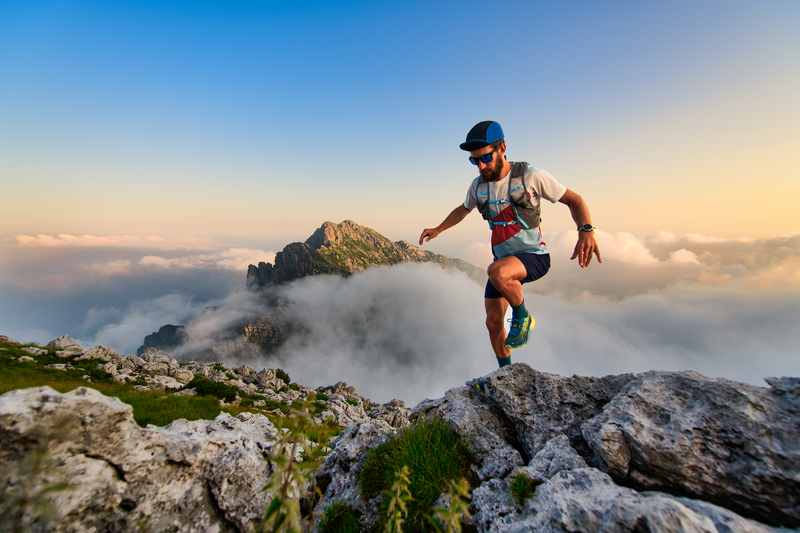
94% of researchers rate our articles as excellent or good
Learn more about the work of our research integrity team to safeguard the quality of each article we publish.
Find out more
REVIEW article
Front. Ind. Microbiol. , 18 January 2024
Sec. Fuels and Chemicals
Volume 1 - 2023 | https://doi.org/10.3389/finmi.2023.1319774
This article is part of the Research Topic Editors' Showcase: Fuels and Chemicals View all 6 articles
Lignocellulose biomass presents a promising and renewable alternative to fossil fuels. Numerous engineered microorganisms have been developed to efficiently utilize this biomass and convert it into valuable platform chemicals. This article provides an overview of the extensive metabolic engineering strategies employed to create robust microbial cell factories for lignocellulose biorefinery. The focus lies on the production of various chemicals including succinic acid, lactic acid, 3-hydroxypropinic acid, xylitol, biohydrocarbons, itaconic acid, 2-phenylethanol, 1,2,4-butanetriol, and 2,3-butanediol from lignocellulose hydrolysate, especially hemicellulose. Additionally, the article briefly discusses the techno-economic analysis, challenges, and future prospects for achieving more sustainable production of these chemicals.
Lignocellulose biomass (LCB) is the most abundant and cost-effective renewable source available on earth with an astonishing annual production rate of 181.5 billion tons worldwide (Dahmen et al., 2019). Notably, out of this total, 1 billion tons of LCB are derived from corn stover, 529 million tons from wheat straw, 731 million tons from rice straw, and 540 million tons from sugarcane bagasse. Perennial grasses also account for 13.5-80.1 tons of LCB (Deng et al., 2023). The remaining quantity of LCB is generated from forestry residues, woody biomass, and various other sources. Nevertheless, a mere 3-4% of LCB is currently used for energy, materials, and fodders. A vast amount of LCB still remains unutilized (Chandel et al., 2020).
The primary aim of the LCB biorefinery is to capitalize on the unused LCB by converting it into various commercially valuable products (Figure 1). Initially, LCB was widely used for the production of biofuels such as ethanol, butanol, and hydrogen (Ashokkumar et al., 2022; Latine et al., 2023). However, in recent times, there has been extensive exploration of LCB for the production of a wide range of bulk and specialty chemicals (Ashokkumar et al., 2022; Lu et al., 2022). More than 200 chemicals are reported to be produced from LCB either through chemical or biological processes (Isikgor and Becer, 2015). In 2004 and 2010, the US Department of Energy listed the top 10 biobased chemicals which included organic acids (succinic acid, lactic acid, 3-hydroxypropionic acid, and levulinic acid), furans (furfural, hydroxymethylfurfural, and furan-2,5-dicarboxylic acid), glycerol and derivatives, sugar alcohol (sorbitol and xylitol), biohydrocarbons (isoprene and others), and ethanol (Bozell and Petersen, 2010). Since the publication of this report, a large number of microbial processes have been developed to utilize LCB for the production of these chemicals, some of them are either already commercialized or nearing commercialization (Table 1). For instance, succinic acid has been successfully produced from various LCB sources such as sugarcane bagasse, corn stover, oil palm trunk, empty fruit bunches, grape pomace, straws, and grasses. Microbes such as Actinobacillus succinogenes, Basfia succiniciproducens, and Saccharomyces cerevisiae have been utilized to produce high titers of succinic acid, ranging from 1.07-40.2 g/L (Bu et al., 2019; Bukhari et al., 2020; Lo et al., 2020; Filippi et al., 2021; Vallecilla-Yepez et al., 2021; Jokodola et al., 2022; Lee et al., 2022; Bukhari et al., 2023; Robertiello et al., 2023; Zevallos Torres et al., 2023). Lactic acid, another chemical, is produced through microbial processes involving Lactobacillus spps, Bacillus coagulans, and Rhizopus oryzae with various feedstocks such as corn stover, straw, sugarcane bagasse and molasses, beechwood, pine, and grasses. The production titer of lactic acid varied widely from 4.4-129.47 g/L (Azaizeh et al., 2020; Karnaouri et al., 2020; Ma et al., 2020; Karnaouri et al., 2021; Pontes et al., 2021; Sivagurunathan et al., 2022; Svetlitchnyi et al., 2022; Yang et al., 2022; D’ambrosio et al., 2023; Yin et al., 2023).
Xylitol, a sugar alcohol with numerous applications, has also been successfully produced through microbial processes. For example, Candida tropicalis was used to produce 25.8-109.5 g/L of xylitol from sugarcane bagasse and rice straw (Junior et al., 2019; Hernández-pérez et al., 2020; Raj and Krishnan, 2020; Singh et al., 2021). Other microbial processes employing microbes such as Kluyveromyces marxianus, and Pichia fermentans have resulted in impressive xylitol production (24.2-86.6 g/L) from LCB sources such as corn cob, olive pits, and sugarcane bagasse (Du et al., 2020; Prabhu et al., 2020a; Narisetty et al., 2021b). Itaconic acid, another chemical of interest, has been produced using the fungus Aspergillus terreus. The process produced 22.43-41.54 g/L of itaconic acid from corn stover, bamboo residue, wheat straw, and wood pulp (Kerssemakers et al., 2020; Yang et al., 2020; Saha et al., 2022). In a study utilizing new isolates of A. japonica, 21.52 g/L of itaconic acid was produced from Citrullus lanatus. (Ramakrishnan et al., 2020). Last but not least, 2,3-butanediol has been produced at titers ranging from 10.30-75.03 g/L using various LCB, with microbial processes involving Klebsiella pneumoniae, K. oxytoca, Pantoea agglomerans, Enterobacter species, and Paenibacillus polymyxa (Cortivo et al., 2019; Hazeena et al., 2019; Pasaye-Anaya et al., 2019; Okonkwo et al., 2021; Rehman et al., 2021; Ma et al., 2022; Li et al., 2023b).
With pressing environmental concerns and the gradual depletion of non-renewable resources, the transition from a chemical-based economy to a bio-based economy has gained much momentum (Amoah et al., 2019; Banu et al., 2021). However, the global production of biobased chemicals and polymers is estimated only to be around 90 million tons, which is approximately 3.5 times lower than the production of petrochemical-based chemicals (Jong et al., 2020). Several key challenges for this disparity include LCB selection and its consistent supply, efficient LCB pretreatment methods, fermentation process, and downstream processing (Liu et al., 2021; Singh et al., 2022). Regarding to fermentation process, it is crucial to develop a robust microbial cell factory that is capable of converting LCB without requiring extensive pre-processing. Additionally, it should be able to efficiently utilize all the resulting monomers, withstand the presence of inhibitors, and produce the maximum amount of the desired target product, whether it be high yields, high titers, or high productivity. Metabolic engineering plays a pivotal role in the development of these robust microbial cell factories optimized for LCB-based biorefinery.
The present review focuses on metabolic engineering strategies employed in the advancement of robust microbial cell factories for LCB biorefinery, emphasizing hemicellulose utilization as a substrate. The study highlights nine chemicals of significant commercial value and the extent of research conducted on them in recent years. These chemicals include succinic acid, lactic acid, 3-hydroxypropionic acid, xylitol, biohydrocarbons, itaconic acid, 2,3-butanediol, 1,2,4-butanetriol, and 2-phenylethanol. Additionally, the review briefly discusses the bioeconomy, addressing the existing challenges and limitations associated with the bioconversion of LCB through metabolic engineering.
Succinic acid, also known as C4-dicarboxylic acid, is one of the top chemicals from biorefinery mentioned in the DOE reports of 2004 and 2010 (Bozell and Petersen, 2010; Jong et al., 2020). It serves as a platform chemical used for the synthesis of several other chemicals such as 1, 4-butanediol, γ-butyrolactone, maleic acid, tetrahydrofuran, N-methyl pyrrolidone, 2-pyrrolidone, and many others. Moreover, it has a wide range of applications in food, medicine, surfactants, detergents, and biodegradable polymer industries. The global production capacity of bio-succinic acid is estimated to be approximately 76-87 kilotons per year (Dickson et al., 2021; Li et al., 2021b).
Several microorganisms such as A. succinogenes, B. succiniciproducens, and Mannheimia succiniciproducens are known to produce succinic acid naturally (Dai et al., 2020; Wang et al., 2022a). These microorganisms employ three distinct metabolic pathways to convert sugar into succinic acid (Figure 2). In the tricarboxylic acid (TCA) cycle, one mole of glucose yields one mole of succinic acid; whereas the glyoxylate pathway results in 1.71 moles of succinic acid per mole of glucose. In the reductive TCA cycle, one mole of glucose yields two moles of succinic acid (Dai et al., 2020). The metabolic engineering approaches used for the production of succinic acid from LCB are summarized in Table 2.
Figure 2 Metabolic pathways for the production of succinic acid, lactic acid, 3-hydroxypropinic acid, xylitol, biohydrocarbons, itaconic acid, 2-phenylethanol, 1,2,4-butanetriol and 2,3-butanediol.
Table 2 Metabolic engineering efforts for the production of chemicals listed in the DOE Top 10 using LCB as feedstocks.
The work conducted by Mao et al. (2018) investigated the production of succinic acid from corn stover. They used Corynebacterium glutamicum as a host strain and implemented several key modifications to the metabolic pathways. They deleted the genes associated with the byproduct formation pathway such as ldh, ackA-pta, pqo, and cat, and replaced the native promoter of the anaplerotic pathway and pentose phosphate pathway genes: pyc (pyruvate carboxylase), ppc (phosphoenolpyruvate carboxylase), talP (transaldolase), and tkt (transketolase) with strong sod promoter to enhance the assimilation of xylose. They also integrated araE from Bacillus subtilis (arabinose symporter) under tuf promoter to improve the influx of xylose. To further optimize succinic acid production and xylose assimilation, pyc, gltA (citrate synthase), sucE (succinate exporter), and Xanthomonas campestris derived xylA/xylB (xylose isomerase and xylulokinase) were overexpressed. In batch fermentation, the resultant engineered strain could produce 98.6 g/L of succinic acid from corn stalk hydrolysate. The obtained yield and productivity were 0.87 g/g total sugar and 4.29 g/L/h respectively, which were comparable to those obtained from a mixture of pure glucose and xylose (Mao et al., 2018). Li et al. (2023a) pursued an alternative approach using a quadruple mutant C. glutamicum (ΔldhΔackA-ptaΔcat) as the host strain. In this study, they overexpressed transcriptional regulator Ncgl0275 along with pyc and ppc to eliminate succinate feed-back inhibition and divert more carbon flux toward succinic acid respectively. Furthermore, two xylose assimilation pathways named the Weimberg pathway (xylBXAC) derived from Caulobacter crescentus and the xylose isomerase pathway (xylABE) derived from Escherichia coli were co-expressed to enhance the strain’s ability to utilize xylose. The final recombinant C. glutamicum could produce 64.16 g/L of succinic acid from corn stover hydrolysate in batch fermentation (Li et al., 2023a).
A study on succinic acid production from sugarcane bagasse was conducted by Ong et al. (2019). They adaptively evolved a single mutant Yarrowia lipolytica (Δsdh5) on glucose to restore glucose metabolism. The evolved Y. lipolytica could produce 33.2 g/L of succinic acid from sugarcane bagasse hydrolysate with a yield of 0.58 g/g total sugar and productivity of 0.33 g/L/h respectively (Ong et al., 2019). In the subsequent study, Prabhu et al. (2020b) overexpressed the xylose oxidoreductase pathway to enable xylose assimilation in the evolved Y. lipolytica. In batch fermentation, the recombinant strain (Δsdh5-XK-XDH-XR++) could produce only 5.60 g/L of succinic acid from sugarcane bagasse (Prabhu et al., 2020b). Similarly, Zhu et al. (2020) explored the possibility of producing succinic acid from woody hydrolysate using engineered E. coli. In this study, they used a previously engineered E. coli (ΔldhAΔadhEΔiclRΔackA-ptaΔptsG) as a host strain and introduced two copy of pycA and one copy of fdh (formate dehydrogenase) derived from Candida boidinii. The recombinant strain successfully produced 287.6 mM of succinic acid from woody hydrolysate. The low production of succinic acid by this strain was mainly attributed to reduced glucose influx caused by the deletion of the ptsG gene. To improve glucose uptake, an additional copy of galP (galactose permease) under the trc promoter was integrated at the galR locus. This modification significantly enhanced glucose consumption and subsequently increased succinic acid production. In batch fermentation, the recombinant strain was able to produce 54.5 g/L of succinate from 60 g/L of woody hydrolysate, with a productivity of 1.81 g/L/h (Zhu et al., 2020).
Huang et al. (2019a) adopted a different approach to produce succinic acid from sweet potato hydrolysates. They overexpressed the ecaA gene under anaerobically-induced nirB promoter in triple mutant Escherichia coli (ΔpflAΔldhAΔptsG). ecaA encodes carbonic anhydrase which catalyzes the interconversion of CO2 and HCO3−.The modified strain exhibited the ability to produce 16.73 g/L of succinic acid from sweet potato waste hydrolysates. Furthermore, when the anaplerotic pathway gene pepc was additionally overexpressed, the production of succinic acid increased to 18.65 g/L (Huang et al., 2019a).
Lactic acid, also known as 2-hydroxypropanoic acid, is one of the new top 10 chemicals listed in the DOE report of 2010 (Bozell and Petersen, 2010; Jong et al., 2020). This versatile compound finds applications across various industries, including food, cosmetics, leather, pharmaceuticals, and the chemical sector. Notably, lactic acid serves as a crucial monomer in the production of biodegradable polylactic acid (PLA) materials, making it a valuable component in the realm of sustainable and environmentally friendly materials. The global production capacity of bio-lactic acid is estimated to be >600 kilotons per year (Jong et al., 2020).
Various microorganisms, including lactic acid bacteria (Lactobacillus, Lactococcus, Enterococcus, and Pediococcus), Bacillus spps (B. coagulans, B. licheniformis), and Rhizopus spps (R. oryzae, R. arrhizus, and R microspores) possess the natural ability to produce lactic acid (Abedi and Hashemi, 2020). These microorganisms use homofermentative and/or heterofermentative routes to convert sugar into lactic acid (Figure 2). In the homofermentative route, the active Embden-Meyerhof-Parnas (EMP) pathway yields two moles of lactic acid from one mole of glucose, whereas if the pentose phosphate pathway is active, only 1.67 moles of lactic acid are produced. In the obligate heterofermentative route, both glucose and pentose produce one mole of lactic acid. Similarly, the facultative heterofermentative route yields two and one moles of lactic acid from one mole of glucose and pentose respectively (Cubas-cano et al., 2018). Table 2 summarizes the various metabolic engineering approaches used for the production of lactic acid from LCB.
Qiu et al. (2018) engineered a single mutant P. acidilactici (ΔldhD) by disrupting phosphoketolase and acetate pathway through deletion of the pkt and ackA2 genes respectively and overexpressing tkt and tal along with xylAB to divert more xylose flux towards the pentose phosphate pathway. To further enhance the assimilation of xylose, engineered P. acidilactici was adaptively evolved on xylose over a period of 66 days. In simultaneous saccharification and fermentation (SSF) with high solids load, the final adapted strain could produce 130.8 g/L of L-lactic acid with a productivity of 1.82 g/L/h from biodetoxified wheat straw hydrolysates (Qiu et al., 2018). Campos et al. (2023) explored the possibility of producing L-lactic acid from softwood hydrolysate using P. acidilactici (ΔldhD). In this study, they adaptively evolved the strain through slow feeding of softwood hydrolysate in the feed batch. With the feeding rate of softwood hydrolysate at 13 ml/h, the evolved strain could produce L-lactic acid with a yield of 0.86 g/g softwood hydrolysate (Campos et al., 2023). In another study, Kong et al. (2019) used engineered Kluyveromyces marxianus for the production of L-lactic acid from corn cob residue. They used previously developed K. marxianus strain as the host strain, and additionally disrupted dld1 (D-lactate dehydrogenase) and co-expressed B. megaterium and Plasmodium falciparum derived ldh genes (L-lactate dehydrogenase) to enhance L-lactic acid production. Furthermore, they introduced an extra copy of native pfk (phosphofructokinase) and S. cerevisiae-derived jen1 genes (monocarboxylate symporter) to redirect carbon flux toward the EMP pathway and facilitate the export of L-lactic acid. The resultant engineered strain could produce 45.43 g/L of L-lactic acid from 120 g/L of corn cob hydrolysate. Moreover, in fed batch-SSF, the strain produced approximately 101.1 and 125.93 g/L of L-lactic acid with a yield of 0.56 and 0.63 g/g using corncob hydrolysates and Jerusalem artichoke tuber powder respectively. Through supplementation with corn steep liquor and defatted soybean meal, the production of L-lactic acid reached 103 g/L L-lactic acid (Kong et al., 2019). Similarly, in the study conducted by Žunar et al. (2020) two L gasseri were developed by deleting either ldhl1 or ldhD to produce optically pure L- or D-lactic acid respectively. The mutant L. gasseri (ΔldhD) was particularly noteworthy, as it successfully produced optically pure L-lactic acid at a concentration of 21.07 g/L from wheat straw hydrolysate.
Qiu et al. (2020a) explored the impact of converting a toxic compound, vanillin, into a non-toxic form on the production of D-lactic acid from corn stover. They utilized a previously engineered strain of P. acidilactici (ΔldhΔpkt-xylAB++tkt-tal++) as a host strain and individually overexpressed various oxidoreductases that act on vanillin. The recombinant strain that overexpressed C. glutamicum cgs9114_RS09725, encoding a short-chain dehydrogenase, demonstrated better performance. It produced 115 g/L of D-lactic acid with a yield of 61.1% from biodetoxified corn stover, while the host strain achieved production of only 101 g/L of D-lactic acid with a yield of 51.6% (Qiu et al., 2020a). In the subsequent study, they adaptively evolved the strain on undetoxified corn cob hydrolysates over a period of 111 days. Eventually, the adapted strain showed a 126.8% increase in D-lactic acid production, yielding 31.30 g/L of D-lactic using undetoxified corn cob hydrolysate. Moreover, in one-pot SSF, the adapted strain could produce 61.9 g/L of D-lactic acid with a yield of 0.48 g/g from undetoxified corn cob hydrolysates (Qiu et al., 2022). The potential of the adapted strain to produce D-lactic acid from sugarcane bagasse was also evaluated. Through optimization of fermentation strategies in one spot SSF, 57.6 g/L of D-lactic acid was produced from undetoxified sugarcane bagasse hydrolysates, with a yield of 0.58 g/g bagasse (Qiu et al., 2023b). Similarly, He et al. (2023) adaptively evolved the engineered P. acidilactici (ΔldhΔpkt-xylAB++tkt-tal++) on a mixture of glucose, xylose, arabinose, mannose, and galactose for a period of 65 days. The final adapted strain showed significant improvement in the simultaneous co-utilization of sugars. In SSF, the strain could finally produce 128 g/L of D-lactic acid with a yield of 0.69 g/g using detoxified wheat straw hydrolysates (He et al., 2023).
3-hydroxypropionic acid (3-HP), also referred to as C3-carboxylic acid, is a notable chemical compound that is mentioned in both the DOE reports of 2004 and 2010 (Bozell and Petersen, 2010; Jong et al., 2020). This versatile compound serves as a substrate for the synthesis of various specific chemicals such as acrylic acid, malonic acid, acrylamide, acrylonitrile, and poly-3-hydroxypropionate. It finds extensive applications in diverse industries such as paints, cosmetics, diapers, adhesives, and polymers (Kumar et al., 2013). The global production capacity of bio-3-HP is not explicitly mentioned in the literature (Jong et al., 2020).
Various microbial biosynthesis pathways for 3-HP have been reported depending on the carbon source (De Fouchécour et al., 2018; Zhao and Tian, 2021). When glucose serves as the substrate, the malonyl-CoA and alanine pathways are involved in the synthesis of 3-HP. These pathways yield two and one mole of 3-HP from one mole of glucose respectively (Figure 2). When glycerol is the substrate, coenzyme-A dependent and independent glycerol oxidation pathways are active and produce one mole of 3-HP from one mole of glycerol. Similarly, one mole of 3-HP is biosynthesized through the oxidation of one mole of 1,3-PDO, and hydrolysis of one mole of 3-hydroxypropionitrile, respectively (De Fouchécour et al., 2018).
The biological production of 3-HP has predominantly been explored using glycerol or glucose as the primary feedstocks. However, there are limited reports on the use of LCB as a feedstock for 3-HP production (Table 2). In a study conducted by Dai et al. (2023) the production of 3-HP from corn stover biomass was investigated. They constructed a recombinant Aspergillus niger by introducing 12 copies of the β−alanine pathway (constructed by including Tribolium castaneum panD, B. cereus bapat, and E. coli hpdh under the control of constitutive gpdA promoter). In addition, they deleted ald6a (encoding malonate semialdehyde dehydrogenase) and introduced 8 copies of pyc2 to reduce 3-HP consumption and improve the 3-HP precursor supply. The final engineered strain could produce 29.4 g/L of 3-HP with a yield of 0.35 mole/mole sugars from corn stover hydrolysate. When an additional 15 copies of the β−alanine pathway were introduced under the promoter of ubi4 and mbfA along with optimization of the fermentation condition, the 3-HP production improved to 36 g/L with a yield of 0.48 mole/mole sugars (Dai et al., 2023). In another study, Liu et al. (2023) focused on the utilization of the malonyl-CoA pathway for the production of 3-HP from corn stover hydrolysate. They developed a recombinant Rhodosporidium toruloides by overexpressing the malonyl-CoA pathway (comprising mcrN and mcrN derived from Chloroflexus aurantiacus). With media optimization in flask-scale experiments, the recombinant strain could produce 10.7 g/L 3-HP from corn stover hydrolysate. When RTO4_8975 was deleted and A. pseudoterreus derived g2945 (3-HP transporter) was overexpressed, the production of 3-HP significantly improved to 18.7 g/L. Further introduction of an additional copy of mcrNC in this engineered strain (ΔRTO4_8975-mcrNC++), 3-HP production reached 19.2 g/L. In fed-batch fermentation, the final strain achieved the production of 45.4 g/L 3-HP (Liu et al., 2023). Liu et al. (2018) also explored the potential of engineered E. coli harboring the malonyl-CoA pathway for the production of 3-HP from corn stover hydrolysate. They deleted the ptsHI gene and mutated the promoter and ribosomal binding site of the glk-galP gene and transcriptional activator soxR (S26G, E32V) for simultaneous co-utilization of mixed sugars and inhibitor tolerance. In addition, they overexpressed the malonyl Co-A pathway constructed by including C. aurantiacus mcr and E. coli ydfG. The final recombinant strain could produce 8 g/L of 3-HP from corn stover hydrolysate containing 1 g/L of furfural (Liu et al., 2018).
Xylitol has also gained recognition as one of the top 10 biorefinery chemicals in the DEA reports of 2004 and 2010 (Bozell and Petersen, 2010; Jong et al., 2020). It is extensively used as a sweetener in the food, odonatological, and pharmaceutical industries, offering the advantage of being low in calories and having a low glycemic index when compared to sucrose. Moreover, xylitol serves as a valuable monomer for synthesizing polymers and various other chemicals such as xylaric acid, xylonic acid, and 1,4-anhydroxylitol (Ahuja et al., 2020). The global production capacity of bio-xylitol acid is reported to be 190 kilotons per year (Jong et al., 2020). Biologically, xylitol is synthesized from xylose through the xylose oxidoreductase pathway, yielding one mole of xylitol from one mole of xylose (Figure 2) (Xu et al., 2019).
The metabolic engineering approaches for xylitol production from LCB are summarized in Table 2. The conversion of xylose to xylitol requires NADPH as a cofactor, and the primary pathway for generating NADPH is the pentose phosphate pathway. In a study by Yuan et al. (2019), they deleted pfkAB (phosphofructokinase) in previously engineered E. coli (ΔptsG△xylAB△ptsF-5 copies of Neurospora crassa mutated xr) to improve carbon flux toward the pentose phosphate pathway. The final engineered E. coli could produce 131.6 g/L of xylitol from hemicellulosic hydrolysate in fed-batch fermentation (Yuan et al., 2019). In another study, they overexpressed two genes of the pentose phosphate pathway, zwf (glucose-6-phoshphate dehydrogenase) and gnd (phosphogluconate dehydrogenase), along with deletion of the pgi gene (glucose-6-phosphate isomerase) in E. coli (ΔptsG△xylAB△ptsF-4 copies of N. crassa xyl1). In fed-batch fermentation, the final recombinant produced 162 g/L of xylitol from corncob hydrolysates, which was 13% higher compared to the host strain (Yuan et al., 2021). In a subsequent study, they mutated the CRP gene in the above E. coli strain to improve the inhibitory tolerance. The newly engineered E. coli mutant (I112L, T127G, A144T) could produce 82 g/L xylitol from un-detoxified condensed corncob hydrolysate via fed-batch fermentation, whereas host-engineered E. coli only produced 30 g/L xylitol (Yuan et al., 2023). Similarly, another mutant (I112L, T127I, and A144T) produced 164 g/L xylitol from detoxified corncob hydrolysate (Yuan et al., 2020). Hua et al. (2019) also studied the production of xylitol from corncob hydrolysate. They used previously engineered K. marxianus (Δhxk1-glk1++) as a host strain to eliminate glucose repression and overexpressed 6 copies of N. crassa xyl1 (xylose reductase) and 3 copies of S. cerevisiae gal2-N376F (xylose transporter). The recombinant strain could produce 69.94 and 118.63 g/L of xylitol from un-detoxified and detoxified corncob hydrolysates respectively (Hua et al., 2019).
Xylose reductase is a key enzyme of the xylitol biosynthesis pathway. Enhancing the efficiency of this enzyme is crucial for improving xylitol production. In a study by Zhang et al. (2022), two xylose reductases derived from C. tropicalis and N. crassa were co-overexpressed in a previously engineered strain of K. marxianus (Δxyl2, 4 copies of glucose/xylose facilitator from C. intermedia, and xyl1 from N. crassa). In fed-batch fermentation supplemented with glycerol, the resulting strain exhibited the ability to produce 105.22 g/L of xylitol with a high productivity rate of 1.25 g/L/h from corncob hydrolysate (Zhang et al., 2022). Similarly, Baptista et al., 2018; Baptista et al., 2020 overexpressed native xylose reductase (gre3) in S. cerevisiae. The recombinant could produce 14.13-29.61 g/L of xylitol from corncob hydrolysate depending upon the fermentation process (Baptista et al., 2018). Further, through optimization of SSF, the resulting recombinant achieved xylitol production of 71.7 g/L from corncob hydrolysate (Baptista et al., 2020). In another study conducted by He et al. (2021), they overexpressed Scheffersomyces stipitis xyl1 in engineered S. cerevisiae (Δxks1, overexpression of native zwf, rki1, tal1, tkl1, and rpe1, and the Meyerozyma guilliermondii-derived mutant xylose transporter mgt05196N360F). The final recombinant strain was able to produce 91 g/L of xylitol in fed batch-SFF fermentation using waste xylose mother liquor and corncob residue together.
In a recent study by Meng et al., 2022, a triple mutant A. niger (ΔladAΔxdhAΔsdhA) was employed for the production of xylitol from different LCBs. The strain could produce 1.49-2.20 mM xylitol from 1% each wheat bran, beechwood xylan, and cotton seed hulls respectively in a shake flask. They additionally deleted xkiA (xylulose kinase) to prevent the diversion of xylulose to the PP pathway. However, the deletion was not beneficial (Meng et al., 2022).
Biohydrocarbons, including isoprene and β-farnesene, have gained prominence as valuable products from biorefineries (Bozell and Petersen, 2010; Jong et al., 2020). However, the global production capacity of biohydrocarbons is not reported (Jong et al., 2020) Isoprene, a C-5 hydrocarbon, is primarily used in the polymer industry. It serves as a key ingredient in the synthesis of various polymer products, including polyisoprene rubber, styrenic thermoplastic elastomer block copolymers, and butyl rubber (Czinkóczky and Németh, 2023). On the other hand, β-farnesene is a C15-alkene, a simple acyclic sesquiterpenoid with diverse applications in the chemical and agriculture industries. It serves as a pesticide and a platform chemical for the production of jet biofuel, vitamin E, cosmetic oil, surfactants, and lubricants (Leavell et al., 2016). Both of these hydrocarbons are biosynthesized from isopentyl phosphate (IPP) and dimethylallyl pyrophosphate, which are generated from either the mevalonate (MVA) or 2-C-methyl-D-erythritol 4-phosphate (MEP) pathways. Isoprene is produced in a single step from IPP, while the synthesis of β-farnesene involves multiple steps (Figure 2) (Czinkóczky and Németh, 2023). One mol of glucose yielded 0.80 and 0.67 mol of isoprene from the MEP and MVA pathways respectively. Similarly, 0.28 mol β-farnesene is biosynthesized from one mol of glucose.
The production of biohydrocarbons from LCB is a relatively underexplored field, as indicated in Table 2. Wang et al. (2017) created the recombinant E. coli overexpressing MVA pathway (containing mvaE and mvaSMT from Enterococcus faecalis) and ipsS (isoprene synthase, from Papulus alba). The strain could produce 249-298 mg/L of isoprene from peanut hull hydrolysate in separate hydrolysis and fermentation, and SSF respectively (Wang et al., 2017). In another study, recombinant E. coli overexpressing the MEP pathway and ipsS was created and employed for the production of isoprene from paper mill sludge hydrolysate. The recombinant strain produced a very low amount of isoprene, 190-470 nmol (Duncan et al., 2020).
The production of β-farnesene from LCB was studied by Bi et al. (2022). They engineered Y. lipolytica by integrating MVA pathway genes (ERG10, tHMGR, ERG12, ERG13, IDI, ERG20, ERG8, and ERG19 under constitutive promoters) into chromosomes. Additionally, extra copies of key enzymes of the MVA pathway (EGR8, EGR19, tHMGR, Silicibacter pomeroyi nadh-HMGR) were overexpressed to further increase flux toward the MVA pathway. By overexpressing aaBFS (encoding β-farnesene synthase) derived from Artemisia annua, the recombinant strain could produce 374-379 mg/L of β-farnesene from lignocellulosic hydrolysate. In fed-batch fermentation with supplementation of Mg2+, the production of β-farnesene reached 7.38 g/L (Bi et al., 2022). You et al. (2019) also employed a similar approach to develop an E. coli strain capable of producing β-farnesene. They overexpressed native atoB; S. cerevisiae derived HMGS, tHMGR, E12, E8, and MVD1; E. coli derived IDI and ispA; and A. annua derived aaBFS. The resultant strain could produce around 1.25 g/L of β-farnesene from corncob hydrolysate. Notably, they found that citrate, generated during the pretreatment of corncob hydrolysate, affected β-farnesene production, while furaldehyde and its derivatives had no impact. When ATP citrate lyase was overexpressed to divert citrate to acetyl-CoA, the β-farnesene production increased to approximately 2.50 g/L. Further, upon switching the host strain, the new recombinant was able to produce 4.06 g/L of β-farnesene in fed-batch fermentation using corncob hydrolysate (You et al., 2019).
Itaconic acid (IA), also known as 2-methylidenebutanedioic acid, was identified as one of the top seven platform chemicals according to the DOE report of 2004. However, this acid was not mentioned in the 2010 report. The applications of itaconic acid span across various industries, including biopolymer production, medical applications, and agriculture. It is biosynthesized through the decarboxylation of cis-aconitate in the TCA cycle (Figure 2) The global production capacity of itaconic acid was reported to be 90 kilotons per year (Jong et al., 2020).
The metabolic engineering approaches used for itaconic acid production from LCB are summarized in Table 3. Yang et al. (2020), generated mutant A. terreus by UV-LiCl mutagenesis and employed it for the production of IA using bamboo residue hydrolysate. The obtained mutant strain achieved a production of 19.35 g/L of IA from bamboo hydrolysates with the addition of corn steep liquor. After optimizing the fermentation process, this mutant strain was able to produce 22.43 g/L of IA in SSF and 41.54 g/L in fed-batch fermentation (Yang et al., 2020). However, it is worth noting that IA concentrations above 20 g/L can be toxic to the producing microorganism and may hinder IA production. In response to this, Wu et al. (2017) developed a mutant strain of A. terreus that was resistant to IA toxicity and could produce 49.65 g/L of IA from wheat bran hydrolysates (Wu et al., 2017). Hanh et al. (2023) studied the production of IA from kraft pulp and developed a recombinant strain of C. glutamicum. This strain overexpressed A. niger cadA (coding for aconitate decarboxylase, a key enzyme in IA production) fused with E. coli malE (maltose binding protein) along with disruption of the lactate pathway. Aconitate decarboxylase is known to have low stability, so the fusion with maltose binding protein aimed to enhance protein stability. The resulting strain was able to produce 6.15 g/L of IA from eucalyptus kraft pulp hydrolysate (Hanh et al., 2023). In another study, Zhao et al. (2018) overexpressed A. niger cadA in N. crassa, and produced 10.4 mg/mL of IA from direct corn stalk and 8.6 mg/mL of IA from direct switch grass.
Table 3 Metabolic engineering efforts for the production of other chemicals using LCB as feedstocks.
1,2,4-Butanetriol (BT), C4 polyol, is a valuable fine chemical with a broad range of applications in the polymer, pharmaceutical, and explosive industries. It is generally used in the synthesis of polyurethane foams and in anti-cancer and anti-viral medications. Additionally, BT is also utilized as an energetic plasticizer (Cao et al., 2015). The synthetic biosynthesis pathway of BT starts from xylose (Figure 2). One mol of xylose yields one mol of BT.
Table 3 summarizes the metabolic engineering approaches used for BT production from LCB. In a study performed by Wang et al. (2018), they identify the efficient enzymes responsible for the conversion of xylonate into bio-based BT. Enzymes from various sources, including E., coli, S. cerevisiae, Caulobacter crescentus, L. lactis, P. putida, Haloferax volcannii, and Pseudomonas sp were screened. A synthetic BT pathway was constructed (containing C. crescentus xylB-xylD, L. lactis kdcA, and E. coli adhP) and overexpressed in E. coli. The recombinant strain could produce 3.4 g/L of BT from corncob hydrolysates through whole-cell bioconversion (Wang et al., 2018). Similarly, Bamba et al. (2019) developed BT-producing S. cerevisiae by integrating another synthetic BT pathway (containing C. crescentus xylB-xylD and L. lactis kdcA) along with deletion of the gre3 gene. Additionally, they deleted bol2 (a negative regulator of iron uptake) and overexpressed ttyw1 (Fe sequester) to improve the xylD enzyme activity and protect cells from Fe toxicity. Xylonate dehydrogenase (xylD) is an iron-containing enzyme and its activity is dependent upon Fe uptake. In the SSF, the final strain could produce 1.1 g/L of BT with a molar yield of 20.5% from diluted rice straw hydrolysate, whereas from undiluted hydrolysate, 0.62 g/L of BT was produced with a molar yield of 5.5% (Bamba et al., 2019). In another study, the production of 4.83 g/L of BT was achieved using ethanol-distilled stillage as feedstock and BT-producing strain Gluconobacter oxydans (overexpressing E. coli yagf, P. putida mdlc, and native gox0313) (Mao et al., 2023). Zhao et al. (2019) developed an interesting two-stage, integrated bioprocess for BT production. In the first stage, glucose and toxic intermediate present sugarcane bagasse hydrolysate were converted to ethanol and non-toxic intermediate by mutant C. glycerinogenes (Δgpd). In the second stage, the obtained ethanol-distilled stillage containing xylose was used as feedstock for BT production. They engineered E. coli (ΔyagEΔydhEΔyiaEΔycdW-mdlCxdh++) by deleting the diversion pathway of xylonate towards pyruvate and pentanoic acid and pushing carbon flux toward BT. The resultant strain could produce 1.3 g/L of BT from ethanol-distilled stillage. Eventually, with additional deletion of crr and optimization of fermentation condition, BT production reached 4.9 g/L of BT with a molar conversion yield of 0.30 mol/mol xylose. This study demonstrates an interesting bioprocess for the co-production of value added products using LCB hydrolysates (Zhao et al., 2019).
2-Phenylethanol (2-PE), also known as 2-phenethyl alcohol, is an alcohol that possesses a delightful fragrance reminiscent of roses. This aromatic compound has wide applications in the food and cosmetic industries, where it serves as a flavoring and fragrance agent. Moreover, it acts as a crucial building block for the synthesis of 2-Phenylethyl acetate, which exhibits a delightful flavor akin to honey and roses. The biosynthesis of 2-PE occurs through the Shikimate and Ehrlich pathways, utilizing either glucose or phenylalanine as a substrate (Figure 2) (Martínez-Avila et al., 2018).
The metabolic engineering approaches used for 2-PE production from LCB are summarized in Table 3. Zhu et al. (2023) studied the production of 2-PE from corncob hydrolysate using C. glutamicum. They initially adaptively evolved C. glutamicum on 2-PE and introduced the Ehrlich pathway by overexpressing S. cerevisiae aro10 and E. coli yjgB. Additional modifications included the overexpression of aroG(S180F), pheA(T326), aroA, ppsA, and tkt genes to enhance carbon flux toward the phenylpyruvate pathway. Finally, they overexpressed Xanthomonas campestris xylAB along with E. coli xylE (xylose transporter) to install the xylose isomerase pathway. The resultant recombinant could produce 3.28 g/L of 2-PE from corn stalk hydrolysate (Zhu et al., 2023). Xu et al. (2023) developed the E. coli mutant to produce 2-PE from sugarcane molasses. They used previously engineered E. coli for 2-PE production from glucose as a host strain and made several modifications to the sucrose catabolism pathway. They replaced the PTS-dependent sucrose transporter with a non-PTS transporter (E. coli cscB) and integrated sucrose phosphorylase (sucP) from Bifidobacterium adolescentis to supply more PEP toward the phenylpyruvate pathway. Additionally, they overexpressed kivD and pheA(T326). The recombinant strain produced 2.21 g/L and 3.59 g/L of 2-PE from untreated and carbon and diatomite pretreated molasses, respectively, in flask-scale experiments. Through fed-batch fermentation and media optimization, they achieved a production of 6.43 g/L of 2-PE with a productivity of 0.11 g/L/h (Xu et al., 2023). Hillman et al. (2021) used the two-stage, integrated bioprocess for 2-PE production from LCB hydrolysate. In the initial stage, different lignocellulosic feedstocks such as corn stover, poplar, and alfalfa were saccharified by Piromyces indianae, eliminating the need for commercial hydrolytic enzymes. In the subsequent stage, 2-PE was produced using P. indianae-fermented hydrolysates as feedstock. They engineered K. marxianus to serve as a 2-PE producer by overexpressing key genes of the Shikimate and Ehrlich pathways [aro4(K221L), aro7(G141S), pha2, and aro10] and deleting diverting pathways (abz1, aro8 and eat1). The final resultant K. marxianus could produce approximately 400 mg/L of 2-PE from P. indianae hydrolysate (corn stover or poplar or alfalfa) (Hillman et al., 2021).
2, 3-butanediol (BDO), is a type of C4-diols, that has extensive applications in the production of synthetic rubber, resin, paints, aviation fuel, and lotions. The biosynthesis of BDO occurs through the mixed acid-BDO pathway, starting from glucose as the primary carbon source (Figure 2) (Hakkim et al., 2020). In this pathway, one mole of glucose yields one mole of BDO.
The metabolic engineering approaches used for BDO production from LCB are summarized in Table 3. Kim et al. (2020) engineered E. aerogenes for the production of BDO from sugarcane bagasse. They used triple mutant E. aerogenes (ΔldhAΔpflBΔptsG) as a host strain and overexpressed the native BDO pathway (budABC operon) and galP with modified ribosomal binding to improve glucose uptake and BDO production. The malic isoenzyme maeA was also co-expressed to reduce PEP accumulation. The resultant recombinant achieved the BDO production of 128.4 g/L with a yield of 0.49 g/g from sugarcane bagasse hydrolysate in flask scale. In fed-batch fermentation, BDO production ranged between 110-114.3 g/L depending on aeration conditions, a significant improvement compared to the triple mutant strain which produced only 66-66.4 g/L of BDO (Kim et al., 2020). In another study, Erian et al. (2018) investigated the potential of another BDO pathway derived from E. cloacae subsp. dissolvens (budABC) using sugarcane molasses. They overexpressed budABC derived from E. cloacae subsp. dissolvens in E. coli W, and achieved a BDO production of 56.30 g/L in pulse-fed batch fermentation. Similarly, Lee and Seo (2019) engineered S. cerevisiae for the production of BDO from cassava hydrolysate. They first deleted pyruvate decarboxylase (pdc1, pdc5, and pdc6) and alcohol dehydrogenase (adh1) to block ethanol production. The BDO pathway (containing B. subtilis alsS and alsD, and native bdh1) was then overexpressed, along with L. lactis noxE for redox balance. The recombinant strain could produce 81.2 g of BDO in batch fermentation, and 132 g/L in fed-batch fermentation using cassava hydrolysates (Lee and Seo, 2019). In a subsequent study, they disrupted the glycerol production pathway by deleting gpd1 and gpd2, and overexpressed C. tropicalis pdc1 to achieve glycerol-free BDO. The resultant recombinant produced 47.4 g/L of BDO with a negligible amount of glycerol from cassava hydrolysate (Kim et al., 2019). Saratale et al. (2018) also reported a production of 10.42 g/L of BDO from kenaf core powder hydrolysate using engineered K. pneumoniae (ΔwabGΔldhAΔpflB).
In a study conducted by Wu et al. (2019), inhibitor-tolerant E. cloacae was generated using the ARTP mutation technique and used for the production of BDO from LCB hydrolysate. One mutant with increased inhibitor tolerance could produce 23.2 g/L of BDO with a yield of 0.44 g/g sugar from corncob hydrolysate (Wu et al., 2019). Amraoui et al. (2022) adopted random mutagenesis using ethyl methane sulfonate (EMS) to mutate E. ludwigii. Through optimized fed-batch fermentation, the mutant strain exhibited impressive BDO production levels of 118.5 g/L from brewers’ spent grain hydrolysate with a sugar-to-BDO conversion efficiency of 0.43 g/g. The mutant strain also produced 68.2 g/L of BDO from detoxified sugarcane bagasse hydrolysate (Narisetty et al., 2021a; Amraoui et al., 2022). For enhanced BDO production from xylose, Cha et al. (2020) conducted adaptive evolution by integrating E. coli xylE into the mgsA loci of engineered K. oxytoca. The adapted strain achieved BDO production levels of nearly 6.11 g/L and 5.82 g/L from sunflower and pine tree hydrolysates, respectively (Cha et al., 2020).
The presence of a wide range of toxic compounds in lignocellulosic hydrolysates is a significant challenge for microbial-based production of value-added chemicals. These toxic compounds can negatively impact microbial growth, metabolism, and product formation. Pretreatment of LCB generates more than 60 different types of toxic chemicals depending upon the types of LCB and the pretreatment process itself. The most commonly generated inhibitors are aldehydes (furfural, methylglyoxal, vanillin), ketones (furanone, pentenone, acetone), phenolic (cresol, guaiacol), acid (acetic acid, formic acid, levulinic acid), and alcohol (methanol, propanol). The mechanism of toxicity widely varies depending upon the types of inhibitors. For example, acetic toxicity is associated with membrane damage, disruption of the proton gradient, and accumulation of anions. Aldehyde and ketone toxicity is related to protein damage. Similarly, phenolic compound and alcohol toxicity is linked to membrane stress and integrity (Jayakody et al., 2022).
The different metabolic engineering approaches used to improve microbial tolerance towards inhibitors derived from LCB are summarized in Table 4. One of the strategies for enhanced inhibitor tolerance is engineering the transcriptional or regulation factor. Luo et al. (2018) mutated the global regulator irre (A43T, D95G, and P261L) derived from Deinococcus radiodurans and overexpressed in S. cerevisiae. The resulting yeast strain exhibited improved growth rates and ethanol productivity in the presence of inhibitors such as furfural, 5-hydroxymethylfurfural, formic acid, and acetic acid, either individually or in combination (Luo et al., 2018). In another study, the two native transcriptional regulators, haa1p and tyeP, were overexpressed in evolution-engineered S. cerevisiae (harboring S. stipitis xyl1 and xyl2, and A. aculeatus bgl1). The final strain could produce 47.50 g/L ethanol with a yield of 0.44 g/g from corn stover hydrolysate, 6.37% higher compared to the host strain (Li et al., 2021a). Similarly, Nouri et al., 2020 overexpressed hfq (transcriptional regulator) and sigE (transcription factor) in Zymomonas mobilis. The overexpression of these regulatory factors improved the strain’s ability to convert sugars to ethanol under inhibitory conditions. The recombinant Z. mobilis produced 1.5-3.6 g/L ethanol with a yield of 44-72% from sugarcane bagasse hydrolysate, while the wild type produced a negligible amount of ethanol (Nouri et al., 2020). Qu et al. (2021) deleted argR1864, the negative regulator of the stress response system (DnaK-DnaJ-GrpE system and GroEL-GroES chaperonin) in Thermoanaerobacterium aotearoense, and the ethanol production from soybean straw, sorghum and wheat hydrolysate was improved by 113.89-366.36% (Qu et al., 2021). An improved tolerance toward furfural and acetic acid in Saitozyma podzolica by deletion of transcriptional regulator creE was also reported by Ran et al. (2023). In another study by Alves et al. (2021), the deletion of arabinose transcriptional regulator araE along with mutation in sigI was reported to be responsible for enhanced tolerance toward acetic acid and 5-hydroxymethyl-furfural in evolved strain C. saccharoperbutylacetonicum (Alves et al., 2021). These studies highlight the significance of genetic engineering and the modulation of transcriptional regulators or factors in improving microbial tolerance to inhibitors, ultimately contributing to the development of more robust and efficient microbial cell factories for lignocellulose biorefinery applications.
Another effective approach for inhibitor tolerance is to engineer microorganisms to metabolize these toxic compounds into non-toxic intermediates. Qiu et al. (2020b), designed a P. acidilactici strain capable of metabolizing aldehyde inhibitors. They used previously developed P. acidilactici (for D-lactic production from LCB hydrolysate) as a host strain and integrated Z. mobilis ZMO1116 gene encoding oxidoreductase, which converts p-benzoquinone to less toxic hydroquinone. This genetic modification allowed the recombinant strain to produce significantly higher levels of D-lactic acid from corn stover hydrolysate containing p-benzoquinone, compared to the host strain. The recombinant could produce 123.8 g/L of D-lactic acid from corn stover containing p-benzoquinone (300 mg/L), while the host strain produced 21.4% lesser D-lactic acid (102 g/L) (Qiu et al., 2020b). Suo et al. (2019) also overexpressed aldo/keto reductase from Clostridium beijerinckii in C. tyrobutyricum. This reductase has activity against furan aldehydes and phenolic compounds, common inhibitors in LCB hydrolysates. The resultant recombinant could produce 18.30 g/L butyric acid from corncob acid hydrolysate in flask scale. Additional co-expression of grosESL further increased the productivity to 20.5 g/L. In batch fermentation, the recombinant could produce 28.1% higher butyric acid (32.8 g/L) with 52.6% better productivity as compared to the wild type (Suo et al., 2019). A study on the identification of vanillin detoxifying enzyme was conducted by Yan et al. (2021). They adaptively evolved Z. mobilis on corn stover hydrolysate and identified zmo3_rs07160 encoding short-chain oxidoreductase as a responsible gene for vanillin tolerance. With the homologous overexpression of zmo3_rs07160, the recombinant strain produced 23.6% higher ethanol in separate hydrolysis and fermentation, and 21.8% higher ethanol in simultaneous saccharification and co-fermentation using corn stover hydrolysate (Yan et al., 2021). In another study, Lam et al. (2021) tested different reductases from S. cerevisiae and S. stipitis showing activity toward furfural and hydroxymethylfurfural. They identified S. cerevisiae gre2 as the most efficient one and evolved it through directed evolution for improved detoxifying activity. Overexpression of the evolved gre2, in combination with other modifications such as elevated K+ and a pH buffering system, significantly enhanced the production of ethanol and D-lactic acid from various lignocellulosic feedstocks. The recombinant S. cerevisiae (ethanol-handicapped strain expressing L. mesenteroides lactate dehydrogenase) could produce nearly 50 g/L of D-lactic acid from wheat straw, 1ow-acid corn stover, and approximately 50 g/L DMR corn stover, while the host strain only produced less than 20 g/L of D-lactic acid. Similarly, recombinant S. cerevisiae (engineered for xylose consumption) produced approximately 76 g/L of D-lactic acid from miscanthus, 70 g/L from high-acid corn stover, and approximately 60 g/L from sugarcane bagasse, while the host strain produced only less than 15 g/L (Lam et al., 2021). Chen et al. (2016) also previously reported production of as high as 86 g/L ethanol (> 10 volume%) from DMR corn stover (without purification or concentration) using recombinant Z. mobilis (Chen et al., 2016). In a subsequent study, the recombinant produced around 60 g/L ethanol from recycled DMR hydrolysate liquors (Chen et al., 2018). In addition to this, several other studies have been conducted to identify and use oxidoreductase for tolerance improvement but were not evaluated using LCB hydrolysate (Kim et al., 2022b; Ramzi et al., 2022; Willson et al., 2022; Chang et al., 2023; Wang et al., 2023).
Zou et al., 2021 generated inhibitor-tolerant P. putida by disrupting the glucose metabolism (phosphorylation and oxidation route) by deleting gtsABCD (ATP-dependent sugar transporter) and gcd (glucose dehydrogenase). The resulting engineered P. putida strain could utilize various LCB-derived inhibitors (furan aldehyde, acetic acid, levulinic acid, vanillin, p-coumarate, vanillate, ferulate, and 4-hdyroxybenzoate) as the sole carbon source. In two-stage microbial co-culture fermentation, B. coagulans produced 35.8 g/L lactic acid from P. putida (ΔgtsABCDΔgcd) detoxified LCB hydrolysate while there was no production with undetoxified LCB hydrolysate (Zou et al., 2021). In a subsequent study, they adaptively evolved P. putida (ΔgtsABCDΔgcd) on furfural to further enhance its tolerance to this specific inhibitor. They identified four key mutations in genes related to an ABC transporter and a hypothetical protein that were responsible for improved furfural tolerance [in pp_rs19785 (A7D) and pp_rs18130 (I22K, R23) encoding ABC transporter; pp_rs20740 (V338F) encoding a hypothetical protein]. These mutations were then overexpressed in the engineered P. putida strain. The resulting recombinant strain exhibited significantly improved cell growth and fermentability in real pretreated lignocellulosic biomass hydrolysates, thus making it a more robust candidate for bioconversion processes (Zou et al., 2022). Jiménez-Bonilla et al. (2020) overexpressed efflux pump-encoding gene srgB from P. putida srgB in C. saccharoperbutylacetonicum. This modification resulted in improved tolerance of the recombinant strain to furfural and ferulic acid, and could produce more than 14 g/L of butanol in the presence of these inhibitors. The production was similar to its performance under regular fermentation conditions (Jiménez-Bonilla et al., 2020). Similarly, Kurgan et al., 2019 identified small multidrug resistance pumps (encoded by mdtJI and sugE) and one glycolate/lactate permease (encoded by lldP) as an efflux transporter of furfural. The integration of mdtJI improved the cell biomass of the engineered strain by 4.8-fold in the presence of 1 g/L of furfural (Kurgan et al., 2019). In another study by Yi et al. (2021), potential transporters responsible for furfural and 5-hydroxymethyl-furfural tolerance were identified. These transporters include ABC transporter (ZMO0799 and ZMO0800), MFS transporter (ZMO1288 and ZMO1856), and RND transporter (ZMO0282 and ZMO0798) (Yi et al., 2021). However, further research was not conducted to demonstrate its role. Qiu et al. (2023a) strengthened the cell wall of P. acidilactici strains for improved inhibitor tolerance. They overexpressed key genes involved in peptidoglycan biosynthesis (murG and/or mraY) in a previously developed P. acidilactici for lactic acid production. All the recombinant strains produced higher lactic acid compared to the host strain; the highest lactic acid and cell growth was shown by the recombinant overexpressing mraY. It produced approximately 9-13 g/L lactic acid from 20% and 50% pretreated corncob liquor (Qiu et al., 2023a).
All the studies discussed above demonstrate diverse approaches, including the engineering of transcriptional regulators, conversion of toxic compounds into non-toxic ones, overexpression of efflux pumps, efflux transporters, and cell wall reinforcement, to enhance the tolerance of microbial strains to LCB hydrolysate inhibitors, enabling more efficient and productive bioconversion processes.
The co-utilization of various sugars derived from LCB is a critical aspect of lignocellulose biorefinery and presents several challenges. Two primary challenges associated with the simultaneous co-utilization of LCB-derived sugars are glucose repression and either the presence of inefficient xylose catabolic pathways or complete absence. Glucose, the most abundant sugar released during LCB hydrolysis, can exert repression on the utilization of other sugars, such as xylose and arabinose, which results in incomplete consumption of sugars like xylose and arabinose. Overcoming glucose repression is essential for achieving efficient co-utilization of multiple sugars. In addition, many microorganisms do not possess an efficient native xylose catabolic pathway. Consequently, these organisms struggle to efficiently ferment xylose, which is one of the major sugars derived from LCB. Engineering microorganisms to develop or enhance xylose catabolic pathways is equally crucial for achieving the co-utilization of glucose and xylose.
Various metabolic engineering approaches have been employed to enable the simultaneous metabolism of all sugars derived from LCB (Table 5). Elmore et al. (2020) engineered P putida to co-utilize a variety of sugars present in corn stover hydrolysate. They deleted genes associated with glucose metabolism/repression (gcd, crc) and introduced pathways for xylose metabolism (xylAB-mutated xylE*) and arabinose metabolism (araACBDE) derived from E. coli and B. amifraia respectively. In addition, PP pathway enzymes tkt, tal, and rpe1 were overexpressed. This resulted in a P. putida strain that efficiently co-consumed all sugars (glucose, xylose, arabinose) including p-coumaric acid and acetic acid derived from corn stover hydrolysate, with maximum sugar utilization rates up to 3.3 g/L/h, sugar specific-utilization rates up to 1.29 g utilized sugar/g cell/h, and growth rates over 0.2 h-1 (Elmore et al., 2020). A similar strategy was used to engineer S. cerevisiae by Huang et al. (2019b). They first overexpressed Bacteroides vulgatus-derived xylose metabolism and L. plantarum-derived arabinose metabolic pathways in S. cerevisiae. Through adaptive evolution on xylose and arabinose, they obtained a strain capable of co-utilizing xylose and glucose, achieving high ethanol production (54.11 g/L) from wheat straw hydrolysate (Huang et al., 2019b). To develop a sugar co-utilizing strain of C. tyrobutylicum, Fu et al. (2022) deleted hprK (associated with glucose repression) and xylR (a negative regulator of the xylose operon). The resulting strain showed no glucose repression and significantly improved xylose consumption by 72-94%. Eventually, the engineered C. tyrobutylicum (ΔhprKΔxylR) could produce 12.4-13.37 g/L butyrate from undetoxified corn cob, wheat straw, and soybean straw hydrolysates, 21-39% higher compared to wild type (Fu et al., 2022). Schizochytrium sp. is a non-efficient xylose-metabolizing strain. Wang et al. (2022b) created an efficient xylose-metabolizing Schizochytrium sp by introducing the xylose isomerase pathway (consisting of Piromyces sp xylose isomerase and S. cerevisiae xylulose kinase). The resultant recombinant completely co-consumed glucose and xylose and produced 5.3 g/L of lipid from corn stover hydrolysate, while the wild strain produced 30.4% less (Wang et al., 2022b). Similarly, non-xylose consuming S. cerevisiae was converted into utilizing xylose by disrupting genes (gre3 and pho13), integrating the xylose isomerase pathway (consisting of Piromyces sp mutated xylA*3, native xylulokinase) and S stipitis tal1, and evolutionary engineering on xylose. The evolved strain simultaneously co-consumed glucose and xylose without affecting the xylose consumption rate (Hoang et al., 2018). In a subsequent investigation, they further modified the evolved strain by introducing an additional copy of xylA*3 and rpe1 onto the asc1 locus to enhance the efficiency of xylose metabolism. As a result, the engineered S. cerevisiae exhibited a notable improvement in the rate of xylose consumption, achieving 0.40 g/L/h during mixed sugar fermentation. During bioethanol fermentation, the strain demonstrated a xylose consumption rate of 0.23 g/g/h and an overall sugar consumption rate of 0.66 g/g/h. Moreover, it produced 30.1 g/L of ethanol with a sugar-to-ethanol yield of 0.48 g/g, utilizing miscantus hydrolysates (Tran et al., 2020). Numerous other studies have also been conducted to develop xylose-consuming strains by incorporating the xylose isomerase pathway (Kim et al., 2022a; Yook et al., 2020; Zan et al., 2021; Ling et al., 2022).
In addition to the xylose isomerase pathway, some studies have explored the xylose oxidative pathway as an alternative route for xylose metabolism. Promdonkoy et al. (2019) conducted a study to screen efficient xylose oxidative pathways for xylose utilization. They introduced xylose oxidative pathways derived from different yeasts, including C. tropicalis, C. shehatae, S. stipitis, and Spathaspora passalidarum into S. cerevisiae. Among these, the recombinant strain overexpressing the S. stipitis xylose oxidative pathway exhibited the highest cell growth on xylose. To further enhance xylose metabolism, they overexpressed a mutant hexose transporter (HXT7-F79S) to improve xylose influx and deleted the genes gre3 and pho13. These modifications resulted in a strain with an improved specific growth rate on xylose (Promdonkoy et al., 2019). Jeong et al. (2020) compared xylose isomerase and oxidative pathway by introducing Orpinomyces sp. xylA-P stipitis xyl3 and P stipitis xyl123 respectively, in the ald6 locus of S. cerevisiae. The recombinant overexpressing xylose oxidative pathway demonstrated consumption of a higher percentage of xylose (90%) compared to the strain using the xylose isomerase pathway (10%) (Jeong et al., 2020). Chattopadhyay et al. (2020) identified the two xylose transporters (CtStp1 and CtStp2) in C. tropicalis that were not influenced by glucose. When these transporters were heterologously overexpressed (CtStp1 or CtStp2) along with the xylose oxidative pathway (P. stipitis xyl1 and xyl2, and S. cerevisiae xks1) in a null mutant S. cerevisiae strain lacking hexose transporters, the recombinants exhibited the simultaneous co-consumption of glucose and xylose (Chattopadhyay et al., 2020).
In addition to this, several other strategies such as the disruption of ptsGHI and overexpression of sugar transporter for the efficient co-utilization of sugars were already discussed in Section 2.
The current global production of bio-based chemicals and polymers is estimated to be approximately 90 million metric tons. This production is expected to contribute significantly to the revenue of the global chemical industry, with an estimated value of US$ 10 billion. The global production capacity and market values of chemicals mentioned above are provided in Table 6.
Mancini et al. (2022) performed a techno-economic analysis (TEA) of 4 different biosuccinic acid production processes using corn stover as feedstock: Reverdia, Bioamber, Michigan State University patents (MSUP), and conceptual. Process I (Reverdia) used engineered S. cerevisiae and produced 43 g/L of succinic acid with a yield of 0.69% and a productivity of 0.45 g/L/h. Process II (Bioamber) employed engineered Issaichenkia orientalis, resulting in the production of 48.2 g/L of succinic acid with a yield of 0.45% and a productivity of 0.97 g/L/h. Process III used A. succinogenes and produced 42.8 g/L of succinic acid with a yield of 0.74% and a productivity of 0.43 g/L/h. Process IV (conceptual) also used A. succinogenes but in an immobilized form. It produced 39.6 g/L of succinic acid with a yield of 0.78% and a productivity of 1.77 g/L/h (or 45.7 g/L with a productivity of 2.16 g/L/h). The calculated product cost (PC) and minimum selling price (MSP) of these processes were as follows: Process I – PC of $33.40 million per annum with an MSP of $2.3 per kilogram; Process II - PC of $51.34 million per annum with an MSP of $3.2 per kilogram; Process III - PC of $37.83 million per annum with an MSP of $2.6 per kilogram; and Process IV - PC of $26.63 million per annum with an MSP of $1.40 per kilogram. Compared to fuel-based succinic acid with an MSP of $2 per kilogram, Process I would be cheaper, both Process I and Process III could be competitive, and Process III may not be profitable (Mancini et al., 2022). In another study by Dickson et al. (2021), a detailed TEA was conducted for succinic acid production using different feedstocks, including glucose, corn stover, glycerol, and seaweed, with A. succinogenes. The lowest MSP for succinic acid (ranging from $1.6 to $1.9 per kilogram) was estimated to be when glycerol was used as the feedstock. With acid-thermal pretreated corn stover, the MSP was slightly higher, ranging from $1.7 to $2 per kilogram. The production of biosuccinic acid using glycerol and corn stover could be cheaper compared to fuel-based succinic acid (Dickson et al., 2021). In conclusion, through metabolic engineering of the host strain and the appropriate choice of feedstock, biosuccinic acid can be made competitive with petroleum-based succinic acid.
In a study conducted by Liu et al. (2015), a TEA was performed for the production of L-lactic acid from corn stover using engineered P. acidilactici. This engineered strain was capable of producing 104.5 g/L of L-lactic acid with an overall yield of 71.5%. When acid-pretreated and biodetoxified corn stover was used as the feedstock, the MSP of L-lactic acid was calculated to be $0.523 per kilogram. They also suggested that further engineering of P. acidilactici to enable the conversion of xylose to L-lactic acid could significantly reduce the price (Liu et al., 2015). However, even with the initial MSP, it was lower compared to the current market price of L-lactic acid, which ranges from $1.7 to $2 per kilogram (Li et al., 2021c). In another TEA conducted by Liu et al. (2020), the production of cellulosic itaconic acid by A. terreus was evaluated. This strain produced 33.6 g/L of itaconic acid with a yield of 0.56 g/g from acid-pretreated and biodetoxified corn stover. The estimated MSP of itaconic acid was $1.65 per kilogram, which was lower than the market price of $1.8 per kilogram. Additionally, the MSP could be further reduced to $0.87 per kilogram through strain engineering to improve yield, achieving 0.22 kilogram of itaconic acid per kilogram of corn stover (Liu et al., 2020).
In a study conducted by Gadkari et al. (2023), a detailed TEA and profitability analysis was performed to assess the conversion of sugarcane bagasse sugar into BDO using a mutant strain of Enterobacter ludwigii. This mutant strain was capable of producing 63.5 g/L of BDO from detoxified sugarcane bagasse hydrolysate. They compared five different scenarios and found that the estimated MSP of BDO varied between $1.86 and $3.99 per kilogram, depending on the specific scenario. Taking into account factors such as a standalone facility, the cost of sugarcane bagasse and utilities, and the complete utilization of carbohydrates derived from sugarcane bagasse, the minimum production cost and MSP of BDO were calculated to be $1.21 per kilogram and $1.86 per kilogram, respectively. It is worth noting that an increase in production capacity from 96 metric tons per day to 2000 metric tons per day could result in a 2.50-fold reduction in production costs (Gadkari et al., 2023). Similarly, in a TEA for the co-production of BDO and furfural/technical lignin from switch grass (pretreated with a deep eutectic solvent) using B. vallismortis, the estimated MSP of BDO ranged from $1.7 to $1.74 per kilogram, depending on the pretreatment process. Notably, this estimated price was approximately half of the current market price for BDO (Zang et al., 2020).
A TEA of xylitol production from sugarcane bagasse using engineered and evolved Y. lipolytica was conducted by Shaji et al. (2022). The MSP of xylitol was estimated to be $6.2 per kilogram. This estimation was based on a fermentation yield of 0.54 grams of xylitol per gram of xylose and a production capacity of 437.4 kilograms per hour (Shaji et al., 2022). Similarly, in a study by Mountraki et al. (2017), a TEA was conducted for xylitol production using Candida yeast and Raney nickel catalyst. The estimated MSP of xylitol produced by biological methods ranged from 2.92 to 3.07 euros per kilogram, which is higher compared to the MSP of xylitol produced by chemical methods, ranging from 1.88 to 1.90 euros per kilogram (Mountraki et al., 2017).
The perfect microbial cell factory for lignocellulose biorefinery should be strong and capable of converting LCB with minimal preprocessing. Moreover, it should be able to efficiently utilize sugars derived from lignocellulose, exhibit tolerance to inhibitors, and produce high concentrations of desired products with exceptional efficiency and productivity. Despite substantial progress in chemical production from lignocellulose, a key challenge is the tolerance to inhibitors. These inhibitors not only impede cell growth but also restrict the concentration, efficiency, and productivity of fermentation processes. The types and quantities of toxic compounds generated during the pretreatment process can vary significantly based on the type of LCB. While various successful attempts have been made to enhance microbial tolerance to individual inhibitors, achieving tolerance to single or multiple inhibitors at higher concentrations remains a challenging area requiring further research to comprehensively understand the underlying molecular mechanisms. Furthermore, it is important to note that many tolerance mechanisms have a substantial metabolic cost, including high energy (ATP) and redox cofactor requirements. This cost, when coupled with the demands of both tolerance mechanisms and desired product pathways, can lead to metabolic imbalances. Therefore, the development of microbial cell factories should consider potential metabolic imbalances that may arise along with the establishment of biosynthetic pathways (natural or synthetic) and the deletion of byproduct pathways.
Although not addressed in this review, another significant challenge in lignocellulose biorefinery is the saccharification of LCB. While several enzymes have been discovered and utilized for this purpose, issues such as high costs, enzyme stability problems, and slow reaction rates can hinder saccharification and fermentation processes, resulting in low yields. An alternative approach for this is direct microbial saccharification, which can substantially reduce saccharification costs. Many microbes are known to produce hydrolytic enzymes and have been widely explored for this purpose. Designing a microbial cell that produces an optimal mix of synergistic lignocellulolytic enzymes would be particularly interesting. Furthermore, engineering enzymes or pathways to enhance enzyme kinetics and stability under harsh conditions could significantly benefit the saccharification process.
In recent years, synthetic and system biology has emerged as a potent tool for designing, constructing, and testing microbial cell factories dedicated to the sustainable and renewable production of chemicals. Various tools, including databases (such as KEGG, MetaCyc, antiSMASH, and BRENDA) along with genome mining software tools (such as ClusterFinder, NP.searcher, NaPDoS, and RetroPath 2.0) have become essential for the discovery and design of potential pathways. Artificial intelligence tools such as BNICE.ch, RetroPath RL, RetroBioCat, novoStoic, GEM-Path, PathPred, ICHO, SCROP, RetroXpert, and G2G would be effectively employed in this process. The designed pathways are further optimized for industrial-level chemical production. This optimization includes the design or engineering of synthetic promoters (utilizing promoter libraries), ribosomal binding sites (using UTR designer, RBS designer, and RBS calculator), terminators, and the chromosomal expression of pathways (employing CRISPR/Cas9, CRAGE, XPORT). Successful microbial cell factory design requires the optimization of the entire biological network rather than focusing solely on central metabolism and target biosynthetic pathways. Computational models, such as genome-scale metabolic models and dynamic metabolic models integrated with kinetic information and internal regulation of metabolism, become powerful tools for understanding metabolism and guiding metabolic engineering. Omics technologies, spanning genomics, transcriptomics, and metabolomics, play a crucial role in elucidating complex phenotypes and providing a comprehensive understanding of cell metabolism at a systems level (Cho et al., 2022).
Microbial valorization of LCB to value-added chemicals has gained much attention due to economic and environmental aspects. It requires extensive metabolic engineering and a comprehensive understanding of microbial catabolic and desired product pathways, and toxicity tolerance pathways. In addition, a TEA of bioprocesses is necessary to check the economic viability at the commercial scale. This review highlighted recent advances in metabolic engineering and TEA for the production of several chemicals including succinic acid, lactic acid, 3-HP, itaconic acid, xylitol, biohydrocarbons, BT, BDO, and 2-PE.
SL: Conceptualization, Writing – original draft, Writing – review & editing, Resources. LPT: Writing – review & editing. SKU: Writing – review & editing. DPG: Writing – review & editing. AS: Writing – review & editing.
The author(s) declare that no financial support was received for the research, authorship, and/or publication of this article.
The authors acknowledge PORIN, Kathmandu University and Tribhuvan University for their support.
The authors declare that the research was conducted in the absence of any commercial or financial relationships that could be construed as a potential conflict of interest. The author SL declared that they were an editorial board member of Frontiers, at the time of submission. This had no impact on the peer review process and the final decision.
All claims expressed in this article are solely those of the authors and do not necessarily represent those of their affiliated organizations, or those of the publisher, the editors and the reviewers. Any product that may be evaluated in this article, or claim that may be made by its manufacturer, is not guaranteed or endorsed by the publisher.
360 Research Reports. (2023). Global bio-3-hydroxypropionic acid market proffessional survery by types, applications, and players, with regional growth rate analysis and dvevelopment situation, from 2023 to 2028; Maia-24721839.
Abedi E., Hashemi S. M. B. (2020). Lactic acid production – producing microorganisms and substrates sources-state of art. Heliyon 6, e04974. doi: 10.1016/j.heliyon.2020.e04974
Ahuja V., Macho M., Ewe D., Singh M., Saha S., Saurav K. (2020). Biological and pharmacological potential of xylitol: A molecular insight of unique metabolism. Foods 9, 1–24. doi: 10.3390/foods9111592
Alves R. F., Zetty-Arenas A. M., Demirci H., Dias O., Rocha I., Basso T. O., et al. (2021). Enhancing acetic acid and 5-hydroxymethyl furfural tolerance of C. saccharoperbutylacetonicum through adaptive laboratory evolution. Process Biochem. 101, 179–189. doi: 10.1016/j.procbio.2020.11.013
Amoah J., Kahar P., Ogino C., Kondo A. (2019). Bioenergy and Biorefinery: feedstock, biotechnological conversion and products. Biotechnol. J. 14, 1800494. doi: 10.1002/biot.201800494
Amraoui Y., Prabhu A. A., Narisetty V., Coulon F., Kumar Chandel A., Willoughby N., et al. (2022). Enhanced 2,3-Butanediol production by mutant Enterobacter ludwigii using Brewers’ spent grain hydrolysate: Process optimization for a pragmatic biorefinery loom. Chem. Eng. J. 427, 130851. doi: 10.1016/j.cej.2021.130851
Ashokkumar V., Venkatkarthick R., Jayashree S., Chuetor S., Dharmaraj S., Kumar G., et al. (2022). Recent advances in lignocellulosic biomass for biofuels and value-added bioproducts-A critical review. Bioresour. Technol. 344, 126195. doi: 10.1016/j.biortech.2021.126195
Azaizeh H., Abu Tayeh H. N., Schneider R., Klongklaew A., Venus J. (2020). Production of lactic acid from carob, banana and sugarcane lignocellulose biomass. Molecules 25, 2956. doi: 10.3390/molecules25132956
Bamba T., Yukawa T., Guirimand G., Inokuma K., Sasaki K., Hasunuma T., et al. (2019). Production of 1,2,4-butanetriol from xylose by Saccharomyces cerevisiae through Fe metabolic engineering. Metab. Eng. 56, 17–27. doi: 10.1016/j.ymben.2019.08.012
Banu J. R., Kavitha S., Kumar V., Gunasekaran M., Parthiba O., Kumar G. (2021). Lignocellulosic biomass based biorefinery: A successful platform towards circular bioeconomy. Fuel 302, 121086. doi: 10.1016/j.fuel.2021.121086
Baptista S. L., Carvalho L. C., Romaní A., Domingues L. (2020). Development of a sustainable bioprocess based on green technologies for xylitol production from corn cob. Ind. Crops Prod. 156, 112867. doi: 10.1016/j.indcrop.2020.112867
Baptista S. L., Cunha J. T., Romaní A., Domingues L. (2018). Xylitol production from lignocellulosic whole slurry corn cob by engineered industrial Saccharomyces cerevisiae PE-2. Bioresour. Technol. 267, 481–491. doi: 10.1016/j.biortech.2018.07.068
Bi H., Xv C., Su C., Feng P., Zhang C., Wang M., et al. (2022). β-Farnesene production from low-cost glucose in lignocellulosic hydrolysate by engineered Yarrowia lipolytica. Fermentation 8, 532. doi: 10.3390/fermentation8100532
Bozell J. J., Petersen G. R. (2010). Technology development for the production of biobased products from biorefinery carbohydrates—the US department of energy’s “Top 10” revisited. Green Chem. 12, 539–554. doi: 10.1039/b922014c
Bu J., Yan X., Wang Y. T., Zhu S. M., Zhu M. J. (2019). Co-production of high-gravity bioethanol and succinic acid from potassium peroxymonosulfate and deacetylation sequentially pretreated sugarcane bagasse by simultaneous saccharification and co-fermentation. Energy Convers. Manage. 186, 131–139. doi: 10.1016/j.enconman.2019.02.038
Bukhari N. A., Jahim J. M., Loh S. K., Nasrin A. B., Harun S., Abdul P. M. (2020). Organic acid pretreatment of oil palm trunk biomass for succinic acid production. Waste Biomass Valorization 11, 5549–5559. doi: 10.1007/s12649-020-00953-2
Bukhari N. A., Luthfi A. A. I., Rahim N. A., Nasrin A. B., Sukiran M. A., Loh S. K. (2023). Biomass deacetylation at moderate solid loading improves sugar recovery and succinic acid production. Fermentation 9, 235. doi: 10.3390/fermentation9030235
Campos J., Tejada L. G., Bao J., Lidén G. (2023). Fed-batch strategies for biodetoxification in production of optically pure lactic acid from softwood hydrolysate using Pediococcus acidilactici. Process Biochem. 125, 162–170. doi: 10.1016/j.procbio.2022.12.027
Cao Y., Niu W., Guo J., Xian M., Liu H. (2015). Biotechnological production of 1,2,4-butanetriol: An efficient process to synthesize energetic material precursor from renewable biomass. Sci. Rep. 5, 18149. doi: 10.1038/srep18149
Cha J. W., Jang S. H., Kim Y. J., Chang Y. K., Jeong K. J. (2020). Engineering of Klebsiella oxytoca for production of 2,3-butanediol using mixed sugars derived from lignocellulosic hydrolysates. GCB Bioenergy 12, 275–286. doi: 10.1111/gcbb.12674
Chandel A. K., Garlapati V. K., Jeevan Kumar S. P., Hans M., Singh A. K., Kumar S. (2020). The role of renewable chemicals and biofuels in building a bioeconomy. Biofuels Bioprod. Biorefining 14, 830–844. doi: 10.1002/bbb.2104
Chang S., Zhang S., Chen T., Xu L., Ge S., Li B., et al. (2023). Efficient synthesis of 5-hydroxymethyl-2-furancarboxylic acid from bio-based high-concentration 5-hydroxymethylfurfural via highly tolerant aldehyde dehydrogenase. Mol. Catal. 538, 112966. doi: 10.1016/j.mcat.2023.112966
Chattopadhyay A., Singh R., Das A. K., Maiti M. K. (2020). Characterization of two sugar transporters responsible for efficient xylose uptake in an oleaginous yeast Candida tropicalis SY005. Arch. Biochem. Biophys. 695, 108645. doi: 10.1016/j.abb.2020.108645
Chen X., Kuhn E., Jennings E. W., Nelson R., Tao L., Zhang M., et al. (2016). DMR (deacetylation and mechanical refining) processing of corn stover achieves high monomeric sugar concentrations (230 g L-1) during enzymatic hydrolysis and high ethanol concentrations (>10% v/v) during fermentation without hydrolysate purification or concentration. Energy Environ. Sci. 9, 1237–1245. doi: 10.1039/c5ee03718b
Chen X., Kuhn E., Nagle N., Nelson R., Tao L., Crawford N., et al. (2018). Recycling of dilute deacetylation black liquor to enable efficient recovery and reuse of spent chemicals and biomass pretreatment waste. Front. Energy Res. 6. doi: 10.3389/fenrg.2018.00051
Cho J. S., Kim G. B., Eun H., Moon C. W., Lee S. Y. (2022). Designing microbial cell factories for the production of chemicals. JACS Au 2, 1781–1799. doi: 10.1021/jacsau.2c00344
Cortivo P. R. D., MaChado J., Hickert L. R., Rossi D. M., Ayub M. A. Z. (2019). Production of 2,3-butanediol by Klebsiella pneumoniae BLh-1 and Pantoea agglomerans BL1 cultivated in acid and enzymatic hydrolysates of soybean hull. Biotechnol. Prog. 35, 1–8. doi: 10.1002/btpr.2793
Cubas-cano E., González-fernández C., Ballesteros M., Tomás-Pejó E. (2018). Biotechnological advances in lactic acid production by lactic acid bacteria: lignocellulose as a novel substrate. Biofuels Bioprod. Biorefining 12, 290–303. doi: 10.1002/bbb.1852
Czinkóczky R., Németh Á. (2023). “Bioproduction of isoprene and isoprenoids,” in Valorization of Biomass to Bioproducts: Biochemicals and Biomaterials (Cambridge, MA, United States: Elsevier), 265–277. doi: 10.1016/B978-0-12-822887-6.00021-8
Dahmen N., Lewandowski I., Zibek S., Weidtmann A. (2019). Integrated lignocellulosic value chains in a growing bioeconomy: Status quo and perspectives. Glob. Change Biol. Bioenergy 11, 107–117. doi: 10.1111/gcbb.12586
Dai Z., Guo F., Zhang S., Zhang W., Yang Q., Dong W., et al. (2020). Bio-based succinic acid: an overview of strain development, substrate utilization, and downstream purification. Biofuels Bioprod. Biorefining 14, 965–985. doi: 10.1002/bbb.2063
Dai Z., Pomraning K. R., Deng S., Kim J., Campbell K. B., Robles A. L., et al. (2023). Metabolic engineering to improve production of 3-hydroxypropionic acid from corn-stover hydrolysate in Aspergillus species. Biotechnol. Biofuels Bioprod. 16, 53. doi: 10.1186/s13068-023-02288-1
D’ambrosio S., Zaccariello L., Sadiq S., D’Albore M., Battipaglia G., D’Agostino M., et al. (2023). Grape stalk valorization: An efficient re-use of lignocellulosic biomass through hydrolysis and fermentation to produce lactic acid from Lactobacillus rhamnosus IMC501. Fermentation 9, 616. doi: 10.3390/fermentation9070616
De Fouchécour F., Sánchez-Castañeda A. K., Saulou-Bérion C., Spinnler H.É. (2018). Process engineering for microbial production of 3-hydroxypropionic acid. Biotechnol. Adv. 36, 1207–1222. doi: 10.1016/j.bioteChadv.2018.03.020
Deng W., Feng Y., Fu J., Guo H., Guo Y., Han B., et al. (2023). Catalytic conversion of lignocellulosic biomass into chemicals and fuels. Green Energy Environ. 8, 10–114. doi: 10.1016/j.gee.2022.07.003
Dickson R., Mancini E., Garg N., Woodley J. M., Gernaey K. V., Pinelo M., et al. (2021). Sustainable bio-succinic acid production: superstructure optimization, techno-economic, and lifecycle assessment. Energy Environ. Sci. 14, 3542–3558. doi: 10.1039/d0ee03545a
Du C., Li Y., Zong H., Yuan T., Yuan W., Jiang Y. (2020). Production of bioethanol and xylitol from non-detoxified corn cob via a two-stage fermentation strategy. Bioresour. Technol. 310, 123427. doi: 10.1016/j.biortech.2020.123427
Duncan S. M., Alkasrawi M., Gurram R., Almomani F., Wiberley-Bradford A. E., Singsaas E. (2020). Paper mill sludge as a source of sugars for use in the production of bioethanol and isoprene. Energies 13, 4662. doi: 10.3390/en13184662
Elmore J. R., Dexter G. N., Salvachúa D., O’Brien M., Klingeman D. M., Gorday K., et al. (2020). Engineered Pseudomonas putida simultaneously catabolizes five major components of corn stover lignocellulose: Glucose, xylose, arabinose, p-coumaric acid, and acetic acid. Metab. Eng. 62, 62–71. doi: 10.1016/j.ymben.2020.08.001
Erian A. M., Gibisch M., Pflügl S. (2018). Engineered E. coli W enables efficient 2,3-butanediol production from glucose and sugar beet molasses using defined minimal medium as economic basis. Microb. Cell Fact. 17, 190. doi: 10.1186/s12934-018-1038-0
Filippi K., Georgaka N., Alexandri M., Papapostolou H., Koutinas A. (2021). Valorisation of grape stalks and pomace for the production of bio-based succinic acid by Actinobacillus succinogenes. Ind. Crops Prod. 168, 113578. doi: 10.1016/j.indcrop.2021.113578
Fu H., Zhang H., Guo X., Yang L., Wang J. (2022). Elimination of carbon catabolite repression in Clostridium tyrobutyricum for enhanced butyric acid production from lignocellulosic hydrolysates. Bioresour. Technol. 357, 127320. doi: 10.1016/j.biortech.2022.127320
Gadkari S., Narisetty V., Maity S. K., Manyar H., Mohanty K., Jeyakumar R. B., et al. (2023). Techno-economic analysis of 2,3-butanediol production from sugarcane bagasse. Ind. Eng. Chem. Res. 11, 8337–8349. doi: 10.1021/acssuschemeng.3c01221
Global Market Insight. (2022). 2-Phenylethanol market - by product (synthetic, natural), by application (cosmetics & personal care, laundry & home care, food & beverages, pharmaceuticals) & Forecast, 2022-2028; GMI5063.
Hakkim S., Sindhu R., Pandey A., Binod P. (2020). Lignocellulosic bio-refinery approach for microbial 2,3-Butanediol production. Bioresour. Technol. 302, 122873. doi: 10.1016/j.biortech.2020.122873
Hanh D. D., Elkasaby T., Kawaguchi H., Tsuge Y., Ogino C., Kondo A. (2023). Enhanced production of itaconic acid from enzymatic hydrolysate of lignocellulosic biomass by recombinant Corynebacterium glutamicum. J. Biosci. Bioeng. 136, 7–12. doi: 10.1016/j.jbiosc.2023.03.011
Hazeena S. H., Nair Salini C., Sindhu R., Pandey A., Binod P. (2019). Simultaneous saccharification and fermentation of oil palm front for the production of 2,3-butanediol. Bioresour. Technol. 278, 145–149. doi: 10.1016/j.biortech.2019.01.042
He N., Chen M., Qiu Z., Fang C., Lidén G., Liu X., et al. (2023). Simultaneous and rate-coordinated conversion of lignocellulose derived glucose, xylose, arabinose, mannose, and galactose into D-lactic acid production facilitates D-lactide synthesis. Bioresour. Technol. 377, 128950. doi: 10.1016/j.biortech.2023.128950
He Y., Li H., Chen L., Zheng L., Ye C., Hou J., et al. (2021). Production of xylitol by Saccharomyces cerevisiae using waste xylose mother liquor and corncob residues. Microb. Biotechnol. 14, 2059–2071. doi: 10.1111/1751-7915.13881
Hillman E. T., Li M., Hooker C. A., Englaender J. A., Wheeldon I., Solomon K. V. (2021). Hydrolysis of lignocellulose by anaerobic fungi produces free sugars and organic acids for two-stage fine chemical production with Kluyveromyces marxianus. Biotechnol. Prog. 37, e3172. doi: 10.1002/btpr.3172
Hernández-pérez A. F., Antunes F. A. F., Almeida G. D. (2020). Valorization of the sugarcane bagasse and straw hemicellulosic hydrolysate through xylitol bioproduction: effect of oxygen availability and sucrose supplementation as key factors. Biomass Convers. Bioref. 12, 4901–4915. doi: 10.1007/s13399-020-00993-7
Hoang P. T. N., Ko J. K., Gong G., Um Y., Lee S. M. (2018). Genomic and phenotypic characterization of a refactored xylose-utilizing Saccharomyces cerevisiae strain for lignocellulosic biofuel production. Biotechnol. Biofuels 11, 268. doi: 10.1186/s13068-018-1269-7
Hua Y., Wang J., Zhu Y., Zhang B., Kong X., Li W., et al. (2019). Release of glucose repression on xylose utilization in Kluyveromyces marxianus to enhance glucose-xylose co-utilization and xylitol production from corncob hydrolysate. Microb. Cell Fact. 18, 24. doi: 10.1186/s12934-019-1068-2
Huang M., Cheng J., Chen P., Zheng G., Wang D., Hu Y. (2019a). Efficient production of succinic acid in engineered Escherichia coli strains controlled by anaerobically-induced nirB promoter using sweet potato waste hydrolysate. J. Environ. Manage. 237, 147–154. doi: 10.1016/j.jenvman.2019.02.041
Huang S., Liu T., Peng B., Geng A. (2019b). Enhanced ethanol production from industrial lignocellulose hydrolysates by a hydrolysate-cofermenting Saccharomyces cerevisiae strain. Bioprocess Biosyst. Eng. 42, 883–896. doi: 10.1007/s00449-019-02090-0
Isikgor F. H., Becer C. R. (2015). Polymer chemistry the production of bio-based chemicals and polymers. Polym. Chem. 6, 4497. doi: 10.1039/c5py00263j
Jayakody L. N., Chinmoy B., Turner T. L. (2022). Trends in valorization of highly-toxic lignocellulosic biomass derived-compounds via engineered microbes. Bioresour. Technol. 346, 126614. doi: 10.1016/j.biortech.2021.126614
Jeong D., Oh E. J., Ko J. K., Nam J. O., Park H. S., Jin Y. S., et al. (2020). Metabolic engineering considerations for the heterologous expression of xylose-catabolic pathways in Saccharomyces cerevisiae. PLoS One 15, e0236294. doi: 10.1371/journal.pone.0236294
Jiménez-Bonilla P., Zhang J., Wang Y., Blersch D., De-Bashan L. E., Guo L., et al. (2020). Enhancing the tolerance of Clostridium saccharoperbutylacetonicum to lignocellulosic-biomass-derived inhibitors for efficient biobutanol production by overexpressing efflux pumps genes from Pseudomonas putida. Bioresour. Technol. 312, 123532. doi: 10.1016/j.biortech.2020.123532
Jokodola E. O., Narisetty V., Castro E., Durgapal S., Coulon F., Sindhu R., et al. (2022). Process optimisation for production and recovery of succinic acid using xylose-rich hydrolysates by Actinobacillus succinogenes. Bioresour. Technol. 344, 126224. doi: 10.1016/j.biortech.2021.126224
Jong E., Stichnothe H., Bell G., Jørgensen H. (2020) Bio-based chemicals: A 2020 update - IEA bioenergy: Task 42. Available at: https://www.ieabioenergy.com/blog/publications/new-publication-bio-based-chemicals-a-2020-update.
Junior W. G. M., Thalyta P. F., Trichez D., Almeida J. R. M., Silva B. (2019). Xylitol production on sugarcane biomass hydrolysate by newly identified Candida tropicalis JA2 strain. Yeast 36, 349–361. doi: 10.1002/yea.3394
Karnaouri A., Asimakopoulou G., Kalogiannis K. G., Lappas A., Topakas E. (2020). Efficient D -lactic acid production by Lactobacillus delbrueckii subsp. bulgaricus through conversion of organosolv pretreated lignocellulosic biomass. Biomass Bioenergy 140, 105672. doi: 10.1016/j.biombioe.2020.105672
Karnaouri A., Asimakopoulou G., Kalogiannis K. G., Lappas A. A., Topakas E. (2021). Efficient production of nutraceuticals and lactic acid from lignocellulosic biomass by combining organosolv fractionation with enzymatic/fermentative routes. Bioresour. Technol. 341, 125846. doi: 10.1016/j.biortech.2021.125846
Kerssemakers A. A. J., Doménech P., Cassano M., Yamakawa C. K., Dragone G., Mussatto S. I. (2020). Production of itaconic acid from cellulose pulp: Feedstock feasibility and process strategies for an efficient microbial performance. Energies 13, 1654. doi: 10.3390/en13071654
Kim J., Hwang S., Lee S. M. (2022a). Metabolic engineering for the utilization of carbohydrate portions of lignocellulosic biomass. Metab. Eng. 71, 2–12. doi: 10.1016/j.ymben.2021.10.002
Kim J. W., Lee Y. G., Kim S. J., Jin Y. S., Seo J. H. (2019). Deletion of glycerol-3-phosphate dehydrogenase genes improved 2,3-butanediol production by reducing glycerol production in pyruvate decarboxylase-deficient Saccharomyces cerevisiae. J. Biotechnol. 304, 31–37. doi: 10.1016/j.jbiotec.2019.08.009
Kim J., Son H. F., Hwang S., Gong G., Ko J. K., Um Y., et al. (2022b). Improving lipid production of Yarrowia lipolytica by the aldehyde dehydrogenase-mediated furfural detoxification. Int. J. Mol. Sci. 23, 4761. doi: 10.3390/ijms23094761
Kim D. G., Yoo S. W., Kim M., Ko J. K., Um Y., Oh M. K. (2020). Improved 2,3-butanediol yield and productivity from lignocellulose biomass hydrolysate in metabolically engineered Enterobacter aerogenes. Bioresour. Technol. 309, 123386. doi: 10.1016/j.biortech.2020.123386
Kong X., Zhang B., Hua Y., Zhu Y., Li W., Wang D., et al. (2019). Efficient L-lactic acid production from corncob residue using metabolically engineered thermo-tolerant yeast. Bioresour. Technol. 273, 220–230. doi: 10.1016/j.biortech.2018.11.018
Kumar V., Ashok S., Park S. (2013). Recent advances in biological production of 3-hydroxypropionic acid. Biotechnol. Adv. 31, 945–961. doi: 10.1016/j.bioteChadv.2013.02.008
Kurgan G., Panyon L. A., Rodriguez-Sanchez Y., Pacheco E., Nieves L. M., Mann R., et al. (2019). Bioprospecting of native efflux pumps to enhance furfural tolerance in ethanologenic Escherichia coli. Appl. Environ. Microbiol. 85, e02985–e02918. doi: 10.1128/AEM.02985-18
Lam F. H., Turanli-Yildiz B., Liu D., Resch M. G., Fink G. R., Stephanopoulos G. (2021). Engineered yeast tolerance enables efficient production from toxified lignocellulosic feedstocks. Sci. Adv. 7, eabf7613. doi: 10.1126/sciadv.abf7613
Latine H., Nicolaï T., Arts W., Raikwar D., Pandalone B., Sels B. (2023). “Lignocellulose biorefinery advances the liquid biofuel platform,” in Handbook of Biofuels Production (Cambridge, MA, United States: Elsevier Ltd), 313–359. doi: 10.1016/B978-0-323-91193-1.00009-3
Leavell M. D., McPhee D. J., Paddon C. J. (2016). Developing fermentative terpenoid production for commercial usage. Curr. Opin. Biotechnol. 37, 114–119. doi: 10.1016/j.copbio.2015.10.007
Lee J. S., Lin C. J., Lee W. C., Teng H. Y., Chuang M. H. (2022). Production of succinic acid through the fermentation of Actinobacillus succinogenes on the hydrolysate of Napier grass. Biotechnol. Biofuels Bioprod. 15, 9. doi: 10.1186/s13068-022-02106-0
Lee Y. G., Seo J. H. (2019). Production of 2,3-butanediol from glucose and cassava hydrolysates by metabolically engineered industrial polyploid Saccharomyces cerevisiae. Biotechnol. Biofuels 12, 1–12. doi: 10.1186/s13068-019-1545-1
Li Y., Bhagwat S. S., Cortés-Penã Y. R., Ki D., Rao C. V., Jin Y. S., et al. (2021c). Sustainable lactic acid production from lignocellulosic biomass. ACS Sustain. Chem. Eng. 9, 1341–1351. doi: 10.1021/acssuschemeng.0c08055
Li K., Li C., Zhao X. Q., Liu C. G., Bai F. W. (2023a). Engineering Corynebacterium glutamicum for efficient production of succinic acid from corn stover pretreated by concentrated-alkali under steam-assistant conditions. Bioresour. Technol. 378, 128991. doi: 10.1016/j.biortech.2023.128991
Li C., Ong K. L., Cui Z., Sang Z., Li X., Patria R. D., et al. (2021b). Promising advancement in fermentative succinic acid production by yeast hosts. J. Hazard. Mater. 401, 123414. doi: 10.1016/j.jhazmat.2020.123414
Li Y. Q., Wang M. J., Gan X. F., Luo C. B. (2023b). Cleaner 2,3-butanediol production from unpretreated lignocellulosic biomass by a newly isolated Klebsiella pneumoniae PX14. Chem. Eng. J. 455, 140479. doi: 10.1016/j.cej.2022.140479
Li B., Wang L., Wu Y. J., Xia Z. Y., Yang B. X., Tang Y. Q. (2021a). Improving acetic acid and furfural resistance of xylose-fermenting Saccharomyces cerevisiae strains by regulating novel transcription factors revealed via comparative transcriptomic analysis. Appl. Environ. Microbiol. 87, e00157–e00121. doi: 10.1128/AEM.00158-21
Ling C., Peabody G. L., Salvachúa D., Kim Y. M., Kneucker C. M., Calvey C. H., et al. (2022). Muconic acid production from glucose and xylose in Pseudomonas putida via evolution and metabolic engineering. Nat. Commun. 13, 4925. doi: 10.1038/s41467-022-32296-y
Liu D., Hwang H. J., Otoupal P. B., Geiselman G. M., Kim J., Pomraning K. R., et al. (2023). Engineering Rhodosporidium toruloides for production of 3-hydroxypropionic acid from lignocellulosic hydrolysate. Metab. Eng. 78, 72–83. doi: 10.1016/j.ymben.2023.05.001
Liu R., Liang L., Garst A. D., Choudhury A., Nogué V. S., Beckham G. T., et al. (2018). Directed combinatorial mutagenesis of Escherichia coli for complex phenotype engineering. Metab. Eng. 47, 10–20. doi: 10.1016/j.ymben.2018.02.007
Liu Y., Liu G., Zhang J., Balan V., Bao J. (2020). Itaconic acid fermentation using activated charcoal-treated corn stover hydrolysate and process evaluation based on Aspen plus model. Biomass Convers. Biorefinery 10, 463–470. doi: 10.1007/s13399-019-00423-3
Liu G., Sun J., Zhang J., Tu Y., Bao J. (2015). High titer l-lactic acid production from corn stover with minimum wastewater generation and techno-economic evaluation based on Aspen plus modeling. Bioresour. Technol. 198, 803–810. doi: 10.1016/j.biortech.2015.09.098
Liu Y., Tang Y., Gao H., Zhang W., Jiang Y., Xin F., et al. (2021). Challenges and future perspectives of promising biotechnologies for lignocellulosic biorefiner. Molecules 26, 5411. doi: 10.3390/molecules26175411
Lo E., Brabo-Catala L., Dogaris I., Ammar E. M., Philippidis G. P. (2020). Biochemical conversion of sweet sorghum bagasse to succinic acid. J. Biosci. Bioeng. 129, 104–109. doi: 10.1016/j.jbiosc.2019.07.003
Lu H., Yadav V., Bilal M., Iqbal H. M. N. (2022). Bioprospecting microbial hosts to valorize lignocellulose biomass–Environmental perspectives and value-added bioproducts. Chemosphere 288, 132574. doi: 10.1016/j.chemosphere.2021.132574
Luo P., Zhang Y., Suo Y., Liao Z., Ma Y., Fu H., et al. (2018). The global regulator IrrE from Deinococcus radiodurans enhances the furfural tolerance of Saccharomyces cerevisiae. Biochem. Eng. J. 136, 69–77. doi: 10.1016/j.bej.2018.05.009
Ma X., Gao M., Yin Z., Zhu W., Liu S., Wang Q. (2020). Lactic acid and animal feeds production from Sophora flavescens residues by Rhizopus oryzae fermentation. Process Biochem. 92, 401–408. doi: 10.1016/j.procbio.2020.01.030
Ma L., Wang J., Zhi R., Lucia L. A., Li G., Deng Y. (2022). Utilization of corncob hydrolysate enables 2,3-butanediol production in Enterobacter cholerae. BioResources 17, 5820–5830. doi: 10.15376/biores.17.4.5820-5830
Mancini E., Dickson R., Fabbri S., Udugama I. A., Ullah H. I., Vishwanath S., et al. (2022). Economic and environmental analysis of bio-succinic acid production: From established processes to a new continuous fermentation approach with in-situ electrolytic extraction. Chem. Eng. Res. Des. 179, 401–414. doi: 10.1016/j.cherd.2022.01.040
Mao Y., Li G., Chang Z., Tao R., Cui Z., Wang Z., et al. (2018). Metabolic engineering of Corynebacterium glutamicum for efficient production of succinate from lignocellulosic hydrolysate. Biotechnol. Biofuels 11, 95. doi: 10.1186/s13068-018-1094-z
Mao X., Qian X., Lin J., Wei D. (2023). Engineering Gluconobacter oxydans for efficient production of 3,4-dihydroxybutunate or 1,2,4-butanetriol from D-xylose. Biochem. Eng. J. 195, 108936. doi: 10.1016/j.bej.2023.108936
Martínez-Avila O., Sánchez A., Font X., Barrena R. (2018). Bioprocesses for 2-phenylethanol and 2-phenylethyl acetate production: current state and perspectives. Appl. Microbiol. Biotechnol. 102, 9991–10004. doi: 10.1007/s00253-018-9384-8
Maximize Market Research. (2023).2,3-Butanediol Market – Global industry analysis and forecast (2023-2029); 65350
Meng J., Chroumpi T., Mäkelä M. R., de Vries R. P. (2022). Xylitol production from plant biomass by Aspergillus Niger through metabolic engineering. Bioresour. Technol. 344, 126199. doi: 10.1016/j.biortech.2021.126199
Mountraki A. D., Koutsospyros K. R., Mlayah B. B., Kokossis A. C. (2017). Selection of biorefinery routes: The case of xylitol and its integration with an organosolv process. Waste Biomass Valorization 8, 2283–2300. doi: 10.1007/s12649-016-9814-8
Narisetty V., Amraoui Y., Abdullah A., Ahmad E., Agrawal D., Parameswaran B., et al. (2021a). High yield recovery of 2,3-butanediol from fermented broth accumulated on xylose rich sugarcane bagasse hydrolysate using aqueous two-phase extraction system. Bioresour. Technol. 337, 125463. doi: 10.1016/j.biortech.2021.125463
Narisetty V., Castro E., Durgapal S., Coulon F., Jacob S. (2021b). High level xylitol production by Pichia fermentans using non-detoxified xylose-rich sugarcane bagasse and olive pits hydrolysates. Bioresour. Technol. 342, 126005. doi: 10.1016/j.biortech.2021.126005
Nouri H., Moghimi H., Marashi S. A., Elahi E. (2020). Impact of hfq and sigE on the tolerance of Zymomonas mobilis ZM4 to furfural and acetic acid stresses. PloS One 15, e0240330. doi: 10.1371/journal.pone.0240330
Okonkwo C. C., Ujor V., Ezeji T. C. (2021). Production of 2,3-Butanediol from non-detoxified wheat straw hydrolysate: Impact of microbial inhibitors on Paenibacillus polymyxa DSM 365. Ind. Crops Prod. 159, 113047. doi: 10.1016/j.indcrop.2020.113047
Ong K. L., Li C., Li X., Zhang Y., Xu J., Lin C. S. K. (2019). Co-fermentation of glucose and xylose from sugarcane bagasse into succinic acid by Yarrowia lipolytica. Biochem. Eng. J. 148, 108–115. doi: 10.1016/j.bej.2019.05.004
Pasaye-Anaya L., Vargas-Tah A., Martínez-Cámara C., Castro-Montoya A. J., Campos-García J. (2019). Production of 2,3-butanediol by fermentation of enzymatic hydrolysed bagasse from agave mezcal-waste using the native Klebsiella oxytoca UM2-17 strain. J. Chem. Technol. Biotechnol. 94, 3915–3923. doi: 10.1002/jctb.6190
Pontes R., Romaní A., Michelin M., Domingues L. (2021). L-lactic acid production from multi-supply autohydrolyzed economically unexploited lignocellulosic biomass. Ind. Crops Prod. 170, 113775. doi: 10.1016/j.indcrop.2021.113775
Prabhu A. A., Bosakornranut E., Amraoui Y., Agrawal D., Coulon F., Vivekanand V., et al. (2020a). Enhanced xylitol production using non−detoxified xylose rich pre−hydrolysate from sugarcane bagasse by newly isolated Pichia fermentans. Biotechnol. Biofuels 13, 209. doi: 10.1186/s13068-020-01845-2
Prabhu A. A., Ledesma-Amaro R., Lin C. S. K., Coulon F., Thakur V. K., Kumar V. (2020b). Bioproduction of succinic acid from xylose by engineered Yarrowia lipolytica without pH control. Biotechnol. Biofuels 13. doi: 10.1186/s13068-020-01747-3
Promdonkoy P., Siripong W., Downes J. J., Tanapongpipat S., Runguphan W. (2019). Systematic improvement of isobutanol production from D-xylose in engineered Saccharomyces cerevisiae. AMB Express 9, 160. doi: 10.1186/s13568-019-0885-3
Qiu Z., Fang C., Gao Q., Bao J. (2020a). A short-chain dehydrogenase plays a key role in cellulosic D-lactic acid fermentability of Pediococcus acidilactici. Bioresour. Technol. 297, 122473. doi: 10.1016/j.biortech.2019.122473
Qiu Z., Fang C., He N., Bao J. (2020b). An oxidoreductase gene ZMO1116 enhances the p-benzoquinone biodegradation and chiral lactic acid fermentability of Pediococcus acidilactici. J. Biotechnol. 323, 231–237. doi: 10.1016/j.jbiotec.2020.08.015
Qiu Z., Gao Q., Bao J. (2018). Engineering Pediococcus acidilactici with xylose assimilation pathway for high titer cellulosic L-lactic acid fermentation. Bioresour. Technol. 249, 9–15. doi: 10.1016/j.biortech.2017.09.117
Qiu Z., Han X., Fu A., Jiang Y., Zhang W., Jin C., et al. (2023b). Enhanced cellulosic D-lactic acid production from sugarcane bagasse by pre-fermentation of water-soluble carbohydrates before acid pretreatment. Bioresour. Technol. 368, 128324. doi: 10.1016/j.biortech.2022.128324
Qiu Z., Han X., He J., Jiang Y., Wang G., Wang Z., et al. (2022). One-pot D-lactic acid production using undetoxified acid-pretreated corncob slurry by an adapted Pediococcus acidilactici. Bioresour. Technol. 363, 127993. doi: 10.1016/j.biortech.2022.127993
Qiu Y., Qiu Z., He A., Xu J., Yang X., Zhang H., et al. (2023a). A novel strategy for enhancing inhibitor tolerance of gram-positive bacteria through overexpression of the peptidoglycan synthesis genes murG and mraY. Ind. Crops Prod. 192, 116112. doi: 10.1016/j.indcrop.2022.116112
Qu C., Dai K., Fu H., Wang J. (2021). Enhanced ethanol production from lignocellulosic hydrolysates by Thermoanaerobacterium aotearoense SCUT27/ΔargR1864 with improved lignocellulose-derived inhibitors tolerance. Renew. Energy 173, 652–661. doi: 10.1016/j.renene.2021.04.018
Ramakrishnan G., Dhandapani B., Krishnamoorthy S., Dhithya V., Palaniyappan H. (2020). Next-generation itaconic acid production using novel Aspergillus japonicas from Citrullus lanatus rind through solid-state fermentation. Bioresour. Technol. Rep. 11, 100544. doi: 10.1016/j.biteb.2020.100544
Ramzi A. B., Minggu M. M., Ruslan U. S., Fikri Khairi M. H., Abdul P. M. (2022). Expression of furfural reductase improved furfural tolerance in antarctic bacterium Pseudomonas extremaustralis. Sains Malaysiana 51, 3163–3170. doi: 10.17576/jsm-2022-5110-04
Ran Y., Yang Q., Zeng J., Li F., Cao Y., Xu Q., et al. (2023). Potential xylose transporters regulated by CreA improved lipid yield and furfural tolerance in oleaginous yeast Saitozyma podzolica zwy-2-3. Bioresour. Technol. 386, 129413. doi: 10.1016/j.biortech.2023.129413
Raj K., Krishnan C. (2020). Improved co-production of ethanol and xylitol from low-temperature aqueous ammonia pretreated sugarcane bagasse using two-stage high solids enzymatic hydrolysis and Candida tropicalis. Renew. Energy 153, 392–403. doi: 10.1016/j.renene.2020.02.042
Rehman S., Khairul Islam M., Khalid Khanzada N., Kyoungjin An A., Chaiprapat S., Leu S. Y. (2021). Whole sugar 2,3-butanediol fermentation for oil palm empty fruit bunches biorefinery by a newly isolated Klebsiella pneumoniae PM2. Bioresour. Technol. 333, 125206. doi: 10.1016/j.biortech.2021.125206
Robertiello A., Romano I., Ventorino V., Faraco V., Pepe O. (2023). Enhancing succinic acid production by sequential adaptation of selected Basfia succiniciproducens strains to Arundo donax hydrolysate. Fermentation 9, 573. doi: 10.3390/fermentation9060573
Saha B. C., Kennedy G. J., Bowman M. J., Qureshi N., Nichols N. N. (2022). Itaconic acid production by Aspergillus terreus from glucose up to pilot scale and from corn stover and wheat straw hydrolysates using new manganese tolerant medium. Biocatal. Agric. Biotechnol. 43, 102418. doi: 10.1016/j.bcab.2022.102418
Saratale R. G., Shin H. S., Ghodake G. S., Kumar G., Oh M. K., Saratale G. D. (2018). Combined effect of inorganic salts with calcium peroxide pretreatment for kenaf core biomass and their utilization for 2,3-butanediol production. Bioresour. Technol. 258, 26–32. doi: 10.1016/j.biortech.2018.02.115
Shaji A., Shastri Y., Kumar V., Ranade V. V., Hindle N. (2022). Sugarcane bagasse valorization to xylitol: Techno-economic and life cycle assessment. Biofuels Bioprod. Biorefining 16, 1214–1226. doi: 10.1002/bbb.2368
Singh S., Kaur D., Yadav S. K., Krishania M. (2021). Process scale-up of an efficient acid-catalyzed steam pretreatment of rice straw for xylitol production by C. Tropicalis MTCC 6192. Bioresour. Technol. 320, 124422–403. doi: 10.1016/j.biortech.2020.124422
Singh N., Rani R., Nigam P. S., Dong C., Patel K., Puri M. (2022). Global status of lignocellulosic biorefinery: Challenges and perspectives. Bioresour. Technol. 344, 126415. doi: 10.1016/j.biortech.2021.126415
Sivagurunathan P., Raj T., Singh P., Kumari P., Satlewal A., Prakash R., et al. (2022). High-titer lactic acid production from pilot-scale pretreated non-detoxified rice straw hydrolysate at high-solid loading. Biochem. Eng. J. 187, 108668. doi: 10.1016/j.bej.2022.108668
Suo Y., Liao Z., Qu C., Fu H., Wang J. (2019). Metabolic engineering of Clostridium tyrobutyricum for enhanced butyric acid production from undetoxified corncob acid hydrolysate. Bioresour. Technol. 271, 266–273. doi: 10.1016/j.biortech.2018.09.095
Svetlitchnyi V. A., Svetlichnaya T. P., Falkenhan D. A., Swinnen S., Knopp D. (2022). Direct conversion of cellulose to L−lactic acid by a novel thermophilic Caldicellulosiruptor strain. Biotechnol. Biofuels Bioprod. 15, 44. doi: 10.1186/s13068-022-02137-7
Tran P.H. N., Ko J. K., Gong G., Um Y., Lee S. M. (2020). Improved simultaneous co-fermentation of glucose and xylose by Saccharomyces cerevisiae for efficient lignocellulosic biorefinery. Biotechnol. Biofuels 13, 12. doi: 10.1186/s13068-019-1641-2
Vallecilla-Yepez L., Ramchandran D., Long D., Saha R., Wilkins M. R. (2021). Corn fiber as a biomass feedstock for production of succinic acid. Bioresour. Technol. Rep. 16, 100868. doi: 10.1016/j.biteb.2021.100868
View Market Research Report. (2023a). Bio-succinic acid market by process type (ammonium sulphate process, direct crystallization process, electrodialysis process), application (1, 4 butanediol, plasticizers, polybutylene succinate), Global forecast 2023-2030; MRR-1221 437D459587F3.
View Market Research Report. (2023b). Lactic acid market by form (dry, liquid), application (biodegradable polymers, food & beverages, personal care products) - Global forecast 2023-2030; MRR-50124643844C.
View Market Research Report. (2023c). Xylitol aarket by form (liquid, powder), application (cosmetics, food & beverages, personal care) - Global forecast 2023-2030; MRR-1227 C002B1C9969C.
View Market Research Report. (2023d). Itaconic acid market by derivative (methyl methacrylate, polyitaconic acid, styrene butadiene itaconic acid), application (chillant dispersant agent, SBR Latex, superabsorbent polymer) - Global forecast 2023-2030; MRR-8A35583B11C2.
View Market Research Report. (2023e). Farnesene market by type (α-farnesene, β-farnesene), application (adhesives & resins, cosmetics & personal care, crop protection) - Global forecast 2023-2030; MRR-AD517FAA7D08 1234
View Market Research Report. (2023f). Isoprene market by product (isobutylene isoprene rubber, polyisoprene, styrene isoprene styrene), grade (chemical, polymer), application - Global forecast 2023-2030|; MRR-C002B1C9950D.
Wang H., Li Q., Zhang Z., Ayepa E., Xiang Q., Yu X., et al. (2023). Discovery of new strains for furfural degradation using adaptive laboratory evolution in Saccharomyces cerevisiae. J. Hazard. Mater. 459, 132090. doi: 10.1016/j.jhazmat.2023.132090
Wang S., Wang Z., Wang Y., Nie Q., Yi X., Ge W., et al. (2017). Production of isoprene, one of the high-density fuel precursors, from peanut hull using the high-efficient lignin-removal pretreatment method. Biotechnol. Biofuels 10, 297. doi: 10.1186/s13068-017-0988-5
Wang X., Xu N., Hu S., Yang J., Gao Q., Xu S., et al. (2018). D-1,2,4-butanetriol production from renewable biomass with optimization of synthetic pathway in engineered Escherichia coli. Bioresour. Technol. 250, 406–412. doi: 10.1016/j.biortech.2017.11.062
Wang J., Zeng A. P., Yuan W. (2022a). Succinic acid fermentation from agricultural wastes: The producing microorganisms and their engineering strategies. Curr. Opin. Environ. Sci. Heal. 25, 100313. doi: 10.1016/j.coesh.2021.100313
Wang L. R., Zhang Z. X., Nong F. T., Li J., Huang P. W., Ma W., et al. (2022b). Engineering the xylose metabolism in Schizochytrium sp. to improve the utilization of lignocellulose. Biotechnol. Biofuels Bioprod. 15, 114. doi: 10.1186/s13068-022-02215-w
Willson B. J., Herman R., Langer S., Thomas G. H. (2022). Improved furfural tolerance in Escherichia coli mediated by heterologous NADH-dependent benzyl alcohol dehydrogenases. Biochem. J. 479, 1045–1058. doi: 10.1042/BCJ20210811
Wu X., Liu Q., Deng Y., Li J., Chen X., Gu Y., et al. (2017). Production of itaconic acid by biotransformation of wheat bran hydrolysate with Aspergillus terreus CICC40205 mutant. Bioresour. Technol. 241, 25–34. doi: 10.1016/j.biortech.2017.05.080
Wu J., Zhou Y. J., Zhang W., Cheng K. K., Liu H. J., Zhang J. A. (2019). Screening of a highly inhibitor-tolerant bacterial strain for 2,3-BDO and organic acid production from non-detoxified corncob acid hydrolysate. AMB Express 9, 1–9. doi: 10.1186/s13568-019-0879-1
Xu Y., Chi P., Bilal M., Cheng H. (2019). Biosynthetic strategies to produce xylitol: an economical venture. Appl. Microbiol. Biotechnol. 103, 5143–5160. doi: 10.1007/s00253-019-09881-1
Xu H., Li Z., Li L., Xie X., Cai D., Wang Z., et al. (2023). Sustainable production of 2-phenylethanol from agro-industrial wastes by metabolically engineered Bacillus licheniformis. LWT-Food Sci. Technol. 173, 114414. doi: 10.1016/j.lwt.2022.114414
Yan Z., Zhang J., Bao J. (2021). Increasing cellulosic ethanol production by enhancing phenolic tolerance of Zymomonas mobilis in adaptive evolution. Bioresour. Technol. 329, 124926. doi: 10.1016/j.biortech.2021.124926
Yang Y., Wang Y., Lu X., Zheng X., Yan D., Xin J., et al. (2022). Highly efficient enzymolysis and fermentation of corn stalk into L-lactic acid by enzyme-bacteria friendly ionic liquid pretreatment. Green Chem. Eng. 3, 321–327. doi: 10.1016/j.gce.2021.11.003
Yang J., Xu H., Jiang J., Zhang N., Xie J., Zhao J., et al. (2020). Itaconic acid production from undetoxified enzymatic hydrolysate of bamboo residues using Aspergillus terreus. Bioresour. Technol. 307, 123208. doi: 10.1016/j.biortech.2020.123208
Yi X., Lin L., Mei J., Wang W. (2021). Transporter proteins in Zymomonas mobilis contribute to the tolerance of lignocellulose-derived phenolic aldehyde inhibitors. Bioprocess Biosyst. Eng. 44, 1875–1882. doi: 10.1007/s00449-021-02567-x
Yin F., Sun X., Zheng W., Yin L., Luo X., Zhang Y., et al. (2023). Development of a strategy for L-lactic acid production by Rhizopus oryzae using Zizania latifolia waste and cane molasses as carbon sources. Molecules 28 6234. doi: 10.3390/molecules28176234
Yook S., Kim J., Gong G., Ko J. K., Um Y., Han S. O., et al. (2020). High-yield lipid production from lignocellulosic biomass using engineered xylose-utilizing Yarrowia lipolytica. GCB Bioenergy 12, 670–679. doi: 10.1111/gcbb.12699
You S., Chang H., Zhang C., Gao L., Qi W., Tao Z., et al. (2019). Recycling strategy and repression elimination for lignocellulosic-based farnesene production with an engineered Escherichia coli. J. Agric. Food Chem. 67, 9858–9867. doi: 10.1021/acs.jafc.9b03907
Yuan X., Cao J., Wang R., Han Y., Zhu J., Lin J., et al. (2023). Genetically engineering Escherichia coli to produce xylitol from corncob hydrolysate without lime detoxification. Molecules 28, 1550. doi: 10.3390/molecules28041550
Yuan X., Mao Y., Tu S., Lin J., Shen H., Yang L., et al. (2021). Increasing NADPH availability for xylitol production via pentose phosphate pathway gene vverexpression and Embden Meyerhof Parnas pathway gene deletion in Escherichia coli. J. Agric. Food Chem. 69, 9625–9631. doi: 10.1021/acs.jafc.1c03283
Yuan X., Tu S., Lin J., Yang L., Shen H., Wu M. (2020). Combination of the CRP mutation and ptsG deletion in Escherichia coli to efficiently synthesize xylitol from corncob hydrolysates. Appl. Microbiol. Biotechnol. 104, 2039–2050. doi: 10.1007/s00253-019-10324-0
Yuan X., Wang J., Lin J., Yang L., Wu M. (2019). Efficient production of xylitol by the integration of multiple copies of xylose reductase gene and the deletion of Embden–Meyerhof–Parnas pathway-associated genes to enhance NADPH regeneration in Escherichia coli. J. Ind. Microbiol. Biotechnol. 46, 1061–1069. doi: 10.1007/s10295-019-02169-3
Zan X., Sun J., Chu L., Cui F., Huo S., Song Y., et al. (2021). Improved glucose and xylose co-utilization by overexpression of xylose isomerase and/or xylulokinase genes in oleaginous fungus Mucor circinelloides. Appl. Microbiol. Biotechnol. 105, 5565–5575. doi: 10.1007/s00253-021-11392-x
Zang G., Shah A., Wan C. (2020). Techno-economic analysis of co-production of 2,3-butanediol, furfural, and technical lignin via biomass processing based on deep eutectic solvent pretreatment. Biofuels Bioprod. Biorefining 14, 326–343. doi: 10.1002/bbb.2081
Zevallos Torres L. A., Lorenci Woiciechowski A., Nishida V. S., Valladares-Diestra K.K., de Souza Vandenberghe L. P., Zandona Filho A., et al. (2023). Imidazole pretreatment of oil palm empty fruit bunches for ethanol and succinic acid co-production by Saccharomyces cerevisiae and Pichia stipitis. Bioenerg. Res. 16, 990–1000. doi: 10.1007/s12155-022-10512-3
Zhang J., Xu T., Wang X., Jing X., Zhang J., Hong J., et al. (2022). Lignocellulosic xylitol production from corncob using engineered Kluyveromyces marxianus. Front. Bioeng. Biotechnol. 10. doi: 10.3389/fbioe.2022.1029203
Zhao C., Chen S., Fang H. (2018). Consolidated bioprocessing of lignocellulosic biomass to itaconic acid by metabolically engineering Neurospora crassa. Appl. Microbiol. Biotechnol. 102, 9577–9584. doi: 10.1007/s00253-018-9362-1
Zhao M., Shi D., Lu X., Zong H., Zhuge B. (2019). Co-production of 1,2,4-butantriol and ethanol from lignocellulose hydrolysates. Bioresour. Technol. 282, 433–438. doi: 10.1016/j.biortech.2019.03.057
Zhao P., Tian P. (2021). Biosynthesis pathways and strategies for improving 3-hydroxypropionic acid production in bacteria. World J. Microbiol. Biotechnol. 37, 117. doi: 10.1007/s11274-021-03091-6
Zhu F., Wang C., San K. Y., Bennett G. N. (2020). Metabolic engineering of Escherichia coli to produce succinate from woody hydrolysate under anaerobic conditions. J. Ind. Microbiol. Biotechnol. 47, 223–232. doi: 10.1007/s10295-020-02259-7
Zhu N., Xia W., Wang G., Song Y., Gao X., Liang J., et al. (2023). Engineering Corynebacterium glutamicum for de novo production of 2-phenylethanol from lignocellulosic biomass hydrolysate. Biotechnol. Biofuels Bioprod. 16, 75. doi: 10.1186/s13068-023-02327-x
Zou L., Jin X., Tao Y., Zheng Z., Ouyang J. (2022). Unraveling the mechanism of furfural tolerance in engineered Pseudomonas putida by genomics. Front. Microbiol. 13. doi: 10.3389/fmicb.2022.1035263
Zou L., Ouyang S., Hu Y., Zheng Z., Ouyang J. (2021). Efficient lactic acid production from dilute acid-pretreated lignocellulosic biomass by a synthetic consortium of engineered Pseudomonas putida and Bacillus coagulans. Biotechnol. Biofuels 14, 227. doi: 10.1186/s13068-021-02078-7
Keywords: lignocellulose biomass, chemicals, metabolic engineering, microbial cell factories, techno-economic
Citation: Lama S, Thapa LP, Upadhayaya SK, Gauchan DP and Singh A (2024) Metabolic engineering in lignocellulose biorefining for high-value chemicals: recent advances, challenges, and outlook for enabling a bioeconomy. Front. Ind. Microbiol. 1:1319774. doi: 10.3389/finmi.2023.1319774
Received: 11 October 2023; Accepted: 20 December 2023;
Published: 18 January 2024.
Edited by:
Stephen R. Decker, National Renewable Energy Laboratory (DOE), United StatesReviewed by:
Hui Wei, National Renewable Energy Laboratory (DOE), United StatesCopyright © 2024 Lama, Thapa, Upadhayaya, Gauchan and Singh. This is an open-access article distributed under the terms of the Creative Commons Attribution License (CC BY). The use, distribution or reproduction in other forums is permitted, provided the original author(s) and the copyright owner(s) are credited and that the original publication in this journal is cited, in accordance with accepted academic practice. No use, distribution or reproduction is permitted which does not comply with these terms.
*Correspondence: Suman Lama, ZGpwbHN1bWFuQGdtYWlsLmNvbQ==
Disclaimer: All claims expressed in this article are solely those of the authors and do not necessarily represent those of their affiliated organizations, or those of the publisher, the editors and the reviewers. Any product that may be evaluated in this article or claim that may be made by its manufacturer is not guaranteed or endorsed by the publisher.
Research integrity at Frontiers
Learn more about the work of our research integrity team to safeguard the quality of each article we publish.