- 1Cell Biotechnology Laboratory, Tianjin Cancer Hospital Airport Hospital, Tianjin, China
- 2National Clinical Research Center for Cancer, Tianjin, China
- 3Haihe Laboratory of Synthetic Biology, Tianjin, China
- 4Tianjin Medical University Cancer Institute & Hospital, Tianjin, China
Neoantigen-based cancer vaccine therapy represents a promising precision oncology strategy that targets unique tumor-specific mutations to elicit a robust immune response. This therapeutic approach is designed to harness the host’s immune response against tumor-specific neoantigens to eliminate cancer cells. The efficacy of neoantigen vaccines dependents on the coordinated action of diverse immune cells, including T lymphocytes, dendritic cells, B lymphocytes, natural killer cells, and macrophages. Each cell type plays a distinct and crucial role in recognizing, targeting, and destroying malignant cells. Understanding the mechanisms governing both individual and collective immune cell dynamics is crucial for therapeutic success. This comprehensive review systematically explores the mechanisms of neoantigen-specific immune cells, their dynamic interactions, and clinical application progress, aiming to unveil their potential value and future development in cancer treatment.
1 Introduction
Neoantigen-based cancer vaccine therapy represents a cutting-edge approach in precision oncology, leveraging unique tumor-specific mutations to elicit a robust and targeted immune response (1–6). Neoantigen-specific immune cells play a pivotal role in the success of neoantigen-based cancer vaccine therapies. A comprehensive characterization of these cells and their intricate interplay within the tumor microenvironment (TME) is essential for optimizing this therapeutic strategy and enhancing its clinical outcomes (7, 8). Tumor-infiltrating lymphocytes (TILs), a critical component of the TME, are lymphocytes that have migrated into the tumor tissue and consist of diverse subsets of T cells, B cells, and natural killer (NK) cells (9–13). The composition and functional states of immune cells within the TME largely determine the immune response to the tumor as well as patient prognosis (14). Each immune cell subset plays a distinct yet complementary role in recognizing and eliminating neoantigen-expressing tumor cells, leading to a potent and specific anti-tumor response (7, 15, 16).
Neoantigens are unique tumor-specific peptides derived from somatic mutations or aberrant gene expression in cancer cells (17). These peptides are recognized as “non-self” by the host immune system, thereby activating targeted anti-tumor immune responses (5, 18). Unlike traditional tumor antigens, neoantigens exhibit high specificity and individualization, providing unique targets for precision immunotherapy. Neoantigen-directed therapy aims to overcome the immunosuppressive TME and intratumoral heterogeneity by selectively activating tumor-specific immune cells and boosting anti-tumor immunity in vivo (19, 20). Neoantigen-based cancer vaccines can be developed as synthetic peptides, mRNA, DNA, or ex vivo-loaded dendritic cell(DC) formulations. These vaccines are tailored to the individual’s tumor mutational profile, ensuring a highly specific immune response (6, 21). Upon administration, these vaccines are processed by antigen-presenting cells (APCs), such as DC, which present the neoantigens to T cells, initiating and amplifying the anti-tumor immune response (22). Clinical trials have demonstrated the efficacy of neoantigen-based vaccines across multiple cancer types, particularly in melanoma, lung cancer, bladder cancer, and pancreatic cancer (23–30).
Despite the promising preclinical and early clinical data of neoantigen-based therapy, regulatory approval for their use in cancer patients remains pending. Currently, the majority of neoantigen-based personalized vaccine therapies are still in early stages of clinical development, focusing on enhancing vaccine formulations, delivery methods, and synergistic combination strategies with other therapies such as checkpoint inhibitors to improve efficacy (7, 8, 21, 31, 32). Notable progress includes the combination of V940 (mRNA-4157), a personalized neoantigen vaccine, with pembrolizumab (KEYTRUDA®) is rapidly advancing and entering Phase 3 clinical trials to evaluate its safety and efficacy compared to KEYTRUDA alone in individuals with resected high-risk (Stage IIB-IV) melanoma (24, 33). Furthermore, a neoantigen-targeting tumor-infiltrating lymphocyte adoptive cell transfer (TIL-ACT) approach combined with pembrolizumab demonstrated favorable effects in a breast cancer patient (34). These clinical findings have also provided insights into the spectrum of immune cell populations that respond to neoantigens, unlocking the potential of neoantigen-directed adoptive cell transfer and cancer vaccination therapy. The complexity and dynamic nature of the Tumor Immune Microenvironment (TIME) critically influence immunotherapy efficacy.
Successful neoantigen-directed immunotherapy relies on the synergistic interactions among diverse immune cell populations to overcome tumor immune evasion and achieve an effective anti-tumor response. Elucidating the phenotypic and functional characteristics of neoantigen-reactive immune cells is imperative for improving the efficacy of neoantigen-driven therapeutics. This review presents a comprehensive discussion of tumor neoantigen-activated immune cells, aiming to guide the development of novel therapeutic strategies and optimize treatment outcomes for neoantigen vaccines.
2 Neoantigen and tumor immune microenvironment
The tumor immune microenvironment (TIME) is a highly complex and dynamically heterogeneous ecosystem that acts as a “double-edged sword”, profoundly influencing the efficacy of immunotherapies and other treatments (35–37). The TIME encompasses neoplastic cells, immune cell populations, stromal components, an intricate network of signaling molecules, and the extracellular matrix, collectively influencing the efficacy of neoantigen-based cancer vaccine therapies (15, 38, 39). Tumor-infiltrating lymphocytes (TILs), a critical component of the TIME, comprise heterogeneous populations of lymphocytes that migrate from circulation into tumor tissue. Their composition varies significantly among tumor types and patients, directly impacting immune responses and clinical outcomes (40, 41). TILs encompass a diverse array of immune cell subsets, including various T cell populations (CD8+ cytotoxic T cells, CD4+ helper T cells, and regulatory T cells), B cells, and natural killer (NK) cells (42). The abundance and functionality of TILs, particularly cytotoxic CD8+ T cells, correlate strongly with treatment success and patient survival (43–45).
The TIME harbors various immunosuppressive elements, including malignant cells, cancer-associated fibroblasts (CAFs), vascular endothelial cells, myeloid-derived suppressor cells (MDSCs), tumor-associated macrophages (TAMs), regulatory T cells (Treg), and regulatory B cells, which collectively contribute to immune evasion and tumor progression (46–50). Within the TIME, A complex network of cytokines and chemokines orchestrates the recruitment, activation, differentiation, and function of immune cells. Pro-inflammatory cytokines, such as interferon-gamma (IFN-γ) and tumor necrosis factor-alpha (TNF-α), can potentiate anti-tumor immunity by promoting T cell activation, enhancing antigen presentation, and inducing tumor cell death (51, 52). In contrast, immunosuppressive cytokines, such as interleukin-10 (IL-10) and transforming growth factor-beta (TGF-β), attenuate immune responses by suppressing T cell proliferation, inhibiting antigen presentation, and promoting the development of regulatory T cells (53–57). Strategically modulating these cytokine and chemokine networks offers a promising approach to enhance the therapeutic efficacy of neoantigen vaccines and overcome immune resistance (56, 58, 59). Elucidating the complex interactions between neoantigen-specific immune cells and the TIME is fundamental for developing effective neoantigen-based therapies and predicting treatment outcomes.
Effective anti-tumor immunity depends on the coordinated response of multiple immune cell subsets within the TIME, particularly TILs, which critically influence the response to neoantigen-based cancer vaccine therapy (60). The phenotypic diversity and functional states of TILs serve as a critical indicator of the immune characteristics of the TIME (61). TILs mediate tumor cell death by recognizing by recognizing neoantigen-MHC complexes and releasing cytotoxic molecules like perforin and granzymes (14). They also secrete pro-inflammatory cytokines like IFN-γ and TNF-α that amplify the anti-tumor immune response and recruit additional immune cells, potentially leading to tumor regression and long-term remission (9, 62). Furthermore, TILs regulate tumor angiogenesis, invasion, and metastasis by modulating vascular endothelial growth factor (VEGF) signaling and matrix metalloproteinase activity (63). However, the immunosuppressive effects of the TIME can impair TIL function and persistence, representing a significant challenge in maintaining effective anti-tumor immunity (64, 65). High densities of functionally active CD8+ TILs are generally associated with improved prognosis, whereas elevated proportions of Tregs may indicate immunosuppression (66–69).
In conclusion, elucidating the complex interplay between TILs, the TIME, and neoantigen-based cancer vaccines is crucial for developing effective and personalized immunotherapeutic strategies. The success of neoantigen vaccines depends not only on the precise identification and selection of appropriate neoantigens but also on the ability to induce and sustain a favorable immune microenvironment that supports robust anti-tumor responses (70, 71). Future research should focus on developing innovative strategies to optimize the TIME, overcome immune evasion mechanisms, and enhance the efficacy of neoantigen-based therapies across diverse cancer types and patient populations. This may involve rational combination therapies, novel delivery systems, and personalized approaches that integrate multi-omics data to account for the unique characteristics of each patient’s tumor and immune landscape, ultimately paving the way for more effective and durable cancer treatments.
3 Neoantigen-specific immune cells
3.1 Neoantigen-specific T cells
Neoantigen-specific T cells function as the primary effectors in neoantigen-based immunotherapies (72–74). Advanced DNA and RNA sequencing technologies, coupled with sophisticated bioinformatics algorithms, have deepened our understanding of these cells (75, 76). Single-cell RNA sequencing (scRNA-seq) and immune repertoire profiling have revolutionized the characterization of T cell features and the ability to predict therapeutic outcomes (77, 78). The integration of paired single-cell T cell receptor sequencing (scTCR-seq) and scRNA-seq enables tracking TCR clonotype frequencies before and after vaccination, while concurrently providing insights into the cellular states and functions of T cells with specific TCR clonotypes (76, 77, 79–82). These findings have led to the identification of neoantigen-specific T cell gene signatures, facilitating the prediction of antitumor responses and the development of more effective immunotherapies.
Over 100 clinical trials of personalized neoantigen vaccines have been registered in ClinicalTrials.gov, with encouraging results in melanoma, lung cancer, glioblastoma, and pancreatic cancer (6, 26, 30, 83–85). Following administration, these vaccines are processed by APCs, triggering T cell-mediated anti-tumor immune responses (86, 87). The resulting neoantigen-reactive T cell clones demonstrate diverse characteristics, including antigen recognition specificity, TCR diversity, functional heterogeneity, and HLA restriction (73, 88, 89). Recent research have revealed the transcriptome profiles of these neoantigen-reactive T cells (90), providing insights that validate vaccine efficacy and inform the development of optimized neoantigen-specific T cell therapies.
Several phase I clinical trials demonstrate that neoantigens activate CD8+ T cells fostering long-term immune memory (26, 29, 30, 85, 88). A phase Ib trial of a personalized neoantigen vaccine for glioblastoma showed that vaccine-induced T cells infiltrate the brain tumor microenvironment and elicit a robust immune response. CD8+ T cells exhibited cytotoxicity markers (granzymes, perforin) without CCR7 expression, while some CD4+ T cells displayed regulatory properties through IL2RA and FOXP3 expression (26). Similar results in pancreatic ductal adenocarcinoma revealed expanded CD8+ T cells expressing cytotoxicity genes (PRF1, GZMB) and IFN-γ (30). Remarkably, numerous studies have identified CXCL13 as a key marker in neoantigen-recognizing T cells, correlating with immune checkpoint blockade (ICB) efficacy (25, 90–93). Lung cancer research has revealed tissue-resident memory T cell programs, including CD103, CD69, HOBIT, LINC02446, and CXCL13 expression (90). CXCL13, CD103, and GZMA can serve as markers for neoantigen-specific CD8+ T cells and show promise as potential predictors of neoantigen-driven therapy efficacy (91, 92). Researchers have developed a neoantigen-reactive T cell signature based on clonotype frequency, CD39 protein, and CXCL13 mRNA expression for identifying reactive TCRs in non-small cell lung cancer (NSCLC) (93). Current research indicates that neoantigen-specific CD8+ T cells express characteristic genes including cytotoxicity genes (PRF1, GZMB), exhaustion genes (PDCD1, LAYN, ENTPD1, TIGIT, LAG3, HAVCR2), and specific markers (CD103, CD95, CXCL13). However, a complete gene profile of neoantigen-specific T cells remains to be established.
Recent advances in paired scRNA-seq and TCR-seq have revealed clonotypic T cell signatures, enabling deeper insights into T cell responses to antigen exposure. A landmark study by Steven A. Rosenberg’s team in 2022 provided a comprehensive transcriptome profiling of neoantigen-specific T cells (94). Through analyzing transcriptomic profiles from CD8+ and CD4+ neoantigen-reactive TILs in 10 metastatic cancer patients, the study revealed distinct molecular patterns. Both CD4+ and CD8+ TILs with neoantigen-specific TCRs (NeoTCRs) expressed chemokine-related genes (CXCL13, CXCR6) and inhibitory/exhaustion markers (TIGIT, PDCD1, CD39, LAG3, TOX). CD8+ NeoTCR TILs specifically showed cytotoxicity genes (GZMA, GZMB, GZMK, IFN-γ, PRF1) and the tissue-residency marker ITGAE (CD103). Most notably, the study identified over 200 NeoTCR signature genes specific to neoantigen-specific CD8+ and CD4+ T cells, opening new possibilities for developing patient-specific neoantigen-targeting TCR immunotherapies.
Notably, neoantigen-reactive CD8+ T cells from peripheral blood lymphocytes (PBLs) exhibit distinct features (95). These rare cells, comprising less than 0.005% of PBLs, express memory and quiescence markers (SELL, LTB, KLF2, LGALS3) while showing reduced expression of dysfunction-associated genes (TOX, CXCL13, PRF1, GZMH, GNLY). The cells show reduced expression of dysfunctional genes (TOX, CXCL13, PRF1, GZMH, GNLY) while maintaining higher levels of immune function-related genes (COTL1, PASK, ALOX5AP, HLA-DRB1, HLA-DPA). The study also identified specific surface markers (CD8, CD45RO, HLA-DR, CD39, CD103) for isolating these cells (95). Supporting these findings, other research groups have identified a minor population of neoantigen-specific CD8+ T cells in peripheral blood, characterized by high expression of CD39, PDCD1, and Ki67 (96). While circulating neoantigen-reactive CD8+ T cells share several markers with their tumor-derived counterparts, including CD39, CD103, IFN-γ, and PDCD1, recent research has uncovered important distinctions. Notably, Circulating cells express higher levels of IFN-γ and MHC class II-associated genes but lower levels of exhaustion-related genes (ENTPD1, PDCD1, LAYN) compared to their tumor-resident counterparts (97).
Despite less extensive study, neoantigen-specific CD4+T cells also play a crucial role in the anti-tumor response, as demonstrated by multiple recent studies (25, 98, 99). Research using B16F10 murine melanoma models has shown that neoantigen-specific CD4+ T cells induce strong, long-lasting antitumor immune responses. The study revealed that CD4+ T cells with high or moderate TCR avidity showed similar in vivo proliferation when exposed to cross-presented tumor antigens, resulting in comparable therapeutic effects (98). This finding is consistent with previous study in neoantigen-specific CD8+ T cells, where high structural avidity correlates with enhanced tumor localization and elimination. These findings establish TCR avidity as a critical determinant of both CD4+ and CD8+ T cell responses in cancer immunotherapy (100). Recent phenotypic profiling have revealed diverse characteristics of neoantigen-reactive CD4+ T cells. In melanoma studies, these cells display CXCL13 expression and can be categorized into distinct subsets, including those expressing memory and T follicular helper markers, cytotoxicity markers (GZMA/K and PRF1), inhibitory receptors (PD1 and TIM3), and IFN-γ (25, 101, 102). Consistent with this, studies of stage IIIB/C or IVM1a/b melanoma patients demonstrated long-term persistence of neoantigen-specific T cell responses, with CD4+ T cells exhibiting both memory and exhaustion phenotypes (27, 101).
These studies have illuminated the genetic characteristics of neoantigen-specific CD4+ and CD8+ T cells, revealing shared markers including exhaustion-associated genes, cytotoxicity markers, and IFN-γ expression. As summarized in Table 1, the comprehensive gene profile of neoantigen-reactive T cells highlights their potential as both predictive biomarkers for vaccine efficacy and therapeutic targets for enhancing antitumor responses.
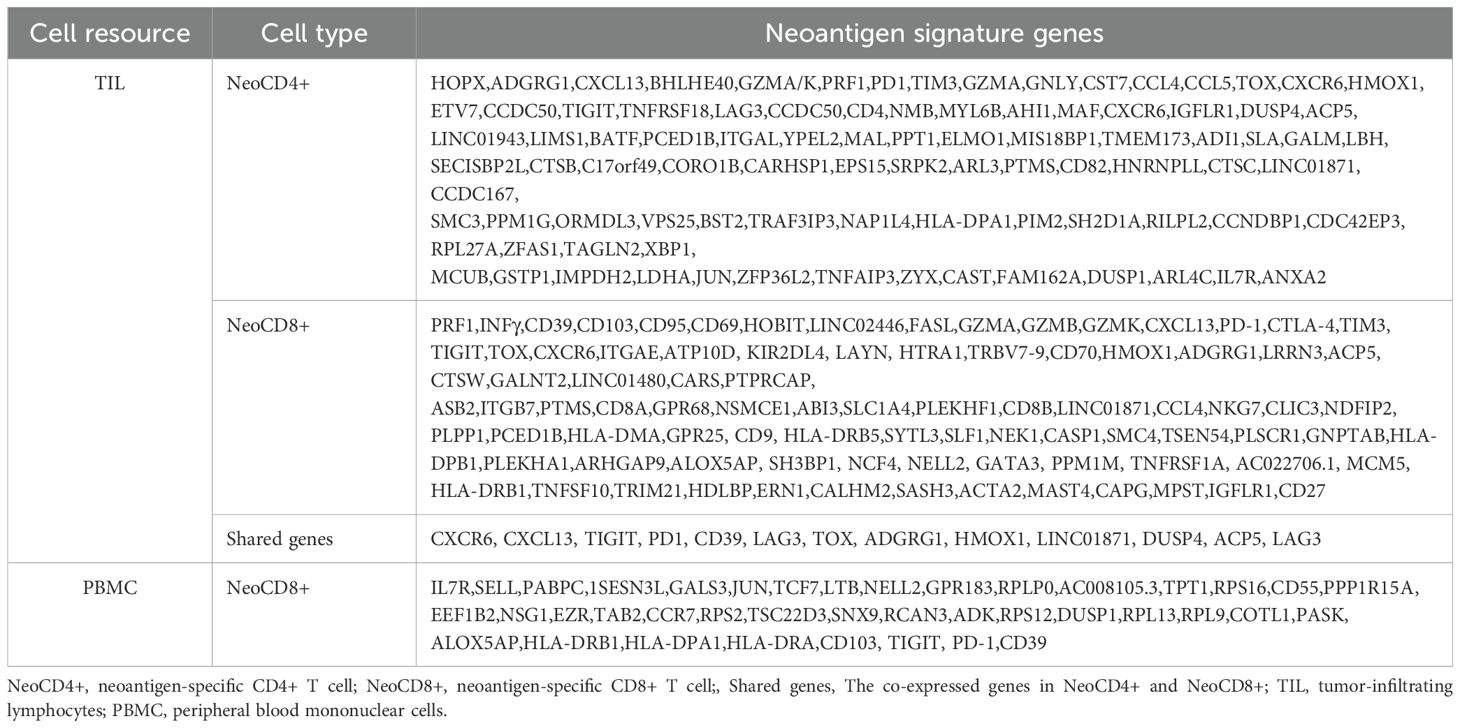
Table 1. Differential gene expression profiles of neoantigen-reactive T cells isolated from TIL and PBMC.
3.2 Neoantigen-specific B cells
B cells contribute to neoantigen-specific immune responses through dual mechanisms: generating antibodies against tumor-specific antigens and serving as APCs (103). These antibodies can mediate antibody-dependent cellular cytotoxicity (ADCC) and opsonization, resulting in the destruction of tumor cells (12, 104–106). In the meanwhile, B cells function as APCs, presenting neoantigens to T cells and facilitating their activation. The role of B cells in neoantigen vaccine responses represents an emerging area of research, holding significant potential for enhancing vaccine efficacy (11, 107).
Within the tumor microenvironment, various B cell populations orchestrate anti-tumor immunity, including follicular B cells, germinal center B cells, and plasma cells. Among them, follicular B cells of the B2 lineage with bimodal IgD expression predominate among tumor-infiltrating B cells (11, 105, 108–111). Different B cell subsets exhibit distinct roles in tumor neoantigen-driven immune responses (11). Specifically, follicular B cells and marginal zone B cells have been implicated in the production of antibodies against tumor neoantigens (103, 112). Furthermore, germinal center (GC) B cells posse the ability to identify neoantigens and actively enhance anti-tumor immunity by orchestrating the activation of autologous CD8+ T cells (113). Essentially, the recognition of neoantigens by B cells triggers tumor-specific responses involving both B cells and T follicular helper (TFH) cells, which subsequently amplify CD8+ T cell anti-tumor immunity via the cytokine IL-21. This mechanism highlights the pivotal role of B cell recognition of neoantigens in eliciting effective anti-tumor responses by CD8+ T cells (113). Further evidence of B cell functionality come from a murine glioblastoma model, where BVax derived from GBM patients induced the generation of 4-1BBL+ B cells, successfully triggering autologous CD8+ T cell activation through CD40/CD40L interaction and IFN-γ stimulation (114). Moreover, B cells can differentiate into memory B cells following neoantigen exposure, providing long-term immunity through their prolonged lifespan and ability to rapidly generate antibodies upon subsequent antigen encounters (115, 116). The clinical significance of these mechanisms is supported by recent findings showing that renal cell carcinoma patients with tumor cells bearing IgG antibodies demonstrate higher response rates to immune checkpoint inhibitors and experience prolonged progression-free survival (PFS) (117).
Tertiary lymphoid structures (TLS) act as sites for the maturation of B cells in situ, resulting in the generation of immunoglobulins (Ig)-producing plasma cells (118, 119). These Ig-producing plasma cells are often found in association with IgG antibodies bound to apoptotic tumor cells, correlating with a positive response to immunotherapy (117, 119, 120). Scientists hypothesize that this correlation stems from the direct anti-tumor effects of these cells, ultimately enhancing treatment efficacy (118). Recent advances in understanding B cell-specific checkpoint molecules have revealed their role in engaging adaptive immunity to promote anti-tumor responses and control tumor growth. Particularly significant is the discovery by Vijay K. Kuchroo’s team that B cell subsets expressing T cell immunoglobulin and mucin domain 1 (TIM-1, encoded by Havcr1) specifically proliferate in draining lymph nodes and enhance anti-tumor CD8+ and CD4+ T cell responses (121). A breakthrough study by Gao and colleagues has provided comprehensive insights into the pan-cancer B cell landscape, establishing a clear connection between TLS maturation, GC B cells, and favorable immunotherapy outcomes. Their work has identified two distinct B cell differentiation pathways in human cancers, with memory B cells (Bm) correlating with improved treatment responses and survival, while atypical memory (AtM) cells are associated with treatment resistance and an exhaustion phenotype developing independently of germinal centers (122). These collective findings highlight B cells as crucial players in neoantigen-driven antitumor immune responses, offering promising opportunities for cancer prevention and treatment. Continued advancement in our understanding of B cell biology and their interactions with other immune cells opens new avenues for developing effective cancer immunotherapies.
3.3 DCs-based neoantigen cancer vaccines
DCs, as highly specialized professional APCs, play a fundamental role in orchestrating cancer immunity (123). These cells are not only integral components of the TME but also possess the distinctive ability to cross-present tumor-associated antigens to T cells, thereby initiating specific antitumor immune responses (124, 125). A remarkable feature of DCs is their capacity to activate between 100 and 3000 T cells individually, demonstrating an efficiency 100-1000 fold higher than that of macrophages and B cells (126, 127).
The DC population exhibit significant heterogeneity and can be classified into different subsets based on their anatomical location, phenotypic characteristics, and functional properties. The principal subsets of DCs encompass conventional DCs (cDCs), plasmacytoid DCs (pDCs), and monocyte-derived DCs (moDCs) (128, 129). cDCs are further delineated into two main subsets: cDC1 and cDC2. Each subset serves distinct functions: cDC1s specialized in cross-presenting antigens to CD8+ T cells, while cDC2s present MHC II-associated antigens to CD4+ T cells, promoting Th1, Th2, and Th17 polarization (129–131). pDCs, predominantly present in the blood and lymphoid organs, are characterized by their production of type I interferons, which are essential for antiviral immune responses. Additionally, pDCs contribute to the activation of other immune cells, including NK cells, and B cells (132). In contrast, moDCs arise through differentiation from monocytes and predominantly reside in inflamed tissues, particularly within the TME. These cells share functional similarities with cDCs in their ability to capture antigens and present them to CD8+ T cells. Of particular significance is their crucial role in neoantigen-driven therapeutic strategies, where they effectively present tumor-specific neoantigens to T cells, thereby initiating and sustaining immune responses against cancer cells (125, 133–135).
Recent research has extensively mapped tumor-infiltrating DC characteristics across various cancer types, such as melanoma, hepatocellular carcinoma, head and neck squamous cell carcinoma, non-small cell lung cancer, cutaneous squamous cell carcinoma, ovarian cancer, breast cancer, and colorectal cancer (136, 137). Of particular interest is the identification of LAMP3+ DC clusters in multiple tumor types, which may originate from cDC1 and cDC2 and migrate to lymph nodes (136, 138, 139). Additionally, scientists have identified a conserved DC cluster enriched in immunoregulatory molecules (CD274, Pdcd1lg2, and CD200) and maturation genes(CD40, CCR7, and IL12b) in non-small-cell lung cancers (140). This DC cluster represents a molecular state acquired by both cDC1s and cDC2s upon sensing or uptake of cell-associated antigens, encompassing the induction of molecular programs specialized in antigen presentation and associated with the induction of regulatory, immunogenic, and migratory gene programs. Recent advances have led to the reclassification of certain cDC2s as ‘DC3s’, which show distinctive capabilities in priming naive CD8+ T cells into tissue-homing CD103+ T cells. DC3s have also been further characterized, and their development has been shown to be dependent on GM-CSF (141, 142).
The development of neoantigen-based DC vaccines has shown remarkable progress, with extensive evidence supporting their efficacy across various cancer types. These highly personalized vaccines utilize DCs derived from autologous peripheral blood monocytes. Multiple clinical studies have demonstrated promising results (143–145). Yang, Li et al. successfully developed a personalized neoantigen peptide-pulsed autologous DC vaccine for lung cancer treatment, demonstrating both safety and efficacy in eliciting neoantigen-specific T-cell responses (134). Similar success has been observed in breast cancer, where neoantigen-pulsed DCs effectively induced cytotoxic T lymphocyte responses when co-cultured with autologous peripheral lymphocytes (146, 147). Studies have explored the combination of personalized neoantigen-loaded DC vaccines with T-cell therapy for hepatocellular carcinoma, while further research demonstrated promising results by combining anti-PD1 with Neo-MoDC vaccines, achieving complete tumor regression in metastatic gastric cancer patients for over 25 months (135, 148).
However, despite these promising results, autologous DC-based vaccines face significant challenges, including limited reproducibility and manufacturing constraints. To overcome these limitations, researchers have developed alternative approaches using allogeneic dendritic cells. A notable breakthrough emerged with the development of an innovative allogeneic plasmacytoid dendritic cell (pDC) line platform. This system was specifically engineered to enhance the priming and expansion of neoantigen-specific T cells for cancer vaccines, offering advantages in both scalability and consistency of production (149). The effectiveness of this novel approach was demonstrated in a phase Ib clinical trial involving nine patients with metastatic stage IV melanoma, where the vaccine successfully primed antitumor CD8+ responses without triggering allogeneic reactions. Particularly encouraging was the observation that two patients showed significant increases in circulating anti-tumor-specific T lymphocytes, with a beneficial transition from naïve to memory phenotype (150). These comprehensive findings support the potential of pDC line-based vaccines, particularly when used in conjunction with immune checkpoint inhibitors, representing a significant advancement in cancer immunotherapy strategies.
3.4 Neoantigen and NK cells
Natural killer (NK) cells represent essential components of the innate immune system, demonstrating remarkable efficacy in cancer immunotherapy, particularly in treating advanced-stage leukemia (151, 152). Their therapeutic value stems from their unique ability to recognize stressed cells independently of neoantigen presentation, enabling them to target tumor cells that have lost MHC class I expression, which represents a common mechanism of tumor immune evasion (153, 154). With the growing recognition of tumor neoantigens as potential immunotherapy targets, research has increasingly focused on understanding NK cell characteristics in neoantigen-driven immune responses. Current investigations are exploring the interactions between NK cells and tumor neoantigens, as well as strategies to enhance NK cell activity for improved cancer treatment outcomes (155, 156). This chapter aims to understand the mechanisms by which NK cells recognize and eliminate neoantigens-presenting tumor cells, ultimately working toward developing strategies to fully harness the potential of NK cells in cancer immunotherapy.
NK cell function is primarily mediated through their recognition of HLA molecules via specific receptors, notably killer cell immunoglobulin-like receptors (KIRs) and natural killer group 2 A (NKG2A). Studies have shown that the interaction affinity between HLA and KIR is peptide-dependent and can influence NK cell effector function (157, 158). Therefore, the altered peptide repertoire displayed by MHC molecules in cancer cells may significantly influence NK cell activity. Supporting this concept, a study has demonstrated that NK cells’ cytotoxic effects on tumor cells are modulated by the binding affinity of KIR peptide-MHC interactions (159). A significant advancement in this field emerged with the development of a cancer vaccine targeting MICA and MICB (MICA/B). This innovative approach induces a coordinated attack by both T cell and NK cell populations, maintaining effectiveness against MHC class I-deficient tumors through the combined action of NK cells and CD4+ T cells (160).
Natural killer T (NKT) cells represent a distinctive cell subset of the innate immune system, characterized by CD1d restriction and lipid antigen reactivity, serving as immunoregulatory T lymphocytes (161, 162). While sharing many phenotypic and functional similarities with conventional T cells in anti-tumor immunity, NKT cells are unique in their ability to recognize lipid-based antigens presented on CD1d molecules rather than peptide-MHC complexes (162–164). The therapeutic potential of this pathway was demonstrated by a single-domain antibody targeting CD1d, which successfully induced robust NKT cell activation and enhanced anti-tumor responses, as validated in multiple myeloma and acute myeloid leukemia models (165). Further supporting their therapeutic relevance, strong evidence indicates that tumor cells generate specific lipid species that function as activating antigens for NKT cells via CD1d presentation, playing a crucial role in cancer immune surveillance (166).
These tumor-associated lipid antigens are unique to tumor cells and can be recognized as foreign by the immune system, particularly through NKT cell-mediated responses.These lipid antigens play a role in the participation of NKT cells in cancer immune surveillance (167). Up to now, various tumor-associated lipid antigens have been identified, such as glycolipids (Globo H, Globo A, Lewis Y, and GM2), phospholipids (phosphatidylinositol and phosphatidylglycerol), glycosphingolipids (GM3, GD2, and GD3), and phosphatidylethanolamines (including PE and PG). Several of these antigens have emerged as promising candidates for cancer vaccine development, owing to their distinctive immunogenic properties (167–169). This recognition mechanism offers potential avenues for immunotherapy and cancer treatment. While scientists are working to harness these antigens to enhance anti-tumor immune responses and overcome immune evasion mechanisms, significant research is still needed to fully understand their role in tumor immunity and optimize therapeutic approaches.
In conclusion, NK cells have shown great potential in cancer immunotherapy due to their ability to recognize and eliminate tumor cells. However, crucial questions remains to be learned about NK cell characteristics and their interactions with neoantigen. Further research is needed to understand the factors that regulate NK cell activation and function within the tumor microenvironment. As our understanding of these processes deepens, it will likely lead to the development of more effective and targeted immunotherapies for cancer treatment.
3.5 Neoantigen-driven tumor-associated macrophages
Tumor-Associated Macrophages (TAMs), a heterogeneous population of myeloid cells infiltrating neoplastic tissues, play a critical role in tumor development and progression. TAMs accumulate within tumor tissues and predominantly exhibit tumor-promoting activity, promoting angiogenesis and immune evasion (170–173). With advancing understanding of tumor neoantigens, research has increasingly focused on characterizing macrophages in neoantigen-driven immune responses.
Macrophages employ multiple mechanisms to recognize tumor cells, including direct recognition through receptors such as CD11c, CD14, and CD40, and indirect recognition via DC presentation. This recognition enables macrophages to interact with various immune cell types, including T cells, B cells, and NK cells, coordinating the immune response against tumors (174–176). Recent experimental studies have validated the antigen-presenting function of MHC II-expressing tumor-associated macrophages (TAMs) to tumor-infiltrating CD4 T cells (177, 178). In a bladder carcinoma mouse model, TAM-mediated antigen presentation to effector CD4 T cells was demonstrated to be crucial for tumor rejection. These immunologically active TAMs exhibited a distinctive immunophenotype characterized by CD11b+, MHCII, F4/80, CD11C, CD80, CD86, CD64, and PD-L1 expression. The antitumor response was found to be dependent on IFN-γ production by CD4 T cells (177).
Macrophages have established themselves as crucial players in tumor neoantigen-driven immune responses. However, significant challenges persist in understanding their complete characteristics within the context of neoantigen-based cancer immunotherapy. Key obstacles include the inherent heterogeneity and plasticity of macrophages within the tumor microenvironment, which complicate effective targeting and manipulation strategies. Moreover, the precise mechanisms governing their recruitment and activation in response to neoantigens require further elucidation.
The collective evidence demonstrates that tumor neoantigens initiate a complex, multifaceted immune response, orchestrated through the coordinated actions of T cells, DCs, B cells, NK cells, NKT cells, and macrophages (Figure 1). While these diverse immune cell populations utilize distinct recognition patterns and mechanistic pathways to mount a comprehensive systemic immune response, several critical challenges remain unresolved. These include gaining a deeper understanding of macrophage heterogeneity and plasticity within the tumor microenvironment, and fully characterizing the mechanisms controlling their recruitment and activation in response to neoantigens.
4 The clinical application of neoantigen-specific immune cell strategy
The emergence of neoantigen-driven TILs has shifted research focus towards neoantigen-specific immune cell therapeutic approaches, offering a personalized treatment modality that harnesses tumor-specific mutations to elicit targeted immune responses. Neoantigen‐reactive T‐cell therapy has emerged as a prosperous branch of adoptive cellular immunotherapy. Neoantigen-reactive T cells can specifically recognize neoantigens expressed by tumor cells and mediate the destruction of these cancer cells (179). This approach involves several critical steps: identifying neoantigens from the patient, developing neoantigen-based vaccines, isolating and expanding neoantigen-reactive T cells in vitro, and ultimately reintroducing the neoantigen-reactive T cell product back into the patient’s body to target and destroy cancer cells. Early clinical trials have demonstrated the feasibility and potential efficacy of this approach in various cancer types, including hepatocellular carcinoma and peripheral T-cell lymphoma (148, 180, 181).
TIL Therapy, pioneered by the Rosenberg research group in 1988, has evolved into a groundbreaking cancer immunotherapy approach, recently culminating in the FDA approval of lifileucel (Amtagvi) for advanced melanoma. This innovative treatment modality involves isolating and expanding TILs from patients’ tumor tissues before reinfusing them back following lymphodepletion, effectively harnessing the patient’s own immune system to combat cancer (182, 183). Clinical implementation of TIL therapy has shown remarkable progress, with studies reporting overall response rates of up to 49% in various cancers, particularly in advanced melanoma, lung cancer, and cervical cancer. Research has demonstrated that TILs play a crucial role in enhancing the efficacy of immune checkpoint blockade (ICB) therapy, with higher TIL infiltration correlating with improved treatment outcomes (60, 184, 185). The therapy’s potential extends beyond direct treatment, as TIL analysis serves as a valuable tool for predicting treatment response and monitoring disease progression, contributing to the advancement of personalized cancer medicine (68). While TIL therapy offers significant advantages, including its highly personalized nature and ability to target multiple tumor antigens simultaneously, several challenges persist. These include the complex and time-consuming manufacturing process, substantial production costs, and potential limitations in efficacy due to the immunosuppressive tumor microenvironment. Despite these challenges, ongoing research focuses on optimizing production protocols, enhancing cell persistence, and developing combination strategies with other therapeutic modalities.
DC vaccines, particularly those loaded with neoantigens, have demonstrated promising results in cancer immunotherapy. Recent clinical studies have shown significant progress across various cancer types, utilizing DCs derived from autologous peripheral blood monocytes (23). Notable successes include a personalized neoantigen peptide-pulsed autologous DC vaccine for lung cancer, which demonstrated both safety and efficacy in eliciting neoantigen-specific T-cell responses. Notable successes include a phase II trial (NCT03067493) combining personalized neoantigen-loaded DC vaccine with neoantigen-activated T-cell therapy in 10 patients with HCC who had undergone curative resection or radiofrequency ablation, showing increased DCs and T-cells infiltration and durable neoantigen-specific T-cell responses (23, 148). Similarly, a phase I clinical trial reported that neoantigen-activated haploidentical T cell therapy for peripheral T-cell lymphoma showing promising preliminary results (ChiCTR1800017440) (180). A remarkable breakthrough was achieved in metastatic gastric cancer, where the combination of anti-PD1 with Neo-MoDC vaccines resulted in complete tumor regression lasting over 25 months. However, challenges persist, including the complex and time-consuming manufacturing process, limited reproducibility of autologous DCs, and high production costs. Innovative approaches, such as allogeneic plasmacytoid DC cell lines and reprogrammed conventional DC1 cells, show therapeutic potential but require further optimization for clinical implementation.
Despite these advances, significant challenges persist, including the need for more comprehensive profiling of neoantigen-specific immune cells, improved clinical trial designs, and better understanding of non-T cell immune populations. While six strategies for neoantigen-reactive T cell therapy have been documented, research on neoantigen-driven B cells, NK cells, and macrophages remains limited (186). As our understanding grows, it’s becoming clear that neoantigen vaccines likely stimulate a complex antitumor immune response involving multiple cell types, pointing towards more effective and personalized future immunotherapies.
5 Perspectives
Neoantigen cancer vaccines represent a promising immunotherapeutic approach that activates diverse immune cells against tumor-specific mutations. While this field shows great potential, comprehensive understanding of neoantigen-specific immune responses remains limited. Current clinical trials predominantly focus on assessing treatment efficacy, often neglecting crucial aspects of post-vaccination immune cell dynamics. This gap in knowledge highlights the need for more detailed investigation into the precise mechanisms and characteristics of neoantigen-specific immune cell responses.
Recent transcriptomic analyses have unveiled complex patterns in neoantigen-driven T cells, including exhaustion markers, memory phenotypes, and cytotoxicity genes (e.g., GZMA, GZMB, GZMK, and IFN-γ). However, a comprehensive understanding of these T cell profiles across various cancer types remains elusive, highlighting the need for broader investigation.
Although T cells are the primary focus, the role of neoantigen-specific B cells, NK cells, and macrophages remain largely underexplored. These cells likely contribute significantly to antitumor response, meriting further research. Some studies suggest neoantigen vaccines may activate these immune cell types, but their characteristics and functions in the immune response to neoantigens are not well-defined. While DCs are studied have been extensively for presenting neoantigens to T cells, the limited availability and reproducibility of autologous DCs have hindered their widespread use in clinical settings. Innovative approaches, including allogeneic plasmacytoid DC (pDC) cell lines and reprogrammed conventional DC1 (cDC1) cells, show therapeutic potential but require further optimization for clinical use.
A critical challenge in neoantigen-based immunotherapy is the presence of immunosuppressive cells within the tumor microenvironment. Tregs, MDSCs, and TAMs can significantly impair therapeutic efficacy by dampening immune responses. Recent findings suggest that combining immunotherapy with strategically dosed chemotherapy may offer a solution. As demonstrated by Wang and Xu, sub-maximal doses of chemotherapeutic agents can selectively eliminate immunosuppressive cells, enhancing the effectiveness of combined immunotherapy approaches, particularly with PD-1 inhibition (37). Understanding and addressing these immunosuppressive mechanisms remains crucial for optimizing neoantigen cancer vaccine outcomes.
In summary, neoantigen vaccine therapy orchestrate complex antitumor immune responses beyond T cells, involving B cells, NK cells, and macrophages. The effectiveness of these responses depends on both the characteristics of neoantigen-reactive immune cells and the immunosuppressive tumor microenvironment. To advance this field, several key aspects need attention: first, deeper investigation of spatial dynamics and molecular profiles of immune cells within the TIME using high-resolution transcriptomics and proteomics techniques; second, optimization of clinical trial designs with systematic immune monitoring at critical time points; and third, development of combination treatment strategies to overcome immunosuppression. Future research must embrace this complexity, studying the interplay between these immune cells. To advance this field further, it is important to consider various factors such as the spatial dynamics of T cells within TIME, high-resolution transcriptomics and proteomics techniques, as well as cancer-specific immune responses when investigating the features of neoantigen-reactive T cells. Looking ahead, continued advances in neoantigen prediction algorithms and delivery platforms, coupled with improved understanding of immune cell interactions, will enhance our ability to design more effective and personalized neoantigen-based cancer immunotherapies.
This figure illustrates the complex interactions between tumor cells, immune cells, and neoantigens in the TME, highlighting various neoantigen presentation mechanisms involved in the anti-tumor immune response. The figure is divided into three main sections demonstrating different aspects of neoantigen-mediated immune responses. Left: The tumor microenvironment (TME), depicting various immune cells interacting with tumor cells and a blood vessel containing circulating immune cells. Center: A cluster of tumor cells releasing neoantigens (represented by red dots) and lipid neoantigens (represented by purple dots). Right: Multiple neoantigen presentation pathways within different immune cells, including (1): Within the Tertiary Lymphoid Structure (TLS), Germinal Center B cells (GCB) presenting to T Follicular Helper cells (TFH) via MHC II/TCR and CD40/CD40L interactions; (2) Type 2 Dendritic Cells (DC2) presenting to CD4+ T cells via MHC II/TCR; (3) Type 1 Dendritic Cells (DC1) presenting to CD8+ T cells via MHC I/TCR; (4) Macrophages presenting to CD4+ T cells via MHC II/TCR; and (5) Dendritic Cells (DC) presenting lipid neoantigens to Natural Killer (NK) cells via CD1d. This figure was created with BioRender (https://www.biorender.com).
Author contributions
WL: Conceptualization, Formal Analysis, Investigation, Writing – original draft, Data curation, Methodology, Visualization. HS: Data curation, Visualization, Methodology, Writing – original draft. PG: Data curation, Visualization, Methodology, Writing – original draft. LH: Data curation, Visualization, Project administration, Methodology, Writing – original draft. XH: Conceptualization, Funding acquisition, Supervision, Writing – review & editing. XL: Writing – review & editing, Conceptualization, Data curation, Formal Analysis, Funding acquisition, Methodology, Project administration, Resources, Supervision, Visualization, Writing – original draft.
Funding
The author(s) declare that financial support was received for the research and/or publication of this article. This research was supported by grants from: National Clinical Research Center for Cancer fund, Haihe Laboratory of Synthetic Biology (Grant No. 22HHSWSS00004), Youth Talent Program of Tianjin Cancer Hospital Airport Hospital (Grant No. KGRCJH001). The funders had no direct role in the study design, data collection, analysis, interpretation, or manuscript preparation.
Conflict of interest
The authors declare that the research was conducted in the absence of any commercial or financial relationships that could be construed as a potential conflict of interest.
Generative AI statement
The author(s) declare that no Generative AI was used in the creation of this manuscript.
Publisher’s note
All claims expressed in this article are solely those of the authors and do not necessarily represent those of their affiliated organizations, or those of the publisher, the editors and the reviewers. Any product that may be evaluated in this article, or claim that may be made by its manufacturer, is not guaranteed or endorsed by the publisher.
References
1. Xie N, Shen G, Gao W, Huang Z, Huang C, Fu L. Neoantigens: promising targets for cancer therapy. Signal Transduction Targeted Ther. (2023) 8:9. doi: 10.1038/s41392-022-01270-x
2. Lin MJ, Svensson-Arvelund J, Lubitz GS, Marabelle A, Melero I, Brown BD, et al. Cancer vaccines: the next immunotherapy frontier. Nat Cancer. (2022) 3:911–26. doi: 10.1038/s43018-022-00418-6
3. Sellars MC, Wu CJ, Fritsch EF. Cancer vaccines: Building a bridge over troubled waters. CELL. (2022) 185:2770–88. doi: 10.1016/j.cell.2022.06.035
4. Carreno BM, Magrini V, Becker-Hapak M, Kaabinejadian S, Hundal J, Petti AA, et al. A dendritic cell vaccine increases the breadth and diversity of melanoma neoantigen-specific T cells. SCIENCE. (2015) 348:803–8. doi: 10.1126/science.aaa3828
5. Lang F, Schrörs B, Löwer M, Türeci Ö, Sahin U. Identification of neoantigens for individualized therapeutic cancer vaccines. Nat Rev Drug Discovery. (2022) 21:261–82. doi: 10.1038/s41573-021-00387-y
6. Li X, You J, Hong L, Liu W, Guo P, Hao X. Neoantigen cancer vaccines: a new star on the horizon. Cancer Biol Med. (2023) 21:1–38. doi: 10.20892/j.issn.2095-3941.2023.0395
7. Lybaert L, Lefever S, Fant B, Smits E, De Geest B, Breckpot K, et al. Challenges in neoantigen-directed therapeutics. Cancer Cell. (2023) 41:15–40. doi: 10.1016/j.ccell.2022.10.013
8. Richard G, Ruggiero N, Steinberg GD, Martin WD, De Groot AS. Neoadjuvant personalized cancer vaccines: the final frontier? Expert Rev Vaccines. (2024) 23:205–12. doi: 10.1080/14760584.2024.2303015
9. Paijens ST, Vledder A, de Bruyn M, Nijman HW. Tumor-infiltrating lymphocytes in the immunotherapy era. Cell Mol Immunol. (2020) 18:842–59. doi: 10.1038/s41423-020-00565-9
10. Monberg TJ, Borch TH, Svane IM, Donia M. TIL therapy: facts and hopes. Clin Cancer Res. (2023) 29:3275–83. doi: 10.1158/1078-0432.CCR-22-2428
11. Engelhard V, Conejo-Garcia JR, Ahmed R, Nelson BH, Willard-Gallo K, Bruno TC, et al. B cells and cancer. Cancer Cell. (2021) 39:1293–6. doi: 10.1016/j.ccell.2021.09.007
12. Laumont CM, Nelson BH. B cells in the tumor microenvironment: Multi-faceted organizers, regulators, and effectors of anti-tumor immunity. Cancer Cell. (2023) 41:466–89. doi: 10.1016/j.ccell.2023.02.017
13. Sivori S, Pende D, Quatrini L, Pietra G, Della Chiesa M, Vacca P, et al. NK cells and ILCs in tumor immunotherapy. Mol Aspects Of Med. (2021) 80:100870. doi: 10.1016/j.mam.2020.100870
14. Betof Warner A, Hamid O, Komanduri K, Amaria R, Butler MO, Haanen J, et al. Expert consensus guidelines on management and best practices for tumor-infiltrating lymphocyte cell therapy. J Immunother Cancer. (2024) 12(2)e008735. doi: 10.1136/jitc-2023-008735
15. Mao X, Xu J, Wang W, Liang C, Hua J, Liu J, et al. Crosstalk between cancer-associated fibroblasts and immune cells in the tumor microenvironment: new findings and future perspectives. Mol Cancer. (2021) 20:131. doi: 10.1186/s12943-021-01428-1
16. Schreiber RD, Old LJ, Smyth MJ. Cancer immunoediting: integrating immunity’s roles in cancer suppression and promotion. Science. (2011) 331:1565–70. doi: 10.1126/science.1203486
17. Schumacher TN, Schreiber RD. Neoantigens in cancer immunotherapy. Science. (2015) 348:69–74. doi: 10.1126/science.aaa4971
18. Schumacher TN, Scheper W, Kvistborg P. Cancer neoantigens. Annu Rev Immunol. (2019) 37:173–200. doi: 10.1146/annurev-immunol-042617-053402
19. Zhang Q, Jia Q, Zhang J, Zhu B. Neoantigens in precision cancer immunotherapy: from identification to clinical applications. Chin Med J (Engl). (2022) 135:1285–98. doi: 10.1097/CM9.0000000000002181
20. Rosenthal R, Cadieux EL, Salgado R, Bakir MA, Moore DA, Hiley CT, et al. Neoantigen-directed immune escape in lung cancer evolution. Nature. (2019) 567:479–85. doi: 10.1038/s41586-019-1032-7
21. Blass E, Ott PA. Advances in the development of personalized neoantigen-based therapeutic cancer vaccines. Nat Rev Clin Oncol. (2021) 18:215–29. doi: 10.1038/s41571-020-00460-2
22. Kratky W, Reis e Sousa C, Oxenius A, Spörri R. Direct activation of antigen-presenting cells is required for CD8+T-cell priming and tumor vaccination. Proc Natl Acad Sci. (2011) 108:17414–9. doi: 10.1073/pnas.1108945108
23. Tang L, Zhang R, Zhang X, Yang L. Personalized neoantigen-pulsed DC vaccines: advances in clinical applications. Front Oncol. (2021) 11:701777. doi: 10.3389/fonc.2021.701777
24. Weber JS, Carlino MS, Khattak A, Meniawy T, Ansstas G, Taylor MH, et al. Individualised neoantigen therapy mRNA-4157 (V940) plus pembrolizumab versus pembrolizumab monotherapy in resected melanoma (KEYNOTE-942): a randomised, phase 2b study. Lancet. (2024) 403:632–44. doi: 10.1016/S0140-6736(23)02268-7
25. Veatch JR, Lee SM, Shasha C, Singhi N, Szeto JL, Moshiri AS, et al. Neoantigen-specific CD4+ T cells in human melanoma have diverse differentiation states and correlate with CD8+ T cell, macrophage, and B cell function. Cancer Cell. (2022) 40:393–409.e9. doi: 10.1016/j.ccell.2022.03.006
26. Keskin DB, Anandappa AJ, Sun J, Tirosh I, Mathewson ND, Li S, et al. Neoantigen vaccine generates intratumoral T cell responses in phase Ib glioblastoma trial. Nature. (2018) 565:234–9. doi: 10.1038/s41586-018-0792-9
27. Hu Z, Leet DE, Allesøe RL, Oliveira G, Li S, Luoma AM, et al. Personal neoantigen vaccines induce persistent memory T cell responses and epitope spreading in patients with melanoma. Nat Med. (2021) 27:515–25. doi: 10.1038/s41591-020-01206-4
28. Ott PA, Hu Z, Keskin DB, Shukla SA, Sun J, Bozym DJ, et al. An immunogenic personal neoantigen vaccine for patients with melanoma. Nature. (2017) 547:217–21. doi: 10.1038/nature22991
29. Ott PA, Hu-Lieskovan S, Chmielowski B, Govindan R, Naing A, Bhardwaj N, et al. A phase ib trial of personalized neoantigen therapy plus anti-PD-1 in patients with advanced melanoma, non-small cell lung cancer, or bladder cancer. Cell. (2020) 183:347–62.e24. doi: 10.1016/j.cell.2020.08.053
30. Rojas LA, Sethna Z, Soares KC, Olcese C, Pang N, Patterson E, et al. Personalized RNA neoantigen vaccines stimulate T cells in pancreatic cancer. Nature. (2023) 618:144–50. doi: 10.1038/s41586-023-06063-y
31. Perrinjaquet M, Richard Schlegel C. Personalized neoantigen cancer vaccines: An analysis of the clinical and commercial potential of ongoing development programs. Drug Discovery Today. (2023) 28:103773. doi: 10.1016/j.drudis.2023.103773
32. Supabphol S, Li L, Goedegebuure SP, Gillanders WE. Neoantigen vaccine platforms in clinical development: understanding the future of personalized immunotherapy. Expert Opin Investig Drugs. (2021) 30:529–41. doi: 10.1080/13543784.2021.1896702
33. Burris HA, Patel MR, Cho DC, Clarke JM, Gutierrez M, Zaks TZ, et al. A phase I multicenter study to assess the safety, tolerability, and immunogenicity of mRNA-4157 alone in patients with resected solid tumors and in combination with pembrolizumab in patients with unresectabl solid tumors. J Of Clin Oncol. (2019) 37:2523. doi: 10.1200/JCO.2019.37.15_suppl.2523
34. Zacharakis N, Huq LM, Seitter SJ, Kim SP, Gartner JJ, Sindiri S, et al. Breast cancers are immunogenic: immunologic analyses and a phase II pilot clinical trial using mutation-reactive autologous lymphocytes. J Of Clin Oncol. (2022) 40:1741–54. doi: 10.1200/JCO.21.02170
35. Lv B, Wang Y, Ma D, Cheng W, Liu J, Yong T, et al. Immunotherapy: reshape the tumor immune microenvironment. Front Immunol. (2022) 13:844142. doi: 10.3389/fimmu.2022.844142
36. Fu T, Dai L-J, Wu S-Y, Xiao Y, Ma D, Jiang Y-Z, et al. Spatial architecture of the immune microenvironment orchestrates tumor immunity and therapeutic response. J Hematol Oncol. (2021) 14:98. doi: 10.1186/s13045-021-01103-4
37. Wang Z-X, Xu R-H. Chemo-immunotherapy in advanced esophageal squamous cell carcinoma: present and future. Holistic Integr Oncol. (2023) 2:5. doi: 10.1007/s44178-023-00028-x
38. Ortiz-Muñoz G, Brown M, Carbone CB, Pechuan-Jorge X, Rouilly V, Lindberg H, et al. In situ tumour arrays reveal early environmental control of cancer immunity. Nature. (2023) 618:827–33. doi: 10.1038/s41586-023-06132-2
39. Binnewies M, Roberts EW, Kersten K, Chan V, Fearon DF, Merad M, et al. Understanding the tumor immune microenvironment (TIME) for effective therapy. Nat Med. (2018) 24:541–50. doi: 10.1038/s41591-018-0014-x
40. Bretz AC, Parnitzke U, Kronthaler K, Dreker T, Bartz R, Hermann F, et al. Domatinostat favors the immunotherapy response by modulating the tumor immune microenvironment (TIME). J ImmunoTherapy Cancer. (2019) 7:294. doi: 10.1186/s40425-019-0745-3
41. Xu L, Zou C, Zhang S, Chu TSM, Zhang Y, Chen W, et al. Reshaping the systemic tumor immune environment (STIE) and tumor immune microenvironment (TIME) to enhance immunotherapy efficacy in solid tumors. J Hematol Oncol. (2022) 15:87. doi: 10.1186/s13045-022-01307-2
42. Tang T, Huang X, Zhang G, Hong Z, Bai X, Liang T. Advantages of targeting the tumor immune microenvironment over blocking immune checkpoint in cancer immunotherapy. Signal Transduction Targeted Ther. (2021) 6:72. doi: 10.1038/s41392-020-00449-4
43. Raskov H, Orhan A, Christensen JP, Gögenur I. Cytotoxic CD8+ T cells in cancer and cancer immunotherapy. Br J Of Cancer. (2020) 124:359–67. doi: 10.1038/s41416-020-01048-4
44. Koh C-H, Lee S, Kwak M, Kim B-S, Chung Y. CD8 T-cell subsets: heterogeneity, functions, and therapeutic potential. Exp Mol Med. (2023) 55:2287–99. doi: 10.1038/s12276-023-01105-x
45. St. Paul M, Ohashi PS. The roles of CD8+ T cell subsets in antitumor immunity. Trends In Cell Biol. (2020) 30:695–704. doi: 10.1016/j.tcb.2020.06.003
46. Jin M-Z, Jin W-L. The updated landscape of tumor microenvironment and drug repurposing. Signal Transduction Targeted Ther. (2020) 5:166. doi: 10.1038/s41392-020-00280-x
47. Li K, Shi H, Zhang B, Ou X, Ma Q, Chen Y, et al. Myeloid-derived suppressor cells as immunosuppressive regulators and therapeutic targets in cancer. Signal Transduction Targeted Ther. (2021) 6:362. doi: 10.1038/s41392-021-00670-9
48. Tie Y, Tang F, Y-q W, Wei XW. Immunosuppressive cells in cancer: mechanisms and potential therapeutic targets. J Hematol Oncol. (2022) 15:61. doi: 10.1186/s13045-022-01282-8
49. Lindau D, Gielen P, Kroesen M, Wesseling P, Adema GJ. The immunosuppressive tumour network: myeloid-derived suppressor cells, regulatory T cells and natural killer T cells. Immunology. (2013) 138:105–15. doi: 10.1111/imm.2013.138.issue-2
50. Togashi Y, Shitara K, Nishikawa H. Regulatory T cells in cancer immunosuppression — implications for anticancer therapy. Nat Rev Clin Oncol. (2019) 16:356–71. doi: 10.1038/s41571-019-0175-7
51. Shen J, Xiao Z, Zhao Q, Li M, Wu X, Zhang L, et al. Anti-cancer therapy with TNFα and IFNγ: A comprehensive review. Cell Proliferation. (2018) 51:e12441. doi: 10.1111/cpr.2018.51.issue-4
52. Mazet JM, Mahale JN, Tong O, Watson RA, Lechuga-Vieco AV, Pirgova G, et al. IFNγ signaling in cytotoxic T cells restricts anti-tumor responses by inhibiting the maintenance and diversity of intra-tumoral stem-like T cells. Nat Commun. (2023) 14:321. doi: 10.1038/s41467-023-35948-9
53. Yi M, Li T, Niu M, Zhang H, Wu Y, Wu K, et al. Targeting cytokine and chemokine signaling pathways for cancer therapy. Signal Transduction Targeted Ther. (2024) 9:176. doi: 10.1038/s41392-024-01868-3
54. Mempel TR, Lill JK, Altenburger LM. How chemokines organize the tumour microenvironment. Nat Rev Cancer. (2023) 24:28–50. doi: 10.1038/s41568-023-00635-w
55. Propper DJ, Balkwill FR. Harnessing cytokines and chemokines for cancer therapy. Nat Rev Clin Oncol. (2022) 19:237–53. doi: 10.1038/s41571-021-00588-9
56. Ozga AJ, Chow MT, Luster AD. Chemokines and the immune response to cancer. Immunity. (2021) 54:859–74. doi: 10.1016/j.immuni.2021.01.012
57. Tauriello DVF, Sancho E, Batlle E. Overcoming TGFβ-mediated immune evasion in cancer. Nat Rev Cancer. (2021) 22:25–44. doi: 10.1038/s41568-021-00413-6
58. Bhat AA, Nisar S, Singh M, Ashraf B, Masoodi T, Prasad CP, et al. Cytokine- and chemokine-induced inflammatory colorectal tumor microenvironment: Emerging avenue for targeted therapy. Cancer Commun. (2022) 42:689–715. doi: 10.1002/cac2.12295
59. Levin N, Kim SP, Marquardt CA, Vale NR, Yu Z, Sindiri S, et al. Neoantigen-specific stimulation of tumor-infiltrating lymphocytes enables effective TCR isolation and expansion while preserving stem-like memory phenotypes. J ImmunoTherapy Cancer. (2024) 12(5):e008645. doi: 10.1136/jitc-2023-008645
60. Sarnaik AA, Hwu P, Mulé JJ, Pilon-Thomas S. Tumor-infiltrating lymphocytes: A new hope. Cancer Cell. (2024) 42:1315–8. doi: 10.1016/j.ccell.2024.06.015
61. Presti D, Dall’Olio FG, Besse B, Ribeiro JM, Di Meglio A, Soldato D. Tumor infiltrating lymphocytes (TILs) as a predictive biomarker of response to checkpoint blockers in solid tumors: A systematic review. Crit Rev oncology/hematology. (2022) 177:103773. doi: 10.1016/j.critrevonc.2022.103773
62. Kumar A, Watkins R, Vilgelm AE. Cell therapy with TILs: training and taming T cells to fight cancer. Front Immunol. (2021) 12:690499. doi: 10.3389/fimmu.2021.690499
63. Zhao H, Wu L, Yan G, Chen Y, Zhou M, Wu Y, et al. Inflammation and tumor progression: signaling pathways and targeted intervention. Signal Transduction Targeted Ther. (2021) 6:263. doi: 10.1038/s41392-021-00658-5
64. Liu Z-L, Chen H-H, Zheng L-L, Sun L-P, Shi L. Angiogenic signaling pathways and anti-angiogenic therapy for cancer. Signal Transduction Targeted Ther. (2023) 8:198. doi: 10.1038/s41392-023-01460-1
65. Lugano R, Ramachandran M, Dimberg A. Tumor angiogenesis: causes, consequences, challenges and opportunities. Cell And Mol Life Sci. (2019) 77:1745–70. doi: 10.1007/s00018-019-03351-7
66. Mauldin IS, Jo J, Wages NA, Yogendran LV, Mahmutovic A, Young SJ, et al. Proliferating CD8+ T cell infiltrates are associated with improved survival in glioblastoma. Cells. (2021) 10(12):3378. doi: 10.3390/cells10123378
67. Palomero J, Panisello C, Lozano-Rabella M, Tirtakasuma R, Díaz-Gómez J, Grases D, et al. Biomarkers of tumor-reactive CD4+and CD8+TILs associate with improved prognosis in endometrial cancer. J ImmunoTherapy Cancer. (2022) 10(12):e005443. doi: 10.1136/jitc-2022-005443
68. Brummel K, Eerkens AL, de Bruyn M, Nijman HW. Tumour-infiltrating lymphocytes: from prognosis to treatment selection. Br J Of Cancer. (2022) 128:451–8. doi: 10.1038/s41416-022-02119-4
69. Stockis J, Roychoudhuri R, Halim TYF. Regulation of regulatory T cells in cancer. IMMUNOLOGY. (2019) 157:219–31. doi: 10.1111/imm.2019.157.issue-3
70. Lee CH, Yelensky R, Jooss K, Chan TA. Update on tumor neoantigens and their utility: why it is good to be different. Trends Immunol. (2018) 39:536–48. doi: 10.1016/j.it.2018.04.005
71. Jiang T, Shi T, Zhang H, Hu J, Song Y, Wei J, et al. Tumor neoantigens: from basic research to clinical applications. J Hematol Oncol. (2019) 12:93. doi: 10.1186/s13045-019-0787-5
72. Donia M, Svane IM. Harnessing neoantigen-specific T cells for precision cancer immunotherapy. Nat Rev Clin Oncol. (2024) 21:253–4. doi: 10.1038/s41571-024-00860-8
73. Li S, Simoni Y, Zhuang S, Gabel A, Ma S, Chee J, et al. Characterization of neoantigen-specific T cells in cancer resistant to immune checkpoint therapies. Proc Natl Acad Sci. (2021) 118(30):e2025570118. doi: 10.1073/pnas.2025570118
74. Tran E. Neoantigen-specific T cells in adoptive cell therapy. Cancer J. (2022) 28:278–84. doi: 10.1097/PPO.0000000000000605
75. Hwang B, Lee JH, Bang D. Single-cell RNA sequencing technologies and bioinformatics pipelines. Exp Mol Med. (2018) 50:1–14. doi: 10.1038/s12276-018-0071-8
76. Song Q, Liu L. Single-cell RNA-seq technologies and computational analysis tools: application in cancer research. Methods Mol Biol. (2022) 2413:245–55. doi: 10.1007/978-1-0716-1896-7_23.
77. Azizi E, Carr AJ, Plitas G, Cornish AE, Konopacki C, Prabhakaran S, et al. Single-cell map of diverse immune phenotypes in the breast tumor microenvironment. CELL. (2018) 174:1293–308.e36. doi: 10.1016/j.cell.2018.05.060
78. Zheng L, Qin S, Si W, Wang A, Xing B, Gao R, et al. Pan-cancer single-cell landscape of tumor-infiltrating T cells. Science. (2021) 374:abe6474. doi: 10.1126/science.abe6474
79. Liu B, Hu X, Feng K, Gao R, Xue Z, Zhang S, et al. Temporal single-cell tracing reveals clonal revival and expansion of precursor exhausted T cells during anti-PD-1 therapy in lung cancer. Nat Cancer. (2021) 3:108–21. doi: 10.1038/s43018-021-00292-8
80. Zhang L, Yu X, Zheng L, Zhang Y, Li Y, Fang Q, et al. Lineage tracking reveals dynamic relationships of T cells in colorectal cancer. NATURE. (2018) 564:268–72. doi: 10.1038/s41586-018-0694-x
81. Sudmeier LJ, Hoang KB, Nduom EK, Wieland A, Neill SG, Schniederjan MJ, et al. Distinct phenotypic states and spatial distribution of CD8+ T cell clonotypes in human brain metastases. Cell Rep Med. (2022) 3:100620. doi: 10.1016/j.xcrm.2022.100620
82. Robins HS, Campregher PV, Srivastava SK, Wacher A, Turtle CJ, Kahsai O, et al. Comprehensive assessment of T-cell receptor β-chain diversity in αβ T cells. BLOOD. (2009) 114:4099–107. doi: 10.1182/blood-2009-04-217604
83. Niemi JVL, Sokolov AV, Schiöth HB. Neoantigen vaccines; clinical trials, classes, indications, adjuvants and combinatorial treatments. Cancers. (2022) 14(20):5163. doi: 10.3390/cancers14205163
84. Zhu P, Li S-Y, Ding J, Fei Z, Sun S-N, Zheng Z-H, et al. Combination immunotherapy of glioblastoma with dendritic cell cancer vaccines, anti-PD-1 and poly I:C. J Pharm Analysis. (2023) 13:616–24. doi: 10.1016/j.jpha.2023.04.012
85. Awad MM, Govindan R, Balogh KN, Spigel DR, Garon EB, Bushway ME, et al. Personalized neoantigen vaccine NEO-PV-01 with chemotherapy and anti-PD-1 as first-line treatment for non-squamous non-small cell lung cancer. Cancer Cell. (2022) 40:1010–26.e11. doi: 10.1016/j.ccell.2022.08.003
86. Biswas N, Chakrabarti S, Padul V, Jones LD, Ashili S. Designing neoantigen cancer vaccines, trials, and outcomes. Front Immunol. (2023) 14:1105420. doi: 10.3389/fimmu.2023.1105420
87. Fan T, Zhang M, Yang J, Zhu Z, Cao W, Dong C. Therapeutic cancer vaccines: advancements, challenges and prospects. Signal Transduction Targeted Ther. (2023) 8:450. doi: 10.1038/s41392-023-01674-3
88. Yarchoan M, Gane EJ, Marron TU, Perales-Linares R, Yan J, Cooch N, et al. Personalized neoantigen vaccine and pembrolizumab in advanced hepatocellular carcinoma: a phase 1/2 trial. Nat Med. (2024) 30:1044–53. doi: 10.1038/s41591-024-02894-y
89. Dolton G, Rius C, Wall A, Szomolay B, Bianchi V, Galloway SAE, et al. Targeting of multiple tumor-associated antigens by individual T cell receptors during successful cancer immunotherapy. Cell. (2023) 186:3333–49.e27. doi: 10.1016/j.cell.2023.06.020
90. Caushi JX, Zhang J, Ji Z, Vaghasia A, Zhang B, Hsiue EH-C, et al. Transcriptional programs of neoantigen-specific TIL in anti-PD-1-treated lung cancers. Nature. (2021) 596:126–32. doi: 10.1038/s41586-021-03752-4
91. Zheng C, Fass JN, Shih YP, Gunderson AJ, Sanjuan Silva N, Huang H, et al. Transcriptomic profiles of neoantigen-reactive T cells in human gastrointestinal cancers. Cancer Cell. (2022) 40:410–23.e7. doi: 10.1016/j.ccell.2022.03.005
92. Workel HH, Lubbers JM, Arnold R, Prins TM, van der Vlies P, de Lange K, et al. A transcriptionally distinct CXCL13+CD103+CD8+ T-cell population is associated with B-cell recruitment and neoantigen load in human cancer. Cancer Immunol Res. (2019) 7:784–96. doi: 10.1158/2326-6066.CIR-18-0517
93. Hanada KI, Zhao C, Gil-Hoyos R, Gartner JJ, Chow-Parmer C, Lowery FJ, et al. A phenotypic signature that identifies neoantigen-reactive T cells in fresh human lung cancers. Cancer Cell. (2022) 40:479–93.e6. doi: 10.1016/j.ccell.2022.03.012
94. Lowery FJ, Krishna S, Yossef R, Parikh NB, Chatani PD, Zacharakis N, et al. Molecular signatures of antitumor neoantigen-reactive T cells from metastatic human cancers. SCIENCE. (2022) 375:877–84. doi: 10.1126/science.abl5447
95. Yossef R, Krishna S, Sindiri S, Lowery FJ, Copeland AR, Gartner JJ, et al. Phenotypic signatures of circulating neoantigen-reactive CD8(+) T cells in patients with metastatic cancers. Cancer Cell. (2023) 41:2154–65 e5. doi: 10.1016/j.ccell.2023.11.005
96. Holm JS, Funt SA, Borch A, Munk KK, Bjerregaard A-M, Reading JL, et al. Neoantigen-specific CD8 T cell responses in the peripheral blood following PD-L1 blockade might predict therapy outcome in metastatic urothelial carcinoma. Nat Commun. (2022) 13:1935. doi: 10.1038/s41467-022-29342-0
97. Chen Y, Wang D, Li Y, Qi L, Si W, Bo Y, et al. Spatiotemporal single-cell analysis decodes cellular dynamics underlying different responses to immunotherapy in colorectal cancer. Cancer Cell. (2024) 42:1268–85.e7. doi: 10.1016/j.ccell.2024.06.009
98. Peng P, Hu H, Liu P, Xu LX. Neoantigen-specific CD4+T-cell response is critical for the therapeutic efficacy of cryo-thermal therapy. J ImmunoTherapy Cancer. (2020) 8(2):e000421. doi: 10.1136/jitc-2019-000421
99. Sultan H, Takeuchi Y, Ward JP, Sharma N, Liu T-T, Sukhov V, et al. Neoantigen-specific cytotoxic Tr1 CD4 T cells suppress cancer immunotherapy. Nature. (2024) 632:182–91. doi: 10.1038/s41586-024-07752-y
100. Schmidt J, Chiffelle J, Perez MAS, Magnin M, Bobisse S, Arnaud M, et al. Neoantigen-specific CD8 T cells with high structural avidity preferentially reside in and eliminate tumors. Nat Commun. (2023) 14:3188. doi: 10.1038/s41467-023-38946-z
101. Brightman SE, Becker A, Thota RR, Naradikian MS, Chihab L, Zavala KS, et al. Neoantigen-specific stem cell memory-like CD4+ T cells mediate CD8+ T cell-dependent immunotherapy of MHC class II-negative solid tumors. Nat Immunol. (2023) 24:1345–57. doi: 10.1038/s41590-023-01543-9
102. Bawden EG, Wagner T, Schröder J, Effern M, Hinze D, Newland L, et al. CD4T cell immunity against cutaneous melanoma encompasses multifaceted MHC II–dependent responses. Sci Immunol. (2024) 9(91):eadi9517. doi: 10.1126/sciimmunol.adi9517
103. Sarvaria A, Madrigal JA, Saudemont A. B cell regulation in cancer and anti-tumor immunity. Cell Mol Immunol. (2017) 14:662–74. doi: 10.1038/cmi.2017.35
104. Laumont CM, Banville AC, Gilardi M, Hollern DP, Nelson BH. Tumour-infiltrating B cells: immunological mechanisms, clinical impact and therapeutic opportunities. Nat Rev Cancer. (2022) 22:414–30. doi: 10.1038/s41568-022-00466-1
105. Cyster JG, Allen CDC. B cell responses: cell interaction dynamics and decisions. Cell. (2019) 177:524–40. doi: 10.1016/j.cell.2019.03.016
106. Wennhold K, Thelen M, Schlößer HA, Haustein N, Reuter S, Garcia-Marquez M, et al. Using antigen-specific B cells to combine antibody and T cell–based cancer immunotherapy. Cancer Immunol Res. (2017) 5:730–43. doi: 10.1158/2326-6066.CIR-16-0236
107. Sabdia MB, Patch A-M, Tsang H, Gandhi MK. Neoantigens – the next frontier in precision immunotherapy for B-cell lymphoproliferative disorders. Blood Rev. (2022) 56:100969. doi: 10.1016/j.blre.2022.100969
108. Young C, Brink R. The unique biology of germinal center B cells. Immunity. (2021) 54:1652–64. doi: 10.1016/j.immuni.2021.07.015
109. Baum CM, Davie JM, McKearn JP. B-cell subsets: functional and structural characteristics. . Crit Rev Immunol. (1985) 5:349–70. doi: 10.1016/B978-0-12-397933-9.00007-2
110. Glass DR, Tsai AG, Oliveria JP, Hartmann FJ, Kimmey SC, Calderon AA, et al. An integrated multi-omic single-cell atlas of human B cell identity. Immunity. (2020) 53:217–32.e5. doi: 10.1016/j.immuni.2020.06.013
111. Wei Y, Huang C-X, Xiao X, Chen D-P, Shan H, He H, et al. B cell heterogeneity, plasticity, and functional diversity in cancer microenvironments. Oncogene. (2021) 40:4737–45. doi: 10.1038/s41388-021-01918-y
112. Ng KW, Boumelha J, Enfield KSS, Almagro J, Cha H, Pich O, et al. Antibodies against endogenous retroviruses promote lung cancer immunotherapy. Nature. (2023) 616:563–73. doi: 10.1038/s41586-023-05771-9
113. Cui C, Wang J, Fagerberg E, Chen P-M, Connolly KA, Damo M, et al. Neoantigen-driven B cell and CD4 T follicular helper cell collaboration promotes anti-tumor CD8 T cell responses. Cell. (2021) 184(25):6101–18.e13. doi: 10.1016/j.cell.2021.11.007
114. Lee-Chang C, Miska J, Hou D, Rashidi A, Zhang P, Burga RA, et al. Activation of 4-1BBL+ B cells with CD40 agonism and IFNγ elicits potent immunity against glioblastoma. J Of Exp Med. (2021) 218(1):e20200913. doi: 10.1084/jem.20200913
115. Inoue T, Kurosaki T. Memory B cells. Nat Rev Immunol. (2023) 24:5–17. doi: 10.1038/s41577-023-00897-3
116. Glaros V, Rauschmeier R, Artemov AV, Reinhardt A, Ols S, Emmanouilidi A, et al. Limited access to antigen drives generation of early B cell memory while restraining the plasmablast response. Immunity. (2021) 54:2005–23.e10. doi: 10.1016/j.immuni.2021.08.017
117. Bi K, He MX, Bakouny Z, Kanodia A, Napolitano S, Wu J, et al. Tumor and immune reprogramming during immunotherapy in advanced renal cell carcinoma. Cancer Cell. (2021) 39:649–61.e5. doi: 10.1016/j.ccell.2021.02.015
118. Meylan M, Petitprez F, Becht E, Bougoüin A, Pupier G, Calvez A, et al. Tertiary lymphoid structures generate and propagate anti-tumor antibody-producing plasma cells in renal cell cancer. Immunity. (2022) 55:527–41.e5. doi: 10.1016/j.immuni.2022.02.001
119. Fridman WH, Meylan M, Petitprez F, Sun C-M, Italiano A, Sautès-Fridman C. B cells and tertiary lymphoid structures as determinants of tumour immune contexture and clinical outcome. Nat Rev Clin Oncol. (2022) 19:441–57. doi: 10.1038/s41571-022-00619-z
120. Kdimati S, Mullins CS, Linnebacher M. Cancer-cell-derived igG and its potential role in tumor development. Int J Of Mol Sci. (2021) 22(21):11597. doi: 10.3390/ijms222111597
121. Bod L, Kye Y-C, Shi J, Torlai Triglia E, Schnell A, Fessler J, et al. B-cell-specific checkpoint molecules that regulate anti-tumour immunity. Nature. (2023) 619:348–56. doi: 10.1038/s41586-023-06231-0
122. Ma J, Wu Y, Ma L, Yang X, Zhang T, Song G, et al. A blueprint for tumor-infiltrating B cells across human cancers. Science. (2024) 384(6695):eadj4857. doi: 10.1126/science.adj4857
123. Heras-Murillo I, Adán-Barrientos I, Galán M, Wculek SK, Sancho D. Dendritic cells as orchestrators of anticancer immunity and immunotherapy. Nat Rev Clin Oncol. (2024) 21:257–77. doi: 10.1038/s41571-024-00859-1
124. Del Prete A, Salvi V, Soriani A, Laffranchi M, Sozio F, Bosisio D, et al. Dendritic cell subsets in cancer immunity and tumor antigen sensing. Cell Mol Immunol. (2023) 20:432–47. doi: 10.1038/s41423-023-00990-6
125. Wculek SK, Cueto FJ, Mujal AM, Melero I, Krummel MF, Sancho D. Dendritic cells in cancer immunology and immunotherapy. Nat Rev Immunol. (2019) 20:7–24. doi: 10.1038/s41577-019-0210-z
126. Banchereau J, Steinman RM. Dendritic cells and the control of immunity. Nature. (1998) 392:245–52. doi: 10.1038/32588
127. Hart DN. Dendritic cells: unique leukocyte populations which control the primary immune response. Blood. (1997) 90:3245–87. doi: 10.1182/blood.V90.9.3245
128. Villar J, Segura E. Decoding the heterogeneity of human dendritic cell subsets. Trends In Immunol. (2020) 41:1062–71. doi: 10.1016/j.it.2020.10.002
129. Segura E. Human dendritic cell subsets: An updated view of their ontogeny and functional specialization. Eur J Of Immunol. (2022) 52:1759–67. doi: 10.1002/eji.202149632
130. Zhu S, Niu C, Chen J. Transcriptional divergence between cDC1s and cDC2s: an AP1–IRF composite element-dependent program. Cell Mol Immunol. (2021) 18:1618–9. doi: 10.1038/s41423-020-00628-x
131. Zhang S, Chopin M, Nutt SL. Type 1 conventional dendritic cells: ontogeny, function, and emerging roles in cancer immunotherapy. Trends In Immunol. (2021) 42:1113–27. doi: 10.1016/j.it.2021.10.004
132. Swiecki M, Colonna M. The multifaceted biology of plasmacytoid dendritic cells. Nat Rev Immunol. (2015) 15:471–85. doi: 10.1038/nri3865
133. Zhao Y, Gao C, Liu L, Wang L, Song Z. The development and function of human monocyte-derived dendritic cells regulated by metabolic reprogramming. J Of Leukocyte Biol. (2023) 114:212–22. doi: 10.1093/jleuko/qiad062
134. Ding Z, Li Q, Zhang R, Xie L, Shu Y, Gao S, et al. Personalized neoantigen pulsed dendritic cell vaccine for advanced lung cancer. Signal Transduct Target Ther. (2021) 6:26. doi: 10.1038/s41392-020-00448-5
135. Guo Z, Yuan Y, Chen C, Lin J, Ma Q, Liu G, et al. Durable complete response to neoantigen-loaded dendritic-cell vaccine following anti-PD-1 therapy in metastatic gastric cancer. NPJ Precis Oncol. (2022) 6:34. doi: 10.1038/s41698-022-00279-3
136. Li W, Pan L, Hong W, Ginhoux F, Zhang X, Xiao C, et al. A single-cell pan-cancer analysis to show the variability of tumor-infiltrating myeloid cells in immune checkpoint blockade. Nat Commun. (2024) 15:6142. doi: 10.1038/s41467-024-50478-8
137. Huang Q, Wang F, Hao D, Li X, Li X, Lei T, et al. Deciphering tumor-infiltrating dendritic cells in the single-cell era. Exp Hematol Oncol. (2023) 12:97. doi: 10.1186/s40164-023-00459-2
138. Wang Z, Ji X, Zhang Y, Yang F, Su H, Zhang H, et al. Interactions between LAMP3+ dendritic cells and T-cell subpopulations promote immune evasion in papillary thyroid carcinoma. J Immunother Cancer. (2024) 12(5):e008983. doi: 10.1136/jitc-2024-008983
139. Cheng S, Li Z, Gao R, Xing B, Gao Y, Yang Y, et al. A pan-cancer single-cell transcriptional atlas of tumor infiltrating myeloid cells. Cell. (2021) 184:792–809.e23. doi: 10.1016/j.cell.2021.01.010
140. Maier B, Leader AM, Chen ST, Tung N, Chang C, LeBerichel J, et al. A conserved dendritic-cell regulatory program limits antitumour immunity. Nature. (2020) 580:257–62. doi: 10.1038/s41586-020-2134-y
141. Shin J-Y, Wang C-Y, Lin C-C, Chu C-L. A recently described type 2 conventional dendritic cell (cDC2) subset mediates inflammation. Cell Mol Immunol. (2020) 17:1215–7. doi: 10.1038/s41423-020-0511-y
142. Minutti CM, Piot C, Pereira da Costa M, Chakravarty P, Rogers N, Huerga Encabo H, et al. Distinct ontogenetic lineages dictate cDC2 heterogeneity. Nat Immunol. (2024) 25:448–61. doi: 10.1038/s41590-024-01745-9
143. Najafi S, Mortezaee K. Advances in dendritic cell vaccination therapy of cancer. Biomedicine Pharmacotherapy. (2023) 164:114954. doi: 10.1016/j.biopha.2023.114954
144. Ingels J, De Cock L, Stevens D, Mayer RL, Théry F, Sanchez GS, et al. Neoantigen-targeted dendritic cell vaccination in lung cancer patients induces long-lived T cells exhibiting the full differentiation spectrum. Cell Rep Med. (2024) 5:101516. doi: 10.1016/j.xcrm.2024.101516
145. Ginhoux F, Guilliams M, Merad M. Expanding dendritic cell nomenclature in the single-cell era. Nat Rev Immunol. (2022) 22:67–8. doi: 10.1038/s41577-022-00675-7
146. Morisaki T, Kubo M, Umebayashi M, Yew PY, Yoshimura S, Park J-H, et al. Neoantigens elicit T cell responses in breast cancer. Sci Rep. (2021) 11:13590. doi: 10.1038/s41598-021-91358-1
147. Zhang X, Kim S, Hundal J, Herndon JM, Li S, Petti AA, et al. Breast cancer neoantigens can induce CD8+ T-cell responses and antitumor immunity. Cancer Immunol Res. (2017) 5:516–23. doi: 10.1158/2326-6066.CIR-16-0264
148. Peng S, Chen S, Hu W, Mei J, Zeng X, Su T, et al. Combination neoantigen-based dendritic cell vaccination and adoptive T-cell transfer induces antitumor responses against recurrence of hepatocellular carcinoma. Cancer Immunol Res. (2022) 10:728–44. doi: 10.1158/2326-6066.CIR-21-0931
149. Hannani D, Leplus E, Laulagnier K, Chaperot L, Plumas J. Leveraging a powerful allogeneic dendritic cell line towards neoantigen-based cancer vaccines. Genes Cancer. (2023) 14:3–11. doi: 10.18632/genesandcancer.229
150. Charles J, Chaperot L, Hannani D, Bruder Costa J, Templier I, Trabelsi S, et al. An innovative plasmacytoid dendritic cell line-based cancer vaccine primes and expands antitumor T-cells in melanoma patients in a first-in-human trial. OncoImmunology. (2020) 9:1738812. doi: 10.1080/2162402X.2020.1738812
151. Crinier A, Narni-Mancinelli E, Ugolini S, Vivier E. SnapShot: natural killer cells. Cell. (2020) 180:1280–.e1. doi: 10.1016/j.cell.2020.02.029
152. Malmberg K-J, Carlsten M, Björklund A, Sohlberg E, Bryceson YT, Ljunggren H-G. Natural killer cell-mediated immunosurveillance of human cancer. Semin In Immunol. (2017) 31:20–9. doi: 10.1016/j.smim.2017.08.002
153. Pan W, Tao T, Qiu Y, Zhu X, Zhou X. Natural killer cells at the forefront of cancer immunotherapy with immune potency, genetic engineering, and nanotechnology. Crit Rev oncology/hematology. (2024) 193:104231. doi: 10.1016/j.critrevonc.2023.104231
154. Vivier E, Rebuffet L, Narni-Mancinelli E, Cornen S, Igarashi RY, Fantin VR. Natural killer cell therapies. Nature. (2024) 626:727–36. doi: 10.1038/s41586-023-06945-1
155. Lv D, Khawar MB, Liang Z, Gao Y, Sun H. Neoantigens and NK cells: “Trick or treat” the cancers? Front Immunol. (2022) 13:931862. doi: 10.3389/fimmu.2022.931862
156. Wolf NK, Kissiov DU, Raulet DH. Roles of natural killer cells in immunity to cancer, and applications to immunotherapy. Nat Rev Immunol. (2022) 23:90–105. doi: 10.1038/s41577-022-00732-1
157. Rehermann B. Peptide-dependent HLA-KIR-mediated regulation of NK cell function. J Of Hepatology. (2016) 65:237–9. doi: 10.1016/j.jhep.2016.05.008
158. Fadda L, Borhis G, Ahmed P, Cheent K, Pageon SV, Cazaly A, et al. Peptide antagonism as a mechanism for NK cell activation. Proc Natl Acad Sci U S A. (2010) 107:10160–5. doi: 10.1073/pnas.0913745107
159. Boyington JC, Motyka SA, Schuck P, Brooks AG, Sun PD. Crystal structure of an NK cell immunoglobulin-like receptor in complex with its class I MHC ligand. Nature. (2000) 405:537–43. doi: 10.1038/35014520
160. Badrinath S, Dellacherie MO, Li A, Zheng S, Zhang X, Sobral M, et al. A vaccine targeting resistant tumours by dual T cell plus NK cell attack. Nature. (2022) 606:992–8. doi: 10.1038/s41586-022-04772-4
161. Rossjohn J, Pellicci DG, Patel O, Gapin L, Godfrey DI. Recognition of CD1d-restricted antigens by natural killer T cells. Nat Rev Immunol. (2012) 12:845–57. doi: 10.1038/nri3328
162. Krijgsman D, Hokland M, Kuppen PJK. The role of natural killer T cells in cancer—A phenotypical and functional approach. Front Immunol. (2018) 9:367. doi: 10.3389/fimmu.2018.00367
163. Netskar H, Pfefferle A, Goodridge JP, Sohlberg E, Dufva O, Teichmann SA, et al. Pan-cancer profiling of tumor-infiltrating natural killer cells through transcriptional reference mapping. Nat Immunol. (2024) 25:1445–59. doi: 10.1038/s41590-024-01884-z
164. Terabe M, Berzofsky JA. Tissue-specific roles of NKT cells in tumor immunity. Front Immunol. (2018) 9:1838. doi: 10.3389/fimmu.2018.01838
165. Lameris R, Shahine A, Pellicci DG, Uldrich AP, Gras S, Le Nours J, et al. A single-domain bispecific antibody targeting CD1d and the NKT T-cell receptor induces a potent antitumor response. Nat Cancer. (2020) 1:1054–65. doi: 10.1038/s43018-020-00111-6
166. Melum E, Jiang X, Baker KD, Macedo MF, Fritsch J, Dowds CM, et al. Control of CD1d-restricted antigen presentation and inflammation by sphingomyelin. Nat Immunol. (2019) 20:1644–55. doi: 10.1038/s41590-019-0504-0
167. Lee MS, Webb TJ. Novel lipid antigens for NKT cells in cancer. Front Immunol. (2023) 14:1173375. doi: 10.3389/fimmu.2023.1173375
168. Dowds CM, Kornell S-C, Blumberg RS, Zeissig S. Lipid antigens in immunity. bchm. (2014) 395:61–81. doi: 10.1515/hsz-2013-0220
169. Furukawa K, Ohmi Y, Hamamura K, Kondo Y, Ohkawa Y, Kaneko K, et al. Signaling domains of cancer-associated glycolipids. Glycoconjugate J. (2022) 39:145–55. doi: 10.1007/s10719-022-10051-1
170. Mantovani A, Marchesi F, Jaillon S, Garlanda C, Allavena P. Tumor-associated myeloid cells: diversity and therapeutic targeting. Cell Mol Immunol. (2021) 18:566–78. doi: 10.1038/s41423-020-00613-4
171. Christofides A, Strauss L, Yeo A, Cao C, Charest A, Boussiotis VA. The complex role of tumor-infiltrating macrophages. Nat Immunol. (2022) 23:1148–56. doi: 10.1038/s41590-022-01267-2
172. Xiang X, Wang J, Lu D, Xu X. Targeting tumor-associated macrophages to synergize tumor immunotherapy. Signal Transduction Targeted Ther. (2021) 6:75. doi: 10.1038/s41392-021-00484-9
173. Jahandideh A, Yarizadeh M, Noei-Khesht-Masjedi M, Fatehnejad M, Jahandideh R, Soheili R, et al. Macrophage’s role in solid tumors: two edges of a sword. Cancer Cell Int. (2023) 23:150. doi: 10.1186/s12935-023-02999-3
174. Muntjewerff EM, Meesters LD, van den Bogaart G. Antigen cross-presentation by macrophages. Front Immunol. (2020) 11:1276. doi: 10.3389/fimmu.2020.01276
175. Martinez-Pomares L, Gordon S. Antigen presentation the macrophage way. Cell. (2007) 131:641–3. doi: 10.1016/j.cell.2007.10.046
176. Stopforth RJ, Ward ES. The role of antigen presentation in tumor-associated macrophages. Crit Rev In Immunol. (2020) 40:205–24. doi: 10.1615/CritRevImmunol.2020034910
177. Perez-Diez A, Liu X, Matzinger P. Neoantigen presentation and IFNγ Signaling on the same tumor-associated macrophage are necessary for CD4 T cell–mediated antitumor activity in mice. Cancer Res Commun. (2022) 2:316–29. doi: 10.1158/2767-9764.CRC-22-0052
178. Wang S, Wang J, Chen Z, Luo J, Guo W, Sun L, et al. Targeting M2-like tumor-associated macrophages is a potential therapeutic approach to overcome antitumor drug resistance. NPJ Precis Oncol. (2024) 8:31. doi: 10.1038/s41698-024-00522-z
179. Zhu Y, Qian Y, Li Z, Li Y, Li B. Neoantigen-reactive T cell: An emerging role in adoptive cellular immunotherapy. MedComm. (2021) 2:207–20. doi: 10.1002/mco2.v2.2
180. Chen Y, Zhao H, Luo J, Liao Y, Dan X, Hu G, et al. A phase I dose-escalation study of neoantigen-activated haploidentical T cell therapy for the treatment of relapsed or refractory peripheral T-cell lymphoma. Front Oncol. (2022) 12:944511. doi: 10.3389/fonc.2022.944511
181. Balzeau J, Ravindran A, Wang X, Maisuria J, Lucchesi A, Yao H, et al. Successful ex vivo expansion of tumor infiltrating lymphocytes with systemic chemotherapy prior to surgical resection. Cancer Immunology Immunother. (2023) 72:3377–85. doi: 10.1007/s00262-023-03500-9
182. Rosenberg SA, Packard BS, Aebersold PM, Solomon D, Topalian SL, Toy ST, et al. Use of tumor-infiltrating lymphocytes and interleukin-2 in the immunotherapy of patients with metastatic melanoma. New Engl J Of Med. (1988) 319:1676–80. doi: 10.1056/NEJM198812223192527
183. Zhao Y, Deng J, Rao S, Guo S, Shen J, Du F, et al. Tumor infiltrating lymphocyte (TIL) therapy for solid tumor treatment: progressions and challenges. Cancers. (2022) 14(17):4160. doi: 10.3390/cancers14174160
184. Klobuch S, Seijkens TTP, Schumacher TN, Haanen JBAG. Tumour-infiltrating lymphocyte therapy for patients with advanced-stage melanoma. Nat Rev Clin Oncol. (2024) 21:173–84. doi: 10.1038/s41571-023-00848-w
185. Gide TN, Quek C, Menzies AM, Tasker AT, Shang P, Holst J, et al. Distinct immune cell populations define response to anti-PD-1 monotherapy and anti-PD-1/anti-CTLA-4 combined therapy. Cancer Cell. (2019) 35:238–55.e6. doi: 10.1016/j.ccell.2019.01.003
Keywords: neoantigen, cancer vaccine, immune cells, precision oncology, antitumor immune response, tumor microenvironment
Citation: Liu W, Su H, Guo P, Hong L, Hao X and Li X (2025) Unveiling the immunological landscape: comprehensive characterization of neoantigen-reactive immune cells in neoantigen cancer vaccines. Front. Immunol. 16:1537947. doi: 10.3389/fimmu.2025.1537947
Received: 02 December 2024; Accepted: 25 February 2025;
Published: 18 March 2025.
Edited by:
Sheefa Mirza, University of the Witwatersrand, South AfricaReviewed by:
Wayne Robert Thomas, University of Western Australia, AustraliaJacopo Chiaro, University of Helsinki, Finland
Copyright © 2025 Liu, Su, Guo, Hong, Hao and Li. This is an open-access article distributed under the terms of the Creative Commons Attribution License (CC BY). The use, distribution or reproduction in other forums is permitted, provided the original author(s) and the copyright owner(s) are credited and that the original publication in this journal is cited, in accordance with accepted academic practice. No use, distribution or reproduction is permitted which does not comply with these terms.
*Correspondence: Xiaoling Li, bGl4aWFvbGluZ0B0bXUuZWR1LmNu; Xishan Hao, eGlzaGFuaGFvQHNpbmEuY29t