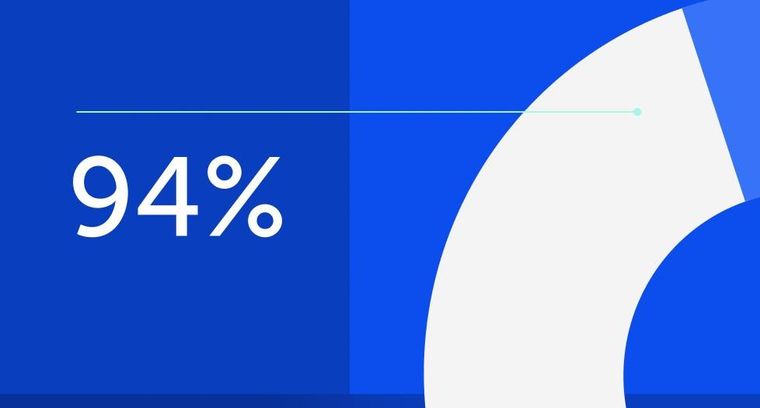
94% of researchers rate our articles as excellent or good
Learn more about the work of our research integrity team to safeguard the quality of each article we publish.
Find out more
REVIEW article
Front. Immunol., 01 April 2025
Sec. Alloimmunity and Transplantation
Volume 16 - 2025 | https://doi.org/10.3389/fimmu.2025.1557707
This article is part of the Research TopicRenal Fibrosis and Renal TransplantationView all 6 articles
Gasdermins (GSDMs), functioning as membrane perforating proteins, can be activated by canonical inflammasomes, noncanonical inflammasomes, as well as non-inflammasomes, leading to cell pyroptosis and the subsequent release of inflammatory mediators. Increasing evidence has implicated that GSDMs are associated with chronic kidney disease (CKD), including diabetes nephropathy, lupus nephritis, obstructive nephropathy, and crystalline nephropathy. This review centers on the role of GSDMs-mediated pyroptosis in the pathogenesis of CKD, providing novel ideas for enhancing the prognosis and therapeutic strategies of CKD.
Chronic kidney disease (CKD), a pressing concern in global public health, imposes a considerable disease burden and poses a major challenge (1). CKD ranked as the 16th most common cause of death globally in 2016 and is expected to rise to 5th by 2040 (2). The global prevalence of CKD is about 9.1%, while awareness of CKD among the general population and high-risk groups, at just only 6% and 10% (3, 4). Currently, the definition of CKD is structural destruction or impaired function of the kidney lasting 3 months or more (4). Renal fibrosis, a common pathological manifestation of CKD, is characterized by glomerulosclerosis, tubular atrophy, interstitial fibrosis, and persistent inflammation (5, 6). With the continuous destruction of renal tissue, renal function progressively declines, and eventually it will develop into end stage renal disease. The ensuing need for renal replacement therapies, including peritoneal dialysis, hemodialysis, and kidney transplantation, undoubtedly further exacerbate the economic burden on patients.
Pyroptosis, a necrotic programmed cell death mediated by gasdermins (GSDMs), is characterized by cell membrane rupture and the release of inflammatory factors such as interleukin-1 (IL-1β) and interleukin-18 (IL-18) (7, 8). GSDMs were initially identified in macrophages in 1992 and subsequently named in 2001 (9, 10). Shao et al. characterized GSDMs as the executors of pyroptosis, and the in 2018, the definition was revised to cell death involving GSDMs-formed membrane pores (7, 11, 12). Pyroptosis primarily occurs in myeloid phagocytes, including macrophages, dendritic cells, and neutrophils, with evidence later confirming its presence in CD4+ T cells, keratinocytes, epithelial cells, and neurons as well (13, 14). Pyroptosis is involved in innate immunity by eliminating pathogens, including bacteria, fungi, and viruses, through the mediation of inflammatory response. However, uncontrolled pyroptosis has the potential to induce inflammation in adjacent cells and tissues, thereby contributing to the initiation and progression of diseases (15–17).
Recently, a wealth of evidence indicates that GSDMs-mediated pyroptosis plays a pivotal role in the pathogenesis and progression of CKD. This paper presents an overview of the fundamental characteristics of the GSDMs family and examines and summarizes their roles and potential mechanisms in CKD, providing novel insights for enhancing the prognosis and treatment of this condition.
Gasdermins were initially discovered in the gastrointestinal tract and skin of mice, hence they were named “Gasdermins (GSDMs)” (18, 19). In humans, there are six GSDMs genes: GSDMA, GSDMB, GSDMC, GSDMD, GSDME (also known as DNFA5), and PJVK (pejvakin, also known as GSDMF, DFNB59). In mice, there are three Gsdma genes (Gsdma1-3), four Gsdmc genes (Gsdmc1-4), Gsdmd, Gsdme, and Pjvk, while the Gsdmb orthologs are lacking (20, 21) (Table 1). In kidney tissue, GSDMD was highly expressed, GSDMC and GSDME were moderately expressed, GSDMB was low, and GSDMA was not detected (21). Except for PJVK, members of the GSDMs protein family are composed of three parts, namely, the N-terminal pore-forming domain (PFD, NTD), the linker part, and the C-terminal inhibitory domain (RD, CTD) (22). When the body is exposed to various internal and external stimuli, GSDMs undergo cleavage by cysteinyl aspartate-specific protease (Caspases) or granzymes, and the dissociated N-terminal structural oligomers punch holes in the cell membrane, mediating the release of inflammatory factors, as well as the flux of water and electrolyte flow, ultimately leading to pyroptosis.
Human GSDMA is located on chromosome 17 and is mainly expressed in epithelial cells of the skin and upper digestive tract (18). Gsdma1 is expressed specifically in the heart region, Gsdma2 is expressed in the fundus and pylorus, and Gsdma3 is mainly expressed in the skin (19). Overexpression of GSDMA is associated with glioma immune escape and poor prognosis in patients, while GSDMA knockdown increases T cell antitumor response via immunotherapy (23). GSDMA can promote the development of esophageal cancer and cisplatin resistance (24). In addition, GSDMA has been implicated in systemic sclerosis, asthma, intestinal bowel disease (IBD), and other immune-related diseases (25–27). However, the role of GSDMA in kidney disease has not been previously reported.
Human GSDMB is also located on chromosome 17 and is mainly expressed in the gastrointestinal tract, thyroid, skin, lungs, and kidneys (18, 28). Besides normal tissues, GSDMB is also highly expressed in tumor tissues, such as bladder cancer, clear cell renal cell carcinoma, gastric cancer, and non-small cell lung cancer (29–32). Furthermore, GSDMB is also associated with IBD, asthma, allergic rhinitis, psoriasis, and other diseases (33–36).
Human GSDMC is located on chromosome 8 (8q24.1), while mouse Gsdmc is located on chromosome 15, and is mainly expressed in skin, spleen, trachea, and gastrointestinal tract (37–39). Under hypoxic conditions, GSDMC transcription was enhanced in breast tumor cells, and GSDMC in turn converted TNF-α-induced apoptosis to pyroptosis (40). In addition, GSDMC is also associated with esophageal cancer, liver cancer, colorectal cancer, pancreatic cancer, and renal clear cell carcinoma (41–46).
Similar to GSDMC, human GSDMD is also located on chromosome 8 (8q24.3), and mouse Gsdmd is located on chromosome 15, and is predominantly expressed in tissues such as the gastrointestinal tract, kidney, and skin (47, 48). GSDMD is the first member of the GSDM family to be found to perform pyroptosis, and the mechanism is well understood (49). GSDMD is mainly cleaved by Caspases at the Asp275 or Asp276 site, Caspase-1/4/5 in humans and Caspase-1/11 in mice (47, 50). GSDMD is involved in a variety of inflammatory diseases such as non-alcoholic steatohepatitis, acute pancreatitis, colitis, sepsis, systemic lupus erythematosus, psoriasis (51–55), and is also associated with acute myocardial infarction, stroke, colon cancer, and other non-inflammatory diseases (56–58). In addition, GSDMD is associated with renal disease, which is described in detail below.
GSDME, also known as deafness, autosomal dominant 5 (DFNA5), is localized on chromosome 7 in humans and chromosome 6 in mice, exhibiting ubiquitous expression across various normal tissues, notably including the gastrointestinal tract and kidney (59). In addition to tumors, GSDME is also involved in hearing loss, neurodegeneration, atherosclerosis, and many renal diseases, which are described in detail below (60–64).
PJVK, situated on chromosome 2 in both humans and mice, is majored expresses in the inner ear and involved in auditory pathways (65). Mutations in PJVK can lead to hearing loss (66). The role of PJVK in renal diseases has not been described.
At present, there are two main types of pyroptosis pathways mediated by Gasdermins: inflammasome-dependent pathway (canonical pathway and non-canonical pathway) and non-inflammasome-dependent pathway (Figures 1, 2).
Figure 1. Inflammasome-dependent pathway (canonical pathway and non-canonical pathway). The canonical pathway: NLRP3, ASC, and pro-Caspase-1 assemble into inflammasome in response to PAMP or DAMP. After inflammasome assembly, activated Caspase-1 cleaves pro-IL-1β and pro-IL-18 to mature IL-1β and IL-18, and simultaneously cleaves GSDMD to release the N terminus of GSDMD. GSDMD-NT oligomerize in the cell membrane to form transmembrane pores, causing K+ efflux, Na+ and water influx, followed by cell swelling and rupture, releasing IL-1β and IL-18, leading to pyroptosis. The non-canonical pathway: inflammasome Caspase-4/5/11 recognizes Lipopolysaccharide (LPS). Activated Caspase-4/5/11 cleaved GSDMD to induce pyroptosis. Caspase-11 activates the Pannexin-1 channel and releases ATP, which further activates the NLRP3 inflammasome to indirectly cut pro-IL-1β and pro-IL-18.
Figure 2. Non-inflammasome-dependent pathway. Streptococcal exotoxin B (SpeB), secreted by human pathogen Group A Streptococcus (GAS), and Caspase-3/4/7 are able to cleave GSDMA to induce pyroptosis. Granzyme A (GZMA), secreted by natural killer cells and cytotoxic T lymphocytes, and Caspase-1/3/4/6/7 cleave GSDMB. α-ketoglutarate (α-KG) induces pyroptosis through Caspase-8 cleavage at GSDMC. In addition, GSDMC is also cleaved by Caspase-6 to induce pyroptosis. Neutrophil elastase (ELANE) and cathepsin G (CatG) can activate GSDMD. Caspase-8 activated by Yersinia can cleave GSDMD and GSDME to trigger pyroptosis. GSDMC and GSDME can transform apoptosis into pyroptosis.
In the canonical pathway, inflammasomes are intricate multi-molecular assemblies that encompass pattern recognition receptors (PRRs), apoptosis-associated speck like protein (ASC), and pro-Caspase-1 (67). As sensors that receive pathogen-associated molecular patterns (PAMP) or damage associated molecular patterns (DAMP) stimuli, PRRs mainly include NLR/NOD-like receptors (NLRP1, NLRP3, NLRP6, NLRP9, NLRC4), Pyrin, and AIM2-like receptor (68). NLRP1 can be activated by Anthrax lethal toxin (LeTx), bacterial muramyl dipeptide, T. gondii, and ATP depletion (69). NLRP3 senses a variety of stimuli, including microbial toxins, viral RNA, ATP, ROS, uric acid crystals, cholesterol, and particulate matter (70–72). Microbial RNA, metabolites, Lipoteichoic acid, and LPS can be used as NLRP6 activation signals (73–75). NLRP9 and AIM2 inflammasomes can recognize pathogen dsDNA (76, 77). Bacterial flagellin can induce the activation of NLRC4 inflammasome (78). Pyrin cannot directly recognize PAMP or DAMP but can be activated when bacterial toxins induce GTPase inactivation (79). PAMP or DAMP activates TLRs/MyD88/NF-κB signaling pathway, leading to transcriptional upregulation of NLRP3, ASC, pro-Caspase-1, pro-IL-1β, and pro-IL-18 (80, 81). After inflammasome assembly, activated Caspase-1 cleaves pro-IL-1β and pro-IL-18 to mature IL-1β and IL-18, and simultaneously cleaves GSDMD to release the N terminus of GSDMD (7, 82). GSDMD-NTs oligomerize in the cell membrane to form transmembrane pores to release IL-1β and IL-18 as well as lead to pyroptosis (15, 83).
In the non-canonical pathway, inflammasomes, human Caspase-4/5 and mouse Caspase-11, specifically recognize the lipopolysaccharide (LPS) by N-terminal CARD (84). The cleavage of GSDMD by Caspase-4/5/11 triggers the process of pyroptosis (47, 50). Caspase-11 fails to cleave pro-IL-1β and pro-IL-18 like Caspase-4/5, but instead activates Pannexin-1 and releases ATP. Subsequently, ATP binds to the P2X7 receptor (P2X7R), causing K+ efflux and further activating NLRP3 inflammasome to indirectly cleave pro-IL-1β and pro-IL-18 (85).
The human pathogen Group A Streptococcus (GAS) secretes the cysteine protein streptococcal exotoxin B (SpeB), which cleaves GSDMA at the junction area of Gln246 and triggers pyroptosis of skin epithelial cells and keratinocytes, independent of Caspase (86, 87). In HEK293T cells, Caspase-3/4/7 are able to cleave GSDMA to induce pyroptosis (88). Granzyme A (GZMA), secreted by natural killer cells and cytotoxic T lymphocytes, cleaves GSDMB at the K244 site of the junction and targets phospholipids on the membrane of Gram-negative bacteria to directly kill bacteria (89, 90). GSDMB can bind to Caspase-1/3/4/6/7 and promote pyroptosis (91, 92). It has also been reported that activated Caspase-7 cuts GSDMB at the D91 site to block GSDMB-mediated pyroptosis (93). α-Ketoglutarate (α-KG) contributes to pyroptosis by enabling Caspase-8 to cleave GSDMC at the Asp240 site, with a similar cleavage occurring at the Asp233 site in mouse GSDMC4 (94). In addition, GSDMC is also cleaved by Caspase-6 to induce pyroptosis (40). Under hypoxia conditions, the increased expression of GSDMC can transform the apoptosis induced by Caspase-8 into pyroptosis (95). Besides Caspase-1/4/5/11, neutrophil elastase (ELANE) and cathepsin G (CatG) can also activate GSDMD (96, 97). Caspase-8 activated by Yersinia can cleave GSDMD and GSDME to trigger pyroptosis (98). GSDME can also be specifically cleaved by Caspase-3 at the Asp270 site to induce pyroptosis, which is transformed into apoptosis after GSDME knockout or promoter demethylation (11, 95, 99, 100).
Inflammation and fibrosis are prevalent pathological characteristics in all forms of CKD, and persistent inflammation can drive fibrosis progression, leading to the pathogenesis of CKD (5, 101). Damaged cells secrete pro-inflammatory cytokines to recruit inflammatory cells for infiltration, and release pro-fibrotic mediators to promote proliferation of myofibroblasts, resulting in the production and deposition of a large amount of extracellular matrix, ultimately causing pathological changes and impaired renal function (101, 102). Recently, a great number of studies has demonstrated that GSDMs-mediate pyroptosis, a pro-inflammatory form of cell death, which is associated with the pathogenesis of various CKD, including diabetic nephropathy, lupus nephritis, obstructive nephropathy, and crystal nephropathy (Table 2, Figure 3).
Figure 3. Summary of potential mechanisms of GSDMD/GSDME-mediated pyroptosis in chronic kidney disease. (A) Diabetic Nephropathy. Under high glucose or high-fat diet/streptozotocin stimulation, GSDMD is activated by Caspase-1/4/11, while GSDME is activated by Caspase-3, triggering pyroptosis, leading to impaired renal function, increased inflammation and fibrosis. (B) Lupus Nephritis. After the generation of autoantibodies by injection of pristane, GSDMD and GSDME can be cleaved by Caspase-1 and Caspase-8, respectively. Subsequently, pyroptosis and renal damage occur. (C) Obstructive Nephropathy. Following obstruction of the ureter, pyroptosis induced by Caspase-1/11/GSDMD and Caspase3/GSDME pathways can be activated, mediating renal injury, inflammation, and fibrosis. (D) Crystalline Nephropathy. Calcium oxalate can induce pyroptosis and cause renal damage through the Caspase-1/11/GSDMD pathway. Uric acid releases cathepsin B through the p53 pathway and subsequently activates the Caspase-1/11/GSDMD pathway. Furthermore, uric acid directly triggered Caspase-8/Caspase-3/NLRP3/GSDME-mediated pyroptosis, resulting in kidney damage.
Diabetic nephropathy (DN) is a prevalent microvascular complication of diabetes mellitus, emerging as a major type of CKD (103). Pathological changes of diabetic nephropathy include cells damage (podocytes, renal tubular epithelial cells (RTECs), endothelial cells), glomerular sclerosis, glomerular basement membrane thickening, mesangial matrix increase, tubular interstitial inflammation, and fibrosis (104).
Recent studies have revealed a correlation between GSDMs-mediated pyroptosis and DN, suggesting a potential mechanistic link between the two processes. In a recent study, high glucose (HG) or streptozotocin (STZ) stimulated pyroptosis in renal cells (podocytes, renal tubules, human glomerular endothelial cells, and mouse glomerular endothelial cells) though the Caspase-11/GSDMD pathway, resulting in impaired renal function, manifested by significant increases in serum nitrogen, serum creatinine, and urinary albumin levels (105). After knocking down the Gsdmd gene in DN mice, pyroptosis and IL-18 were markedly reduced, resulting in improved renal function (105).An et al. showed that punicalagin effectively inhibit NLRP3/Caspase-1/GSDMD/IL-1β pathway, and significantly ameliorates renal function, glomerular sclerosis, renal interstitial fibrosis and other pathological changes in diabetic mice (106). Cheng et al. also demonstrated that the expression levels of Caspase-4/11, GSDMD-N, IL-1β, and IL-18 were markedly elevated in HG-treated podocytes or podocytes from diabetic mice, and the deficiency of Caspase-11 or Gsdmd alleviated inflammation and podocytes loss in diabetic mice (107). Immunohistochemistry staining showed that the levels of fibronectin, alpha-smooth muscle actin, and the number of F4/80+ macrophages in DN mice were higher than the control group (108). Western blotting results indicated that the expressions of GSDMD-FL, GSDMD-NT, IL-1β, IL-18, and α-SMA were increased in DN mice, suggesting that pyroptosis was associated with renal tubule injury and interstitial inflammation (108). In the rat DN model and HG-treated human kidney proximal tubular epithelial cells (HK-2 cells), Caspase-1/3, GSDME, GSDME-NT, and IL-1β were significantly upregulated. In rats that underwent renal cortical injection of AAV9-shGSDME to knockdown Gsdme in the kidney, reduced renal injury and pyroptosis were observed, demonstrating that Caspase-3/GSDME promotes pyroptosis and contributes to renal injury in DN (109).
In addition, pyroptosis was also detected in renal biopsy tissues obtained from DN patients. When compared with patients with glomerular minor lesion, the expressions of Caspase-1 and GSDMD in renal tubular cells were increased in DN patients (108). Increased expression of GSDMD in DN patients was positively correlated with renal tubulointerstitial fibrosis and negatively correlated with renal function (110). Additionally, the expression of NLRP3 was upregulated in DN patients, and demonstrating a positive association with tubulointerstitial injury (111).
These studies indicate that GSDMD and GSDME are activated in DN, and GSDMD or GSMDE mediated-pyroptosis promotes the pathogenesis of DN.
Systemic lupus erythematosus (SLE) is a multisystem autoimmune disease characterized by autoantibody formation, immune complex deposition, and chronic inflammation (112). Kidneys are the affected organs in SLE, with up to 60% of patients developing lupus nephritis (LN) (113). The pathological mechanism of LN involves the deposition of immune complexes, infiltration of inflammatory cells, and injury or death of glomerular and tubular cell (114).
Studies have shown that characteristic autoantibodies and typical clinical and renal pathological changes of SLE can be produced after intraperitoneal injection of pristane (115, 116). Increased expressions of pyroptosis related proteins (NLRP3, GSDMD, and Caspase-1) and inflammatory factors (TNF-α and IL-1β) were observed in pristane-induced LN mice models (117). Cao et al. treated LN patients and MRL/lpr mice with a combination of mycophenolate mortiate, calcineurin inhibitors, and steroids, resulting in significant inhibition of Caspase-1/GSDMD-mediated pyroptosis and alleviation of disease progression (118). GSDME was observed to be highly expressed in the renal tubules of SLE patients and pristane-induced lupus mice, while Caspase-3/GSDME-mediated pyroptosis, autoantibody, glomerular IgG deposition and renal lesions were ameliorated in Gsdme-/- lupus mice (119).
The causes of obstructive nephropathy (ON) include urinary calculi, congenital stenosis, and tumor masses. The main characteristics of ON include renal dysfunction, inflammation, and renal interstitial fibrosis, which may progress to CKD with the progression of the disease (120, 121).
Several studies have used the unilateral ureteral obstruction (UUO) model to investigate the role of GSDMs in ON. In UUO model, Caspase-11 was activated to cleave GSDMD to mediate pyroptosis, which induced the formation neutrophil extracellular trap and the transformation of macrophage to myofibroblast, promoting inflammation and renal fibrosis (122). Furthermore, Li et al.’s research suggested that Caspase3/GSDME-mediated pyroptosis of renal tubular cells promoted the progression of renal injury and fibrosis induced by ureteral obstruction (63). In the UUO mice model, inhibition of Caspase-3 or loss of Gsdme was found to alleviate renal fibrosis (123). Disulfiram has been reported to directly inhibit GSDMD cleavage, thereby suppressing pyroptosis and cell fibrosis; however, it is ineffective in inhibiting pyroptosis once the process has already been initiated (124). Furthermore, Tongluo Yishen Detection has been proven to effectively inhibit pyroptosis and improve renal function and fibrosis by targeting NLRP3/Caspase-1/GSDMD pathway (125). YiShen HuoXue decoction can also inhibit this pathway to improve renal fibrosis and inflammation (126).
Crystals, including calcium oxalate (CaOx) and uric acid (UA), that deposit in the renal tubules and interstitium induce ischemia, tubule fibrosis, and inflammation through mechanisms involving tubular obstruction and cytotoxicity, ultimately leading to crystalline nephropathy (CN) (127, 128).
Oxalate nephropathy, although rare, has a poor prognosis and often develops into the final stage of CKD (129, 130). Studies have shown that CaOx induced RTECs and HK-2 cells injury through NLRP3/Caspase-1/GSDMD pathway (131, 132). H3 relaxin, with its anti-inflammatory and antioxidant properties, exerted a protective effect against oxalate nephropathy in rats by targeting the NLRP3/Caspase-1/GSDMD axis (133). Melatonin alleviated RTECs damage and renal CaOx crystal deposition by inhibiting non-canocial inflammasome mediated pyroptosis by NLRP3/Caspase-11/GSDMD (134).
Recent studies have shown that hyperuricemia plays a pathogenic role in the progression of kidney disease, leading to CKD (hyperuricemic nephropathy, HN) (135–137). GSDMD was significantly upregulated in renal tissue of experimental HN mice, and the loss of renal function and renal tubule fibrosis were improved after Gsdmd knockout (138). A study has shown that UA activated autophagy through the p53 pathway and released cathepsin B, which subsequently activated the NLRP3/Caspase-1,11/GSDMD pathway to participate in the development of experimental HN (139). A. manihot L. flower mitigates UA-induced RTECs damage by inhibiting Caspase-8/Caspase-3/NLRP3/GSDME-mediated pyroptosis (140).
Current studies have shown that GSDMs-mediated pyroptosis is involved in experimental CN, but its role in human CN remains to be determined.
Animal models of CKD provide powerful experimental basis for further exploring the pathogenesis of CKD, verifying the effectiveness of treatment methods. 5/6 nephrectomy (5/6Nx), by removing one whole kidney and two-thirds of the opposite kidney, simulates human CKD manifestations including renal fibrosis and decreased kidney function (141, 142). It is shown that Gsdme deletion significantly improves renal function and renal interstitial fibrosis in mice underwent 5/6Nx (123). Adenine-induced renal injury is also commonly used to mimic human CKD. Data indicated that pyroptosis-related proteins such as GSDMD were upregulated in adenine-induced CKD mice, and pyroptosis was significantly alleviated after butyrate intervention (143).
Here, we provide an overview indicating that GSDMD/GSDME-mediated pyroptosis is an important factor in the pathogenesis of CKD. However, several issues remain to be explored and elucidated through further research. First, it has been established that GSDMD and GSDME are associated with multiple types of CKD; however, the role of GSDMDA, GSDMB, GSDMC, and PJVK in CKD remains unclear. Second, in addition to being activated by Caspases to trigger pyroptosis, GSDMs can convert apoptosis to pyroptosis in some cases, so whether there is mutual conversion or interaction between pyroptosis and apoptosis in CKD. Third, at present, in animal and cellular experiments, some drugs and knocking out/down GSDMs can improve CKD; however, clinical trials are needed to verify. Therefore, we hope that future studies will focus on the design and development of drugs that specifically target GSDMD/GSDME, with the aim of improving the quality of life of CKD patients and will also further explore the functional roles by other members of GSDMs.
HG: Writing – original draft, Writing – review & editing. TX: Visualization, Writing – original draft, Writing – review & editing. YL: Investigation, Writing – original draft. ZX: Writing – review & editing. ZS: Writing – original draft. HY: Writing – original draft. HZ: Writing – original draft. WL: Writing – original draft. CY: Supervision, Writing – original draft. BG: Supervision, Writing – original draft, Writing – review & editing. SL: Funding acquisition, Writing – original draft, Writing – review & editing, Project administration. LY: Funding acquisition, Writing – original draft, Writing – review & editing.
The author(s) declare that financial support was received for the research and/or publication of this article. This article was supported by Shenzhen Longhua District Foundation of Science and Technology (Grant/Award Number: SZLHQJCYJ202002); Shenzhen Longhua District Science and Technology Innovation Special Fund Project (Grant/Award Numbers: 11501A20220923BF59236, 11501A20220923BE5B6B3, 11501A20220923BD5F291); Guangdong Engineering Technology Research Center (Grant/Award Numbers: 507204531040); Guangzhou Development Zone entrepreneurship leading talent project (Grant/Award Numbers: 2017-L153); Guangdong High tech Research and Development Center Project (Grant/Award Numbers: 2023B01012000010); Science and Technology Projects in Guangzhou (Grant/Award Numbers: 202201010196); Guangzhou entrepreneurship leading team(Grant/Award Numbers: 202009030005). Guangdong Basic and Applied Basic Research Foundation, Grant/Award Number: 2025A1515012512; Shenzhen Foundation of Science and Technology, Grant/Award Number: JCYJ20240813153002004; Shenzhen Longhua District Healthcare Institutions Scientific Research Project: 2022041.
The authors declare that the research was conducted in the absence of any commercial or financial relationships that could be construed as a potential conflict of interest.
The author(s) declare that no Generative AI was used in the creation of this manuscript.
All claims expressed in this article are solely those of the authors and do not necessarily represent those of their affiliated organizations, or those of the publisher, the editors and the reviewers. Any product that may be evaluated in this article, or claim that may be made by its manufacturer, is not guaranteed or endorsed by the publisher.
1. Ke C, Liang J, Liu M, Liu S, Wang C. Burden of chronic kidney disease and its risk-attributable burden in 137 low-and middle-income countries, 1990-2019: results from the global burden of disease study 2019. BMC Nephrol. (2022) . 23:17. doi: 10.1186/s12882-021-02597-3
2. Foreman KJ, Marquez N, Dolgert A, Fukutaki K, Fullman N, McGaughey M, et al. Forecasting life expectancy, years of life lost, and all-cause and cause-specific mortality for 250 causes of death: reference and alternative scenarios for 2016-40 for 195 countries and territories. Lancet. (2018) . 392:2052–90. doi: 10.1016/S0140-6736(18)31694-5
3. Collaboration, G.B.D.C.K.D. Global, regional, and national burden of chronic kidney disease, 1990-2017: a systematic analysis for the Global Burden of Disease Study 2017. Lancet. (2020) . 395:709–33. doi: 10.1016/S0140-6736(20)30045-3
4. Kidney Disease: Improving Global Outcomes, C.K.D.W.G. KDIGO 2024 clinical practice guideline for the evaluation and management of chronic kidney disease. Kidney Int. (2024) . 105:S117–314. doi: 10.1016/j.kint.2023.10.018
5. Liu Y. Cellular and molecular mechanisms of renal fibrosis. Nat Rev Nephrol. (2011) . 7:684–96. doi: 10.1038/nrneph.2011.149
6. Webster AC, Nagler EV, Morton RL, Masson P. Chronic kidney disease. Lancet. (2017) . 389:1238–52. doi: 10.1016/S0140-6736(16)32064-5
7. Shi J, Zhao Y, Wang K, Shi X, Wang Y, Huang H, et al. Cleavage of GSDMD by inflammatory caspases determines pyroptotic cell death. Nature. (2015) . 526:660–5. doi: 10.1038/nature15514
8. Shi J, Gao W, Shao F. Pyroptosis: gasdermin-mediated programmed necrotic cell death. Trends Biochem Sci. (2017) . 42:245–54. doi: 10.1016/j.tibs.2016.10.004
9. Zychlinsky A, Prevost MC, Sansonetti PJ. Shigella flexneri induces apoptosis in infected macrophages. Nature. (1992) . 358:167–9. doi: 10.1038/358167a0
10. Cookson BT, Brennan MA. Pro-inflammatory programmed cell death. Trends Microbiol. (2001) . 9:113–4. doi: 10.1016/s0966-842x(00)01936-3
11. Wang Y, Gao W, Shi X, Ding J, Liu W, He H, et al. Chemotherapy drugs induce pyroptosis through caspase-3 cleavage of a gasdermin. Nature. (2017) . 547:99–103. doi: 10.1038/nature22393
12. Galluzzi L, Vitale I, Aaronson SA, Abrams JM, Adam D, Agostinis P, et al. Molecular mechanisms of cell death: recommendations of the Nomenclature Committee on Cell Death 2018. Cell Death Differ. (2018) . 25:486–541. doi: 10.1038/s41418-017-0012-4
13. Vande WL, Lamkanfi M. Pyroptosis. Curr Biol. (2016) . 26:R568–72. doi: 10.1016/j.cub.2016.02.019
14. Zhang KJ, Wu Q, Jiang SM, Ding L, Liu CX, Xu M, et al. Pyroptosis: A new frontier in kidney diseases. Oxid Med Cell Longev. (2021) . 2021:6686617. doi: 10.1155/2021/6686617
15. Kovacs SB, Miao EA. Gasdermins: effectors of pyroptosis. Trends Cell Biol. (2017) . 27:673–84. doi: 10.1016/j.tcb.2017.05.005
16. Kesavardhana S, Malireddi RKS, Kanneganti TD. Caspases in cell death, inflammation, and pyroptosis. Annu Rev Immunol. (2020) . 38:567–95. doi: 10.1146/annurev-immunol-073119-095439
17. Zheng X, Chen W, Gong F, Chen Y, Chen E. The role and mechanism of pyroptosis and potential therapeutic targets in sepsis: A review. Front Immunol. (2021) . 12:711939. doi: 10.3389/fimmu.2021.711939
18. Saeki N, Kuwahara Y, Sasaki H, Satoh H, Shiroishi T. Gasdermin (Gsdm) localizing to mouse Chromosome 11 is predominantly expressed in upper gastrointestinal tract but significantly suppressed in human gastric cancer cells. Mamm Genome. (2000) . 11:718–24. doi: 10.1007/s003350010138
19. Tamura M, Tanaka S, Fujii T, Aoki A, Komiyama H, Ezawa K, et al. Members of a novel gene family, Gsdm, are expressed exclusively in the epithelium of the skin and gastrointestinal tract in a highly tissue-specific manner. Genomics. (2007) . 89:618–29. doi: 10.1016/j.ygeno.2007.01.003
20. Broz P, Pelegrin P, Shao F. The gasdermins, a protein family executing cell death and inflammation. Nat Rev Immunol. (2020) . 20:143–57. doi: 10.1038/s41577-019-0228-2
21. De Schutter E, Roelandt R, Riquet FB, Van Camp G, Wullaert A, Vandenabeele P. Punching holes in cellular membranes: biology and evolution of gasdermins. Trends Cell Biol. (2021) . 31:500–13. doi: 10.1016/j.tcb.2021.03.004
22. Ding J, Wang K, Liu W, She Y, Sun Q, Shi J, et al. Pore-forming activity and structural autoinhibition of the gasdermin family. Nature. (2016) . 535:111–6. doi: 10.1038/nature18590
23. Zhang R, Song Q, Lin X, Du B, Geng D, Gao D. GSDMA at the crossroads between pyroptosis and tumor immune evasion in glioma. Biochem Biophys Res Commun. (2023) . 686:149181. doi: 10.1016/j.bbrc.2023.149181
24. Wang H, Wang H, Chen J, Liu P, Xiao X. Overexpressed FAM111B degrades GSDMA to promote esophageal cancer tumorigenesis and cisplatin resistance. Cell Oncol (Dordr). (2024) . 47:343–59. doi: 10.1007/s13402-023-00871-0
25. Terao C, Kawaguchi T, Dieude P, Varga J, Kuwana M, Hudson M, et al. Transethnic meta-analysis identifies GSDMA and PRDM1 as susceptibility genes to systemic sclerosis. Ann Rheum Dis. (2017) . 76:1150–8. doi: 10.1136/annrheumdis-2016-210645
26. Yu J, Kang MJ, Kim BJ, Kwon JW, Song YH, Choi WA, et al. Polymorphisms in GSDMA and GSDMB are associated with asthma susceptibility, atopy and BHR. Pediatr Pulmonol. (2011) . 46:701–8. doi: 10.1002/ppul.21424
27. Soderman J, Berglind L, Almer S. Gene expression-genotype analysis implicates GSDMA, GSDMB, and LRRC3C as contributors to inflammatory bowel disease susceptibility. BioMed Res Int. (2015) . 2015:834805. doi: 10.1155/2015/834805
28. Hu Y, Wang B, Li S, Yang S. Pyroptosis, and its role in central nervous system disease. J Mol Biol. (2022) . 434:167379. doi: 10.1016/j.jmb.2021.167379
29. He H, Yi L, Zhang B, Yan B, Xiao M, Ren J, et al. USP24-GSDMB complex promotes bladder cancer proliferation via activation of the STAT3 pathway. Int J Biol Sci. (2021) . 17:2417–29. doi: 10.7150/ijbs.54442
30. Cui Y, Zhou Z, Chai Y, Zhang Y. Upregulated GSDMB in clear cell renal cell carcinoma is associated with immune infiltrates and poor prognosis. J Immunol Res. (2021) . 2021:7753553. doi: 10.1155/2021/7753553
31. Saeki N, Komatsuzaki R, Chiwaki F, Yanagihara K, Sasaki H. A GSDMB enhancer-driven HSV thymidine kinase-expressing vector for controlling occult peritoneal dissemination of gastric cancer cells. BMC Cancer. (2015) . 15:439. doi: 10.1186/s12885-015-1436-1
32. Zhang X, Liu R. Pyroptosis-related genes GSDMB, GSDMC, and AIM2 polymorphisms are associated with risk of non-small cell lung cancer in a Chinese Han population. Front Genet. (2023) . 14:1212465. doi: 10.3389/fgene.2023.1212465
33. Rana N, Privitera G, Kondolf HC, Bulek K, Lechuga S, De Salvo C, et al. GSDMB is increased in IBD and regulates epithelial restitution/repair independent of pyroptosis. Cell. (2022) . 185:283–298.e17. doi: 10.1016/j.cell.2021.12.024
34. Li X, Christenson SA, Modena B, Li H, Busse WW, Castro M, et al. Genetic analyses identify GSDMB associated with asthma severity, exacerbations, and antiviral pathways. J Allergy Clin Immunol. (2021) . 147:894–909. doi: 10.1016/j.jaci.2020.07.030
35. Shamsi BH, Chen H, Yang X, Liu M, Liu Y. Association between polymorphisms of the GSDMB gene and allergic rhinitis risk in the Chinese population: a case-control study. J Asthma. (2023) . 60:1751–60. doi: 10.1080/02770903.2023.2185893
36. Ji X, Chen H, Xie L, Chen S, Huang S, Tan Q, et al. The study of GSDMB in pathogenesis of psoriasis vulgaris. PloS One. (2023) . 18:e0279908. doi: 10.1371/journal.pone.0279908
37. Watabe K, Ito A, Asada H, Endo Y, Kobayashi T, Nakamoto K, et al. Structure, expression and chromosome mapping of MLZE, a novel gene which is preferentially expressed in metastatic melanoma cells. Jpn J Cancer Res. (2001) . 92:140–51. doi: 10.1111/j.1349-7006.2001.tb01076.x
38. Wu C, Orozco C, Boyer J, Leglise M, Goodale J, Batalov S, et al. BioGPS: an extensible and customizable portal for querying and organizing gene annotation resources. Genome Biol. (2009) . 10:R130. doi: 10.1186/gb-2009-10-11-r130
39. Zheng Z, Deng W, Lou X, Bai Y, Wang J, Zeng H, et al. Gasdermins: pore-forming activities and beyond. Acta Biochim Biophys Sin (Shanghai). (2020) . 52:467–74. doi: 10.1093/abbs/gmaa016
40. Hou J, Zhao R, Xia W, Chang CW, You Y, Hsu JM, et al. PD-L1-mediated gasdermin C expression switches apoptosis to pyroptosis in cancer cells and facilitates tumour necrosis. Nat Cell Biol. (2020) . 22:1264–75. doi: 10.1038/s41556-020-0575-z
41. Li M, Jiang Q, Liu X, Han L, Chen S, Xue R. The pyroptosis-related signature composed of GSDMC predicts prognosis and contributes to growth and metastasis of hepatocellular carcinoma. Front Biosci (Landmark Ed). (2023) . 28:235. doi: 10.31083/j.fbl2810235
42. Yan C, Niu Y, Li F, Zhao W, Ma L. System analysis based on the pyroptosis-related genes identifies GSDMC as a novel therapy target for pancreatic adenocarcinoma. J Transl Med. (2022) . 20:455. doi: 10.1186/s12967-022-03632-z
43. Xia XH, Yin WJ, Mao JF, Liu P, Qin CD, Hu JJ, et al. The expression profile of Gasdermin C-related genes predicts the prognosis and immunotherapy response of pancreatic adenocarcinoma. Am J Cancer Res. (2023) . 13:1240–58.
44. Cui YQ, Meng F, Zhan WL, Dai ZT, Liao X. High expression of GSDMC is associated with poor survival in kidney clear cell cancer. BioMed Res Int. (2021) . 2021:5282894. doi: 10.1155/2021/5282894
45. Tang D, Zheng Y, Wang G, Sheng C, Liu Z, Wang B, et al. HPV18 E6 inhibits alpha-ketoglutarate-induced pyroptosis of esophageal squamous cell carcinoma cells via the P53/MDH1/ROS/GSDMC pathway. FEBS Open Bio. (2023) . 13:1522–35. doi: 10.1002/2211-5463.13666
46. Miguchi M, Hinoi T, Shimomura M, Adachi T, Saito Y, Niitsu H, et al. Gasdermin C is upregulated by inactivation of transforming growth factor beta receptor type II in the presence of mutated apc, promoting colorectal cancer proliferation. PloS One. (2016) . 11:e0166422. doi: 10.1371/journal.pone.0166422
47. Burdette BE, Esparza AN, Zhu H, Wang S. Gasdermin D in pyroptosis. Acta Pharm Sin B. (2021) . 11:2768–82. doi: 10.1016/j.apsb.2021.02.006
48. Saeki N, Usui T, Aoyagi K, Kim DH, Sato M, Mabuchi T, et al. Distinctive expression and function of four GSDM family genes (GSDMA-D) in normal and Malignant upper gastrointestinal epithelium. Genes Chromosomes Cancer. (2009) . 48:261–71. doi: 10.1002/gcc.20636
49. Kayagaki N, Stowe IB, Lee BL, O’Rourke K, Anderson K, Warming S, et al. Caspase-11 cleaves gasdermin D for non-canonical inflammasome signalling. Nature. (2015) . 526:666–71. doi: 10.1038/nature15541
50. Devant P, Kagan JC. Molecular mechanisms of gasdermin D pore-forming activity. Nat Immunol. (2023) . 24:1064–75. doi: 10.1038/s41590-023-01526-w
51. Xu B, Jiang M, Chu Y, Wang W, Chen D, Li X, et al. Gasdermin D plays a key role as a pyroptosis executor of non-alcoholic steatohepatitis in humans and mice. J Hepatol. (2018) . 68:773–82. doi: 10.1016/j.jhep.2017.11.040
52. Silva CMS, Wanderley CWS, Veras FP, Sonego F, Nascimento DC, Goncalves AV, et al. Gasdermin D inhibition prevents multiple organ dysfunction during sepsis by blocking NET formation. Blood. (2021) . 138:2702–13. doi: 10.1182/blood.2021011525
53. Miao N, Wang Z, Wang Q, Xie H, Yang N, Wang Y, et al. Oxidized mitochondrial DNA induces gasdermin D oligomerization in systemic lupus erythematosus. Nat Commun. (2023) . 14:872. doi: 10.1038/s41467-023-36522-z
54. Gao L, Dong X, Gong W, Huang W, Xue J, Zhu Q, et al. Acinar cell NLRP3 inflammasome and gasdermin D (GSDMD) activation mediates pyroptosis and systemic inflammation in acute pancreatitis. Br J Pharmacol. (2021) . 178:3533–52. doi: 10.1111/bph.15499
55. Gao H, Cao M, Yao Y, Hu W, Sun H, Zhang Y, et al. Dysregulated microbiota-driven gasdermin D activation promotes colitis development by mediating IL-18 release. Front Immunol. (2021) . 12:750841. doi: 10.3389/fimmu.2021.750841
56. Wang J, Yao J, Liu Y, Huang L. Targeting the gasdermin D as a strategy for ischemic stroke therapy. Biochem Pharmacol. (2021) . 188:114585. doi: 10.1016/j.bcp.2021.114585
57. Jiang K, Tu Z, Chen K, Xu Y, Chen F, Xu S, et al. Gasdermin D inhibition confers antineutrophil-mediated cardioprotection in acute myocardial infarction. J Clin Invest. (2022) . 132:e151268. doi: 10.1172/JCI151268
58. Tanaka S, Orita H, Kataoka T, Miyazaki M, Saeki H, Wada R, et al. Gasdermin D represses inflammation-induced colon cancer development by regulating apoptosis. Carcinogenesis. (2023) . 44:341–9. doi: 10.1093/carcin/bgad005
59. Van Laer L, Huizing EH, Verstreken M, van Zuijlen D, Wauters JG, Bossuyt PJ, et al. Nonsyndromic hearing impairment is associated with a mutation in DFNA5. Nat Genet. (1998) . 20:194–7. doi: 10.1038/2503
60. Zhang Z, Zhang Y, Xia S, Kong Q, Li S, Liu X, et al. Gasdermin E suppresses tumour growth by activating anti-tumour immunity. Nature. (2020) . 579:415–20. doi: 10.1038/s41586-020-2071-9
61. Neel DV, Basu H, Gunner G, Bergstresser MD, Giadone RM, Chung H, et al. Gasdermin-E mediates mitochondrial damage in axons and neurodegeneration. Neuron. (2023) . 111:1222–1240.e9. doi: 10.1016/j.neuron.2023.02.019
62. Wei Y, Lan B, Zheng T, Yang L, Zhang X, Cheng L, et al. GSDME-mediated pyroptosis promotes the progression and associated inflammation of atherosclerosis. Nat Commun. (2023) . 14:929. doi: 10.1038/s41467-023-36614-w
63. Li Y, Yuan Y, Huang ZX, Chen H, Lan R, Wang Z, et al. GSDME-mediated pyroptosis promotes inflammation and fibrosis in obstructive nephropathy. Cell Death Differ. (2021) . 28:2333–50. doi: 10.1038/s41418-021-00755-6
64. Li W, Sun J, Zhou X, Lu Y, Cui W, Miao L. Mini-review: GSDME-mediated pyroptosis in diabetic nephropathy. Front Pharmacol. (2021) . 12:780790. doi: 10.3389/fphar.2021.780790
65. Delmaghani S, Del CFJ, Michel V, Leibovici M, Aghaie A, Ron U, et al. Mutations in the gene encoding pejvakin, a newly identified protein of the afferent auditory pathway, cause DFNB59 auditory neuropathy. Nat Genet. (2006) . 38:770–8. doi: 10.1038/ng1829
66. Mujtaba G, Bukhari I, Fatima A, Naz S. A p.C343S missense mutation in PJVK causes progressive hearing loss. Gene. (2012) . 504:98–101. doi: 10.1016/j.gene.2012.05.013
67. Rathinam VA, Fitzgerald KA. Inflammasome complexes: emerging mechanisms and effector functions. Cell. (2016) . 165:792–800. doi: 10.1016/j.cell.2016.03.046
68. Lamkanfi M, Dixit VM. Mechanisms and functions of inflammasomes. Cell. (2014) . 157:1013–22. doi: 10.1016/j.cell.2014.04.007
69. Chavarria-Smith J, Vance RE. The NLRP1 inflammasomes. Immunol Rev. (2015) . 265:22–34. doi: 10.1111/imr.12283
70. Huang Y, Xu W, Zhou R. NLRP3 inflammasome activation and cell death. Cell Mol Immunol. (2021) . 18:2114–27. doi: 10.1038/s41423-021-00740-6
71. Fu J, Wu H. Structural mechanisms of NLRP3 inflammasome assembly and activation. Annu Rev Immunol. (2023) . 41:301–16. doi: 10.1146/annurev-immunol-081022-021207
72. Xu J, Nunez G. The NLRP3 inflammasome: activation and regulation. Trends Biochem Sci. (2023) . 48:331–44. doi: 10.1016/j.tibs.2022.10.002
73. Li R, Zhu S. NLRP6 inflammasome. Mol Aspects Med. (2020) . 76:100859. doi: 10.1016/j.mam.2020.100859
74. Hara H, Seregin SS, Yang D, Fukase K, Chamaillard M, Alnemri ES, et al. The NLRP6 inflammasome recognizes lipoteichoic acid and regulates gram-positive pathogen infection. Cell. (2018) . 175:1651–1664.e14. doi: 10.1016/j.cell.2018.09.047
75. Levy M, Thaiss CA, Zeevi D, Dohnalova L, Zilberman-Schapira G, Mahdi JA, et al. Microbiota-modulated metabolites shape the intestinal microenvironment by regulating NLRP6 inflammasome signaling. Cell. (2015) . 163:1428–43. doi: 10.1016/j.cell.2015.10.048
76. Mullins B, Chen J. NLRP9 in innate immunity and inflammation. Immunology. (2021) . 162:262–7. doi: 10.1111/imm.13290
77. Lugrin J, Martinon F. The AIM2 inflammasome: Sensor of pathogens and cellular perturbations. Immunol Rev. (2018) . 281:99–114. doi: 10.1111/imr.12618
78. Duncan JA, Canna SW. The NLRC4 inflammasome. Immunol Rev. (2018) . 281:115–23. doi: 10.1111/imr.12607
79. Schnappauf O, Chae JJ, Kastner DL, Aksentijevich I. The pyrin inflammasome in health and disease. Front Immunol. (2019) . 10:1745. doi: 10.3389/fimmu.2019.01745
80. Yang Y, Wang H, Kouadir M, Song H, Shi F. Recent advances in the mechanisms of NLRP3 inflammasome activation and its inhibitors. Cell Death Dis. (2019) . 10:128. doi: 10.1038/s41419-019-1413-8
81. Mangan MSJ, Olhava EJ, Roush WR, Seidel HM, Glick GD, Latz E. Targeting the NLRP3 inflammasome in inflammatory diseases. Nat Rev Drug Discovery. (2018) . 17:588–606. doi: 10.1038/nrd.2018.97
82. Miao EA, Rajan JV, Aderem A. Caspase-1-induced pyroptotic cell death. Immunol Rev. (2011) . 243:206–14. doi: 10.1111/j.1600-065X.2011.01044.x
83. Liu X, Zhang Z, Ruan J, Pan Y, Magupalli VG, Wu H, et al. Inflammasome-activated gasdermin D causes pyroptosis by forming membrane pores. Nature. (2016) . 535:153–8. doi: 10.1038/nature18629
84. Shi J, Zhao Y, Wang Y, Gao W, Ding J, Li P, et al. Inflammatory caspases are innate immune receptors for intracellular LPS. Nature. (2014) . 514:187–92. doi: 10.1038/nature13683
85. Yang D, He Y, Munoz-Planillo R, Liu Q, Nunez G. Caspase-11 requires the pannexin-1 channel and the purinergic P2X7 pore to mediate pyroptosis and endotoxic shock. Immunity. (2015) . 43:923–32. doi: 10.1016/j.immuni.2015.10.009
86. Deng W, Bai Y, Deng F, Pan Y, Mei S, Zheng Z, et al. Streptococcal pyrogenic exotoxin B cleaves GSDMA and triggers pyroptosis. Nature. (2022) . 602:496–502. doi: 10.1038/s41586-021-04384-4
87. LaRock DL, Johnson AF, Wilde S, Sands JS, Monteiro MP, LaRock CN. Group A Streptococcus induces GSDMA-dependent pyroptosis in keratinocytes. Nature. (2022) . 605:527–31. doi: 10.1038/s41586-022-04717-x
88. Li S, Song J, Liu J, Zhou S, Zhao G, Li T, et al. African swine fever virus infection regulates pyroptosis by cleaving gasdermin A (GSDMA) via active caspase-3 and caspase-4. J Biol Chem. (2024) . 300:107307. doi: 10.1016/j.jbc.2024.107307
89. Zhou Z, He H, Wang K, Shi X, Wang Y, Su Y, et al. Granzyme A from cytotoxic lymphocytes cleaves GSDMB to trigger pyroptosis in target cells. Science. (2020) 368:eaaz7548. doi: 10.1126/science.aaz7548
90. Hansen JM, de Jong MF, Wu Q, Zhang LS, Heisler DB, Alto LT, et al. Pathogenic ubiquitination of GSDMB inhibits NK cell bactericidal functions. Cell. (2021) . 184:3178–3191.e18. doi: 10.1016/j.cell.2021.04.036
91. Feng Y, Li M, Yangzhong X, Zhang X, Zu A, Hou Y, et al. Pyroptosis in inflammation-related respiratory disease. J Physiol Biochem. (2022) . 78:721–37. doi: 10.1007/s13105-022-00909-1
92. Chen Q, Shi P, Wang Y, Zou D, Wu X, Wang D, et al. GSDMB promotes non-canonical pyroptosis by enhancing caspase-4 activity. J Mol Cell Biol. (2019) . 11:496–508. doi: 10.1093/jmcb/mjy056
93. Li X, Zhang T, Kang L, Xin R, Sun M, Chen Q, et al. Apoptotic caspase-7 activation inhibits non-canonical pyroptosis by GSDMB cleavage. Cell Death Differ. (2023) . 30:2120–34. doi: 10.1038/s41418-023-01211-3
94. Zhang JY, Zhou B, Sun RY, Ai YL, Cheng K, Li FN, et al. The metabolite alpha-KG induces GSDMC-dependent pyroptosis through death receptor 6-activated caspase-8. Cell Res. (2021) . 31:980–97. doi: 10.1038/s41422-021-00506-9
95. Tsuchiya K. Switching from apoptosis to pyroptosis: gasdermin-elicited inflammation and antitumor immunity. Int J Mol Sci. (2021) . 22:426. doi: 10.3390/ijms22010426
96. Burgener SS, Leborgne NGF, Snipas SJ, Salvesen GS, Bird PI, Benarafa C. Cathepsin G inhibition by serpinb1 and serpinb6 prevents programmed necrosis in neutrophils and monocytes and reduces GSDMD-driven inflammation. Cell Rep. (2019) . 27:3646–3656.e5. doi: 10.1016/j.celrep.2019.05.065
97. Kambara H, Liu F, Zhang X, Liu P, Bajrami B, Teng Y, et al. Gasdermin D exerts anti-inflammatory effects by promoting neutrophil death. Cell Rep. (2018) . 22:2924–36. doi: 10.1016/j.celrep.2018.02.067
98. Sarhan J, Liu BC, Muendlein HI, Li P, Nilson R, Tang AY, et al. Caspase-8 induces cleavage of gasdermin D to elicit pyroptosis during Yersinia infection. Proc Natl Acad Sci U S A. (2018) . 115:E10888–97. doi: 10.1073/pnas.1809548115
99. Rogers C, Fernandes-Alnemri T, Mayes L, Alnemri D, Cingolani G, Alnemri ES. Cleavage of DFNA5 by caspase-3 during apoptosis mediates progression to secondary necrotic/pyroptotic cell death. Nat Commun. (2017) . 8:14128. doi: 10.1038/ncomms14128
100. Jiang M, Qi L, Li L, Li Y. The caspase-3/GSDME signal pathway as a switch between apoptosis and pyroptosis in cancer. Cell Death Discovery. (2020) . 6:112. doi: 10.1038/s41420-020-00349-0
101. Meng XM. Inflammatory mediators and renal fibrosis. Adv Exp Med Biol. (2019) . 1165:381–406. doi: 10.1007/978-981-13-8871-2_18
102. Huang R, Fu P, Ma L. Kidney fibrosis: from mechanisms to therapeutic medicines. Signal Transduct Target Ther. (2023) . 8:129. doi: 10.1038/s41392-023-01379-7
103. Ruiz-Ortega M, Rodrigues-Diez RR, Lavoz C, Rayego-Mateos S. Special issue “Diabetic nephropathy: diagnosis, prevention and treatment. J Clin Med. (2020) . 9:813. doi: 10.3390/jcm9030813
104. Kriz W, Lowen J, Grone HJ. The complex pathology of diabetic nephropathy in humans. Nephrol Dial Transplant. (2023) . 38:2109–19. doi: 10.1093/ndt/gfad052
105. Shao Y, Deng S, Tang W, Huang L, Xie Y, Yuan S, et al. Molecular mechanism of GSDMD mediated glomerular endothelial cells pyroptosis: An implying in the progression of diabetic nephropathy. Int Immunopharmacol. (2023) . 122:110632. doi: 10.1016/j.intimp.2023.110632
106. An X, Zhang Y, Cao Y, Chen J, Qin H, Yang L. Punicalagin protects diabetic nephropathy by inhibiting pyroptosis based on TXNIP/NLRP3 pathway. Nutrients. (2020) . 12:1516. doi: 10.3390/nu12051516
107. Cheng Q, Pan J, Zhou ZL, Yin F, Xie HY, Chen PP, et al. Caspase-11/4 and gasdermin D-mediated pyroptosis contributes to podocyte injury in mouse diabetic nephropathy. Acta Pharmacol Sin. (2021) . 42:954–63. doi: 10.1038/s41401-020-00525-z
108. Cui X, Li Y, Yuan S, Huang Y, Chen X, Han Y, et al. Alpha-kinase1 promotes tubular injury and interstitial inflammation in diabetic nephropathy by canonical pyroptosis pathway. Biol Res. (2023) . 56:5. doi: 10.1186/s40659-023-00416-7
109. Li S, Feng L, Li G, Liu R, Ma C, Wang L, et al. GSDME-dependent pyroptosis signaling pathway in diabetic nephropathy. Cell Death Discovery. (2023) . 9:156. doi: 10.1038/s41420-023-01452-8
110. Wang Y, Zhu X, Yuan S, Wen S, Liu X, Wang C, et al. TLR4/NF-kappaB signaling induces GSDMD-related pyroptosis in tubular cells in diabetic kidney disease. Front Endocrinol (Lausanne). (2019) . 10:603. doi: 10.3389/fendo.2019.00603
111. Han Y, Xu X, Tang C, Gao P, Chen X, Xiong X, et al. Reactive oxygen species promote tubular injury in diabetic nephropathy: The role of the mitochondrial ros-txnip-nlrp3 biological axis. Redox Biol. (2018) . 16:32–46. doi: 10.1016/j.redox.2018.02.013
112. Hoi A, Igel T, Mok CC, Arnaud L. Systemic lupus erythematosus. Lancet. (2024) . 403:2326–38. doi: 10.1016/S0140-6736(24)00398-2
113. Anders HJ, Saxena R, Zhao MH, Parodis I, Salmon JE, Mohan C. Lupus nephritis. Nat Rev Dis Primers. (2020) . 6:7. doi: 10.1038/s41572-019-0141-9
114. Lech M, Anders HJ. The pathogenesis of lupus nephritis. J Am Soc Nephrol. (2013) . 24:1357–66. doi: 10.1681/ASN.2013010026
115. Satoh M, Reeves WH. Induction of lupus-associated autoantibodies in BALB/c mice by intraperitoneal injection of pristane. J Exp Med. (1994) . 180:2341–6. doi: 10.1084/jem.180.6.2341
116. Satoh M, Kumar A, Kanwar YS, Reeves WH. Anti-nuclear antibody production and immune-complex glomerulonephritis in BALB/c mice treated with pristane. Proc Natl Acad Sci U S A. (1995) . 92:10934–8. doi: 10.1073/pnas.92.24.10934
117. Zhang Z, Song W, Yan R. Gbp3 is associated with the progression of lupus nephritis by regulating cell proliferation, inflammation and pyroptosis. Autoimmunity. (2023) . 56:2250095. doi: 10.1080/08916934.2023.2250095
118. Cao H, Liang J, Liu J, He Y, Ke Y, Sun Y, et al. Novel effects of combination therapy through inhibition of caspase-1/gasdermin D induced-pyroptosis in lupus nephritis. Front Immunol. (2021) . 12:720877. doi: 10.3389/fimmu.2021.720877
119. Luo G, He Y, Yang F, Zhai Z, Han J, Xu W, et al. Blocking GSDME-mediated pyroptosis in renal tubular epithelial cells alleviates disease activity in lupus mice. Cell Death Discovery. (2022) . 8:113. doi: 10.1038/s41420-022-00848-2
120. Norregaard R, Mutsaers HAM, Frokiaer J, Kwon TH. Obstructive nephropathy and molecular pathophysiology of renal interstitial fibrosis. Physiol Rev. (2023) . 103:2827–72. doi: 10.1152/physrev.00027.2022
121. Wang K, Liao Q, Chen X. Research progress on the mechanism of renal interstitial fibrosis in obstructive nephropathy. Heliyon. (2023) . 9:e18723. doi: 10.1016/j.heliyon.2023.e18723
122. Wang Y, Li Y, Chen Z, Yuan Y, Su Q, Ye K, et al. GSDMD-dependent neutrophil extracellular traps promote macrophage-to-myofibroblast transition and renal fibrosis in obstructive nephropathy. Cell Death Dis. (2022) . 13:693. doi: 10.1038/s41419-022-05138-4
123. Wu M, Xia W, Jin Q, Zhou A, Wang Q, Li S, et al. Gasdermin E deletion attenuates ureteral obstruction- and 5/6 nephrectomy-induced renal fibrosis and kidney dysfunction. Front Cell Dev Biol. (2021) . 9:754134. doi: 10.3389/fcell.2021.754134
124. Zhang Y, Zhang R, Han X. Disulfiram inhibits inflammation and fibrosis in a rat unilateral ureteral obstruction model by inhibiting gasdermin D cleavage and pyroptosis. Inflammation Res. (2021) . 70:543–52. doi: 10.1007/s00011-021-01457-y
125. Jia Q, Zhang X, Hao G, Zhao Y, Lowe S, Han L, et al. Tongluo yishen decoction ameliorates renal fibrosis via NLRP3-mediated pyroptosis in vivo and in vitro. Front Pharmacol. (2022) . 13:936853. doi: 10.3389/fphar.2022.936853
126. Feng M, Luo F, Wu H, Chen Y, Zuo J, Weng X, et al. Network pharmacology analysis and machine-learning models confirmed the ability of yiShen huoXue decoction to alleviate renal fibrosis by inhibiting pyroptosis. Drug Des Devel Ther. (2023) . 17:3169–92. doi: 10.2147/DDDT.S420135
127. Perazella MA, Herlitz LC. The crystalline nephropathies. Kidney Int Rep. (2021) . 6:2942–57. doi: 10.1016/j.ekir.2021.09.003
128. Piani F, Sasai F, Bjornstad P, Borghi C, Yoshimura A, Sanchez-Lozada LG, et al. Hyperuricemia and chronic kidney disease: to treat or not to treat. J Bras Nefrol. (2021) . 43:572–9. doi: 10.1590/2175-8239-JBN-2020-U002
129. Ermer T, Eckardt KU, Aronson PS, Knauf F. Oxalate, inflammasome, and progression of kidney disease. Curr Opin Nephrol Hypertens. (2016) . 25:363–71. doi: 10.1097/MNH.0000000000000229
130. Llanos M, Kwon A, Herlitz L, Shafi T, Cohen S, Gebreselassie SK, et al. The clinical and pathological characteristics of patients with oxalate nephropathy. Kidney360. (2024) . 5:65–72. doi: 10.34067/KID.0000000000000340
131. Gan XG, Wang ZH, Xu HT. Mechanism of miRNA-141-3p in Calcium Oxalate-Induced Renal Tubular Epithelial Cell Injury via NLRP3-Mediated Pyroptosis. Kidney Blood Press Res. (2022) . 47:300–8. doi: 10.1159/000521795
132. Chen Y, Yang S, Kong H, Wang Q, Chen S, Wang X, et al. Oxalate−induced renal pyroptotic injury and crystal formation mediated by NLRP3−GSDMD signaling in vitro and in vivo. Mol Med Rep. (2023) . 28:209. doi: 10.3892/mmr.2023.13096
133. Liu J, Yang K, Jin Y, Liu Y, Chen Y, Zhang X, et al. H3 relaxin protects against calcium oxalate crystal-induced renal inflammatory pyroptosis. Cell Prolif. (2020) . 53:e12902. doi: 10.1111/cpr.12902
134. Yao K, Zhang ZH, Liu MD, Niu FW, Li X, Ding DM, et al. Melatonin alleviates intrarenal CaOx crystals deposition through inhibiting LPS-induced non-canonical inflammasome-mediated renal tubular epithelial cells pyroptosis. Int Immunopharmacol. (2023) 124:110796. doi: 10.1016/j.intimp.2023.110796
135. Pan J, Zhang C, Shi M, Guo F, Liu J, Li L, et al. Ethanol extract of Liriodendron chinense (Hemsl.) Sarg barks attenuates hyperuricemic nephropathy by inhibiting renal fibrosis and inflammation in mice. J Ethnopharmacol. (2021) . 264:113278. doi: 10.1016/j.jep.2020.113278
136. Yang L, Wang B, Ma L, Fu P. Traditional Chinese herbs and natural products in hyperuricemia-induced chronic kidney disease. Front Pharmacol. (2022) . 13:971032. doi: 10.3389/fphar.2022.971032
137. Johnson RJ. Intestinal hyperuricemia as a driving mechanism for CKD. Am J Kidney Dis. (2023) . 81:127–30. doi: 10.1053/j.ajkd.2022.08.001
138. Ma L, Shen R, Jiao J, Lin X, Zhai B, Xu A, et al. Gasdermin D promotes hyperuricemia-induced renal tubular injury through RIG-I/caspase-1 pathway. iScience. (2023) . 26:108463. doi: 10.1016/j.isci.2023.108463
139. Hu Y, Shi Y, Chen H, Tao M, Zhou X, Li J, et al. Blockade of autophagy prevents the progression of hyperuricemic nephropathy through inhibiting NLRP3 inflammasome-mediated pyroptosis. Front Immunol. (2022) . 13:858494. doi: 10.3389/fimmu.2022.858494
140. Ding Z, Zhao J, Wang X, Li W, Chen C, Yong C, et al. Total extract of Abelmoschus manihot L. alleviates uric acid-induced renal tubular epithelial injury via inhibition of caspase-8/caspase-3/NLRP3/GSDME signaling. Front Pharmacol. (2022) . 13:907980. doi: 10.3389/fphar.2022.907980
141. Kujal P, Vernerova Z. 5/6 nephrectomy as an experimental model of chronic renal failure and adaptation to reduced nephron number. Cesk Fysiol. (2008) . 57:104–9.
142. Adam RJ, Williams AC, Kriegel AJ. Comparison of the surgical resection and infarct 5/6 nephrectomy rat models of chronic kidney disease. Am J Physiol Renal Physiol. (2022) 322:F639–54. doi: 10.1152/ajprenal.00398.2021
Keywords: gasdermins, pyroptosis, chronic kidney disease, inflammation, renal fibrosis
Citation: Gao H, Xie T, Li Y, Xu Z, Song Z, Yu H, Zhou H, Li W, Yun C, Guan B, Luan S and Yin L (2025) Role of gasdermins in chronic kidney disease. Front. Immunol. 16:1557707. doi: 10.3389/fimmu.2025.1557707
Received: 09 January 2025; Accepted: 14 March 2025;
Published: 01 April 2025.
Edited by:
Lili Zhou, Southern Medical University, ChinaReviewed by:
Edmund Chung, Children’s Hospital at Westmead, AustraliaCopyright © 2025 Gao, Xie, Li, Xu, Song, Yu, Zhou, Li, Yun, Guan, Luan and Yin. This is an open-access article distributed under the terms of the Creative Commons Attribution License (CC BY). The use, distribution or reproduction in other forums is permitted, provided the original author(s) and the copyright owner(s) are credited and that the original publication in this journal is cited, in accordance with accepted academic practice. No use, distribution or reproduction is permitted which does not comply with these terms.
*Correspondence: Chen Yun, bmljazY2NjQ1NkAxMjYuY29t; Shaodong Luan, c3praWRuZXkzQDE2My5jb20=; Baozhang Guan, Mzk2MzQ5OTMwQHFxLmNvbQ==; Lianghong Yin, MTM3MjUyNTE0NThAMTI2LmNvbQ==
†These authors have contributed equally to this work and share first authorship
Disclaimer: All claims expressed in this article are solely those of the authors and do not necessarily represent those of their affiliated organizations, or those of the publisher, the editors and the reviewers. Any product that may be evaluated in this article or claim that may be made by its manufacturer is not guaranteed or endorsed by the publisher.
Research integrity at Frontiers
Learn more about the work of our research integrity team to safeguard the quality of each article we publish.