, 35).
Most of our understanding of RNA modifications including tRNA, rRNA and mRNA modifications comes from model organisms such as E. coli, S. cerevisiae, mice, zebrafish, and fruit flies (2, 4, 10, 36, 37). It remains largely unexplored about the specific role and mechanism of RNA modifications in the development and physiology of non-Drosophila insects. As the most successful animal group, insects develop in diverse and distinguished patterns, have astonishing behaviors, and adapt to almost every corner of the earth (38). It is anticipated that RNA modifications, which are crucial for regulating cellular function including gene expression, play critical roles in different aspects of insect biology. In the last few years, entomologists have begun to investigate the role of mRNA m6A in different insects, uncovering various functions of m6A in insects. This review aims to present an overview of recent advances in understanding RNA modifications in insects, specifically focusing on mRNA m6A which has been suggested to regulate insect development and the response of insects to the environment. The final section of this review also addresses the functions of tRNA and rRNA modifications in insects. We did not cover other non-m6A mRNA modifications and other RNA modifications due to the limited number of studies on these modifications in insects.
2 The role of m6A RNA modifications in insect development and reproduction
Insects exhibit diverse life cycles, but most holometabolous insects adhere to a general pattern, including egg, larva, pupa, and adult life stages. The cycle begins with the eggs laid by adults in a desirable environment. After completing embryonic development, larvae hatch from the eggs and undergo a rapid growth phase with massive feeding and several molts as they significantly increase body size. Following the larval stage, insects undergo pupation with extensive tissue remodeling and transform into pupae. Finally, the adults emerge from pupal cases, complete their reproductive development, and are ready to produce the next generation. The hemimetabolous insects skip the pupal stage as the nymphs undergo metamorphosis to become adults. Insect development and reproduction require the temporal and spatial regulation of gene expression to meet the needs of different tissues at various stages. As a critical epigenetic factor, the role of m6A in mRNA metabolism during insect development and reproduction is an intriguing question.
2.1 Embryogenesis
During the first few hours of embryogenesis, the maternal RNAs play a critical role in embryonic development. As the development progresses, the zygotic genome starts to synthesize its own RNAs. In vertebrate embryonic development, the m6A pathway is important by regulating both maternal and zygotic mRNAs. Notably, a significant portion of maternal mRNAs in the embryos of zebrafish and mice are marked with m6A labels, which are critical for the maternal-to-zygotic transition (MZT) (37, 39). Several studies also have highlighted the importance of m6A in post-MZT embryonic development (16, 40). Disturbing zygotic m6A by knocking out the Mettl3 gene in the embryo caused death at an early embryonic stage in mice by failing to regulate naïve pluripotency (16). Similarly, morpholino-mediated knockdown of Mettl3 in zebrafish embryos caused tissue differentiation defects and increased apoptosis (40).
In insects, m6A modifications on maternal mRNA are also important for successful embryonic development. In Drosophila melanogaster, m6A has been shown to contribute to the degradation of maternal RNAs (41). A study utilizing RNA-seq analysis of embryos derived from both wild type and Mettl3–Mettl14 double maternal mutants revealed that, in the eggs laid by the mutants, a significant portion of m6A-marked maternal RNAs remains stable during the maternal-to-zygotic transition (41). Approximately 40% of eggs laid by Mettl3-Mettl14 double maternal mutants failed to hatch (41). A more severe effect was observed in the red flour beetle Tribolium castaneum; eggs laid by Mettl3 dsRNA-treated females stopped embryonic development at an early embryonic stage before nuclear division and migration (42).
The role of m6A in post-MZT embryonic development varies in different insects. Mettl3 null mutants of D. melanogaster remain viable and can survive to adult stages (27). However, in the silkworm Bombyx mori, knockdown of Mettl3 by embryonic injection of siRNA affected several signaling pathways, including Wnt and Toll/Imd signaling required for embryogenesis (43). Similarly, in the fall armyworm, Spodoptera frugiperda, methylated RNA immunoprecipitation-seq, and RNA-seq suggested that m6A regulates the expression of genes required for organogenesis during embryogenesis (44).
2.2 Post-embryonic development
After hatching from eggs, insect larvae undergo several molts and metamorphosis and ultimately develop into the adult stage with reproduction capability. Insects exhibit a remarkable diversity in their post-embryonic development modes, including different molting times, various body structures, specialized organs, and unique behavioral characteristics. These distinct features are shaped by determinantal genes, which are precisely expressed and regulated to ensure accurate and appropriate development. As a major epigenetic marker, mRNA m6A regulates insect post-embryonic development. While m6A deficiency does not cause acute lethal effects in the post-embryonic stage of insects (17, 42), recent research has uncovered its role in fine-tuning gene expression and delicately moderating post-embryonic development in various insect species.
Several recent studies suggest that m6A plays a critical role in caste differentiation in social insects. The western honeybee, Apis mellifera, is a widely used model for studying the development and behaviors of social insects. A recent investigation has found a critical role of m6A modification in larval development and caste differentiation of honeybees (45). For the genetically identical female larvae of honeybees, worker larvae contain hypermethylated m6A than queen larvae (45). Chemical suppression of m6A in worker larvae can induce the development of queen features (45). In addition, another group showed that the m6A pathway might regulate the behavior of honeybee workers; different Mettl3 expression and m6A levels have been observed in the brain and fat body of young and old adult honeybee workers inferring the role of m6A in regulating behavior switch during age progression (46, 47).
As mentioned above, D. melanogaster is the most extensively studied insect regarding the function of m6A (17, 21, 27, 36, 48, 49). Deficiency of Mettl3 does not cause lethal effects in D. melanogaster post-embryonic development (17). However, Mettl3 mutant flies show defects in locomotion and memory, which are mediated by different m6A readers (17, 48). These behavior and cognitive defects are consistent with the detected high enrichment of m6A in the D. melanogaster nervous system (49). The effect of m6A on fly behavior may be induced by disturbed axonal overgrowth and misguidance of the fly nervous system (50). More interestingly, D. melanogaster sex determination is also regulated by the m6A pathway (17, 27, 49). The splicing of Sex-lethal (Sxl, the master sex determination factor) mRNA is regulated by the m6A pathway. Females with m6A deficiency produce male-specific Sxl isoform and have relatively high mortality, while males are unaffected (17, 49). The regulation of sex determination seems to be specific for D. melanogaster. The function of m6A in regulating sex determination was not observed in insects such as T. castaneum that do not utilize Sxl as a sex determination regulator (42).
m6A also affects various aspects of other insects. In T. castaneum, the m6A pathway plays a crucial role in the ecdysis process during eclosion; knocking down m6A writers causes the failure of shedding old pupal cuticles without affecting the formation of adult structures (42). In the silkworm B. mori, m6A was found to specifically mediate the function of the posterior silk gland regulated by juvenile hormone analog (51). In summary, the role of m6A in insect post-embryonic development appears to be very diverse and species-specific (Figure 1).
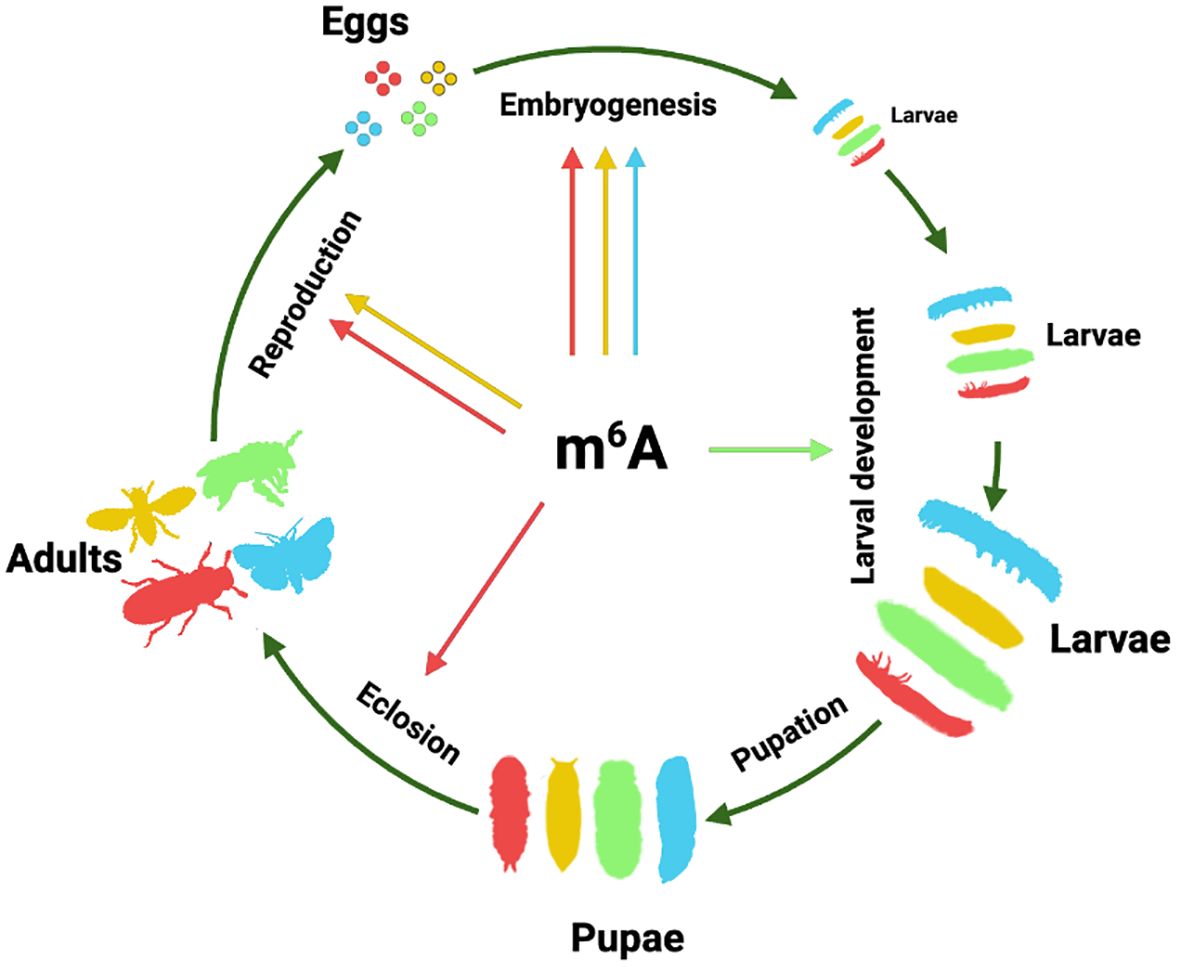
Figure 1. The role of m6A RNA modification in insect development and reproduction. Hypothetical insect life cycles are used to represent the function of m6A in various developmental stages of different insects. Yellow represents Diptera drawing with the silhouette of D. melanogaster; green represents Hymenoptera using A. mellifera silhouette; red represents Coleoptera using T. castaneum silhouette; and blue represents Lepidoptera using S. frugiperda silhouette. The arrow sourced from the central m6A symbol indicates the existence of reported evidence in this insect order. The silhouettes were obtained from PhyloPic (http://phylopic.org) or created by the authors.
2.3 Reproduction
The m6A-mediated epigenetic regulation has been reported to be involved in many reproductive diseases of mammals (52). In mice, disturbing the m6A pathway by knocking out m6A players has been shown to affect the fertility of both males and females (29, 39, 53–55). The development of insect reproductive systems also requires the m6A pathway. In D. melanogaster, ovary development defects were observed in Mettl3 mutants (17, 56). Meanwhile, male adults of Drosophila Mettl3 mutants showed reduced fertility, and the effect of m6A on sperm spermatogenesis is likely mediated by regulating chickadee mRNA (57). Similarly, in T. castaneum, the knockdown of Mettl3 affects the fertility of both females and males; the reduced number and size of female oocytes were observed in Mettl3 knockdown females (42). A recent transcriptome-wide study conducted in Anopheles sinensis revealed that several genes associated with spermatogenesis are m6A-modulated and may be regulated by the m6A pathway (58). While existing studies indicate a broad involvement of m6A in regulating insect reproduction, the precise mechanisms through which m6A influences the development of insect reproductive systems and whether it acts through shared pathways or genes in various insect species remain unclear.
3 The role of m6A RNA modifications in insect stress response
Insects are confronted with a wide range of external factors, including both biotic and abiotic factors, and have evolved various strategies to survive and thrive under these challenging conditions (59–62). Recent studies have shed light on the involvement of the m6A pathway in the adaptive responses of insects to various external challenges, including virus infections and exposure to insecticide (Figure 2).
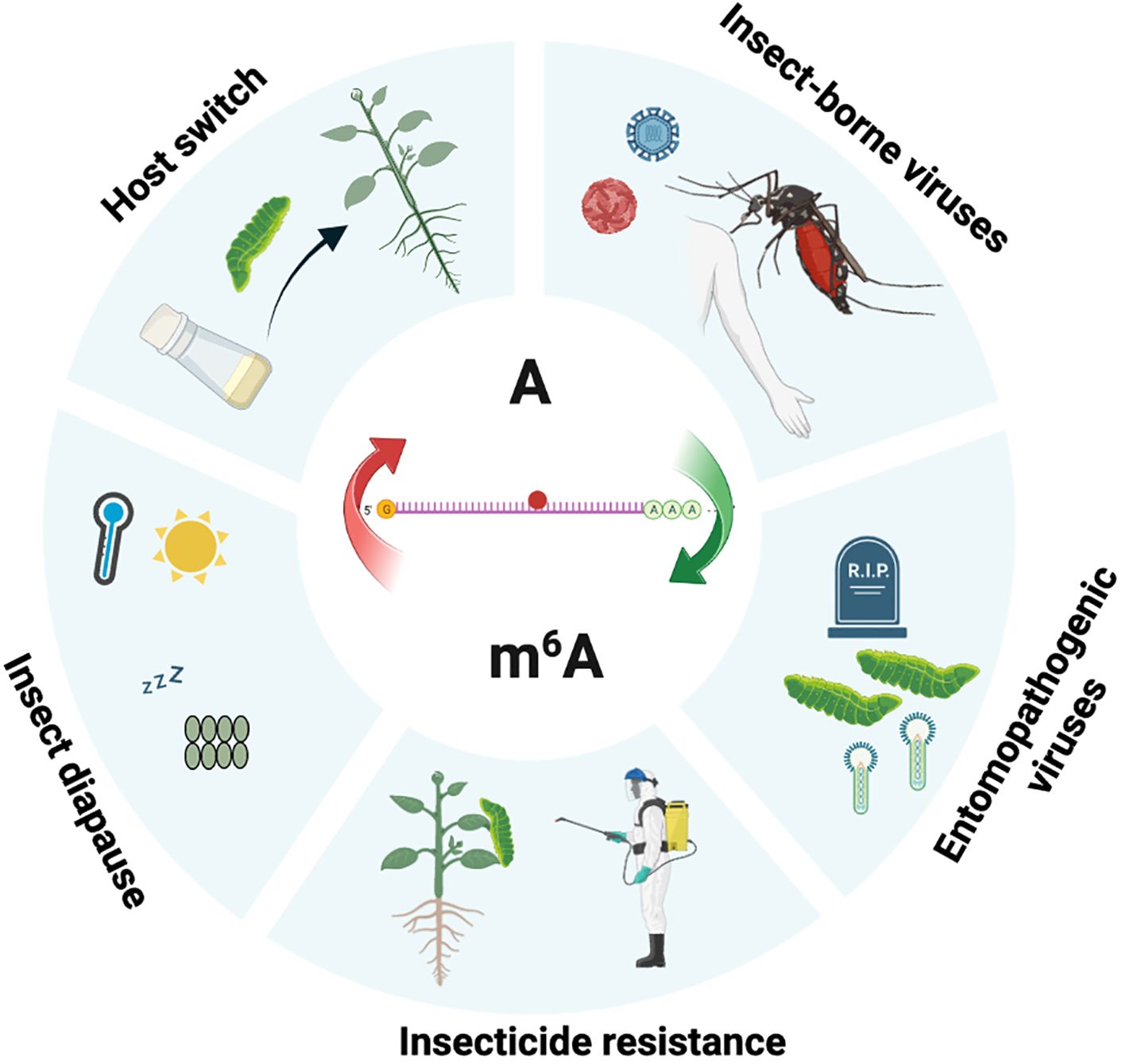
Figure 2. The role of m6A modification in insects interacting with external factors. Schematic representation shows the involvement of m6A in the insect adaptation to the environment. This figure was created utilizing Biorender.
3.1 Entomopathogenic viruses
Entomopathogenic viruses are one of the major threats to insect colony maintenance and farming, but meanwhile, serve as a promising pest control strategy. The silkworm, B. mori, is an economically and culturally important insect in Asia. B. mori nucleopolyhedrovirus (BmNPV) is one of the top threats to the silkworm industry. The m6A immunoprecipitation sequencing revealed that m6A levels of B. mori are associated with BmNPV infection (63, 64). A significant increase in m6A peaks was observed in B. mori midguts following BmNPV infection (63, 64). However, contrasting functions of the m6A pathway in the BmNPV infection were observed. One investigation showed that knocking down BmMettl3 in BmN cells led to an increased BmNPV infection, while overexpression reduced the infection (64). On the contrary, in another study (63), it has been shown that low expression levels of BmMettl3 enhance the resistance to BmNPV infection. Despite the established involvement of m6A in the BmNPV infection of silkworms, the precise mechanisms through which m6A mediates the entomopathogenic virus infection remain elusive.
3.2 Insect-borne viruses
Unlike entomopathogenic viruses, insect-borne viruses typically do not significantly affect insect survival; however, they pose a significant threat to food safety and human health by causing plant and human diseases (65, 66). Recent studies have shed light on the regulatory role of the m6A pathway in viral proliferation by acting on viral RNA or the host’s genes (67–70).
The presence of m6A sites has been identified in the RNA genomes of Flaviviridae viruses, including dengue, Zika, yellow fever, and West Nile virus (71). It has been revealed that the m6A machinery of the human host can directly regulate the viral replication by modulating RNA modifications of the Zika viral mRNA; the methylation of viral mRNA promotes the degradation of viral mRNA mediated by YTHDFs (69). A later study has further shown that Flaviviridae infection also induces the m6A changes in human cellular mRNAs, which regulates the viral infection (68). Similarly, within the mosquito host, the m6A pathway of mosquitos also plays a regulatory role in virus proliferation (67). In the yellow fever mosquito, Aedes aegypti, the m6A pathway in mosquitoes has been found to regulate the proliferation of dengue virus (67). The regulation of m6A modification is mediated by affecting ubiquitin carrier protein 9 (Ubc9) gene expression of Ae. aegypti; knockdown and overexpression of Ubc9 led to reduced and increased dengue virus infection (67). Inhibition of m6A, either by applying a chemical inhibitor of m6A or silencing m6A methyltransferase genes, reduced the abundance and stability of Ubc9 mRNA and thus decreased dengue virus infection (67). A case of m6A-mediated regulation of plant pathogenic virus replication in insect vectors has also been reported (72). The study showed that the infection of rice black-streaked dwarf virus (RBSDV) decreases the m6A level in midgut cells of the insect vector, small brown planthopper, Laodelphax striatellus. Silencing of m6A methyltransferase in L. striatellus significantly induced RBSDV titer; however, it is not clear how m6A mediates the replication of RBSDV (72).
In recent reports, the role of m6A in insect vector-virus interactions has been highlighted. However, conflicting roles of m6A in the virus life cycle have been observed. This discrepancy may arise from different modes of action, such as directly acting on viral RNA or through targeting genes of the insect host that are critical for virus infection. Since many insect-borne viruses are RNA viruses, they are likely subject to modifications by the m6A pathway of insect hosts and may further be regulated by the m6A pathway. We anticipate that further investigations will uncover additional roles of the host and vector m6A pathways in regulating insect-viruses interactions.
3.3 Other external factors
Besides viruses, recent studies have also revealed the involvement of the m6A pathway in insects’ response to other external stresses. Many insects can rapidly develop pesticide resistance through natural selection, causing tremendous economic and environmental losses (73). A recent study indicated that the m6A pathway contributes to the insecticide thiamethoxam resistance in a global invasive agricultural pest, the whitefly Bemisia tabaci (74). m6A levels were found to be higher in resistance strains than the susceptible strain, and the introduction of a m6A site at a P450 gene promoter region by a mutation in the resistance strains is one of the main causes for the resistance emergence (74). The m6A pathway has also been revealed to be involved in the host plant adaption of diamondback moth Plutella xylostella (75). Compared to the wild-type insects, P. xylostella mutants of Mettl3 and Mettl14 showed a significant deduction of larval and pupal weight when transferring from the artificial diet to the host plant (75). m6A was also found to regulate juvenile hormone (JH) titer in the diamondback moth P. xylostella to enhance the pest fitness when resisting the Bt Cry1Ac toxin (76).
Insect diapause is a crucial and adaptive trait that enables insects to survive in extreme environments, usually triggered by environmental factors such as photoperiod and low temperature (77). A study on silkworm B. mori showed that the diapause‐destined eggs have a relatively greater m6A content than nondiapause‐destined eggs (78). The follow-up studies from the same group further revealed that the m6A pathway regulates the diapause of B. mori eggs by acting on genes in the insect hormones synthesis pathway (79, 80).
4 tRNA and rRNA modifications in insects
Despite the important roles that mRNA modifications play, among all RNA types, tRNA has the highest proportion of modifications, followed by rRNA. Many modifications of tRNA and rRNA are in the functional regions and thus are critical for maintaining RNA structure and facilitating their functions (2–5, 81). The loss of these important modifications can result in translation deficiency and cause developmental delay and/or lethal effects. Some modifications are installed by non-conserved enzymes or altered in response to cellular environmental change, playing less critical roles in translation (3, 81). However, the functions of these modifications in insects including the model insect D. melanogaster are not well studied. Given that tRNA and rRNA have the most abundant modifications, understanding how these modifications affect insects could provide valuable insights into insect development and developing pest control strategies. Therefore, in this section, we summarize the studies that have investigated various tRNA and rRNA modifications in insects, although there are a limited number of them.
4.1 Transfer RNA modifications
Studies in D. melanogaster have focused on specific tRNA modifications and shown that some are critical for Drosophila development and maintenance of normal lifespan. For example, N6-threonylcarbamoyl-adenosine (t6A) is a universally conserved modification in the tRNA anticodon region, which is critical for decoding ANN codons and supports proper translation (82). Loss of t6A induced by knockdown or mutations of t6A biosynthesis factors caused various developmental deficiencies, including melanotic growth and reduced developmental rate (83, 84). At the tissue level, proliferating tissues, such as wing discs, are more affected by t6A deficiency than non-proliferating tissues (83, 84). In a recent study, research through a genome-wide screen revealed that two tRNA 2’-O-methylation methyltransferases are critical for small RNA pathways (85). Mutants are viable and fertile but exhibit a shorter lifespan and increased sensibility to RNA virus infection (85).
The tRNA modifications are involved in mosquito reproduction (86). The abundance of tRNA modifications is sex-associated in mosquitoes (86). Higher tRNA modifications were detected in females compared to males of Ae. aegypti, Culex pipiens, and Anopheles stephensi mosquitoes (86). The high tRNA modifications in females likely are contributed by the increased expression of tRNA-modifying enzymes in female ovaries, suggesting tRNA modification may be critical for mosquito reproduction (86).
4.2 Ribosomal RNA modifications
2’-O-methylation and pseudouridine (Ψ) are the two most abundant rRNA modifications, which are modified by box C/D and box H/ACA sRNP complexes, respectively. Many of these modifications are clustered in the functional region of the ribosome and thus are critical for cell function and survival (87, 88). D. melanogaster null mutants of the box H/ACA Ψ synthetase Nop60B results in lethality, while partial loss-of-function mutations of Nop60B caused developmental delay, reduced body size, hatched abdominal cuticle, and decreased female fertility (89, 90). In T. castaneum, knockdown of box C/D methyltransferase fibrillarin (TC002569) and the box H/ACA Ψ synthetase TcNop60B (TC010569) causes high mortality (unpublished data from the authors) (91).
Besides 2’-O-methylation and Ψ, rRNA contains many other modifications, including methylation, acetylation, etc. These rRNA modifications are usually introduced by stand-alone enzymes (3). For example, in D. melanogaster, methyltransferase NSUN5 was predicted to modify cytosine 3620 of 28S rRNA (92). Loss of NSUN5 can increase the lifespan and stress resistance of D. melanogaster (92). This is likely due to the loss of methylation, causing the ribosome structure to change and making ribosomes favor the recruitment of oxidative stress-responsive mRNAs (92). D. melanogaster Mettl5 is specifically required to deposit m6A near the decoding center of 18S ribosomal RNA (93). However, Mettl5 does not affect ribosome maturation but is required for the orientation and locomotion of adult flies (93).
5 Conclusions and future perspectives
In the past few years, RNA modifications, including mRNA m6A and other RNA modifications, have been revealed to be fundamental in RNA transcription and translation, which add another layer of epigenetic factors for gene regulation (1). Among RNA modifications, the function of mRNA m6A in insects is most well-understood and plays an important role in the regulation of the insect life cycle. Loss of function studies such as knockdown or knockout of the key players in the m6A methyltransferases complex showed that the m6A pathway is critical for insect embryogenesis and reproduction, and the regulation is likely conserved among insects. However, the impact of the m6A pathway on insect post-embryonic development is either a sublethal/minor effect or insect species dependent. Furthermore, more and more studies suggest that m6A is likely involved in insects’ environmental adaptation when insects face biotic or abiotic environmental factors such as virus infection and natural and artificial xenobiotics, which suggests that the mRNA m6A could serve as a critical factor in understanding the mechanisms behind insect pest outbreaks and evolution. In future studies, it is important to understand how m6A regulates different insect life processes. The genes affected by m6A could serve as new targets for pest control or pesticide resistance management.
Research on the function of tRNA and rRNA modifications in insects is generally limited and has been mostly focused on Drosophila. However, many of these modifications serve as important functional sites in tRNA and rRNA, and their deficiency often causes severe lethal effects. Thus, genes involved in tRNA and rRNA modifications could serve as potential targets for RNAi pest control strategies.
Author contributions
YJ: Formal analysis, Writing – review & editing, Writing – original draft, Conceptualization. SP: Resources, Project administration, Funding acquisition, Writing – review & editing, Writing – original draft, Conceptualization.
Funding
The author(s) declare financial support was received for the research, authorship, and/or publication of this article. Research in the Palli laboratory is supported by grants from the National Institutes of Health (GM070559-15 and 1R21AI131427-01), the National Science Foundation (Industry/University Cooperative Research Centers, the Center for Arthropod Management Technologies under grant IIP-1821936), and the USDA/NIFA (under Hatch Project 2351177000 and Agriculture and Food Research Initiative Competitive Grant 2019-67013-29351). The content is solely the author’s responsibility and does not necessarily represent the official views of funders.
Conflict of interest
The authors declare that the research was conducted in the absence of any commercial or financial relationships that could be construed as a potential conflict of interest.
The author(s) declared that they were an editorial board member of Frontiers, at the time of submission. This had no impact on the peer review process and the final decision.
Publisher’s note
All claims expressed in this article are solely those of the authors and do not necessarily represent those of their affiliated organizations, or those of the publisher, the editors and the reviewers. Any product that may be evaluated in this article, or claim that may be made by its manufacturer, is not guaranteed or endorsed by the publisher.
References
1. Roundtree IA, Evans ME, Pan T, He C. Dynamic RNA modifications in gene expression regulation. Cell. (2017) 169:1187–200. doi: 10.1016/j.cell.2017.05.045
2. Jackman JE, Alfonzo JD. Transfer RNA modifications: nature's combinatorial chemistry playground. Wiley Interdiscip Rev RNA. (2013) 4:35–48. doi: 10.1002/wrna.1144
3. Sloan KE, Warda AS, Sharma S, Entian KD, Lafontaine DLJ, Bohnsack MT. Tuning the ribosome: The influence of rRNA modification on eukaryotic ribosome biogenesis and function. RNA Biol. (2017) 14:1138–52. doi: 10.1080/15476286.2016.1259781
4. Decatur WA, Fournier MJ. rRNA modifications and ribosome function. Trends Biochem Sci. (2002) 27:344–51. doi: 10.1016/S0968-0004(02)02109-6
5. Anger AM, Armache JP, Berninghausen O, Habeck M, Subklewe M, Wilson DN, et al. Structures of the human and Drosophila 80S ribosome. Nature. (2013) 497:80–5. doi: 10.1038/nature12104
6. Polikanov YS, Melnikov SV, Soll D, Steitz TA. Structural insights into the role of rRNA modifications in protein synthesis and ribosome assembly. Nat Struct Mol Biol. (2015) 22:342–4. doi: 10.1038/nsmb.2992
7. Gilbert WV, Nachtergaele S. mRNA regulation by RNA modifications. Annu Rev Biochem. (2023) 92:175–98. doi: 10.1146/annurev-biochem-052521-035949
8. Owens MC, Zhang C, Liu KF. Recent technical advances in the study of nucleic acid modifications. Mol Cell. (2021) 81:pp.4116–4136. doi: 10.1016/j.molcel.2021.07.036
9. Dominissini D, Moshitch-Moshkovitz S, Salmon-Divon M, Amariglio N, Rechavi G. Transcriptome-wide mapping of N6-methyladenosine by m6A-seq based on immunocapturing and massively parallel sequencing. Nat Protoc. (2013) 8:176–89. doi: 10.1038/nprot.2012.148
10. Dominissini D, Moshitch-Moshkovitz S, Schwartz S, Salmon-Divon M, Ungar L, Osenberg S, et al. Topology of the human and mouse m6A RNA methylomes revealed by m6A-seq. Nature. (2012) 485:201–6. doi: 10.1038/nature11112
11. Linder B, Grozhik AV, Olarerin-George AO, Meydan C, Mason CE, Jaffrey SR. Single-nucleotide-resolution mapping of m6A and m6Am throughout the transcriptome. Nat Methods. (2015) 12:767–72. doi: 10.1038/nmeth.3453
12. Meyer KD. DART-seq: an antibody-free method for global m6A detection. Nat Methods. (2019) 16:1275–80. doi: 10.1038/s41592-019-0570-0
13. Perry RP, Kelley DE. Existence of methylated messenger-RNA in mouse L cells. Cell. (1974) 1:37–42. doi: 10.1016/0092-8674(74)90153-6
14. Chen X-Y, Zhang J, Zhu J-S. The role of m6A RNA methylation in human cancer. Mol Cancer. (2019) 18:1–9. doi: 10.1186/s12943-019-1033-z
15. Fustin J-M, Doi M, Yamaguchi Y, Hida H, Nishimura S, Yoshida M, et al. RNA-methylation-dependent RNA processing controls the speed of the circadian clock. Cell. (2013) 155:793–806. doi: 10.1016/j.cell.2013.10.026
16. Geula S, Moshitch-Moshkovitz S, Dominissini D, Mansour AA, Kol N, Salmon-Divon M, et al. m6A mRNA methylation facilitates resolution of naïve pluripotency toward differentiation. Science. (2015) 347:1002–6. doi: 10.1126/science.1261417
17. Kan L, Grozhik AV, Vedanayagam J, Patil DP, Pang N, Lim KS, et al. The m6A pathway facilitates sex determination in Drosophila. Nat Commun. (2017) 8:15737. doi: 10.1038/ncomms15737
18. Murakami S, Jaffrey SR. Hidden codes in mRNA: Control of gene expression by m6A. Mol Cell. (2022) 82:2236–51. doi: 10.1016/j.molcel.2022.05.029
19. Wei G, Almeida M, Pintacuda G, Coker H, Bowness JS, Ule J, et al. Acute depletion of METTL3 implicates N6-methyladenosine in alternative intron/exon inclusion in the nascent transcriptome. Genome Res. (2021) 31:1395–408. doi: 10.1101/gr.271635.120
20. Zaccara S, Jaffrey SR. A unified model for the function of YTHDF proteins in regulating m6A-modified mRNA. Cell. (2020) 181:1582–95. doi: 10.1016/j.cell.2020.05.012
21. Guo J, Tang HW, Li J, Perrimon N, Yan D. Xio is a component of the Drosophila sex determination pathway and RNA N6-methyladenosine methyltransferase complex. Proc Natl Acad Sci U S A. (2018) 115:3674–9. doi: 10.1073/pnas.1720945115
22. Meyer KD, Jaffrey SR. Rethinking m6A readers, writers, and erasers. Annu Rev Cell Dev Biol. (2017) 33:319–42. doi: 10.1146/annurev-cellbio-100616-060758
23. Ruzicka K, Zhang M, Campilho A, Bodi Z, Kashif M, Saleh M, et al. Identification of factors required for m6A mRNA methylation in Arabidopsis reveals a role for the conserved E3 ubiquitin ligase HAKAI. New Phytol. (2017) 215:157–72. doi: 10.1111/nph.14586
24. Flamand MN, Tegowski M, Meyer KD. The proteins of mRNA modification: writers, readers, and erasers. Annu Rev Biochem. (2023) 92:145–73. doi: 10.1146/annurev-biochem-052521-035330
25. Patil DP, Pickering BF, Jaffrey SR. Reading m6A in the transcriptome: m6A-binding proteins. Trends Cell Biol. (2018) 28:113–27. doi: 10.1016/j.tcb.2017.10.001
26. Dai Z, Asgari S. ALKBH8 as a potential N6-methyladenosine (m6A) eraser in insects. Insect Mol Biol. (2023) 32:461–8. doi: 10.1111/imb.12843
27. Haussmann IU, Bodi Z, Sanchez-Moran E, Mongan NP, Archer N, Fray RG, et al. m6A potentiates Sxl alternative pre-mRNA splicing for robust Drosophila sex determination. Nature. (2016) 540:301–4. doi: 10.1038/nature20577
28. Xiao W, Adhikari S, Dahal U, Chen YS, Hao YJ, Sun BF, et al. Nuclear m6A reader YTHDC1 regulates mRNA splicing. Mol Cell. (2016) 61:507–19. doi: 10.1016/j.molcel.2016.01.012
29. Kasowitz SD, Ma J, Anderson SJ, Leu NA, Xu Y, Gregory BD, et al. Nuclear m6A reader YTHDC1 regulates alternative polyadenylation and splicing during mouse oocyte development. PLoS Genet. (2018) 14:e1007412. doi: 10.1371/journal.pgen.1007412
30. Edens BM, Vissers C, Su J, Arumugam S, Xu Z, Shi H, et al. FMRP modulates neural differentiation through m6A-dependent mRNA nuclear export. Cell Rep. (2019) 28:845–54. doi: 10.1016/j.celrep.2019.06.072
31. Roundtree IA, Luo G-Z, Zhang Z, Wang X, Zhou T, Cui Y, et al. YTHDC1 mediates nuclear export of N6-methyladenosine methylated mRNAs. eLife. (2017) 6:e31311. doi: 10.7554/eLife.31311
32. Mauer J, Luo X, Blanjoie A, Jiao X, Grozhik AV, Patil DP, et al. Reversible methylation of m6Am in the 5’cap controls mRNA stability. Nature. (2017) 541:371–5. doi: 10.1038/nature21022
33. Lee Y, Choe J, Park OH, Kim YK. Molecular mechanisms driving mRNA degradation by m6A modification. Trends Genet. (2020) 36:177–88. doi: 10.1016/j.tig.2019.12.007
34. Coots RA, Liu X-M, Mao Y, Dong L, Zhou J, Wan J, et al. m6A facilitates eIF4F-independent mRNA translation. Mol Cell. (2017) 68:504–14. doi: 10.1016/j.molcel.2017.10.002
35. Shi H, Wei J, He C. Where, when, and how: context-dependent functions of RNA methylation writers, readers, and erasers. Mol Cell. (2019) 74:640–50. doi: 10.1016/j.molcel.2019.04.025
36. Lence T, Soller M, Roignant JY. A fly view on the roles and mechanisms of the m6A mRNA modification and its players. RNA Biol. (2017) 14:1232–40. doi: 10.1080/15476286.2017.1307484
37. Zhao BS, Wang X, Beadell AV, Lu Z, Shi H, Kuuspalu A, et al. m6A-dependent maternal mRNA clearance facilitates zebrafish maternal-to-zygotic transition. Nature. (2017) 542:475–8. doi: 10.1038/nature21355
38. Stork NE. How many species of insects and other terrestrial arthropods are there on Earth? Annu Rev Entomol. (2018) 63:31–45. doi: 10.1146/annurev-ento-020117-043348
39. Ivanova I, Much C, Di Giacomo M, Azzi C, Morgan M, Moreira PN, et al. The RNA m6A reader YTHDF2 is essential for the post-transcriptional regulation of the maternal transcriptome and oocyte competence. Mol Cell. (2017) 67:1059–67. doi: 10.1016/j.molcel.2017.08.003
40. Ping XL, Sun BF, Wang L, Xiao W, Yang X, Wang WJ, et al. Mammalian WTAP is a regulatory subunit of the RNA N6-methyladenosine methyltransferase. Cell Res. (2014) 24:177–89. doi: 10.1038/cr.2014.3
41. Zhang G, Xu Y, Wang X, Zhu Y, Wang L, Zhang W, et al. Dynamic FMR1 granule phase switch instructed by m6A modification contributes to maternal RNA decay. Nat Commun. (2022) 13:859. doi: 10.1038/s41467-022-28547-7
42. Jiao Y, Palli SR. N6-adenosine (m6A) mRNA methylation is required for Tribolium castaneum development and reproduction. Insect Biochem Mol Biol. (2023) 159:103985. doi: 10.1016/j.ibmb.2023.103985
43. Liu SQ, Jia SZ, Tian H, Li YH, Hu KW, Tao JG, et al. Evolution of m6A-related genes in insects and the function of METTL3 in silkworm embryonic development. Insect Mol Biol. (2023) 32:316–27. doi: 10.1111/imb.12832
44. Chen Y, Lai Y, Liu R, Yao L, Yu XQ, Wang X. Transcriptome-wide analysis of mRNA N6-methyladenosine modification in the embryonic development of Spodoptera frugiperda. Insect Sci. (2023) 30:1229–44. doi: 10.1111/1744-7917.13172
45. Wang M, Xiao Y, Li Y, Wang X, Qi S, Wang Y, et al. RNA m6A modification functions in larval development and caste differentiation in honeybee (Apis mellifera). Cell Rep. (2021) 34:108580. doi: 10.1016/j.celrep.2020.108580
46. Bataglia L, Simoes ZLP, Nunes FMF. Active genic machinery for epigenetic RNA modifications in bees. Insect Mol Biol. (2021) 30:566–79. doi: 10.1111/imb.12726
47. Bataglia L, Simoes ZLP, Nunes FMF. Transcriptional expression of m6A and m5C RNA methyltransferase genes in the brain and fat body of honey bee adult workers. Front Cell Dev Biol. (2022) 10:921503. doi: 10.3389/fcell.2022.921503
48. Kan L, Ott S, Joseph B, Park ES, Dai W, Kleiner RE, et al. A neural m6A/Ythdf pathway is required for learning and memory in Drosophila. Nat Commun. (2021) 12:1458. doi: 10.1038/s41467-021-21537-1
49. Lence T, Akhtar J, Bayer M, Schmid K, Spindler L, Ho CH, et al. m6A modulates neuronal functions and sex determination in Drosophila. Nature. (2016) 540:242–7. doi: 10.1038/nature20568
50. Worpenberg L, Paolantoni C, Longhi S, Mulorz MM, Lence T, Wessels HH, et al. Ythdf is a N6-methyladenosine reader that modulates Fmr1 target mRNA selection and restricts axonal growth in Drosophila. EMBO J. (2021) 40:e104975. doi: 10.15252/embj.2020104975
51. Liu S, Tian H, Xu Y, Wang H. Juvenile hormone regulates silk gene expression by m6A RNA methylation. Cell Mol Life Sci. (2023) 80:331. doi: 10.1007/s00018-023-04996-1
52. Sui X, Klungland A, Gao L. RNA m6A modifications in mammalian gametogenesis and pregnancy. Reproduction. (2023) 165:R1–8. doi: 10.1530/REP-22-0112
53. Hsu PJ, Zhu Y, Ma H, Guo Y, Shi X, Liu Y, et al. Ythdc2 is an N6-methyladenosine binding protein that regulates mammalian spermatogenesis. Cell Res. (2017) 27:1115–27. doi: 10.1038/cr.2017.99
54. Qi M, Sun H, Guo Y, Zhou Y, Gu X, Jin J, et al. m6A reader protein YTHDF2 regulates spermatogenesis by timely clearance of phase-specific transcripts. Cell Prolif. (2022) 55:e13164. doi: 10.1111/cpr.13164
55. Sui X, Hu Y, Ren C, Cao Q, Zhou S, Cao Y, et al. METTL3-mediated m6A is required for murine oocyte maturation and maternal-to-zygotic transition. Cell Cycle. (2020) 19:391–404. doi: 10.1080/15384101.2019.1711324
56. Hongay CF, Orr-Weaver TL. Drosophila Inducer of MEiosis 4 (IME4) is required for Notch signaling during oogenesis. Proc Natl Acad Sci U S A. (2011) 108:14855–60. doi: 10.1073/pnas.1111577108
57. Rockwell AL, Hongay CF. Dm Ime4 depletion affects permeability barrier and Chic function in Drosophila spermatogenesis. Mech Dev. (2020) 164:103650. doi: 10.1016/j.mod.2020.10365
58. Liu C, Cao J, Zhang H, Wu J, Yin J. Profiling of transcriptome-wide N6-methyladenosine (m6A) modifications and identifying m6A associated regulation in sperm tail formation in Anopheles sinensis. Int J Mol Sci. (2022) 23(9):4630. doi: 10.3390/ijms23094630
59. Chow J, Kagan JC. The fly way of antiviral resistance and disease tolerance. Adv Immunol. (2018) 140:59–93. doi: 10.1016/bs.ai.2018.08.002
60. Dillon ME, Lozier JD. Adaptation to the abiotic environment in insects: the influence of variability on ecophysiology and evolutionary genomics. Curr Opin Insect Sci. (2019) 36:131–9. doi: 10.1016/j.cois.2019.09.003
61. Heckel DG. Insect detoxification and sequestration strategies. Annu Plant Reviews: Insect-Plant Interact. (2014) 47:77–114. doi: 10.1002/9781118829783.ch3
62. Teets NM, Gantz J, Kawarasaki Y. Rapid cold hardening: ecological relevance, physiological mechanisms and new perspectives. J Exp Biol. (2020) 223:jeb203448. doi: 10.1242/jeb.203448
63. Xue P, Jiang T, Zhu J, Wang M, Zhao Q, Huang J, et al. Low METTL3 level in midgut of the Bombyx mori inhibit the proliferation of nucleopolyhedrovirus. J Asia Pac Entomol. (2021) 24:42–9. doi: 10.1016/j.aspen.2020.10.015
64. Zhang X, Zhang Y, Dai K, Liang Z, Zhu M, Pan J, et al. N6-methyladenosine level in silkworm midgut/Ovary cell line is associated with Bombyx mori nucleopolyhedrovirus infection. Front Microbiol. (2020) 10:2988. doi: 10.3389/fmicb.2019.02988
65. Halbach R, Junglen S, van Rij RP. Mosquito-specific and mosquito-borne viruses: evolution, infection, and host defense. Curr Opin Insect Sci. (2017) 22:16–27. doi: 10.1016/j.cois.2017.05.004
66. Ye J, Zhang L, Zhang X, Wu X, Fang R. Plant defense networks against insect-borne pathogens. Trends Plant Sci. (2021) 26:272–87. doi: 10.1016/j.tplants.2020.10.009
67. Dai Z, Etebari K, Asgari S. N6-methyladenosine modification of the Aedes aEgypti transcriptome and its alteration upon dengue virus infection in Aag2 cell line. Commun Biol. (2022) 5:607. doi: 10.1038/s42003-022-03566-8
68. Gokhale NS, McIntyre ABR, Mattocks MD, Holley CL, Lazear HM, Mason CE, et al. Altered m6A modification of specific cellular transcripts affects Flaviviridae infection. Mol Cell. (2020) 77:542–55. doi: 10.1016/j.molcel.2019.11.007
69. Lichinchi G, Zhao BS, Wu Y, Lu Z, Qin Y, He C, et al. Dynamics of human and viral RNA methylation during Zika virus infection. Cell Host Microbe. (2016) 20:666–73. doi: 10.1016/j.chom.2016.10.002
70. Williams GD, Gokhale NS, Horner SM. Regulation of viral infection by the RNA modification N6-methyladenosine. Annu Rev Virol. (2019) 6:235–53. doi: 10.1146/annurev-virology-092818-015559
71. Gokhale NS, McIntyre ABR, McFadden MJ, Roder AE, Kennedy EM, Gandara JA, et al. N6-methyladenosine in Flaviviridae viral RNA genomes regulates infection. Cell Host Microbe. (2016) 20:654–65. doi: 10.1016/j.chom.2016.09.015
72. Tian S, Wu N, Zhang L, Wang X. RNA N6-methyladenosine modification suppresses replication of rice black streaked dwarf virus and is associated with virus persistence in its insect vector. Mol Plant Pathol. (2021) 22:1070–81. doi: 10.1111/mpp.13097
73. Hawkins NJ, Bass C, Dixon A, Neve P. The evolutionary origins of pesticide resistance. Biol Rev. (2019) 94:135–55. doi: 10.1111/brv.12440
74. Yang X, Wei X, Yang J, Du T, Yin C, Fu B, et al. Epitranscriptomic regulation of insecticide resistance. Sci Adv. (2021) 7:eabe5903. doi: 10.1126/sciadv.abe5903
75. Wang BB, Lai YF, Li FF, Jiao L, Qiao QX, Li SY, et al. Functional characterization of two RNA methyltransferase genes METTL3 and METTL14 uncovers the roles of m6A in mediating adaptation of Plutella xylostella to host plants. Int J Mol Sci. (2022) 23. doi: 10.3390/ijms231710013
76. Guo Z, Bai Y, Zhang X, Guo L, Zhu L, Sun D, et al. RNA m6A methylation suppresses insect juvenile hormone degradation to minimize fitness costs in response to a pathogenic attack. Adv Sci. (2024) 11:2307650. doi: 10.1002/advs.202307650
77. Hahn DA, Denlinger DL. Energetics of insect diapause. Annu Rev Entomol. (2011) 56:103–21. doi: 10.1146/annurev-ento-112408-085436
78. Jiang T, Li J, Qian P, Xue P, Xu J, Chen Y, et al. The role of N6-methyladenosine modification on diapause in silkworm (Bombyx mori) strains that exhibit different voltinism. Mol Reprod Dev. (2019) 86:1981–92. doi: 10.1002/mrd.23283
79. Chen Y-H, Jiang T, Yasen A, Fan B-Y, Zhu J, Wang M-X, et al. m6A-dependent mevalonate kinase in juvenile hormone synthesis pathway regulates the diapause process of bivoltine silkworm (Bombyx mori). Mol Biol Rep. (2023) 50:5295–306. doi: 10.1007/s11033-023-08489-z
80. Chen Y, Fan B, Yasen A, Zhu J, Wang M, Shen X. YTHDF3 is involved in the diapause process of bivoltine Bombyx mori strains by regulating the expression of Cyp307a1 and Cyp18a1 genes in the ecdysone synthesis pathway. Biomolecules. (2022) 12:1127. doi: 10.3390/biom12081127
81. Suzuki T. The expanding world of tRNA modifications and their disease relevance. Nat Rev Mol Cell Biol. (2021) 22:375–92. doi: 10.1038/s41580-021-00342-0
82. Grosjean H, Sprinzl M, Steinberg S. Posttranscriptionally modified nucleosides in transfer RNA: their locations and frequencies. Biochimie. (1995) 77:139–41. doi: 10.1016/0300-9084(96)88117-X
83. Lin CJ, Smibert P, Zhao X, Hu JF, Ramroop J, Kellner SM, et al. An extensive allelic series of Drosophila kae1 mutants reveals diverse and tissue-specific requirements for t6A biogenesis. RNA. (2015) 21:2103–18. doi: 10.1261/rna.053934.115
84. Rojas-Benitez D, Eggers C, Glavic A. Modulation of the proteostasis machinery to overcome stress caused by diminished levels of t6A-modified tRNAs in Drosophila. Biomolecules. (2017) 7:25. doi: 10.3390/biom7010025
85. Angelova MT, Dimitrova DG, Da Silva B, Marchand V, Jacquier C, Achour C, et al. tRNA 2'-O-methylation by a duo of TRM7/FTSJ1 proteins modulates small RNA silencing in Drosophila. Nucleic Acids Res. (2020) 48:2050–72. doi: 10.1093/nar/gkaa002
86. Kelley M, Uhran M, Herbert C, Yoshida G, Watts ER, Limbach PA, et al. Abundances of transfer RNA modifications and transcriptional levels of tRNA-modifying enzymes are sex-associated in mosquitoes. Insect Biochem Mol Biol. (2022) 143:103741. doi: 10.1016/j.ibmb.2022.103741
87. Esguerra J, Warringer J, Blomberg A. Functional importance of individual rRNA 2’-O-ribose methylations revealed by high-resolution phenotyping. RNA. (2008) 14:649–56. doi: 10.1261/rna.845808
88. Tollervey D, Lehtonen H, Jansen R, Kern H, Hurt EC. Temperature-sensitive mutations demonstrate roles for yeast fibrillarin in pre-rRNA processing, pre-rRNA methylation, and ribosome assembly. Cell. (1993) 72:443–57. doi: 10.1016/0092-8674(93)90120-F
89. Giordano E, Peluso I, Senger S, Furia M. minifly, a Drosophila gene required for ribosome biogenesis. J Cell Biol. (1999) 144:1123–33. doi: 10.1083/jcb.144.6.1123
90. Kauffman T, Tran J, DiNardo S. Mutations in Nop60B, the Drosophila homolog of human dyskeratosis congenita 1, affect the maintenance of the germ-line stem cell lineage during spermatogenesis. Dev Biol. (2003) 253:189–99. doi: 10.1016/S0012-1606(02)00013-1
91. Schmitt-Engel C, Schultheis D, Schwirz J, Ströhlein N, Troelenberg N, Majumdar U, et al. The iBeetle large-scale RNAi screen reveals gene functions for insect development and physiology. Nat Commun. (2015) 6:7822. doi: 10.1038/ncomms8822
92. Schosserer M, Minois N, Angerer TB, Amring M, Dellago H, Harreither E, et al. Methylation of ribosomal RNA by NSUN5 is a conserved mechanism modulating organismal lifespan. Nat Commun. (2015) 6:6158. doi: 10.1038/ncomms7158
Keywords: m6A, epigenetics, insect development, environmental adaptation, reproduction
Citation: Jiao Y and Palli SR (2024) RNA modifications in insects. Front. Insect Sci. 4:1448766. doi: 10.3389/finsc.2024.1448766
Received: 13 June 2024; Accepted: 05 August 2024;
Published: 26 August 2024.
Edited by:
Yolanda H. Chen, University of Vermont, United StatesReviewed by:
Zhaojiang Guo, Chinese Academy of Agricultural Sciences, ChinaCopyright © 2024 Jiao and Palli. This is an open-access article distributed under the terms of the Creative Commons Attribution License (CC BY). The use, distribution or reproduction in other forums is permitted, provided the original author(s) and the copyright owner(s) are credited and that the original publication in this journal is cited, in accordance with accepted academic practice. No use, distribution or reproduction is permitted which does not comply with these terms.
*Correspondence: Subba Reddy Palli, cnBhbGxpQHVreS5lZHU=