- Department of Plant Pathology, Entomology and Microbiology, Iowa State University, Ames, IA, United States
Mosquitoes are the most important animal vector of disease on the planet, transmitting a variety of pathogens of both medical and veterinary importance. Mosquito-borne diseases display distinct seasonal patterns driven by both environmental and biological variables. However, an important, yet unexplored component of these patterns is the potential for seasonal influences on mosquito physiology that may ultimately influence vector competence. To address this question, we selected Culex pipiens, a primary vector of the West Nile virus (WNV) in the temperate United States, to examine the seasonal impacts on mosquito physiology by examining known immune and bacterial components implicated in mosquito arbovirus infection. Semi-field experiments were performed under spring, summer, and late-summer conditions, corresponding to historically low-, medium-, and high-intensity periods of WNV transmission, respectively. Through these experiments, we observed differences in the expression of immune genes and RNA interference (RNAi) pathway components, as well as changes in the distribution and abundance of Wolbachia in the mosquitoes across seasonal cohorts. Together, these findings support the conclusion that seasonal changes significantly influence mosquito physiology and components of the mosquito microbiome, suggesting that seasonality may impact mosquito susceptibility to pathogen infection, which could account for the temporal patterns in mosquito-borne disease transmission.
Introduction
Several mosquito-borne pathogens, such as malaria, dengue, and West Nile virus (WNV), display seasonal patterns in their transmission (1–6) that are driven by environmental variables that influence mosquito life-history traits (7–9), physiology (10, 11), and abundance (11–14), which ultimately shape mosquito-borne disease transmission (15). However, there is little information on how seasonal trends may influence mosquito susceptibility to pathogen infection.
The ability of a mosquito to transmit disease, otherwise known as vector competence, is a multivariate trait incorporating aspects of innate immunity, the microbiota, and host physiology (16–21). RNA interference (RNAi) pathways serve as the primary mechanism for controlling virus infection in the mosquito host (18–20). This includes the prominent roles of Dicer-2 (DCR2) and Argonaute-2 (AGO2) in the production of small interfering RNAs (siRNAs) that cause the targeted degradation of viral RNAs (18–20). In addition, the activation of the JAK-STAT, Toll, and IMD immune signaling pathways initiates the expression of downstream effector genes [such as cecropin (22, 23) and Vago (24, 25)] that limit virus infection (18, 19). The mosquito microbiome can also have a significant impact on vector competence, influencing infection outcomes for a variety of mosquito-borne pathogens (26–28).
The endosymbiont Wolbachia pipientis naturally infects a wide range of arthropods, including the mosquito Culex pipiens (29). Although Wolbachia is often associated with host alterations to reproduction (30), the presence of Wolbachia has been shown to influence mosquito arbovirus infection in both natural (31) and artificial hosts (32, 33). When paired with the previous observation that Wolbachia-induced arbovirus resistance is density dependent (32, 34), the presence of Wolbachia can have a significant impact on mosquito vector competence.
Temperature has been implicated as the primary driver of seasonal transmission dynamics (15, 35–41) and vector competence (37, 42), with known impacts on mosquito immune function (such as melanization and phagocytosis) (43, 44) and immune gene expression (43–45), which serve as the first line of defense against invading pathogens. Temperature has also been implicated in the shaping of the mosquito microbiota (46), most notably that of Wolbachia (47–49), which can have positive or negative impacts on arbovirus infection (32, 33). Moreover, temperature can also influence the efficacy of the RNAi pathway (50), which is integral to mosquito antiviral immunity (18–20). Although these studies demonstrate the complexity and widespread influence of temperature on the mosquito vector, it is currently unclear how temperature in the context of other seasonal factors, such as photoperiod and relative humidity, may together influence mosquito vector competence.
To approach this question, we performed semi-field experiments with a laboratory-derived strain of Culex pipiens, a primary vector of WNV in the United States, to examine potential seasonal differences in mosquito host physiology that could influence vector competence. We demonstrate that adult female Cx. pipiens reared under spring, summer, and late-summer conditions display distinct differences in the expression of immune genes and RNAi pathway components, and in Wolbachia abundance. These data suggest that mosquito physiology and vector competence is dynamic, where different seasonal environmental conditions may have a significant influence on mosquito susceptibility to pathogen infection. As a result, our data suggest that environmental conditions at different times of the year likely influence vector susceptibility and seasonal trends of mosquito-borne disease transmission that are potentially transferable to other vector–pathogen systems.
Materials and methods
Culex pipiens rearing and maintenance
A laboratory colony of Cx. pipiens mosquitoes, originally isolated from field collections in Ames, IA, United States, was maintained at 25°C, 85% relative humidity, and 16 : 8 hours (L : D; light : dark) on 10% sucrose ad libitum. Larvae were maintained using an equal mix of crushed Milk-Bone® and TetraMin® fish food, while commercial sheep’s blood (Hemostat Laboratories), provided via artificial membrane feeding, was used for blood feeding and subsequent egg production.
Semi-field studies
To determine how different seasonal environmental conditions might influence mosquito physiology, we performed semi-field experiments with a laboratory colony of Cx. pipiens, in a similar manner to previous studies (11) at different times of the year. Initiated with first-instar larvae, mosquitoes were reared to adulthood under spring (week 19, May), summer (week 30, July), and late-summer (week 35, late August) conditions (Figure 1A). These time points are representative of periods of little-to-no WNV activity, increasing WNV activity, and peak WNV activity, respectively, which ultimately shape the seasonality of WNV transmission (5, 6).
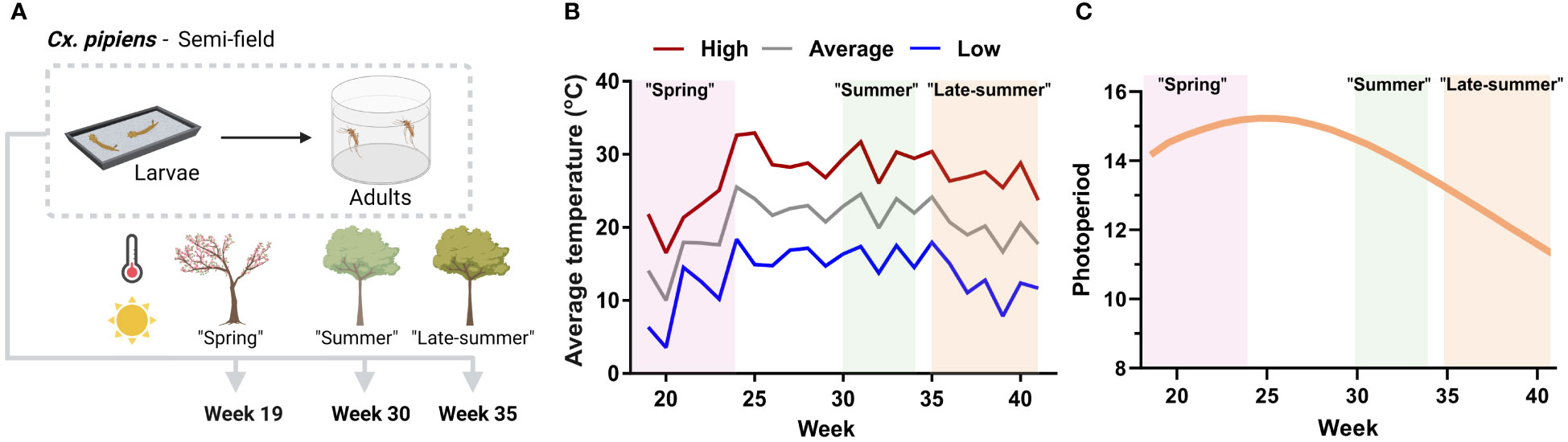
Figure 1 Overview and temperature conditions of the semi-field experiments examining Culex pipiens seasonality. (A) Graphical overview of semi-field experiments where first-instar Culex pipiens larvae were reared outside at three time points (week 19, 30, and 35 of the year) to represent spring, summer, and late-summer conditions. Mosquitoes were reared outside for their entire development, first in metal trays for larval growth, then the pupae were collected and placed in eclosion chambers to allow for adult emergence. Adult female mosquitoes were collected 5–8 days post emergence for further molecular analysis. Mosquitoes were obtained from a laboratory colony of Cx. pipiens. (B) Conditions experienced by each study group, with temperatures reflecting the weekly average of the daily high, average, and low temperatures for Ames, IA, United States, in 2021 when the experiments were performed. (C) Photoperiod (daylight) averages are displayed by week, highlighting conditions for each study group. Graphics in (A) were created with BioRender.com.
For the semi-field studies, first-instar larvae from our laboratory colony of Cx. pipiens were placed in metal rearing trays covered by a tempered glass pane to provide protection from the elements, contaminants, or potential predation. Rearing trays were placed at two locations in Ames, IA, United States, at epidemiological weeks 19 (9 May), 30 (25 July), and 35 (29 August) in 2021, coinciding with periods of differing mosquito activity and WNV transmission intensity in the state of Iowa (5, 11, 14, 51, 52). The use of adult mosquitoes at week 30 corresponded with previous semi-field studies (11), with the resulting mosquito samples shared between experiments. Larval density in each experimental condition was approximately 300 per tray in 1 L of distilled water, with larvae maintained on an equal mix of crushed Milk-Bone® and TetraMin® fish food. Resulting pupae were collected and placed in special eclosion chambers (BioQuip) directly adjacent to the larval rearing trays where, following emergence, adults were maintained on 10% sucrose under semi-field conditions until the collection of mosquito samples and sheltered under a metal cage to provide protection from animal disturbance. The last cup of adults was collected on 15 October (week 41), terminating the semi-field study.
Environmental data
Daily high and low temperatures (°C) were collected for the study period from May to October using the southeast Ames station (IA0203) of the Iowa State University Mesonet (https://mesonet.agron.iastate.edu) to understand semi-field temperature conditions. A 30-year average was provided from the central Iowa station (IA50014) to place the study year’s values in a larger context. Diurnal temperature ranges were determined using the central Iowa station as the difference between the average daily high temperature and average daily low temperature for each week. Photoperiod data were obtained from an online sunrise/sunset table for Des Moines, IA, United States (www.timeanddate.com), with hours of daylight displayed as average weekly values. Data were visualized in R (version 3.6.3) using the ggplot2 package.
Gene expression analysis
Adult female Cx. pipiens (5–8 days post eclosion) were collected from laboratory or semi-field samples and stored at –80°C for later processing. Mosquitoes were pooled into groups of 5–10 mosquitoes for analysis, with three or more replicates per experimental treatment. RNA was extracted from stored samples using Trizol® and cDNA was synthesized using the RevertAid cDNA kit (Thermo Fisher Scientific). Immune genes previously implicated in antiviral immunity were selected from previous studies (24, 50), with primer sequences listed in Table S1. Relative gene expression was measured via quantitative reverse transcription-polymerase chain reaction (qRT-PCR) using a Quant Studio 3 (Thermo Fisher Scientific) and PowerUp SYBR Green (Thermo Fisher Scientific) under the following conditions: 50°C for 2 min, 95°C for 15 s, and 60°C for 1 min for 40 cycles.
Wolbachia quantification in Culex pipiens
An initial PCR was performed to amplify an approximately 900-bp fragment of the Wolbachia 16S ribosomal RNA gene from our laboratory colony of Cx. pipiens using previously described methods with Wolbachia-specific primers (53) (Table S2). Following cloning and Sanger sequencing, the resulting 16S sequence was deposited in GenBank (accession number OQ034610). From this sequence, specific Wolbachia primers derived from our Cx. pipiens colony (ISU Wolb), amplifying a 249-bp amplicon, were designed to determine relative Wolbachia titers when normalized to transcript levels of ribosomal protein L32 (Rpl32) (48, 54) (Table S2). To determine Wolbachia titers across laboratory and seasonal conditions, dissected ovaries and ovariectomized carcasses were stored at –80°C until further processing. DNA was isolated using the Marriot DNA extraction procedure as described previously (5, 55–57) and stored at –20°C prior to future use. Amplification was performed by qRT-PCR using a Quant Studio 3 (Thermo Fisher) and PowerUp SYBR Green (Thermo Fisher) under the following conditions: 50°C for 2 min, 95°C for 15 s, and 60°C for 1 min for 40 cycles. Wolbachia titers were determined by calculating the delta Ct of Wolbachia copies relative to Rpl32 and transformed (2n) to produce relative Wolbachia density estimates for each tissue sample (48, 54).
Statistical analyses
All statistical analyses were performed using GraphPad (Prism version 7). One-way non-parametric ANOVA tests (Kruskal–Wallis) with Dunn’s post hoc analysis being used to test differences in gene expression and Wolbachia titers.
Results
Semi-field studies to address mosquito seasonality
To assess the seasonal conditions of our semi-field studies (outlined in Figure 1A), we examined the effects of temperature (Figure 1B) and photoperiod (Figure 1C) for each of the semi-field cohorts during our study period.
The spring cohort experienced the largest variation in temperature, recording the coldest weekly low temperature of 3.6°C in week 20 and the warmest weekly high of 32.6°C in week 24 of our study period (Figure 1B, Table 1). In contrast, the summer cohort experienced the most stable temperature conditions, with little fluctuation in daily temperature ranges, compared with the other experimental groups (Figures 1, S1). The late-summer cohort faced declining temperatures during its development, with daily lows dipping below 10°C in week 39 (Figure 1B). Although life-history traits were not directly collected in these experiments, the summer (week 30) cohort had the shortest developmental time and highest percentage of larvae reaching adulthood. By comparison, mosquito development in both the spring and late-summer cohorts was extended. Together, these data suggest that weekly temperature fluctuations are much more dynamic in the spring and late summer, such that the effects of temperature may influence mosquito development and physiology very differently than the more uniform summer conditions.
An additional measurable aspect of our seasonal conditions is the photoperiod, for which the spring cohort experienced the highest levels, averaging 14 hours and 46 minutes of daylight (Figure 1C). The amount of daylight decreased across the other seasonal cohorts, with the summer condition experiencing a slight decrease (14 hours and 12 minutes) and the late-summer cohort subjected to a prominent decrease in photoperiod of approximately 2 hours (12 hours and 23 minutes; Figure 1C).
Seasonality influences key components of mosquito antiviral immunity
With previous studies demonstrating the effects of temperature on mosquito immune function (43–45, 50), we examined immune gene expression in the context of our seasonal conditions. With RNA interference (RNAi) considered the primary mechanism of antiviral immunity in mosquitoes (58, 59), we determined the relative gene expression of two primary RNAi pathway components, Dicer-2 (DCR2) and Argonaute-2 (AGO2) (50, 58) in the context of our semi-field experiments. Both genes displayed significantly increased expression during the summer cohort when compared with the laboratory colony or other experimental semi-field groups (Figure 2A), suggesting elevated RNAi activity and increased antiviral defenses during this time period.
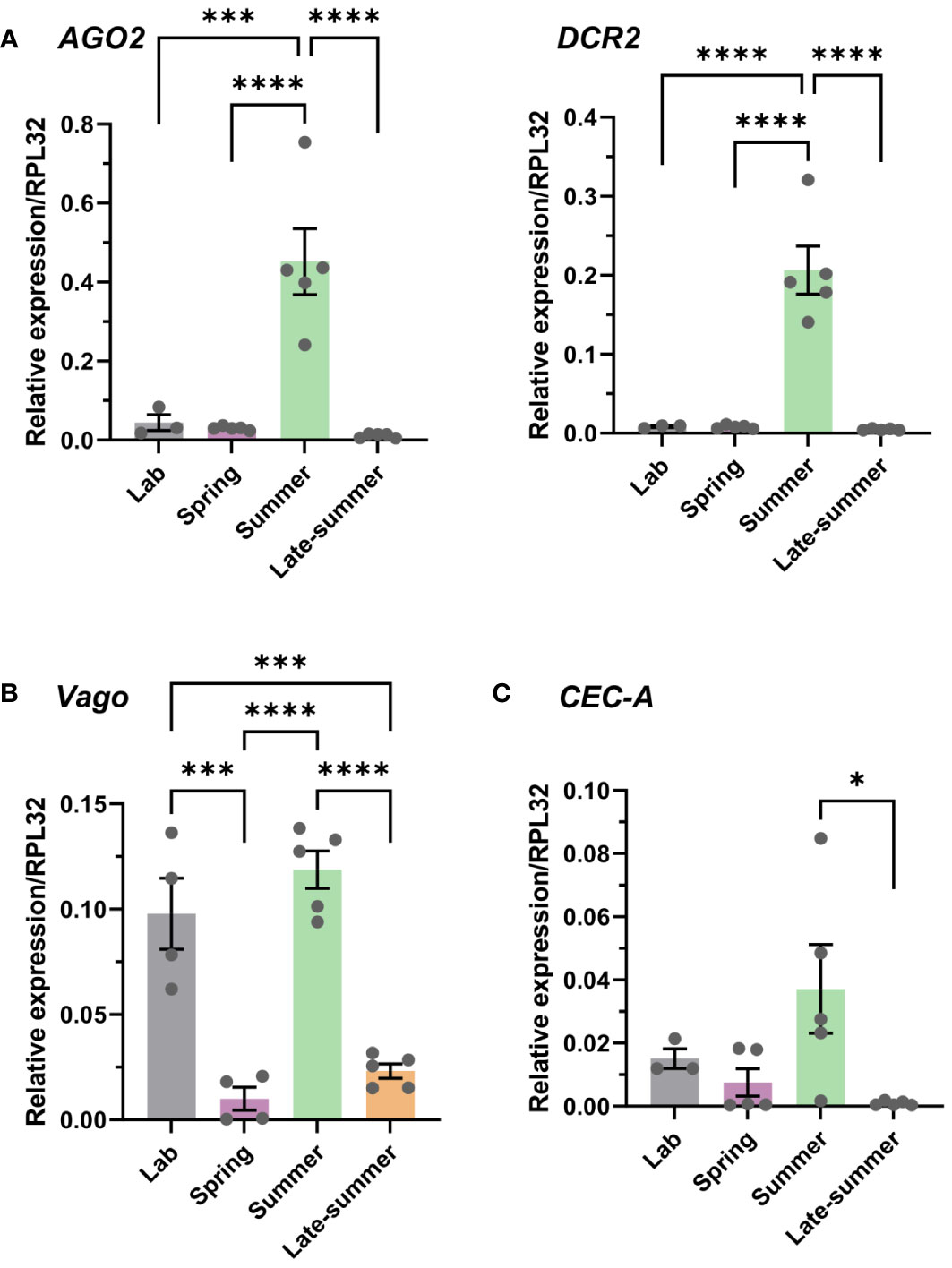
Figure 2 Seasonality of Culex pipiens immune gene expression. Gene expression was examined using samples from our Cx. pipiens colony under standard rearing conditions (laboratory) and across seasonal conditions (spring, summer, and late summer) from semi-field experiments. Expression of (A) genes involved in the RNA interference (RNAi) pathway (AGO2 and DCR2), (B) the immune factor Vago, or (C) the antimicrobial gene CEC-A were evaluated by quantitative reverse transcription- polymerase chain reaction (qRT-PCR). Each replicate contained groups of four pooled mosquitoes, with three or more independent samples per experimental condition. Data are displayed as the mean ± SEM, with significance determined using a one-way ANOVA with a Tukey’s multiple comparison test. Significant differences between conditions are denoted by asterisks (*, p < 0.05; ***, p < 0.001; ****, p < 0.0001); all other comparisons between conditions were not significant. SEM, standard error of the mean.
In addition, we also examined Vago and cecropin A (CEC-A) expression, immune genes previously implicated in the Culex antiviral response to WNV (24, 25) and as an important antimicrobial peptide acting on a wide range of pathogens (60, 61) including viruses, respectively (22, 23). Vago displayed reduced levels of expression during the spring and late-summer conditions (Figure 2B), whereas CEC-A displayed a similar, less pronounced phenotype with significantly reduced expression during the late-summer time period (Figure 2C). This suggests that Vago- and CEC-A-mediated immune responses may be attenuated during these seasonal time points. Together, these data suggest that important mediators of the mosquito immune response and antiviral immunity are influenced by seasonality, suggesting that mosquito susceptibility to pathogen infection may be highly variable during the course of the season.
Seasonality influences Wolbachia abundance and tissue localization
Cx. pipiens are natural hosts of Wolbachia, insect endosymbionts that influence mosquito vector competence for arbovirus infection (32, 33). Although Wolbachia dynamics in response to temperature have been explored extensively in other mosquito species (47–49), the impacts of temperature on Wolbachia in Cx. pipiens have not been previously explored. Although Wolbachia are commonly associated with germ-line tissues, Wolbachia can also be found within somatic tissues of Culex species (33, 54, 62). For this reason, we specifically examined Wolbachia titers by qRT-PCR (54) in the ovaries and the dissected ovariectomized carcass of adult females reared in the laboratory and our semi-field conditions (Figure 3). When compared with the results from laboratory-reared mosquitoes, the Wolbachia titers in the ovaries of mosquitos reared in semi-field conditions were significantly reduced across seasonal conditions, with the reduction in Wolbachia the most prominent at the spring time point (Figure 3), thus demonstrating that Wolbachia abundance in the mosquito ovary notably changes throughout the year. As expected, Wolbachia abundance was substantially higher in germline tissue than in somatic tissue (Figure 3). Wolbachia levels were slightly reduced in somatic tissues during the late summer, yet did not display similar differences across seasonal time points (Figure 3).
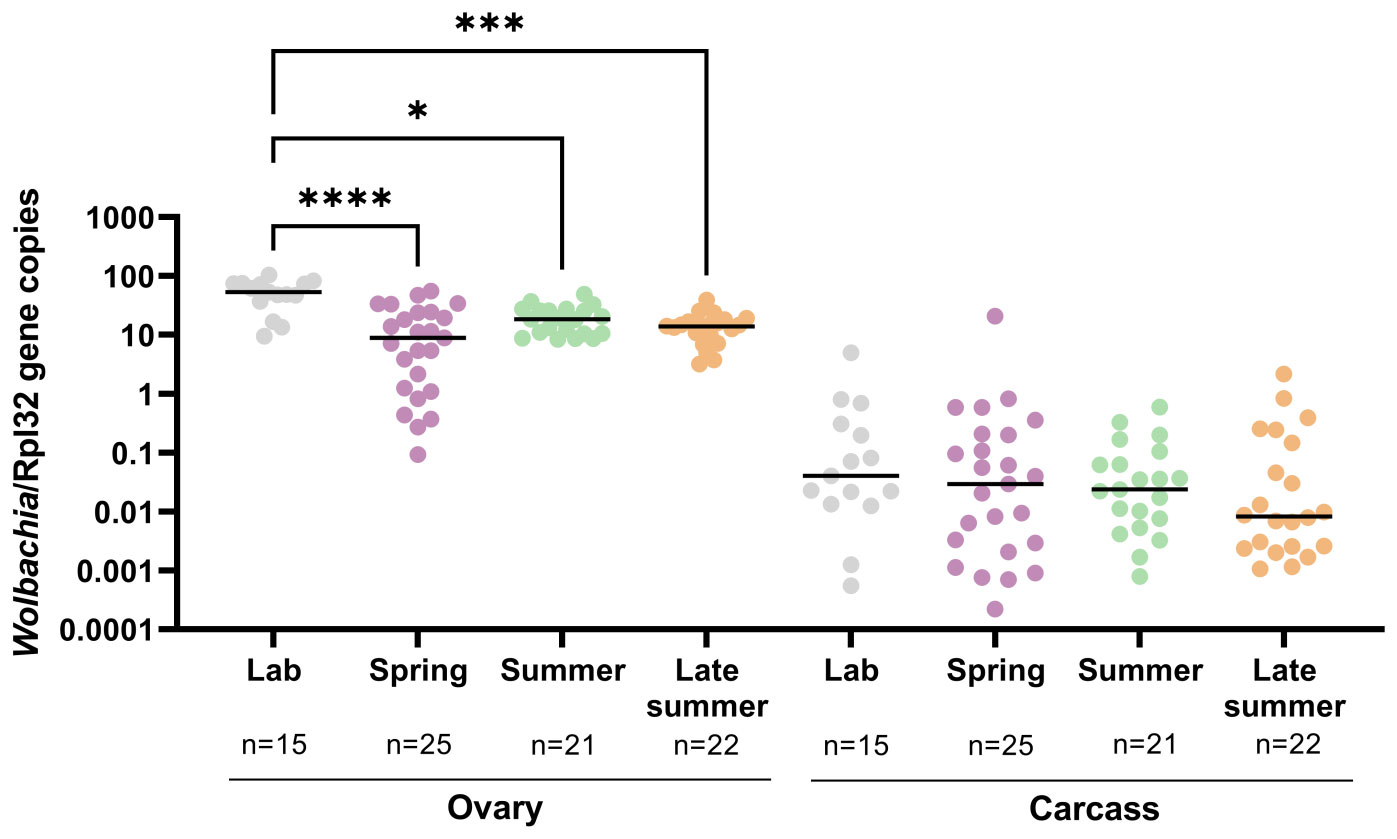
Figure 3 Seasonality of Wolbachia titers in Culex pipiens ovary and carcass samples. Wolbachia titers (16s RNA) were examined in individual Cx. pipiens ovary or carcass (remaining tissue following ovary dissection) samples reared in the laboratory or under spring, summer, and late-summer semi-field conditions by quantitative reverse transcription- polymerase chain reaction (qRT-PCR) and normalized to Rpl32 expression. A one-way non-parametric ANOVA (Kruskal–Wallis) with a Dunn’s multiple comparison test was used to determine significance. Significant differences between conditions are denoted by asterisks (*, p < 0.05; ***, p < 0.001; ****, p < 0.0001).
Discussion
Although the seasonal trends of mosquito-borne diseases are well established, few studies have attempted to understand the mechanisms by which mosquito vector competence may change across seasons. To begin to address this question, we performed semi-field studies to measure changes in components that influence mosquito vector competence (immune gene expression and Wolbachia dynamics) in Cx. pipiens across seasonal time points that reflect periods of low- to high-WNV transmission trends.
WNV exhibits clear seasonal transmission trends in Culex populations and human cases, with infections peaking in the late summer across the United States (5, 6), yet the mechanisms driving these seasonal trends have been largely unexplored. Although previous studies have suggested that the spatiotemporal abundance of Culex vector populations in the summer and late summer can lead to increased levels of WNV infection in mosquito populations (14, 52), overall Culex abundance in Iowa typically peaks much earlier in the season (i.e., May/June) (5, 11, 14, 51, 52). Therefore, combining both mosquito abundance and infection data (vector index) is more predictive than mosquito abundance alone in predicting human WNV cases (63–66), demonstrating the importance of identifying the physiological components that contribute to mosquito infection and transmission of WNV. Although climate conditions are known to influence interannual differences in mosquito populations (14) and promote virus amplification (35, 41), the influence of seasonal changes on mosquito physiology and vector competence have not been adequately addressed outside of the context of diapause (11, 67, 68). Through the use of seasonal cohorts in our semi-field studies, we have demonstrated that components of the RNAi pathway and immune-related genes are differentially expressed over the spring, summer, and late-summer seasons, suggesting that the susceptibility of Cx. pipiens to infection is highly variable throughout the year. In addition, differences in Wolbachia abundance and localization in somatic and germline tissues may similarly contribute to Cx. pipiens physiology and vector competence.
Temperature, a key component of seasonality, has previously been found to have an effect on mosquito immune function (43) and vector competence in virus infection (38–40). In Culex tarsalis, increasing temperatures resulted in fewer infected females following exposure to WEE (69), suggesting that the antiviral immune response was stronger at higher temperatures. This is further supported by the results from a study by Adelman et al. (50), which used a transgenic Aedes aegypti sensor strain to investigate the impacts of temperature on the RNAi pathway, demonstrating the destabilization of RNAi at cooler temperatures (18°C). Our results show that the summer-reared group (experiencing uniformly warm temperatures) displayed the highest expression of the RNAi machinery components Dcr-2 and Ago-2. These genes were expressed at much lower levels during the spring and late summer when mosquitoes experienced cooler temperatures. This indicates that the RNAi pathway may be attenuated during these seasonal time frames, corresponding with the initiation of viral cycling in the spring and increased frequency of mosquito WNV infections in the late summer. However, these observations should be directly tested in the future through WNV infection experiments under seasonal conditions, and, as a result, the lack of direct testing represents a limitation in the interpretation of our current study.
Similar trends were also found for other components of the immune system, such as Vago and CEC-A, which also displayed reduced levels of expression in the spring and late summer. Vago has been described as an interferon-like cytokine that activates the Jak-STAT pathway to limit WNV infection in Culex quinquefasciatus (24), whereas cecropins and other antimicrobial peptides (AMPs) have been implicated in antiviral immunity in insects (22, 23). Although the functional role of Vago has not previously been explored in the context of seasonality, previous studies have demonstrated that cecropin expression is influenced by temperature (42, 43). Together, our data suggest that the downregulation of these genes during the spring and late summer likely contribute to seasonal differences in Culex vector competence and susceptibility to WNV infection, although these conclusions need to be further tested in future WNV infection experiments.
In addition to components of the innate immune system, microbes can also modulate mosquito physiology and vector competence in important ways. Wolbachia are insect endosymbionts that affect many aspects of host biology, from reproduction (30, 70) to pathogen infection (32, 33, 71, 72). Wolbachia are distinct from the general gut microbiota, as they are intracellular bacteria distributed in both somatic and germ-line tissues (62). Although our data suggest that changes in ovary Wolbachia titers could influence cytoplasmic incompatibility and reproduction, we did not directly address whether or not these changes have measurable reproductive phenotypes and will look to address these questions in future experiments. Similar to previous studies evaluating Wolbachia in field-collected Cx. pipiens (54), we found that somatic titers of Wolbachia were highly variable in laboratory samples and in samples from each of our semi-field conditions. Considering previous evidence that indicates that somatic infection densities of Wolbachia may contribute to WNV resistance (54), it is possible that the decrease in somatic Wolbachia levels in the late summer could influence Cx. pipiens vector competence and susceptibility to WNV infection. However, the lack of significant differences in somatic Wolbachia titers across our seasonal conditions suggests that any alterations to vector competence result from the highly variable somatic Wolbachia infections between individual mosquito samples as opposed to seasonal conditions.
Diurnal temperature fluctuations (i.e., the change between day and night temperatures) are most pronounced during the spring and late summer in temperate climates, and have previously been suggested to influence mosquito vector competence (73–77). This includes recent studies where diurnal temperature fluctuations influenced WNV titers in both Cx. tarsalis and Cx. quinquefasciatus; however, these differences in viral titers did not significantly influence infection, dissemination, or transmission (76). However, these data suggest that the variations in diurnal temperature correspond with seasonal changes, which may have additional impacts on mosquito host physiology and antiviral immunity. As a result, the seasonal differences in immune gene expression and Wolbachia abundance identified in our study may account for these physiological changes, thereby warranting further laboratory studies to distinguish the effects of seasonal temperature differences and diurnal temperature fluctuations.
Additional seasonal influences beyond that of temperature, such as changes in photoperiod and humidity, have been less explored in the context of mosquito vector competence. Although the combined effects of photoperiod and temperature are essential for promoting mosquito diapause (11, 78), the evidence suggests that photoperiod alone can influence mosquito size, lifespan, and propensity for blood-feeding (79). This suggests that other aspects of mosquito physiology may be influenced by photoperiod, yet these have not been adequately explored to date. Although humidity is also an important determinant of mosquito lifespan, recent evidence indicates that mosquito dehydration can influence carbohydrate metabolism and blood-feeding behavior, with the potential to increase pathogen transmission (80). However, future studies are needed to fully determine the impacts of humidity and dehydration on mosquito vector competence.
Although these experiments were conducted in mosquitoes reared under semi-field conditions, we believe that our results can similarly be used to interpret trends in natural populations of Cx. pipiens. Our seasonal observations of elevated immune expression in the summer, contrasted by impaired immune function in the spring and late summer, closely correspond to previous studies demonstrating seasonal differences in the vector competence of Cx. tarsalis populations collected from the field (81). Reisen et al. show that higher levels of western equine encephalitis virus were required to infect mosquitoes during the summer months, whereas mosquitoes were much more susceptible to virus infection in the spring and late-summer/fall months, demonstrating important seasonal differences in mosquito vector competence (81). As Cx. tarsalis is not naturally infected with Wolbachia such as Cx. pipiens (33), it is possible that changes to the efficiency of RNAi or immune expression, similar to those in our own results, could account for these observations of seasonal differences in infection outcomes.
Although further studies are required to fully delineate the seasonal influences on mosquito physiology and their impacts on mosquito-borne pathogen infection, we believe that our experiments are an important first step in evaluating the seasonal influences that determine mosquito vector competence.
Data availability statement
The datasets presented in this study can be found in online repositories. The names of the repository/repositories and accession number(s) can be found in the article/Supplementary Material.
Author contributions
EF and RS designed the study. EF performed experiments. EF and RS performed the analysis and wrote the manuscript. Both authors contributed to the article and approved the submitted version.
Funding
This research was supported in part by the USDA National Institute of Food and Agriculture (Hatch Project 101071) and the Midwest Center of Excellence for Vector-Borne Disease by Cooperative Agreement #U01 CK000505, funded by the Centers for Disease Control and Prevention. Its contents are solely the responsibility of the authors and do not necessarily represent the official views of the Centers of Disease Control and Prevention or the Department of Health and Human Services.
Conflict of interest
The authors declare that the research was conducted in the absence of any commercial or financial relationships that could be construed as a potential conflict of interest.
Publisher’s note
All claims expressed in this article are solely those of the authors and do not necessarily represent those of their affiliated organizations, or those of the publisher, the editors and the reviewers. Any product that may be evaluated in this article, or claim that may be made by its manufacturer, is not guaranteed or endorsed by the publisher.
Supplementary material
The Supplementary Material for this article can be found online at: https://www.frontiersin.org/articles/10.3389/finsc.2023.1144072/full#supplementary-material
References
1. Briët OJT, Vounatsou P, Amerasinghe PH. Malaria seasonality and rainfall seasonality in Sri Lanka are correlated in space. Geospat Health (2008) 2:183–90. doi: 10.4081/gh.2008.242
2. Olayemi K, Ande AT, Ayanwale AV, Mohammed AZ, Bello TM, Idris B, et al. Seasonal trends in epidemiological and entomological profiles of malaria transmission in north central Nigeria. Pakistan J Biol Sci (2011) 14:293–9. doi: 10.3923/pjbs.2011.293.299
3. Polwiang S. The time series seasonal patterns of dengue fever and associated weather variables in Bangkok (2003-2017). BMC Infect Dis (2020) 20:208. doi: 10.1186/s12879-020-4902-6
4. Yuan HY, Liang J, Lin PS, Sucipto K, Tsegaye MM, Wen TH, et al. The effects of seasonal climate variability on dengue annual incidence in Hong Kong: a modelling study. Sci Rep (2020) 10:4297. doi: 10.1038/s41598-020-60309-7
5. Dunphy BM, Kovach KB, Gehrke EJ, Field EN, Rowley WA, Bartholomay LC, et al. Long-term surveillance defines spatial and temporal patterns implicating Culex tarsalis as the primary vector of West Nile virus. Sci Rep (2019) 9:6637. doi: 10.1038/s41598-019-43246-y
6. Petersen LR. Epidemiology of West Nile virus in the United States: implications for arbovirology and public health. J Med Entomol (2019) 56:1456–62. doi: 10.1093/jme/tjz085
7. Ciota AT, Matacchiero AC, Marm Kilpatrick A, Kramer LD. The effect of temperature on life history traits of Culex mosquitoes. J Med Entomol (2014) 51:55–62. doi: 10.1603%2Fme13003
8. Wilke ABB, Medeiros-Sousa AR, Ceretti-Junior W, Marrelli MT. Mosquito populations dynamics associated with climate variations. Acta Trop (2017) 166:343–50. doi: 10.1016/j.actatropica.2016.10.025
9. Agyekum TP, Botwe PK, Arko-Mensah J, Issah I, Acquah AA, Hogarh JN, et al. A systematic review of the effects of temperature on Anopheles mosquito development and survival: implications for malaria control in a future warmer climate. Int J Environ Res Public Health (2021) 18:7255. doi: 10.3390/ijerph18147255
10. Denlinger DL, Armbruster PA. Mosquito diapause. Annu Rev Entomol (2014) 59:73–93. doi: 10.1146/annurev-ento-011613-162023
11. Field EN, Shepard JJ, Clifton ME, Price KJ, Witmier BJ, Johnson K, et al. Semi-field and surveillance data define the natural diapause timeline for Culex pipiens across the United States. Commun Biol (2022) 5:1300. doi: 10.1038/s42003-022-04276-x
12. Andraud M, Hens N, Beutels P. A simple periodic-forced model for dengue fitted to incidence data in Singapore. Math Biosci (2013) 244:22–8. doi: 10.1016/j.mbs.2013.04.001
13. Valentine MJ, Ciraola B, Jacobs GR, Arnot C, Kelly PJ, Murdock CC. Effects of seasonality and land use on the diversity, relative abundance, and distribution of mosquitoes on St. Kitts, West indies. Parasites Vectors (2020) 13:543. doi: 10.1186/s13071-020-04421-7
14. Tokarz RE, Smith RC. Crossover dynamics of Culex (Diptera: Culicidae) vector populations determine WNV transmission intensity. J Med Entomol (2020) 57:289–96. doi: 10.1093/jme/tjz122
15. Cator LJ, Johnson LR, Mordecai EA, El Moustaid F, Smallwood TRC, LaDeau SL, et al. The role of vector trait variation in vector-borne disease dynamics. Front Ecol Evol (2020) 8:189. doi: 10.3389/fevo.2020.00189
16. Dennison NJ, Jupatanakul N, Dimopoulos G. The mosquito microbiota influences vector competence for human pathogens. Curr Opin Insect Sci (2014) 3:6–13. doi: 10.1016/j.cois.2014.07.004
17. Smith RC, Vega-Rodríguez J, Jacobs-Lorena M. The Plasmodium bottleneck: malaria parasite losses in the mosquito vector. Mem Inst Oswaldo Cruz (2014) 109:644–61. doi: 10.1590/0074-0276130597
18. Cheng G, Liu Y, Wang P, Xiao X. Mosquito defense strategies against viral infection. Trends Parasitol (2016) 32:177–86. doi: 10.1016/j.pt.2015.09.009
19. Lee WS, Webster JA, Madzokere ET, Stephenson EB, Herrero LJ. Mosquito antiviral defense mechanisms: a delicate balance between innate immunity and persistent viral infection. Parasites Vectors (2019) 12:165. doi: 10.1186/s13071-019-3433-8
20. Blair CD. Mosquito RNAi is the major innate immune pathway controlling arbovirus infection and transmission. Future Microbiol (2011) 6:265–77. doi: 10.2217/fmb.11.11
21. Brackney DE, LaReau JC, Smith RC. Frequency matters: how successive feeding episodes by blood-feeding insect vectors influences disease transmission. PloS Pathog (2021) 17:e1009590. doi: 10.1371/journal.ppat.1009590
22. Feng M, Fei S, Xia J, Labropoulou V, Swevers L, Sun J. Antimicrobial peptides as potential antiviral factors in insect antiviral immune response. Front Immunol (2020) 11:2030. doi: 10.3389/fimmu.2020.02030
23. Huang Z, Kingsolver MB, Avadhanula V, Hardy RW. An antiviral role for antimicrobial peptides during the arthropod response to alphavirus replication. J Virol (2013) 87:42472–80. doi: 10.1128/JVI.03360-12
24. Paradkar PN, Trinidad L, Voysey R, Duchemin J-BJB, Walker PJ. Secreted vago restricts West Nile virus infection in Culex mosquito cells by activating the Jak-STAT pathway. Proc Natl Acad Sci (2012) 109:18915–20. doi: 10.1073/pnas.1205231109
25. Paradkar PN, Duchemin JB, Voysey R, Walker PJ. Dicer-2-Dependent activation of Culex vago occurs via the TRAF-Rel2 signaling pathway. PloS Negl Trop Dis (2014) 8:e0002823. doi: 10.1371/journal.pntd.0002823
26. Cirimotich CM, Dong Y, Clayton AM, Sandiford SL, Souza-Neto JA, Mulenga M, et al. Natural microbe-mediated refractoriness to Plasmodium infection in Anopheles gambiae. Science (2011) 332:855–8. doi: 10.1126/science.1201618
27. Ramirez JL, Short SM, Bahia AC, Saraiva RG, Dong Y, Kang S, et al. Chromobacterium Csp_P reduces malaria and dengue infection in vector mosquitoes and has entomopathogenic and In vitro anti-pathogen activities. PloS Pathog (2014) 10:e1004398. doi: 10.1371/journal.ppat.1004398
28. Barletta ABF, Nascimento-Silva MCL, Talyuli OAC, Oliveira JHM, Pereira LOR, Oliveira PL, et al. Microbiota activates IMD pathway and limits sindbis infection in Aedes aegypti. Parasites Vectors (2017) 10:103. doi: 10.1186/s13071-017-2040-9
29. Rasgon JL, Scott TW. An initial survey for Wolbachia (Rickettsiales:Rickettsiaceae) infections in selected California mosquitoes (Diptera: Culicidae). J Med Entomol (2004) 41:255–7. doi: 10.1603/0022-2585-41.2.255
30. Correa CC, Ballard JWO. Wolbachia associations with insects: winning or losing against a master manipulator. Front Ecol Evol (2016) 3:153. doi: 10.3389/fevo.2015.00153
31. Glaser RL, Meola MA. The native Wolbachia endosymbionts of Drosophila melanogaster and Culex quinquefasciatus increase host resistance to West Nile virus infection. PloS One (2010) 5:e0011977. doi: 10.1371/journal.pone.0011977
32. Moreira LA, Iturbe-Ormaetxe I, Jeffery JA, Lu G, Pyke AT, Hedges LM, et al. AA Wolbachia symbiont in Aedes aegypti limits infection with dengue, chikungunya, and Plasmodium. Cell (2009) 139:1268–78. doi: 10.1016/j.cell.2009.11.042
33. Dodson BL, Hughes GL, Paul O, Matacchiero AC, Kramer LD, Rasgon JL. Wolbachia enhances West Nile virus (WNV) infection in the mosquito Culex tarsalis. PloS Negl Trop Dis (2014) 8:e0002965. doi: 10.1371/journal.pntd.0002965
34. Lu P, Bian G, Pan X, Xi Z. Wolbachia induces density-dependent inhibition to dengue virus in mosquito cells. PloS Negl Trop Dis (2012) 6:e0001754. doi: 10.1371/journal.pntd.0001754
35. Shocket MS, Verwillow AB, Numazu MG, Slamani H, Cohen JM, El Moustaid F, et al. Transmission of West Nile and five other temperate mosquito-borne viruses peaks at temperatures between 23˚C and 26˚C. Elife (2020) 9:e58511. doi: 10.7554/elife.58511
36. Couper LI, Farner JE, Caldwell JM, Childs ML, Harris MJ, Kirk DG, et al. How will mosquitoes adapt to climate warming? Elife (2021) 10:e69630. doi: 10.7554/eLife.69630
37. Bellone R, Failloux AB. The role of temperature in shaping mosquito-borne viruses transmission. Front Microbiol (2020) 11:584846. doi: 10.3389/fmicb.2020.584846
38. Dohm DJ, O’Guinn ML, Turell MJ. Effect of environmental temperature on the ability of Culex pipiens (Diptera: Culicidae) to transmit West Nile virus. J Med Entomol (2002) 39:221–5. doi: 10.1603/0022-2585-39.1.221
39. Turell MJ. Effect of environmental temperature on the vector competence of Aedes taeniorhynchus for Rift Valley fever and Venezuelan equine encephalitis viruses. Am J Trop Med Hyg (1993) 49:672–6. doi: 10.4269/ajtmh.1993.49.672
40. Tsai CH, Chen TH, Lin C, Shu PY, Su CL, Teng HJ. The impact of temperature and Wolbachia infection on vector competence of potential dengue vectors Aedes aegypti and Aedes albopictus in the transmission of dengue virus serotype 1 in southern Taiwan. Parasites Vectors (2017) 10:551. doi: 10.1186/s13071-017-2493-x
41. Paz S. Climate change impacts on West Nile virus transmission in a global context. Philos Trans R Soc B Biol Sci (2015) 370:20130561. doi: 10.1098/rstb.2013.0561
42. Murdock CC, Paaijmans KP, Cox-Foster D, Read AF, Thomas MB. Rethinking vector immunology: the role of environmental temperature in shaping resistance. Nat Rev Microbiol (2012) 10:869–76. doi: 10.1038/nrmicro2900
43. Murdock CC, Paaijmans KP, Bells AS, King JG, Hillyer JF, Read AF, et al. Complex effects of temperature on mosquito immune function. Proc R Soc B Biol Sci (2012) 279:3357–66. doi: 10.1098/rspb.2012.0638
44. Ferreira PG, Tesla B, Horácio ECA, Nahum LA, Brindley MA, de Oliveira Mendes TA, et al. Temperature dramatically shapes mosquito gene expression with consequences for mosquito–Zika virus interactions. Front Microbiol (2020) 11:901. doi: 10.3389/fmicb.2020.00901
45. Wimalasiri-Yapa BMCR, Barrero RA, Stassen L, Hafner LM, McGraw EA, Pyke AT, et al. Temperature modulates immune gene expression in mosquitoes during arbovirus infection: temperature, mosquitoes and arboviruses. Open Biol (2021) 11:200246. doi: 10.1098/rsob.200246
46. Onyango GM, Bialosuknia MS, Payne FA, Mathias N, Ciota TA, Kramer DL. Increase in temperature enriches heat tolerant taxa in Aedes aegypti midguts. Sci Rep (2020) 10:19135. doi: 10.1038/s41598-020-76188-x
47. Lau MJ, Ross PA, Endersby-Harshman NM, Hoffmann AA. Impacts of low temperatures on Wolbachia (Rickettsiales: Rickettsiaceae)-infected Aedes aegypti (Diptera: Culicidae). J Med Entomol (2020) 57:1567–74. doi: 10.1093/jme/tjaa074
48. Ross PA, Axford JK, Yang Q, Staunton KM, Ritchie SA, Richardson KM, et al. Heatwaves cause fluctuations in wMel Wolbachia densities and frequencies in Aedes aegypti. PloS Negl Trop Dis (2020) 14:e0007958. doi: 10.1371/journal.pntd.0007958
49. Ross PA, Wiwatanaratanabutr I, Axford JK, White VL, Endersby-Harshman NM, Hoffmann AA. Wolbachia infections in Aedes aegypti differ markedly in their response to cyclical heat stress. PloS Pathog (2017) 13:e1006006. doi: 10.1371/journal.ppat.1006006
50. Adelman ZN, Anderson MAE, Wiley MR, Murreddu MG, Samuel GH, Morazzani EM, et al. Cooler temperatures destabilize RNA interference and increase susceptibility of disease vector mosquitoes to viral infection. PloS Negl Trop Dis (2013) 7:e0002239. doi: 10.1371/journal.pntd.0002239
51. Sucaet Y, Van Hemert J, Tucker B, Bartholomay LC. A web-based relational database for monitoring and analyzing mosquito population dynamics. J Med Entomol (2008) 45:775–84. doi: 10.1093/jmedent/45.4.775
52. Adelman JS, Tokarz RE, Euken AE, Field EN, Russell MC, Smith RC. Relative influence of land use, mosquito abundance, and bird communities in defining West Nile virus infection rates in Culex mosquito populations. Insects (2022) 13:758. doi: 10.3390/insects13090758
53. O’Neill SL, Giordano R, Colbert AME, Karr TL, Robertson HM. 16S rRNA phylogenetic analysis of the bacterial endosymbionts asociated with cytoplasmic incompatibility in insects. Proc Natl Acad Sci U.S.A. (1992) 89:2699–702. doi: 10.1073%2Fpnas.89.7.2699
54. Micieli MV, Glaser RL. Somatic wolbachia (Rickettsiales: Rickettsiaceae) levels in Culex quinquefasciatus and Culex pipiens (Diptera: Culicidae) and resistance to West Nile virus infection. J Med Entomol (2014) 51:189–99. doi: 10.1603/ME13152
55. Post RJ, Flook PK, Millest AL. Methods for the preservation of insects for DNA studies. Biochem Syst Ecol (1993) 21:85–92. doi: 10.1016/0305-1978(93)90012-G
56. Field EN, Gehrke EJ, Ruden RM, Adelman JS, Smith RC. An improved multiplex polymerase chain reaction (PCR) assay for the identification of mosquito (Diptera: Culicidae) blood meals. J Med Entomol (2020) 57:557–62. doi: 10.1093/jme/tjz182
57. Hall DR, Tokarz RE, Field EN, Smith RC, Studies G. Surveillance and genetic data support the introduction and establishment of Aedes albopictus in Iowa, USA. Sci Rep (2022) 12:2143. doi: 10.1038/s41598-022-06294-5
58. Olson KE, Blair CD. Arbovirus-mosquito interactions: RNAi pathway. Curr Opin Virol (2015) 15:119–26. doi: 10.1016/j.coviro.2015.10.001
59. Wynant N, Santos D, Vanden Broeck J. Biological mechanisms determining the success of RNA interference in insects. Int Rev Cell Mol Biol (2014) 312:139–67. doi: 10.1016/b978-0-12-800178-3.00005-1
60. Hillyer JF. Insect immunology and hematopoiesis. Dev Comp Immunol (2016) 58:102–18. doi: 10.1016/j.dci.2015.12.006
61. Yi HY, Chowdhury M, Huang YD, Yu XQ. Insect antimicrobial peptides and their applications. Appl Microbiol Biotechnol (2014) 98:5807–22. doi: 10.1007/s00253-014-5792-6
62. Pietri JE, DeBruhl H, Sullivan W. The rich somatic life of Wolbachia. Microbiologyopen (2016) 5:923–36. doi: 10.1002/mbo3.390
63. Bolling BG, Barker CM, Moore CG, Pape WJ, Eisen L. Seasonal patterns for entomological measures of risk for exposure to Culex vectors and West Nile virus in relation to human disease cases in northeastern Colorado. J Med Entomol (2009) 46:1519–31. doi: 10.1603/033.046.0641
64. Kilpatrick AM, Pape WJ. Predicting human West Nile virus infections with mosquito surveillance data. Am J Epidemiol (2013) 178:829–35. doi: 10.1093/aje/kwt046
65. Jones RC, Weaver KN, Smith S, Blanco C, Flores C, Gibbs K, et al. Use of the vector index and geographic information system to prospectively inform West Nile virus interventions. J Am Mosq Control Assoc (2011) 27:315–9. doi: 10.2987/10-6098.1
66. Division of Vector-Borne Diseases. West Nile virus in the United States: guidelines for surveillance, prevention, and control (2013). Available at: http://www.cdc.gov/westnile/resources/pdfs/wnvGuidelines.pdf.
67. Robich RM, Denlinger DL. Diapause in the mosquito Culex pipiens evokes a metabolic switch from blood feeding to sugar gluttony. Proc Natl Acad Sci U.S.A. (2005) 102:15912–7. doi: 10.1073/pnas.0507958102
68. Kang DS, Cotten MA, Denlinger DL, Sim C. Comparative transcriptomics reveals key gene expression differences between diapausing and non-diapausing adults of Culex pipiens. PloS One (2016) 11:e0154892. doi: 10.1371/journal.pone.0154892
69. Kramer LD, Hardy JL, Presser SB. Effect of temperature of extrinsic incubation on the vector competence of Culex tarsalis for western equine encephalomyelitis virus. Am J Trop Med Hyg (1983) 32:1130–9. doi: 10.4269/ajtmh.1983.32.1130
70. Altinli M, Gunay F, Alten B, Weill M, Sicard M. Wolbachia diversity and cytoplasmic incompatibility patterns in Culex pipiens populations in Turkey. Parasites Vectors (2018) 11:198. doi: 10.1186/s13071-018-2777-9
71. Dutra HLC, Rocha MN, Dias FBS, Mansur SB, Caragata EP, Moreira LA. Wolbachia blocks currently circulating Zika virus isolates in Brazilian Aedes aegypti mosquitoes. Cell Host Microbe (2016) 19:771–4. doi: 10.1016/j.chom.2016.04.021
72. Hussain M, Lu G, Torres S, Edmonds JH, Kay BH, Khromykh AA, et al. Effect of Wolbachia on replication of West Nile virus in a mosquito cell line and adult mosquitoes. J Virol (2013) 87:851–8. doi: 10.1128/JVI.01837-12
73. Carrington LB, Seifert SN, Willits NH, Lambrechts L, Scott TW. Large diurnal temperature fluctuations negatively influence Aedes aegypti (Diptera: Culicidae) life-history traits. J Med Entomol (2013) 50:43–51. doi: 10.1603/ME11242
74. Lambrechts L, Paaijmans KP, Fansiri T, Carrington LB, Kramer LD, Thomas MB, et al. Impact of daily temperature fluctuations on dengue virus transmission by Aedes aegypti. Proc Natl Acad Sci (2011) 108:7460–5. doi: 10.1073/pnas.1101377108
75. Alto BW, Wiggins K, Eastmond B, Ortiz S, Zirbel K, Lounibos LP. Diurnal temperature range and chikungunya virus infection in invasive mosquito vectors. J Med Entomol (2018) 55:217–24. doi: 10.1093/jme/tjx182
76. McGregor BL, Kenney JL, Connelly CR. The effect of fluctuating incubation temperatures on West Nile virus infection in Culex mosquitoes. Viruses (2021) 13:1822. doi: 10.3390/v13091822
77. Paaijmans KP, Blanford S, Bell AS, Blanford JI, Read AF, Thomas MB. Influence of climate on malaria transmission depends on daily temperature variation. Proc Natl Acad Sci U.S.A. (2010) 107:15135–9. doi: 10.1073/pnas.1006422107
78. Eldridge BF. Environmental control of ovarian development in mosquitoes of the Culex pipiens complex. Am Assoc Adv Sci (1966) 151:826–8. doi: 10.1126/science.151.3712.826
79. Costanzo KS, Schelble S, Jerz K, Keenan M. The effect of photoperiod on life history and blood-feeding activity in Aedes albopictus and Aedes aegypti (Diptera: Culicidae). J Vector Ecol (2015) 40:164–71. doi: 10.1111/jvec.12146
80. Hagan RW, Didion EM, Rosselot AE, Holmes CJ, Siler SC, Rosendale AJ, et al. Dehydration prompts increased activity and blood feeding by mosquitoes. Sci Rep (2018) 8:6804. doi: 10.1038/s41598-018-24893-z
81. Reisen WK, Hardy JL, Presser SB, Chiles RE. Seasonal variation in the vector competence of Culex tarsalis (Diptera: Culicidae) from the coachella valley of California for Western equine encephalomyelitis and St. Louis Encephalitis Viruses J Med Entomol (1996) 33:433–7. doi: 10.1093/jmedent/33.3.433
Keywords: seasonality, Culex pipiens, mosquito, semi-field, physiology, Wolbachia, gene expression, RNAi
Citation: Field EN and Smith RC (2023) Seasonality influences key physiological components contributing to Culex pipiens vector competence. Front. Insect Sci. 3:1144072. doi: 10.3389/finsc.2023.1144072
Received: 13 January 2023; Accepted: 12 May 2023;
Published: 25 May 2023.
Edited by:
Yoonseong Park, Kansas State University, United StatesReviewed by:
L. Paulina Maldonado-Ruiz, Kansas State University, United StatesBerlin L. Londono-Renteria, Tulane University, United States
Copyright © 2023 Field and Smith. This is an open-access article distributed under the terms of the Creative Commons Attribution License (CC BY). The use, distribution or reproduction in other forums is permitted, provided the original author(s) and the copyright owner(s) are credited and that the original publication in this journal is cited, in accordance with accepted academic practice. No use, distribution or reproduction is permitted which does not comply with these terms.
*Correspondence: Ryan C. Smith, c21pdGhyQGlhc3RhdGUuZWR1