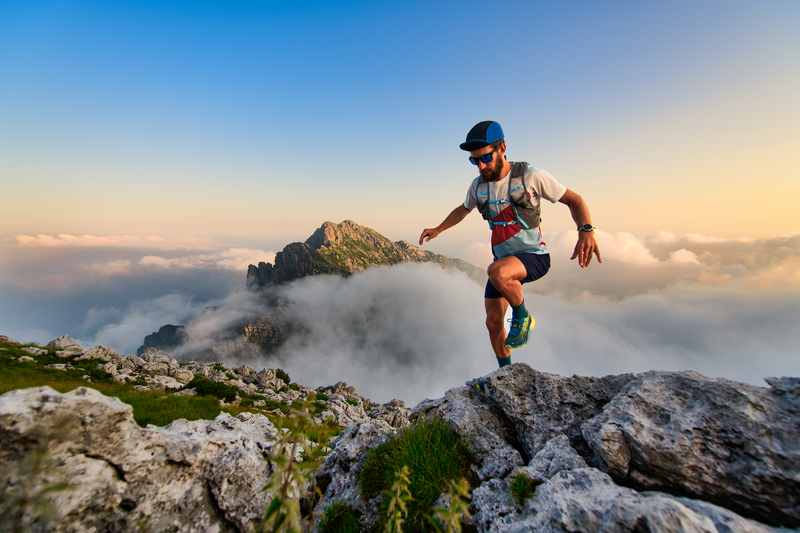
95% of researchers rate our articles as excellent or good
Learn more about the work of our research integrity team to safeguard the quality of each article we publish.
Find out more
ORIGINAL RESEARCH article
Front. Immunol. , 03 April 2025
Sec. Microbial Immunology
Volume 16 - 2025 | https://doi.org/10.3389/fimmu.2025.1538425
Klebsiella pneumoniae is the causative agent of a wide range of antibiotic-resistant infections, including nosocomial pneumonia and neonatal sepsis. We investigate here the mechanisms underlying innate immune recognition of this pathogen by focusing on the role of endosomal Toll-like receptors (TLRs), which sense prokaryotic nucleic acids, in comparison with TLR4, which recognizes the cell-wall lipopolysaccharide component. Lack of functional endosomal TLRs made mice more susceptible to pulmonary infection by K. pneumoniae, in association with reduced production of proinflammatory and chemotactic cytokines and reduced neutrophil recruitment to the lung. This phenotype was as severe as that of TLR4-deficient mice and only moderately milder than that of mice lacking the TLR adaptor MyD88. Notably, macrophages lacking at the same time TLR7, 9 and 13 were more defective than those lacking only TLR9 in their ability to produce proinflammatory cytokines, suggesting a role for the RNA sensing TLR7 and 13 receptors in K. pneumoniae recognition. Collectively, our results unveil the presence of an integrated system of DNA and RNA sensing TLRs that cooperates with TLR4 in immune detection and clearance of K. pneumoniae. These data may be useful to devise alternative therapeutic approaches aimed at stimulating responses against antibiotic-resistant K. pneumoniae strains.
Klebsiella species are ubiquitous Gram-negative microorganisms found in the environment, including water and soil, as well as in association with animals (1, 2). Klebsiella pneumoniae, an opportunistic encapsulated human pathogen, frequently colonizes the gastrointestinal mucosa and the oropharynx without causing disease. However, from these sites, K. pneumoniae can spread to other tissues, leading to severe conditions including urinary tract infections (UTIs), pneumonia, meningitis and septicemia (3–5). K. pneumoniae also frequently colonizes medical devices, being one of the most commonly isolated nosocomial pathogens in intensive care units (4, 5). An alarming proportion of K. pneumoniae clinical isolates is highly resistant to a wide variety of antibiotics, such as fluoroquinolones, third-generation cephalosporins, aminoglycosides and carbapenems, thus severely limiting available therapeutic options (4). Furthermore, recent studies suggest that emerging hypervirulent K. pneumoniae clones have simultaneously acquired virulence and antimicrobial genes, raising the potential for untreatable infections even in healthy individuals (6–8). In addition, K. pneumoniae can finely regulate the expression of virulence factors and antibiotic resistance genes through Two-Component Systems (TCSs) capable of sensing and responding to environmental signals (9–12). Due to these factors, the World Health Organization (WHO) recently identified K. pneumoniae as a critical public health threat and a major antimicrobial resistant (AMR) pathogen (13, 14), highlighting an urgent need to develop alternative therapeutic strategies, such as those that enhance host defenses. Defenses against K. pneumoniae and other microbes rely on the host’s ability to recognize invading pathogens through pattern-recognition receptors (PRRs) that are primarily found in cells of the mononuclear phagocyte system (such as monocytes, macrophages, and dendritic cells) as well as in granulocytes, including neutrophils. PRRs are strategically located in subcellular compartments where they detect conserved microbial components, known as pathogen-associated molecular patterns (PAMPs), and initiate intracellular signaling pathways that activate or enhance the host’s antimicrobial defenses (15). Toll-like receptors (TLRs) are one of the best-characterized classes of PRRs and are highly conserved across a wide range of species, from invertebrates to vertebrates (16–18). Upon recognition of microbial PAMPs, TLRs trigger complex downstream signaling pathways leading to the production and release of cytokines and chemokines that orchestrate a variety of innate and adaptive responses (19). Signaling molecules that are sequentially engaged by TLRs include the adaptor MyD88 (myeloid differentiation primary response gene 88), which leads to transcription of anti-microbial genes through the activation of mitogen-activated protein kinases, nuclear factor-κB and interferon releasing factors (20). In addition, TLR3 and TLR4 can activate MyD88-independent pathways that require the adaptor molecule TRIF (TIR domain-containing adaptor inducing interferon-β) (21).
TLR4, a cell surface-exposed receptor, has been historically identified as the most important TLR for the recognition of K. pneumoniae (22–25), due to its capacity to sense lipopolysaccharide (LPS), the major component of the outer membrane of Gram-negative bacteria (26). TLR2, an additional surface-exposed receptor, has also been reported to play a role in anti-K. pneumoniae defenses (27, 28). Finally, the endosomal receptor TLR9, which can sense unmethylated CpG-rich DNA motifs that are abundant in prokaryotic genomes, also participates in anti-K. pneumoniae defenses (20). Interestingly, in dendritic cells, TLR9 can cooperate with TLR4 to maximally amplify the production of IL-23 and IL-17 (22). Other in vivo studies focused on the involvement of MyD88 (which mediates signaling by all TLRs except TLR3) or TIRAP (an essential adaptor for TLR1, TLR2, TLR4 and TLR6 signaling), confirming the important role of TLR-initiated pathways in K. pneumoniae recognition (21, 29).
Although recent studies highlighted a crucial role of RNA-sensing TLRs, such as TLR7 and 13, in the recognition of Gram-positive bacterial pathogens (30–33), little is known of the functions of these receptors in the context of infection by K. pneumoniae or other Gram-negative pathogens. In the present study, by using mouse strains with defective TLR signaling, we demonstrate that endosomal receptors play a major role in sensing the presence of K. pneumoniae and in sustaining the production of a distinctive set of proinflammatory and chemotactic cytokines, particularly IL-12p70. We find that absence of nucleic acid-sensing TLR function is associated with markedly enhanced susceptibility to lung infection due to defective neutrophil recruitment. Moreover, K. pneumoniae RNA was found to be more potent than DNA in inducing cytokine production in macrophages, primarily through its ability to engage TLR7 and 13. These findings may provide valuable insights for the development of alternative therapeutic strategies that target innate immune receptors, offering a potential approach for treating infections caused by antibiotic-resistant K. pneumoniae.
Wild type (WT) C57BL/6 female mice were purchased from Charles River Laboratories (Charles River, Calco, Italy). Mice lacking MyD88, TLR2, TLR3, TLR4, TLR7 and TLR9 on a C57BL/6 background were a generous gift of Shizuo Akira (from Osaka University, Suita, Japan). TLR13-/- mice were obtained by intercrossing TLR13+/- animals generated at the University of California, Davis Mouse Biology Program (www.mousebiology.org) and provided by the Knockout (KO) Mouse Project Repository (www.komp.org), as previously described (32). Triple KO mice simultaneously lacking TLR7, 9 and 13 have also been previously described (32, 33). Mice bearing the H412R mutation of UNC93B1 (or “3d” mice) were donated by Bruce Beutler (University of Texas Southwestern Medical Center, Texas). Individually ventilated cages were used to house all mice strains under specific pathogen-free conditions. K. pneumoniae AC133 (34) was used throughout the present investigation. Bacteria were grown to the mid-log phase in Luria Bertani broth, washed three times in nonpyrogenic phosphate-buffered saline (PBS) (0.01 M phosphate, 0.15 M NaCl [pH 7.4]; Thermo Fisher Scientific), and resuspended to the appropriate concentration in Luria Bertani broth before intranasal challenge (or in PBS before addition to phagocyte cultures) at the indicated multiplicities of infection (MOI, see below).
Six-week-old female mice were anesthetized by the intraperitoneal injection of tiletamine-zolazepam (0.1 mg/mouse) and xylazine (0.16 mg/mouse) and inoculated intranasally (i.n.) with 20 µl of the bacterial suspension (1x107 CFU/mouse). Clinical conditions were evaluated every 12h for 10 days after inoculation. Animals with irreversible signs of disease, as assessed by a scoring system based on predefined clinical criteria (34), were humanely euthanized using cervical dislocation. To study the ability of bacteria to grow in vivo, the animals were euthanized at the indicated times after challenge and transcardially perfused with PBS (20 ml), prior to lung collection, as previously described (30). To obtain lung homogenates, the organs were minced and enzymatically digested with collagenase, as described previously (30). Bacterial CFU were measured in organ homogenates by plating serial dilutions on agar plates by standard techniques, while neutrophil numbers were determined by flow cytometry as described below.
3d, MyD88-/-, TLR4-/- and TLR2-/- mice were used as source of bone marrow-derived and bronchial washing cells that were isolated as previously described (34). Briefly, bone marrow cells were obtained from the extremities of the long bones after cutting off epiphyses through centrifugation at 400xg for 15 min, washed and resuspended in PBS. To obtain neutrophils, cells were centrifuged on a density Percoll gradient, as described (33). To obtain macrophages, marrow cells were cultured for 6 to 7 days in RPMI 1640 supplemented with heat-inactivated 10% FCS, penicillin (50 IU/ml), streptomycin (50 g/ml) and macrophage colony-stimulating factor (M-CSF; 100 ng/ml) or granulocyte-macrophage colony-stimulating factor (GM-CSF; 20 ng/ml), both from PeproTech. Frozen bone marrow derived macrophages originally obtained from TLR3-/-, TLR7-/-, TLR9-/-, TLR13-/- and TLR7/9/13-/- mice (33) were thawed and cultured in the presence of GM-CSF to obtain GM-CSF-polarized macrophages. To obtain alveolar macrophages (AM), the lungs of WT, 3d, MyD88-/-, TLR4-/- or TLR2-/- mice were lavaged with an intratracheal catheter with PBS supplemented with 1mM EDTA. The bronchoalveolar lavage fluid was centrifuged at 300xg for 10 min and cells were resuspended to a cell concentration of 5x106/ml in RPMI 1640 supplemented with heat-inactivated 10% FCS, penicillin (50 IU/ml), streptomycin (50 g/ml), and 100 ng/ml M-CSF. Isolated cells were incubated at 37°C with 5% CO2 for 24 h and three washes were done to gently remove nonadherent cells.
Macrophages were infected with PBS suspensions of K. pneumoniae at the indicated MOIs. A low-speed centrifugation step (400xg for 10 min) was carried out to facilitate interactions between bacteria and macrophages. After a 1 h incubation at 37°C with 5% CO2, gentamycin (100 μg/ml) was subsequently added to infected cells for killing extracellular bacteria, as previously described (35–38). Control wells were stimulated with Escherichia coli K-12 ultrapure LPS (InvivoGen). Cell culture supernatants were then collected at various times after infection and stored at 80°C for cytokine measurements. In selected experiments, bone marrow-derived macrophages (BMDMs) were stimulated with DNA and RNA extracted from K. pneumoniae. Briefly, bacterial nucleic acids were extracted and purified as previously described (33) and complexed with N-[1-(2,3-dioleoyloxy) propyl]-N, N, N-trimethylammonium methyl-sulfate (DOTAP) before cell stimulation, as previously described (32, 33, 39).
For phagocytosis/killing assays, WT and 3d macrophages (5x105 cells/well) were infected with K. pneumoniae (1x107 CFU/well) and incubated for 1 h at 37°C with 5% CO2. After three washes with PBS and killing of extracellular bacteria through the addition of medium containing gentamicin (100 μg/ml), macrophages were washed, detached, and lysed with 0.025% Triton X-100 to release intracellular bacteria. Recovered bacteria were plated on agar plates for CFU counts.
Commercial enzyme-linked immunosorbent assay (ELISA) kits were used to measure cytokine and chemokines levels in lung homogenates and culture supernatants, as described (40). Keratinocyte-derived chemokine (KC; Cxcl1), macrophage inflammatory protein 2 (MIP-2; Cxcl2), IL-1β, TNF-α, IL-12 p70, IFN-β, and IFN-ℽ were determined in triplicate with the following ELISA kits (all from R&D Systems): CXCL1/KC Quantikine, DuoSet CXCL2/MIP-2, IL-1β/IL-1F2 Quantikine, DuoSet TNF-α, DuoSet IL-12 p70, IFN-β DuoSet and Duoset IFN-ℽ. The lowest detection limits of these assays were, respectively, 15.6, 15.6, 12.5, 16.0, 39.1, 12.5, and 31.3 pg/ml.
Total RNA was extracted from macrophages (1x107) using the RNeasy mini kit (Qiagen) according to the manufacturer’s instructions as previously described (41, 42). After determining RNA quality and yield by agarose gel electrophoresis and spectrophotometry, cDNA was synthesized using the Moloney murine leukemia virus reverse transcriptase kit (M-MLV RT; Invitrogen), and expression of cytokine encoding genes was determined by qPCR by the delta-delta threshold cycle (ΔΔCT) method normalized against the β-actin housekeeping gene, as described (30).
Neutrophils were enumerated in lung homogenates during K. pneumoniae infection by flow cytometry on a FACS Canto II flow cytometer (BD Biosciences) using BD Trucount beads (BD Biosciences), as previously described (33). Briefly, single cell suspensions obtained from lung homogenates were incubated for 20 min with mouse Fc block (rat anti-mouse CD16/CD32 clone 2.4G2, BD Pharmingen) and then stained for 20 min with antibodies directed against Ly6G (phycoerythrin [PE]–rat anti-mouse Ly6G clone 1A8; BD Pharmingen) or with an isotype control monoclonal antibody. Data analysis was performed using Flowing Software 2.5.1.
CellROX Deep Red Flow Cytometry Assay Kit (Thermo Fisher Scientific) was used to measure bacteria-induced reactive oxygen species (ROS) production, according to the manufacturer’s instructions. Briefly, bone marrow-derived neutrophils from WT or immune-deficient mice were seeded at a concentration of 5 x 105 per well in RPMI 1640 supplemented with 10% FCS and stimulated for 30 min with the indicated doses of K. pneumoniae. Then, stimulated neutrophils were stained with the CellROX fluorescent reagent (5µM) for 30 min at 37°C. Cells were washed three times, fixed with 3.7% formaldehyde for 15 min and analyzed with a BD FACSCanto II instrument. Flowing Software 2.5.1 was used for data analysis.
Differences in CFU counts and in cytokine or chemokine levels were assessed by Mann-Whitney test. Survival data obtained in in vivo assays were analyzed by the log-rank (Mantel-Cox) test. Differences were considered statistically significant when p values were less than 0.05. Statistical analyses were performed with GraphPad Prism 8.2.0 (GraphPad Software, Inc., San Diego, CA).
All animal studies were performed in strict accordance with the European Union guidelines for the use of laboratory animals. The procedures were approved by the Animal Welfare Committee of the University of Messina (OPBA) and by the Ministero della Salute of Italy (permit numbers 785/2018-PR and 1023/2024-PR).
To investigate the role of endosomal TLRs in immune sensing of K. pneumoniae, we used “triple-defective” (3d) mice carrying a missense mutation in UNC93B1, a chaperone protein responsible for trafficking and localization of endosomal TLRs. As a result, 3d mice are deficient in signaling through nucleic acid-sensing TLRs, such as TLR3, 7, 9, 11, and 13 (43). To assess the susceptibility of 3d mice to K. pneumoniae, we employed a pulmonary infection model in which bacteria were administered intranasally (i.n.) and replicated in the lungs. For comparison, we also included mice lacking MyD88, TLR4, or TLR2, as these molecules are known to contribute to anti-K. pneumoniae immune responses (27, 28, 44). Mice were challenged with a low, sublethal bacterial dose (as determined in preliminary experiments), and survival was monitored for up to 10 days. As expected, all wild-type (WT) mice survived without signs of disease. In contrast, MyD88-deficient mice displayed extreme susceptibility, with all succumbing within 3 days (Figure 1A). Although 3d mice survived longer than MyD88-deficient mice, they ultimately succumbed early during the infection, showing similar susceptibility to K. pneumoniae as TLR4-deficient mice. In comparison, TLR2-deficient mice exhibited greater resistance, with only 40% requiring humane euthanasia at late time points (Figure 1A). To determine whether increased lethality in immune-defective mice is due to a reduced ability to control bacterial replication, we assessed bacterial burden in the blood and lungs at 24 and 48 h post-challenge. At both time points, K. pneumoniae was detected in the lungs of all wild-type (WT) mice but only a few developed bacteremia (Figures 1B–E), indicating their ability to largely contain the infection within the lungs. In contrast, bacteria were detected in the blood of all 3d, MyD88-deficient, and TLR4-deficient mice, with significantly higher bacterial loads compared to WT animals (Figures 1B–E). These findings suggest that 3d mice are hypersusceptible to K. pneumoniae due to impaired control of bacterial replication in the lung and its spread to the bloodstream. Furthermore, the severity of the 3d phenotype is comparable to that of TLR4-deficient mice and more severe than that of TLR2-deficient mice.
Figure 1. Mice with non-functional UNC93B1 (3d mice) are highly susceptible to (K) pneumoniae infection. (A) Survival of WT, 3d, MyD88-/-, TLR4-/- and TLR2-/- mice after i.n. challenge with 1 x 107 CFU of (K) pneumoniae. Shown are cumulative data from two experiments, each involving 8 animals per group. **, p<0.01; ***, p<0.001 versus WT mice, as determined by the log-rank test. (B–E) Log CFU numbers in the lungs or blood of WT, 3d, TLR4-/-, MyD88-/- and TLR2-/- mice at 24 and 48 h after i.n. infection. Horizontal red bars indicate mean values. The dashed lines indicate the limit of detection of the test. Each determination was conducted on a different animal in the course of two experiments, each involving 4 animals per group. ns, not significant, *, p<0.05; **, p<0.01; ***, p<0.001 versus WT mice as determined by the Mann-Whitney test.
Given their hypersusceptibility to K. pneumoniae infection, we next assessed the ability of 3d mice to mount defensive proinflammatory responses against the pathogen. Mice were challenged intranasally, as described previously, and cytokine concentrations, neutrophil infiltration, and bacterial burden were measured in lung homogenates. In wild-type (WT) mice, bacterial numbers steadily increased during the initial hours of infection, peaking at 24 hours and slowly declining thereafter (Figure 2A). In comparison, pathogen growth was faster in 3d mice, with bacterial numbers almost twice as high at 6 hours post-challenge. Neutrophil infiltration paralleled bacterial growth early during infection, peaking at 6 hours in both groups (Figure 2B). However, neutrophils influx was delayed and significantly reduced in 3d mice, despite higher bacterial loads. Neutrophils recruitment was associated with the production of several chemotactic and proinflammatory cytokines. As shown in Figures 2C, D, the neutrophil-attracting chemokines Cxcl1 and Cxcl2 were the first to increase above baseline in WT mice, being detectable as early as 1-hour post-challenge, whereas their production was delayed and diminished in 3d mice. Similarly, levels of TNF-α and IL-1β were lower in 3d mice early in infection (Figures 2E, F). Notably, both groups of mice exhibited a peak in IFN-γ at 24 hours, i.e. considerably later than other proinflammatory mediators (Figures 2G, H). Additionally, IL-12p70 and IFN-γ responses were more severely reduced in 3d mice compared to other cytokines. These findings suggest that endosomal receptors are critical for controlling K. pneumoniae infection in the lungs by promoting early chemotactic and proinflammatory cytokine production, as well as neutrophil recruitment. Furthermore, these receptors play a particularly important role in mediating IL-12p70 and IFN-γ responses to K. pneumoniae.
Figure 2. Mice with non-functional UNC93B1 (3d mice) are defective in neutrophil recruitment and cytokine responses during (K) pneumoniae pneumonitis. CFU numbers (A), neutrophil counts (B) and cytokine concentrations (C–H) in the lungs at various times after i.n. challenge with 1 x 107 CFU/mouse of (K) pneumoniae. Shown are results from one experiment involving 3 animals per time point. *, p<0.05 as determined by the Mann-Whitney test. Points and bars indicate means plus standard deviations. The dotted lines indicate the limits of detection of the tests.
Given the significant reduction in neutrophil infiltration and cytokine production observed during in vivo infection, we sought to investigate in vitro cytokine responses to K. pneumoniae in macrophages, as these cells are key producers of inflammatory mediators. To this end, we cultured WT and 3d bone marrow-derived macrophages in the presence of macrophage colony-stimulating factor (M-CSF-MΦ) and assessed their bactericidal activity and inflammatory mediator production. 3d M-CSF-MΦ displayed no significant defects in bacterial internalization or intracellular killing compared to WT M-CSF-MΦ (Figure 3A). However, 3d cells were significantly, albeit partially, impaired in their ability to release Cxcl2, IL-1β and TNF-α in response to K. pneumoniae compared to WT cells (Figures 3B–D). These defects were specific for stimulation with whole bacteria, since 3d macrophages exhibited normal responses to LPS, a well-known TLR4 agonist. Moreover, the extent of the reduction in pro-inflammatory cytokine production in 3d macrophages was similar to that observed in cells deficient in TLR4, which is known to play a crucial role in immune sensing of Gram-negative bacteria, including K. pneumoniae.
Figure 3. Endosomal TLRs participate in chemokine and cytokine responses to K. pneumoniae by M-CSF polarized macrophages. (A) Bacterial killing assay in bone marrow macrophages cultured in the presence of M-CSF obtained from wild type (WT) and 3d mice. Cells (5x105) were exposed to K. pneumoniae (1x107 CFU) for 1 h at 37°C in 5% CO2. Extracellular bacteria were then killed through the addition of gentamicin (100 µg/ml), and cultures were further incubated for 1, 3, and 6 h before determination of CFU numbers in cell lysates. Shown are means plus standard deviations from three experiments conducted in triplicate. Statistical analysis was conducted by the Mann-Whitney test. (B–D) Cxcl2, IL-1β and TNF-α concentrations in 24-h culture supernatants of bone marrow macrophages cultured in the presence of M-CSF (M-CSF-MΦ) isolated from WT, TLR4-/- or 3d mice and stimulated with increasing amounts (MOIs of 5, 10, and 20) of bacteria or with LPS (0.1 µg/ml). ns, not significant; *, p<0.05 as determined by the Mann-Whitney test. The dotted lines indicate the limits of detection of the tests.
In view of the important role of GM-CSF in macrophage differentiation, we also used bone marrow-derived MΦ cultured in the presence of this growth factor. Notably, while WT GM-CSF-MΦ produced high levels of IL-12p70, IL-1β and IFN-β in response to K. pneumoniae, the production of IL-12p70 and IL-1β was markedly impaired in 3d GM-CSF-MΦ, with the extent of reduction surpassing that observed in TLR4-deficient macrophages. (Figures 4A. B). However, the levels of IFN-β, Cxcl1, Cxcl2 and TNF-α were decreased to a similar degree in 3d and TLR4-KO MΦ (Figures 4C–F). All together, these data revealed that endosomal TLRs are crucial for the production of pro-inflammatory cytokines, particularly IL-12p70 and IL-1β, by bone marrow derived macrophages stimulated with K. pneumoniae. Furthermore, the presence of TLR4 cannot compensate for this defect.
Figure 4. Cytokine responses to K. pneumoniae in GM-CSF polarized macrophages. (A–F) IL-12p70, IL-1β, IFN-β, Cxcl1, Cxcl2 and TNF-α protein levels in 24-h culture supernatants of macrophages (GM-CSF-MΦ) obtained from the bone marrows of WT, TLR4-/- or 3d mice, cultured in the presence of GM-CSF and stimulated with increasing amounts (MOI of 5, 10, and 20) of bacteria or with LPS (0.1 µg/ml). Shown are means plus standard deviations from three experiments conducted in triplicate. *, p<0.05 as determined by the Mann-Whitney test. The dotted lines indicate the limits of detection of the tests.
In subsequent experiments, we investigated the role of endosomal TLRs in the recognition of K. pneumoniae by alveolar macrophages (AM), given the critical role that these cells play in immune defenses against pulmonary infections. Consistent with our previous findings in bone marrow-derived macrophages, the levels of TNF-α, Cxcl2, IL-12p70, and IFN-β were significantly reduced in 3d AM following stimulation with K. pneumoniae. These results suggest that nucleic acid-sensing TLRs play a key role in the response of alveolar macrophages to K. pneumoniae infection (Figures 5A–D). To explore whether these effects are linked to transcriptional regulation, we assessed mRNA levels for various cytokines in AM stimulated with K. pneumoniae (Figures 5E–H). Cytokine mRNA levels increased significantly within the first few hours after bacterial stimulation, peaking at 2-4h post-infection. While IL-12p40, IL-1β, and Cxcl2 mRNA levels were only moderately impaired in 3d AM, IL-12p35 mRNA expression was markedly reduced, suggesting that the pronounced decrease in IL-12p70 levels observed in AM supernatants is secondary to a selective reduction in IL-12p35 mRNA transcription (Figure 5F).
Figure 5. Role of endosomal TLRs in cytokine response to K. pneumoniae by alveolar macrophages. (A–D) Alveolar macrophages (MΦ) from WT, TLR4-/- or 3d mice were stimulated with K. pneumoniae (MOI of 10), and cytokine concentrations were measured in 24-h culture supernatants. The dotted lines indicate the limits of detection of the tests. Means ± SD from three independent experiments conducted in triplicate. ns, not significant, *, p<0.05 as determined by the Mann-Whitney test. The dotted lines indicate the limits of detection of the tests. (E–H) RT-qPCR assessment of cytokine mRNA levels in GM-CSF-polarized bone marrow macrophages at different times after stimulation with K. pneumoniae (MOI of 10). *, p<0.05 as determined by the Mann-Whitney test.
Overall, impaired cytokine gene transcription and increased susceptibility to infection in the absence of nucleic acid-sensing endosomal receptors suggest a role for K. pneumoniae DNA and/or RNA in stimulating innate immune responses. To gain further insights, we purified K. pneumoniae DNA and RNA and used them to stimulate GM-CSF-polarized macrophages. Both nucleic acid types could induce the release of IFN-β and IL-12p70 from these cells in a dose-dependent manner, with RNA showing significantly higher potency (Figures 6A, B). Responses to nucleic acids, but not to LPS, were completely abrogated in 3d macrophages. Moreover, GM-CSF-polarized macrophages lacking TLR7 or TLR13 were partially unable to respond to RNA, while macrophages lacking TLR9 were defective in their ability respond DNA (Figures 6C, D).
Figure 6. RNA from K. pneumoniae induces cytokine production by activating endosomal TLRs in GM-CSF-polarized bone marrow macrophages. (A–D) Production of IFN-β and IL-12p70 in GM-CSF-polarized bone marrow macrophages obtained from the indicated mouse strains and stimulated with graded doses (0.01, 0.1, 1 and 10 μg/ml; A, B), or with 10 μg/ml (C, D) of purified DNA or RNA from K. pneumoniae. DNA and RNA were complexed with N-[1-(2,3-dioleoyloxy) propyl]-N, N, N-trimethylammonium methyl-sulfate (DOTAP) before cell stimulation. (E–G) Production of IL-12p70, IL-1β and IFN-β in GM-CSF-polarized bone marrow macrophages obtained from the indicated mouse strains and stimulated with K. pneumoniae (MOI 10). In all experiments, cytokine concentrations were determined in culture supernatants obtained at 24 h after stimulation, using E. coli LPS (0.1 μg/ml) as a positive control. The dotted lines indicate the limits of detection of the tests. Data are expressed as means ± SD of three independent experiments conducted in duplicate. *, p<0.05; as determined by the Mann-Whitney test. ns, not significant.
To assess the contribution of bacterial RNA to the overall ability of whole K. pneumoniae to induce cytokine production, we tested macrophages from mice lacking one or more RNA-sensing TLRs (TLR3, 7 and 13) in comparison to the DNA-sensing receptor TLR9. Moderate, though significant, reductions in IL-12p70, IL-1β and IFN-β responses to K. pneumoniae were observed in GM-CSF-MΦ from mice lacking TLR7, 9 or 13, but not in cells lacking TLR3 (Figures 6E–G). However, such responses were almost completely abrogated in triple-KO cells simultaneously lacking TLR7, 9 and 13, thereby phenocopying 3d cells (Figures 6E–G). Collectively, these data indicate that K. pneumoniae nucleic acids are potent inducers of proinflammatory cytokines, particularly IL-12p70, in macrophages, and that recognition of bacterial RNA through TLR7 or TLR13 contributes to these responses, similar to TLR9-mediated DNA recognition.
Defective reactive oxygen species (ROS) production has been linked to hypersusceptibility to K. pneumoniae (45). Given the crucial role of neutrophils in bacterial killing, we investigated whether neutrophils lacking endosomal TLRs are impaired in their ability to produce ROS in response to K. pneumoniae. Bone marrow-derived neutrophils were exposed to increasing concentrations of K. pneumoniae, and ROS production was assessed using a fluorescence flow cytometry assay. Under these conditions, K. pneumoniae induced ROS production, which was primarily dependent on TLR signaling, as evidenced by an over 80% reduction in ROS levels in neutrophils lacking the TLR adaptor MyD88. Notably, neutrophils from 3d or TLR4-deficient mice were also significantly impaired in their ROS production (Figure 7A). Additionally, bactericidal activity was significantly, though moderately, reduced in 3d and TLR4-deficient neutrophils (Figure 7B). Collectively, these data indicate that TLRs, and in particular endosomal TLRs and TLR4, play an important role in neutrophil ROS production and in the ability of these cells to kill K. pneumoniae.
Figure 7. Endosomal TLRs are required for optimal (K) pneumoniae killing. (A) Fluorescence intensities (ΔMFI) of bone marrow derived neutrophils obtained from WT, TLR4-/- or 3d mice stimulated with the indicated MOIs of (K) pneumoniae and examined by fluorescence flow cytometry using the CellROX fluorescent reagent. Data are expressed as means ± SD of three independent experiments conducted in duplicate. *, p<0.05 as determined by Mann-Whitney test. (B) Killing of (K) pneumoniae by bone marrow-derived neutrophils from WT, TLR4-/- or 3d mice. Cells were exposed to (K) pneumoniae (1x105 CFU/well) and bacterial viability was determined at the indicated time points. Data are expressed as means ± standard deviations of three independent experiments conducted in duplicate. *, p<0.05 as determined by the Mann-Whitney test.
Considerable efforts have been made in recent years to understand the mechanisms underlying innate immune responses to K. pneumoniae infections, as this knowledge is crucial for elucidating the pathogenesis of the disease and developing alternative therapeutic strategies (46). Previous studies have highlighted the central role of TLRs in K. pneumoniae recognition with marked susceptibility to infection observed in the absence of TLR adaptors such as MyD88, TRIF, and TIRAP (47, 48). While the roles of a few isolated receptors (including TLR2, TLR4, and TLR9) have been explored, the relative contributions of these and other TLRs to the host’s overall ability to control infection, as well as the potential interactions between these receptors, remain poorly understood. In particular, the role of nucleic acid-sensing TLRs in defense against K. pneumoniae have not been fully examined, with only a few studies addressing this aspect (20, 22, 49). Curiously, while the absence of TLR9 impairs host defenses to a lesser extent than the absence of TLR4 (20), the lack of TLR3 paradoxically enhances bacterial clearance via a macrophage-dependent mechanism (46).
Here we show that when the function of multiple nucleic acids sensing TLRs is compromised —such as in 3d mice with a loss-of-function mutation in the UNC93B1 chaperone— anti-K. pneumoniae host defenses are markedly impaired. This phenotype is as severe as that observed in TLR4-KO mice and nearly as severe as in MyD88-deficient mice. Notably, the increased lethality observed in all these mouse strains is linked to an impaired ability to produce optimal levels of chemotactic and pro-inflammatory cytokines in vivo. This deficiency leads to defective neutrophil recruitment to the lungs, uncontrolled pathogen growth, and systemic bacterial dissemination. The reduced cytokine levels observed in vivo were mirrored by reduced cytokine production by both bone marrow derived and alveolar macrophages infected in vitro. However, the contribution of other cell types (such as epithelial cells) cannot be ruled out and this requires further investigation.
Interestingly, we found that 3d macrophages are more severely impaired than TLR4 KO macrophages in their ability to produce IL-12p70 in response to K. pneumoniae, although both cell types show similar deficits in producing other cytokines. The defective IL-12p70 release by 3d macrophages was linked to impaired activation of the gene encoding the IL-12p35 subunit, which may be associated with selective targeting of this gene by nucleic acid-sensing TLRs through the activation of IFN regulatory transcription factors 1 and 5 (30, 50, 51). IL-12p70 is primarily produced by mononuclear phagocytes and plays a key role in inducing IFN-γ, a critical antibacterial factor, in group 1 innate lymphoid cells and T lymphocytes (49). In this study, IFN-γ peaked significantly later than IL-12p70 in the lungs of infected animals, suggesting that the reduced IFN-γ levels observed in 3d mice may, at least in part, be due to defective IL-12p70 production by mononuclear phagocytes early during infection.
Notably, macrophages lacking isolated endosomal receptors such as TLR3, 7, 9 and 13 exhibited no or only slight defects in cytokine production, suggesting that these receptors can compensate for each other’s absence. This redundancy may have evolved to prevent pathogens from evading immune detection. However, the combined loss of TLR7, 9 and 13 resulted in severely impaired cytokine production, indicating that the presence of cell surface receptors (e.g. TLR4 and TLR2) and cytosolic innate immune sensors cannot compensate for lack of endosomal TLR function. Indeed, the absence of TLR2 had no effect on cytokine responses to K. pneumoniae and was associated with only a slight increase in lethality late during infection, consistent with previous studies (26). Thus, while confirming the fundamental importance of LPS recognition by TLR4 in K. pneumoniae sensing, we demonstrate here that an equally essential role is played by a complementary endosomal receptor system by which TLR7, 9 and 13 cooperate in detecting the presence of different forms of K. pneumoniae nucleic acids, likely released by bacterial digestion in phagolysosomes (30).
As mentioned above, triple KO cells simultaneously lacking TLR7, 9 and 13 produced much lower cytokine levels than those lacking only TLR9. This suggests that, in addition to the DNA-sensing TLR9, RNA-sensing TLR7 and 13 play significant roles in cytokine responses against K. pneumoniae, as supported by the potent immune-stimulating effects of bacterial RNA compared to DNA. Collectively, our data highlight the significant role of prokaryotic RNA in K. pneumoniae immune sensing, extending to Gram-negative bacteria similar findings previously observed with pyogenic Gram-positive cocci (30, 32, 33, 52–54). These results lend support to the emerging view that RNA is an important target of bacterial recognition even in pathogens where LPS has traditionally been considered the dominant immune-activating signal. For example, blockade of the RNA sensing TLR8 receptor significantly impaired the production of IL-12p70 and IL-1β in human monocytes infected with P. aeruginosa (51). It should be noted, in this respect, that human TLR8 plays an important role in recognizing pathogens that are sensed predominantly by TLR13 in murine systems, as TLR13 is absent in humans, while TLR8 is almost completely nonfunctional in mice (31–33, 55, 56). Finally, we reveal a novel function of TLRs in anti-K. pneumoniae defenses, linked to the ability of TLR4 and endosomal TLRs to enhance ROS production and bactericidal activity in neutrophils. These findings align with the emerging view that TLRs can promote ROS responses to bacteria (13, 14).
In conclusion, our data demonstrate that defective endosomal receptor function leads to hypersusceptibility to K. pneumoniae infection, primarily due to impaired cytokine production by macrophages, reduced neutrophil recruitment and defective bacterial killing by neutrophils. Future studies will investigate the contributions of other cell types, such as epithelial cells and innate lymphoid cells, to TLR-dependent anti-K. pneumoniae responses. Of note, our findings also highlight the significant role of RNA as a target for innate immune recognition of K. pneumoniae. These insights may prove valuable in developing strategies to stimulate these receptors, offering potential therapeutic avenues for treating infections caused by antibiotic-resistant strains.
The authors acknowledge that the data presented in this study have been deposited and are publicly available at the following links https://figshare.com/s/cb9e1c04eb7d44b7d50a and doi: 10.6084/m9.figshare.28677941.
The animal study was approved by Animal Welfare Committee of the University of Messina (OPBA) and Ministero della Salute of Italy. The study was conducted in accordance with the local legislation and institutional requirements.
GD: Conceptualization, Data curation, Investigation, Methodology, Writing – original draft, Writing – review & editing. AF: Conceptualization, Data curation, Investigation, Methodology, Writing – original draft, Writing – review & editing. GL: Conceptualization, Investigation, Writing – original draft, Writing – review & editing. FC: Investigation, Writing – review & editing. MV: Conceptualization, Supervision, Writing – review & editing. IV: Conceptualization, Supervision, Writing – review & editing. PL: Conceptualization, Writing – review & editing. AB: Investigation, Methodology, Writing – review & editing. FG: Investigation, Methodology, Writing – review & editing. LF: Investigation, Methodology, Writing – review & editing. GT: Conceptualization, Data curation, Investigation, Writing – original draft, Writing – review & editing. CB: Conceptualization, Funding acquisition, Investigation, Writing – original draft, Writing – review & editing.
The author(s) declare that financial support was received for the research and/or publication of this article. This study was supported by Research Projects of National Interest (Prin 2022 PNRR, CUP J53D23014110001; identification code P2022BNCKS) funding awarded to C.B by the Ministry of University and Research (MUR) of Italy. Publication expenses were sustained by the University of Messina through the APC initiative. The author(s) declare that financial support was received for the research, authorship, and/or publication of this article.
Authors GT, CB were employed by Scylla Biotech Srl.
The remaining authors declare that the research was conducted in the absence of any commercial or financial relationships that could be construed as a potential conflict of interest.
The author(s) declare that no Generative AI was used in the creation of this manuscript.
All claims expressed in this article are solely those of the authors and do not necessarily represent those of their affiliated organizations, or those of the publisher, the editors and the reviewers. Any product that may be evaluated in this article, or claim that may be made by its manufacturer, is not guaranteed or endorsed by the publisher.
1. Podschun R, Ullmann U. Klebsiella spp. as nosocomial pathogens: epidemiology, taxonomy, typing methods, and pathogenicity factors. Clin Microbiol Rev. (1998) 11:589–603. doi: 10.1128/CMR.11.4.589
2. Podschun R, Pietsch S, Höller C, Ullmann U. Incidence of Klebsiella species in surface waters and their expression of virulence factors. Appl Environ Microbiol. (2001) 67:3325–7. doi: 10.1128/AEM.67.7.3325-3327.2001
3. Paczosa MK, Mecsas J. Klebsiella pneumoniae: Going on the offense with a strong defense. Microbiol Mol Biol Rev. (2016) 80:629–61. doi: 10.1128/MMBR.00078-15
4. Gorrie CL, Mirčeta M, Wick RR, Judd LM, Lam MMC, Gomi R, et al. Genomic dissection of Klebsiella pneumoniae infections in hospital patients reveals insights into an opportunistic pathogen. Nat Commun. (2022) 13:3017. doi: 10.1038/s41467-022-30717-6
5. Barbadoro P, Labricciosa FM, Recanatini C, Gori G, Tirabassi F, Martini E, et al. Catheter-associated urinary tract infection: Role of the setting of catheter insertion. Am J Infect Control. (2015) 43:707–10. doi: 10.1016/j.ajic.2015.02.011
6. Holt KE, Wertheim H, Zadoks RN, Baker S, Whitehouse CA, Dance D, et al. Genomic analysis of diversity, population structure, virulence, and antimicrobial resistance in Klebsiella pneumoniae, an urgent threat to public health. Proc Natl Acad Sci U S A. (2015) 112:E3574–81. doi: 10.1073/pnas.1501049112
7. Lam MMC, Wick RR, Wyres KL, Gorrie CL, Judd LM, Jenney AWJ, et al. Genetic diversity, mobilisation and spread of the yersiniabactin-encoding mobile element ICEKp in Klebsiella pneumoniae populations. Microb Genom. (2018) 4(9):e000196. doi: 10.1099/mgen.0.000196
8. Wyres KL, Holt KE. Klebsiella pneumoniae as a key trafficker of drug resistance genes from environmental to clinically important bacteria. Curr Opin Microbiol. (2018) 45:131–9. doi: 10.1016/j.mib.2018.04.004
9. De Gaetano GV, Lentini G, Famà A, Coppolino F, Beninati C. In vivo role of two-component regulatory systems in models of urinary tract infections. Pathogens. (2023) 12(1):119. doi: 10.3390/pathogens12010119
10. De Gaetano GV, Lentini G, Famà A, Coppolino F, Beninati C. Antimicrobial resistance: Two-component regulatory systems and multidrug efflux pumps. Antibiotics (Basel). (2023) 12(6):965. doi: 10.3390/antibiotics12060965
11. Li L, Ma J, Cheng P, Li M, Yu Z, Song X, et al. Roles of two-component regulatory systems in Klebsiella pneumoniae: Regulation of virulence, antibiotic resistance, and stress responses. Microbiol Res. (2023) 272:127374. doi: 10.1016/j.micres.2023.127374
12. Coppolino F, Berbiglia A, Lentini G, Famà A, Pietrocola G, Teti G, et al. Role of the saeRS two-component regulatory system in group B streptococcus biofilm formation on human fibrinogen. Microorganisms. (2024) 12(10):2096. doi: 10.3390/microorganisms12102096
13. Ballén V, Gabasa Y, Ratia C, Ortega R, Tejero M, Soto S. Antibiotic resistance and virulence profiles of klebsiella pneumoniae strains isolated from different clinical sources. Front Cell Infect Microbiol. (2021) 11:738223. doi: 10.3389/fcimb.2021.738223
14. Available online at: https://www.who.int/publications/m/item/pathogens-prioritization-a-scientific-framework-for-epidemic-and-pandemic-research-preparedness.
15. Li D, Wu M. Pattern recognition receptors in health and diseases. Signal Transduct Target Ther. (2021) 6:291. doi: 10.1038/s41392-021-00687-0
16. Vijay K. Toll-like receptors in immunity and inflammatory diseases: Past, present, and future. Int Immunopharmacol. (2018) 59:391–412. doi: 10.1016/j.intimp.2018.06.044
17. Gerold G, Zychlinsky A, de Diego JL. What is the role of Toll-like receptors in bacterial infections? Semin Immunol. (2007) 19:41–7. doi: 10.1016/j.smim.2006.12.003
18. Liu G, Zhang H, Zhao C. Evolutionary history of the toll-like receptor gene family across vertebrates. Genome Biol Evol. (2020) 12:3615–34. doi: 10.1093/gbe/evz266
19. Cen X, Liu S, Cheng K. The role of toll-like receptor in inflammation and tumor immunity. Front Pharmacol. (2018) 9:878. doi: 10.3389/fphar.2018.00878
20. Bhan U, Lukacs NW, Osterholzer JJ, Newstead MW, Zeng X, Moore TA, et al. TLR9 is required for protective innate immunity in Gram-negative bacterial pneumonia: role of dendritic cells. J Immunol. (2007) 179:3937–46. doi: 10.4049/jimmunol.179.6.3937
21. Jeyaseelan S, Young SK, Yamamoto M, Arndt PG, Akira S, Kolls JK, et al. Toll/IL-1R domain-containing adaptor protein (TIRAP) is a critical mediator of antibacterial defense in the lung against Klebsiella pneumoniae but not Pseudomonas aeruginosa. J Immunol. (2006) 177:538–47. doi: 10.4049/jimmunol.2200522
22. Bhan U, Ballinger MN, Zeng X, Newstead MJ, Cornicelli MD, Standiford TJ. Cooperative interactions between TLR4 and TLR9 regulate interleukin 23 and 17 production in a murine model of gram negative bacterial pneumonia. PloS One. (2010) 5:e9896. doi: 10.1371/journal.pone.0009896
23. Hernandez A, Zhou J, Bohannon JK, McBride MA, Gibson-Corley KN, Patil NK, et al. Intrapulmonary treatment with a novel tlr4 agonist confers protection against klebsiella pneumonia. Shock. (2022) 58:295–303. doi: 10.1097/SHK.0000000000001977
24. Hunt JJ, Astley R, Wheatley N, Wang JT, Callegan MC. TLR4 contributes to the host response to Klebsiella intraocular infection. Curr Eye Res. (2014) 39:790–802. doi: 10.3109/02713683.2014.883412
25. Li S, Yang P, Xu L, Li M. Blocking of Birc3/TLR4/Myd88 signaling protects carbapenem-resistant klebsiella pneumoniae in a mouse model of infection. Transpl Immunol. (2021) 69:101464. doi: 10.1016/j.trim.2021.101464
26. Schurr JR, Young E, Byrne P, Steele C, Shellito JE, Kolls JK. Central role of toll-like receptor 4 signaling and host defense in experimental pneumonia caused by Gram-negative bacteria. Infect Immun. (2005) 73:532–45. doi: 10.1128/IAI.73.1.532-545.2005
27. Regueiro V, Moranta D, Campos MA, Margareto J, Garmendia J, Bengoechea JA. Klebsiella pneumoniae increases the levels of Toll-like receptors 2 and 4 in human airway epithelial cells. Infect Immun. (2009) 77:714–24. doi: 10.1128/IAI.00852-08
28. Wieland CW, van Lieshout MH, Hoogendijk AJ, van der Poll T. Host defence during Klebsiella pneumonia relies on haematopoietic-expressed Toll-like receptors 4 and 2. Eur Respir J. (2011) 37:848–57. doi: 10.1183/09031936.00076510
29. Cai S, Batra S, Shen L, Wakamatsu N, Jeyaseelan S. Both TRIF- and MyD88-dependent signaling contribute to host defense against pulmonary Klebsiella infection. J Immunol. (2009) 183:6629–38. doi: 10.4049/jimmunol.0901033
30. Mancuso G, Gambuzza M, Midiri A, Biondo C, Papasergi S, Akira S, et al. Bacterial recognition by TLR7 in the lysosomes of conventional dendritic cells. Nat Immunol. (2009) 10:587–94. doi: 10.1038/ni.1733
31. Signorino G, Mohammadi N, Patanè F, Buscetta M, Venza M, Venza I, et al. Role of Toll-like receptor 13 in innate immune recognition of group B streptococci. Infect Immun. (2014) 82:5013–22. doi: 10.1128/IAI.02282-14
32. Famà A, Midiri A, Mancuso G, Biondo C, Lentini G, Galbo R, et al. Nucleic acid-sensing toll-like receptors play a dominant role in innate immune recognition of pneumococci. mBio. (2020) 11(2):e00415–20. doi: 10.1128/mBio.00415-20
33. Lentini G, Famà A, De Gaetano GV, Galbo R, Coppolino F, Venza M, et al. Role of endosomal TLRs in staphylococcus aureus infection. J Immunol. (2021) 207:1448–55. doi: 10.4049/jimmunol.2100389
34. Lentini G, Famà A, De Gaetano GV, Coppolino F, Mahjoub AK, Ryan L, et al. Caspase-8 inhibition improves the outcome of bacterial infections in mice by promoting neutrophil activation. Cell Rep Med. (2023) 4:101098. doi: 10.1016/j.xcrm.2023.101098
35. De Gaetano GV, Pietrocola G, Romeo L, Galbo R, Lentini G, Giardina M, et al. The Streptococcus agalactiae cell wall-anchored protein PbsP mediates adhesion to and invasion of epithelial cells by exploiting the host vitronectin/α. Mol Microbiol. (2018) 110:82–94. doi: 10.1111/mmi.14084
36. De Gaetano GV, Lentini G, Galbo R, Coppolino F, Famà A, Teti G, et al. Invasion and trafficking of hypervirulent group B streptococci in polarized enterocytes. PloS One. (2021) 16:e0253242. doi: 10.1371/journal.pone.0253242
37. De Gaetano GV, Coppolino F, Lentini G, Famà A, Cullotta C, Raffaele I, et al. Streptococcus pneumoniae binds collagens and C1q via the SSURE repeats of the PfbB adhesin. Mol Microbiol. (2022) 117:1479–92. doi: 10.1111/mmi.14920
38. De Gaetano GV, Lentini G, Coppolino F, Famà A, Pietrocola G, Beninati C. Engagement of α3β1 and α2β1 integrins by hypervirulent Streptococcus agalactiae in invasion of polarized enterocytes. Front Microbiol. (2024) 15:1367898. doi: 10.3389/fmicb.2024.1367898
39. Lentini G, De Gaetano GV, Famà A, Galbo R, Coppolino F, Mancuso G, et al. Neutrophils discriminate live from dead bacteria by integrating signals initiated by Fprs and TLRs. EMBO J. (2022) 41:e109386. doi: 10.15252/embj.2021109386
40. Coppolino F, Romeo L, Pietrocola G, Lentini G, De Gaetano GV, Teti G, et al. Lysine residues in the MK-rich region are not required for binding of the pbsP protein from group B streptococci to plasminogen. Front Cell Infect Microbiol. (2021) 11:679792. doi: 10.3389/fcimb.2021.679792
41. Coppolino F, De Gaetano GV, Claverie C, Sismeiro O, Varet H, Legendre R, et al. The SaeRS two-component system regulates virulence gene expression in group B. mBio. (2024) 15(9):e0197524. doi: 10.1128/mbio.01975-24
42. Claverie C, Coppolino F, Mazzuoli MV, Guyonnet C, Jacquemet E, Legendre R, et al. Signal-independent activation reveals two component regulatory networks. Nat Commun. (2024) 15:9175. doi: 10.1038/s41467-024-53439-3
43. Tabeta K, Hoebe K, Janssen EM, Du X, Georgel P, Crozat K, et al. The Unc93b1 mutation 3d disrupts exogenous antigen presentation and signaling via Toll-like receptors 3, 7 and 9. Nat Immunol. (2006) 7:156–64. doi: 10.1038/ni1297
44. Jeon HY, Park JH, Park JI, Kim JY, Seo SM, Ham SH, et al. Cooperative interactions between toll-like receptor 2 and toll-like receptor 4 in murine. J Microbiol Biotechnol. (2017) 27:1529–38. doi: 10.4014/jmb.1704.04039
45. Nguyen GT, Shaban L, Mack M, Swanson KD, Bunnell SC, Sykes DB, et al. SKAP2 is required for defense against K. pneumoniae infection and neutrophil respiratory burst. Elife. (2020) 9:e56656. doi: 10.7554/eLife.56656
46. Bengoechea JA, Sa Pessoa J. Klebsiella pneumoniae infection biology: living to counteract host defences. FEMS Microbiol Rev. (2019) 43:123–44. doi: 10.1093/femsre/fuy043
47. van Lieshout MH, Blok DC, Wieland CW, de Vos AF, van ‘t Veer C, van der Poll T. Differential roles of MyD88 and TRIF in hematopoietic and resident cells during murine gram-negative pneumonia. J Infect Dis. (2012) 206:1415–23. doi: 10.1093/infdis/jis505
48. van Lieshout MH, Florquin S, Van’t Veer C, de Vos AF, van der Poll T. TIR-domain-containing adaptor-inducing interferon-β (TRIF) mediates antibacterial defense during gram-negative pneumonia by inducing interferon-x03B3. J Innate Immun. (2015) 7:637–46. doi: 10.1159/000430913
49. Suresh MV, Dolgachev VA, Zhang B, Balijepalli S, Swamy S, Mooliyil J, et al. TLR3 absence confers increased survival with improved macrophage activity against pneumonia. JCI Insight. (2019) 4(23):e131195. doi: 10.1172/jci.insight.131195
50. Negishi H, Fujita Y, Yanai H, Sakaguchi S, Ouyang X, Shinohara M, et al. Evidence for licensing of IFN-gamma-induced IFN regulatory factor 1 transcription factor by MyD88 in Toll-like receptor-dependent gene induction program. Proc Natl Acad Sci U S A. (2006) 103:15136–41. doi: 10.1073/pnas.0607181103
51. Moen SH, Ehrnström B, Kojen JF, Yurchenko M, Beckwith KS, Afset JE, et al. Human toll-like receptor 8 (TLR8) is an important sensor of pyogenic bacteria, and is attenuated by cell surface TLR signaling. Front Immunol. (2019) 10:1209. doi: 10.3389/fimmu.2019.01209
52. Deshmukh SD, Kremer B, Freudenberg M, Bauer S, Golenbock DT, Henneke P. Macrophages recognize streptococci through bacterial single-stranded RNA. EMBO Rep. (2011) 12:71–6. doi: 10.1038/embor.2010.189
53. Gratz N, Siller M, Schaljo B, Pirzada ZA, Gattermeier I, Vojtek I, et al. Group A streptococcus activates type I interferon production and MyD88-dependent signaling without involvement of TLR2, TLR4, and TLR9. J Biol Chem. (2008) 283:19879–87. doi: 10.1074/jbc.M802848200
54. Lentini G, Famà A, Biondo C, Mohammadi N, Galbo R, Mancuso G, et al. Neutrophils enhance their own influx to sites of bacterial infection via endosomal TLR-dependent cxcl2 production. J Immunol. (2020) 204:660–70. doi: 10.4049/jimmunol.1901039
55. Ehrnström B, Beckwith KS, Yurchenko M, Moen SH, Kojen JF, Lentini G, et al. Toll-like receptor 8 is a major sensor of group B streptococcus but not escherichia coli in human primary monocytes and macrophages. Front Immunol. (2017) 8:1243. doi: 10.3389/fimmu.2017.01243
Keywords: gram negative bacteria, pneumonitis, pattern recognition receptors, neutrophils, cytokines, bacterial RNA
Citation: De Gaetano GV, Famà A, Lentini G, Coppolino F, Venza M, Venza I, Laganà P, Berbiglia A, Grasso F, Fiore L, Teti G and Beninati C (2025) Role of endosomal toll-like receptors in immune sensing of Klebsiella pneumoniae. Front. Immunol. 16:1538425. doi: 10.3389/fimmu.2025.1538425
Received: 02 December 2024; Accepted: 18 March 2025;
Published: 03 April 2025.
Edited by:
Felix Ngosa Toka, Ross University School of Veterinary Medicine, Saint Kitts and NevisCopyright © 2025 De Gaetano, Famà, Lentini, Coppolino, Venza, Venza, Laganà, Berbiglia, Grasso, Fiore, Teti and Beninati. This is an open-access article distributed under the terms of the Creative Commons Attribution License (CC BY). The use, distribution or reproduction in other forums is permitted, provided the original author(s) and the copyright owner(s) are credited and that the original publication in this journal is cited, in accordance with accepted academic practice. No use, distribution or reproduction is permitted which does not comply with these terms.
*Correspondence: Giuseppe Teti, Z2lvdGV0aUBtYWMuY29t
†These authors have contributed equally to this work
Disclaimer: All claims expressed in this article are solely those of the authors and do not necessarily represent those of their affiliated organizations, or those of the publisher, the editors and the reviewers. Any product that may be evaluated in this article or claim that may be made by its manufacturer is not guaranteed or endorsed by the publisher.
Research integrity at Frontiers
Learn more about the work of our research integrity team to safeguard the quality of each article we publish.