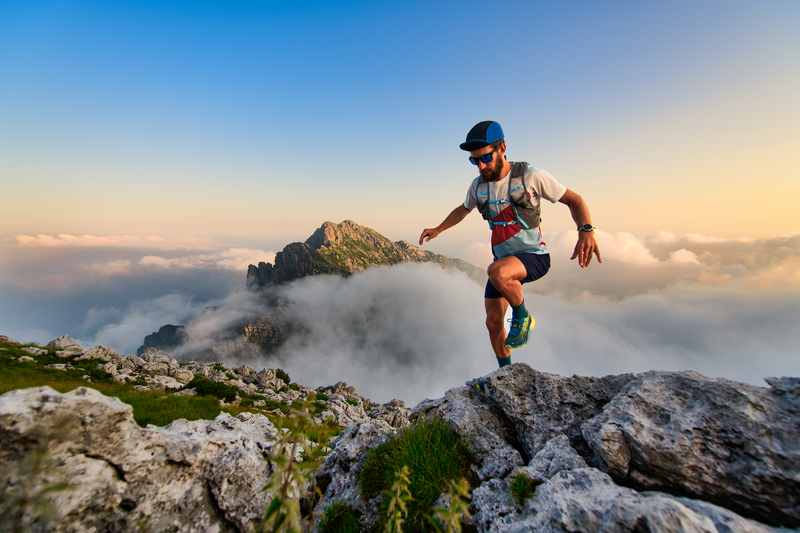
95% of researchers rate our articles as excellent or good
Learn more about the work of our research integrity team to safeguard the quality of each article we publish.
Find out more
MINI REVIEW article
Front. Immunol. , 18 March 2025
Sec. Cancer Immunity and Immunotherapy
Volume 16 - 2025 | https://doi.org/10.3389/fimmu.2025.1579822
Osteosarcoma remains a highly aggressive bone malignancy with limited therapeutic options, necessitating novel treatment strategies. Immunotherapy has emerged as a promising approach, yet its efficacy in osteosarcoma is hindered by an immunosuppressive tumor microenvironment and resistance mechanisms. This review explores recent advancements in checkpoint blockade, cellular therapies, and combination strategies aimed at enhancing immune responses. We highlight key challenges, including tumor heterogeneity, poor immune infiltration, and the need for predictive biomarkers. By integrating immunotherapy with chemotherapy, radiotherapy, and targeted therapy, emerging approaches seek to improve treatment outcomes. This review provides a comprehensive analysis of the evolving landscape of osteosarcoma immunotherapy, offering insights into future directions and potential breakthroughs. Researchers and clinicians will benefit from understanding these developments, as they pave the way for more effective and personalized therapeutic strategies in osteosarcoma.
Osteosarcoma is the most common primary malignant bone tumor, predominantly affecting children, adolescents, and young adults (1). The global incidence of osteosarcoma is estimated at 3–5 cases per million annually, with a peak occurrence in the second decade of life, coinciding with periods of rapid bone growth. Despite significant advances in multimodal treatment strategies, including surgery and chemotherapy, the long-term prognosis for patients with metastatic or recurrent osteosarcoma remains poor (2). The current five-year survival rate for localized osteosarcoma approaches 60–70%, whereas it drops to less than 30% in cases with metastasis, particularly involving the lungs. The aggressive nature of osteosarcoma, coupled with its high propensity for distant dissemination and resistance to conventional therapies, underscores the urgent need for novel and more effective treatment approaches. Historically, the standard-of-care for osteosarcoma has comprised neoadjuvant chemotherapy, surgical resection, and adjuvant chemotherapy. While this regimen has improved survival rates over past decades, it has largely reached a therapeutic plateau, with minimal advances in overall survival observed in recent years. Moreover, the efficacy of traditional cytotoxic agents is often hindered by severe adverse effects, multidrug resistance, and inadequate control of micro-metastatic disease. Radiotherapy, though occasionally used in unresectable or palliative settings, has limited effectiveness due to the relative radioresistance of osteosarcoma cells (3). Given these limitations, there is a pressing demand for alternative therapeutic strategies that can enhance treatment efficacy while minimizing systemic toxicity.
Immunotherapy has emerged as a promising approach to overcoming the limitations of conventional treatments by leveraging the body’s immune system to recognize and eradicate tumor cells (4). Recent advances in tumor immunology have revealed that osteosarcoma, despite being historically considered immunologically “cold,” exhibits an immunosuppressive tumor microenvironment (TME) that can be modulated to improve immune responsiveness (5, 6). Among immunotherapeutic strategies, immune checkpoint blockade (ICB) targeting the programmed cell death protein 1 (PD-1)/PD-1 ligand (PD-L1) and cytotoxic T-lymphocyte antigen 4 (CTLA-4)pathways has demonstrated clinical success in several cancers and is now being actively explored in osteosarcoma (7, 8). Additionally, cellular therapies, including chimeric antigen receptor (CAR)-T cells, CAR-natural killer (NK) cells, and dendritic cell (DC) vaccines, offer novel avenues for enhancing anti-tumor immunity (9, 10). Given the complex interplay between immune evasion mechanisms in OS, combination strategies integrating immunotherapy with chemotherapy, radiotherapy, or targeted agents are being investigated to potentiate therapeutic efficacy.
This review provides a comprehensive overview of emerging immunotherapies in osteosarcoma, with a particular focus on checkpoint blockade, cellular therapies, and combinatorial approaches. By discussing current advancements, challenges, and future directions, we aim to highlight the potential of immunotherapy in reshaping the treatment landscape for OS and improving patient outcomes.
OS is characterized by a highly immunosuppressive TME, which plays a crucial role in tumor progression, immune evasion, and therapeutic resistance. Unlike immunologically “hot” tumors that are heavily infiltrated with cytotoxic T cells, osteosarcoma exhibits a predominantly immune-excluded or immune-suppressed phenotype, limiting the efficacy of immunotherapy (11, 12). The osteosarcoma TME is composed of various immune cells, including tumor-associated macrophages (TAMs), T cells, NK cells, and regulatory T cells (Tregs), which collectively contribute to an immunosuppressive niche. Additionally, osteosarcoma cells exploit immune checkpoint pathways, such as PD-1/PD-L1, CTLA-4, lymphocyte activation gene-3(LAG-3), and T cell immunoglobulin and mucin domain-containing protein 3(TIM-3), to evade immune surveillance. The cytokine and chemokine milieu further modulates immune cell infiltration and activity, reinforcing the immune-suppressive landscape of the tumor. Understanding the mechanisms underlying this immunosuppressive TME is critical for developing effective immunotherapeutic strategies for osteosarcoma.
The cellular composition of the osteosarcoma TME plays a pivotal role in dictating the immune response. Various immune cell populations, including TAMs, T cells, NK cells, and Tregs, contribute to the immunosuppressive state of osteosarcoma, promoting tumor progression and resistance to immune-based therapies.
TAMs are a dominant immune cell population in osteosarcoma, often displaying an M2-like, pro-tumorigenic phenotype. These macrophages promote tumor progression through multiple mechanisms, including immune suppression, angiogenesis, and extracellular matrix remodeling (13, 14). TAMs in osteosarcoma secrete high levels of immunosuppressive cytokines such as interleukin-10 (IL-10) and transforming growth factor-beta (TGF-β), which inhibit the activation and proliferation of cytotoxic T lymphocytes (CTLs) and NK cells (15). For instance, high infiltration of CD163+ M2-like macrophages in osteosarcoma correlates with poor prognosis and increased metastatic potential (16). Additionally, TAMs contribute to immune evasion by upregulating the expression of PD-L1, which interacts with PD-1 on T cells to induce T cell exhaustion (17). Targeting TAMs with colony-stimulating factor receptor (CSF1R) inhibitors or repolarizing them toward an M1-like phenotype using toll-like receptor (TLR) agonists has been proposed as a potential strategy to overcome macrophage-mediated immunosuppression in osteosarcoma (18).
Although osteosarcoma tumors contain T cell infiltrates, their functionality is severely compromised. CD8+ cytotoxic T cells, which are critical for anti-tumor immunity, exhibit reduced effector function due to chronic antigen exposure and persistent immune checkpoint signaling. The phenomenon of T cell exhaustion is well-documented in osteosarcoma, with tumor-infiltrating T cells expressing high levels of PD-1, TIM-3, and LAG-3—markers associated with dysfunctional and anergic T cells (19, 20). A study analyzing osteosarcoma biopsies found that while CD8+ T cells were present in the TME, they lacked the ability to proliferate and secrete effector cytokines such as interferon-gamma (IFN-γ) and tumor necrosis factor-alpha (TNF-α) (19, 21). Moreover, the presence of CD4+ helper T cells is often skewed towards Treg phenotype, which further suppresses cytotoxic responses (22). Efforts to restore T cell function in osteosarcoma have focused on checkpoint blockade therapy, but the response rates have remained suboptimal, suggesting the need for combination strategies to reinvigorate T cell-mediated immunity.
NK cells are innate immune effectors capable of recognizing and eliminating tumor cells without prior antigen sensitization (23). However, in osteosarcoma, the activity of NK cells is significantly suppressed due to multiple factors. Tumor-derived TGF-β downregulates the expression of activating receptors such as NKG2D on NK cells, impairing their ability to recognize and kill osteosarcoma cells (24). Furthermore, osteosarcoma cells frequently shed soluble ligands for NKG2D, further inhibiting NK cell-mediated cytotoxicity. Increasing NK cell infiltration through adoptive NK cell transfer or cytokine stimulation (e.g., IL-15 administration) can enhance anti-tumor immunity in osteosarcoma (25). Additionally, CAR-NK cell therapies targeting osteosarcoma-associated antigens such as B7-H3 are under investigation as potential strategies to overcome NK cell dysfunction in osteosarcoma (26).
Tregs play a crucial role in maintaining immune tolerance, but in the context of cancer, they act as potent suppressors of anti-tumor immunity. osteosarcoma tumors often exhibit an increased frequency of CD4+CD25+FoxP3+ Tregs, which suppress the activity of CTLs and NK cells through the secretion of IL-10 and TGF-β (27, 28). Tregs interact with osteoblastic, endothelial, and myeloid cells through C-X-C motif chemokine ligand (CXCL) signaling, particularly affecting the expression of C-X-C motif chemokine receptor 4 (CXCR4). These interactions, primarily mediated by CXCL12 and transforming growth factor β1 (TGFB1), collectively facilitate tumor growth and progression (28). Additionally, Tregs express high levels of CTLA-4, which competes with CD28 for binding to B7 molecules on antigen-presenting cells, thereby dampening T cell activation. Agents such as anti-CD25 antibodies and CTLA-4 inhibitors are being explored for their potential to reduce Treg-mediated immunosuppression and restore effective anti-tumor responses.
The cytokine and chemokine landscape in osteosarcoma play a pivotal role in shaping immune cell recruitment and function, ultimately influencing tumor progression and immune evasion. Immunosuppressive cytokines such as TGF-β and IL-10 are prominently involved in promoting Treg expansion and suppressing effector T cell activity, thereby fostering an immunosuppressive TME. Conversely, pro-inflammatory cytokines like IFN-γ and IL-12 are critical for enhancing anti-tumor immunity; however, their expression is frequently suppressed in osteosarcoma, limiting their protective effects. Chemokines further contribute to this complex interplay: CCL2 and CCL5 facilitate the recruitment of TAMs and Tregs, while CXCL9 and CXCL10, which are essential for promoting T cell infiltration, are often downregulated in osteosarcoma (29, 30). To counteract these immunosuppressive mechanisms, therapeutic strategies aimed at modulating the cytokine and chemokine milieu are under investigation. For instance, IL-12 gene therapy has shown potential in restoring anti-tumor immunity (31), and CXCR4 antagonists are being explored to disrupt chemokine-mediated immune suppression (32). Osteosarcoma cells also secrete CXCL14 that activates integrinα11β1 on fibroblasts to form a lung metastatic niche (33).
Immune checkpoint blockade has revolutionized cancer immunotherapy by enhancing the immune system’s ability to recognize and eliminate tumor cells (34). However, in osteosarcoma, the efficacy of checkpoint inhibitors remains suboptimal due to the inherently immunosuppressive TME and various resistance mechanisms. Although osteosarcoma has historically been classified as an immunologically “cold” tumor with low levels of T cell infiltration, growing evidence suggests that modulating immune checkpoints reshape the TME and enhance anti-tumor immunity (7). The most widely studied immune checkpoints in osteosarcoma include PD-1/PD-L1 pathway and the CTLA-4 pathway. However, newer checkpoint molecules such as LAG-3, TIM-3, T cell immunoreceptor with Ig and ITIM domains (TIGIT), V-domain Ig suppressor of T cell activation (VISTA), and B7-H3 (CD276) have gained increasing attention as potential targets in osteosarcoma.
The PD-1/PD-L1 axis plays a critical role in immune evasion in osteosarcoma. PD-L1 is frequently upregulated on osteosarcoma cells, particularly in response to IFN-γ signaling. Engagement of PD-L1 with PD-1 on T cells leads to T cell exhaustion, reducing their ability to proliferate and produce effector cytokines such as IFN-γ and TNF-α.
Clinical trials evaluating PD-1 inhibitors (e.g., pembrolizumab, nivolumab) and PD-L1 inhibitors (e.g., atezolizumab, durvalumab) in osteosarcoma patients have demonstrated mixed outcomes. The SARC028 trial (NCT02301039), a phase II study investigating pembrolizumab in bone and soft tissue sarcomas, reported limited efficacy in osteosarcoma, with an overall response rate (ORR) of only 5% (35). Similarly, nivolumab monotherapy trials have shown minimal clinical benefit, as disease progression was observed in the majority of osteosarcoma patients (36). However, these trials have limitations, including small sample sizes, lack of biomarker-driven patient selection, and heterogeneous treatment responses. For example, most trials do not stratify patients based on PD-L1 expression levels, tumor mutational burden (TMB), or the composition of TME, which may impact treatment outcomes. In contrast, combination approaches have shown more promising results; for example, a trial combining pembrolizumab with axitinib, a VEGFR inhibitor, demonstrated improved disease control, suggesting that targeting the tumor vasculature may enhance the efficacy of immunotherapy in osteosarcoma (37). These findings highlight the potential of combination strategies to overcome the limitations of monotherapy in this challenging disease.
The limited efficacy of PD-1/PD-L1 blockade in osteosarcoma can be attributed to several key factors. First, osteosarcoma exhibits a relatively low TMB compared to malignancies such as melanoma or lung cancer, resulting in fewer neoantigens available for immune recognition (38). Low TMB reduces the likelihood of generating sufficient tumor-specific neoantigens that can be recognized by T cells, thereby limiting the efficacy of immune checkpoint blockade. Recent studies suggest that enhancing TMB through genetic or epigenetic modifications, such as using DNA methyltransferase inhibitors, may improve response rates to checkpoint inhibitors in osteosarcoma. Second, deficiencies in antigen presentation further contribute to resistance. Osteosarcoma cells often downregulate major histocompatibility complex class I (MHC-I) molecules, which are critical for presenting tumor antigens to cytotoxic T cells. Loss of MHC-I expression impairs immune recognition, leading to immune evasion. Strategies to restore antigen presentation, such as IFN-γ treatment or histone deacetylase inhibitors (HDACis), have been explored as potential approaches to enhance immune checkpoint therapy efficacy in osteosarcoma. Third, the immunosuppressive TME in osteosarcoma inhibits T cell function and undermine anti-tumor immunity (6). Fourth, heterogeneous PD-L1 expression among osteosarcoma patients further limits the uniform effectiveness of PD-1/PD-L1 blockade, as not all patients exhibit sufficient PD-L1 levels to benefit from this therapy (39). To address these challenges, combination strategies that target multiple resistance mechanisms are actively being explored. For example, combining PD-1/PD-L1 blockade with therapies aimed at increasing antigen presentation (e.g., HDAC inhibitors), reprogramming the TME (e.g., CSF1R inhibitors, IL-10 blockade), or enhancing TMB through epigenetic modulation (e.g., DNA methyltransferase inhibitors) represents a promising approach to improve therapeutic outcomes.
CTLA-4 is an inhibitory receptor expressed on T cells that competes with CD28 for binding to B7 molecules on antigen-presenting cells (APCs). By preventing co-stimulatory signaling, CTLA-4 inhibits T cell activation and expansion. Preclinical and clinical studies investigating CTLA-4 inhibitors in osteosarcoma have yielded limited but promising insights. Ipilimumab, an anti-CTLA-4 antibody, has demonstrated significant efficacy in melanoma; however, its clinical benefit in osteosarcoma remains uncertain. A pilot study evaluating ipilimumab in pediatric sarcomas reported modest disease stabilization, suggesting potential yet limited activity in osteosarcoma. Similarly, tremelimumab, another CTLA-4 inhibitor, has been tested in combination with PD-L1 inhibitors, but no significant survival benefit has been observed in osteosarcoma to date (40). These findings highlight the need for further research to optimize CTLA-4 blockade strategies, potentially through combination therapies or biomarker-driven patient selection, to enhance therapeutic outcomes in osteosarcoma.
Combination strategies are being explored to improve the limited efficacy of CTLA-4 monotherapy. One such approach is combining CTLA-4 blockade with PD-1 inhibition. In melanoma, the combination of ipilimumab (CTLA-4 inhibitor) and nivolumab (PD-1 inhibitor) has shown significant improvements in survival rates compared to monotherapy (41, 42). A phase I/II trial evaluated the combination of nivolumab and ipilimumab in children and young adults with recurrent/refractory osteosarcoma tumors. The study tested two dose levels (DL1 and DL2) for safety and established the recommended phase II dose (RP2D) for pediatric patients. However, this trial also faced challenges, including immune-related adverse events (irAEs), variability in response rates, and the need for longer follow-up to determine overall survival benefits. The RP2D combination was well tolerated and demonstrated some clinical activity in these pediatric patients with solid tumors (43). Preclinical studies in osteosarcoma have also suggested that dual checkpoint inhibition could enhance anti-tumor immunity. Another promising combination is CTLA-4 blockade with radiotherapy. Radiation therapy, by inducing immunogenic cell death, can increase tumor antigen release and immune cell infiltration. For example, studies using murine models have shown that the combination of CTLA-4 blockade and radiation therapy results in improved tumor control and enhanced immune responses, suggesting this combination may be effective in treating various cancers. Future trials are focusing on refining patient selection criteria by incorporating immune profiling and genomic analyses to identify responders more accurately. For example, assessing tumor neoantigen load and immune cell infiltration levels may help predict which patients will benefit most from dual checkpoint blockade.
LAG-3 is co-expressed with PD-1 on exhausted T cells and contributes to immune suppression, making it an attractive target for immune checkpoint therapy (44, 45). Blocking LAG-3 enhances T cell proliferation and cytokine production, suggesting a potential strategy to restore immune function in tumors (46). LAG-3 is highly expressed in osteosarcoma (47).One promising combination is the use of relatlimab (anti-LAG-3) alongside nivolumab (anti-PD-1), which has shown encouraging results in melanoma and other solid tumors (48, 49). This combination is now being investigated in osteosarcoma to determine its potential efficacy (50). Preclinical models of osteosarcoma have demonstrated that LAG-3 blockade, when combined with PD-1 inhibition, results in increased tumor regression in murine models, further supporting the therapeutic potential of targeting LAG-3 in osteosarcoma.
TIM-3 is another key exhaustion marker on T cells and plays a significant role in immune evasion (51). It is also expressed on TAMs, where it contributes to their immunosuppressive function (52). A phase I/II study evaluated the safety and efficacy of sabatolimab (MBG453), an anti-TIM-3 monoclonal antibody, with or without spartalizumab (anti-PD-1), in patients with advanced solid tumors. The results showed that sabatolimab plus spartalizumab was well tolerated and demonstrated preliminary signs of antitumor activity (53). But studies in osteosarcoma for co-blockade of TIM-3 and PD-1 require further study. It could be a promising strategy to overcome immune suppression and improve therapeutic outcomes in osteosarcoma.
TIGIT is an emerging immune checkpoint that suppresses the activity of both NK cells and T cells, contributing to immune evasion in tumors (54). Tiragolumab, an anti-TIGIT antibody, has shown promise when combined with PD-L1 blockade in non-small cell lung cancer and is currently being investigated in osteosarcoma (55). In preclinical models of osteosarcoma, blocking TIGIT has been shown to enhance NK cell-mediated cytotoxicity, suggesting that targeting TIGIT could improve the anti-tumor immune response (56, 57). This combination strategy holds potential for overcoming immune suppression and improving treatment outcomes in osteosarcoma.
Despite the promise of checkpoint inhibitors, resistance remains a significant challenge in cancer therapy. Several mechanisms of resistance have been identified. The low tumor mutational burden (TMB) of osteosarcoma leads to reduced neoantigen availability, limiting T cell recognition and immune activation. Enhancing TMB through mutagenic therapies or epigenetic modulation has been proposed to overcome this challenge. Additionally, antigen presentation deficiencies, particularly the downregulation of MHC-I molecules, impair T cell recognition of osteosarcoma cells. Strategies such as IFN-γ stimulation or the use of histone deacetylase inhibitors (HDACis) have been explored to restore MHC-I expression and improve antigen presentation. The adaptive upregulation of other immune checkpoints, such as LAG-3, TIM-3, or VISTA, following PD-1 blockade, necessitates the development of multi-target approaches to overcome this compensatory immune evasion. Additionally, TME-mediated resistance, characterized by the suppression of T cell activation through MDSCs, Tregs, and TAMs, further contributes to immune escape. Additionally, deficient antigen presentation, such as the downregulation of MHC-I molecules in osteosarcoma cells, impairs T cell recognition and limits the effectiveness of immune checkpoint inhibitors. Addressing these resistance mechanisms through combination therapies and strategies to enhance antigen presentation is critical for improving response rates and overcoming therapeutic resistance.
Several strategies have been proposed to overcome resistance to checkpoint inhibitors. Epigenetic modulators, such as hypomethylating agents, upregulate immune-related genes, thereby enhancing the efficacy of checkpoint inhibitors. Cytokine-based therapies, including IL-12 and IL-15, promote the activation of T cells and NK cells, potentially improving immune responses (58). Additionally, oncolytic viruses, which are engineered to selectively infect and kill tumor cells, can increase tumor antigen release, further enhancing the efficacy of immune checkpoint blockade.
CAR-T cell therapy has demonstrated remarkable success in hematologic malignancies, yet its efficacy in solid tumors like osteosarcoma remains limited (59, 60). The development of CAR-T therapy for osteosarcoma has focused on identifying suitable tumor-associated antigens (TAAs) that are highly expressed in osteosarcoma cells while sparing normal tissues. However, despite promising preclinical data, clinical translation has been challenging due to factors such as antigen heterogeneity, T cell exhaustion, and the immunosuppressive tumor.
Targeting specific antigens in osteosarcoma has emerged as a promising therapeutic strategy. GD2, a disialoganglioside, is highly expressed in pediatric solid tumors, including osteosarcoma (61, 62) (Figure 1). Preclinical studies have shown that GD2-targeted CAR-T cells exhibit potent anti- osteosarcoma activity in vitro and in mouse models. However, a phase I clinical trial (NCT02107963) evaluating GD2-CAR-T therapy in osteosarcoma patients demonstrated limited efficacy, with only transient tumor regression observed in some patients. The lack of durable responses was attributed to T cell exhaustion and immune escape mechanisms (9). HER2, a human epidermal growth factor receptor, is variably expressed in osteosarcoma, but targeting it remains a viable approach (63, 64). A phase I clinical trial (NCT00902044) testing HER2-CAR-T cells in osteosarcoma patients showed safety but only modest efficacy, with a lack of sustained responses linked to T cell exhaustion and antigen heterogeneity (65). One of the key challenges has been antigen heterogeneity, leading to immune escape and limited response rates. Combination approaches, such as combining HER2-CAR-T cells with checkpoint inhibitors like PD-1 blockade, have shown improved persistence and tumor clearance in preclinical models. The bispecific antibodies (BsAbs) targeting GD2 and HER2 in osteosarcoma demonstrated potent anti-tumor effects both in vitro and in vivo. Preclinical studies indicate that combining BsAb therapy with anti-PD-L1 blockade enhances T cell activation and tumor clearance. However, clinical validation is still needed to determine the optimal patient selection criteria and therapeutic combinations. T cells armed with these BsAbs showed significant anti-tumor activity, and the combination of BsAbs with anti-PD-L1 antibodies further enhanced the anti-tumor response. These findings support clinical trials investigating GD2 and HER2-targeted T-BsAb therapy in combination with immune checkpoint inhibitors to improve treatment outcomes for osteosarcoma patients (66).
Figure 1. Immunotherapy and cell therapy for osteosarcoma. This figure illustrates the mechanisms of immune evasion in osteosarcoma and highlights potential immunotherapeutic and cell-based therapeutic strategies. T cells express immune checkpoint receptors such as PD-1, CTLA-4, TIM-3, and TIGIT, which interact with their respective ligands (e.g., PD-L1 and B7-H3) on osteosarcoma cells, leading to T cell exhaustion and immune evasion. The presence of HER2-CAR-T and GD2-CAR-T cells represents promising adoptive cell therapy approaches to enhance T cell-mediated tumor killing. Macrophages interact with osteosarcoma cells through the CD47-SIRPα axis, a “don’t eat me” signal that inhibits phagocytosis; blocking this pathway is a potential strategy to enhance macrophage-mediated tumor clearance. Osteosarcoma cells express immune checkpoint ligands such as PD-L1, B7-H3, CD70, and MCAM, which contribute to immune evasion by suppressing T cell and macrophage activity. Additionally, natural killer (NK) cells, which express TIM-3, play a role in targeting osteosarcoma cells, but their activity can be inhibited by tumor-derived signals. Enhancing NK cell function through immunotherapies, such as CAR-NK cells targeting CD70 or MCAM, is another promising therapeutic approach. Collectively, the figure underscores the importance of targeting immune checkpoint pathways (e.g., PD-1/PD-L1, CTLA-4, CD47-SIRPα) and utilizing adoptive cell therapies (e.g., CAR-T and CAR-NK cells) to overcome immune evasion in osteosarcoma and improve treatment outcomes.
B7-H3 (CD276) is overexpressed in osteosarcoma and plays a significant role in immune evasion (67). The B7-H3-targeting antibody-drug conjugate (ADC) m276-SL-PBD has demonstrated significant antitumor activity in pediatric solid tumor models, including patient-derived (PDX) and cell line-derived xenografts (CDX). In randomized trials, m276-SL-PBD achieved a 92.3% response rate, with 61.5% of models showing a maintained complete response (68). These findings support the clinical development of m276-SL-PBD for high-risk pediatric solid malignancies. Preclinical studies have demonstrated that B7-H3 CAR-T cells induce robust tumor regression in osteosarcoma mouse models. Early-phase clinical trials for B7-H3 CAR-T therapy are ongoing, with promising initial results, highlighting its potential as a target for osteosarcoma immunotherapy. Additionally, recent studies in canine models have shown that B7-H3 CAR-T cells can specifically target and kill B7-H3-expressing canine osteosarcoma cells both in vitro and in vivo. Furthermore, co-expressing a chemokine receptor (CXCR2) with the B7-H3 CAR construct significantly enhanced the anti-tumor activity of canine CAR-T cells, suggesting a potential strategy to improve CAR-T cell efficacy in osteosarcoma treatment (69).
CAR-modified NK cells offer several advantages over CAR-T cells in cancer therapy (70). First, they present a lower risk of cytokine release syndrome (CRS) and graft-versus-host disease (GVHD), which are common complications associated with CAR-T cell therapy. Second, NK cells have an innate tumor-killing capacity that is independent of antigen specificity, enabling them to target a wider range of tumor cells. Additionally, CAR-NK cells can function effectively in immunosuppressive tumor microenvironments, making them a promising therapeutic option in resistant cancers. However, a major limitation is the relatively short lifespan of adoptively transferred NK cells, which may reduce long-term efficacy in clinical settings.
CAR-NK cell therapy has emerged as a promising immunotherapeutic approach for osteosarcoma. Unlike traditional T-cell therapies, CAR-NK cells offer several advantages, including reduced risk of cytokine release syndrome, neurotoxicity, and graft-versus-host disease, thereby enhancing safety profiles (71). Preclinical studies have shown that CAR-NK cells targeting CD70 exhibit enhanced cytotoxicity against osteosarcoma cell lines, but the clinical relevance of CD70 expression in osteosarcoma patients remains to be validated (72, 73). Additionally, anti-melanoma cell adhesion molecule (MCAM) CAR-NK cells have shown significant antitumor activity in osteosarcoma models, suggesting that targeting MCAM could be a viable strategy for OS immunotherapy (74). However, the lack of standardized patient selection criteria and limited data on in vivo persistence remain key challenges. Combination approaches are being explored to enhance CAR-NK cell efficacy. For example, the IL-15 agonist NKTR-255 has been shown to prolong NK cell survival and enhance tumor-killing activity in preclinical models (25, 75). Similarly, CD47 blockade using magrolimab (MAG) has been reported to augment CAR-NK cell-mediated cytotoxicity by enhancing macrophage phagocytosis (75). While these approaches are promising, clinical data on the efficacy and durability of CAR-NK cell therapy in osteosarcoma are still limited, highlighting the need for further studies.
DCs are potent APCs that prime T cell responses against tumors. DC-based vaccines aim to enhance anti-tumor immunity by delivering tumor antigens to the immune system. DC-based vaccines represent a promising strategy for osteosarcoma immunotherapy. One approach involves autologous DC vaccines, where patient-derived DCs are loaded with osteosarcoma -associated antigens, such as tumor lysates or synthetic peptides, and reinfused into the patient to stimulate an anti-tumor immune response. A clinical trial using a DC vaccine pulsed with osteosarcoma tumor lysates reported prolonged progression-free survival in a subset of patients, but overall response rates remained low (76). In addition to autologous vaccines, combination strategies have been explored to enhance their effectiveness. Combining DC vaccines with checkpoint inhibitors, such as anti-PD-1 therapy, has shown improved tumor rejection in osteosarcoma models, indicating a synergistic effect (77). Another promising approach is combining DC vaccines with oncolytic viruses. These viruses, engineered to express tumor antigens, can enhance DC-mediated T cell priming, further boosting the anti-tumor immune response (78). Despite these advancements, limited clinical efficacy has been observed, highlighting the need for further optimization of DC vaccine platforms to achieve more consistent and durable therapeutic outcomes (5).
Given the complexity of osteosarcoma’s immune landscape, monotherapy approaches often fail to achieve durable responses. Combination strategies that integrate multiple immunotherapeutic modalities or pair immunotherapy with conventional treatments (e.g., chemotherapy, radiotherapy, and targeted therapy) have demonstrated enhanced efficacy in overcoming immune resistance. Emerging approaches, including oncolytic virotherapy, TLR agonists, and neoantigen-based vaccines, offer new opportunities to boost anti-tumor immunity.
A growing body of evidence suggests that combining immunotherapy with conventional treatment modalities enhance therapeutic efficacy in osteosarcoma by modifying the tumor microenvironment, increasing antigen presentation, and mitigating immunosuppressive mechanisms. Chemotherapy has long been a cornerstone of osteosarcoma treatment, and certain agents such as doxorubicin, cisplatin, and methotrexate have been shown to induce immunogenic cell death (ICD) (79). This process not only leads to tumor cell apoptosis but also enhances the exposure of tumor-associated antigens, thereby stimulating an adaptive immune response. Preclinical studies indicate that checkpoint blockade therapies combined with chemotherapy augment T cell activation and improve response rates in osteosarcoma models (80). However, the immunosuppressive effects of chemotherapy on lymphocytes and antigen-presenting cells remain a challenge, necessitating precise optimization of drug selection, sequencing, and dosing to preserve immune function while maximizing anti-tumor immunity.
Similarly, radiotherapy has emerged as a potent immunomodulator in osteosarcoma by promoting antigen release, increasing tumor immunogenicity, and upregulating immune checkpoint molecule expression such as PD-L1 (81). Ionizing radiation has been shown to enhance T cell infiltration into tumors, rendering osteosarcoma more susceptible to immunotherapy. The concept of the “abscopal effect,” wherein localized radiation induces systemic anti-tumor immune responses, has gained attention as a potential mechanism to enhance immunotherapy efficacy. Despite promising preclinical data, clinical evidence demonstrating the abscopal effect in osteosarcoma remains limited, highlighting the need for well-designed clinical trials. Current pre-clinical exploring the combination of radiotherapy with PD-1/PD-L1 inhibitors, as well as CAR-T cell therapy, to assess their synergistic potential in overcoming immune evasion and improving treatment outcomes in osteosarcoma patients are pending.
Targeted therapies have also been integrated into immunotherapy strategies for osteosarcoma, particularly tyrosine kinase inhibitors (TKIs) such as cabozantinib and sorafenib (82). These agents exhibit immunomodulatory properties by enhancing T cell infiltration, reducing Treg activity, and modulating MDSC populations. Preclinical studies suggest that TKIs increases tumor susceptibility to checkpoint blockade therapy, and clinical trials are currently assessing their combination with PD-1 inhibitors in various solid tumors, including osteosarcoma (83–85). In addition, indoleamine 2,3-dioxygenase (IDO) inhibitors, which target tryptophan metabolism, have been proposed as a strategy to reverse immune suppression in osteosarcoma by limiting the production of immunosuppressive metabolites that impair T cell function (86). However, their clinical success has been inconsistent, suggesting that further mechanistic insights into metabolic immune regulation in osteosarcoma are needed to refine their therapeutic application. Additionally, resistance to immune checkpoint blockade in osteosarcoma is influenced by multiple factors, including tumor mutational burden (TMB), antigen presentation deficiencies, and the immunosuppressive TME. Osteosarcoma is generally characterized by a low TMB, which may contribute to reduced neoantigen presentation and limited T cell recognition. Moreover, defects in antigen processing and presentation, such as the downregulation of MHC-I molecules, can impair immune detection and contribute to therapeutic resistance. The TME further exacerbates resistance by fostering an immunosuppressive milieu dominated by MDSCs, Tregs, and TAMs, all of which inhibit effective anti-tumor immunity. Addressing these resistance mechanisms through combination approaches, such as incorporating epigenetic modulators, metabolic reprogramming strategies, and novel immune checkpoint targets, is essential for enhancing response rates and improving clinical outcomes in osteosarcoma.
Despite the promise of combination strategies, several challenges remain in optimizing their clinical application. The timing, dosing, and sequencing of these therapies must be carefully calibrated to balance tumor cytotoxicity with immune stimulation while minimizing toxicity. Furthermore, osteosarcoma exhibits significant molecular heterogeneity, necessitating a personalized approach that incorporates molecular profiling and immune biomarkers to tailor treatment regimens for individual patients (87, 88). A deeper understanding of osteosarcoma’s immune microenvironment heterogeneity is crucial to identifying patient subgroups that may benefit most from specific combination strategies.
Advances in systems biology, machine learning, and high-throughput screening may aid in identifying optimal combination strategies that maximize therapeutic efficacy while mitigating adverse effects.
Immunotherapy has emerged as a promising strategy for osteosarcoma, a malignancy with historically limited treatment options and poor outcomes in relapsed or metastatic cases (5). Over the past decade, significant advances have been made in understanding the immunosuppressive tumor microenvironment, leading to the development of various immune-based interventions. Checkpoint blockade therapies, cellular therapies such as CAR-T and CAR-NK cells, and combination strategies integrating oncolytic virotherapy, microbiome modulation, Toll-like receptor agonists, and neoantigen-based vaccines have shown potential in preclinical and early-phase clinical studies. However, despite these advances, the clinical efficacy of immunotherapy in osteosarcoma remains inconsistent due to tumor heterogeneity, immune evasion mechanisms, and insufficient immune infiltration.
To translate these promising findings into meaningful clinical benefits, further translational research and well-designed clinical trials are essential. The identification of predictive biomarkers will be crucial for patient stratification, ensuring that the most suitable patients receive immunotherapy-based interventions. Currently, a major limitation is the lack of validated biomarkers for predicting responses to immunotherapy, necessitating further exploration of molecular and immune profiling strategies. Additionally, the development of next-generation cellular therapies, including multi-targeted CAR-T cells, armored CAR-NK cells, and gene-edited immune cells, holds great promise for overcoming resistance and improving treatment durability.
Looking ahead, a multidisciplinary approach that combines immunotherapy with conventional treatments such as chemotherapy, radiotherapy, and targeted therapy may pave the way for more effective, personalized treatment regimens. The integration of AI-driven precision medicine and liquid biopsy-based monitoring may refine patient selection and treatment adaptation, increasing therapeutic success rates. As research continues to advance, overcoming key barriers—such as immune evasion, inadequate T cell infiltration, and therapy resistance—will be essential for improving clinical outcomes. Further investigation into emerging strategies, including the use of oncolytic viruses to enhance tumor immunogenicity, microbiome-based interventions to modulate systemic immunity, and neoantigen-based vaccines for personalized immunotherapy, may provide novel avenues to enhance treatment efficacy. Cellular and immune-based therapies are expected to play an increasingly central role in improving osteosarcoma outcomes, offering new hope for patients with this aggressive malignancy.
GC: Resources, Writing – review & editing. ZH: Resources, Writing – original draft. DW: Conceptualization, Writing – review & editing.
The author(s) declare that no financial support was received for the research and/or publication of this article.
All the pictures were generated via BioRenders, https://BioRender.com
The authors declare that the research was conducted in the absence of any commercial or financial relationships that could be construed as a potential conflict of interest.
The authors declare that no Gen AI was used in the creation of this manuscript.
All claims expressed in this article are solely those of the authors and do not necessarily represent those of their affiliated organizations, or those of the publisher, the editors and the reviewers. Any product that may be evaluated in this article, or claim that may be made by its manufacturer, is not guaranteed or endorsed by the publisher.
ADC, antibody-drug conjugate; AI, artificial intelligence; APCs, antigen-presenting cells; BsAbs, bispecific antibodies; CAR-T, chimeric antigen receptor –T; CDX, cell line-derived xenografts; CRS, cytokine release syndrome; CTLs, cytotoxic T lymphocytes; CXCL, C-X-C motif chemokine ligand; CXCR4, C-X-C motif chemokine receptor 4; DC, dendritic cell; GVHD, graft-versus-host disease; ICB, immune checkpoint blockade; ICD, immunogenic cell death; IDO, indoleamine 2,3-dioxygenase; IFN-γ, interferon-gamma; IL-10, interleukin-10; IL-15, interleukin-15; LAG-3, lymphocyte activation gene-3; MAG, magrolimab; MCAM, melanoma cell adhesion molecule; PDX, patient-derived; PD-1, programmed cell death protein 1; PD-L1, PD-1 ligand; TAAs, tumor-associated antigens; TAMs, tumor-associated macrophages; TGF-β, transforming growth factor-beta; TGFβ1,transforming growth factor β1; TIGIT, T cell immunoreceptor with Ig and ITIM domains; TIM-3, T cell immunoglobulin and mucin domain-containing protein 3; TKIs, tyrosine kinase inhibitors; TLR, toll-like receptor; TMB, tumor mutational burden; TME, tumor microenvironment; TNF-α, tumor necrosis factor-alpha; Tregs, regulatory T cells; VISTA, V-domain Ig suppressor of T cell activation;ADC: antibody-drug conjugate.
1. Meltzer PS, Helman LJ. New horizons in the treatment of osteosarcoma. N Engl J Med. (2021) 385:2066–76. doi: 10.1056/NEJMra2103423
2. Gill J, Gorlick R. Advancing therapy for osteosarcoma. Nat Rev Clin Oncol. (2021) 18:609–24. doi: 10.1038/s41571-021-00519-8
3. Zhang J, Tan Y, Shou Y, Fang L, Li X, Lai J. The relationship between adjuvant radiotherapy and survival of osteosarcoma: a case-control study. Biotechnol Genet Eng Rev. (2023) 39:796–809. doi: 10.1080/02648725.2022.2163805
4. Liu Y, Wang Y, Yang Y, Weng L, Wu Q, Zhang J, et al. Emerging phagocytosis checkpoints in cancer immunotherapy. Signal Transduct Target Ther. (2023) 8:104. doi: 10.1038/s41392-023-01365-z
5. Yu S, Yao X. Advances on immunotherapy for osteosarcoma. Mol Cancer. (2024) 23:192. doi: 10.1186/s12943-024-02105-9
6. Wu C, Gong S, Duan Y, Deng C, Kallendrusch S, Berninghausen L, et al. A tumor microenvironment-based prognostic index for osteosarcoma. J BioMed Sci. (2023) 30:23. doi: 10.1186/s12929-023-00917-3
7. Chen C, Xie L, Ren T, Huang Y, Xu J, Guo W. Immunotherapy for osteosarcoma: Fundamental mechanism, rationale, and recent breakthroughs. Cancer Lett. (2021) 500:1–10. doi: 10.1016/j.canlet.2020.12.024
8. Ge YX, Zhang TW, Zhou L, Ding W, Liang HF, Hu ZC, et al. Enhancement of anti-PD-1/PD-L1 immunotherapy for osteosarcoma using an intelligent autophagy-controlling metal organic framework. Biomaterials. (2022) 282:121407. doi: 10.1016/j.biomaterials.2022.121407
9. Kaczanowska S, Murty T, Alimadadi A, Contreras CF, Duault C, Subrahmanyam PB, et al. Immune determinants of CAR-T cell expansion in solid tumor patients receiving GD2 CAR-T cell therapy. Cancer Cell. (2024) 42:35–51.e38. doi: 10.1016/j.ccell.2023.11.011
10. Hidalgo L, Somovilla-Crespo B, Garcia-Rodriguez P, Morales-Molina A, Rodriguez-Milla MA, Garcia-Castro J. Switchable CAR T cell strategy against osteosarcoma. Cancer Immunol Immunother. (2023) 72:2623–33. doi: 10.1007/s00262-023-03437-z
11. Cillo AR, Mukherjee E, Bailey NG, Onkar S, Daley J, Salgado C, et al. Ewing sarcoma and osteosarcoma have distinct immune signatures and intercellular communication networks. Clin Cancer Res. (2022) 28:4968–82. doi: 10.1158/1078-0432.CCR-22-1471
12. Liu Y, Feng W, Dai Y, Bao M, Yuan Z, He M, et al. Single-cell transcriptomics reveals the complexity of the tumor microenvironment of treatment-naive osteosarcoma. Front Oncol. (2021) 11:709210. doi: 10.3389/fonc.2021.709210
13. Cersosimo F, Lonardi S, Bernardini G, Telfer B, Mandelli GE, Santucci A, et al. Tumor-associated macrophages in osteosarcoma: from mechanisms to therapy. Int J Mol Sci. (2020) 21(15):5207. doi: 10.3390/ijms21155207
14. Zhao Y, Zhang B, Zhang Q, Ma X, Feng H. Tumor-associated macrophages in osteosarcoma. J Zhejiang Univ Sci B. (2021) 22:885–92. doi: 10.1631/jzus.B2100029
15. Huang H, Wang X, Zhang S, Bai X, Griffin N, Shan Y, et al. In vitro and in vivo killing effects of methionine enkephalin on osteosarcoma. Int Immunopharmacol. (2023) 125:111226. doi: 10.1016/j.intimp.2023.111226
16. Li Y, Li M, Wei R, Wu J. Identification and functional analysis of EPOR(+) tumor-associated macrophages in human osteosarcoma lung metastasis. J Immunol Res. (2020) 2020:9374240. doi: 10.1155/2020/9374240
17. Fang W, Zhou T, Shi H, Yao M, Zhang D, Qian H, et al. Progranulin induces immune escape in breast cancer via up-regulating PD-L1 expression on tumor-associated macrophages (TAMs) and promoting CD8(+) T cell exclusion. J Exp Clin Cancer Res. (2021) 40:4. doi: 10.1186/s13046-020-01786-6
18. Huang Q, Liang X, Ren T, Huang Y, Zhang H, Yu Y, et al. The role of tumor-associated macrophages in osteosarcoma progression - therapeutic implications. Cell Oncol (Dordr). (2021) 44:525–39. doi: 10.1007/s13402-021-00598-w
19. Fan Q, Wang Y, Cheng J, Pan B, Zang X, Liu R, et al. Single-cell RNA-seq reveals T cell exhaustion and immune response landscape in osteosarcoma. Front Immunol. (2024) 15:1362970. doi: 10.3389/fimmu.2024.1362970
20. Cheng D, Zhang Z, Liu D, Mi Z, Tao W, Fu J, et al. Unraveling T cell exhaustion in the immune microenvironment of osteosarcoma via single-cell RNA transcriptome. Cancer Immunol Immunother. (2024) 73:35. doi: 10.1007/s00262-023-03585-2
21. Chen Y, Yan W, Wang H, Ou Z, Chen H, Huang Z, et al. The prognostic model established by the differential expression genes based on CD8(+) T cells to evaluate the prognosis and the response to immunotherapy in osteosarcoma. Mediators Inflammation. (2023) 2023:6563609. doi: 10.1155/2023/6563609
22. Lu J, Kang X, Wang Z, Zhao G, Jiang B. The activity level of follicular helper T cells in the peripheral blood of osteosarcoma patients is associated with poor prognosis. Bioengineered. (2022) 13:3751–9. doi: 10.1080/21655979.2022.2031387
23. Netskar H, Pfefferle A, Goodridge JP, Sohlberg E, Dufva O, Teichmann SA, et al. Pan-cancer profiling of tumor-infiltrating natural killer cells through transcriptional reference mapping. Nat Immunol. (2024) 25:1445–59. doi: 10.1038/s41590-024-01884-z
24. Razmara AM, Judge SJ, Gingrich AA, Cruz SM, Culp WTN, Kent MS, et al. Natural killer and T cell infiltration in canine osteosarcoma: clinical implications and translational relevance. Front Vet Sci. (2021) 8:771737. doi: 10.3389/fvets.2021.771737
25. Omer N, Nicholls W, Ruegg B, Souza-Fonseca-Guimaraes F, Rossi GR. Enhancing natural killer cell targeting of pediatric sarcoma. Front Immunol. (2021) 12:791206. doi: 10.3389/fimmu.2021.791206
26. Bareke H, Ibanez-Navarro A, Guerra-Garcia P, Gonzalez Perez C, Rubio-Aparicio P, Plaza Lopez de Sabando D, et al. Prospects and advances in adoptive natural killer cell therapy for unmet therapeutic needs in pediatric bone sarcomas. Int J Mol Sci. (2023) 24(9):8324. doi: 10.3390/ijms24098324
27. Cheng S, Wang H, Kang X, Zhang H. Immunotherapy innovations in the fight against osteosarcoma: emerging strategies and promising progress. Pharmaceutics. (2024) 16(2):251. doi: 10.3390/pharmaceutics16020251
28. Cheng D, Zhang Z, Mi Z, Tao W, Liu D, Fu J, et al. Deciphering the heterogeneity and immunosuppressive function of regulatory T cells in osteosarcoma using single-cell RNA transcriptome. Comput Biol Med. (2023) 165:107417. doi: 10.1016/j.compbiomed.2023.107417
29. Regan DP, Chow L, Das S, Haines L, Palmer E, Kurihara JN, et al. Losartan blocks osteosarcoma-elicited monocyte recruitment, and combined with the kinase inhibitor toceranib, exerts significant clinical benefit in canine metastatic osteosarcoma. Clin Cancer Res. (2022) 28:662–76. doi: 10.1158/1078-0432.CCR-21-2105
30. Petrosiute A, Musvicaite J, Petroska D, Scerbaviciene A, Arnold S, Matuliene J, et al. CCL2-CCR2 axis inhibition in osteosarcoma cell model: the impact of oxygen level on cell phenotype. J Cell Physiol. (2025) 240:e31489. doi: 10.1002/jcp.31489
31. Hu J, Lazar AJ, Ingram D, Wang WL, Zhang W, Jia Z, et al. Cell membrane-anchored and tumor-targeted IL-12 T-cell therapy destroys cancer-associated fibroblasts and disrupts extracellular matrix in heterogenous osteosarcoma xenograft models. J Immunother Cancer. (2024) 12(1): e006991. doi: 10.1136/jitc-2023-006991
32. Zhu Y, Tang L, Zhao S, Sun B, Cheng L, Tang Y, et al. CXCR4-mediated osteosarcoma growth and pulmonary metastasis is suppressed by MicroRNA-613. Cancer Sci. (2018) 109:2412–22. doi: 10.1111/cas.13653
33. Xu Y, Deng C, Chen H, Song Y, Xu H, Song G, et al. Osteosarcoma cells secrete CXCL14 that activates integrin alpha11beta1 on fibroblasts to form a lung metastatic niche. Cancer Res. (2024) 84:994–1012. doi: 10.1158/0008-5472.CAN-23-1307
34. Anwar MA, El-Baba C, Elnaggar MH, Elkholy YO, Mottawea M, Johar D, et al. Novel therapeutic strategies for spinal osteosarcomas. Semin Cancer Biol. (2020) 64:83–92. doi: 10.1016/j.semcancer.2019.05.018
35. Tawbi HA, Burgess M, Bolejack V, Van Tine BA, Schuetze SM, Hu J, et al. Pembrolizumab in advanced soft-tissue sarcoma and bone sarcoma (SARC028): a multicentre, two-cohort, single-arm, open-label, phase 2 trial. Lancet Oncol. (2017) 18:1493–501. doi: 10.1016/S1470-2045(17)30624-1
36. Zheng B, Ren T, Huang Y, Sun K, Wang S, Bao X, et al. PD-1 axis expression in musculoskeletal tumors and antitumor effect of nivolumab in osteosarcoma model of humanized mouse. J Hematol Oncol. (2018) 11:16. doi: 10.1186/s13045-018-0560-1
37. Velayutham NK, Thamaraikani T, Wahab S, Khalid M, Ramachawolran G, Abullais SS, et al. Stylopine: A potential natural metabolite to block vascular endothelial growth factor receptor 2 (VEGFR2) in osteosarcoma therapy. Front Pharmacol. (2023) 14:1150270. doi: 10.3389/fphar.2023.1150270
38. Sayles LC, Breese MR, Koehne AL, Leung SG, Lee AG, Liu HY, et al. Genome-informed targeted therapy for osteosarcoma. Cancer Discovery. (2019) 9:46–63. doi: 10.1158/2159-8290.CD-17-1152
39. Yang C, Lai Y, Wang J, Chen Q, Pan Q, Xu C, et al. Spatial heterogeneity of PD-1/PD-L1 defined osteosarcoma microenvironments at single-cell spatial resolution. Lab Invest. (2024) 104:102143. doi: 10.1016/j.labinv.2024.102143
40. Somaiah N, Conley AP, Parra ER, Lin H, Amini B, Solis Soto L, et al. Durvalumab plus tremelimumab in advanced or metastatic soft tissue and bone sarcomas: a single-centre phase 2 trial. Lancet Oncol. (2022) 23:1156–66. doi: 10.1016/S1470-2045(22)00392-8
41. Blank CU, Lucas MW, Scolyer RA, van de Wiel BA, Menzies AM, Lopez-Yurda M, et al. Neoadjuvant nivolumab and ipilimumab in resectable stage III melanoma. N Engl J Med. (2024) 391:1696–708. doi: 10.1056/NEJMoa2402604
42. Wolchok JD, Chiarion-Sileni V, Rutkowski P, Cowey CL, SChadendorf D, Wagstaff J, et al. Final, 10-year outcomes with nivolumab plus ipilimumab in advanced melanoma. N Engl J Med. (2025) 392:11–22. doi: 10.1056/NEJMoa2407417
43. Davis KL, Fox E, Isikwei E, Reid JM, Liu X, Minard CG, et al. A phase I/II trial of nivolumab plus ipilimumab in children and young adults with relapsed/refractory solid tumors: A children’s oncology group study ADVL1412. Clin Cancer Res. (2022) 28:5088–97. doi: 10.1158/1078-0432.CCR-22-2164
44. Andrews LP, Butler SC, Cui J, Cillo AR, Cardello C, Liu C, et al. LAG-3 and PD-1 synergize on CD8(+) T cells to drive T cell exhaustion and hinder autocrine IFN-gamma-dependent anti-tumor immunity. Cell. (2024) 187:4355–4372.e4322. doi: 10.1016/j.cell.2024.07.016
45. Cillo AR, Cardello C, Shan F, Karapetyan L, Kunning S, Sander C, et al. Blockade of LAG-3 and PD-1 leads to co-expression of cytotoxic and exhaustion gene modules in CD8(+) T cells to promote antitumor immunity. Cell. (2024) 187:4373–4388.e4315. doi: 10.1016/j.cell.2024.06.036
46. Aggarwal V, Workman CJ, Vignali DAA. LAG-3 as the third checkpoint inhibitor. Nat Immunol. (2023) 24:1415–22. doi: 10.1038/s41590-023-01569-z
47. Pu F, Chen F, Zhang Z, Qing X, Lin H, Zhao L, et al. TIM-3 expression and its association with overall survival in primary osteosarcoma. Oncol Lett. (2019) 18:5294–300. doi: 10.3892/ol.2019.10855
48. Huuhtanen J, Kasanen H, Peltola K, Lonnberg T, Glumoff V, Bruck O, et al. Single-cell characterization of anti-LAG-3 and anti-PD-1 combination treatment in patients with melanoma. J Clin Invest. (2023) 133(6):e164809. doi: 10.1172/JCI164809
49. Mao C, Xiong A, Qian J, Wang W, Liu Y, Zhang T, et al. Dual inhibition of LAG-3 and PD-1 with IBI110 and sintilimab in advanced solid tumors: the first-in-human phase Ia/Ib study. J Hematol Oncol. (2024) 17:132. doi: 10.1186/s13045-024-01651-5
50. Ligon JA, Choi W, Cojocaru G, Fu W, Hsiue EH, Oke TF, et al. Pathways of immune exclusion in metastatic osteosarcoma are associated with inferior patient outcomes. J Immunother Cancer. (2021) 9(5):e001772. doi: 10.1136/jitc-2020-001772
51. Zhang Z, Ren C, Xiao R, Ma S, Liu H, Dou Y, et al. Palmitoylation of TIM-3 promotes immune exhaustion and restrains antitumor immunity. Sci Immunol. (2024) 9:eadp7302. doi: 10.1126/sciimmunol.adp7302
52. Dixon KO, Lahore GF, Kuchroo VK. Beyond T cell exhaustion: TIM-3 regulation of myeloid cells. Sci Immunol. (2024) 9:eadf2223. doi: 10.1126/sciimmunol.adf2223
53. Curigliano G, Gelderblom H, Mach N, Doi T, Tai D, Forde PM, et al. Phase I/ib clinical trial of sabatolimab, an anti-TIM-3 antibody, alone and in combination with spartalizumab, an anti-PD-1 antibody, in advanced solid tumors. Clin Cancer Res. (2021) 27:3620–9. doi: 10.1158/1078-0432.CCR-20-4746
54. Chauvin JM, Zarour HM. TIGIT in cancer immunotherapy. J Immunother Cancer. (2020) 8(2):e000957. doi: 10.1136/jitc-2020-000957
55. Chu X, Tian W, Wang Z, Zhang J, Zhou R. Co-inhibition of TIGIT and PD-1/PD-L1 in cancer immunotherapy: mechanisms and clinical trials. Mol Cancer. (2023) 22:93. doi: 10.1186/s12943-023-01800-3
56. Hui X, Farooq MA, Chen Y, Ajmal I, Ren Y, Xue M, et al. A novel strategy of co-expressing CXCR5 and IL-7 enhances CAR-T cell effectiveness in osteosarcoma. Front Immunol. (2024) 15:1462076. doi: 10.3389/fimmu.2024.1462076
57. Judge SJ, Darrow MA, Thorpe SW, Gingrich AA, O’Donnell EF, Bellini AR, et al. Analysis of tumor-infiltrating NK and T cells highlights IL-15 stimulation and TIGIT blockade as a combination immunotherapy strategy for soft tissue sarcomas. J Immunother Cancer. (2020) 8(2):e001355. doi: 10.1136/jitc-2020-001355
58. Propper DJ, Balkwill FR. Harnessing cytokines and chemokines for cancer therapy. Nat Rev Clin Oncol. (2022) 19:237–53. doi: 10.1038/s41571-021-00588-9
59. Anderson ND, Birch J, Accogli T, Criado I, Khabirova E, Parks C, et al. Transcriptional signatures associated with persisting CD19 CAR-T cells in children with leukemia. Nat Med. (2023) 29:1700–9. doi: 10.1038/s41591-023-02415-3
60. Zhu J, Simayi N, Wan R, Huang W. CAR T targets and microenvironmental barriers of osteosarcoma. Cytotherapy. (2022) 24:567–76. doi: 10.1016/j.jcyt.2021.12.010
61. Nazha B, Inal C, Owonikoko TK. Disialoganglioside GD2 expression in solid tumors and role as a target for cancer therapy. Front Oncol. (2020) 10:1000. doi: 10.3389/fonc.2020.01000
62. Fu Y, Yu J, Liatsou I, Du Y, Josefsson A, Nedrow JR, et al. Anti-GD2 antibody for radiopharmaceutical imaging of osteosarcoma. Eur J Nucl Med Mol Imaging. (2022) 49:4382–93. doi: 10.1007/s00259-022-05888-5
63. Nakano K. The future of HER2-targeted treatment for osteosarcoma: lessons from the negative trastuzumab deruxtecan results. Int J Mol Sci. (2023) 24(23):16823. doi: 10.3390/ijms242316823
64. Xiao H, Jensen PE, Chen X. Elimination of osteosarcoma by necroptosis with graphene oxide-associated anti-HER2 antibodies. Int J Mol Sci. (2019) 20. doi: 10.3390/ijms20184360
65. Hegde M, Navai S, DeRenzo C, Joseph SK, Sanber K, Wu M, et al. Autologous HER2-specific CAR T cells after lymphodepletion for advanced sarcoma: a phase 1 trial. Nat Cancer. (2024) 5:880–94. doi: 10.1038/s43018-024-00749-6
66. Park JA, Cheung NV. GD2 or HER2 targeting T cell engaging bispecific antibodies to treat osteosarcoma. J Hematol Oncol. (2020) 13:172. doi: 10.1186/s13045-020-01012-y
67. Majzner RG, Theruvath JL, Nellan A, Heitzeneder S, Cui Y, Mount CW, et al. CAR T cells targeting B7-H3, a pan-cancer antigen, demonstrate potent preclinical activity against pediatric solid tumors and brain tumors. Clin Cancer Res. (2019) 25:2560–74. doi: 10.1158/1078-0432.CCR-18-0432
68. Kendsersky NM, Lindsay J, Kolb EA, Smith MA, Teicher BA, Erickson SW, et al. The B7-H3-targeting antibody-drug conjugate m276-SL-PBD is potently effective against pediatric cancer preclinical solid tumor models. Clin Cancer Res. (2021) 27:2938–46. doi: 10.1158/1078-0432.CCR-20-4221
69. Cao JW, Lake J, Impastato R, Chow L, Perez L, Chubb L, et al. Targeting osteosarcoma with canine B7-H3 CAR T cells and impact of CXCR2 Co-expression on functional activity. Cancer Immunol Immunother. (2024) 73:77. doi: 10.1007/s00262-024-03642-4
70. Myers JA, Miller JS. Exploring the NK cell platform for cancer immunotherapy. Nat Rev Clin Oncol. (2021) 18:85–100. doi: 10.1038/s41571-020-0426-7
71. Pan K, Farrukh H, Chittepu V, Xu H, Pan CX, Zhu Z. CAR race to cancer immunotherapy: from CAR T, CAR NK to CAR macrophage therapy. J Exp Clin Cancer Res. (2022) 41:119. doi: 10.1186/s13046-022-02327-z
72. Rav E, Maegawa S, Gopalakrishnan V, Gordon N. Overview of CD70 as a potential therapeutic target for osteosarcoma. J Immunol. (2023) 211:1067–72. doi: 10.4049/jimmunol.2200591
73. Rav ES, Anjier A, Acharya S, Basar R, Wang Y, Daw N, et al. Targeting CD70 using CAR NK cells to enhance NK cells cytolytic effect against osteosarcoma. J Immunol. (2023) 210:142.102. doi: 10.4049/jimmunol.210.Supp.142.02
74. Gardenswartz A, Luo W, Rosenblum JM, Ayello J, Cairo MS. Abstract 6594: Targeting Ewing sarcoma and osteosarcoma with anti-MCAM chimeric antigen receptor modified NK cells. Cancer Res. (2020) 80:6594–4. doi: 10.1158/1538-7445.Am2020-6594
75. Luo W, Hoang H, Zhu H, Miller K, Mo X, Eguchi S, et al. Circumventing resistance within the Ewing sarcoma microenvironment by combinatorial innate immunotherapy. J Immunother Cancer. (2024) 12(9):e009726. doi: 10.1136/jitc-2024-009726
76. Laureano RS, Sprooten J, Vanmeerbeerk I, Borras DM, Govaerts J, Naulaerts S, et al. Trial watch: Dendritic cell (DC)-based immunotherapy for cancer. Oncoimmunology. (2022) 11:2096363. doi: 10.1080/2162402X.2022.2096363
77. Yang Y, Zhou Y, Wang J, Zhou Y, Watowich SS, Kleinerman ES. CD103(+) cDC1 dendritic cell vaccine therapy for osteosarcoma lung metastases. Cancers (Basel). (2024) 16(19):3251. doi: 10.3390/cancers16193251
78. Sun C, Ma X, Zhou C, Zhang Z, Guo J. Irreversible electroporation combined with dendritic cell-based vaccines for the treatment of osteosarcoma. Anticancer Res. (2023) 43:3389–400. doi: 10.21873/anticanres.16514
79. Zhai J, Gu X, Liu Y, Hu Y, Jiang Y, Zhang Z. Chemotherapeutic and targeted drugs-induced immunogenic cell death in cancer models and antitumor therapy: An update review. Front Pharmacol. (2023) 14:1152934. doi: 10.3389/fphar.2023.1152934
80. Liu X, He S, Wu H, Xie H, Zhang T, Deng Z. Blocking the PD-1/PD-L1 axis enhanced cisplatin chemotherapy in osteosarcoma in vitro and in vivo. Environ Health Prev Med. (2019) 24:79. doi: 10.1186/s12199-019-0835-3
81. Fu Z, Li K, Wang H, Li Y, Zhang J, Zhou J, et al. Spectral computed tomography-guided radiotherapy of osteosarcoma utilizing BiOI nanosheets. Acta Biomater. (2023) 166:615–26. doi: 10.1016/j.actbio.2023.05.026
82. Assi A, Farhat M, Hachem MCR, Zalaquett Z, Aoun M, Daher M, et al. Tyrosine kinase inhibitors in osteosarcoma: Adapting treatment strategiesa. J Bone Oncol. (2023) 43:100511. doi: 10.1016/j.jbo.2023.100511
83. Just MA, Van Mater D, Wagner LM. Receptor tyrosine kinase inhibitors for the treatment of osteosarcoma and Ewing sarcoma. Pediatr Blood Cancer. (2021) 68:e29084. doi: 10.1002/pbc.29084
84. Fleuren EDG, Vlenterie M, van der Graaf WTA. Recent advances on anti-angiogenic multi-receptor tyrosine kinase inhibitors in osteosarcoma and Ewing sarcoma. Front Oncol. (2023) 13:1013359. doi: 10.3389/fonc.2023.1013359
85. Shi B, Chang J, Sun X, Ma X, Zhao P, Zhou C, et al. A meta-analysis: the clinical value of PD-1 inhibitor or protein tyrosine kinase inhibitors in the treatment of advanced osteosarcoma. Front Oncol. (2023) 13:1148735. doi: 10.3389/fonc.2023.1148735
86. Yang D, Chen Y, He ZNT, Wang Y, Ke C, Luo Y, et al. Indoleamine 2,3-dioxygenase 1 promotes osteosarcoma progression by regulating tumor-derived exosomal miRNA hsa-miR-23a-3p. Front Pharmacol. (2023) 14:1194094. doi: 10.3389/fphar.2023.1194094
87. Zhou Y, Yang D, Yang Q, Lv X, Huang W, Zhou Z, et al. Single-cell RNA landscape of intratumoral heterogeneity and immunosuppressive microenvironment in advanced osteosarcoma. Nat Commun. (2020) 11:6322. doi: 10.1038/s41467-020-20059-6
Keywords: osteosarcoma, immunotherapy, checkpoint blockade, immune cell therapy, tumor microenvironment
Citation: Han Z, Chen G and Wang D (2025) Emerging immunotherapies in osteosarcoma: from checkpoint blockade to cellular therapies. Front. Immunol. 16:1579822. doi: 10.3389/fimmu.2025.1579822
Received: 19 February 2025; Accepted: 04 March 2025;
Published: 18 March 2025.
Edited by:
Xiangpeng Dai, Jilin University, ChinaReviewed by:
Rui Dong, Stanford University, United StatesCopyright © 2025 Han, Chen and Wang. This is an open-access article distributed under the terms of the Creative Commons Attribution License (CC BY). The use, distribution or reproduction in other forums is permitted, provided the original author(s) and the copyright owner(s) are credited and that the original publication in this journal is cited, in accordance with accepted academic practice. No use, distribution or reproduction is permitted which does not comply with these terms.
*Correspondence: Dongchen Wang, d2FuZ2RvbmdjaGVuMDEwM0AxNjMuY29t
Disclaimer: All claims expressed in this article are solely those of the authors and do not necessarily represent those of their affiliated organizations, or those of the publisher, the editors and the reviewers. Any product that may be evaluated in this article or claim that may be made by its manufacturer is not guaranteed or endorsed by the publisher.
Research integrity at Frontiers
Learn more about the work of our research integrity team to safeguard the quality of each article we publish.