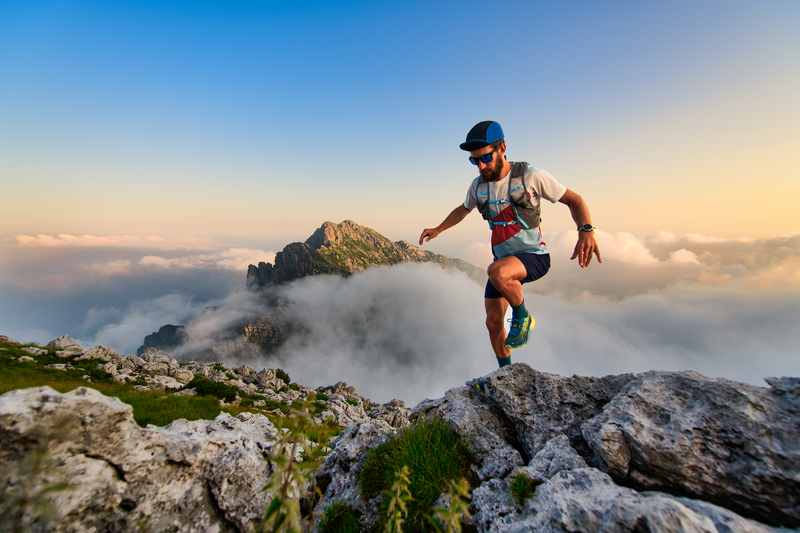
95% of researchers rate our articles as excellent or good
Learn more about the work of our research integrity team to safeguard the quality of each article we publish.
Find out more
REVIEW article
Front. Immunol. , 20 March 2025
Sec. Autoimmune and Autoinflammatory Disorders : Autoimmune Disorders
Volume 16 - 2025 | https://doi.org/10.3389/fimmu.2025.1567833
This article is part of the Research Topic Community Series in The Role of Monocytes/Macrophages in Autoimmunity and Autoinflammation: Volume II View all 5 articles
Idiopathic inflammatory myopathies (IIMs) are heterogeneous autoimmune disorders characterized by muscle inflammation, weakness, and extramuscular manifestations such as interstitial lung disease, skin rash, arthritis, dysphagia, myocarditis and other systemic organ involvement. Although T and B cells have historically been central to the understanding of IIM immunopathology, monocytes and their differentiated progenitor cells, macrophages, are increasingly being recognized as critical mediators of both tissue damage and repair. In subtypes such as dermatomyositis, immune-mediated necrotizing myopathy and antisynthetase syndrome, macrophages infiltrate skeletal muscle and other affected tissues, contributing to inflammation via production of pro-inflammatory cytokines, chemokines, and reactive oxygen species. Dysregulated interferon signaling, mitochondrial stress, and aberrant metabolic states in these cells further perpetuate tissue injury in IIMs. Conversely, certain macrophage subsets can support muscle fiber regeneration and dampen inflammation, underscoring the dual roles these cells can play. Future research into the heterogeneity of monocytes and macrophages, including single-cell transcriptomic and metabolomic approaches, will help clarify disease mechanisms, identify biomarkers of disease activity and prognosis, and guide novel therapeutic strategies targeting these innate immune cells in IIM.
Idiopathic inflammatory myopathies (IIMs) represent a diverse group of autoimmune diseases characterized by immune-mediated damage to skeletal muscle. IIM subgroups include dermatomyositis (DM), antisynthetase syndrome (ASyS), polymyositis (PM), immune-mediated necrotizing myopathy (IMNM), and inclusion body myositis, each of which exhibits distinct clinical and pathological features (1). IIM manifestations extend beyond muscle weakness, frequently involving skin rashes, arthritis/arthralgia, interstitial lung disease (ILD), and cardiac complications (1–3). While early research focused primarily on the roles of T and B lymphocytes and dendritic cells, a growing body of evidence highlights the crucial involvement of monocytes and macrophages in the initiation, progression and resolution of muscle inflammation in IIMs (4–7). Monocytes are recruited to affected tissues where they can be activated, differentiating into various forms of macrophages that contribute to tissue damage, modulate the local inflammatory milieu and participate in repair mechanisms. Given the increasing recognition of the roles of monocytes and macrophages in IIM pathology, this review aims to delineate their diverse functions, explore their contribution to disease mechanisms, and discuss potential targeted therapeutic strategies.
The myeloid cell compartment, comprising monocytes, macrophages, granulocytes and dendritic cells, serves not only as the first line of immune defense but also plays a pivotal role in mediating communication between innate and adaptive immunity (8, 9). Traditional flow cytometry methods for classifying blood monocytes have relied on the distinction among classical (CD14++CD16−), intermediate (CD14++CD16+), and non-classical (CD14+CD16++) subsets (10). However, single-cell RNA sequencing (scRNA-seq) has revolutionized our understanding of monocyte heterogeneity, revealing a more complex and dynamic picture of their subsets. This technology demonstrates a spectrum of transcriptional states within these subsets, each characterized by specific cytokine profiles, interferon (IFN) signatures, chemokine receptor expression and metabolic activity (11, 12). This refined understanding will be crucial for dissecting the specific roles of different monocyte and macrophage populations in the pathogenesis of IIMs.
While not all macrophages are derived from monocytes (13), in the context of myositis, monocytes are recruited to affected muscle tissues, where they differentiate into monocyte-derived macrophages (14). Once within the muscle tissue, these monocytes undergo further differentiation into functionally plastic macrophages, exhibiting a wide range of activation states (15). Macrophages are traditionally categorized as M1-like (pro-inflammatory) or M2-like (anti-inflammatory and tissue-repairing) subsets; however, in vivo macrophage phenotypes are considerably more nuanced and context-dependent (16). Indeed, macrophages exhibit pro-inflammatory, pro-wound-healing, pro-fibrotic, anti-inflammatory, anti-fibrotic, pro-resolving, and tissue-regenerating properties, often with overlapping or sequential expression (16). M1-like macrophages are often activated by IFN-γ and bacterial products via pathways including the IFN-γ/Janus kinase (JAK)/signal transducer and activator of transcription 1 (STAT1), Toll-like receptor (TLR) activation of TIR domain-containing adaptor inducing interferon-β/interferon regulatory factor 3 for IFN secretion, and TLR/MyD88/NF-κB for cytokine secretion, and these macrophages secrete inflammatory cytokines and chemokines (e.g., tumor necrosis factor (TNF)-α, interleukins (IL-1, IL-6), chemokines (e.g., C-C motif ligand (CCL)2/4, and C-X-C motif chemokine ligand (CXCL)8/11), nitric oxide, and reactive oxygen species (ROS) (15). These factors exacerbate local inflammation and contribute to muscle fiber necrosis.
Macrophages also play a crucial role in resolving inflammation by clearing cellular debris. M2-like macrophages, often induced by IL-4 and IL-10 via the IL-4/IL-13/JAK1/JAK3/STAT6 and IL-10/STAT3 pathways, respectively, secrete anti-inflammatory mediators (e.g., IL-10) and the growth factors IGF-1 and transforming growth factor β, which can both promote tissue repair and, in some contexts, contribute to fibrosis (17). They may also promote myogenic differentiation and facilitate muscle fiber regeneration. However, the M1/M2 paradigm should be considered a spectrum, with considerable plasticity in macrophage phenotypes influenced by the local microenvironment (16). Understanding these diverse macrophage phenotypes lays the groundwork for examining changes in circulating monocyte subsets and their contribution to the tissue-specific pathology of IIMs.
Studies of circulating monocyte populations in IIMs have revealed alterations in subset distribution and activation status. Notably, in patients with active anti-melanoma differentiation-associated protein 5 (MDA5) antibody-positive DM, the proportion of circulating classical monocytes was increased compared with healthy controls (18). Classical monocytes are characterized by their production of S100 family proteins, leading to pro-inflammatory responses (19, 20). Furthermore, monocyte subsets in IIM, including classical monocytes, show elevated expression of genes involved in neutrophil and monocyte activation, such as S100A8, S100A9, S100A12, FCGR3B, and CXCR2 (18). Similarly, mass cytometry of peripheral blood mononuclear cells (PBMCs) revealed an increased proportion of CD14+CD16-CD19-CD3- classical monocytes in IIM patients compared with healthy controls (21). Furthermore, bulk RNA-seq analysis of PBMCs from patients with IIMs, along with deconvolution analysis using CIBERSORTx algorithm (22), also indicates an increased proportion of monocytes compared to healthy controls (23). In the study, flow cytometry analysis further confirmed the increased proportions of classical and intermediate monocyte subsets in IIM patients compared to healthy controls. These changes in PBMC populations have been identified in patients with both anti-MDA5 antibody-positive DM and anti-Jo-1 antibody-positive IIMs (23). Notably, scRNA-seq of PBMCs detected IFI27-expressing CD14+ monocytes as a key feature in patients with active DM positive for anti-MDA5 antibodies (24). Since IFI27 is an IFN-inducible gene, this finding suggests that IFN signature and activated monocytes are contributing to the pathogenesis of anti-MDA5 antibody-positive DM.
In the pathogenesis of myositis, monocytes are actively recruited from the circulation to inflamed muscle tissue. This process is driven by intricate interplay among chemokines and adhesion molecules (14). Notably, elevated levels of several chemokines, including CCL2, CCL3, CCL4, CXCL8, CXCL9, and CXCL10, have been identified within the muscle tissue of IIM patients (25–31). These chemokines act as chemoattractants, guiding monocytes and lymphocytes to the site of inflammation (31). Specifically, CCL2, CCL3, CCL4, CXCL8, and CXCL10 levels are also increased in the peripheral blood of patients with IIM (28, 32, 33), suggesting their role in the systemic inflammatory response simultaneously. Moreover, the serum CXCL8 level has been identified as a predictive marker for rapidly progressing ILD (RP-ILD) among patients with IIM-associated ILD (34). Similarly, the serum CXCL10 level is correlated with the Cutaneous Dermatomyositis Disease Area and Severity Index (CDASI) score in patients with DM (35). Further emphasizing this connection, the serum CXCL10 levels in anti-MDA5 antibody-positive DM patients are markedly elevated at disease onset and decrease upon treatment (36). This highlights the systemic nature of inflammation and the involvement of chemokines in multi-organ manifestations of IIMs. Interestingly, monocytes have been shown to produce CXCL10 in a dose-dependent manner upon type I IFN stimulation in vivo (36). This suggests a positive feedback loop wherein monocyte activation and type I IFN signaling amplify inflammation. In addition, increased levels of vascular adhesion molecules, such as vascular cell adhesion molecule 1, are also observed in patients with DM, particularly those who develop severe ILD (37). This increased expression of adhesion molecules likely further facilitates the migration of monocytes into the damaged organs.
Histopathological studies consistently reveal significant infiltration of monocytes and macrophages within the muscle tissue of IIM patients (1, 14). Macrophages are often found distributed around the endomysium and perimysium in patients with DM. However, the distribution of macrophages in affected muscle can vary considerably depending on the IIM subtype and specific autoantibody profile. For instance, muscle specimens from juvenile patients with anti-nuclear matrix protein (NXP) 2 and anti-transcriptional intermediary factor (TIF) 1-γ antibodies show pronounced diffuse endomysial macrophage infiltration (38). Conversely, muscle biopsies from anti-MDA5 antibody-positive DM patients typically show only mild inflammatory cell infiltration (38). However, biopsies of juvenile DM patients with anti-Mi2 antibodies in one study tended to show greater necrosis or perifascicular atrophy rather than purely diffuse macrophage infiltration (39). These infiltrations mirrored those found in adult DM; anti-NXP2, anti-TIF1-γ, and anti-Mi2 antibody-positive DM displayed similar CD68+ infiltration in both endomysial and perimysial areas, while anti-MDA5-positive DM showed less CD68+ cell infiltration (40) (Supplementary Figure S1). In patients with ASyS (41) and IMNM (42), macrophages are diffusely distributed within the endomysium. This implies that the degree and pattern of macrophage infiltration reflect distinct underlying pathogenic mechanisms in different IIM subtypes. Once recruited to muscle tissues, macrophages contribute to myophagocytosis, the process of engulfing damaged muscle fibers, and release pro-inflammatory cytokines, including IL-1, IL-6, and TNF (43). These actions amplify the inflammatory response and directly contribute to muscle damage.
Our previous transcriptomic analysis revealed a strong association between increased monocyte infiltration into muscle tissue and muscle damage (6). By applying a deconvolution algorithm to bulk RNA-seq data from muscle tissue of IIM patients, we quantified the proportions of infiltrating immune cell types. We found that the estimated proportions of CD16+ and CD16- monocytes, as well as myeloid dendritic cells, are positively correlated with serum creatine kinase and aldolase levels, further reinforcing their involvement in muscle damage. Similarly, gene modules associated with phagocytosis also exhibit a positive correlation with these muscle enzymes. Supporting these findings, histological analysis of muscle tissue from patients refractory to standard immunosuppressive treatment for myositis revealed a larger area occupied by CD68+ macrophages compared with muscle tissue from patients who responded to treatment (44). Additionally, a negative correlation was observed between the manual muscle testing 8 score at baseline and the area occupied by CD68+ cells before treatment. This suggests that the extent of macrophage infiltration may be a predictive marker of disease severity and treatment response.
While direct evidence of monocyte and/or macrophage infiltration in lung tissues from IIM patients is limited, this is largely because bronchoalveolar lavage fluid (BALF) and lung biopsies are not necessary for clinical diagnosis and do not substantially contribute to clinical decision-making. This is especially true when radiological patterns of ILD are evident through imaging or serological markers such as myositis-specific antibodies or myositis-associated antibodies are positive (45, 46).
Nonetheless, there is growing evidence suggesting that macrophages likely play a significant role in the development of IIM-associated ILD. One immunohistochemical analysis showed that CD163+ macrophages were found to infiltrate the alveolar spaces in the lungs of patients with DM-related ILD and were more severe in the lungs of a non-surviving patient (47). The serum level of soluble CD163, a type I transmembrane protein and a marker of macrophage activation, is also elevated in DM and PM patients with ILD compared to those without ILD, and it correlates with disease activity (48). Additionally, recent scRNA-seq of BALF from patients with DM revealed an upregulation of genes associated with IFN-related pathways, including IFIT1 and CXCL10, within monocytes/macrophages (49). Interestingly, a viral response signature, characterized by MX1 expression, was detected not only in lung-resident immune cells but also in circulating monocytes, neutrophils, B cells, and plasma cells in the blood of DM patients. This suggests a systemic immune response with a prominent pulmonary component in these patients.
Several serum markers associated with macrophage activation have been reported to predict prognosis in IIM-associated ILD. Ferritin is described as a macrophage activation marker and is introduced alongside other macrophage markers such as chitinase-3-like protein 1, soluble CD206, galectin-9, and neopterin in the context of anti-MDA5 antibody-positive DM (50–53). Although the precise cellular origin of ferritin in this condition remains unclear, the positive correlation between an increased percentage of HLA-DRlow CD14+ monocytes and both serum ferritin and IL-6 levels (54) suggests that these monocytes, in addition to macrophages, may also be a source of ferritin (55). Moreover, macrophages recycle iron from senescent erythrocytes, storing it intracellularly in ferritin. In inflammatory states, an elevated level of hepcidin leads to the degradation of ferroportin, causing macrophages to retain more iron. This results in increased ferritin synthesis and release, thus elevating the serum ferritin concentration. During macrophage activation, pro-inflammatory cytokines (e.g., IL-1β, IL-6) and hepcidin signals converge to upregulate ferritin expression (56). Additionally, the heavy subunits of ferritin may also function as a pro-inflammatory factor (57). While the ferritin level is linked to disease progression, particularly in patients with RP-ILD, the serum CXCL10 level has been reported to reflect the early treatment response more effectively compared with the ferritin level (36). In addition to ferritin, serum soluble CD206, a mannose receptor and marker for M2 macrophages (58) is also elevated in anti-MDA5 antibody-positive DM-associated ILD (59) and in RP-ILD (60). Furthermore, its serum level is correlated with a poor prognosis, indicating a potential pathogenic role (61). Although M2 macrophages generally play an anti-inflammatory role, CD206+ lung macrophages in bleomycin-induced lung fibrosis in mice contribute to lung fibrosis, suggesting a pathogenic fibrotic role in IIM-ILD (62). Neopterin, primarily produced by activated macrophages and monocytes stimulated with IFN-γ, exhibits higher serum levels in DM patients compared with healthy controls (63). Anti-MDA5 antibody-positive DM patients exhibit the highest levels of serum neopterin, and a high serum neopterin level was identified as an independent risk factor for mortality (63). Additionally, the serum level of YKL-40, primarily secreted by macrophages and neutrophils, has also been shown to predict the occurrence of RP-ILD and to indicate a poor prognosis (64). These findings underscore the complex interplay among macrophage activation, IFN signaling, and ILD disease severity, though interpretation should be approached with caution given the plasticity and heterogeneity of macrophages.
Emerging evidence suggests that macrophage polarization is not strictly binary (65), particularly in tissue-resident macrophages such as alveolar macrophages (AM). Indeed, AM from individuals in distinct tissue localization consistently express features of both M1 (CD80, CD86, CD64) and M2 (CD206 and CD163) polarized macrophages, and the CD206hiCD86hi AM subset remains the dominant population even after in vivo exposure to pneumococci or human immunodeficiency virus (66). Additionally, M2 macrophages can express CD86, an M1 marker, in response to TLR2 stimulation and exhibit a pro-inflammatory phenotype, further demonstrating the phenotypic and functional plasticity of macrophages. Moreover, macrophages exhibit significant heterogeneity within the M2 spectrum, encompassing distinct subsets (M2a, M2b, M2c, and M2d) with diverse roles in wound healing, immunoregulation, and tissue remodeling (65, 67). Given this complexity, the relationship between M2 macrophages and M1 markers cannot be simply defined, and macrophage-associated parameters must be interpreted within specific biological contexts.
The histology of DM skin is characterized by perivascular infiltrates of immune cells, including T cells and macrophages (68). Immunohistochemical analysis of DM skin lesions (specifically Gottron’s sign) in patients with anti-MDA5 antibody-positive DM revealed that the majority of CXCL10+ cells are also CD68+, indicating their monocyte/macrophage lineage (36). Furthermore, in addition to the presence of MxA, a protein specifically induced by type I IFN, CXCL10 is moderately to strongly expressed in DM skin, primarily within the upper dermis and epidermis (69). These skin areas also exhibit elevated numbers of CXC-chemokine receptor 3 (CXCR3)+ T cells. This suggests a potential mechanism wherein type I IFN-induced CXCL10 production by macrophages attracts T cells, contributing to the characteristic skin rash in DM.
While IFN signatures in muscle tissue are reportedly enriched in DM and, to a lesser extent, in ASyS (6, 70), studies employing immunofluorescence and highly multiplexed imaging mass cytometry showed that both ASyS and DM skin exhibit similarly elevated type I IFN signaling. This is evidenced by elevated levels of IFN-β and MxA compared with healthy controls (71). Interestingly, macrophage subsets, specifically those positive for phosphorylated stimulator of interferon genes (STING), display heightened production of pro-inflammatory mediators, such as TNF, IL-17, and IFN-β, in skin involvement in ASyS relative to those in DM (71). In contrast, the overall composition and activation of other immune cell populations, including dendritic and T cells, are largely similar between the two diseases. These findings demonstrate that while both ASyS and DM skin share common features of type I IFN activation, macrophages positive for phosphorylated STING may play a distinct role in modulating inflammation and contributing to the unique clinical features of cutaneous signs in ASyS.
The activation of monocytes in IIMs is influenced by complex interplay among factors such as galectin-9, IFN signaling, and mitochondrial dysfunction. Galectin-9, a β-galactose-binding protein secreted by vascular endothelial cells, promotes angiogenesis (72). In particular, the serum galectin-9 level is significantly increased in patients with anti-MDA5 antibody-positive DM compared with healthy controls (73–75). Furthermore, increased gene expression of galectin-9 is observed in both the serum and lung tissues of patients who developed RP-ILD (75). In vitro experiments confirmed that stimulation with galectin-9 leads to increased secretion of CCL2 from lung fibroblasts (75). Given that galectin-9 promotes differentiation into M2-type macrophages (76), these findings suggest that galectin-9 can stimulate monocyte/macrophage activity and contribute to lung fibrosis especially in anti-MDA5 antibody-positive DM. Interestingly, a positive correlation was observed between galectin-9 and type I IFN-inducible genes, such as MX1 and IFIH1, at the mRNA level in PBMCs, suggesting a potential synergistic or interconnected role of galectin-9 and type I IFN signaling in fibrosis associated with anti-MDA5 antibody-positive DM (75). This further emphasizes the intricate interplay of different inflammatory mediators in IIM pathogenesis.
Lately, emerging evidence has also highlighted the contribution of monocyte mitochondrial dysfunction to IIM pathology. In juvenile dermatomyositis (JDM), mitochondrial abnormalities in CD14+ monocytes, including the presence of “megamitochondria” and enhanced oxidative phosphorylation, promote the production of oxidized mitochondrial DNA (77). This oxidized mitochondrial DNA can activate the cyclic GMP-AMP synthase/STING pathway, driving further IFN production and muscle fiber damage. Additionally, dysregulated expression of mitochondrial-associated genes has been correlated with increased expression of IFN-stimulated genes in JDM CD14+ monocytes, highlighting this mechanistic link (77). Indeed, IFNs activate the transcription factor STAT1, which in turn induces the expression of M1 markers, including CXCL9 and CXCL10, via the IFN-α/β receptor (78, 79). These findings underscore the role of IFN signaling in promoting pro-inflammatory macrophage activation. Moreover, aberrant changes to mitochondrial morphology and cellular metabolism are key features of mitochondrial stress, associated with decreased oxidative phosphorylation and increased ROS levels (15, 80, 81). These observations highlight dysregulated mitochondrial dynamics and oxidative stress as critical pathological features linking monocyte abnormalities to IIM disease progression.
Monocytes and macrophages do not function in isolation in IIM; rather, they interact extensively with other immune cells, including CD8+ cytotoxic T cells, CD4+ helper T cells, and B cells (82). Activated macrophages initiate a cycle of inflammation by releasing pro-inflammatory cytokines and chemokines. These signals attract more immune cells, activate helper and cytotoxic T cells, which leads to tissue damage, and stimulate further macrophage activity. C-X3-C motif ligand 1 (CX3CL1), which is highly expressed in inflamed endothelial cells induced by type I IFN (83), plays a crucial role in attracting monocytes and T cells. An elevated CX3CL1 level in patients with anti-MDA5 antibody-positive DM shows a significant correlation with anti-MDA5 antibody titers (84). Notably, CX3CL1 can induce recruitment of CX3CR1+ M2 macrophages in the lungs (83, 85), potentially associated with lung fibrosis, suggesting complex interplay among endothelial activation, monocyte recruitment, and tissue fibrosis. In addition, dysregulation of regulatory T cells, which normally regulate macrophage phagocytosis, can contribute to disease development (86). These intricate cellular interactions might be critical to the pathogenesis of muscle damage. Unlike in healthy conditions, muscle fibers themselves can produce chemokines and express MHC class I in IIMs (82, 87), resulting in dynamic interplay in which both immune and muscle cells influence the other’s phenotypes and activities. Furthermore, monocyte-derived dendritic cells can facilitate myoblast proliferation and migration while upregulating the expression of HLA-ABC, HLA-DR, VLA-5, and VLA-6 in myoblasts (88). This further indicates that monocyte-derived cells directly influence muscle regeneration and repair processes in IIMs.
A deeper understanding of the multifaceted roles of monocytes and macrophages in IIM pathogenesis is paving the way for the development of more targeted therapeutic strategies. However, the current therapies targeting these cells in IIMs are not fully optimized. The development of treatments that directly address monocyte recruitment, modulate their activation, shift their polarization states, or address the underlying mechanisms of their aberrant activation (e.g., by addressing mitochondrial dysfunction or aberrant type I IFN signaling) are active and important areas of investigation.
Current standard of care treatments for IIM, including glucocorticoids, methotrexate, azathioprine, calcineurin inhibitors, cyclophosphamide and intravenous immunoglobulin (IVIg) (1, 89), likely exert some of their therapeutic effects by modulating monocyte and macrophage function, although the precise mechanisms are not fully elucidated. Glucocorticoids can directly and indirectly influence immune cell differentiation, exerting distinct effects on monocytes versus mature macrophages. Indeed, they alter a greater number of mRNAs in monocytes than in differentiated macrophages (90) and can inhibit proinflammatory mediators while enhancing anti-inflammatory factors, partly by stimulating the adenosine receptor A3 to promote a shift toward M2 macrophages (91, 92). In rheumatoid arthritis, glucocorticoids similarly foster an anti-inflammatory macrophage phenotype, suppress cytokine production, boost phagocytic capacity, and help protect joint structures—partly via interactions with fibroblast-like synoviocytes (93). These effects also facilitate clearance of apoptotic cells, often through stromal cell interactions.
Classical immunosuppressants, while acting on a broad spectrum of immune cells, also impact monocyte and macrophage function through diverse mechanisms. Methotrexate induces apoptosis in monocytes and promotes the transformation of M1 macrophages into M2 macrophages through adenosine signaling. The levels of adenosine increase in response to methotrexate administration, binding to adenosine receptors on monocytes (94). In bleomycin-induced pulmonary fibrosis mouse models, tacrolimus inhibits JAK2/STAT3 signaling, reducing profibrotic factor production by M2 macrophages and modulating their polarization, suggesting a significant anti-fibrotic effect through macrophage targeting (95). However, tacrolimus can also promote M2-like polarization in monocytes/macrophages from healthy volunteers and inhibit p38MAPK phosphorylation at higher concentrations (96), indicating a complex and potentially concentration-dependent effect on macrophage function. Azathioprine’s metabolites, 6-MP and 6-T-GTP, dampen macrophage-mediated inflammation. While this effect is largely Rac1-independent, Rac1-mediated suppression of iNOS also contributes (97). Cyclophosphamide reduces the production of pro-inflammatory cytokines IL-1 and TNF by monocytes, acting both directly on these cells and indirectly through effects on lymphocytes and the hematopoietic system (98). Moreover, IVIg, though its mechanisms of action are complex, inhibits IFN-γ signaling in macrophages by suppressing the Fc receptor FcγRIII (99). Despite the ability of current immunosuppressants to reduce inflammation and modulate macrophage activity, they are often limited by side effects and may not fully resolve monocyte/macrophage-driven pathology. This underscores the need for more targeted therapeutic strategies aimed at specifically addressing the role of these cells in disease.
Monocyte migration to inflamed tissues is a critical step in the amplification of inflammatory responses. Strategies designed to interfere with this process are of considerable interest. For instance, antibodies targeting the chemokine receptor CCR2, which is crucial for CCL2 (MCP-1)-mediated chemotaxis, have been explored as a therapeutic approach. While anti-CCR2 antibodies did not demonstrate substantial efficacy in rheumatoid arthritis trials (100), their potential in the context of IIMs, in which CCL2 is often elevated, deserves further consideration. Similarly, monoclonal antibodies targeting granulocyte-macrophage colony-stimulating factor, a key cytokine in monocyte and macrophage differentiation, are being explored for their ability to inhibit macrophage proliferation and activation (101). Although these antibodies are not currently under investigation specifically for IIM treatment, they are in clinical trials for treating other autoimmune conditions (e.g., rheumatoid arthritis, psoriasis, and multiple sclerosis) (101).
The colony-stimulating factor 1 receptor (CSF-1R) plays a crucial role in the survival, proliferation, differentiation, recruitment, and function of mononuclear phagocytes, including macrophages and monocytes (102). Therefore, monoclonal antibodies targeting CSF-1R are being actively investigated as well (103). A strategy to reduce monocyte and macrophage activation and recruitment to inflamed tissues involves targeting oxidative stress. In experimental autoimmune myositis models, increased ROS and decreased NF-E2-related factor 2 (Nrf2) levels are observed, and studies have shown that overexpressing Nrf2 in experimental autoimmune myositis macrophages inhibits their migration and reduces the levels of pro-inflammatory factors while increasing antioxidative stress enzymes (104). Hence, activating the Nrf2/antioxidant response element pathway can promote the degradation of ROS and reduce the expression of pro-inflammatory factors, thus potentially mitigating macrophage infiltration and inflammation in IIMs.
The inherent plasticity of macrophages can allow a shift in their activation from a pro-inflammatory M1-like phenotype toward a reparative M2-like state. This concept is appealing, and several approaches to achieve this shift are under evaluation (105–107). However, as noted earlier, M2-like macrophages have also been implicated in IIM pathogenesis, particularly in the context of fibrosis. Since M2-like macrophages may be recruited in response to inflammation to aid in its resolution (108), it is important to recognize that the M2 phenotype is not monolithic. Indeed, M2 macrophages are further classified into subsets with distinct functions, including pro-fibrotic, immunomodulatory and anti-inflammatory, and immunosuppressive and pro-fibrotic roles (67). A shift toward anti-inflammatory and immunosuppressive subtypes may have therapeutic potential in IIM.
Modulating signaling molecules such as peroxisome proliferator-activated receptors (PPARs), particularly PPAR-γ, holds promise. PPAR-γ expression is increased by IL-4 and is a key regulator of inflammation and lipid metabolism, promoting an alternatively activated macrophage phenotype (109, 110). Mesenchymal stem cell-derived exosomes represent another avenue for modulating macrophage polarization. These vesicles can transfer diverse molecules, including DNA, mRNA, and proteins, to recipient cells, including macrophages, to promote tissue repair and suppress inflammatory M1 cells (111, 112). Additionally, exosomes derived from other cell types, such as endothelial cells (e.g., containing miR-10a) and adipose tissue-derived stem cells (e.g., carrying active STAT3, which induces arginase-1 expression in macrophages), can also modulate macrophage phenotypes (113, 114). As such, understanding which exosomes promote a more reparative macrophage phenotype is important for exploring mesenchymal stem cell-derived exosome therapy for IIMs.
The complex interplay between dysregulated mitochondrial function and heightened IFN signatures, especially in conditions like DM and JDM (1, 77, 115–119), highlights opportunities for intervention. Given that type I IFN signaling utilizes JAK1 and tyrosine kinase 2 (TYK2) as signal transducers, JAK inhibitors—some of which are already approved for rheumatoid arthritis (120) —show potential by blocking IFN-α and IFN-γ signaling, particularly in monocytes, as well as in other immune cells (121). Additionally, they promote the generation of monocyte-derived macrophages with an anti-inflammatory transcriptional and functional profile (122), thereby reducing the production of pro-inflammatory cytokines and chemokines. Indeed, some reports have shown the efficacy of JAK inhibitors, particularly in managing anti-MDA5 antibody-positive DM (123–131). This may stem from the robust type I IFN signature observed in anti-MDA5 antibody-positive DM. Furthermore, anifrolumab, a human monoclonal antibody targeting the type I IFN receptor subunit 1, which has been approved for SLE (132), is another potential therapeutic option. In addition to its broader effects on IFN signaling, anifrolumab has been shown to attenuate the inflammatory response in models of myocardial ischemia/reperfusion injury by reducing monocyte/macrophage polarization toward the pro-inflammatory M1 phenotype and decreasing their phagocytic activity (133). In fact, case reports have shown rapid improvement of skin rashes in IIM patients treated with anifrolumab (134, 135). Likewise, dazukibart, a monoclonal antibody against IFN-β, also showed promising results in a phase 2 trial for adult DM, especially for skin manifestations (136).
While direct targeting of inflammatory cytokines, such as TNF-α (137–142) and IL-6 (143, 144) is a therapeutic strategy being explored for IIMs, the efficacy of these agents varies and, in some cases, may cause harm (145–149). TNF-α inhibitors regulate the polarization of inflammatory M1 macrophages while also enhancing phagocytosis (150). Meanwhile, IL-6 inhibitors reduce superoxide anion production by monocytes/macrophages and upregulate PPAR-γ expression in monocytes and monocyte-derived macrophages, promoting a shift toward an anti-inflammatory phenotype (151). Further research is needed to identify and validate specific therapeutic targets, optimize drug use, and establish efficacy in well-defined IIM patient populations based on molecular data (152).
Monocytes and macrophages drive tissue inflammation, damage, and fibrosis in IIMs via complex interactions with innate and adaptive immunity (Figure 1). Their infiltration patterns differ according to subtype, reflecting distinct immunopathological pathways. Mitochondrial dysfunction and metabolic shifts regulate monocyte activation and cytokine production, complicating the traditional M1/M2 framework. In addition to therapies targeting various molecules and emerging technologies (153, 154), strategies are being developed to inhibit macrophage activation, shift them toward anti-inflammatory phenotypes, block key receptors (e.g., CSF-1R), or target their released cytokines.
Figure 1. Involvement of monocytes and macrophages in IIMs. Monocytes from the bone marrow circulate in the blood (classical, intermediate, non-classical) and are recruited to affected tissues, such as muscle, skin, and lung, by elevated chemokines and adhesion molecules. In tissues, monocytes differentiate into pro-inflammatory (M1-like) macrophages or tissue-repairing (M2-like) macrophages under the influence of cytokines such as IFN-γ, IL-4, and IL-10. In the muscle, these macrophages contribute to phagocytosis and tissue damage, while in the lung, they are associated with interstitial lung disease (ILD), marked by elevated levels of ferritin and other biomarkers. Type I IFN responses are observed in affected organs. Potential therapeutic targets to address monocyte and macrophage involvement in IIMs include anti-CCR2, anti-GM-CSF, anti-CSF-1R, Nrf2 activators, PPAR-γ agonists, mesenchymal stem cell-derived exosomes (MSC-Exos), JAK inhibitors, anifrolumab, and dazukibart.
Further studies are needed to clarify how autoantibody profiles and clinical phenotypes shape monocyte/macrophage phenotypes and clinical outcomes. Moreover, a recent large-scale retrospective cohort study revealed a higher risk of ischemic heart disease in PM and DM (hazard ratio: 1.61 [1.15–2.25]) (155). Given that one cause of atherosclerosis is the accumulation of cholesterol-laden macrophages in the arterial wall and that atherosclerotic plaques also contain monocytes (156), the increased risk of heart disease in IIM may be explained by another pathogenic role of monocytes and macrophages. The role of tissue-repairing macrophages in IIM remains poorly understood (86), and local tissue factors like hypoxia and muscle-derived signals also impact macrophage polarization. Advanced profiling methods (scRNA-seq, spatial transcriptomics) are vital for uncovering disease-specific subsets (157). Therefore, a personalized approach targeting dysregulated pathways of monocyte/macrophage biology in IIMs holds the potential to transform patient care, enabling mechanism-based therapies that balance inflammation, tissue repair, and fibrosis more effectively.
SI: Conceptualization, Investigation, Writing – original draft, Writing – review & editing. TK: Conceptualization, Writing – original draft, Writing – review & editing. YT: Conceptualization, Writing – review & editing. HT: Conceptualization, Writing – review & editing. TO: Conceptualization, Funding acquisition, Supervision, Writing – review & editing. KF: Conceptualization, Funding acquisition, Supervision, Writing – review & editing.
The author(s) declare that financial support was received for the research and/or publication of this article. This work was supported by a Grant-in-Aid for JSPS Research Fellow (JSPS KAKENHI Grant Numbers 22J20047 and 22KJ0995 to SI), The Center of Innovation Program from the Japan Science and Technology Agency (JPMJCE1304 to KF), The Ministry of Education, Culture, Sports, and the Japan Agency for Medical Research and Development (JP21tm0424221 to KF, JP21zf0127004 to KF, JP22ek0410074 to KF, JP223fa627001 to KF, JP233fa627001 to KF, and JP23gm1810005 to KF and TO), JSPS Grant-in-Aid for Scientific Research (B) (JP19H03697 and JP22H03110 to TO), Grant-in-Aid for Early-Career Scientists (22K16355 to TK). The authors declare that this study received funding from Chugai Pharmaceutical Co., Ltd. The funder was not involved in the study design, collection, analysis, interpretation of data, the writing of this article, or the decision to submit it for publication.
All the figures were created with BioRender.com.
TO belonged to the Social Cooperation Program of the Department of Functional Genomics and Immunological Diseases, supported by Chugai Pharmaceutical. TK has received speaking fees and/or honoraria from Tanabe Mitsubishi, Kissei, Pfizer, Chugai, Daiichi-Sankyo, Eli Lilly, Novartis, Eisai, Asahi Kasei, AbbVie, and Janssen and received grants and speaking fees from GlaxoSmithKline. YT has received speaking fees and/or honoraria from AbbVie, Janssen, GlaxoSmithKline, Astellas Pharma Inc., Daiichi Sankyo, Eli Lilly, Novartis, Eisai, Asahi Kasei Corporation, and AstraZeneca. HT has received speaking fees and/or honoraria from AbbVie, Amgen, Asahi Kasei, Astellas, Bristol-Myers Squibb, Chugai, Daiichi-Sankyo, Eisai, Eli Lilly, Gilead, Jansen, Novartis, Sanofi, Tanabe Mitsubishi, and UCB, as well as research grants from AbbVie, Mochida, and Takeda. KF received grants, consulting fees, speaking fees, and/or honoraria from Chugai Pharmaceutical Co., Ltd., Asahi Kasei Pharma, AstraZeneca, Tsumura & Co., AbbVie, Bristol Myers Squibb, Eisai, Taisho Pharmaceutical, Eli Lilly, Daiichi Sankyo, GlaxoSmithKline, Mitsubishi Tanabe Pharma, Gilead Sciences, Pfizer Inc., Astellas Pharma Inc., Novartis, and Alexion Pharmaceuticals, Inc.
The remaining authors declare that the research was conducted in the absence of any commercial or financial relationships that could be construed as a potential conflict of interest.
The author(s) declare that no Generative AI was used in the creation of this manuscript.
All claims expressed in this article are solely those of the authors and do not necessarily represent those of their affiliated organizations, or those of the publisher, the editors and the reviewers. Any product that may be evaluated in this article, or claim that may be made by its manufacturer, is not guaranteed or endorsed by the publisher.
The Supplementary Material for this article can be found online at: https://www.frontiersin.org/articles/10.3389/fimmu.2025.1567833/full#supplementary-material
Supplementary Figure 1 | Macrophage infiltration patterns in muscle across selected subtypes of IIM. Macrophage infiltration in the perimysium and endomysium among different dermatomyositis subtypes. TIF1-γ- and Mi-2-positive patients demonstrate the higher endomysial infiltration, whereas MDA5-positive patients show comparatively lower macrophage infiltration in both perimysial and endomysial areas. IIM, idiopathic inflammatory myopathies; MDA5, melanoma differentiation-associated gene 5; TIF1-γ, transcription intermediary factor 1-γ.
1. Lundberg IE, Fujimoto M, Vencovsky J, Aggarwal R, Holmqvist M, Christopher-Stine L, et al. Idiopathic inflammatory myopathies. Nat Rev Dis Primers. (2021) 7:86. doi: 10.1038/s41572-021-00321-x
2. Izuka S, Komai T, Shoda H, Fujio K. Long-term risks of Malignancy in myositis-specific antibody-positive idiopathic inflammatory myopathy. Rheumatol Int. (2023) 43:335–43. doi: 10.1007/s00296-022-05214-0
3. Ushijima TS, Komai T, Izuka S, Shoda H, Fujio K. Characteristics of anti-melanoma differentiation associated gene 5 antibody-positive dermatomyositis with thrombotic microangiopathy. Mod Rheumatol. (2024) 34(5):973–7. doi: 10.1093/mr/roae009
4. Wu Y, Deshpande A, Geraci N, Budde P, Sellers V, Velisetty P, et al. TLR7/8 activation in immune cells and muscle by RNA-containing immune complexes: Role in inflammation and the pathogenesis of myositis. Arthritis Rheumatol. (2025) 77(2):190–201. doi: 10.1002/art.42989
5. Fukada A, Fujisawa T, Hozumi H, Koda K, Akamatsu T, Oyama Y, et al. Prognostic role of interferon-λ3 in anti-melanoma differentiation-associated gene 5-positive dermatomyositis-associated interstitial lung disease. Arthritis Rheumatol. (2024) 76:796–805. doi: 10.1002/art.42785
6. Izuka S, Umezawa N, Komai T, Sugimori Y, Kimura N, Mizoguchi F, et al. Muscle tissue transcriptome of idiopathic inflammatory myopathy reflects the muscle damage process by monocytes and presence of skin lesions. Arthritis Rheumatol. (2025) 77:99–106. doi: 10.1002/art.42972
7. Gono T, Okazaki Y, Kuwana M. Antiviral proinflammatory phenotype of monocytes in anti-MDA5 antibody-associated interstitial lung disease. Rheumatol (Oxford). (2022) 61:806–14. doi: 10.1093/rheumatology/keab371
8. Kania G, Rudnik M, Distler O. Involvement of the myeloid cell compartment in fibrogenesis and systemic sclerosis. Nat Rev Rheumatol. (2019) 15:288–302. doi: 10.1038/s41584-019-0212-z
9. Netea MG, Domínguez-Andrés J, Barreiro LB, Chavakis T, Divangahi M, Fuchs E, et al. Defining trained immunity and its role in health and disease. Nat Rev Immunol. (2020) 20:375–88. doi: 10.1038/s41577-020-0285-6
10. Ziegler-Heitbrock L, Ancuta P, Crowe S, Dalod M, Grau V, Hart DN, et al. Nomenclature of monocytes and dendritic cells in blood. Blood. (2010) 116:e74–80. doi: 10.1182/blood-2010-02-258558
11. Villani A-C, Satija R, Reynolds G, Sarkizova S, Shekhar K, Fletcher J, et al. Single-cell RNA-seq reveals new types of human blood dendritic cells, monocytes, and progenitors. Science. (2017) 356. doi: 10.1126/science.aah4573
12. Zhang B, Moorlag SJ, Dominguez-Andres J, Bulut Ö, Kilic G, Liu Z, et al. Single-cell RNA sequencing reveals induction of distinct trained-immunity programs in human monocytes. J Clin Invest. (2022) 132. doi: 10.1172/JCI147719
13. Guilliams M, Ginhoux F, Jakubzick C, Naik SH, Onai N, Schraml BU, et al. Dendritic cells, monocytes and macrophages: a unified nomenclature based on ontogeny. Nat Rev Immunol. (2014) 14:571–8. doi: 10.1038/nri3712
14. Rostasy KM, Piepkorn M, Goebel H-H, Menck S, Hanefeld F, Schulz-Schaeffer WJ. Monocyte/macrophage differentiation in dermatomyositis and polymyositis. Muscle Nerve. (2004) 30:225–30. doi: 10.1002/mus.20088
15. Chen S, Saeed AFUH, Liu Q, Jiang Q, Xu H, Xiao GG, et al. Macrophages in immunoregulation and therapeutics. Signal Transduct Target Ther. (2023) 8:207. doi: 10.1038/s41392-023-01452-1
16. Wynn TA, Vannella KM. Macrophages in tissue repair, regeneration, and fibrosis. Immunity. (2016) 44:450–62. doi: 10.1016/j.immuni.2016.02.015
17. Rigamonti E, Zordan P, Sciorati C, Rovere-Querini P, Brunelli S. Macrophage plasticity in skeletal muscle repair. BioMed Res Int. (2014) 2014:560629. doi: 10.1155/2014/560629
18. Sugimori Y, Iwasaki Y, Takeshima Y, Okubo M, Kobayashi S, Hatano H, et al. Transcriptome profiling of immune cell types in peripheral blood reveals common and specific pathways involved in the pathogenesis of myositis-specific antibody-positive inflammatory myopathies. ACR Open Rheumatol. (2023) 5:93–102. doi: 10.1002/acr2.11521
19. Wong KL, Tai JJ-Y, Wong W-C, Han H, Sem X, Yeap W-H, et al. Gene expression profiling reveals the defining features of the classical, intermediate, and nonclassical human monocyte subsets. Blood. (2011) 118:e16–31. doi: 10.1182/blood-2010-12-326355
20. Lira-Junior R, Holmström SB, Clark R, Zwicker S, Majster M, Johannsen G, et al. S100A12 expression is modulated during monocyte differentiation and reflects periodontitis severity. Front Immunol. (2020) 11:86. doi: 10.3389/fimmu.2020.00086
21. Wilfong EM, Bartkowiak T, Vowell KN, Westlake CS, Irish JM, Kendall PL, et al. High-dimensional analysis reveals distinct endotypes in patients with idiopathic inflammatory myopathies. Front Immunol. (2022) 13:756018. doi: 10.3389/fimmu.2022.756018
22. Newman AM, Steen CB, Liu CL, Gentles AJ, Chaudhuri AA, Scherer F, et al. Determining cell type abundance and expression from bulk tissues with digital cytometry. Nat Biotechnol. (2019) 37:773–82. doi: 10.1038/s41587-019-0114-2
23. Li M, Zhang Y, Zhang W, Sun J, Liu R, Pan Z, et al. Type 1 interferon signature in peripheral blood mononuclear cells and monocytes of idiopathic inflammatory myopathy patients with different myositis-specific autoantibodies. Front Immunol. (2023) 14:1169057. doi: 10.3389/fimmu.2023.1169057
24. He J, Liu Z, Cao Y, Zhang X, Yi C, Zhou Y, et al. Single-cell landscape of peripheral immune response in patients with anti-melanoma differentiation-associated gene 5 dermatomyositis. Rheumatol (Oxford). (2024) 63(8):2284–94. doi: 10.1093/rheumatology/kead597
25. Confalonieri P, Bernasconi P, Megna P, Galbiati S, Cornelio F, Mantegazza R. Increased expression of beta-chemokines in muscle of patients with inflammatory myopathies. J Neuropathol Exp Neurol. (2000) 59:164–9. doi: 10.1093/jnen/59.2.164
26. De Bleecker JL, De Paepe B, Vanwalleghem IE, Schröder JM. Differential expression of chemokines in inflammatory myopathies. Neurology. (2002) 58:1779–85. doi: 10.1212/WNL.58.12.1779
27. Raju R, Vasconcelos O, Granger R, Dalakas MC. Expression of IFN-gamma-inducible chemokines in inclusion body myositis. J Neuroimmunol. (2003) 141:125–31. doi: 10.1016/S0165-5728(03)00218-2
28. Szodoray P, Alex P, Knowlton N, Centola M, Dozmorov I, Csipo I, et al. Idiopathic inflammatory myopathies, signified by distinctive peripheral cytokines, chemokines and the TNF family members B-cell activating factor and a proliferation inducing ligand. Rheumatol (Oxford). (2010) 49:1867–77. doi: 10.1093/rheumatology/keq151
29. Civatte M, Bartoli C, Schleinitz N, Chetaille B, Pellissier JF, Figarella-Branger D. Expression of the beta chemokines CCL3, CCL4, CCL5 and their receptors in idiopathic inflammatory myopathies. Neuropathol Appl Neurobiol. (2005) 31:70–9. doi: 10.1111/j.1365-2990.2004.00591.x
30. Allenbach Y, Chaara W, Rosenzwajg M, Six A, Prevel N, Mingozzi F, et al. Th1 response and systemic treg deficiency in inclusion body myositis. PloS One. (2014) 9:e88788. doi: 10.1371/journal.pone.0088788
31. Mageriu V, Manole E, Bastian AE, Staniceanu F. Role of myokines in myositis pathogenesis and their potential to be new therapeutic targets in idiopathic inflammatory myopathies. J Immunol Res. (2020) 2020:9079083. doi: 10.1155/2020/9079083
32. Zhao Q, Chen Y, Diao L, Zhang S, Wu D, Xue F, et al. Identification of distinct cytokine/chemokine profiles in dermatomyositis with anti-transcriptional intermediary factor 1-γ antibody. Rheumatol (Oxford). (2022) 61:2176–84. doi: 10.1093/rheumatology/keab625
33. Loaiza-Félix J, Moreno-Ramírez M, Pérez-García FL, Jiménez-Rojas V, Sánchez-Muñoz F, Amezcua-Guerra ML. Serum levels of adipokines in patients with idiopathic inflammatory myopathies: a pilot study. Rheumatol Int. (2017) 37:1341–5. doi: 10.1007/s00296-017-3752-z
34. Shimizu T, Koga T, Furukawa K, Horai Y, Fujikawa K, Okada A, et al. IL-15 is a biomarker involved in the development of rapidly progressive interstitial lung disease complicated with polymyositis/dermatomyositis. J Intern Med. (2021) 289:206–20. doi: 10.1111/joim.v289.2
35. Chen M, Quan C, Diao L, Xue F, Xue K, Wang B, et al. Measurement of cytokines and chemokines and association with clinical severity of dermatomyositis and clinically amyopathic dermatomyositis. Br J Dermatol. (2018) 179:1334–41. doi: 10.1111/bjd.2018.179.issue-6
36. Kokuzawa A, Nakamura J, Kamata Y, Sato K. Potential role of type I interferon/IP-10 axis in the pathogenesis of anti-MDA5 antibody-positive dermatomyositis. Clin Exp Rheumatol. (2023) 41:275–84. doi: 10.55563/clinexprheumatol/em67zx
37. Lin M, Yang C, Liu X, Zhao S, Tian B, Hou X, et al. Increased levels of VCAM-1 in Sera and VLA-4 expression on neutrophils in dermatomyositis with interstitial lung disease. Immunol Invest. (2022) 51:980–92. doi: 10.1080/08820139.2021.1897611
38. Yasin SA, Schutz PW, Deakin CT, Sag E, Varsani H, Simou S, et al. Histological heterogeneity in a large clinical cohort of juvenile idiopathic inflammatory myopathy: analysis by myositis autoantibody and pathological features. Neuropathol Appl Neurobiol. (2019) 45:495–512. doi: 10.1111/nan.2019.45.issue-5
39. Fornaro M, Girolamo F, Cavagna L, Franceschini F, Giannini M, Amati A, et al. Severe muscle damage with myofiber necrosis and macrophage infiltrates characterize anti-Mi2 positive dermatomyositis. Rheumatology. (2021) 60:2916–26. doi: 10.1093/rheumatology/keaa739
40. Tanboon J, Inoue M, Saito Y, Tachimori H, Hayashi S, Noguchi S, et al. Dermatomyositis: Muscle pathology according to antibody subtypes. Neurology. (2022) 98:e739–49. doi: 10.1212/WNL.0000000000013176
41. Da Silva LM, Borges IB, Shinjo SK. High prevalence of necrotising myopathy pattern in muscle biopsies of patients with anti-Jo-1 antisynthetase syndrome. Clin Exp Rheumatol. (2023) 41(2):238–46. doi: 10.55563/clinexprheumatol/zq7x68
42. Allenbach Y, Arouche-Delaperche L, Preusse C, Radbruch H, Butler-Browne G, Champtiaux N, et al. Necrosis in anti-SRP+ and anti-HMGCR+myopathies: Role of autoantibodies and complement. Neurology. (2018) 90:e507–17. doi: 10.1212/WNL.0000000000004923
43. Allenbach Y, Benveniste O, Stenzel W, Boyer O. Immune-mediated necrotizing myopathy: clinical features and pathogenesis. Nat Rev Rheumatol. (2020) 16:689–701. doi: 10.1038/s41584-020-00515-9
44. Tang Q, Gheorghe KR, Zhang X-M, Lindroos E, Alexanderson H, Wick C, et al. Features of repeated muscle biopsies and phenotypes of monocytes in paired blood samples and clinical long-term response to treatment in patients with idiopathic inflammatory myopathy: a pilot study. Clin Exp Rheumatol. (2020) 38:42–9.
45. Mehta P, Aggarwal R, Porter JC, Gunawardena H. Management of interstitial lung disease (ILD) in myositis syndromes: A practical guide for clinicians. Best Pract Res Clin Rheumatol. (2022) 36:101769. doi: 10.1016/j.berh.2022.101769
46. Shappley C, Paik JJ, Saketkoo LA. Myositis-related interstitial lung diseases: Diagnostic features, treatment, and complications. Curr Treatm Opt Rheumatol. (2019) 5:56–83. doi: 10.1007/s40674-018-0110-6
47. Enomoto Y, Suzuki Y, Hozumi H, Mori K, Kono M, Karayama M, et al. Clinical significance of soluble CD163 in polymyositis-related or dermatomyositis-related interstitial lung disease. Arthritis Res Ther. (2017) 19:9. doi: 10.1186/s13075-016-1214-8
48. Peng Q-L, Zhang Y-L, Shu X-M, Yang H-B, Zhang L, Chen F, et al. Elevated serum levels of soluble CD163 in polymyositis and dermatomyositis: Associated with macrophage infiltration in muscle tissue. J Rheumatol. (2015) 42:979–87. doi: 10.3899/jrheum.141307
49. Hirano A, Sakashita A, Fujii W, Baßler K, Tsuji T, Kadoya M, et al. Immunological characteristics of bronchoalveolar lavage fluid and blood across connective tissue disease-associated interstitial lung diseases. Front Immunol. (2024) 15:1408880. doi: 10.3389/fimmu.2024.1408880
50. Gono T, Kawaguchi Y, Satoh T, Kuwana M, Katsumata Y, Takagi K, et al. Clinical manifestation and prognostic factor in anti-melanoma differentiation-associated gene 5 antibody-associated interstitial lung disease as a complication of dermatomyositis. Rheumatol (Oxford). (2010) 49:1713–9. doi: 10.1093/rheumatology/keq149
51. Gono T, Sato S, Kawaguchi Y, Kuwana M, Hanaoka M, Katsumata Y, et al. Anti-MDA5 antibody, ferritin and IL-18 are useful for the evaluation of response to treatment in interstitial lung disease with anti-MDA5 antibody-positive dermatomyositis. Rheumatol (Oxford). (2012) 51:1563–70. doi: 10.1093/rheumatology/kes102
52. So J, So H, Wong VT-L, Ho R, Wu TY, Wong PC-H, et al. Predictors of rapidly progressive interstitial lung disease and mortality in patients with autoantibodies against melanoma differentiation-associated protein 5 dermatomyositis. Rheumatol (Oxford). (2022) 61:4437–44. doi: 10.1093/rheumatology/keac094
53. Lu X, Peng Q, Wang G. Anti-MDA5 antibody-positive dermatomyositis: pathogenesis and clinical progress. Nat Rev Rheumatol. (2024) 20:48–62. doi: 10.1038/s41584-023-01054-9
54. Shi J, Pei X, Peng J, Wu C, Lv Y, Wang X, et al. Monocyte-macrophage dynamics as key in disparate lung and peripheral immune responses in severe anti-melanoma differentiation-associated gene 5-positive dermatomyositis-related interstitial lung disease. Clin Transl Med. (2025) 15:e70226. doi: 10.1002/ctm2.70226
55. Cohen LA, Gutierrez L, Weiss A, Leichtmann-Bardoogo Y, Zhang D-L, Crooks DR, et al. Serum ferritin is derived primarily from macrophages through a nonclassical secretory pathway. Blood. (2010) 116:1574–84. doi: 10.1182/blood-2009-11-253815
56. Ganz T, Nemeth E. Iron homeostasis in host defence and inflammation. Nat Rev Immunol. (2015) 15:500–10. doi: 10.1038/nri3863
57. Ruscitti P, Di Benedetto P, Berardicurti O, Panzera N, Grazia N, Lizzi AR, et al. Pro-inflammatory properties of H-ferritin on human macrophages, ex vivo and in vitro observations. Sci Rep. (2020) 10:12232. doi: 10.1038/s41598-020-69031-w
58. Hussell T, Bell TJ. Alveolar macrophages: plasticity in a tissue-specific context. Nat Rev Immunol. (2014) 14:81–93. doi: 10.1038/nri3600
59. Li Y, Liu X, Tian M, Zou R, Gao Y, Huang M, et al. Soluble CD206 levels correlate with disease deterioration and predict prognosis of anti-MDA5 antibody-positive dermatomyositis related interstitial lung disease. Clin Respir J. (2023) 17:507–15. doi: 10.1111/crj.13616
60. Shen Y-W, Zhang Y-M, Huang Z-G, Wang G-C, Peng Q-L. Increased levels of soluble CD206 associated with rapidly progressive interstitial lung disease in patients with dermatomyositis. Mediators Inflammation. (2020) 2020:7948095. doi: 10.1155/2020/7948095
61. Horiike Y, Suzuki Y, Fujisawa T, Yasui H, Karayama M, Hozumi H, et al. Successful classification of macrophage-mannose receptor CD206 in severity of anti-MDA5 antibody positive dermatomyositis associated ILD. Rheumatol (Oxford). (2019) 58:2143–52. doi: 10.1093/rheumatology/kez185
62. Pommerolle L, Beltramo G, Biziorek L, Truchi M, Dias AMM, Dondaine L, et al. CD206+ macrophages are relevant non-invasive imaging biomarkers and therapeutic targets in experimental lung fibrosis. Thorax. (2024) 79:1124–35. doi: 10.1136/thorax-2023-221168
63. Peng Q-L, Zhang Y-M, Liang L, Liu X, Ye L-F, Yang H-B, et al. A high level of serum neopterin is associated with rapidly progressive interstitial lung disease and reduced survival in dermatomyositis. Clin Exp Immunol. (2020) 199:314–25. doi: 10.1111/cei.13404
64. Jiang L, Wang Y, Peng Q, Shu X, Wang G, Wu X. Serum YKL-40 level is associated with severity of interstitial lung disease and poor prognosis in dermatomyositis with anti-MDA5 antibody. Clin Rheumatol. (2019) 38:1655–63. doi: 10.1007/s10067-019-04457-w
65. Kulakova K, Lawal TR, Mccarthy E, Floudas A. The contribution of macrophage plasticity to inflammatory arthritis and their potential as therapeutic targets. Cells. (2024) 13:1586. doi: 10.3390/cells13181586
66. Mitsi E, Kamng’ona R, Rylance J, Solórzano C, Jesus Reiné J, Mwandumba HC, et al. Human alveolar macrophages predominately express combined classical M1 and M2 surface markers in steady state. Respir Res. (2018) 19:66. doi: 10.1186/s12931-018-0777-0
67. Jiang Y, Cai R, Huang Y, Zhu L, Xiao L, Wang C, et al. Macrophages in organ fibrosis: from pathogenesis to therapeutic targets. Cell Death Discovery. (2024) 10:487. doi: 10.1038/s41420-024-02247-1
68. Caproni M, Torchia D, Cardinali C, Volpi W, Del Bianco E, D’Agata A, et al. Infiltrating cells, related cytokines and chemokine receptors in lesional skin of patients with dermatomyositis. Br J Dermatol. (2004) 151:784–91. doi: 10.1111/j.1365-2133.2004.06144.x
69. Wenzel J, Schmidt R, Proelss J, Zahn S, Bieber T, Tüting T. Type I interferon-associated skin recruitment of CXCR3+ lymphocytes in dermatomyositis. Clin Exp Dermatol. (2006) 31:576–82. doi: 10.1111/j.1365-2230.2006.02150.x
70. Pinal-Fernandez I, Casal-Dominguez M, Derfoul A, Pak K, Plotz P, Miller FW, et al. Identification of distinctive interferon gene signatures in different types of myositis. Neurology. (2019) 93:e1193–204. doi: 10.1212/WNL.0000000000008128
71. Patel J, Ravishankar A, Maddukuri S, Vazquez T, Grinnell M, Werth VP. Identification of similarities between skin lesions in patients with antisynthetase syndrome and skin lesions in patients with dermatomyositis by highly multiplexed imaging mass cytometry. Arthritis Rheumatol. (2022) 74:882–91. doi: 10.1002/art.42050
72. Yang R, Sun L, Li C-F, Wang Y-H, Yao J, Li H, et al. Galectin-9 interacts with PD-1 and TIM-3 to regulate T cell death and is a target for cancer immunotherapy. Nat Commun. (2021) 12:832. doi: 10.1038/s41467-021-21099-2
73. Bellutti Enders F, van Wijk F, Scholman R, Hofer M, Prakken BJ, van-Royen-Kerkhof A, et al. Correlation of CXCL10, tumor necrosis factor receptor type II, and galectin 9 with disease activity in juvenile dermatomyositis. Arthritis Rheumatol. (2014) 66:2281–9. doi: 10.1002/art.38676
74. Wienke J, Bellutti Enders F, Lim J, Mertens JS, van den Hoogen LL, Wijngaarde CA, et al. Galectin-9 and CXCL10 as biomarkers for disease activity in juvenile dermatomyositis: A longitudinal cohort study and multicohort validation. Arthritis Rheumatol. (2019) 71:1377–90. doi: 10.1002/art.40881
75. Liang L, Zhang Y-M, Shen Y-W, Song A-P, Li W-L, Ye L-F, et al. Aberrantly expressed galectin-9 is involved in the immunopathogenesis of anti-MDA5-positive dermatomyositis-associated interstitial lung disease. Front Cell Dev Biol. (2021) 9:628128. doi: 10.3389/fcell.2021.628128
76. Lv R, Bao Q, Li Y. Regulation of M1−type and M2−type macrophage polarization in RAW264.7 cells by Galectin−9. Mol Med Rep. (2017) 16:9111–9. doi: 10.3892/mmr.2017.7719
77. Wilkinson MGL, Moulding D, McDonnell TCR, Orford M, Wincup C, Ting JYJ, et al. Role of CD14+ monocyte-derived oxidised mitochondrial DNA in the inflammatory interferon type 1 signature in juvenile dermatomyositis. Ann Rheum Dis. (2023) 82:658–69. doi: 10.1136/ard-2022-223469
78. Wang N, Liang H, Zen K. Molecular mechanisms that influence the macrophage M1—M2 polarization balance. Front Immunol. (2014) 5:614. doi: 10.3389/fimmu.2014.00614
79. Hu X, Chakravarty SD, Ivashkiv LB. Regulation of interferon and Toll-like receptor signaling during macrophage activation by opposing feedforward and feedback inhibition mechanisms. Immunol Rev. (2008) 226:41–56. doi: 10.1111/j.1600-065X.2008.00707.x
80. Walsh MC, Lee J, Choi Y. Tumor necrosis factor receptor- associated factor 6 (TRAF6) regulation of development, function, and homeostasis of the immune system. Immunol Rev. (2015) 266:72–92. doi: 10.1111/imr.2015.266.issue-1
81. Klein K, He K, Younes AI, Barsoumian HB, Chen D, Ozgen T, et al. Role of mitochondria in cancer immune evasion and potential therapeutic approaches. Front Immunol. (2020) 11:573326. doi: 10.3389/fimmu.2020.573326
82. Dalakas MC. Inflammatory muscle diseases. N Engl J Med. (2015) 372:1734–47. doi: 10.1056/NEJMra1402225
83. Nakano M, Fujii T, Hashimoto M, Yukawa N, Yoshifuji H, Ohmura K, et al. Type I interferon induces CX3CL1 (fractalkine) and CCL5 (RANTES) production in human pulmonary vascular endothelial cells. Clin Exp Immunol. (2012) 170:94–100. doi: 10.1111/j.1365-2249.2012.04638.x
84. Takada T, Aoki A, Asakawa K, Sakagami T, Moriyama H, Narita I, et al. Serum cytokine profiles of patients with interstitial lung disease associated with anti-CADM-140/MDA5 antibody positive amyopathic dermatomyositis. Respir Med. (2015) 109:1174–80. doi: 10.1016/j.rmed.2015.07.004
85. Ishida Y, Kimura A, Nosaka M, Kuninaka Y, Hemmi H, Sasaki I, et al. Essential involvement of the CX3CL1-CX3CR1 axis in bleomycin-induced pulmonary fibrosis via regulation of fibrocyte and M2 macrophage migration. Sci Rep. (2017) 7. doi: 10.1038/s41598-017-17007-8
86. Li Z, Liu H, Xie Q, Yin G. Macrophage involvement in idiopathic inflammatory myopathy: pathogenic mechanisms and therapeutic prospects. J Inflammation (Lond). (2024) 21:48. doi: 10.1186/s12950-024-00422-w
87. Dalakas MC. Pathogenesis and therapies of immune-mediated myopathies. Autoimmun Rev. (2012) 11:203–6. doi: 10.1016/j.autrev.2011.05.013
88. Ladislau L, Portilho DM, Courau T, Solares-Pérez A, Negroni E, Lainé J, et al. Activated dendritic cells modulate proliferation and differentiation of human myoblasts. Cell Death Dis. (2018) 9:551. doi: 10.1038/s41419-018-0426-z
89. Oddis CV, Aggarwal R. Treatment in myositis. Nat Rev Rheumatol. (2018) 14:279–89. doi: 10.1038/nrrheum.2018.42
90. Wang C, Nanni L, Novakovic B, Megchelenbrink W, Kuznetsova T, Stunnenberg HG, et al. Extensive epigenomic integration of the glucocorticoid response in primary human monocytes and in vitro derived macrophages. Sci Rep. (2019) 9:2772. doi: 10.1038/s41598-019-39395-9
91. Hardy RS, Raza K, Cooper MS. Therapeutic glucocorticoids: mechanisms of actions in rheumatic diseases. Nat Rev Rheumatol. (2020) 16:133–44. doi: 10.1038/s41584-020-0371-y
92. Barczyk K, Ehrchen J, Tenbrock K, Ahlmann M, Kneidl J, Viemann D, et al. Glucocorticoids promote survival of anti-inflammatory macrophages via stimulation of adenosine receptor A3. Blood. (2010) 116:446–55. doi: 10.1182/blood-2009-10-247106
93. Eiers A-K, Vettorazzi S, Tuckermann JP. Journey through discovery of 75 years glucocorticoids: evolution of our knowledge of glucocorticoid receptor mechanisms in rheumatic diseases. Ann Rheum Dis. (2024) 83:1603–13. doi: 10.1136/ard-2023-225371
94. Cronstein BN, Aune TM. Methotrexate and its mechanisms of action in inflammatory arthritis. Nat Rev Rheumatol. (2020) 16:145–54. doi: 10.1038/s41584-020-0373-9
95. Liu B, Jiang Q, Chen R, Gao S, Xia Q, Zhu J, et al. Tacrolimus ameliorates bleomycin-induced pulmonary fibrosis by inhibiting M2 macrophage polarization via JAK2/STAT3 signaling. Int Immunopharmacol. (2022) 113:109424. doi: 10.1016/j.intimp.2022.109424
96. Kannegieter NM, Hesselink DA, Dieterich M, Kraaijeveld R, Rowshani AT, Leenen PJM, et al. The effect of tacrolimus and mycophenolic acid on CD14+ monocyte activation and function. PloS One. (2017) 12:e0170806. doi: 10.1371/journal.pone.0170806
97. Marinković G, Hamers AAJ, de Vries CJM, de Waard V. 6-mercaptopurine reduces macrophage activation and gut epithelium proliferation through inhibition of GTPase Rac1. Inflammation Bowel Dis. (2014) 20:1487–95. doi: 10.1097/MIB.0000000000000122
98. McBride WH, Hoon DB, Jung T, Naungayan J, Nizze A, Morton DL. Cyclophosphamide-induced alterations in human monocyte functions. J Leukoc Biol. (1987) 42:659–66. doi: 10.1002/jlb.42.6.659
99. Park-Min K-H, Serbina NV, Yang W, Ma X, Krystal G, Neel BG, et al. FcgammaRIII-dependent inhibition of interferon-gamma responses mediates suppressive effects of intravenous immune globulin. Immunity. (2007) 26:67–78. doi: 10.1016/j.immuni.2006.11.010
100. Vergunst CE, Gerlag DM, Lopatinskaya L, Klareskog L, Smith MD, van den Bosch F, et al. Modulation of CCR2 in rheumatoid arthritis: a double-blind, randomized, placebo-controlled clinical trial. Arthritis Rheum. (2008) 58:1931–9. doi: 10.1002/art.23591
101. Lotfi N, Thome R, Rezaei N, Zhang G-X, Rezaei A, Rostami A, et al. Roles of GM-CSF in the pathogenesis of autoimmune diseases: An update. Front Immunol. (2019) 10:1265. doi: 10.3389/fimmu.2019.01265
102. Hume DA, MacDonald KPA. Therapeutic applications of macrophage colony-stimulating factor-1 (CSF-1) and antagonists of CSF-1 receptor (CSF-1R) signaling. Blood. (2012) 119:1810–20. doi: 10.1182/blood-2011-09-379214
103. Peyraud F, Cousin S, Italiano A. CSF-1R inhibitor development: Current clinical status. Curr Oncol Rep. (2017) 19:70. doi: 10.1007/s11912-017-0634-1
104. Liu Y, Gao Y, Yang J, Shi C, Wang Y, Xu Y. Nrf2/ARE pathway inhibits inflammatory infiltration by macrophage in rats with autoimmune myositis. Mol Immunol. (2019) 105:165–72. doi: 10.1016/j.molimm.2018.11.014
105. Mantovani A, Sica A. Macrophages, innate immunity and cancer: balance, tolerance, and diversity. Curr Opin Immunol. (2010) 22:231–7. doi: 10.1016/j.coi.2010.01.009
106. Duluc D, Corvaisier M, Blanchard S, Catala L, Descamps P, Gamelin E, et al. Interferon-gamma reverses the immunosuppressive and protumoral properties and prevents the generation of human tumor-associated macrophages. Int J Cancer. (2009) 125:367–73. doi: 10.1002/ijc.v125:2
107. Beatty GL, Chiorean EG, Fishman MP, Saboury B, Teitelbaum UR, Sun W, et al. CD40 agonists alter tumor stroma and show efficacy against pancreatic carcinoma in mice and humans. Science. (2011) 331:1612–6. doi: 10.1126/science.1198443
108. Funes SC, Rios M, Escobar-Vera J, Kalergis AM. Implications of macrophage polarization in autoimmunity. Immunology. (2018) 154:186–95. doi: 10.1111/imm.2018.154.issue-2
109. Bouhlel MA, Derudas B, Rigamonti E, Dièvart R, Brozek J, Haulon S, et al. PPARgamma activation primes human monocytes into alternative M2 macrophages with anti-inflammatory properties. Cell Metab. (2007) 6:137–43. doi: 10.1016/j.cmet.2007.06.010
110. Stasiulewicz A, Znajdek K, Grudzień M, Pawiński T, Sulkowska AJI. A guide to targeting the endocannabinoid system in drug design. Int J Mol Sci. (2020) 21:2778. doi: 10.3390/ijms21082778
111. Zhao J, Li X, Hu J, Chen F, Qiao S, Sun X, et al. Mesenchymal stromal cell-derived exosomes attenuate myocardial ischaemia-reperfusion injury through miR-182-regulated macrophage polarization. Cardiovasc Res. (2019) 115:1205–16. doi: 10.1093/cvr/cvz040
112. Sun W, Yan S, Yang C, Yang J, Wang H, Li C, et al. Mesenchymal stem cells-derived exosomes ameliorate lupus by inducing M2 macrophage polarization and regulatory T cell expansion in MRL/lpr mice. Immunol Invest. (2022) 51:1785–803. doi: 10.1080/08820139.2022.2055478
113. Njock M-S, Cheng HS, Dang LT, Nazari-Jahantigh M, Lau AC, Boudreau E, et al. Endothelial cells suppress monocyte activation through secretion of extracellular vesicles containing antiinflammatory microRNAs. Blood. (2015) 125:3202–12. doi: 10.1182/blood-2014-11-611046
114. Zhao H, Shang Q, Pan Z, Bai Y, Li Z, Zhang H, et al. Exosomes from adipose-derived stem cells attenuate adipose inflammation and obesity through polarizing M2 macrophages and beiging in white adipose tissue. Diabetes. (2018) 67:235–47. doi: 10.2337/db17-0356
115. Musai J, Mammen AL, Pinal-Fernandez I. Recent updates on the pathogenesis of inflammatory myopathies. Curr Rheumatol Rep. (2024) 26(12):421–30. doi: 10.1007/s11926-024-01164-7
116. Abad C, Pinal-Fernandez I, Guillou C, Bourdenet G, Drouot L, Cosette P, et al. IFNγ causes mitochondrial dysfunction and oxidative stress in myositis. Nat Commun. (2024) 15:5403. doi: 10.1038/s41467-024-49460-1
117. Sevim E, Kobrin D, Casal-Dominguez M, Pinal-Fernandez I. A comprehensive review of dermatomyositis treatments - from rediscovered classics to promising horizons. Expert Rev Clin Immunol. (2024) 20:197–209. doi: 10.1080/1744666X.2023.2270737
118. Amici DR, Pinal-Fernandez I, Christopher-Stine L, Mammen AL, Mendillo ML. A network of core and subtype-specific gene expression programs in myositis. Acta Neuropathol. (2021) 142:887–98. doi: 10.1007/s00401-021-02365-5
119. Pinal-Fernandez I, Casal-Dominguez M, Derfoul A, Pak K, Miller FW, Milisenda JC, et al. Machine learning algorithms reveal unique gene expression profiles in muscle biopsies from patients with different types of myositis. Ann Rheum Dis. (2020) 79:1234–42. doi: 10.1136/annrheumdis-2019-216599
120. Gadina M, Le MT, Schwartz DM, Silvennoinen O, Nakayamada S, Yamaoka K, et al. Janus kinases to jakinibs: from basic insights to clinical practice. Rheumatol (Oxford). (2019) 58:i4–i16. doi: 10.1093/rheumatology/key432
121. Traves PG, Murray B, Campigotto F, Galien R, Meng A, Di Paolo JA. JAK selectivity and the implications for clinical inhibition of pharmacodynamic cytokine signalling by filgotinib, upadacitinib, tofacitinib and baricitinib. Ann Rheum Dis. (2021) 80:865–75. doi: 10.1136/annrheumdis-2020-219012
122. López-Navarro B, Simón-Fuentes M, Ríos I, Schiaffino MT, Sanchez A, Torres-Torresano M, et al. Macrophage re-programming by JAK inhibitors relies on MAFB. Cell Mol Life Sci. (2024) 81:152. doi: 10.1007/s00018-024-05196-1
123. Kurasawa K, Arai S, Namiki Y, Tanaka A, Takamura Y, Owada T, et al. Tofacitinib for refractory interstitial lung diseases in anti-melanoma differentiation-associated 5 gene antibody-positive dermatomyositis. Rheumatol (Oxford). (2018) 57:2114–9. doi: 10.1093/rheumatology/key188
124. Ida T, Furuta S, Takayama A, Tamura J, Hayashi Y, Abe K, et al. Efficacy and safety of dose escalation of tofacitinib in refractory anti-MDA5 antibody-positive dermatomyositis. RMD Open. (2023) 9:e002795. doi: 10.1136/rmdopen-2022-002795
125. Hama S, Akiyama M, Higashida-Konishi M, Oshige T, Takei H, Izumi K, et al. Successful treatment with tofacitinib for relapse of rapidly progressive interstitial lung disease in anti-melanoma differentiation-associated gene 5 antibody-positive clinically amyopathic dermatomyositis. Mod Rheumatol Case Rep. (2023) 7:92–5. doi: 10.1093/mrcr/rxac049
126. Hosokawa Y, Oiwa H. A case of refractory interstitial lung disease in anti-MDA5-positive dermatomyositis that improved after switching to tofacitinib. J Clin Rheumatol. (2021) 27:S661–2. doi: 10.1097/RHU.0000000000001645
127. Kato M, Ikeda K, Kageyama T, Kasuya T, Kumagai T, Furuya H, et al. Successful treatment for refractory interstitial lung disease and pneumomediastinum with multidisciplinary therapy including tofacitinib in a patient with anti-MDA5 antibody-positive dermatomyositis. J Clin Rheumatol. (2021) 27:S574–7. doi: 10.1097/RHU.0000000000000984
128. Li S, Li S, Wang J, Zhang L, Duan J, Lu X, et al. Efficacy and safety of tofacitinib in anti-melanoma differentiation-associated 5 gene antibody-positive dermatomyositis. J Clin Rheumatol. (2023) 29:281–4. doi: 10.1097/RHU.0000000000002010
129. Chen Z, Wang X, Ye S. Tofacitinib in amyopathic dermatomyositis-associated interstitial lung disease. N Engl J Med. (2019) 381:291–3. doi: 10.1056/NEJMc1900045
130. Wang Y, Luo J, Lv X, Li Y, An Q, Mo L, et al. Tofacitinib for new-onset adult patients with anti-melanoma differentiation-associated 5 gene antibody positive dermatomyositis. Clin Rheumatol. (2023) 42:1847–53. doi: 10.1007/s10067-023-06567-y
131. Harada H, Shoda H, Tsuchiya H, Misaki M, Sawada T, Fujio K. Baricitinib for anti-melanoma differentiation-associated protein 5 antibody-positive dermatomyositis-associated interstitial lung disease: a case series and literature review on Janus kinase inhibitors for the disease. Rheumatol Int. (2024) 44:961–71. doi: 10.1007/s00296-024-05551-2
132. Morand EF, Furie R, Tanaka Y, Bruce IN, Askanase AD, Richez C, et al. Trial of anifrolumab in active systemic lupus erythematosus. N Engl J Med. (2020) 382:211–21. doi: 10.1056/NEJMoa1912196
133. Zhang L, Jiang Y, Jia W, Le W, Liu J, Zhang P, et al. Modelling myocardial ischemia/reperfusion injury with inflammatory response in human ventricular cardiac organoids. Cell Prolif. (2025) 58(3):e13762. doi: 10.1111/cpr.13762
134. Shaw KS, Reusch DB, Castillo RL, Hashemi KB, Sundel R, Dedeoglu F, et al. Rapid improvement in recalcitrant cutaneous juvenile dermatomyositis with anifrolumab treatment. JAMA Dermatol. (2024) 160:237–8. doi: 10.1001/jamadermatol.2023.4744
135. Ang PS, Ezenwa E, Ko K, Hoffman MD. Refractory dermatomyositis responsive to anifrolumab. JAAD Case Rep. (2024) 43:27–9. doi: 10.1016/j.jdcr.2023.10.023
136. Fiorentino D, Mangold AR, Werth VP, Christopher-Stine L, Femia A, Chu M, et al. Efficacy, safety, and target engagement of dazukibart, an IFNβ specific monoclonal antibody, in adults with dermatomyositis: a multicentre, double-blind, randomised, placebo-controlled, phase 2 trial. Lancet. (2025) 405:137–46. doi: 10.1016/S0140-6736(24)02071-3
137. Hengstman GJD, van den Hoogen FHJ, Barrera P, Netea MG, Pieterse A, van de Putte LBA, et al. Successful treatment of dermatomyositis and polymyositis with anti-tumor-necrosis-factor-alpha: preliminary observations. Eur Neurol. (2003) 50:10–5. doi: 10.1159/000070852
138. Anandacoomarasamy A, Howe G, Manolios N. Advanced refractory polymyositis responding to infliximab. Rheumatol (Oxford). (2005) 44:562–3. doi: 10.1093/rheumatology/keh539
139. Efthimiou P, Schwartzman S, Kagen LJ. Possible role for tumour necrosis factor inhibitors in the treatment of resistant dermatomyositis and polymyositis: a retrospective study of eight patients. Ann Rheum Dis. (2006) 65:1233–6. doi: 10.1136/ard.2005.048744
140. Iannone F, Scioscia C, Falappone PCF, Covelli M, Lapadula G. Use of etanercept in the treatment of dermatomyositis: a case series. J Rheumatol. (2006) 33:1802–4.
141. Muscle Study Group. A randomized, pilot trial of etanercept in dermatomyositis. Ann Neurol. (2011) 70:427–36. doi: 10.1002/ana.22477
142. Selva-O’Callaghan A, Martínez-Costa X, Solans-Laque R, Mauri M, Capdevila JA, Vilardell-Tarrés M. Refractory adult dermatomyositis with pneumatosis cystoides intestinalis treated with infliximab. Rheumatol (Oxford). (2004) 43:1196–7. doi: 10.1093/rheumatology/keh285
143. Narazaki M, Hagihara K, Shima Y, Ogata A, Kishimoto T, Tanaka T. Therapeutic effect of tocilizumab on two patients with polymyositis. Rheumatol (Oxford). (2011) 50:1344–6. doi: 10.1093/rheumatology/ker152
144. Aggarwal R, Rider LG, Ruperto N, Bayat N, Erman B, Feldman BM, et al. 2016 American college of rheumatology/European league against rheumatism criteria for minimal, moderate, and major clinical response in adult dermatomyositis and polymyositis: An international myositis assessment and clinical studies group/paediatric rheumatology international trials organisation collaborative initiative. Arthritis Rheumatol. (2017) 69:898–910. doi: 10.1002/art.40064
145. Dastmalchi M, Grundtman C, Alexanderson H, Mavragani CP, Einarsdottir H, Helmers SB, et al. A high incidence of disease flares in an open pilot study of infliximab in patients with refractory inflammatory myopathies. Ann Rheum Dis. (2008) 67:1670–7. doi: 10.1136/ard.2007.077974
146. Schiffenbauer A, Garg M, Castro C, Pokrovnichka A, Joe G, Shrader J, et al. A randomized, double-blind, placebo-controlled trial of infliximab in refractory polymyositis and dermatomyositis. Semin Arthritis Rheum. (2018) 47:858–64. doi: 10.1016/j.semarthrit.2017.10.010
147. Ishikawa Y, Yukawa N, Ohmura K, Hosono Y, Imura Y, Kawabata D, et al. Etanercept-induced anti-Jo-1-antibody-positive polymyositis in a patient with rheumatoid arthritis: a case report and review of the literature. Clin Rheumatol. (2010) 29:563–6. doi: 10.1007/s10067-009-1370-1
148. Klein R, Rosenbach M, Kim EJ, Kim B, Werth VP, Dunham J. Tumor necrosis factor inhibitor-associated dermatomyositis. Arch Dermatol. (2010) 146:780–4. doi: 10.1001/archdermatol.2010.142
149. Riolo G, Towheed TE. Anti-tumor necrosis factor inhibitor therapy-induced dermatomyositis and fasciitis. J Rheumatol. (2012) 39:192–4.
150. Degboé Y, Rauwel B, Baron M, Boyer J-F, Ruyssen-Witrand A, Constantin A, et al. Polarization of rheumatoid macrophages by TNF targeting through an IL-10/STAT3 mechanism. Front Immunol. (2019) 10:3. doi: 10.3389/fimmu.2019.00003
151. Obeng JA, Amoruso A, Camaschella GLE, Sola D, Brunelleschi S, Fresu LG. Modulation of human monocyte/macrophage activity by tocilizumab, abatacept and etanercept: an in vitro study. Eur J Pharmacol. (2016) 780:33–7. doi: 10.1016/j.ejphar.2016.03.028
152. Izuka S, Komai T, Itamiya T, Ota M, Nagafuchi Y, Shoda H, et al. Machine learning-driven immunophenotypic stratification of mixed connective tissue disease corroborating the clinical heterogeneity. Rheumatol (Oxford). (2025) 64(3):1409–16. doi: 10.1093/rheumatology/keae158
153. Saygin D, Werth V, Paik JJ, Park JK, Needham M, Lundberg IE, et al. Current myositis clinical trials and tribulations. Ann Rheum Dis. (2024) 83:826–9. doi: 10.1136/ard-2023-224652
154. Izuka S, Sen P, Komai T, Fujio K, Knitza J, Gupta L. Digital approaches in myositis. Health Policy Technol. (2024) 13(4):100906. doi: 10.1016/j.hlpt.2024.100906
155. Fisher L, Ben-Shabat N, Gendelman O, Sharif K, Ehrenberg S, Shani U, et al. Risk of atherosclerosis-related diseases in polymyositis and dermatomyositis patients: A large-scale population-based study. Atherosclerosis. (2025) 401:119100. doi: 10.1016/j.atherosclerosis.2024.119100
156. Moore KJ, Sheedy FJ, Fisher EA. Macrophages in atherosclerosis: a dynamic balance. Nat Rev Immunol. (2013) 13:709–21. doi: 10.1038/nri3520
Keywords: idiopathic inflammatory myopathy, myositis, dermatomyositis, monocytes, macrophages, interstitial lung disease, interferon, mitochondrial dysfunction
Citation: Izuka S, Komai T, Tsuchida Y, Tsuchiya H, Okamura T and Fujio K (2025) The role of monocytes and macrophages in idiopathic inflammatory myopathies: insights into pathogenesis and potential targets. Front. Immunol. 16:1567833. doi: 10.3389/fimmu.2025.1567833
Received: 28 January 2025; Accepted: 03 March 2025;
Published: 20 March 2025.
Edited by:
Mitsuhiro Takeno, Nippon Medical School Musashi Kosugi Hospital, JapanReviewed by:
Tsuyoshi Shirai, Tohoku University, JapanCopyright © 2025 Izuka, Komai, Tsuchida, Tsuchiya, Okamura and Fujio. This is an open-access article distributed under the terms of the Creative Commons Attribution License (CC BY). The use, distribution or reproduction in other forums is permitted, provided the original author(s) and the copyright owner(s) are credited and that the original publication in this journal is cited, in accordance with accepted academic practice. No use, distribution or reproduction is permitted which does not comply with these terms.
*Correspondence: Toshihiko Komai, S09NQUlULUlOVEBoLnUtdG9reW8uYWMuanA=; Keishi Fujio, RlVKSU9LLUlOVEBoLnUtdG9reW8uYWMuanA=
Disclaimer: All claims expressed in this article are solely those of the authors and do not necessarily represent those of their affiliated organizations, or those of the publisher, the editors and the reviewers. Any product that may be evaluated in this article or claim that may be made by its manufacturer is not guaranteed or endorsed by the publisher.
Research integrity at Frontiers
Learn more about the work of our research integrity team to safeguard the quality of each article we publish.