- 1Department of Biochemistry, Virginia Polytechnic Institute and State University, Blacksburg, VA, United States
- 2Department of Entomology, University of California Riverside, Riverside, CA, United States
Aedes aegypti, the vector for dengue, chikungunya, yellow fever, and Zika, poses a growing global epidemiological risk. Despite extensive research on Ae. aegypti’s life history traits and behavior, critical knowledge gaps persist, particularly in integrating these findings across varied experimental contexts. The plasticity of Ae. aegypti’s traits throughout its life cycle allows dynamic responses to environmental changes, yet understanding these variations within heterogeneous study designs remains challenging. A critical aspect often overlooked is the impact of using lab-adapted lines of Ae. aegypti, which may have evolved under laboratory conditions, potentially altering their life history traits and behavioral responses compared to wild populations. Therefore, incorporating field-derived populations in experimental designs is essential to capture the natural variability and adaptability of Ae. aegypti. The relationship between larval growing conditions and adult traits and behavior is significantly influenced by the specific context in which mosquitoes are studied. Laboratory conditions may not replicate the ecological complexities faced by wild populations, leading to discrepancies in observed traits and behavior. These discrepancies highlight the need for ecologically relevant experimental conditions, allowing mosquito traits and behavior to reflect field distributions. One effective approach is semi-field studies involving field-collected mosquitoes housed for fewer generations in the lab under ecologically relevant conditions. This growing trend provides researchers with the desired control over experimental conditions while maintaining the genetic diversity of field populations. By focusing on variations in life history traits and behavioral plasticity within these varied contexts, this review highlights the intricate relationship between larval growing conditions and adult traits and behavior. It underscores the significance of transstadial effects and the necessity of adopting study designs and reporting practices that acknowledge plasticity in adult traits and behavior, considering variations due to larval rearing conditions. Embracing such approaches paves the way for a comprehensive understanding of contextual variations in mosquito life history traits and behavior. This integrated perspective enables the synthesis of research findings across laboratory, semi-field, and field-based investigations, which is crucial for devising targeted intervention strategies tailored to specific ecological contexts to combat the health threat posed by this formidable disease vector effectively.
1 Introduction
The global epidemiological risk associated with Aedes aegypti is a significant concern. This invasive mosquito species is a crucial vector for several mosquito-borne viruses causing dengue, chikungunya, yellow fever, and Zika. These viruses are responsible for frequent outbreaks of diseases, leading to morbidity and mortality and substantial economic burdens worldwide. Dengue, in particular, has become a significant public health concern over the past decade, with approximately 3.9 billion people at risk of infection in over 128 countries (1, 2). Dengue incidence in the Americas, Southeast Asia, and the Western Pacific regions surged to approximately 5.5 million in 2020, as reported by the Pan American Health Organization (3). This worrisome upward trajectory has persisted, with 4.6 million dengue cases in the Americas alone in 2023, and over 9.3 million cases already reported as of June 2024 (4). In the United States, where cases were typically associated with international travel, the local transmission of dengue fever was reported in Arizona, California, Florida, Hawaii, and Texas in 2023, raising new challenges for local public health departments. Besides posing health risks, the cumulative economic costs of mitigating Aedes-borne diseases from 1975 to 2020 are estimated at 310.8 billion USD worldwide (5).
Ae. aegypti is the primary vector of dengue, chikungunya, Zika, and yellow fever viruses. Ae. albopictus also serves as a vector for these arboviruses, contributing to their transmission in various regions (6, 7). Since 1920, the estimated global abundance of Ae. aegypti has risen by approximately 9.5%, and future projections indicate a 30% increase by the end of the 21st century (8). By 2080, Ae. aegypti is predicted to be reported in as many as 162 countries, including countries documenting their presence for the first time (9).
Given these escalating risks, understanding the adaptability of Ae. aegypti to changing conditions becomes paramount for understanding its success as one of the most invasive mosquito species. Adaptability in Ae. aegypti encompasses genetic variation and phenotypic plasticity, each playing crucial roles in the mosquito’s ability to respond to environmental changes. Genetic variation provides the raw material for natural selection, enabling populations to evolve over time (10, 11). Influenced by larval environments, phenotypic plasticity allows individual mosquitoes to adjust their traits in response to immediate conditions. Moreover, plasticity can evolve within populations over time, potentially interacting with genetic differences. This means that the degree of genetic differentiation underlying various traits can vary within a population, leading to intrapopulation diversity in plastic responses that enhance adaptability (12, 13).
The plasticity of traits throughout their life cycle allows them to respond dynamically to environmental changes (14, 15). However, despite the acknowledged importance of adaptations and trait plasticity in Ae. aegypti, significant gaps persist in how we investigate and perceive behavior and life history trait variations, given the contextual complexity arising from heterogeneous study design and methodology. It is important to note that not all plastic responses are adaptive; some may arise from physiological or environmental constraints (16). Understanding genetic and plastic contributions to adaptability provides a comprehensive view of how Ae. aegypti can thrive in diverse environments.
While this review primarily focuses on Ae. aegypti, it is essential to consider insights from studies on other mosquito species to understand phenotypic variation and adaptability comprehensively. For instance, studies on Ae. albopictus and other mosquito species have highlighted similar adaptive responses to environmental pressures, suggesting broader patterns that can inform our understanding of Ae. aegypti (7). These comparisons can reveal fundamental principles of mosquito biology and adaptation, enhancing our ability to predict and manage vector populations.
This review highlights such knowledge gaps, specifically leveraging findings across laboratory, semi-field, and field-based investigations (17). By integrating insights from various Aedes species and other mosquitoes, this review emphasizes the importance of adopting study designs and reporting practices that acknowledge plasticity in adult behavior while also considering variation arising from differences in larval and adult traits due to larval growing conditions, also referred to as transstadial effects. Embracing such approaches paves the way for a comprehensive understanding of contextual variation in mosquito life history traits and behavior. This integrated perspective enables the synthesis of research findings across different study contexts, ultimately improving our capacity to devise targeted intervention strategies in the field tailored to specific ecological contexts to effectively combat the health threat posed by this formidable disease vector.
2 Variation in life-history traits
Ae. aegypti exhibits considerable variation in life history traits, contributing to its adaptability and vector potential. This review focuses on several key life history traits, including longevity, fecundity, adult body size, age of reproduction, and reproductive effort. These traits are influenced by both genetic and environmental factors, with phenotypic plasticity playing a crucial role in the mosquito’s adaptability. Understanding these variations is crucial because they provide insights into the mechanisms driving the adaptability and invasiveness of Ae. aegypti.
Ae. aegypti is a globally distributed and highly variable species, with significant variation in traits both within and among populations. Differences in genetic composition and environmental conditions across various geographical regions can lead to substantial differences in traits such as fecundity, longevity, development time, and vector competence (18, 19). This intra-species variability influences how different populations respond to environmental pressures and control measures, and it can affect disease transmission dynamics and invasion potential (20).
Mosquito life history traits and behavior have been well studied across multiple species (Figure 1A). Among these studies, population-specific variation in traits have been documented, showing that mosquitoes from different regions exhibit varying levels of insecticide resistance, which can impact the effectiveness of control strategies (21). Additionally, the genetic diversity within populations can influence their capacity to adapt to new environments, making some populations more successful invaders than others (22). Therefore, it is essential to consider genetic and environmental factors when studying the life history traits and vector potential of Ae. aegypti.
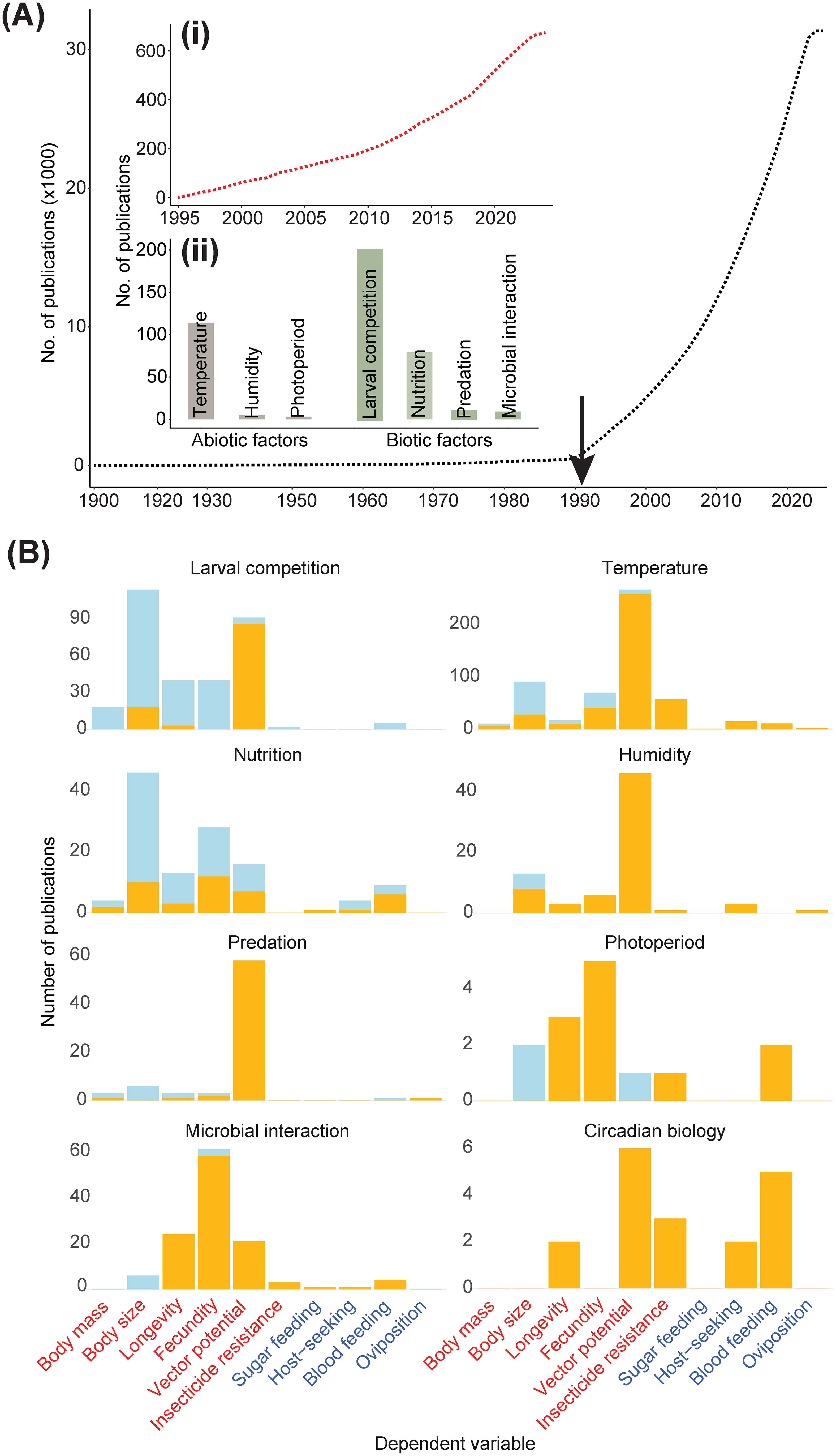
Figure 1. Trends in Mosquito Research Publications: (A) The cumulative number of publications from 1900 to 2024 focusing on life history traits and behavior of all mosquito species. The black arrow indicates the first description of the influence of transstadial effects. Insets: (i) Cumulative number of publications until 2024 specifically focused on Ae. aegypti traits and behavior. (ii) Cumulative number of publications investigating the impact of specific abiotic and biotic factors on Ae. aegypti life history and behavior. (B) A visualization of the literature trend investigating the effects of abiotic and biotic factors (independent variable) on larval and adult traits and behavior across all mosquito species. The bar plots in the left column represent the data on the number of studies investigating the effects of five biotic (larval competition, nutrition, predation, microbial interaction, circadian biology) and three abiotic factors (temperature, humidity, photoperiod) across all mosquito species. Along the X-axis are the most commonly investigated dependent variables; names denoted in red and blue denote adult traits and adult behavior, respectively. Yellow-shaded bars denote studies that have not considered the influence of transstadial effects on adult traits and behavior stemming from larval growing conditions. Blueshaded bars denote studies that have factored in transstadial effects. Data source: Clarivate Web of Science.
Several studies have documented adaptive plasticity in life-history traits, resistance to desiccation and insecticides, preference towards urban environments, and degree of anthropophily. However, the influence of environmental and physiological factors (i.e., context-specificity) in shaping the variations in these plastic traits (i.e., the direction and magnitude of effects) needs more attention. Many studies have investigated the influence of temperature on plasticity in life history traits, so we will discuss these findings considering the abundance of available data (Figure 1B). Additionally, we will discuss context-specificity in neuroethological studies because sensory processes involved with host detection and location have been extensively studied.
Ae. aegypti predominantly thrives in habitats with temperatures ranging from 18°C to 38°C, with the median temperature ranging between 25°C and 32°C (23, 24). While they are also found in much colder and warmer habitats, temperatures between 18°C and 38°C facilitate their complete metamorphosis, survival, and reproduction. These temperatures account for approximately two-thirds of their current geographical range (25, 26). Not surprisingly, the poleward shifts in their global distribution are predicted to covary strongly with mosquitoes’ adaptations to more extreme temperatures (26, 27).
The relationship between temperature and life history traits in Ae. aegypti is complex and often nonlinear. Many researchers view traits such as egg viability and larval survival as having optimal temperature ranges where the traits are maximized, with reduced viability and survival at temperature extremes (28, 29). For instance, egg viability typically peaks at intermediate temperatures and decreases at both lower and higher extremes (30). Similarly, larval survival rates tend to be highest within a moderate temperature range and drop off at temperatures outside this range, reflecting a nonlinear response (31). It is essential to recognize that temperature effects on most life history traits are better described by nonlinear or non-monotonic relationships, with trait performance often peaking at optimal temperatures and declining at suboptimal extremes.
While development rate is one of the few traits that might exhibit a more linear relationship with temperature within a limited range, even this relationship can become nonlinear at higher temperatures where development may fail due to mortality (32). The slope of this linear relationship corresponds to the cumulative effect of temperature variations on the development rate, and the intercept represents the theoretical temperature at which development ceases to occur, also known as the developmental zero (32, 33). However, empirical data from multiple studies suggests that this linear relationship is likely only true for mosquitoes developing within the median temperature range, i.e., 25°C and 32°C (34).
Outside the median temperature range, as temperatures approach the warmer or colder extremes, the magnitude of temperature-mediated effects scale non-linearly per unit change in temperature. For example, the egg hatch rates exhibited a non-linear decline with rising temperature, decreasing to 1.6% at 35°C. Similarly, lower temperatures also have had a non-linear impact on egg hatchability, albeit with a lesser magnitude of decline compared to higher temperatures: from 72% at 20°C to 55% at 18°C, 60% at 16°C, 53% at 14°C, and 43% at 12°C (30). Likewise, larval rearing at 27°C resulted in a pupation rate of 98.5% seven days post egg hatch, but this decreased to 97.2%, 87.4%, and 74.2% at 30°C, 33°C, and 35°C, respectively (35).
Even within the median temperature range, the relationship is often nonlinear when the effects of temperature have been studied in interaction with other environmental factors. For instance, larval competition and resource availability affect the temperature dependence of Ae. albopictus’s fitness (36). In particular, in resource-scarce or high-competition environments, the temperature facilitating optimal development and fitness drops by ~6°C. Furthermore, these interactive effects result in a ~10°C reduction in the width of Ae. aegypti’s thermal niche, i.e., the range of temperatures that facilitates the species’ survival and reproduction (37). To better visualize this context, Figures 2A–C presents a hypothetical illustration highlighting the differences between modeling the environment-trait-fitness relationship as linear versus nonlinear. Figure 2A depicts a classical linear environment-trait relationship. Figure 2B illustrates the nonlinear relationship, as discussed in the examples above on temperature-mediated effects on life history traits. Figure 2C shows how these environmentally-mediated trait variations shape mosquito fitness.
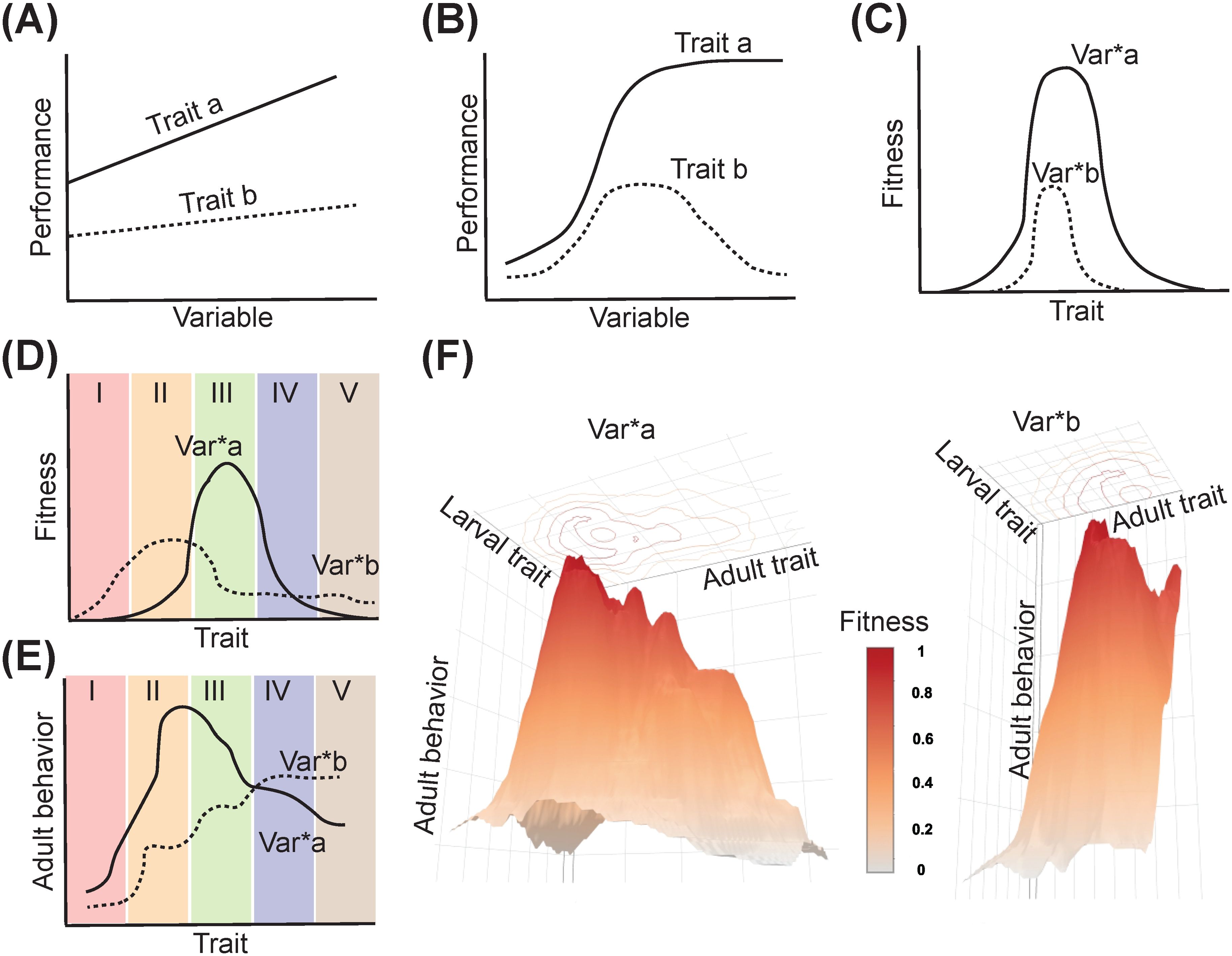
Figure 2. Adaptive trait-environment relationships in Ae. aegypti: (A) Classic representation of trait plasticity in response to environmental variables, (B) Representation of non-linear relationships between traits and environmental variables, (C) Influence of non-linear trait-environment relationships on mosquito fitness, (D, E) Existing methods fail to consider the covariation in larval and adult traits and its impact on adult behavior and fitness within the framework of environment-trait relationships. Sections I-V illustrate hypothetical segments of the overall trait distribution and demonstrate how sampling only a subset of this distribution affects the interpretation of relationships between fitness, adult behavior, and life-history traits, (F) A comprehensive framework that visualizes the data in (D, E) by depicting the environment-trait-fitness relationship while accounting for the covariations in larval and adult traits influenced by transstadial effects. This visualization assumes a 1:1 correlation between environment-trait variables and fitness; therefore, the color gradient mirrors the 3D surface. However, this correlation may vary across specific experiments. Asterik (*) symbol in (C–F) denote the interaction between two variables.
In addition, the interactions between temperature and relative humidity, together with variation in adult body size, strongly correlate with the longevity of adult Ae. aegypti mosquitoes (38). Low resource larval environments at 26.4°C resulted in females with shorter lifespans (6.9 days) compared to larval environments with similar resource availability at 30.1°C (10.7 days) and 35.1°C (8.5 days) (38). Similarly, the time taken to reach pupation decreased progressively, exhibiting a non-linear decline, with durations of 21.97 days at 15.2°C, 14.46 days at 17.9°C, 9.83 days at 21.6°C, and 8.67 days at 25.3°C (39). These non-linear temperature-mediated effects highlight the complexity of Ae. aegypti’s response to temperature fluctuations and suggest potential analogous trends in other life-history responses to various biotic and abiotic factors.
In the context of reproduction, higher temperatures lead to reduced egg production in Ae. aegypti females, reduced the latency to oviposition, and altered oviposition patterns. For instance, at 25°C and 80% humidity, Ae. aegypti females lived twice as long and produced 40% more eggs than at 35°C and 80% humidity. At high temperatures and high humidity, mosquitoes survived less and produced fewer eggs. At 35°C and 60% humidity, only 15% of females laid more than 100 eggs, and 45% of the females did not oviposit any eggs. Egg fertility also decreased with rising temperatures at lower humidity levels (40). The interaction between temperature and humidity plays a crucial role in the survival of eggs. High humidity levels enhance egg viability and hatching rates at optimal temperatures, while low humidity leads to desiccation and reduced viability, even if the temperature is within a favorable range (41). This interaction highlights the complexity of environmental factors affecting mosquito life history traits. Moreover, the magnitude of any larval environmental effect on adult traits differs between male and female mosquitoes due to protandry, whereby female mosquitoes exhibit a slower growth rate than their male counterparts (42, 43). Consequently, females spend more time in larval habitats, rendering the quality of larval growing conditions significantly more influential on female larval and adult traits than males. For example, suboptimal larval growing conditions generally lead to higher female mortality rates and a skewed sex ratio towards males (43). Density-dependent female larval mortality is also a critical determinant of adult body size and other traits (44). Thus, evaluating plasticity in mosquito traits requires a sex-specific approach.
Understanding the distribution of life history traits is essential for comprehending the biological and ecological factors contributing to Ae. aegypti’s success as an invasive species and a vector. Longevity and fecundity, for example, are directly related to the mosquito’s ability to sustain and spread infections over time (45). Variation in the age of reproduction can lead to differences in generation time, affecting how quickly populations can grow and adapt to new environments (46). Adult body size is another critical factor influencing mosquitoes’ survival, fecundity, and vector competence (23). Larger adult mosquitoes generally have higher fecundity and longer lifespans, making them formidable disease vectors (1). However, body size is highly influenced by larval rearing conditions, such as temperature, food availability, and density. For example, larval competition and limited resources can lead to smaller adult sizes, which may reduce individual survival and reproductive success (47). Notably, these factors can result in similar phenotypic outcomes through different mechanisms. The reproductive effort reflects the balance between the number of offspring produced and the investment in each offspring’s quality, significantly impacting population dynamics and resilience (1). These life history traits are influenced by both genetic and environmental factors, with phenotypic plasticity playing a crucial role in how Ae. aegypti adapts to varying conditions (14, 43). Therefore, deciphering the interplay between these environmental factors and life history traits enhances our comprehension of the complex biology of Ae. aegypti and sheds light on the adaptive mechanisms that make them such formidable disease vectors.
3 Genetic variation and phenotypic plasticity
While phenotypic plasticity plays a crucial role in the adaptability of Ae. aegypti, it is essential to recognize the genetic components underlying these traits. Phenotypic traits such as host choice, behavior, and adult size exhibit significant genetic variation, which interacts with environmental factors to shape the observed phenotypic outcomes (48, 49). This interaction between genetic variation and phenotypic plasticity is pivotal for understanding the adaptability and vector potential of Ae. aegypti (15, 50).
Host choice exemplifies a trait influenced by both genetic and environmental factors. Studies have shown that Ae. aegypti’s preference for human hosts has a strong genetic basis, with certain populations exhibiting innate tendencies towards anthropophily (51). Specific genetic loci associated with the preference for human odors underscore the genetic underpinnings of this behavior. However, environmental conditions, such as the availability of hosts and habitat characteristics, also modulate this preference, showcasing epigenetic plasticity.
Behavioral traits, including feeding and oviposition behaviors, also exhibit genetic variation. For instance, the genetic differentiation between sylvatic and domestic forms of Ae. aegypti influences their behavior and habitat preferences (52). Sylvatic populations tend to feed on a broader range of hosts and oviposit in natural habitats, while domestic populations strongly prefer human hosts and artificial containers for oviposition. These inherent genetic differences are further influenced by environmental factors, such as the availability of breeding sites and host density, leading to context-specific behavioral adaptations.
Adult size is another trait where genetic variation and phenotypic plasticity intersect. Body size is determined by both genetic factors and larval rearing conditions, such as temperature and resource availability (53). Genetic differences between populations can result in varying growth rates and adult size at emergence (15). Heritability plays a significant role in determining adult body size, yet environmental factors like larval density and nutrition levels also induce plastic responses, affecting size-related traits such as longevity and fecundity. This interaction between genetic predisposition and environmental conditions underscores the complexity of size variation and its implications for vector competence (15).
Understanding the interplay between genetic variation and phenotypic plasticity is crucial for predicting how Ae. aegypti populations might respond to changing environments (49). This knowledge is vital for devising effective vector control strategies, as both genetic adaptation and plastic responses can influence the success of interventions. For example, genetic differences in insecticide resistance can interact with environmental factors, such as exposure levels and habitat characteristics, to shape resistance dynamics (48). Recognizing the contributions of both genetic variation and phenotypic plasticity will enhance our ability to anticipate and manage the evolutionary responses of Ae. aegypti to control measures.
4 Behavioral plasticity
The success of Ae. aegypti as an invasive species is largely attributed to its anthropophilic behavior, with a strong preference towards human hosts for blood meals. While its host-seeking behavior has been extensively studied, its sugar-feeding habits and preferences, crucial for metabolic sustenance, remain relatively unexplored (54). Given the exclusive sugar-feeding diet of males and the importance of carbohydrates for females’ metabolism, this is a crucial contributor to the species’ invasion potential (55). Despite Ae. aegypti mosquitoes predominantly inhabiting human-dominated areas, it is essential to acknowledge the existence of known sylvatic populations that defy this trend and also feed on non-human hosts (52).
The variation between sylvatic and domestic forms of Ae. aegypti is a key example of both genetic differentiation and behavioral plasticity. Sylvatic Ae. aegypti primarily inhabit forested areas and utilize natural water containers for breeding, while domestic Ae. aegypti thrive in urban environments, breeding in artificial containers and frequently entering human dwellings. Multiple studies provide substantial evidence for a genetic basis underlying the plasticity in mosquito behavior (51, 56–58). These studies demonstrate that genetic differences are responsible for variations in behaviors such as host preference, habitat selection, and oviposition site choice. In addition to genetic differentiation, both Ae. aegypti and Ae. albopictus exhibit considerable flexibility in their feeding behaviors, which further reflects their adaptability to varying environmental conditions. For instance, while longevity typically decreases in the absence of sugar, fecundity can actually increase when females rely solely on human blood without sugar supplementation (59). This plasticity in feeding and reproductive strategies underscores the adaptability of these species, allowing them to thrive under different environmental pressures. Such flexibility is a testament to the complex interplay between diet, genetic predispositions, and life history traits that enable these mosquitoes to exploit a wide range of ecological niches. This combination of genetic factors and behavioral plasticity is central to the success of Ae. aegypti in invading tropical and subtropical regions. The inherent genetic differences between sylvatic and domestic forms are a result of their adaptation to specific environments, while behavioral plasticity enables them to respond dynamically to varying habitat conditions.
To identify and locate their hosts, Ae. aegypti females rely on the synergistic integration of sensory cues, including olfactory, visual, thermal, and gustatory cues (60). Olfactory cues and carbon dioxide facilitate long-range attraction, and other sensory cues enable medium-to-short-range attraction. Finally, thermal cues are primarily effective at short distances since temperature gradients created by convection around a human host diminish quickly (61–63). While there is a consensus on the relative significance of these sensory cues, the contribution of thermal cues in host-seeking behaviors is still debated, most likely due to contextual differences in the experimental paradigms used in studies. For example, some studies found that the attraction of Ae. aegypti to thermal cues depend on their ability to sense CO2 (64), while others showed that their attraction to thermal cues could also occur independently of CO2 (61). In the absence of CO2, the sensory integration of thermal and chemosensory cues (host volatiles) likely drives the host-seeking behavior (65). Nevertheless, there is evidence that thermal cues alone (specifically convective but not radiative heat) are sufficient for females to locate heat sources in the range of potential hosts’ temperature (66).
In the context of long-range attraction, the response to CO2 predominantly drives host-seeking and reduces the response threshold to human-derived odors. Ae. aegypti’s preferences for visual cues of specific wavelengths are also gated by the detection of CO2 (60). However, within a short range to the human host, orientation and landing behaviors are mediated by olfactory cues, not CO2 or a co-located visual cue (67, 68). Further, several hundred human-derived chemoattractants have been identified (69, 70). The valence of these chemoattractants can vary significantly, both in isolation and in synergistic combinations, and these variations are influenced by physiological and environmental factors (51, 71, 72).
While host-seeking is typically regarded as a female mosquito trait, Ae. aegypti males also display attraction to humans (73, 74). Mature males are particularly drawn to human-derived chemosensory cues, which drive their swarming behavior in pursuit of potential mates and contribute to their mating success in natural populations (66, 75). Amidst debates on mosquitoes’ attraction to sensory cues and multimodal sensory integration, responses to environmental cues in Ae. aegypti are often interpreted as phenotypic plasticity, where organisms modify their behavior or traits in response to changing environmental conditions (13). For instance, variations in host-seeking behavior can be influenced by larval rearing conditions, indicating plastic responses to environmental cues (23). However, some responses are considered innate, meaning they are hardwired into the organism’s genetic makeup and are not easily altered by the environment (51). These innate responses can still exhibit context-specific variations, as the expression of innate traits can be modulated by environmental factors (76). Thus, distinguishing between plastic and innate responses requires careful consideration of both genetic and environmental influences (77). Unfortunately, many studies overlook this context specificity, focusing on mechanistic effects under controlled conditions. While mechanistic insights are valuable, the broader relevance of these mechanisms in real-world contexts is needed to bridge findings from the lab to their applicability in field conditions.
Extrinsically, factors like temperature, humidity, and photoperiod significantly shape mosquito behavior. As poikilotherms, the ambient environmental temperature dictates mosquitoes’ body temperature and activity. Warmer temperatures, for example, increase their metabolism, leading to heightened activity and increased sugar feeding from plants to meet their nutritional needs (78). Besides elevated activity levels, temperature influences the sensitivity to chemosensory cues as Ae. aegypti females were more attracted to CO2 when tested at 30°C compared to 20°C and 25°C (79). Electrophysiological recordings indicate that odorant-specific changes in antennal sensitivity to odors mediate this effect of temperature on olfactory behavior (79). The differences in response to odorants could also be partly due to temperature-induced alterations in the characteristics of odorant compounds. Indeed, one can expect temperature changes to affect the chemicals’ partial vapor pressure, impacting their diffusion and subsequent interaction with odorant binding receptors in the chemosensory organs of Ae. aegypti (80, 81).
Intrinsically, as observed in many other insect models, the olfactory sensitivity of Ae. aegypti varies with the time of day (82), with several genes involved in olfactory processes being regulated by the mosquito’s circadian clock (83, 84). Furthermore, sleep deprivation detrimentally affects both host-seeking and blood-feeding behaviors in Ae. aegypti, potentially linked to alterations in time-dependent olfactory sensitivity (85). It is thus critical to synchronize mosquitoes to test behavioral and physiological responses to host cues in the proper (or most relevant) temporal context. It is also essential to report temporal information in publications as the norm. Overall, the physiological state of the insect (e.g., age, reproductive status, feeding state, chronobiology, sleep patterns, prior experience, etc.) significantly affects its responses to resource-associated cues (86). For example, older females, who typically exhibit a higher propensity for seeking hosts, display increased sensitivity to CO2 compared to their younger counterparts in the first few days post-emergence. (87). Additionally, mating and blood-feeding suppress host-seeking behavior, with a return to baseline levels after oviposition (88–90).
Altogether, this underscores the importance of considering the interplay between mosquitoes’ physiology and behavior. Overall, extrinsic and intrinsic factors modulate mechanisms at peripheral and central levels to drive behavioral variation tailored to different physiological and ecological contexts.
5 Using transstadial effects to navigate contextual complexity in studying life history and behavior
The variation in life history traits and behavior of Ae. aegypti described in the literature are primarily influenced by decisions made by experimenters, i.e., the study design employed to quantify traits. While simple experimental designs offer more explicit contexts, their capacity to fully capture the breadth of variation in mosquito life history and behavior is debatable. On the other hand, complex study designs reporting multivariate and interactive effects encounter challenges in discerning the relative contribution of each independent variable to the magnitude and direction of observed effects on traits and their underlying distribution (91). These studies also hinder experimenters’ ability to dissect the mechanistic underpinnings of the observed behaviors (49). This complexity arises when multiple experimental variables are manipulated simultaneously, potentially interacting in ways that obscure context-dependent effects unless addressed explicitly in the study design (92–94).
Numerous studies have explored the impact of larval competition and nutrition on adult mosquito body size at emergence. Typically, heightened larval competition and lower nutrition reduce per capita resource availability, resulting in smaller adult mosquitoes (95, 96). Consequently, a diverse range of mosquito size distributions resulting from varying levels of larval competition and nutrition have been reported in the literature (43, 97–99). However, it remains challenging to determine if these varying size distributions across studies, often overlapping, genuinely reflect the ecologically relevant limits (i.e., in larval density and food availability). To address this concern, some studies integrate field-derived preliminary data on larval density or food availability in natural habitats, ensuring that experimental variables are manipulated within ecologically relevant bounds (100, 101). Others employ experimental variables that may not strictly adhere to these limits but conduct standardization trials under controlled conditions to establish the range within which variables can adequately capture a trait’s distribution (102, 103). When manipulated individually, varying larval densities and food levels could still produce adults with similar size distributions, although due to different physiological responses. Nevertheless, more needs to be understood about whether these adults, despite their similar size distribution, share similarities in their physiological, behavioral, and life history characteristics. When these variables interact, untangling their influence on adult size distribution becomes complex and challenging. Nonetheless, these intricacies are frequently disregarded in many studies, highlighting the significance of interpreting effect sizes on mosquito traits and behavior while considering the distribution’s shape, especially at the tails, where sampling efforts may be limited. These gaps in approach will likely impact the perceived relationship between environmental factors and life history traits, the extent of variation, and the plasticity window for those traits (Figures 2D, E).
It is important to consider whether the source of size variation, or any other phenotypic variation, influences how these traits relate to fitness and disease transmission. Different sources of variation, whether genetic, physiological, or environmental, might have distinct effects on these outcomes. Investigating these differences could provide valuable insights into the adaptive strategies of Ae. aegypti. This question merits further exploration in the context of understanding vector competence and developing effective control strategies. For example, resource limitation and competition during larval development often result in smaller adults, which can exhibit greater susceptibility to several arboviruses and, in some cases, higher oral transmission rates (47, 104). Conversely, other studies have found that smaller individuals from high-density rearing conditions may have reduced vector competence (105, 106). Additionally, research on Ae. albopictus has indicated that higher temperatures during larval development can produce smaller adults with lower susceptibility to some arboviruses.
Transstadial effects, such as resource limitation, fluctuations in temperature and humidity, predator presence, parasites and several other factors, during larval development, significantly influence the adult size of mosquitoes, primarily through phenotypic plasticity. As previously noted, these factors may lead to similar phenotypic outcomes through different mechanisms. However, the role of selection among individuals with inherent genetic differences in size in shaping adult size distributions under various larval growing conditions has been less frequently considered. Additionally, size distributions can vary among populations due to inherent genetic differences (107). Future research investigating the specific contributions of genetic and environmental factors to mosquito size variation, mediated by transstadial effects, is crucial for understanding their impact on fitness traits and vector competence in Ae. aegypti and other mosquitoes.
Multiple studies have revealed intriguing trends linking variation in temperature with the host-seeking behavior of female Ae. aegypti. However, a crucial aspect is often overlooked: mosquitoes’ complex life history and the influence of larval growing conditions that cascade to modulate adult traits and behavior (43, 76, 108, 109). Temperature-driven variation in host-seeking behavior have often been reported during behavioral studies (110, 111). The complex life cycle of Aedes mosquitoes involves distinct habitats for larvae and pupae versus adults, which often results in them experiencing different thermal environments. Larvae and pupae are confined to aquatic environments, typically in small volumes of water (single containers, tree holes, etc.), with a narrow thermal range during their development. In contrast, adult females are highly mobile and traverse various aerial and terrestrial environments, exposing them to a wide range of microclimatic conditions as they seek sugar sources, hosts for blood meal, oviposition, and resting sites (112, 113).
Given these differing thermal exposures, it is essential to consider how transstadial effects might influence sensory and behavioral responses. The thermal mismatch or match between larval and adult environments could significantly impact adult mosquitoes’ phenotypic traits and behaviors. For example, a stable larval thermal environment might lead to different developmental outcomes compared to the variable thermal exposures experienced by adults, affecting their survival, reproduction, and vector competence (28 and 114–116). Studies have shown that discrepancies in thermal environments between life stages can alter adult behavior and physiology, emphasizing the need for experimental designs to account for these differences (114). Therefore, the thermal history of both larval and adult stages is crucial for accurately interpreting the effects of temperature on mosquito biology and behavior. This consideration helps ensure that experimental results are ecologically relevant and reflective of the natural conditions experienced by mosquitoes throughout their life cycle. However, the adult mosquitoes used in most behavioral studies often originate from larval environments chosen primarily for optimizing the yield of colonies, needing more alignment with the experimental context. (64, 117). Also, it is essential to note that most behavioral studies need to accurately report the larval growing conditions, which hinders contextualizing the reported effect sizes on adult behavior.
Laboratory studies often focus on controlled conditions to ensure repeatability and reliability of results. However, these studies might not fully capture the complexity and variability of mosquito behavior in the wild. For instance, despite artificial blood feeding over many generations, laboratory strains of Ae. aegypti maintain a strong preference for human hosts, demonstrating that some behaviors are robust and persist even under artificial rearing conditions (19, 56, 118). Nevertheless, many other behaviors and responses might be context-specific and influenced by the natural environment (e.g., circadian rhythms, activity patterns, etc.), which laboratory settings fail to replicate completely (119). Therefore, a hypothetical framework is needed to interpret the environment-trait-fitness relationship, considering the covariations in larval and adult traits influenced by transstadial effects (Figure 2F). This framework emphasizes the importance of visualizing the covariations in larval and adult traits, considering the influences of independent variables across developmental stages along the two axes. Such an approach is crucial for accurately interpreting their effects on adult behavior (third axis) and its implications for mosquito fitness (fourth dimension). By accounting for these relationships between environmental variables, transtadially-mediated trait variation and adult fitness, we can better contextualize experimental findings and highlight their ecological relevance.
Furthermore, adult females selected for behavioral assays from laboratory colonies may exhibit trait distributions specific to their rearing conditions, such as larval crowding, feeding regime, temperature, and humidity (120–124). As described in several studies’ methodology, the typical “standard larval rearing condition” often does not yield adult Aedes mosquitoes representing the full spectrum of their trait distributions for use as subjects in behavioral experiments (28, 32, 125–127). Critically, this limitation extends beyond laboratory-reared mosquitoes to field-collected mosquitoes utilized in laboratory and semi-field experiments (128, 129). In such instances, the comprehensive trait distribution is frequently disregarded, resulting in the unintentional selection of mosquitoes with a subset of trait values or characteristics as experimental subjects. Due to the strong covariation between life-history traits and several adult behaviors, studies conducted with these mosquitoes, representing only a subset of the overall trait distribution, may only incompletely capture the variation associated with a specific behavioral repertoire. This limitation not only narrows the range of contexts in which study results can be interpreted but also affects the magnitude and direction of reported effect sizes, presenting challenges for reproducibility and generalization to broader contexts.
6 Discussion
From a vector-borne disease control perspective, studies on mosquito life history traits and behavior aim to elucidate their significance in influencing vector potential, insecticide resistance, and invasion potential. This review highlights how neglecting context-specific effects significantly undermines the accuracy of the relationships quantified between experimental variables. By integrating findings from studies on other species, we can draw broader conclusions and identify patterns that may apply across mosquitoes. For example, similar phenotypic plasticity and adaptability mechanisms observed in Ae. albopictus, another important vector species, can provide comparative insights that enhance our understanding of Ae. aegypti. Such cross-species comparisons are particularly valuable for identifying generalizable principles of mosquito biology and vector management, which can inform strategies to control multiple species simultaneously.
Moreover, considering genetic and phenotypic variation across mosquito species helps us recognize the evolutionary pressures and environmental factors shaping these traits. This understanding is crucial for anticipating how mosquito populations may respond to environmental changes, such as climate variability, urbanization, and habitat modification. For instance, the genetic diversity within populations can influence their capacity to develop insecticide resistance, necessitating the development of dynamic and adaptable control measures. Additionally, recognizing the role of phenotypic plasticity in facilitating rapid adaptation to new environments can help predict and mitigate the spread of invasive mosquito species.
The lack of context specificity in reported effects, along with difficulties in experimentally quantifying population-specific variation in mosquito traits and behavior, complicates the parameterization of data for predicting mosquito demography, distribution, and disease transmission dynamics (44, 49, 130–132). For instance, the impact of larval rearing conditions on adult traits is often studied using laboratory-bred strains under controlled laboratory conditions, which may not accurately reflect the variability encountered in natural settings. This discrepancy can lead to over- or underestimation of the effects of environmental factors on mosquito populations.
Unfortunately, interactive effects are frequently viewed as epistemically precarious due to their variability, sometimes leading to the belief that observed effect sizes are unpredictable (133–136). This perspective stems from the challenge of isolating specific variables in multifactorial experiments and the inherent complexity of ecological interactions. However, this review stresses that while interactive effects vary significantly across contexts, their variability does not imply unpredictability. With appropriate study design measures established a priori, or at least detailed, a posteriori reporting of experimental methods, their variability across contexts can be systematically studied, allowing knowledge to be rigorously extrapolated. Incorporating field-derived data into laboratory experiments is one such approach, enabling researchers to better simulate natural conditions and account for context-specific effects.
There are, however, notable exceptions to this issue. Some studies have successfully accounted for interactive effects, offering valuable insights into mosquito life-history traits. For example, Carrington et al. (2013) critically analyzed the effects of fluctuating daily temperatures on Ae. aegypti, emphasizing the interaction between mean temperatures and temperature fluctuations. Similarly, Muturi et al. (137) examined the interactive effects of temperature and insecticide exposure on the life-history traits of Culex restuans and Ae. albopictus, providing a nuanced understanding of how these factors together influence development time, survival, and adult size. Additional studies by Alto and Juliano (138) on temperature and larval density and Yeap et al. (139) on temperature and Wolbachia infection further illustrate how these environmental factors jointly shape mosquito traits and vector competence. These examples highlight the importance of considering interactive effects in mosquito research to avoid misinterpretation of biological outcomes and to ensure the ecological validity of laboratory findings.
By addressing these interactive effects in mosquito research, we can fully leverage the extensive knowledge gained from laboratory and semi-field studies, which outnumber field-based studies, to apply these findings effectively in field contexts. This integration is essential for developing accurate mosquito behavior and population dynamics models, which are critical for predicting or, eventually, mitigating vector populations and vector-borne diseases. Furthermore, understanding the interaction between genetic variation and phenotypic plasticity can help identify potential targets for genetic modification or biological control strategies, offering new avenues for disease prevention.
Overall, this review underscores the necessity of a holistic approach incorporating genetic, phenotypic, and environmental factors to understand mosquito ecology comprehensively. Such an approach will enhance our ability to develop robust, context-sensitive interventions that can adapt to the dynamic nature of mosquito populations and the environments they inhabit.
Author contributions
CV: Conceptualization, Data curation, Formal analysis, Investigation, Visualization, Writing – original draft, Writing – review & editing. KC: Conceptualization, Data curation, Formal analysis, Investigation, Visualization, Writing – original draft, Writing – review & editing.
Funding
The author(s) declare that no financial support was received for the research, authorship, and/or publication of this article.
Conflict of interest
The authors declare that the research was conducted in the absence of any commercial or financial relationships that could be construed as a potential conflict of interest.
Publisher’s note
All claims expressed in this article are solely those of the authors and do not necessarily represent those of their affiliated organizations, or those of the publisher, the editors and the reviewers. Any product that may be evaluated in this article, or claim that may be made by its manufacturer, is not guaranteed or endorsed by the publisher.
References
1. Brady OJ, Gething PW, Bhatt S, Messina JP, Brownstein JS, Hoen AG, et al. Refining the global spatial limits of dengue virus transmission by evidence-based consensus. PloS Negl Trop Dis. (2012) 6:e1760. doi: 10.1371/journal.pntd.0001760
2. World Health Organization. Dengue and severe dengue (2023). Available online at: https://www.who.int/news-room/fact-sheets/detail/dengue-and-severe-dengue (Accessed June 23, 2024).
3. Ryff KR. Epidemiologic trends of dengue in US territories 2010–2020. MMWR. Surveillance Summaries. (2023) 72:1–11.
4. Pan American Health Organization, World Health Organization. Epidemiological Update: Increase in dengue cases in the Region of the Americas. Washington, D.C: PAHO/WHO (2024).
5. Roiz D, Pontifes P, Jourdain F, Diagne C, Leroy B, Vaissière AC, et al. The rising global economic costs of Aedes and Aedes-borne diseases. Research Square (2023). Available online at: https://www.doi.org/10.21203/rs.3.rs-2679030/v1 (Accessed March 08, 2024). Preprint.
6. Gratz NG. Critical review of the vector status of Aedes albopictus. Med Veterinary Entomology. (2004) 18:215–27. doi: 10.1111/j.0269-283X.2004.00513.x
7. Kraemer MUG, Sinka ME, Duda KA, Mylne AQN, Shearer FM, Barker CM, et al. The global distribution of the arbovirus vectors Aedes aegypti and Ae. albopictus. eLife. (2015) 4:e08347. doi: 10.7554/eLife.08347
8. Liu-Helmersson J, Rocklöv J, Sewe M, Brännström Å. Climate change may enable Aedes aegypti infestation in major European cities by 2100. Environ Res. (2019) 172:693–9. doi: 10.1016/j.envres.2019.02.026
9. Kraemer MU, Reiner JRC, Brady OJ, Messina JP, Gilbert M, Pigott DM, et al. Past and future spread of the arbovirus vectors Aedes aegypti and Aedes albopictus. Nat Microbiol. (2019) 4:854–63. doi: 10.1038/s41564-019-0376-y
10. Endler JA. Natural Selection in the Wild. New Jersey, United States of America: Princeton University Press (1986).
11. Futuyma DJ. Natural selection and adaptation. Evolution. (2009), 279–301. doi: 10.1515/9781400848065-026
12. Pigliucci M. Phenotypic Plasticity: Beyond Nature and Nurture. Maryland, USA: Johns Hopkins University Press (2001).
13. West-Eberhard MJ. Developmental Plasticity and Evolution. New York, USA: Oxford University Press (2003).
14. Nylin S, Gotthard K. Plasticity in life-history traits. Annu Rev entomology. (1998) 43:63–83. doi: 10.1146/annurev.ento.43.1.63
15. Schneider JR, Chadee DD, Mori A, Romero-Severson J, Severson DW. Heritability and adaptive phenotypic plasticity of adult body size in the mosquito Aedes aegypti with implications for dengue vector competence. Infection Genet Evol. (2011) 11:11–6. doi: 10.1016/j.meegid.2010.10.019
16. DeWitt TJ, Sih A, Wilson DS. Costs and limits of phenotypic plasticity. Trends Ecol Evol. (1998) 13:77–81. doi: 10.1016/S0169-5347(97)01274-3
17. Ritchie SA, Johnson PH, Freeman AJ, Odell RG, Graham N, DeJong PA, et al. A secure semi-field system for the study of Aedes aegypti. PloS Negl Trop Dis. (2011) 5:e988. doi: 10.1371/journal.pntd.0000988
18. Lounibos LP. Invasions by insect vectors of human disease. Annu Rev Entomology. (2002) 47:233–66. doi: 10.1146/annurev.ento.47.091201.145206
19. Powell JR, Tabachnick WJ. History of domestication and spread of Aedes aegypti - A Review. Memórias do Instituto Oswaldo Cruz. (2013) 108:11–7. doi: 10.1590/0074-0276130395
20. Dickens BL, Sun H, Jit M, Cook AR, Carrasco LR. Determining environmental and anthropogenic factors which explain the global distribution of Aedes aegypti and Ae. albopictus. Parasites Vectors. (2018) 11:310. doi: 10.1136/bmjgh-2018-000801
21. Smith LB, Kasai S, Scott JG. Pyrethroid resistance in Aedes aegypti and Aedes albopictus: Important mosquito vectors of human diseases. Pesticide Biochem Physiol. (2016) 133:1–12. doi: 10.1016/j.pestbp.2016.03.005
22. Deming R, Manrique-Saide P, Medina Barreiro A, Cardeña E, Che-Mendoza A, Jones B, et al. Spatial variation of insecticide resistance in the dengue vector Aedes aegypti presents unique vector control challenges. Parasites Vectors. (2016) 9:67. doi: 10.1186/s13071-016-1346-3
23. Reinhold JM, Lazzari CR, Lahondère C. Effects of the environmental temperature on Aedes aegypti and Aedes albopictus mosquitoes: a review. Insects. (2018) 9:158. doi: 10.3390/insects9040158
24. Wint W, Jones P, Kraemer M, Alexander N, Schaffner F. Past, present and future distribution of the yellow fever mosquito Aedes aegypti: The European paradox. Sci Total Environ. (2022) 847:157566. doi: 10.1016/j.scitotenv.2022.157566
25. Monaghan AJ, Sampson KM, Steinhoff DF, Ernst KC, Ebi KL, Jones B, et al. The potential impacts of 21st century climatic and population changes on human exposure to the virus vector mosquito Aedes aegypti. Climatic Change. (2018) 146:487–500. doi: 10.1007/s10584-016-1679-0
26. Ryan SJ, Carlson CJ, Mordecai EA, Johnson LR. Global expansion and redistribution of Aedes-borne virus transmission risk with climate change. PloS Negl Trop Dis. (2019) 13:e0007213. doi: 10.1371/journal.pntd.0007213
27. Lahondère C. Recent advances in insect thermoregulation. J Exp Biol. (2023) 226:jeb245751. doi: 10.1242/jeb.245751
28. Carrington LB, Armijos MV, Lambrechts L, Barker CM, Scott TW. Effects of fluctuating daily temperatures at critical thermal extremes on Aedes aegypti life-history traits. PloS One. (2013a) 8:e58824. doi: 10.1371/journal.pone.0058824
29. Clements AN. The biology of mosquitoes. In: Development, Nutrition and Reproduction, vol. 1. United Kingdom: Chapman & Hall (1992).
30. Byttebier B, De Majo MS, Fischer S. Hatching response of Aedes aegypti (Diptera: Culicidae) eggs at low temperatures: effects of hatching media and storage conditions. J Med Entomology. (2014) 51:97–103. doi: 10.1603/ME13066
31. Rueda LM, Patel KJ, Axtell RC, Stinner RE. Temperature-dependent development and survival rates of Culex quinquefasciatus and Aedes aegypti (Diptera: Culicidae). J Med Entomology. (1990) 27:892–8. doi: 10.1093/jmedent/27.5.892
32. Couret J, Benedict MQ. A meta-analysis of the factors influencing development rate variation in Aedes aegypti (Diptera: Culicidae). BMC Ecol. (2014) 14:1–15. doi: 10.1186/1472-6785-14-3
33. Eisen L, Monaghan AJ, Lozano-Fuentes S, Steinhoff DF, Hayden MH, Bieringer PE. The impact of temperature on the bionomics of Aedes (Stegomyia) aegypti, with special reference to the cool geographic range margins. J Med entomology. (2014) 51:496–516. doi: 10.1603/ME13214
34. Edillo F, Ymbong RR, Bolneo AA, Hernandez RJ, Fuentes BL, Cortes G, et al. Temperature, season, and latitude influence development-related phenotypes of Philippine Aedes aegypti (Linnaeus): Implications for dengue control amidst global warming. Parasites Vectors. (2022) 15:74. doi: 10.1186/s13071-022-05186-x
35. Mohammed A, Chadee DD. Effects of different temperature regimens on the development of Aedes aegypti (L.)(Diptera: Culicidae) mosquitoes. Acta tropica. (2011) 119:38–43. doi: 10.1016/j.actatropica.2011.04.004
36. Ezeakacha NF, Yee DA. The role of temperature in affecting carry-over effects and larval competition in the globally invasive mosquito Aedes albopictus. Parasites Vectors. (2019) 12:1–11. doi: 10.1186/s13071-019-3391-1
37. Huxley PJ, Murray KA, Pawar S, Cator LJ. Competition and resource depletion shape the thermal response of population fitness in Aedes aegypti. Commun Biol. (2022) 5:66. doi: 10.1038/s42003-022-03030-7
38. Gutiérrez J,EH, Walker KR, Ernst KC, Riehle MA, Davidowitz G. Size as a proxy for survival in Aedes aegypti (Diptera: Culicidae) mosquitoes. J Med Entomology. (2020) 57:1228–38. doi: 10.1093/jme/tjaa055
39. Grech MG, Sartor PD, Almirón WR, Ludueña-Almeida FF. Effect of temperature on life history traits during immature development of Aedes aegypti and Culex quinquefasciatus (Diptera: Culicidae) from Córdoba city, Argentina. Acta tropica. (2015) 146:1–6. doi: 10.1016/j.actatropica.2015.02.010
40. Costa EAPDA, Santos EMDM, Correia JC, Albuquerque CMRD. Impact of small variations in temperature and humidity on the reproductive activity and survival of Aedes aegypti (Diptera, Culicidae). Rev Bras Entomologia. (2010) 54:488–93. doi: 10.1590/S0085-56262010000300021
41. Juliano SA, O’Meara GF, Morrill JR, Cutwa MM. Desiccation and thermal tolerance of eggs and the coexistence of competing mosquitoes. Oecologia. (2002) 130:458–69. doi: 10.1007/s004420100811
42. Bedhomme S, Agnew P, Sidobre C, Michalakis Y. Sex-specific reaction norms to intraspecific larval competition in the mosquito Aedes aegypti. J Evolutionary Biol. (2003) 16:721–30. doi: 10.1046/j.1420-9101.2003.00576.x
43. Chandrasegaran K, Kandregula SR, Quader S, Juliano SA. Context-dependent interactive effects of non-lethal predation on larvae impact adult longevity and body composition. PloS One. (2018) 13:e0192104. doi: 10.1371/journal.pone.0192104
44. Walker M, Chandrasegaran K, Vinauger C, Robert MA, Childs LM. Modeling the effects of Aedes aegypti’s larval environment on adult body mass at emergence. PloS Comput Biol. (2021) 17:e1009102. doi: 10.1371/journal.pcbi.1009102
45. Styer LM, Carey JR, Wang JL, Scott TW. Mosquitoes do senesce: departure from the paradigm of constant mortality. Am J Trop Med Hygiene. (2007) 76:111–7. doi: 10.4269/ajtmh.2007.76.111
46. Christophers SR. Aedes aegypti (L.) the yellow fever mosquito: its life history, bionomics and structure. United Kingdom: Cambridge University Press (1960).
47. Alto BW, Reiskind MH, Lounibos LP. Size alters susceptibility of vectors to dengue virus infection and dissemination. Am J Trop Med Hygiene. (2005) 72:688–93.
48. Carrasco D, Lefèvre T, Moiroux N, Pennetier C, Chandre F, Cohuet A. Behavioural adaptations of mosquito vectors to insecticide control. Curr Opin Insect Sci. (2019) 34:48–54. doi: 10.1016/j.cois.2019.03.005
49. Chandrasegaran K, Lahondère C, Escobar LE, Vinauger C. Linking mosquito ecology, traits, behavior, and disease transmission. Trends Parasitol. (2020) 36:393–403. doi: 10.1016/j.pt.2020.02.001
50. Moreno-García M, Lanz-Mendoza H, Córdoba-Aguilar A. Genetic variance and genotype-by-environment interaction of immune response in Aedes aegypti (Diptera: Culicidae). J Med entomology. (2014) 47:111–20. doi: 10.1093/jmedent/47.2.111
51. McBride CS, Baier F, Omondi AB, Spitzer SA, Lutomiah J, Sang R, et al. Evolution of mosquito preference for humans linked to an odorant receptor. Nature. (2014) 515:222–7. doi: 10.1038/nature13964
52. Aubry F, Dabo S, Manet C, Filipović I, Rose NH, Miot EF, et al. Enhanced Zika virus susceptibility of globally invasive Aedes aegypti populations. Science. (2020) 370:991–6. doi: 10.1126/science.abd3663
53. Costanzo KS, Westby KM, Medley KA. Genetic and environmental influences on the size-fecundity relationship in Aedes albopictus (Diptera: Culicidae): impacts on population growth estimates? PloS One. (2018) 13:e0201465. doi: 10.1371/journal.pone.0201465
54. Lahondère C, Vinauger C, Okubo RP, Wolff GH, Chan JK, Akbari OS, et al. The olfactory basis of orchid pollination by mosquitoes. Proc Natl Acad Sci. (2020) 117:708–16. doi: 10.1073/pnas.1910589117
55. Upshur IF, Fehlman M, Parikh V, Vinauger C, Lahondère C. Sugar feeding by invasive mosquito species on ornamental and wild plants. Sci Rep. (2023) 13:22121. doi: 10.1038/s41598-023-48089-2
56. Mukwaya LG. Genetic control of feeding preferences in the mosquitoes Aedes (Stegomyia) simpsoni and aegypti. Physiol Entomology. (1977) 2:133–45. doi: 10.1111/j.1365-3032.1977.tb00091.x
57. Leahy SM, Vandehey RC, Booth KS. Differential response to oviposition site by feral and domestic populations of Aedes aegypti (L.)(Diptera: Culicidae). Bull Entomological Res. (1978) 68:455–63. doi: 10.1017/S0007485300009433
58. Trpis M, Hausermann W. Genetics of house-entering behaviour in East African populations of Aedes aegypti (L.)(Diptera: Culicidae) and its relevance to speciation. Bull Entomological Res. (1978) 68:521–32. doi: 10.1017/S0007485300009494
59. Braks MAH, Juliano SA, Lounibos LP. Superior reproductive success on human blood without sugar is not limited to highly anthropophilic mosquito species. Med veterinary entomology. (2006) 20:53–9. doi: 10.1111/j.1365-2915.2006.00612.x
60. Alonso San Alberto D, Rusch C, Zhan Y, Straw AD, Montell C, Riffell JA. The olfactory gating of visual preferences to human skin and visible spectra in mosquitoes. Nat Commun. (2022) 13:555. doi: 10.1038/s41467-022-28195-x
61. Van Breugel F, Riffell J, Fairhall A, Dickinson MH. Mosquitoes use vision to associate odor plumes with thermal targets. Curr Biol. (2015) 25:2123–9. doi: 10.1016/j.cub.2015.06.046
62. Sorrells TR, Pandey A, Rosas-Villegas A, Vosshall LB. A persistent behavioral state enables sustained predation of humans by mosquitoes. Elife. (2022) 11:e76663. doi: 10.7554/eLife.76663
63. Laursen WJ, Tang R, Garrity PA. Hunting with heat: thermosensory-driven foraging in mosquitoes, snakes and beetles. J Exp Biol. (2023) 226:jeb229658. doi: 10.1242/jeb.229658
64. Liu MZ, Vosshall LB. General visual and contingent thermal cues interact to elicit attraction in female Aedes aegypti mosquitoes. Curr Biol. (2019) 29:2250–7. doi: 10.1016/j.cub.2019.06.001
65. McMeniman CJ, Corfas RA, Matthews BJ, Ritchie SA, Vosshall LB. Multimodal integration of carbon dioxide and other sensory cues drives mosquito attraction to humans. Cell. (2014) 156:1060–71. doi: 10.1016/j.cell.2013.12.044
66. Zermoglio PF, Robuchon E, Leonardi MS, Chandre F, Lazzari CR. What does heat tell a mosquito? Characterization of the orientation behaviour of Aedes aegypti towards heat sources. J Insect Physiol. (2017) 100:9–14. doi: 10.1016/j.jinsphys.2017.04.010
67. Lacey ES, Ray A, Carde RT. Close encounters: contributions of carbon dioxide and human skin odour to finding and landing on a host in Aedes aegypti. Physiol entomology. (2014) 39:60–8. doi: 10.1111/phen.12048
68. Sumner BD, Cardé RT. Primacy of human odors over visual and heat cues in inducing landing in female aedes aegypti mosquitoes. J Insect Behav. (2022) 35:31–43. doi: 10.1007/s10905-022-09796-2
69. Bello JE, Cardé RT. Compounds from human odor induce attraction and landing in female yellow fever mosquitoes (Aedes aegypti). Sci Rep. (2022) 12:15638. doi: 10.1038/s41598-022-19254-w
70. Geier M, Sass H, Boeckh J. A search for components in human body odour that attract females of Aedes aegypti. In: Ciba Foundation Symposium 200-Olfaction in Mosquito-Host Interactions. John Wiley & Sons, Ltd, Chichester, UK (2007). p. 132–48.
71. De Obaldia ME, Morita T, Dedmon LC, Boehmler DJ, Jiang CS, Zeledon EV, et al. Differential mosquito attraction to humans is associated with skin-derived carboxylic acid levels. Cell. (2022) 185:4099–116. doi: 10.1016/j.cell.2022.09.034
72. VanderGiessen M, Tallon AK, Damico B, Lahondère C, Vinauger C. Soap application alters mosquito-host interactions. iScience. (2023) 26:106667. doi: 10.1016/j.isci.2023.106667
73. Basrur NS, De Obaldia ME, Morita T, Herre M, von Heynitz RK, Tsitohay YN, et al. Fruitless mutant male mosquitoes gain attraction to human odor. Elife. (2020) 9:e63982. doi: 10.7554/eLife.63982
74. Amos BA, Hoffmann AA, Staunton KM, Lau MJ, Burkot TR, Ross PA. Long-range but not short-range attraction of male Aedes aegypti (Diptera: Culicidae) mosquitoes to humans. J Med Entomology. (2022) 59:83–8. doi: 10.1093/jme/tjab164
75. Tallon AK, Hill SR, Ignell R. Sex and age modulate antennal chemosensory-related genes linked to the onset of host seeking in the yellow-fever mosquito, Aedes aegypti. Sci Rep. (2019) 9:43. doi: 10.1038/s41598-018-36550-6
76. Juliano SA, Ribeiro GS, Maciel-de-Freitas R, Castro MG, Codeço C, Lourenço-de-Oliveira R, et al. She’sa femme fatale: low-density larval development produces good disease vectors. Memorias do Instituto Oswaldo Cruz. (2014) 109:1070–7. doi: 10.1590/0074-02760140455
77. Scheiner SM. Genetics and evolution of phenotypic plasticity. Annu Rev Ecol systematics. (1993) 24:35–68. doi: 10.1146/annurev.es.24.110193.000343
78. Upshur IF, Bose EA, Hart C, Lahondère C. Temperature and sugar feeding effects on the activity of a laboratory strain of Aedes aegypti. Insects. (2019) 10:347. doi: 10.3390/insects10100347
79. Lahondère C, Vinauger C, Liaw JE, Tobin KK, Joiner JM, Riffell JA. Effect of temperature on mosquito olfaction. Integrative and Comparative Biology. (2023) 63:356–67. doi: 10.1093/icb/icad066
80. Ignell R, Lazzari CR, Lorenzo MG, Hill SR. Sensory ecology of disease vectors. Netherlands: Wageningen Academic Publishers (2022).
81. Pitts RJ, Bouzada LI, Guerenstein PG. Chapter 2: Comparative morphology of the peripheral olfactory system of disease vector arthropods. In: Ignell R, Lazzari CR, Lorenzo MG, Hill &, S. R, editors. Sensory ecology of disease vectors. Netherlands: Wageningen Academic Publishers (2022). p. 29–70.
82. Eilerts DF, VanderGiessen M, Bose EA, Broxton K, Vinauger C. Odor-specific daily rhythms in the olfactory sensitivity and behavior of Aedes aegypti mosquitoes. Insects. (2018) 9:147. doi: 10.3390/insects9040147
83. Leming MT, Rund SS, Behura SK, Duffield GE, O’Tousa JE. A database of circadian and diel rhythmic gene expression in the yellow fever mosquito Aedes aegypti. BMC Genomics. (2014) 15:1–9. doi: 10.1186/1471-2164-15-1128
84. Shetty V, Meyers JI, Zhang Y, Merlin C, Slotman MA. Impact of disabled circadian clock on yellow fever mosquito Aedes aegypti fitness and behaviors. Sci Rep. (2022) 12:6899. doi: 10.1038/s41598-022-10825-5
85. Ajayi OM, Marlman JM, Gleitz LA, Smith ES, Piller BD, Krupa JA, et al. Behavioral and postural analyses establish sleep-like states for mosquitoes that can impact host landing and blood feeding. J Exp Biol. (2022) 225:jeb244032. doi: 10.1242/jeb.244032
86. Wynne NE, Lorenzo MG, Vinauger C. Mechanism and plasticity of vectors’ host-seeking behavior. Curr Opin Insect Sci. (2020) 40:1–5. doi: 10.1016/j.cois.2020.02.001
87. Grant AJ, O’Connell RJ. Age-related changes in female mosquito carbon dioxide detection. J Med entomology. (2007) 44:617–23. doi: 10.1093/jmedent/44.4.617
88. Klowden MJ. The endogenous regulation of mosquito reproductive behavior. Experientia. (1990) 46:660–70. doi: 10.1007/BF01939928
89. Alonso DP, Campos M, Troca H, Kunii R, Tripet F, Ribolla PEM. Gene expression profile of Aedes aegypti females in courtship and mating. Sci Rep. (2019) 9:15492. doi: 10.1038/s41598-019-52268-5
90. Camargo C, Ahmed-Braimah YH, Amaro IA, Harrington LC, Wolfner MF, Avila FW. Mating and blood-feeding induce transcriptome changes in the spermathecae of the yellow fever mosquito Aedes aegypti. Sci Rep. (2020) 10:14899. doi: 10.1038/s41598-020-71904-z
91. Scheiner SM, Gurevitch J eds. Design and analysis of ecological experiments. United Kingdom: Oxford University Press (2001).
92. Daugherty MP, Juliano SA. Testing for context-dependence in a processing chain interaction among detritus-feeding aquatic insects. Ecol entomology. (2002) 27:541–53. doi: 10.1046/j.1365-2311.2002.00446.x
93. Juliano SA. Species interactions among larval mosquitoes: context dependence across habitat gradients. Annu Rev entomology. (2009) 54:37–56. doi: 10.1146/annurev.ento.54.110807.090611
94. Tsurim I, Silberbush A, Ovadia O, Blaustein L, Margalith Y. Inter-and intra-specific density-dependent effects on life history and development strategies of larval mosquitoes. PloS One. (2013) 8:e57875. doi: 10.1371/journal.pone.0057875
95. Couret J, Dotson E, Benedict MQ. Temperature, larval diet, and density effects on development rate and survival of Aedes aegypti (Diptera: Culicidae). PloS One. (2014) 9:e87468. doi: 10.1371/journal.pone.0087468
96. Heath K, Paton RS, Wilson AJ, Bonsall M. Nutritional availability and larval density dependence in Aedes aegypti. Ecol Entomology. (2020) 45:929–44. doi: 10.1111/een.12877
97. Steinwascher K. Competition among Aedes aegypti larvae. PloS One. (2018) 13:e0202455. doi: 10.1371/journal.pone.0202455
98. Souza RS, Virginio F, Riback TIS, Suesdek L, Barufi JB, Genta FA. Microorganism-based larval diets affect mosquito development, size and nutritional reserves in the yellow fever mosquito Aedes aegypti (Diptera: Culicidae). Front Physiol. (2019) 10:152. doi: 10.3389/fphys.2019.00152
99. Yan J, Kibech R, Stone CM. Differential effects of larval and adult nutrition on female survival, fecundity, and size of the yellow fever mosquito, Aedes aegypti. Front zoology. (2021) 18:1–9. doi: 10.1186/s12983-021-00395-z
100. Focks DA, Alexander N, Villegas E, World Health Organization. Multicountry study of Aedes aegypti pupal productivity survey methodology: findings and recommendations (No. TDR/IRM/DEN/06.1). World Health Organization (2006).
101. Williams CR, Johnson PH, Ball TS, Ritchie SA. Productivity and population density estimates of the dengue vector mosquito Aedes aegypti (Stegomyia aegypti) in Australia. Med veterinary entomology. (2013) 27:313–22. doi: 10.1111/j.1365-2915.2012.01051.x
102. Banerjee S, Pramanik S, Banerjee S, Saha GK, Aditya G. Effects of density dependent larval competition on the life history traits of Aedes aegypti and Aedes albopictus (Diptera: Culicidae). Fragmenta entomologica. (2017) 49:97–107. doi: 10.4081/fe.2017.238
103. Krol L, Gorsich EE, Hunting ER, Govender D, Van Bodegom PM, Schrama M. Eutrophication governs predator-prey interactions and temperature effects in Aedes aegypti populations. Parasites Vectors. (2019) 12:1–10. doi: 10.1186/s13071-019-3431-x
104. Alto BW, Reiskind MH, Lounibos LP. Size alters susceptibility of vectors to dengue virus infection and dissemination. Am J Trop Med hygiene. (2008) 79:688. doi: 10.4269/ajtmh.2008.79.688
105. Nasci RS, Mitchell CJ. Larval diet, adult size, and susceptibility of Aedes aegypti (Diptera: Culicidae) to infection with Ross River virus. J Med Entomology. (1994) 31:123–6. doi: 10.1093/jmedent/31.1.123
106. Sumanochitrapon W, Strickman D, Sithiprasasna R, Kittayapong P, Innis BL. Effect of size and geographic origin of Aedes aegypti on oral infection with dengue-2 virus. Am J Trop Med hygiene. (1998) 58:283–6. doi: 10.4269/ajtmh.1998.58.283
107. Wilk-da-Silva R, de Souza Leal Diniz MMC, Marrelli MT, Wilke ABB. Wing morphometric variability in Aedes aegypti (Diptera: Culicidae) from different urban built environments. Parasites Vectors. (2018) 11:1–9. doi: 10.1186/s13071-018-3154-4
108. Alto BW, Lord CC. Transstadial effects of Bti on traits of Aedes aegypti and infection with dengue virus. PloS Negl Trop Dis. (2016) 10:e0004370. doi: 10.1371/journal.pntd.0004370
109. Chandrasegaran K, Juliano SA. How do trait-mediated non-lethal effects of predation affect population-level performance of mosquitoes? Front Ecol Evol. (2019) 7:25. doi: 10.3389/fevo.2019.00025
110. Roiz D, Rosa R, Arnoldi D, Rizzoli A. Effects of temperature and rainfall on the activity and dynamics of host-seeking Aedes albopictus females in northern Italy. Vector-borne zoonotic Dis. (2010) 10:811–6. doi: 10.1089/vbz.2009.0098
111. Costanzo K, Occhino D. Effects of temperature on blood feeding and activity levels in the tiger mosquito, aedes albopictus. Insects. (2023) 14:752. doi: 10.3390/insects14090752
112. Honório NA, Silva WDC, Leite PJ, Gonçalves JM, Lounibos LP, Lourenço-de-Oliveira R. Dispersal of Aedes aegypti and Aedes albopictus (Diptera: Culicidae) in an urban endemic dengue area in the State of Rio de Janeiro, Brazil. Memórias do Instituto Oswaldo Cruz. (2003) 98:191–8. doi: 10.1590/S0074-02762003000200005
113. Maciel-de-Freitas R, Codeço CT, Lourenço-de-Oliveira R. Body size-associated survival and dispersal rates of Aedes aegypti in Rio de Janeiro. Med veterinary entomology. (2007) 21:284–92. doi: 10.1111/j.1365-2915.2007.00694.x
114. Carrington LB, Seifert SN, Armijos MV, Lambrechts L, Scott TW. Reduction of Aedes aegypti vector competence for dengue virus under large temperature fluctuations. Am J Trop Med hygiene. (2013b) 88:689. doi: 10.4269/ajtmh.12-0488
115. Lambrechts L, Paaijmans KP, Fansiri T, Carrington LB, Kramer LD, Thomas MB, et al. Impact of daily temperature fluctuations on dengue virus transmission by Aedes aegypti. Proc Natl Acad Sci. (2011) 108:7460–5. doi: 10.1073/pnas.1101377108
116. De Majo MS, Zanotti G, Campos RE, Fischer S. Effects of constant and fluctuating low temperatures on the development of Aedes aegypti (Diptera: Culicidae) from a temperate region. J Med Entomology. (2019) 56:1661–8. doi: 10.1093/jme/tjz087
117. Raji JI, Melo N, Castillo JS, Gonzalez S, Saldana V, Stensmyr MC, et al. Aedes aegypti mosquitoes detect acidic volatiles found in human odor using the IR8a pathway. Curr Biol. (2019) 29:1253–62. doi: 10.1016/j.cub.2019.02.045
118. Wohl MP, McMeniman CJ. Overview of Aedes aegypti and use in laboratory studies. Cold Spring Harbor Protoc. (2023) 2023:pdb–top107651. doi: 10.1101/pdb.top107651
119. Camara TNDL. Activity patterns of Aedes aegypti and Aedes albopictus (Diptera: Culicidae) under natural and artificial conditions. Oecologia Australis. (2010) 14:737–44.
120. McLean-Cooper N, Achee N, Foggie T, Grieco J, Williams J. Space optimizing methods for laboratory rearing of Aedes aegypti. J Am Mosq Control Assoc. (2008) 24:460–2. doi: 10.2987/5649.1
121. Zheng ML, Zhang DJ, Damiens DD, Lees RS, Gilles JR. Standard operating procedures for standardized mass rearing of the dengue and chikungunya vectors Aedes aegypti and Aedes albopictus (Diptera: Culicidae)-II-Egg storage and hatching. Parasites Vectors. (2015) 8:1–7. doi: 10.1186/s13071-015-0951-x
122. Bond JG, Ramírez-Osorio A, Marina CF, Fernández-Salas I, Liedo P, Dor A, et al. Efficiency of two larval diets for mass-rearing of the mosquito Aedes aegypti. PloS One. (2017) 12:e0187420. doi: 10.1371/journal.pone.0187420
123. Puggioli A, Carrieri M, Dindo ML, Medici A, Lees RS, Gilles JRL, et al. Development of Aedes albopictus (Diptera: Culicidae) larvae under different laboratory conditions. J Med entomology. (2017) 54:142–9. doi: 10.1093/jme/tjw127
124. Contreras-Perera Y, Flores-Pech JP, Pérez-Carillo S, Puerta-Guardo H, Geded-Moreno E, Correa-Morales F, et al. Different larval diets for Aedes aegypti (Diptera: Culicidae) under laboratory conditions: in preparation for a mass-rearing system. Biologia. (2023) 78:3387–99. doi: 10.1007/s11756-023-01469-5
125. Padmanabha H, Correa F, Legros M, Nijhout HF, Lord C, Lounibos LP. An eco-physiological model of the impact of temperature on Aedes aegypti life history traits. J Insect Physiol. (2012) 58:1597–608. doi: 10.1016/j.jinsphys.2012.09.015
126. Sasmita HI, Tu WC, Bong LJ, Neoh KB. Effects of larval diets and temperature regimes on life history traits, energy reserves and temperature tolerance of male Aedes aegypti (Diptera: Culicidae): optimizing rearing techniques for the sterile insect programmes. Parasites Vectors. (2019) 12:1–16. doi: 10.1186/s13071-019-3830-z
127. David MR, Dantas ES, Maciel-de-Freitas R, Codeço CT, Prast AE, Lourenço-de-Oliveira R. Influence of larval habitat environmental characteristics on Culicidae immature abundance and body size of adult Aedes aegypti. Front Ecol Evol. (2021) 9:626757. doi: 10.3389/fevo.2021.626757
128. Bong LJ, Tu WC, Neoh KB. Interpopulation variations in life history traits and reproductive tactics in Aedes aegypti: A test on populations 50 km apart. Acta Tropica. (2021) 213:105750. doi: 10.1016/j.actatropica.2020.105750
129. Muttis E, Balsalobre A, Chuchuy A, Mangudo C, Ciota AT, Kramer LD, et al. Factors related to Aedes aegypti (Diptera: Culicidae) populations and temperature determine differences on life-history traits with regional implications in disease transmission. J Med entomology. (2018) 55:1105–12. doi: 10.1093/jme/tjy057
130. Chown SL, Nicolson S. Insect physiological ecology: mechanisms and patterns. United Kingdom: Oxford University Press (2004).
131. Ellis AM, Garcia AJ, Focks DA, Morrison AC, Scott TW. Parameterization and sensitivity analysis of a complex simulation model for mosquito population dynamics, dengue transmission, and their control. Am J Trop Med hygiene. (2011) 85:257. doi: 10.4269/ajtmh.2011.10-0516
132. Wu SL, Sánchez C HM, Henry JM, Citron DT, Zhang Q, Compton K, et al. Vector bionomics and vectorial capacity as emergent properties of mosquito behaviors and ecology. PloS Comput Biol. (2020) 16:e1007446. doi: 10.1371/journal.pcbi.1007446
133. Engqvist L. The mistreatment of covariate interaction terms in linear model analyses of behavioural and evolutionary ecology studies. Anim Behav. (2005) 70:967–71. doi: 10.1016/j.anbehav.2005.01.016
134. Gelman A, Hill J. Data analysis using regression and multilevel/hierarchical models. United Kingdom: Cambridge university press (2007).
135. Schielzeth H. Simple means to improve the interpretability of regression coefficients. Methods Ecol Evol. (2010) 1:103–13. doi: 10.1111/j.2041-210X.2010.00012.x
136. Beck CW, Bliwise NG. Interactions are critical. CBE—Life Sci Educ. (2014) 13:371–2. doi: 10.1187/cbe.14-05-0086
137. Muturi EJ, Lampman R, Costanzo K, Alto BW. Effect of temperature and insecticide stress on life-history traits of Culex restuans and Aedes albopictus (Diptera: Culicidae). J Med entomology. (2011) 48:243–50. doi: 10.1603/ME10017
138. Alto BW, Juliano SA. Temperature effects on the dynamics of Aedes albopictus (Diptera: Culicidae) populations in the laboratory. J Med entomology. (2001) 38:548–56. doi: 10.1603/0022-2585-38.4.548
Keywords: Aedes aegypti, context-specific variation, life history traits, behavioral plasticity, transstadial effects
Citation: Vinauger C and Chandrasegaran K (2024) Context-specific variation in life history traits and behavior of Aedes aegypti mosquitoes. Front. Insect Sci. 4:1426715. doi: 10.3389/finsc.2024.1426715
Received: 02 May 2024; Accepted: 19 August 2024;
Published: 25 September 2024.
Edited by:
Peter M. Piermarini, The Ohio State University, United StatesReviewed by:
Steven A. Juliano, Illinois State University, United StatesMatthew DeGennaro, Florida International University, United States
Copyright © 2024 Vinauger and Chandrasegaran. This is an open-access article distributed under the terms of the Creative Commons Attribution License (CC BY). The use, distribution or reproduction in other forums is permitted, provided the original author(s) and the copyright owner(s) are credited and that the original publication in this journal is cited, in accordance with accepted academic practice. No use, distribution or reproduction is permitted which does not comply with these terms.
*Correspondence: Karthikeyan Chandrasegaran, a2FydGhpa2NAdWNyLmVkdQ==
†These authors have contributed equally to this work