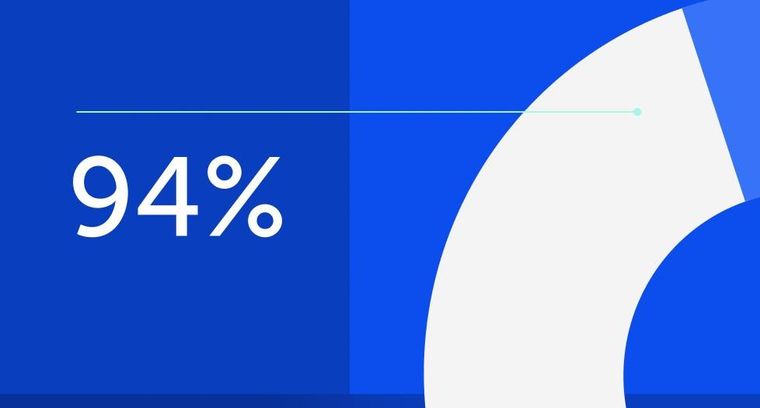
94% of researchers rate our articles as excellent or good
Learn more about the work of our research integrity team to safeguard the quality of each article we publish.
Find out more
MINI REVIEW article
Front. Immunol., 24 March 2025
Sec. Vaccines and Molecular Therapeutics
Volume 16 - 2025 | https://doi.org/10.3389/fimmu.2025.1575133
This article is part of the Research TopicVaccines and Molecular Therapeutics for TuberculosisView all 6 articles
The hallmark tissue lesions of tuberculosis (TB) are granulomas. These multicellular structures exhibit varying degrees of cellular complexity, are dynamic, and show considerable diversity within and between hosts. Categorization based on gross pathologic features, particularly caseation and necrosis, was historically coined prior to the identification of mycobacteria as the causative agent of TB. More recently, granuloma zonation based on immune cell composition, metabolite abundance, and physical characteristics has gained attention. With the advent of single-cell analyses, distinct microenvironments and cellular ecosystems within TB granulomas have been identified. We summarize the architecture of TB granulomas and highlight their cellular heterogeneity, including cell niches as well as physical factors such as oxygen gradients that modulate lesion fate. We discuss opportunities for therapy, highlighting new models and the power of in silico modeling to unravel granuloma features and trajectories. Understanding the relevance of the granuloma microenvironment to disease pathophysiology will facilitate the development of more effective interventions, such as host-directed therapies for TB.
Tuberculosis (TB) is a major global health threat (1). TB is caused by Mycobacterium tuberculosis (Mtb), which usually infects the lungs. The pathological hallmark of TB and the center of bacteria-host interaction are the tuberculous granulomas. These are organized cellular structures generated by the host’s immune response in order to contain Mtb (2). However, the bacteria may override the immune control in these lesions (3). Granulomas are highly heterogenous, comprising microenvironments with diverse cellular compositions and various biochemical characteristics including gas, metabolite and nutrient gradients (4, 5). Such heterogeneity profoundly influences host immunity and Mtb biology, affecting disease progression, latency, and potential reactivation.
Mtb actively drives granuloma biogenesis to its own benefit (6). Accordingly, granuloma characteristics are relevant to both TB chemotherapy and host-directed therapies (HDTs) (7, 8). Current treatment regimens for drug-sensitive TB involve a prolonged course of multiple antibiotics, which poses challenges in terms of adherence and efficacy. The emergence of drug-resistant TB further complicates the treatment. Given the limitations of conventional chemotherapy and the threat of increasing drug resistance, HDTs have emerged as a promising alternative (1). HDTs aim to enhance the host immune response to effectively eliminate Mtb or limit deleterious inflammation, potentially shortening treatment duration, preventing the development of resistance, and improving the efficacy of existing anti-TB drugs. This review focuses on microenvironments within pulmonary TB granulomas, specifically zonation, molecular gradients, and cellular ecosystems revealed by spatial biology. We discuss the role of microenvironments in disease pathogenesis and highlight their relevance for HDT against TB.
The granuloma as a histopathologic hallmark of TB was described already in the 19th century (9, 10). Prior to the introduction of TB chemotherapy, knowledge of the granuloma was obtained from autopsies. Today, animal models recapitulate the complexity of TB granulomas to varying degrees (8). Mouse models contributed greatly to the understanding of immune responses in TB, however usually lack organized granulomas, cavitation and fibrosis (11). Natural TB hosts such as cattle, pigs and non-human primates (NHPs) present well-organized and histopathological diverse lung granulomas which resemble lesions in humans (12, 13). Guinea pigs (14) and rabbits (15) develop necrotic lesions and cavities, yet the immunological toolbox is restricted for these species. Thus, spatial insights about TB granulomas emerge from few mammalian TB models. Granulomas contain aggregations of immune cells, initially macrophages, and are formed by chronic mycobacterial stimulation (Figure 1). They range in size from a few mm to 20 mm (16, 17) and are round, ellipsoid, elongated or even branched (18, 19). Histopathology, immunohistochemistry and ultrastructural microscopy revealed the cellular composition of developing granulomas. As the disease progresses, monocyte-derived macrophages, dendritic cells and granulocytes, including neutrophils and eosinophils, are recruited proximally to the Mtb-infected macrophages. The cellular influx promotes granuloma biogenesis and macrophages within these structures rapidly progress toward epithelioid differentiation as shown in guinea pigs (14), mice (20), cattle (12), rabbits (15) and NHPs (13). These nascent granulomas increase in size and complexity. T- and B-cells surround the macrophage core of the mature TB granuloma. Non-immune cells including fibroblasts also contribute to granuloma architecture and maintenance (21, 22). The timing of immune cell afflux defines the architecture of the lung granuloma, including cellular neighborhoods and cell-cell communication. Lymphocytes enriched in the outer layer do not interact with the inner myeloid cell compartment due to spatial distance (23). This limits the mycobactericidal arsenal of the phagocytes (24, 25). Even within an individual, granulomas can have distinct immune signature (26, 27) and fates, ranging from sterilizing to caseating entities that facilitate Mtb dissemination (28, 29). Spatial mapping of individual immune cells revealed a superstructural histopathologic feature of the human TB granulomas (4) with distinct non-necrotizing cellular aggregates proximal to necrotizing granulomas. The fate of TB granulomas is determined by their cellular composition. An excess of neutrophils accompanies caseating granulomas and immunopathology (30, 31), whereas an early CD4 T-cell accumulation and local macrophage activation prevent intra-granulomatous necrosis (31).
Figure 1. Tuberculous granuloma microenvironment and host-directed therapies. Granulomas are hallmark lesions of tuberculosis (TB) and exhibit distinct cellular zonation, gradients of gases such as oxygen or carbon monoxide (A, B), nutrients such as amino acids and glucose, and various metabolites (C). (D-F) Distinct cellular ecosystems have been identified in TB granulomas. These are defined by polarized macrophages and T-cell immune responses, distinct features of immune cells including transformed macrophages, and cell clusters with distinct spatial positioning. (G) Approaches for host-directed therapies (HDTs) targeting granuloma biology include modulation of inflammation, tissue matrix, and neovascularization. Ala, alanine; Arg, arginine; CBS, cystathionine β-synthase; CO, carbon monoxide; H2S, hydrogen sulfide; Glu, glucose; HIF, hypoxia-inducible factor; HO-1, hemeoxygenase-1; IDO, indoleamine dioxygenase; IFN, interferon; IL, interleukin; iNOS, inducible nitric oxide synthase; Mφ, macrophage; MMP, matrix metalloprotease; NO, nitric oxide; PDE, phosphodiesterase; PD-L1, programmed cell death ligand 1; PGE, prostaglandin; T, T-cell; Th, T-helper cell; TNF, tumor necrosis factor; Trp, tryptophan; VEGF, vascular endothelial growth factor. Figure created with Biorender.
Macrophages acquire distinct features that define the zonation of TB granulomas. Foamy and multinucleated giant cells (MNGCs) are distributed in different regions while epithelioid macrophages surround the core of Mtb-infected cells. Epithelization is driven by chronic mTORC1 stimulation (32) in combination with the IL-4/STAT6 signaling pathway which triggers E-cadherin expression on macrophages (33). In addition to the upregulation of genes regulating tight junctions and desmosomes, mainly E-cadherin seems critical for granuloma organization (34). This tight layer seals Mtb within the necrotic core and also limits drug penetration. Granuloma zonation has also been linked to macrophage polarization and to specific myeloid cell subpopulations. The M1 and M2 macrophage dichotomy fails to describe the continuum of macrophage activation, yet markers associated with these phenotypes occupy distinct granuloma niches. Anti-inflammatory (M2-like) macrophages enriched in arginase 1 (Arg1) versus inducible nitric oxide (iNOS) (35) are proximal to caseating foci within primate granulomas. Such patterns differ in other natural hosts. Porcine TB granulomas accumulate Arg1+ cells regardless of the lesion stage whereas iNOS-expressing M1-like cells populate bovine early-stage granulomas (36). Subsets of M2-like macrophages identified as CD16+CD163+MerTK+pSTAT3+ cells are associated with lesion severity in humans (37). iNOS/eNOS, Arg1 expression and additional factors, e.g. IL-10 signaling, drive regional macrophage polarization within TB granulomas. Subsets of neutrophils releasing neutrophil extracellular traps in a type I interferon (IFN-I)-dependent process are associated with central granuloma caseation (38). The early influx of IFN-I-producing plasmacytoid dendritic cells and interstitial macrophages may influence neutrophil function, and subsequent lesion zonation and outcome (39, 40). Fatty acid oxidation (FAO)-dependent neutrophils are recruited to granulomas (41) and cells with increased mitochondrial metabolism, similar to FAO-relying alveolar macrophages (42), support Mtb replication and induce immunopathology (43). Adaptive immunity in TB is mainly defined by B and T cells in the periphery of the TB granuloma. CD8+ T cells are essential in early control of TB in macaques (44). On the other hand, CD4+ T cells have a significant role in protection against reinfection (45). Granuloma-associated lymphoid tissue containing Mtb-specific B cells proximal to T follicular helper-like cells appear essential for disease control (46). Further diversity of CD3+ and CD8+ T-cell and B cell distribution within individual human TB granulomas was unveiled by quantitative multiplexed immunofluorescence which emphasizes presence of distinct immune environments (47).
High-resolution single cell analyses suggest further a zonation of granulomas into microenvironments and cellular ecosystems (Figure 1). Microenvironments with highly localized immunoregulatory programs, e.g. indoleamine 2,3-dioxygenase 1 (IDO1)- and PD-L1-expressing myeloid cells or proliferating regulatory T-cells, have been described (21). Variable multicellular ecosystems endow granulomas with Mtb restrictive functions (13). Bacilli persist in granulomas showing a type 2 immunity and wound healing programs. Mtb control requires niches enriched for type 1/type 17, stem-like, and cytotoxic T-cells. Spatial transcriptomics revealed regions containing SPP1+ macrophages distributed from the periphery to the granuloma center (48, 49). These engage with fibroblasts in bilateral cross-talks possibly contributing to lesion outcome. The spatial organization of TB granulomas follows pro- and anti-inflammatory mediators (50). Granuloma centers are rich in reactive oxygen species and pro-inflammatory eicosanoids whereas an anti-inflammatory signature surrounds the caseum. This physical segregation of the mediators may stem from various cellular niches. Thus, different cellular phenotypes and secretory products localize in specific areas, control bacilli growth and cell death events within TB granulomas.
One of the key features of TB granulomas is a gradual decrease in oxygen levels towards the center (51, 52). Certain host species, including guinea pigs, rabbits, NHPs, and selected mouse strains, i.e. C3HeB/FeJ, exhibit hypoxic and well-defined granulomas (53, 54). Although organized granulomas were described in very-low dose (20) and ultra-low dose (55) infected C57BL/6 mice the oxygen abundances within remain unknown. Hypoxia promotes a non-replicating, persistent Mtb state that is also induced by nitric oxide (NO) and carbon monoxide (CO) gas gradients (56, 57). The hypoxic granuloma core is often necrotic, regardless of whether the infection is latent or active (58, 59). Disease exacerbation by necrosis is enhanced under hypoxia, leading to extracellular matrix destruction through the upregulation of collagenase and elastases (60).
Hypoxia-mediated inflammation is conserved across species (61). It triggers the stabilization of HIF-1α and the subsequent adaptation of immune cells to oxygen limitation. Depending on their spatial distribution, certain immune cell populations face oxygen deprivation within the TB granuloma. Hypoxia affects the architecture and dynamics of intracellular organelles relevant to defense against Mtb and these changes may be independent of HIF1-α stabilization (62–66). Nevertheless, HIF-1α stabilization reduces the influx of CD4+ and CD8+ T-cells, impairing Mtb control (67). Mtb produces d-serine under hypoxia, which hampers CD8-mediated IFN-γ secretion via dysregulated mTORC1 functions (68). Hypoxia related immune regulation and spatial reorganization within granulomas have also been demonstrated for innate immune cells including macrophages (69, 70), and neutrophils (71, 72). Abundances of macrophage Arg1 and neutrophil proteases, such as cathepsin G, are altered under hypoxia. The macrophage-rich core splits into two metabolic zones: a hypoxic, high-burden zone and an IDOhigh zone that limits lymphocyte infiltration, weakening immunity in the NHP model (73). Thus, both innate and adaptive immune cells are affected by hypoxia in TB. Certain pro-angiogenetic factors such as vascular endothelial growth factor (VEGF), which are regulated by hypoxia, modulate granuloma architecture. While VEGF is highly abundant in the necrotic core and in surrounding macrophages (74) of human and rabbit (75) granulomas, microvessels are mainly present in the granuloma periphery and exhibit spatial and morphological heterogeneity (75). Propensity of macrophages to produce VEGF upon Mtb infection was shown with human cells (76). Inhibition of VEGF results in resolution of hypoxia, reduced inflammation, survival and vascular normalization in rabbits and mice (74, 75).
Changes within TB granulomas are not limited to oxygen levels. Mtb stimulates the expression of heme oxygenase 1 (HO-1) in macrophages which is highly abundant in the lungs of Mtb-infected mice (77). HO-1 produces CO, which remains elusive in the context of TB granulomas. Another gas, hydrogen sulphide (H2S) recently gained attention in TB. H2S is produced by several host enzymes including cystathionine β-synthase (CBS). H2S is a vasodilator that can cause inflammation via NF-κB activation (78). It may also negatively regulate iNOS and NO production (79). Mice deficient in CBS or treated with enzyme inhibitors survive longer with reduced bacterial load and lung inflammation (80). Despite these recent findings, there is still a knowledge gap about roles of these gases in pathophysiology of the TB granuloma.
Hypoxia is linked to glycolysis in the TB granuloma. Increased glycolysis triggers the formation of acidic by-products such as lactate which acidifies the lesion microenvironment in guinea pigs and mice (81–83). Lung tissue from human TB patients may reach acidic (pH ≤5.5) pH (84). The pH in rabbit caseum within granulomas increases from 6.4 to 7.4 upon lesion maturation (85), which is accompanied by changes in cellular composition. Thus, existence of zones with different pH within individual granulomas or within certain granuloma subtypes is plausible. On the other hand, in situ lesion pH in C3HeB/FeJ mice does not reach a pH below 7 (86).
Regarding the tricarboxylic acid cycle, the intermediate itaconate modulates host immunity in TB. Itaconate triggers anti-inflammatory responses, influences bacterial clearance, and affects tissue pathology in mouse models (87). Given the intracellular localization and limited cell permeability of itaconate, its potential influence on the microenvironment of TB granulomas requires further investigation.
Local hypoxia leads to a shift in the metabolic landscape beyond glycolysis. In humans, caseating granulomas are associated with increased lipid metabolism, especially cholesterol, cholesterol esters and triacylglycerols (88). Arginine and tryptophan are metabolized by the host via Arg1 or NOS, and IDO, respectively. Unavailability of Arg1 in the absence of iNOS leads to increased Mtb burden in hypoxic granulomatous structures (70). Epithelioid macrophages express higher levels of eNOS and iNOS than Arg1 with immunosuppressive macrophage phenotypes proximal to the lymphocyte cuff (35). Thus, the spatial availability of arginine may be critical for the pathophysiology of TB granulomas. The enzymes IDO1 and IDO2 perform the first-rate limiting step of L-tryptophan conversion to L-kynurenine and are highly expressed in macrophages within the granuloma. IDOhigh macrophages are present in caseating granulomas in the NHP model (89). They may also interact proximally with T-cells and thus may be involved in the modulation of T-cell functions (90). In guinea pig granulomas, upregulation of many metabolites including amino acids such as alanine, glutamate and aspartate mimics metabolic changes in solid tumors (83). L-alanine promotes NF-κB activation and antimicrobial peptide production. Mtb expresses the alanine dehydrogenase Rv2780 which hydrolyzes the intracellular L-alanine pool and thereby suppresses the NF-κB-mediated expression of defensins in the murine TB model (91), but the relevance of this process to granuloma biology requires further investigation.
Metabolites of the arachidonic acid (AA) pathway are also critical for the pathophysiology of TB granulomas. These (e.g. products of ALOX5, ALOX5AP and LTA4H) are distributed throughout the granuloma with elevated levels in cavitary and caseous human granulomas (50). Within solid lesions, LTA4H is mainly distributed among MNGCs. In caseous and cavitary granulomas as well as alveolar inflammatory infiltrates, it is mainly distributed in macrophages/DCs and MNGCs (50). Caseous and cavitary granulomas show low levels of cyclooxygenases (COX1 and 2), which are responsible for the synthesis of prostaglandins (PGH2 and PGE2 or PGD2) (92). Since Mtb inhibits the eicosanoid pathway and negatively affects innate and adaptive immunity as well as cellular necrosis (93, 94), zonation of these metabolites affects granuloma stability.
In summary, the TB granuloma is a highly dynamic entity shaped by metabolic shifts, cell interactions, and oxygen deprivation. Metabolic adaptations further shape the immune landscape of granulomas, adding another layer of complexity (Figure 1). Because timing and location control the composition of the cellular microenvironment and Mtb permissiveness, a thorough understanding of the spatial immunobiology of the TB granuloma is essential for advancing the understanding of TB.
Standard TB chemotherapy consists of a combination of antibiotics over several months. Compliance is often poor due to the long duration of treatment and drug side effects (95), necessitating additional treatment options. Moreover, TB granulomas ‘wall off’ mycobacteria and also antibiotics. For example, in situ analysis of fluoroquinolones showed a variable distribution in immune cells within granuloma zones (96). Therefore, novel antibiotics should take granuloma biology into account, as lesion penetration is essential for good clinical outcomes. We focus on adjunctive HDTs in the context of pulmonary TB granulomas.
As diverse as granulomas in TB patients are, so are the requirements for HDTs (97). In the context of granuloma biology, several adjunctive HDTs are attractive (Figure 1). Type 4 phosphodiesterase inhibitors (PDE) such as CC-11050 exert their anti-inflammatory effects by limiting cAMP degradation. Increased cAMP levels lead to suppression of pro-inflammatory cytokines, prevention of inflammatory cell proliferation and slowing down of fibrotic processes (98). The downregulation of TNF-α networks by CC-11050 treatment is particularly relevant in TB (99, 100), given the role of this cytokine in the early formation and maintenance of granulomas (101, 102). Combined with antibiotics PDE inhibitors reduced the bacillary load in the lungs of rabbits and mice (99, 100) and improved TB outcomes in patients. CC-11050 completed a phase 2a clinical trial with increased lung recovery and a good preliminary safety profile (NCT02968927) (103). Moreover, CC-11050 and CC-3052 (another PDE inhibitor) modulated the wound healing networks in a rabbit model of TB (99, 104). Factors involved in this process were matrix metalloproteases (MMPs). MMPs include collagenases (e.g. MMP1 and MMP13), gelatinases (e.g. MMP2 and MMP9), stromelysins (e.g. MMP3), elastases (e.g. MMP12), and membrane-anchored proteases (e.g. MMP14) (105). They are involved in connective tissue homeostasis and fibrotic processes associated with TB granulomas. Excessive expression of MMPs in TB leads to lung tissue damage and cavitation. The use of an anti-MMP9 antibody as an adjunct to TB drugs reduced TB relapse rates (106). Hydroxamate-based compounds have a broad-spectrum activity against MMPs by mimicking collagen. Marimastat belongs to this class of drug and inhibited early granuloma formation besides reducing Mtb growth in a lung tissue model (107). Marimasat and Batimastat (a compound in the same class) in combination with anti-TB drugs reduced vascular leakage, stabilized blood vessels and improved drug penetration by targeting MMP2 and 9 (108). Doxycycline, an FDA-approved antibiotic and systemic MMP inhibitor (109) is being evaluated for off-target use in pulmonary (NCT02774993, NCT06477185) and central nervous system TB (NCT06446245). Besides bactericidal and sustained immunomodulatory effects via downregulation of IFN I and II, doxycycline reduces cavitary volume in the lungs of TB patients (109).
Granuloma formation is closely related to angiogenesis (27, 110). Normalization of Mtb-induced angiogenesis results in reduced bacillary growth and dissemination (110). Administration of losartan, an AT1 receptor antagonist, and bevacizumab, a human anti-VEGF monoclonal antibody, showed regularized blood vessels and extracellular matrix in granuloma microenvironment. These agents reduced Mtb burden in a rabbit model of TB alone and in combination with TB drugs (111). Delivery of an anti-VEGF antibody also resulted in reduced hypoxic regions and increased small molecule delivery (75). As discussed above, host enzymes associated with macrophage polarization control Mtb replication and the inflammatory milieu within the TB granuloma. Targeting the AA pathway seems amenable for the development of HDTs (112). Targeting IDO as adjunct to chemotherapy also showed promising results in NHP models (113). HDTs could balance the immune response within the granuloma considering its various microenvironments. Current solutions are limited to specific molecules or pathways, and many regulators of granuloma zonation remain to be targeted.
Multi-omics approaches in spatial biology currently advance the study of diseased tissues at unprecedented resolution. However, there is a growing need for novel ex vivo models to complement animal models that develop human-like TB granulomas. We envision that integrative approaches incorporating patient biopsies, animal samples, and ex vivo models will mutually facilitate a detailed understanding of the spatiotemporal cues of the TB granuloma.
Opportunities to advance knowledge of TB granulomas are provided by new technologies, high performance computing and modeling. Granuloma models based on computational methods (114, 115) and 3D spheroids (116, 117) facilitate studies of cellular responses under specific conditions such as hypoxia. Spheroids mimic in vivo granulomas, including the dormant state of Mtb (118, 119). Further opportunities include the integration of novel features into 3D models and/or in silico simulations. New experimental data link macrophage ontogeny (120), phenotype (enzymes, cell surface markers e.g. CD38) (121) and immunometabolism (FAO or glycolysis) to Mtb killing propensity, but the spatial context is still lacking. Similarly, clarifying the non-redundant roles of CD4+ and CD8+ lymphocyte subsets (44, 45) or positioning of granuloma-associated lymphoid tissue (46) in the spatial context could help define correlates of protection. In this respect, precise localization and interactors of B cell clusters including plasma cells, as detected in NHPs (122), and zonation of Mtb-specific antibodies remain elusive. The TB granuloma has already been mathematically modeled (123). Refinements and AI implementation to integrate multi-omics could help predict granuloma trajectories. New in vivo tracers of inflammation (124) and AI-based imaging algorithms could predict responses to therapies and accelerate HDTs.
The challenges in determining granuloma heterogeneity lie in the diverse lifestyle of Mtb. In addition to its intracellular and extracellular localization, Mtb’s subcellular lifestyle adds a layer of complexity and modulates host responses. Visualization of the subcellular localization of Mtb within infected tissue using correlative light electron microscopy is feasible, but limited to a few specialized laboratories and precludes high-throughput scale (125). Drug penetrance is conditioned by subcellular niches (126), and thus uncovering microenvironments with distinct subcellular Mtb residency is relevant for TB chemotherapy. Mtb genetic diversity influences disease severity and lung pathology (127), and must be considered. Attempts to model granuloma responses to distinct Mtb lineages (128) encourage further studies aiming to elucidate the extent to which Mtb lineage alters zonation or cellular niches.
Singular HDT approaches are currently being tested, but future therapy of TB patients could be guided by clinical data and individualized. Complex diseases require personalized therapies, and perhaps TB endotypes (129) are based on granuloma variability. Embracing heterogeneity at the microenvironmental level paves the way for exciting discoveries about granuloma biology and TB therapy.
GK: Visualization, Writing – original draft, Writing – review & editing. SF: Visualization, Writing – original draft, Writing – review & editing. AD: Conceptualization, Writing – original draft, Writing – review & editing.
The author(s) declare that financial support was received for the research and/or publication of this article. AD acknowledges funding from Deutsche Forschungsgemeinschaft “Research Training Group 2719” and “NFDI4Immuno”; GK acknowledges stipend from “Landesgraduiertenförderung Mecklenburg-Vorpommern”.
The authors declare that the research was conducted in the absence of any commercial or financial relationships that could be construed as a potential conflict of interest.
The author(s) declare that no Generative AI was used in the creation of this manuscript.
All claims expressed in this article are solely those of the authors and do not necessarily represent those of their affiliated organizations, or those of the publisher, the editors and the reviewers. Any product that may be evaluated in this article, or claim that may be made by its manufacturer, is not guaranteed or endorsed by the publisher.
2. Ulrichs T, Kaufmann SH. New insights into the function of granulomas in human tuberculosis. J Pathol. (2006) 208:261–9. doi: 10.1002/path.v208:2
3. Dorhoi A, Reece ST, Kaufmann SH. For better or for worse: the immune response against Mycobacterium tuberculosis balances pathology and protection. Immunol Rev. (2011) 240:235–51. doi: 10.1111/j.1600-065X.2010.00994.x
4. Sawyer AJ, Patrick E, Edwards J, Wilmott JS, Fielder T, Yang Q, et al. Spatial mapping reveals granuloma diversity and histopathological superstructure in human tuberculosis. J Exp Med. (2023) 220. doi: 10.1084/jem.20221392
5. Qualls JE, Murray PJ. Immunometabolism within the tuberculosis granuloma: amino acids, hypoxia, and cellular respiration. Semin Immunopathol. (2016) 38:139–52. doi: 10.1007/s00281-015-0534-0
6. Davis JM, Ramakrishnan L. The role of the granuloma in expansion and dissemination of early tuberculous infection. Cell. (2009) 136:37–49. doi: 10.1016/j.cell.2008.11.014
7. Kaufmann SHE, Dorhoi A, Hotchkiss RS, Bartenschlager R. Host-directed therapies for bacterial and viral infections. Nat Rev Drug Discovery. (2018) 17:35–56. doi: 10.1038/nrd.2017.162
8. Lenaerts A, Barry CE 3rd, Dartois V. Heterogeneity in tuberculosis pathology, microenvironments and therapeutic responses. Immunol Rev. (2015) 264:288–307. doi: 10.1111/imr.2015.264.issue-1
9. Canetti G. The Tubercle Bacillus in the Pulmonary Lesion of Man Vol. 226. Yale University, New Haven, Connecticut, USA: Springer Publishing Company (1955).
10. Elie M. Lectures on the Comparative Pathology of Inflammation, delivered at the Pasteur Instutute in 1891. Paris, France (1893).
11. Corleis B, Bastian M, Hoffmann D, Beer M, Dorhoi A. Animal models for COVID-19 and tuberculosis. Front Immunol. (2023) 14:1223260. doi: 10.3389/fimmu.2023.1223260
12. Cassidy JP, Bryson DG, Pollock JM, Evans RT, Forster F, Neill SD. Early lesion formation in cattle experimentally infected with Mycobacterium bovis. J Comp Pathol. (1998) 119:27–44. doi: 10.1016/S0021-9975(98)80069-8
13. Gideon HP, Hughes TK, Tzouanas CN, Wadsworth MH 2nd, Tu AA, Gierahn TM, et al. Multimodal profiling of lung granulomas in macaques reveals cellular correlates of tuberculosis control. Immunity. (2022) 55:827–46 e10. doi: 10.1016/j.immuni.2022.04.004
14. Larenas-Munoz F, Ruedas-Torres I, Hunter L, Bird A, Agullo-Ros I, Winsbury R, et al. Characterisation and development of histopathological lesions in a Guinea pig model of Mycobacterium tuberculosis infection. Front Vet Sci. (2023) 10:1264200. doi: 10.3389/fvets.2023.1264200
15. Shima K, Dannenberg AM Jr., Ando M, Chandrasekhar S, Seluzicki JA, Fabrikant JI. Macrophage accumulation, division, maturation, and digestive and microbicidal capacities in tuberculous lesions. I. Studies involving their incorporation of tritiated thymidine and their content of lysosomal enzymes and bacilli. Am J Pathol. (1972) 67:159–80.
16. Gil O, Diaz I, Vilaplana C, Tapia G, Diaz J, Fort M, et al. Granuloma encapsulation is a key factor for containing tuberculosis infection in minipigs. PloS One. (2010) 5:e10030. doi: 10.1371/journal.pone.0010030
17. Goo JM, Im JG, Do KH, Yeo JS, Seo JB, Kim HY, et al. Pulmonary tuberculoma evaluated by means of FDG PET: findings in 10 cases. Radiology. (2000) 216:117–21. doi: 10.1148/radiology.216.1.r00jl19117
18. Wells G, Glasgow JN, Nargan K, Lumamba K, Madansein R, Maharaj K, et al. Micro-computed tomography analysis of the human tuberculous lung reveals remarkable heterogeneity in three-dimensional granuloma morphology. Am J Respir Crit Care Med. (2021) 204:583–95. doi: 10.1164/rccm.202101-0032OC
19. Wells G, Glasgow JN, Nargan K, Lumamba K, Madansein R, Maharaj K, et al. A high resolution 3D atlas of the spectrum of tuberculous and COVID19 lung lesions. EMBO Mol Med. (2022) 14:e16283. doi: 10.15252/emmm.202216283
20. Donovan ML, Bielefeldt-Ohmann H, Rollo RF, McPherson SJ, Schultz TE, Mori G, et al. Distinct contributions of the innate immune receptors TLR2 and RP105 to formation and architecture of structured lung granulomas in mice infected with Mycobacterium tuberculosis. Immunology. (2023) 169:13–26. doi: 10.1111/imm.v169.1
21. McCaffrey EF, Donato M, Keren L, Chen Z, Delmastro A, Fitzpatrick MB, et al. The immunoregulatory landscape of human tuberculosis granulomas. Nat Immunol. (2022) 23:318–29. doi: 10.1038/s41590-021-01121-x
22. Qiu X, Zhong P, Yue L, Li C, Yun Z, Si G, et al. Spatial transcriptomic sequencing reveals immune microenvironment features of Mycobacterium tuberculosis granulomas in lung and omentum. Theranostics. (2024) 14:6185–201. doi: 10.7150/thno.99038
23. Kaplan G, Post FA, Moreira AL, Wainwright H, Kreiswirth BN, Tanverdi M, et al. Mycobacterium tuberculosis growth at the cavity surface: a microenvironment with failed immunity. Infect Immun. (2003) 71:7099–108. doi: 10.1128/IAI.71.12.7099-7108.2003
24. Ernst JD, Cornelius A, Desvignes L, Tavs J, Norris BA. Limited antimycobacterial efficacy of epitope peptide administration despite enhanced antigen-specific CD4 T-cell activation. J Infect Dis. (2018) 218:1653–62. doi: 10.1093/infdis/jiy142
25. Kauffman KD, Sallin MA, Sakai S, Kamenyeva O, Kabat J, Weiner D, et al. Defective positioning in granulomas but not lung-homing limits CD4 T-cell interactions with Mycobacterium tuberculosis-infected macrophages in rhesus macaques. Mucosal Immunol. (2018) 11:462–73. doi: 10.1038/mi.2017.60
26. Subbian S, Tsenova L, Kim MJ, Wainwright HC, Visser A, Bandyopadhyay N, et al. Lesion-specific immune response in granulomas of patients with pulmonary tuberculosis: A pilot study. PloS One. (2015) 10:e0132249. doi: 10.1371/journal.pone.0132249
27. Ulrichs T, Kosmiadi GA, Jorg S, Pradl L, Titukhina M, Mishenko V, et al. Differential organization of the local immune response in patients with active cavitary tuberculosis or with nonprogressive tuberculoma. J Infect Dis. (2005) 192:89–97. doi: 10.1086/jid.2005.192.issue-1
28. Barry CE, Boshoff HI, Dartois V, Dick T, Ehrt S, Flynn J, et al. The spectrum of latent tuberculosis: rethinking the biology and intervention strategies. Nat Rev Microbiol. (2009) 7:845–55. doi: 10.1038/nrmicro2236
29. Lin PL, Ford CB, Coleman MT, Myers AJ, Gawande R, Ioerger T, et al. Sterilization of granulomas is common in active and latent tuberculosis despite within-host variability in bacterial killing. Nat Med. (2014) 20:75–9. doi: 10.1038/nm.3412
30. Marzo E, Vilaplana C, Tapia G, Diaz J, Garcia V, Cardona PJ. Damaging role of neutrophilic infiltration in a mouse model of progressive tuberculosis. Tuberculosis (Edinb). (2014) 94:55–64. doi: 10.1016/j.tube.2013.09.004
31. Gern BH, Klas JM, Foster KA, Cohen SB, Plumlee CR, Duffy FJ, et al. CD4-mediated immunity shapes neutrophil-driven tuberculous pathology. bioRxiv. (2024). doi: 10.1101/2024.04.12.589315
32. Linke M, Pham HT, Katholnig K, Schnoller T, Miller A, Demel F, et al. Chronic signaling via the metabolic checkpoint kinase mTORC1 induces macrophage granuloma formation and marks sarcoidosis progression. Nat Immunol. (2017) 18:293–302. doi: 10.1038/ni.3655
33. Cronan MR, Hughes EJ, Brewer WJ, Viswanathan G, Hunt EG, Singh B, et al. A non-canonical type 2 immune response coordinates tuberculous granuloma formation and epithelialization. Cell. (2021) 184:1757–74 e14. doi: 10.1016/j.cell.2021.02.046
34. Cronan Mark R, Beerman Rebecca W, Rosenberg Allison F, Saelens Joseph W, Johnson Matthew G, Oehlers Stefan H, et al. Macrophage epithelial reprogramming underlies mycobacterial granuloma formation and promotes infection. Immunity. (2016) 45:861–76. doi: 10.1016/j.immuni.2016.09.014
35. Mattila JT, Ojo OO, Kepka-Lenhart D, Marino S, Kim JH, Eum SY, et al. Microenvironments in tuberculous granulomas are delineated by distinct populations of macrophage subsets and expression of nitric oxide synthase and arginase isoforms. J Immunol. (2013) 191:773–84. doi: 10.4049/jimmunol.1300113
36. Larenas-Munoz F, Hamed MG, Ruedas-Torres I, Maria-Sanchez-Carvajal J, Dominguez J, Jose Pallares F, et al. Macrophage polarization in lymph node granulomas from cattle and pigs naturally infected with Mycobacterium tuberculosis complex. Vet Pathol. (2024) 61:792–802. doi: 10.1177/03009858241231606
37. Lastrucci C, Benard A, Balboa L, Pingris K, Souriant S, Poincloux R, et al. Tuberculosis is associated with expansion of a motile, permissive and immunomodulatory CD16(+) monocyte population via the IL-10/STAT3 axis. Cell Res. (2015) 25:1333–51. doi: 10.1038/cr.2015.123
38. Chowdhury CS, Kinsella RL, McNehlan ME, Naik SK, Lane DS, Talukdar P, et al. Type I IFN-mediated NET release promotes Mycobacterium tuberculosis replication and is associated with granuloma caseation. Cell Host Microbe. (2024) 32:2092–111 e7. doi: 10.1016/j.chom.2024.11.008
39. Esaulova E, Das S, Singh DK, Choreno-Parra JA, Swain A, Arthur L, et al. The immune landscape in tuberculosis reveals populations linked to disease and latency. Cell Host Microbe. (2021) 29:165–78 e8. doi: 10.1016/j.chom.2020.11.013
40. Kotov DI, Lee OV, Fattinger SA, Langner CA, Guillen JV, Peters JM, et al. Early cellular mechanisms of type I interferon-driven susceptibility to tuberculosis. Cell. (2023) 186:5536–53 e22. doi: 10.1016/j.cell.2023.11.002
41. Sankar P, Ramos RB, Corro J, Mishra LK, Nafiz TN, Bhargavi G, et al. Fatty acid metabolism in neutrophils promotes lung damage and bacterial replication during tuberculosis. PloS Pathog. (2024) 20:e1012188. doi: 10.1371/journal.ppat.1012188
42. Pisu D, Huang L, Grenier JK, Russell DG. Dual RNA-seq of mtb-infected macrophages in vivo reveals ontologically distinct host-pathogen interactions. Cell Rep. (2020) 30:335–50 e4. doi: 10.1016/j.celrep.2019.12.033
43. Andrews JT, Zhang Z, Prasad G, Huey F, Nazarova EV, Wang J, et al. Metabolically active neutrophils represent a permissive niche for Mycobacterium tuberculosis. Mucosal Immunol. (2024) 17:825–42. doi: 10.1016/j.mucimm.2024.05.007
44. Winchell CG, Nyquist SK, Chao MC, Maiello P, Myers AJ, Hopkins F, et al. CD8+ lymphocytes are critical for early control of tuberculosis in macaques. J Exp Med. (2023) 220. doi: 10.1084/jem.20230707
45. Bromley JD, Ganchua SKC, Nyquist SK, Maiello P, Chao M, Borish HJ, et al. CD4(+) T cells re-wire granuloma cellularity and regulatory networks to promote immunomodulation following Mtb reinfection. Immunity. (2024) 57:2380–98 e6. doi: 10.1016/j.immuni.2024.08.002
46. Swanson RV, Gupta A, Foreman TW, Lu L, Choreno-Parra JA, Mbandi SK, et al. Antigen-specific B cells direct T follicular-like helper cells into lymphoid follicles to mediate Mycobacterium tuberculosis control. Nat Immunol. (2023) 24:855–68. doi: 10.1038/s41590-023-01476-3
47. Abengozar-Muela M, Esparza MV, Garcia-Ros D, Vasquez CE, Echeveste JI, Idoate MA, et al. Diverse immune environments in human lung tuberculosis granulomas assessed by quantitative multiplexed immunofluorescence. Mod Pathol. (2020) 33:2507–19. doi: 10.1038/s41379-020-0600-6
48. Katano H, Hebisawa A, Sato Y, Hoshino Y. Identification of SPP1-positive macrophages by single-cell spatial analysis in human lung tissues with mycobacterial infection. bioRxiv. (2025):612778. doi: 10.1101/2024.09.12.612778
49. Yu X, Wang J, Wang P, Liu X, Li C, Ju Y, et al. Single-cell and spatial transcriptomic analyses deciphering the three-layer architecture of human tuberculosis granulomas. bioRxiv. (2024):603490. doi: 10.1101/2024.07.15.603490
50. Marakalala MJ, Raju RM, Sharma K, Zhang YJ, Eugenin EA, Prideaux B, et al. Inflammatory signaling in human tuberculosis granulomas is spatially organized. Nat Med. (2016) 22:531–8. doi: 10.1038/nm.4073
51. Bresser PL, Reed J, Sathekge MM, Vorster M. (68) Ga-nitroimidazole PET/CT imaging of hypoxia in tuberculosis: A case series. J Med Radiat Sci. (2022) 69:518–24. doi: 10.1002/jmrs.v69.4
52. Belton M, Brilha S, Manavaki R, Mauri F, Nijran K, Hong YT, et al. Hypoxia and tissue destruction in pulmonary TB. Thorax. (2016) 71:1145–53. doi: 10.1136/thoraxjnl-2015-207402
53. Harper J, Skerry C, Davis SL, Tasneen R, Weir M, Kramnik I, et al. Mouse model of necrotic tuberculosis granulomas develops hypoxic lesions. J Infect Dis. (2012) 205:595–602. doi: 10.1093/infdis/jir786
54. Via LE, Lin PL, Ray SM, Carrillo J, Allen SS, Eum SY, et al. Tuberculous granulomas are hypoxic in Guinea pigs, rabbits, and nonhuman primates. Infect Immun. (2008) 76:2333–40. doi: 10.1128/IAI.01515-07
55. Plumlee CR, Duffy FJ, Gern BH, Delahaye JL, Cohen SB, Stoltzfus CR, et al. Ultra-low dose aerosol infection of mice with mycobacterium tuberculosis more closely models human tuberculosis. Cell Host Microbe. (2021) 29:68–82 e5. doi: 10.1016/j.chom.2020.10.003
56. Kumar A, Toledo JC, Patel RP, Lancaster JR, Steyn AJC. Mycobacterium tuberculosis DosS is a redox sensor and DosT is a hypoxia sensor. Proc Natl Acad Sci. (2007) 104:11568–73. doi: 10.1073/pnas.0705054104
57. Voskuil MI, Schnappinger D, Visconti KC, Harrell MI, Dolganov GM, Sherman DR, et al. Inhibition of respiration by nitric oxide induces a Mycobacterium tuberculosis dormancy program. J Exp Med. (2003) 198:705–13. doi: 10.1084/jem.20030205
58. Hudock TA, Foreman TW, Bandyopadhyay N, Gautam US, Veatch AV, LoBato DN, et al. Hypoxia Sensing and Persistence Genes Are Expressed during the Intragranulomatous Survival of Mycobacterium tuberculosis. Am J Respir Cell Mol Biol. (2017) 56:637–47. doi: 10.1165/rcmb.2016-0239OC
59. Mehra S, Foreman TW, Didier PJ, Ahsan MH, Hudock TA, Kissee R, et al. The dosR regulon modulates adaptive immunity and is essential for mycobacterium tuberculosis persistence. Am J Respir Crit Care Med. (2015) 191:1185–96. doi: 10.1164/rccm.201408-1502OC
60. Ong CWM, Fox K, Ettorre A, Elkington PT, Friedland JS. Hypoxia increases neutrophil-driven matrix destruction after exposure to Mycobacterium tuberculosis. Sci Rep. (2018) 8:11475. doi: 10.1038/s41598-018-29659-1
61. Mirchandani AS, Jenkins SJ, Bain CC, Sanchez-Garcia MA, Lawson H, Coelho P, et al. Hypoxia shapes the immune landscape in lung injury and promotes the persistence of inflammation. Nat Immunol. (2022) 23:927–39. doi: 10.1038/s41590-022-01216-z
62. Sekine H, Takeda H, Takeda N, Kishino A, Anzawa H, Isagawa T, et al. PNPO-PLP axis senses prolonged hypoxia in macrophages by regulating lysosomal activity. Nat Metab. (2024) 6:1108–27. doi: 10.1038/s42255-024-01053-4
63. Hong J, Wuest TR, Min Y, Lin PC. Oxygen tension regulates lysosomal activation and receptor tyrosine kinase degradation. Cancers (Basel). (2019) 11:1653. doi: 10.3390/cancers11111653
64. Hao T, Yu J, Wu Z, Jiang J, Gong L, Wang B, et al. Hypoxia-reprogramed megamitochondrion contacts and engulfs lysosome to mediate mitochondrial self-digestion. Nat Commun. (2023) 14:4105. doi: 10.1038/s41467-023-39811-9
65. Sutendra G, Dromparis P, Wright P, Bonnet S, Haromy A, Hao Z, et al. The role of Nogo and the mitochondria-endoplasmic reticulum unit in pulmonary hypertension. Sci Transl Med. (2011) 3:88ra55. doi: 10.1126/scitranslmed.3002194
66. Sharma D, Barhwal KK, Biswal SN, Srivastava AK, Bhardwaj P, Kumar A, et al. Hypoxia-mediated alteration in cholesterol oxidation and raft dynamics regulates BDNF signalling and neurodegeneration in hippocampus. J Neurochem. (2019) 148:238–51. doi: 10.1111/jnc.2019.148.issue-2
67. Liu R, Muliadi V, Mou W, Li H, Yuan J, Holmberg J, et al. HIF-1 stabilization in T cells hampers the control of Mycobacterium tuberculosis infection. Nat Commun. (2022) 13:5093. doi: 10.1038/s41467-022-32639-9
68. Cheng H, Ji Z, Wang Y, Li S, Tang T, Wang F, et al. Mycobacterium tuberculosis produces d-serine under hypoxia to limit CD8+ T cell-dependent immunity in mice. Nat Microbiol. (2024) 9:1856–72. doi: 10.1038/s41564-024-01701-1
69. Bucsan AN, Veatch A, Singh DK, Akter S, Golden NA, Kirkpatrick M, et al. Response to hypoxia and the ensuing dysregulation of inflammation impacts mycobacterium tuberculosis pathogenicity. Am J Respir Crit Care Med. (2022) 206:94–104. doi: 10.1164/rccm.202112-2747OC
70. Duque-Correa MA, Kuhl AA, Rodriguez PC, Zedler U, Schommer-Leitner S, Rao M, et al. Macrophage arginase-1 controls bacterial growth and pathology in hypoxic tuberculosis granulomas. Proc Natl Acad Sci U S A. (2014) 111:E4024–32. doi: 10.1073/pnas.1408839111
71. Yang CT, Cambier CJ, Davis JM, Hall CJ, Crosier PS, Ramakrishnan L. Neutrophils exert protection in the early tuberculous granuloma by oxidative killing of mycobacteria phagocytosed from infected macrophages. Cell Host Microbe. (2012) 12:301–12. doi: 10.1016/j.chom.2012.07.009
72. Reece ST, Loddenkemper C, Askew DJ, Zedler U, Schommer-Leitner S, Stein M, et al. Serine protease activity contributes to control of Mycobacterium tuberculosis in hypoxic lung granulomas in mice. J Clin Invest. (2010) 120:3365–76. doi: 10.1172/JCI42796
73. McCaffrey EF, Delmastro CA, Fitzhugh I, Ranek JS, Douglas S, Peters MJ, et al. The immunometabolic topography of tuberculosis granulomas governs cellular organization and bacterial control. bioRxiv. (2025). doi: 10.1101/2025.02.18.638923
74. Harding JS, Herbath M, Chen Y, Rayasam A, Ritter A, Csoka B, et al. VEGF-A from granuloma macrophages regulates granulomatous inflammation by a non-angiogenic pathway during mycobacterial infection. Cell Rep. (2019) 27:2119–31 e6. doi: 10.1016/j.celrep.2019.04.072
75. Datta M, Via LE, Kamoun WS, Liu C, Chen W, Seano G, et al. Anti-vascular endothelial growth factor treatment normalizes tuberculosis granuloma vasculature and improves small molecule delivery. Proc Natl Acad Sci. (2015) 112:1827–32. doi: 10.1073/pnas.1424563112
76. Polena H, Boudou F, Tilleul S, Dubois-Colas N, Lecointe C, Rakotosamimanana N, et al. Mycobacterium tuberculosis exploits the formation of new blood vessels for its dissemination. Sci Rep. (2016) 6:33162. doi: 10.1038/srep33162
77. Shiloh MU, Manzanillo P, Cox JS. Mycobacterium tuberculosis senses host-derived carbon monoxide during macrophage infection. Cell Host Microbe. (2008) 3:323–30. doi: 10.1016/j.chom.2008.03.007
78. Bhatia M. Role of hydrogen sulfide in the pathology of inflammation. Sci (Cairo). (2012) 2012:159680. doi: 10.6064/2012/159680
79. Kunota TTR, Rahman MA, Truebody BE, Mackenzie JS, Saini V, Lamprecht DA, et al. Mycobacterium tuberculosis H(2)S functions as a sink to modulate central metabolism, bioenergetics, and drug susceptibility. Antioxid (Basel). (2021) 10:1285. doi: 10.3390/antiox10081285
80. Saini V, Chinta KC, Reddy VP, Glasgow JN, Stein A, Lamprecht DA, et al. Hydrogen sulfide stimulates Mycobacterium tuberculosis respiration, growth and pathogenesis. Nat Commun. (2020) 11:557. doi: 10.1038/s41467-019-14132-y
81. Shi L, Salamon H, Eugenin EA, Pine R, Cooper A, Gennaro ML. Infection with Mycobacterium tuberculosis induces the Warburg effect in mouse lungs. Sci Rep. (2015) 5:18176. doi: 10.1038/srep18176
82. Shin JH, Yang JY, Jeon BY, Yoon YJ, Cho SN, Kang YH, et al. (1)H NMR-based metabolomic profiling in mice infected with Mycobacterium tuberculosis. J Proteome Res. (2011) 10:2238–47. doi: 10.1021/pr101054m
83. Somashekar BS, Amin AG, Rithner CD, Troudt J, Basaraba R, Izzo A, et al. Metabolic profiling of lung granuloma in Mycobacterium tuberculosis infected Guinea pigs: ex vivo 1H magic angle spinning NMR studies. J Proteome Res. (2011) 10:4186–95. doi: 10.1021/pr2003352
84. Kempker RR, Heinrichs MT, Nikolaishvili K, Sabulua I, Bablishvili N, Gogishvili S, et al. Lung tissue concentrations of pyrazinamide among patients with drug-resistant pulmonary tuberculosis. Antimicrob Agents Chemother. (2017) 61. doi: 10.1128/AAC.00226-17
85. Weiss C, Tabachnick J, Cohen HP. Mechanism of softening of tubercles. III. Hydrolysis of protein and nucleic acid during anaerobic autolysis of normal and tuberculous lung tissue in vitro. AMA Arch Pathol. (1954) 57:179–93.
86. Lanoix JP, Lenaerts AJ, Nuermberger EL. Heterogeneous disease progression and treatment response in a C3HeB/FeJ mouse model of tuberculosis. Dis Model Mech. (2015) 8:603–10. doi: 10.1242/dmm.019513
87. Nair S, Huynh JP, Lampropoulou V, Loginicheva E, Esaulova E, Gounder AP, et al. Irg1 expression in myeloid cells prevents immunopathology during M. tuberculosis infection. J Exp Med. (2018) 215:1035–45. doi: 10.1084/jem.20180118
88. Kim MJ, Wainwright HC, Locketz M, Bekker LG, Walther GB, Dittrich C, et al. Caseation of human tuberculosis granulomas correlates with elevated host lipid metabolism. EMBO Mol Med. (2010) 2:258–74-74. doi: 10.1002/emmm.201000079
89. Mehra S, Alvarez X, Didier PJ, Doyle LA, Blanchard JL, Lackner AA, et al. Granuloma correlates of protection against tuberculosis and mechanisms of immune modulation by Mycobacterium tuberculosis. J Infect Dis. (2013) 207:1115–27. doi: 10.1093/infdis/jis778
90. Gautam US, Foreman TW, Bucsan AN, Veatch AV, Alvarez X, Adekambi T, et al. In vivo inhibition of tryptophan catabolism reorganizes the tuberculoma and augments immune-mediated control of Mycobacterium tuberculosis. Proc Natl Acad Sci USA. (2018) 115:E62–71. doi: 10.1073/pnas.1711373114
91. Peng C, Cheng Y, Ma M, Chen Q, Duan Y, Liu S, et al. Mycobacterium tuberculosis suppresses host antimicrobial peptides by dehydrogenating L-alanine. Nat Commun. (2024) 15:4216. doi: 10.1038/s41467-024-48588-4
92. Rangel Moreno J, Estrada Garcia I, de la Luz Garcia Hernandez M, Aguilar Leon D, Marquez R, Hernandez Pando R. The role of prostaglandin E2 in the immunopathogenesis of experimental pulmonary tuberculosis. Immunology. (2002) 106:257–66. doi: 10.1046/j.1365-2567.2002.01403.x
93. Divangahi M, Desjardins D, Nunes-Alves C, Remold HG, Behar SM. Eicosanoid pathways regulate adaptive immunity to Mycobacterium tuberculosis. Nat Immunol. (2010) 11:751–8. doi: 10.1038/ni.1904
94. Chen M, Divangahi M, Gan H, Shin DS, Hong S, Lee DM, et al. Lipid mediators in innate immunity against tuberculosis: opposing roles of PGE2 and LXA4 in the induction of macrophage death. J Exp Med. (2008) 205:2791–801. doi: 10.1084/jem.20080767
95. Dobbs Thomas E, Webb Risa M. Chemotherapy of tuberculosis. Microbiol Spectrum. (2017) 5. doi: 10.1128/microbiolspec.tnmi7-0040-2017
96. Blanc L, Daudelin IB, Podell BK, Chen PY, Zimmerman M, Martinot AJ, et al. High-resolution mapping of fluoroquinolones in TB rabbit lesions reveals specific distribution in immune cell types. Elife. (2018) 7. doi: 10.7554/eLife.41115
97. Cubillos-Angulo JM, Nogueira BMF, Arriaga MB, Barreto-Duarte B, Araujo-Pereira M, Fernandes CD, et al. Host-directed therapies in pulmonary tuberculosis: Updates on anti-inflammatory drugs. Front Med (Lausanne). (2022) 9:970408. doi: 10.3389/fmed.2022.970408
98. Li H, Zuo J, Tang W. Phosphodiesterase-4 inhibitors for the treatment of inflammatory diseases. Front Pharmacol. (2018) 9:1048. doi: 10.3389/fphar.2018.01048
99. Subbian S, Tsenova L, Holloway J, Peixoto B, O’Brien P, Dartois V, et al. Adjunctive phosphodiesterase-4 inhibitor therapy improves antibiotic response to pulmonary tuberculosis in a rabbit model. EBioMedicine. (2016) 4:104–14. doi: 10.1016/j.ebiom.2016.01.015
100. Subbian S, Koo MS, Tsenova L, Khetani V, Zeldis JB, Fallows D, et al. Pharmacologic inhibition of host phosphodiesterase-4 improves isoniazid-mediated clearance of mycobacterium tuberculosis. Front Immunol. (2016) 7:238. doi: 10.3389/fimmu.2016.00238
101. Saunders BM, Tran S, Ruuls S, Sedgwick JD, Briscoe H, Britton WJ. Transmembrane TNF is sufficient to initiate cell migration and granuloma formation and provide acute, but not long-term, control of Mycobacterium tuberculosis infection. J Immunol. (2005) 174:4852–9. doi: 10.4049/jimmunol.174.8.4852
102. Flynn JL, Goldstein MM, Chan J, Triebold KJ, Pfeffer K, Lowenstein CJ, et al. Tumor necrosis factor-alpha is required in the protective immune response against Mycobacterium tuberculosis in mice. Immunity. (1995) 2:561–72. doi: 10.1016/1074-7613(95)90001-2
103. Wallis RS, Ginindza S, Beattie T, Arjun N, Likoti M, Edward VA, et al. Adjunctive host-directed therapies for pulmonary tuberculosis: a prospective, open-label, phase 2, randomised controlled trial. Lancet Respir Med. (2021) 9:897–908. doi: 10.1016/S2213-2600(20)30448-3
104. Subbian S, Tsenova L, O’Brien P, Yang G, Koo MS, Peixoto B, et al. Phosphodiesterase-4 inhibition combined with isoniazid treatment of rabbits with pulmonary tuberculosis reduces macrophage activation and lung pathology. Am J Pathol. (2011) 179:289–301. doi: 10.1016/j.ajpath.2011.03.039
105. Elkington PT, Friedland JS. Matrix metalloproteinases in destructive pulmonary pathology. Thorax. (2006) 61:259–66. doi: 10.1136/thx.2005.051979
106. Ordonez AA, Pokkali S, Kim S, Carr B, Klunk MH, Tong L, et al. Adjunct antibody administration with standard treatment reduces relapse rates in a murine tuberculosis model of necrotic granulomas. PloS One. (2018) 13:e0197474. doi: 10.1371/journal.pone.0197474
107. Parasa VR, Muvva JR, Rose JF, Braian C, Brighenti S, Lerm M. Inhibition of tissue matrix metalloproteinases interferes with mycobacterium tuberculosis-induced granuloma formation and reduces bacterial load in a human lung tissue model. Front Microbiol. (2017) 8:2370. doi: 10.3389/fmicb.2017.02370
108. Xu Y, Wang L, Zimmerman MD, Chen KY, Huang L, Fu DJ, et al. Matrix metalloproteinase inhibitors enhance the efficacy of frontline drugs against Mycobacterium tuberculosis. PloS Pathog. (2018) 14:e1006974. doi: 10.1371/journal.ppat.1006974
109. Miow QH, Vallejo AF, Wang Y, Hong JM, Bai C, Teo FS, et al. Doxycycline host-directed therapy in human pulmonary tuberculosis. J Clin Invest. (2021) 131. doi: 10.1172/JCI141895
110. Oehlers SH, Cronan MR, Scott NR, Thomas MI, Okuda KS, Walton EM, et al. Interception of host angiogenic signalling limits mycobacterial growth. Nature. (2015) 517:612–5. doi: 10.1038/nature13967
111. Datta M, Via LE, Dartois V, Weiner DM, Zimmerman M, Kaya F, et al. Normalizing granuloma vasculature and matrix improves drug delivery and reduces bacterial burden in tuberculosis-infected rabbits. Proc Natl Acad Sci U S A. (2024) 121:e2321336121. doi: 10.1073/pnas.2321336121
112. Young C, Walzl G, Du Plessis N. Therapeutic host-directed strategies to improve outcome in tuberculosis. Mucosal Immunol. (2020) 13:190–204. doi: 10.1038/s41385-019-0226-5
113. Singh B, Moodley C, Singh DK, Escobedo RA, Sharan R, Arora G, et al. Inhibition of indoleamine dioxygenase leads to better control of tuberculosis adjunctive to chemotherapy. JCI Insight. (2023) 8. doi: 10.1172/jci.insight.163101
114. Petrucciani A, Hoerter A, Kotze L, Du Plessis N, Pienaar E. Agent-based model predicts that layered structure and 3D movement work synergistically to reduce bacterial load in 3D in vitro models of tuberculosis granuloma. PloS Comput Biol. (2024) 20:e1012266. doi: 10.1371/journal.pcbi.1012266
115. Budak M, Via LE, Weiner DM, Barry CE 3rd, Nanda P, Michael G, et al. A systematic efficacy analysis of tuberculosis treatment with BPaL-containing regimens using a multiscale modeling approach. CPT Pharmacometr Syst Pharmacol. (2024) 13:673–85. doi: 10.1002/psp4.13117
116. Reichmann MT, Tezera LB, Vallejo AF, Vukmirovic M, Xiao R, Reynolds J, et al. Integrated transcriptomic analysis of human tuberculosis granulomas and a biomimetic model identifies therapeutic targets. J Clin Invest. (2021) 131. doi: 10.1172/JCI148136
117. Kotze LA, Beltran CGG, Lang D, Loxton AG, Cooper S, Meiring M, et al. Establishment of a patient-derived, magnetic levitation-based, three-dimensional spheroid granuloma model for human tuberculosis. mSphere. (2021) 6:e0055221. doi: 10.1128/mSphere.00552-21
118. Arbues A, Brees D, Chibout SD, Fox T, Kammuller M, Portevin D. TNF-alpha antagonists differentially induce TGF-beta1-dependent resuscitation of dormant-like Mycobacterium tuberculosis. PloS Pathog. (2020) 16:e1008312. doi: 10.1371/journal.ppat.1008312
119. Kapoor N, Pawar S, Sirakova TD, Deb C, Warren WL, Kolattukudy PE. Human granuloma in vitro model, for TB dormancy and resuscitation. PloS One. (2013) 8:e53657. doi: 10.1371/journal.pone.0053657
120. Pisu D, Huang L, Narang V, Theriault M, Le-Bury G, Lee B, et al. Single cell analysis of M. tuberculosis phenotype and macrophage lineages in the infected lung. J Exp Med. (2021) 218. doi: 10.1084/jem.20210615
121. Pisu D, Johnston L, Mattila JT, Russell DG. The frequency of CD38(+) alveolar macrophages correlates with early control of M. tuberculosis in the murine lung. Nat Commun. (2024) 15:8522. doi: 10.1038/s41467-024-52846-w
122. Phuah JY, Mattila JT, Lin PL, Flynn JL. Activated B cells in the granulomas of nonhuman primates infected with Mycobacterium tuberculosis. Am J Pathol. (2012) 181:508–14. doi: 10.1016/j.ajpath.2012.05.009
123. Millar JA, Butler JR, Evans S, Mattila JT, Linderman JJ, Flynn JL, et al. Spatial organization and recruitment of non-specific T cells may limit T cell-macrophage interactions within mycobacterium tuberculosis granulomas. Front Immunol. (2020) 11:613638. doi: 10.3389/fimmu.2020.613638
124. Salehi Farid A, Rowley JE, Allen HH, Kruger IG, Tavakolpour S, Neeley K, et al. CD45-PET is a robust, non-invasive tool for imaging inflammation. Nature. (2025) 639:214–24. doi: 10.1038/s41586-024-08441-6
125. Fearns A, Greenwood DJ, Rodgers A, Jiang H, Gutierrez MG. Correlative light electron ion microscopy reveals in vivo localisation of bedaquiline in Mycobacterium tuberculosis-infected lungs. PloS Biol. (2020) 18:e3000879. doi: 10.1371/journal.pbio.3000879
126. Greenwood DJ, Dos Santos MS, Huang S, Russell MRG, Collinson LM, MacRae JI, et al. Subcellular antibiotic visualization reveals a dynamic drug reservoir in infected macrophages. Science. (2019) 364:1279–82. doi: 10.1126/science.aat9689
127. Sousa J, Ca B, Maceiras AR, Simoes-Costa L, Fonseca KL, Fernandes AI, et al. Mycobacterium tuberculosis associated with severe tuberculosis evades cytosolic surveillance systems and modulates IL-1beta production. Nat Commun. (2020) 11:1949. doi: 10.1038/s41467-020-15832-6
128. Arbués A, Schmidiger S, Reinhard M, Borrell S, Gagneux S, Portevin D. CXCL9, granzyme B and TNF-α orchestrate protective in vitro granulomatous responses across Mycobacterium tuberculosis complex lineages. bioRxiv. (2024):595219. doi: 10.1101/2024.05.21.595219
Keywords: tuberculosis, granuloma, spatial biology, host-directed therapy, immunity
Citation: Krueger G, Faisal S and Dorhoi A (2025) Microenvironments of tuberculous granuloma: advances and opportunities for therapy. Front. Immunol. 16:1575133. doi: 10.3389/fimmu.2025.1575133
Received: 11 February 2025; Accepted: 03 March 2025;
Published: 24 March 2025.
Edited by:
Andreas Kupz, James Cook University, AustraliaReviewed by:
Selvakumar Subbian, Rutgers, The State University of New Jersey, United StatesCopyright © 2025 Krueger, Faisal and Dorhoi. This is an open-access article distributed under the terms of the Creative Commons Attribution License (CC BY). The use, distribution or reproduction in other forums is permitted, provided the original author(s) and the copyright owner(s) are credited and that the original publication in this journal is cited, in accordance with accepted academic practice. No use, distribution or reproduction is permitted which does not comply with these terms.
*Correspondence: Anca Dorhoi, YW5jYS5kb3Job2lAZmxpLmRl
†These authors have contributed equally to this work
Disclaimer: All claims expressed in this article are solely those of the authors and do not necessarily represent those of their affiliated organizations, or those of the publisher, the editors and the reviewers. Any product that may be evaluated in this article or claim that may be made by its manufacturer is not guaranteed or endorsed by the publisher.
Research integrity at Frontiers
Learn more about the work of our research integrity team to safeguard the quality of each article we publish.