- National Renewable Energy Laboratory, BioEnergy Science and Technology Center, Golden, CO, United States
Microbes drive our complex biosphere by regulating the global ecosystem through cycling elements and energy. Humankind has barely begun leveraging this biotransformation capacity to impact global economies and ecologies. Advances in genetic engineering, molecular analysis, metabolic flux modeling, microbial consortia/biome mapping and engineering, cell-free bioproduction, artificial intelligence/machine learning and the ever expanding -omics frontiers have set the stage for paradigm changes to how humankind produces, uses, transforms, and recycles carbon and energy through microbes. Harnessing this enormous potential could drive a global bioeconomy and manage carbon at a planetary level but requires understanding and application at a grand scale across a broad range of science and engineering disciplines. The penultimate manifestation of these advances is the “bio-refinery”, which is often referenced, but is a long way from being fully developed as a global carbon management platform. Broadening the feed stocks, processing operations, and product portfolio to a sequential cascade optimizing the conversion as a whole instead of limited outputs could greatly advance deployment and stability of a bioeconomy.
Introduction
The “bio-refinery” is envisioned as “biomass in - products out” via a sequence of mechanical, thermochemical, and biological processes (Takkellapati et al., 2018) (Figure 1A) over a range of feed stocks and products. Setting aside grain/sugar feed stocks, lignocellulosic inputs dominate this landscape, often limited to one or a few closely related materials. Residual carbon represents an untapped resource for additional products or carbon sequestration. Proposed dedicated bio-refineries include starch (Koutinas et al., 2007; Laufer, 2019; Parchami et al., 2021; Marzo-Gago et al., 2022; He et al., 2023), sugarcane (Pereira et al., 2021; Valladares-Diestra et al., 2021; Deeba et al., 2022; Fernando Herrera Adarme et al., 2022), or wheat straw to ethanol (Kaparaju et al., 2009), lignocellulosics to BDO (Huang et al., 2013; Li et al., 2014; Forte et al., 2016; Hazeena et al., 2020; Rehman et al., 2021), waste gases to ethanol (Arslan et al., 2019; De Tissera et al., 2019; Liu et al., 2022; Tharak and Mohan, 2022), trees to paper pulp (Huang et al., 2010; Hundt et al., 2014; Gottumukkala et al., 2016), wet waste to biogas and fertilizer (Bhaskar et al., 2016; Dahiya et al., 2018; Ge et al., 2018; Desmond-Le Quemener et al., 2019), biomass to hydrocarbon fuels (Davis et al., 2020; Klein et al., 2021), and biomass to biochar, bio-oil, or syngas (Wang et al., 2013; Sarkar et al., 2015; Yuan and Macquarrie, 2015; Hong et al., 2017; De Bhowmick et al., 2019a; De Tissera et al., 2019; Sun et al., 2020). “Niche” platforms have been proposed for specific feed stocks, conversion processes, and products. Usmani et al. published a review of lignocellulosic bio-refineries in 2021 (Usmani et al., 2021)and the IEA published a limited bio-refinery status in 2022, not including the US or Canada among others (Annevelink et al., 2022), which included “extended” pulp/paper, anaerobic digestion, or sugar/starch-based bio-refineries and only nine convert lignocellulosics to ethanol, most with mixed product streams. Feed-stocks for the remaining are variable and include wood processing waste, wet wastes, pulp and paper waste, used/primary vegetable oils/animal fats, textile waste, seed/starch based, grasses/ag crops/residues, and MSW/other wastes. Table 1 is a compilation of selected lignocellulose/waste-based bio-refineries compiled from several websites (Ethanol biorefinery locations; Biorefineries in Europe; Global biorefinery status report 2022; Facilities).
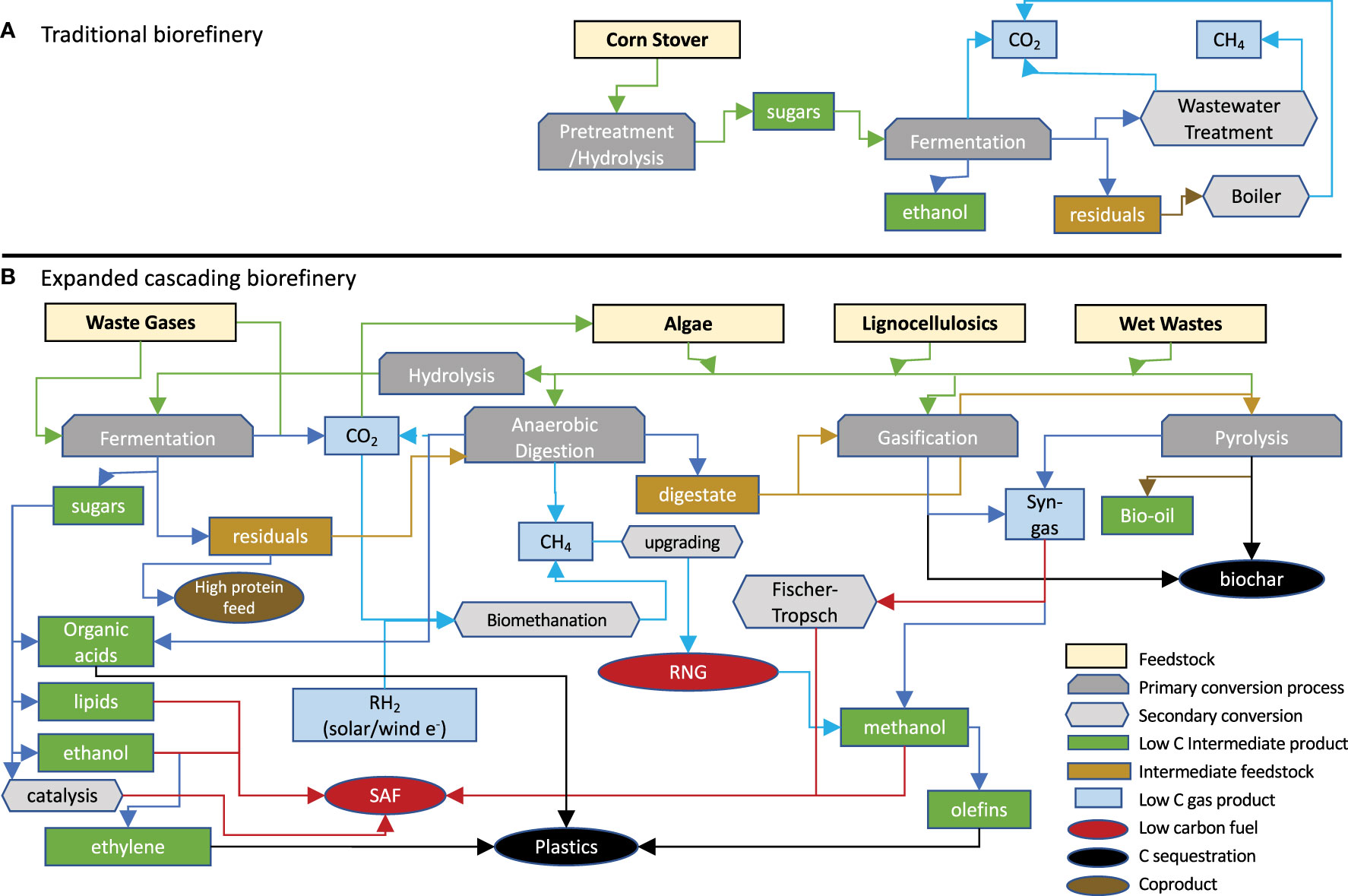
Figure 1 (A) Typical bio-refinery process scheme where limited feed stocks are transformed by a linear process into limited bio-products. (B) Expanded bio-refinery concept using multiple conversion processes to transform a range of feed stocks into an array of bio-products and sequestering untransformed carbon. RNG, Renewable Natural Gas; SAF, Sustainable Aviation Fuel.
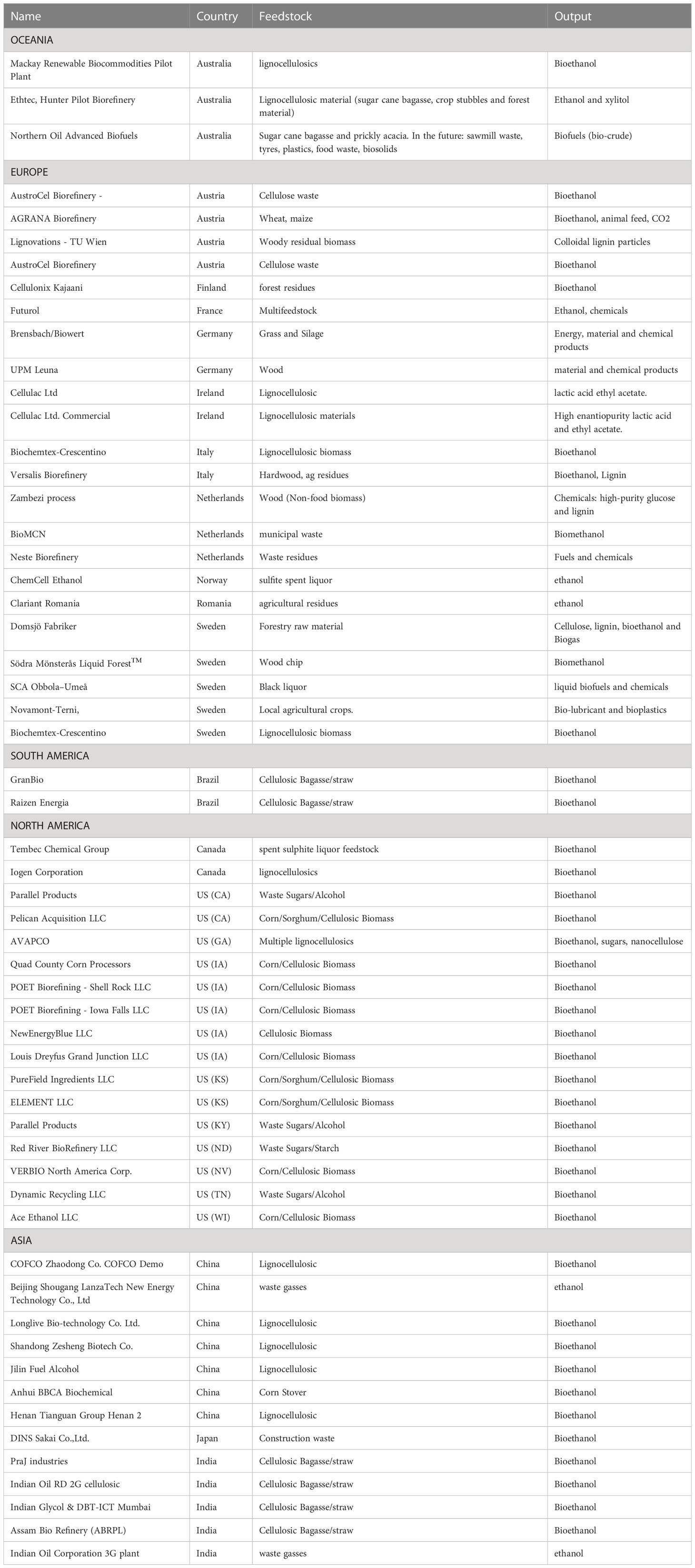
Table 1 Snapshot of global lignocellulose/waste-based bio-refineries operating at the time of this article’s development.
Lignocellulosic bio-refineries transforming the energy landscape through renewable fuels has had numerous social, political, and technical obstacles. Food vs. fuel, land-use, and carbon emissions have been used as arguments against biomass conversion. And while lack of incentives and credits are implied, failure points are mainly technical, i.e. conversion of recalcitrant plant polysaccharides to sugars (Yogalakshmi et al., 2023). Lignin is a problem as is crystalline cellulose and the heterogeneity of hemicelluloses, requiring complex processing and expensive enzymes. Dozens of pretreatments have been tested and generally failed, many due to focusing too tightly on a single product and devaluing the remainder (Yogalakshmi et al., 2023). Fibrous or woody structure requires energy to overcome and collection and transport impose additional costs and supply issues (Saini et al., 2020). Feedstock complexity leads to process design, construction, and operational complexity, other points of failure (Saini et al., 2020). A few examples speak to the primary causes. Dirty feed stocks wreaked havoc at Beta-Renewables and Poet’s Project Liberty had feed stock feeding and pretreatment issues. KiOR failed to scale their facility correctly and low production could not maintain operational capacity while the ADM/Metabolix bioplastic venture failed due to uncertainties in design, production, and market adoption (Saini et al., 2020). Complexity failed INEOS’ Indian River facility with blame being put on wet wood, hydrogen cyanide production, and a range of equipment and power failures. (Investigation: INEOS failed despite $129 million in taxpayer subsidies) Basically, lab-based processes and TEA/LCA models failed at demonstration or commercial scale.
Dedicated bio-refineries based on localized feed stocks and targeted bio-products and continued advancement of all biological aspects of conversion are essential. However, a new paradigm valorizing carbon capture, mitigation, management, and sequestration is emerging; flexible bio-refineries using expanded feed stocks, cascading conversion technologies, and a portfolio of bio-products and sequestered carbon (Figure 1B). Carbon management as income provides flexibility in processing, obviating the constraint for high specific yields as subsequent processes can take partially converted residuals into a new production stream. Branching and cascading processes can trade decreased yield for increased throughput, lower capital and operating costs, and feed stock and product flexibility to fit local or changing markets while increasing overall carbon conversion yield through the inclusion of carbon sequestration.
A broader range of feed stocks and wider product portfolio, requires synergy and co-development in numerous areas and disciplines to optimize the overall system. This will include biology and biochemistry, chemical and mechanical engineering, thermochemical processing, chemical catalysis, techno-economic and life cycle analyses, and even other renewable energy sources to supply low-carbon power and electrons. A “true” bio-refinery will operate much like a petro- refinery; where feed stock is converted into an array of bio-products using multiple technologies optimized holistically. This concept has had limited effort to date, primarily exploration of gaseous feed stocks and the use of algae to capture CO2 and serve as a feed stock or production system (Subhadra and Grinson, 2011; Morais et al., 2015; Butti and Mohan, 2017; Kassim and Meng, 2017; Wiesberg et al., 2017; De Bhowmick et al., 2019b; North, 2019; Yadav et al., 2019; Banerjee et al., 2021), however various social and political pressures to valorize carbon management and sequestration will undoubtedly lead to higher interest in more extensive carbon utilization.
While BioEnergy with Carbon Capture and Sequestration (BECCS) is still uncertain (Jones and Albanito, 2020), technologies such as pyrolysis to form biochar waste carbon to concrete, plastics, and other durable materials (Arehart et al., 2021) could be a simpler option for carbon management (Lefebvre et al., 2020; Papageorgiou et al., 2021). The global bioeconomy is poised to emerge and impact planetary carbon reduction, but needed underlying science and engineering is just being developed.
Artificial intelligence and machine learning
This new holistic approach can be further enhanced with the use of advanced machine learning techniques and artificial intelligence developed in the past decade allowing the exploration of data sets from the benchtop scale to the bio-refinery scale and pushing the boundary for real-time predictive systems that are tailored for both microbes and feed stocks (Oruganti et al., 2023). These data driven approaches have proven to be a powerful tool in assisting design and understanding of biological production of fuels and chemicals. Already studied for optimizing algae growth as a feed stock (Oruganti et al., 2023), this approach can improve yield, product purity, analytics, and guide genetic engineering strategies (Yang et al., 2023) and enzyme engineering (Foroozandeh Shahraki et al., 2021). Reducing severity, time, Capex, and Opex and providing flexible product portfolios driven by prevailing markets are potential areas limited by the current model emphasizing high yields of limited products.
Feed-stocks
Starch, sugars, and lignocellulosics
Microbiology has been used for centuries to produce bio-products and biochemicals from various biomass feed stocks (Buchholz and Collins, 2013). Most well-known is alcoholic fermentation by yeast, whether for beverage or fuel. Additional yeasts and bacterial systems are being developed and production of industrial chemicals such organic acids are heavily based in fungal fermentation of sugars (Grewal and Kalra, 1995; Francisco et al., 2020; Xue et al., 2021; Shikina et al., 2022; Upton et al., 2022; Chib et al., 2023).
The U. S. Department of Energy focuses on lignocellulosic biomass crops such as hybrid poplar, switchgrass, miscanthus, and other grasses and fast-growing hardwoods (Feedstock technologies). Other lignocellulosics include agricultural residues such as corn stover, sugarcane bagasse, and wheat, oat, and rice straws, forestry thinnings, and wood processing residues. Proposed feed stocks include everything from municipal solid wastes and gases to algae. Any lignocellulosic biomass can be converted given the right process and market conditions and the myriad of proposed process technologies in “dedicated” bio-refineries are usually linear and focused on optimizing yield of one or a few products from a limited feed stock input.
Wet wastes and plastics
Food waste, manures, municipal solid waste, and sewage offer wide opportunities for conversion processes and products. As landfills increasingly reject organic wastes, alternative disposal routes are needed. Bio-refining is being proposed for many industrial food waste streams (Kumar et al., 2022) while sewage bio-solids are limited in traditional land-application for disposal (Collivignarelli et al., 2020). Expansion of municipal wastewater systems is often limited by urban sprawl so faster, more efficient options are needed (Jing et al., 2021). Manure is often concentrated by localized high volume ranching, farming, and processing operations. Some of these materials are used to generate biogas by anaerobic digestion, often in co-digestion with other ag residues, however residual digestate contains a large fraction of the original carbon and disposal is still problematic (Chiumenti et al., 2018).
Thermochemical treatment and land filling are the primary means of plastic disposal, however biological deconstruction for renewable plastic generation or other bio-products are being investigated (Bertocchini and Arias, 2023; Malik et al., 2023). Recycling and up-cycling plastics to biodegradable plastics bio-products are two approaches that can help restore the damage caused by this polymer (Kochanska et al., 2022; Morici et al., 2022). Plastics can serve as an excellent source of carbon for microbes, provided the bonds are hydrolysable. While microorganisms metabolize many natural recalcitrant compounds, they have not evolved to breaking down these recently developed man-made materials (Kim et al., 2022; Lim and Thian, 2022; Mat Yasin et al., 2022; Crystal Thew et al., 2023; Thew et al., 2023).
The grand challenge associated with biological conversion of plastics is multidimensional. Bio-catalysts that crack tough chemical bonds in plastics are limited. Enzyme engineering needs to be applied to develop enzymes for individual plastic types. Bio-conversion research on plastics has primarily focused on PET metabolism and research on other plastics is quite rudimentary. Metagenomic approaches to identify novel organisms and enzymes with plastic degrading properties must be explored to determine naturally evolving bio-catalysts from plastic enriched microbiomes, such as the landfills and oceans. Furthermore, integrated microbial and chemical approaches to deconstruct and valorize plastic carbon to bio-products will be critical to a circular bioeconomy.
Algae
Micro- and macro-algae present a massive biological resource for sequestering carbon and have long been proposed as bio-refinery feed stocks and catalysts. Algae take up CO2 directly and often have very high productivity rates. Algae’s ability to use HCO3- directly enables 5-7-fold higher CO2 absorption than wood (Jang et al., 2012) and biomass productivities nearly 4-fold higher than sugarcane (Adams et al., 2008). They can be grown without land or freshwater and do not compete for food production resources. In 2019, over 35 million tons of algae was harvested worldwide, with ~97% by aquaculture. Over 99.8% was macroalgae and 0.16% microalgae (Cai et al., 2021).
Microalgae are used to capture CO2 and waste nutrients for production of bio-products and feed stock biomass. They have high neutral lipid concentrations (up to 70%), driving interest for biodiesel and biofuel production (Chisti, 2007; Sajjadi et al., 2018). Microalgae accumulate other storage compounds such as starch, which can serve for fermentative conversion to biofuels. The flexibility of growing microalgae in open ponds and enclosed photobioreactors under photoautotrophic, heterotrophic, or mixotrophic conditions make them an attractive system for bioproduct applications, though photobioreactors are generally considered too expensive and small scale for production of commodities such as biofuels.
Macroalgae (seaweeds) are starting to be recognized for applications such as waste-water treatments and natural fertilizer applications (Farghali et al., 2023). Their high carbohydrate content (over 60%), in comparison to less than 20% in microalgae (Jung et al., 2013; Jambo et al., 2016) and general lack of lignin and crystalline cellulose point towards easier biological conversion than lignocellulose, however harvest is a challenge. Their unusual polysaccharide chemistry and sometimes high protein content offer both challenges and opportunities. Alginates, carrageenan, fucoidan and laminarin found in the cell walls of macroalgae are recognized for their biological protective activities in humans, highlighting their pharmacological importance (Praveen et al., 2019). They serve as hydrocolloids or functional ingredients in the food industry. Pigments in the form of carotenoids and chlorophyll can serve as replacement for synthetic colors in the food industry (Biris-Dorhoi et al., 2020). The macroalgae industry can impact direct CO2 removal efficiency by sequestering carbon in the form of biochar or via a bio-refinery approach (Farghali et al., 2023).
Most of the genetic engineering efforts have focused on microalgae, macroalgae are only starting to get some attention (Charrier et al., 2015; Cao et al., 2022). Significant efforts are underway to engineer microalgae for biofuel production, despite considerable scientific challenges resulting in several unsuccessful commercialization attempts. Nevertheless, the field of microalgal research has come a long way towards realizing the potential of these photosynthetic organisms. Genetic engineering in macroalgae is just developing, leaving a lot of scope to be explored. While microalgae must be engineered to improve robustness and productivity, large scale cultivation of macroalgae supported by genetic engineering efforts must be achieved for biofuel and specific bioproduct-based applications.
Cyanobacteria have properties similar to micro- and macro-algae and show promise as an environmentally friendly feed stock for production of fuels and plastics alternatives (Farrokh et al., 2019; Afreen et al., 2021). They are known to produce pigments applicable in the food and cosmetics industries, while also serving as food supplement themselves (Zahra et al., 2020). Interesting bioactivities of their metabolites have also been reported, suggesting clinical importance. With smaller genome sizes, challenges associated with improving productivity and product diversity for industrial scale deployment can be addressed effectively using synthetic biology approaches in comparison to the more-cumbersome higher algae.
CO2 and other gases
Bio-generated CO2 from fermentation and CH4/CO2 from anaerobic digestion represent point-source concentrated feed stocks for carbon capture, cycling, and utilization. Bio-conversion routes can generate renewable natural gas and methanol as well as ethanol which can be further upgraded to jet fuel and other products. Microbial engineering to enhance this capture and conversion is only now beginning. Syngas is already used as a feed stock for biorefining with several pilot and demonstration plants currently operating (Dahmen et al., 2019; De Bhowmick et al., 2019b; De Tissera et al., 2019; Yadav et al., 2019). LanzaTech uses flue gas bioconversion for ethanol and other bio-products and subsequent upgrading to jet fuel using the LanzaJet process.
Bio-driven GHG mitigation is likely the only viable global-scale option in the near-term and bio-refineries are primed to contributed. Biology has been capturing CO2 since the beginning of life at a planetary scale, the energy is sunlight, and biomass represents high-density carbon. The key will be balancing economical bio-products and sequestering the low-value carbon. In contrast, Direct Air Capture using adsorbants, desorption, and underground sequestration is too energy intensive. According to the International Energy Agency, DAC CO2 requires between 6.5 and 10 GJ/t CO2 or 1.8 to 2.7 MWh per ton (Budinis). In simple terms, sequestering 1 GT of CO2 using DAC would require between 1800 and 2700 TWh, roughly half of the total U.S. output in 2021 of ~4000 TWh. The largest operating DAC facility, Climeworks ORCA plant in Iceland, sequesters 4000 MT/year, necessitating 250,000 similar plants to capture 1 GT CO2/year.
Bio-catalysts
Biocatalysis forms the core of any circular bioeconomy, bio-refinery, or industrial biotechnology process. Biology’s ability to rapidly catalyze biochemical reactions sequentially at low temperature and high specificity forms the basis of industrial biotechnology. Classical bio-catalysts such as microbes and enzymes have been used for centuries. More recently, immobilized cells and cell-free systems have gained attention as means to accomplish certain biochemical pathways without “wasting” carbon and energy maintaining viable cells. Regardless of form, bio-catalysts are fundamental to industrial production of biofuels, biochemicals, and bio-products.
Microbes
Bacteria, yeast, fungi, and other whole cell bio-catalysts dominate industrial microbiology in biofuel and biochemical production. Examples include fungal production of organic acids and lipids (Grewal and Kalra, 1995; Carvalho et al., 2019; Chib et al., 2023), alcohols and lipids in yeast (Olson et al., 2004; Hull et al., 2014; Cai et al., 2016a; Pendon et al., 2021), alcohols and biochemicals in bacteria (Cai et al., 2016b), lipids and carotenoids in microalgae (Lopes da Silva et al., 2019; Monte et al., 2020; Papachristou et al., 2021; Oh et al., 2022), and a myriad of other bio-products from a range of microbes. Advances in molecular biology have enabled rapid and targeted metabolic engineering for increased product rate and titer, biofunneling to increase yields, expanded substrate utilization, redox balancing, and other cellular redesigns. Cutting edge technologies such as high through-put sequencing and targeted genome modification tools have opened a Pandora’s box of opportunities in microbial metabolic engineering. The dawn of the -omics age has extended these opportunities even further as metabolomics, proteomics, fluxomics, genomics, transcriptomics, lipidomics, and glycomics continue to increase our understanding of cellular metabolism and pathways. Metagenomics, epigenomics, microbiomics, and secretomics have led us to the edge of engineering and directing microbial consortia in specific and targeted manner. These data-intensive techniques are tailor-made for big data applications of artificial intelligence and machine learning and as this interface of biology and data science continues to expand, we expect leaps forward in our understanding and manipulation of these cellular processes.
Enzymes
Enzymatic bioconversion of lignocellulosic biomass in the past 40+ years has evolved from relatively simple models of fungal cellulases such as Cel7A from Trichoderma reesei (Taylor et al., 2018), to a broader and more comprehensive understating of mesoscale deconstruction mechanisms employed by multifunctional bacterial enzymes such as CelA from Caldicellulosiruptor bescii (Brunecky et al., 2017), synthetic multifunctional cellulases (Brunecky et al., 2020), and megaDalton sized cellulosomal complexes utilized by CBP organisms like Clostridium thermocellum (Hirano et al., 2016). Moreover, the critical debranching roles of accessory enzymes acting on xylan and other hemicelluloses (Moon et al., 2011; Barr et al., 2012; Pryor et al., 2012; Lagaert et al., 2014; Cao et al., 2015; Goncalves et al., 2015; Hu et al., 2015; Laothanachareon et al., 2015; Sun et al., 2015; Yang et al., 2018; Ogunyewo et al., 2021) and the discovery of Lytic Polysaccharide Mono-Oxygenase enzymes have also been critical in the development of modern commercial cellulases (Agger et al., 2014; Muller et al., 2015; Bernardi et al., 2020; Cheng et al., 2020; Calderaro et al., 2021).
Two primary challenges will be understanding deconstruction of lignocellulosic materials by novel enzymes and enzyme classes and leveraging that understanding to develop advanced commercial enzymes. Future enzyme cocktails will apply these deconstruction strategies to minimally pretreated lignocellulosic feed stocks in a cost-effective manner. Facile, robust, and low-cost production of enzymes must be developed significantly beyond the current state of the art to be applicable at global commodity scale.
Cell-free systems
Bio-based fuels and chemicals rely on living microbial cells, presenting challenges in engineering and optimizing metabolic pathways for these compounds. Mass transfer and pathway optimization are constrained by cell membranes and intracellular processes (Lu, 2017; Wilding et al., 2018) and much of the carbon ends up in cell biomass, reducing overall carbon efficiency. A high carbon efficiency bioeconomy could benefit from a move toward highly efficient cell-free synthetic biology (Sheldon and Woodley, 2018; Wilding et al., 2018). Cell-free synthetic biology is an emerging interdisciplinary approach utilizing enzymes and cofactor components that are engineered and optimized without the use of living cells, allowing direct control of transcription, translation, and metabolism in an open environment (Lu, 2017).
The primary advantages of cell free enzyme systems are facile manipulation of substrate ratios, and careful adjustment of high energy flux ratios that are either difficult or impossible to control in microbial systems. Enzyme activity and temperature optima are tuned through careful enzyme selection. In contrast, living systems issues include metabolite competition, generation of side products, suboptimal enzyme ratios, and variable temperature optima for the cell (Bergquist et al., 2020) and devotes significant energy and effort in keeping itself alive and reproducing. Unfortunately, key problems for cell free systems remain, largely related to robust and facile protein expression, where post translational modifications are key. Recycling energy carriers such as NADH/NADPH or ATP is also an problem, however there are some approaches using whole cell lysates or redox balancing reactions (Ullah et al., 2016). Examples of possible reactions are too many to list, but two common substrates, glucose and glycerol can be utilized to produce a variety of products (Bergquist et al., 2020).
Discussion
We are on the cusp of a carbon-management-based global bioeconomy driven by reducing GHG levels, decarbonizing a wide range of industries, and equilibrating bioenergy and bio-products opportunities across geographical, cultural, economic, and social barriers. The bio-refinery will play a central role in this new paradigm and will function from niche to regional commodity scale. Feed-stocks and products will be extremely narrow or exceedingly broad based on local opportunities and the science and engineering needed will vary accordingly. The social, political, and economic factors will likely stay in flux for years, however the underlying need to solve global carbon levels will only continue to increase. Bio-based technologies offer the best and possibly only opportunity to achieve this planetary effort at scale and in the shortest time, but developing the myriad technologies needed to implement the solution will require constant and ongoing dissemination of results, collaborations across disciplines, and rigorous peer review to advance the bio-refinery to a meaningful level to solve rising carbon levels worldwide.
Data availability statement
The original contributions presented in the study are included in the article/supplementary material. Further inquiries can be directed to the corresponding author.
Author contributions
SD: Overall concept and framework, principal writing for abstract, introduction, Feed-stocks (starch, sugars, LCs, wet wastes, CO2 and gases), Biocatalysts (microbes), edited and contributed to other sections, handles references, final editing and submission. RB: Principal writing for enzymes and cell-free sections, general contributions to rest of article, JY: Principal writing for AI/ML and cell-free. VS: Principal writing for algae, plastics, and microbes sections, general contributions to rest of article. All authors contributed to the article and approved the submitted version.
Funding
Funding provided by U.S. Department of Energy Office of Energy Efficiency and Renewable Energy Bioenergy Technologies Office. The views expressed in the article do not necessarily represent the views of the DOE or the U.S. Government.
Acknowledgments
This work was authored by Alliance for Sustainable Energy, LLC, the Manager and Operator of the National Renewable Energy Laboratory for the U.S. Department of Energy (DOE) under Contract No. DE-AC36-08GO28308.
Conflict of interest
The author SRD declared that they were an editorial board member of Frontiers, at the time of submission. This had no impact on the peer review process and the final decision.
The remaining authors declare that the research was conducted in the absence of any commercial or financial relationships that could be construed as a potential conflict of interest.
Publisher’s note
All claims expressed in this article are solely those of the authors and do not necessarily represent those of their affiliated organizations, or those of the publisher, the editors and the reviewers. Any product that may be evaluated in this article, or claim that may be made by its manufacturer, is not guaranteed or endorsed by the publisher.
References
Adams J. M., Gallagher J. A., Donnison I. S. (2008). Fermentation study on Saccharina latissima for bioethanol production considering variable pre-treatments. J. Appl. Phycology 21 (5), 569–574. doi: 10.1007/s10811-008-9384-7
Afreen R., Tyagi S., Singh G. P., Singh M. (2021). Challenges and perspectives of polyhydroxyalkanoate production from microalgae/cyanobacteria and bacteria as microbial factories: an assessment of hybrid biological system. Front. Bioengineering Biotechnol. 9. doi: 10.3389/fbioe.2021.624885
Agger J. W., Isaksen T., Varnai A., Vidal-Melgosa S., Willats W. G., Ludwig R., et al. (2014). Discovery of LPMO activity on hemicelluloses shows the importance of oxidative processes in plant cell wall degradation. Proc. Natl. Acad. Sci. U.S.A. 111 (17), 6287–6292. doi: 10.1073/pnas.1323629111
Annevelink B., Chavez L. G., Ree R. V., Gursel I. V. (2022). Global biorefinery status report 2022. (IEA Bioenergy).
Arehart J. H., Hart J., Pomponi F., D’Amico B. (2021). Carbon sequestration and storage in the built environment. Sustain. Production Consumption 27, 1047–1063. doi: 10.1016/j.spc.2021.02.028
Arslan K., Bayar B., Nalakath Abubackar H., Veiga M. C., Kennes C. (2019). Solventogenesis in Clostridium aceticum producing high concentrations of ethanol from syngas. Bioresour Technol. 292, 121941. doi: 10.1016/j.biortech.2019.121941
Ethanol biorefinery locations. Available at: https://ethanolrfa.org/resources/ethanol-biorefinery-locations.
Biorefineries in europe. Available at: https://task42.ieabioenergy.com/wp-content/uploads/sites/10/2021/02/Cepi_Biorefineries-in-Europe_master_161220_finalappendixes.pdf.
Global biorefinery status report 2022. Available at: https://www.ieabioenergy.com/wp-content/uploads/2022/09/IEA-Bioenergy-Task-42-Global-biorefinery-status-report-2022-220712.pdf.
Facilities. Available at: https://www.ieabioenergy.com/installations.
Investigation: INEOS failed despite $129 million in taxpayer subsidies. Available at: https://advancedbiofuelsusa.info/investigation-ineos-failed-despite-129-million-in-taxpayer-subsidies.
Feedstock technologies. Available at: https://www.energy.gov/eere/bioenergy/feedstock-technologies (Accessed 28 March 2023).
Banerjee S., Ray A., Das D. (2021). Optimization of Chlamydomonas reinhardtii cultivation with simultaneous CO(2) sequestration and biofuels production in a biorefinery framework. Sci. Total Environ. 762, 143080. doi: 10.1016/j.scitotenv.2020.143080
Barr C. J., Mertens J. A., Schall C. A. (2012). Critical cellulase and hemicellulase activities for hydrolysis of ionic liquid pretreated biomass. Bioresour Technol. 104, 480–485. doi: 10.1016/j.biortech.2011.10.101
Bergquist P. L., Siddiqui S., Sunna A. (2020). Cell-free biocatalysis for the production of platform chemicals. Front. Energy Res. , 8. doi: 10.3389/fenrg.2020.00193
Bernardi A. V., Gerolamo L. E., de Gouvea P. F., Yonamine D. K., Pereira L. M. S., de Oliveira A. H. C., et al. (2020). LPMO afAA9_B and cellobiohydrolase afCel6A from A. fumigatus boost enzymatic saccharification activity of cellulase cocktail. Int. J. Mol. Sci. 22 (1), 276–299. doi: 10.1007/s10811-008-9384-7
Bertocchini F., Arias C. F. (2023). Why have we not yet solved the challenge of plastic degradation by biological means? PloS Biol. 21 (3), e3001979. doi: 10.1371/journal.pbio.3001979
Bhaskar T., Chang J. S., Khanal S., Lee D. J., Venkata Mohan S., Rittmann B. E. (2016). Waste biorefinery - advocating circular economy. Bioresour Technol. 215, 1. doi: 10.1016/j.biortech.2016.06.020
Biris-Dorhoi E.-S., Michiu D., Pop C. R., Rotar A. M., Tofana M., Pop O. L., et al. (2020). Macroalgae—A sustainable source of chemical compounds with biological activities. Nutrients 12 (10), 3085. doi: 10.3390/nu12103085
Brunecky R., Donohoe B. S., Yarbrough J. M., Mittal A., Scott B. R., Ding H., et al. (2017). The Multi Domain Caldicellulosiruptor bescii CelA Cellulase Excels at the Hydrolysis of Crystalline Cellulose. Sci. Rep. 7 (1), 9622. doi: 10.1038/s41598-017-08985-w
Brunecky R., Subramanian V., Yarbrough J. M., Donohoe B. S., Vinzant T. B., Vanderwall T. A., et al. (2020). Synthetic fungal multifunctional cellulases for enhanced biomass conversion. Green Chem. 22 (2), 478–489. doi: 10.1039/C9GC03062J
Buchholz K., Collins J. (2013). The roots–a short history of industrial microbiology and biotechnology. Appl. Microbiol. Biotechnol. 97 (9), 3747–3762. doi: 10.1007/s00253-013-4768-2
Budinis S. Direct air capture- technology deep dive. Available at: https://www.iea.org/reports/direct-air-capture (Accessed 03/31).
Butti S. K., Mohan S. V. (2017). Autotrophic biorefinery: dawn of the gaseous carbon feedstock. FEMS Microbiol. Lett. 364 (18), 1–8. doi: 10.1093/femsle/fnx166
Cai D., Dong Z., Wang Y., Chen C., Li P., Qin P., et al. (2016a). Biorefinery of corn cob for microbial lipid and bio-ethanol production: An environmental friendly process. Bioresour Technol. 211, 677–684. doi: 10.1016/j.biortech.2016.03.159
Cai D., Dong Z., Wang Y., Chen C., Li P., Qin P., et al. (2016b). Co-generation of microbial lipid and bio-butanol from corn cob bagasse in an environmentally friendly biorefinery process. Bioresour Technol. 216, 345–351. doi: 10.1016/j.biortech.2016.05.073
Cai J., Lovatelli A., Aguilar-Manjarrez J., Cornish L., Dabbadie L., Desrochers A., et al. (2021). Seaweeds and microalgae: an overview for unlocking their potential in global aquaculture development. FAO Fisheries Aquaculture 1–28. doi: 10.4060/cb5670en
Calderaro F., Bevers L. E., van den Berg M. A. (2021). Oxidative power: tools for assessing LPMO activity on cellulose. Biomolecules 11 (8), 1098–1119. doi: 10.3390/biom11081098
Cao X., Kong F., Sun B., Yin J., Ren H., Yue H., et al. (2022). A toolbox for constructing a stable genetic transformation platform allowing foreign fragment integration in the genome of neopyropia yezoensis. Front. Mar. Sci. 9. doi: 10.3389/fmars.2022.856790
Cao G., Ximenes E., Nichols N. N., Frazer S. E., Kim D., Cotta M. A., et al. (2015). Bioabatement with hemicellulase supplementation to reduce enzymatic hydrolysis inhibitors. Bioresour Technol. 190, 412–415. doi: 10.1016/j.biortech.2015.04.064
Carvalho A. K. F., Bento H. B. S., Reis C. E. R., De Castro H. F. (2019). Sustainable enzymatic approaches in a fungal lipid biorefinery based in sugarcane bagasse hydrolysate as carbon source. Bioresour Technol. 276, 269–275. doi: 10.1016/j.biortech.2018.12.118
Charrier B., Rolland E., Gupta V., Reddy C. R. K. (2015). Production of genetically and developmentally modified seaweeds: exploiting the potential of artificial selection techniques. Front. Plant Sci. 6. doi: 10.3389/fpls.2015.00127
Cheng C., Haider J., Liu P., Yang J., Tan Z., Huang T., et al. (2020). Engineered LPMO significantly boosting cellulase-catalyzed depolymerization of cellulose. J. Agric. Food Chem. 68 (51), 15257–15266. doi: 10.1021/acs.jafc.0c05979
Chib S., Jamwal V. L., Kumar V., Gandhi S. G., Saran S. (2023). Fungal production of kojic acid and its industrial applications. Appl. Microbiol. Biotechnol. 107 (7-8), 2111–2130. doi: 10.1007/s00253-023-12451-1
Chisti Y. (2007). Biodiesel from microalgae. Biotechnol. Adv. 25 (3), 294–306. doi: 10.1016/j.biotechadv.2007.02.001
Chiumenti A., da Borso F., Limina S. (2018). Dry anaerobic digestion of cow manure and agricultural products in a full-scale plant: Efficiency and comparison with wet fermentation. Waste Manag 71, 704–710. doi: 10.1016/j.wasman.2017.03.046
Collivignarelli M. C., Abba A., Benigna I. (2020). The reuse of biosolids on agricultural land: Critical issues and perspective. Water Environ. Res. 92 (1), 11–25. doi: 10.1002/wer.1196
Crystal Thew X. E., Lo S. C., Ramanan R. N., Tey B. T., Huy N. D., Chien Wei O. (2023). Enhancing plastic biodegradation process: strategies and opportunities. Crit. Rev. Biotechnol., 1–18. doi: 10.1080/07388551.2023.2170861
Dahiya S., Kumar A. N., Shanthi J. Sravan, Chatterjee S., Sarkar O., Mohan S. V. (2018). Food waste biorefinery: Sustainable strategy for circular bioeconomy. Bioresour Technol. 248 (Pt A), 2–12. doi: 10.1016/j.biortech.2017.07.176
Dahmen N., Henrich E., Henrich T. (2019). Synthesis gas biorefinery. Adv. Biochem. Eng. Biotechnol. 166, 217–245. doi: 10.1007/10_2016_63
Davis R., Tao L., Bartling A. (2020). Biochemical conversion of lignocellulosic biomass to hydrocarbon fuels and products: 2019 state of technology and future research (Golden, CO: National Renewable Energy Laboratory).
De Bhowmick G., Sarmah A. K., Sen R. (2019a). Zero-waste algal biorefinery for bioenergy and biochar: A green leap towards achieving energy and environmental sustainability. Sci. Total Environ. 650 (Pt 2), 2467–2482. doi: 10.1016/j.scitotenv.2018.10.002
De Bhowmick G., Sarmah A. K., Sen R. (2019b). Performance evaluation of an outdoor algal biorefinery for sustainable production of biomass, lipid and lutein valorizing flue-gas carbon dioxide and wastewater cocktail. Bioresour Technol. 283, 198–206. doi: 10.1016/j.biortech.2019.03.075
Deeba F., Kiran Kumar K., Ali Wani S., Kumar Singh A., Sharma J., Gaur N. A. (2022). Enhanced biodiesel and beta-carotene production in Rhodotorula pacifica INDKK using sugarcane bagasse and molasses by an integrated biorefinery framework. Bioresour Technol. 351, 127067. doi: 10.1016/j.biortech.2022.127067
Desmond-Le Quemener E., Bridier A., Tian J. H., Madigou C., Bureau C., Qi Y., et al. (2019). Biorefinery for heterogeneous organic waste using microbial electrochemical technology. Bioresour Technol. 292, 121943. doi: 10.1016/j.biortech.2019.121943
De Tissera S., Kopke M., Simpson S. D., Humphreys C., Minton N. P., Durre P. (2019). Syngas biorefinery and syngas utilization. Adv. Biochem. Eng. Biotechnol. 166, 247–280. doi: 10.1007/10_2017_5
Farghali M., Mohamed I. M. A., Osman A. I., Rooney D. W. (2023). Seaweed for climate mitigation, wastewater treatment, bioenergy, bioplastic, biochar, food, pharmaceuticals, and cosmetics: a review. Environ. Chem. Lett. 21 (1), 97–152. doi: 10.1007/s10311-022-01520-y
Farrokh P., Sheikhpour M., Kasaeian A., Asadi H., Bavandi R. (2019). Cyanobacteria as an eco-friendly resource for biofuel production: A critical review. Biotechnol. Prog. 35 (5), e2835. doi: 10.1002/btpr.2835
Fernando Herrera Adarme O., Eduardo Lobo Baeta B., Cardoso Torres M., Camilo Otalora Tapiero F., Vinicius Alves Gurgel L., de Queiroz Silva S., et al. (2022). Biogas production by anaerobic co-digestion of sugarcane biorefinery byproducts: Comparative analyses of performance and microbial community in novel single-and two-stage systems. Bioresour Technol. 354, 127185. doi: 10.1016/j.biortech.2022.127185
Foroozandeh Shahraki M., Farhadyar K., Kavousi K., Azarabad M. H., Boroomand A., Ariaeenejad S., et al. (2021). A generalized machine-learning aided method for targeted identification of industrial enzymes from metagenome: A xylanase temperature dependence case study. Biotechnol. Bioengineering 118 (2), 759–769. doi: 10.1002/bit.27608
Forte A., Zucaro A., Basosi R., Fierro A. (2016). LCA of 1,4-Butanediol Produced via Direct Fermentation of Sugars from Wheat Straw Feedstock within a Territorial Biorefinery. Materials (Basel) 9 (7), 563–585. doi: 10.3390/ma9070563
Francisco J. C. E., Rivera W. L., Vital P. G. (2020). Influences of carbohydrate, nitrogen, and phosphorus sources on the citric acid production by fungal endophyte Aspergillus fumigatus P3I6. Prep Biochem. Biotechnol. 50 (3), 292–301. doi: 10.1080/10826068.2019.1689510
Ge S., Usack J., Ma B. (2018). Advances and challenges at the waste-to-bioenergy/biorefinery nexus. BioMed. Res. Int. 2018, 3642363. doi: 10.1155/2018/3642363
Goncalves G. A., Takasugi Y., Jia L., Mori Y., Noda S., Tanaka T., et al. (2015). Synergistic effect and application of xylanases as accessory enzymes to enhance the hydrolysis of pretreated bagasse. Enzyme Microb. Technol. 72, 16–24. doi: 10.1016/j.enzmictec.2015.01.007
Gottumukkala L. D., Haigh K., Collard F. X., van Rensburg E., Gorgens J. (2016). Opportunities and prospects of biorefinery-based valorisation of pulp and paper sludge. Bioresour Technol. 215, 37–49. doi: 10.1016/j.biortech.2016.04.015
Grewal H. S., Kalra K. L. (1995). Fungal production of citric acid. Biotechnol. Adv. 13 (2), 209–234. doi: 10.1016/0734-9750(95)00002-8
Hazeena S. H., Sindhu R., Pandey A., Binod P. (2020). Lignocellulosic bio-refinery approach for microbial 2,3-Butanediol production. Bioresour Technol. 302, 122873. doi: 10.1016/j.biortech.2020.122873
He C., Zhang X., Zhang Z., Wang C., Wang D., Wei G. (2023). Whole-crop biorefinery of corn biomass for pullulan production by Aureobasidium pullulans. Bioresour Technol. 370, 128517. doi: 10.1016/j.biortech.2022.128517
Hirano K., Kurosaki M., Nihei S., Hasegawa H., Shinoda S., Haruki M., et al. (2016). Enzymatic diversity of the Clostridium thermocellum cellulosome is crucial for the degradation of crystalline cellulose and plant biomass. Sci. Rep. 6, 35709. doi: 10.1038/srep35709
Hong Y., Chen W., Luo X., Pang C., Lester E., Wu T. (2017). Microwave-enhanced pyrolysis of macroalgae and microalgae for syngas production. Bioresour Technol. 237, 47–56. doi: 10.1016/j.biortech.2017.02.006
Hu J., Chandra R., Arantes V., Gourlay K., Susan van Dyk J., Saddler J. N. (2015). The addition of accessory enzymes enhances the hydrolytic performance of cellulase enzymes at high solid loadings. Bioresour Technol. 186, 149–153. doi: 10.1016/j.biortech.2015.03.055
Huang C. F., Jiang Y. F., Guo G. L., Hwang W. S. (2013). Method of 2,3-butanediol production from glycerol and acid-pretreated rice straw hydrolysate by newly isolated strains: pre-evaluation as an integrated biorefinery process. Bioresour Technol. 135, 446–453. doi: 10.1016/j.biortech.2012.10.141
Huang H. J., Ramaswamy S., Al-Dajani W. W., Tschirner U. (2010). Process modeling and analysis of pulp mill-based integrated biorefinery with hemicellulose pre-extraction for ethanol production: a comparative study. Bioresour Technol. 101 (2), 624–631. doi: 10.1016/j.biortech.2009.07.092
Hull C. M., Loveridge E. J., Donnison I. S., Kelly D. E., Kelly S. L. (2014). Co-production of bioethanol and probiotic yeast biomass from agricultural feedstock: application of the rural biorefinery concept. AMB Express 4, 64. doi: 10.1186/s13568-014-0064-5
Hundt M., Engel N., Schnitzlein K., Schnitzlein M. G. (2014). Combining the effects of pulping severity and alkali concentration to optimize the lignocellulose-based AlkaPolP biorefinery concept. Bioresour Technol. 166, 411–419. doi: 10.1016/j.biortech.2014.05.050
Jambo S. A., Abdulla R., Mohd Azhar S. H., Marbawi H., Gansau J. A., Ravindra P. (2016). A review on third generation bioethanol feedstock. Renewable Sustain. Energy Rev. 65, 756–769. doi: 10.1016/j.rser.2016.07.064
Jang S. -S., Shirai Y., Uchida M., Wakisaka M. (2012). Production of mono sugar from acid hydrolysis of seaweed. Afr. J. Biotechnol. 11 (8), 1953–1963. doi: 10.5897/ajb10.1681
Jing H., Wang H., Lin C. S. K., Zhuang H., To M. H., Leu S. Y. (2021). Biorefinery potential of chemically enhanced primary treatment sewage sludge to representative value-added chemicals - A de novo angle for wastewater treatment. Bioresour Technol. 339, 125583. doi: 10.1016/j.biortech.2021.125583
Jones M. B., Albanito F. (2020). Can biomass supply meet the demands of bioenergy with carbon capture and storage (BECCS)? Glob Chang Biol. 26 (10), 5358–5364. doi: 10.1111/gcb.15296
Jung K. A., Lim S. R., Kim Y., Park J. M. (2013). Potentials of macroalgae as feedstocks for biorefinery. Bioresour Technol. 135, 182–190. doi: 10.1016/j.biortech.2012.10.025
Kaparaju P., Serrano M., Thomsen A. B., Kongjan P., Angelidaki I. (2009). Bioethanol, biohydrogen and biogas production from wheat straw in a biorefinery concept. Bioresour Technol. 100 (9), 2562–2568. doi: 10.1016/j.biortech.2008.11.011
Kassim M. A., Meng T. K. (2017). Carbon dioxide (CO(2)) biofixation by microalgae and its potential for biorefinery and biofuel production. Sci. Total Environ. 584-585, 1121–1129. doi: 10.1016/j.scitotenv.2017.01.172
Kim D. W., Ahn J. H., Cha C. J. (2022). Biodegradation of plastics: mining of plastic-degrading microorganisms and enzymes using metagenomics approaches. J. Microbiol. 60 (10), 969–976. doi: 10.1007/s12275-022-2313-7
Klein B., McNamara I., Davis R., Mittal A., Johnson D. (2021). Techno-economic assessment for the production of hydrocarbon fuels via catalytic upgrading of furans (Golden, Colorado: National Renewable Energy Laboratory).
Kochanska E., Wozniak K., Nowaczyk A., Piedade P. J., de Almeida Lavorato M. L., Almeida A. M., et al. (2022). Global ban on plastic and what next? Are consumers ready to replace plastic with the second-generation bioplastic? Results of the snowball sample consumer research in china, western and eastern europe, north america and brazil. Int. J. Environ. Res. Public Health 19 (21), 13970–13995. doi: 10.3390/ijerph192113970
Koutinas A. A., Arifeen N., Wang R., Webb C. (2007). Cereal-based biorefinery development: integrated enzyme production for cereal flour hydrolysis. Biotechnol. Bioeng 97 (1), 61–72. doi: 10.1002/bit.21206
Kumar V., Sharma N., Umesh M., Selvaraj M., Al-Shehri B. M., Chakraborty P., et al. (2022). Emerging challenges for the agro-industrial food waste utilization: A review on food waste biorefinery. Bioresour Technol. 362, 127790. doi: 10.1016/j.biortech.2022.127790
Lagaert S., Pollet A., Courtin C. M., Volckaert G. (2014). beta-xylosidases and alpha-L-arabinofuranosidases: accessory enzymes for arabinoxylan degradation. Biotechnol. Adv. 32 (2), 316–332. doi: 10.1016/j.biotechadv.2013.11.005
Laothanachareon T., Bunterngsook B., Suwannarangsee S., Eurwilaichitr L., Champreda V. (2015). Synergistic action of recombinant accessory hemicellulolytic and pectinolytic enzymes to Trichoderma reesei cellulase on rice straw degradation. Bioresour Technol. 198, 682–690. doi: 10.1016/j.biortech.2015.09.053
Laufer A. (2019). Starch biorefinery enzymes. Adv. Biochem. Eng. Biotechnol. 166, 137–152. doi: 10.1007/10_2016_60
Lefebvre D., Williams A., Meersmans J., Kirk G. J. D., Sohi S., Goglio P., et al. (2020). Modelling the potential for soil carbon sequestration using biochar from sugarcane residues in Brazil. Sci. Rep. 10 (1), 19479. doi: 10.1038/s41598-020-76470-y
Li L., Li K., Wang K., Chen C., Gao C., Ma C., et al. (2014). Efficient production of 2,3-butanediol from corn stover hydrolysate by using a thermophilic Bacillus licheniformis strain. Bioresour Technol. 170, 256–261. doi: 10.1016/j.biortech.2014.07.101
Lim B. K. H., Thian E. S. (2022). Biodegradation of polymers in managing plastic waste - A review. Sci. Total Environ. 813, 151880. doi: 10.1016/j.scitotenv.2021.151880
Liu G., Yang G., Peng X., Wu J., Tsubaki N. (2022). Recent advances in the routes and catalysts for ethanol synthesis from syngas. Chem. Soc. Rev. 51 (13), 5606–5659. doi: 10.1039/D0CS01003K
Lopes da Silva T., Moniz P., Silva C., Reis A. (2019). The dark side of microalgae biotechnology: A heterotrophic biorefinery platform directed to omega-3 rich lipid production. Microorganisms 7 (12), 670–691. doi: 10.3390/microorganisms7120670
Lu Y. (2017). Cell-free synthetic biology: Engineering in an open world. Synth Syst. Biotechnol. 2 (1), 23–27. doi: 10.1016/j.synbio.2017.02.003
Malik S., Maurya A., Khare S. K., Srivastava K. R. (2023). Computational exploration of bio-degradation patterns of various plastic types. Polymers (Basel) 15 (6), 1540–1552. doi: 10.3390/polym15061540
Marzo-Gago C., Venus J., Lopez-Gomez J. P. (2022). Production of lactic acid from pasta wastes using a biorefinery approach. Biotechnol. Biofuels Bioprod 15 (1), 128. doi: 10.1186/s13068-022-02222-x
Mat Yasin N., Akkermans S., Van Impe J. F. M. (2022). Enhancing the biodegradation of (bio)plastic through pretreatments: A critical review. Waste Manag 150, 1–12. doi: 10.1016/j.wasman.2022.06.004
Monte J., Ribeiro C., Parreira C., Costa L., Brive L., Casal S., et al. (2020). Biorefinery of Dunaliella salina: Sustainable recovery of carotenoids, polar lipids and glycerol. Bioresour Technol. 297, 122509. doi: 10.1016/j.biortech.2019.122509
Moon Y. H., Iakiviak M., Bauer S., Mackie R. I., Cann I. K. (2011). Biochemical analyses of multiple endoxylanases from the rumen bacterium Ruminococcus albus 8 and their synergistic activities with accessory hemicellulose-degrading enzymes. Appl. Environ. Microbiol. 77 (15), 5157–5169. doi: 10.1128/AEM.00353-11
Morais A. R., da Costa Lopes A. M., Bogel-Lukasik R. (2015). Carbon dioxide in biomass processing: contributions to the green biorefinery concept. Chem. Rev. 115 (1), 3–27. doi: 10.1021/cr500330z
Morici E., Carroccio S. C., Bruno E., Scarfato P., Filippone G., Dintcheva N. T. (2022). Recycled (Bio)Plastics and (Bio)Plastic composites: A trade opportunity in a green future. Polymers (Basel) 14 (10), 2038–2062. doi: 10.3390/polym14102038
Muller G., Varnai A., Johansen K. S., Eijsink V. G., Horn S. J. (2015). Harnessing the potential of LPMO-containing cellulase cocktails poses new demands on processing conditions. Biotechnol. Biofuels 8, 187. doi: 10.1186/s13068-015-0376-y
North M. (2019). Across the board: michael north on carbon dioxide biorefinery. ChemSusChem 12 (8), 1763–1765. doi: 10.1002/cssc.201900676
Ogunyewo O. A., Upadhyay P., Rajacharya G. H., Okereke O. E., Faas L., Gomez L. D., et al. (2021). Accessory enzymes of hypercellulolytic Penicillium funiculosum facilitate complete saccharification of sugarcane bagasse. Biotechnol. Biofuels 14 (1), 171. doi: 10.1186/s13068-021-02020-x
Oh Y. K., Kim S., Ilhamsyah D. P. A., Lee S. G., Kim J. R. (2022). Cell disruption and lipid extraction from Chlorella species for biorefinery applications: Recent advances. Bioresour Technol. 366, 128183. doi: 10.1016/j.biortech.2022.128183
Olson E. S., Sharma R. K., Aulich T. R. (2004). Higher-alcohols biorefinery: improvement of catalyst for ethanol conversion. Appl. Biochem. Biotechnol. 113-116, 913–932. doi: 10.1385/ABAB:115:1-3:0913
Oruganti R. K., Biji A. P., Lanuyanger T., Show P. L., Sriariyanun M., Upadhyayula V. K. K., et al. (2023). Artificial intelligence and machine learning tools for high-performance microalgal wastewater treatment and algal biorefinery: A critical review. Sci. Total Environ. 876, 162797. doi: 10.1016/j.scitotenv.2023.162797
Papachristou I., Akaberi S., Silve A., Navarro-Lopez E., Wustner R., Leber K., et al. (2021). Analysis of the lipid extraction performance in a cascade process for Scenedesmus almeriensis biorefinery. Biotechnol. Biofuels 14 (1), 20. doi: 10.1186/s13068-020-01870-1
Papageorgiou A., Azzi E. S., Enell A., Sundberg C. (2021). Biochar produced from wood waste for soil remediation in Sweden: Carbon sequestration and other environmental impacts. Sci. Total Environ. 776, 145953. doi: 10.1016/j.scitotenv.2021.145953
Parchami M., Ferreira J. A., Taherzadeh M. J. (2021). Starch and protein recovery from brewer’s spent grain using hydrothermal pretreatment and their conversion to edible filamentous fungi - A brewery biorefinery concept. Bioresour Technol. 337, 125409. doi: 10.1016/j.biortech.2021.125409
Pendon M. D., Madeira J. V. Jr., Romanin D. E., Rumbo M., Gombert A. K., Garrote G. L. (2021). A biorefinery concept for the production of fuel ethanol, probiotic yeast, and whey protein from a by-product of the cheese industry. Appl. Microbiol. Biotechnol. 105 (9), 3859–3871. doi: 10.1007/s00253-021-11278-y
Pereira B., Marcondes W. F., Carvalho W., Arantes V. (2021). High yield biorefinery products from sugarcane bagasse: Prebiotic xylooligosaccharides, cellulosic ethanol, cellulose nanofibrils and lignin nanoparticles. Bioresour Technol. 342, 125970. doi: 10.1016/j.biortech.2021.125970
Praveen M. A., Parvathy K. R. K., Balasubramanian P., Jayabalan R. (2019). An overview of extraction and purification techniques of seaweed dietary fibers for immunomodulation on gut microbiota. Trends Food Sci. Technol. 92, 46–64. doi: 10.1016/j.tifs.2019.08.011
Pryor S. W., Karki B., Nahar N. (2012). Effect of hemicellulase addition during enzymatic hydrolysis of switchgrass pretreated by soaking in aqueous ammonia. Bioresour Technol. 123, 620–626. doi: 10.1016/j.biortech.2012.07.040
Rehman S., Khairul Islam M., Khalid Khanzada N., Kyoungjin An A., Chaiprapat S., Leu S. Y. (2021). Whole sugar 2,3-butanediol fermentation for oil palm empty fruit bunches biorefinery by a newly isolated Klebsiella pneumoniae PM2. Bioresour Technol. 333, 125206. doi: 10.1016/j.biortech.2021.125206
Saini R., Osorio-Gonzalez C. S., Hegde K., Brar S. K., Magdouli S., Vezina P., et al. (2020). Lignocellulosic biomass-based biorefinery: an insight into commercialization and economic standout. Curr. Sustainable/Renewable Energy Rep. 7 (4), 122–136. doi: 10.1007/s40518-020-00157-1
Sajjadi B., Chen W.-Y., Raman A. A. A., Ibrahim S. (2018). Microalgae lipid and biomass for biofuel production: A comprehensive review on lipid enhancement strategies and their effects on fatty acid composition. Renewable Sustain. Energy Rev. 97, 200–232. doi: 10.1016/j.rser.2018.07.050
Sarkar O., Agarwal M., Naresh Kumar A., Venkata Mohan S. (2015). Retrofitting hetrotrophically cultivated algae biomass as pyrolytic feedstock for biogas, bio-char and bio-oil production encompassing biorefinery. Bioresour Technol. 178, 132–138. doi: 10.1016/j.biortech.2014.09.070
Sheldon R. A., Woodley J. M. (2018). Role of biocatalysis in sustainable chemistry. Chem. Rev. 118 (2), 801–838. doi: 10.1021/acs.chemrev.7b00203
Shikina E. V., Kovalevsky R. A., Shirkovskaya A. I., Toukach P. V. (2022). Prospective bacterial and fungal sources of hyaluronic acid: A review. Comput. Struct. Biotechnol. J. 20, 6214–6236. doi: 10.1016/j.csbj.2022.11.013
Subhadra B., Grinson G. (2011). Algal biorefinery-based industry: an approach to address fuel and food insecurity for a carbon-smart world. J. Sci. Food Agric. 91 (1), 2–13. doi: 10.1002/jsfa.4207
Sun F. F., Hong J., Hu J., Saddler J. N., Fang X., Zhang Z., et al. (2015). Accessory enzymes influence cellulase hydrolysis of the model substrate and the realistic lignocellulosic biomass. Enzyme Microb. Technol. 79-80, 42–48. doi: 10.1016/j.enzmictec.2015.06.020
Sun Q., Meng J., Sarkar B., Lan Y., Lin L., Li H., et al. (2020). Long-term influence of maize stover and its derived biochar on soil structure and organo-mineral complexes in Northeast China. Environ. Sci. pollut. Res. Int. 27 (22), 28374–28383. doi: 10.1007/s11356-020-08171-y
Takkellapati S., Li T., Gonzalez M. A. (2018). An overview of biorefinery derived platform chemicals from a cellulose and hemicellulose biorefinery. Clean Technol. Environ. Policy 20 (7), 1615–1630. doi: 10.1007/s10098-018-1568-5
Taylor L. E. 2nd;, Knott B. C., Baker J. O., Alahuhta P. M., Hobdey S. E., Linger J. G., et al. (2018). Engineering enhanced cellobiohydrolase activity. Nat. Commun. 9 (1), 1186. doi: 10.1038/s41467-018-03501-8
Tharak A., Mohan S. V. (2022). Syngas fermentation to acetate and ethanol with adaptative electroactive carboxydotrophs in single chambered microbial electrochemical system. Micromachines (Basel) 13 (7), 980–997. doi: 10.3390/mi13070980
Thew C. X. E., Lee Z. S., Srinophakun P., Ooi C. W. (2023). Recent advances and challenges in sustainable management of plastic waste using biodegradation approach. Bioresour Technol. 374, 128772. doi: 10.1016/j.biortech.2023.128772
Ullah M. W., Khattak W. A., Ul-Islam M., Khan S., Park J. K. (2016). Metabolic engineering of synthetic cell-free systems: Strategies and applications. Biochem. Eng. J. 105, 391–405. doi: 10.1016/j.bej.2015.10.023
Upton D. J., Kaushal M., Whitehead C., Faas L., Gomez L. D., McQueen-Mason S. J., et al. (2022). Integration of Aspergillus niger transcriptomic profile with metabolic model identifies potential targets to optimise citric acid production from lignocellulosic hydrolysate. Biotechnol. Biofuels Bioprod 15 (1), 4. doi: 10.1186/s13068-021-02099-2
Usmani Z., Sharma M., Awasthi A. K., Lukk T., Tuohy M. G., Gong L., et al. (2021). Lignocellulosic biorefineries: The current state of challenges and strategies for efficient commercialization. Renewable Sustain. Energy Rev. 148, 111258. doi: 10.1016/j.rser.2021.111258
Valladares-Diestra K. K., Porto de Souza Vandenberghe L., Soccol C. R. (2021). A biorefinery approach for enzymatic complex production for the synthesis of xylooligosaccharides from sugarcane bagasse. Bioresour Technol. 333, 125174. doi: 10.1016/j.biortech.2021.125174
Wang C., Thygesen A., Liu Y., Li Q., Yang M., Dang D., et al. (2013). Bio-oil based biorefinery strategy for the production of succinic acid. Biotechnol. Biofuels 6, 74. doi: 10.1186/1754-6834-6-74
Wiesberg I. L., Brigagao G. V., de Medeiros J. L. (2017). de Queiroz Fernandes Araujo, O., Carbon dioxide utilization in a microalga-based biorefinery: Efficiency of carbon removal and economic performance under carbon taxation. J. Environ. Manage 203 (Pt 3), 988–998. doi: 10.1016/j.jenvman.2017.03.005
Wilding K. M., Schinn S. M., Long E. A., Bundy B. C. (2018). The emerging impact of cell-free chemical biosynthesis. Curr. Opin. Biotechnol. 53, 115–121. doi: 10.1016/j.copbio.2017.12.019
Xue X., Bi F., Liu B., Li J., Zhang L., Zhang J., et al. (2021). Improving citric acid production of an industrial Aspergillus niger CGMCC 10142: identification and overexpression of a high-affinity glucose transporter with different promoters. Microb. Cell Fact 20 (1), 168. doi: 10.1186/s12934-021-01659-3
Yadav G., Dash S. K., Sen R. (2019). A biorefinery for valorization of industrial waste-water and flue gas by microalgae for waste mitigation, carbon-dioxide sequestration and algal biomass production. Sci. Total Environ. 688, 129–135. doi: 10.1016/j.scitotenv.2019.06.024
Yang C. T., Kristiani E., Leong Y. K., Chang J. S. (2023). Big data and machine learning driven bioprocessing - Recent trends and critical analysis. Bioresour Technol. 372, 128625. doi: 10.1016/j.biortech.2023.128625
Yang Y., Yang J., Liu J., Wang R., Liu L., Wang F., et al. (2018). The composition of accessory enzymes of Penicillium chrysogenum P33 revealed by secretome and synergistic effects with commercial cellulase on lignocellulose hydrolysis. Bioresour Technol. 257, 54–61. doi: 10.1016/j.biortech.2018.02.028
Yogalakshmi K. N., Usman T.M. M., Kavitha S., Sachdeva S., Thakur S., Kumar S. A., et al. (2023). Lignocellulosic biorefinery technologies: A perception into recent advances in biomass fractionation, biorefineries, economic hurdles and market outlook. Fermentation 9 (3), 238. doi: 10.3390/fermentation9030238
Yuan Y., Macquarrie D. J. (2015). Microwave assisted step-by-step process for the production of fucoidan, alginate sodium, sugars and biochar from Ascophyllum nodosum through a biorefinery concept. Bioresour Technol. 198, 819–827. doi: 10.1016/j.biortech.2015.09.090
Keywords: bio-refinery, bioconversion, biofuels, bio-products, carbon management, bioeconomy, industrial microbiology
Citation: Decker SR, Brunecky R, Yarbrough JM and Subramanian V (2023) Perspectives on biorefineries in microbial production of fuels and chemicals. Front. Ind. Microbiol. 1:1202269. doi: 10.3389/finmi.2023.1202269
Received: 07 April 2023; Accepted: 17 July 2023;
Published: 04 August 2023.
Edited by:
Lynn M. Wendt, Idaho National Laboratory (DOE), United StatesReviewed by:
Nilesh Kumar Sharma, Praj Industries Limited, IndiaCopyright © 2023 Decker, Brunecky, Yarbrough and Subramanian. This is an open-access article distributed under the terms of the Creative Commons Attribution License (CC BY). The use, distribution or reproduction in other forums is permitted, provided the original author(s) and the copyright owner(s) are credited and that the original publication in this journal is cited, in accordance with accepted academic practice. No use, distribution or reproduction is permitted which does not comply with these terms.
*Correspondence: Stephen R. Decker, c3RldmUuZGVja2VyQG5yZWwuZ292