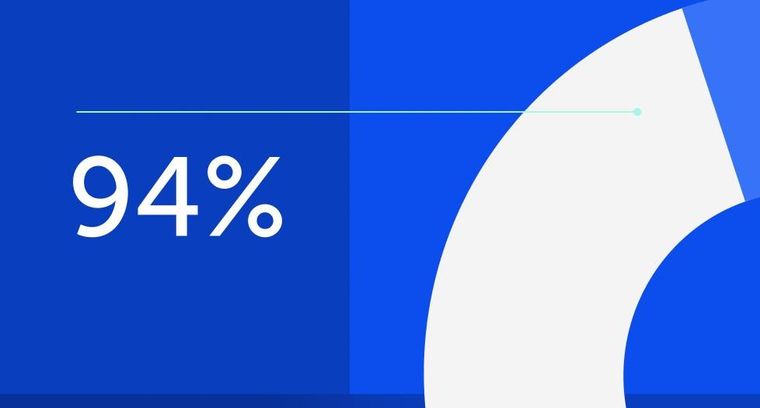
94% of researchers rate our articles as excellent or good
Learn more about the work of our research integrity team to safeguard the quality of each article we publish.
Find out more
REVIEW article
Front. Insect Sci., 29 August 2023
Sec. Insect Health and Pathology
Volume 3 - 2023 | https://doi.org/10.3389/finsc.2023.1195254
This article is part of the Research TopicPhysiological Alterations of Nematodes Influenced by Cross-Phylum SymbiosesView all 5 articles
The term “microbial control” has been used to describe the use of microbial pathogens (bacteria, viruses, or fungi) or entomopathogenic nematodes (EPNs) to control various insect pest populations. EPNs are among the best biocontrol agents, and major developments in their use have occurred in recent decades, with many surveys having been conducted all over the world to identify EPNs that may have potential in the management of insect pests. For nematodes, the term “entomopathogenic” means “causing disease to insects” and is mainly used in reference to the bacterial symbionts of Steinernema and Heterorhabditis (Xenorhabdus and Photorhabdus, respectively), which cause EPN infectivity. A compendium of our multiannual experiences on EPN surveys and on their collection, identification, characterization, and use in agro-forestry ecosystems is presented here to testify and demonstrate once again that biological control with EPNs is possible and offers many advantages over chemicals, such as end-user safety, minimal damage to natural enemies, and lack of environmental pollution, which are essential conditions for an advanced IPM strategy.
The majority of nematodes are free-living organisms found in soil or in water. One-quarter of all nematodes are parasites of plants or animals (1), and, among the latter, some species are associated in various ways with insects. These relationships range from phoresy to symbiosis, and from commensalism to facultative or obligate parasitism (2, 3), and may involve insect parasitic nematodes (e.g., the natural parasite Hexamermis sp.) (Figure 1) or entomopathogenic nematodes (EPNs). They are frequently found in nature, infesting their hosts on the exoskeleton, or in the hemocoel, or nested in the reproductive, respiratory, digestive, or excretory systems. They can induce sterility; reduce fecundity, longevity, and the host’s ability to move; induce developmental delay; cause morphological changes; and ultimately host death may occur (4). Among more than 30 families of nematodes associated with insects, the families Steinernematidae Filipjev, 1934, and Heterorhabditidae Poinar, 1976 (order Rhabditida), are the ones that arouse the most interest. From a practical point of view in terms of hexapod control, nematodes within these two families are more accurately called entomopathogens because they exert their action in association with symbiotic bacteria (5–7).
Figure 1 Hexamermis sp. (Nematoda, Mermithidae), entomoparasitic nematode predating on Thaumetopoea pityocampa larva.
The biological cycle begins with young females laying eggs in the substrate, but when they become older or hermaphrodite, the eggs hatch in the mother’s uterus (endotokia matricida) (8). In both Steinernematidae and Heterorhabditidae, it is the third stage, also known as the infective juvenile (IJ) stage, that initiates the infection. After reproduction and multiplication, the IJs abandon the cadaver, retaining the cuticle of the previous stage that protects them from dehydration, attacks from pathogenic fungi, and other forms of stress (9). Their subsequent movement through the soil in search of new hosts leads to the loss of this protective cuticle. Having identified the target host, IJs penetrate its body, preferably through natural openings (i.e., the mouth, anus, or stigmas) (Figure 2), after drilling its tracheae or intestines (10). Heterorhabditis spp., having a tooth in the anterior part of the body, have a comparative advantage because they are also able to pass through intersegmental membranes (11). The Steinernema species, although lacking such a structure, are also able to pass through the integument favored by the high hydrostatic pressure characteristic of small nematodes, the size of the anterior part of their body (ca. 8–15 μm), and the secretion of histolytic enzymes. Thus, due to the absence of an epicuticular protective layer that would otherwise block the action of the histolytic enzymes produced by the IJs, Steinernema feltiae overcome the tegument barrier of the larvae of the Diptera species Tipula paludosa Meigen and T. oleracea L (12). Once in the hemolymph, the IJs release the symbiotic bacteria present in their gut: the Steinernema release Xenorhabdus spp. and the Heterorhabditis release Photorhabdus spp. The nematodes act like a small syringe to inject the bacteria. In the hemolymph, these bacteria multiply rapidly and produce a wide range of toxins and exoenzymes that kill the host, turning its tissues into a kind of soup on which the nematodes feed to reach the adult stage after four stages of development. Antimicrobial substances that are also secreted promote the development of symbiotic bacteria and nematodes (13). Additionally, the nematodes themselves make significant contributions to killing the host (14–19). When they reach the adult stage, Steinernema spp. mate and produce successive generations, whereas IJs of the Heterorhabditis spp. develop into self-fertilizing hermaphroditic females that will produce males and females in the next generation. The cycle is completed within a few days, and hundreds of thousands of new IJs will emerge from the now- destroyed host in search of new victims. Invasion of a victim by a single individual of Heterorhabditis is enough to produce a new generation, whereas at least two IJs are needed in the case of Steinernema, being gonochoric (20). When the food supply becomes scarce, development stops at the IJ stage and the cadaver is abandoned (Figure 3). Steinernema and Heterorhabditis spp. parasitize a wide range of insect species, and the duration of the biological cycle is influenced by both the environmental temperature and the species/strain of the nematode itself. Usually, the death of the host occurs quickly, as 48 hours is sufficient time for the bacteria to take effect.
Figure 2 Infective juvenile (IJ) stage of Steinernema feltiae penetrating through the mouth of a young Capnodis tenebrionis larva.
Symbiosis with bacteria is the reason why Steinernematidae and Heterorhabditidae are more correctly called entomopathogenic nematodes and not entomoparasites. Photorhabdus and Xenorhabdus, symbionts of Steinernema and Heterorhabditis, respectively, belong to the family Enterobacteriaceae and are elongated (0.5 × 1–10 μm for the former, 0.3–2 × 2–10 μm for the latter) asporigenous Gram-negative and facultative anaerobic bacteria (21, 22). These entomopathogenic bacteria are obligate symbionts of EPN species; they biosynthesize and release secondary metabolites on artificial substrates with antibiotic activity against Gram-positive and Gram-negative bacteria and with antifungal, nematicidal, anti-ulcer, antiviral, and antitumor activity (23–25). The nematode–bacterium symbiotic relationship results in the nematode protecting the bacterium from the external environment and introducing it into the host, with the bacterium providing food for the nematode to develop. Xenorhabdus reside in a special bladder located just behind the basal bulb of the esophagus present in the infective stages of Steinernema (26), whereas Phothorabdus are housed in the middle part of the intestine of Heterorhabditis IJs (27). Although bacteria and nematodes can reproduce separately, together they have high specificity (26). However, there are some exceptions, such as X. bovienii (which is a symbiont not only of S. affine but also of S. feltiae, S. ichnusae, S. kraussei, S. intermedium, and S. weiseri) and X. kozodoii (which is associated with S. arenarium and S. apuliae) (28, 29). Among the Photorhabdus, P. luminescens and P. luminescens laumondii are simultaneously present in H. bacteriophora (30). Insects killed by the bacteria take on characteristic colorations: victims of Xenorhabdus become grayish or creamy yellow, whereas those with Photorhabdus in their tissues are deep red, or in rare cases greenish, and moderately luminescent in the dark (Figure 4). Both kinds of bacteria, when multiplying on artificial substrate for a long period of time, produce cells of the second type (or phase II), which have altered properties compared with cells isolated from nematodes (or phase I) and are not infectious. Phase II does not occur naturally in nematodes. Recently, in the context of this nematode–bacteria symbiosis, an infectious contribution has also been demonstrated for the bacterium Pseudomonas protegens (7) and, as a corollary of the description presented in this paragraph, it is important to point out the significant work of updating and deepening the field’s knowledge of EPN-associated microbiota that has been carried out by the Gaudriault lab (31).
Figure 4 Characteristic coloring of larvae (Galleria mellonella) due to the action of symbiotic bacteria: Photorhabdus gives the larva a red or greenish color, Xenorhabdus a grayish or yellow-cream color.
The strategies used by IJs to approach their victims vary according to the species of nematode. There are two types: the ambush strategy and the cruiser strategy (32). Species that use the ambush strategy include Steinernema carpocapsae and S. scapterisci, which wait for the victim with an upright body and reach it with a jump up to 5 mm long; their action is mainly directed against arthropods that are present and active on the soil surface. Heterorhabditis spp., Steinernema glaseri, and S. apuliae are more mobile and actively search for prey in the soil (the cruiser strategy), while S. riobrave, S. feltiae, and S. ichnusae (33) use an intermediate strategy. The success of the attack is also influenced by the soil type, which may hinder or favor the movement of the nematodes in search of a victim (34, 35). One of the most important systems of insect defense against pests is encapsulation (36). However, this is ineffective against microorganisms and parasites that have developed strategies to bypass or inactivate the immune system of the host. In other cases, the behavior of the potential victim is more direct and tends to avoid penetration by the IJs. This behavior is observed in the larvae of Popillia japonica Newman (Coleoptera, Scarabaeidae), which dispose of the IJs of H. bacteriophora by wiping their body with their legs and rubbing the posterior part of the abdomen to avoid penetration via the anal opening (37).
The most practical method for obtaining IJs from soil is to collect representative soil samples rather than taking a single large sample or using a soil probe (38, 39). This is because taking a single sample reduces the probability of obtaining EPNs. In the laboratory, samples weighing approximately 1 kg are then placed in plastic bags, moistened with water as needed, and placed in a wire mesh cage containing between two and five larvae of the lepidopteran Galleria mellonella L. (Lepidoptera, Pyralidae) to attract nematodes. Other researchers prefer to use wire mesh tea filters instead of cages to prevent Galleria larvae from dispersing in the soil and being attacked by predators (Figure 5). These filters are equipped with a long stem and can also be inserted directly into the soil in the open field. For in vivo nematode production, last-stage larvae of G. mellonella are infested with suspensions of IJs in a Petri dish containing two moist filter papers at the bottom, or alternatively, a 2- to 3-mm-thick layer of well-washed and sterilized sea sand. After 3 to 5 days, the dead larvae are transferred to “White traps” (Figure 6) to promote the multiplication of EPNs, and the IJs are subsequently collected (40). These are then washed several times in sterile water and stored in the refrigerator. Pieces of sponge soaked in suspensions of IJs in water are used for this purpose, consisting of 500–1,000 IJs per cm2 of sponge in a tightly sealed plastic bag to avoid dehydration. At 5°C–10°C, the life span of the infective stages is between 1–3 months and several years, depending on the species. This type of preservation requires little skill and little energy, and the nematodes are of good quality. The disadvantage is the high cost (approximately €1 per million nematodes) and the lack of scale efficiency. In addition to refrigeration, entomopathogenic nematodes can be stored indefinitely in liquid nitrogen (41). Experiments on cryopreservation have been and are currently being conducted in Italy, with interesting results (42, 43). Bioreactors are used for industrial production (44), but currently not in Italy, where there are no production centers at present, although the country has greatly contributed to the development of technologies for the mass production of EPNs in recent decades (e.g., the Ecogen Europe biofactory, operative in Pantalla di Todi in the 1980s). In EPNs, as in all other nematodes, morphological and morphometric characters are very important for species identification. However, the high uniformity of the blueprint of EPNs, combined with the high intraspecific variability, makes identification based on morphology alone very difficult. The molecular approach involving the sequencing of specific loci, which has recently been increasingly used in the identification of animal and plant organisms in conjunction with morphological studies, has now made species identification of EPNs easier and more reliable. Under the morphological examination approach, in both families (Steinernematidae and Heterorhabditidae), the form primarily considered for species identification is the infectious juvenile third stage, i.e., the IJ.
Genus Steinernema Travassos, 1927 (Steinernematidae, Panagrolaimomorpha, and Strongyloidoidea) (Figure 7): first and second generation of amphimictic adults. Oviparous or ovoviviparous. The genus Neosteinernema Nguyen et Smart, 1994 (found only in America), also belongs to the same family. The genus Steinernema currently includes 108 species. Of the Steinernema species described, 15 have been found or reported in Europe and/or non-European Mediterranean regions.
Genus Heterorhabditis Poinar, 1976 (Heterorhabditidae, Rhabditomorpha, and Strongyloidea) (Figure 8): the first generation of adults is hermaphroditic, the second generation of adults is amphimictic. Oviparous or ovoviviparous. Fourteen species are currently classified in the genus Heterorhabditis, four of which have been found or reported in Europe (for details on the systematics of EPNs, see (45).
The first data on the isolation of a strain of EPNs in Italy date back to the early post-war period and concern the discovery of a small number of specimens of the beet weevil Temnorhinus mendicus Gyll. (Coleoptera, Curculionidae) infested with nematodes, which were described as Neoaplectana menozii Travassos, now a species inquirenda (37). However, the first samplings aiming to assess the spread of entomopathogenic nemotodes in the soil, which were carried out in the Emilia-Romagna region, date back only to 1983 (46). Subsequent research, involving almost all Italian regions, has provided and continues to provide very interesting data, including the discovery of new species. To date, more than 8,000 soil samples have been taken from different localities and biotopes. Among all sampled areas, 50% are agricultural habitats (olive groves, vineyards, orchards of various types, and vegetable and cereal fields), 35% are wooded environments (pine forests, oak forests, and chestnut forests), 10% are coastal areas, and the remaining 5% are pastures, fallow land, salt marshes, and lakeshores. To date, a total of more than 150 EPN strains have been isolated in Italy: 48 isolates of Heterorhabditis bacteriophora, 1 of H. downesi, 58 of Steinernema feltiae, 11 of S. affine, 4 of S. kraussei, 8 of S. apuliae, 4 of S. ichnusae, 12 of S. carpocapsae, 1 of S. vulcanicum, 4 of S. arenarium, and 1 of Oscheius onirici (the genus Oscheius includes several species of nematodes, of which only a few share similarities in parasitic action toward insects with EPNs). Some recently isolated strains from Lombardy have also been identified (47, 48). Steinernema kraussei has been isolated only in the soils of the chestnut groves of Etna, Sicily (47); S. apuliae, S. ichnusae, S. vulcanicum, and O. onirici are four new species presently recorded only in Italy, with the first isolated on the Apulian coast, the second in Sardinia and Campania, the third on the slopes of Etna in Sicily, and the fourth in Tuscany (49). A survey of the presence and characterization of entomopathogenic nematodes in Italy showed that EPNs were found in all habitats studied, indicating a wide distribution of species in different ecosystems. Steinernematidae occurred more frequently than Heterorhabditidae, with S. feltiae and H. bacteriophora being the most widespread species. Steinernema feltiae was isolated in most habitats, with a preference for sandy soils. H. bacteriophora is also a fairly common species, also found in volcanic soils (on the volcanic island of Pantelleria) but never in deciduous forests, showing a preference for sandy soils (58% of strains). Aside from the two dominant species, S. feltiae and H. bacteriophora, EPNs tended to correlate with a specific habitat: S. kraussei and S. affine, for example, were found in forests at fairly high altitudes; S. affine was isolated from different soil types, but almost exclusively in deciduous forests; and S. kraussei was isolated in chestnut forests with sandy soils on Mount Etna in Sicily (46). Concerning habitat preferences for other species, S. apuliae was isolated from different habitats, but always near coastal areas, showing a clear preference for sandy soils, while S. carpocapsae was isolated in the northern part of Apulia and in Tuscany, Emilia-Romagna, Lombardy, and Veneto, mostly in fallow soils (47). Sampling in Sicily revealed the first Italian strains of H. megidis and H. downesi, indicating that all Heterorhabditidae species reported in Europe are present in Sicily. Soil characteristics also influence the presence of EPNs, and our survey showed a clear correlation between EPN presence and soil texture, with EPNs exhibiting a preference for sandy and medium-textured soils. No strains were isolated from clay soils. This is most likely because sandy and medium-textured soils promote EPN mobility and survival, whereas clay-rich soils restrict nematode movement. The importance of two natural habitats, pine forests and oak forests, is suggested by this study of EPN distribution among habitats. These two forest environments have the highest concentrations of isolated EPN strains, with only three of the seven species found in the forests also being found in other habitats. The data on the biodiversity of EPNs collected thus far do not exhaustively cover all geographic areas and habitats in Italy, but they still contribute significantly to our understanding of the presence and geographic distribution of EPNs in relation to the wide variety of habitats found in Italy (50).
Molecular approaches show particular accuracy where morphological characterizations at the species level are not able to discriminate between closely related species. Molecular techniques based on PCR allow the analysis of informative DNA markers and genes from individual nematodes. A molecular marker is any DNA sequence that is widespread throughout the genome showing polymorphism (51) that can be detected using molecular techniques. Markers must also be stable, enabling their identification in each developmental stage of nematodes (52). The target regions used for EPN identification are nuclear, ribosomal, and mitochondrial DNA. The cistron of ribosomal DNA contains three highly conserved genes, 18S rRNA, 5.8S, and 28S, and highly variable regions such as the internal transcribed spacer (ITS; ITS1 and ITS2), IGS (intergenic spacer), and ETS (external transcribed spacer) regions. The ITS and expansion domains of the 28S gene are the most variable regions of rDNA and, along with partial mitochondrial genes, such as NADH dehydrogenase subunit 4 (nd4) or cytochrome oxidase (COI), and protein-coding genes, such as cmd-1, unc-87, hsp70 (53, 54), and hsp90 (55), are very useful markers for species differentiation. Furthermore, they allow the detection of misidentifications in order to establish phylogenetic relationships within and among EPNs (56–64). Recent studies by Spiridonov and Subbotin (65) and by Dhakal et al. (64) have confirmed that the ITS, the 28S rRNA gene, the COI, nuclear cmd-1, and unc-87 sequences are useful markers for EPN identification, confirming the occurrence of three groups within the Heterorhabditis genus, namely, “Indica”, “Bacteriophora”, and “Megidis”. Sequence analyses have also enabled the detection of misidentifications in GenBank and correction of their taxonomic status within the Heterorhabditis species. Furthermore, pairwise analyses permit the estimation of intraspecific and interspecific sequence variability within most of the Heterorhabditis and Steinernema species and strains (64). For Italian EPNs (Heterorhabditis, Steinernema, and Oscheius strains), the markers mainly used are ITS regions, the most conserved region of the D2D3 domain (LSU) for ribosomal DNA, the cytochrome oxidase I (COI) locus, and a partial portion of hsp90 (55, 66). Fanelli et al. (55) used degenerate primers to obtain partial hsp90 gene sequences from several entomopathogenic nematodes, including H. bacteriophora and O. myriophilus, and demonstrated that phylogenetic trees based on hsp90 sequences showed equal resolution, and in most cases were congruent with those inferred from ribosomal markers.
Comparison of the ITS sequences among Steinernema species has also showed higher variability than either the D2-D3 expansion domains of the 28SrRNA gene or the partial 18S rRNA gene (61). Analysis of partial mitochondrial COI sequences among Steinernema species has revealed that fewer clades could be resolved by this method than with ITS (61), despite the plurality of informative sites available for COI sequences.
Massive sequencing techniques have produced a large number of ITS and COI sequences for EPNs, leading to the development of the “DNA barcoding” protocol for the rapid identification of nematodes. This is a molecular method dedicated to the identification of biological identity that involves testing the variability of a relevant marker (67, 68). This approach exploits conserved regions of these genes, comparing all sequences present in the database in order to design universal primers that amplify short DNA fragments to be used as a barcode, and identifying even the cryptic nematode species (which have the same morphology but which are genetically different) and larval stages present in a soil sample, even in the absence of morphological identification (2, 67). Molecular barcodes can group an unknown EPN specimen phylogenetically into its correct position compared to known reference sequences (Figure 9).
Figure 9 Phylogenetic relationships of COI among different Italian strains of entomopathogenic nematodes, based on sequencing of several clones of each strain. (Colored boxes indicate the Italian strains).
Sequence differences in PCR products from different EPN spp. or strains can be analyzed by digestion with restriction enzymes. Six restriction enzymes are sufficient to differentiate almost all nematode species. Restriction fragment length polymorphism (RFLP) profiles of the ITS region are accurate and reproducible, allowing characterization of Steinernema and Heterorhabditis species (43, 69). ITS-RFLP analyses can also reveal the presence of different strains within EPN species, thus indicating genetic variability due to geographical origin or insect host (70, 71).
The random amplification of polymorphic DNA (RAPD) technique enables measurement of genetic diversity in EPNs, even if no prior sequence information is available. This technique involves the amplification of gDNA fragments using a single primer of 10 nucleotides with an arbitrary (random) sequence that can bind to complementary sequences present in the DNA. The profiles of the fragments generated are species-specific and can be compared with those of other EPN species (72). The presence or absence of a fragment in a sample represents a diagnostic marker, allowing researchers to discriminate among and within Steinernema spp. and Heterorhabditis spp (73–75).
Several tools, such as amplified fragment length polymorphism (AFLP), simple sequence repeats (SSR), inter-simple sequence repeats (ISSR), single-strand conformation polymorphism (SSCP), and sequence-related amplified polymorphism (SRAP), are also used to characterize genetic diversity in EPN species (76–79). SRAP has been successfully used to evaluate genetic diversity in EPN species, and a study by Youssef et al. (80) revealed that 12 different SRAP primer pairs can be used to differentiate among seven Steinernema species, with this method being more accurate than RAPD.
The real-time PCR (qPCR) technique enables monitoring of the amplification in real time and quantification of the number of copies of a specific target region. Copy number quantification of the target region is carried out during the exponential phase of PCR, producing much more accurate results than the traditional PCR “end point”. Quantification of the product is achieved by adding fluorescent compounds that are incorporated into each copy of the amplified product in each cycle, and fluorescence is proportional to the quantity of the amplified product. At present, several real-time qPCR probes are available to characterize EPN assemblages in the field (81–84). Species-specific primers and probes have been designed based on the ITS rDNA sequences of several Steinernema and Heterorhabditis species, and are available to the scientific community (82, 84, 85).
Real-time PCR (qPCR) enables not only discrimination among different species but also exploration of the relationships and the functions of different organisms within ecosystems (83). Thus, qPCR and species-specific primer–probe combinations allow the simultaneous detection, identification, and quantification of EPNs, as well as their antagonists and competitors, in soil samples (83, 84).
Sequencing technologies, such as high-throughput sequencing (HTS) and next-generation sequencing (NGS), are evolving rapidly, allowing accurate EPN identification and analysis of soil nematode communities (86). Furthermore, metagenomic analysis can explore EPN biogeography and the factors that modulate the presence and abundance of these organisms in soil samples (87).
Recently, loop-mediated isothermal amplification (LAMP) assay has been employed for rapid detection of Heterorhabditis spp. and Steinernema spp. from total soil DNA (88).
LAMP was developed in 2000 (87, 88) and has been used for the identification of animal and plant pathogens, including plant parasitic nematodes. It combines the simplicity and rapidity of reaction setup with ready data interpretation. The advantage of the LAMP method is that DNA amplification reactions take place at a constant temperature. LAMP is based on the isothermal amplification of nucleic acids by thermostable polymerase, with “strand displacement” activity isolated from Bacillus stearothermophilus (BSt polymerase) and on six specific primers which recognize eight regions on the selected target DNA (F3c, F2c, and F1c in the 3′ direction and B1, B2, and B3 in the 5′ direction). LAMP is inexpensive, fast, and reproducible, and can be conducted even in poorly equipped laboratories (89–91).
LAMP primers for Heterorhabditis and Steinernema have been designed by aligning sequences of the ITS region of rDNA for Heterorhabditis spp. and the 18S rDNA sequences for Steinernema spp. that were retrieved from GenBank. Five separate sets of LAMP primers are available for each EPN genus (88). The LAMP technique reduces the time and labor costs associated with the insect-baiting technique and provides information that can be used in the development of detection kits for the diagnosis of EPNs in the field without the need for trained and experienced personnel.
Commercial products containing Heterorhabditis bacteriophora, H. megidis, Steinernema feltiae, and S. carpocapsae have been successfully used to control various species of Heteroptera, Lepidoptera, Coleoptera, Diptera, and Hymenoptera (50). In Italy, EPN formulations have been effectively tested and used to control various species, including but not limited to the following: the scolytid Tomicus piniperda (92) and the lepidopteran Thaumetopoea pityocampa on pines (93) (Figure 10); the lepidopterans Pammene fasciana, Cydia splendana, and C. fagiglandana and the coleopterans Curculio elephas and C. glandium on chestnut trees (94, 95); the lepidopteran Cydia pomonella and the hymenoptera Hoplocampa brevis on pear trees (95, 96); the curculionid Rhytidoderes plicatus on cabbage roots (97); the chrysomelid Xanthogaleruca luteola on elm trees (98) (Figure 11); the tingid Corythucha ciliata on sycamores (99) (Figure 12); and the curculionid Otiorhynchus sulcatus on ornamental plants (100) and on strawberries (101, 102). In the Mediterranean region, because of the spread of exotic palm weevils, promising experiments to contain the spread of the weevil Rynchophorus ferrugineus with entomopathogenic EPN nematodes have been carried out in several countries, such as Egypt, Spain, and Italy (Figure 13). Other applications of beneficial nematodes have been effective in controlling sciarid dipteran in ornamental nurseries and mushroom farms, the weevil curculionid Curculio nucum on hazels (103), the thrips Frankliniella occidentalis (104), the lepidopteran Tuta absoluta on tomato, the chrysomelid Diabrotica virgifera on corn (105), and slugs and snails in horticulture, with the latter case involving the use of the specific nematode Phasmarhabditis hermaphrodita (49). Entomopathogenic nematodes have also been found to be effective against xylophagous insects residing in cryptic habitats, such as Capnodis tenebrionis, Arhopalus syriacus, Cossus cossus, and Parahypopta caestrum (106). Recently, these biological control agents have found broad application in integrated control programs of the Scarab beetle Popillia japonica in large cultivated and non-cultivated areas of Lombardy and Piedmont (105, 106), and of the cossid Parahypopta caestrum in the asparagus fields of Apulia (107).
Figure 13 Rhynchophorus ferrugineus adults infested by Heterorhabditis bacteriophora, Italian strain.
The larvae of Coleoptera Curculionidae of the genus Otiorhynchus represent the classic example of a target pest of EPNs, to the extent that this type of biological control is currently widely tested and used around the world. The most suitable nematodes against Otiorhynchus larvae belong to the genus Heterorhabditis, although satisfactory results have been achieved with formulations based on S. feltiae and, to a lesser extent, on S. carpocapsae (108, 109). Formulas based on H. bacteriophora are marketed in Italy in packages containing 50 to 250 million IJs. The doses used correspond to 200,000 to 400,000 nematodes per m2, or 25,000 to 40,000 nematodes per plant (50). The end of summer is the best time for application, so as to target the early larval stages of the insects, which are more sensitive and are at the beginning of their phytophagous action, under optimum soil temperatures of 18°C –22°C (110). Spring treatments on larger overwintering larvae and newly formed pupae are equally effective if soil temperatures are above 15°C and a dose of 500,000 nematodes per m2 is used (50). The crops targeted for this type of application are typically ornamental plants in nurseries and strawberry crops in open field and protected cultivations. Another example EPN application, whose use has expanded in recent years to cover a surface area of 800 ha in Emilia-Romagna and approximately 1,500 ha throughout northern Italy, is that of the autumn treatments used against overwintering larvae of Cydia pomonella on pear and apple (50). The nematodes used belong to the species S. feltiae and S. carpocapsae, which are applied to the trunks and lower branches of the trees, where the larvae overwinter in bark crevices, protected in a light cocoon that the nematodes can perforate (49). The application dose corresponds to 1.5 × 109 IJ diluted in 15 hL of water per ha, distributed by the company atomizer, for which it is necessary to close the highest nozzles and remove the filters (49). Thorough moistening of the plants and soil before and after the application of entomopathogenic nematodes and constant moistening of the substrate promote good efficacy of the biological treatment and ensure parasitization of the insect; if water availability is limited, it is advisable to inject the EPN suspension into the soil. Xylophagous insects, such as Lepidoptera Cossidae (Cossus L. and Zeuzera pyrina L.), Coleoptera Cerambycidae (Saperda carcharias L.), and Buprestidae (Capnodis tenebrionis L.) can also be effectively controlled with injections of IJ suspensions into the penetration holes (also with the addition of chitosan) or by the obstruction of these openings with pieces of sponge soaked in nematodes. EPNs are typically applied to culture systems and substrates that are regularly treated with other chemicals, including natural soil improvers and fertilizers. Heterorhabditis bacteriophora, S. carpocapsae, and S. feltiae are in most cases compatible with plant protection products, but can interact with such substances, in some cases even producing antagonistic or synergistic effects (111).
EPNs are ubiquitous organisms that are not dangerous to higher animals or humans and do not have any side effects for plants. Before being used, entomopathogenic organisms should, in general, be subject to registration screening, with the exception of ENPs, which are generally exempted due to their pluricellular structure and recognized specificity to insects. At the European level, Regulation (EC) No. 1107/2009 of the European Parliament and of the Council of 21 October 2009, which repealed Commission Directive 91/414 concerning the placing on the market of plant protection products, applies to active substances, including micro-organisms, safeners, synergists, co-formulants, and adjuvants, that have a general or specific action against harmful organisms. This regulation provides for the registration of three “categories” of formulations: biocides, microorganisms, and viruses. Nematodes and macro-organisms (insects and auxiliary mites) are not mentioned, pursuant to the principle of forgoing the registration process for products with low environmental impact, based on the unequivocal interpretation of the countries of the European Union. However, unlike those countries that currently do not require any registration (such as Denmark, Finland, France, Greece, Germany, Italy, Portugal, and Spain), others do require some form of registration: Austria requires the same form of registration as that used for chemicals; Belgium and the Netherlands require registration only for new formulations; Poland, the Czech Republic, and Hungary provide for a preliminary field trial; Ireland, Switzerland, Norway, and Sweden require the registration of all biological control agents; and in the UK, no registration is required for indigenous ENPs, but the introduction of non-native strains in the wild in the country is controlled by the Wildlife and Countryside Act (112). In Japan, registration is required for ENPs as well as for chemicals, whereas in other non-European countries (such as Australia, Canada, and the United States), no registration is needed, provided that the ENPs are indigenous. In New Zealand, in contrast, native species must also be registered and are protected. All these states have also enacted specific legislation to regulate the import and release of non-native species. The REBECA Action (Regulation of Biological Control Agents, 113), funded by the European Union for the correct use and marketing of EPNs, has made several recommendations on the use of entomopathogenic nematodes, such as knowledge of the exact identity (specific identification) of the ENPs and accurate identification of the symbiotic bacterium of Heterorhabditis indica, to exclude the presence of Photorhabdus asymbiotica, which is harmful to humans. At the same time, it has also been pointed out that no precautions are necessary if one uses native EPNs and is provided with comprehensive information on the “environmental risk assessment” (ERA) criteria used for insects and auxiliary mites. Thus, molecular biology and phylogenetic reconstruction play an important role in elucidating the systematics, cospeciation, and coadaptation of entomopathogenic nematodes and their symbiotic bacteria when new EPNs are used as biological control agents (114–116).
All authors listed have made a substantial, direct, and intellectual contribution to the work and approved it for publication.
Puglia Regional Fundings “Aspara” and “Psr-Leg” projects; Italian National funding "Covexy" project.
The authors declare that the research was conducted in the absence of any commercial or financial relationships that could be construed as a potential conflict of interest.
The author ET declared that they were an editorial board member of Frontiers at the time of submission. This had no impact on the peer review process and the final decision.
All claims expressed in this article are solely those of the authors and do not necessarily represent those of their affiliated organizations, or those of the publisher, the editors and the reviewers. Any product that may be evaluated in this article, or claim that may be made by its manufacturer, is not guaranteed or endorsed by the publisher.
The Supplementary Material for this article can be found online at: https://www.frontiersin.org/articles/10.3389/finsc.2023.1195254/full#supplementary-material
1. Blaxter M, Koutsovoulos G. The evolution of parasitism in Nematoda. Parasitology (2015) 142:S26–39. doi: 10.1017/S0031182014000791
2. Blaxter ML, De Ley P, Garey JR, Liu LX, Scheldeman P, Vierstraete A, et al. A molecular evolutionary framework for the phylum Nematoda. Nature (1998) 392:71–5. doi: 10.1038/32160
3. Schmid-Hempel P. Parasite immune evasion: a momentous molecular war. Trends Ecol Evol (2008) 23:318–26. doi: 10.1016/j.tree.2008.02.011
4. Georgis R. Present and future prospects for entomopathogenic nematode products. Biocontrol Sci Techn (1992) 2:83–99. doi: 10.1080/09583159209355222
5. Kaya HK, Gaugler R. Entomopathogenic nematodes. Ann Rev Entomol (1993) 38:181–206. doi: 10.1146/annurev.en.38.010193.001145
6. White B. Biological control of insect pests. Sci e-Resources (2019). ED-Tech Press UK298 p. 5:219–81.
7. Ruiu L, Marche MG, Mura ME, Tarasco E. Involvement of a novel Pseudomonas protegens strain associated with entomopathogenic nematode infective juveniles in insect pathogenesis. Pest Manag Sci (2022) 78:5437–43. doi: 10.1002/ps.7166
8. Johnigk SA, Ehlers RU. Endotokia matricida in hermaphrodites of Heterorhabditis spp. and the effect of the food supply. Nematology (1999) 1:717–26. doi: 10.1163/156854199508748
9. Dunphy GB, Webster JM. Partially characterized components of the epicuticle of dauer juvenile Steinernema feltiae and their influence on hemocyte activity in Galleria mellonella. J Parasitol (1987) 73:584–8. doi: 10.2307/3282140
10. Ishibashi N, Kondo E. Behavior of infective juveniles. In: Entomopathogenic nematodes in biological control. Boca Raton, FL: CRC Press (1990). p. 139–50.
11. Bedding RA, Molyneux AS. Penetration of insect cuticle by infective juveniles of Heterorhabditis spp. (Heterorhabditidae: Nematoda). Nematologica (1982) 28:354–9. doi: 10.1163/187529282X00402
12. Peters A, Ehlers RU. Susceptibility of leatherjackets (Tipula paludosa and Tipula oleracea; Tipulidae; Nematocera) to the entomopathogenic nematode Steinernema feltiae. J Invertebr Pathol (1994) 63:163–71. doi: 10.1006/jipa.1994.1031
13. Ji D, Yi Y, Kang GH, Choi YH, Kim P, Baek NI, et al. Identification of an antibacterial compound, benzylideneacetone, from Xenorhabdus nematophila against major plant-pathogenic bacteria. FEMS Microbiol Lett (2004) 239:241–8. doi: 10.1016/j.femsle.2004.08.041
14. Han R, Ehlers RU. Pathogenicity, development, and reproduction of Heterorhabditis bacteriophora and Steinernema carpocapsae under axenic in vivo conditions. J Invertebr Pathol (2000) 75:55–8. doi: 10.1006/jipa.1999.4900
15. Burman M. Neoaplectana carpocapsae Ðtoxin production by axenic insect parasitic nematodes. Nematologica (1982) 28(1):62–70. doi: 10.1163/187529282X00510
16. Toubarro D, Avila MM, Hao YJ, Balasubramanian N, Jing YJ, Montiel R, et al. A serpin released by an entomopathogen impairs clot formation in insect defense system. PloS One (2013) 8(7):e69161. doi: 10.1371/journal.pone.0069161
17. Toubarro D, Lucena-Robles M, Nascimento G, Costa G, Montiel R, Coelho AV, et al. An apoptosis inducing serine protease secreted by the entomopathogenic nematode Steinernema carpocapsae. Int J Parasit (2009) 39(12):1319–30. doi: 10.1016/j.ijpara.2009.04.013
18. Lu D, Macchietto M, Chang D, Barros MM, Baldwin J, Mortazavi A, et al. Activated entomopathogenic nematode infective juveniles release lethal venom proteins. PloS Pathog (2017) 13:e1006302. doi: 10.1371/journal.ppat.1006302
19. Chang DZ, Serra L, Lu D, Mortazavi A, Dillman AR. A core set of venom proteins is released by entomopathogenic nematodes in the genus Steinernema. PloS Pathog (2019) 15(5):e1007626. doi: 10.1371/journal.ppat.1007626
20. Wang J, Bedding RA. Population development of Heterorhabditis bacteriophora and Steinernema carpocapsae in the larvae of Galleria mellonella. Fund App Nematol (1996) 19:363–8.
21. Boemare N, Akhurst R. Biochemical and physiological characterization of colony form variants in Xenorhabdus spp. (Enterobacteriaceae). J Gen Microbiol (1988) 134:751–61. doi: 10.1099/00221287-134-3-751
22. Thomas GM, Poinar GO. Xenorhabdus gen. nov., a genus of entomopathogenic, nematophilic bacteria of the family Enterobacteriaceae. Int J Syst Bacteriol (1979) 29:352–60. doi: 10.1099/00207713-29-4-352
23. Forst S, Dowds B, Boemare N, Stackebrandt E. Xenorhabdus and Photorhabdus spp.: bugs that kill bugs. Ann Rev Micr (1997) 51:47–72. doi: 10.1146/annurev.micro.51.1.47
24. Fodor A, Gualtieri M, Zeller M, Tarasco E, Klein MG, Fodor, et al. Type strains of entomopathogenic nematode-symbiotic bacterium species, Xenorhabdus szentirmaii (EMC) and X. budapestensis (EMA), are exceptional sources of non-ribosomal templated, large-target-spectral, thermotolerant-antimicrobial peptides (by both), and iodinin (by EMC). Pathogens (2022) 11:342. doi: 10.3390/pathogens11030342
25. Fodor A, Vellai T, Hess C, Makrai L, Dublecz K, Pál L, et al. XENOFOOD - An Autoclaved Feed Supplement Containing Autoclavable Antimicrobial Peptides—Exerts Anticoccidial GI Activity, and Causes Bursa Enlargement, but Has No Detectable Harmful Effects in Broiler Cockerels despite in vitro Detectable Cytotoxicity on LHM Cells. Pathogens (2023) 12:458. doi: 10.3390/pathogens12030458
26. Martens Eric C, Heugens K, Goodrich-Blair H. Early Colonization Events in the Mutualistic Association between Steinernema carpocapsae Nematodes and Xenorhabdus nematophila Bacteria. J Bacteriol (2003) 185:3147–3154. doi: 10.1128/JB.185.10.3147-3154.2003
27. Ciche TA, Kim KS, Kaufmann-Daszczuk B, Nguyen KC, Hall DH. Cell invasion and matricide during Photorhabdus luminescens transmission by Heterorhabditis bacteriophora nematodes. Appl Env Micr (2008) 74:2275–87. doi: 10.1128/AEM.02646-07
28. Tailliez P, Pages S, Ginibre N, Boemare N. New insight into diversity in the genus Xenorhabdus, including the description of ten novel species. Int J Syst Evol Microbiol (2006) 56:2805–18. doi: 10.1099/ijs.0.64287-0
29. Rappazzo G, Salvo E, Tarasco E, Petronio Petronio G, Buccheri MA, Furneri PM, et al. Endo symbionts of Entomopathogenic Nematodes from South Italy: a phenotypic study. Redia (2018) 101:183–8. doi: 10.19263/REDIA-101.18.24
30. Fischer-Le Saux M, Viallard V, Brunel B, Normand P, Boemare NE. Polyphasic classification of the genus Photorhabdus and proposal of new taxa: P. luminescens subsp. luminescens subsp. nov., P. luminescens subsp. akhurstii subsp. nov., P. luminescens subsp. laumondii subsp. nov., P. temperata sp. nov., P. temperata subsp. temperata subsp. nov. and P. asymbiotica sp. nov. Int J Syst Evol Micr (1999) 49:1645–56. doi: 10.1099/00207713-49-4-1645
31. Ogier JC, Pagès S, Frayssinet M, Gaudriault S. Entomopathogenic nematode-associated microbiota: from monoxenic paradigm to Pathobiome. Microbiome (2020) 8:25. doi: 10.1186/s40168-020-00800-5
32. Lortkipanidze MA, Gorgadze OA, Kajaia GS, Gratiashvili NG, Kuchava MA. Foraging behavior and virulence of some entomopathogenic nematodes. Ann Agrarian Sci (2016) 14:99–103. doi: 10.1016/j.aasci.2016.05.009
33. Tarasco E, Mrácek Z, Nguyen KB, Triggiani O. Steinernema ichnusae sp. n. (Nematoda: Steinernematidae) a new entomopathogenic nematode from Sardinia Island (Italy). J Invertebr Pathol (2008) 99:173–85. doi: 10.1016/j.jip.2008.05.001
34. Kruitbos LM, Heritage S, Hapca S, Wilson MJ. The influence of habitat quality on the foraging strategies of the entomopathogenic nematodes Steinernema carpocapsae and Heterorhabditis megidis. Parasitol (2010) 137:303–9. doi: 10.1017/S0031182009991326
35. Wilson MJ, Ehlers RU, Glazer I. Entomopathogenic nematode foraging strategies–is Steinernema carpocapsae really an ambush forager. Nematology (2012) 14:389–94. doi: 10.1163/156854111X617428
36. Götz P, Boman HG. Insect immunity. In: Kerkut GA, Gilbert LI, editors. Comprehensive Insect Physiology, Biochemistry, and Pharmacology, vol. 3 . Oxford, Pergamon Press (1985). p. 453–485.
37. Gaugler R, Wang YI, Campbell JF. Aggressive and evasive behaviors in Popillia japonica (Coleoptera: Scarabaeidae) larvae: defenses against entomopathogenic nematode attack. J Inver Path (1994) 64:193–9. doi: 10.1016/S0022-2011(94)90150-3
38. Tarasco E, Kary NE, Fanelli E, Mohammadi D, Xingyue L, Ali Mehrvar A, et al. Modified bait insect technique in entomopathogens’ survey from the Arasbaran Biosphere Reserve (Iran). Redia (2020) 103:129–32. doi: 10.19263/REDIA-103.20.20
39. Tarasco E, Kary NE, Fanelli E, Mohammadi D, Mehrvar A, De Luca F, et al. Survey on entomopathogens from the arasbaran biosphere reserve (Iran) with a Modified bait insect technique. Geol Earth Mar Sci (2020) 2:1–3.
40. Orozco RA, Lee MM, Stock SP. Soil sampling and isolation of entomopathogenic nematodes (Steinernematidae, Heterorhabditidae). J Vis Exp (2014) 89:e52083. doi: 10.3791/52083
41. Lacey LA. Manual of techniques in insect pathology. Academic Press (1997). doi: 10.1016/B978-0-12-432555-5.X5000-3
42. Cosi E, Triggiani O, Irdani T, Tarasco E, Roversi PF. First results of entomopathogenic nematodes cryopreservation in liquid nitrogen and storage at– 140° C. Redia (2008) 91:181–3.
43. Torrini G, Landi S, Benvenuti C, De Luca F, Fanelli E, Troccoli A, et al. Morphological and molecular characterization of a Steinernema carpocapsae (Nematoda Steinernematidae) strain isolated in Veneto region (Italy). Redia (2014) 97:89–94.
44. Cortés-Martínez CI, Chavarría-Hernández N. Production of entomopathogenic nematodes in submerged monoxenic culture: a review. Biotechnol Bioeng (2020) 117:3968–85. doi: 10.1002/bit.27515
45. Nguyen K, Hunt D. Entomopathogenic nematodes: systematics, phylogeny and bacterial symbionts. Leiden: Brill (2007).
46. Tarasco E, Clausi M, Rappazzo G, Vinciguerra M, Longo A, Triggiani O. Could Italy be considered a favorite place in Europe for EPN biodiversity? IOBC-WPRS Bullet (2009) 45:387–9.
47. Tarasco E, Clausi M, Rappazzo G, Panzavolta T, Curto G, Sorino R, et al. Biodiversity of entomopathogenic nematodes in Italy. J Helminthol (2015) 89:359–66. doi: 10.1017/S0022149X14000194
48. Glazer I, Santoiemma G, Battisti A, De Luca F, Fanelli E, Troccoli A, et al. Invasion of Popillia japonica in Lombardy, Italy: Interactions with soil entomopathogenic nematodes and native grubs. Agr For Entomol (2022) 24:600–8. doi: 10.1111/afe.12524
49. Tarasco E, Ragni A, Curto G. Status of entomopathogenic nematodes in integrated pest management strategies in Italy. In: Abd-Elgawad MMM, Askary TH, Coupland J, editors. Biocontrol Agents: Entomopathogenic and Slug Parasitic Nematodes. CABI International (2017). p. 429–44. doi: 10.1079/9781786390004.0429
50. De Luca F, Clausi M, Troccoli A, Curto G, Rappazzo G, Tarasco E. Entomopathogenic Nematodes in Italy: Occurrence and Use in Microbial Control Strategies. In: Campos Herrera R, editor. Nematode Pathogenesis of Insects and Other Pests. Switzerland: Springer International Publishing (2015). p. 431–49.
51. Li XY, Li J, Zhao ZJ, Yang F, Fu QW, Liu HS, et al. Sequence-related amplified polymorphism (SRAP) for studying genetic diversity and population structure of plants and other living organisms. J Anim Plant Sci (2014) 24:1478–86.
52. Agarwal M, Shrivastava N, Padh H. Advances in molecular marker techniques and their applications in plant sciences. Plant Cell Rep (2008) 27:617–31. doi: 10.1007/s00299-008-0507-z
53. Liu J, Berry RE, Blouin MS. Molecular differentiation and phylogeny of entomopathogenic nematodes (Rhabditida: Heterorhabditidae) based on ND4 gene sequences of mitochondrial DNA. J Parasitol (1999) 85:709–15. doi: 10.2307/3285747
54. Saeb AT. Heat shock protein Hsp70 multigene family as a new genetic target for the differentiation and identification of entomopathogenic nematodes (Rhabditida: Heterorhabditidae). Ad Life Sci Health (2015) 2:16–30.
55. Fanelli E, Troccoli A, Tarasco E, De Luca F. Molecular characterization and functional analysis of the Hb-Hsp 90-1 gene in relation to temperature changes in Heterorhabditis bacteriophora. Front Physiol (2021) 12:615653. doi: 10.3389/fphys.2021.615653
56. Nguyen KB, Shapiro-Ilan DI, Mbata G. Heterorhabditis Georgiana n. sp. (Rhabditida: Heterorhabditidae) from Georgia, USA. Nematology (2008) 10:433–48. doi: 10.1163/156854108783900276
57. Lulamba TE, Serepa-Dlamini MH. Molecular identification of a Heterorhabditis entomopathogenic nematode isolated from the northernmost region of South Africa. Egypt J Biol Pest Contr (2020) 30:77. doi: 10.1186/s41938-020-00279-0
58. Malan AP, Knoetze R, Tiedt L. Heterorhabditis noenieputensis n. sp. (Rhabditida: Heterorhabditidae), a new entomopathogenic nematode from South Africa. J Helminthol (2014) 88:139–51. doi: 10.1017/s0022149x12000806
59. Phan KL, Subbotin SA, Nguyen NC, Moens M. Heterorhabditis baujardi sp. n. (Rhabditida: Heterorhabditidae) from Vietnam and morphometric data for H. indica populations. Nematology (2003) 5:367–82. doi: 10.1163/156854103769224368
60. Kuwata R, Yoshiga T, Yoshida M, Kondo E. Phylogenetic relationships of Japanese Heterorhabditis nematodes and their symbiotic Photorhabdus bacteria. Jap J Nematol (2007) 37:39–50. doi: 10.3725/jjn.37.39
61. Gorgadze O, Fanelli E, Lortkhipanidze M, Troccoli A, Burjanadze M, Tarasco E, et al. Steinernema borjomiense n. sp. (Rhabditida: Steinernematidae), a new entomopathogenic nematode from Georgia. Nematology (2018) 20:653–69. doi: 10.1163/15685411-00003167
62. Noujeim E, Sakr J, Fanelli E, Troccoli A, Pages S, Tarasco E, et al. Phylogenetic relationships of entomopathogenic nematodes and their bacterial symbionts from coastal areas in Lebanon. Redia (2016) 99:127–37. doi: 10.19263/REDIA-99.16.16
63. Bhat AH, Chaubey AK, Shokoohi E, Mashela PW. Study of steinernema hermaphroditum (Nematoda, rhabditida) from the west uttar pradesh, India. Acta Parasitol (2019) 64:720–37. doi: 10.2478/s11686-019-00061-9
64. Dhakal M, Nguyen KB, Hunt DJ, Ehlers R, Spiridonov SE, Subbotin SA. Molecular identification, phylogeny and phylogeography of the entomopathogenic nematodes of the genus Heterorhabditis Poinar, 1976: a multigene approach. Nematology (2020) 23:451–66. doi: 10.1163/15685411-bja10052
65. Spiridonov SE, Subbotin SA. Phylogeny and phylogeography of heterorhabditis and steinernema. Adv entomopathog nematopdes taxonomy phylogeny (2016) 12:413–27. Brill Eds. Nematology Monographs and Perspectives. doi: 10.1163/9789004285347_007
66. Torrini G, Mazza G, Carletti B, Benvenuti C, Roversi PF, Fanelli E, et al. Oscheius onirici sp. n. (Nematoda: Rhabditidae): a new entomopathogenic nematode from an Italian cave. Zootaxa (2015) 3937(3):533–48. doi: 10.11646/zootaxa.3937.3.6
67. Floyd R, Abebe E, Papert A, Blaxter M. Molecular barcodes for soil nematode identification. Mol Ecol (2002) 11:839–50. doi: 10.1046/j.1365-294x.2002.01485.x
68. Hebert PD, Ratnasingham S, De Waard JR. Barcoding animal life: cytochrome c oxidase subunit 1 divergences among closely related species. Proc R Soc Lon Ser B: Biol Sci (2003) 270:S96–9. doi: 10.1098/rsbl.2003.0025
69. Reid AP, Hominick WM. Cloning of the rDNA repeat unit from a British entomopathogenic nematode (Steinernematidae) and its potential for species identification. Parasitol (1993) 107:529–36. doi: 10.1017/S0031182000068104
70. Stock SP, Mracek Z, Webster M. Morphological variation between allopatric populations of Steinernema krausei (Steiner, 1923) (Rhabditida: Steinernematidae). Nematology (2000) 2:143–52. doi: 10.1163/156854100509033
71. Razia MK, Padmanaban R, Karthik Raja P, Chellapandi P, Sivaramakrishnan S. PCR-RFLP pattern analysis of entomopathogenic nematodes isolated from agro-ecosystem for implicating their genetic diversity. Munis Entomol Zool (2011) 6:404–11.
72. Marin DH, Kaplan DT Opperman CH. Randomly Amplified Polymorphic DNA differs with burrowing nematode collection site, but not with host range. J Nematol (1999) 31:232–9.
73. Shapiro DI, Glazer I, Segal D. Genetic diversity in wild and laboratory population of Heterorhabditis bacteriophora as determined by RAPD–PCR analysis. Fund App Nematol (1997) 20:581–5.
74. Ibrahim SAM. Genetic diversity and phylogenetic relationships of some entomopathogenic nematode species (Steinernematidae and Heterorhabditidae). Int J Nematol (2009) 19:144–50.
75. Padmanaban K, Karthik RR, Razia M, Chellapandi P, Sivaramakrishnan S. Genetic diversity of entomopathogenic nematodes in non-agricultural ecosystem revealed with PCR-RAPD markers. Int J Nematol (2014) 24:1–8.
76. Budak H, Shearman RC, Parmaksiz I, Dweikat I. Comparative analysis of seeded and vegetative biotype buffalo grasses based on phylogenetic relationship using ISSRs, SSRs, RAPDs, and SRAPs. Theor Appl Genet (2004) 109:280–8. doi: 10.1007/s00122-004-1630-z
77. Gasser RB, Hu M, Chilton NB, Campbell BE, Jex AJ, Otranto D, et al. Single-strand conformation polymorphism (SSCP) for the analysis of genetic variation. Nat Protoc (2006) 1:3121–8. doi: 10.1038/nprot.2006.485
78. Mutlu N, Boyaci FH, Göçmen MM, Abak K. Development of SRAP, SRAPRGA, RAPD and SCAR markers linked with a Fusarium wilt resistance gene in eggplant. Theor Appl Genet (2008) 117:1303–12. doi: 10.1007/s00122-008-0864-6
79. Abedian M, Talebi M, Golmohammdi HR, Sayed Tabatabaei BE. Genetic diversity and population structure of mahaleb cherry (Prunus mahaleb L.) and sweet cherry (Prunus avium L.) using SRAP markers. Biochem Syst Ecol (2012) 40:112–7. doi: 10.1016/j.bse.2011.10.005
80. Youssef SS, Ibrahim SA, Abdel Azim AM, Khashaba EH, El-Sharkawy AM. Genetic variation among five Egyptian clover cultivars using Random Amplified Polymorphic DNA (RAPD) and Sequence-Related Amplified Polymorphism (SRAP) Molecular Markers. Egypt Acad J Biol Sci s C Physiol Mol Biol (2019) 11:37–45. doi: 10.21608/eajbsc.2019.31758
81. Torr P, Spiridonov SE, Heritage S, Wilson MJ. Habitat associations of two entomopathogenic nematodes: a quantitative study using real-time quantitative polymerase chain reactions. J Anim Ecol (2007) 76:238–45. doi: 10.1111/j.1365-2656.2006.01196.x
82. Campos-Herrera R, El-Borai FE, Stuart RJ, Graham JH, Duncan LW. Entomopathogenic nematodes, phoretic Paenibacillus spp., and the use of real time quantitative PCR to explore soil food webs in Florida citrus groves. J Invertebr Pathol (2011) 108:30–9. doi: 10.1016/j.jip.2011.06.005
83. Campos-Herrera R, Ali JG, Díaz BM, Duncan LW. Analyzing spatial patterns linked to the ecology of herbivores and their natural enemies in the soil. Front Plant Sci (2013) 4:1–18. doi: 10.3389/fpls.2013.00378
84. Campos-Herrera R, Jaffuel G, Chiriboga X, Blanco-Perez R, Fesselet M, Púza V, et al. Traditional and molecular detection methods reveal intense interguild competition and other multitrophic interactions associated with native entomopathogenic nematodes in Swiss tillage soils. Plant Soil (2015) 389:237–55. doi: 10.1007/s11104-014-2358-4
85. Campos-Herrera R, El-Borai FE, Larry LW. Wide interguild relationships among entomopathogenic and free-living nematodes in soil as measured by real time qPCR. J Invertebr Pathol (2012) 111:126–35. doi: 10.1016/j.jip.2012.07.006
86. Treonis AM, Unangst SK, Kepler RM, Buyer JS, Cavigelli MA, Mirsky SB, et al. Characterization of soil nematode communities in three cropping systems through morphological and DNA metabarcoding approaches. Sci Rep (2018) 8:2004. doi: 10.1038/s41598-018-20366-5
87. Dritsoulas A, Campos-Herrera R, Blanco-Pérez R, Duncan LW. Comparing high throughput sequencing and real time qPCR for characterizing entomopathogenic nematode biogeography. Soil Biol Biochem (2020) 145:107793. doi: 10.1016/j.soilbio.2020.107793
88. Singh G, Ahuja A, Rao U, Singh Somvanshi V. Loop-mediated isothermal amplification based identification of entomopathogenic nematodes Heterorhabditis spp. and Steinernema spp. from soil DNA. BioControl (2021) 66:701–12. doi: 10.1007/s10526-021-10103-9
89. Tomita N, Mori Y, Kanda H, Notomi T. Loop-mediated isothermal amplification (LAMP) of gene sequences and simple visual detection of products. Nat Prot (2008) 3:877–82. doi: 10.1038/nprot.2008.57
90. Goto M, Honda E, Ogura A, Nomoto A. Hanaki KI Colorimetric detection of loop-mediated isothermal amplification reaction by using hydroxy naphthol blue. Biotechniques (2009) 46:167–72. doi: 10.2144/000113072
91. Mori Y, Notomi T. Loop-mediated isothermal amplification (LAMP): a rapid, accurate, and cost-effective diagnostic method for infectious diseases. J Infect Chemother (2009) 15:62–9. doi: 10.1007/s10156-009-0669-9
92. Triggiani O. Sensibilità del Tomicus (Blastophagus) piniperda L. (Coleoptera: Scolytidae) a nematodi della famiglia Steinernematidae e Heterorhabditidae. Entomologica (1983) 18:215–23.
93. Triggiani O, Tarasco E. Efficacy and persistence of entomopathogenic nematodes in controlling larval populations of Thaumetopoea pityocampa (Lepidoptera: Thaumetopoeidae). Biocontrol Sci Techn (2002) 12:747–52. doi: 10.1080/0958315021000039923
94. Vinciguerra MT, Clausi M. Biological control of chestnut insect pests by means of entomopathogenic nematodes. Adv Horticul Sci (2006) 20:40–4.
95. Curto G, Caruso S, Reggiani A, Vergnani S. Efficacia dei nematodi entomopatogeni nel contenimento delle larve di Cydia pomonella L. nell'Italia Settentrionale Frutticolt (2009) 3:45–9.
96. Reggiani A, Curto G, Vergnani S, Caruso S, Boselli M. Effectiveness of entomopathogenic nematodes in the control of Cydia pomonella overwintering larvae in Northern Italy. IOBC WPRS Bullet (2008) 31:287–93.
97. Tarasco E, Triggiani O. Biocontrol of Rhytidoderes plicatus Oliv.(Coleoptera, Curculionidae) in potted savoy cabbageswith entompathogenic nematodes. Entomologica (2002) 36:157–63.
98. Triggiani O, Tarasco E. Applying entomopathogenic nematode to Xanthogaleruca luteola (Coleoptera Chrysomelidae) infested foliage. Redia (2007) 90:29–31.
99. Tarasco E, Triggiani O. Evaluation and comparison of entomopathogenic nematodes and fungi to control Corythucha ciliata Say (Rhynchota Tingidae). Redia (2006) 89:51–4.
100. Georgis R, Poinar GO. Nematodes as bioinsecticides in turf and ornamentals. In: Handbook of integrated pest management for turf and ornamentals, London: CRC Press (2020). p. 477–87. doi: 10.1201/9780138752798
101. Willmott DM, Hart AJ, Long SJ, Edmondson RN, Richardson PN. Use of a cold-active entomopathogenic nematode Steinernema kraussei to control overwintering larvae of the black vine weevil Otiorhynchus sulcatus (Coleoptera: Curculionidae) in outdoor strawberry plants. Nematology (2002) 4:925–32. doi: 10.1163/156854102321122548
102. Shields EJ, Testa AM. Multi-year biological control of black vine weevil, Otiorhynchus sulcatus, with persistent entomopathogenic nematodes. Great Lakes Entomol (2020) 53:7.
103. Batalla-Carrera L, Morton A, García-del-Pino F. Field efficacy against the hazelnut weevil, Curculio nucum and short-term persistence of entomopathogenic nematodes. Spanish J Agr Res (2013) 11:1112–9. doi: 10.5424/sjar/2013114-4210
104. Ebssa L, Borgemeister C, Berndt O, Poehling HM. Impact of entomopathogenic nematodes on different soil-dwelling stages of western flower thrips, Frankliniella occidentalis (Thysanoptera: Thripidae), in the laboratory and under semi-field conditions. Biocontrol Sci Techn (2001) 11:515–25. doi: 10.1080/09583150120067544
105. Toepfer S, Knuth P, Glas M, Kuhlmann U. Successful application of entomopathogenic nematodes for the biological control of western corn rootworm larvae in Europe: a mini review. Julius-Kühn-Archiv (2014) 444:59.
106. El Khoury Y, Noujeim E, Ravlić J, Oreste M, Addante R, Nemer N, et al. The effect of entomopathogenic nematodes and fungi against four xylophagous pests. Biocontrol Sci Techn. (2020) 30:983–95. doi: 10.1080/09583157.2020.1781059
107. Tarasco E, Bubici G, Oreste M. Biological notes on Parahypopta caestrum and first microbiological control assays. Bull Insectol (2016) 69:259–62.
108. Backhaus GF. (1994). Biological control of Otiorhynchus sulcatus F. by use of entomopathogenic nematodes of the genus Heterorhabditis. Acta Hortic 364:131–42. doi: 10.17660/ActaHortic.1994.364.16
109. Long SJ, Richardson PN, Fenlon JS. Influence of temperature on the infectivity of entomopathogenic nematodes (Steinernema and Heterorhabditis spp.) to larvae and pupae of the vine weevil Otiorhynchus sulcatus (Coleoptera: Curculionidae). Nematology (2000) 2:309–17. doi: 10.1163/156854100509187
110. El Khoury Y, Oreste M, Noujeim E, Nemer N, Tarasco E. Effect of temperature on the pathogenicity of Mediterranean native entomopathogenic nematodes (Steinernematidae and Heterorhabditidae) from natural ecosystems. Redia (2018) 101:123–7. doi: 10.19263/REDIA-101.18.16
111. El-Ashry RM, El-Marzoky AM. Compatibility of entomopathogenic nematodes, Heterorhabditis bacteriophora Poimar and Steinernema carpocapsae Weiser with some chemical and biopesticides. Zagazig J Agric Res (2018) 45:905–16. doi: 10.21608/zjar.2018.49129
112. Richardson PN. British and European legislation regulating rhabditid nematodes. Biocontrol Sci Techn (1996) 6:449–64. doi: 10.1080/09583159631415
113. Ralf-Udo Ehlers. Regulation of Biological Control Agents, Springer Ed. (2011). pp. 416. doi: 10.1007/978-90-481-3664-3
114. Notomi T, Okayama H, Masubuchi H, Yonekawa T, Watanabe K, Amino N, et al. Loop-mediated isothermal amplification of DNA. Nucleic Acids Res (2000) 15:28. doi: 10.1093/nar/28.12.e63
115. Marianelli L, Paoli F, Torrini G, Mazza G, Benvenuti C, Binazzi F, et al. Entomopathogenic nematodes as potential biological control agents of Popillia japonica (Coleoptera, Scarabaeidae) in Piedmont Region (Italy). J Appl Entomol (2018) 142:311–8. doi: 10.1111/jen.12470
Keywords: Steinernematidae, Heterorhabditidae, microbial control, survey, EPN native strains
Citation: Tarasco E, Fanelli E, Salvemini C, El-Khoury Y, Troccoli A, Vovlas A and De Luca F (2023) Entomopathogenic nematodes and their symbiotic bacteria: from genes to field uses. Front. Insect Sci. 3:1195254. doi: 10.3389/finsc.2023.1195254
Received: 28 March 2023; Accepted: 04 August 2023;
Published: 29 August 2023.
Edited by:
Jay Daniel Evans, Agricultural Research Service (USDA), United StatesReviewed by:
Adler Ray Dillman, University of California, Riverside, United StatesCopyright © 2023 Tarasco, Fanelli, Salvemini, El-Khoury, Troccoli, Vovlas and De Luca. This is an open-access article distributed under the terms of the Creative Commons Attribution License (CC BY). The use, distribution or reproduction in other forums is permitted, provided the original author(s) and the copyright owner(s) are credited and that the original publication in this journal is cited, in accordance with accepted academic practice. No use, distribution or reproduction is permitted which does not comply with these terms.
*Correspondence: Eustachio Tarasco, ZXVzdGFjaGlvLnRhcmFzY29AdW5pYmEuaXQ=
Disclaimer: All claims expressed in this article are solely those of the authors and do not necessarily represent those of their affiliated organizations, or those of the publisher, the editors and the reviewers. Any product that may be evaluated in this article or claim that may be made by its manufacturer is not guaranteed or endorsed by the publisher.
Research integrity at Frontiers
Learn more about the work of our research integrity team to safeguard the quality of each article we publish.