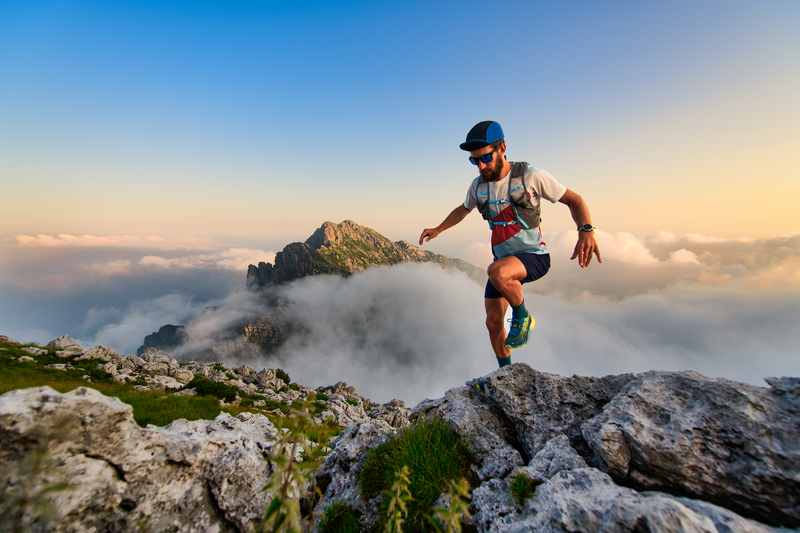
95% of researchers rate our articles as excellent or good
Learn more about the work of our research integrity team to safeguard the quality of each article we publish.
Find out more
MINI REVIEW article
Front. Immunol. , 31 March 2025
Sec. Cytokines and Soluble Mediators in Immunity
Volume 16 - 2025 | https://doi.org/10.3389/fimmu.2025.1555414
This article is part of the Research Topic Polysaccharides for Health and Nutrition - Extraction, Isolation, Bioactivity and Innovative Applications View all articles
Polysaccharides, as complex carbohydrates, play a pivotal role in immune modulation and interactions with the gut microbiota. The diverse array of dietary polysaccharides influences gut microbial ecology, impacting immune responses, metabolism, and overall well-being. Despite their recognized benefits, there is limited understanding of the precise mechanisms by which polysaccharides modulate the immune system through the gut microbiota. A comprehensive search of Web of Science, PubMed, Google Scholar, and Embase up to May 2024 was conducted to identify relevant studies. This study employs a systematic approach to explore the interplay between polysaccharides and the gut microbiota, focusing on cytokine-mediated and short-chain fatty acid (SCFA)-mediated pathways. The findings underscore the significant role of polysaccharides in shaping the composition and function of the gut microbiota, thereby influencing immune regulation and metabolic processes. However, further research is necessary to elucidate the detailed molecular mechanisms and translate these findings into clinical applications.
The mammalian gut houses a diverse range of microorganisms, including bacteria, archaea, viruses, fungi, and protozoa, which interact beneficially with the host (1). These gut microbiota influence various processes through the gut-brain axis, affecting metabolism, immune responses, and behavior. With approximately 10¹³ bacteria in the large intestine, they are crucial for immune system development and metabolic capacity (2). The microbiota interact closely with host immunity, distinguishing pathogens from healthy tissues (3). Research shows that short-chain fatty acids (SCFAs) from fiber-rich diets are potential therapies for inflammatory bowel disease and allergic asthma (4). Additionally, disruptions in the microbiota can alter the gut environment, affecting both microbial and host metabolites and compromising immunity (5). Polysaccharides are key carbohydrates involved in energy storage, structural integrity, and immune modulation. Typically well-tolerated, they are found in various foods (6). Plant-derived polysaccharides offer diverse health benefits, including immune regulation, anti-inflammatory effects, antiviral capabilities, antitumor effects, and hypoglycemic potential, all linked to their structural attributes (7). These polysaccharides influence the gut microbiota, with dietary polysaccharides being broken down by specialized enzymes from the gut microbiome (8). A diet rich in polysaccharides supports beneficial gut microbes and enhances overall well-being. For example, inulin can reduce endotoxins and stimulate gut bacteria growth (9). Recent studies suggest that polysaccharides may modulate immune responses and help manage conditions like SARS-CoV-2 infection (10). This study focuses on the effects of polysaccharides on gut microbes and immune regulation, specifically through cytokine and SCFA pathways.
Polysaccharides exhibit a wide range of structural and functional properties that significantly influence their interactions with the gut microbiota and the immune system. This section examines polysaccharides derived from plants, algae, and fungi, highlighting their structural characteristics and biological activities.
Plants are a primary source of polysaccharides, which play essential roles in both structure and function. Polysaccharides like pectin, found in plant cell walls, and homogalacturonans, composed of α-(1–4)-linked D-galacturonic acid, are particularly important. Cellulose, a major component of green plants and algae, consists of β(1→4)-linked D-glucose units. Insulins, mainly composed of fructose units linked by β(2→1) glycosidic bonds, are commonly found in various plants. Plant polysaccharides have been shown to impact health conditions such as diabetes and liver fibrosis. Liu et al. (11) found that polysaccharides from Dendrobium officinale (DOP) enhance glycogen synthesis and stability, inhibit glycogen degradation, and suppress gluconeogenesis. Wang et al. (12) demonstrated that DOP reduces inflammation by inhibiting LPS/TLR4/NF-κB signaling, decreasing TGF-β and TNF-α levels, lowering collagen I and α-SMA expression, and increasing IL-10 expression.
Algae, unique among photosynthetic organisms, possess polysaccharides that resist human digestive enzymes but can be fermented by gut bacteria. These polysaccharides, including sulfated types such as carrageenans, agars, porphyrin, and xylan found in red algae, exhibit notable biological properties. They are particularly significant for their anticoagulant effects, which help prevent thrombus formation and reduce the risk of clotting. Tang et al. (13) highlighted the anticoagulant and antioxidant properties of polysaccharides from Grateloupia livida. Jia et al. (14) found that algal polysaccharides positively affect blood glucose, triglycerides, cholesterol, alanine transaminase, and blood urea nitrogen levels in type 2 diabetic rats, indicating their potential as natural pharmaceuticals.
Edible fungi contain a variety of polysaccharides, including heteropolysaccharides and glucans, which exhibit biological activities such as immunomodulation, antioxidation, and potential health benefits. These polysaccharides enhance the nutritional value of edible fungi and hold promise for functional foods and natural medicines. Observational studies have shown their roles in addressing obesity, immunity, and cognitive function. Chang et al. (15) noted that high molecular weight polysaccharides from Ganoderma lucidum may improve gut health and help manage metabolic disorders linked to obesity. Luo et al. (16) found that polysaccharides from Ganoderma lucidum may alleviate colorectal cancer by influencing gut bacteria and gene expression. Poria cocos polysaccharides, mainly (1→3)-β-glucans with (1→6)-β-glucose side chains, are key bioactive components with properties such as cancer-fighting, immunomodulation, anti-inflammatory, anti-aging, antioxidant, anti-diabetes, and anti-hemorrhagic fever effects (6).
Polysaccharides play a crucial role in shaping the gut microbiota, providing probiotics with a competitive advantage over pathogenic bacteria. Additionally, the gut microbiota are significantly influenced by polysaccharides with various chemical structures. Together, polysaccharides function as prebiotics, helping to regulate the microecological environment of the gut. Figure 1 illustrates the interaction between polysaccharides and gut microbiota.
Evidence suggests that the composition of gut microbiota is closely linked to polysaccharide intake. Thomson et al. (17) demonstrated that co-cultures of Bacteroides dorei and Clostridium symbiosum grew rapidly on xylan, leading to a reduction in inflammation. Population studies have shown that barley β-glucan increases the abundance of Bacteroides and Bifidobacteria in older adults, highlighting its prebiotic potential (18). In vitro studies by Yang et al. (19) found that pectin, inulin, and β-glucan promoted the proliferation of Bifidobacteria and Erysipelotrichaceae. Shang et al. (20) proposed that fucoidans may help improve intestinal dysbiosis by increasing Akkermansia, potentially alleviating metabolic syndrome.
The symbiotic gut microbiota harnesses energy and produces a wide array of molecules and metabolites, including key SCFAs such as acetate, propionate, and butyrate (21–23). SCFAs exert their effects through various mechanisms, including acidifying the intestinal environment and maintaining intestinal barrier function. For instance, SCFAs play a significant role in mammalian energy metabolism by facilitating the fermentation of dietary fiber by anaerobic bacteria in the gut (24). Another study found higher concentrations of SCFAs in the proximal colon, where enterocytes either utilize them locally or transport them across the intestinal epithelium (25). These findings highlight the importance of SCFAs in maintaining gut and immune homeostasis. Acetate, a common anion in biological systems, is utilized by organisms as acetyl coenzyme A, a critical substrate. Intraperitoneal administration of sodium acetate at varying doses has been observed to trigger headaches in sensitized rats. It is postulated that acetate, a byproduct of ethanol oxidation, contributes to the manifestation of hangover symptoms. Elevated serum acetate levels lead to adenosine accumulation in various tissues, including the brain. Interestingly, post-ethanol treatment with the adenosine receptor antagonist caffeine was found to alleviate nociceptive responses in rat models. Furthermore, acetate has well-documented immunomodulatory properties, impacting the innate immune response against pathogens like Haemophilus influenzae, a major respiratory pathogen. Meanwhile, butyrate has emerged as a key regulator in immune homeostasis, acting locally in the gastrointestinal tract and systemically through circulation. By inhibiting class I histone deacetylases and stimulating G-protein coupled receptors such as GPR109A, GPR43, and GPR41, it exerts immunomodulatory effects (26). Among the various SCFAs, butyrate uniquely serves as a ligand for HCA2 in intestinal regulatory T cells in experimental settings (27). Its critical role in modulating colonic inflammation has made it a potential agent for both preventing and treating inflammation-related conditions like ulcerative colitis and colorectal cancer.
It is the intricate enzymatic machinery of the intestinal microbiota that generates SCFAs from polysaccharides (21). Noteworthy microbial inhabitants are involved in SCFA synthesis in response to polysaccharide stimuli. For instance, the Wood-Ljungdahl pathway utilized by Blautia hydrogenotrophica facilitates acetate production within this metabolic framework (28). Propionate biosynthesis occurs via the succinate pathway within the Bacteroidetes phylum, which includes taxa such as Lachnospiraceae and Negativicutes (29). Metagenomic analyses highlight that gut microbiota species are crucial to butyrate production, with specific subsets of Lachnospiraceae capable of converting lactate and acetate into butyrate (30). Furthermore, Akkermansia plays a significant role in converting mucin into SCFAs, adding another layer of complexity to the gut ecosystem’s metabolic interactions (20). Research suggests that fucoidan supplements can promote the proliferation of specific microbes, enhancing SCFA production. In vitro and animal studies further support this, showing significant increases in SCFA synthesis with high barley β-glucan intake (18). Interventions involving plant-derived polysaccharides, in combination with cross-feeding mechanisms between lactate-producing bacteria, likely contribute to maintaining a well-functioning microbial community capable of producing butyrate (31). Additionally, Astragalus membranaceus polysaccharides have been shown to modulate SCFA composition and increase the Bacteroidetes-to-Firmicutes ratio (a marker of gut dysbiosis) in diabetic mice (32).
Polysaccharides, as complex carbohydrates, regulate immune responses by interacting with specific receptors on host cells (33). The molecular weight, degree of branching, and functional groups of polysaccharides influence their immunomodulatory mechanisms. Below are the mechanisms by which different structural polysaccharides regulate immune responses through specific receptors or pathways:
(1) β-Glucans: β-Glucans, commonly found in fungi, yeast, and algae, primarily modulate immune responses via pattern recognition receptors (PRRs). Specifically, Dectin-1 is the main receptor for β-glucans. Binding of β-glucans to Dectin-1 activates the Syk signaling pathway, which in turn triggers NF-κB activation, promoting the production of pro-inflammatory cytokines such as TNF-α and IL-6 (34). Additionally, β-glucans can activate macrophages through Toll-like receptor (TLR) 2, enhancing their phagocytic capabilities and strengthening the host’s defense against pathogens. (2) Arabinogalactan: Arabinogalactan, abundant in plants, particularly in traditional medicinal herbs like Astragalus membranaceus, primarily activates immune responses by modulating TLR4 (35). Upon binding to TLR4, arabinogalactan activates the MyD88-dependent signaling pathway, inducing the secretion of IL-12 and promoting T cell polarization and activation. (3) Pectins: Pectins, complex polysaccharides derived from plant cell walls, possess highly branched structures. Their immunomodulatory effects are primarily mediated through interactions with Toll-like receptors (TLR2 and TLR4) on macrophages. Studies have shown that pectins can inhibit the NF-κB pathway, reducing the production of pro-inflammatory cytokines and exerting anti-inflammatory effects (36). Furthermore, pectins can interact with C-type lectin receptors (CLRs), enhancing antigen presentation and adaptive immune responses. (4) Sulfated Polysaccharides: Sulfated polysaccharides, such as carrageenan from seaweed, exert immunomodulatory effects closely linked to their sulfate groups. They interact with C-type lectin receptors (e.g., DC-SIGN) to regulate dendritic cell and T cell functions (37). Additionally, sulfated polysaccharides can inhibit complement activation, reducing inflammatory responses.
SCFAs, including acetate, propionate, and butyrate, are metabolic products produced by gut microbiota through the fermentation of dietary fibers. These SCFAs play critical roles in immune regulation, with each fatty acid modulating the immune system through distinct mechanisms. (1) Acetate: Acetate is one of the most abundant SCFAs and plays multiple roles in gut immunity. It strengthens the intestinal mucosal barrier, preventing pathogen invasion. Acetate binds to G-protein coupled receptors, particularly GPR43, which activates anti-inflammatory pathways, including the promotion of IL-10 production. IL-10 is a key regulatory cytokine that controls immune responses and prevents excessive inflammation (38). Additionally, acetate suppresses the production of pro-inflammatory cytokines, exerting systemic anti-inflammatory effects. Through these mechanisms, acetate plays a vital role in maintaining intestinal homeostasis and overall immune balance. (2) Propionate: Propionate primarily influences immune function by modulating metabolic pathways. Like acetate, propionate interacts with GPR43 and GPR41 receptors to regulate gut immune responses (39). A critical function of propionate is the promotion of regulatory T cell (Treg) generation. Tregs are specialized immune cells that suppress inflammatory responses and maintain immune tolerance (40). By enhancing Treg activity, propionate helps reduce inflammation, particularly in chronic inflammatory conditions. Additionally, propionate influences fat and glucose metabolism, indirectly affecting immune function through metabolic regulation. (3) Butyrate: Butyrate serves as the primary energy source for colonocytes and regulates immune function through several mechanisms (41). One key mechanism is the inhibition of histone deacetylases (HDACs), which modulates gene expression in immune cells. This action not only promotes the generation of regulatory T cells but also suppresses the release of pro-inflammatory cytokines, reducing inflammation. Butyrate has demonstrated significant effects in preventing and treating inflammatory bowel diseases (IBDs) such as ulcerative colitis and Crohn’s disease (42). By maintaining the integrity of the intestinal epithelial barrier and reducing inflammation, butyrate aids in alleviating disease symptoms and promoting gut healing.
A summary of the immune regulation mechanisms mediated by cytokines and SCFAs is illustrated in Figure 2.
A diverse array of immune cells, including macrophages, B lymphocytes, T lymphocytes, mast cells, endothelial cells, fibroblasts, and others, produce cytokines, which are essential signaling molecules (43). These cytokines exert their effects through cell surface receptors and play crucial roles in both pro-inflammatory and anti-inflammatory pathways. Additionally, cytokines are being explored as potential therapeutic targets for alleviating pathological pain resulting from inflammation or peripheral nerve injury (44). Furthermore, the modulation of pro-inflammatory cytokines by polysaccharides is being investigated as a strategy to mitigate immunosuppressive effects in patients undergoing treatment for immune-related conditions. In contrast, anti-inflammatory cytokines play regulatory roles in dampening inflammatory responses and balancing the actions of pro-inflammatory cytokines (45). Key cytokines that influence immune system function include IL-4, IL-10, IL-11, and IL-13.
Macrophages are key players in initiating various immune responses. Polysaccharides have been shown to activate macrophages, influencing cytokine secretion through diverse signaling pathways. Gupta et al. (46) demonstrated that polysaccharides extracted from Tinospora cordifolia (TCPs) can enhance the expression of nitric oxide synthase (NOS2), leading to increased nitric oxide production and enhanced microbicidal capabilities of macrophages. However, certain polysaccharides have immunosuppressive properties, inhibiting NF-κB and MAPK-mediated inflammation triggered by lipopolysaccharides (LPS). T cells consist of various helper subsets, each with distinct functions mediated by the secretion of specific cytokine profiles. Chen et al. (47) revealed that, through its interaction with Toll-like receptors, YCP serves as a secondary signal that activates T cells, promotes proliferation, and increases IFN production. Furthermore, by activating TLR-4 on dendritic cells, YCP promotes IL-12 secretion and upregulates CD80, CD86, and MHC II markers (48). Activated T cells are crucial for promoting the secretion of IFN-γ and IL-12 while suppressing IL-4 production, a mechanism vital for enhancing immune responses to foreign antigens (49). In contrast, immunoglobulins produced by B cells play a significant role in immune defense against exogenous antigens (50). Additionally, microglia, the innate immune cells of the central nervous system, produce various inflammatory cytokines in response to injury or infection.
In the context of gut microbiota-driven anaerobic fermentation, the breakdown of complex polysaccharides generates a diverse array of microbiota-derived metabolites. Among these, SCFAs are key modulators of immune function. Growing evidence highlights the ongoing regulatory influence of SCFAs on both local and systemic immune homeostasis, as well as immune cell functionality (51). SCFAs exert their effects through the inhibition of histone deacetylases and the activation of G protein-coupled receptors (GPCRs), which confer beneficial effects on immune cells. In a study by Chang et al. (52), the bacterial metabolite n-butyrate was shown to modulate intestinal macrophages by inhibiting histone deacetylases, making them less responsive to commensal microbes in the colon. SCFAs also play a crucial role in promoting the generation of anti-inflammatory cytokines. They activate the inflammasome in immune cells through a GPCR-mediated mechanism, involving hyperpolarization and calcium mobilization (53). This pathway leads to the synthesis of IL-10, a key anti-inflammatory cytokine, which depends on the suppression of histone deacetylase activity and activation of GPR43. Moreover, Ouyang et al. (54) emphasized the critical role of IL-22 in maintaining intestinal barrier integrity, balancing intestinal equilibrium, and protecting against inflammation. Recent studies have shown that innate lymphoid cells and CD4+ T cells produce IL-22 in response to SCFAs through the activation of GPR41 and inhibition of histone deacetylases (55).
The conformation of polysaccharides plays a significant role in their interaction with the immune system. For example, Satitmanwiwat et al. (56) found that triple-helix conformations exhibit greater immunomodulatory efficacy compared to β-glucans, as they stimulate TNF-α release by immune cells. The molecular weight of polysaccharides also influences immune responses, with higher molecular weight polysaccharides generally showing stronger effects. Zhao et al. (57) demonstrated that the immune effects of Schisandra chinensis polysaccharides are inversely related to their molecular weight. On the other hand, Chen et al. (58) found that lower molecular weight polysaccharides, with simpler structures, are more likely to cross cellular barriers. Functional groups in polysaccharides also impact immune responses in distinct ways. For instance, higher acetylation levels may reduce immunomodulatory effectiveness (59), while sulfate groups in pectic polysaccharides can enhance macrophage and neutrophil function, potentially improving immune responses (60). Additionally, branching in polysaccharides—driven by monosaccharide residues or chains—can affect immune regulation, such as IL-6 secretion induced by LPS (61). The details of these relationships are summarized in Table 1. Despite these insights, further research is needed to fully understand the structure-function relationships in immune-regulating polysaccharides and explore their potential applications in functional foods.
Table 1. Structural features and therapeutic applications of polysaccharides in immune-related diseases.
Bacteria ferment indigestible dietary fibers anaerobically to produce SCFAs, including acetate, butyrate, and propionate. These SCFAs can influence systemic health by signaling through G-protein-coupled receptors, such as GPR41, GPR43, and GPR109a (62, 63). They play a crucial role in regulating gut homeostasis and maintaining epithelial barriers. Butyrate, for example, acts as an inhibitor of histone deacetylase, promoting colonocyte proliferation, inhibiting stem cell proliferation, and regulating the polarization of anti-inflammatory macrophages (64). Additionally, butyrate directly stimulates colonic mucus secretion by upregulating Muc2 and glycoltransferase expression (65) and indirectly through autophagy. SCFAs also provide anti-inflammatory protection by regulating Tregs (66). High-fiber diets protect against allergies in mice by impairing Th2 differentiation and enhancing dendritic cell phagocytosis, whereas low-fiber diets exacerbate allergic inflammation (67). In type 1 diabetes, increased SCFA levels are linked to improved symptoms, which may result from a reduction in autoreactive T cells, induction of Tregs, and enhanced gut barrier function (68). It has been proposed that SCFAs can exert beneficial effects by directly influencing gut-derived hormones like GLP-1 and peptide YY, in addition to impacting systemic circulation (69). Beyond metabolic benefits, SCFAs stimulate intestinal gluconeogenesis (70). When administered alone or in combination—whether through drinking water, nanoparticles, or acetate supplementation—propionate, butyrate, and acetate can improve host metabolism and physiology (71). Notably, the effects of propionate are believed to be mediated through peripheral nerve stimulation of the vagus nerve. In contrast, high-fat diets increase gut microbiota-mediated acetate turnover, which promotes hyperphagia by increasing ghrelin secretion and enhancing energy storage (72). These effects appear to depend on the specific site where SCFAs are stimulated, emphasizing the need for further research into their role in regulating obesity.
Despite the growing interest in understanding the interaction between SCFAs and the immune system, several knowledge gaps remain. More research is needed to elucidate the molecular mechanisms by which SCFAs—such as acetate, butyrate, and propionate—affect immune cells, including Tregs, macrophages, and dendritic cells. The context-dependent effects of SCFAs in diseases like inflammatory bowel disease, type 2 diabetes, and allergic asthma also require further investigation to fully realize their therapeutic potential (73). Additionally, the role of SCFAs in the gut-brain axis, personalized nutrition, and long-term health impacts warrants deeper exploration. Developing innovative therapeutic formulations aimed at modulating SCFA levels, as well as understanding their interactions with other gut-derived metabolites, are promising yet underexplored areas. Finally, further research is needed to explore the relationship between SCFAs and obesity, as well as other metabolic disorders, including their feedback mechanisms with the nervous system. Addressing these gaps may lead to the development of novel therapeutic strategies for treating immune-related and metabolic diseases.
Several critical areas for further exploration regarding the interaction between SCFAs and the immune system include understanding the molecular mechanisms through which SCFAs modulate immune cell functions. This knowledge could lead to therapeutic applications for autoimmune and inflammatory diseases. Additionally, investigating the role of SCFAs in diseases such as inflammatory bowel disease, type 2 diabetes, and allergic asthma is essential to determine how SCFAs influence disease progression and treatment outcomes. The gut-brain axis represents another frontier, particularly in understanding how SCFAs affect neurological diseases and behaviors through mechanisms like inflammation, appetite regulation, and neural signaling. Personalized nutrition, tailored to an individual’s microbiome and SCFA profile, could optimize health outcomes. However, the long-term effects of sustained SCFA modulation via diet or supplementation remain unclear, highlighting the need for longitudinal studies. Developing therapeutic formulations to modulate SCFA levels or mimic their effects offers exciting new treatment possibilities. Moreover, understanding the interactions between SCFAs and other metabolites, such as bile acids and amino acid derivatives, is crucial for a more comprehensive understanding of their roles in health and disease.
Recent studies highlight the therapeutic potential of polysaccharide prebiotics in cancer and autoimmune disorders. For instance, fucoidan in colorectal cancer patients enhances chemotherapy sensitivity by upregulating Akkermansia abundance and suppressing TLR4/NF-κB signaling (74). In rheumatoid arthritis, β-glucan activates Dectin-1 to promote Treg differentiation and reduce pro-inflammatory cytokines (e.g., IL-17, TNF-α) (75). A clinical trial (NCT04128072) demonstrated that inulin combined with immune checkpoint inhibitors improved melanoma patients’ ORR (45% vs 28%) (76). Future research should focus on dose optimization and targeted delivery strategies for clinical translation.
The gut microbiota are well-known for their role in chronic autoimmune diseases, particularly brain-related disorders. These conditions involve complex signaling pathways that include neurotransmitters, immune cells, and microbiota-derived metabolites. While manipulating the microbiota to treat diseases such as multiple sclerosis (MS) shows promise, more systematic research is needed. Dietary interventions, including polysaccharides, have the potential to enhance immunity by regulating gut microbiota. Polysaccharides exhibit immunomodulatory and prebiotic effects, although their use in boosting immunity is not yet widespread. Future research should focus on understanding how these substances balance immune enhancement and immunosuppression, as well as determining specific intake patterns to modulate pro-inflammatory cytokines. Additionally, further investigation is needed to better understand the mechanisms promoting MS.
TZ: Conceptualization, Writing – original draft, Writing – review & editing. CW: Investigation, Writing – original draft, Writing – review & editing. YL: Writing – original draft, Writing – review & editing. BL: Methodology, Writing – original draft. MS: Writing – review & editing. WZ: Validation, Writing – original draft. CZ: Conceptualization, Supervision, Validation, Writing – original draft, Writing – review & editing.
The author(s) declare that no financial support was received for the research and/or publication of this article.
The authors declare that the research was conducted in the absence of any commercial or financial relationships that could be construed as a potential conflict of interest.
The author(s) declare that Generative AI was used in the creation of this manuscript. We use AI to correct word and grammar errors.
All claims expressed in this article are solely those of the authors and do not necessarily represent those of their affiliated organizations, or those of the publisher, the editors and the reviewers. Any product that may be evaluated in this article, or claim that may be made by its manufacturer, is not guaranteed or endorsed by the publisher.
1. Parker A, Fonseca S, Carding SR. Gut microbes and metabolites as modulators of blood-brain barrier integrity and brain health. Gut Microbes. (2020) 11:135–57. doi: 10.1080/19490976.2019.1638722
2. White EC, Houlden A, Bancroft AJ, Hayes KS, Goldrick M, Grencis RK, et al. Manipulation of host and parasite microbiotas: Survival strategies during chronic nematode infection. Sci Adv. (2018) 4:eaap7399. doi: 10.1126/sciadv.aap7399
3. Zheng D, Liwinski T, Elinav E. Interaction between microbiota and immunity in health and disease. Cell Res. (2020) 30:492–506. doi: 10.1038/s41422-020-0332-7
4. Sun M, Wu W, Liu Z, Cong Y. Microbiota metabolite short chain fatty acids, GPCR, and inflammatory bowel diseases. J Gastroenterol. (2017) 52:1–8. doi: 10.1007/s00535-016-1242-9
5. Pakpour S, Bhanvadia A, Zhu R, Amarnani A, Gibbons SM, Gurry T, et al. Identifying predictive features of Clostridium difficile infection recurrence before, during, and after primary antibiotic treatment. Microbiome. (2017) 5:148. doi: 10.1186/s40168-017-0368-1
6. Li X, Ma L, Zhang L. Molecular basis for Poria cocos mushroom polysaccharide used as an antitumor drug in China. J Cell Mol Med. (2019) 23:4–20. doi: 10.1111/jcmm.2019.23.issue-1
7. Wang L, Chen L, Li J, Di L, Wu H. Structural elucidation and immune-enhancing activity of peculiar polysaccharides fractioned from marine clam Meretrix meretrix (Linnaeus). Carbohydr Res. (2018) 338:2393–402. doi: 10.1016/j.carbpol.2018.08.106
8. El Kaoutari A, Armougom F, Gordon JI, Raoult D, Henrissat B. The abundance and variety of carbohydrate-active enzymes in the human gut microbiota. Nat Rev Microbiol. (2013) 11:497–504. doi: 10.1038/nrmicro3050
9. Li M, Yue H, Wang Y, Guo C, Du Z, Jin C, et al. Intestinal microbes derived butyrate is related to the immunomodulatory activities of Dendrobium officinale polysaccharide. Int J Biol Macromol. (2020) 149:717–23. doi: 10.1016/j.ijbiomac.2020.01.305
10. Barbosa JR, de Carvalho Junior RN. Polysaccharides obtained from natural edible sources and their role in modulating the immune system: Biologically active potential that can be exploited against COVID-19. Trends Food Sci Technol. (2021) 108:223–35. doi: 10.1016/j.tifs.2020.12.026
11. Yuan L, Zhong ZC, Liu Y. Structural characterisation and immunomodulatory activity of a neutral polysaccharide from Sambucus adnata Wall. Int J Biol Macromol. (2020) 154:1400–7. doi: 10.1016/j.ijbiomac.2019.11.021
12. Wang K, Yang X, Wu Z, et al. Dendrobium officinale polysaccharide protected CCl4-induced liver fibrosis through intestinal homeostasis and the LPS-TLR4-NF-κB signaling pathway. Front Pharmacol. (2020) 11:240. doi: 10.3389/fphar.2020.00240
13. Yu Y, Shen M, Song Q, Wang H, Li Q, Mei H, et al. Biological activities and pharmaceutical applications of polysaccharide from natural resources: A review. Carbohydr Polym. (2018) 183:91–101. doi: 10.1016/j.carbpol.2017.12.009
14. Jia RB, Wu J, Li ZR, Xie J. Structural characterization of polysaccharides from three seaweed species and their hypoglycemic and hypolipidemic activities in type 2 diabetic rats. Int J Biol Macromol. (2020) 155:1040–9. doi: 10.1016/j.ijbiomac.2019.11.068
15. Chang CJ, Lin CS, Lu CC, Ou ZR, Lin L, Sun B, et al. Ganoderma lucidum reduces obesity in mice by modulating the composition of the gut microbiota. Nat Commun. (2015) 6:7489. doi: 10.1038/ncomms8489
16. Luo JM, Zhang C, Liu R, Martel J, Ko YF, Ojcius DM, et al. Ganoderma lucidum polysaccharide alleviating colorectal cancer by alteration of special gut bacteria and regulation of gene expression of colonic epithelial cells. J Funct Foods. (2018) 47:127–35. doi: 10.1016/j.jff.2018.05.041
17. Thomson P, Medina DA, Ortuzar V, Gao LJ, Ou SY, Liu L, et al. Anti-inflammatory effect of microbial consortia during the utilization of dietary polysaccharides. Food Res Int. (2018) 109:14–23. doi: 10.1016/j.foodres.2018.04.008
18. Mitsou EK, Panopoulou N, Turunen K, Gotteland M, Garrido D. Prebiotic potential of barley derived β-glucan at low intake levels: A randomised, double-blinded, placebo-controlled clinical study. Food Res Int. (2010) 43:1086–92. doi: 10.1016/j.foodres.2010.01.020
19. Yang J, Martinez I, Walter J, Spiliotis V, Kyriacou A. In vitro characterization of the impact of selected dietary fibers on fecal microbiota composition and short chain fatty acid production. Anaerobe. (2013) 23:74–81. doi: 10.1016/j.anaerobe.2013.06.012
20. Shang QS, Song GR, Zhang MF, Shi JJ, Xu CY, Hao JJ, et al. Dietary fucoidan improves metabolic syndrome in association with increased Akkermansia population in the gut microbiota of high-fat diet-fed mice. J Funct Foods. (2017) 28:138–46. doi: 10.1016/j.jff.2016.11.002
21. Martin-Gallausiaux C, Marinelli L, Blottiere HM, Shi JJ, Xu CY, Hao JJ, et al. SCFA: Mechanisms and functional importance in the gut. Proc Nutr Soc. (2021) 80:37–49. doi: 10.1017/S0029665120006916
22. Rooks MG, Garrett WS. Gut microbiota, metabolites and host immunity. Nat Rev Immunol. (2016) 16:341–52. doi: 10.1038/nri.2016.42
23. Yang W, Cong Y. Gut microbiota-derived metabolites in the regulation of host immune responses and immune-related inflammatory diseases. Cell Mol Immunol. (2021) 18:866–77. doi: 10.1038/s41423-021-00661-4
24. den Besten G, van Eunen K, Groen AK, Venema K, Reijngoud DJ, Bakker BM. The role of short-chain fatty acids in the interplay between diet, gut microbiota, and host energy metabolism. J Lipid Res. (2013) 54:2325–40. doi: 10.1194/jlr.R036012
25. Tan J, McKenzie C, Potamitis M, et al. The role of short-chain fatty acids in health and disease. Adv Immunol. (2014) 121:91–119. doi: 10.1016/B978-0-12-800100-4.00003-9
26. Wang G. Human antimicrobial peptides and proteins. Pharmaceutics. (2014) 7:545–94. doi: 10.3390/ph7050545
27. Hoeppli RE, Wu D, Cook L, et al. The environment of regulatory T cell biology: cytokines, metabolites, and the microbiome. Front Immunol. (2015) 6:61. doi: 10.3389/fimmu.2015.00061
28. Miller TL, Wolin MJ. Pathways of acetate, propionate, and butyrate formation by the human fecal microbial flora. Appl Environ Microbiol. (1996) 62:1589–92. doi: 10.1128/aem.62.5.1589-1592.1996
29. Reichardt N, Duncan SH, Young P, Belenguer A, McWilliam Leitch C, Scott KP, et al. Phylogenetic distribution of three pathways for propionate production within the human gut microbiota. ISME J. (2014) 8:1323–35. doi: 10.1038/ismej.2014.14
30. Louis P, Flint HJ. Diversity, metabolism and microbial ecology of butyrate-producing bacteria from the human large intestine. FEMS Microbiol Lett. (2009) 294:1–8. doi: 10.1111/j.1574-6968.2009.01514.x
31. Vital M, Howe AC, Tiedje JM. Revealing the bacterial butyrate synthesis pathways by analyzing (meta)genomic data. mBio. (2014) 5:e00889. doi: 10.1128/mBio.00889-14
32. Liu Y, Liu W, Li J, Tang S, Wang M, Huang W, et al. A polysaccharide extracted from Astragalus membranaceus residue improves cognitive dysfunction by altering gut microbiota in diabetic mice. Carbohydr Polym. (2019) 205:500–12. doi: 10.1016/j.carbpol.2018.10.041
33. Nam J, Kim A, Kim K, Moon JH, Baig J, Phoo M, et al. Engineered polysaccharides for controlling innate and adaptive immune responses. Nat Rev Bioeng. (2024) 2:733–51. doi: 10.1038/s44222-024-00193-2
34. Brown G. Dectin-1: a signalling non-TLR pattern-recognition receptor. Nat Rev Immunol. (2006) 6:33–43. doi: 10.1038/nri1745
35. Lai CY, Yang LC, Lin WC. Type II arabinogalactan from Anoectochilus formosanus induced dendritic cell maturation through TLR2 and TLR4. Phytomedicine. (2015) 22:1207–14. doi: 10.1016/j.phymed.2015.10.010
36. Donadio JLS, Fabi JP, Sztein MB, Salerno-Gonçalves R. Dietary fiber pectin: challenges and potential anti-inflammatory benefits for preterms and newborns. Front Nutr. (2024) 10:1286138. doi: 10.3389/fnut.2023.1286138
37. Švajger U, Anderluh M, Jeras M, Obermajer N. C-type lectin DC-SIGN: An adhesion, signalling and antigen-uptake molecule that guides dendritic cells in immunity. Cell Signal. (2010) 22:1397–405. doi: 10.1016/j.cellsig.2010.03.018
38. Kendrick SF, O’Boyle G, Mann J, Zeybel M, Palmer J, Jones DE, et al. Acetate, the key modulator of inflammatory responses in acute alcoholic hepatitis. Hepatology. (2010) 51:1988–97. doi: 10.1002/hep.23572
39. Ikeda T, Nishida A, Yamano M, et al. Short-chain fatty acid receptors and gut microbiota as therapeutic targets in metabolic, immune, and neurological diseases. Pharmacol Ther. (2022) 239:108273. doi: 10.1016/j.pharmthera.2022.108273
40. Okeke EB, Uzonna JE. The pivotal role of regulatory T cells in the regulation of innate immune cells. Front Immunol. (2019) 10:680. doi: 10.3389/fimmu.2019.00680
41. Donohoe DR, Garge N, Zhang X, et al. The microbiome and butyrate regulate energy metabolism and autophagy in the mammalian colon. Cell Metab. (2011) 13:517–26. doi: 10.1016/j.cmet.2011.02.018
42. Recharla N, Geesala R, Shi XZ. Gut microbial metabolite butyrate and its therapeutic role in inflammatory bowel disease: A literature review. Nutrients. (2023) 15:2275. doi: 10.3390/nu15102275
43. Cui H, Zhang L, Shi Y. Biomaterials-mediated ligation of immune cell surface receptors for immunoengineering. Immuno-Oncol Technol. (2024) 21:100695. doi: 10.1016/j.iotech.2023.100695
44. Vanderwall AG, Milligan ED. Cytokines in pain: harnessing endogenous anti-inflammatory signaling for improved pain management. Front Immunol. (2019) 10:3009. doi: 10.3389/fimmu.2019.03009
45. Opal SM, DePalo VA. Anti-inflammatory cytokines. Chest. (2000) 117:1162–72. doi: 10.1378/chest.117.4.1162
46. Gupta PK, Rajan MGR, Kulkarni S. Activation of murine macrophages by G1–4A, a polysaccharide from Tinospora cordifolia, in TLR4/MyD88 dependent manner. Int Immunopharmacol. (2017) 50:168–77. doi: 10.1016/j.intimp.2017.06.025
47. Chen S, Ding R, Zhou Y, et al. Immunomodulatory effects of polysaccharide from marine fungus Phoma herbarum YS4108 on T cells and dendritic cells. Mediators Inflamm. (2014) 2014:738631. doi: 10.1155/2014/738631
48. Dong C. Cytokine regulation and function in T cells. Annu Rev Immunol. (2021) 39:51–76. doi: 10.1146/annurev-immunol-061020-053702
49. Garver LS, Xi Z, Dimopoulos G. Immunoglobulin superfamily members play an important role in the mosquito immune system. Dev Comp Immunol. (2008) 32:519–31. doi: 10.1016/j.dci.2007.09.007
50. Ney LM, Wipplinger M, Grossmann M, Engert N, Wegner VD, Mosig AS. Short chain fatty acids: key regulators of the local and systemic immune response in inflammatory diseases and infections. Open Biol. (2023) 13:230014. doi: 10.1098/rsob.230014
51. Chang PV, Hao L, Offermanns S, Medzhitov R. The microbial metabolite butyrate regulates intestinal macrophage function via histone deacetylase inhibition. Proc Natl Acad Sci USA. (2014) 111:2247–52. doi: 10.1073/pnas.1322269111
52. Macia L, Tan J, Vieira AT, Leach K, Stanley D, Luong S, et al. Metabolite-sensing receptors GPR43 and GPR109A facilitate dietary fibre-induced gut homeostasis through regulation of the inflammasome. Nat Commun. (2015) 6:6734. doi: 10.1038/ncomms7734
53. Ouyang W, O’Garra A. IL-10 family cytokines IL-10 and IL-22: From basic science to clinical translation. Immunity. (2019) 50:871–91. doi: 10.1016/j.immuni.2019.03.020
54. Yang W, Yu T, Huang X, Bilotta AJ, Xu L, Lu Y, et al. Intestinal microbiota-derived short-chain fatty acids regulation of immune cell IL-22 production and gut immunity. Nat Commun. (2020) 11:4457. doi: 10.1038/s41467-020-18262-6
55. Satitmanwiwat S, Ratanakhanokchai K, Laohakunjit N, Chao LK, Chen ST, Pason P, et al. Improved purity and immunostimulatory activity of beta-(1→3)(1→6)-glucan from Pleurotus sajor-caju using cell wall-degrading enzymes. J Agric Food Chem. (2012) 60:5423–30. doi: 10.1021/jf300354x
56. Zhao T, Feng Y, Li J, Mao R, Zou Y, Feng W, et al. Schisandra polysaccharide evokes immunomodulatory activity through TLR4-mediated activation of macrophages. Int J Biol Macromol. (2014) 65:33–40. doi: 10.1016/j.ijbiomac.2014.01.018
57. Chen N, Zhou M, Dong X, Qu J, Gong F, Han Y, et al. Epidemiological and clinical characteristics of 99 cases of 2019 novel coronavirus pneumonia in Wuhan, China: A descriptive study. Lancet. (2020) 395:507–13. doi: 10.1016/S0140-6736(20)30211-7
58. Wang XS, Liu L, Fang JN, Fang JN. Immunological activities and structure of pectin from Centella asiatica. Carbohydr Polym. (2005) 60:95–101. doi: 10.1016/j.carbpol.2004.11.031
59. Xie G, Schepetkin IA, Siemsen DW, Kirpotina LN, Wiley JA, Quinn MT. Fractionation and characterization of biologically-active polysaccharides from Artemisia tripartita. Phytochemistry. (2008) 69:1359–71. doi: 10.1016/j.phytochem.2008.01.009
60. Ishisono K, Yabe T, Kitaguchi K. Citrus pectin attenuates endotoxin shock via suppression of Toll-like receptor signaling in Peyer’s patch myeloid cells. J Nutr Biochem. (2017) 50:38–45. doi: 10.1016/j.jnutbio.2017.07.016
61. Brown AJ, Goldsworthy SM, Barnes AA, Eilert MM, Tcheang L, Daniels D, et al. The orphan G protein-coupled receptors GPR41 and GPR43 are activated by propionate and other short chain carboxylic acids. J Biol Chem. (2003) 278:11312–9. doi: 10.1074/jbc.M211609200
62. Koh A, De Vadder F, Kovatcheva-Datchary P, Bäckhed F, Bäckhed F. From dietary fiber to host physiology: short-chain fatty acids as key bacterial metabolites. Cell. (2016) 165:1332–45. doi: 10.1016/j.cell.2016.05.041
63. Fukuda S, Toh H, Hase K, Oshima K, Nakanishi Y, Yoshimura K, et al. Bifidobacteria can protect from enteropathogenic infection through production of acetate. Nature. (2011) 469:543–7. doi: 10.1038/nature09646
64. Paulson JC, Colley KJ. Glycosyltransferases. Structure, localization, and control of cell type-specific glycosylation. J Biol Chem. (1989) 264:17615–8. doi: 10.1016/S0021-9258(19)84610-0
65. Smith PM, Howitt MR, Panikov N, Michaud M, Gallini CA, Bohlooly-Y M, et al. The microbial metabolites, short-chain fatty acids, regulate colonic Treg cell homeostasis. Science. (2013) 341:569–73. doi: 10.1126/science.1241165
66. Trompette A, Gollwitzer ES, Yadava K, Sichelstiel AK, Sprenger N, Ngom-Bru C, et al. Gut microbiota metabolism of dietary fiber influences allergic airway disease and hematopoiesis. Nat Med. (2014) 20:159–66. doi: 10.1038/nm.3444
67. Marino E, Richards JL, McLeod KH, Stanley D, Yap YA, Knight J, et al. Gut microbial metabolites limit the frequency of autoimmune T cells and protect against type 1 diabetes. Nat Immunol. (2017) 18:552–62. doi: 10.1038/ni.3713
68. Brooks L, Viardot A, Tsakmaki A, Stolarczyk E, Howard JK, Cani PD, et al. Fermentable carbohydrate stimulates FFAR2-dependent colonic PYY cell expansion to increase satiety. Mol Metab. (2017) 6:48–60. doi: 10.1016/j.molmet.2016.10.011
69. De Vadder F, Kovatcheva-Datchary P, Goncalves D, Vinera J, Zitoun C, Duchampt A, et al. Microbiota-generated metabolites promote metabolic benefits via gut-brain neural circuits. Cell. (2014) 156:84–96. doi: 10.1016/j.cell.2013.12.016
70. Lin HV, Frassetto A, Kowalik EJ Jr, Nawrocki AR, Lu MM, Kosinski JR, et al. Butyrate and propionate protect against diet-induced obesity and regulate gut hormones via free fatty acid receptor 3-independent mechanisms. PLoS One. (2012) 7:e35240. doi: 10.1371/journal.pone.0035240
71. Perry RJ, Peng L, Barry NA, Cline GW, Zhang D, Cardone RL, et al. Acetate mediates a microbiome-brain-beta-cell axis to promote metabolic syndrome. Nature. (2016) 534:213–7. doi: 10.1038/nature18309
72. Campos-Perez W, Martinez-Lopez E. Effects of short chain fatty acids on metabolic and inflammatory processes in human health. Biochim Biophys Acta Mol Cell Biol Lipids. (2021) 1866:158900. doi: 10.1016/j.bbalip.2021.158900
73. Guo C, Huo YJ, Li Y, Han Y, Zhou D. Gut-brain axis: Focus on gut metabolites short-chain fatty acids. World J Clin Cases. (2022) 10:1754–63. doi: 10.12998/wjcc.v10.i6.1754
74. Zhang T, Gao G, Kwok LY, Sun Z. Gut microbiome-targeted therapies for Alzheimer’s disease. Gut Microbes. (2023) 15:2271613. doi: 10.1080/19490976.2023.2271613
75. Li T, Liu T, Zhao Z, Pan Y, Xu X, Zhang Y, et al. Antifungal immunity mediated by C-type lectin receptors may be a novel target in immunotherapy for urothelial bladder cancer. Front Immunol. (2022) 13:911325. doi: 10.3389/fimmu.2022.911325
Keywords: polysaccharides, immune regulation, gut microbiota, SCFAs, cytokines
Citation: Zhao T, Wang C, Liu Y, Li B, Shao M, Zhao W and Zhou C (2025) The role of polysaccharides in immune regulation through gut microbiota: mechanisms and implications. Front. Immunol. 16:1555414. doi: 10.3389/fimmu.2025.1555414
Received: 04 January 2025; Accepted: 05 March 2025;
Published: 31 March 2025.
Edited by:
Barbara E. Teixeira-Costa, Fluminense Federal University, BrazilReviewed by:
Jayvadan Jayantilal Vaishnav, Parul University, IndiaCopyright © 2025 Zhao, Wang, Liu, Li, Shao, Zhao and Zhou. This is an open-access article distributed under the terms of the Creative Commons Attribution License (CC BY). The use, distribution or reproduction in other forums is permitted, provided the original author(s) and the copyright owner(s) are credited and that the original publication in this journal is cited, in accordance with accepted academic practice. No use, distribution or reproduction is permitted which does not comply with these terms.
*Correspondence: Wuyang Zhao, OTE3OTAyOTgzQHFxLmNvbQ==; Chuang Zhou, emhvdWNodWFuZzIwMjQwOEAxNjMuY29t
†These authors share first authorship
Disclaimer: All claims expressed in this article are solely those of the authors and do not necessarily represent those of their affiliated organizations, or those of the publisher, the editors and the reviewers. Any product that may be evaluated in this article or claim that may be made by its manufacturer is not guaranteed or endorsed by the publisher.
Research integrity at Frontiers
Learn more about the work of our research integrity team to safeguard the quality of each article we publish.