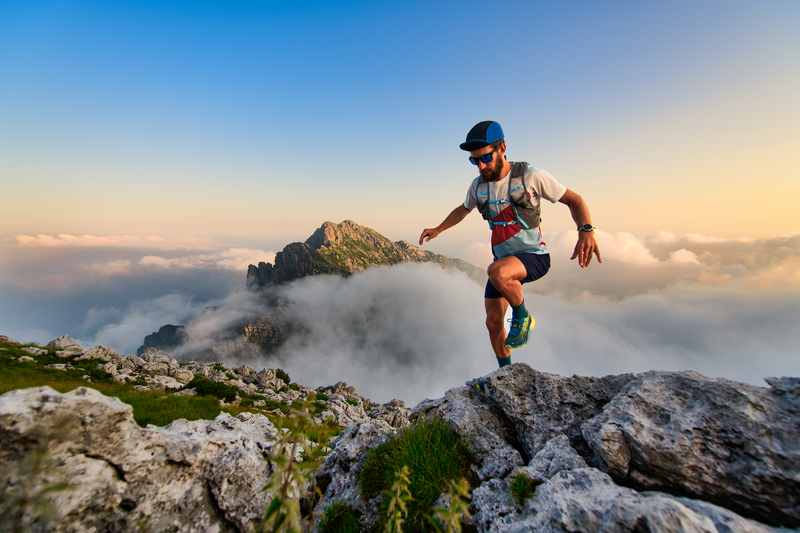
94% of researchers rate our articles as excellent or good
Learn more about the work of our research integrity team to safeguard the quality of each article we publish.
Find out more
REVIEW article
Front. Immunol. , 23 January 2025
Sec. Cancer Immunity and Immunotherapy
Volume 16 - 2025 | https://doi.org/10.3389/fimmu.2025.1542844
This article is part of the Research Topic Crosstalk in Ferroptosis, Immunity & Inflammation View all 24 articles
Ferroptosis is a novel form of cell death distinct from traditional mechanisms, characterized by the accumulation of iron ions and the production of lipid peroxides. It not only affects the survival of tumor cells but is also closely linked to changes in the tumor microenvironment. Lung cancer is one of the leading malignancies worldwide in terms of incidence and mortality, and its complex biological mechanisms and resistance make treatment challenging. Recent studies have shown that ferroptosis plays a key role in the onset and progression of lung cancer, with its intricate regulatory mechanisms influencing tumor development and response to therapy. As research into ferroptosis deepens, related molecular pathways, such as glutamate metabolism, iron metabolism, and antioxidant defense, have been gradually revealed. However, in clinical practice, ferroptosis-based therapeutic strategies for lung cancer are still in their early stages. Challenges remain, including the incomplete understanding of the specific mechanisms of ferroptosis, insufficient research on related regulatory factors, and limited insight into the interactions within the tumor microenvironment. Therefore, effective modulation of ferroptosis to enhance lung cancer treatment remains an urgent issue. This review summarizes the biological mechanisms of ferroptosis, analyzes the regulatory factors of ferroptosis in lung cancer cells and their interaction with the tumor microenvironment, and further explores potential therapeutic strategies targeting ferroptosis. By synthesizing the latest research, this paper aims to provide new perspectives and directions for lung cancer treatment, with the goal of advancing clinical applications.
Lung cancer is one of the most common cancers worldwide and has an extremely high mortality rate (1). According to global cancer statistics, the annual death toll from lung cancer has surpassed 2 million, making it one of the leading causes of cancer-related deaths (2). In some regions, the incidence and mortality rates of lung cancer continue to rise year by year, posing a significant public health challenge (3–6). The high mortality rate of lung cancer is primarily attributed to factors such as the lack of obvious early symptoms, late diagnosis, and treatment difficulties. Additionally, smoking, air pollution, occupational exposure, and other factors are considered major risk factors for lung cancer, with their impact varying significantly across different regions (7).
Traditional treatment methods for lung cancer mainly include surgery, radiotherapy, and chemotherapy. However, these approaches have significant limitations when treating advanced-stage lung cancer, especially small cell lung cancer (SCLC) and non-small cell lung cancer (NSCLC) (8). Surgical treatment is effective for early-stage cases, but in patients with locally advanced or metastatic lung cancer, chemotherapy and radiotherapy often show limited effectiveness and are accompanied by high toxic side effects (9, 10). Moreover, due to tumor heterogeneity and drug resistance, many patients still face the risk of recurrence and metastasis after receiving conventional treatments (11). Despite advancements in early screening and treatment methods for lung cancer in recent years, the prognosis remains poor, with a five-year survival rate of less than 20% (12). Therefore, there is an urgent need to explore new treatment strategies to improve the survival rate and quality of life for lung cancer patients. A comparison of the advantages and disadvantages of various lung cancer treatment methods is shown in Table 1:
Iron plays a crucial role in cellular metabolism, as it is involved not only in oxygen transport and energy metabolism, but also in DNA synthesis and repair (13). However, excessive iron accumulation can lead to an increase in reactive oxygen species (ROS) within cells, triggering oxidative stress that damages cellular DNA and other macromolecules, thereby promoting tumor initiation and progression (14, 15). In recent years, increasing evidence has shown that dysregulation of iron metabolism is closely associated with the development of various cancers, including lung cancer (16). Iron is not only a key element required for tumor cell growth but also a critical regulator of cell death. In particular, in lung cancer, abnormal iron metabolism may represent an important feature of the tumor microenvironment, influencing tumor cell growth and metastasis.
In this context, ferroptosis, a newly discovered form of programmed cell death, has gradually gained attention from researchers. Ferroptosis is a form of cell death driven by the accumulation of iron-dependent lipid peroxides, and it differs from traditional forms of cell death such as apoptosis and necrosis (17, 18). For instance, cells undergoing ferroptosis typically exhibit membrane rupture and organelle swelling, changes not typically observed in apoptosis. Ferroptosis was initially identified in tumor research, but more recent studies have indicated that it also plays a significant role in various neurodegenerative diseases, such as Alzheimer’s disease and Parkinson’s disease, as well as other pathological conditions. The mechanism of ferroptosis involves multiple biological processes, including iron metabolism, lipid metabolism, and antioxidant responses (19, 20). Importantly, ferroptosis plays a critical role in the tumor microenvironment, and inducing ferroptosis may offer novel therapeutic strategies for cancer treatment (21). By modulating iron metabolism and lipid peroxidation, ferroptosis can be induced in tumor cells, thereby inhibiting tumor growth and metastasis, and overcoming resistance to traditional therapies (22, 23).
In lung cancer, the expression profiles of GPX4 (glutathione peroxidase 4) and SLC7A11 (solute carrier family 7 member 11) are closely associated with patient prognosis. Studies have shown that high expression of GPX4 in lung adenocarcinoma (LUAD) is linked to tumor aggressiveness and drug resistance, with its expression level negatively correlated with patient survival. Similarly, high expression of SLC7A11 is considered a marker of poor prognosis in lung cancer patients. Specifically, overexpression of SLC7A11 is associated with tumor stage, lymph node metastasis, and chemotherapy resistance. Furthermore, the expression patterns of ferroptosis-related genes can serve as biomarkers to predict survival and therapeutic response in lung cancer patients. For example, one study developed a prognostic model based on ferroptosis-related genes using RNA-seq data from NSCLC patients, revealing that patients in the high-risk group had significantly lower overall survival compared to those in the low-risk group (24).
These findings indicate that ferroptosis-related genes not only play a pivotal role in the initiation and progression of lung cancer but also have potential clinical applications, providing a foundation for the development of personalized treatment strategies. Therefore, exploring the role of ferroptosis in lung cancer not only aids in understanding the biological characteristics of the disease but may also provide important insights for the development of novel therapeutic approaches.
Ferroptosis is a novel form of programmed cell death that differs significantly from traditional mechanisms of cell death in its biological characteristics. The hallmark of ferroptosis is the accumulation of iron-dependent lipid peroxides, leading to cell membrane rupture and cell death. Additionally, the morphological features of ferroptosis cells differ from other forms of cell death, typically exhibiting cell shrinkage, disruption of cell membrane integrity, and the formation of intracellular lipid vesicles (25, 26). Ferroptosis is closely associated with excessive intracellular iron accumulation and the generation of ROS, which together lead to lipid peroxidation and cellular damage. Research has shown that ferroptosis not only plays a crucial role in cancer cells but is also closely linked to the pathogenesis of various diseases, including neurodegenerative diseases, cardiovascular diseases, and kidney disorders (27–32).
The regulation of ferroptosis involves multiple molecular pathways and signaling cascades. Key molecules, such as GPX4 and SLC7A11, are central to ferroptosis regulation (Figure 1). GPX4 plays a critical protective role during ferroptosis, and its loss of function increases the cell’s susceptibility to ferroptosis. By catalyzing the reduction of lipid peroxides, GPX4 protects cells from oxidative damage and is therefore considered an inhibitor of ferroptosis. On the other hand, SLC7A11 is responsible for the uptake of cysteine, which is essential for glutathione synthesis, thereby enhancing the cell’s resistance to oxidative stress and suppressing ferroptosis.
Figure 1. Mechanism of ferroptosis. SLC7A11, the cystine transporter solute carrier family member 11; GSH, glutathione; ROS, reactive oxygen species; GPX4, glutathione peroxidase 4; mTORC1, mammalian target of rapamycin complex 1; GPD2, glycerol-3-phosphate dehydrogenase 2; DHODH, dihydroorotate dehydrogenase; CoQH2, ubiquinol; TfR, transferrin receptor; NCOA4, nuclear receptor coactivator 4; PUFA, polyunsaturated fatty acid; ACSL4, Acyl-CoA synthetase long-chain family member 4; LPCAT3, lysophosphatidylcholine acyltransferase 3; PUFA-PL, PUFA-containing phospholipids.
In lung cancer, abnormal expression of ferroptosis-related molecules is considered closely linked to tumor initiation and progression. The expression levels of GPX4 and SLC7A11 are significantly upregulated in lung cancer cells, and this upregulation correlates with the malignancy and prognosis of the tumor, making them potential therapeutic targets (33, 34). Studies have shown that high expression of SLC7A11 is closely associated with the proliferation and survival of lung cancer cells, while the expression of GPX4 is linked to chemo resistance (35). Additionally, other ferroptosis-related molecules, such as NCOA4 (nuclear receptor coactivator 4) and TXNIP (thioredoxin-interacting protein), also play important roles in ferroptosis in lung cancer (36). Therefore, targeted therapeutic strategies aimed at these molecules may offer new treatment options for lung cancer patients, particularly in the management of drug-resistant tumors (37). Furthermore, ferroptosis inducers and inhibitors affect its occurrence through different signaling pathways, further highlighting its complex biological characteristics (38).
The study of ferroptosis provides new insights into the mechanisms of cell death and its role in disease, especially in the development of therapeutic strategies for cancer and neurodegenerative disorders. Thus, a deeper understanding of the biological features and underlying mechanisms of ferroptosis is crucial for developing targeted treatments for related diseases.
The molecular mechanisms of ferroptosis primarily involve iron metabolism, lipid peroxidation, and related signaling pathways (Figure 1). Excessive iron accumulation in cells can generate ROS through the Fenton reaction, leading to lipid peroxidation (39). Lipid peroxidation is one of the core events in ferroptosis, as excessive ROS generation oxidizes polyunsaturated fatty acids (PUFAs) in cell membranes, forming lipid peroxides that further trigger cell death (40–42). Studies have shown that ferroptosis is closely related to the depletion of glutathione (GSH), an important antioxidant. A lack of GSH reduces the cell’s ability to resist oxidative stress, thereby promoting ferroptosis (43, 44). Furthermore, GSH depletion is often associated with iron overload, a state that predisposes cells to ferroptosis, which has been validated in various diseases, such as acute kidney injury and neurodegenerative diseases (45).
GPX4 is a critical regulator in preventing ferroptosis, as it inhibits ferroptosis by catalyzing the reduction of lipid peroxides (Figure 1). Therefore, dysfunction of GPX4 leads to increased ferroptosis, while iron deficiency or failure of related antioxidant systems can exacerbate this process (46, 47). Additionally, ferroptosis is also associated with the activation of several signaling pathways, with the Nrf2 pathway playing a key role in regulating the cellular antioxidant capacity (48). Recent research has increasingly highlighted the role of non-coding RNAs (such as miRNAs and lncRNAs) in the regulation of ferroptosis. These molecules can influence the process of ferroptosis by modulating the expression of related genes (49–52). In summary, the molecular mechanisms of ferroptosis are complex and involve interactions among multiple biological pathways (Figure 1). By modulating lipid metabolism and glutathione levels, new strategies for treating related diseases may emerge. Research in this field is continuously advancing, providing a theoretical foundation for clinical applications.
Oxidative stress refers to the imbalance in the cellular environment caused by excessive ROS production and insufficient antioxidant capacity, leading to cell damage and death (Figure 1). There is a close interplay between iron metabolism and oxidative stress. Iron, an essential trace element, participates in various biochemical reactions within cells, but excess iron can promote ROS generation, triggering oxidative stress (53, 54). Oxidative stress not only directly damages cell membranes and organelles but also activates several signaling pathways that further exacerbate iron accumulation and cellular damage. In diseases affecting organs such as the kidneys and liver, abnormal iron metabolism is closely related to oxidative stress, and together they promote cellular damage and dysfunction (27, 55). For instance, in conditions of iron overload, increased ROS within cells can further amplify iron accumulation, creating a vicious cycle that ultimately leads to ferroptosis (56, 57). Moreover, oxidative stress can also influence the expression of proteins involved in iron metabolism, creating a feedback loop that accelerates cell death under the combined pressures of oxidative stress and iron metabolism imbalance (58). Understanding the relationship between iron metabolism and oxidative stress is crucial for developing new therapeutic strategies to address related diseases (Figure 1).
Ferroptosis plays a complex role in tumor immunity. Recent studies have shown that ferroptosis not only serves as a form of tumor cell death but also influences immune cell functions within the tumor microenvironment. Ferroptosis can induce immunogenic cell death, and the released cellular contents (such as damage-associated molecular patterns, or DAMPs) can activate dendritic cells, thereby enhancing anti-tumor immune responses (59). However, inhibition of ferroptosis may allow tumor cells to escape immune surveillance, reducing the effectiveness of immunotherapy (60). Additionally, the combination of ferroptosis and immune checkpoint inhibition (ICI) therapy has shown potential synergistic effects, possibly improving the efficacy of cancer treatments (61). Studies suggest that ferroptosis can enhance the effects of immunotherapy by inducing immunogenic death of tumor cells and activating anti-tumor immune responses. However, the oxidative byproducts released during ferroptosis may also inhibit immune cells in the tumor microenvironment, leading to immune tolerance, which in turn affects the outcome of immunotherapy (62). For example, tumor-associated macrophages can secrete certain metabolites that promote tumor cell resistance to ferroptosis, further influencing tumor progression and the effectiveness of immunotherapy (63). Therefore, in-depth research into the role of ferroptosis in tumor immunity not only helps us understand the mechanisms of immune escape in tumors but may also provide a theoretical foundation for developing new immunotherapeutic strategies.
Iron metabolism in lung cancer cells is notably dysregulated, and this dysregulation is closely linked to the occurrence of ferroptosis. Studies show that lung cancer cells typically exhibit excessive iron accumulation, which leads to an increase in ROS, triggering lipid peroxidation and ultimately resulting in ferroptosis. In lung cancer cells, the disruption of iron metabolism is characterized by upregulation of iron transport proteins such as transferrin (Tf) and hepcidin, while the expression of ferroportin, an iron export protein, is reduced (Figure 1). These alterations affect the cell’s ability to uptake and store iron, thus promoting tumorigenesis and progression (64–66). Additionally, some studies have found abnormal expression of ferroptosis-related genes in lung cancer patients, suggesting that the alteration of iron metabolism could serve as a new therapeutic target for lung cancer treatment (67, 68). Therefore, regulating iron metabolism and enhancing ferroptosis to inhibit tumor growth may represent a novel therapeutic strategy for lung cancer.
Iron metabolism plays a crucial role in the onset and progression of lung cancer. Research indicates that dysregulated iron metabolism not only promotes tumor growth but is also closely linked to metastasis and drug resistance. The cellular state of iron (such as ferrous iron Fe2+ and ferric iron Fe3+) exerts different effects on the growth and survival of lung cancer cells. For instance, studies have shown that the accumulation of ferrous iron inhibits the proliferation of lung cancer cells, whereas ferric iron has little impact on cell growth (69). Moreover, cancer-associated fibroblasts (CAFs) regulate the iron metabolism of lung cancer cells by secreting exosomes, which in turn affect the occurrence of ferroptosis. This process involves the interaction of long non-coding RNAs (such as ROR1-AS1) with genes related to iron metabolism. Disruption of iron metabolism not only accelerates lung cancer progression but may also contribute to treatment resistance. Therefore, further investigation into the interplay between iron metabolism and lung cancer progression could provide new strategies and targets for therapy. Overall, the interaction between ferroptosis and iron metabolism offers new insights into the onset and development of lung cancer, highlighting the importance of iron as a potential therapeutic target.
Ferroptosis plays a dual role in lung cancer cell proliferation. On one hand, the induction of ferroptosis can suppress lung cancer cell proliferation. Research suggests that by regulating iron metabolism and inducing ferroptosis, the growth and spread of lung cancer cells can be effectively inhibited. For example, ubiquitin-specific protease 35 (USP35), a deubiquitinase, when overexpressed in lung cancer cells, stabilizes ferroportin and prevents ferroptosis, thus promoting cell proliferation (70). In contrast, inhibiting USP35 expression leads to an increase in ferroptosis and a suppression of lung cancer cell proliferation. On the other hand, inhibition of ferroptosis may accelerate the proliferation of lung cancer cells, which is closely associated with the malignancy and chemotherapy resistance of the tumor (71). Additionally, long non-coding RNAs (lncRNAs) also play a crucial role in regulating ferroptosis. For example, the upregulation of LUCAT1 is associated with increased lung cancer cell proliferation, while its inhibition promotes ferroptosis. Therefore, modulating ferroptosis pathways may offer new therapeutic approaches for lung cancer, particularly in overcoming chemotherapy resistance, by inducing ferroptosis to enhance tumor cell sensitivity to treatment (71, 72).
Ferroptosis also plays an important role in the metastasis of lung cancer. Studies have found that ferroptosis can promote lung cancer metastasis by modulating the tumor microenvironment and affecting immune cell function. For example, the occurrence of ferroptosis may lead to the release of pro-inflammatory cytokines from tumor cells, which alters the tumor microenvironment and influences the metastatic capacity of the cancer (19). Some studies suggest that after ferroptosis, immune cells such as macrophages and neutrophils in the tumor microenvironment become activated and release pro-inflammatory factors, further promoting tumor growth and metastasis (71, 73). Moreover, ferroptosis may influence the epithelial-mesenchymal transition (EMT) process in tumor cells, thus enhancing the invasiveness and metastatic potential of lung cancer cells (74). Research indicates that inhibiting ferroptosis could aid in suppressing lung cancer cell metastasis. For instance, CAFs secrete lncRNAs (such as ROR1-AS1) in exosomes to inhibit ferroptosis in lung cancer cells, thereby promoting metastasis (75). Furthermore, the dysregulation of iron metabolism is closely associated with the metastatic ability of tumors. Iron accumulation not only promotes tumor cell proliferation but also enhances oxidative stress and induces inflammation, which can further promote metastasis (76). Therefore, therapeutic strategies targeting ferroptosis may help inhibit lung cancer metastasis and improve patient prognosis. By modulating iron metabolism and inducing ferroptosis, new anti-metastasis therapies could be developed, offering better treatment options for lung cancer patients.
Ferroptosis is an iron-dependent form of cell death, characterized by the accumulation of lipid peroxides in the cell membrane. The generation of lipid peroxides is closely linked to intracellular iron levels, with excessive iron accumulation driving the production of ROS through the Fenton reaction, which, in turn, exacerbates lipid peroxidation (Figure 1). Studies have shown that the sensitivity to ferroptosis is regulated by various lipid metabolic enzymes, such as long-chain acyl-CoA synthetase 4 (ACSL4) and lipoxygenases (LOX) (Figure 1). These enzymes play critical roles at different stages of lipid peroxidation, influencing the cell’s response to ferroptosis. For example, an increase in ACSL4 expression promotes the accumulation of polyunsaturated fatty acids (PUFAs), which enhances the sensitivity of cells to ferroptosis (Figure 1). The regulation of these metabolic enzymes not only affects cell survival but is also closely associated with the development of various diseases, including cancer and neurodegenerative disorders (77, 78). Furthermore, the presence of PUFAs in the cell membrane significantly impacts the occurrence of ferroptosis, as these PUFAs are highly susceptible to peroxidation, leading to membrane damage and cell death (39). Therefore, regulating the activity of lipid metabolic enzymes and maintaining the balance of lipid components are crucial strategies for modulating ferroptosis, providing new insights and targets for the treatment of related diseases (79–81).
Antioxidants play a crucial role in the regulation of ferroptosis. The occurrence of ferroptosis is often accompanied by the dysregulation of the intracellular antioxidant system, particularly the loss of function of GPX4, which significantly reduces the cell’s ability to defend against lipid peroxidation (Figure 1). For example, in the tumor microenvironment, the imbalance of antioxidants can lead to the accumulation of iron and the increase of lipid peroxides, thereby inducing ferroptosis. Therefore, the role of antioxidants is highly dependent on the cellular environment and specific physiological and pathological conditions (82, 83). Research has shown that antioxidants such as vitamin E and other natural compounds can effectively inhibit the occurrence of ferroptosis by enhancing the cell’s antioxidant capacity and mitigating the impact of lipid peroxidation. Additionally, nuclear factor erythroid 2-related factor 2 (Nrf2), a key transcription factor, can regulate the expression of antioxidant genes within the cell, thereby influencing the sensitivity to ferroptosis. Thus, utilizing antioxidants to boost the cell’s antioxidant capacity may become a novel strategy for treating diseases associated with ferroptosis (84–86).
The tumor microenvironment is one of the key regulators of ferroptosis. The metabolic characteristics of tumor cells in this environment, such as hypoxia, acidity, and nutrient deprivation, can influence iron metabolism and the oxidative state of lipids, thereby modulating the sensitivity to ferroptosis (87). Studies have shown that the interactions between immune cells and tumor cells in the tumor microenvironment may affect tumor progression and metastasis by regulating ferroptosis pathways. For example, certain immune cells in the tumor microenvironment might promote ferroptosis in tumor cells or help them resist ferroptosis through the release of cytokines and metabolic byproducts. Moreover, tumor cells’ ability to adapt to ferroptosis may contribute to resistance to anticancer therapies. Additionally, the high iron concentration and oxidative stress present in the tumor microenvironment can also promote the occurrence of ferroptosis, a mechanism that plays a crucial role in tumor progression and metastasis. Therefore, further research into the influence of the tumor microenvironment on ferroptosis will not only help to understand tumor biology but also provide a basis for the development of new therapeutic strategies (81, 88, 89).
Ferroptosis is a newly identified form of programmed cell death, primarily driven by iron-dependent lipid peroxidation, leading to cellular death. In contrast, apoptosis is executed through intracellular signaling pathways, particularly via the activation of caspases. Therefore, ferroptosis differs significantly from classical apoptosis. Studies have shown a complex interplay between ferroptosis and apoptosis. On one hand, in certain contexts, ferroptosis can induce apoptosis by promoting the generation of ROS within the cell. For instance, lncRNA LINC00618 has been found to promote both apoptosis and ferroptosis in leukemia cells, suggesting an interaction between these two cell death mechanisms (90). On the other hand, ferroptosis may inhibit apoptosis. For example, in some cancer cells, the activation of ferroptosis may suppress apoptotic pathways, thereby affecting tumor growth and metastasis (91). Furthermore, in some cases, ferroptosis may occur prior to apoptosis, resulting in cell death before apoptotic signals emerge (92). Thus, a deeper understanding of the interaction between ferroptosis and apoptosis not only helps unravel the complexity of cell death mechanisms but may also provide new avenues for cancer therapy (93).
Necroptosis is a regulated form of necrosis, typically triggered by intracellular death receptor signaling, and shares morphological and biochemical features with ferroptosis. Both processes involve rupture of the cell membrane and the release of cellular contents, triggering inflammatory responses (94). Research has shown that ferroptosis and necroptosis interact in certain contexts. For instance, iron overload can promote necroptosis, and the activation of necroptosis can exacerbate ferroptosis by promoting iron release (95). Excessive iron accumulation can not only induce ferroptosis but may also promote necroptotic cell death by affecting mitochondrial function and the integrity of the cell membrane (96). Additionally, certain inhibitors, such as KW-2449 and Necrostatin-1, have been found to suppress both cell death pathways simultaneously, suggesting that they may share common regulatory mechanisms. In pathological conditions like ischemia-reperfusion injury, ferroptosis and necroptosis work together in the cellular damage process, regulating the type and extent of cell death (97). Therefore, combined intervention targeting these two forms of cell death may offer new strategies for treating related diseases.
Autophagy is a cellular self-degradation process that clears damaged organelles and proteins, maintaining intracellular homeostasis (Figure 2). Recent studies have revealed that autophagy plays a crucial role in promoting ferroptosis. By regulating intracellular iron levels and lipid metabolism, autophagy facilitates the occurrence of ferroptosis. For example, autophagy has been shown to increase the levels of free iron in cells by degrading ferritin, the iron storage protein, thereby accelerating lipid peroxidation and leading to ferroptosis (98). Additionally, oxidative stress is considered a key factor in autophagy-induced ferroptosis. Research indicates that ROS can activate autophagy, further promoting the onset of ferroptosis (99). Therefore, autophagy is not only an important regulatory factor in ferroptosis but also a protective mechanism in cells responding to iron overload and oxidative stress. Inhibition of autophagy significantly impacts ferroptosis. Studies have shown that when autophagy is suppressed, cells become less sensitive to ferroptosis. For example, in cells lacking autophagy-related genes, iron accumulation and lipid peroxidation levels are significantly reduced, thus protecting the cells from ferroptosis (98). Moreover, autophagy inhibitors like 3-methyladenine (3-MA) reduce cell sensitivity to ferroptosis inducers, suggesting that autophagy plays a critical role in regulating ferroptosis (100). In certain pathological conditions, such as atherosclerosis, there is a close interplay between autophagy inhibition and ferroptosis activation, where autophagy suppression alleviates ferroptosis-related cellular damage (101). Therefore, modulating autophagic activity may provide new therapeutic strategies for diseases associated with ferroptosis.
Figure 2. Mechanism diagram of autophagy and cuproptosis. SLC7A11, the cystine transporter solute carrier family member 11; GSH, glutathione; GPX4, glutathione peroxidase 4.
Cuproptosis is a form of cell death induced by excessive copper, involving mitochondrial dysfunction and protein aggregation (102). Studies have shown that there is a complex interaction between these two cell death mechanisms (Figure 2). For example, copper can enhance ferroptosis by promoting the degradation of GPX4 (103). Additionally, copper deficiency increases cellular sensitivity to ferroptosis, suggesting a metabolic crossover between iron and copper. This may occur through the regulation of antioxidant mechanisms and lipid metabolism, thereby influencing each other (104). Understanding this metabolic intersection is crucial for uncovering the regulatory mechanisms of cell death and its role in pathological conditions such as cancer and neurodegenerative diseases. The crosstalk between ferroptosis and cuproptosis involves multiple signaling pathways (Figure 2). Research has found that copper accumulation can influence the occurrence of ferroptosis by modulating iron metabolism pathways within mitochondria. This mechanism includes increasing intracellular iron levels by inhibiting enzymes associated with iron metabolism (105). Meanwhile, ferroptosis inducers such as erastin can enhance copper-dependent lipid acylation and protein aggregation, thereby promoting the occurrence of cuproptosis (102). Furthermore, the interaction between iron and copper may also affect cell death fate by regulating intracellular ROS levels. Excessive ROS accumulation causes cellular damage and may promote cell death through the activation of various signaling pathways (106). Therefore, in-depth exploration of the interactions between these signaling pathways could help develop combined therapeutic strategies targeting both ferroptosis and cuproptosis.
As emerging mechanisms of cell death, ferroptosis and cuproptosis show great potential in cancer therapy (Figure 2). By targeting and inducing either ferroptosis or cuproptosis, researchers hope to overcome tumor resistance to conventional treatments. For instance, combining ferroptosis inducers with chemotherapy or immunotherapy drugs could significantly enhance anti-tumor efficacy (107). Additionally, copper ion carriers and copper compounds are also being explored as new therapeutic strategies, especially for tumor types that are resistant to standard treatments (108). Moreover, the interaction between ferroptosis and cuproptosis provides new insights for cancer therapy. Studies suggest that targeting both cell death mechanisms simultaneously may yield a synergistic effect, improving treatment outcomes (109). Therefore, a deeper understanding of the mechanisms underlying ferroptosis and cuproptosis and their applications in cancer therapy may offer new treatment options for cancer patients.
The comparison table of ferroptosis and other cell death modes is shown in Table 2.
In recent years, the development of ferroptosis inducers has emerged as a novel strategy for cancer treatment. Numerous studies have shown that ferroptosis inducers can effectively induce ferroptosis in tumor cells by targeting iron metabolism and lipid peroxidation, thereby inhibiting tumor growth. In clinical applications, ferroptosis inducers have demonstrated the potential to overcome chemotherapy resistance in certain cancers (111, 112). For example, small molecules such as Erastin, RSL3, and FIN56 have been proven to induce ferroptosis by inhibiting GPX4, offering a new approach for cancer treatment (113, 114).
Furthermore, advances in nanotechnology have made it possible to target the delivery of ferroptosis inducers, enhancing the bioavailability and therapeutic efficacy of these drugs while reducing toxicity to normal cells (115, 116). Researchers are exploring the use of nanocarriers to improve the specificity and effectiveness of ferroptosis inducers, thus achieving precise targeting of tumor cells (117). Additionally, strategies targeting iron-sulfur clusters (ISCs) have been proposed to induce ferroptosis in cancer cells. By modulating intracellular iron metabolism and lipid peroxidation, ferroptosis inducers are expected to address the issue of resistance in cancer therapies (118, 119). These advances offer new perspectives and possibilities for the clinical application of ferroptosis inducers. A comparison of various ferroptosis inducers is shown in Figure 3.
Combination therapy strategies have shown promising prospects in cancer treatment, particularly when ferroptosis inducers are used in conjunction with other therapeutic modalities. This is because inducing ferroptosis can enhance the sensitivity of tumor cells to other treatments while reducing immune suppression in the tumor microenvironment (120). For example, combining ferroptosis inducers with immunotherapy or targeted therapy has been shown to significantly improve treatment outcomes. Ferroptosis can overcome the resistance of cancer cells to conventional therapies, especially in highly heterogeneous tumors such as glioblastoma and melanoma (121, 122). Studies have found that ferroptosis inducers can enhance the antitumor activity of BRAF inhibitors and immune checkpoint inhibitors, thereby improving the prognosis of patients with malignant melanoma (123).
Radiotherapy combined with ferroptosis inducers has also demonstrated enhanced efficacy, as radiotherapy promotes ferroptosis by increasing intracellular iron accumulation (124). Specifically, radiotherapy induces ROS and activates lipid metabolism enzymes (such as ACSL4), which in turn promote ferroptosis. Moreover, the induction of ferroptosis can enhance the effects of radiotherapy, increasing tumor cell sensitivity and improving treatment outcomes. For instance, certain ferroptosis inducers, when used alongside radiotherapy, significantly enhance the antitumor effects of radiotherapy by increasing the accumulation of lipid peroxides inside cells (125, 126). Clinical studies have shown that combining ferroptosis inducers with radiotherapy can significantly inhibit tumor growth and overcome resistance to radiotherapy in some cancers (127–129).
In the context of chemotherapy, ferroptosis is also considered a novel strategy for overcoming chemotherapy resistance by enhancing the cytotoxic effects of chemotherapeutic agents. Research has shown that combining chemotherapy drugs like cisplatin with ferroptosis inducers can reverse tumor cell resistance, thereby improving the efficacy of chemotherapy (130, 131). This combination strategy not only increases the tumor cell death rate but may also improve patient prognosis, especially in the case of refractory cancers (132, 133). This approach not only overcomes the resistance associated with single therapies but also targets tumor cells through multiple mechanisms, thus enhancing overall treatment efficacy (134).
Studies have also shown that combining therapies with different mechanisms can effectively improve patient response rates and reduce the risk of tumor recurrence (135). However, the specific mechanisms, optimal dosages, and treatment regimens for combination therapy still require further clinical trials and basic research to determine, in order to achieve better therapeutic outcomes and minimize side effects. Therefore, research into the combination of ferroptosis inducers with traditional therapies offers new treatment options and improved prognosis for cancer patients (136).
Currently, therapeutic strategies targeting ferroptosis have made significant progress in preclinical and clinical research. Several studies have validated the antitumor effects of ferroptosis inducers in animal models, demonstrating their potential applications across different cancer types. For example, research on colorectal cancer has shown that ferroptosis inducers can effectively overcome chemotherapy resistance and significantly increase tumor cell death rates (137, 138). In terms of clinical research, although still in its early stages, some small-scale clinical trials are assessing the safety and efficacy of ferroptosis inducers. These studies lay the foundation for future clinical applications and highlight the potential of ferroptosis as a novel therapeutic target (139). Furthermore, these studies not only focus on the direct role of ferroptosis in cancer therapy but also explore its synergistic effects in radiotherapy and immunotherapy (140). While the current findings are promising, more clinical trials are needed to validate the practical effects and indications of ferroptosis-targeted therapy in different cancer types. As our understanding of ferroptosis mechanisms deepens and related drugs continue to be developed, ferroptosis-targeted therapies are expected to become a new option for cancer treatment in the near future.
Research has also explored the impact of modulating ferroptosis-related genes on the prognosis of lung cancer patients, with results indicating that specific ferroptosis-related genes could serve as prognostic biomarkers to predict patient survival. Additionally, clinical trials are exploring strategies to combine ferroptosis inducers with traditional chemotherapy drugs to overcome resistance in lung cancer. Although clinical applications targeting ferroptosis are still in their infancy, the available research results provide a foundation for future clinical trials, suggesting that modulation of ferroptosis could become a new direction for lung cancer treatment.
Ferroptosis, as a novel targeted therapy strategy, demonstrates potential in the treatment of lung cancer. Studies indicate that lung cancer cells are particularly sensitive to ferroptosis, especially in cases of chemotherapy resistance, making ferroptosis induction a promising therapeutic approach (141). For example, certain natural compounds have been found to enhance the effectiveness of chemotherapy for lung cancer by inducing ferroptosis, offering a new solution to chemotherapy resistance. Additionally, researchers are developing new ferroptosis inducers that not only exhibit good selectivity and bioavailability but can also be combined with other treatments such as radiotherapy and immunotherapy to improve therapeutic outcomes. In conclusion, ferroptosis, as a new direction in targeted therapy, holds great promise in the future treatment of lung cancer, particularly in overcoming resistance and enhancing treatment efficacy.
In this review, we explored the significance and mechanisms of ferroptosis in lung cancer, emphasizing its role in the tumor microenvironment and its impact on the survival and proliferation of lung cancer cells. Ferroptosis, an iron-dependent form of programmed cell death, has been shown to play a crucial role in various cancer types, particularly in lung cancer. Its importance is highlighted by the complex relationship between ferroptosis and tumor cell survival, proliferation, and drug resistance. Research suggests that ferroptosis not only influences tumor cell survival and proliferation by regulating intracellular iron homeostasis, lipid peroxidation, and antioxidant responses, but it may also alter the sensitivity of tumors to conventional therapies. These findings shed light on the complexity of ferroptosis and its feasibility as a therapeutic target in lung cancer.
Targeting ferroptosis offers a novel perspective for lung cancer treatment. Studies have shown that promoting ferroptosis can effectively suppress tumor cell growth while enhancing responses to chemotherapy and immunotherapy. The potential of this new therapeutic strategy is particularly evident in lung cancer cases resistant to existing treatments. Therefore, the development of drugs or therapies targeting iron metabolism could become a significant direction in lung cancer treatment, warranting further attention from clinical researchers and drug developers. Future research should focus on further elucidating the molecular mechanisms of ferroptosis and its role in the heterogeneity of lung cancer. Additionally, exploring the differences in ferroptosis characteristics between different types of lung cancer (e.g., small cell lung cancer and non-small cell lung cancer) and combining it with other therapeutic approaches will be an important part of future studies. Moreover, understanding the complex interactions between ferroptosis and the tumor microenvironment is crucial for identifying new biomarkers and therapeutic targets.
However, despite the clear potential of ferroptosis in lung cancer treatment, we must be cautious in interpreting existing research results. The factors influencing ferroptosis and the associated signaling pathways vary across different experimental conditions, cell models, and animal studies. There are also some discrepancies in the mechanisms of ferroptosis and its role in lung cancer across different studies. Therefore, it is essential to adopt more standardized experimental designs and conduct multi-center clinical trials to validate the true potential of ferroptosis in lung cancer therapy. Future research should focus on clarifying the molecular mechanisms of ferroptosis and how to effectively leverage this mechanism in clinical practice. Exploring the regulatory factors of ferroptosis in different tumor microenvironments and assessing its combined use with other therapeutic strategies will be key areas of future research. Additionally, developing new ferroptosis inducers and screening biomarkers to predict patients’ responses to ferroptosis-targeted therapies will provide important insights for personalized treatment. Future studies need to be more systematic and in-depth to unravel the complex mechanisms of ferroptosis in the occurrence, progression, and outcome of lung cancer. This will not only enhance our understanding of tumor biology but also lay the foundation for the development of new targeted therapeutic strategies.
Ferroptosis-related therapies hold great potential for clinical application but also face several challenges. Balancing the promotion and inhibition of ferroptosis, ensuring the selectivity and safety of these therapies, is a critical issue that researchers must address. Furthermore, individual differences and the complexity of the tumor microenvironment will affect the clinical efficacy of ferroptosis-based treatments. Therefore, future research should emphasize interdisciplinary collaboration, combining basic research with clinical trials, to facilitate the translation of ferroptosis-related therapies into clinical practice.
In conclusion, ferroptosis, as a novel strategy for lung cancer treatment, shows broad developmental prospects. By integrating multidisciplinary research efforts and delving deeper into the mechanisms of ferroptosis and its potential for clinical application, we hope to provide more effective treatment options for lung cancer patients.
GG: Writing – original draft. XZ: Conceptualization, Writing – review & editing.
The author(s) declare that no financial support was received for the research, authorship, and/or publication of this article.
We appreciate the convenience provided by FigDraw in Figure 2.
The authors declare that the research was conducted in the absence of any commercial or financial relationships that could be construed as a potential conflict of interest.
The author(s) declare that no Generative AI was used in the creation of this manuscript.
All claims expressed in this article are solely those of the authors and do not necessarily represent those of their affiliated organizations, or those of the publisher, the editors and the reviewers. Any product that may be evaluated in this article, or claim that may be made by its manufacturer, is not guaranteed or endorsed by the publisher.
1. Thandra KC, Barsouk A, Saginala K, Aluru JS, Barsouk A. Epidemiology of lung cancer. Contemp Oncol (Pozn). (2021) 25:45–52. doi: 10.5114/wo.2021.103829
2. Chauhan P, Pandey P, Ramniwas S, Khan F, Maqsood R. Deciphering the correlation between the emergence of lung carcinoma associated with tuberculosis-related inflammation. Endocr Metab Immune Disord Drug Targets. (2024) 25:291–9. doi: 10.2174/0118715303301146240522095638
3. Oliver AL. Lung cancer: epidemiology and screening. Surg Clin North Am. (2022) 102:335–44. doi: 10.1016/j.suc.2021.12.001
4. Salehiniya H, Bahadori M, Ghanizadeh G, Raei M. Epidemiological study of lung cancer in Iran: A systematic review. Iran J Public Health. (2022) 51:306–17. doi: 10.18502/ijph.v51i2.8683
5. Miranda-Filho A, Piñeros M, Bray F. The descriptive epidemiology of lung cancer and tobacco control: a global overview 2018. Salud Publica Mex. (2019) 61:219–29. doi: 10.21149/10140
6. Sun H, Zhang H, Cai H, Yuan W, Wang F, Jiang Y, et al. Burden of lung cancer in China, 1990-2019: findings from the global burden of disease study 2019. Cancer Control. (2023) 30:10732748231198749. doi: 10.1177/10732748231198749
7. Barta JA, Powell CA, Wisnivesky JP. Global epidemiology of lung cancer. Ann Glob Health. (2019) 85:8. doi: 10.5334/aogh.2419
8. Zou K, Sun P, Huang H, Zhuo H, Qie R, Xie Y, et al. Etiology of lung cancer: Evidence from epidemiologic studies. J Natl Cancer Cent. (2022) 2:216–25. doi: 10.1016/j.jncc.2022.09.004
9. Lin H-T, Liu F-C, Wu C-Y, Kuo C-F, Lan W-C, Yu H-P. Epidemiology and survival outcomes of lung cancer: A population-based study. BioMed Res Int. (2019) 2019:8148156. doi: 10.1155/2019/8148156
10. Huang J, Deng Y, Tin MS, Lok V, Ngai CH, Zhang L, et al. Distribution, risk factors, and temporal trends for lung cancer incidence and mortality: A global analysis. Chest. (2022) 161:1101–11. doi: 10.1016/j.chest.2021.12.655
11. Zumsteg ZS, Luu M, Rosenberg PS, Elrod JK, Bray F, Vaccarella S, et al. Global epidemiologic patterns of oropharyngeal cancer incidence trends. J Natl Cancer Inst. (2023) 115:1544–54. doi: 10.1093/jnci/djad169
12. Lu T, Yang X, Huang Y, Zhao M, Li M, Ma K, et al. Trends in the incidence, treatment, and survival of patients with lung cancer in the last four decades. Cancer Manag Res. (2019) 11:943–53. doi: 10.2147/CMAR.S187317
13. Luo L, Wang H, Tian W, Zeng J, Huang Y, Luo H. Targeting ferroptosis for cancer therapy: iron metabolism and anticancer immunity. Am J Cancer Res. (2021) 11(11):5508–25.
14. Forciniti S, Greco L, Grizzi F, Malesci A, Laghi L. Iron metabolism in cancer progression. Int J Mol Sci. (2020) 21:2257. doi: 10.3390/ijms21062257
15. Zhang Y-Y, Han Y, Li W-N, Xu R-H, Ju H-Q. Tumor iron homeostasis and immune regulation. Trends Pharmacol Sci. (2024) 45:145–56. doi: 10.1016/j.tips.2023.12.003
16. Kuang Y, Wang Q. Iron and lung cancer. Cancer Lett. (2019) 464:56–61. doi: 10.1016/j.canlet.2019.08.007
17. Wang W, Xie Y, Malhotra A. Potential of curcumin and quercetin in modulation of premature mitochondrial senescence and related changes during lung carcinogenesis. J Environ Pathol Toxicol Oncol. (2021) 40:53–60. doi: 10.1615/JEnvironPatholToxicolOncol.2021039371
18. Andreani C, Bartolacci C, Scaglioni PP. Ferroptosis: A specific vulnerability of RAS-driven cancers? Front Oncol. (2022) 12:923915. doi: 10.3389/fonc.2022.923915
19. Li Y, Tuerxun H, Zhao Y, Liu X, Li X, Wen S, et al. The new era of lung cancer therapy: Combining immunotherapy with ferroptosis. Crit Rev Oncol Hematol. (2024) 198:104359. doi: 10.1016/j.critrevonc.2024.104359
20. Sheng X-H, Han L-C, Gong A, Meng X-S, Wang X-H, Teng L-S, et al. Discovery of novel ortho-aminophenol derivatives targeting lipid peroxidation with potent antiferroptotic activities. J Med Chem. (2024) 67:9536–51. doi: 10.1021/acs.jmedchem.4c00600
21. Walters R, Mousa SA. Modulations of ferroptosis in lung cancer therapy. Expert Opin Ther Targets. (2022) 26:133–43. doi: 10.1080/14728222.2022.2032651
22. Lei H, Hou G, Liu L, Pei Z, Chen Y, Lu Y, et al. A two-pronged nanostrategy of iron metabolism disruption to synergize tumor therapy by triggering the paraptosis-apoptosis hybrid pathway. ACS Nano. (2024) 18:22257–74. doi: 10.1021/acsnano.4c06199
23. Zhao Y-Y, Lian J-X, Lan Z, Zou K-L, Wang W-M, Yu G-T. Ferroptosis promotes anti-tumor immune response by inducing immunogenic exposure in HNSCC. Oral Dis. (2023) 29:933–41. doi: 10.1111/odi.14077
24. Liu T, Luo H, Zhang J, Hu X, Zhang J. Molecular identification of an immunity- and Ferroptosis-related gene signature in non-small cell lung Cancer. BMC Cancer. (2021) 21:783. doi: 10.1186/s12885-021-08541-w
25. Chen H-X, Wu Y-P, Li W, Shen H-H, Chen Z-H. Ferroptosis in respiratory diseases. Sheng Li Xue Bao. (2020) 72:575–85. doi: 10.13294/j.aps.2020.0064
26. Naderi S, Khodagholi F, Janahmadi M, Motamedi F, Torabi A, Batool Z, et al. Ferroptosis and cognitive impairment: Unraveling the link and potential therapeutic targets. Neuropharmacology. (2025) 263:110210. doi: 10.1016/j.neuropharm.2024.110210
27. Wang F, He J, Xing R, Sha T, Sun B. Molecular mechanisms of ferroptosis and their role in inflammation. Int Rev Immunol. (2023) 42:71–81. doi: 10.1080/08830185.2021.2016739
28. Lei J, Chen Z, Song S, Sheng C, Song S, Zhu J. Insight into the role of ferroptosis in non-neoplastic neurological diseases. Front Cell Neurosci. (2020) 14:231. doi: 10.3389/fncel.2020.00231
29. Feng Q, Yu X, Qiao Y, Pan S, Wang R, Zheng B, et al. Ferroptosis and acute kidney injury (AKI): molecular mechanisms and therapeutic potentials. Front Pharmacol. (2022) 13:858676. doi: 10.3389/fphar.2022.858676
30. Feng Q, Yang Y, Ren K, Qiao Y, Sun Z, Pan S, et al. Broadening horizons: the multifaceted functions of ferroptosis in kidney diseases. Int J Biol Sci. (2023) 19:3726–43. doi: 10.7150/ijbs.85674
31. Wang H, Yu X, Liu D, Qiao Y, Huo J, Pan S, et al. VDR activation attenuates renal tubular epithelial cell ferroptosis by regulating nrf2/HO-1 signaling pathway in diabetic nephropathy. Adv Sci (Weinh). (2024) 11:e2305563. doi: 10.1002/advs.202305563
32. Li S, Han Q, Liu C, Wang Y, Liu F, Pan S, et al. Role of ferroptosis in chronic kidney disease. Cell Commun Signal. (2024) 22:113. doi: 10.1186/s12964-023-01422-8
33. Yang H, Zhao L, Gao Y, Yao F, Marti TM, Schmid RA, et al. Pharmacotranscriptomic analysis reveals novel drugs and gene networks regulating ferroptosis in cancer. Cancers (Basel). (2020) 12:3273. doi: 10.3390/cancers12113273
34. Lu C, Cai Y, Liu W, Peng B, Liang Q, Yan Y, et al. Aberrant expression of KDM1A inhibits ferroptosis of lung cancer cells through up-regulating c-Myc. Sci Rep. (2022) 12:19168. doi: 10.1038/s41598-022-23699-4
35. Wen MG, Zheng HX, Zhao YZ, Xia P. Distinct roles and molecular mechanisms of nicotine and benzo(a)pyrene in ferroptosis of lung adenocarcinoma and lung squamous cell carcinoma. Tob Induc Dis. (2024) 22:121. doi: 10.18332/tid/189490
36. Zheng Y, Yang W, Wu W, Jin F, Lu D, Gao J, et al. Diagnostic and predictive significance of the ferroptosis-related gene TXNIP in lung adenocarcinoma stem cells based on multi-omics. Transl Oncol. (2024) 45:101926. doi: 10.1016/j.tranon.2024.101926
37. Xie B, Chen Q, Dai Z, Jiang C, Sun J, Guan A, et al. Prognostic significance of a 3-gene ferroptosis-related signature in lung cancer via LASSO analysis and cellular functions of UBE2Z. Comput Biol Chem. (2024) 113:108192. doi: 10.1016/j.compbiolchem.2024.108192
38. Liu J. Research advances in the understanding of how exosomes regulate ferroptosis in cancer. Clin Transl Oncol. (2023) 25:1906–15. doi: 10.1007/s12094-023-03089-6
39. Li Y, Zhang H, Yang F, Zhu D, Chen S, Wang Z, et al. Mechanisms and therapeutic potential of disulphidptosis in cancer. Cell Prolif. (2024) 58:e13752. doi: 10.1111/cpr.13752
40. Balakrishnan M, Kenworthy AK. Lipid peroxidation drives liquid-liquid phase separation and disrupts raft protein partitioning in biological membranes. J Am Chem Soc. (2024) 146:1374–87. doi: 10.1021/jacs.3c10132
41. Su L-J, Zhang J-H, Gomez H, Murugan R, Hong X, Xu D, et al. Reactive oxygen species-induced lipid peroxidation in apoptosis, autophagy, and ferroptosis. Oxid Med Cell Longev. (2019) 2019:5080843. doi: 10.1155/2019/5080843
42. Dai Z, Zhang W, Zhou L, Huang J. Probing lipid peroxidation in ferroptosis: emphasizing the utilization of C11-BODIPY-based protocols. Methods Mol Biol. (2023) 2712:61–72. doi: 10.1007/978-1-0716-3433-2_6
43. Liu M, Kong X-Y, Yao Y, Wang X-A, Yang W, Wu H, et al. The critical role and molecular mechanisms of ferroptosis in antioxidant systems: a narrative review. Ann Transl Med. (2022) 10:368. doi: 10.21037/atm-21-6942
44. Hu X, Xu Y, Xu H, Jin C, Zhang H, Su H, et al. Progress in understanding ferroptosis and its targeting for therapeutic benefits in traumatic brain and spinal cord injuries. Front Cell Dev Biol. (2021) 9:705786. doi: 10.3389/fcell.2021.705786
45. Ma S, Adzavon YM, Wen X, Zhao P, Xie F, Liu M, et al. Novel insights in the regulatory mechanisms of ferroptosis in hepatocellular carcinoma. Front Cell Dev Biol. (2022) 10:873029. doi: 10.3389/fcell.2022.873029
46. Lai L, Tan M, Hu M, Yue X, Tao L, Zhai Y, et al. Important molecular mechanisms in ferroptosis. Mol Cell Biochem. (2024) 24:5009. doi: 10.1007/s11010-024-05009-w
47. Liu W, Zhang F, Yang K, Yan Y. Comprehensive analysis regarding the prognostic significance of downregulated ferroptosis-related gene AKR1C2 in gastric cancer and its underlying roles in immune response. PloS One. (2023) 18:e0280989. doi: 10.1371/journal.pone.0280989
48. Ma T-L, Zhou Y, Wang C, Wang L, Chen J-X, Yang H-H, et al. Targeting ferroptosis for lung diseases: exploring novel strategies in ferroptosis-associated mechanisms. Oxid Med Cell Longev. (2021) 2021:1098970. doi: 10.1155/2021/1098970
49. Shaghaghi Z, Motieian S, Alvandi M, Yazdi A, Asadzadeh B, Farzipour S, et al. Ferroptosis inhibitors as potential new therapeutic targets for cardiovascular disease. Mini Rev Med Chem. (2022) 22:2271–86. doi: 10.2174/1389557522666220218123404
50. Xu C, Liu Z, Xiao J. Ferroptosis: A double-edged sword in gastrointestinal disease. Int J Mol Sci. (2021) 22:12403. doi: 10.3390/ijms222212403
51. Zuo Y-B, Zhang Y-F, Zhang R, Tian J-W, Lv X-B, Li R, et al. Ferroptosis in cancer progression: role of noncoding RNAs. Int J Biol Sci. (2022) 18:1829–43. doi: 10.7150/ijbs.66917
52. Zhang J, Guo C. Current progress of ferroptosis in cardiovascular diseases. Front Cardiovasc Med. (2023) 10:1259219. doi: 10.3389/fcvm.2023.1259219
53. Galaris D, Barbouti A, Pantopoulos K. Iron homeostasis and oxidative stress: An intimate relationship. Biochim Biophys Acta Mol Cell Res. (2019) 1866:118535. doi: 10.1016/j.bbamcr.2019.118535
54. Gensluckner S, Wernly B, Datz C, Aigner E. Iron, oxidative stress, and metabolic dysfunction-associated steatotic liver disease. Antioxidants (Basel). (2024) 13:208. doi: 10.3390/antiox13020208
55. Cui F, Mi H, Wang R, Du Y, Li F, Chang S, et al. The effect of chronic intermittent hypobaric hypoxia improving liver damage in metabolic syndrome rats through ferritinophagy. Pflugers Arch. (2023) 475:1251–63. doi: 10.1007/s00424-023-02860-6
56. Zhang M, Gao J, Deng G, Guo C, Bi Y, Guo D. Regulation of plant iron homeostasis by abscisic acid: a review. Sheng Wu Gong Cheng Xue Bao. (2022) 38:2725–37. doi: 10.13345/j.cjb.220072
57. Emri T, Antal K, Varga K, Gila BC, Pócsi I. The oxidative stress response highly depends on glucose and iron availability in aspergillus fumigatus. J Fungi (Basel). (2024) 10:221. doi: 10.3390/jof10030221
58. Zhang J, Song J, Liu S, Zhang Y, Qiu T, Jiang L, et al. m6A methylation-mediated PGC-1α contributes to ferroptosis via regulating GSTK1 in arsenic-induced hepatic insulin resistance. Sci Total Environ. (2023) 905:167202. doi: 10.1016/j.scitotenv.2023.167202
59. Zhai X, Lin Y, Zhu L, Wang Y, Zhang J, Liu J, et al. Ferroptosis in cancer immunity and immunotherapy: Multifaceted interplay and clinical implications. Cytokine Growth Factor Rev. (2024) 75:101–9. doi: 10.1016/j.cytogfr.2023.08.004
60. Lu Y, Xie X, Luo L. Ferroptosis crosstalk in anti-tumor immunotherapy: molecular mechanisms, tumor microenvironment, application prospects. Apoptosis. (2024) 29:1914–43. doi: 10.1007/s10495-024-01997-8
61. Gu X, Liu YE, Dai X, Yang Y-G, Zhang X. Deciphering the potential roles of ferroptosis in regulating tumor immunity and tumor immunotherapy. Front Immunol. (2023) 14:1137107. doi: 10.3389/fimmu.2023.1137107
62. Zheng Y, Sun L, Guo J, Ma J. The crosstalk between ferroptosis and anti-tumor immunity in the tumor microenvironment: molecular mechanisms and therapeutic controversy. Cancer Commun (Lond). (2023) 43:1071–96. doi: 10.1002/cac2.12487
63. Lin H, Tison K, Du Y, Kirchhoff P, Kim C, Wang W, et al. Itaconate transporter SLC13A3 impairs tumor immunity via endowing ferroptosis resistance. Cancer Cell. (2024) 42:2032–44. doi: 10.1016/j.ccell.2024.10.010
64. Chen K, Zhang S, Jiao J, Zhao S. Ferroptosis and its potential role in lung cancer: updated evidence from pathogenesis to therapy. J Inflammation Res. (2021) 14:7079–90. doi: 10.2147/JIR.S347955
65. Li Y, Li X, Li J. Ferroptosis in lung cancer: dual role, multi-level regulation, and new therapeutic strategies. Front Oncol. (2024) 14:1360638. doi: 10.3389/fonc.2024.1360638
66. Peng Y, Ouyang L, Zhou Y, Lai W, Chen Y, Wang Z, et al. AhR Promotes the Development of Non-small cell lung cancer by Inducing SLC7A11-dependent Antioxidant Function. J Cancer. (2023) 14:821–34. doi: 10.7150/jca.82066
67. Gao C, Xiao F, Zhang L, Sun Y, Wang L, Liu X, et al. SENP1 inhibition suppresses the growth of lung cancer cells through activation of A20-mediated ferroptosis. Ann Transl Med. (2022) 10:224. doi: 10.21037/atm-21-6909
68. Xing N, Du Q, Guo S, Xiang G, Zhang Y, Meng X, et al. Ferroptosis in lung cancer: a novel pathway regulating cell death and a promising target for drug therapy. Cell Death Discovery. (2023) 9:110. doi: 10.1038/s41420-023-01407-z
69. Hinokuma H, Kanamori Y, Ikeda K, Hao L, Maruno M, Yamane T, et al. Distinct functions between ferrous and ferric iron in lung cancer cell growth. Cancer Sci. (2023) 114:4355–64. doi: 10.1111/cas.15949
70. Tang Z, Jiang W, Mao M, Zhao J, Chen J, Cheng N. Deubiquitinase USP35 modulates ferroptosis in lung cancer via targeting ferroportin. Clin Transl Med. (2021) 11:e390. doi: 10.1002/ctm2.390
71. Wang Y, Hu J, Fleishman JS, Li Y, Ren Z, Wang J, et al. Inducing ferroptosis by traditional medicines: a novel approach to reverse chemoresistance in lung cancer. Front Pharmacol. (2024) 15:1290183. doi: 10.3389/fphar.2024.1290183
72. Zhang B, Hou Q, Zhang X, Ma Y, Yuan J, Li S, et al. Anesthetic propofol inhibits ferroptosis and aggravates distant cancer metastasis via Nrf2 upregulation. Free Radic Biol Med. (2023) 195:298–308. doi: 10.1016/j.freeradbiomed.2022.12.092
73. Zhou Q, Xu T, Li J, Tan J, Mao Q, Liu T, et al. Identification of the potential ferroptosis key genes in lung cancer with bone metastasis. J Thorac Dis. (2023) 15:2708–20. doi: 10.21037/jtd-23-539
74. Tai F, Zhai R, Ding K, Zhang Y, Yang H, Li H, et al. Long non−coding RNA lung cancer−associated transcript 1 regulates ferroptosis via microRNA−34a−5p−mediated GTP cyclohydrolase 1 downregulation in lung cancer cells. Int J Oncol. (2024) 64:64. doi: 10.3892/ijo.2024.5652
75. Yao F, Zhao Y, Wang G, Zhao M, Hong X, Ye Z, et al. Exosomal lncRNA ROR1-AS1 from cancer-associated fibroblasts inhibits ferroptosis of lung cancer cells through the IGF2BP1/SLC7A11 signal axis. Cell Signal. (2024) 120:111221. doi: 10.1016/j.cellsig.2024.111221
76. Chang W-M, Li L-J, Chiu IA, Lai T-C, Chang Y-C, Tsai H-F, et al. The aberrant cancer metabolic gene carbohydrate sulfotransferase 11 promotes non-small cell lung cancer cell metastasis via dysregulation of ceruloplasmin and intracellular iron balance. Transl Oncol. (2022) 25:101508. doi: 10.1016/j.tranon.2022.101508
77. Murray MB, Dixon SJ. Ferroptosis regulation by Cap'n'collar family transcription factors. J Biol Chem. (2024) 300:107583. doi: 10.1016/j.jbc.2024.107583
78. Rabitha R, Shivani S, Showket Y, Sudhandiran G. Ferroptosis regulates key signaling pathways in gastrointestinal tumors: Underlying mechanisms and therapeutic strategies. World J Gastroenterol. (2023) 29:2433–51. doi: 10.3748/wjg.v29.i16.2433
79. Pope LE, Dixon SJ. Regulation of ferroptosis by lipid metabolism. Trends Cell Biol. (2023) 33:1077–87. doi: 10.1016/j.tcb.2023.05.003
80. Lee J-Y, Kim WK, Bae K-H, Lee SC, Lee E-W. Lipid metabolism and ferroptosis. Biol (Basel). (2021) 10:184. doi: 10.3390/biology10030184
81. Lupica-Tondo GL, Arner EN, Mogilenko DA, Voss K. Immunometabolism of ferroptosis in the tumor microenvironment. Front Oncol. (2024) 14:1441338. doi: 10.3389/fonc.2024.1441338
82. Forcina GC, Pope L, Murray M, Dong W, Abu-Remaileh M, Bertozzi CR, et al. Ferroptosis regulation by the NGLY1/NFE2L1 pathway. Proc Natl Acad Sci U S A. (2022) 119:e2118646119. doi: 10.1073/pnas.2118646119
83. Shen C, Wang Y. Ferroptosis biomarkers for predicting prognosis and immunotherapy efficacy in adrenocortical carcinoma. Arch Med Res. (2023) 54:45–55. doi: 10.1016/j.arcmed.2022.12.003
84. Lan J, Liu L, Zhao W, Li Z, Zeng R, Fang S, et al. Unlocking the anticancer activity of gambogic acid: a shift towards ferroptosis via a GSH/Trx dual antioxidant system. Free Radic Biol Med. (2024) 218:26–40. doi: 10.1016/j.freeradbiomed.2024.03.023
85. Fan G-B, Li Y, Xu G-S, Zhao AY, Jin H-J, Sun S-Q, et al. Propofol inhibits ferroptotic cell death through the nrf2/gpx4 signaling pathway in the mouse model of cerebral ischemia-reperfusion injury. Neurochem Res. (2023) 48:956–66. doi: 10.1007/s11064-022-03822-7
86. Nehring H, Meierjohann S, Friedmann Angeli JP. Emerging aspects in the regulation of ferroptosis. Biochem Soc Trans. (2020) 48:2253–9. doi: 10.1042/BST20200523
87. Deng X, Chu W, Zhang H, Peng Y. Nrf2 and ferroptosis: A new research direction for ischemic stroke. Cell Mol Neurobiol. (2023) 43:3885–96. doi: 10.1007/s10571-023-01411-y
88. Li D, Zhang Z, Wang L. Emerging role of tumor microenvironmental nutrients and metabolic molecules in ferroptosis: Mechanisms and clinical implications. BioMed Pharmacother. (2024) 179:117406. doi: 10.1016/j.biopha.2024.117406
89. Bi Q, Sun Z-J, Wu J-Y, Wang W. Ferroptosis-mediated formation of tumor-promoting immune microenvironment. Front Oncol. (2022) 12:868639. doi: 10.3389/fonc.2022.868639
90. Wang Z, Chen X, Liu N, Shi Y, Liu Y, Ouyang L, et al. A nuclear long non-coding RNA LINC00618 accelerates ferroptosis in a manner dependent upon apoptosis. Mol Ther. (2021) 29:263–74. doi: 10.1016/j.ymthe.2020.09.024
91. Li S, Huang Y. Ferroptosis: an iron-dependent cell death form linking metabolism, diseases, immune cell and targeted therapy. Clin Transl Oncol. (2022) 24:1–12. doi: 10.1007/s12094-021-02669-8
92. Zhang P, Chen L, Zhao Q, Du X, Bi M, Li Y, et al. Ferroptosis was more initial in cell death caused by iron overload and its underlying mechanism in Parkinson's disease. Free Radic Biol Med. (2020) 152:227–34. doi: 10.1016/j.freeradbiomed.2020.03.015
93. Vinik Y, Maimon A, Dubey V, Raj H, Abramovitch I, Malitsky S, et al. Programming a ferroptosis-to-apoptosis transition landscape revealed ferroptosis biomarkers and repressors for cancer therapy. Adv Sci (Weinh). (2024) 11:e2307263. doi: 10.1002/advs.202307263
94. Woo Y, Lee H-J, Jung YM, Jung Y-J. Regulated necrotic cell death in alternative tumor therapeutic strategies. Cells. (2020) 9:2709. doi: 10.3390/cells9122709
95. Zhou Y, Liao J, Mei Z, Liu X, Ge J. Insight into crosstalk between ferroptosis and necroptosis: novel therapeutics in ischemic stroke. Oxid Med Cell Longev. (2021) 2021:9991001. doi: 10.1155/2021/9991001
96. Zhao Y, Wang Q, Zhu J, Cai J, Feng X, Song Q, et al. Identification of KW-2449 as a dual inhibitor of ferroptosis and necroptosis reveals that autophagy is a targetable pathway for necroptosis inhibitors to prevent ferroptosis. Cell Death Dis. (2024) 15:764. doi: 10.1038/s41419-024-07157-9
97. Zhou P, Zhang S, Wang M, Zhou J. The induction mechanism of ferroptosis, necroptosis, and pyroptosis in inflammatory bowel disease, colorectal cancer, and intestinal injury. Biomolecules. (2023) 13:820. doi: 10.3390/biom13050820
98. Park E, Chung SW. ROS-mediated autophagy increases intracellular iron levels and ferroptosis by ferritin and transferrin receptor regulation. Cell Death Dis. (2019) 10:822. doi: 10.1038/s41419-019-2064-5
99. Lee S, Hwang N, Seok BG, Lee S, Lee S-J, Chung SW. Autophagy mediates an amplification loop during ferroptosis. Cell Death Dis. (2023) 14:464. doi: 10.1038/s41419-023-05978-8
100. Hu G, Yuan Z, Wang J. Autophagy inhibition and ferroptosis activation during atherosclerosis: Hypoxia-inducible factor 1α inhibitor PX-478 alleviates atherosclerosis by inducing autophagy and suppressing ferroptosis in macrophages. BioMed Pharmacother. (2023) 161:114333. doi: 10.1016/j.biopha.2023.114333
101. Xie Y, Zhou Y, Wang J, Du L, Ren Y, Liu F. Ferroptosis, autophagy, tumor and immunity. Heliyon. (2023) 9:e19799. doi: 10.1016/j.heliyon.2023.e19799
102. Wang W, Lu K, Jiang X, Wei Q, Zhu L, Wang X, et al. Ferroptosis inducers enhanced cuproptosis induced by copper ionophores in primary liver cancer. J Exp Clin Cancer Res. (2023) 42:142. doi: 10.1186/s13046-023-02720-2
103. Xue Q, Yan D, Chen X, Li X, Kang R, Klionsky DJ, et al. Copper-dependent autophagic degradation of GPX4 drives ferroptosis. Autophagy. (2023) 19:1982–96. doi: 10.1080/15548627.2023.2165323
104. Li F, Wu X, Liu H, Liu M, Yue Z, Wu Z, et al. Copper depletion strongly enhances ferroptosis via mitochondrial perturbation and reduction in antioxidative mechanisms. Antioxidants (Basel). (2022) 11:2084. doi: 10.3390/antiox11112084
105. Song R, Yin S, Wu J, Yan J. Neuronal regulated cell death in aging-related neurodegenerative diseases: key pathways and therapeutic potentials. Neural Regener Res. (2025) 20:2245–63. doi: 10.4103/NRR.NRR-D-24-00025
106. Jiayi H, Ziyuan T, Tianhua X, Mingyu Z, Yutong M, Jingyu W, et al. Copper homeostasis in chronic kidney disease and its crosstalk with ferroptosis. Pharmacol Res. (2024) 202:107139. doi: 10.1016/j.phrs.2024.107139
107. Gao X, Zhao H, Liu J, Wang M, Dai Z, Hao W, et al. Enzalutamide sensitizes castration-resistant prostate cancer to copper-mediated cell death. Adv Sci (Weinh). (2024) 11:e2401396. doi: 10.1002/advs.202401396
108. Ji P, Wang P, Chen H, Xu Y, Ge J, Tian Z, et al. Potential of copper and copper compounds for anticancer applications. Pharm (Basel). (2023) 16:234. doi: 10.3390/ph16020234
109. Wang J, Li J, Liu J, Chan K-Y, Lee H-S, Lin KN, et al. Interplay of ferroptosis and cuproptosis in cancer: dissecting metal-driven mechanisms for therapeutic potentials. Cancers (Basel). (2024) 16:512. doi: 10.3390/cancers16030512
110. Li Y, Zhang H, Zhu D, Yang F, Wang Z, Wei Z, et al. Notochordal cells: A potential therapeutic option for intervertebral disc degeneration. Cell Prolif. (2024) 57:e13541. doi: 10.1111/cpr.13541
111. Hu B, Yin Y, Li S, Guo X. Insights on ferroptosis and colorectal cancer: progress and updates. Molecules. (2022) 28:243. doi: 10.3390/molecules28010243
112. Zhou Q, Meng Y, Li D, Yao L, Le J, Liu Y, et al. Ferroptosis in cancer: From molecular mechanisms to therapeutic strategies. Signal Transduct Target Ther. (2024) 9:55. doi: 10.1038/s41392-024-01769-5
113. Sun S, Shen J, Jiang J, Wang F, Min J. Targeting ferroptosis opens new avenues for the development of novel therapeutics. Signal Transduct Target Ther. (2023) 8:372. doi: 10.1038/s41392-023-01606-1
114. Yin W, Chang J, Sun J, Zhang T, Zhao Y, Li Y, et al. Nanomedicine-mediated ferroptosis targeting strategies for synergistic cancer therapy. J Mater Chem B. (2023) 11:1171–90. doi: 10.1039/d2tb02161g
115. Guan Z, Li S, Liu Y, Zhang X, Liu Y, Zou F, et al. A specific targeted enhanced nanotherapy strategy for inducing ferroptosis by regulating the iron pool levels in tumor cells. ACS Appl Mater Interfaces. (2024) 16:56837–49. doi: 10.1021/acsami.4c13534
116. Ashoub MH, Razavi R, Heydaryan K, Salavati-Niasari M, Amiri M. Targeting ferroptosis for leukemia therapy: exploring novel strategies from its mechanisms and role in leukemia based on nanotechnology. Eur J Med Res. (2024) 29:224. doi: 10.1186/s40001-024-01822-7
117. Xiang D, Zhou L, Yang R, Yuan F, Xu Y, Yang Y, et al. Advances in ferroptosis-inducing agents by targeted delivery system in cancer therapy. Int J Nanomedicine. (2024) 19:2091–112. doi: 10.2147/IJN.S448715
118. Lee J, Roh J-L. Targeting iron-sulfur clusters in cancer: opportunities and challenges for ferroptosis-based therapy. Cancers (Basel). (2023) 15:2694. doi: 10.3390/cancers15102694
119. Mokhtarpour K, Razi S, Rezaei N. Ferroptosis as a promising targeted therapy for triple negative breast cancer. Breast Cancer Res Treat. (2024) 207:497–513. doi: 10.1007/s10549-024-07387-7
120. Zhang X, Li X, Xia R, Zhang H-S. Ferroptosis resistance in cancer: recent advances and future perspectives. Biochem Pharmacol. (2024) 219:115933. doi: 10.1016/j.bcp.2023.115933
121. Zhuo S, He G, Chen T, Li X, Liang Y, Wu W, et al. Emerging role of ferroptosis in glioblastoma: Therapeutic opportunities and challenges. Front Mol Biosci. (2022) 9:974156. doi: 10.3389/fmolb.2022.974156
122. Manzari Tavakoli G, Mirzapour MH, Razi S, Rezaei N. Targeting ferroptosis as a cell death pathway in Melanoma: From molecular mechanisms to skin cancer treatment. Int Immunopharmacol. (2023) 119:110215. doi: 10.1016/j.intimp.2023.110215
123. Ta N, Jiang X, Zhang Y, Wang H. Ferroptosis as a promising therapeutic strategy for melanoma. Front Pharmacol. (2023) 14:1252567. doi: 10.3389/fphar.2023.1252567
124. Zheng X, Jin X, Ye F, Liu X, Yu B, Li Z, et al. Ferroptosis: a novel regulated cell death participating in cellular stress response, radiotherapy, and immunotherapy. Exp Hematol Oncol. (2023) 12:65. doi: 10.1186/s40164-023-00427-w
125. Jewell K, Kostos L, Emmerson B, Hofman MS. Combination strategies and targeted radionuclide therapies. Semin Nucl Med. (2024) 54:612–21. doi: 10.1053/j.semnuclmed.2024.05.011
126. Ye LF, Chaudhary KR, Zandkarimi F, Harken AD, Kinslow CJ, Upadhyayula PS, et al. Radiation-induced lipid peroxidation triggers ferroptosis and synergizes with ferroptosis inducers. ACS Chem Biol. (2020) 15:469–84. doi: 10.1021/acschembio.9b00939
127. An W-X, Gupta R, Zhai K, Wang Y-R, Xu W-H, Cui Y. Current and potential roles of ferroptosis in bladder cancer. Curr Med Sci. (2024) 44:51–63. doi: 10.1007/s11596-023-2814-6
128. Kobayashi H, Yoshimoto C, Matsubara S, Shigetomi H, Imanaka S. Current understanding of and future directions for endometriosis-related infertility research with a focus on ferroptosis. Diagnostics (Basel). (2023) 13:1926. doi: 10.3390/diagnostics13111926
129. Yeon Kim S, Tang M, Lu T, Chih SY, Li W. Ferroptosis in glioma therapy: advancements in sensitizing strategies and the complex tumor-promoting roles. Brain Res. (2024) 1840:149045. doi: 10.1016/j.brainres.2024.149045
130. Zhan M, Ding Y, Huang S, Liu Y, Xiao J, Yu H, et al. Lysyl oxidase-like 3 restrains mitochondrial ferroptosis to promote liver cancer chemoresistance by stabilizing dihydroorotate dehydrogenase. Nat Commun. (2023) 14:3123. doi: 10.1038/s41467-023-38753-6
131. Xu J, Zheng B, Wang W, Zhou S. Ferroptosis: a novel strategy to overcome chemoresistance in gynecological Malignancies. Front Cell Dev Biol. (2024) 12:1417750. doi: 10.3389/fcell.2024.1417750
132. Bae C, Hernández Millares R, Ryu S, Moon H, Kim D, Lee G, et al. Synergistic effect of ferroptosis-inducing nanoparticles and X-ray irradiation combination therapy. Small. (2024) 20:e2310873. doi: 10.1002/smll.202310873
133. Ning J, Chen L, Zeng Y, Xiao G, Tian W, Wu Q, et al. The scheme, and regulative mechanism of pyroptosis, ferroptosis, and necroptosis in radiation injury. Int J Biol Sci. (2024) 20:1871–83. doi: 10.7150/ijbs.91112
134. Jiang X, Yu M, Wang W-K, Zhu L-Y, Wang X, Jin H-C, et al. The regulation and function of Nrf2 signaling in ferroptosis-activated cancer therapy. Acta Pharmacol Sin. (2024) 45:2229–40. doi: 10.1038/s41401-024-01336-2
135. Guan X-Y, Guan X-L, Zhu J-R. Mechanisms and applications of ferroptosis-associated regulators in cancer therapy and drug resistance. J Chemother. (2023) 35:671–88. doi: 10.1080/1120009X.2023.2177808
136. Ishimaru K, Ikeda M, Miyamoto HD, Furusawa S, Abe K, Watanabe M, et al. Deferasirox targeting ferroptosis synergistically ameliorates myocardial ischemia reperfusion injury in conjunction with cyclosporine A. J Am Heart Assoc. (2024) 13:e031219. doi: 10.1161/JAHA.123.031219
137. Cheng X, Zhao F, Ke B, Chen D, Liu F. Harnessing ferroptosis to overcome drug resistance in colorectal cancer: promising therapeutic approaches. Cancers (Basel). (2023) 15:5209. doi: 10.3390/cancers15215209
138. Feng Y, Wei H, Lyu M, Yu Z, Chen J, Lyu X, et al. Iron retardation in lysosomes protects senescent cells from ferroptosis. Aging (Albany NY). (2024) 16:7683–703. doi: 10.18632/aging.205777
139. Wang L, Piao Y, Guo F, Wei J, Chen Y, Dai X, et al. Current progress of pig models for liver cancer research. BioMed Pharmacother. (2023) 165:115256. doi: 10.1016/j.biopha.2023.115256
140. Li L, Liu X, Han C, Tian L, Wang Y, Han B. Ferroptosis in radiation-induced brain injury: roles and clinical implications. BioMed Eng Online. (2024) 23:93. doi: 10.1186/s12938-024-01288-y
Keywords: lung cancer, ferroptosis, cell death mechanisms, tumor microenvironment, therapeutic strategies, molecular pathways
Citation: Gao G and Zhang X (2025) Broadening horizons: research on ferroptosis in lung cancer and its potential therapeutic targets. Front. Immunol. 16:1542844. doi: 10.3389/fimmu.2025.1542844
Received: 10 December 2024; Accepted: 03 January 2025;
Published: 23 January 2025.
Edited by:
Qi Feng, First Affiliated Hospital of Zhengzhou University, ChinaReviewed by:
Liangxue Zhou, Sichuan University, ChinaCopyright © 2025 Gao and Zhang. This is an open-access article distributed under the terms of the Creative Commons Attribution License (CC BY). The use, distribution or reproduction in other forums is permitted, provided the original author(s) and the copyright owner(s) are credited and that the original publication in this journal is cited, in accordance with accepted academic practice. No use, distribution or reproduction is permitted which does not comply with these terms.
*Correspondence: Xindi Zhang, emhhbmd4aW5kaTQwMEAxNjMuY29t
Disclaimer: All claims expressed in this article are solely those of the authors and do not necessarily represent those of their affiliated organizations, or those of the publisher, the editors and the reviewers. Any product that may be evaluated in this article or claim that may be made by its manufacturer is not guaranteed or endorsed by the publisher.
Research integrity at Frontiers
Learn more about the work of our research integrity team to safeguard the quality of each article we publish.