- 1Liaoning Cancer Hospital & Institute, Shenyang, China
- 2First Affiliated Hospital of Dalian Medical University, Dalian, China
Thymidine phosphorylase (TYMP), a protein found in both prokaryotic and eukaryotic cells, is encoded by a gene located in the q13 region of chromosome 22. With a relative molecular mass of 55,000, TYMP exists as a homodimer. Recent research has increasingly illuminated the diverse functions of TYMP. It is known to facilitate platelet activation, osteoclast differentiation, and angiogenesis. Mutations in the TYMP gene are linked to mitochondrial neurogastrointestinal encephalomyopathy. Beyond its physiological roles, TYMP contributes significantly to tumor growth and cancer progression, where it promotes angiogenesis, modulates epigenetic genes, inhibits apoptosis, and acts as a critical enzyme in the nucleoside metabolic rescue pathway. Moreover, TYMP holds substantial implications in cancer treatment and prognosis. Given its involvement in cancer progression, TYMP inhibitors may prove valuable in inhibiting tumor growth and metastasis. Interestingly, while TYMP can drive tumor growth, certain concentrations of TYMP also enhance the cytotoxic effects of chemotherapy drugs such as 5-fluorouracil (5-FU). Although challenges exist—such as the potential disruption of normal physiological functions when inhibiting TYMP—the protein remains a promising target for cancer treatment. Ongoing research on TYMP could deepen our understanding of human physiology and the pathogenesis of cancer and open new avenues for therapeutic interventions. This article provides a comprehensive review of TYMP’s structure, physiological functions, and its role in tumorigenesis and anti-tumor therapy.
1 Introduction
Thymidine phosphorylase (TYMP) was first isolated and purified from animal tissues by Friedkin and Roberts in 1954, with the enzyme subsequently named thymidine phosphorylase, now commonly referred to as TYMP (1). Due to the technological limitations of the time, research on TYMP was limited following its discovery. It wasn’t until 1978 that Kubilus and Baden isolated and purified human TYMP from human amniotic membrane, confirming its existence in humans (2). This marked the beginning of a deeper exploration into the presence and functions of TYMP within the human body. Over time, TYMP’s essential biological roles, including promoting platelet activation, osteoclast differentiation, angiogenesis, and its involvement in tumor angiogenesis, epigenetic gene modification, apoptosis resistance, and nucleoside metabolic salvage, have been gradually unveiled. As such, TYMP has become a critical target in cancer research. Despite significant advancements, ongoing studies continue to deepen our understanding of TYMP’s full potential, yet much remains to be uncovered.
Cancer remains the leading cause of death worldwide (3–5). Its insidious nature means that by the time clinical symptoms become apparent, the disease is often in its advanced stages (6). While outcomes vary among patients, many advanced cancer cases can benefit from careful preoperative assessment and postoperative neoadjuvant chemotherapy, leading to improved prognoses (7, 8). However, these approaches do not diminish the significance of targeted cancer therapies in the treatment landscape. In recent years, the development of targeted cancer therapies, coupled with clinical trials, has provided promising alternative treatment options, offering patients greater choices. Therefore, understanding the underlying mechanisms of cancer and identifying viable therapeutic targets, such as TYMP, has become an essential focus in cancer research.
2 Structure of TYMP
2.1 Gene and molecular structure of TYMP
The TYMP gene is located on chromosome 22q13.32-qter (9). Whether in mammals or bacteria, TYMP exhibits structural conservation, existing as an anionic protein composed of two homodimers with a relative molecular mass of 55,000. Notably, when investigating the three-dimensional structure of Escherichia coli thymidine phosphorylase, researchers determined its active site by differentiating thymine and thymidine binding. This marked the first reported identification of a possible molecular structure of TYMP with thymine in the active site (10). Furthermore, human TYMP shares 39% sequence homology with Escherichia coli TYMP (11). Human TYMP possesses a proline-rich N-terminus (12), a feature absent in bacterial TYMP, potentially explaining the functional differences between the two. For instance, human TYMP plays a role in promoting platelet activation and hemostasis, functions that bacteria do not require.
2.2 TYMP enzyme activity and TYMP inhibitors
TYMP serves as a rate-limiting enzyme (13), exhibiting the common characteristic where enzyme structure influences its activity (14–16). Tipiracil hydrochloride (TPI) has been identified as a potent TYMP inhibitor, demonstrating high binding affinity to the enzyme. Moreover, TPI can function as an imaging agent for assessing TYMP expression in vivo, owing to its stability post-18 F labeling (17). Another notable TYMP inhibitor is the tritylated inosine derivative 5’-O-triynyl sugar (formerly KIN59), which is a non-competitive inhibitor (16). KIN59 binds to TYMP, inducing a conformational change that inhibits enzymatic activity without affecting substrate binding, underscoring the impact of TYMP conformational alterations on its enzymatic function. Additionally, Karen et al. identified polycyclic nitrogen heterocycles as potential TYMP inhibitors (18). Utilizing molecular docking techniques, their research demonstrated the interaction between polycyclic nitrogen heterocycles and TYMP’s active binding site. These findings highlight TYMP inhibitors as a promising class of drugs with significant research potential. For the development of TYMP-targeted therapies in the medical field, understanding the binding site and conformational changes of TYMP will be crucial for optimizing drug efficacy.
3 Physiological functions of TYMP
3.1 Downstream activation pathways of TYMP
TYMP performs a variety of functions, with its activities often relying on multiple signal transduction pathways. In recent years, research into TYMP has advanced significantly, leading to a deeper understanding of its mechanisms. Among the key mechanisms of TYMP are the following:
Platelets, which play a pivotal role in thrombosis, rely on platelet activation as a critical factor in the thrombotic process. Collagen-induced platelet activation is primarily mediated through glycoprotein VI (GPVI). GPVI exists in both monomeric and dimeric forms on the cell surface and is associated with various effectors, including the Fc receptor γ chain (FcRγ), spleen tyrosine kinase, phospholipase Cγ, protein kinase Cδ, and bisphosphoinositide polyphosphate phosphodiesterase 1 (19). During platelet activation, LYN and FYN kinases bind to the cytoplasmic domain of one GPVI molecule, while the other GPVI molecule associates with the FcRγ chain dimer, thereby activating the GPVI signaling pathway that mediates platelet activation (20). Src family kinases (SFKs) play a pivotal role in this activation cascade (21), with FYN acting as the primary stimulator of SFKs, which in turn influence platelet activation. LYN can suppress collagen-induced platelet activation by promoting phosphorylation of the immunoreceptor tyrosine inhibitory motif (ITIM) domain of platelet endothelial cell adhesion molecule 1 (PECAM1) (22, 23). TYMP interacts with the phosphate group on p-LYN, removing it and impairing LYN’s ability to mediate PECAM-1/ITIM phosphorylation. This action reduces LYN’s inhibitory function on collagen-induced platelet activation, thereby indirectly promoting platelet activation and thrombosis (12). This mechanism of TYMP’s role in platelet activation is summarized in Figure 1. Consequently, targeting TYMP with specific drugs may offer a promising approach for developing new anti-platelet and anti-thrombotic treatments.
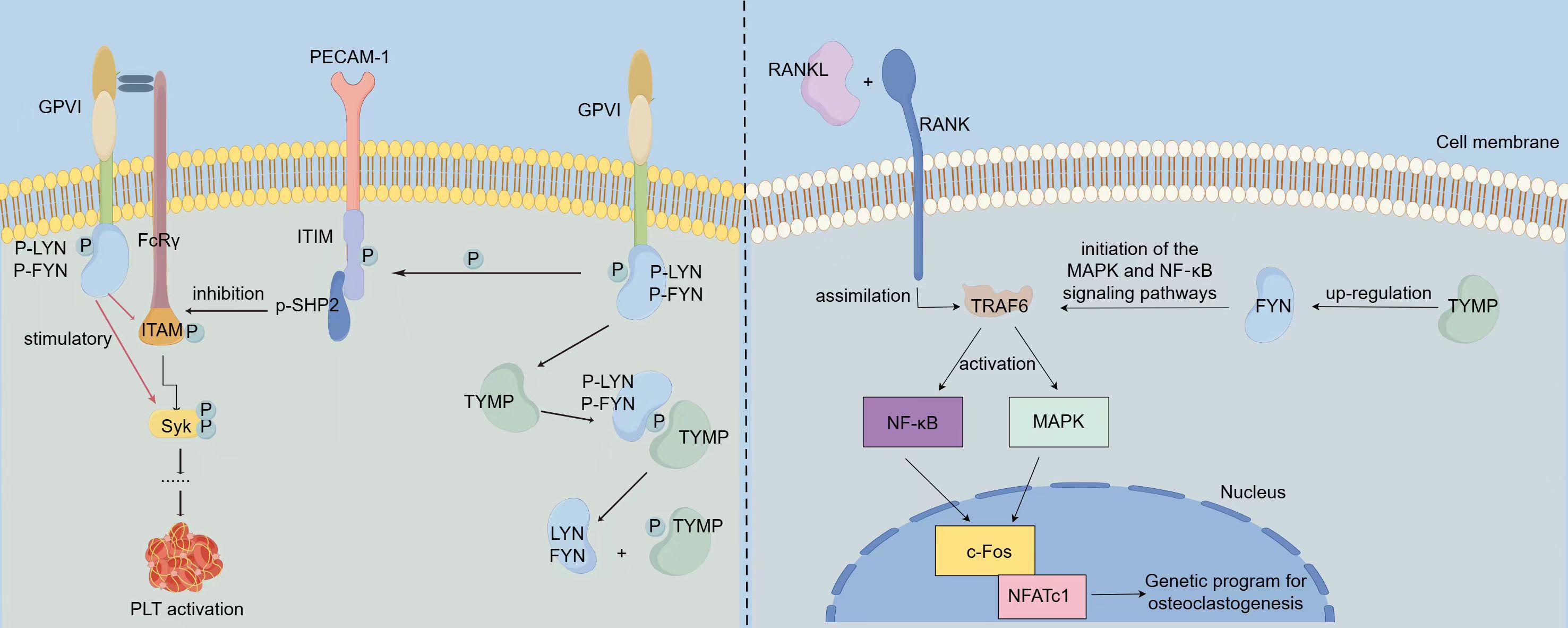
Figure 1. Mechanisms via which TYMP promotes platelet activation and osteoclast differentiation. LYN and FYN bind to the cytoplasmic domain of one GPVI molecule, while the other GPVI molecule interacts with the FcRγ chain dimer, thereby initiating the GPVI signaling pathway. Activation of this pathway results in LYN and FYN stimulating the phosphorylation of ITAM and Syk, which in turn activates platelet activation. Concurrently, LYN inhibits collagen-induced platelet activation by promoting the phosphorylation of the immunoreceptor tyrosine inhibitory motif (ITIM) domain of platelet endothelial cell adhesion molecule 1 (PECAM1). TYMP binds to the phosphate group of p-LYN, dephosphorylating it and causing the loss of its ability to mediate PECAM-1/ITIM phosphorylation. This action attenuates LYN’s inhibitory function on collagen-induced platelet activation, thereby indirectly promoting platelet activation and contributing to thrombosis. In OC precursor cells, RANKL binds to the RANK receptor on OC precursors, leading to the recruitment of TRAF6, which activates NF-κB and AP-1 transcription factors, triggering downstream signaling pathways. This activation promotes the expression of c-Fos and NFATc1, key drivers of osteoclast differentiation. In TYMP-stimulated cells, the expression of FYN is significantly increased. TYMP activates FYN signaling, which subsequently promotes the activation of MAPK and NF-κB signaling pathways, thereby facilitating osteoclast differentiation.
The receptor activator of nuclear factor-κB ligand (RANKL)-associated signaling pathway plays a critical role in osteoclastogenesis and is potentially linked to inflammation (24). Osteoclasts (OCs) are multinucleated hematopoietic cells capable of bone resorption. Their formation is supported by macrophage colony-stimulating factor (M-CSF) and RANKL, which binds to the RANK receptor on OC precursors (25). The RANK-RANKL interaction recruits tumor necrosis factor receptor-associated factor 6 (TRAF6), which activates the NF-κB and AP-1 transcription factors (26, 27), initiating the signaling cascade. This activation promotes the expression of key molecules such as c-Fos, Cathepsin K, and T cell nuclear factor cytoplasmic 1 (NFATc1), thereby facilitating OC differentiation (28, 29) (Figure 1). Once differentiated, OC cells are rapidly driven toward apoptosis. FYN plays a key role in stimulating osteoclastogenesis by enhancing the proliferation and differentiation of OC precursors (30). This effect is primarily mediated through phosphorylation. A Japanese research team identified TYMP as a factor that induces osteoclast differentiation by activating FYN signaling. After TYMP stimulation, they observed a marked increase in phosphorylated FYN levels and enhanced FYN gene expression in macrophages. Additionally, sedimentation experiments revealed that TYMP binds to a complex containing integrin β1 (ITGβ1) and FYN. Furthermore, in TYMP-stimulated cells, there was a significant upregulation of phosphorylated protein kinase 1/2 (MEK1/2) and phosphorylated extracellular signal-regulated kinase 1/2 (ERK1/2). These findings suggest that TYMP activates FYN signaling, leading to the activation of MAPK and NF-κB pathways, thereby promoting osteoclast differentiation (31). The detailed mechanism by which TYMP promotes osteoclast differentiation is summarized in Figure 1.
3.2 Pro-angiogenic function of TYMP
Angiogenesis, the process of forming new blood vessels from existing ones, is promoted by TYMP. TYMP is highly expressed not only in tumor cells but also in normal cells, including macrophages, stromal cells, glial cells, and certain epithelial cells (32). Notably, the elevated expression of TYMP in macrophages and skin plays a critical role in maintaining systemic thymidine homeostasis.
TYMP catalyzes the conversion of thymidine (TdR) into deoxyribose-1-phosphate (dR-1-P) and thymine, producing a molecular structure that contains deoxyribose (dR) (33). This dR component can further participate in angiogenesis (34). Since TYMP catalyzes this reaction and indirectly influences angiogenesis, its pro-angiogenic effect is closely tied to its enzymatic activity. Consequently, inhibiting TYMP’s enzymatic activity with TYMP inhibitors to block its pro-angiogenic effects presents a potential therapeutic strategy. Furthermore, TYMP can directly induce angiogenic factors such as interleukin-8, basic fibroblast growth factor, and tumor necrosis factor α, which promote angiogenesis and stimulate endothelial cell migration and invasion (33). However, research into TYMP’s role in angiogenesis remains limited, and it is unclear whether additional pro-angiogenic mechanisms exist. Therefore, the full extent of TYMP’s involvement in angiogenesis is not yet fully understood, and further investigation is necessary.
3.3 Genetic disorders caused by TYMP deficiency
Mitochondrial neurogastrointestinal encephalomyopathy (MNGIE) is a rare autosomal recessive disorder caused by mutations in the TYMP gene, resulting in the loss of TYMP function (35). This disease leads to dysfunction in the digestive and nervous systems, presenting with clinical symptoms such as cachexia, ptosis, external ophthalmoplegia, peripheral neuropathy, and leukoencephalopathy (36–38). While no significant side effects have been reported for TYMP inhibitors as emerging drugs, the existence of MNGIE serves as a reminder that TYMP inhibitors could have previously unrecognized side effects. Therefore, extensive research is still needed before TYMP inhibitors can be applied clinically.
4 Role of TYMP in the occurrence and progression of cancer
TYMP is overexpressed in various cancers, including breast cancer (38), gastric cancer (39), esophageal cancer (40), oral squamous cell carcinoma (41), lung cancer (42), colorectal cancer (43), cervical cancer (44), and bladder cancer (45). Furthermore, plasma levels of TYMP in individuals with certain cancers are significantly higher than in healthy individuals (46) (Figure 2).
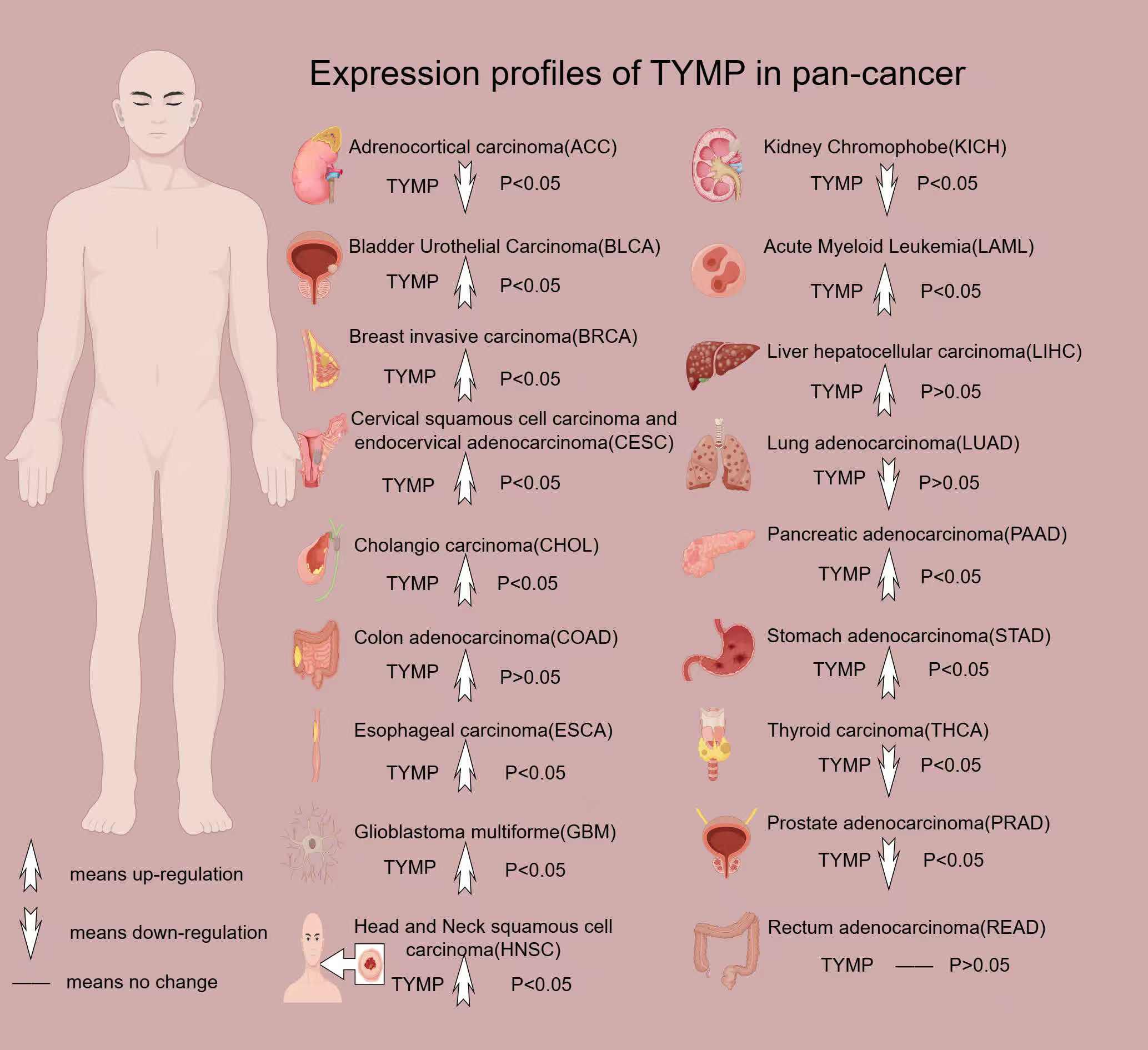
Figure 2. Expression profile of TYMP in pan-cancer. All data in this image are sourced from the GEPIA2 platform.
4.1 TYMP-driven tumorigenesis through angiogenesis
The most well-established function of TYMP is its role in promoting angiogenesis. For tumors to proliferate extensively, they require a constant supply of oxygen and nutrients, which depend on blood circulation. Thus, a fully developed vascular system is essential for tumor growth and progression. TYMP’s angiogenesis-promoting effect addresses this need. As previously mentioned, TYMP facilitates angiogenesis through the dR component in its metabolites and by directly stimulating angiogenic factors, which in turn influence tumor growth and development. Consequently, inhibiting TYMP enzymatic activity using TYMP inhibitors has emerged as a viable therapeutic approach for slowing cancer progression. However, not all tumors depend on TYMP for angiogenesis. Some tumors, despite having dense microvascular networks, show no significant increase in TYMP levels (47). This suggests that additional, yet unexplored, mechanisms may also drive tumor angiogenesis. Nonetheless, it is clear that tumors with high TYMP expression are likely to have dense microvascular systems.
4.2 TYMP-driven tumorigenesis through epigenetic modification
TYMP also influences tumor progression through its role in DNA methylation regulation. A 2016 study revealed that TYMP catalyzes the conversion of thymidine to thymine and 2-deoxy-D-ribose (2DDR), which then binds to integrin αVβ3/α5β1 on progenitor cells, activating the PI3K/Akt signaling pathway. This results in increased expression of DNA methyltransferase 3A (DNMT3A), leading to hypermethylation of key genes such as RUNX2, osterix, and IRF8 (48) (Figure 3). This mechanism is particularly significant in myeloma, where TYMP-induced hypermethylation of these genes contributes to reduced bone formation and enhanced bone resorption. TYMP overexpression is commonly observed in bone metastatic tumors, further supporting the potential of TYMP-targeted therapies for treating myeloma-related bone diseases.
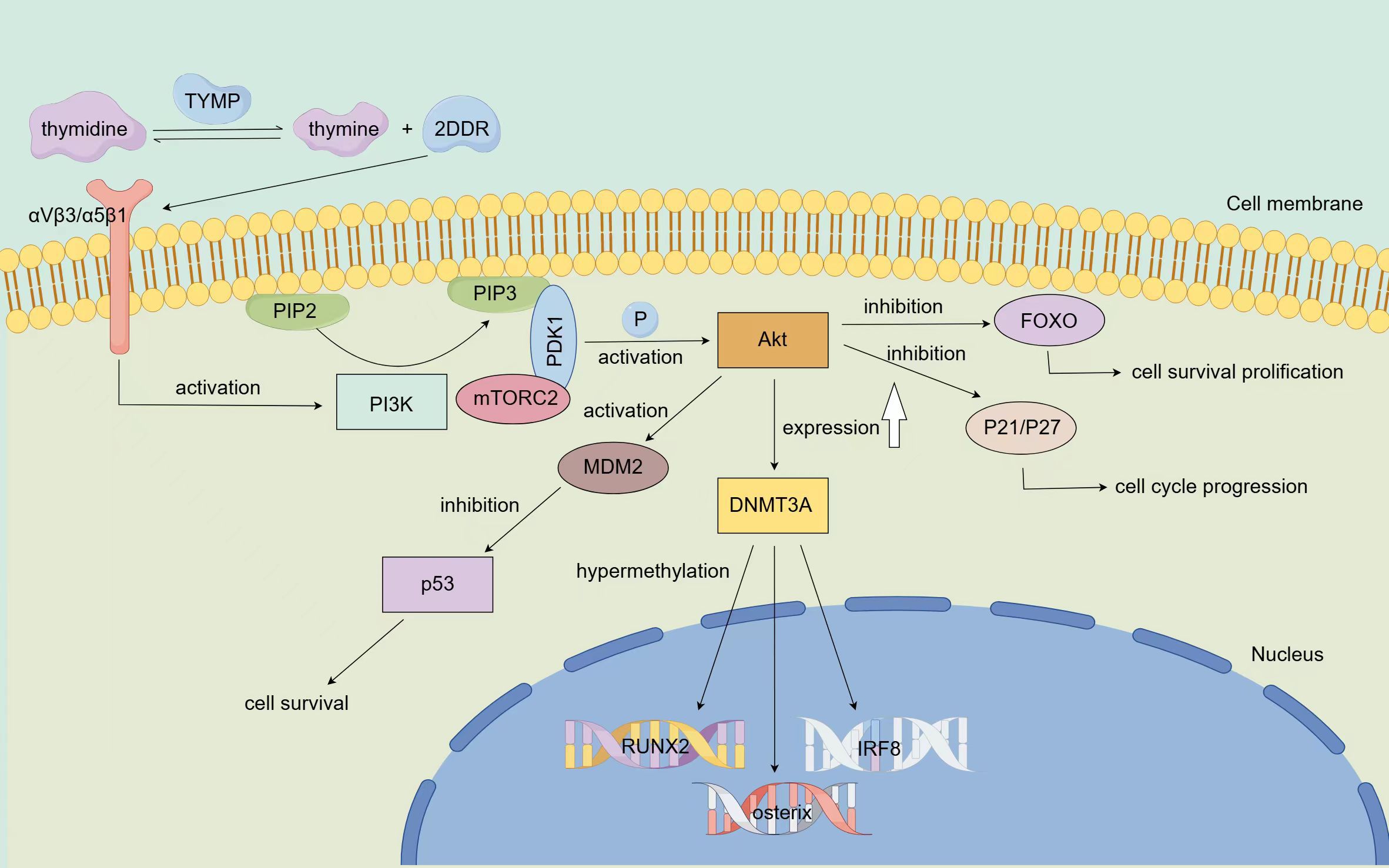
Figure 3. Mechanism via which TYMP modifies epigenetic and resists apoptosis TYMP catalyzes the conversion of thymidine to thymine and 2DDR, which binds to integrin αVβ3/α5β1 on progenitor cells, thereby activating the PI3K pathway. Once activated, PI3K promotes the conversion of PIP2 to PIP3, leading to the phosphorylation of Akt via PDK1 and mTORC2, completing Akt activation. Activated Akt inhibits FOXO, a key initiator of apoptosis, thereby promoting cell survival. Additionally, Akt can enhance tumor growth by suppressing the expression of tumor suppressor proteins P21 and P27. Furthermore, Akt acts on the MDM2 oncoprotein, negatively regulating the p53 tumor suppressor, thus resisting apoptosis and promoting tumor cell survival. Activated Akt also upregulates the expression of DNMT3A, leading to the hypermethylation of key genes such as RUNX2, osterix, and IRF8.
4.3 TYMP-driven tumorigenesis through anti-apoptotic pathways
TYMP plays a key role in helping tumor cells resist apoptosis induced by hypoxia. Kitazono’s study demonstrated that TYMP’s metabolites, 2-deoxy-D-ribose and thymine, can partially counteract hypoxia-induced apoptosis in KB/TP cells by transfecting them with endothelial cell growth factor/thymidine phosphorylase (PD-ECGF/TP) cDNA (49). Additionally, research involving myocardial ischemia in dogs showed that PD-ECGF/TP improved ischemic conditions, alleviating apoptosis caused by hypoxia (50, 51). Furthermore, TYMP activates the PI3K/Akt signaling pathway (48), a key pathway involved in regulating cell apoptosis (52). Upon PI3K activation, phosphatidylinositol-4,5-bisphosphate (PIP2) is converted into phosphatidylinositol-3,4,5-triphosphate (PIP3) (53), leading to the phosphorylation of Akt via phosphoinositide-dependent kinase 1 (PDK1) and mammalian target of rapamycin complex 2 (mTORC2), thereby activating Akt (54). Activated Akt inhibits the Forkhead box O (FOXO) transcription factors, which initiate apoptosis, thereby promoting cell survival (55). Additionally, Akt can inhibit the expression of cell cycle regulators P21 and P27, further supporting tumor growth (56). Akt also negatively regulates the p53 tumor suppressor protein via MDM2, resisting apoptosis and promoting tumor cell survival (57) (Figure 3). Thus, TYMP can prevent cell apoptosis by activating the PI3K/Akt pathway, supporting tumor cells in resisting apoptosis induced by various treatments such as the immune response, hypoxia, radiotherapy, and chemotherapy. Understanding TYMP’s anti-apoptotic role could enhance cancer therapies and offer insights into treating diseases caused by cellular hypoxia. The main signaling pathways of TYMP are summarized in Figure 3.
4.4 TYMP-driven tumorigenesis through stabilizing the thymidine pool
As a key enzyme in the nucleoside metabolic salvage pathway, TYMP helps maintain thymine pool stability, a key factor for DNA synthesis. Tumor cells reprogram their metabolic pathways to meet growth demands, with pyrimidine metabolism playing a central role. Studies have shown that the expression levels of thymidylate synthase (TYMS) and TYMP in tumor tissues are significantly higher than in adjacent normal tissues. Both TYMS and TYMP mRNA have the potential to serve as reliable diagnostic indicators for colon cancer (CC), but further research is required to confirm this (58). Given that tumor growth depends on cell division, TYMP’s involvement in cell proliferation and tumor development suggests its critical role in cancer progression. Further investigation into the relationship between TYMP and tumors is needed.
5 Roles of TYMP in anti-cancer therapy
5.1 TYMP functions as a target for cancer therapy
Although TYMP exerts several promoting effects on tumor cell growth and development, it also holds therapeutic potential in cancer treatment. As a molecular target, TYMP is being explored for the development of cancer therapeutics (59). Investigating its biological functions in tumors may lead to the synthesis of TYMP inhibitors, which could prevent angiogenesis and slow tumor metastasis. However, research has indicated that certain TYMP activities can activate a variety of chemotherapeutic agents (40), which is crucial for cancer therapy. For instance, bevacizumab can enhance the metabolic activation of 5-fluorouracil (5-FU) through upregulation of TYMP (60). Furthermore, recent studies suggest that utilizing human mesenchymal stem cells (hMSCs) as carriers to deliver TYMP to cancer cells may facilitate the conversion of docifluridine (5′-DFUR) into the toxic 5-FU, promoting cancer cell death (61).
The activation of nucleic acid sensors in endothelial cells (ECs) triggers inflammation in various diseases, including cancer. Specifically, activation of the cytoplasmic RNA sensor retinoic acid-induced gene 1 (RIG-I) significantly contributes to decreased EC survival, with TYMP being the most upregulated gene in this process. Therefore, inhibiting TYMP could potentially alleviate endothelial dysfunction and enhance cancer treatment (62).
As previously discussed, TYMP activity can activate chemotherapy drugs, while TYMP inhibitors could mitigate its angiogenic effects, thereby indirectly impeding tumor cell growth. We summarized the TYMP inhibitors that are currently of certain research value and the chemotherapeutic drugs that rely on TYMP activity activation (Table 1). Although TYMP inhibitors may hold promise in cancer therapy (69), their safety remains uncertain, and long-term research is necessary to determine their clinical viability.
5.2 Targeting TYMP to alleviate resistance to cancer immunotherapy and chemotherapy
Immunotherapy and chemotherapy are widely used in cancer treatment; however, their efficacy is not always consistent. Over prolonged exposure to these therapies, tumor cells often develop drug resistance, which diminishes treatment effectiveness. This phenomenon is a key factor contributing to the continued challenges in achieving significant progress in cancer treatment.
Peri et al. observed the upregulation of TYMP in gastric cancer cells that were induced to become resistant to 5-fluorouracil, identifying TYMP gene mutations in tumor cells as a common cause of 5-fluorouracil resistance. Such mutations can enhance angiogenesis in gastric cancer cell lines (70). Another study found that TYMP-induced T cell exhaustion plays a critical role in immunotherapy resistance in colorectal cancer (CRC) (71). Targeted TYMP therapies could potentially improve the effectiveness of immunotherapy. In 2022, a research team explored whether demethylation of TYMP would increase cancer cell sensitivity to 5-FU. While the results did not demonstrate that TYMP demethylation alone enhanced 5-FU sensitivity, they suggested a potential strategy combining TYMP with other metabolic pathways to boost 5-FU responsiveness (72). These findings provide novel insights into drug resistance in tumor cells, thereby enhancing cancer treatment strategies.
5.3 Role of TYMP in prognostic evaluation of tumor therapies
The expression of TYMP is closely associated with rectal cancer treatment outcomes (73). Preoperative radiotherapy or chemoradiotherapy (CRT) is considered the standard treatment for locally advanced rectal cancer (74, 75), and studies have shown that TYMP expression can help predict the efficacy of CRT (76). Furthermore, TYMP’s significance in CRC progression is evident, as a study demonstrated that the rs11479 polymorphism could predict the prognosis of patients with CRC receiving capecitabine-based adjuvant chemotherapy (77). This highlights that modulating TYMP mRNA expression could enhance the effectiveness of capecitabine-based therapy, thus improving survival rates in patients with CRC.
Additionally, TYMP expression in CRC tumor epithelial cells is linked to recurrence-free survival (RFS) in patients with CRC. Elevated TYMP expression may correlate with poor prognosis in these patients (78). Furthermore, research has shown that TYMP polymorphisms can influence the prognosis of patients with CRC undergoing chemotherapy by modulating TYMP mRNA expression (79, 80).
TYMP also serves as a valuable immune prognostic marker in various cancers, including renal clear cell carcinoma (81, 82) and low-grade glioma (83). Recent studies have shown that a reduction in TYMP expression can significantly affect the proliferation, migration, and invasion of renal cell carcinoma (RCC) cells in vitro (84). Furthermore, a 2016 study indicated a potential link between TYMP levels and the survival rate of patients with localized gastric cancer following radical gastrectomy (85). Additionally, the combined expression of TYMP and hypoxia-inducible factor α (HIF-1α) has been shown to predict the prognosis of patients with rectal cancer undergoing neoadjuvant chemoradiotherapy with oxaliplatin and capecitabine (XELOXART) (86). These findings underscore the pivotal role of TYMP in cancer treatment and prognosis prediction.
Despite its promising potential in cancer therapy, TYMP has certain limitations. Due to individual differences among patients, TYMP-targeting drugs may be effective for some cancer individuals, while less effective for others. Moreover, TYMP not only promotes tumor progression but also influences normal physiological functions, making it crucial to consider the potential side effects of TYMP-targeted therapies. Further research and clinical trials are needed to validate these effects. While significant progress has been made in understanding TYMP’s role in disease, many aspects remain to be explored. The mechanisms underlying TYMP’s involvement in tumors are complex, and the clinical effects of inhibiting TYMP have yet to be fully established. The potential for unknown side effects of TYMP inhibitors also warrants further investigation. Despite these uncertainties, TYMP remains a promising therapeutic target. It holds considerable potential in the understanding and treatment of cancer, making ongoing research into TYMP essential. However, considering the current state of research, significant progress is still required before TYMP-targeted therapies can be fully developed and applied.
6 Conclusions
TYMP, a protein found extensively in both prokaryotic and eukaryotic cells, performs critical physiological functions, including promoting platelet activation, osteoclast differentiation, and angiogenesis. Mutations in the TYMP gene can lead to genetic disorders such as MNGIE. Beyond its role in normal cells, TYMP is also pivotal in cancer cells, where it facilitates tumor angiogenesis, modifies epigenetics, and helps resist cell apoptosis. Additionally, TYMP functions as a key enzyme in the nucleoside metabolic rescue pathway. Consequently, studying TYMP enhances our understanding of cancer mechanisms and is vital for cancer treatment. Targeting TYMP has emerged as a promising strategy for cancer therapy, and TYMP-activated anti-tumor drugs represent an important therapeutic approach. Despite existing limitations, further research on TYMP holds significant potential for advancing cancer treatment.
Author contributions
BH: Data curation, Investigation, Writing – original draft. QY: Writing – original draft, Conceptualization, Supervision. JS: Conceptualization, Supervision, Writing – original draft. CW: Writing – review & editing, Data curation, Investigation. DY: Conceptualization, Supervision, Writing – review & editing.
Funding
The author(s) declare that no financial support was received for the research and/or publication of this article.
Acknowledgments
We acknowledge Bullet Edits Limited for their linguistic editing and proofreading of the manuscript. We also extend our gratitude to the FigDraw platform for facilitating the creation of the illustrations. The three images included in this manuscript were designed with the assistance of the FigDraw platform and received approval. The approval numbers for the images are as follows: Figure 1 ID: UWPSO8ccc4; Figure 2 ID: RYUII43b78; Figure 3 ID: TIWUU7ee76.
Conflict of interest
The authors declare that the research was conducted in the absence of any commercial or financial relationships that could be construed as a potential conflict of interest.
Generative AI statement
The author(s) declare that no Generative AI was used in the creation of this manuscript.
Publisher’s note
All claims expressed in this article are solely those of the authors and do not necessarily represent those of their affiliated organizations, or those of the publisher, the editors and the reviewers. Any product that may be evaluated in this article, or claim that may be made by its manufacturer, is not guaranteed or endorsed by the publisher.
References
1. Friedkin M, Roberts D. The enzymatic synthesis of nucleosides. I. Thymidine phosphorylase in mammalian tissue. J Biol Chem. (1954) 207:245–56. doi: 10.1016/S0021-9258(18)71264-7
2. Kubilus J, Lee LD, Baden HP. Purification of thymidine phosphorylase from human amniochorion. Biochim Biophys Acta. (1978) 527:221–8. doi: 10.1016/0005-2744(78)90271-1
3. Bray F, Laversanne M, Sung H, Ferlay J, Siegel RL, Soerjomataram I, et al. Global cancer statistics 2022: GLOBOCAN estimates of incidence and mortality worldwide for 36 cancers in 185 countries. CA Cancer J Clin. (2024) 74:229–63. doi: 10.3322/caac.21834
4. de Martel C, Georges D, Bray F, Ferlay J, Clifford GM. Global burden of cancer attributable to infections in 2018: a worldwide incidence analysis. Lancet Glob Health. (2020) 8:e180–90. doi: 10.1016/S2214-109X(19)30488-7
5. Siegel RL, Miller KD, Fuchs HE, Jemal A. Cancer statistics, 2022. CA Cancer J Clin. (2022) 72:7–33. doi: 10.3322/caac.21708
6. Yuan Q, Sun J, Hong Z, Shang D. Determining a robust prognostic biomarker for 804 patients with pancreatic cancer using a machine learning computational framework. Int J Surg. (2025) 111:1561–3. doi: 10.1097/JS9.0000000000002016
7. Pu N, Wu W, Liu S, Xie Y, Yin H, Chen Q, et al. Survival benefit and impact of adjuvant chemotherapy following systemic neoadjuvant chemotherapy in patients with resected pancreas ductal adenocarcinoma: a retrospective cohort study. Int J Surg. (2023) 109:3137–46. doi: 10.1097/JS9.0000000000000589
8. Liang H, Yan X, Li Z, Chen X, Qiu Y, Li F, et al. Clinical outcomes of conversion surgery following immune checkpoint inhibitors and chemotherapy in stage IV gastric cancer. Int J Surg. (2023) 109:4162–72. doi: 10.1097/JS9.0000000000000738
9. Nishino I, Spinazzola A, Hirano M. Thymidine phosphorylase gene mutations in MNGIE, a human mitochondrial disorder. Science. (1999) 283:689–92. doi: 10.1126/science.283.5402.689
10. Walter MR, Cook WJ, Cole LB, Short SA, Koszalka GW, Krenitsky TA, et al. Three-dimensional structure of thymidine phosphorylase from Escherichia coli at 2.8 A resolution. J Biol Chem. (1990) 265:14016–22. doi: 10.1016/S0021-9258(18)77450-4
11. Barton GJ, Ponting CP, Spraggon G, Finnis C, Sleep D. Human platelet-derived endothelial cell growth factor is homologous to Escherichia coli thymidine phosphorylase. Protein Sci. (1992) 1:688–90. doi: 10.1002/pro.5560010514
12. Li W, Gigante A, Perez-Perez MJ, Yue H, Hirano M, McIntyre TM, et al. Thymidine phosphorylase participates in platelet signaling and promotes thrombosis. Circ Res. (2014) 115:997–1006. doi: 10.1161/CIRCRESAHA.115.304591
13. Tabata S, Yamamoto M, Goto H, Hirayama A, Ohishi M, Kuramoto T, et al. Thymidine catabolism as a metabolic strategy for cancer survival. Cell Rep. (2017) 19:1313–21. doi: 10.1016/j.celrep.2017.04.061
14. Mitsiki E, Papageorgiou AC, Iyer S, Thiyagarajan N, Prior SH, Sleep D, et al. Structures of native human thymidine phosphorylase and in complex with 5-iodouracil. Biochem Biophys Res Commun. (2009) 386:666–70. doi: 10.1016/j.bbrc.2009.06.104
15. Miyadera K, Sumizawa T, Haraguchi M, Yoshida H, Konstanty W, Yamada Y, et al. Role of thymidine phosphorylase activity in the angiogenic effect of platelet derived endothelial cell growth factor/thymidine phosphorylase. Cancer Res. (1995) 55:1687–90.
16. Bronckaers A, Aguado L, Negri A, Camarasa MJ, Balzarini J, Pérez-Pérez MJ, et al. Identification of aspartic acid-203 in human thymidine phosphorylase as an important residue for both catalysis and non-competitive inhibition by the small molecule “crystallization chaperone” 5’-O-tritylinosine (KIN59). Biochem Pharmacol. (2009) 78:231–40. doi: 10.1016/j.bcp.2009.04.011
17. Grierson JR, Brockenbrough JS, Rasey JS, Wiens L, Vesselle H. Synthesis and in vitro evaluation of 5-fluoro-6-[(2-iminopyrrolidin-1-YL)methyl]uracil, TPI(F): an inhibitor of human thymidine phosphorylase (TP). Nucleosides Nucleotides Nucleic Acids. (2010) 29:49–54. doi: 10.1080/15257770903451603
18. Aknin K, Bontemps A, Farce A, Merlet E, Belmont P, Helissey P, et al. Polycyclic nitrogen heterocycles as potential thymidine phosphorylase inhibitors: synthesis, biological evaluation, and molecular docking study. J Enzyme Inhib Med Chem. (2022) 37:252–68. doi: 10.1080/14756366.2021.2001806
19. Babur Ö, Melrose AR, Cunliffe JM, Klimek J, Pang J, Sepp AI, et al. Phosphoproteomic quantitation and causal analysis reveal pathways in GPVI/ITAM-mediated platelet activation programs. Blood. (2020) 136:2346–58. doi: 10.1182/blood.2020005496
20. Dütting S, Bender M, Nieswandt B. Platelet GPVI: a target for antithrombotic therapy?! Trends Pharmacol Sci. (2012) 33:583–90. doi: 10.1016/j.tips.2012.07.004
21. Séverin S, Nash CA, Mori J, Zhao Y, Abram C, Lowell CA, et al. Distinct and overlapping functional roles of Src family kinases in mouse platelets. J Thromb Haemost. (2012) 10:1631–45. doi: 10.1111/j.1538-7836.2012.04814.x
22. Cicmil M, Thomas JM, Sage T, Barry FA, Leduc M, Bon C, et al. Collagen, convulxin, and thrombin stimulate aggregation-independent tyrosine phosphorylation of CD31 in platelets. Evidence for the involvement of Src family kinases. J Biol Chem. (2000) 275:27339–47. doi: 10.1016/S0021-9258(19)61516-4
23. Ming Z, Hu Y, Xiang J, Polewski P, Newman PJ, Newman DK. Lyn and PECAM-1 function as interdependent inhibitors of platelet aggregation. Blood. (2011) 117:3903–6. doi: 10.1182/blood-2010-09-304816
24. Wei W, Peng C, Gu R, Yan X, Ye J, Xu Z, et al. Urolithin A attenuates RANKL-induced osteoclastogenesis by co-regulating the p38 MAPK and Nrf2 signaling pathway. Eur J Pharmacol. (2022) 921:174865. doi: 10.1016/j.ejphar.2022.174865
25. Teitelbaum SL. Osteoclasts: what do they do and how do they do it? Am J Pathol. (2007) 170:427–35. doi: 10.2353/ajpath.2007.060834
26. Karin M, Cao Y, Greten FR, Li ZW. NF-kappaB in cancer: from innocent bystander to major culprit. Nat Rev Cancer. (2002) 2:301–10. doi: 10.1038/nrc780
27. Teitelbaum SL, Ross FP. Genetic regulation of osteoclast development and function. Nat Rev Genet. (2003) 4:638–49. doi: 10.1038/nrg1122
28. Xue C, Luo H, Wang L, Deng Q, Kui W, Da W, et al. Aconine attenuates osteoclast-mediated bone resorption and ferroptosis to improve osteoporosis via inhibiting NF-κB signaling. Front Endocrinol (Lausanne). (2023) 14:1234563. doi: 10.3389/fendo.2023.1234563
29. Takayanagi H, Kim S, Koga T, Nishina H, Isshiki M, Yoshida H, et al. Induction and activation of the transcription factor NFATc1 (NFAT2) integrate RANKL signaling in terminal differentiation of osteoclasts. Dev Cell. (2002) 3:889–901. doi: 10.1016/S1534-5807(02)00369-6
30. Kim HJ, Warren JT, Kim SY, Chappel JC, DeSelm CJ, Ross FP, et al. Fyn promotes proliferation, differentiation, survival and function of osteoclast lineage cells. J Cell Biochem. (2010) 111:1107–13. doi: 10.1002/jcb.v111:5
31. Matsumae G, Shimizu T, Tian Y, Takahashi D, Ebata T, Alhasan H, et al. Targeting thymidine phosphorylase as a potential therapy for bone loss associated with periprosthetic osteolysis. Bioeng Transl Med. (2021) 6:e10232. doi: 10.1002/btm2.10232
32. Fox SB, Moghaddam A, Westwood M, Turley H, Bicknell R, Gatter KC, et al. Platelet-derived endothelial cell growth factor/thymidine phosphorylase expression in normal tissues: an immunohistochemical study. J Pathol. (1995) 176:183–90. doi: 10.1002/path.1711760212
33. Bijnsdorp IV, Capriotti F, Kruyt FA, Losekoot N, Fukushima M, Griffioen AW, et al. Thymidine phosphorylase in cancer cells stimulates human endothelial cell migration and invasion by the secretion of angiogenic factors. Br J Cancer. (2011) 104:1185–92. doi: 10.1038/bjc.2011.74
34. Hotchkiss KA, Ashton AW, Schwartz EL. Thymidine phosphorylase and 2-deoxyribose stimulate human endothelial cell migration by specific activation of the integrins alpha 5 beta 1 and alpha V beta 3. J Biol Chem. (2003) 278:19272–9. doi: 10.1074/jbc.M212670200
35. Kučerová L, Dolina J, Dastych M, Bartušek D, Honzík T, Mazanec J, et al. Mitochondrial neurogastrointestinal encephalomyopathy imitating Crohn’s disease: a rare cause of malnutrition. J Gastrointestin Liver Dis. (2018) 27:321–5. doi: 10.15403/jgld.2014.1121.273.kuc
36. Shah SAY, Shakeel HA, Hassan WU. Rare pathogenic mutation in the thymidine phosphorylase gene (TYMP) causing mitochondrial neurogastrointestinal encephalomyelopathy. BMJ Neurol Open. (2022) 4:e000287. doi: 10.1136/bmjno-2022-000287
37. Mojtabavi H, Fatehi F, Shahkarami S, Rezaei N, Nafissi S. Novel mutations of the TYMP gene in mitochondrial neurogastrointestinal encephalomyopathy: case series and literature review. J Mol Neurosci. (2021) 71:2526–33. doi: 10.1007/s12031-021-01822-w
38. Filosto M, Cotti Piccinelli S, Caria F, Gallo Cassarino S, Baldelli E, Galvagni A, et al. Mitochondrial neurogastrointestinal encephalomyopathy (MNGIE-MTDPS1). J Clin Med. (2018) 7(11):389. doi: 10.3390/jcm7110389
39. Wang L, Huang X, Chen Y, Jin X, Li Q, Yi TN. Prognostic value of TP/PD-ECGF and thrombocytosis in gastric carcinoma. Eur J Surg Oncol. (2012) 38:568–73. doi: 10.1016/j.ejso.2012.04.008
40. Bronckaers A, Gago F, Balzarini J, Liekens S. The dual role of thymidine phosphorylase in cancer development and chemotherapy. Med Res Rev. (2009) 29:903–53. doi: 10.1002/med.20159
41. Yao L, Itoh S, Furuta I. Thymidine phosphorylase expression in oral squamous cell carcinoma. Oral Oncol. (2002) 38:584–90. doi: 10.1016/S1368-8375(01)00113-0
42. Chujo M, Miura T, Kawano Y, Miyawaki M, Imakiire T, Hayashita Y, et al. Thymidine phosphorylase levels and dihydropyrimidine dehydrogenase levels in non-small cell lung cancer tissues. Oncol Rep. (2006) 16:777–80. doi: 10.3892/or.16.4.777
43. Sadahiro S, Suzuki T, Tanaka A, Okada K, Nagase H, Uchida J. Association of right-sided tumors with high thymidine phosphorylase gene expression levels and the response to oral uracil and tegafur/leucovorin chemotherapy among patients with colorectal cancer. Cancer Chemother Pharmacol. (2012) 70:285–91. doi: 10.1007/s00280-012-1909-8
44. Hasegawa K, Okamoto H, Kawamura K, Kato R, Kobayashi Y, Sekiya T, et al. The effect of chemotherapy or radiotherapy on thymidine phosphorylase and dihydropyrimidine dehydrogenase expression in cancer of the uterine cervix. Eur J Obstetrics Gynecology Reprod Biol. (2012) 163:67–70. doi: 10.1016/j.ejogrb.2012.03.014
45. Shimabukuro T, Matsuyama H, Baba Y, Jojima K, Suyama K, Aoki A, et al. Expression of thymidine phosphorylase in human superficial bladder cancer. Int J Urol. (2005) 12:29–34. doi: 10.1111/j.1442-2042.2004.00992.x
46. Bijnsdorp IV, de Bruin M, Laan AC, Fukushima M, Peters GJ. The role of platelet-derived endothelial cell growth factor/thymidine phosphorylase in tumor behavior. Nucleosides Nucleotides Nucleic Acids. (2008) 27:681–91. doi: 10.1080/15257770802143988
47. O’Byrne KJ, Koukourakis MI, Giatromanolaki A, Cox G, Turley H, Steward WP, et al. Vascular endothelial growth factor, platelet-derived endothelial cell growth factor and angiogenesis in non-small-cell lung cancer. Br J Cancer. (2000) 82:1427–32. doi: 10.1054/bjoc.1999.1129
48. Liu H, Liu Z, Du J, He J, Lin P, Amini B, et al. Thymidine phosphorylase exerts complex effects on bone resorption and formation in myeloma. Sci Transl Med. (2016) 8:353ra113. doi: 10.1126/scitranslmed.aad8949
49. Kitazono M, Takebayashi Y, Ishitsuka K, Takao S, Tani A, Furukawa T, et al. Prevention of hypoxia-induced apoptosis by the angiogenic factor thymidine phosphorylase. Biochem Biophys Res Commun. (1998) 253:797–803. doi: 10.1006/bbrc.1998.9852
50. Li W, Tanaka K, Ihaya A, Fujibayashi Y, Takamatsu S, Morioka K, et al. Gene therapy for chronic myocardial ischemia using platelet-derived endothelial cell growth factor in dogs. Am J Physiol Heart Circ Physiol. (2005) 288:H408–15. doi: 10.1152/ajpheart.00176.2004
51. Li W, Tanaka K, Morioka K, Takamori A, Handa M, Yamada N, et al. Long-term effect of gene therapy for chronic ischemic myocardium using platelet-derived endothelial cell growth factor in dogs. J Gene Med. (2008) 10:412–20. doi: 10.1002/jgm.v10:4
52. Fresno Vara JA, Casado E, de Castro J, Cejas P, Belda-Iniesta C, González-Barón M. PI3K/Akt signalling pathway and cancer. Cancer Treat Rev. (2004) 30:193–204. doi: 10.1016/j.ctrv.2003.07.007
53. Miricescu D, Totan A, Stanescu-Spinu II, Badoiu SC, Stefani C, Greabu M. PI3K/AKT/mTOR signaling pathway in breast cancer: from molecular landscape to clinical aspects. Int J Mol Sci. (2021) 22:173. doi: 10.3390/ijms22010173
54. Tian L-Y, Smit DJ, Jücker M. The role of PI3K/AKT/mTOR signaling in hepatocellular carcinoma metabolism. Int J Mol Sci. (2023) 24:2652. doi: 10.3390/ijms24032652
55. Farhan M, Wang H, Gaur U, Little PJ, Xu J, Zheng W. FOXO signaling pathways as therapeutic targets in cancer. Int J Biol Sci. (2017) 13:815–27. doi: 10.7150/ijbs.20052
56. Guo Q, Xiong Y, Song Y, Hua K, Gao S. ARHGAP17 suppresses tumor progression and up-regulates P21 and P27 expression via inhibiting PI3K/AKT signaling pathway in cervical cancer. Gene. (2019) 692:9–16. doi: 10.1016/j.gene.2019.01.004
57. Mayo LD, Donner DB. A phosphatidylinositol 3-kinase/Akt pathway promotes translocation of Mdm2 from the cytoplasm to the nucleus. Proc Natl Acad Sci U.S.A. (2001) 98:11598–603. doi: 10.1073/pnas.181181198
58. Ramadan RA, Moghazy TF, Hafez R, Morsi H, Samir M, Shamesya M. Significance of expression of pyrimidine metabolizing genes in colon cancer. Arab J Gastroenterol. (2020) 21:189–93. doi: 10.1016/j.ajg.2020.07.006
59. Deves C, Rostirolla DC, Martinelli LK, Bizarro CV, Santos DS, Basso LA. The kinetic mechanism of Human Thymidine Phosphorylase - a molecular target for cancer drug development. Mol Biosyst. (2014) 10:592–604. doi: 10.1039/C3MB70453J
60. Liu W, Zhang J, Yao X, Jiang C, Ni P, Cheng L, et al. Bevacizumab-enhanced antitumor effect of 5-fluorouracil via upregulation of thymidine phosphorylase through vascular endothelial growth factor A/vascular endothelial growth factor receptor 2-specificity protein 1 pathway. Cancer Sci. (2018) 109:3294–304. doi: 10.1111/cas.2018.109.issue-10
61. Tarar A, Alyami EM, Peng CA. Mesenchymal stem cells anchored with thymidine phosphorylase for doxifluridine-mediated cancer therapy. RSC Adv. (2021) 11:1394–403. doi: 10.1039/D0RA10263F
62. Baris A, Fraile-Bethencourt E, Eubanks J, Khou S, Anand S. Thymidine phosphorylase facilitates retinoic acid inducible gene-I induced endothelial dysfunction. Cell Death Dis. (2023) 14:294. doi: 10.1038/s41419-023-05821-0
63. Bera H, Ojha Pk, Tan BJ, Sun L, Dolzhenko AV, Chui WK, et al. Discovery of mixed type thymidine phosphorylase inhibitors endowed with antiangiogenic properties: synthesis, pharmacological evaluation and molecular docking study of 2-thioxo-pyrazolo[1,5-a][1,3,5]triazin-4-ones. Part II. Eur J Med Chem. (2014) 78:294–303. doi: 10.1016/j.ejmech.2014.03.063
64. Shahzad SA, Sarfraz A, Yar M, Khan ZA, Naqvi SAR, Naz S, et al. Synthesis, evaluation of thymidine phosphorylase and angiogenic inhibitory potential of ciprofloxacin analogues: Repositioning of ciprofloxacin from antibiotic to future anticancer drugs. Bioorg Chem. (2020) 100:103876. doi: 10.1016/j.bioorg.2020.103876
65. Bronckaers A, Aguado L, Negri A, Camarasa MJ, Balzarini J, Pérez-Pérez MJ, et al. Identification of aspartic acid-203 in human thymidine phosphorylase as an important residue for both catalysis and non-competitive inhibition by the small molecule "crystallization chaperone" 5'-O-tritylinosine (KIN59). Biochem Pharmacol. (2009) 78:231–40. doi: 10.1016/j.bcp.2009.04.011
66. Belcher A, Zulfiker AHM, Li OQ, Yue H, Gupta AS, Li W. Targeting thymidine phosphorylase with tipiracil hydrochloride attenuates thrombosis without increasing risk of bleeding in mice. Arterioscler Thromb Vasc Biol. (2021) 41:668–82. doi: 10.1161/ATVBAHA.120.315109
67. Wang X, Peng I, Peng CA. Eradication of cancer cells using doxifluridine and mesenchymal stem cells expressing thymidine phosphorylase. Bioengineering (Basel). (2024) 11(12):1194. doi: 10.3390/bioengineering11121194
68. Toi M, Bando H, Horiguchi S, Takada M, Kataoka A, Ueno T, et al. Modulation of thymidine phosphorylase by neoadjuvant chemotherapy in primary breast cancer. Br J Cancer. (2004) 90:2338–43. doi: 10.1038/sj.bjc.6601845
69. Pérez-Pérez MJ, Priego EM, Hernández AI, Camarasa MJ, Balzarini J, Liekens S. Thymidine phosphorylase inhibitors: recent developments and potential therapeutic applications. Mini Rev Med Chem. (2005) 5:1113–23. doi: 10.2174/138955705774933301
70. Peri S, Biagioni A, Versienti G, Andreucci E, Staderini F, Barbato G, et al. Enhanced vasculogenic capacity induced by 5-fluorouracil chemoresistance in a gastric cancer cell line. Int J Mol Sci. (2021) 22(14):7698. doi: 10.3390/ijms22147698
71. Paladhi A, Daripa S, Mondal I, Hira SK. Targeting thymidine phosphorylase alleviates resistance to dendritic cell immunotherapy in colorectal cancer and promotes antitumor immunity. Front Immunol. (2022) 13:988071. doi: 10.3389/fimmu.2022.988071
72. Koyama M, Osada E, Akiyama N, Eto K, Manome Y. Effect of thymidine phosphorylase gene demethylation on sensitivity to 5-fluorouracil in colorectal cancer cells. Anticancer Res. (2022) 42:837–44. doi: 10.21873/anticanres.15541
73. Derwinger K, Lindskog EB, Palmqvist E, Wettergren Y. Changes in thymidine phosphorylase gene expression related to treatment of rectal cancer. Anticancer Res. (2013) 33:2447–51.
74. Peeters KC, Marijnen CA, Nagtegaal ID, Kranenbarg EK, Putter H, Wiggers T, et al. The TME trial after a median follow-up of 6 years: increased local control but no survival benefit in irradiated patients with resectable rectal carcinoma. Ann Surg. (2007) 246:693–701. doi: 10.1097/01.sla.0000257358.56863.ce
75. Sauer R, Liersch T, Merkel S, Fietkau R, Hohenberger W, Hess C, et al. Preoperative versus postoperative chemoradiotherapy for locally advanced rectal cancer: results of the German CAO/ARO/AIO-94 randomized phase III trial after a median follow-up of 11 years. J Clin Oncol. (2012) 30:1926–33. doi: 10.1200/JCO.2011.40.1836
76. Sadahiro S, Suzuki T, Tanaka A, Okada K, Saito G, Kamijo A, et al. Increase in gene expression of TYMP, DPYD and HIF1A are associated with response to preoperative chemoradiotherapy including S-1 or UFT for rectal cancer. Anticancer Res. (2016) 36:2433–40.
77. Jia X, Zhang T, Sun J, Lin H, Bai T, Qiao Y, et al. Rs11479 in thymidine phosphorylase associated with prognosis of patients with colorectal cancer who received capecitabine-based adjuvant chemotherapy. Pharmgenomics Pers Med. (2023) 16:277–89. doi: 10.2147/PGPM.S397382
78. Kaidi D, Szeponik L, Yrlid U, Wettergren Y, Bexe Lindskog E. Impact of thymidine phosphorylase and CD163 expression on prognosis in stage II colorectal cancer. Clin Transl Oncol. (2022) 24:1818–27. doi: 10.1007/s12094-022-02839-2
79. Goto T, Shinmura K, Yokomizo K, Sakuraba K, Kitamura Y, Shirahata A, et al. Expression levels of thymidylate synthase, dihydropyrimidine dehydrogenase, and thymidine phosphorylase in patients with colorectal cancer. Anticancer Res. (2012) 32:1757–62.
80. Du YB, Zhang TF, Cui K, Jin SL, Xi Y, Ma W. The influence of Thymidine Phosphorylase genetic variation on clinical outcomes and safety of colorectal cancer patients received adjuvant chemotherapy after R0 resection. Zhonghua Yi Xue Za Zhi. (2018) 98:2569–73. doi: 10.3760/cma.j.issn.0376-2491.2018.32.007
81. Chen SA, Zhang JP, Wang N, Chen J. Identifying TYMP as an immune prognostic marker in clear cell renal cell carcinoma. Technol Cancer Res Treat. (2023) 22:15330338231194555. doi: 10.1177/15330338231194555
82. Liu M, Pan Q, Xiao R, Yu Y, Lu W, Wang L. A cluster of metabolism-related genes predict prognosis and progression of clear cell renal cell carcinoma. Sci Rep. (2020) 10:12949. doi: 10.1038/s41598-020-67760-6
83. Yang Y, Jiang L, Wang S, Chen H, Yi M, Wu Y, et al. A comprehensive pan-cancer analysis on the immunological role and prognostic value of TYMP in human cancers. Transl Cancer Res. (2022) 11:3187–208. doi: 10.21037/tcr-22-502
84. Li Y, Fan C, Hu Y, Zhang W, Li H, Wang Y, et al. Multi-cohort validation: A comprehensive exploration of prognostic marker in clear cell renal cell carcinoma. Int Immunopharmacol. (2024) 135:112300. doi: 10.1016/j.intimp.2024.112300
85. Huang L, Liu S, Lei Y, Wang K, Xu M, Chen Y, et al. Systemic immune-inflammation index, thymidine phosphorylase and survival of localized gastric cancer patients after curative resection. Oncotarget. (2016) 7:44185–93. doi: 10.18632/oncotarget.v7i28
Keywords: nucleotide metabolism, thymidine phosphorylase, physiological functions, tumorigenesis, anticancer therapy
Citation: Huang B, Yuan Q, Sun J, Wang C and Yang D (2025) Thymidine phosphorylase in nucleotide metabolism: physiological functions and its implications in tumorigenesis and anti-cancer therapy. Front. Immunol. 16:1561560. doi: 10.3389/fimmu.2025.1561560
Received: 16 January 2025; Accepted: 28 March 2025;
Published: 15 April 2025.
Edited by:
Renjun Gu, Nanjing University of Chinese Medicine, ChinaCopyright © 2025 Huang, Yuan, Sun, Wang and Yang. This is an open-access article distributed under the terms of the Creative Commons Attribution License (CC BY). The use, distribution or reproduction in other forums is permitted, provided the original author(s) and the copyright owner(s) are credited and that the original publication in this journal is cited, in accordance with accepted academic practice. No use, distribution or reproduction is permitted which does not comply with these terms.
*Correspondence: Dong Yang, eWFuZ2Rvbmd5aTIwMTBAMTI2LmNvbQ==
†These authors have contributed equally to this work