- 1Biosciences Center, National Renewable Energy Laboratory, Golden, CO, United States
- 2Bioenergy Science and Technology, National Renewable Energy Laboratory, Golden, CO, United States
The field of industrial microbiology has great potential to tackle the most pressing challenges facing society, but today, this entails much more than classical fermentation technology followed by product upgrading. Methods are needed to confer unit-operation-level functionality and whole-system-level robustness, as well as flexibility. As examples of this concept, we focus here on methodologies often deployed at industrial scale as discrete processes—such as biological, chemical, and electrochemical unit operations—that are also capable of substantial complementarity when combined sequentially and operated continuously. A key and relatively new type of conversion step is made possible by catalyst immobilization methods at both the enzymatic and cellular level. Cases drawn from electro-fermentation for acetoin production and syngas-mediated microbial electrosynthesis of ethanol exemplify the synergy of combined operational approaches. Because modular processes can be treated as a series of reactor elements, considerable flexibility is possible. As discussed, however, not all processes are amenable to this intensification.
1 Introduction
Industrial microbiology and fermentation play a crucial role in the production of chemicals and fuels, enabling the generation of high-value products such as biofuels, biodegradable plastics, enzymes, pharmaceuticals, and food additives. In this rapidly changing world, industrial microbiology is facing a range of major challenges, including economic pressures that require the development of more sustainable and efficient production processes, dealing with the environmental impact of climate change on microbial systems, and addressing the ethical and regulatory compliance issues surrounding the use of genetically modified organisms. Among these challenges, the economic pressures of industrial microbiology are significant. The cost of research and development is high, and competition is ever present. Industrial microbiologists must seek new ways to reduce costs and increase efficiency while maintaining quality.
Conventional approaches to industrial microbiology often rely on either a single unit operation or a limited set of unit operations, which can limit the efficiency and yield of the production process. To meet the challenges of reducing costs and increasing efficiency in industrial microbiology, a multimodal strategy of combing biological, chemical, and electrochemical unit operations can be utilized to maximize the yield and quality of the desired product. We explore the idea of combining continuous biological, chemical, and electrochemical unit operations sequentially in industrial microbiology to produce high-value products.
2 Design and deployment of continuous multimodal processes
As shown in Figure 1 (upper inset), the common unit equipment today consists of stirred tank fermenters/chemical reactors, microfiltration/ultrafiltration systems, and flow reactors charged with solid chemical catalysts or immobilized enzymes (e.g., xylose isomerase). These systems are designed for batch or semi-continuous operation, often requiring separate concentration/dilution steps, as well as temporary storage of the intermediates. One can envision adaptation, in many cases, to a fully continuous operation. For example, the initial chemical feedstocks are often produced via classical submerged cultures of fungi, yeast, or bacteria. These cultures can be operated in a continuous fed-batch mode or use a “culture train” of stirred tank fermenters, where the chemical feedstock is produced in semi-continuous mode. A continuous stream can be generated using a “surge tank” facility. Usually, products in spent microbial growth media are dilute. This challenge can be overcome using continuous ultrafiltration/microfiltration systems. Ultrafiltration systems are often used in the single-stage mode to concentrate macromolecules and/or chemicals from water. Reverse osmosis is the analogous process that produces clean water as the permeate (e.g., approximately 1-Å porosity). Using hydrolysis of biomass by biomass-degrading enzymes as an example, in the two-stage mode, ultrafiltration/microfiltration systems can compartmentalize milled biomass combined with biomass-degrading enzymes separately from the products of hydrolysis, monosaccharides. For such systems, the ultrafiltration membrane pore sizes should correspond to 10–30 kDa, or about 0.002 microns. As mentioned, these sugars could then be concentrated using a reverse-osmosis-type second stage.
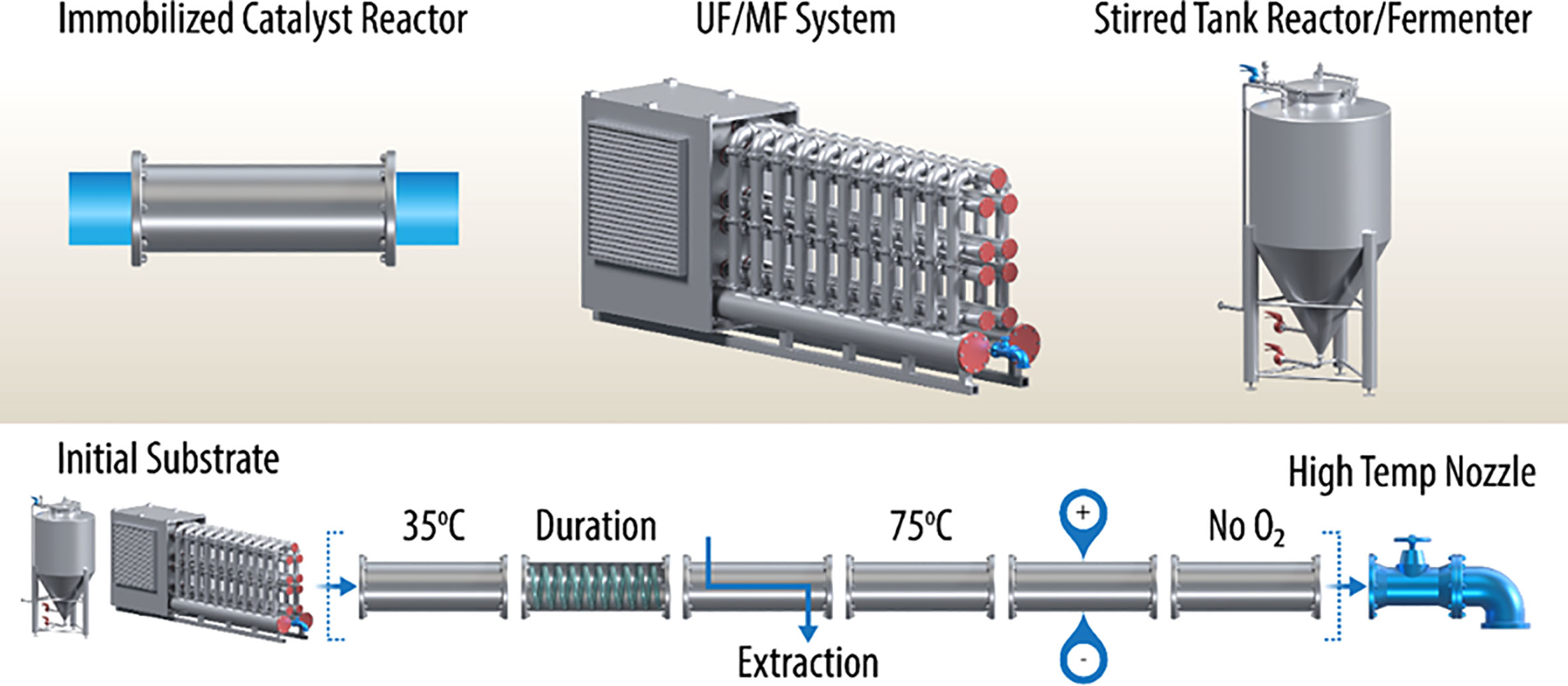
Figure 1 Continuous multimodal processing permits the possibility of (1) a high number of conversion steps, (2) segments that can be optimized independently, (3) combinations of biochemical and chemical catalytic segments, (4) extension of reaction duration with long-residence-time segments, (5) extraction using non-miscible solvents and membranes, (6) redox segments capable of electron injection, and (7) high-temperature nozzle for control of polymerization or sterility of final product. However, all segments must be operated at the same volumetric flow rate, which may be challenging. Additionally, wherever possible, free space for mixing should be avoided, using Raschig rings or similar inert inserts in appropriate segments for example, to maintain an approximation of plug flow.
Subsequent process steps can follow some or all of the examples shown in Figure 1; however, these flow reactors must be carefully designed to permit optimum reaction conditions, plug flow fluid dynamics, and containment. Examples of immobilized enzyme and chemical catalyst reactors are well known, but linking these stages has not been demonstrated often. One concept shown here is the use of a flow reactor segment with an internal tubing raceway, which effectively extends the residence time in this segment. There may be other ways of doing this (e.g., using a large volume mixing tank), but this would destroy the plug flow nature of the proposed continuous modular process. Finally, process finishing segments can be considered to produce a pasteurized product or a polymerized resin, for example. Other likely final steps include further dewatering, stabilization with chemical adjuvants, and packaging.
3 Biological, chemical, and electrochemical unit operations
3.1 Biological unit segments
Examples of biological unit operations include fermentation and bioreactor processing, where microorganisms are used to convert substrate(s) into desired products such as ethanol, butanol, lactic acid, and many others. Biological unit operations have the advantage of being highly specific and selective, which can lead to high product purity. Traditionally, fermentations using native microorganisms have met many product goals in food, fuels, and pharmaceutical industries. With the advent of effective cloning tools such as CRISPR-Cas9 and heterologous enzymes, entire metabolic pathways can be efficiently introduced into so-called “expression chassis,” allowing new industries to emerge. Typically, fermentation technology is done in batch mode (Li et al., 2014), although some examples of successful continuous fermentation methods have been reported as elaborated in later sections. In continuous fermentation, the cells must be retained in the primary fermenter as the products are introduced and recovered simultaneously. Depending on the system at hand, this can be very challenging at large scale. Industrial-scale biological processing began with simple stirred tank monoculture fermentations, and this format is still effective for many processes.
Another example of a relatively new continuous biological processing method is cell-free reactors, which use immobilized enzymes. Immobilization can be conferred by direct covalent crosslinking to reactor packing materials, entrapment in gels, or even confinement in lysosomes. In all cases that are successful, the enzymes must be retained in a system that does not impair their ability to remain functional (e.g., sites of product entry and release and cofactor binding remain accessible). Many immobilized metabolic-type enzymes require electron cycling via cofactors or mediator molecules, and this has proven to be challenging in a flow reactor environment. Examples of successful application at industrial scale for immobilized enzymes include the fructose from glucose production industry.
Intact cells can also be immobilized. In fact, immobilized yeast cells have been maintained in steady-state culture for years at the lab scale (Moreno-García et al., 2018). Immobilization of cells has some advantages over immobilization of enzymes. Briefly, (1) metabolic pathway enzymes are not intended for exposure to environments beyond the cytosol, and (2) it is difficult to ensure the spatial arrangement of immobilized enzymes to represent a pathway. Compared to the local organization afforded by some organelles (eukaryotes) and cytosol-facing cell membranes (prokaryotes), the presumption is that cofactor charging will continue to function in the cellular environment upon cell immobilization.
Finally, the immobilization of enzymes and cells permits a new concept for complex processing schemes, that of sequentially placing segmented reactors capable of disparate unit conversions (Figure 1).
3.2 Chemical unit segments
Chemical unit operations use chemical reactions to convert substrates to desired products. In the context of industrial microbiology, chemical unit operations can refer to processing ranging from upstream to downstream of biological unit operations. Examples in upstream processing could be pretreatments used to modify the substrate to make it more suitable for microbial processing, whereas downstream processing chemical unit operations refer primarily to “upgrading” of the microbial product (i.e., to chemically modify the microbial product to the finished product or another intermediate). For example, chemical unit operations such as hydrolysis, esterification, dehydrogenation, hydrogenation, and transesterification are commonly used to modify substrates and produce chemicals and fuels, such as biodiesel (Mandari and Devarai, 2021).
It is noteworthy that chemical unit operations often have a lower intrinsic variability compared with biological processes (Becker et al., 2023). In addition, chemical unit operations have the advantage of being fast, highly flexible, and adaptable. Therefore, integrating chemical unit operations upstream and/or downstream can be a feasible approach to enhance performance in industrial microbiology. However, the possible deleterious effects that residual solvents or byproducts of the chemical reaction must be weighed for continuous operation. An example is the very high toxicity of some commonly used organic solvents (Gullón et al., 2020).
3.3 Electrochemical unit segments
Electrochemical unit operations involve the use of electrical energy to drive chemical reactions and have the advantage of being highly efficient and selective. For example, the conversion of bio-oil obtained from lignocellulosic biomass into usable fuels or chemicals demands energy-intensive refining. Traditional methods such as hydrodeoxygenation are conducted under high temperatures (200°–400°C) and high pressure (200 bar) of exogenous hydrogen. In contrast, electrochemical hydrogenation functions at lower temperatures (<80°C) and ambient pressure, with no need for added hydrogen (Page et al., 2023). It is also possible that direct injection of electrons into immobilized cell and enzyme reactors may enhance energy charge and thus relieve some of the dependency on molecular cofactors (Schlager et al., 2016). Many practical questions remain to be answered in this regard, such as the best way of delivering electrons to a reactor filled with aqueous media. Should one use DC or AC power? AC power may be useful for diminishing the biological fouling of reactor electrodes (Mecheri et al., 2018). And what are the best configurations for reactor electrodes? As always, this technology also signals safety concerns for the operators as well.
3.4 Special unit segments
We have proposed several segments of special interest, such as a reaction residence time extension segment and an organic solvent segment (membrane-assisted liquid extraction). The latter is useful for impurity removal. Long removed from the biological segments, downstream product upgrading can deploy high temperature (with back-pressure control) for purposes of final reaction setting (i.e., resin polymerization) or pasteurization. Certainly, other special processing configurations will be proposed as adaptation of continuous processing to new and existing manufacturing processes is contemplated.
4 Multimodality from combining biological, chemical, and electrochemical unit operations
As described above, each unit operation has complementary strengths in its design and construction, which makes it readily evident that there is substantial potential for synergy in the integrated application of these unit operations. In general, the use of biological unit operations can allow for the selective production of a desired product or complex molecules, whereas chemical and electrochemical unit operations can be used to separate and modify the molecule as needed (Salvachua et al., 2021). Utilizing multiple unit operations can facilitate the enhancement of individual stages in the entire process, leading to increased efficiency and output.
5 Continuous integrated manufacturing: from concepts in biopharmaceutical industry to emerging implementation in industrial microbiology
Continuous integrated processing is gaining traction in the biopharmaceutical industry, with major manufacturers worldwide developing continuous process platforms. The integration of continuous unit operations, such as spray drying, dry blending, granulation, and extrusion, has the potential to revolutionize synthesis processes (Rogers and Jensen, 2019). However, even in the biopharmaceutical industry, such a holistic approach to continuous manufacturing requires overcoming logistical challenges and legacy (i.e., outdated) infrastructure, as well as process integration challenges (e.g., establishing control systems and process monitoring strategies), ambiguous regulatory guidelines, and a shift toward novel therapies. The true impact of continuous manufacturing in the biopharmaceutical industry will be most pronounced when manufacturing costs represent a significant portion of product development expenses, as elaborated in two recent review papers (Rogers and Jensen, 2019; Rathore et al., 2023).
To ensure process robustness and product quality, continuous manufacturing in the biopharmaceutical industry needs to consider process understanding, real-time monitoring and control, and release testing. Furthermore, implementing increased levels of controls and incorporating these functionalities into the control strategy will also be crucial to ensure process performance and product quality (Rogers and Jensen, 2019).
In contrast, continuous integrated processing may have arguably gained even more traction and made significantly more progress in industrial microbiology than in the biopharmaceutical industry, partially due to relatively more relaxed regulatory guidelines and easier regulatory approval. Thus, the cases for the continuous processing reviewed herein in industrial microbiology are the unique examples of such technology being systematically applied today.
6 Syngas and microbial electrosynthesis (MES)-oriented multimodality in unit operations
Syngas, a mixture of hydrogen and carbon monoxide, is a promising substrate to produce chemicals and fuels, including ethanol. It can be efficiently produced via reverse water–gas shift and water electrolysis (Badgett et al., 2021). In addition, technologies such as solar-driven electrolysis (Schmidt et al., 2017), supercritical water (scH2O) gasification (Okolie et al., 2019), and biomass pyrolysis-gasification (Dou et al., 2019) further enable the sustainable production of molecular hydrogen (H2). Together, these technologies will undoubtedly enable the utilization of the reducing power of H2 for the in vivo biosynthesis of chemicals and facilitate downstream scale-up (Wei et al., 2023). In this context, the conversion of syngas to acetate, ethanol, and other chemicals has been an active area of research in the past several decades.
MES is a promising approach that employs microbial cells to transform CO2 into valuable chemicals by utilizing electrons supplied to microbes either directly by an electrode or indirectly via mediators, such as H2 (Claassens et al., 2019). This technique holds great potential as a green and ecologically favorable method for fixing CO2, as microbes are self-replicating and have endogenous pathways to catalyze reductive CO2 conversion (Zhu et al., 2022).
Nevertheless, the industrial adoption of MES is hampered by numerous bottlenecks, such as low carbon sequestration efficiency and low titers of products. In addition to synthetic biology engineering, other approaches such as flexible stack designs with flat or tubular MES modules and new anodic materials have also been explored to boost MES cost-effectiveness (Dessì et al., 2021).
Advances in continuous, sustainable generation of syngas and the boost of MES cost-effectiveness have also provided new impetus and momentum to implement multimodality strategies for the conversion of syngas to acetate, ethanol, and other chemicals. Recent studies employing multimodal approaches, as described in below sections, reveal a variety of strategies for utilizing a combination of engineering methods to accomplish a range of objectives that were otherwise unattainable.
7 Multimodality in industrial unit operations
The following examples of multimodality may benefit from continuous processing (listed in Table 1), given the proper economic and market share incentives.
7.1 Case 1. Anode-assisted electro-fermentation for acetoin production
While traditional fermentation processes rely on microbial activity for the conversion of organic matter into high-value products, electro-fermentation involves the use of electrodes to drive microbial metabolism, which emerged as a promising alternative to conventional fermentation. Anode-assisted electro-fermentation has been recently used to optimize industrial aerobic bioprocesses for boosting acetoin production by Bacillus subtilis, with a yield of 0.78 ± 0.04 mol product/mol glucose, which is twice as high as without anode potential. Limited air/oxygen supply enables the bacterial cells to donate excess electrons to the anode, thereby leading to steered carbon flux (Sun et al., 2023).
7.2 Case 2. Anode-assisted electro-fermentation for rhamnolipid production
Rhamnolipid production by engineered P. putida KT2440 under aerobic conditions was challenged by costly aeration and the subsequent reactor foaming (Tiso et al., 2016; Tiso et al., 2017). To solve this issue, Askitosari and coworkers used anodic electro-fermentation to reduce the oxygen demand of aerobic bioprocesses by enhancing the production of glycolipid surfactants under oxygen-limited conditions. In that approach, the heterologously co-produced, extracellular phenazines were used to link the anode to bacterial metabolism and serve as redox mediators in scaled integrated electro-bioreactors (Askitosari et al., 2020).
7.3 Case 3. Electrocatalyst-assisted MES for converting CO2 to chemicals
A range of microbes can utilize single-carbon (C1) feedstocks—such as carbon monoxide and formate, which can be generated from a CO2 reduction reaction—as a source of both carbon and energy (Stöckl et al., 2022; Chu et al., 2023). Zhu et al. (Zhu et al., 2022) reported the use of electrocatalyst-assisted MES to produce acetate and ethanol from CO2 valorization by using Clostridium ljungdahlii. By integrating cobalt phthalocyanine into the porous 3D MES cathodes, the researchers found a more reliable method for generating CO (0.08–0.06 mL/min) and H2 (0.16–0.06 mL/min) from CO2, which resulted in an increase in the maximum levels of acetate (5.1 g/L) and ethanol (1.2 g/L) titers. Although not yet at a high technology readiness level for industrial application, it provided evidence that the novel cathode materials showed substantial advancements toward more effective and stable MES operations (Zhu et al., 2022).
7.4 Case 4. Multimodal unit operations for syngas to ethanol at commercial scale
There are mainly two routes for converting syngas to ethanol. The first route is the direct chemical conversion of syngas to ethanol using catalysts; this route has not yet been commercialized due to poor selectivity, low yield, and mixed alcohols as products (Spivey and Egbebi, 2007; Choi and Liu, 2009; Dieterich et al., 2020).
The second route is the production of ethanol from syngas fermentation, which was reported to be at commercial scale by a joint venture between LanzaTech and Shougang Group. The reported feed gas composition in molar ratio is 5:1:1 for H2:CO : CO2 in syngas fermentation for ethanol production (Huang et al., 2020; Badgett et al., 2021; Lee et al., 2021). In the product of ethanol, half of the moles of carbon were assumed to be from CO, and the other half from CO2 (Huang et al., 2020). The production of ethanol using fermentation exhibits greater carbon utilization and selectivities compared to the direct chemical conversion of syngas to ethanol with catalysts (Badgett et al., 2021). Furthermore, LanzaTech also partnered with the U.S. Department of Energy’s national laboratories to upgrade the produced ethanol into aviation fuels through catalytic conversion (Badgett et al., 2021; Owoade et al., 2023), which manifests the implementation of multimodality strategy by utilizing multiple unit operation to achieve otherwise unattainable goals in producing complex end products.
It is noteworthy that in addition to LanzaTech, there are several other organizations—including Ineos Bio (Vero Beach, FL, USA), Coskata (Warrenville, IL, USA), Genomatica (San Diego, CA, USA), and Kiverdi (Pleasanton, CA, USA)—that are also working toward the commercialization of syngas fermentation for the production of ethanol and other chemicals, as discussed in a recent review (Owoade et al., 2023).
8 Challenges for assembling continuous multi-unit operations for manufacturing scale
While the complexity and variability inherent to each unit operation already pose mostly known challenges for manufacturing processes, the propagation of variances and the interplay of different operations along a manufacturing process path will add another layer of complexity. The alignment and compatibility among these individual unit processing operations must be carefully considered.
9 Concluding remarks and future outlook
Multimodality of an industrial system implies the use of two or more sequential unit operations to improve the system’s overall performance and sustainability. We propose that in some cases, this can be accomplished in a continuous operation. For industrial-scale biotechnology to be realized, the deployment of continuous sequential unit operations will likely be preceded by design, implementation, and optimization at the laboratory and then pilot plant scale. In other cases, engineering workflows may be limited to discontinuous unit operations – limited by technical readiness. As industrial microbiology progressively targets more complex systems, knowledge gaps will remain for the foreseeable future. To fill these gaps, continued development of novel tools is needed to boost system engineering capabilities. One area of particular interest is the development of machine learning and big data analytics. These new computational tools will be especially useful for optimizing and facilitating the development the multimodality technology.
Data availability statement
The raw data supporting the conclusions of this article will be made available by the authors, without undue reservation.
Author contributions
MH and HW conceived the outline and prepared the manuscript. Both authors contributed to the article and approved the submitted version.
Funding
This work was authored by Alliance for Sustainable Energy, LLC, the Manager and Operator of the National Renewable Energy Laboratory for the U.S. Department of Energy (DOE) under Contract No. DE-AC36-08GO28308. Funding provided by U.S. Department of Energy Office of Energy Efficiency and Renewable Energy Bioenergy Technologies Office.
Acknowledgments
The views expressed in the article do not necessarily represent the views of the DOE or the U.S. Government. The U.S. Government retains and the publisher, by accepting the article for publication, acknowledges that the U.S. Government retains a nonexclusive, paid-up, irrevocable, worldwide license to publish or reproduce the published form of this work, or allow others to do so, for U.S. Government purposes. The funder was not involved in the study design, collection, analysis, interpretation of data, the writing of this article or the decision to submit it for publication. All authors declare no other competing interests.
Conflict of interest
The authors declare that the research was conducted in the absence of any commercial or financial relationships that could be construed as a potential conflict of interest.
The author MH declared that they were an editorial board member of Frontiers, at the time of submission. This had no impact on the peer review process and the final decision.
Publisher’s note
All claims expressed in this article are solely those of the authors and do not necessarily represent those of their affiliated organizations, or those of the publisher, the editors and the reviewers. Any product that may be evaluated in this article, or claim that may be made by its manufacturer, is not guaranteed or endorsed by the publisher.
References
Askitosari T. D., Berger C., Tiso T., Harnisch F., Blank L. M., Rosenbaum M. A. (2020). Coupling an electroactive Pseudomonas putida KT2440 with bioelectrochemical rhamnolipid production. Microorganisms 8, 1959. doi: 10.3390/microorganisms8121959
Badgett A., Xi W., Ruth M. (2021). The Potential for Electrons to Molecules Using Solar Energy (United States: National Renewable Energy Lab.(NREL), Golden, CO).
Becker L., Sturm J., Eiden F., Holtmann D. (2023). Analyzing and understanding the robustness of bioprocesses. Trends Biotechnol. 41, 1013–1026 doi: 10.1016/j.tibtech.2023.03.002
Choi Y., Liu P. (2009). Mechanism of ethanol synthesis from syngas on Rh (111). J. Am. Chem. Soc. 131, 13054–13061. doi: 10.1021/ja903013x
Chu N., Jiang Y., Liang Q., Liu P., Wang D., Chen X., et al. (2023). Electricity-driven microbial metabolism of carbon and nitrogen: A waste-to-resource solution. Environ. Sci. Technol. 57, 4379–4395. doi: 10.1021/acs.est.2c07588
Claassens N. J., Cotton C. A., Kopljar D., Bar-Even A. (2019). Making quantitative sense of electromicrobial production. Nat. Catalysis 2, 437–447. doi: 10.1038/s41929-019-0272-0
Dessì P., Rovira-Alsina L., Sánchez C., Dinesh G. K., Tong W., Chatterjee P., et al. (2021). Microbial electrosynthesis: Towards sustainable biorefineries for production of green chemicals from CO2 emissions. Biotechnol. Adv. 46, 107675. doi: 10.1016/j.biotechadv.2020.107675
Dieterich V., Buttler A., Hanel A., Spliethoff H., Fendt S. (2020). Power-to-liquid via synthesis of methanol, DME or Fischer–Tropsch-fuels: a review. Energy Environ. Sci. 13, 3207–3252. doi: 10.1039/D0EE01187H
Dou B., Zhang H., Song Y., Zhao L., Jiang B., He M., et al. (2019). Hydrogen production from the thermochemical conversion of biomass: issues and challenges. Sustain. Energy Fuels 3, 314–342. doi: 10.1039/C8SE00535D
Gullón P., Gullón B., ROmaní A., Rocchetti G., Lorenzo J. M. (2020). Smart advanced solvents for bioactive compounds recovery from agri-food by-products: A review. Trends Food Sci. Technol. 101, 182–197. doi: 10.1016/j.tifs.2020.05.007
Huang Z., Grim G., Schaidle J., Tao L. (2020). Using waste CO2 to increase ethanol production from corn ethanol biorefineries: Techno-economic analysis. Appl. Energy 280, 115964. doi: 10.1016/j.apenergy.2020.115964
Lee U., Hawkins T. R., Yoo E., Wang M., Huang Z., Tao L. (2021). Using waste CO2 from corn ethanol biorefineries for additional ethanol production: life-cycle analysis. Biofuels Bioprod. Biorefining 15, 468–480. doi: 10.1002/bbb.2175
Li T., Chen X. B., Chen J. C., Wu Q., Chen G. Q. (2014). Open and continuous fermentation: products, conditions and bioprocess economy. Biotechnol. J. 9, 1503–1511. doi: 10.1002/biot.201400084
Mandari V., Devarai S. K. (2021). Biodiesel production using homogeneous, heterogeneous, and enzyme catalysts via transesterification and esterification reactions: A critical review. Bioenergy Res. 15, 935–961. doi: 10.1007/s12155-021-10333-w
Mecheri B., Gokhale R., Santoro C., Costa De Oliveira M. A., D’epifanio A., Licoccia S., et al. (2018). Oxygen reduction reaction electrocatalysts derived from iron salt and benzimidazole and aminobenzimidazole precursors and their application in microbial fuel cell cathodes. ACS Appl. Energy materials 1, 5755–5765. doi: 10.1021/acsaem.8b01360
Moreno-García J., García-Martínez T., Mauricio J. C., Moreno J. (2018). Yeast immobilization systems for alcoholic wine fermentations: actual trends and future perspectives. Front. Microbiol. 9, 241. doi: 10.3389/fmicb.2018.00241
Okolie J. A., Rana R., Nanda S., Dalai A. K., Kozinski J. A. (2019). Supercritical water gasification of biomass: a state-of-the-art review of process parameters, reaction mechanisms and catalysis. Sustain. Energy fuels 3, 578–598. doi: 10.1039/C8SE00565F
Owoade A., Alshami A. S., Levin D., Onaizi S., Malaibari Z. O. (2023). Progress and development of syngas fermentation processes toward commercial bioethanol production. Biofuels Bioprod. Biorefining. doi: 10.1002/bbb.2481
Page J. R., Manfredi Z., Bliznakov S., Valla J. A. (2023). Recent progress in electrochemical upgrading of bio-oil model compounds and bio-oils to renewable fuels and platform chemicals. Materials 16, 394. doi: 10.3390/ma16010394
Rathore A. S., Thakur G., Kateja N. (2023). Continuous integrated manufacturing for biopharmaceuticals: A new paradigm or an empty promise? Biotechnol. Bioeng. 120, 333–351. doi: 10.1002/bit.28235
Rogers L., Jensen K. F. (2019). Continuous manufacturing–the Green Chemistry promise? Green Chem. 21, 3481–3498. doi: 10.1039/C9GC00773C
Salvachua D., Saboe P. O., Nelson R. S., Singer C., Mcnamara I., Del Cerro C., et al. (2021). Process intensification for the biological production of the fuel precursor butyric acid from biomass. Cell Rep. Phys. Sci. 2, 100587. doi: 10.1016/j.xcrp.2021.100587
Schlager S., Dumitru L. M., Haberbauer M., Fuchsbauer A., Neugebauer H., Hiemetsberger D., et al. (2016). Electrochemical reduction of carbon dioxide to methanol by direct injection of electrons into immobilized enzymes on a modified electrode. ChemSusChem 9, 631–635. doi: 10.1002/cssc.201501496
Schmidt O., Gambhir A., Staffell I., Hawkes A., Nelson J., Few S. (2017). Future cost and performance of water electrolysis: An expert elicitation study. Int. J. hydrogen Energy 42, 30470–30492. doi: 10.1016/j.ijhydene.2017.10.045
Spivey J. J., Egbebi A. (2007). Heterogeneous catalytic synthesis of ethanol from biomass-derived syngas. Chem. Soc. Rev. 36, 1514–1528. doi: 10.1039/b414039g
Stöckl M., Claassens N., Lindner S., Klemm E., Holtmann D. (2022). Coupling electrochemical CO2 reduction to microbial product generation–identification of the gaps and opportunities. Curr. Opin. Biotechnol. 74, 154–163. doi: 10.1016/j.copbio.2021.11.007
Sun Y., Kokko M., Vassilev I. (2023). Anode-assisted electro-fermentation with Bacillus subtilis under oxygen-limited conditions. Biotechnol. Biofuels Bioprod. 16, 1–12. doi: 10.1186/s13068-022-02253-4
Tiso T., Sabelhaus P., Behrens B., Wittgens A., Rosenau F., Hayen H., et al. (2016). Creating metabolic demand as an engineering strategy in Pseudomonas putida–Rhamnolipid synthesis as an example. Metab. Eng. Commun. 3, 234–244. doi: 10.1016/j.meteno.2016.08.002
Tiso T., Zauter R., Tulke H., Leuchtle B., Li W.-J., Behrens B., et al. (2017). Designer rhamnolipids by reduction of congener diversity: production and characterization. Microbial Cell factories 16, 1–14. doi: 10.1186/s12934-017-0838-y
Wei H., Wang W., Chou Y.-C., Himmel M. E., Chen X., Bomble Y. J., et al. (2023). Prospects for engineering Ralstonia eutropha and Zymomonas mobilis for the autotrophic production of 2, 3-butanediol from CO2 and H2. Eng. Microbiol. 3, 100074. doi: 10.1016/j.engmic.2023.100074
Keywords: industrial microbiology, multimodal processing, continuous processing, process engineering, rational design, electrons to molecules, electro-fermentation, redox enzymes
Citation: Wei H and Himmel ME (2023) Continuous multimodal technologies in industrial microbiology: potential for achieving high process performance and agility. Front. Ind. Microbiol. 1:1234241. doi: 10.3389/finmi.2023.1234241
Received: 03 June 2023; Accepted: 31 July 2023;
Published: 16 August 2023.
Edited by:
Falk Harnisch, Helmholtz Association of German Research Centres (HZ), GermanyReviewed by:
Nilesh Kumar Sharma, Praj Industries Limited, IndiaLászló Koók, University of Pannonia, Hungary
Copyright © 2023 Wei and Himmel. This is an open-access article distributed under the terms of the Creative Commons Attribution License (CC BY). The use, distribution or reproduction in other forums is permitted, provided the original author(s) and the copyright owner(s) are credited and that the original publication in this journal is cited, in accordance with accepted academic practice. No use, distribution or reproduction is permitted which does not comply with these terms.
*Correspondence: Hui Wei, aHVpLndlaUBucmVsLmdvdg==; Michael E. Himmel, bWlrZS5oaW1tZWxAbnJlbC5nb3Y=