- 1Laboratorio de Investigaciones Bioquímicas de la Facultad de Medicina (LIBIM), Instituto de Química Básica y Aplicada del Nordeste Argentino (IQUIBA-NEA), Universidad Nacional del Nordeste, Consejo Nacional de Investigaciones Científicas y Técnicas (UNNE-CONICET), Corrientes, Argentina
- 2Instituto de Biología y Genética Molecular, Consejo Superior de Investigaciones Científicas Uva, Valladolid, Spain
- 3Departamento de Bioquímica y Biología Molecular y Fisiología, Universidad de Valladolid, Valladolid, Spain
- 4Centro de Investigación Biomédica en Red de Diabetes y Enfermedades Metabólicas Asociadas (CIBERDEM), Instituto de Salud Carlos III, Madrid, Spain
Macrophages, crucial innate immune cells, defend against pathogens and resolve inflammation, maintaining tissue balance. They perform phagocytosis, present antigens to T cells, and bond innate and adaptive immunity through various activation states. Classical activation is associated with Th1 responses and interferon γ production, while alternative activation, induced by interleukin 4, is characterized by increased endocytosis, reduced secretion of pro-inflammatory cytokines, and roles in immunoregulation and tissue remodeling. Although these represent opposite extremes observed in vitro, the remarkable plasticity of macrophages allows for a wide spectrum of activation phenotypes that are complex to characterize experimentally. While the application of omics techniques has resulted in significant advances in the characterization of macrophage polarization, lipidomic studies have received lesser attention. Beyond their role as structural components and energy sources, lipids function as signaling molecules that regulate macrophage activation and polarization, thereby shaping immune responses. This work reviews the interaction between lipid signaling and macrophage polarization, exploring how lipid metabolism influences macrophage phenotype and function. These insights offer potential therapeutic strategies for immune-mediated diseases and inflammation-related disorders, including inflammaging.
1 Introduction
Macrophages are tissue-resident cells that act as sentinels of the immune system, regulating a delicate balance between defense against pathogens and initiation/resolution of inflammation, thus maintaining tissue homeostasis (1–3). Macrophages exhibit a high degree of plasticity and diversity. It is widely acknowledged that these cells originate from progenitors starting from embryogenesis onwards and continuing throughout an individual’s lifespan. Many tissue-resident macrophages are derived from embryonic progenitors and are capable of self-renewal without relying on adult blood monocytes. In steady-state conditions, fetal-derived and monocyte-derived macrophages co-exist, both contributing to tissue homeostasis. During inflammation, monocytes are recruited to generate macrophages that play crucial roles in either promoting local inflammation or facilitating its resolution (4–6). This guarantees a permanent presence of macrophages in adult tissues through self-renewal, and increases in their number during inflammatory circumstances (7, 8).
The concept of classical macrophage activation was first introduced by Mackaness (9) to describe the antigen-dependent but nonspecific enhanced microbicidal activity of macrophages following secondary pathogen exposure. This activity, later linked to Th1 (CD4+ T helper lymphocytes) responses and interferon γ (IFNγ) production, was also associated with cytotoxic and antitumoral properties (9, 10). In contrast, IFNγ was found to inhibit the expression of the macrophage mannose receptor, while interleukin 4 (IL4) enhanced its expression and induced an alternative activation, characterized by increased endocytic clearance and reduced pro-inflammatory cytokine secretion (11, 12). Mills and colleagues (13–15) later proposed the M1/M2 classification, observing that macrophages from Leishmania major–resistant Th1 mouse strains produced more nitric oxide when activated by IFNγ or bacterial lipopolysaccharide (LPS) compared to macrophages from Th2 strains, which metabolized arginine to ornithine instead. Since nitric oxide inhibits cell division and ornithine promotes it, the M1 and M2 phenotypes were hypothesized to have opposing roles in inflammation. Over time, research has revealed a spectrum of macrophage activation states between M1 and M2, depending largely on the type of stimuli (16, 17). Today, M1 macrophages are recognized as classically activated (LPS plus IFNγ), pro-inflammatory cells that express inducible nitric oxide synthase (iNOS) and produce pro-inflammatory cytokines, initiating inflammation. In contrast, M2 macrophages are alternatively activated (IL4 or IL13), anti-inflammatory/reparative cells that express high levels of arginase and produce anti-inflammatory cytokines, promoting the resolution of inflammation.
Macrophage polarization leads to notable alterations in gene expression, precluding the definition of a specific activation state by a single gene. This uncertainty is often mitigated by using multiple markers to characterize activation outcomes. Many laboratories have expanded marker assignments to include transcription factors, cytokines, chemokines, and cell surface markers, aiming for a comprehensive understanding of macrophage activation (18–21). These aspects highlight the complexity of macrophage polarization and the need to refine our understanding of this paradigm beyond conventional approaches. The shift from traditional gene and protein markers toward omics approaches, such as transcriptomics and proteomics, has improved our understanding of macrophage activation (22–25). However, lipidomics has received lesser attention. Beyond their traditional roles as structural components and energy sources, lipids function as signaling molecules that influence numerous cellular processes (26, 27). In fact, lipid metabolism and signaling pathways have emerged as key regulators of macrophage activation and polarization, thereby shaping immune responses and inflammatory outcomes (28). In this review, we comprehensively explore the sophisticated interplay between lipid signaling and macrophage polarization, focusing on how lipid metabolism influences macrophage phenotype and function and vice versa.
2 Intermediary metabolism and its influence on macrophage polarization
2.1 Immunometabolism
Energy production is crucial for the proper functioning of cells, whether they are in a resting state or activated. Cells primarily generate energy through two key pathways: glycolysis and oxidative phosphorylation (OXPHOS). Similar to other normal cells, macrophages in their resting state largely rely on mitochondrial OXPHOS and catabolic metabolism to meet their energy requirements. However, when activated and, especially during classical activation, there is an increase in aerobic glycolysis, and reduction of OXPHOS, even in the presence of adequate oxygen levels. This phenomenon is referred to as metabolic reprogramming or Warburg effect (29). Studies on immunometabolism have demonstrated that metabolic reprogramming not only governs macrophage phenotype, but also influences their plasticity, depending on the primary immune specific function they need to fulfill (30, 31). It is clear that an interplay exists between the phenotype and the metabolic energy status of macrophages. Several high-quality review articles have been published on this topic, and the interested reader is kindly referred to them (28, 31–37). Figures 1, 2 provide a summary of these contributions, highlighting their connection to lipid metabolism and positioning acetyl-CoA and acyl-CoA at the core of intermediary metabolism. Notably, the origin and fate of acetyl-CoA are key factors that help distinguish between M1 and M2 (38).
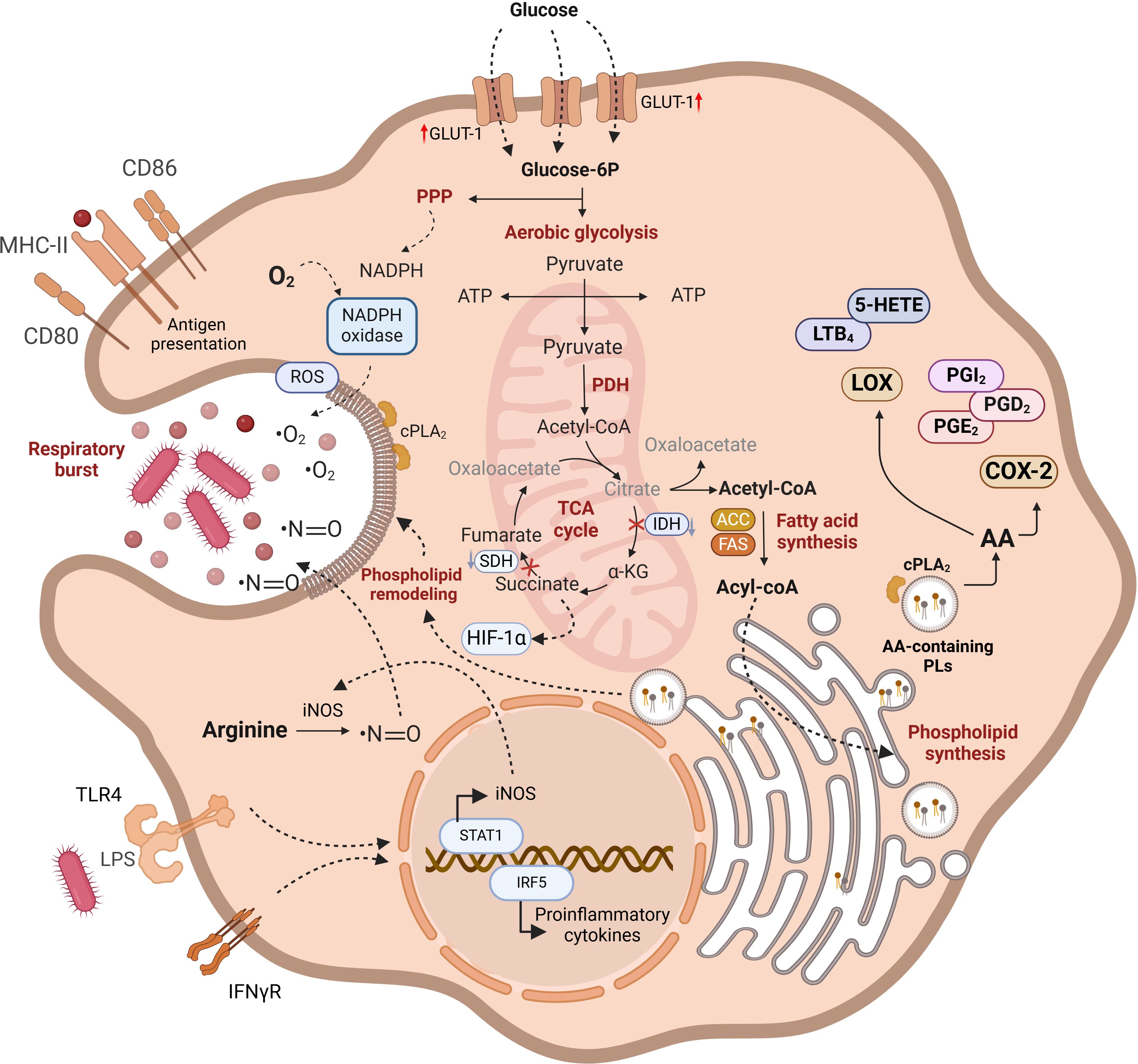
Figure 1. Functional characteristics of M1 macrophages. Lipid metabolism plays a crucial role in the metabolic and functional characteristics of M1 macrophages. Increased glucose uptake via GLUT-1 enhances glycolysis, but glucose-6P is also diverted to the pentose phosphate pathway (PPP) for NADPH and ROS production. Disruptions in the TCA cycle lead to the mitochondrial export of citrate and succinate, with citrate being converted into acetyl-CoA, which is pivotal for the synthesis of acyl-CoA and phospholipids. These lipids are vital for membrane remodeling during phagosome formation. TLR4 and IFNγR signaling enhance proinflammatory cytokine production and iNOS synthesis, contributing to ROS generation. Free arachidonic acid arising from phospholipid hydrolysis serves as a substrate for cyclooxygenase-2 and 5-lipoxygenase (5-LOX), resulting in the production of proinflammatory eicosanoids. Lipid pathways are in bold highlighting the importance of lipid metabolism in maintaining M1 macrophage functionality. AA-containing phospholipids are produced from preexistig phospholipids via deacylation/reacylation reactions. For further details see text.
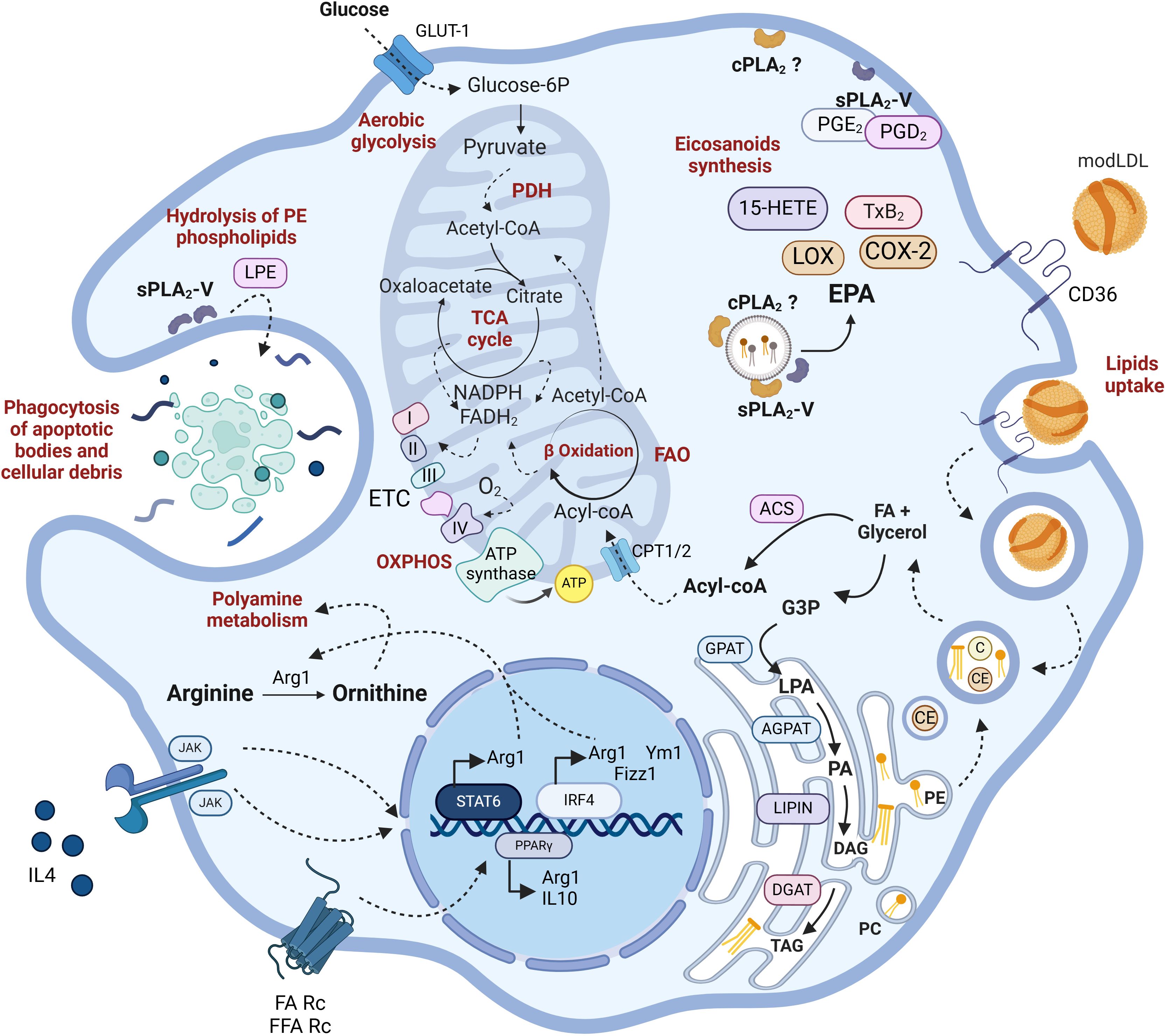
Figure 2. Lipid metabolism in M2 macrophages. IL4R signaling leads to the upregulation of typical M2 markers such as Arg1, YM1, or Fizz1. Additionally, PPARγ is upregulated by receptor-mediated signaling stimulated by free FA (FFA) or IL4 itself. M2 macrophages possess fully functional glycolytic and TCA cycles in contrast to M1. They metabolize arginine to produce ornithine and other polyamines that promote cell proliferation. They exhibit a high rate of phagocytosis focused on degrading cellular debris, dead bacteria, and opsonized particles. Through CD36-mediated endocytosis, they internalize modified LDLs, whose lipids serve for membrane remodeling, fueling FAO, and ETC. While the involvement of sPLA2-V in phagocytosis, via generation of ethanolamine lysophospholipids (LPE) has been well established, the role of cPLA2α is less characterized. Arg1, arginase-1; Chi3l3, chitinase-like protein 3; Fizz1, found in inflammatory zone-1 (also known as resistin-like alpha, Retnla); PPARγ, peroxisome proliferator-activated receptor γ. For further details see text.
In a nutshell, pro-inflammatory M1 macrophages are metabolically characterized by a high reliance on aerobic glycolysis and present two key breaks in the TCA cycle, leading to the accumulation of citrate, succinate, and itaconate. The excess succinate contributes to the stabilization of HIF1α, which subsequently activates the transcription of glycolytic genes, thereby sustaining the glycolytic metabolism in M1 macrophages. Additionally, M1 macrophages express high levels of iNOS, which produces nitric oxide from arginine. Nitric oxide, a free radical with microbicidal properties, inhibits the ETC, further reducing mitochondrial respiration. Additionally, M1 macrophages show increased production of ROS and fatty acid synthesis (Figure 1). In contrast, M2 macrophages are distinguished by their expression of the enzyme arginase, which catalyzes the hydrolysis of arginine into ornithine and urea, reducing the availability of arginine for nitric oxide production (Figure 2). M2 macrophages typically depend on OXPHOS, with an intact TCA cycle that provides substrates necessary for the ETC.
2.2 Fatty acid metabolism in polarized macrophages
Macrophages incorporate fatty acids (FA) from lipoprotein lipids via specific or scavenger receptors. In addition, they possess all the machinery necessary to synthesize FA via de novo pathways. Synthesis occurs in the cytosol using acetyl-CoA that is produced by ATP-citrate lyase (ACLY). This enzyme acts on the citrate that was originally generated in the mitochondria by the TCA cycle. Citrate leaves the organelle in exchange for malate in a process known as the citrate shuttle (Figure 1). Cytosolic acetyl-CoA is transformed to malonyl-CoA by acetyl-CoA carboxylase (ACC), participating in the synthesis of FA by repetitive additions of two carbons, catalyzed by fatty acid synthase (FAS), until the generation of a 16-carbon fatty acid, palmitic acid. Acetyl-CoA is also used for the synthesis of cholesterol in a complex multistep cytosolic pathway that is known as the mevalonate pathway. FA can be esterified to glycerol-3-phosphate or cholesterol to ultimately form phospholipids, triacylglycerols or cholesterol esters. Conversely, FA can be β-oxidized in the mitochondrial matrix, generating energy for the cell in a process that takes place through a repetitive cyclic series of reactions catalyzed by acyl CoA dehydrogenase, enoyl CoA hydratase, β-hydroxyl acyl CoA dehydrogenase, and β-keto thiolase. To enter the mitochondrial matrix, FA are transformed to fatty acyl-CoA by the fatty acyl-CoA synthetase and cross mitochondrial membranes using the transporters carnitine palmitoyl transferase I (CPT1) and carnitine palmitoyl transferase II (CPT-2) located in the outer membrane and the inner membrane respectively (Figure 2).
After palmitic acid is synthesized by FAS, FA diversity is created by the action of elongases and desaturases, which supply the cells with a varied number of FAs for membrane phospholipid synthesis and neutral lipid formation. FA desaturation and elongation enzymes are integral to macrophage physiology, influencing membrane composition, signaling pathways, and functional responses. Desaturases introduce double bonds into FA chains, altering their degree of unsaturation, while elongases extend the carbon chain length of FAs (39). These modifications are crucial for maintaining the fluidity and integrity of cellular membranes, which in turn affect receptor function and signal transduction in macrophages (40, 41). For instance, the activity of stearoyl-CoA desaturase 1, the enzyme that converts palmitic acid to palmitoleic acid, and stearic acid to oleic acid, has been implicated in modulating lipid composition, thereby influencing macrophage activation and inflammatory responses (42).
The balance between saturated and unsaturated FAs, regulated by both desaturases and elongases, appears to be essential for macrophage polarization. Alterations in desaturase activity can skew macrophage polarization, impacting immune responses and disease progression. In this regard, it has been shown that impairment of systemic docosahexaenoic acid (DHA, 22:6n–3) synthesis, which involves desaturase and elongase activities, affects macrophage plasticity and polarization (43). Thus, the absence of the elongase Elovl2 results in the increased expression of M1 markers in both M1 and M2 macrophages, and the reduced expression of M2 markers in M2 macrophages. Consequently, this elongase plays a crucial role in regulating the balance between pro-inflammatory and anti-inflammatory processes. Importantly, DHA supplementation in animals counteracts these effects (43). Other studies found that changes in FA desaturation were associated with insulin resistance, highlighting the broader implications of these enzymes in metabolic regulation (44). Moreover, the interplay between FA metabolism and macrophage function extends to disease contexts such as idiopathic pulmonary fibrosis. Dysregulation of FA elongation and desaturation pathways has been observed in this disorder, suggesting that targeting these metabolic processes could offer therapeutic avenues (45).
Numerous studies have described specific roles for FAs in regulating the inflammatory response of macrophages. Saturated FA such as palmitic acid and stearic acid, when in excess, generally promote inflammation and are associated with type 1 immune responses (46). In contrast, monounsaturated fatty acids such as oleic acid and palmitoleic acid can have anti-inflammatory effects, aligning with type 2 immune responses (47–49). Mammalian cells cannot synthesize long-chain polyunsaturated FAs (PUFA) of the n–6 series, (e.g. arachidonic acid, AA, 20:4n–6), or those of the n-3 series (e.g. DHA, 22:6n–3) de novo. However, they can produce them through the elongation and desaturation of their essential precursors, linoleic acid (18:2n–6) and α-linolenic acid (18:3n–3), which must be obtained from dietary sources. Because of the key roles of PUFAs in immune cell function, dietary habits significantly influence macrophage polarization. Several lines of research suggest that PUFAs of the n–3 series promote an M2 anti-inflammatory macrophage phenotype, which aids in tissue repair and resolution of inflammation (50–52). High dietary intake of n–3 PUFAs enhances M2 macrophage polarization and chemotaxis, thereby regulating tissue inflammation and metabolic health (53–56). Whether these effects are mediated by the FA itself or an oxygenated metabolite(s) is currently a matter of strong debate (57, 58). Conversely, diets high in n–6 PUFAs, which are abundant in animal fats, may predispose macrophages toward a pro-inflammatory M1 state (50–52). This shift can contribute to chronic inflammatory conditions. For example, a positive correlation has been found between the consumption of animal fats and the presence of pro-inflammatory macrophages in human adipose tissue, suggesting that dietary FA composition directly affects macrophage behavior (54–56, 59, 60). Therefore, maintaining a balanced intake of n–3 and n–6 PUFAs may be crucial for modulating macrophage polarization and supporting optimal immune function.
During proinflammatory activation of macrophages (TLR4 activation by LPS), AMPK activity decreases. As a consequence, phosphorylation of ACC is reduced, which increases its activity and promotes the conversion of acetyl-CoA to malonyl-CoA. Since malonyl-CoA is an inhibitor of CPT-1, M1 macrophages display reduced FAO and increased lipogenesis (61). In fact, lipid biogenesis, coupled with aerobic glycolysis, drives in M1 macrophages a metabolic shift akin to the Warburg effect (Figure 1). The source of acetyl-CoA necessary for FA synthesis in M1 macrophages is the glycolysis. As in the rest of the cells, glycolysis generates pyruvate from glucose that can be converted to lactate and secreted, or undergo decarboxylation in the mitochondria to render acetyl-CoA. Acetyl-CoA enters the TCA as citrate after condensation with oxaloacetate (OAA). Because in M1 macrophages there is a reduced expression of the TCA cycle enzyme isocitrate dehydrogenase, citrate increases in mitochondria and is shuttled to the cytosol to be used for lipid synthesis (62, 63). The mitochondrial citrate carrier, responsible for the transport of citrate from the mitochondria to the cytosol, and the following three cytosolic key enzymes participating in FA synthesis, namely ACLY, ACC and FAS, have all been implicated in LPS-induced inflammatory processes by participating in the production of NO, ROS and prostaglandins (CIC and ACLY); inducing epigenetic remodeling through promotion of histone acetylation (ACLY); helping during an early metabolic switch to glycolysis, and remodeling of the macrophage lipidome (ACC); altering the cell membrane order and composition, and disrupting Rho GTPase signaling, which is crucial for cell adhesion, migration, and activation (FAS) (63–66).
Transcriptional regulation of FA biosynthesis is controlled in a coordinated and positive manner by sterol regulatory element binding proteins (SREBPs) and by liver X receptors (LXR). LXR induces the expression of SREBP1, and both together participate in the upregulation of FA synthetic genes, especially ACC and FAS (67). However, in macrophages, these transcription factors have also other roles and fates. LXR has an anti-inflammatory role, antagonizing NF-κB action on inflammatory genes during TLR4 activation (68, 69). By participating in the upregulation of the plasma membrane cholesterol transporter ABCA1, LXR contributes to alter membrane cholesterol homeostasis, inhibiting the recruitment of the TLR adaptors MyD88 and TRAF6, and blocking in this way the activation of MAPK and NF-κB during TLR4 stimulation (70). In contrast, SREBP1 regulates the levels of some inflammasome receptors participating in IL1β production and inflammation development during LPS activation (71). Interestingly, it has also been described that SREBP1 activity exhibits a late increase during TLR4 challenge, thereby participating in the expression of genes that regulate the synthesis of anti-inflammatory PUFAs (72). In this manner, SREBP1 may participate in the resolution of inflammation.
In contrast with M1 macrophages, TAG uptake and FA release through lysosomal hydrolysis and oxidation appear to be essential for M2 macrophages (73, 74) (Figure 2). There is some controversy regarding the importance of FAO for M2 polarization. The first suggestion in support of this idea was a study using rather high doses of the CPT1 inhibitor etomoxir (≥ 50 µM) (75). Subsequent studies in human and mouse macrophages failed to confirm an effect of etomoxir on IL4 responses (33, 37, 76). The controversy was resolved by a study demonstrating that low concentrations of etomoxir (≤ 3 µM), while effectively inhibiting CPT-1, did not influence IL4 polarization (77). Moreover, high doses of etomoxir (>100 µM) deplete free intracellular CoA and IL4 driven polarization, even in the absence of CPT-1 or CPT-2. The effect is rescued by exogenous CoA treatment (77). While this study demonstrates that CPT-1 is dispensable for macrophage polarization to M2 and highlights the importance of CoA availability, future research using genetic approaches should shed more light on the importance of FAO in M2 polarization (36).
Another distinctive feature of IL4-treated macrophages is the up-regulation of CD36 by PPARγ, a signaling receptor and FA transporter that participates in macrophage alternative activation (73). CD36 plays a role in the uptake of LDL and VLDL particles, whose TAG molecules are metabolized by lysosomal acid lipases to produce free FA. These FA are sent to the mitochondria for β–oxidation, thereby enhancing OXPHOS in the M2 macrophages. In this way, CD36 influences the expression of M2 markers during IL-4 responses (73).
Interestingly, given that CD36 has multiple ligands, the receptor may support both anti- or pro-inflammatory macrophage activation depending on the environment, the ligands and the coreceptors used for signaling. For example, it has been described that in atherosclerosis, oxidized low-density lipoproteins (oxLDLs) in the intima of the arteries bind to CD36 with high affinity, activating signaling cascades that help during lipoprotein internalization and foam cell formation (78, 79). oxLDLs induce the expression of FA transport proteins (FABP4, ACSL1, CPT1, CPT2) that sequentially carry exogenous FA into the mitochondrial matrix. Contrary to the alternative activation, the oxLDL/CD36 axis reduces OXPHOS and switches metabolism to glycolysis, causing increased accumulation of free FA in the mitochondria and a parallel mitochondrial ROS production. Mitochondrial ROS activates the NF-κB pathway and promotes pro-inflammatory macrophage activation (80, 81). In this scenario, CD36 also participates in the activation of the inflammasome and IL1β production by facilitating the accumulation of cholesterol crystals that promote lysosomal disruption (82).
Microbiota-derived short-chain fatty acids (SCFAs), including acetate, propionate, and butyrate, have garnered significant attention for their impact on macrophage physiology. These metabolites, produced during the fermentation of dietary fibers by gut microbiota, play crucial roles in regulating immune responses and inflammation. For instance, butyrate has been shown to reduce the development of IFNγ generating cells while promoting the development of regulatory T cells, thereby modulating immune responses (83). Furthermore, SCFAs influence macrophage functions by promoting phagosome-lysosome fusion, increasing reactive oxygen species production, and balancing cytokine responses. These effects are particularly relevant in the context of pulmonary fungal infections, where SCFAs have been explored for their therapeutic potential (84). Additionally, SCFAs have been linked to a decrease in allergic inflammation in asthma, highlighting their role in modulating immune responses (85–87).
2.3 Oxylipins regulate macrophage polarization
The oxylipins, including the AA-derived eicosanoids, are crucial endogenous mediators in physiological and pathological processes, synthesized by different cells but especially inflammatory cells. They are generated from PUFAs by various enzymatic pathways and include the prostaglandins (PG) and thromboxane (TX) (COX pathway), leukotrienes (LTs), lipoxins (LX) and hydroxyeicosatetraenoic acids (HETEs) (LOX pathway), and epoxyeicosatrienoic acids (ETEs) (via cytochrome P450). A fourth pathway, nonenzymatic, generates the isoprostanes (88, 89) (Figure 3).
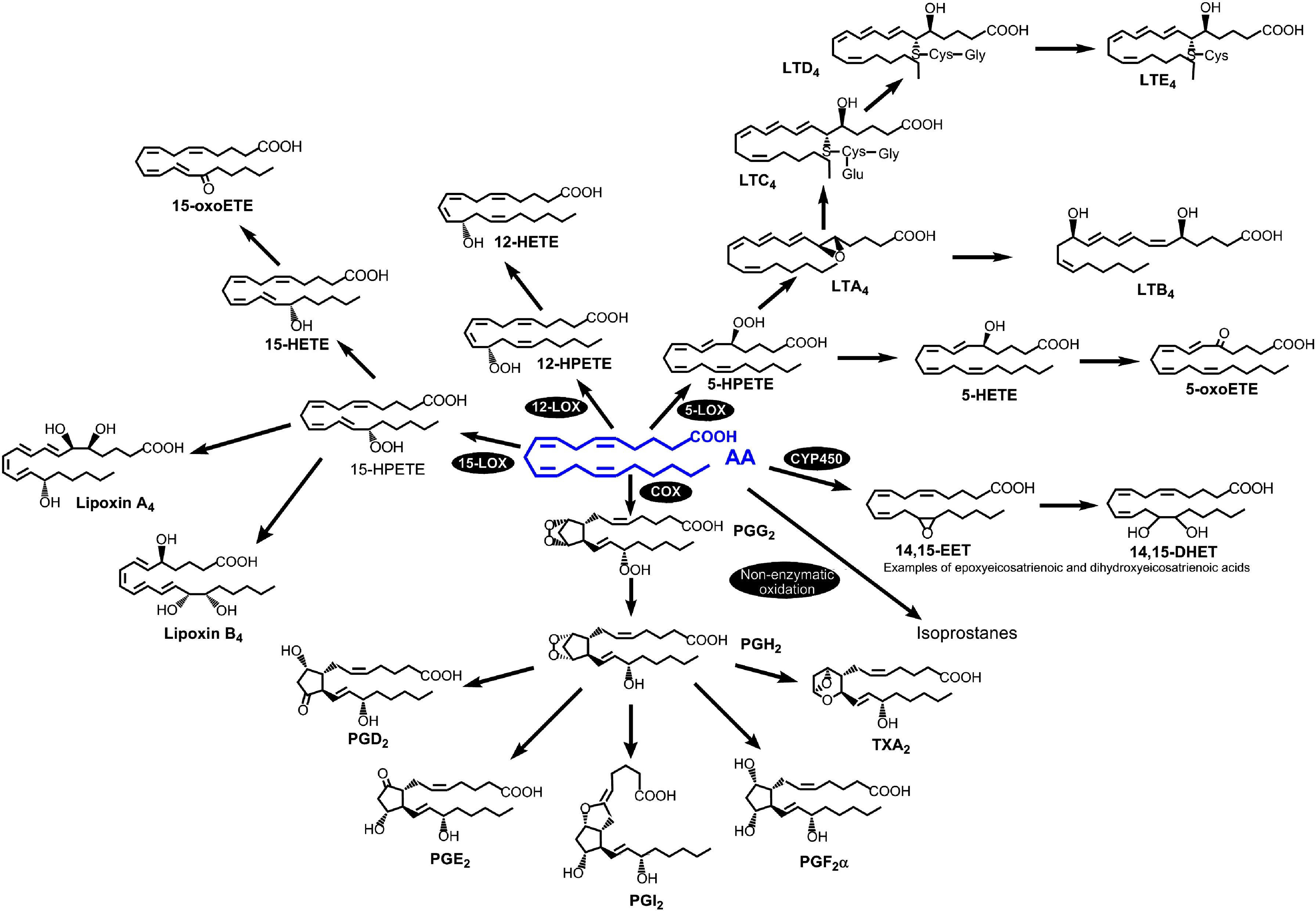
Figure 3. The AA-derived eicosanoid family. The four pathways for AA metabolism into eicosanoids are as follows: cyclooxygenase, lipoxygenase, cytochrome-P450 and non-enzymatic oxidation reactions. Reprinted with permission from ref. 88.
It is widely known that the eicosanoids play a key role in inflammation, and are linked to cardiovascular diseases, metabolic syndrome, and cancer (89, 90). Thus, the identification of eicosanoid biomarkers and/or therapeutic targets are prominent areas of research. Even before the introduction of the concept of macrophage polarization, different laboratories showed how proinflammatory stimuli induce the synthesis or repression of enzymes that mediate eicosanoid metabolism, and produce specific profiles of eicosanoids (91). Gupta and co-workers (92) generated a comprehensive quantitative lipidomic data set of eicosanoids produced upon stimulation with the TLR4 ligand, Kdo2 lipid A (KLA) in RAW264 macrophage-like cells. Subsequently, Norris et al. (93) compared quantitative temporal differences in eicosanoid levels with the expression of enzymatic transcripts after TLR4 stimulation with KLA in four different types of macrophages (resident or thioglycollate-elicited peritoneal macrophages, bone marrow-derived macrophages, and RAW246.7 macrophage-like cells). All macrophage phenotypes produced primarily COX metabolites, with notable variations in PGI2 synthase expression and PGI2 production among them (93). Studies in human macrophages under multiple polarization conditions confirmed that the COX products PGE2 and PGD2 are the major metabolites produced under all polarization states; however, no changes were detected in the levels of these metabolites among the different conditions (94). Strikingly however, M1 macrophages displayed a remarkable synthesis of TXB2 which was not found in the other polarization phenotypes (94).
From another perspective, Lukic and colleagues (95) analyzed inflammatory lipid mediator profiles in macrophage phenotypes before full activation, using GM-CSF or M-CSF to prime cells towards M1 or M2 phenotypes. They considered this scenario because this is likely reflecting the state of the cell when pathogens are encountered. In resting conditions, both phenotypes released pro-resolving lipid mediators. Upon bacterial stimulation, M-CSF macrophages shifted towards proinflammatory eicosanoids, including increased 5-LOX products. However, GM-CSF cells exhibited higher 5-LOX expression, forming consequently high amounts of 5-HETE. This suggests pre-existing pathway preferences before full M1/M2 activation that influence the inflammatory response (95). Also working with pathogens, Werz and colleagues showed that human macrophages respond uniquely to bacteria (96). M1 macrophages, activated by E. coli and S. aureus, predominantly produce proinflammatory eicosanoids such as LTB4 and PGE2. In contrast, M2 macrophages respond to these pathogens by generating specialized pro-resolving mediators. This distinction in responses underscores the diverse roles of M1 and M2 macrophages in inflammatory or pro-resolving contexts during infections (96).
More recently, another study determined eicosanoid formation during macrophage polarization, and its subsequent role in inflammation. Cui and co-workers (97) developed an acute inflammatory model in mice after LPS inoculation and studied the inflammatory cytokine secretion (TNFα and IL6) along with the phenotype changes of peritoneal macrophages over time. They observed an increase in eicosanoids from the COX pathway and a decrease in products from the LOX pathway in the macrophages after LPS administration. To further clarify the relationship between macrophage polarization and eicosanoid metabolism, they differentiated THP-1 cells into M1 and M2 phenotypes in vitro and analyzed their eicosanoid content. The results showed that M1 cells utilized AA in preference, while M2 cells preferred EPA as a substrate, suggesting a potential mechanism for the anti-inflammatory properties of M2 macrophages (97).
2.4 Phospholipase A2s and acyltransferases modulate lipid signaling and macrophage function
Phospholipases are the enzymes responsible for cleaving ester bonds within phospholipids. Their hydrolytic activity produces a variety of lipid products that play crucial roles in cellular signaling. Among the phospholipase superfamily, phospholipase A2s (PLA2s) are particularly important, as these are the enzymes that generate the free polyunsaturated fatty acids that serve as precursors for oxylipin biosynthesis (88, 98, 99). The PLA2 enzymes were initially classified into groups according to structural and sequence criteria. At the time of this writing, 16 groups have been described, with many of them including several subgroups (100, 101). However there is an alternative classification, frequently used, that categorizes the PLA2s on the basis of biochemical and functional similarities (100, 101). According to this classification, PLA2s are grouped into 6 major families, namely: (i) the sPLA2s or secreted enzymes; (ii) the cPLA2s, or calcium-dependent cytosolic enzymes; (iii) the iPLA2s, or calcium-independent enzymes; (iv) the platelet-activating factor acetyl hydrolases (PAF-AH); (v) the L-PLA2 or lysosomal enzymes and (vi) the adPLA2 or adipose tissue-specific enzymes (Figure 4).
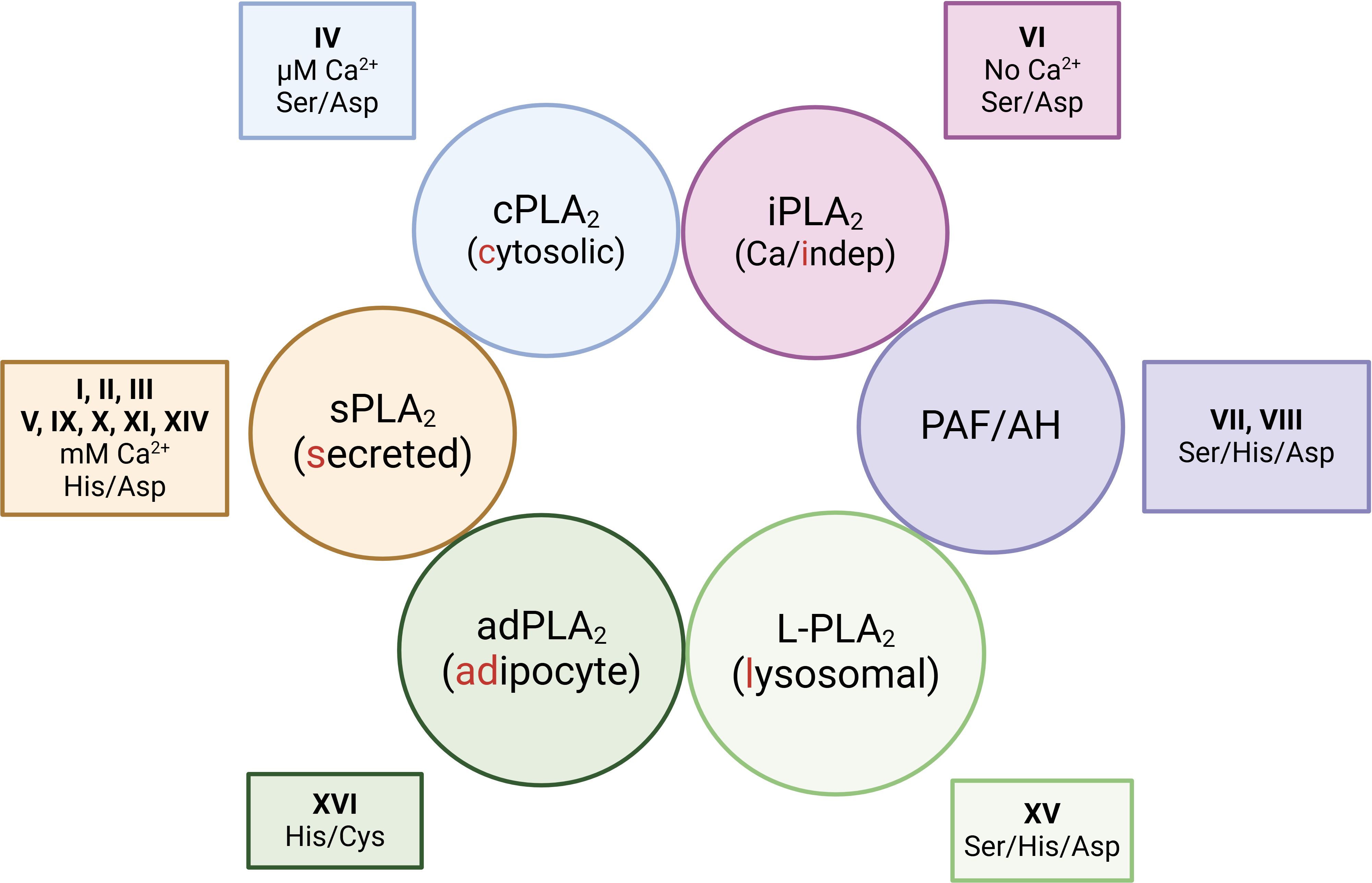
Figure 4. Functional classification of phospholipase A2s. The enzymes are grouped into 6 major families depending on biochemical commonalities i.e. whether they are secreted or reside in specific subcellular compartments; and whether they need Ca2+ for activity. The figure also includes the group numbers that each family belongs to, and the catalytic amino acid diads/triads of each family.
Since PLA2s usually display cellular expression specificities and defined substrate preferences, it has been long speculated that differentially polarized macrophages could exhibit select PLA2 profiles and, therefore, generate specific lipidomic signatures for each polarized activation state. Particularly important in this regard would be the expression profiles of the group IVA calcium-dependent cytosolic phospholipase A2 (cPLA2α), and the sPLA2s belonging to groups IIA, V, and X, as these are the enzymes most commonly implicated in regulating AA mobilization and attendant eicosanoid biosynthesis (100–103). Of note, in addition to regulating lipid signaling, cPLA2α is also critical to sustain another major macrophage function, i.e. phagocytosis. This enzyme is well documented to translocate to the phagosome during phagocytosis (104–106). cPLA2α seems essential for the cell to effectively internalize the ingested particles through mechanisms involving both its catalytic activity and the specific interaction of its C2 and polyphosphoinositide-binding domains with the phagosome membrane (107–109) (Figure 5).
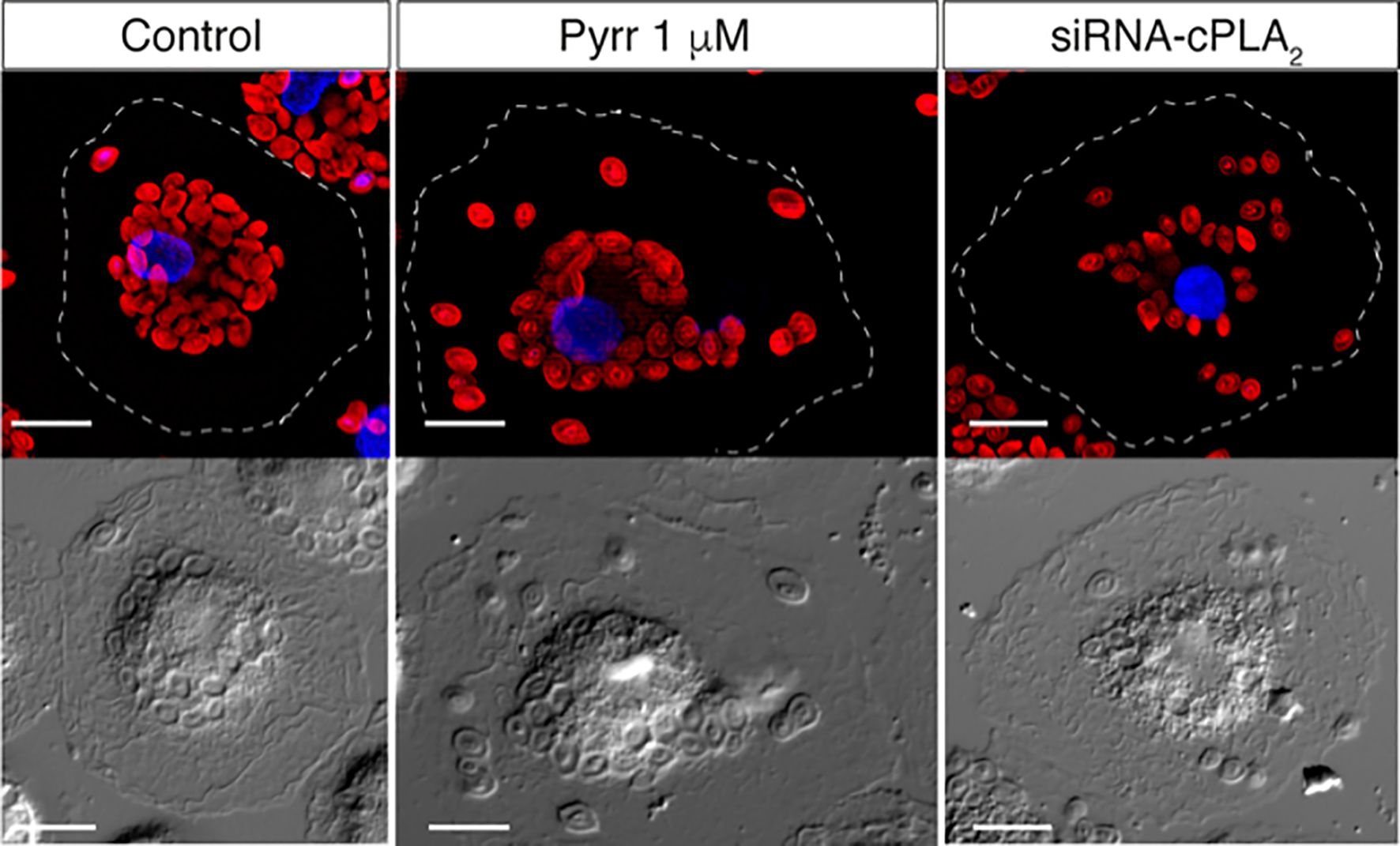
Figure 5. cPLA2α regulates phagosome internalization. Group IVA cytosolic phospholipase A2α (cPLA2α), the major enzyme controlling AA mobilization for production of eicosanoids during phagocytosis, is known to translocate to the phagosome membrane. The figure shows the internalization of IgG-opsonized Alexa Fluor594-conjugated zymosan particles by human monocyte-derived macrophages, as analyzed by epifluorescence microscopy. In control untreated cells, the particles are internalized deep inside the cell, concentrating in close proximity to the nucleus. In contrast, in cells treated with the inhibitor pyrrophenone (Pyrr) or cells depleted of the enzyme by specific siRNA silencing (siRNA-cPLA2α), a more disperse internalization pattern is observed, with a significant number of particles dispersed all over the cytoplasm, far away from the nucleus. Thus, cPLA2α actively modulates the internalization of the IgG-opsonized particles. Magnification, 10 µm. For further details, see refs. 106 and 107.
In studies with murine peritoneal and bone marrow-derived macrophages, Ashley and co-workers assessed the expression of a number of PLA2 enzymes, namely cPLA2α and the calcium-independent enzymes iPLA2β (group VIA PLA2) and iPLA2γ (group VIC PLA2) (110). Of these, only cPLA2α was found to slightly increase upon activation with either LPS plus IFNγ (M1 conditions) or IL4 (M2 conditions). Interestingly however, analyses of macrophages from iPLA2β–/– k.o. mice suggested the involvement of this enzyme, not cPLA2α, in macrophage polarization. Stimulation of iPLA2β-deficient macrophages with IL4 resulted in the increased expression of M2 markers, as compared with macrophages from wild type animals. These findings suggested that the absence of iPLA2β drives the macrophages to an M2 phenotype, while its presence favors an M1 phenotype (110). Further studies from the same group concluded that iPLA2β also participates in the regulation of the production of pro-inflammarory oxylipins by the macrophages (111).
In contrast with the above data, Klement and co-workers (112) reported that persistent treatment of mice with LPS markedly increases the mRNA expression level of pla2g4a, the gene coding for cPLA2α. Moreover, a trend and significant further upregulation of pla2g4a was observed in male and female iPLA2β–/– k.o. mice, respectively, suggesting that the lack of iPLA2β up-regulates cPLA2α, this resulting in elevated production of eicosanoids (112). The same work also showed that specific iPLA2β deficiency in myeloid cells leads to the promotion of adaptive autoimmune and LPS-innate inflammatory responses associated with an activation of MIP-1α/CCL3 (112). Thus the absence of iPLA2β exacerbating inflammation suggests that a major role for iPLA2β in macrophage physiology is to regulate lipid signaling pathways that orchestrate the generation of protective, anti-inflammatory M2-type responses (113). Consistent with this view, iPLA2β has repeatedly been observed to function as the major PLA2 effecting the mobilization of anti-inflammatory FAs such as adrenic acid (114, 115), DHA (116–118), and palmitoleic acid (119, 120). The key role that iPLA2β appears to play in clearing oxidized membrane phospholipids (121, 122), is also in agreement with an anti-inflammatory role for this enzyme in macrophages.
Among the PLA2 family of enzymes, the secreted PLA2s (sPLA2) constitute the largest class, with at least 11 different enzymes being expressed by mammalian cells. In particular, sPLA2 enzymes are abundantly present in innate immune cells, where they play specific roles in regulating a wide variety of innate immune functions, ranging from the production of bioactive oxylipins to membrane rearrangement or the degradation of the membrane of invading pathogens or extracellular vesicles (123). Among the sPLA2s, the group V enzyme (sPLA2-V) represents a very interesting case because of its bi-faceted role in immunity (103, 124, 125), i.e. the enzyme may play both pro- and anti-inflammatory roles depending on conditions, cell type, and species. This enzyme hydrolyzes phospholipid-bound oleic and linoleic acid residues in preference to AA residues (126, 127), suggesting varied roles for this enzyme in addition to or independent of its role in the eicosanoid metabolic cascade (123, 128). As regards to the latter, studies utilizing sPLA2-V-deficient mice have conclusively demonstrated the involvement of this enzyme in regulating AA mobilization and the attendant eicosanoid generation of macrophages during phagocytosis of zymosan particles (129). Subsequent studies demonstrated as well the involvement of sPLA2-V on stimulation of the macrophages via TLR1/2, TLR2, TLR3, TLR4, TLR6/2 and TLR7, but not TLR5 or TLR9 (130–132). It is interesting to note in this regard that clear variations in the lipidome of macrophages stimulated via different receptors have been described (133). This suggests that, depending on the receptor involved, different phospholipases may be implicated in shaping the cellular lipidome and thus in guiding the macrophages to their effector functions. During TLR4 stimulation, sPLA2-V is thought to amplify the action of cPLA2α, which is the key enzyme in effecting the release of AA (134). While the molecular details governing cross-talk between the two PLA2s remain to be fully clarified, it has been shown that sPLA2-V regulates cPLA2α phosphorylation (132, 135). Alternatively, sPLA2-V-catalyzed phospholipid hydrolysis may produce lipid metabolite(s) that directly regulate(s) the activity of the cPLA2α, or vice versa (136–138).
The involvement of sPLA2-V in regulating the production of AA-derived proinflammatory lipid mediators suggests detrimental roles for this enzyme under certain circumstances. However, comprehensive analyses of PLA2 expression in macrophages from different types and species has revealed that sPLA2-V is very strongly induced by stimuli that promote polarization to an anti-inflammatory M2 phenotype, including IL4, IL13, IL10, and M-CSF (139–141). Increased sPLA2-V expression in M2 polarized human macrophages correlates with the greater phagocytic capacity that these cells exhibit, suggesting that sPLA2-V-regulated lipid changes may be crucial for the execution of this response (141–143). In keeping with this view, lipidomic analyses of human macrophages identified the ethanolamine lysoplasmalogens as sPLA2-V-derived metabolites that effectively restore phagocytosis in sPLA2-V-deficient cells (141, 144). More recent work using bone marrow-derived macrophages from sPLA2-V deficient mice, confirmed the essential role that this enzyme plays in phagocytosis and in the activation of select lipid pathways during polarization towards an M2 phenotype, in particular those involving the hydrolysis of ethanolamine phospholipids (125). Importantly, the latter study also confirmed the role of sPLA2-V in regulating AA mobilization and eicosanoid production regardless of the polarization activation regime, thus stressing again the bi-faceted role of sPLA2-V, modulating both pro- and anti-inflammatory actions. Under both polarization regimes, PGE2 and PGD2, were reduced in macrophages lacking sPLA2-V (125).
PUFAs are not typically incorporated into phospholipids during de novo biosynthesis in the endoplasmic reticulum; instead, they are integrated at a later stage via a deacylation/reacylation cycle known as the Lands cycle (145–148). In this cycle, PLA2 enzymes, particularly the calcium-independent ones (149–151), cleave existing phospholipids to generate 2-lysophospholipids, which are then rapidly reacylated with PUFAs by CoA-dependent lysophospholipid acyltransferases (145–148). These latter enzymes thus play a crucial role in lipid remodeling and the generation of bioactive lipids. By modulating the composition of cellular membranes, the CoA-dependent acyltransferases influence various cellular functions, including membrane fluidity, curvature, and signaling pathways. In macrophages and other innate immune cells, these enzymes are crucial for maintaining lipid homeostasis and facilitating appropriate responses to environmental stimuli (152–154). The activity and expression of specific acyltransferases have significant implications for macrophage biology. For instance, lysophosphatidylcholine acyltransferase 2 (LPCAT2) is known to co-localize with cyclooxygenase-2 (COX-2) in lipid droplets within macrophages (155). This association suggests a role for LPCAT2 in the production of inflammatory mediators, as lipid droplets are described as sites for the synthesis of eicosanoids (156). Moreover, LPCAT2 is required for macrophage cytokine gene expression and release in response to TLR4 and TLR2 ligand stimulation, but not for TLR-independent stimuli, indicating its selective involvement in pathogen-induced inflammatory responses (157). Additionally, the regulation of lysophospholipid levels by acyltransferases can impact macrophage activation states. Elevated levels of lysophospholipids, such as lysophosphatidylcholine (LPC), have been associated with pro-inflammatory macrophage activation (158, 159). By controlling the reacylation of LPC, enzymes like LPCAT3 help modulate the inflammatory status of innate immune cells (154). Characterization of human LPCAT3 has shown its robust activity in esterifying lysophosphatidylcholine with PUFA, especially AA (160, 161), thereby limiting the abundance of bioactive lysophospholipids that can act as signaling molecules in inflammation.
2.5 Glycerophospholipids and sphingolipids in the dynamics of macrophage polarization
In addition to liberating free FAs, PLA2 activation leads to the production of lysophospholipids, many of which are biologically active (162). Lysophospholipids play a crucial role in macrophage biology, influencing various aspects of immune function, inflammation, and cellular signaling. Macrophages utilize these lipids to regulate their activation states, migration, and cytokine production in response to infections or tissue injury. Through interactions with specific G-protein-coupled receptors lysophospholipids modulate intracellular signaling cascades that influence pro-inflammatory or anti-inflammatory responses depending on the context (163, 164). As indicated in the preceding section, ethanolamine lysoplasmalogens appear to play a crucial role in regulating the elevated phagocytic response exhibited by M2 macrophages (141, 144). LPC has been implicated in promoting inflammatory responses by stimulating TLR pathways and, as a consequence, inducing COX-2 and the production of cytokines such as TNFα and IL6 (131, 165). On the other hand, lysophosphatidic acid signaling can enhance macrophage survival and migration, facilitating tissue remodeling and resolution of inflammation (163). These differential effects highlight the importance of lysophospholipid metabolism in fine-tuning immune responses during infections, autoimmune diseases, and tissue repair. Additionally, lysophospholipids may contribute to macrophage-driven pathologies, such as atherosclerosis and chronic inflammatory diseases. Oxidized phospholipids, including oxidized LPC, are known to accumulate in atherosclerotic plaques and modulate macrophage foam cell formation, promoting disease progression (159, 166).
During pro-inflammatory activation of macrophages, the levels of many glycerophospholipid species show significant increases (133, 167, 168). Dennis and colleagues first conducted analyses of lipid molecular species using quantitative mass spectrometry to delineate the macrophage lipidome following stimulation of RAW264.7 macrophage-like cells with Kdo2-lipid A, the active component of LPS (167). The data stressed that activation of the innate immune system by inflammatory mediators leads to alterations across a wide range of mammalian lipid categories (167). In general agreement with these data, Lee and collaborators (168) also characterized the lipid profile of RAW264.7 cells stimulated with different concentrations of LPS. This work showed that 11 classes of lipids, including TAG, DAG, ChE, PE, PS, PI, PA, LPC, LysoPE, Cer, and dCer, were increased, and that three classes, cholesterol, PC, and LysoPA, were decreased in an LPS concentration-dependent manner. Similar studies utilizing human macrophages also demonstrated profound changes in the cellular phospholipidome on stimulation with innate immune stimuli (94). In these studies, a lipidomic analysis of 4 macrophage phenotypes (M1, M2a, M2c and controls) was performed, and a principal component analysis revealed substantial changes in the lipid profile of all activation states. M1 macrophages were the furthest apart in PC1, while both M2 groups were distinct from controls and M1, yet closely clustered in PC1 and PC2. However, in PC3, substantial differences between M2a and M2c were recognized. This study also reported the phospholipid composition of each macrophage phenotype, finding interesting variations in each phospholipid class. Species such as PE(34:1), PI(34:2) and PI(38:5) showed slight increases (differentiated cells compared to controls) in contrast to PE(38:4) or PI(38:4), which decreased (94). Decreases in these latter species along with other species such as PC(36:4), PC(38:4) and PC(40:5) have also been documented in other studies, and are indicative of stimulus-induced AA mobilization (169–173). However, in other cases, the phospholipid alterations appear to be driven by an upregulation of de novo pathways for lipid synthesis. In support of this, the removal of key enzymes involved in this process, such as acetyl-CoA carboxylase, attenuates glycerophospholipid enrichment (66). Additionally, the levels of glycerolipid synthesis intermediates—particularly saturated and monounsaturated species of phosphatidic acid and its derivative diacylglycerol—increase during TLR4 activation (167), reinforcing further the role of the de novo synthesis in this context.
Subcellular lipidomic analyses have suggested that, during activation of macrophages via TLR4, glycerophospholipids changes occur not only in the plasma membrane but also in intracellular membranes (Figure 6). After separation of different cellular fractions by centrifugation, Andreyev and co-workers (174) noted that the nuclear and mitochondrial fractions increase their enrichment in PC, PE, and PS; the endoplasmic reticulum fraction increases its content of PC and PA, and the plasma membrane fraction increases its content of PE while decreasing PC and PS. Interestingly, species of PI primarily increase in the nuclear fraction. Therefore, the nucleus and mitochondria are the organelles that show the greatest increase in glycerolipid content during the pro-inflammatory activation of macrophages (174). These changes are likely related with the finding that specific intracellular sites exist for the different signal-activated phospholipases to mediate phospholipid turnover and signaling (99, 175, 176).
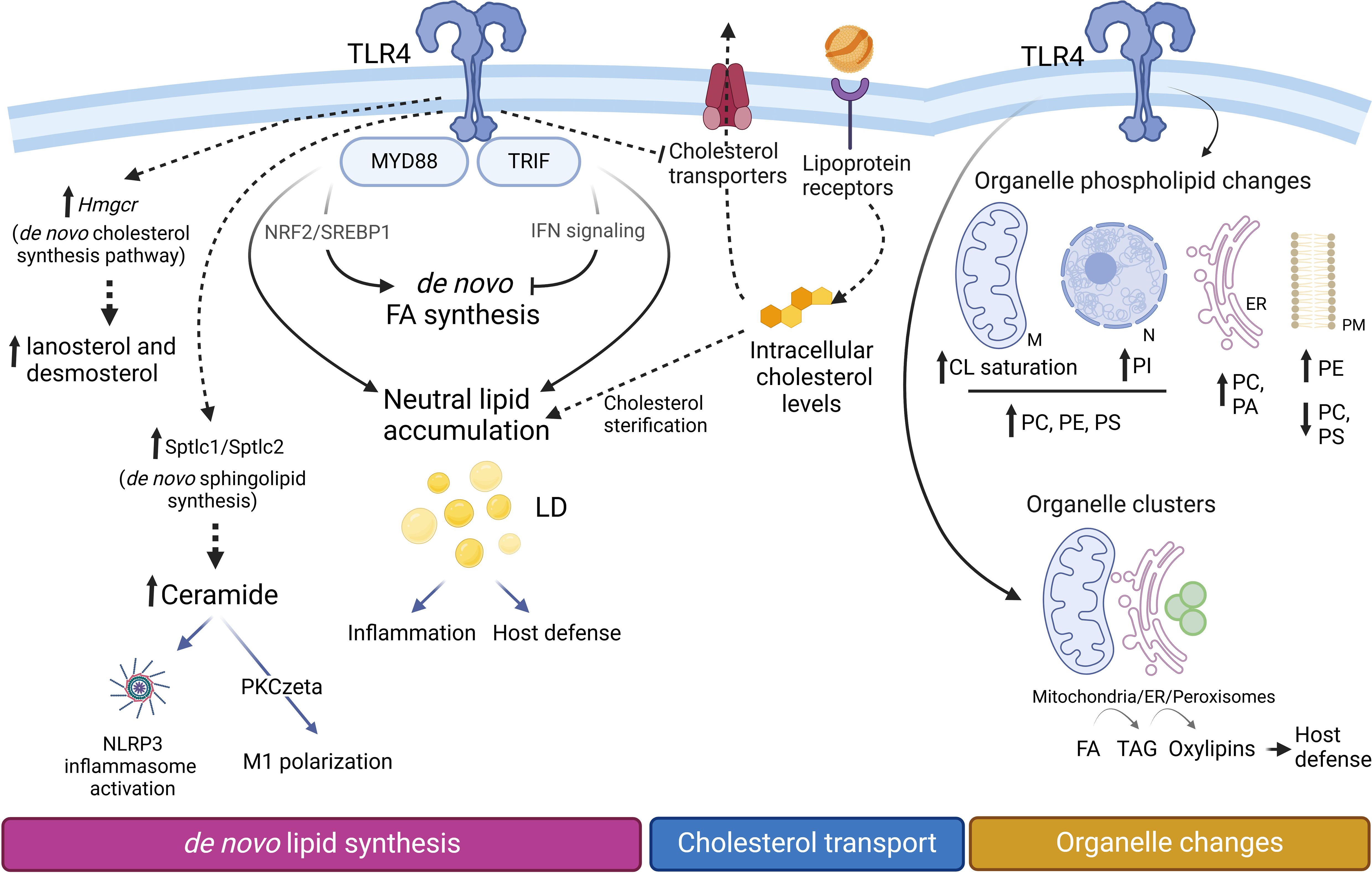
Figure 6. Lipid changes during M1 polarization. Proinflammatory macrophages accumulate triacylglycerol (TAG) and cholesterol esters (CE) within lipid droplets (LD), increasing their number and size. TAG synthesis, critical for LD formation, supports inflammation by providing precursors for lipid mediators and contributes to host defense mechanisms. Upregulation of Hmgcr facilitates the production of cholesterol biosynthetic intermediates such as lanosterol and desmosterol. However, cellular levels of cholesterol and CE are primarily regulated through lipoprotein internalization and alterations in the expression of cholesterol transporters. Increased expression levels of Sptlc1/Sptlc2 promote an elevation of de novo sphingolipid synthesis. The glycerophospholipid composition of cellular organelles undergoes significant changes. In mitochondria, cardiolipins (CL) become more saturated, while PC, PE, and PS increase in both mitochondria (M) and the nucleus (N). The endoplasmic reticulum (ER) becomes enriched in phosphatidic acid (PA), whereas the plasma membrane (PM) experiences an increase in PE and a concomitant decrease in PC and PS levels. Organelle clustering facilitates the biosynthetic flow of FA (from mitochondria) to TAG (in the ER) and oxylipins (in peroxisomes), enabling coordinated lipid metabolism and inflammatory responses.
Cardiolipins are glycerophospholipids that reside specifically in the mitochondrial inner membrane. These complex lipids, containing four FAs, undergo extensive turnover after macrophage activation, leading to cardiolipin species with fewer unsaturations and shorter chains (174). Due to the important role of these lipids in the maintenance of cristae morphology and stability and function of ETC complexes, cardiolipin remodeling is suggested to participate in the modulation of respiratory chain function during inflammatory reprograming of macrophages (177, 178).
Sphingolipids are crucial regulators of macrophage function, particularly during inflammatory responses and polarization. Molecules such as sphingosine and ceramide and their phosphorylated derivatives were originally described as having opposing roles. Phosphorylated sphingolipids, including ceramide-1-phosphate and sphingosine-1-phosphate, support cell survival by promoting proliferation, inflammation, and motility. Conversely, their non-phosphorylated counterparts, ceramide and sphingosine, induce apoptosis, cell cycle arrest and senescence, collectively forming the “sphingolipid rheostat” (179). Similarly, ceramide and sphingosine-1-phosphate exhibit distinct roles in macrophage polarization (180). Ceramide drives pro-inflammatory M1 polarization by activating pathways such as NLRP3, leading to the production of IL1β (181). For example, C2-ceramide reprograms tumor-associated macrophages toward an M1 phenotype through protein kinase Cζ-dependent mechanisms, enhancing pro-inflammatory cytokine secretion and improving CD8+ T cell cytotoxicity within the tumor microenvironment. These findings underscore the contribution of ceramide to antitumor immunity (182, 183). In contrast, the influence of sphingosine 1-phosphate on macrophage functionality is more nuanced. It signals through five G protein-coupled receptors (S1PR1–S1PR5), each linked to distinct pathways. Depending on the receptor involved and the microenvironment, sphingosine 1-phosphate can promote either pro-inflammatory or anti-inflammatory responses, emphasizing its dual role in immune regulation (184, 185).
Macrophage activation through TLR4 also leads to acute alterations in sphingomyelin levels via sphingomyelin synthase (186, 187). At longer times, an increase in palmitoyl-CoA and the expression of serine palmitoyltransferase, the rate-limiting enzyme in sphingolipid synthesis, drives the de novo production of sphingolipids (167). This synthesis contributes to the lipid remodeling that facilitates adaptation of the macrophages to their microenvironment and phenotypic shifts. Hence, targeting these pathways may offer promising therapeutic potential to redirect macrophage polarization, either to mitigate chronic inflammatory diseases or to bolster antitumor immunity.
2.6 Neutral lipids: Triacylglycerol and cholesterol esters
Triacylglycerol (TAG), an energy-storing neutral lipid, significantly increases its levels under proinflammatory conditions, and plays important roles in regulating inflammation (133, 167, 188). This lipid, along with cholesterol esters, is stored in lipid droplets, resulting in a notable increase in both the number and size of these organelles in proinflammatory macrophages (Figure 6) (133, 188, 189). Interestingly, the inhibition of TAG synthesis during LPS activation appears to prevent not only lipid droplet development but also the production of inflammatory mediators (188). Although the primary function of lipid droplets has traditionally been described as energy storage for ATP production via mitochondrial FAO, they are now recognized as hubs for antibacterial protein accumulation and reservoirs of precursors for proinflammatory lipids (188–190). Thus increased TAG synthesis and its accumulation in lipid droplets may support host defense (191), but it can also exacerbate atherosclerosis development during infections and inflammatory diseases (192).
Recent studies using a multi-spectral organelle imaging approach that enables the simultaneous visualization and behavioral analysis of several organelles, have shown that during M1 polarization, a flux of FA exists within clusters of endoplasmic reticulum and mitochondria to supply the necessary FA for lipid droplet growth. The subsequent recruitment of peroxisomes to these clusters supports the hydrolysis of TAG to release PUFA which may putatively mediate lipid signaling via oxylipin formation (190). The molecular mechanism for TAG hydrolysis and effector(s) involved in FA release under these conditions remain to be clarified, as cPLA2α, an enzyme that does not hydrolyze TAG, is well established as the essential enzyme for eicosanoid formation in major immunoinflammatory cells such as macrophages and mast cells (193–196). Conversely, cPLA2α is known to regulate lipid droplet formation in human phagocytes (197, 198).
Other studies, using a combination of shotgun lipidomics and stable-isotope tracing, revealed how macrophages change their neutral lipid profile when activated through TLRs and also during polarization to M1 (LPS plus IFNγ) (133). Macrophages activated only through TLR4 or activated with LPS plus IFNγ accumulate lipids differently. Combining LPS with IFNγ enhances the reprograming of the lipidome promoted by LPS alone, further increasing the accumulation of cholesterol esters and TAG. Although both MyD88 and TRIF adaptor proteins contribute to neutral lipid accumulation during TLR4 activation, they have opposing effects on de novo FA synthesis. MyD88 signaling promotes de novo synthesis of long chain FA, particularly monounsaturated FA such as oleic acid, which depends on NRF2 and SREBP1 transcriptional activities. In contrast, TRIF signaling, which is involved in the upregulation of IFN, opposes MyD88 in FA synthesis through autocrine type I interferon signaling. However, both MyD88 and TRIF participate in the accumulation of neutral lipids during TLR4 activation, which possibly reflects different roles in lipid uptake and traffic (133, 199).
Proinflammatory activation of macrophages leads to elevated levels of intermediates of the de novo synthesis of cholesterol, including lanosterol and desmosterol. Lanosterol accumulation attenuates the activation of STAT1-STAT2 signaling pathways, resulting in decreased secretion of proinflammatory cytokines (200). Desmosterol accumulation suppresses inflammasome activation and modulates inflammatory responses in macrophages through pathways independent of LXR activation, highlighting the complexity of cholesterol metabolism in immune regulation (201). Likewise, other oxysterols such as 25-hydroxycholesterol and 24,25-epoxy-cholesterol are also elevated (167, 200, 202). These oxysterols influence macrophage polarization primarily through the activation of LXRs. When activated by oxysterols, LXRs promote the expression of genes responsible for cholesterol efflux and anti-inflammatory responses, thereby facilitating the polarization of macrophages toward an anti-inflammatory phenotype (203). In addition, these increases are accompanied by a significant upregulation of the expression of HMG-CoA reductase (Hmgcr), a limiting step in the pathway, and of cholesterol 25-hydroxylase (Cho25h), which participates in the production of 25-hydroxycholesterol (167). The most pronounced changes involve the up-regulation of sterol-O-acyl transferases -1 and -2, and the accumulation of cholesterol esters. Unexpectedly however, the latter changes appear to be driven by different processes. While the cholesterol biosynthesis pathway contributes to the accumulation of intermediates, the final concentration of cholesterol and its esters seems to be primarily regulated by the uptake of lipoproteins through membrane receptors (Ldlr, Cd36, Msr1, etc.), along with changes in the expression of transporters (Abca1, Abcg1) that export cholesterol (167) (Figure 6).
M2-polarized macrophages exhibit lower levels of both TAG and cholesterol esters compared to M1 macrophages. They also exhibit a markedly different behavior in response to an overload of exogenous free FA. While M1 macrophages primarily accumulate these FA in TAG, M2 macrophages accumulate them in glycerophospholipids and sphingophospholipids (204). This behavior, along with the differences between these two populations regarding the utilization of FA for energy, could explain the disparities in lipotoxicity that M1 and M2 macrophages exhibit in pathophysiological conditions such as obesity (205). Future investigation should shed light into how these different macrophage populations channel de novo fatty acids into different families of complex lipids.
3 Macrophage lipid metabolism in aging: Some considerations
Aging and age-related diseases are linked by fundamental mechanisms that often revolve around inflammation. As we age, a persistent, non-infectious, low-level inflammation state known as “inflammaging” emerges, playing a role in the development of age-related diseases (206–208) (Figure 7). Aging impairs macrophage polarization, leading to dysfunctional phenotypes that do not easily fit into the typical M1 or M2 categories. In general, tissue macrophages become more proinflammatory and less phagocytic with age. The altered tissue macrophages also contribute to organ deterioration in aging, as the decrease in cellular repair processes perpetuates damage at the level of nucleic acids and proteins.
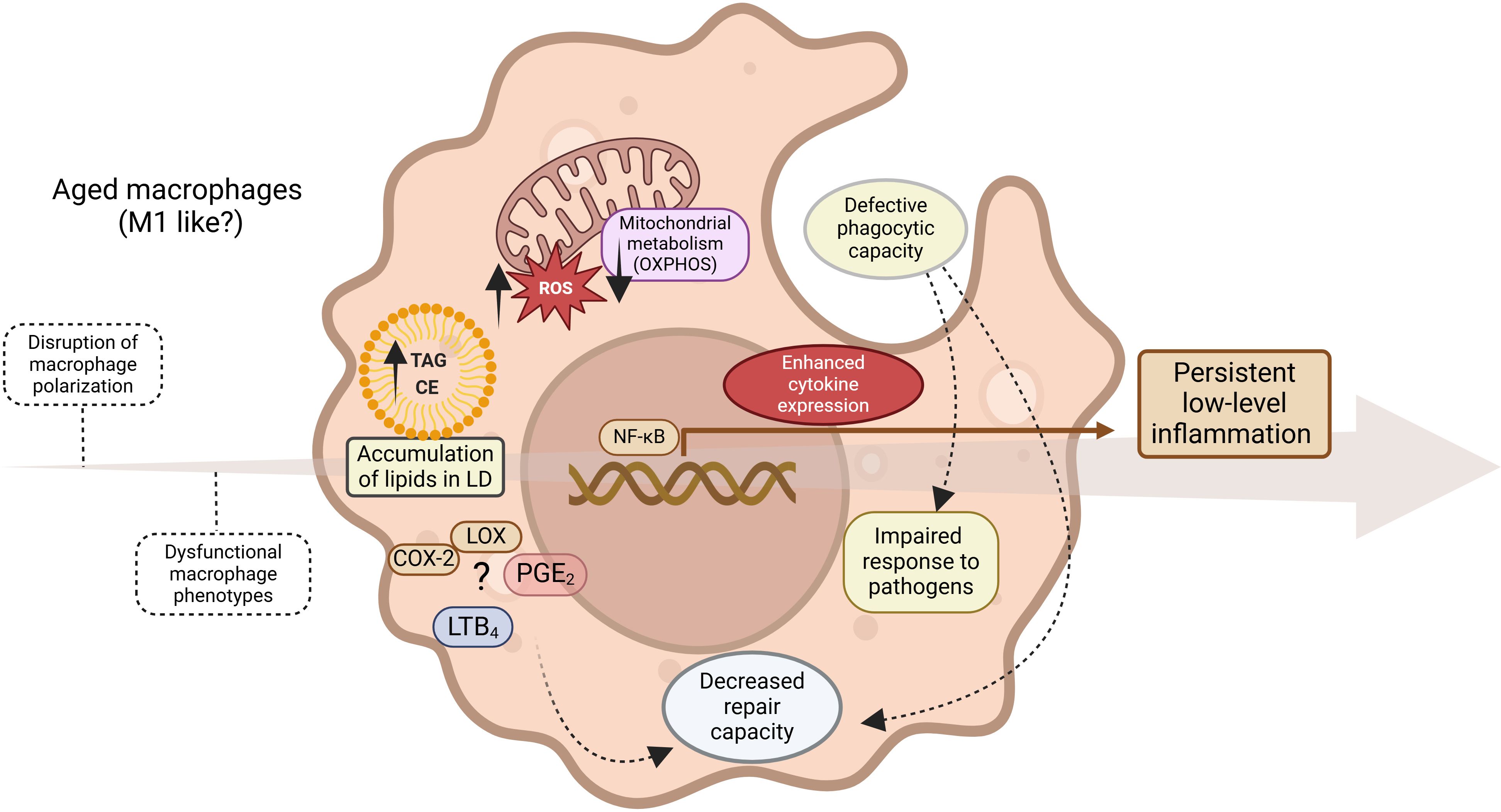
Figure 7. Inflammaging. Progressive evolution (arrow) of persistent non-infectious inflammation driven by multiple events, including dysregulation of lipid metabolism, results in a dysfunctional, predominantly proinflammatory macrophage phenotype with impaired immune functions.
Tissue resident macrophages adapt their metabolism to the microenvironment and the function that they have in each tissue (209, 210). In accordance with this, the changes that macrophages experience during aging are generally related to alterations in their metabolism triggered by local tissue perturbations (211), albeit distal perturbations such as changes in the gut microbiota may also contribute (212). Many tissue resident macrophages, especially alveolar macrophages, large peritoneal macrophages, Langerhans cells, Kupffer cells and splenic red pulp macrophages, are completely dependent on OXPHOS to survive, as deletion of OXPHOS genes reduces their viability (208). In this regard, aged tissue macrophages decrease their mitochondrial metabolism, especially through reduction of OXPHOS activity, increase ROS production and store large amounts of neutral lipids in lipid droplets (213–215).
In the aged brain, microglia (the brain macrophages) display accumulation of lipids in lipid droplets, defective phagocytosis, and increased ROS levels and proinflammatory cytokine production during LPS challenge (215). Lipid droplet-laden microglia display altered expression of multiple genes, including those related with the phagosomal maturation pathway, genes related with the synthesis of FA, and genes related with FA β-oxidation (215). These alterations seem to be dependent on lipid accumulation. Interestingly, lipid droplet-laden microglia displaying a dysfunctional phenotype are present in a mouse model of frontotemporal dementia (215). Other studies have shown that the prostaglandin receptor EP2 on myeloid cells participates in the suppression of bioenergetic metabolism, a process mediated by PGE2. Mechanistically, PGE2 drives the conversion of glucose to glycogen, with a concomitant reduction of glycolysis and OXPHOS and, consequently, ATP production. As a result, there is an increased proinflammatory immune response and cognitive decline of the aged animals. Importantly, inhibition of myeloid or peripheral EP2 prevents the cognitive decline (216). Finally, knock-in mouse models that express the human APOE4 allele, related with the late-onset of Alzheimer disease, have shown that the microglia of aged animals strongly express APOE. When subject to an inflammatory challenge, these cells respond by increasing glycolysis, Hif1a expression and reducing mitochondrial respiration, a phenotype that is characteristic of classically activated macrophages (217).
Recently, Schädel and collaborators used lipidomic profiling to demonstrate that aged peritoneal macrophages produce significantly decreased amounts of pro-inflammatory PGE2, LTB4, and specialized pro-resolving mediators such as maresin-1 and protectin DX (218). These declines were correlated with reduced expression of COX-1 and FLAP (5-lipoxygenase activating protein). This study is significant because it challenges the concept that aging solely increases the pro-inflammatory activation of macrophages (208–210). Rather, it is the disruption of lipid metabolism and signaling as a whole that affects immune functions.
Lipid metabolism is intricately linked to the development of cardiometabolic diseases, including atherosclerosis. As individuals age, dysregulation in lipid homeostasis contributes to the accumulation of lipids, inflammation, and vascular dysfunction, ultimately increasing the risk of a wide range of cardiovascular and metabolic disorders. Type 2 diabetes often coexists with coronary artery disease, contributing to heightened inflammatory infiltrates and enlarged necrotic cores, which worsen atherosclerosis. In this context, Bi and colleagues (219) showed that PGE2 regulates M2 polarization via the cyclic AMP-responsive element binding/brain-derived neurotrophic factor/tyrosine kinase receptor B pathway. Thus, in situ synthesis of PGE2 could be an attractive target to improve treatment strategies. Along the same lines, another significant complication is diabetic cardiomyopathy, characterized by cardiac inflammation and metabolic alteration. During this condition, altered FA metabolism drives macrophage polarization toward the pro-inflammatory M1 phenotype. Sreedhar et al. (220) demonstrated that the 14-3-3η protein plays a pathogenic role, as its silencing increases the expression of FAS and M1 cellular markers, and decreases M2 markers. In turn these data support a potential therapeutic target role for this cardiac protein in diabetic cardiomiopathy (220).
Emerging evidence suggests that lipid metabolism also plays a pivotal role in age-related cancer progression, linking metabolic reprogramming to tumor development and immune dysfunction in aging tissues (221, 222). Tumor-associated macrophages (TAM), natural immune cells abundant in the tumor microenvironment, can be categorized into the anti-tumor M1 subtype and pro-tumor M2 subtype. Given the high plasticity of TAM, the shift from the M1 to M2 phenotype in the hypoxic and hypoglycemic tumor microenvironment accelerates cancer progression, closely tied to lipid metabolism. Since lipid metabolism reprogramming profoundly impacts TAM function, it holds potential as a promising therapeutic target for cancer (Figure 8). Key players of lipid metabolism in TAMs, including PPARγ, PI 3-kinase, and the lipoxygenases, promote the formation of a tumor immunosuppressive microenvironment and facilitate immune escape (222–225). In this sense, Wu and collaborators (226) revealed that the immunosuppressive state of TAMs is modulated by long-chain unsaturated FA metabolism, specifically that of oleic acid. By targeting crucial organelles in myeloid TAM, with chemical inhibitors, TAM polarization was blocked in vitro, and tumor growth was inhibited in vivo (226). Likewise, Goossens et al. (227) characterized TAM in a metastatic ovarian cancer mouse model, revealing that ovarian cancer cells induce membrane-cholesterol efflux and deplete lipid rafts from macrophages. Enhanced cholesterol efflux facilitates IL4-mediated reprogramming, inhibiting IFNγ-induced gene expression. Deleting ABC transporters, which mediate cholesterol efflux, reverses the tumor-promoting functions of TAM, reducing tumor progression. These findings highlight a previously unrecognized role for membrane-cholesterol efflux in driving TAM-mediated tumor progression, suggesting a potential novel anti-tumor therapeutic approach (179). Finally, Prima and colleagues (228) showed that the COX-2/mPGES1/PGE2 pathway plays a role in regulating PD-L1 (programed death ligand-1, CD274) expression in tumor-infiltrating myeloid cells. Consequently, altering PGE2 metabolism in the tumor microenvironment could help reduce immune suppression in the tumor host.
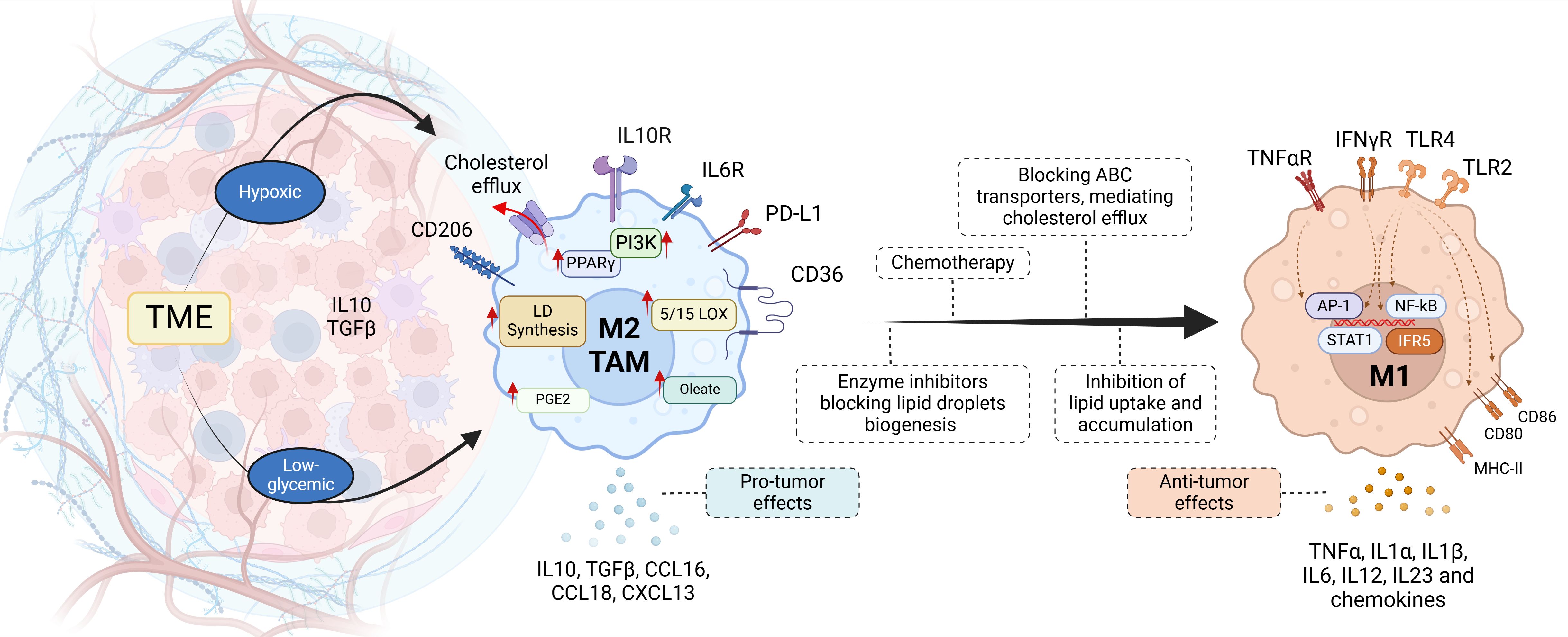
Figure 8. Tumor-associated macrophages. Recent therapeutic strategies focused on lipid metabolism aim to repolarize M2 macrophages in the tumor microenvironment (TME). Chemotherapy induces the production of ROS and the activation of HIF1α, reprogramming TAM metabolism to M1 with the release of pro-inflammatory mediators (cytokines and chemokines). M2 TAMs are characterized by exacerbated lipid synthesis pathways, mediated by the overexpression of PPARγ or PI 3-kinase (PI3K), leading to lipid accumulation. Thus the inhibition of key enzymes that mediate the synthesis of fatty acids, phospholipids, cholesterol, lipid droplets, or cholesterol efflux transporters, could represent therapeutic targets for pharmacological blockade.
4 Conclusions and perspectives
Despite the significant progress in macrophage biology, great challenges remain to precisely define the polarization states of macrophages and identify robust biomarkers beyond the classic ones available today. The characteristic plasticity of these cells implies additional experimental complexity, since they exhibit very diverse phenotypes under normal and pathological conditions. However, this biological characteristic represents at the same time an extraordinary possibility of therapeutic intervention in an enormous number of pathologies. The lipidomic analyses of macrophages stress that, throughout the polarized activation process, several key enzymes involved in lipid biosynthetic pathways are induced, expressed, and activated to different degrees. Therefore, the changes in the levels of the different lipid classes are the consequence of highly orchestrated enzymatic processes, many of which are still not fully characterized or understood. Clearly, further research will be needed to understand how signaling pathways and enzymatic processes coordinate the reshaping of macrophage lipid composition with different phenotypes under different stimuli, which is crucial for their function. To this end, efforts should be made to integrate multi-omics approaches, including transcriptomics, proteomics, and metabolomics, to comprehensively characterize macrophage lipid metabolism within the whole polarization landscape, and identify suitable biomarkers. In this regard, several studies have employed single-cell and spatial transcriptomic techniques, often integrated with lipidomic analyses, to investigate lipid metabolism in relation to macrophage phenotypes. For instance, a study utilizing single-cell RNA sequencing identified distinct macrophage populations with unique lipid metabolism profiles, shedding light on their roles in various physiological and pathological contexts (229). Another research effort combined single-cell sequencing with spatial transcriptomics to explore the tumor microenvironment, revealing that M1 macrophages can suppress lipid metabolism in pancreatic adenocarcinoma cells, thereby affecting tumor progression (230). Additionally, spatial transcriptomics has been applied to examine perivascular macrophages, uncovering alterations in lipid metabolism that influence their function and contribution to disease states (231). These studies collectively demonstrate the utility of advanced transcriptomic and metabolomic approaches in elucidating the complex relationship between lipid metabolism and macrophage phenotypes. These advances will improve our understanding of macrophage biology and facilitate the discovery of targeted therapies for various diseases.
Author contributions
JR: Conceptualization, Writing – original draft, Writing – review & editing. JC: Conceptualization, Writing – original draft, Writing – review & editing. MB: Conceptualization, Funding acquisition, Supervision, Writing – original draft, Writing – review & editing. JB: Conceptualization, Funding acquisition, Project administration, Validation, Writing – original draft, Writing – review & editing.
Funding
The author(s) declare that financial support was received for the research, authorship, and/or publication of this article. The authors declare that financial support was received for the research, authorship, and/or publication of this article. This work was supported by grant PID2022-140764OB-I00, funded by the Spanish Ministry of Science, Innovation, and Universities, Agencia Estatal de Investigación (MICIN/AEI/10.13039/501100011033), and by grant CB07/08/0004 from CIBERDEM-Instituto de Salud Carlos III.
Acknowledgments
Figures were created with BioRender.com.
Conflict of interest
The authors declare that the research was conducted in the absence of any commercial or financial relationships that could be construed as a potential conflict of interest.
Author M.B. declared that she was an Associate Editor of Frontiers in Cell and Developmental Biology at the time of submission. This had no impact on the peer review process and the final decision.
Author J.B. declared that he was an Associate Editor of Frontiers in Cell and Developmental Biology and of Frontiers in Immunology at the time of submission. This had no impact on the peer review process and the final decision.
The author(s) declared that they were an editorial board member of Frontiers, at the time of submission. This had no impact on the peer review process and the final decision.
Generative AI statement
The author(s) declare that no Generative AI was used in the creation of this manuscript.
Publisher’s note
All claims expressed in this article are solely those of the authors and do not necessarily represent those of their affiliated organizations, or those of the publisher, the editors and the reviewers. Any product that may be evaluated in this article, or claim that may be made by its manufacturer, is not guaranteed or endorsed by the publisher.
Glossary
AA: arachidonic acid
ACLY: ATP-citrate lyase
ACC: acetyl-CoA carboxylase
ACSL: acyl-CoA synthetase, long chain
BMDM: bone marrow-derived macrophages
COX: cyclooxygenase
CPT1: carnitine palmitoyltransferase 1
DAG: diacylglycerol
DHA: docosahexaenoic acid
EPA: eicosapentaenoic acid
ETC: electron transport chain
FABP4: fatty acid binding protein 4
FA: fatty acid
FAO: fatty acid β-oxidation
FAS: fatty acid synthase
GM-CSF: granulocyte-macrophage colony-stimulating factor
HMG-CoA: 3-hydroxy-3-methylglutaryl-CoA
IFNγ: interferon γ
IL: interleukin
KLA: Kdo2 lipid A
LDL: low-density lipoprotein
LOX: lipoxygenase
LPC: lysophosphatidylcholine
LPS: lipopolysaccharide
LT: leukotriene
LXR: liver X receptor
M1/M2: macrophage activation phenotypes (pro-inflammatory and anti-inflammatory)
M-CSF: macrophage colony-stimulating factor
MyD88: myeloid differentiation primary response 88
NADPH: nicotinamide adenine dinucleotide phosphate (reduced)
NF-κB: nuclear factor kappa-light-chain-enhancer of activated B cells
NO: nitric oxide
OXPHOS: oxidative phosphorylation
PA: phosphatidic acid
PAF-AH: platelet-activating factor acetyl hydrolases
PC: choline-containing glycerophospholipids
PE: ethanolamine-containing glycerophospholipids
PG: prostaglandin
PI: phosphatidylinositol
PLA2: phospholipase A2
PS: phosphatidylserine
PUFA: polyunsaturated fatty acid
ROS: reactive oxygen species
SCFA: short-chain fatty acid
SREBP1: sterol regulatory element-binding protein 1
TAG: triacylglycerol
TAM: tumor-associated macrophage
TCA: tricarboxylic acid cycle
Th: T-helper lymphocytes
TLR: Toll-like receptor
TRAF6: TNF receptor-associated factor 6
TX: thromboxane.
References
1. Haniffa M, Bigley V, Collin M. Human mononuclear phagocyte system reunited. Semin Cell Dev Biol. (2015) 41:59–69. doi: 10.1016/j.semcdb.2015.05.004
2. Locati M, Curtale G, Mantovani A. Diversity, mechanisms, and significance of macrophage plasticity. Annu Rev Pathol Mech Dis. (2020) 15:123–47. doi: 10.1146/annurev-pathmechdis-012418-012718
3. Murray PJ. Macrophage polarization. Annu Rev Physiol. (2017) 79:541–66. doi: 10.1146/annurev-physiol-022516-034339
4. Hashimoto D, Chow A, Noizat C, Teo P, Beasley MB, Leboeuf M, et al. Tissue-resident macrophages self-maintain locally throughout adult life with minimal contribution from circulating monocytes. Immunity. (2013) 38:792–804. doi: 10.1016/j.immuni.2013.04.004
5. Gomez-Perdiguero E, Klapproth K, Schulz C, Busch K, Azzoni E, Crozet L, et al. Tissue-resident macrophages originate from yolk-sac-derived erythro-myeloid progenitors. Nature. (2015) 518:547–51. doi: 10.1038/nature13989
6. Chakarov S, Lim HY, Tan L, Lim SY, See P, Lum J, et al. Two distinct interstitial macrophage populations coexist across tissues in specific subtissular niches. Science. (2019) 363:eaau0964. doi: 10.1126/science.aau0964
7. Gentek R, Molawi K, Sieweke MH. Tissue macrophage identity and self-renewal. Immunol Rev. (2014) 262:56–73. doi: 10.1111/imr.12224
8. Sanin DE, Ge Y, Marinkovic E, Kabat AM, Castoldi A, Caputa G, et al. A common framework of monocyte-derived macrophage activation. Sci Immunol. (2022) 7:eabl7482. doi: 10.1126/sciimmunol.abl7482
9. Mackaness GB. Cellular resistance to infection. J Exp Med. (1962) 116:381–406. doi: 10.1084/jem.116.3.381
10. Nathan CF, Murray HW, Wiebe ME, Rubin BY. Identification of interferon-gamma as the lymphokine that activates human macrophage oxidative metabolism and antimicrobial activity. J Exp Med. (1983) 158:670–89. doi: 10.1084/jem.158.3.670
11. Mokoena T, Gordon S. Human macrophage activation. Modulation of mannosyl, fucosyl receptor activity in vitro by lymphokines, gamma and alpha interferons, and dexamethasone. J Clin Invest. (1985) 75:624–31. doi: 10.1172/JCI111740
12. Stein M, Keshav S, Harris N, Gordon S. Interleukin 4 potently enhances murine macrophage mannose receptor activity: A marker of alternative immunologic macrophage activation. J Exp Med. (1992) 176:287–92. doi: 10.1084/jem.176.1.287
13. Mills CD, Kincaid K, Alt JM, Heilman MJ, Hill AM. M-1/M-2 macrophages and the Th1/Th2 paradigm. J Immunol. (2000) 164:6166–73. doi: 10.4049/jimmunol.164.12.6166
14. Mills CD. M1 and M2 macrophages: Oracles of health and disease. Crit Rev Immunol. (2012) 32:463–88. doi: 10.1615/critrevimmunol.v32.i6.10
15. Mills CD. Anatomy of a discovery: M1 and M2 macrophages. Front Immunol. (2015) 6:212. doi: 10.3389/fimmu.2015.00212
16. Mantovani A, Sica A, Sozzani S, Allavena P, Vecchi A, Locati M. The chemokine system in diverse forms of macrophage activation and polarization. Trends Immunol. (2004) 25:677–86. doi: 10.1016/j.it.2004.09.015
17. Ferrante CJ, Pinhal-Enfield G, Elson G, Cronstein BN, Hasko G, Outram S, et al. The adenosine-dependent angiogenic switch of macrophages to an M2-like phenotype is independent of interleukin-4 receptor alpha (IL-4Rα) signaling. Inflammation. (2013) 36:921–31. doi: 10.1007/s10753-013-9621-3
18. Jablonski KA, Amici SA, Webb LM, Ruiz-Rosado JDD, Popovich PG, Partida-Sanchez S, et al. Novel markers to delineate murine M1 and M2 macrophages. PloS One. (2015) 10:5–11. doi: 10.1371/journal.pone.0145342
19. Jha AK, Huang SCC, Sergushichev A, Lampropoulou V, Ivanova Y, Loginicheva E, et al. Network integration of parallel metabolic and transcriptional data reveals metabolic modules that regulate macrophage polarization. Immunity. (2015) 42:419–30. doi: 10.1016/j.immuni.2015.02.005
20. Yao Y, Xu XH, Jin L. Macrophage polarization in physiological and pathological pregnancy. Front Immunol. (2019) 10:792. doi: 10.3389/fimmu.2019.00792
21. Zhang Q, Sioud M. Tumor-associated macrophage subsets: shaping polarization and targeting. Int J Mol Sci. (2023) 24:7493. doi: 10.3390/ijms24087493
22. He L, Jhong JH, Chen Q, Huang KY, Strittmatter K, Kreuzer J, et al. Global characterization of macrophage polarization mechanisms and identification of M2-type polarization inhibitors. Cell Rep. (2021) 37:109955. doi: 10.1016/j.celrep.2021.109955
23. Liu P-S, Wang H, Li X, Chao T, Teav T, Christen S, et al. [amp]]alpha;-Ketoglutarate orchestrates macrophage activation through metabolic and epigenetic reprogramming. Nat Immunol. (2017) 18:985–94. doi: 10.1038/ni.3796
24. Okabe Y, Medzhitov R. Tissue-specific signals control reversible program of localization and functional polarization of macrophages. Cell. (2014) 157:832–44. doi: 10.1016/j.cell.2014.04.016
25. Orecchioni M, Ghosheh Y, Pramod AB, Ley K. Macrophage polarization: Different gene signatures in M1(Lps+) vs. classically and M2(LPS-) vs. alternatively activated macrophages. Front Immunol. (2019) 10:1084. doi: 10.3389/fimmu.2019.01084
26. Kihara Y. Druggable lipid signaling pathways. Adv Exp Med Biol. (2020) 1274:1–4. doi: 10.1007/978-3-030-50621-6_1
27. Yoon H, Shaw JL, Haigis MC, Greka A. Lipid metabolism in sickness and in health: Emerging regulators of lipotoxicity. Mol Cell. (2021) 81:3708–30. doi: 10.1016/j.molcel.2021.08.027
28. Ross EA, Devitt A, Johnson JR. Macrophages: The good, the bad, and the gluttony. Front Immunol. (2021) 12:708186. doi: 10.3389/fimmu.2021.708186
29. Warburg O, Wind F, Negelein E. The metabolism of tumors in the body. J Gen Physiol. (1927) 8:519–30. doi: 10.1085/jgp.8.6.519
30. Mantovani A, Biswas SK, Galdiero MR, Sica A, Locati M. Macrophage plasticity and polarization in tissue repair and remodelling. J Pathol. (2013) 229:176–85. doi: 10.1002/path.4133
31. Jung J, Zeng H, Horng T. Metabolism as a guiding force for immunity. Nat Cell Biol. (2019) 21:85–93. doi: 10.1038/s41556-018-0217-x
32. Viola A, Munari F, Sánchez-Rodríguez R, Scolaro T, Castegna A. The metabolic signature of macrophage responses. Front Immunol. (2019) 10:1462. doi: 10.3389/fimmu.2019.01462
33. Van den Bossche J, O’Neill LA, Menon D. Macrophage immunometabolism: where are we (going)? Trends Immunol. (2017) 38:395–406. doi: 10.1016/j.it.2017.03.001
34. Verdeguer F, Aouadi M. Macrophage heterogeneity and energy metabolism. Exp Cell Res. (2017) 360:35–40. doi: 10.1016/j.yexcr.2017.03.043
35. O’Neill LAJ, Kishton RJ, Rathmell J. A guide to immunometabolism for immunologists. Nat Rev Immunol. (2016) 16:553–65. doi: 10.1038/nri.2016.70
36. Nomura M, Liu J, Rovira II, Gonzalez-Hurtado E, Lee J, Wolfgang MJ, et al. Fatty acid oxidation in macrophage polarization. Nat Immunol. (2016) 17:216–7. doi: 10.1038/ni.3366
37. Namgaladze D, Brüne B. Macrophage fatty acid oxidation and its roles in macrophage polarization and fatty acid-induced inflammation. Biochim Biophys Acta. (2016) 1861:1796–807. doi: 10.1016/j.bbalip.2016.09.002
38. Mehta MM, Weinberg SE, Chandel NS. Mitochondrial control of immunity: Beyond ATP. Nat Rev Immunol. (2017) 17:608–20. doi: 10.1038/nri.2017.66
39. Zhuang XY, Zhang YH, Xiao AF, Zhang AH, Fang BS. Key enzymes in fatty acid synthesis pathway for bioactive lipid biosynthesis. Front Nutr. (2022) 9:851402. doi: 10.3389/fnut.2022.851402
40. Zhang C, Wang Y, Wang F, Wang Z, Lu Y, Xu Y, et al. Quantitative profiling of glycerophospholipids during mouse and human macrophage differentiation using targeted mass spectrometry. Sci Rep. (2017) 7:412. doi: 10.1038/s41598-017-00341-2
41. Ting HC, Chen LT, Chen JY, Huang YL, Xin RC, Chan JF, et al. Double bonds of unsaturated fatty acids differentially regulate mitochondrial cardiolipin remodeling. Lipids Health Dis. (2019) 18:53. doi: 10.1186/s12944-019-0990-y
42. Kindt A, Liebisch G, Clavel T, Haller D, Hörmannsperger G, Yoon H, et al. The gut microbiota promotes hepatic fatty acid desaturation and elongation in mice. Nat Commun. (2018) 9:3760. doi: 10.1038/s41467-018-05767-4
43. Talamonti E, Pauter AM, Asadi A, Fischer AW, Chiurchiù V, Jacobsson A. Impairment of systemic DHA synthesis affects macrophage plasticity and polarization: implications for DHA supplementation during inflammation. Cell Mol Life Sci. (2017) 74:2815–26. doi: 10.1007/s00018-017-2498-9
44. Vessby B, Gustafsson IB, Tengblad S, Boberg M, Andersson A. Desaturation and elongation of fatty acids and insulin action. Ann N.Y. Acad Sci. (2002) 967:183–95. doi: 10.1111/j.1749-6632.2002.tb04275.x
45. Geng J, Liu Y, Dai H, Wang C. Fatty acid metabolism and idiopathic pulmonary fibrosis. Front Physiol. (2022) 12:794629. doi: 10.3389/fphys.2021.794629
46. Valdearcos. M, Esquinas E, Meana C, Peña L, Gil-de-Gómez L, Balsinde J, et al. Lipin-2 reduces proinflammatory signaling induced by saturated fatty acids in macrophages. J Biol Chem. (2012) 287:10894–108904. doi: 10.1074/jbc.M112.342915
47. Guijas C, Meana C, Astudillo AM, Balboa MA, Balsinde J. Foamy monocytes are enriched in cis-7-hexadecenoic fatty acid, 16:1n-9, a possible biomarker for early detection of cardiovascular disease. Cell Chem Biol. (2016) 23:689–99. doi: 10.1016/j.chembiol.2016.04.012
48. Astudillo AM, Meana C, Guijas C, Pereira L, Lebrero P, Balboa MA, et al. Occurrence and biological activity of palmitoleic acid isomers in phagocytic cells. J Lipid Res. (2018) 59:237–49. doi: 10.1194/jlr.M079145
49. Bermúdez MA, Pereira L, Fraile C, Valerio L, Balboa MA, Balsinde J. Roles of palmitoleic acid and its positional isomers, hypogeic and sapienic acids, in inflammation, metabolic diseases and cancer. Cells. (2022) 11:2146. doi: 10.3390/cells11142146
50. Oh DY, Talukdar S, Bae EJ, Imamura T, Morinaga H, Fan W, et al. GPR120 is an omega-3 fatty acid receptor mediating potent anti-inflammatory and insulin-sensitizing effects. Cell. (2010) 142:687–98. doi: 10.1016/j.cell.2010.07.041
51. Innes JK, Calder PC. Omega-6 fatty acids and inflammation. Prostaglandins Leukot Essent Fat. Acids. (2018) 132:41–8. doi: 10.1016/j.plefa.2018.03.004
52. Lee-Okada HC, Xue C, Yokomizo T. Recent advances on the physiological and pathophysiological roles of polyunsaturated fatty acids and their biosynthetic pathway. Biochim Biophys Acta. (2025) 1870:159564. doi: 10.1016/j.bbalip.2024.159564
53. Song MY, Wang J, Lee Y, Lee J, Kwon KS, Bae EJ, et al. Enhanced M2 macrophage polarization in high n-3 polyunsaturated fatty acid transgenic mice fed a high-fat diet. Mol Nutr Food Res. (2016) 60:2481–92. doi: 10.1002/mnfr.201600014
54. Poledne R, Malinska H, Kubatova H, Fronek J, Thieme F, Kauerova S, et al. Polarization of macrophages in human adipose tissue is related to the fatty acid spectrum in membrane phospholipids. Nutrients. (2020) 2:8. doi: 10.3390/nu12010008
55. Videla LA, Valenzuela R, Del Campo A, Zúñiga-Hernández J. Omega-3 lipid mediators: modulation of the M1/M2 macrophage phenotype and its protective role in chronic liver diseases. Int J Mol Sci. (2023) 24:15528. doi: 10.3390/ijms242115528
56. Hung HC, Tsai SF, Chou HW, Tsai MJ, Hsu PL, Kuo YM. Dietary fatty acids differentially affect secretion of pro-inflammatory cytokines in human THP-1 monocytes. Sci Rep. (2023) 13:5511. doi: 10.1038/s41598-023-32710-5
57. Schebb NH, Kühn H, Kahnt AS, Rund KM, O’Donnell VB, Flamand N, et al. Formation, signaling and occurrence of specialized pro-resolving lipid mediators-What is the evidence so far? Front Pharmacol. (2022) 13:838782. doi: 10.3389/fphar.2022.838782
58. O’Donnell VB, Schebb NH, Milne GL, Murphy MP, Thomas CP, Steinhilber D, et al. Failure to apply standard limit-of-detection or limit-of-quantitation criteria to specialized pro-resolving mediator analysis incorrectly characterizes their presence in biological samples. Nat Commun. (2023) 14:7172. doi: 10.1038/s41467-023-41766-w
59. Li Q, Cui K, Wu M, Xu D, Mai K, Ai Q. Polyunsaturated fatty acids influence LPS-induced inflammation of fish macrophages through differential modulation of pathogen recognition and p38 MAPK/NF-κB signaling. Front Immunol. (2020) 11:559332. doi: 10.3389/fimmu.2020.559332
60. Balestrieri B, Di Costanzo D, Dwyer DF. Macrophage-mediated immune responses: from fatty acids to oxylipins. Molecules. (2022) 27:152. doi: 10.3390/molecules27010152
61. Tadie JM, Bae HB, Deshane JS, Bell CP, Lazarowski ER, Chaplin DD, et al. Toll-like receptor 4 engagement inhibits adenosine 5’-monophosphate-activated protein kinase activation through a high mobility group box 1 protein-dependent mechanism. Mol Med. (2012) 18:659–68. doi: 10.2119/molmed.2011.00401
62. Infantino V, Convertini P, Cucci L, Panaro MA, Di Noia MA, Calvello R, et al. The mitochondrial citrate carrier: a new player in inflammation. Biochem J. (2011) 438:433–6. doi: 10.1042/BJ20111275
63. Infantino V, Iacobazzi V, Palmieri F, Menga A. ATP-citrate lyase is essential for macrophage inflammatory response. Biochem Biophys Res Commun. (2013) 440:105–11. doi: 10.1016/j.bbrc.2013.09.037
64. Wei X, Song H, Yin L, Rizzo MG, Sidhu R, Covey DF, et al. Fatty acid synthesis configures the plasma membrane for inflammation in diabetes. Nature. (2016) 539:294–8. doi: 10.1038/nature20117
65. Lauterbach MA, Hanke JE, Serefidou M, Mangan MSJ, Kolbe CC, Hess T, et al. Toll-like receptor signaling rewires macrophage metabolism and promotes histone acetylation via ATP-citrate lyase. Immunity. (2019) 51:997–1011. doi: 10.1016/j.immuni.2019.11.009
66. Yeudall S, Upchurch CM, Seegren PV, Pavelec CM, Greulich J, Lemke MC, et al. Macrophage acetyl-CoA carboxylase regulates acute inflammation through control of glucose and lipid metabolism. Sci Adv. (2022) 8:eabq1984. doi: 10.1126/sciadv.abq1984
67. Schultz JR, Tu H, Luk A, Repa JJ, Medina JC, Li L, et al. Role of LXRs in control of lipogenesis. Genes Dev. (2000) 14:2831–8. doi: 10.1101/gad.850400
68. Castrillo A, Joseph SB, Vaidya SA, Haberland M, Fogelman AM, Cheng G, et al. Crosstalk between LXR and toll-like receptor signaling mediates bacterial and viral antagonism of cholesterol metabolism. Mol Cell. (2003) 12:805–16. doi: 10.1016/s1097-2765(03)00384-8
69. Joseph SB, Castrillo A, Laffitte BA, Mangelsdorf DJ, Tontonoz P. Reciprocal regulation of inflammation and lipid metabolism by liver X receptors. Nat Med. (2003) 9:213–9. doi: 10.1038/nm820
70. Ito A, Hong C, Rong X, Zhu X, Tarling EJ, Hedde PN, et al. LXRs link metabolism to inflammation through Abca1-dependent regulation of membrane composition and TLR signaling. eLife. (2015) 4:e08009. doi: 10.7554/eLife.08009
71. Im S-S, Yousef L, Blaschitz C, Liu JZ, Edwards RA, Young SG, et al. Linking lipid metabolism to the innate immune response in macrophages through sterol regulatory element binding protein-1a. Cell Metab. (2011) 13:540–9. doi: 10.1016/j.cmet.2011.04.001
72. Oishi Y, Spann NJ, Link VM, Muse ED, Strid T, Edillor C, et al. SREBP1 contributes to resolution of pro-inflammatory TLR4 signaling by reprogramming fatty acid metabolism. Cell Metab. (2017) 25:412–27. doi: 10.1016/j.cmet.2016.11.009
73. Huang SC-C, Everts B, Ivanova Y, O’Sullivan D, Nascimento M, Smith AM, et al. Cell-intrinsic lysosomal lipolysis is essential for alternative activation of macrophages. Nat Immunol. (2014) 15:846–55. doi: 10.1038/ni.2956
74. Biswas SK, Mantovani A. Orchestration of metabolism by macrophages. Cell Metab. (2012) 15:432–7. doi: 10.1016/j.cmet.2011.11.013
75. Vats D, Mukundan L, Odegaard JI, Zhang L, Smith KL, Morel CR, et al. Oxidative metabolism and PGC-1β attenuate macrophage-mediated inflammation. Cell Metab. (2006) 4:13–24. doi: 10.1016/j.cmet.2006.05.011
76. Tan Z, Xie N, Cui H, Moellering DR, Abraham E, Thannickal VJ, et al. Pyruvate dehydrogenase kinase 1 participates in macrophage polarization via regulating glucose metabolism. J Immunol. (2015) 194:6082–6089. doi: 10.4049/jimmunol.1402469
77. Divakaruni AS, Hsieh WY, Minarrieta L, Duong TN, Kim KKO, Desousa BR, et al. Etomoxir inhibits macrophage polarization by disrupting CoA homeostasis. Cell Metab. (2018) 28:490–503. doi: 10.1016/j.cmet.2018.06.001
78. Podrez EA, Febbraio M, Sheibani N, Schmitt D, Silverstein RL, Hajjar DP, et al. Macrophage scavenger receptor CD36 is the major receptor for LDL modified by monocyte-generated reactive nitrogen species. J Clin Invest. (2000) 105:1095–108. doi: 10.1172/JCI8574
79. Rahaman SO, Lennon DJ, Febbraio M, Podrez EA, Hazen SL, Silverstein RL. A CD36-dependent signaling cascade is necessary for macrophage foam cell formation. Cell Metab. (2006) 4:211–21. doi: 10.1016/j.cmet.2006.06.007
80. Chen Y, Yang M, Huang W, Chen W, Zhao Y, Schulte ML, et al. Mitochondrial metabolic reprogramming by CD36 signaling drives macrophage inflammatory responses. Circ Res. (2019) 125:1087–102. doi: 10.1161/CIRCRESAHA.119.315833
81. Chen Y, Zhang J, Cui W, Silverstein RL. CD36, a signaling receptor and fatty acid transporter that regulates immune cell metabolism and fate. J Exp Med. (2022) 219:1–15. doi: 10.1084/jem.20211314
82. Sheedy FJ, Moore KJ. IL-1 signaling in atherosclerosis: sibling rivalry. Nat Immunol. (2013) 14:1030–10302. doi: 10.1038/ni.2711
83. Duan H, Wang LJ, Huangfu M, Li H. The impact of microbiota-derived short-chain fatty acids on macrophage activities in disease: Mechanisms and therapeutic potentials. Biomed Pharmacother. (2023) 165:115276. doi: 10.1016/j.biopha.2023.115276
84. Xie Q, Li Q, Fang H, Zhang R, Tang H, Chen L. Gut-derived short-chain fatty acids and macrophage modulation: exploring therapeutic potentials in pulmonary fungal infections. Clin Rev Allergy Immunol. (2024) 66:316–27. doi: 10.1007/s12016-024-08999-z
85. Cong J, Zhou P, Zhang R. Intestinal microbiota-derived short chain fatty acids in host health and disease. Nutrients. (2022) 14:1977. doi: 10.3390/nu14091977
86. Fusco W, Bernabeu Lorenzo M, Cintoni M, Porcari S, Rinninella E, Kaitsas F, et al. Short-chain fatty-acid-producing bacteria: Key components of the human gut microbiota. Nutrients. (2023) 15:2211. doi: 10.3390/nu15092211
87. Mann ER, Lam YK, Uhlig HH. Short-chain fatty acids: linking diet, the microbiome and immunity. Nat Rev Immunol. (2024) 24:577–95. doi: 10.1038/s41577-024-01014-8
88. Astudillo AM, Balgoma D, Balboa MA, Balsinde J. Dynamics of arachidonic acid mobilization by inflammatory cells. Biochim Biophys Acta. (2012) 1821:249–56. doi: 10.1016/j.bbalip.2011.11.006
89. Dennis EA, Norris PC. Eicosanoid storm in infection and inflammation. Nat Rev Immunol. (2015) 15:511–23. doi: 10.1038/nri3859
90. Leuti A, Fazio D, Fava M, Piccoli A, Oddi S, Maccarrone M. Bioactive lipids, inflammation and chronic diseases. Adv Drug Deliv. Rev. (2020) 159:133–69. doi: 10.1016/j.addr.2020.06.028
91. Radmark O. Formation of eicosanoids and other oxylipins in human macrophages. Biochem Pharmacol. (2022) 204:115210. doi: 10.1016/j.bcp.2022.115210
92. Gupta S, Maurya MR, Stephens DL, Dennis EA, Subramaniam S. An integrated model of eicosanoid metabolism and signaling based on lipidomics flux analysis. Biophys J. (2009) 96:4542–51. doi: 10.1016/j.bpj.2009.03.011
93. Norris PC, Reichart D, Dumlao DS, Glass CK, Dennis EA. Specificity of eicosanoid production depends on the TLR-4-stimulated macrophage phenotype. J Leukoc. Biol. (2011) 90:563–74. doi: 10.1189/jlb.0311153
94. Montenegro-Burke JR, Sutton JA, Rogers LM, Milne GL, McLean JA, Aronoff DM. Lipid profiling of polarized human monocyte-derived macrophages. Prostaglandins Other Lipid Mediat. (2016) 127:1–8. doi: 10.1016/j.prostaglandins.2016.11.002
95. Lukic A, Larssen P, Fauland A, Samuelsson B, Wheelock CE, Gabrielsson S, et al. GM-CSF– and M-CSF–primed macrophages present similar resolving but distinct inflammatory lipid mediator signatures. FASEB J. (2017) 31:4370–81. doi: 10.1096/fj.201700319R
96. Werz O, Gerstmeier J, Libreros S, de la Rosa X, Werner M, Norris PC, et al. Human macrophages differentially produce specific resolvin or leukotriene signals that depend on bacterial pathogenicity. Nat Commun. (2018) 9:59. doi: 10.1038/s41467-017-02538-5
97. Cui J, Shan K, Yang Q, Chen W, Feng N, Chen YQ. Eicosanoid production by macrophages during inflammation depends on the M1/M2 phenotype. Prostaglandins Other Lipid Mediat. (2022) 160:106635. doi: 10.1016/j.prostaglandins.2022.106635
98. Astudillo AM, Balboa MA, Balsinde J. Selectivity of phospholipid hydrolysis by phospholipase A2 enzymes in activated cells leading to polyunsaturated fatty acid mobilization. Biochim Biophys Acta. (2019) 1864:772–83. doi: 10.1016/j.bbalip.2018.07.002
99. Mouchlis VD, Dennis EA. Phospholipase A2 catalysis and lipid mediator lipidomics. Biochim Biophys Acta. (2019) 1864:766–71. doi: 10.1016/j.bbalip.2018.08.010
100. Dennis EA, Cao J, Hsu YH, Magrioti V, Kokotos G. Phospholipase A2 enzymes: Physical structure, biological function, disease implication, chemical inhibition, and therapeutic intervention. Chem Rev. (2011) 111:6130–85. doi: 10.1021/cr200085w
101. Murakami M. The phospholipase A2 superfamily as a central hub of bioactive lipids and beyond. Pharmacol Ther. (2023) 244:108382. doi: 10.1016/j.pharmthera.2023.108382
102. Balsinde J, Winstead MV, Dennis EA. Phospholipase A2 regulation of arachidonic acid mobilization. FEBS Lett. (2002) 531:2–6. doi: 10.1016/s0014-5793(02)03413-0
103. Murakami M, Sato H, Miki Y, Yamamoto K, Taketomi Y. A new era of secreted phospholipase A2s. J Lipid Res. (2015) 56:1248–61. doi: 10.1194/jlr.R058123
104. Girotti M, Evans JH, Burke D, Leslie CC. Cytosolic phospholipase A2 translocates to forming phagosomes during phagocytosis of zymosan in macrophages. J Biol Chem. (2004) 279:19113–21. doi: 10.1074/jbc.M313867200
105. Suram S, Brown GD, Ghosh M, Gordon S, Loper R, Taylor PR, et al. Regulation of cytosolic phospholipase A2 activation and cyclooxygenase-2 expression in macrophages by the β-glucan receptor. J Biol Chem. (2006) 281:5506–14. doi: 10.1074/jbc.M509824200
106. Casas J, Meana C, Esquinas E, Valdearcos M, Pindado J, Balsinde J, Balboa MA. Requirement of JNK-mediated phosphorylation for translocation of group IVA phospholipase A2 to phagosomes in human macrophages. J Immunol. (2009) 183:2767–74. doi: 10.4049/jimmunol.0901530
107. Casas J, Valdearcos M, Pindado J, Balsinde J, Balboa MA. The cationic cluster of group IVA phospholipase A2 (Lys488/Lys541/Lys543/Lys544) is involved in translocation of the enzyme to phagosomes in human macrophages. J Lipid Res. (2010) 51:388–99. doi: 10.1194/jlr.M001461
108. Zizza P, Iurisci C, Bonazzi M, Cossart P, Leslie CC, Corda D, et al. Phospholipase A2 IVα regulates phagocytosis independent of its enzymatic activity. J Biol Chem. (2012) 287:16849–59. doi: 10.1074/jbc.M111.309419
109. Dabral D, van den Bogaart G. The roles of phospholipase A2 in phagocytes. Front Cell Dev Biol. (2021) 9:673502. doi: 10.3389/fcell.2021.673502
110. Ashley JW, Hancock WD, Nelson AJ, Bone RN, Tse HM, Wohltmann M, et al. Polarization of macrophages toward M2 phenotype is favored by reduction in iPLA2β (group VIA phospholipase A2). J Biol Chem. (2016) 291:23268–81. doi: 10.1074/jbc.M116.754945
111. Nelson AJ, Stephenson DJ, Cardona CL, Lei X, Almutairi A, White TD, et al. Macrophage polarization is linked to Ca2+-independent phospholipase A2β-derived lipids and cross-cell signaling in mice. J Lipid Res. (2020) 61:143–8. doi: 10.1194/jlr.RA119000281
112. Klement L, Jansakun C, Yan B, Staffer S, Tuma-Kellner S, Altamura S, et al. Myeloid-specific deletion of group VIA calcium-independent phospholipase A2 induces pro-inflammatory LPS response predominantly in male mice via MIP-1α activation. Biochim Biophys Acta. (2024) 1870:167016. doi: 10.1016/j.bbadis.2024.167016
113. Astudillo AM, Balboa MA, Balsinde J. Compartmentalized regulation of lipid signaling in oxidative stress and inflammation: plasmalogens, oxidized lipids and ferroptosis as new paradigms of bioactive lipid research. Prog Lipid Res. (2023) 89:101207. doi: 10.1016/j.plipres.2022.101207
114. Brouwers H, Jónasdóttir HS, Kuipers ME, Kwekkeboom JC, Auger JL, Gonzalez-Torres M, et al. Anti-inflammatory and proresolving effects of the omega-6 polyunsaturated fatty acid adrenic acid. J Immunol. (2020) 205:2840–9. doi: 10.4049/jimmunol.1801653
115. Monge P, Garrido A, Rubio JM, Magrioti V, Kokotos G, Balboa MA, et al. The contribution of cytosolic group IVA and calcium-independent group VIA phospholipase A2s to adrenic acid mobilization in murine macrophages. Biomolecules. (2020) 10:542. doi: 10.3390/biom10040542
116. Cheon Y, Kim HW, Igarashi M, Modi HR, Chang L, Ma K, et al. Disturbed brain phospholipid and docosahexaenoic acid metabolism in calcium-independent phospholipase A2-VIA (iPLA2β)- knockout mice. Biochim Biophys Acta. (2012) 1821:1278–86. doi: 10.1016/j.bbalip.2012.02.003
117. Basselin M, Rosa AO, Ramadan E, Cheon Y, Chang L, Chen M, et al. Imaging decreased brain docosahexaenoic acid metabolism and signaling in iPLA2β (VIA)-deficient mice. J Lipid Res. (2010) 51:3166–73. doi: 10.1194/jlr.M008334
118. Peltner LK, Gluthmann L, Börner F, Pace S, Hoffstetter RK, Kretzer C, et al. Cannabidiol acts as molecular switch in innate immune cells to promote the biosynthesis of inflammation-resolving lipid mediators. Cell Chem Biol. (2023) 30:1508–24. doi: 10.1016/j.chembiol.2023.08.001
119. Astudillo AM, Meana C, Bermúdez MA, Pérez-Encabo A, Balboa MA, Balsinde J. Release of anti-inflammatory palmitoleic acid and its positional isomers by mouse peritoneal macrophages. Biomedicines. (2020) 8:480. doi: 10.3390/biomedicines8110480
120. Bermúdez MA, Garrido A, Pereira L, Garrido T, Balboa MA, Balsinde J. Rapid movement of palmitoleic acid from phosphatidylcholine to phosphatidylinositol in activated human monocytes. Biomolecules. (2024) 14:707. doi: 10.3390/biom14060707
121. Sun WY, Tyurin VA, Mikulska-Ruminska K, Shrivastava IH, Anthonymuthu TS, Zhai Y-J, et al. Phospholipase iPLA2β averts ferroptosis by eliminating a redox lipid death signal. Nat Chem Biol. (2021) 17:465–76. doi: 10.1038/s41589-020-00734-x
122. Chen D, Chu B, Yang X, Liu Z, Jin Y, Kon N, et al. iPLA2β-mediated lipid detoxification controls p53-driven ferroptosis independent of GPX4. Nat Commun. (2021) 12:3644. doi: 10.1038/s41467-021-23902-6
123. Murakami M, Sato H, Taketomi. Y. Modulation of immunity by the secreted phospholipase A2 family. Immunol Rev. (2023) 317:42–70. doi: 10.1111/imr.13205
124. Balestrieri B, Hsu VW, Gilbert H, Leslie CC, Han WK, Bonventre JV, et al. Group V secretory phospholipase A2 translocates to the phagosome after zymosan stimulation of mouse peritoneal macrophages and regulates phagocytosis. J Biol Chem. (2006) 281:6691–8. doi: 10.1074/jbc.M508314200
125. Koganesawa M, Yamaguchi M, Samuchiwal SK, Balestrieri B. Lipid profile of activated macrophages and contribution of group V phospholipase A2. Biomolecules. (2021) 11:25. doi: 10.3390/biom11010025
126. Balsinde J, Balboa MA, Yedgar S, Dennis EA. Group V phospholipase A2-mediated oleic acid mobilization in lipopolysaccharide-stimulated P388D1 macrophages. J Biol Chem. (2000) 275:4783–6. doi: 10.1074/jbc.275.7.4783
127. Watanabe K, Taketomi Y, Miki Y, Kugiyama K, Murakami M. Group V secreted phospholipase A2 plays a protective role against aortic dissection. J Biol Chem. (2020) 295:10092–111. doi: 10.1074/jbc.RA120.013753
128. Sendetski M, Wedel S, Furutani K, Hahnefeld L, Angioni C, Heering J, et al. Oleic acid released by sensory neurons inhibits TRPV1-mediated thermal hypersensitivity via GPR40. iScience. (2024) 27:110552. doi: 10.1016/j.isci.2024.110552
129. Satake Y, Diaz BL, Balestrieri B, Lam BK, Kanaoka Y, Grusby MJ, et al. Role of group V phospholipase A2 in zymosan-induced eicosanoid generation and vascular permeability revealed by targeted gene disruption. J Biol Chem. (2004) 279:16488–94. doi: 10.1074/jbc.M313748200
130. Pindado J, Balsinde J, Balboa MA. TLR3-dependent induction of nitric oxide synthase in RAW 264.7 macrophage-like cells via a cytosolic phospholipase A2/cyclooxygenase-2 pathway. J Immunol. (2007) 179:4821–8. doi: 10.4049/jimmunol.179.7.4821
131. Ruipérez V, Casas J, Balboa MA, Balsinde J. Group V phospholipase A2-derived lysophosphatidylcholine mediates cyclooxygenase-2 induction in lipopolysaccharide-stimulated macrophages. J Immunol. (2007) 179:631–8. doi: 10.4049/jimmunol.179.1.631
132. Ruipérez V, Astudillo AM, Balboa MA, Balsinde J. Coordinate regulation of TLR-mediated arachidonic acid mobilization in macrophages by group IVA and group V phospholipase A2s. J Immunol. (2009) 182:3877–83. doi: 10.4049/jimmunol.0804003
133. Hsieh WY, Zhou QD, York AG, Williams KJ, Scumpia PO, Kronenberger EB, et al. Toll-like receptors induce signal-specific reprogramming of the macrophage lipidome. Cell Metab. (2020) 32:128–43. doi: 10.1016/j.cmet.2020.05.003
134. Kita Y, Shindou H, Shimizu T. Cytosolic phospholipase A2 and lysophospholipid acyltransferases. Biochim Biophys Acta. (2019) 1864:838–45. doi: 10.1016/j.bbalip.2018.08.006
135. Kikawada. E, Bonventre JV, Arm JP. Group V secretory PLA2 regulates TLR2-dependent eicosanoid generation in mouse mast cells through amplification of ERK and cPLA2α activation. Blood. (2007) 110:561–7. doi: 10.1182/blood-2006-10-052258
136. Balsinde J, Shinohara H, Lefkowitz LJ, Johnson CA, Balboa MA, Dennis EA. Group V phospholipase A2-dependent induction of cyclooxygenase-2 in macrophages. J Biol Chem. (1999) 274:25967–70. doi: 10.1074/jbc.274.37.25967
137. Balboa MA, Perez R, Balsinde J. Amplification mechanisms of inflammation: paracrine stimulation of arachidonic acid mobilization by secreted phospholipase A2 is regulated by cytosolic phospholipase A2-derived hydroperoxyeicosatetraenoic acid. J Immunol. (2003) 171:989–94. doi: 10.4049/jimmunol.171.2.989
138. Han WK, Sapirstein A, Hung CC, Alessandrini A, Bonventre JV. Cross-talk between cytosolic phospholipase A2α (cPLA2α) and secretory phospholipase A2 (sPLA2) in hydrogen peroxide-induced arachidonic acid release in murine mesangial cells. J Biol Chem. (2003) 278:24153–63. doi: 10.1074/jbc.m300424200
139. Sato H, Taketomi Y, Ushida A, Isogai Y, Kojima T, Hirabayashi T, et al. The adipocyte-inducible secreted phospholipases PLA2G5 and PLA2G2E play distinct roles in obesity. Cell Metab. (2014) 20:119–32. doi: 10.1016/j.cmet.2014.05.002
140. Ohta S, Imamura M, Xing W, Boyce JA, Balestrieri B. Group V secretory phospholipase A2 is involved in macrophage activation and is sufficient for macrophage effector functions in allergic pulmonary inflammation. J Immunol. (2013) 190:5927–38. doi: 10.4049/jimmunol.1203202
141. Rubio JM, Rodríguez JP, Gil-de-Gómez L, Guijas C, Balboa MA, Balsinde J. Group V secreted phospholipase A2 is upregulated by IL-4 in human macrophages and mediates phagocytosis via hydrolysis of ethanolamine phospholipids. J Immunol. (2015) 194:3327–39. doi: 10.4049/jimmunol.1401026
142. Balestrieri B, Maekawa A, Xing W, Gelb MH, Katz HR, Arm JP. Group V secretory phospholipase A2 modulates phagosome maturation and regulates the innate immune response against Candida albicans. J Immunol. (2009) 182:4891–8. doi: 10.4049/jimmunol.0803776
143. Boilard E, Lai Y, Larabee K, Balestrieri B, Ghomashchi F, Fujioka D, et al. A novel anti-inflammatory role for secretory phospholipase A2 in immune complex-mediated arthritis. EMBO Mol Med. (2010) 2:172–87. doi: 10.1002/emmm.201000072
144. Rubio JM, Astudillo AM, Casas J, Balboa MA, Balsinde J. Regulation of phagocytosis in macrophages by membrane ethanolamine plasmalogens. Front Immunol. (2018) 9:1723. doi: 10.3389/fimmu.2018.01723
145. Lands WEM. Stories about acyl chains. Biochim Biophys Acta. (2000) 1483:1–14. doi: 10.1016/s1388-1981(99)00177-8
146. Chilton FH, Fonteh AN, Surette ME, Triggiani M, Winkler JD. Control of arachidonate levels within inflammatory cells. Biochim Biophys Acta. (1996) 299:1–15. doi: 10.1016/0005-2760(95)00169-7
147. Pérez-Chacón G, Astudillo AM, Balgoma D, Balboa MA, Balsinde J. Control of free arachidonic acid levels by phospholipases A2 and lysophospholipid acyltransferases. Biochim Biophys Acta. (2009) 1791:1103–13. doi: 10.1016/j.bbalip.2009.08.007
148. Murphy RC, Folco G. Lysophospholipid acyltransferases and leukotriene biosynthesis: Intersection of the Lands cycle and the arachidonate PI cycle. J Lipid Res. (2019) 60:219–26. doi: 10.1194/jlr.S091371
149. Balsinde J. Roles of various phospholipases A2 in providing lysophospholipid acceptors for fatty acid phospholipid incorporation and remodelling. Biochem J. (2002) 364:695–702. doi: 10.1042/BJ20020142
150. Balboa MA, Sáez Y, Balsinde J. Calcium-independent phospholipase A2 is required for lysozyme secretion in U937 promonocytes. J Immunol. (2003) 170:5276–80. doi: 10.4049/jimmunol.170.10.5276
151. Pérez R, Melero R, Balboa MA, Balsinde J. Role of group VIA calcium-independent phospholipase A2 in arachidonic acid release, phospholipid fatty acid incorporation, and apoptosis in U937 cells responding to hydrogen peroxide. J Biol Chem. (2004) 279:40385–91. doi: 10.1074/jbc.M402562200
152. Shindou H, Hishikawa D, Harayama T, Eto M, Shimizu T. Generation of membrane diversity by lysophospholipid acyltransferases. J Biochem. (2013) 154:21–8. doi: 10.1093/jb/mvt048
153. Yamashita A, Hayashi Y, Nemoto-Sasaki Y, Ito M, Oka S, Tanikawa T, et al. Acyltransferases and transacylases that determine the fatty acid composition of glycerolipids and the metabolism of bioactive lipid mediators in mammalian cells and model organisms. Prog Lipid Res. (2014) 53:18–81. doi: 10.1016/j.plipres.2013.10.001
154. Valentine WJ, Shimizu T, Shindou H. Lysophospholipid acyltransferases orchestrate the compositional diversity of phospholipids. Biochimie. (2023) 215:24–33. doi: 10.1016/j.biochi.2023.08.012
155. Jarc E, Petan T. A twist of FATe: Lipid droplets and inflammatory lipid mediators. Biochimie. (2020) 169:69–87. doi: 10.1016/j.biochi.2019.11.016
156. Bozza PT, Bakker-Abreu I, Navarro-Xavier RA, Bandeira-Melo C. Lipid body function in eicosanoid synthesis: An update. Prostaglandins Leukot Essent Fatty Acids. (2011) 85:205–13. doi: 10.1016/j.plefa.2011.04.020
157. Abate W, Alrammah H, Kiernan M, Tonks AJ, Jackson SK. Lysophosphatidylcholine acyltransferase 2 (LPCAT2) co-localises with TLR4 and regulates macrophage inflammatory gene expression in response to LPS. Sci Rep. (2020) 10:10355. doi: 10.1038/s41598-020-67000-x
158. Huang YH, Schäfer-Elinder L, Wu R, Claesson HE, Frostegård J. Lysophosphatidylcholine (LPC) induces proinflammatory cytokines by a platelet-activating factor (PAF) receptor-dependent mechanism. Clin Exp Immunol. (1999) 116:326–31. doi: 10.1046/j.1365-2249.1999.00871.x
159. Prabutzki P, Schiller J, Engel KM. Phospholipid-derived lysophospholipids in (patho)physiology. Atherosclerosis. (2024) 398:118569. doi: 10.1016/j.atherosclerosis.2024.118569
160. Jain S, Zhang X, Khandelwal PJ, Saunders AJ, Cummings BS, Oelkers P. Characterization of human lysophospholipid acyltransferase 3. J Lipid Res. (2009) 50:1563–70. doi: 10.1194/jlr.M800398-JLR200
161. Pérez-Chacón G, Astudillo AM, Ruipérez V, Balboa MA, Balsinde J. Signaling role for lysophospholipid acyltransferase 3 in receptor-regulated arachidonic acid reacylation reactions in human monocytes. J Immunol. (2010) 184:1071–8. doi: 10.4049/jimmunol.0902257
162. Kano K, Aoki J, Hla T. Lysophospholipid mediators in health and disease. Annu Rev Pathol. (2021) 17:459–83. doi: 10.1146/annurev-pathol-050420-025929
163. Jiang S, Yang H, Li M. Emerging roles of lysophosphatidic acid in macrophages and inflammatory diseases. Int J Mol Sci. (2023) 24:12524. doi: 10.3390/ijms241512524
164. Masquelier J, Alhouayek M, Terrasi R, Bottemanne P, Paquot A, Muccioli GG. Lysophosphatidylinositols in inflammation and macrophage activation: Altered levels and anti-inflammatory effects. Biochim Biophys Acta. (2018) 1863:1458–68. doi: 10.1016/j.bbalip.2018.09.003
165. Qin X, Qiu C, Zhao L. Lysophosphatidylcholine perpetuates macrophage polarization toward classically activated phenotype in inflammation. Cell Immunol. (2014) 289:185–90. doi: 10.1016/j.cellimm.2014.04.010
166. Li YF, Li RS, Samuel SB, Cueto R, Li XY, Wang H, et al. Lysophospholipids and their G protein-coupled receptors in atherosclerosis. Front Biosci (Landmark). (2016) 21:70–88. doi: 10.2741/4377
167. Dennis EA, Deems RA, Harkewicz R, Quehenberger O, Brown HA, Milne SB, et al. A mouse macrophage lipidome. J Biol Chem. (2010) 285:39976–29985. doi: 10.1074/jbc.M110.182915
168. Lee JW, Mok HJ, Lee DY, Park SC, Kim GS, Lee SE, et al. UPLC-QqQ/MS-based lipidomics approach to characterize lipid alterations in inflammatory macrophages. J Proteome Res. (2017) 16:1460–9. doi: 10.1021/acs.jproteome.6b00848
169. Rouzer CA, Ivanova PT, Byrne MO, Milne SB, Brown HA, Marnett LJ. Lipid profiling reveals glycerophospholipid remodeling in zymosan-stimulated macrophages. Biochemistry. (2007) 46:6026–42. doi: 10.1021/bi0621617
170. Balgoma D, Astudillo AM, Pérez-Chacón G, Montero O, Balboa MA, Balsinde J. Markers of monocyte activation revealed by lipidomic profiling of arachidonic acid-containing phospholipids. J Immunol. (2010) 184:3857–65. doi: 10.4049/jimmunol.0902883
171. Balsinde J, Balboa MA, Insel PA, Dennis EA. Differential regulation of phospholipase D and phospholipase A2 by protein kinase C in P388D1 macrophages. Biochem J. (1997) 321:805–946. doi: 10.1042/bj3210805
172. Gil-de-Gómez L, Astudillo AM, Guijas C, Magrioti V, Kokotos G, Balboa MA, et al. Cytosolic group IVA and calcium-independent group VIA phospholipase A2s act on distinct phospholipid pools in zymosan-stimulated mouse peritoneal macrophages. J Immunol. (2014) 192:752–62. doi: 10.4049/jimmunol.1302267
173. Lebrero P, Astudillo AM, Rubio JM, Fernández-Caballero J, Kokotos G, Balboa MA, et al. Cellular plasmalogen content does not influence arachidonic acid levels or distribution in macrophages: a role for cytosolic phospholipase A2γ in phospholipid remodeling. Cells. (2019) 8:799. doi: 10.3390/cells8080799
174. Andreyev A,Y, Fahy E, Guan Z, Kelly S, Li X, McDonald JG, et al. Subcellular organelle lipidomics in TLR-4-activated macrophages. J Lipid Res. (2010) 51:2785–97. doi: 10.1194/jlr.M008748
175. Balboa MA, Shirai Y, Gaietta G, Ellisman MH, Balsinde J, Dennis EA. Localization of group V phospholipase A2 in caveolin-enriched granules in activated P388D1 macrophage-like cells. J Biol Chem. (2003) 278:48059–65. doi: 10.1074/jbc.M305904200
176. Shirai Y, Balsinde J, Dennis EA. Localization and functional interrelationships among cytosolic group IV, secreted group V, and Ca2+-independent group VI phospholipase A2s in P388D1 macrophages using GFP/RFP constructs. Biochim Biophys Acta. (2005) 1735:119–29. doi: 10.1016/j.bbalip.2005.05.005
177. Pennington ER, Funai K, Brown DA, Shaikh SR. The role of cardiolipin concentration and acyl chain composition on mitochondrial inner membrane molecular organization and function. Biochim Biophys Acta. (2019) 1864:1039–52. doi: 10.1016/j.bbalip.2019.03.012
178. Reynolds MB, Hong HS, Michmerhuizen BC, Lawrence AE, Zhang L, Knight JS, et al. Cardiolipin coordinates inflammatory metabolic reprogramming through regulation of complex II disassembly and degradation. Sci Adv. (2023) 9:eade8701. doi: 10.1126/sciadv.ade8701
179. Wymann MP, Schneiter R. Lipid signalling in disease. Nat Rev Mol Cell Biol. (2008) 9:162–76. doi: 10.1038/nrm2335
180. Sun H, Sun S, Chen G, Xie H, Yu S, Lin X, et al. Ceramides and sphingosine-1-phosphate mediate the distinct effects of M1/M2-macrophage infusion on liver recovery after hepatectomy. Cell Death Dis. (2021) 12:324. doi: 10.1038/s41419-021-03616-9
181. Camell CD, Nguyen KY, Jurczak MJ, Christian BE, Shulman GI, Shadel GS, et al. Macrophage-specific de novo synthesis of ceramide is dispensable for inflammasome-driven inflammation and insulin resistance in obesity. J Biol Chem. (2015) 290:29402–13. doi: 10.1074/jbc.M115.680199
182. Afrin F, Mateen S, Oman J, Lai JCK, Barrott JJ, Pashikanti S. Natural products and small molecules targeting cellular ceramide metabolism to enhance apoptosis in cancer cells. Cancers. (2023) 15:4645. doi: 10.3390/cancers15184645
183. Li RZ, Wang XR, Wang J, Xie C, Wang XX, Pan HD, et al. The key role of sphingolipid metabolism in cancer: New therapeutic targets, diagnostic and prognostic values, and anti-tumor immunotherapy resistance. Front Oncol. (2022) 12:941643. doi: 10.3389/fonc.2022.941643
184. Weigert A, Olesch C, Brüne B. Sphingosine-1-phosphate and macrophage biology. How the sphinx tames the big eater. Front Immunol. (2019) 10:1706. doi: 10.3389/fimmu.2019.01706
185. Sun G, Wang B, Wu X, Cheng J, Ye J, Wang C, et al. How do sphingosine-1-phosphate affect immune cells to resolve inflammation? Front Immunol. (2024) 15:1362459. doi: 10.3389/fimmu.2024.1362459
186. Balsinde J, Balboa MA, Dennis EA. Inflammatory activation of arachidonic acid signaling in murine P388D1 macrophages via sphingomyelin synthesis. J Biol Chem. (1997) 272:20373–7. doi: 10.1074/jbc.272.33.20373
187. Olona A, Hateley C, Muralidharan S, Wenk MR, Torta F, Behmoaras J. Sphingolipid metabolism during Toll-like receptor 4 (TLR4)-mediated macrophage activation. Br J Pharmacol. (2021) 178:4575–87. doi: 10.1111/bph.15642
188. Castoldi A, Monteiro LB, van Teijlingen Bakker N, Sanin DE, Rana N, Corrado M, et al. Triacylglycerol synthesis enhances macrophage inflammatory function. Nat Commun. (2020) 11:4107. doi: 10.1038/s41467-020-17881-3
189. Bosch M, Sánchez-Álvarez M, Fajardo A, Kapetanovic R, Steiner B, Dutra F, et al. Mammalian lipid droplets are innate immune hubs integrating cell metabolism and host defense. Science. (2020) 370:eaay8085. doi: 10.1126/science.aay8085
190. Zimmermann JA, Lucht K, Stecher M, Badhan C, Glaser KM, Epple MW, et al. Functional multi-organelle units control inflammatory lipid metabolism of macrophages. Nat Cell Biol. (2024) 26:1261–73. doi: 10.1038/s41556-024-01457-0
191. Safi R, Sánchez-Álvarez M, Bosch M, Demangel C, Parton RG, Pol A. Defensive-lipid droplets: cellular organelles designed for antimicrobial immunity. Immunol Rev. (2023) 317:113–36. doi: 10.1111/imr.13199
192. Bermúdez MA, Balboa MA, Balsinde J. Lipid droplets, phospholipase A2, arachidonic acid, and atherosclerosis. Biomedicines. (2021) 9:1891. doi: 10.3390/biomedicines9121891
193. Leslie CC. Cytosolic phospholipase A2s: physiological function and role in disease. J Lipid Res. (2015) 56:1386–402. doi: 10.1194/jlr.R057588
194. Gijón MA, Spencer DM, Siddiqi AR, Bonventre JV, Leslie CC. Cytosolic phospholipase A2 is required for macrophage arachidonic acid release by agonists that do and do not mobilize calcium. J Biol Chem. (2000) 275:20146–56. doi: 10.1074/jbc.M908941199
195. Balboa MA, Balsinde J, Dillon DA, Carman GM, Dennis EA. Proinflammatory macrophage-activating properties of the novel phospholipid diacylglycerol pyrophosphate. J Biol Chem. (1999) 274:522–6. doi: 10.1074/jbc.274.1.522
196. Taketomi Y, Murakami M. Regulatory roles of phospholipase A2 enzymes and bioactive lipids in mast cell biology. Front Immunol. (2022) 13:923265. doi: 10.3389/fimmu.2022.923265
197. Guijas C, Pérez-Chacón G, Astudillo AM, Rubio JM, Gil-de-Gómez L, Balboa MA, et al. Simultaneous activation of p38 and JNK by arachidonic acid stimulates the cytosolic phospholipase A2-dependent synthesis of lipid droplets in human monocytes. J Lipid Res. (2012) 53:2343–54. doi: 10.1194/jlr.M028423
198. Guijas C, Rodríguez JP, Rubio JM, Balboa MA, Balsinde J. Phospholipase A2 regulation of lipid droplet formation. Biochim Biophys Acta. (2014) 1841:1661–71. doi: 10.1016/j.bbalip.2014.10.004
199. Feingold KR, Shigenaga JK, Kazemi MR, McDonald CM, Patzek SM, Cross AS, et al. Mechanisms of triglyceride accumulation in activated macrophages. J Leukoc. Biol. (2012) 92:829–39. doi: 10.1189/jlb.1111537
200. Araldi E, Fernández-Fuertes M, Canfrán-Duque A, Tang W, Cline GW, Madrigal-Matute J, et al. Lanosterol modulates TLR4-mediated innate immune responses in macrophages. Cell Rep. (2017) 19:2743–55. doi: 10.1016/j.celrep.2017.05.093
201. Zhang X, McDonald JG, Aryal B, Fernández-Hernando C. Desmosterol suppresses macrophage inflammasome activation and protects against vascular inflammation and atherosclerosis. Proc Natl Acad Sci USA. (2021) 118:e2107682118. doi: 10.1073/pnas.2107682118
202. Spann NJ, Garmire LX, McDonald JG, Myers DS, Milne SB, Shibata N, et al. Regulated accumulation of desmosterol integrates macrophage lipid metabolism and inflammatory responses. Cell. (2012) 151:138–52. doi: 10.1016/j.cell.2012.06.054
203. Hong C, Walczak R, Dhamko H, Bradley MN, Marathe C, Boyadjian R, et al. Constitutive activation of LXR in macrophages regulates metabolic and inflammatory gene expression: identification of ARL7 as a direct target. J Lipid Res. (2011) 52:531–9. doi: 10.1194/jlr.M010686
204. Morgan PK, Huynh K, Pernes G, Miotto PM, Mellett NA, Giles C, et al. Macrophage polarization state affects lipid composition and the channeling of exogenous fatty acids into endogenous lipid pools. J Biol Chem. (2021) 297:101341. doi: 10.1016/j.jbc.2021.101341
205. Prieur X, Mok CY, Velagapudi VR, Núñez V, Fuentes L, Montaner D, et al. Differential lipid partitioning between adipocytes and tissue macrophages modulates macrophage lipotoxicity and M2/M1 polarization in obese mice. Diabetes. (2011) 60:797–809. doi: 10.2337/db10-0705
206. van Beek AA, Van del Bossche J, Mastroberardino PG, de Winther MPJ, Leenen PJM. Metabolic alterations in aging macrophages: ingredients for inflammaging? Trends Immunol. (2019) 40:113–27. doi: 10.1016/j.it.2018.12.007
207. Fulop T, Larbi A, Pawelec G, Khalil A, Cohen AA, Hirokawa K, et al. Immunology of aging: the birth of inflammaging. Clin Rev Allergy Immunol. (2023) 64:109–22. doi: 10.1007/s12016-021-08899-6
208. Wculek SK, Heras-Murillo I, Mastrangelo A, Mañanes D, Galán M, Miguel V, et al. Oxidative phosphorylation selectively orchestrates tissue macrophage homeostasis. Immunity. (2023) 56:516–30. doi: 10.1016/j.immuni.2023.01.011
209. Lavin Y, Winter D, Blacher-Gonnen R, David E, Karen-Shaul H, Merad M, et al. Tissue-resident macrophage enhancer landscapes are shaped by the local microenvironment. Cell. (2014) 159:1312–26. doi: 10.1016/j.cell.2014.11.018
210. Wculek SK, Forisch S, Miguel V, Sancho D. Metabolic homeostasis of tissue macrophages across the lifespan. Trends Endocrinol Metab. (2024) 35:793–808. doi: 10.1016/j.tem.2024.04.017
211. McQuattie-Pimentel AC, Ren Z, Joshi N, Watanabe S, Stoeger T, Chi M, et al. The lung microenvironment shapes a dysfunctional response of alveolar macrophages in aging. J Clin Invest. (2021) 131:e140299. doi: 10.1172/JCI140299
212. Mossad O, Batut B, Yilmaz B, Dokalis N, Mezö C, Nent E, et al. Gut microbiota drives age-related oxidative stress and mitochondrial damage in microglia via the metabolite N6-carboxymethyllysine. Nat Neurosci. (2022) 25:295–305. doi: 10.1038/s41593-022-01027-3
213. Vida C, Martínez de Toda I, Cruces J, Garrido A, González-Sánchez M, de la Fuente M. Role of macrophages in age-related oxidative stress and lipofuscin accumulation in mice. Redox Biol. (2017) 12:423–37. doi: 10.1016/j.redox.2017.03.005
214. Burns JC, Cotleur B, Waltcher DM, Bajrami B, Rubino SJ, Wei R, et al. Differential accumulation of storage bodies with aging defines discrete subsets of microglia in the healthy brain. eLife. (2020) 9:e57495. doi: 10.7554/eLife.57495
215. Marschallinger J, Iram T, Zardeneta M, Lee SE, Lehallier B, Haney MS, et al. Lipid-droplet-accumulating microglia represent a dysfunctional and proinflammatory state in the aging brain. Nat Neurosci. (2020) 23:194–208. doi: 10.1038/s41593-019-0566-1
216. Minhas PS, Latif-Hernandez A, McReynolds MR, Durairaj AS, Wang Q, Rubin A, et al. Restoring metabolism of myeloid cells reverses cognitive decline in ageing. Nature. (2021) 590:122–8. doi: 10.1038/s41586-020-03160-0
217. Lee S, Devenney NA, Golden LR, Smith CT, Schwartz JL, Walsh AE, et al. APOE modulates microglial immunometabolism in response to age, amyloid pathology, and inflammatory challenge. Cell Rep. (2023) 42:112196. doi: 10.1016/j.celrep.2023.112196
218. Schädel P, Czapka A, Gebert N, Jacobsen ID, Ori A, Werz O. Metabololipidomic and proteomic profiling reveals aberrant macrophage activation and interrelated immunomodulatory mediator release during aging. Aging Cell. (2023) 22:13856. doi: 10.1111/acel.13856
219. Bi C, Fu Y, Zhang Z, Li B. Prostaglandin E2 confers protection against diabetic coronary atherosclerosis by stimulating M2 macrophage polarization via the activation of the 1366 CREB/BDNF/TrkB signaling pathway. FASEB J (2020) 34:7360–71. doi: 10.1096/fj.201902055R1367
220. Sreedhar R, Arumugam S, Thandavarayan RA, Karuppagounder V, Koga Y, Nakamura T, et al. Role of 14-3-3η protein on cardiac fatty acid metabolism and macrophage 1369 polarization after high fat diet induced type 2 diabetes mellitus. Int J Biochem Cell Biol (2017) 88:92–9. doi: 10.1016/j.biocel.2017.05.0091371
221. Wang Y, Wang D, Yang L, Zhang Y. Metabolic reprogramming in the immunosuppression of tumor-associated macrophages. Chin Med J (2022) 135:2405–16. doi: 10.1097/CM9.00000000000024261374
222. Qiao X, Hu Z, Xiong F, Yang Y, Peng C, Wang D, et al. Lipid metabolism reprogramming in tumor-associated macrophages and implications for therapy. Lipids Health Dis (2023) 1376 22:45. doi: 10.1186/s12944-023-01807-11377
223. Wang S, Liu R, Yu Q, Dong L, Bi Y, Liu G. Metabolic reprogramming of macrophages during infections and cancer. Cancer Lett (2019) 452:14–22. doi: 10.1016/j.canlet.2019.03.0151379
224. Kerneur C, Cano CE, Olive D. Major pathways involved in macrophage polarization in cancer. Front Immunol (2022) 13:1026954. doi: 10.3389/fimmu.2022.1026954
225. Boutilier AJ, Elsawa SF. Macrophage polarization states in the tumor microenvironment. Int J Mol Sci (2021) 22:6995. doi: 10.3390/ijms221369951383
226. Wu H, Han Y, Rodriguez Sillke Y, Deng H, Siddiqui S, Treese C, et al. Lipid droplet-dependent fatty acid metabolism controls the immune suppressive phenotype of tumor-1385 associated macrophages. EMBO Mol Med (2019) 11:e10698. doi: 10.15252/emmm.201910698
227. Goossens P, Rodriguez-Vita J, Etzerodt A, Masse M, Rastoin O, Gouirand V, et al. Membrane cholesterol efflux drives tumor-associated macrophage reprogramming and tumor progression. Cell Metab. (2019) 29:1376–1389.e4. doi: 10.1016/j.cmet.2019.02.016
228. Prima V, Kaliberova LN, Kaliberov S, Curiel DT, Kusmartsev S. COX2/mPGES1/PGE2 pathway regulates PD-L1 expression in tumor-associated macrophages and myeloid-derived suppressor cells. Proc Natl Acad Sci USA. (2017) 114:1117–22. doi: 10.1073/pnas.1612920114
229. Sun H, Zhang L, Wang Z, Gu D, Zhu M, Cai Y, et al. Single-cell transcriptome analysis indicates fatty acid metabolism-mediated metastasis and immunosuppression in male breast cancer. Nat Commun. (2023) 14:5590. doi: 10.1038/s41467-023-41318-2
230. Zhan T, Zou Y, Han Z, Tian XR, Chen M, Liu J, et al. Single-cell sequencing combined with spatial transcriptomics reveals that the IRF7 gene in M1 macrophages inhibits the occurrence of pancreatic cancer by regulating lipid metabolism-related mechanisms. Clin Transl Med. (2024) 14:e1799. doi: 10.1002/ctm2.1799
Keywords: macrophage phenotype, lipidomic profiling, phospholipase a2 signaling, lipid remodeling, inflammaging
Citation: Rodríguez JP, Casas J, Balboa MA and Balsinde J (2025) Bioactive lipid signaling and lipidomics in macrophage polarization: Impact on inflammation and immune regulation. Front. Immunol. 16:1550500. doi: 10.3389/fimmu.2025.1550500
Received: 23 December 2024; Accepted: 28 January 2025;
Published: 14 February 2025.
Edited by:
Fabrizia Bonacina, University of Milan, ItalyReviewed by:
Toshiyuki Murai, Osaka University, JapanJeroen Bogie, University of Hasselt, Belgium
Luca De Feo, Humanitas Research Hospital, Italy
Copyright © 2025 Rodríguez, Casas, Balboa and Balsinde. This is an open-access article distributed under the terms of the Creative Commons Attribution License (CC BY). The use, distribution or reproduction in other forums is permitted, provided the original author(s) and the copyright owner(s) are credited and that the original publication in this journal is cited, in accordance with accepted academic practice. No use, distribution or reproduction is permitted which does not comply with these terms.
*Correspondence: Jesús Balsinde, amJhbHNpbmRlQHV2YS5lcw==