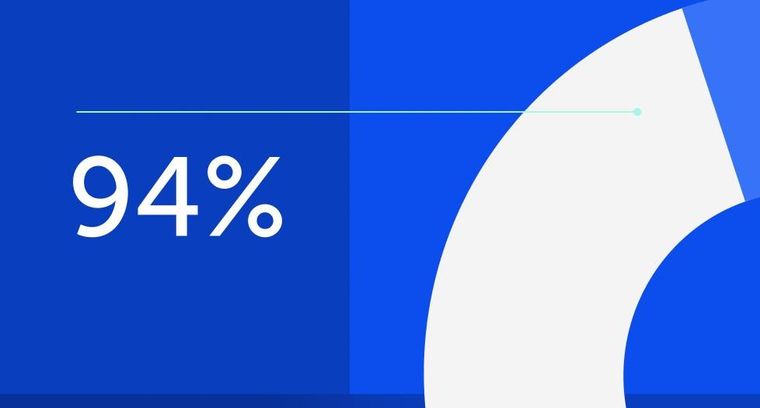
94% of researchers rate our articles as excellent or good
Learn more about the work of our research integrity team to safeguard the quality of each article we publish.
Find out more
REVIEW article
Front. Immunol., 31 March 2025
Sec. Systems Immunology
Volume 16 - 2025 | https://doi.org/10.3389/fimmu.2025.1563484
This article is part of the Research TopicUnveiling the Host's Acute Immune Response to Infectious Mucosal Diseases: Insights and ImplicationsView all 4 articles
Lipopolysaccharide (LPS), also known as an endotoxin, is derived from Gram-negative bacteria. The intravenous administration of LPS induces an inflammatory response and causes systemic inflammation, such as cytokine storm. Gram-negative bacteria that produce LPS are found in the environment and digestive tract. The mucous membrane, the primary barrier between the interior of the body and the external environment, is constantly exposed to LPS. Moreover, no toxicity is observed when administering LPS through the mucous membranes of the mouth or skin. The presence of LPS in the mucous membranes is necessary not only for maintaining health but also for inducing preventive and therapeutic effects against multiple diseases when administered orally or topically. LPS is an environmental substance that is useful when administered to mucous membranes. The general information emphasizes the role of LPS as an inflammatory substance that occurs when administered intravenously. Therefore, the valuable role of LPS is unknown. Thus, mucosal administration of LPS has received little attention, and the mechanism underlying the expression of its beneficial effects has not been fully elucidated. We proposed a comprehensive concept, the “macrophage network,” which proposes a regulatory system in which the mucosa receives environmental information, membrane-bound cytokines are expressed in phagocytes (macrophages), and these macrophages migrate distally to exert effects, such as anti-inflammatory and tissue repair effects, on distal tissues through cell-to-cell communication (juxtacrine signaling) with tissue macrophages. This macrophage network is effective not only for preventing and treating diseases but also for increasing the efficacy of pharmaceuticals. This review aims to investigate the preventive and therapeutic effects of oral and transdermal administration of LPS on various diseases and present an introduction to the concept of the macrophage network and the latest findings.
Living organisms must maintain homeostasis and survive despite being exposed to diverse environmental changes. They must eliminate invading pathogens, such as viruses and bacteria, and tissue damage caused by other factors, such as ultraviolet rays, chemicals, and heat. Living organisms have developed mechanisms to maintain homeostasis by actively using environmental information that is useful for survival. In the context of immune responses, the primary reception of this information is mainly carried out by innate immunity. Phagocytes, the main cells responsible for innate immunity, not only identify and eliminate foreign invaders, such as bacteria and viruses, but also respond to a wide range of other environmental stimuli, such as heat, pressure, and low-molecular-weight substances, such as adenosine and salt, and play a significant role in maintaining the homeostasis of the individual.
Until recently, little attention has been paid to the biological responses to these everyday environmental stimuli to maintain homeostasis. This may be due to the difficulty of extracting the necessary information from miscellaneous environmental information and of understanding how an individual organism maintains homeostasis even when this information is extracted. Innate immunity is a well-understood mechanism for maintaining homeostasis. The research focusing on the identification of lipopolysaccharide (LPS) in the outer membrane of Gram-negative bacteria and its receptor, Toll-like receptor 4 (TLR4), has dramatically advanced our understanding of the molecular level of innate immunity involving macrophages, dendritic cells, neutrophils, epithelial cells, etc. (1–3). LPS is a substance that activates these natural immune system cells in minute quantities (4) and is well known to induce systemic inflammation when administered intravascularly, even at low doses (5). We focused on innate immunity and searched for environmental factors that control macrophages via the mucosa. We found that mucosal administration of LPS is highly useful as a molecule that controls innate immunity (6). The administration of LPS via mucosal routes, such as oral or transdermal delivery, not only exhibits no toxicity but also, contrary to conventional understanding, demonstrates anti-inflammatory effects and tissue repair and regeneration capabilities. The mechanism by which LPS contributes to the maintenance of systemic homeostasis via mucosal innate immunity is still largely unknown. To elucidate this mechanism, we have focused on macrophages, which maintain tissue homeostasis, and have hypothesized that a regulatory system called the “macrophage network” (7, 8). Additionally, in vitro test systems using cultured cells are another factor that hinders our understanding of the physiological effects of LPS. Until now, LPS has been used as an inflammatory agent by stimulating monocyte-derived macrophage cell cultures or bone marrow-derived cells at high concentrations (≥100 ng/ml) or by injecting it into the abdominal cavity or blood vessels of animals (9). This model could be a useful tool for treating sepsis and inflammatory diseases. However, LPS is naturally present in the skin, intestinal tract, and respiratory tract, and it cannot be treated the same way as in the injection model. It has become clear that LPS has useful physiological activity when administered orally or topically and has a previously unknown homeostatic maintenance effect (10).
This review aims to demonstrate that oral and transdermal administration of LPS is beneficial for preventing and treating chronic inflammatory diseases, such as allergies and dementia, which are difficult to treat with current medical treatments. Additionally, this review discusses the role of LPS as an environmental substance in maintaining homeostasis in individuals via the mucosal membrane and the macrophage network hypothesis.
Innate self-healing ability is a critical physiological mechanism for maintaining individual health. Its role in eliminating exogenous agents, such as viruses and bacteria, remains a key research area. At sites of foreign invasion, tissue-resident macrophages initiate the molecular recognition of pathogens through pathogen-associated molecular pattern recognition receptors, including TLRs, Nod-like receptors, C-type lectin receptors, and RIG-I-like receptors. The activation of these pattern recognition receptors triggers macrophage-mediated responses, including the recruitment of neutrophils and monocytes, generation of reactive oxygen species (ROS), and production of chemokines and cytokines (11). These events manifest as inflammatory symptoms, such as edema, erythema, fever, and pain, which are traditionally viewed under pathological conditions (12).
Inflammation has been increasingly recognized as a physiological process that plays an important role in tissue repair and healing. During immune challenges, such as injury and infection, tissue macrophages recognize pathogens and initiate an acute inflammatory response. This process involves tissue destruction via ROS production to eliminate the offending agent, followed by a highly regulated process of tissue repair and regeneration. The repair phase involves debris phagocytosis, angiogenesis, and tissue remodeling (13). The concept of physiological inflammation was first proposed by Metchnikoff, who asserted its essential role in maintaining homeostasis through its dual function of pathogen clearance and tissue repair (14, 15). As key mediators of innate immunity, macrophages play an important role in the inflammatory process, participating from its onset to resolution (13).
The theories of Metchnikoff on physiological inflammation are being revisited, extending the role of innate immunity beyond classical inflammation to encompass broader self-healing mechanisms (16). Initially, innate immunity was understood as a defense system that recognizes and neutralizes exogenous pathogens, such as bacteria, viruses, and fungi (17). Today, it is considered a frontline mechanism capable of eliminating endogenous aberrant elements, such as senescent or apoptotic cells, denatured proteins, and oxidized lipids, which disrupt homeostasis (18–21). This orchestrated process, which is referred to as physiological inflammation, enables the clearance and repair of damaged tissues, thereby promoting recovery (22).
Physiological inflammation is particularly related to scenarios in which tissue damage is caused by pathogens, aging, or malignancies. Macrophage-mediated processes clear damaged cells and tissues, facilitating their repair and regeneration (18). Thus, innate immunity is primarily driven by macrophages and is the cornerstone of self-healing.
Failure to effectively eliminate pathological stimuli through the physiological inflammatory process may lead to the accumulation of foreign substances and sustained cellular damage, thereby leading to chronic inflammation and impaired tissue function and homeostasis. Therefore, the decline in innate immune function compromises the recognition and resolution of internal and external aberrations, ultimately reducing the organism’s self-healing ability.
Significant attention has been paid to the role of innate immunity in infection prevention, particularly during the spread of novel coronaviruses (23). Evidence suggests that the Bacillus Calmette–Guérin (BCG) vaccine promotes a rapid immune response to COVID-19, which potentially mitigates disease progression through the early suppression of viral replication mediated by interferon-alpha (IFN-α) induction (24). Furthermore, the BCG vaccine enhances IFN-α production during viral entry through epigenetic modifications in macrophages (25). This durable infection-preventive effect, known as “trained immunity” or “innate immune memory,” indicates the enduring nature of such responses (26). Therefore, the maintenance of robust innate immune functionality is increasingly recognized as an important strategy for preventing and treating infectious diseases.
The prevalence of lifestyle-related diseases, such as cancer, diabetes, and Alzheimer’s disease, has increased over the past decade (27–29). Although these conditions are associated with lifestyle factors, such as physical inactivity and excessive consumption of high-calorie diets, aging also plays a critical role. Aging is associated with decreased macrophage phagocytic activity, reduced clearance of foreign substances, and chronic inflammation, which is driven by phenotypic shifts from tissue-repairing M2 macrophages to proinflammatory M1 macrophages (30). Additionally, disruption of homeostatic mechanisms can exacerbate these conditions (30, 31). As proposed in the “hygiene hypothesis,” modern urban lifestyles reduce exposure to symbiotic microbiota and innate immune stimuli, such as LPS. This reduction alters the balance of acquired immunity, thereby increasing the prevalence of allergic diseases (32–34). These observations underscore the importance of innate immunity in maintaining physiological homeostasis.
Macrophages are heterogeneous immune cells with high plasticity that adapt to environmental cues and exhibit distinct functional phenotypes. M1 macrophages are responsible for the clearance of pathogens through the production of inflammatory cytokines, chemokines, and ROS. In contrast, M2 macrophages mediate anti-inflammatory responses and promote tissue repair and regeneration through the secretion of anti-inflammatory cytokines and growth factors (13, 35–40). Recent therapeutic approaches targeting M2 macrophages have shown promise in managing Alzheimer’s disease (41), maintaining skin health (18, 42), restoring mobility after spinal cord injury, and treating amyotrophic lateral sclerosis and liver cirrhosis (43–48). Schwartz et al. reported that inducing tissue-reparative macrophages can regulate physiological inflammation and provide therapeutic potential for traditionally challenging conditions, such as spinal cord injuries (49, 50).
Therefore, the activation of innate immunity plays an important role not only in preventing infection but also in strengthening self-healing mechanisms across various diseases, including lifestyle-related conditions. This perspective provides valuable insights into the scientific exploration of self-healing, an essential and broad concept in health maintenance and disease management.
Macrophages are widely distributed across tissues and play central roles in immune responses and homeostasis. Macrophages constitute approximately 50% of the total immune cell mass in the human body (51), highlighting their significant importance in immune defense mechanisms. Tiemeijer et al. reported that macrophage interactions vary according to density and the environmental context, revealing heterogeneity at the single-cell level (52). This quantitative and qualitative diversity indicates that macrophages significantly contribute to the maintenance of organismal homeostasis. However, the mechanism underlying macrophage-mediated homeostatic regulation is not fully elucidated. Tissue macrophages are increasingly recognized as integral to the clearance of dead cells, cellular debris, immune complexes, bacteria, and other waste products. Additionally, they play an essential role in organ development and tissue homeostasis (31). Crosstalk between tissue-resident macrophages and local cells has been reported in the heart (53) and adipose tissue (54), where mitochondria serve as signaling intermediaries. In addition to their tissue-specific roles, macrophages are believed to function as part of a systemic network (Figure 1). This “macrophage network” hypothesis posits that macrophages in specific tissues communicate with counterparts in distant tissues to regulate homeostasis (8). Recent studies have suggested that this macrophage network operates through integrin-mediated motor control and inter-macrophage communication, a mechanism observed even in primitive multicellular organisms, such as sponges, where migratory macrophage-like cells maintain organismal integrity in the absence of the nervous system (55, 56). Macrophage-mediated signaling relies on cytokines. Information transmission occurs through paracrine or juxtacrine signaling, in which membrane-expressed cytokines, such as tumor necrosis factor (TNF), interleukin-1 alpha, Fas ligand, TNF-related apoptosis-inducing ligand, stem cell factor, and transforming growth factor alpha, facilitate direct communication between macrophages and target cells (57). Examples of juxtacrine signaling include tumor necrosis factor (TNF) (58), IL-1 alpha (59), Fas ligand (60), TRAIL (61), SCF (62), and TGF-alpha (63).
Figure 1. The macrophage network concept. The macrophage network hypothesis is based on the idea that the transmission of information is mediated by secreted cytokines (paracrine) and/or by cell-to-cell signaling (juxtacrine) via membrane-bound cytokines expressed on macrophages. Oral and transdermal administration of LPS activates macrophages of the intestinal mucosa, which induce the activation of systemic macrophages in a paracrine manner and/or membrane-bound cytokine-expressing macrophages migrate to tissues and communicate with systemic macrophages in a juxtacrine manner. mCSF1, TNF-α, FasL, OX40L, and other signaling molecules have been proposed to be involved in propagating the activation of mucosal macrophage to systemic macrophage. FasL, Fas ligand; LPS, lipopolysaccharide; mCSF1, membrane-bound colony stimulating factor-1; OX40L, OX40 ligand; TLR4, toll-like receptor 4; TNF-α, tumor necrosis factor-alpha.
Activated macrophages in one tissue may migrate to distal tissues, thereby transmitting information to local cellular populations. Migratory macrophages serve as critical mediators of systemic immune regulation in higher organisms. The systemic signaling capacity of macrophages is highlighted in several examples. During cardiac stress, such as exercise, cardiac macrophages are activated by colony-stimulating factor 2 (CSF2) secreted by renal macrophages, facilitating cardiomyocyte proliferation (64). Similarly, oral administration of LPS induces the expression of membrane-bound CSF1 in peripheral leukocytes, enhancing the phagocytic capacity of microglia in the brain and converting them into neuroprotective M2-type macrophages (65). In the gastrointestinal system, LPS activates intestinal macrophages to induce bone morphogenetic protein-2, which stimulates enteric neurons and enhances peristalsis, whereas neurons secrete CSF2 to promote macrophage proliferation (66). These processes are mediated by TLR4 ligands derived from the gut microbiota (67). These findings support the existence of a tissue macrophage-mediated signaling system, suggesting that a functional macrophage network extends across multiple organ systems (68–72). In Western medicine, drug delivery systems target local areas to enhance therapeutic efficacy. However, environmental stimuli, such as those received by the skin and mucosa, often affect distant tissues. Similar phenomena have been observed in adaptive immunity, wherein dendritic cells and monocytes transport pathogen antigens from the site of infection or vaccination to the lymph nodes, activating CD4+ and CD8+ T cells in lymphoid tissues (73). The diverse population of tissue macrophages that can receive environmental signals and transmit them across the body supports the hypothesis that the macrophage network plays a pivotal role in maintaining systemic health. Therefore, macrophages act as a highly versatile network that integrates environmental signals and orchestrates immune responses across tissues. The macrophage network is a critical component of innate immune regulation and systemic homeostasis, indicating its potential as a therapeutic target for promoting health and treating diseases.
Improvements in healthcare access, nutrition, and sanitation have contributed to increased longevity in developed countries. However, the incidence of allergies, lifestyle-related diseases, emerging infectious diseases, and cancers that are challenging to treat is increasing in modern societies (74–77). This is often attributed to enhanced sanitation in urban environments and the overuse of antibiotics, which lead to a decline in the natural functionality of the immune system (34, 78, 79). LPS, as a potential missing environmental factor, has emerged as a focus of interest for compensating for diminished innate immune function.
LPS is a major component of the outer membrane of Gram-negative bacteria. It is a macromolecule with a molecular weight of approximately 5–100 kDa. It has three main components: lipid A, core polysaccharide, and O-antigen. The biological activity of LPS is primarily mediated by its interaction with TLR4. LPS can be categorized based on the bacterial source into lipid A with six to seven fatty acids (e.g., Gammaproteobacteria) and lipid A with four to five fatty acids (e.g., Bacteroides). The former exhibits a 100-fold greater binding affinity for TLR4 and higher biological activity (80, 81). Enterobacteriaceae-derived LPS, which is part of the high-affinity group, activates macrophages at picogram concentrations, making it one of the most potent innate immune activators (4). However, excessive systemic LPS through intravascular administration can induce a cytokine storm characterized by the release of interleukin-6, IFN-γ, and TNF-α, thereby leading to endotoxemia (82).
Symbiotic Gram-negative bacteria inhabit mucosal surfaces, such as the gastrointestinal tract, skin, and oral cavity, releasing LPS into the environment as extracellular vesicles, also known as outer membrane vesicles. In these contexts, LPS contributes more to health maintenance than inflammation. Previous studies have shown that TLR4-deficient mice exhibit increased susceptibility to allergic reactions, reduced macrophage activity, impaired intestinal motility, and decreased neuronal survival, highlighting the role of LPS-TLR4 signaling in maintaining innate immune homeostasis (67, 83, 84). Oral administration of LPS enhances macrophage phagocytic activity without inducing systemic inflammation and confers neuroprotective effects by transforming microglia into a neuroprotective phenotype (65, 85). Thus, LPS can safely regulate innate immunity when administered orally or through mucosal routes.
The “hygiene hypothesis” posits that improved sanitation in urban environments reduces exposure to microbial components, such as LPS, leading to an increased prevalence of allergic diseases (86). This has been linked to an imbalance in T-helper cell responses, with a dominance of Th2 over Th1 immunity (83, 87). Children with gut microbiota dominated by low-LPS-producing Bacteroides have been reported to be more prone to allergies than those with high-LPS-producing Enterobacteriaceae (84). These findings indicate that variations in gut microbiota-derived LPS influence allergy susceptibility and can be mitigated by dietary interventions. As mentioned above, LPS can be broadly divided into two types. One is LPS represented by the genus Gammaproteobacteria, which is a strong TLR4 stimulator. The second is LPS represented by the genus Bacteroides, which is a weaker TLR4 stimulator than the former. The difference between these LPS is mainly due to differences in their affinity for TLR4 (88). Therefore, we believe that the difference in function is due to quantitative issues with the LPS functioning, and not due to differences in function between LPS types.
Furthermore, the widespread use of antibiotics, although pivotal in infection control, has disrupted the gut microbiota composition, leading to a reduction in Gram-negative bacterial populations and associated LPS signaling (89, 90). When mice are given antibiotics orally, the density and diversity of intestinal bacteria in their feces decreases by approximately one-tenth or more (89), and the amount of antimicrobial peptide (RegIIIγ) secreted by Paneth cells in the small intestine decreases by one-fifth (90). This antimicrobial peptide suppresses the growth of vancomycin-resistant enterococci (VRE) among intestinal bacteria, and although VRE grows when antibiotics are administered, the growth of VRE is also suppressed by oral administration of LPS (90). Disturbances to the intestinal flora caused by antibiotics are particularly concerning in early childhood. Exposure to antibiotics in the neonatal period has been shown to increase the odds of developing allergies by 2-3 times when assessed between the ages of 2 and adulthood (91), suggesting that it is associated with an increased risk of allergic diseases in later life. Therefore, oral administration of LPS has been suggested as a potential countermeasure to restore innate immune function in such scenarios (90).
With the increasing prevalence of allergic diseases, particularly in urban settings, maintaining adequate environmental exposure to LPS is essential for immune homeostasis. Additionally, developing safe and effective technologies to modulate LPS levels is urgently needed to address these urban-associated diseases. In such cases, it is thought that the LPS of the genus Gammaproteobacteria, which acts in small quantities, is more effective (92).
LPS is routinely ingested through the intestinal mucosa and contributes to health maintenance. However, many biologists do not accept the notion that LPS is an inflammatory substance and sensibly useful. The intravascular administration of LPS induces an extremely strong systemic cytokine storm. The maximum-tolerated dose of the intravenous administration of LPS in humans is as low as 4 ng/kg, and it is an extremely strong inflammatory agent (4). Given that roughly half of the symbiotic bacteria present in the mucous membranes of the intestinal tract, oral cavity, airways, and skin are Gram-negative bacteria, which constantly release outer membrane vesicles, including LPS, it is not surprising that LPS is a substance that hardly induces inflammation in the oral cavity and skin.
Our research has shown that humans are likely to consume LPS daily. Many plants, including vegetables and cereals, have been found to contain more than 10 µg/g of LPS (93, 94). The LPS content in brown rice reaches 10 µg/g, and plant-derived LPS may be involved in maintaining health through daily consumption (6). The levels of LPS in 414 herbal extracts were measured and reported to range from ≥10 ng/g to >100 µg/g (overall mean value 17.4 µg/g). Although the origin of LPS in plants is unknown, it is believed to be derived from Gram-negative bacteria that are in symbiosis with plants in the rhizosphere, where plant hairy roots and soil composition interact with each other, and bacterial density increases by tens to hundreds of times (95). Many symbiotic bacteria in the rhizosphere have been reported to exhibit beneficial effects on plant growth, such as nitrogen fixation, solubilization of insoluble phosphates, and penetration into the plant interior for infection control (96). Additionally, many Enterobacteriaceae bacteria, such as Escherichia coli, Salmonella, and Pantoea, possess LPS, which activates innate immunity in minute quantities (10). Therefore, LPS can be ingested orally in large quantities on a daily basis, and it is considered safe. In fact, when LPS derived from Pantoea agglomerans, which is symbiotic with many plants, such as wheat and rice, was administered at a dose of 4500 mg/kg body weight/day for at least 90 days, no adverse effects were observed in terms of clinical symptoms, body weight, food intake, or clinical pathology, and no macroscopic or microscopic findings related to the test substance were observed (97, 98). The LPS derived from Pantoea agglomerans has already received Generally Recognized as Safe status, and it has been granted New Dietary Ingredient certifications by the US Food and Drug Administration as an active nutritional ingredient, ensuring its safety.
One concern about the oral administration of LPS, which belongs to the genus Gammaproteobacteria and activates macrophages in small quantities, is that endotoxemia caused by LPS absorbed through the intestinal tract may exacerbate diabetes and atherosclerosis (99, 100). These studies regard high blood LPS levels in mice fed a high-fat diet as endotoxemia. However, this increase in blood LPS levels is known to be due to LPS being absorbed from the intestinal tract together with fat and being bound to lipoprotein particles (chylomicrons) that transport fat in the blood (101). Additionally, LPS bound to chylomicrons is thought to be excreted from the body without causing inflammation (101). Therefore, even though high-fat diets are related to increased blood LPS levels, it cannot be determined as the cause. Furthermore, a previous study (99) introduced a continuous LPS infusion model using a subcutaneous infusion pump as a demonstration experiment to show that high-fat diet-induced endotoxemia exacerbates diabetes. Injected LPS differs significantly from LPS in the blood after oral intake. As mentioned previously, it causes systemic inflammation in minute quantities. In other words, this continuous LPS administration model leads to the worsening of diabetes due to systemic chronic inflammation and has a different mechanism of action from oral LPS administration.
Oral administration of LPS derived from P. agglomerans, which belongs to the genus Gammaproteobacteria, has demonstrated a protective effect against diabetes in a mouse model of obese diabetes (KK-Ay) (102), which is contrary to common knowledge. This discrepancy is attributed to the recognition of endotoxemia as a high blood LPS level in mice fed a high-fat diet. However, the increase in blood LPS levels associated with a high-fat diet is based on the fact that LPS is associated with lipoprotein particles (chylomicrons) that are absorbed with fat and transport fat in the blood (101).
LPS has been reported to induce inflammation in certain pathological conditions in the leaky gut, which is characterized by loss of intestinal barrier integrity (99, 100). However, in a mouse model of ulcerative colitis with loss of the intestinal barrier, symptoms have been reported to exacerbate in mice lacking TLR4 of the LPS receptor, and removal of the intestinal microbiota by antibiotic cocktail administration exacerbates ulcerative colitis symptoms, whereas oral LPS administration improves symptoms (103). In other words, LPS in the intestinal tract suppresses enteritis. These findings indicate that the current common knowledge that LPS is responsible for chronic inflammation caused by a leaky gut is open to reconsideration.
As discussed thus far, lipopolysaccharides (LPS) derived from symbiotic bacteria present in the environment or on mucosal surfaces are believed to exert beneficial effects on the innate immune system, primarily through macrophage activation, thereby contributing to the maintenance of individual health. Specifically, LPS is thought to play a crucial role in sustaining and enhancing self-healing ability. However, in the modern era, reduced environmental exposure to LPS and the widespread use of antibiotics have led to a situation in which the intentional intake of LPS via mucosal surfaces is actively promoted. Several studies have investigated the role of oral administration of LPS in activating tissue macrophages and inducing prevention and treatment effects (6, 8, 104–106).
The mechanism of action of orally administered LPS leading to self-healing remains unclear. However, the innate immune system may increase its responsiveness to foreign body elimination through epigenetic regulation triggered by activation. The potentiating effect of BCG on the secondary stimulation response of macrophages, which are cells of the innate immune system, is driven by epigenetic regulation and the acquisition of resistance to SARS-CoV2 (107). Similar epigenetic regulation occurs in the phenomenon where stimulation of the LPS receptor TLR4 enhances NF-κB reactivity and drives innate immune system training (108). Such phenomena by LPS have been reported in self-healing related macrophages (6, 8, 104–106, 109), mast cells (110), microglia (110, 111), neutrophils (112), and monocytes (113).
The preventive and therapeutic effects of oral administration of LPS on various diseases have been reported in animal and human intervention studies, including reduction or synergistic effect of anti-cancer drugs (114–118), promotion of skin ulcer healing (human intractable skin wounds) (119), promotion of skin ulcer healing (human intractable skin wounds) (119), influenza virus sublingual vaccine adjuvant effect (suppression of influenza infection deaths) (120), suppression of deaths due to toxoplasma infection (121), suppression of plateau reactivity of type I allergy (suppression of IgE-dependent allergic reactions) (8, 122), amelioration of high-fat diet-induced hyperlipidemia (suppression of atherosclerosis) in ApoE-deficient mice (123), suppression of salt-induced blood pressure increase in rats (124), improvement of diabetes and increased adiponectin induction in KK-Ay mice (102), and hair growth (hair growth promotion) (125). Recent studies have reported that the oral administration of LPS promotes the removal of foreign substances by microglia and prevents Alzheimer’s disease (65, 123, 126). Additionally, clinical studies have shown that it is effective in cancer (cancer shrinkage) (127), atopy (improved remission) (128), diabetes (improved markers) (129, 130), capillary dilation (increased) (131), wound healing (accelerated healing of intractable wounds) (132), and developmental disorders (improved) (133) (Figure 2).
Figure 2. The various diseases and conditions which from oral and/or transdermal administration of LPS and its effects as observed by animal experiments and human clinical studies. RCT, randomized clinical trial; LDL/TG, low-density lipopolyprotein/triglyceride.
Kobayashi et al. reported that the oral administration of LPS improved learning function assessed using the Morris water maze test in age-accelerated mice (SAM-P8) fed a high-fat diet as a model of Alzheimer’s disease (123). Additionally, it has been reported to enhance the phagocytic capacity of microglia, the so-called brain-resident macrophages, and induce a significant decrease in amyloid-β levels in the brain and an ameliorating effect on lifestyle-related diseases with significant decreases in diabetes markers, such as HbA1c, oral glucose tolerance test, and fasting blood glucose (134).
Dementia has long been thought to be caused by amyloid-β accumulating in the brain. However, meta-analysis studies have shown that this does not always translate into a therapeutic effect (135). Diabetes in the brain may be a major solitary dementia cause (136). Oral administration of LPS to mice with intracerebroventricular streptozotocin-induced diabetic dementia in the brain has been found to improve learning and memory performance and alter gene expression patterns in the microglia of brain tissue macrophages, exhibiting anti-inflammatory, tissue repair, and neuroprotective effects (65). Furthermore, in this model, learning and memory deficits improved when oral administration of LPS was initiated after cognitive decline, indicating a possible therapeutic effect (137).
Oral administration of LPS has been shown to reduce memory impairment due to dementia in the aging model and diabetes in the brain model. The mechanism underlying this effect involves the removal and repair of damaged neurons by microglia. These findings indicate that oral administration of LPS may be a therapeutic agent that, unlike conventional dementia drugs, repairs and regenerates brain damage via tissue macrophages to exert preventive and therapeutic effects.
Oral and nasal administration of LPS also plays a role in preventing infectious diseases. When mice were given free drinking administration of LPS and infected with Salmonella via intraperitoneal injection, the LPS treatment significantly prolonged survival (6). In addition, when mice were given LPS intranasally 3 to 1/2 days before infection with influenza virus, it significantly prevented death from influenza (138). Furthermore, sublingual administration of LPS significantly increases the production of immunoglobulin A in the mucosa of the influenza vaccine, and reduces mortality (120). Since influenza is a respiratory tract infection that is neutralized by immunoglobulin A in the respiratory tract mucus before it enters the body, it is possible to develop an effective sublingual vaccine.
Chronic inflammation caused by obesity is a significant factor in many lifestyle-related diseases, such as dementia, atherosclerosis, diabetes, and cancer. Oral administration of LPS has been reported to have the ability to prevent obesity. Yamamoto et al. reported that LPS intake in KK-Ay mice improved fasting blood glucose, insulin sensitivity, and HbA1c, and notably, in adipose tissue, adiponectin protein and gene expression significantly increased. However, no changes in inflammatory cytokines were observed (102). Adiponectin is an anti-inflammatory, anti-obesity cytokine produced by adipocytes (139), a phenomenon that is opposite to the fact that LPS is considered a diabetes-increasing factor (140, 141) and awaits further analysis.
The health-maintaining effects of LPS exposure may be analogous to the hormesis effects of radiation exposure. High-dose radiation is harmful to health, whereas low-dose radiation may contribute to health maintenance (142). In this light, the human body may possess a mechanism by which innate immune activation by environmental stimuli at levels where the response is temporarily undetectable is responsible for health maintenance, and the regulation of innate immune activation by oral administration of LPS may be one such system.
The skin is considered the second most important interface for interaction with symbiotic bacteria from the external environment after the intestinal tract. This section explores the role of lipopolysaccharides (LPS) in maintaining and enhancing self-healing ability, primarily through macrophage activation, when applied to the skin. LPS exhibits a high affinity for sebum within the stratum corneum. However, the passage of molecules larger than 500 Da, such as LPS, is restricted by tight junctions in the granular layer of the lower epidermis (143). Despite this barrier, LPS interacts with TLR4 expressed on Langerhans cells. These cells extend their dendrites beyond tight junctions, facilitating the binding of LPS to TLR4 (144). Langerhans cells, historically categorized as dendritic cells, have been reclassified as macrophages specific to skin tissue (145). These cells exhibit functional changes upon stimulation with LPS, including the downregulation of the allergic chemokine thymus-activation-regulated chemokine. This suppression of thymus-activation-regulated chemokine expression is associated with a reduction in allergic responses, highlighting the potential role of LPS-stimulated Langerhans cells in mitigating allergic inflammation (144). In the skin, TLR4 is expressed not only on Langerhans cells but also transiently on damaged epidermal keratinocytes within 24 h of injury. Stimulation with LPS has been reported to enhance the wound-healing capacity of epidermal cells (146). In vitro studies using human epidermal keratinocyte models (HaCaT cells) have shown that LPS promotes cell migration, a critical step in wound closure (147). Furthermore, similar effects of TLR4 activation by LPS have been observed in other epithelial tissues. Lung and corneal epithelial cells upregulate TLR4 expression in response to physical stimuli. LPS stimulation in these cells has been reported to promote wound healing, whereas blocking TLR4 signaling with specific inhibitors or antibodies delays healing (146, 147). These findings indicate that LPS directly influences epidermal and epithelial cells by activating TLR4, thereby enhancing their wound-healing properties.
LPS has been reported in cell studies to interact with keratinocytes at the apical side of tight junctions, inducing antimicrobial peptide production and enhancing the epidermal barrier by upregulating the expression of filaggrin and claudin-1, which are key components associated with tight junction integrity (148). In human intervention studies, LPS derived from Pantoea vagans has demonstrated additional benefits, including skin hypersensitivity suppression, increased stratum corneum hydration, and reduced transepidermal water loss, in individuals with sensitive skin (149). Similarly, a double-blind human intervention study has reported that applying LPS derived fromP. agglomerans to the skin significantly reduces the itching of mild atopic dermatitis and improves the skin condition (128). These findings indicate that transdermal application of LPS contributes to skin homeostasis by preventing and reducing skin disorders. Mechanistically, LPS enhances skin barrier function and regulates moisture balance, indicating its potential as a therapeutic agent for managing sensitive or compromised skin conditions. Additionally, activation of skin tissue by LPS may help prevent skin aging. It has been shown in cell models and ex-vivo models using human skin that senescent cells in the dermis are phagocytosed and removed via receptors such as STAB1 (phosphatidylserine receptor), CD36, and advanced glycation end-product receptor (18, 150). Macrophages activated by LPS exhibit increased phagocytic activity in co-culture condition, facilitating the removal of senescent cells and foreign substances (151). In vitro studies have shown that LPS-stimulated macrophages modulate the properties of primary human fibroblasts, enhancing cell proliferation, hyaluronic acid synthesis, and elastin expression. These findings indicate that transdermal administration of LPS activates dermal macrophages, thereby contributing to the removal of senescent cells and the prevention of skin aging. Furthermore, a recent study reported that the thinned epidermis of aging mice regained thickness compared with that of younger mice following LPS application (122).
Consequently, transdermal application of LPS supports skin homeostasis by improving epidermal barrier function, alleviating skin sensitivity, and promoting dermal repair mechanisms, resulting in enhancing self-healing ability. Additionally, LPS can prevent skin aging by activating macrophages, enhancing fibroblast function, and clearing senescent cells. These findings underscore the self-healing potential of LPS in addressing skin disorders and age-related changes in skin integrity.
In this review, we introduced the concept of the macrophage network as an innate immune mechanism responsible for health maintenance and natural healing. Although there have been many reports of LPS showing toxicity depending on the type and administration methods (152, 153), we will outline the latest findings and applications regarding the prevention and treatment effects of various diseases through oral and transdermal administration of LPS.
Humans are constantly exposed to the risk of infection by viral, bacterial, and other pathogens, such as coronaviruses that have emerged in the last few years. The human body eliminates emerging infectious pathogens mainly through innate immune function. However, if the body is continuously exposed to excessive stress levels, the innate immune system is weakened, the organism’s homeostasis is severely impaired, and the body loses its ability to resist emerging infectious pathogens (6, 154). Therefore, establishing measures to prevent the weakening of innate immunity is important (155–157). Further advances in our understanding of self-healing based on innate immune function can contribute to the development of effective disease prevention and treatment strategies, such as oral and transdermal administration of LPS.
Determining the state of innate immune function is necessary for assessing health and innate healing. To this end, a comprehensive index of innate immunity should be established, test methods should be identified, and assessment criteria should be developed. However, because innate immune responses are diverse and heterogeneous, focusing on a single group of cells is insufficient. The development of non-invasive, user-friendly methods to assess innate immune responses is an important challenge. Despite being challenging, further efforts should be made to design effective devices for the routine monitoring of innate immune function.
Even healthy individuals may be carriers of hidden premorbid conditions, such as pre-diabetes, hypertension, and dyslipidemia (124, 155). These diseases are associated with reduced innate immune function and impaired self-healing. Using pharmaceuticals to treat these supposedly healthy but disease-prevalent conditions is highly problematic. Therefore, proper functioning of the innate immune system is essential for achieving optimal health. Furthermore, in diseased individuals, restoring the innate immune function can maximize the effectiveness of medicinal products. The significance of maintaining innate immune function and using LPS is expected to get wider recognition in the coming years.
G-IS: Writing – review & editing. MO: Writing – original draft, Writing – review & editing. VT: Writing – review & editing. CK: Writing – review & editing. HI: Writing – original draft, Writing – review & editing.
The author(s) declare that financial support was received for the research and/or publication of this article. This study was funded by the Control of Innate Immunity Collaborative Innovation Partnership, the Cross-ministerial Strategic Innovation Promotion Program (SIP-No. 14533073) of the Council for Science from Technology and Innovation in the Cabinet Office of the Japanese Government and the National Agriculture and Food Research Organization, and JSPS KAKENHI (Grant No. 24K10213).
We gratefully acknowledge the work of past and present members of our laboratory.
The authors declare that the research was conducted in the absence of any commercial or financial relationships that could be construed as a potential conflict of interest.
The author(s) declare that no Generative AI was used in the creation of this manuscript.
All claims expressed in this article are solely those of the authors and do not necessarily represent those of their affiliated organizations, or those of the publisher, the editors and the reviewers. Any product that may be evaluated in this article, or claim that may be made by its manufacturer, is not guaranteed or endorsed by the publisher.
1. Beutler B. Tlr4: central component of the sole mammalian lps sensor. Curr Opin Immunol. (2000) 12:20–6. doi: 10.1016/s0952-7915(99)00046-1
2. Poltorak A, He X, Smirnova I, Liu MY, Van Huffel C, Du X, et al. Defective lps signaling in C3h/hej and C57bl/10sccr mice: mutations in tlr4 gene. Science. (1998) 282:2085–8. doi: 10.1126/science.282.5396.2085
3. Rock FL, Hardiman G, Timans JC, Kastelein RA, Bazan JF. A family of human receptors structurally related to drosophila toll. Proc Natl Acad Sci U.S.A. (1998) 95:588–93. doi: 10.1073/pnas.95.2.588
4. Deng H, Maitra U, Morris M, Li L. Molecular mechanism responsible for the priming of macrophage activation. J Biol Chem. (2013) 288:3897–906. doi: 10.1074/jbc.M112.424390
5. Mohammad S, Thiemermann C. Role of metabolic endotoxemia in systemic inflammation and potential interventions. Front Immunol. (2020) 11:594150. doi: 10.3389/fimmu.2020.594150
6. Gen-Ichiro S, Hiroyuki I, Takashi N, Noriko Y, Yoshie T, Chie K. Preventative and therapeutic potential of lipopolysaccharide derived from edible gram-negative bacteria to various diseases. Curr Drug Ther. (2008) 3:26–32. doi: 10.2174/157488508783331243
7. Kohchi C, Inagawa H, Hino M, Oda M, Nakata K, Yoshida AYA, et al. Utilization of macrophages in anticancer therapy: the macrophage network theory. Anticancer Res. (2004) 24:3311.
8. Kohchi C, Inagawa H, Nishizawa T, Yamaguchi T, Nagai S, Soma G. Applications of lipopolysaccharide derived from pantoea agglomerans (Ip-pa1) for health care based on macrophage network theory. J Biosci Bioeng. (2006) 102:485–96. doi: 10.1263/jbb.102.485
9. Honda T, Inagawa H. Utility of in vitro cellular models of low-dose lipopolysaccharide in elucidating the mechanisms of anti-inflammatory and wound-healing-promoting effects of lipopolysaccharide administration in vivo. Int J Mol Sci. (2023) 24:14387. doi: 10.3390/ijms241814387
10. Dutkiewicz J, Mackiewicz B, Lemieszek MK, Golec M, Milanowski J. Pantoea agglomerans: A mysterious bacterium of evil and good. Part iv. Beneficial effects. Ann Agric Environ Med. (2016) 23:206–22. doi: 10.5604/12321966.1203879
11. Shafqat A, Khan JA, Alkachem AY, Sabur H, Alkattan K, Yaqinuddin A, et al. How neutrophils shape the immune response: reassessing their multifaceted role in health and disease. Int J Mol Sci. (2023) 24:17583. doi: 10.3390/ijms242417583
12. Chen L, Deng H, Cui H, Fang J, Zuo Z, Deng J, et al. Inflammatory responses and inflammation-associated diseases in organs. Oncotarget. (2018) 9:7204–18. doi: 10.18632/oncotarget.23208
13. Wynn TA, Vannella KM. Macrophages in tissue repair, regeneration, and fibrosis. Immunity. (2016) 44:450–62. doi: 10.1016/j.immuni.2016.02.015
14. Metchnikoff É. Leçons sur la pathologie comparée de L'inflammation. LIBRAIRE L'ACADÉMIE MÉDECINE. (1892).
15. Steven JL. Metchnikoff on the comparative pathology of inflammation. Glasgow Med J. (1892) 38:195–205.
16. Gordon S. Elie metchnikoff, the man and the myth. J Innate Immun. (2016) 8:223–7. doi: 10.1159/000443331
17. Kirkland TN, Fierer J. Innate immune receptors and defense against primary pathogenic fungi. Vaccines (Basel). (2020) 8:303. doi: 10.3390/vaccines8020303
18. Li X, Yang K, Gao S, Zhao J, Liu G, Chen Y, et al. Carnosine stimulates macrophage-mediated clearance of senescent skin cells through activation of the akt2 signaling pathway by cd36 and rage. Front Pharmacol. (2020) 11:593832. doi: 10.3389/fphar.2020.593832
19. Kale A, Sharma A, Stolzing A, Desprez PY, Campisi J. Role of immune cells in the removal of deleterious senescent cells. Immun Ageing. (2020) 17:16. doi: 10.1186/s12979-020-00187-9
20. McCullough JL, Kelly KM. Prevention and treatment of skin aging. Ann N Y Acad Sci. (2006) 1067:323–31. doi: 10.1196/annals.1354.044
21. McHugh D, Gil J. Senescence and aging: causes, consequences, and therapeutic avenues. J Cell Biol. (2018) 217:65–77. doi: 10.1083/jcb.201708092
22. Medzhitov R. Origin and physiological roles of inflammation. Nature. (2008) 454:428–35. doi: 10.1038/nature07201
23. Giamarellos-Bourboulis EJ, Tsilika M, Moorlag S, Antonakos N, Kotsaki A, Domínguez-Andrés J, et al. Activate: randomized clinical trial of bcg vaccination against infection in the elderly. Cell. (2020) 183:315–23.e9. doi: 10.1016/j.cell.2020.08.051
24. Tsilika M, Taks E, Dolianitis K, Kotsaki A, Leventogiannis K, Damoulari C, et al. Corrigendum: Activate-2: A Double-Blind Randomized Trial of Bcg Vaccination against Covid-19 in Individuals at Risk. Front Immunol. (2022) 13:1018384. doi: 10.3389/fimmu.2022.1018384
25. Saeed S, Quintin J, Kerstens HH, Rao NA, Aghajanirefah A, Matarese F, et al. Epigenetic programming of monocyte-to-macrophage differentiation and trained innate immunity. Science. (2014) 345:1251086. doi: 10.1126/science.1251086
26. Netea MG, Quintin J, van der Meer JW. Trained immunity: A memory for innate host defense. Cell Host Microbe. (2011) 9:355–61. doi: 10.1016/j.chom.2011.04.006
27. Kocarnik JM, Compton K, Dean FE, Fu W, Gaw BL, Harvey JD, et al. Cancer incidence, mortality, years of life lost, years lived with disability, and disability-adjusted life years for 29 cancer groups from 2010 to 2019: A systematic analysis for the global burden of disease study 2019. JAMA Oncol. (2022) 8:420–44. doi: 10.1001/jamaoncol.2021.6987
28. Liu J, Ren ZH, Qiang H, Wu J, Shen M, Zhang L, et al. Trends in the incidence of diabetes mellitus: results from the global burden of disease study 2017 and implications for diabetes mellitus prevention. BMC Public Health. (2020) 20:1415. doi: 10.1186/s12889-020-09502-x
29. Li X, Feng X, Sun X, Hou N, Han F, Liu Y. Global, regional, and national burden of alzheimer's disease and other dementias, 1990-2019. Front Aging Neurosci. (2022) 14:937486. doi: 10.3389/fnagi.2022.937486
30. Linehan E, Fitzgerald DC. Ageing and the immune system: focus on macrophages. Eur J Microbiol Immunol (Bp). (2015) 5:14–24. doi: 10.1556/eujmi-d-14-00035
31. Mass E, Nimmerjahn F, Kierdorf K, Schlitzer A. Tissue-specific macrophages: how they develop and choreograph tissue biology. Nat Rev Immunol. (2023) 23:563–79. doi: 10.1038/s41577-023-00848-y
32. Renz H, Blümer N, Virna S, Sel S, Garn H. The immunological basis of the hygiene hypothesis. Chem Immunol Allergy. (2006) 91:30–48. doi: 10.1159/000090228
33. Pfefferle PI, Keber CU, Cohen RM, Garn H. The hygiene hypothesis - learning from but not living in the past. Front Immunol. (2021) 12:635935. doi: 10.3389/fimmu.2021.635935
34. Braun-Fahrländer C, Riedler J, Herz U, Eder W, Waser M, Grize L, et al. Environmental exposure to endotoxin and its relation to asthma in school-age children. N Engl J Med. (2002) 347:869–77. doi: 10.1056/NEJMoa020057
35. Mirza R, DiPietro LA, Koh TJ. Selective and specific macrophage ablation is detrimental to wound healing in mice. Am J Pathol. (2009) 175:2454–62. doi: 10.2353/ajpath.2009.090248
36. Ingman WV, Wyckoff J, Gouon-Evans V, Condeelis J, Pollard JW. Macrophages promote collagen fibrillogenesis around terminal end buds of the developing mammary gland. Dev Dyn. (2006) 235:3222–9. doi: 10.1002/dvdy.20972
37. Mantovani A, Biswas SK, Galdiero MR, Sica A, Locati M. Macrophage plasticity and polarization in tissue repair and remodelling. J Pathol. (2013) 229:176–85. doi: 10.1002/path.4133
38. Shapouri-Moghaddam A, Mohammadian S, Vazini H, Taghadosi M, Esmaeili SA, Mardani F, et al. Macrophage plasticity, polarization, and function in health and disease. J Cell Physiol. (2018) 233:6425–40. doi: 10.1002/jcp.26429
39. Tsai CF, Chen GW, Chen YC, Shen CK, Lu DY, Yang LY, et al. Regulatory effects of quercetin on M1/M2 macrophage polarization and oxidative/antioxidative balance. Nutrients. (2021) 14:67. doi: 10.3390/nu14010067
40. Sica A, Mantovani A. Macrophage plasticity and polarization: in vivo veritas. J Clin Invest. (2012) 122:787–95. doi: 10.1172/jci59643
41. Schwartz M, Shechter R. Systemic inflammatory cells fight off neurodegenerative disease. Nat Rev Neurol. (2010) 6:405–10. doi: 10.1038/nrneurol.2010.71
42. Bocciarelli C, Cordel N, Leschiera R, Talagas M, Le-Gall-Ianotto C, Hu W, et al. New human in vitro co-culture model of keratinocytes and sensory neurons like cells releasing substance P with an evaluation of the expression of zikv entry receptors: A potent opportunity to test zika virus entry and to study zika virus' Infection in neurons? Exp Dermatol. (2023) 32:1563–8. doi: 10.1111/exd.14870
43. Zhang J, Hu D, Li L, Qu D, Shi W, Xie L, et al. M2 microglia-derived exosomes promote spinal cord injury recovery in mice by alleviating A1 astrocyte activation. Mol Neurobiol. (2024) 61:7009–25. doi: 10.1007/s12035-024-04026-6
44. Shiraishi W, Yamasaki R, Hashimoto Y, Ko S, Kobayakawa Y, Isobe N, et al. Clearance of peripheral nerve misfolded mutant protein by infiltrated macrophages correlates with motor neuron disease progression. Sci Rep. (2021) 11:16438. doi: 10.1038/s41598-021-96064-6
45. Watanabe Y, Tsuchiya A, Seino S, Kawata Y, Kojima Y, Ikarashi S, et al. Mesenchymal stem cells and induced bone marrow-derived macrophages synergistically improve liver fibrosis in mice. Stem Cells Transl Med. (2019) 8:271–84. doi: 10.1002/sctm.18-0105
46. Gordon S, Martinez FO. Alternative activation of macrophages: mechanism and functions. Immunity. (2010) 32:593–604. doi: 10.1016/j.immuni.2010.05.007
47. Murray PJ, Wynn TA. Obstacles and opportunities for understanding macrophage polarization. J Leukoc Biol. (2011) 89:557–63. doi: 10.1189/jlb.0710409
48. Wynn TA, Chawla A, Pollard JW. Macrophage biology in development, homeostasis and disease. Nature. (2013) 496:445–55. doi: 10.1038/nature12034
49. Rapalino O, Lazarov-Spiegler O, Agranov E, Velan GJ, Yoles E, Fraidakis M, et al. Implantation of stimulated homologous macrophages results in partial recovery of paraplegic rats. Nat Med. (1998) 4:814–21. doi: 10.1038/nm0798-814
50. Knoller N, Auerbach G, Fulga V, Zelig G, Attias J, Bakimer R, et al. Clinical experience using incubated autologous macrophages as a treatment for complete spinal cord injury: phase I study results. J Neurosurg Spine. (2005) 3:173–81. doi: 10.3171/spi.2005.3.3.0173
51. Sender R, Weiss Y, Navon Y, Milo I, Azulay N, Keren L, et al. The total mass, number, and distribution of immune cells in the human body. Proc Natl Acad Sci U.S.A. (2023) 120:e2308511120. doi: 10.1073/pnas.2308511120
52. Tiemeijer BM, Heester S, Sturtewagen AYW, Smits A, Tel J. Single-cell analysis reveals tlr-induced macrophage heterogeneity and quorum sensing dictate population wide anti-inflammatory feedback in response to lps. Front Immunol. (2023) 14:1135223. doi: 10.3389/fimmu.2023.1135223
53. Nicolás-Ávila JA, Lechuga-Vieco AV, Esteban-Martínez L, Sánchez-Díaz M, Díaz-García E, Santiago DJ, et al. A network of macrophages supports mitochondrial homeostasis in the heart. Cell. (2020) 183:94–109.e23. doi: 10.1016/j.cell.2020.08.031
54. Brestoff JR, Wilen CB, Moley JR, Li Y, Zou W, Malvin NP, et al. Intercellular mitochondria transfer to macrophages regulates white adipose tissue homeostasis and is impaired in obesity. Cell Metab. (2021) 33:270–82.e8. doi: 10.1016/j.cmet.2020.11.008
55. Paterson N, Lämmermann T. Macrophage network dynamics depend on haptokinesis for optimal local surveillance. Elife. (2022) 11:e75354. doi: 10.7554/eLife.75354
56. Cebrian E, Uriz MJ, Turon X. Sponges as biomonitors of heavy metals in spatial and temporal surveys in northwestern mediterranean: multispecies comparison. Environ Toxicol Chem. (2007) 26:2430–9. doi: 10.1897/07-292.1
57. Armingol E, Officer A, Harismendy O, Lewis NE. Deciphering cell-cell interactions and communication from gene expression. Nat Rev Genet. (2021) 22:71–88. doi: 10.1038/s41576-020-00292-x
58. Zhang J, Yang Y, Zhou S, He X, Cao X, Wu C, et al. Membrane-bound tnf mediates microtubule-targeting chemotherapeutics-induced cancer cytolysis via juxtacrine inter-cancer-cell death signaling. Cell Death Differ. (2020) 27:1569–87. doi: 10.1038/s41418-019-0441-3
59. Sasu S, Cooper AL, Beasley D. Juxtacrine effects of il-1 alpha precursor promote inos expression in vascular smooth muscle cells. Am J Physiol Heart Circ Physiol. (2001) 280:H1615–23. doi: 10.1152/ajpheart.2001.280.4.H1615
60. Hill LL, Shreedhar VK, Kripke ML, Owen-Schaub LB. A critical role for fas ligand in the active suppression of systemic immune responses by ultraviolet radiation. J Exp Med. (1999) 189:1285–94. doi: 10.1084/jem.189.8.1285
61. Nair PM, Ngu H, Torres E, Marsters S, Lawrence DA, Stephan JP, et al. Membrane display and functional analysis of juxtacrine ligand-receptor signaling. Biotechniques. (2015) 59:231–8. doi: 10.2144/000114342
62. Mazzoldi EL, Pavan S, Pilotto G, Leone K, Pagotto A, Frezzini S, et al. A juxtacrine/paracrine loop between C-kit and stem cell factor promotes cancer stem cell survival in epithelial ovarian cancer. Cell Death Dis. (2019) 10:412. doi: 10.1038/s41419-019-1656-4
63. Bush MR, Mele JM, Couchman GM, Walmer DK. Evidence of juxtacrine signaling for transforming growth factor alpha in human endometrium. Biol Reprod. (1998) 59:1522–9. doi: 10.1095/biolreprod59.6.1522
64. Fujiu K, Shibata M, Nakayama Y, Ogata F, Matsumoto S, Noshita K, et al. A heart-brain-kidney network controls adaptation to cardiac stress through tissue macrophage activation. Nat Med. (2017) 23:611–22. doi: 10.1038/nm.4326
65. Mizobuchi H, Yamamoto K, Yamashita M, Nakata Y, Inagawa H, Kohchi C, et al. Prevention of diabetes-associated cognitive dysfunction through oral administration of lipopolysaccharide derived from pantoea agglomerans. Front Immunol. (2021) 12:650176. doi: 10.3389/fimmu.2021.650176
66. Muller PA, Koscsó B, Rajani GM, Stevanovic K, Berres ML, Hashimoto D, et al. Crosstalk between muscularis macrophages and enteric neurons regulates gastrointestinal motility. Cell. (2014) 158:300–13. doi: 10.1016/j.cell.2014.04.050
67. Anitha M, Vijay-Kumar M, Sitaraman SV, Gewirtz AT, Srinivasan S. Gut microbial products regulate murine gastrointestinal motility via toll-like receptor 4 signaling. Gastroenterology. (2012) 143:1006–16.e4. doi: 10.1053/j.gastro.2012.06.034
68. Mantovani A, Sozzani S, Locati M, Allavena P, Sica A. Macrophage polarization: tumor-associated macrophages as a paradigm for polarized M2 mononuclear phagocytes. Trends Immunol. (2002) 23:549–55. doi: 10.1016/s1471-4906(02)02302-5
69. Ying W, Fu W, Lee YS, Olefsky JM. The role of macrophages in obesity-associated islet inflammation and B-cell abnormalities. Nat Rev Endocrinol. (2020) 16:81–90. doi: 10.1038/s41574-019-0286-3
70. Parri M, Ippolito L, Cirri P, Ramazzotti M, Chiarugi P. Metabolic cell communication within tumour microenvironment: models, methods and perspectives. Curr Opin Biotechnol. (2020) 63:210–9. doi: 10.1016/j.copbio.2020.03.001
71. Najafi M, Hashemi Goradel N, Farhood B, Salehi E, Nashtaei MS, Khanlarkhani N, et al. Macrophage polarity in cancer: A review. J Cell Biochem. (2019) 120:2756–65. doi: 10.1002/jcb.27646
72. Mammana S, Fagone P, Cavalli E, Basile MS, Petralia MC, Nicoletti F, et al. The role of macrophages in neuroinflammatory and neurodegenerative pathways of alzheimer's disease, amyotrophic lateral sclerosis, and multiple sclerosis: pathogenetic cellular effectors and potential therapeutic targets. Int J Mol Sci. (2018) 19:831. doi: 10.3390/ijms19030831
73. Hampton HR, Chtanova T. Lymphatic migration of immune cells. Front Immunol. (2019) 10:1168. doi: 10.3389/fimmu.2019.01168
74. Vandenbulcke L, Bachert C, Van Cauwenberge P, Claeys S. The innate immune system and its role in allergic disorders. Int Arch Allergy Immunol. (2006) 139:159–65. doi: 10.1159/000090393
75. Dagenais A, Villalba-Guerrero C, Olivier M. Trained immunity: A "New" Weapon in the fight against infectious diseases. Front Immunol. (2023) 14:1147476. doi: 10.3389/fimmu.2023.1147476
76. Katoh N, Ohya Y, Ikeda M, Ebihara T, Katayama I, Saeki H, et al. Japanese guidelines for atopic dermatitis 2020. Allergol Int. (2020) 69:356–69. doi: 10.1016/j.alit.2020.02.006
77. Devereux G, Matsui EC, Burney PGJ. Epidemiology of asthma and allergic airway diseases. Middleton's Allergy. (2014), 754–89. doi: 10.1016/b978-0-323-08593-9.00049-8
78. von Mutius E. The "Hygiene hypothesis" and the lessons learnt from farm studies. Front Immunol. (2021) 12:635522. doi: 10.3389/fimmu.2021.635522
79. Morcos MM, Morcos WM, Ibrahim MA, Shaheen MA. Environmental exposure to endotoxin in rural and urban Egyptian school children and its relation to asthma and atopy. Minerva Pediatr. (2011) 63:19–26.
80. Vatanen T, Kostic AD, d'Hennezel E, Siljander H, Franzosa EA, Yassour M, et al. Variation in microbiome lps immunogenicity contributes to autoimmunity in humans. Cell. (2016) 165:842–53. doi: 10.1016/j.cell.2016.04.007
81. Rhee SH. Lipopolysaccharide: basic biochemistry, intracellular signaling, and physiological impacts in the gut. Intest Res. (2014) 12:90–5. doi: 10.5217/ir.2014.12.2.90
82. Karki R, Kanneganti TD. The 'Cytokine storm': molecular mechanisms and therapeutic prospects. Trends Immunol. (2021) 42:681–705. doi: 10.1016/j.it.2021.06.001
83. Bashir ME, Louie S, Shi HN, Nagler-Anderson C. Toll-like receptor 4 signaling by intestinal microbes influences susceptibility to food allergy. J Immunol. (2004) 172:6978–87. doi: 10.4049/jimmunol.172.11.6978
84. Vazquez-Torres A, Vallance BA, Bergman MA, Finlay BB, Cookson BT, Jones-Carson J, et al. Toll-like receptor 4 dependence of innate and adaptive immunity to salmonella: importance of the kupffer cell network. J Immunol. (2004) 172:6202–8. doi: 10.4049/jimmunol.172.10.6202
85. Inagawa H, Kobayashi Y, Kohchi C, Zhang R, Shibasaki Y, Soma G. Primed activation of macrophages by oral administration of lipopolysaccharide derived from pantoea agglomerans. In Vivo. (2016) 30:205–11.
86. Nicolaou N, Siddique N, Custovic A. Allergic disease in urban and rural populations: increasing prevalence with increasing urbanization. Allergy. (2005) 60:1357–60. doi: 10.1111/j.1398-9995.2005.00961.x
87. Yoshida A, Kohchi C, Inagawa H, Nishizawa T, Soma G. Improvement of allergic dermatitis via regulation of the th1/th2 immune system balance by macrophages activated with lipopolysaccharide derived from pantoea agglomerans (Ip-pa1). Anticancer Res. (2009) 29:4867–70.
88. Mohr AE, Crawford M, Jasbi P, Fessler S, Sweazea KL. Lipopolysaccharide and the gut microbiota: considering structural variation. FEBS Lett. (2022) 596:849–75. doi: 10.1002/1873-3468.14328
89. Stefka AT, Feehley T, Tripathi P, Qiu J, McCoy K, Mazmanian SK, et al. Commensal bacteria protect against food allergen sensitization. Proc Natl Acad Sci U.S.A. (2014) 111:13145–50. doi: 10.1073/pnas.1412008111
90. Brandl K, Plitas G, Mihu CN, Ubeda C, Jia T, Fleisher M, et al. Vancomycin-resistant enterococci exploit antibiotic-induced innate immune deficits. Nature. (2008) 455:804–7. doi: 10.1038/nature07250
91. Farooqi IS, Hopkin JM. Early childhood infection and atopic disorder. Thorax. (1998) 53:927–32. doi: 10.1136/thx.53.11.927
92. Kohchi C, Uehiro M, Fukaya T, Watanabe N, Inagawa H, Soma G-I. Pantoea agglomerans lipopolysaccharide controls nasal discomfort—a placebo-controlled, randomized, double-blind, parallel-group comparison trial. Int J Trans Med. (2024) 4:126–39. doi: 10.3390/ijtm4010006
93. Inagawa H, Saika T, Nisizawa T, Kohchi C, Uenobe M, Soma G. Dewaxed brown rice contains a significant amount of lipopolysaccharide pointing to macrophage activation via tlrs. Anticancer Res. (2016) 36:3599–605.
94. Tjendana Tjhin V, Oda M, Yamashita M, Iwaki T, Fujita Y, Wakame K, et al. Baseline data collections of lipopolysaccharide content in 414 herbal extracts and its role in innate immune activation. Sci Rep. (2024) 14:15394. doi: 10.1038/s41598-024-66081-2
95. Bakker PA, Berendsen RL, Doornbos RF, Wintermans PC, Pieterse CM. The rhizosphere revisited: root microbiomics. Front Plant Sci. (2013) 4:165. doi: 10.3389/fpls.2013.00165
96. Timofeeva AM, Galyamova MR, Sedykh SE. Plant growth-promoting soil bacteria: nitrogen fixation, phosphate solubilization, siderophore production, and other biological activities. Plants (Basel). (2023) 12:4074. doi: 10.3390/plants12244074
97. Phipps KR, Sulaiman C, Simon R, Holalagoudar S, Kohchi C, Nakata Y. Subchronic (90-day) toxicity assessment of somacy-fp100, a lipopolysaccharide-containing fermented wheat flour extract from pantoea agglomerans. J Appl Toxicol. (2020) 40:1342–52. doi: 10.1002/jat.3987
98. Taniguchi Y, Yoshioka N, Nishizawa T, Inagawa H, Kohchi C, Soma G. Utility and safety of lps-based fermented flour extract as a macrophage activator. Anticancer Res. (2009) 29:859–64.
99. Pussinen PJ, Havulinna AS, Lehto M, Sundvall J, Salomaa V. Endotoxemia is associated with an increased risk of incident diabetes. Diabetes Care. (2011) 34:392–7. doi: 10.2337/dc10-1676
100. Violi F, Cammisotto V, Bartimoccia S, Pignatelli P, Carnevale R, Nocella C. Gut-derived low-grade endotoxaemia, atherothrombosis and cardiovascular disease. Nat Rev Cardiol. (2023) 20:24–37. doi: 10.1038/s41569-022-00737-2
101. Ghoshal S, Witta J, Zhong J, de Villiers W, Eckhardt E. Chylomicrons promote intestinal absorption of lipopolysaccharides. J Lipid Res. (2009) 50:90–7. doi: 10.1194/jlr.M800156-JLR200
102. Yamamoto K, Yamashita M, Oda M, Tjendana Tjhin V, Inagawa H, Soma GI. Oral administration of lipopolysaccharide enhances insulin signaling-related factors in the kk/ay mouse model of type 2 diabetes mellitus. Int J Mol Sci. (2023) 24:4619. doi: 10.3390/ijms24054619
103. Rakoff-Nahoum S, Paglino J, Eslami-Varzaneh F, Edberg S, Medzhitov R. Recognition of commensal microflora by toll-like receptors is required for intestinal homeostasis. Cell. (2004) 118:229–41. doi: 10.1016/j.cell.2004.07.002
104. Inagawa H, Kohchi C, Soma G. Oral administration of lipopolysaccharides for the prevention of various diseases: benefit and usefulness. Anticancer Res. (2011) 31:2431–6.
105. Murray PJ, Allen JE, Biswas SK, Fisher EA, Gilroy DW, Goerdt S, et al. Macrophage activation and polarization: nomenclature and experimental guidelines. Immunity. (2014) 41:14–20. doi: 10.1016/j.immuni.2014.06.008
106. Soma G, Inagawa H. Methods to prevent or treat refractory diseases by focusing on intestinal microbes using lps and macrophages. Anticancer Res. (2015) 35:4393–6.
107. Netea MG, Giamarellos-Bourboulis EJ, Domínguez-Andrés J, Curtis N, van Crevel R, van de Veerdonk FL, et al. Trained immunity: A tool for reducing susceptibility to and the severity of sars-cov-2 infection. Cell. (2020) 181:969–77. doi: 10.1016/j.cell.2020.04.042
108. Chen S, Yang J, Wei Y, Wei X. Epigenetic regulation of macrophages: from homeostasis maintenance to host defense. Cell Mol Immunol. (2020) 17:36–49. doi: 10.1038/s41423-019-0315-0
109. Rusek P, Wala M, Druszczyńska M, Fol M. Infectious agents as stimuli of trained innate immunity. Int J Mol Sci. (2018) 19:456. doi: 10.3390/ijms19020456
110. De Zuani M, Dal Secco C, Tonon S, Arzese A, Pucillo CEM, Frossi B. Lps guides distinct patterns of training and tolerance in mast cells. Front Immunol. (2022) 13:835348. doi: 10.3389/fimmu.2022.835348
111. Lajqi T, Lang GP, Haas F, Williams DL, Hudalla H, Bauer M, et al. Memory-like inflammatory responses of microglia to rising doses of lps: key role of pi3kγ. Front Immunol. (2019) 10:2492. doi: 10.3389/fimmu.2019.02492
112. Lajqi T, Braun M, Kranig SA, Frommhold D, Pöschl J, Hudalla H. LPS induces opposing memory-like inflammatory responses in mouse bone marrow neutrophils. Int J Mol Sci. (2021) 22:9803. doi: 10.3390/ijms22189803
113. Yuan R, Geng S, Li L. Molecular mechanisms that underlie the dynamic adaptation of innate monocyte memory to varying stimulant strength of tlr ligands. Front Immunol. (2016) 7:497. doi: 10.3389/fimmu.2016.00497
114. Honda T, Inagawa H, Yamamoto I. Expression of chemotaxis- and angiogenesis-related factors in human monocytes following interaction with colon cancer cells is suppressed by low-dose lipopolysaccharide. Anticancer Res. (2014) 34:4609–13.
115. Honda T, Yamamoto I, Inagawa H. Angiogenesis-, metastasis- and signaling pathway-related factor dynamics in human colon cancer cells following interaction with monocytes. Anticancer Res. (2013) 33:2895–900.
116. Honda T, Inagawa H, Yamamoto I. Differential expression of mrna in human monocytes following interaction with human colon cancer cells. Anticancer Res. (2011) 31:2493–7.
117. Hino M, Kohchi C, Nishizawa T, Yoshida A, Nakata K, Inagawa H, et al. Innate-immune therapy for lung carcinoma based on tissue-macrophage activation with lipopolysaccharide. Anticancer Res. (2005) 25:3747–54.
118. Ohno S, Inagawa H, Dhar DK, Fujii T, Ueda S, Tachibana M, et al. The degree of macrophage infiltration into the cancer cell nest is a significant predictor of survival in gastric cancer patients. Anticancer Res. (2003) 23:5015–22.
119. Inagawa H, Saitoh F, Iguchi M, Nishizawa T, Okutomi T, Morikawa A, et al. Homeostasis as regulated by activated macrophage. Iii. Protective effect of lpsw (Lipopolysaccharide (Lps) of wheat flour) on gastric ulcer in mice as compared with those of other lps from various sources. Chem Pharm Bull (Tokyo). (1992) 40:998–1000. doi: 10.1248/cpb.40.998
120. Fukasaka M, Asari D, Kiyotoh E, Okazaki A, Gomi Y, Tanimoto T, et al. A lipopolysaccharide from pantoea agglomerans is a promising adjuvant for sublingual vaccines to induce systemic and mucosal immune responses in mice via tlr4 pathway. PloS One. (2015) 10:e0126849. doi: 10.1371/journal.pone.0126849
121. Suzuki Y, Kobayashi A, Nishizawa T, Inagawa H, Morikawa A, Soma G, et al. Homeostasis as regulated by activated macrophage. Vi. Protective effect of lpsw (a lipopolysaccharide from wheat flour) against acute infection by toxoplasma gondii in mice. Chem Pharm Bull (Tokyo). (1992) 40:1266–7. doi: 10.1248/cpb.40.1266
122. Tanaka M, Kohchi C, Inagawa H, Ikemoto T, Hara-Chikuma M. Effect of topical application of lipopolysaccharide on contact hypersensitivity. Biochem Biophys Res Commun. (2022) 586:100–6. doi: 10.1016/j.bbrc.2021.11.045
123. Kobayashi Y, Inagawa H, Kohchi C, Kazumura K, Tsuchiya H, Miwa T, et al. Oral administration of pantoea agglomerans-derived lipopolysaccharide prevents metabolic dysfunction and alzheimer's disease-related memory loss in senescence-accelerated prone 8 (Samp8) mice fed a high-fat diet. PloS One. (2018) 13:e0198493. doi: 10.1371/journal.pone.0198493
124. Zhang R, Inagawa H, Uchibori S, Masaki T, Kobara H, Soma GI. Preventive effect of oral administration of pantoea agglomerans-derived lps in a hypertensive rat model upon salt loading. Anticancer Res. (2019) 39:4503–9. doi: 10.21873/anticanres.13626
125. Wakame K, Okawa H, Komatsu KI, Nakata A, Sato K, Ingawa H, et al. Immunopotentiator from pantoea agglomerans 1 (Ip-pa1) promotes murine hair growth and human dermal papilla cell gene expression. Anticancer Res. (2016) 36:3687–92.
126. Uenobe M, Saika T, Waku N, Ohno M, Inagawa H. Efficacy of continuous ingestion of dewaxed brown rice on the cognitive functions of the residents of elderly welfare facilities: A pilot test using crossover trial. Food Sci Nutr. (2019) 7:3520–6. doi: 10.1002/fsn3.1202
127. Morishima A, Inagawa H. Clinical effects of orally administered lipopolysaccharide derived from pantoea agglomerans on Malignant tumors. Anticancer Res. (2016) 36:3747–51.
128. Nakai K, Kubota Y, Soma GI, Kohchi C. The effect of lipopolysaccharide-containing moisturizing cream on skin care in patients with mild atopic dermatitis. In Vivo. (2019) 33:109–14. doi: 10.21873/invivo.11446
129. Iguchi M, Inagawa H, Nishizawa T, Okutomi T, Morikawa A, Soma GI, et al. Homeostasis as regulated by activated macrophage. V. Suppression of diabetes mellitus in non-obese diabetic mice by lpsw (a lipopolysaccharide from wheat flour). Chem Pharm Bull (Tokyo). (1992) 40:1004–6. doi: 10.1248/cpb.40.1004
130. Nakata K, Taniguchi Y, Yoshioka N, Yoshida A, Inagawa H, Nakamoto T, et al. A mixture of salacia oblonga extract and ip-pa1 reduces fasting plasma glucose (Fpg) and low-density lipoprotein (Ldl) cholesterol levels. Nutr Res Pract. (2011) 5:435–42. doi: 10.4162/nrp.2011.5.5.435
131. Nakata Y, Kohchi C, Ogawa K, Nakamoto T, Yoshimura H, Soma GI. Effects of 3 months continuous intake of supplement containing pantoea agglomerans lps to maintain normal bloodstream in adults: parallel double-blind randomized controlled study. Food Sci Nutr. (2018) 6:197–206. doi: 10.1002/fsn3.547
132. Morishima A, Inagawa H. Improvement in protracted wound healing by topical cream containing lipopolysaccharide derived from pantoea agglomerans. Anticancer Res. (2018) 38:4375–9. doi: 10.21873/anticanres.12739
133. Morishima A, Zhang R, Nagaoka T, Inagawa H. Useful cases of patients with developmental disorders improved by oral administration of lps derived from pantoea agglomerans. Anticancer Res. (2020) 40:4755–62. doi: 10.21873/anticanres.14477
134. Kobayashi Y, Inagawa H, Kohchi C, Okazaki K, Zhang R, Kobara H, et al. Lipopolysaccharides derived from pantoea agglomerans can promote the phagocytic activity of amyloid B in mouse microglial cells. Anticancer Res. (2017) 37:3917–20. doi: 10.21873/anticanres.11774
135. Ackley SF, Zimmerman SC, Brenowitz WD, Tchetgen Tchetgen EJ, Gold AL, Manly JJ, et al. Effect of reductions in amyloid levels on cognitive change in randomized trials: instrumental variable meta-analysis. Bmj. (2021) 372:n156. doi: 10.1136/bmj.n156
136. Kandimalla R, Thirumala V, Reddy PH. Is alzheimer's disease a type 3 diabetes? A critical appraisal. Biochim Biophys Acta Mol Basis Dis. (2017) 1863:1078–89. doi: 10.1016/j.bbadis.2016.08.018
137. Inagawa H, Oda M, Tjhin VT, Kohchi C, Soma GI. Restoration of spatial learning through oral administration of lipopolysaccharides in diabetes-related cognitive dysfunction. In Vivo. (2024) 38:2190–6. doi: 10.21873/invivo.13682
138. Shinya K, Ito M, Makino A, Tanaka M, Miyake K, Eisfeld AJ, et al. The tlr4-trif pathway protects against H5n1 influenza virus infection. J Virol. (2012) 86:19–24. doi: 10.1128/jvi.06168-11
139. Fu Y, Luo N, Klein RL, Garvey WT. Adiponectin promotes adipocyte differentiation, insulin sensitivity, and lipid accumulation. J Lipid Res. (2005) 46:1369–79. doi: 10.1194/jlr.M400373-JLR200
140. Mehta NN, McGillicuddy FC, Anderson PD, Hinkle CC, Shah R, Pruscino L, et al. Experimental endotoxemia induces adipose inflammation and insulin resistance in humans. Diabetes. (2010) 59:172–81. doi: 10.2337/db09-0367
141. Anhê FF, Barra NG, Cavallari JF, Henriksbo BD, Schertzer JD. Metabolic endotoxemia is dictated by the type of lipopolysaccharide. Cell Rep. (2021) 36:109691. doi: 10.1016/j.celrep.2021.109691
142. Ren H, Shen J, Tomiyama-Miyaji C, Watanabe M, Kainuma E, Inoue M, et al. Augmentation of innate immunity by low-dose irradiation. Cell Immunol. (2006) 244:50–6. doi: 10.1016/j.cellimm.2007.02.009
143. Sugibayashi K. Theory, practical application and future expectation of percutaneous absorption. Oleoscience. (2017) 17:549–58. doi: 10.5650/oleoscience.17.549
144. Wakame K, Komatsu K, Inagawa H, Nishizawa T. Immunopotentiator from pantoea agglomerans prevents atopic dermatitis induced by dermatophagoides farinae extract in nc/nga mouse. Anticancer Res. (2015) 35:4501–8.
145. Otsuka M, Egawa G, Kabashima K. Uncovering the mysteries of langerhans cells, inflammatory dendritic epidermal cells, and monocyte-derived langerhans cell-like cells in the epidermis. Front Immunol. (2018) 9:1768. doi: 10.3389/fimmu.2018.01768
146. Eslani M, Movahedan A, Afsharkhamseh N, Sroussi H, Djalilian AR. The role of toll-like receptor 4 in corneal epithelial wound healing. Invest Ophthalmol Vis Sci. (2014) 55:6108–15. doi: 10.1167/iovs.14-14736
147. Inagawa H, Nishizawa T, Kohchi C, Soma GI. Lipopolysaccharide derived from pantoea agglomerans directly promotes the migration of human keratinocytes. In Vivo. (2024) 38:2172–8. doi: 10.21873/invivo.13680
148. Seo SJ, Ahn SW, Hong CK, Ro BI. Expressions of beta-defensins in human keratinocyte cell lines. J Dermatol Sci. (2001) 27:183–91. doi: 10.1016/s0923-1811(01)00135-9
149. Kohchi C, Hara-Chikuma M, Hamada K, Ikemoto T, Tsutsui S, Inagawa H, et al. Effectiveness of lipopolysaccharide for sensitive skin. J Soc Cosmetic Chem Japan. (2021) 55:338–45. doi: 10.5107/sccj.55.338
150. Ogata Y, Yamada T, Hasegawa S, Sugiura K, Akamatsu H. Changes of senescent cell accumulation and removal in skin tissue with ageing. Exp Dermatol. (2023) 32:1159–61. doi: 10.1111/exd.14818
151. Ogata Y, Yamada T, Hasegawa S, Sanada A, Iwata Y, Arima M, et al. Sasp-induced macrophage dysfunction may contribute to accelerated senescent fibroblast accumulation in the dermis. Exp Dermatol. (2021) 30:84–91. doi: 10.1111/exd.14205
152. Ashwood P. Preliminary evidence of differentially induced immune responses by microparticle-adsorbed lps in patients with crohn's disease. J Cell Immunol. (2022) 4:211–8. doi: 10.33696/immunology.4.152
153. Caradonna L, Amati L, Magrone T, Pellegrino NM, Jirillo E, Caccavo D. Enteric bacteria, lipopolysaccharides and related cytokines in inflammatory bowel disease: biological and clinical significance. J Endotoxin Res. (2000) 6:205–14.
154. Nakamoto T, Yoshimura H, Honda T, Nakata K, Taniguchi Y, Yoshida A, et al. Treatments for the activating macrophages that reduces surgical stress and postoperative mortalities from bacterial infections and tumor metastases. In Vivo. (2007) 21:357–64.
155. Engelich G, Wright DG, Hartshorn KL. Acquired disorders of phagocyte function complicating medical and surgical illnesses. Clin Infect Dis. (2001) 33:2040–8. doi: 10.1086/324502
156. Netea MG, Domínguez-Andrés J, Barreiro LB, Chavakis T, Divangahi M, Fuchs E, et al. Defining trained immunity and its role in health and disease. Nat Rev Immunol. (2020) 20:375–88. doi: 10.1038/s41577-020-0285-6
Keywords: lipopolysaccharide, macrophage, macrophage network, innate immunity, self-healing ability
Citation: Soma G-I, Oda M, Tjhin VT, Kohchi C and Inagawa H (2025) Oral and transdermal administration of lipopolysaccharide safely enhances self-healing ability through the macrophage network. Front. Immunol. 16:1563484. doi: 10.3389/fimmu.2025.1563484
Received: 20 January 2025; Accepted: 13 March 2025;
Published: 31 March 2025.
Edited by:
Farha Naz, University of Virginia, United StatesCopyright © 2025 Soma, Oda, Tjhin, Kohchi and Inagawa. This is an open-access article distributed under the terms of the Creative Commons Attribution License (CC BY). The use, distribution or reproduction in other forums is permitted, provided the original author(s) and the copyright owner(s) are credited and that the original publication in this journal is cited, in accordance with accepted academic practice. No use, distribution or reproduction is permitted which does not comply with these terms.
*Correspondence: Gen-Ichiro Soma, Z2Vuc29tYUBzaGl6ZW5tZW5la2kub3Jn
Disclaimer: All claims expressed in this article are solely those of the authors and do not necessarily represent those of their affiliated organizations, or those of the publisher, the editors and the reviewers. Any product that may be evaluated in this article or claim that may be made by its manufacturer is not guaranteed or endorsed by the publisher.
Research integrity at Frontiers
Learn more about the work of our research integrity team to safeguard the quality of each article we publish.