- School of Biological Sciences, Illinois State University, Normal, IL, United States
Diet can have an array of both direct and indirect effects on an organism’s health and fitness, which can influence the outcomes of host-pathogen interactions. Land use changes, which could impact diet quantity and quality, have imposed foraging stress on important natural and agricultural pollinators. Diet related stress could exacerbate existing negative impacts of pathogen infection. Accounting for most of its nutritional intake in terms of protein and many micronutrients, pollen can influence bee health through changes in immunity, infection, and various aspects of individual and colony fitness. We investigate how adult pollen consumption, pollen type, and pollen diversity influence bumble bee Bombus impatiens survival and infection outcomes for a microsporidian pathogen Nosema (Vairimorpha) bombi. Experimental pathogen exposures of larvae occurred in microcolonies and newly emerged adult workers were given one of three predominantly monofloral, polyfloral, or no pollen diets. Workers were assessed for size, pollen consumption, infection 8-days following adult-eclosion, survival, and the presence of extracellular microsporidian spores at death. Pollen diet treatment, specifically absence of pollen, and infection independently reduced survival, but we saw no effects of pollen, pollen type, or pollen diet diversity on infection outcomes. The latter suggests infection outcomes were likely already set, prior to differential diets. Although infection outcomes were not altered by pollen diet in our study, it highlights both pathogen infection and pollen availability as important for bumble bee health, and these factors may interact at different stages of bumble bee development, at the colony level, or under different dietary regimes.
Introduction
An organism’s diet, through its nutrition or other elements, can have a profound influence on its ecology, including biotic interactions (1). This includes important effects of diet on the outcomes of pathogen infection, including pathogen traits of infectivity and transmission and host traits of health and fitness. In humans, the link between malnutrition and infectious disease is longstanding, with deficiencies in the diet compromising immunity and thus resistance to infection (2). Variation in host diet can influence immunity (3–5) and infection outcomes relating to the levels of infection and survival tolerance to infection (5–7). Non-nutritional compounds can also reduce infection (8). Diets can enhance the growth of commensal microbes that compete with pathogenic bacteria, indirectly reducing the opportunity for infection, or promoting immunity (9). Conversely, a highly diverse diet can catalyze the growth of symbiotic bacteria to levels that lead to costly upregulation of immunity (10). Diet composition can also affect strategic life history trait shifts, such as investment in reproduction upon parasite infection (11). As shown by these examples above, the evidence for diet-dependence of infection outcomes is widespread, but we still lack knowledge of how ecologically relevant variation in diet, including diversity, may affect many important host-pathogen interactions.
The health of pollinators globally is currently of great concern. Agricultural and other land use changes combined with other factors like nutrition, changing climate, pathogens and pesticides have been touted as being responsible for declines of several species of insect pollinators, including bees (12, 13). These factors deemed detrimental to pollinator health will have negative impacts on important agricultural and natural ecosystem pollination, increasing the need to elucidate the mechanisms by which they affect pollinator populations. In addition, the factors highlighted above have the potential to interact with one another, potentially to the further detriment of pollinator health (12, 14–16). This work focuses on the potential interaction between adult diet and infection outcomes in a bumble bee and microsporidian pathogen system.
Floral resources available to bees, which dictate their diet, have the potential to influence individual development and condition but also the health of social bee colonies (17, 18). Bee nectar and pollen consumption are responsible for being the main source of carbohydrates and protein, respectively, with pollen also providing lipids and micronutrients (19). Different flowers provide nectar that varies in carbohydrates and organic compounds important for pollinator attraction and floral defense (20), and pollen that varies in protein, lipid, sterol and essential amino acids (21–24). The available resources for bees may vary in quality and quantity, and land use changes, such as intense agriculture, can decrease floral diversity (25).
Available quantity, quality and diversity of pollen resources for important bee pollinators may induce foraging stress and have other direct and indirect effects on individual and overall colony health. Under such conditions bees may be faced with increased energetic costs of foraging (26) and nutritional deprivation. For example, Bombus impatiens colony-level performance strongly correlates with total amount of pollen collected and total macronutrient quantity (27). In addition, in intense agricultural environments depauperate in floral diversity, foragers of the bumble bee B. terrestris return with less diverse pollen loads compared with more diverse areas, and the diversity of collected pollen is positively correlated with a surrogate of colony growth and weight (28). Pollen availability and type has also been shown to influence individual development, size and survival, queen oviposition, and colony development (29–35). Even if pollen diversity is not important per se, but rather individual components of the pollen diet influence bee health (24), pollen diet diversity can contribute to buffering against poor quality pollen (34) and allow for diet optimization (23, 36).
There are likely many connections between nutrition and bee pathogen infection, including interactions that influence health (37). For example, choice of floral resources may affect pathogen exposure (38) and landscape simplification altering diet breadth appears to affect pathogen prevalence (39). Conversely, pathogen infection may influence foraging behavior, and hence diet. Bombus impatiens foraging efforts are impaired, specifically in discriminatory learning of rewarding and nonrewarding flowers, under the stress of infection by the trypanosome Crithida bombi (40). However, the focus here is on how diet may interact directly or indirectly, through bee host physiology and condition, with pathogen infections.
Pollen feeding alters C. bombi development in bumble bees (Bombus terrestris), with important effects on colony-level transmission (41). In addition, an increase in pollen consumption may indirectly benefit the host without necessarily influencing pathogen clearance. Honey bees (Apis mellifera) fed higher pollen quantity showed higher Nosema cerenae infection intensities but also had higher survival (42). Diverse poly-floral pollen also increased honey bee tolerance to N. ceranae infection above all but the most protein rich mono-floral diets (43). A similar benefit of poly-floral pollen was seen for honey bee larvae infected with an opportunistic fungal pathogen Aspergillus fumigatus (44). High diet diversity in honey bees increases glucose oxidase activity, a social immune measure important for an antimicrobial secretion in larval food and honey (45). Pollen diet quality can also affect bumble bee immunity (46), and immune gene expression in response to a trypanosome infection are reduced following pollen deprivation (47). Some pollen types, such as sunflower, have also been declared medicinal due to reductions in bumble bee trypanosome infection (48–50). These effects may not come from nutrition, but rather protective anti-microbial effects of phytochemicals in the diet (37). It is clear then, that both pollen diversity and pollen quality may be important for mitigating the negative effects of infection.
Many North American bumble bee species have declined in abundance and range over the last decades, with an apparent association between species declines and infection with the microsporidian pathogen Nosema (Vairimorpha) bombi (12, 51–53). Recently, there was a proposal to reclassify Nosema as Vairimorpha (54). However, while we acknowledge uncertainty in the classification, we maintain our use of the genus name Nosema given a lack of additional evidence that would be required for official reclassification (55). Nosema bombi has been shown to decrease bee fitness traits, including survival, sperm counts (56), and colony size (57). Adult workers deposit environmentally resistant spores in their feces (58), which contaminate nest material and floral resources. Larval stages are infected (59) and the intra-cellular pathogen stage hijacks ATP from host cells to develop and reproduce (60). Due to the drain by N. bombi on host energy reserves, infection outcomes may be expected to depend on nutritional resources, including those in the pollen diet. Although it has been shown that the isoflavanoid biochanin A, found in clover pollen, reduces N. bombi spores in adults but not larvae (61), no other studies have investigated such effects of bumble bee diet on infection with N. bombi.
There is limited understanding on how important pathogen and host parameters in chronic infections, including those of key pollinators, are influenced by diet diversity. In this study, we analyze the influence of adult pollen diet availability and diversity on outcomes of existing N. bombi infections in the bumble bee B. impatiens. We hypothesize that a pollen diet and the diversity of that diet will influence either resistance or tolerance to infection (62), based on the multiple stressor hypothesis of increased detrimental effects upon combined stressors (14). We use experimental pathogen inoculations during the larval stage combined with adult diets of no pollen, three predominantly mono-floral pollen diets (Salix sp., Gleditsia triacanthos, Rubus sp.) and one poly-floral pollen diet, combining these monofloral pollens, to assess the effects on diet consumption over time, pathogen spore loads, and host survival. We predict that pollen deprived bees and those fed mono-floral pollen diets will have lower resistance to infection (spore load) and that an interaction between pollen diet and Nosema infection on survival will be present because of further reduced tolerance to infection in pollen deprived and mono-floral pollen fed bees.
Materials and methods
Overview of experimental design to assess individual and combined pollen and pathogen effects
To assess individual and combined effects of diet treatment and N. bombi exposure and infection, developing larvae were either exposed or not exposed to a fixed number of spores during early development within their microcolonies. Subsequently, adults emerging in the microcolonies were isolated and provisioned with no pollen, one of three predominantly mono-floral pollen diets (confirmed molecularly as Salix sp., Gleditsia triacanthos, Rubus sp.), or a poly-floral diet consisting of an equal mix of the three mono-floral diets. Workers were assessed for effects of Nosema exposure or infection and pollen diet treatment on: (i) adult body size (n=200), (ii) pollen consumption (n=169), (iii) sporulating infection prevalence and spore count 8-days after adult emergence in a subset of bees killed at that time (n=76), (iv) infection prevalence and spore count at the time of death in a survival group and (v) their adult survival (n=166).
Bumble bee source colonies
Microcolonies were established from ten commercial colonies (Koppert Biological Systems, Howell, Michigan, USA) and additional data for 8-day infection outcomes were collected from microcolonies sourced from a further three lab-reared colonies from field-caught queens. Queens of the lab-reared colonies were collected with the permission of the ParkLands Foundation (http://www.parklandsfoundation.org/) from the Mackinaw River Study Area (Lexington, IL., U.S.A.). All established colonies were confirmed free of common pathogen infections (including N. bombi) and maintained under standard laboratory conditions (14).
Nosema bombi inoculum
Abdomens from three workers infected with a single Nosema isolate (lab identification: 017.01) were processed as in Calhoun et al. (14) and aliquots were stored at -80°C. When larvae were ready to receive the Nosema spore inoculum, aliquots were thawed on dry bath at 32°C. The aliquot was centrifuged at 3000 g for five minutes, and supernatant was removed. The pellet was then suspended in 1:1 v/v ultrapure water and 50% sugar water blended with five grains of fresh frozen pollen. Spore solutions were adjusted to 20,000 spores/μL for inoculation.
Microcolony set-up and larval pathogen inoculation
Worker larvae in queenless microcolonies were designated to receive either N. bombi or a control solution containing only sugar water and pollen. Queenless microcolonies were constructed by randomly isolating five workers from a source colony into a plastic box with three medium petri dishes used to hold a brood clump of early instar (2nd) larvae and their surrounding wax that had been removed from the queen containing source colony, a standard poly-floral pollen pellet that was replaced three times per week, and a feeding tube providing sugar water ad libitum. All microcolonies were housed under red-light illumination at 26 ± 1.5°C.
Two-days after microcolony establishment, individual larva received a 2 μL inoculum of either 40,000 N. bombi spores suspended in a sugar water/pollen grain solution or a comparable solution without pathogen spores. Larval brood were temporarily separated from their respective microcolony, the wax of the larval casing was carefully peeled back utilizing soft forceps and a straight tip dissecting needle, exposing the larvae. Once uncovered, the 2 μL inoculum was delivered with a micropipette to the ventral side of each individual, allowing for the solution to adhere and be consumed by the larva. Each larval brood was left separated from the microcolony for five minutes to ensure full consumption of the treatment and placed back into their respective microcolony, where the wax was repaired by the nursing workers. This process was observed to ensure that larvae were not rejected. The original adult workers were marked with a spot of correction fluid on the top of the thorax and microcolonies were observed daily for adult emergence of the experimental individuals. Adults that emerged from each microcolony were removed within 24 hours and allocated uniformly to treatments within each replicate block. Removed individuals were isolated into plastic deli dishes (10 × 5 × 8 cm) with a 15 mL feeding tube containing sterile sugar water, paper substrate, and a 35mm petri dish with a pollen pellet for their respective diet treatment (see below). Deli dishes were replaced every 8 days for bees in the survival group. Body size from the radial cell length of the forewings for all individual (63) were measured using ImageJ software. The mean was taken from each pair of forewings for further analyses.
Pollen diet treatments and consumption
Adults emerging from the microcolonies were provided with one of five pollen diet treatments. To produce three mono-floral diets, honey bee collected pollen loads were sorted based on color and the three most consistent and abundant were chosen (green, orange, yellow). A poly-floral blend was made by combining equal weights, to the nearest milligram, of the three mono-floral blends. Honey bees are known to predominantly collect one type of pollen per foraging trip (64). However, to confirm purity of mono-floral pollen diets and the diversity of the mono-floral versus poly-floral diets, four samples of each pollen type were suspended in ultrapure water and pollen grains counted in a FastRead 102 chamber under 400x magnification. All mono-floral diets had high levels of the focal pollen type, with the green 92% (s.d. 0.020%), orange 99% (s.d. 0.001%), and yellow 97% (s.d. 0.004%) purity. The Shannon Diversity Index, determined through microscopy for all pollen, the poly-floral treatment (1.20) was much higher than the green (0.27), orange (0.08) and yellow (0.17) mono-floral diets, indicating the desired difference between the treatments in diet diversity. The identity of the pollen was determined by DNA extraction from three replicates of individual pollen loads from each type using an IBI Scientific Mini Genomic Plant DNA Kit following the manufacturer’s instructions. PCR reactions were then set up using established primers and conditions for the trnL-trnF and ITS2 barcoding regions (65). All replicates for both regions were consistently identified as Salix sp. (Willow, orange), Gleditsia triacanthos (Honey locust, yellow), Rubus sp. (bramble, green) (Supplementary Table 1).
All pollen diets were made of the same ratio of sugar water to pollen and shaped into pellets using a 1 mL syringe, with the pollen cut every 100μl. Pellets were made fresh and replaced every 4 days. Consumption of diet treatments was measured by the difference in dry mass relative to the mean of 30 pre-weighed identically made reference pollen pellets of each diet. Dry weight was taken following a 2 day drying period in a drying oven at 60°C. All pellets were weighed on an XA Analytical Balance (Fisher Scientific) to the nearest milligram. A fifth pollen diet treatment consisted of no pollen, where workers were only fed sugar water ad libitum.
Infection and survival responses
Infection was measured in a group of bees that were killed at eight-days post-eclosion to ensure a fixed timepoint for assessment of infection across individuals at a time when spores have been produced. These bees were stored at -20°C until processing. Whole bee abdomens were removed, homogenized in 1 mL ringer solution with an Omni THQ digital tissue homogenizer with a hard tissue tip. 10 μL of the homogenate was placed onto a hemocytometer and observed under a phase contrast microscope (Labomed Lx 500, Fremont, CA, USA) at 400x total magnification. Transmission ready spores were counted and converted to total spores per individual for further analysis. When spores are present, they are proportional to the overall infection intensity of intra-cellular stages (14).
Those bees assigned to the survival group were tracked daily for survival. Upon death, the date was recorded, and Nosema spores on death were quantified as above. Bees that did not die before the end of the experiment were included in analyses as right-censored observations.
Statistical analyses
All analyses were performed in R version 3.6.3 “Holding the Windsock” for Mac (66). The coxme package (67) was used for Mixed Effect Cox Proportional Hazards Models and the lme4 package for Generalized Linear Models (68). For each response variable, potential distributions were assessed for model fit and adherence to model assumptions. Initial models were simplified by sequentially eliminating non-significant terms based on likelihood ratio tests (LRTs) and nested models were selected using AIC (69). Statistics for terms not in final models were taken from the step before their removal. The package emmeans (70) was used to calculate estimated marginal means and their confidence intervals for levels of model terms and for post hoc contrasts.
For all models the original source colony and micro-colony, nested within original source colony, were included as random effects. A Linear Mixed Effects Model was fit with Nosema exposure treatment as a fixed effect to test the effect of larval exposure to the pathogen on adult body size. Additionally, a second model was fit only to the data from the Nosema exposed group to assess the influence of infection status, with the presence of spores when sampled taken as evidence of infection and the lack of spores as no infection. For a Linear Mixed Effects Model to analyze pollen consumption from 0 to 4 days and 4 to 8 days after adult eclosion, individual bee ID was included as an additional random effect to account for the repeated measures design. Further, the initial fitted model included body size, time period (0-4 and 4-8 days), Nosema exposure, pollen diet treatment, and all two-way interactions and the three-way interaction between time period, Nosema exposure, and pollen diet treatment. A second model was again fit with infection status within Nosema exposed bees replacing Nosema exposure treatment. Fixed time point infection prevalence at 8 days post-adult eclosion were analyzed based on binary infection prevalence (0/1). Infection prevalence was analyzed with a Generalized Linear Mixed Model fit with a binomial distribution and logit link function, including body size and diet in the initial model. The total number of spores was analyzed with a Linear Mixed Effects Model initially including body size and pollen diet treatment. The response variable of total spores was log transformed to meet model assumptions, and this approach was preferred as it produced a better fitting model than Generalized Linear Mixed Models with distributions including log link functions. These same analyses were repeated for spore presence and numbers at death in the survival group. A Mixed Effect Cox Proportional Hazards Model was used to evaluate the effect of body size, pollen treatment, Nosema exposure treatment, and the interaction between pollen and Nosema exposure treatments on individual survival. A similar model but only on individuals exposed to Nosema, replaced Nosema exposure treatment with infection status, as with body size and pollen consumption above.
Results
Body size
Comparing bees exposed as larvae to Nosema and unexposed bees, there was no significant difference in adult size (χ2 = 0.316, d.f. = 1, p = 0.574). However, within Nosema exposed bees, those showing signs of infection (i.e., the presence of spores) were significantly smaller than those with no apparent infection (χ2 = 4.01, d.f. = 1, p = 0.045) (Supplementary Figure 1).
Pollen diet consumption
There was a significant effect of time period on pollen consumption (Table 1), with bees consuming more in their first four days than between day four and eight (Figure 1). Additionally, larger bees consumed more pollen (β = 0.346) (Table 1). There was, however, no effect of Nosema exposure, diet, or the interactions between them and time period on pollen consumption (p > 0.25) (Table 1). These outcomes did not change when comparing bees with evidence of infection and those without in the Nosema exposed group (Table 1).
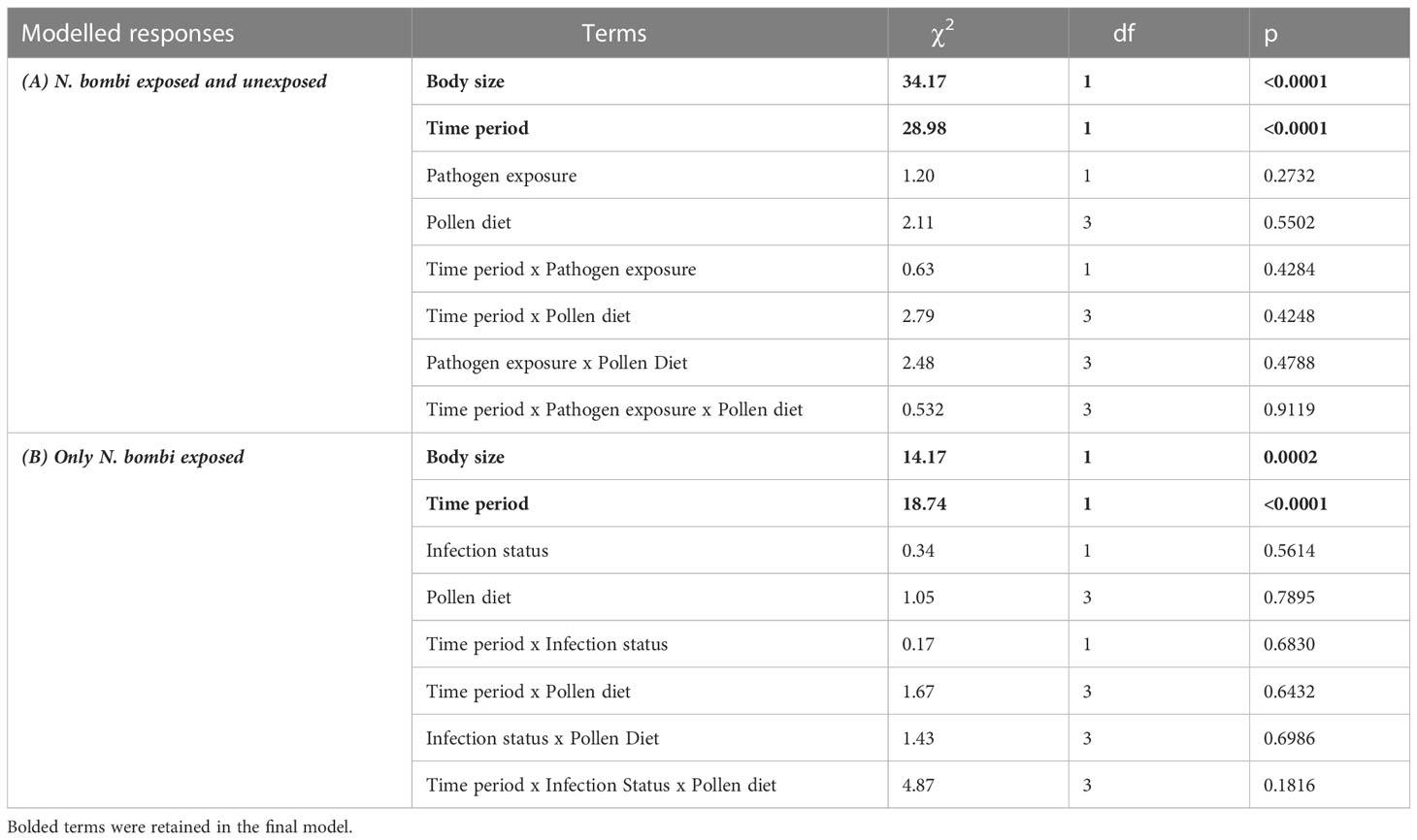
Table 1 Fixed effect model terms for pollen diet consumption for (A) Nosema bombi exposed and unexposed bees, and (B) within N. bombi exposed bees, those with evidence of infection and those without.
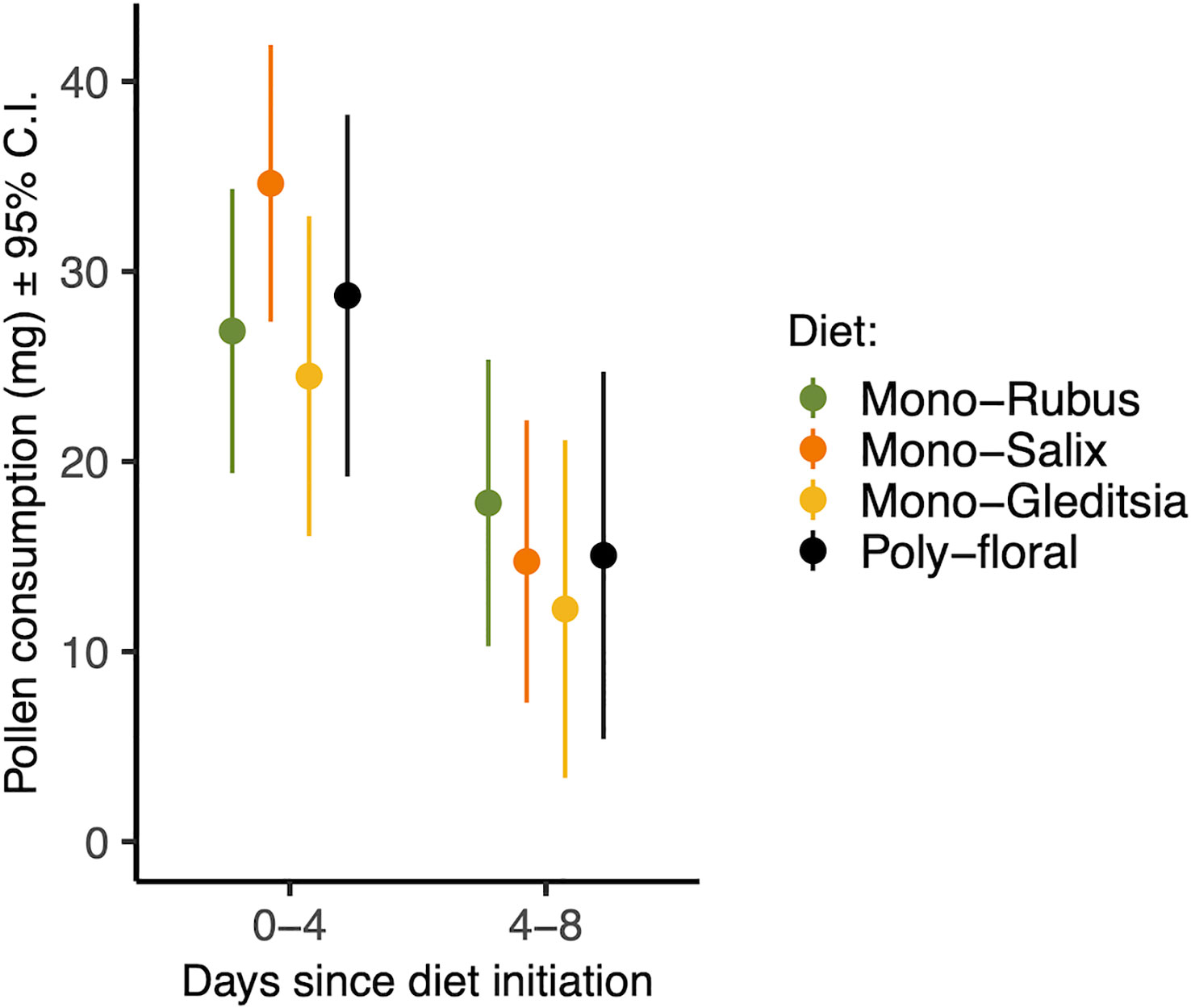
Figure 1 Total pollen consumed from days 0-4 and 4-8 for each pollen diet treatment. Points represent estimated marginal means and error bars represent 95% confidence intervals.
Pathogen spores at 8 days after adult eclosion
At 8 days following adult eclosion, 45% of bees exposed to Nosema as larvae had spores present. There was no significant effect of the diet treatment on the presence of spores (χ2 = 0.69, d.f. = 4, p = 0.953) (Figure 2), nor an effect of body size (χ2 = 1.66, d.f. = 1, p = 0.198). Of those bees with spores present, there was no significant difference in spore numbers based on diet (χ2 = 0.53, d.f. = 4, p = 0.970) (Supplementary Figure 2) or body size (χ2 = 2.90, d.f. = 1, p = 0.088).
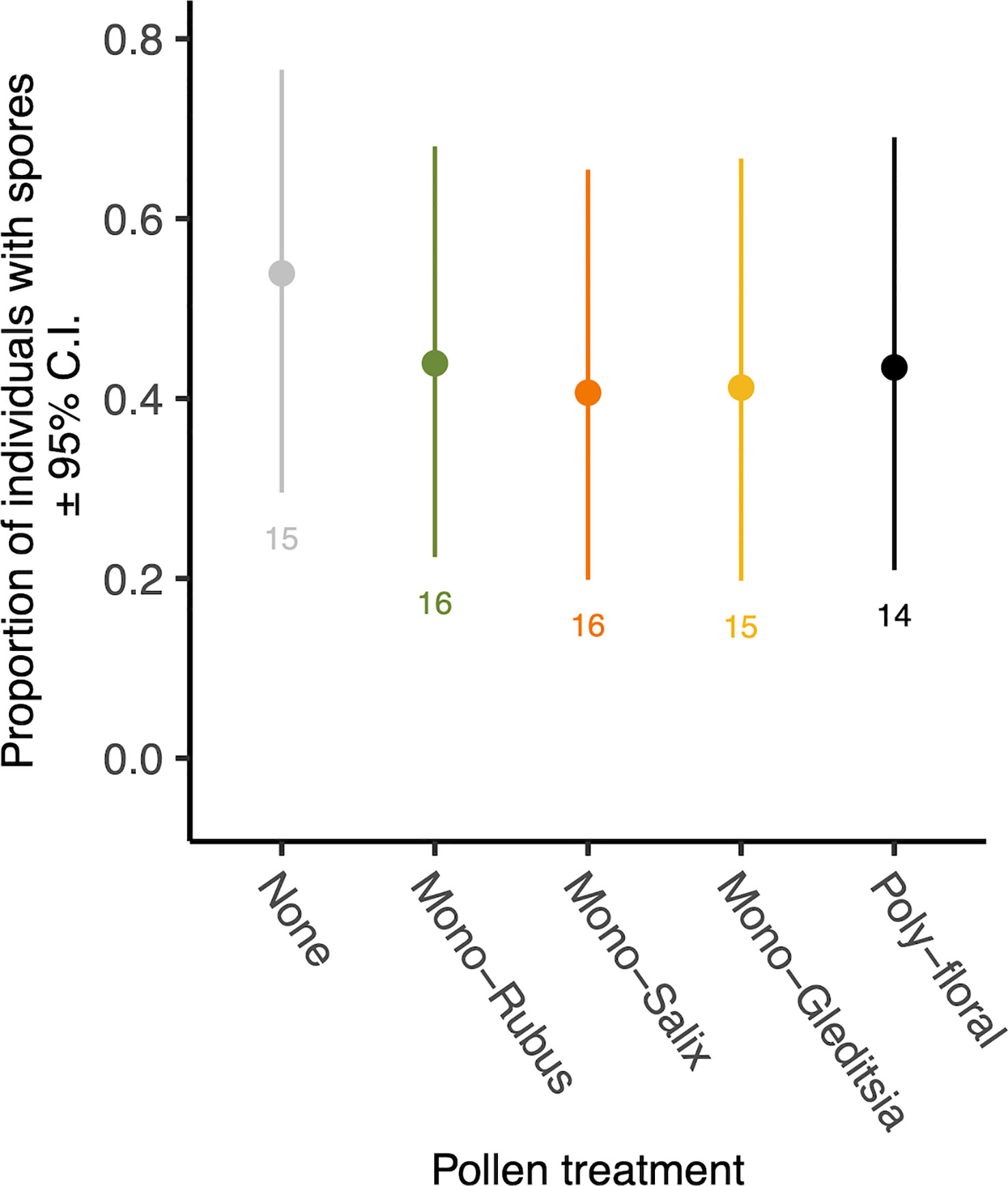
Figure 2 The proportion of 8-day post eclosion adult workers with N. bombi spores by pollen diet treatment. Points represent diet treatment estimated marginal means and error bars represent 95% confidence intervals. Numbers below bars represent sample sizes of each diet treatment.
Pathogen spores on death
At the time of their death, 47.5% of those bees exposed to Nosema had spores present. There was no effect of pollen diet treatment (χ2 = 2.39, d.f. = 4, p = 0.664, Figure 3) or body size (χ2 = 1.38, d.f. = 1, p = 0.240) on the proportion of bees with spores on death. Of those with spores present when they died, there was no significant difference in spore numbers based on diet (χ2 = 2.06, d.f. = 4, p = 0.724) (Supplementary Figure 3). There was, however, a significant effect of body size (χ2 = 5.35, d.f. = 1, p = 0.021), with larger bees having more spores when they died (Supplementary Figure 4).
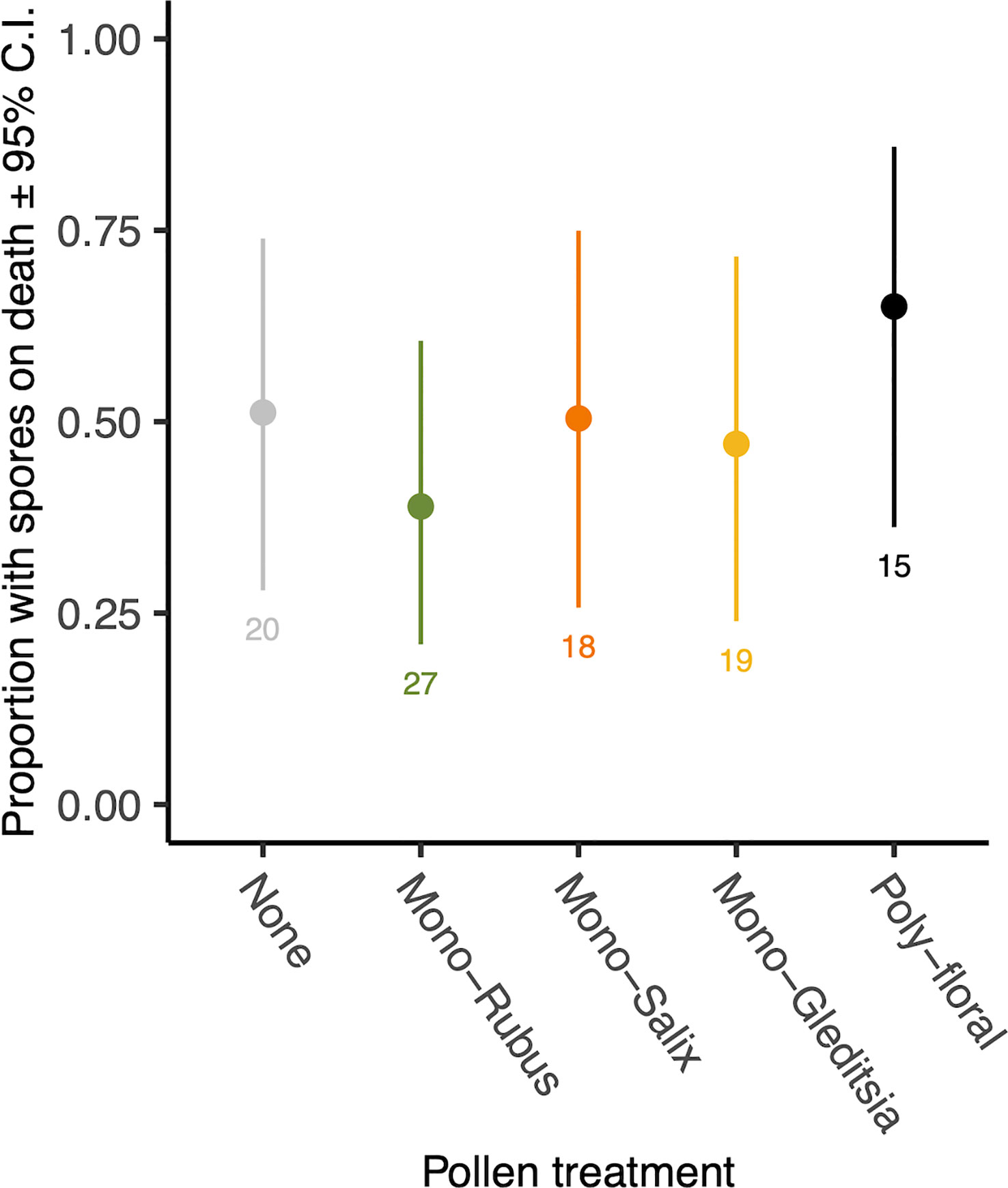
Figure 3 Proportion of workers with N. bombi spores at the time of death across pollen diet treatments. Points represent diet treatment estimated marginal means and error bars represent 95% confidence intervals. Numbers below bars represent sample sizes of each diet treatment.
Survival
There was no significant interaction between Nosema exposure and pollen diet on survival (χ2 = 7.33, d.f. = 4, p = 0.119). However, pathogen exposed bees had a decreased likelihood of survival (χ2 = 4.53, d.f. = 1, p = 0.033) (Figure 4). There was also a significant effect of pollen diet treatment on mortality (χ2 = 27.40, d.f. = 4, p < 0.001, Figure 5), with post hoc tests indicating significantly increased probability of survival in individuals provided with pollen versus those not (Hazard ratio = 3.32, s.e. = 0.79, p < 0.001). Larger individuals also had a higher probability of survival (χ2 = 5.62, d.f. = 1, p = 0.018). When only examining bees exposed to Nosema, and comparing bees with evidence of infection and those without, these outcomes were largely consistent for the interaction of pollen diet treatment and infection status (χ2 = 1.75, d.f. = 4, p = 0.782), infection status (χ2 = 3.97, d.f. = 4, p = 0.046), pollen diet treatment (χ2 = 15.06, d.f. = 4, p = 0.005) and body size (χ2 = 3.53, d.f. = 1, p = 0.060).
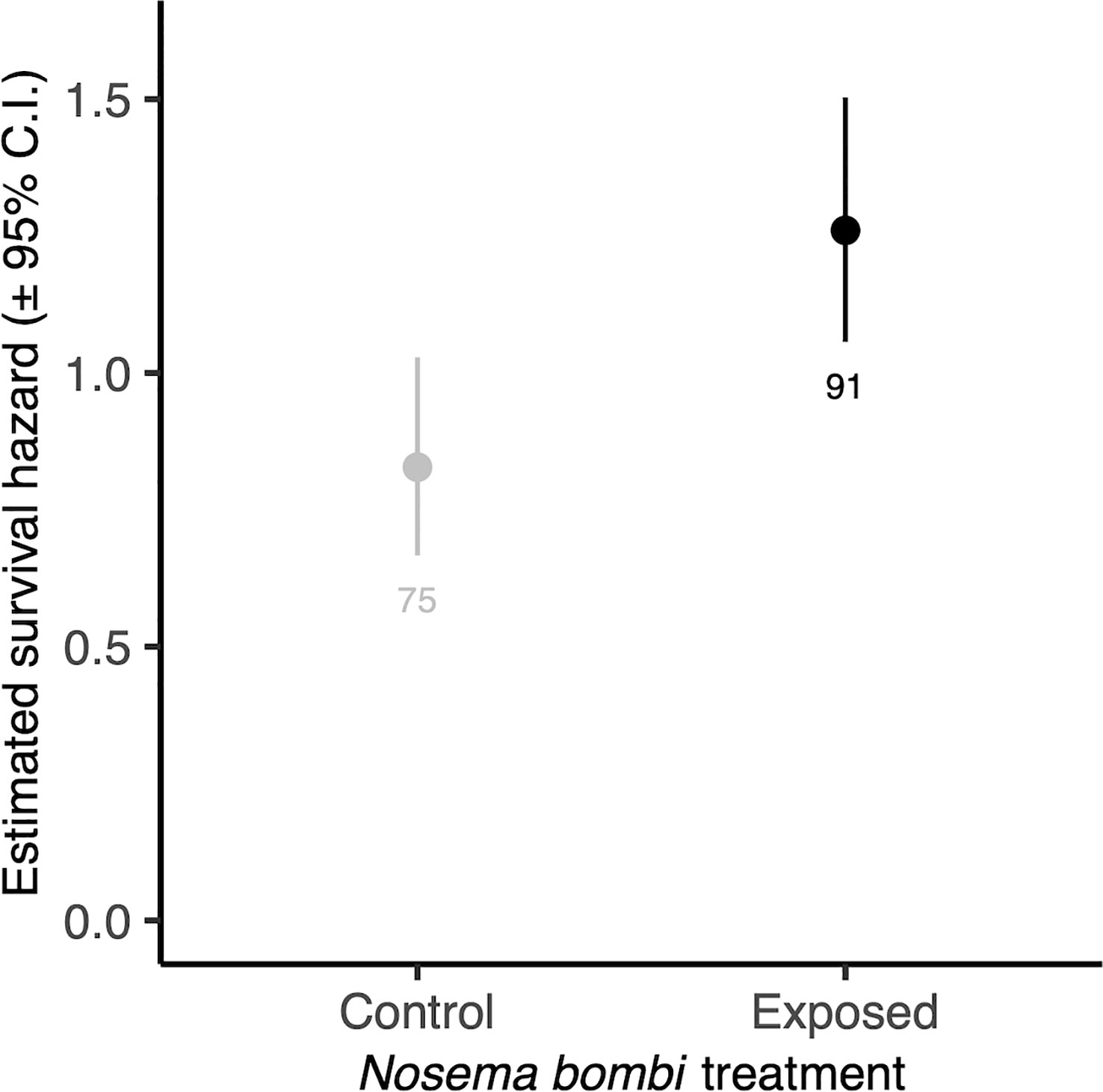
Figure 4 Estimated survival hazards of worker bees exposed to N. bombi and those not. Points represent model estimated survival hazard values and error bars represent 95% confidence interval. Numbers below bars represent sample sizes.
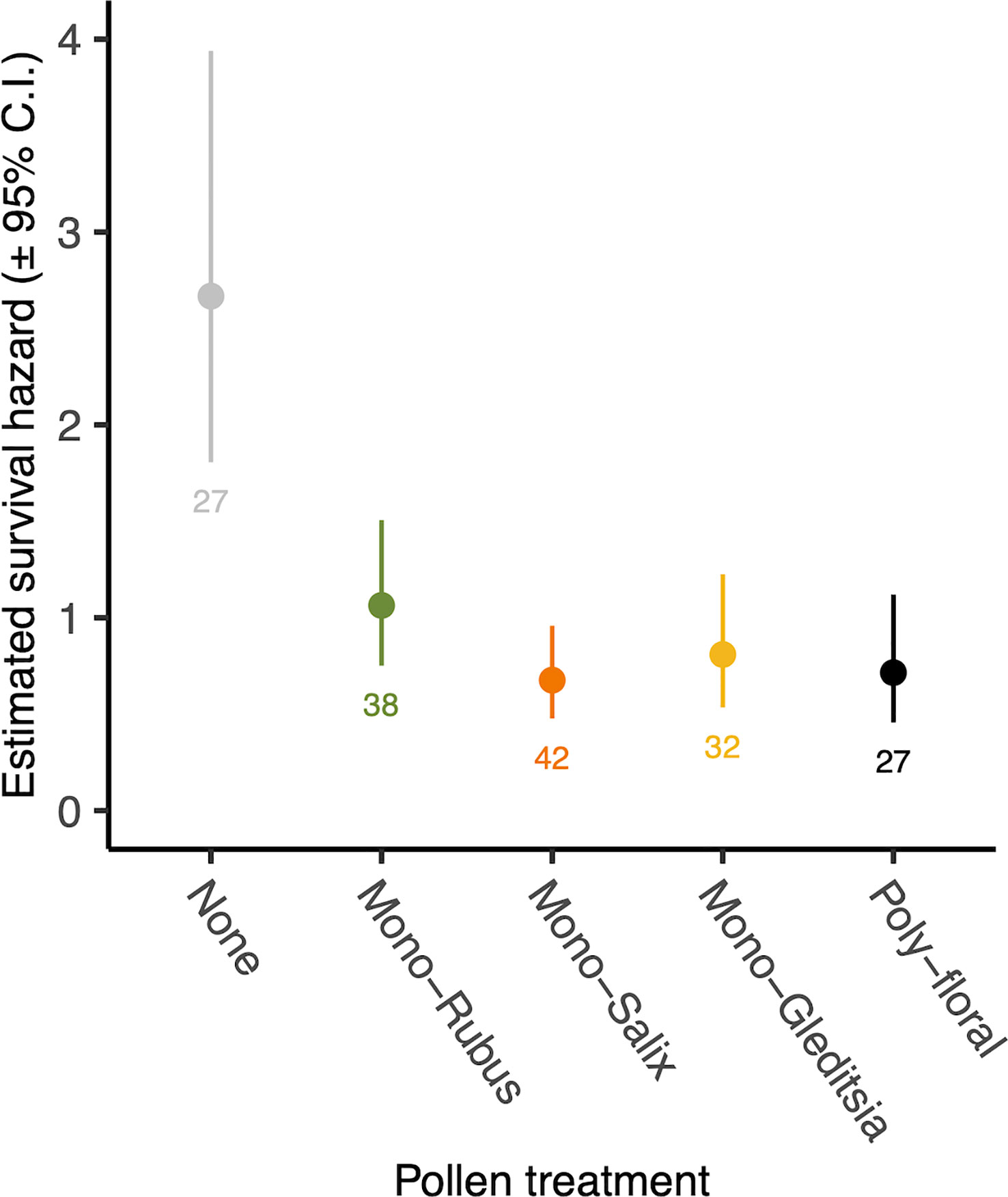
Figure 5 Estimated survival hazard of worker bees across pollen diet treatments. Points represent model estimated survival hazard values and error bars represent 95% confidence interval. Numbers below bars represent sample sizes.
Discussion
The pollen diet treatments employed in our study did not influence resistance to N. bombi infection, with prevalence of spores in eight-day adults, prevalence of spores upon death, and spore loads in dying bees not differing significantly across diet treatments. However, adult body size, something that can be affected by larval diet (31), affected some infection outcomes. Nosema infection negatively affected survival, but we did not find this to be affected by pollen diet treatment. However, pollen diet treatment itself significantly influenced survival, which was driven by reduced survival in pollen deprived adults. This supports other work suggesting the importance of pollen not just for larval development but also for adult bumble bee health (47, 71).
Contrary to our hypothesis, we did not find support that pollen diet, either pollen presence or diversity, influences N. bombi infection outcomes measured. This is in contrast to studies in honey bees infected with other Nosema spp. showing increased infection levels with increased pollen consumption (72, 73) and increased tolerance with greater pollen consumption (42) and with high diverse diets (43). Such effects may have been expected in bumble bees too, where the immune response to and infection by an adult bumble bee gut-infecting trypanosome, C. bombi, is affected in various ways by pollen availability and type (41, 47, 48). We suspect that in our study the exact infection outcomes of spore load and survival had been determined prior to the implementation of the different pollen diet treatments, given that infection establishment and development predominantly occur pre-adult. However, an interaction between pathogen infection and diet could be found under a different experimental regime. Nosema bombi infection establishes most successfully early in bumble bee development in larval instars (59). In our study, larval diet was consistent across all treatments, with pollen diet treatments only imposed during adulthood. It could be that by the time adulthood is reached, the trajectory of the infection is set. However, work showing that an isoflavonoid present in certain pollen types influences N. bombi spore load when consumed by adult but not larval bumble bees (61), suggests that this is not necessarily the case.
In addition to the temporal aspect between larval and adult diets, only three select pollen types were tested in our experiment, with pollen of Salix sp., Gleditsia triacanthos, and Rubus sp. In nature, a greater diversity or availability of different pollen may have different effects based on their distinct profiles of protein, lipid, amino acids, and secondary compounds. It is known that different pollen sources differ in important ways that may affect bumble bee individual and colony health (24, 33, 35), but a diverse poly-floral pollen diet is only expected to be beneficial if the mono-floral diets differ in parameters relevant for health. It has been previously shown that Salix and Rubus differ in protein:lipid ratios (19), but these differences were apparently not sufficient to drive different outcomes in terms of survival alone or infection outcomes documented. In nature, bumble bees may also regulate their intake of pollen in polyfloral landscapes to meet their nutritional demands (23, 27). If different infection outcomes were found under other varied pollen diets, it is feasible that bumble bees could balance their intake to minimize infection-related health losses, as has been seen in other insect systems (74, 75).
In addition to effects on infection resistance, the possibility that all individuals experienced similar diets during important stages of development may be an underlying reason that explains host survival in our study. Similar diets would result in similar anatomical development of Malpighian tubules, thorax muscles, fat body tissue and nerve tissues, all where N. bombi infects (76). The lack of any interaction between pollen diet treatment and infection is perhaps most surprising when contrasting the pollen provisioned and pollen deprived groups, given N. bombi’s metabolic need and energy depletion of host cells (60) and the reduced survival of pollen deprived bees. It is plausible that infected bees in the absence of pollen compensated the loss of energy through increased carbohydrate consumption through sugar water, which was provided ad libitum and was not measured in this study. Future studies should focus on varied pollen compositions and their influence during larval development on immunity and infection.
Although infection outcomes were not affected by diet, we observed negative effects on survival and adult body size in N. bombi infected bees. This study in B. impatiens adds to demonstrations from other bumble bee species of several detrimental impacts of individuals and colonies (56, 57, 77; but see 14). The documented effects on size and survival could come from the cost of infection directly due to tissue damage or energy utilization of the microsporidian (60, 76) or from a shift in resource allocation from development and other traits to upregulate costly immunity (78).
We found that larger bees had higher numbers of spores on death. An increased area in the gut has been proposed to be a potential cause for an increase in N. bombi infection (79), which could link body size, infection, and eventual spore numbers on death. However, a significant effect of size was not seen on infection at eight days post-adult eclosion. It is also possible that bees surviving longer could have more time for spores to accumulate. In honey bees, N. ceranae spore loads increased as bees aged (80). With survival positively associated with body size, the relationship between body size and spore number on death could be explained by a similar relationship between age and spore loads.
In conclusion, we did not find that infections were mitigated in adult workers given pollen or given poly-floral diets compared to mono-floral diets. However, we found evidence that both factors are important for determining bumble bee health, but in the experimental infection and diet regimes assessed here they act independently. Nevertheless, combinations of the stressors of pathogens and a dearth of pollen in bumble bee populations will be more detrimental than each alone, due to their additive effects. Moreover, at the colony or bee pollinator community level, it is plausible that pollen diets could interact with infection or dietary effects on pathogen infection could emerge. With anthropogenic stressors threatening floral resources, and therefore pollen availability, future work should further investigate such potential diet and infectious disease links.
Data availability statement
The original contributions presented in the study are included in the article/Supplementary Material. Further inquiries can be directed to the corresponding author.
Ethics statement
The manuscript presents research on animals that do not require ethical approval for their study.
Author contributions
AM, AC, and BS conceived and designed the experiments. AM and AC performed the experiments. AM and BS analyzed the data. AM and BS wrote the manuscript; AC provided editorial advice. All authors contributed to the article and approved the submitted version
Funding
This work was supported by the National Institutes of Health [grant R15 GM129681-01 to BS], the United States Department of Agriculture [grant 2017-67013-26536 to BS], the Great Lakes Restoration Initiative through the U.S. Fish and Wildlife Service’s Threatened and Endangered Species Template [grant F22AP02271-00 to BS], and a Sigma Xi Grant-in-aid of research to AM.
Acknowledgments
The authors thank other Sadd lab members for assistance with colony maintenance and discussion.
Conflict of interest
The authors declare that the research was conducted in the absence of any commercial or financial relationships that could be construed as a potential conflict of interest.
The author BS declared that they were an editorial board member of Frontiers at the time of submission. This had no impact on the peer review process and the final decision.
Publisher’s note
All claims expressed in this article are solely those of the authors and do not necessarily represent those of their affiliated organizations, or those of the publisher, the editors and the reviewers. Any product that may be evaluated in this article, or claim that may be made by its manufacturer, is not guaranteed or endorsed by the publisher.
Supplementary material
The Supplementary Material for this article can be found online at: https://www.frontiersin.org/articles/10.3389/finsc.2023.1207058/full#supplementary-material
References
1. Raubenheimer D, Simpson SJ, Mayntz D. Nutrition, ecology and nutritional ecology: toward an integrated framework. Funct Ecol (2009) 23:4–16. doi: 10.1111/j.1365-2435.2009.01522.x
2. Katona P, Katona-Apte J. The interaction between nutrition and infection. Clin Infect Dis (2008) 46:1582–8. doi: 10.1086/587658
3. Taylor CH, Young S, Fenn J, Lamb AL, Lowe AE, Poulin B, et al. Immune state is associated with natural dietary variation in wild mice Mus musculus domesticus. Funct Ecol (2019) 33:1425–35. doi: 10.1111/1365-2435.13354
4. Costantin EC, Viol DL, Del Puppo NP, Elliot SL. Realism in immune ecology studies: artificial diet enhances a caterpillar’s immune defense but does not mask the effects of a plastic immune strategy. Front Insect Sci (2022) 1:754571. doi: 10.3389/finsc.2021.754571
5. Pirk CW, Scheiner R. The effects of diet on health in insects. Front Insect Sci (2023) 3:1186027. doi: 10.3389/finsc.2023.1186027
6. Howick VM, Lazzaro BP. Genotype and diet shape resistance and tolerance across distinct phases of bacterial infection. BMC Evolutionary Biol (2014) 14:56. doi: 10.1186/1471-2148-14-56
7. Frizzera D, Ray AM, Seffin E, Zanni V, Annoscia D, Grozinger CM, et al. The beneficial effect of pollen on Varroa infested bees depends on its influence on behavioral maturation genes. Front Insect Sci (2022) 2:864238. doi: 10.3389/finsc.2022.864238
8. Gowler CD, Leon KE, Hunter MD, de Roode JC. Secondary defense chemicals in milkweed reduce parasite infection in monarch butterflies, Danaus plexippus. J Chem Ecol (2015) 41:520–3. doi: 10.1007/s10886-015-0586-6
9. Jiang JF, Song XM, Wu JL, Jiang YQ. Effects of alfalfa meal on the intestinal microbial diversity and immunity of growing ducks. J Anim Physiol Anim Nutr (2014) 98:1039–46. doi: 10.1111/jpn.12167
10. Krams IA, Kecko S, Jõers P, Trakimas G, Elferts D, Krams R, et al. Microbiome symbionts and diet diversity incur costs on the immune system of insect larvae. J Exp Biol (2017) 220:4204–12. doi: 10.1242/jeb.169227
11. Hudson AL, Moatt JP, Vale PF. Terminal investment strategies following infection are dependent on diet. J Evolutionary Biol (2019) 33:309–17. doi: 10.1111/jeb.13566
12. Cameron SA, Sadd BM. Global trends in bumble bee health. Annu Rev Entomol (2020) 65:209–32. doi: 10.1146/annurev-ento-011118-111847
13. Winfree R, Bartomeus I, Cariveau DP. Native pollinators in anthropogenic habitats. Annu Rev Ecology Evolution Systematics (2011) 42:1–22. doi: 10.1146/annurev-ecolsys-102710-145042
14. Calhoun AC, Harrod AE, Bassingthwaite TA, Sadd BM. Testing the multiple stressor hypothesis: chlorothalonil exposure alters transmission potential of a bumblebee pathogen but not individual host health. Proc R Soc B: Biol Sci (2021) 288:20202922. doi: 10.1098/rspb.2020.2922
15. Vanbergen AJ, The Insect Pollinators Initiative. Threats to an ecosystem service: pressures on pollinators. Front Ecol Environ (2013) 11:251–9. doi: 10.1890/120126
16. Goulson D, Nicholls E, Botías C, Rotheray EL. Bee declines driven by combined stress from parasites, pesticides, and lack of flowers. Science (2015) 347:1255957. doi: 10.1126/science.1255957
17. Woodard SH. Bumble bee ecophysiology: integrating the changing environment and the organism. Curr Opin Insect Sci (2017) 22:101–8. doi: 10.1016/j.cois.2017.06.001
18. Woodard SH, Jha S. Wild bee nutritional ecology: predicting pollinator population dynamics, movement, and services from floral resources. Curr Opin Insect Sci (2017) 21:83–90. doi: 10.1016/j.cois.2017.05.011
19. Vaudo AD, Tooker JF, Patch HM, Biddinger DJ, Coccia M, Crone MK, et al. Pollen protein: lipid macronutrient ratios may guide broad patterns of bee species floral preferences. Insects (2020) 11:132. doi: 10.3390/insects11020132
20. Heil M. Nectar: generation, regulation and ecological functions. Trends Plant Sci (2011) 16:191–200. doi: 10.1016/j.tplants.2011.01.003
21. Tasei JN, Aupinel P. Nutritive value of 15 single pollens and pollen mixes tested on larvae produced by bumblebee workers (Bombus terrestris, Hymenoptera: Apidae). Apidologie (2008) 39:397–409. doi: 10.1051/apido:2008017
22. Weiner CN, Hilpert A, Werner M, Linsenmair KE, Blüthgen N. Pollen amino acids and flower specialisation in solitary bees. Apidologie (2010) 41:476–87. doi: 10.1051/apido/2009083
23. Vaudo AD, Patch HM, Mortensen DA, Tooker JF, Grozinger CM. Macronutrient ratios in pollen shape bumble bee (Bombus impatiens) foraging strategies and floral preferences. Proc Natl Acad Sci U S A (2016) 113:E4035–42. doi: 10.1073/pnas.1606101113
24. Moerman R, Vanderplanck M, Fournier D, Jacquemart AL, Michez D. Pollen nutrients better explain bumblebee colony development than pollen diversity. Insect Conserv Diversity (2017) 10:171–9. doi: 10.1111/icad.12213
25. Shuaib M, Ali K, Ahmed S, Hussain F, Ilyas M, Hassan N, et al. Impact of rapid urbanization on the floral diversity and agriculture land of district Dir, Pakistan. Acta Ecologica Sin (2018) 38:394–400. doi: 10.1016/j.chnaes.2018.04.002
26. Heinrich B. Energetics of pollination. Annu Rev Ecol Systematics (1975) 6:139–70. doi: 10.1146/annurev.es.06.110175.001035
27. Vaudo AD, Farrell LM, Patch HM, Grozinger CM, Tooker JF. Consistent pollen nutritional intake drives bumble bee (Bombus impatiens) colony growth and reproduction across different habitats. Ecology and Evolution (2018) 8:5765–76. doi: 10.1002/ece3.4115
28. Hass AL, Brachmann L, Batáry P, Clough Y, Behling H, Tscharntke T. Maize-dominated landscapes reduce bumblebee colony growth through pollen diversity loss. J Appl Ecol (2019) 56:294–304. doi: 10.1111/1365-2664.13296
29. Génissel A, Aupinel P, Bressac C, Tasei JN, Chevrier C. Influence of pollen origin on performance of Bombus terrestris micro-colonies. Entomologia Experimentalis Applicata (2002) 104:329–36. doi: 10.1046/j.1570-7458.2002.01019.x
30. Leza M, Watrous K, Bratu J, Woodard SH. Effects of neonicotinoid insecticide exposure and monofloral diet on nest-founding bumblebee queens. Proc R Soc B: Biol Sci (2018) 285:20180761. doi: 10.1098/rspb.2018.0761
31. Sutcliffe GH, Plowright RC. The effects of food supply on adult size in the bumble bee Bombus terricola Kirby (Hymenoptera: Apidae). Can Entomologist (1988) 120:1051–8. doi: 10.4039/Ent1201051-12
32. Yoon HJ, Kim SE, Lee KY, Lee SB, Park IG. Oviposition and colony development of the bumblebees, Bombus ignitus and B. terrestris depending on different pollen. Int J Ind Entomol (2005) 11:99–105.
33. Moerman R, Roger N, De Jonghe R, Michez D, Vanderplanck M. Interspecific variation in bumblebee performance on pollen diet: new insights for mitigation strategies. PloS One (2016) 11:e0168462. doi: 10.1371/journal.pone.0168462
34. McAulay MK, Forrest JRK. How do sunflower pollen mixtures affect survival of queenless microcolonies of bumblebees (Bombus impatiens)? Arthropod-Plant Interact (2019) 13:517–29. doi: 10.1007/s11829-018-9664-3
35. Watrous KM, Duennes MA, Woodard SH. Pollen diet composition impacts early nesting success in queen bumble bees Bombus impatiens Cresson (Hymenoptera: Apidae). Environ Entomol (2019) 48:711–7. doi: 10.1093/ee/nvz043
36. Vaudo AD, Stabler D, Patch HM, Tooker JF, Grozinger CM, Wright GA. Bumble bees regulate their intake of essential protein and lipid pollen macronutrients. J Exp Biol (2016) 219:3962–70. doi: 10.1242/jeb.140772
37. Koch H, Brown MJ, Stevenson PC. The role of disease in bee foraging ecology. Curr Opin Insect Sci (2017) 21:60–7. doi: 10.1016/j.cois.2017.05.008
38. Piot N, Meeus I, Kleijn D, Scheper J, Linders T, Smagghe G. Establishment of wildflower fields in poor quality landscapes enhances micro-parasite prevalence in wild bumble bees. Oecologia (2019) 189:149–58. doi: 10.1007/s00442-018-4296-y
39. Figueroa LL, Grab H, Ng WH, Myers CR, Graystock P, McFrederick QS, et al. Landscape simplification shapes pathogen prevalence in plant-pollinator networks. Ecol Lett (2020) 23:1212–22. doi: 10.1111/ele.13521
40. Gegear RJ, Otterstatter MC, Thomson JD. Bumble-bee foragers infected by a gut parasite have an impaired ability to utilize floral information. Proc R Soc B: Biol Sci (2006) 273:1073–8. doi: 10.1098/rspb.2005.3423
41. Logan A, Ruiz-González MX, Brown MJF. The impact of host starvation on parasite development and population dynamics in an intestinal trypanosome parasite of bumble bees. Parasitology (2005) 130:637–42. doi: 10.1017/S0031182005007304
42. Jack CJ, Uppala SS, Lucas HM, Sagili RR. Effects of pollen dilution on infection of Nosema ceranae in honey bees. J Insect Physiol (2016) 87:12–9. doi: 10.1016/j.jinsphys.2016.01.004
43. Di Pasquale G, Salignon M, Le Conte Y, Belzunces LP, Decourtye A, Kretzschmar A, et al. Influence of pollen nutrition on honey bee health: do pollen quality and diversity matter? PloS One (2013) 8:e72016. doi: 10.1371/journal.pone.0072016
44. Foley K, Fazio G, Jensen AB, Hughes WO. Nutritional limitation and resistance to opportunistic Aspergillus parasites in honey bee larvae. J Invertebrate Pathol (2012) 111:68–73. doi: 10.1016/j.jip.2012.06.006
45. Alaux C, Ducloz F, Crauser D, Le Conte Y. Diet effects on honeybee immunocompetence. Biol Lett (2010) 6:562–5. doi: 10.1098/rsbl.2009.0986
46. Roger N, Michez D, Wattiez R, Sheridan C, Vanderplanck M. Diet effects on bumblebee health. J Insect Physiol (2017) 96:128–33. doi: 10.1016/j.jinsphys.2016.11.002
47. Brunner FS, Schmid-Hempel P, Barribeau SM. Protein-poor diet reduces host-specific immune gene expression in Bombus terrestris. Proc R Soc B: Biol Sci (2014) 281:20140128. doi: 10.1098/rspb.2014.0128
48. Giacomini JJ, Leslie J, Tarpy DR, Palmer-Young EC, Irwin RE, Adler LS. Medicinal value of sunflower pollen against bee pathogens. Sci Rep (2018) 8:1–10. doi: 10.1038/s41598-018-32681-y
49. Fowler AE, Sadd BM, Bassingthwaite T, Irwin RE, Adler LS. Consuming sunflower pollen reduced pathogen infection but did not alter measures of immunity in bumblebees. Philos Trans R Soc B (2022) 377:20210160. doi: 10.1098/rstb.2021.0160
50. Figueroa LL, Fowler A, Lopez S, Amaral VE, Koch H, Stevenson PC, et al. Sunflower spines and beyond: mechanisms and breadth of pollen that reduce gut pathogen infection in the common eastern bumble bee. Funct Ecol (2023) 37:1757–69. doi: 10.1111/1365-2435.14320
51. Cameron SA, Lozier JD, Strange JP, Koch JB, Cordes N, Solter LF, et al. Patterns of widespread decline in North American bumble bees. Proc Natl Acad Sci U S A (2011) 108:662–7. doi: 10.1073/pnas.1014743108
52. Cameron SA, Lim HC, Lozier JD, Duennes MA, Thorp R. Test of the invasive pathogen hypothesis of bumble bee decline in North America. Proc Natl Acad Sci U S A (2016) 113:4386–91. doi: 10.1073/pnas.1525266113
53. Malfi RL, Roulston TAH. Patterns of parasite infection in bumble bees (Bombus spp.) of Northern Virginia. Ecol Entomol (2014) 39:17–29. doi: 10.1111/een.12069
54. Tokarev YS, Huang WF, Solter LF, Malysh JM, Becnel JJ, Vossbrinck CR. A formal redefinition of the genera Nosema and Vairimorpha (Microsporidia: Nosematidae) and reassignment of species based on molecular phylogenetics. J Invertebrate Pathol (2020) 169:107279. doi: 10.1016/j.jip.2019.107279
55. Aime MC, Miller AN, Aoki T, Bensch K, Cai L, Crous PW, et al. How to publish a new fungal species, or name, version 3.0. IMA fungus (2021) 12:1–15. doi: 10.1186/s43008-021-00063-1
56. Otti O, Schmid-Hempel P. Nosema bombi: a pollinator parasite with detrimental fitness effects. J Invertebrate Pathol (2007) 96:118–24. doi: 10.1016/j.jip.2007.03.016
57. Otti O, Schmid-Hempel P. A field experiment on the effect of Nosema bombi in colonies of the bumblebee Bombus terrestris. Ecol Entomol (2008) 33:577–82. doi: 10.1111/j.1365-2311.2008.00998.x
58. Rutrecht ST, Brown MJ. The life-history impact and implications of multiple parasites for bumble bee queens. Int J Parasitol (2008) 38:799–808. doi: 10.1016/j.ijpara.2007.11.004
59. Rutrecht ST, Klee J, Brown MJF. Horizontal transmission success of Nosema bombi to its adult bumble bee hosts: effects of dosage, spore source and host age. Parasitology (2007) 134:1719–26. doi: 10.1017/S0031182007003162
60. Williams BA. Unique physiology of host–parasite interactions in microsporidia infections. Cell Microbiol (2009) 11:1551–60. doi: 10.1111/j.1462-5822.2009.01362.x
61. Folly AJ, Stevenson PC, Brown MJ. Age-related pharmacodynamics in a bumblebee–microsporidian system mirror similar patterns in vertebrates. J Exp Biol (2020) 223:jeb217828. doi: 10.1242/jeb.217828
62. Råberg L, Sim D, Read AF. Disentangling genetic variation for resistance and tolerance to infectious diseases in animals. Science (2007) 318:812–4. doi: 10.1126/science.1148526
63. Müller CB, Blackburn TM, Schmid-Hempel P. Field evidence that host selection by conopid parasitoids is related to host body size. Insectes Sociaux (1996) 43:227–33. doi: 10.1007/BF01242924
64. Castiglioni S, Astolfi P, Conti C, Monaci E, Stefano M, Carloni P. Morphological, physicochemical and FTIR spectroscopic properties of bee pollen loads from different botanical origin. Molecules (2019) 24:3974. doi: 10.3390/molecules24213974
65. Kamo T, Kusumoto Y, Tokuoka Y, Okubo S, Hayakawa H, Yoshiyama M, et al. A DNA barcoding method for identifying and quantifying the composition of pollen species collected by European honeybees, Apis mellifera (Hymenoptera: Apidae). Appl Entomol Zoology (2018) 53:353–61. doi: 10.1007/s13355-018-0565-9
66. R Core Team. R: A language and environment for statistical computing. Vienna, Austria: R Foundation for Statistical Computing (2020).
67. Therneau T. Mixed effects Cox models in R. Release 2.2-16 (2020). Available at: https://CRAN.R-project.org/package=coxme.
68. Bates D, Mächler M, Bolker B, Walker S. Fitting linear mixed-effects models using lme4. J Stat Software (2015) 67:1–48. doi: 10.18637/jss.v067.i01
69. Burnham KP, Anderson DR, Huyvaert KP. AIC model selection and multimodel inference in behavioral ecology: some background, observations, and comparisons. Behav Ecol Sociobiol (2011) 65:23–35. doi: 10.1007/s00265-010-1029-6
70. Lenth R, Singmann H, Love J, Buerkner P, Herve M. emmeans: estimated marginal means, aka least-squares means. Release 1.4.5 (2020). Available at: https://CRAN.R-project.org/package=emmeans.
71. Smeets P, Duchateau MJ. Longevity of Bombus terrestris workers (Hymenoptera: Apidae) in relation to pollen availability, in the absence of foraging. Apidologie (2003) 34:333–7. doi: 10.1051/apido:2003026
72. Rinderer TE, Dell Elliott K. Worker honey bee response to infection with Nosema apis: influence of diet. J Economic Entomol (1977) 70:431–3. doi: 10.1093/jee/70.4.431
73. Fleming JC, Schmehl DR, Ellis JD. Characterizing the impact of commercial pollen substitute diets on the level of Nosema spp. in honey bees (Apis mellifera L.). PloS One (2015) 10:e0132014. doi: 10.1371/journal.pone.0132014
74. Povey S, Cotter SC, Simpson SJ, Wilson K. Dynamics of macronutrient self-medication and illness-induced anorexia in virally infected insects. J Anim Ecol (2014) 83:245–55. doi: 10.1111/1365-2656.12127
75. de Roode JC, Hunter MD. Self-medication in insects: when altered behaviors of infected insects are a defense instead of a parasite manipulation. Curr Opin Insect Sci (2019) 33:1–6. doi: 10.1016/j.cois.2018.12.001
76. Fries I, De Ruijter AAD, Paxton RJ, da Silva AJ, Slemenda SB, Pieniazek NJ. Molecular characterization of Nosema bombi (Microsporidia: Nosematidae) and a note on its sites of infection in Bombus terrestris (Hymenoptera: Apoidea). J Apicultural Res (2001) 40:91–6. doi: 10.1080/00218839.2001.11101056
77. van Der Steen JJ. Infection and transmission of Nosema bombi in Bombus terrestris colonies and its effect on hibernation, mating and colony founding. Apidologie (2008) 39:273–82. doi: 10.1051/apido:2008006
78. Sadd BM, Schmid-Hempel P. Principles of ecological immunology. Evolutionary Appl (2009) 2:113–21. doi: 10.1111/j.1752-4571.2008.00057.x
79. Larsson JR. Cytological variation and pathogenicity of the bumble bee parasite Nosema bombi (Microspora, Nosematidae). J Invertebrate Pathol (2007) 94:1–11. doi: 10.1016/j.jip.2006.07.006
Keywords: diet diversity, infection, Nosema bombi, pollinator health, pollen, parasite, Bombus
Citation: Martinez A, Calhoun AC and Sadd BM (2023) Investigating the influence of diet diversity on infection outcomes in a bumble bee (Bombus impatiens) and microsporidian (Nosema bombi) host-pathogen system. Front. Insect Sci. 3:1207058. doi: 10.3389/finsc.2023.1207058
Received: 17 April 2023; Accepted: 01 August 2023;
Published: 17 August 2023.
Edited by:
Fabio Manfredini, University of Aberdeen, United KingdomReviewed by:
Chuan Ma, Chinese Academy of Agricultural Sciences, ChinaArran James Folly, Animal and Plant Health Agency, United Kingdom
Copyright © 2023 Martinez, Calhoun and Sadd. This is an open-access article distributed under the terms of the Creative Commons Attribution License (CC BY). The use, distribution or reproduction in other forums is permitted, provided the original author(s) and the copyright owner(s) are credited and that the original publication in this journal is cited, in accordance with accepted academic practice. No use, distribution or reproduction is permitted which does not comply with these terms.
*Correspondence: Ben M. Sadd, Ym1zYWRkQGlsc3R1LmVkdQ==
†Present address: Abraham Martinez, Department of Cell and Developmental Biology, University of Colorado, Anschutz Medical Campus, Aurora, CO, United States