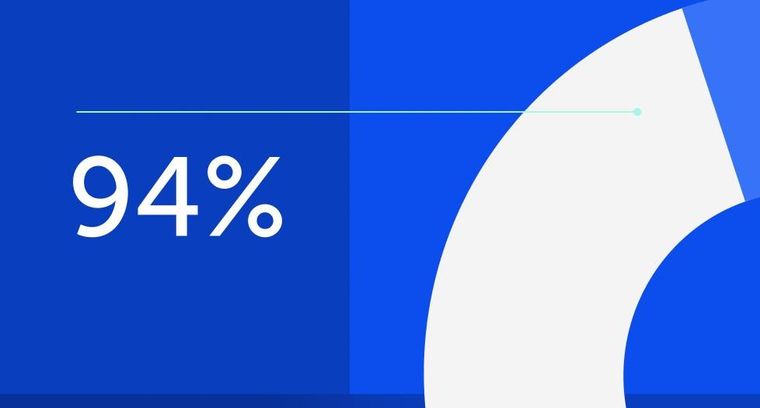
94% of researchers rate our articles as excellent or good
Learn more about the work of our research integrity team to safeguard the quality of each article we publish.
Find out more
REVIEW article
Front. Immunol., 17 April 2025
Sec. Cancer Immunity and Immunotherapy
Volume 16 - 2025 | https://doi.org/10.3389/fimmu.2025.1536355
Boron Neutron Capture Therapy (BNCT), often referred to as the ‘cell knife,’ represents a binary, tumor-selective therapeutic modality that minimizes damage to surrounding healthy tissues. This review provides a comprehensive clinical perspective on BNCT, addressing the radiobiological mechanisms and summarizing related clinical trials, with a particular emphasis on glioma and head and neck cancers. Furthermore, the paper touches upon the synergistic potential of BNCT when integrated with other treatment modalities, such as proton and carbon ion radiotherapy, alternative neutron capture therapies, ultrasound, and immunotherapy. These combined approaches may offer promising avenues for future research, potentially enhancing the therapeutic index and expanding the applicability of BNCT in oncological practice.
Boron neutron capture therapy (BNCT) is a highly accurate form of radiotherapy (RT) that combines targeted therapy with heavy ion RT. Ideally, nonradioactive 10B is taken up only by tumor cells. When 10B is irradiated with low-energy thermal neutrons, the unstable isotope 11B is created. Then, 11B undergoes instantaneous nuclear fission into recoiling 7Li nuclei and high-energy alpha particles (4He), which deposit their energies in the range of 5–9 µm (shorter than the cell diameter) (Figure 1). Hence, the harmful effects are limited to tumor cells (1). In recent years, monumental breakthroughs have been made in emerging methods of cancer treatment, such as targeted therapy, proton RT and heavy ion RT. However, several limitations and shortcomings remain. Targeted drugs kill tumor cells by targeting a link in the process of metabolism or proliferation. However, these links can be blocked or compensated for easily, resulting in drug resistance (2). Proton radiotherapy and heavy ion radiotherapy, high linear energy transfer (high-LET) methods, have shown significant cell-killing effects. These methods are more accurate than conventional RT according to the Bragg peaks. However, some healthy tissues are still exposed to radiation before the ray reaches the tumor. In addition, healthy tissues surrounding the tumor inevitably receive the same amount of irradiation (3).
Figure 1. Schematic diagram of boron neutron capture therapy (BNCT) selectively killing tumor cells. Boron agents are administered and selectively accumulate in tumor cells. After the application of neutron beams, the neutrons are captured by boron-10 (¹0B) within the tumor cells, leading to the formation of helium-4 (4He) and lithium-7 (7Li), along with the emission of high-energy gamma rays. This reaction causes the death of the tumor cells while sparing the surrounding normal cells.
At present, BNCT has entered the era of the accelerator, and new boron agents are being widely investigated. The development of imaging technology for dynamic monitoring has gradually increased. In 2020, permission for manufacturing and sailing accelerator-based BNCT equipment and boropharan was obtained from Japan for the first time (4). BNCT studies have since increased in countries such as America, Europe, Japan and China. In this review, the radiobiological mechanism of BNCT is introduced. The clinical results are summarized, with a focus on glioma and head and neck cancer (HNC). Perspectives on the combination of BNCT with other antitumor treatments are discussed. In addition, studies relevant to immunotherapy are presented.
The treatment planning system (TPS) serves as the hub supporting the technology of BNCT. Compared with traditional photon or proton radiotherapy planning systems, BNCT-TPS faces three unique challenges: The first dimension is the complexity of the energy field, where neutron interactions with biological tissues result in secondary particle cascade reactions; the second dimension is pharmacokinetics, which involves accurately determining boron concentrations; and the third dimension is the specificity of biological effect calculations, which require the transformation of physical doses into biologically effective doses. The multi-physical field coupling characteristic of BNCT poses significant technical barriers in terms of algorithm innovation, data integration, and computational efficiency.
The planning module is the core of the TPS, which is based on the Monte Carlo method to calculate the dose distribution of patients in a mixed neutron-photon field (5, 6). The physical doses induced by neutrons consist of the boron doses produced by the 10B(n, α)7Li reaction, the nitrogen doses produced by the 14N(n, p)14C reaction, and the hydrogen doses produced by the 1H(n, n)p reaction (7). In BNCT, dose prescriptions refer to biological effective doses. Therefore, the planning module needs to perform calculations from neutron flux to physical dose to biological dose (while also considering the impact of γ rays in the mixed neutron-photon field and secondary γ rays produced by neutron interactions with biological tissues on the total biological dose). The calculated results are then returned to the TPS in the form of three-dimensional dose cloud maps and dose-volume histogram (DVH) diagrams. The formula for computing the physical dose is as follows. The absorbed dose Dn produced by the reaction of the neutron with each atom is given as follows:
Where f is the factor releasing kinetic energy or dose conversion factor of photons in neutron matter, and ϕ(t) is the neutron flux or photon at a point. The value of f varies with the radiation energy. The dose component DwoB is expressed as follows:
Where DwoB is expressed as the sum of the nonboron dose components (8). DN, DH, and Dγ are the nitrogen, hydrogen and γ dose components, respectively. The formula for calculating the biological dose is as follow:
Where ED is equivalent dose. CB is the boron concentration. DB is the boron dose component. CBEB is compound biological effectiveness, which depends on the behavior of boron compound in each tissue. RBEN, RBEH, and RBEγ are nitrogen, hydrogen, and γ absorbed doses of relative biological effectiveness, respectively.
Ionizing radiation is characterized by its biological effects and is related to linear energy transfer. BNCT is a mixed-field irradiation technique comprising components with varying LET characteristics. These absorbed dose components are generally considered to act independently of each other (9, 10). Investigating the radiobiological mechanisms induced by BNCT will help researchers identify the cellular response markers and possible signaling pathways (Figure 2), thereby increasing therapeutic efficacy and reducing toxicity (11–13).
Figure 2. Radiobiological mechanisms of BNCT, including DNA damage, DNA repair, and cell cycle arrest and apoptosis. DNA double strand breaks (DSBs) initiate DNA repair, cell cycle arrest and apoptosis. DNA ligase IV and Ku70 are crucial for nonhomologous end joining (NHEJ), while Rad51 and Rad54 are integral to homologous recombination (HR). Cyclins and checkpoint proteins, such as Cyclin B1, CDK1, play important role in regulation of cell cycle. Bax activation and Bcl-2 downregulation are involvement in the apoptosis triggered by BNCT.
DNA is the primary target of radiation damage, whether caused by phonons, protons, heavy ions, or neutrons (14, 15). γH2AX serves as a key marker for DNA double strand breaks (DSBs), initiating the recruitment of DNA repair proteins and playing a crucial role in maintaining genomic stability after irradiation (16). Masutani et al. reported an increase in γH2AX at 6 h after BNCT in a lymphosarcoma model. γH2AX and poly (ADP-ribosylation) (PAR) staining persisted at 20 h after BNCT (17). DNA damage increases with increasing radiation LET (18). High-LET particle components principally induce direct damage to DNA, causing irreparable DSBs, referred to as ‘complex DSBs’. Low-LET radiation primarily causes indirect, reparable DNA single-strand breaks (SSBs) (1, 14, 19–21). Epithermal neutrons can cause more than 50% of the DNA strands to break, and with increasing 10B concentration, more DNA strands break. Compared with photon RT, BNCT produces larger and more complex micronuclei in tumor cells (22, 23). BNCT produced a significantly larger focus size of γH2AX than phonon treatment did in a thyroid follicular cancer cell line (12).
DNA damage activates the DNA repair system (24). In mammalian species, DSBs are repaired through nonhomologous end joining (NHEJ) in most cases. Natsuko Kondo et al. reported that BNCT-induced DNA damage can be partially repaired by the NHEJ repair protein DNA ligase IV (25). Ku70 is crucial for NHEJ, a faster but less accurate repair pathway primarily active in the G1 phase. In contrast, Rad51 and Rad54 are integral to homologous recombination (HR), a high-fidelity repair mechanism active in the S and G2 phases of the cell cycle (12, 14). Rodriguez et al. reported that the mRNA expression of Rad51 and Rad54 increased, but that of Ku70 did not significantly change (12). Perona et al. reported that Ku70 expression increased at different times after irradiation with neutrons but decreased after BNCT (neutrons plus BOPP). This decrease in Ku70 expression after BNCT explains the increase in sensitization to radiation in the BNCT treatment group (26).
DNA damage naturally initiates cell cycle checkpoints, which provides cells with the time required for repair or to decide on programmed cell death if damage is irreparable (1, 27, 28). Cell cycle analysis revealed that BNCT induced G2/M arrest at 24 and 48 hours after irradiation (26). G2/M arrest has been associated with specific regulatory cyclin B1 (proteins associated with G2 arrest), and an inhibition or a delay in the activation of CDK1 (29, 30). Similarly, Sun et al. found a decreased expression of cyclin B1 and CDK1 proteins after BNCT (31). Fujita et al. and Kamida et al. also reported that Wee1, cdc2, and cyclin B1 were altered in the oral squamous cell carcinoma (OSCC) cell line SAS. Caspase 3 induces both G1 and G2 arrest, whereas apoptosis is related to G1 arrest (18, 32). BNCT inhibits OSCC cells in both p53-dependent and p53-independent manners. P53 is necessary for G1 arrest-associated apoptosis (1, 18, 32, 33). However, Seki et al. reported that DNA damage induced by BNCT was not dependent on p53 function (33). Wang et al. reported that the BNCT-induced apoptosis of glioma cells may be associated with Bax activation and Bcl-2 downregulation (34). However, Aromando et al. suggested that apoptosis may not play a significant role in BNCT-induced tumor control (35). To date, the role of apoptotic machinery after BNCT is still being explored.
Cells in S/G2/M phase having higher uptake of boronophenylalanine (BPA) than that in the G1/S phase due to metabolic activity (36). This effect was stronger with BPA than with borocaptate sodium (BSH), as BPA relies on cellular uptake, whereas BSH is a diffusion drug (36–38). Hypoxia-inducible factor 1α (HIF-1α) mediates adaptive responses to hypoxia and controls L-type amino acid transporter (LAT1) expression in hypoxic tumor cells (39, 40). In a lymphosarcoma model, high mobility group box-1 (HMGB1) levels increased 6 hours after BNCT and decreased at 20 hours (17). Unexpectedly, BNCT increased the metastatic potential of high-grade gliomas. This effect occurs because of bystander effects in adjacent cells; that is, BNCT can induce mutations in normal cells near boron-containing tumor cells, and NF-κB may be involved in the response (41). BNCT altered the extracellular matrix by decreasing collagen synthesis and elevated the levels of the tumor necrosis factor (TNF) receptor and cleaved caspases 3, 7, 8 and 9 in melanoma. These findings suggest that multiple pathways associated with cell cycle arrest and apoptosis are involved in the treatment of tumors by BNCT (42).
Recently, BNCT has been successfully used to treat high-grade gliomas and recurrent/metastatic HNC. Furthermore, it has potential for treating melanoma, breast cancer, angiosarcoma, etc (43). We summarize the clinical trials in which BNCT was used to treat patients with high-grade gliomas and HNC since 1994 in Tables 1 and 2 and present the landmark studies in Figure 3. In addition, the registered trials are summarized in Table 3.
Table 3. Clinical studies of BNCT(ICTRP) https://trialsearch.who.int/.
High-grade glioma is a category of aggressive primary brain tumors with limited therapeutic options and poor prognosis (88–90). Although glioblastoma (GBM) rarely metastasizes to other organs, it exhibits a highly infiltrative growth pattern (44). Traditional photon RT cannot kill infiltrating GBM cells, as the radiation dose required to eliminate tumor cells would also induce necrosis in the surrounding healthy brain tissue (44, 91, 92). Gliomas, account for most attempts to use BNCT in clinical settings, using the terminally differentiated nature of neurons to the advantage of a therapy based on lethal-upon-replication genome damage (13). GBM was chosen as the initial clinical target for phase I and II trials of BNCT (44). In 1951, Sweet and Javid reported the first case at the Brookhaven Graphite Research Reactor for primary brain cancer treated with BNCT (93). Forty brain tumor patients subsequently participated in the clinical trial. Unfortunately, patients experienced serious side effects, including scalp radiation damage, brain radionecrosis, cerebral edema and intractable shock, because the penetration force of the neutron sources used for treatment at the time was weak and the targeting of the boron agents was poor (94–99). Hence, the US completely discontinued clinical trials of BNCT in 1961. Hatanaka continued this research in Japan. In 1990, he reported that 120 patients with Grade III-IV gliomas whose tumors were within the limits of maximum therapeutic depth had a very satisfying 5-year survival rate of 58% (100). Four years later, he reported that 9 patients had lived longer than 10 years (101). This was an unexpected result and encouraged researchers to proceed with BNCT studies. In the 1990s, the USA initiated several clinical trials of BNCT with BPA and epithermal neutron beams (44–46). From September 1994 to May 1999, fifty-three primary GBM patients at the Brookhaven National Laboratory received BNCT after surgery via one, two or three irradiation fields. The median survival times (MST) were 14.8, 12.1 and 11.9 months, respectively. Extended exposure to thermal neutron beams was linked to increased neurotoxicity but was not positively correlated with improved local control or survival. This is indirect proof that BNCT has a greater advantage at low neutron irradiation doses (44, 45). The EORTC trial 11961 was launched in Germany in 1997 and included 26 GBM patients treated with BSH-based BNCT. This trial demonstrated the safety of BSH for clinical application at a dose rate of 1 mg/kg/min and a dose of 100 mg/kg. However, cerebral radiological changes, such as cerebral atrophy and white matter changes, appeared in half of the patients within the first year after BNCT (48–50). In the same period, intraoperative NCT and external beam NCT were compared in Japan. They found the MST of the two groups were 23.3 and 27.1 months, respectively (59). Twenty-two selected malignant glioma (MG) patients with progression after surgery and traditional RT entered a phase I study (NCT00115440) in Finland between 2001 and 2008. The MST after BNCT was 7 months, and the 1-year overall survival (OS) was 36% (52). In 2001, Sweden carried out two clinical studies in which the infusion of BPA was increased to 900 mg/kg body weight and was administered via a 6-hour intravenous infusion to increase the boron concentration in tumor cells. One study included 30 GBM patients, 27 of whom underwent debulking surgery, and reported that the boron concentration in the blood during irradiation ranged from 15.2-33.7 µg/g. Although the efficacy of BNCT was comparable to that of conventional photon RT, it worsened quality of life. Interestingly, patients treated with temozolomide (TMZ) at recurrence had a longer survival rate (17.7 months) than did those treated with BNCT alone (11.5 months) (53). The results of the other trial, which included 12 patients with recurrent GBM, were more encouraging. BNCT was reported to be as effective as concentration RT for recurrent GBM (54). Later, radiotherapy plus concomitant and adjuvant temozolomide was shown to be an optimal therapy for GBM (102). J.W. Hopewell et al. and Anja Sander et al. attempted to compare the OS between BNCT and RT+TMZ by reanalyzing the published data. Regrettably, no high-confidence results were found because of high patient heterogeneity across different trials (103, 104). Twenty-one newly diagnosed glioblastoma patients treated with BNCT had an MST of 15.6 months after diagnosis, which was significantly better than that of patients treated with postoperative radiotherapy and chemotherapy. There was a significant prolongation of survival in the BNCT+XRT boost group (MST, 23.5 months after diagnosis) compared with the BNCT alone group (MST, 14.1 months after diagnosis) (63). On the basis of these findings, a multicentric phase II clinical study was performed in Japan (NCT00974987), which planed enrolled 32 participants treated with BNCT, X-ray radiation treatment and TMZ. The outcome was not reported. In addition to TMZ, bevacizumab (BV) is an efficient antitumor drug for GBM. Twenty-five GBM patients with recurrent malignant glioma who were treated with BNCT and BV achieved prolonged OS and progression-free survival (PFS) compared to those achieved in prior BNCT-only studies. In addition, combining bevacizumab with BNCT may mitigate adverse effects such as pseudo-progression and radiation necrosis (64, 65). A multi-institutional, open-label, phase II clinical trial for 27 recurrent MG patients was conducted with the abovementioned accelerator-based BNCT system (JG002). BV-naïve MG patients who experienced recurrence after standard treatment were enrolled between February 2016 and June 2018. In that study, the 1-year survival rate was 79.2% and the MST was 18.7 months in recurrent GBM patients, whereas those of JO22506 patients were 34.5% and 10.5 months, respectively (https://meetings.asco.org/abstracts-presentations/190090). These results are exciting, but the monitoring efficacy of magnetic resonance imaging cannot provide sufficient information on the biological features of the tumor to identify pseudo-progression. Therefore, the use of 18F-BPA-PET to monitor treatment efficacy and evaluate patient prognosis is promising. If the trial is successful, recurrent MG may have a new indicated therapy (JPRN-UMIN000022850).
With successful clinical trials for the treatment of MG underway in the 1990s, researchers began to focus on other cancers. BNCT has achieved great success in treating HNC. Before the advent of immunotherapy, therapeutic approaches for recurrent or locally advanced HNC included only RT, platinum drugs and cetuximab. Thus, there is much room for the development of BNCT to treat HNC. The first patient with recurrent HNC received BNCT in 2001. In the 2000s, several clinical trials were performed in Finland. In a prospective, single-center phase I-II study (NCT00114790), twelve patients with locally advanced inoperable HNC were treated with BNCT. The outcomes included partial response (PR), complete response (CR) in 7 patients, and stable disease (SD) in 2 patients, and the 1-year OS was 66.7%. BNCT was thus shown to be an effective and safe treatment modality for locally advanced inoperable HNCs that recur at previously irradiated sites (67). Another clinical trial (NCT00114790) involving 30 patients with inoperable, locally recurrent HNC also utilized BNCT. The MST after BNCT was 13 months, and the 2-year OS was 30%. BNCT has shown efficacy in treating patients with cancer recurrence at previously irradiated sites, although recurrence remains common (51, 68, 69). The EORTC 11001 study explored the feasibility of BNCT for head and neck squamous cell carcinoma (HNSCC). Prior to the planned tumor resection, three patients received BSH, and three received BPA (71, 72). At that time, several trials were performed in Japan that combined BSH and BPA. There was a cohort of 62 patients, and primary severe Grade 3 or 4 toxicities were manageable (75, 81). In 2008, the dream of “from reactors to accelerators” came true. One interesting trial, including 9 patients with recurrent HNC, was performed in Taiwan, China, in the era of intensity-modulated radiation therapy (IMRT) and suggested that BNCT combined with compensated IMRT can increase treatment homogeneity and conformity compared with BNCT alone, particularly for tumor volumes exceeding 100 cm3, and may improve local tumor control (86). The JHN002 trial included patients with recurrent SCC or with recurrent/locally advanced non-SCC (R/LAnSCC). The ORR for all patients was 71%, and the 2-year OS rates for R-SCC and R/LAnSCC were 58% and 100%, respectively (81). Because of the success of JHN002, the Japanese government approved accelerator-based BNCT equipment, boropharan and health insurance coverage of BNCT for HNC in 2020 (4, 105). Under the Japan National Health Insurance System, a retrospective analysis investigated the first 47 patients treated with BNCT between May 2020 and February 2021 in Japan. All patients had undergone RT. The minimum dose administered to the tumor was 27.4 Gy-Eq, with a range of 13.3–45.2 Gy-Eq. The overall survival rates at 1 and 2 years were 86.1% and 66.5%, indicating high degrees of efficacy and safety (82). Similarly, the other retrospective study included 36 hypopharyngeal/laryngeal cancer patients with prior head and neck irradiation. The CR rate was 72%, and the objective response rate (ORR) was 84%. The MST was 15.5 months, and the 2-year OS was 79.8%. No acute G4–5 adverse events (AEs) were observed except for hyperamylasemia, and no late-phase G3 or higher AEs occurred. This finding demonstrates again that BNCT can achieve a good tumor response while preserving the larynx without severe AEs (83). In addition, a prospective phase I/II trial enrolled 14 patients, and 12 patients received combined treatment. The median BNCT average dose for the gross tumor volume (GTV) was 21.6 Gy-Eq, and the median image-guided intensity-modulated radiotherapy (IG-IMRT) dose for the PTV was 46.8 Gy/26 fractions. The 1-year OS and local PFS rates were 56% and 21%, respectively. Although the trial showed a high response rate (64%), it also experienced a significant incidence of in-field and marginal failure. Future research could explore combining BNCT with non-radiation modalities (87).
Dual-modality cancer treatment may synergistically enhance treatment efficacy. In recent years, the use of BNCT plus another therapeutic modality has gained increasing attention, although such approaches have yet to be widely adopted in clinical practice. Several combined regimens have been proposed, such as BNCT-photon (see clinical trials), BNCT-proton, BNCT-carbon ion radiotherapy (CIRT), BNCT-GdNCT, and BNCT-Ultrasound.
Proton therapy is a promising radiotherapy modality for treating deep-seated and unresectable tumors. However, its biological advantages have not yet been addressed. In 2014, Do-Kun et al. first proposed the idea of proton-boron capture therapy (PBCT), a modality that combines the concepts of proton RT and BNCT (106). Four years later, Cirrone et al. experimentally tested this idea for the first time by using the p+11B→3α nuclear fusion reaction to increase the biological effectiveness of protons (107). Two subsequent reports demonstrated that osteosarcoma cells (108), prostate cancer cells and glioblastoma cells (109) treated with PBCT exhibited reduced survival and increased chromosomal aberrations compared with those treated with protons alone. Furthermore, Manandhar et al. first reported the use of DSBs as a surrogate measure of the dose enhancement effect of alpha particles arising from the proton–boron reaction. They discovered that BSH radiosensitized cells to protons, but this effect was independent of DNA damage (110).
CIRT is a therapeutic modality that relies on the Bragg peak of carbon ions to achieve precise and conformal dose deposition in tumors (111). Theoretically, the combined BNCT–CIRT modality can offer a more homogeneous tumor dose distribution and lower normal tissue toxicity by integrating the biological targeting capabilities of BNCT with the intensity modulation capabilities of CIRT. Han et al. assessed the feasibility and potential advantages of integrating BNCT with CIRT. BNCT–CIRT ensures uniform delivery within the clinical tumor volume (CTV) via the reversed gradient effect from the CIRT component. BNCT–CIRT can thus minimize damage to normal brain tissue and skin (112).
GdNCT is another neutron capture therapy (NCT). BNCT and GdNCT have their own merits and limitations. Higher levels of DNA damage are caused by the release of secondary high-LET particles during BNCT, but improved dose uniformity is expected for the GdNCT technique because secondary particles with lower LET values (electrons and photons) are released during the GdNCT, and 157Gd has a higher neutron capture cross-section than 10B, allowing the use of a lower neutron flux for the NCT technique. In addition, the secondary particles released after neutron capture by 157Gd have long ranges inside the target volume. Therefore, a combination of 10B and 157Gd may improve treatment efficiency in terms of dose uniformity and the relative biological effectiveness (RBE) of DNA damage. Shamsabadi et al. reported that the combined Gd/BNCT technique increases tumor coverage at relatively high doses but reduces the RBE model of DSB induction, potentially affecting the clinical efficacy of NCT (113).
The mechanism of ultrasound differs from that of RT (direct DNA damage). First, ultrasound enhances the sensitivity of tumor cells to radiotherapy (114–116). Second, ultrasound with microbubbles is expected to increase BPA uptake in tumor cells, thereby increasing the boron adsorption capacity. Ultrasound-induced cavitation can compromise the integrity of endothelial and tumor cells by disrupting cell–cell junctions and causing leakage of transport molecules from blood vessels into the tumor microenvironment (TME) (117). Notably, previous study has shown that focused ultrasound (FUS), when combined with a microbubble agent, has ability to temporarily disrupt blood-brain barrier (118). Microbubble-based sonoporation has been shown to reduce the expression of P-glycoprotein in the blood-brain barrier in rats (119). This would increase drug penetration and accumulation in the central nervous system (120), which is one of the excellent advantages for the treatment of glioma using BNCT.
It is now widely acknowledged that high-LET irradiation may be more immunogenic (121). BNCT combined with immunotherapies will thus be a natural future direction (Table 4). As early as 2000, Smilowitz et al. used a malignant rat glioma model of high immunogenicity to evaluate the efficacy of the combination of BNCT and immunoprophylaxis. Half of the rats died after treatment with BNCT alone, but survival was higher after combined treatment. Most surviving rats display immune memory six months or longer after treatment (122). Although second-generation boron agents, including BPA and BSH, have been put into clinical application, they are still not ideal because of insufficient tumor specificity. Synthetic, highly selective and safe boron delivery drugs constitute the key way to overcome the bottleneck of BNCT. Ali Khan et al. reported that the presence of boron-rich liposomes in the blood is crucial for the inhibitory effects of BNCT, whereas direct injection showed no additional benefit in a breast cancer BALB/c mouse model. Compared with other blood components, peripheral blood mononuclear cells (PBMCs) are more likely to assimilate boron-rich liposomes. However, irradiation did not damage the boron-carrying PBMCs. BNCT in PBMCs caused these cells to adopt an antitumor phenotype characterized by increased IL-12 and decreased IL-10 levels. These findings indicate that boron-rich liposome-based BNCT can increase antitumor immunomodulatory effects (123). Shi et al. engineered a neutron-activated boron capsule that synergizes BNCT and controlled immune adjuvant release to elicit a strong antitumor immune response. Like photon radiation, BNCT can remodel the tumor immune microenvironment. Single-cell RNA-Seq analysis indicated that PEG-B-COF+ neutron-treated tumors presented elevated levels of CD4+, CD8+, and Natural killer (NK) cells and a reduced proportion of myeloid cells. The expression of protumoral and immunosuppressive genes was downregulated, whereas the expression of proinflammatory chemokine genes, T/NK cell activation genes, and T/NK cell effector genes was upregulated. Moreover, BNCT can induce immunogenic cell death and exert an abscopal effect (125). Kinashi et al. reported that low-energy head-neutron irradiation damages immune organs in radiosensitive SCID and BALB/c mice and that the combination of BNCT and immunotherapy may not only increase the efficacy but also attenuate damage caused by BNCT (126).
Currently, PD‐1/PD‐L1 inhibitors are the most widely used for immunotherapy. Fujimoto et al. first demonstrated the abscopal effect induced by the combination of BNCT and an anti-PD-1 antibody in an immune checkpoint inhibitor (ICI)-resistant melanoma model (131). Almost simultaneously, Chiu et al. developed amphiphilic PEG-b-PVBE block copolymer micelles and combined these micelles with PD-L1 antibody treatment in a melanoma model. Compared with BNCT alone, combination therapy more effectively inhibited tumor growth and significantly increased T-cell infiltration and activation at tumor sites, indicating a stronger immune response (129).
Myeloid-derived suppressor cells (MDSCs) are a heterogeneous group consisting of granulocytic (G-MDSC) and monocytic (M-MDSC) subsets, each of which inhibits immune function through distinct mechanisms (132, 133). One study revealed that MDSC depletion (CSF-1R inhibitor) combined with BNCT extended mouse survival and promoted tumor immunity by reducing the number of tumor-associated macrophages and increasing the number of CD8+ T cells (127). CD47-blocking immunotherapy activates macrophage-mediated phagocytosis, increases adaptive immunity, and decreases the risk of recurrence. Chen et al. designed a multifunctional nanoliposome delivery system to transport a CD47 targeted CRISPR–Cas9 gene knockout plasmid and a boron delivery drug to the nucleus of tumor tissue in a GBM mouse model, thus increasing antitumor effectiveness (124). Dendritic cells (DCs) are optimal targets for delivering immunogenic cargo because of their strong antigen-presenting abilities. Recently, Lv et al. prepared BMDCs pulsed with BNCT-irradiated tumor cell-derived extracellular vesicles (BEVs) as a tumor vaccine candidate (named BEV@BMDCs), and this treatment elicited strong antitumor immunity in vivo. Vaccination with BEV@BMDCs suppressed primary tumor growth, prevented the formation of metastatic foci, and induced a long-lasting immune response (130).
In addition to being combined with immunotherapy, BNCT combined with anti-inflammatory and anticancer substances may elicit much stronger immune responses. Frydryk Benitez et al. combined (BPA/borophenylalanine+GB-10/decahydrodecaborate)-BNCT (Comb-BNCT) with oligo-fucoidan or glutamine in colon cancer models. They reported that, compared with BPA-BNCT, Comb-BNCT increased therapeutic efficacy, reduced radiotoxicity, and induced both an immune response and an abscopal effect (128).
BNCT is a type of binary therapy for cancer treatment designed to address resistance to conventional treatment. BNCT has notable advantages for the treatment of multiple tiny metastatic foci, and the whole process requires only 1–2 treatments, saving cost and time. This review inspired us several directions for future study. First, understanding the essential molecular mechanisms of BNCT is important. However, mechanistic studies of events in the cell following BNCT are scarce, and most current considerations on this topic are largely inferred from information about the biological effects of high-LET radiation from other sources and of radiomimetic drugs. Therefore, this study could be helpful for researchers and practitioners to better understand the similarities between BNCT-induced damage and other types of radiation damage, as well as the cellular responses to this damage. Second, although clinical trials in glioma and HNC patients have achieved outstanding success, while a strong heterogeneity has been revealed among trials. No randomized controlled trials are currently comparing the first-line treatment of patients. Further optimization and well-designed randomized controlled trials are needed to further validate the efficacy and safety of BNCT in other cancer types. In addition, since BNCT is typically considered during local recurrence, it faces significant challenges in curing tumors during the initial treatment phase. It is important to standardize the treatment protocol of BNCT. Future studies should focus on standardizing treatment protocols and addressing limitations to guide clinical decision-making. Finally, a more profound understanding of the TME and increasingly mature BNCT techniques are needed. We to some extent fill the gap about the role of BNCT on the TME. Combined treatments, including photon, proton, CIRT, GdNCT, and ultrasound, may be new directions for future research. Thus, preclinical studies are needed to understand the radiobiological characteristics and immunomodulatory mechanisms of BNCT. BNCT has a long history, but comprehensive studies are subject to limitations because of the upper threshold level. In particular, BNCT is an intrinsically multidisciplinary field that requires cooperation from researchers in nuclear physics, chemistry, pharmacology, oncology, imaging, computer science and other areas. Additionally, the development of small BNCT devices based on miniaturized accelerators or small neutron sources, such as Cf-252 sources, are needed to reduce the treatment costs of BNCT.
ZZ: Writing – original draft, Writing – review & editing. YC: Writing – original draft, Writing – review & editing. YW: Writing – original draft, Writing – review & editing. YH: Writing – original draft. HG: Writing – original draft. FH: Writing – original draft. FF: Writing – original draft. XL: Conceptualization, Writing – review & editing. RZ: Conceptualization, Funding acquisition, Writing – review & editing. BX: Conceptualization, Writing – review & editing.
The author(s) declare that financial support was received for the research and/or publication of this article. This work was supported by Fujian Provincial Natural Science Foundation of China (No.2022J02037) and Excellent Young Scholars Cultivation Project of Fujian Medical University Union Hospital (2022XH034).
The authors declare that the research was conducted in the absence of any commercial or financial relationships that could be construed as a potential conflict of interest.
The author(s) declare that no Generative AI was used in the creation of this manuscript
All claims expressed in this article are solely those of the authors and do not necessarily represent those of their affiliated organizations, or those of the publisher, the editors and the reviewers. Any product that may be evaluated in this article, or claim that may be made by its manufacturer, is not guaranteed or endorsed by the publisher.
AEs: adverse events
BEVs: BNCT radiated tumor cell-derived extracellular vesicles
BNCT: Boron neutron capture therapy
BPA: Boronophenylalanine
BSH: borocaptate sodium
BV: bevacizumab
CIRT: carbon ion radiotherapy
CR: complete response
CT: computed tomography
CTV: clinical tumor volume
DCs: Dendritic cells
DSBs: double strand breaks
DVH: dose-volume histogram
FUS: focused ultrasound
GBM: glioblastoma
Gd: gadolinium
GdNCT: Gd neutron capture therapy
G-MDSCs: granulocytic MDSCs
GTV: gross tumor volume
HIF-1α: Hypoxia-inducible factor 1α
HMGB1: high mobility group box-1
HNC: head-neck cancer
HNSCC: head and neck squamous cell carcinoma
HR: homologous recombination
ICI: immune checkpoint inhibitor
IMRT: intensity-modulated radiation therapy
LAT1: L-type amino acid transporters
7Li: lithium-7
LET: linear energy transfer
MDSCs: Myeloid-derived suppressor cells
MG: malignant glioma
M-MDSCs: monocytic MDSCs
MRI: magnetic resonance imaging
MST: Median survival times
NCT: neutron capture therapy
NHEJ: non-homologous end joining
NK: natural killer
ORR: objective response rate
OS: overall survival
OSCC: oral squamous cell carcinoma
PAR: Poly(ADP-ribosylation)
PBCT: proton-boron capture therapy
PBMCs: peripheral blood mononuclear cells
PFS: progression-free survival
PR: partial response
RBE: relative biological effectiveness
R/LAnSCC: recurrent/locally advanced non-SCC
RT: radiotherapy
SD: stable disease
SSBs: single-strand breaks
TME: tumor microenvironment
TMZ: temozolomide
TNF: tumor necrosis factor
TPS: treatment planning system
1. Dymova MA, Taskaev SY, Richter VA, Kuligina EV. Boron neutron capture therapy: Current status and future perspectives. Cancer Commun (London England). (2020) 40:406–21. doi: 10.1002/cac2.12089
2. Suski JM, Braun M, Strmiska V, Sicinski P. Targeting cell-cycle machinery in cancer. Cancer Cell. (2021) 39:759–78. doi: 10.1016/j.ccell.2021.03.010
3. Schaue D, McBride WH. Opportunities and challenges of radiotherapy for treating cancer. Nat Rev Clin Oncol. (2015) 12:527–40. doi: 10.1038/nrclinonc.2015.120
4. Nakahara Y, Ito H, Masuoka J, Abe T. Boron neutron capture therapy and photodynamic therapy for high-grade meningiomas. Cancers. (2020) 12:1334. doi: 10.3390/cancers12051334
5. Kumada H, Takada K, Aihara T, Matsumura A, Sakurai H, Sakae T. Verification for dose estimation performance of a Monte-Carlo based treatment planning system in University of Tsukuba. Appl Radiat Isot. (2020) 166:109222. doi: 10.1016/j.apradiso.2020.109222
6. Hu N, Tanaka H, Kakino R, Yoshikawa S, Miyao M, Akita K, et al. Evaluation of a treatment planning system developed for clinical boron neutron capture therapy and validation against an independent Monte Carlo dose calculation system. Radiat Oncol (London England). (2021) 16:243. doi: 10.1186/s13014-021-01968-2
7. Kumada H, Takada K. Treatment planning system and patient positioning for boron neutron capture therapy. Ther Radiol Oncol. (2018) 2:50. doi: 10.21037/tro.2018.10.12
8. Horiguchi H, Sato T, Kumada H, Yamamoto T, Sakae T. Estimation of relative biological effectiveness for boron neutron capture therapy using the PHITS code coupled with a microdosimetric kinetic model. J Radiat Res. (2015) 56:382–90. doi: 10.1093/jrr/rru109
9. Coderre JA, Morris GM. The radiation biology of boron neutron capture therapy. Radiat Res. (1999) 151:1–18. doi: 10.2307/3579742
10. Masunaga S, Sakurai Y, Tanaka H, Tano K, Suzuki M, Kondo N, et al. The dependency of compound biological effectiveness factors on the type and the concentration of administered neutron capture agents in boron neutron capture therapy. SpringerPlus. (2014) 3:128. doi: 10.1186/2193-1801-3-128
11. Sato A, Itoh T, Imamichi S, Kikuhara S, Fujimori H, Hirai T, et al. Proteomic analysis of cellular response induced by boron neutron capture reaction in human squamous cell carcinoma SAS cells. Appl Radiat Isot. (2015) 106:213–9. doi: 10.1016/j.apradiso.2015.08.001
12. Rodriguez C, Carpano M, Curotto P, Thorp S, Casal M, Juvenal G, et al. In vitro studies of DNA damage and repair mechanisms induced by BNCT in a poorly differentiated thyroid carcinoma cell line. Radiat Environ Biophys. (2018) 57:143–52. doi: 10.1007/s00411-017-0729-y
13. Mechetin GV, Zharkov DO. DNA damage response and repair in boron neutron capture therapy. Genes. (2023) 14:127. doi: 10.3390/genes14010127
14. Zhou C, Parsons JL. The radiobiology of HPV-positive and HPV-negative head and neck squamous cell carcinoma. Expert Rev Mol Med. (2020) 22:e3. doi: 10.1017/erm.2020.4
15. Isozaki T, Fujita M, Yamada S, Imadome K, Shoji Y, Yasuda T, et al. Effects of carbon ion irradiation and X-ray irradiation on the ubiquitylated protein accumulation. Int J Oncol. (2016) 49:144–52. doi: 10.3892/ijo.2016.3504
16. Wang Y, Pandey RN, York AJ, Mallela J, Nichols WC, Hu YC, et al. The EYA3 tyrosine phosphatase activity promotes pulmonary vascular remodeling in pulmonary arterial hypertension. Nat Commun. (2019) 10:4143. doi: 10.1038/s41467-019-12226-1
17. Masutani M, Baiseitov D, Itoh T, Hirai T, Berikkhanova K, Murakami Y, et al. Histological and biochemical analysis of DNA damage after BNCT in rat model. Appl Radiat Isot. (2014) 88:104–8. doi: 10.1016/j.apradiso.2014.03.003
18. Fujita Y, Kato I, Iwai S, Ono K, Suzuki M, Sakurai Y, et al. Role of p53 mutation in the effect of boron neutron capture therapy on oral squamous cell carcinoma. Radiat Oncol (London England). (2009) 4:63. doi: 10.1186/1748-717X-4-63
19. Okamoto E, Yamamoto T, Nakai K, Fumiyo Y, Matsumura A. Detection of DNA double-strand breaks in boron neutron capture reaction. Appl Radiat Isot. (2015) 106:185–8. doi: 10.1016/j.apradiso
20. Maliszewska-Olejniczak K, Kaniowski D, Araszkiewicz M, Tymińska K, Korgul A. Molecular mechanisms of specific cellular DNA damage response and repair induced by the mixed radiation field during boron neutron capture therapy. Front Oncol. (2021) 11:676575. doi: 10.3389/fonc.2021.676575
21. Kinashi Y, Yokomizo N, Takahashi S. DNA double-strand breaks induced byFractionated neutron beam irradiation for boron neutron capture therapy. Anticancer Res. (2017) 37:1681–5. doi: 10.21873/anticanres.11499
22. Dagrosa A, Carpano M, Perona M, Thomasz L, Nievas S, Cabrini R, et al. Studies for the application of boron neutron capture therapy to the treatment of differentiated thyroid cancer. Appl Radiat Isot. (2011) 69:1752–5. doi: 10.1016/j.apradiso.2011.02.030
23. Dagrosa MA, Crivello M, Perona M, Thorp S, Santa Cruz GA, Pozzi E, et al. First evaluation of the biologic effectiveness factors of boron neutron capture therapy (BNCT) in a human colon carcinoma cell line. Int J Radiat Oncol Biol Phys. (2011) 79:262–8. doi: 10.1016/j.ijrobp.2010.07.020
24. Basu A, Krishnamurthy S. Cellular responses to Cisplatin-induced DNA damage. J Nucleic Acids. (2010) 2010:201367. doi: 10.4061/2010/201367
25. Kondo N, Sakurai Y, Hirota Y, Tanaka H, Watanabe T, Nakagawa Y, et al. DNA damage induced by boron neutron capture therapy is partially repaired by DNA ligase IV. Radiat Environ Biophys. (2016) 55:89–94. doi: 10.1007/s00411-015-0625-2
26. Perona M, Pontiggia O, Carpano M, Thomasz L, Thorp S, Pozzi E, et al. In vitro studies of cellular response to DNA damage induced by boron neutron capture therapy. Appl Radiat Isot. (2011) 69:1732–6. doi: 10.1016/j.apradiso.2011.03.044
27. Chen KH, Lai ZY, Li DY, Lin YC, Chou FI, Chuang YJ. Analysis of DNA damage responses after boric acid-mediated boron neutron capture therapy in hepatocellular carcinoma. Anticancer Res. (2019) 39:6661–71. doi: 10.21873/anticanres.13881
28. Fujita Y, Yamamoto N, Kato I, Iwai S, Ono K, Sakurai Y, et al. Induction of multinucleation in oral squamous cell carcinoma tissue with mutated p53 surviving boron neutron capture therapy. Int J Radiat Biol. (2011) 87:293–301. doi: 10.3109/09553002.2011.530336
29. Nurse P. A long twentieth century of the cell cycle and beyond. Cell. (2000) 100:71–8. doi: 10.1016/S0092-8674(00)81684-0
30. Hu X, Bryington M, Fisher AB, Liang X, Zhang X, Cui D, et al. Ubiquitin/proteasome-dependent degradation of D-type cyclins is linked to tumor necrosis factor-induced cell cycle arrest. J Biol Chem. (2002) 277:16528–37. doi: 10.1074/jbc.M109929200
31. Sun T, Zhang Z, Li B, Chen G, Xie X, Wei Y, et al. Boron neutron capture therapy induces cell cycle arrest and cell apoptosis of glioma stem/progenitor cells in vitro. Radiat Oncol (London England). (2013) 8:195. doi: 10.1186/1748-717X-8-195
32. Kamida A, Fujita Y, Kato I, Iwai S, Ono K, Suzuki M, et al. Effect of neutron capture therapy on the cell cycle of human squamous cell carcinoma cells. Int J Radiat Biol. (2008) 84:191–9. doi: 10.1080/09553000801902125
33. Seki K, Kinashi Y, Takahashi S. Influence of p53 status on the effects of boron neutron capture therapy in glioblastoma. Anticancer Res. (2015) 35:169–74.
34. Wang P, Zhen H, Jiang X, Zhang W, Cheng X, Guo G, et al. Boron neutron capture therapy induces apoptosis of glioma cells through Bcl-2/Bax. BMC Cancer. (2010) 10:661. doi: 10.1186/1471-2407-10-661
35. Aromando RF, Heber EM, Trivillin VA, Nigg DW, Schwint AE, Itoiz ME. Insight into the mechanisms underlying tumor response to boron neutron capture therapy in the hamster cheek pouch oral cancer model. J Pathol Med. (2009) 38:448–54. doi: 10.1111/j.1600-0714.2008.00720.x
36. Yoshida F, Matsumura A, Shibata Y, Yamamoto T, Nakauchi H, Okumura M, et al. Cell cycle dependence of boron uptake from two boron compounds used for clinical neutron capture therapy. Cancer Lett. (2002) 187:135–41. doi: 10.1016/S0304-3835(02)00380-4
37. Masunaga SI, Sakurai Y, Tanaka H, Takata T, Suzuki M, Sanada Y, et al. Usefulness of combination with both continuous administration of hypoxic cytotoxin and mild temperature hyperthermia in boron neutron capture therapy in terms of local tumor response and lung metastatic potential. Int J Radiat Biol. (2019) 95:1708–17. doi: 10.1080/09553002.2019.1666214
38. Masunaga S, Ono K. Significance of the response of quiescent cell populations within solid tumors in cancer therapy. J Radiat Res. (2002) 43:11–25. doi: 10.1269/jrr.43.11
39. Harada T, Hirose K, Wada Y, Sato M, Ichise K, Aoki M, et al. YC-1 sensitizes the antitumor effects of boron neutron capture therapy in hypoxic tumor cells. J Radiat Res. (2020) 61:524–34. doi: 10.1093/jrr/rraa024
40. Sanada Y, Takata T, Tanaka H, Sakurai Y, Watanabe T, Suzuki M, et al. HIF-1α affects sensitivity of murine squamous cell carcinoma to boron neutron capture therapy with BPA. Int J Radiat Biol. (2021) 97:1441–9. doi: 10.1080/09553002.2021.1956004
41. Kondo N, Barth RF, Miyatake SI, Kawabata S, Suzuki M, Ono K, et al. Cerebrospinal fluid dissemination of high-grade gliomas following boron neutron capture therapy occurs more frequently in the small cell subtype of IDH1(R132H) mutation-negative glioblastoma. J Neuro-oncol. (2017) 133:107–18. doi: 10.1007/s11060-017-2408-x
42. Faião-Flores F, Coelho PR, Toledo Arruda-Neto JD, Maria-Engler SS, Tiago M, Capelozzi VL, et al. Apoptosis through Bcl-2/Bax and cleaved caspase up-regulation in melanoma treated by boron neutron capture therapy. PloS One. (2013) 8:e59639. doi: 10.1371/journal.pone.0059639
43. Malouff TD, Seneviratne DS, Ebner DK, Stross WC, Waddle MR, Trifiletti DM, et al. Boron neutron capture therapy: A review of clinical applications. Front Oncol. (2021) 11:601820. doi: 10.3389/fonc.2021.601820
44. Diaz AZ. Assessment of the results from the phase I/II boron neutron capture therapy trials at the Brookhaven National Laboratory from a clinician’s point of view. J Neuro-oncol. (2003) 62:101–9. doi: 10.1007/BF02699937
45. Chadha M, Capala J, Coderre JA, Elowitz EH, Iwai J, Joel DD, et al. Boron neutron-capture therapy (BNCT) for glioblastoma multiforme (GBM) using the epithermal neutron beam at the Brookhaven National Laboratory. Int J Radiat Oncol Biol Phys. (1998) 40:829–34. doi: 10.1016/S0360-3016(97)00891-2
46. Palmer MR, Goorley JT, Kiger WS, Busse PM, Riley KJ, Harling OK, et al. Treatment planning and dosimetry for the Harvard-MIT Phase I clinical trial of cranial neutron capture therapy. Int J Radiat Oncol Biol Phys. (2002) 53:1361–79. doi: 10.1016/S0360-3016(02)02862-6
47. Kiger WS 3rd, Lu XQ, Harling OK, Riley KJ, Binns PJ, Kaplan J, et al. Preliminary treatment planning and dosimetry for a clinical trial of neutron capture therapy using a fission converter epithermal neutron beam. Appl Radiat Isot. (2004) 61:1075–81. doi: 10.1016/j.apradiso.2004.05.008
48. Vos MJ, Turowski B, Zanella FE, Paquis P, Siefert A, Hideghéty K, et al. Radiologic findings in patients treated with boron neutron capture therapy for glioblastoma multiforme within EORTC trial 11961. Int J Radiat Oncol Biol Phys. (2005) 61:392–9. doi: 10.1016/j.ijrobp.2004.06.008
49. Hideghéty K, Sauerwein W, Wittig A, Götz C, Paquis P, Grochulla F, et al. Tissue uptake of BSH in patients with glioblastoma in the EORTC 11961 phase I BNCT trial. J Neuro-oncol. (2003) 62:145–56. doi: 10.1007/BF02699941
50. Sauerwein W, Moss R, Rassow J, Stecher-Rasmussen F, Hideghéty K, Wolbers JG, et al. Organisation and management of the first clinical trial of BNCT in Europe (EORTC protocol 11961).EORTC BNCT study group. Strahlenther und Onkol. (1999) 175 Suppl 2:108–11. doi: 10.1007/BF03038906
51. Joensuu H, Kankaanranta L, Seppälä T, Auterinen I, Kallio M, Kulvik M, et al. Boron neutron capture therapy of brain tumors: clinical trials at the finnish facility using boronophenylalanine. J Neuro-oncol. (2003) 62:123–34. doi: 10.1007/BF02699939
52. Kankaanranta L, Seppälä T, Koivunoro H, Välimäki P, Beule A, Collan J, et al. L-boronophenylalanine-mediated boron neutron capture therapy for Malignant glioma progressing after external beam radiation therapy: a Phase I study. Int J Radiat Oncol Biol Phys. (2011) 80:369–76. doi: 10.1016/j.ijrobp.2010.02.031
53. Henriksson R, Capala J, Michanek A, Lindahl SA, Salford LG, Franzén L, et al. Boron neutron capture therapy (BNCT) for glioblastoma multiforme: a phase II study evaluating a prolonged high-dose of boronophenylalanine (BPA). Radiother Oncol. (2008) 88:183–91. doi: 10.1016/j.radonc.2006.04.015
54. Pellettieri L, H-Stenstam B, Rezaei A, Giusti V, Sköld K. An investigation of boron neutron capture therapy for recurrent glioblastoma multiforme. Acta Neurol Scand. (2008) 117:191–7. doi: 10.1111/j.1600-0404.2007.00924.x
55. Kageji T, Nagahiro S, Matsuzaki K, Mizobuchi Y, Toi H, Nakagawa Y, et al. Boron neutron capture therapy using mixed epithermal and thermal neutron beams in patients with Malignant glioma-correlation between radiation dose and radiation injury and clinical outcome. Int J Radiat Oncol Biol Phys. (2006) 65:1446–55. doi: 10.1016/j.ijrobp.2006.03.016
56. Kageji T, Mizobuchi Y, Nagahiro S, Nakagawa Y, Kumada H. Clinical results of boron neutron capture therapy (BNCT) for glioblastoma. Appl Radiat Isot. (2011) 69:1823–5. doi: 10.1016/j.apradiso.2011.05.029
57. Kageji T, Mizobuchi Y, Nagahiro S, Nakagawa Y, Kumada H. Long-survivors of glioblatoma treated with boron neutron capture therapy (BNCT). Appl Radiat Isot. (2011) 69:1800–2. doi: 10.1016/j.apradiso.2011.03.021
58. Yamamoto T, Matsumura A, Nakai K, Shibata Y, Endo K, Sakurai F, et al. Current clinical results of the Tsukuba BNCT trial. Appl Radiat Isot. (2004) 61:1089–93. doi: 10.1016/j.apradiso.2004.05.010
59. Yamamoto T, Nakai K, Kageji T, Kumada H, Endo K, Matsuda M, et al. Boron neutron capture therapy for newly diagnosed glioblastoma. Radiother Oncol. (2009) 91:80–4. doi: 10.1016/j.radonc.2009.02.009
60. Miyatake S, Kawabata S, Kajimoto Y, Aoki A, Yokoyama K, Yamada M, et al. Modified boron neutron capture therapy for Malignant gliomas performed using epithermal neutron and two boron compounds with different accumulation mechanisms: an efficacy study based on findings on neuroimages. J Neurosurg. (2005) 103:1000–9. doi: 10.3171/jns.2005.103.6.1000
61. Kawabata S, Miyatake S, Kuroiwa T, Yokoyama K, Doi A, Iida K, et al. Boron neutron capture therapy for newly diagnosed glioblastoma. J Radiat Res. (2009) 50:51–60. doi: 10.1269/jrr.08043
62. Miyatake S, Kawabata S, Yokoyama K, Kuroiwa T, Michiue H, Sakurai Y, et al. Survival benefit of Boron neutron capture therapy for recurrent Malignant gliomas. J Neuro-oncol. (2009) 91:199–206. doi: 10.1007/s11060-008-9699-x
63. Kawabata S, Miyatake S, Hiramatsu R, Hirota Y, Miyata S, Takekita Y, et al. Phase II clinical study of boron neutron capture therapy combined with X-ray radiotherapy/temozolomide in patients with newly diagnosed glioblastoma multiforme–study design and current status report. Appl Radiat Isot. (2011) 69:1796–9. doi: 10.1016/j.apradiso.2011.03.014
64. Furuse M, Kawabata S, Wanibuchi M, Shiba H, Takeuchi K, Kondo N, et al. Boron neutron capture therapy and add-on bevacizumab in patients with recurrent Malignant glioma. Jpn J Clin Oncol. (2022) 52:433–40. doi: 10.1093/jjco/hyac004
65. Shiba H, Takeuchi K, Hiramatsu R, Furuse M, Nonoguchi N, Kawabata S, et al. Boron neutron capture therapy combined with early successive bevacizumab treatments for recurrent Malignant gliomas - A pilot study. Neurol Medico-chir. (2018) 58:487–94. doi: 10.2176/nmc.oa.2018-0111
66. Miyatake S, Kawabata S, Goto H, Narita Y, Suzuki M, Hirose K, et al. Accelerator-based BNCT in rescue treatment of patients with recurrent GBM: A multicenter phase II study. J Clin Oncol. (2020) 38:15_suppl. doi: 10.1200/JCO.2020.38.15_suppl.2536
67. Kankaanranta L, Seppälä T, Koivunoro H, Saarilahti K, Atula T, Collan J, et al. Boron neutron capture therapy in the treatment of locally recurred head and neck cancer. Int J Radiat Oncol Biol Phys. (2007) 69:475–82. doi: 10.1016/j.ijrobp.2007.03.039
68. Kankaanranta L, Seppälä T, Koivunoro H, Saarilahti K, Atula T, Collan J, et al. Boron neutron capture therapy in the treatment of locally recurred head-and-neck cancer: final analysis of a phase I/II trial. Int J Radiat Oncol Biol Phys. (2012) 82:e67–75. doi: 10.1016/j.ijrobp.2010.09.057
69. Kouri M, Kankaanranta L, Seppälä T, Tervo L, Rasilainen M, Minn H, et al. Undifferentiated sinonasal carcinoma may respond to single-fraction boron neutron capture therapy. Radiother Oncol. (2004) 72:83–5. doi: 10.1016/j.radonc.2004.03.016
70. Haapaniemi A, Kankaanranta L, Saat R, Koivunoro H, Saarilahti K, Mäkitie A, et al. Boron neutron capture therapy in the treatment of recurrent laryngeal cancer. Int J Radiat Oncol Biol Phys. (2016) 95:404–10. doi: 10.1016/j.ijrobp.2015.11.010
71. Wittig A, Collette L, Appelman K, Bührmann S, Jäckel MC, Jöckel KH, et al. EORTC trial 11001: distribution of two 10B-compounds in patients with squamous cell carcinoma of head and neck, a translational research/phase 1 trial. J Cell Mol Med. (2009) 13:1653–65. doi: 10.1111/j.1582-4934.2009.00856.x
72. Wittig A, Malago M, Collette L, Huiskamp R, Bührmann S, Nievaart V, et al. Uptake of two 10B-compounds in liver metastases of colorectal adenocarcinoma for extracorporeal irradiation with boron neutron capture therapy (EORTC Trial 11001). Int J Cancer. (2008) 122:1164–71. doi: 10.1002/ijc.v122:5
73. Kato I, Ono K, Sakurai Y, Ohmae M, Maruhashi A, Imahori Y, et al. Effectiveness of BNCT for recurrent head and neck Malignancies. Appl Radiat Isot. (2004) 61:1069–73. doi: 10.1016/j.apradiso.2004.05.059
74. Kato I, Fujita Y, Maruhashi A, Kumada H, Ohmae M, Kirihata M, et al. Effectiveness of boron neutron capture therapy for recurrent head and neck Malignancies. Appl Radiat Isot. (2009) 67:S37–42. doi: 10.1016/j.apradiso.2009.03.103
75. Suzuki M, Kato I, Aihara T, Hiratsuka J, Yoshimura K, Niimi M, et al. Boron neutron capture therapy outcomes for advanced or recurrent head and neck cancer. J Radiat Res. (2014) 55:146–53. doi: 10.1093/jrr/rrt098
76. Aihara T, Morita N, Kamitani N, Kumada H, Ono K, Hiratsuka J, et al. Boron neutron capture therapy for advanced salivary gland carcinoma in head and neck. Int J Clin Oncol. (2014) 19:437–44. doi: 10.1007/s10147-013-0580-3
77. Aihara T, Morita N, Kamitani N, Kumada H, Ono K, Hiratsuka J, et al. BNCT for advanced or recurrent head and neck cancer. Appl Radiat Isot. (2014) 88:12–5. doi: 10.1016/j.apradiso.2014.04.007
78. Kimura Y, Ariyoshi Y, Shimahara M, Miyatake S, Kawabata S, Ono K, et al. Boron neutron capture therapy for recurrent oral cancer and metastasis of cervical lymph node. Appl Radiat Isot. (2009) 67:S47–9. doi: 10.1016/j.apradiso.2009.03.019
79. Kato I, Fujita Y, Ohmae M, Sakurai Y, Suzuki M, Masunaga S, et al. Boron neutron capture therapy in patients with recurrent head and neck cancers who have no other treatment options. J Clin Oncol. (2014) 32:15_suppl. doi: 10.1200/jco.2014.32.15_suppl.6046
80. Morita T, Kurihara H, Hiroi K, Honda N, Igaki H, Hatazawa J, et al. Dynamic changes in (18)F-borono-L-phenylalanine uptake in unresectable, advanced, or recurrent squamous cell carcinoma of the head and neck and Malignant melanoma during boron neutron capture therapy patient selection. Radiat Oncol (London England). (2018) 13:4. doi: 10.1186/s13014-017-0949-y
81. Hirose K, Konno A, Hiratsuka J, Yoshimoto S, Kato T, Ono K, et al. Boron neutron capture therapy using cyclotron-based epithermal neutron source and borofalan ((10)B) for recurrent or locally advanced head and neck cancer (JHN002): An open-label phase II trial. Radiother Oncol. (2021) 155:182–7. doi: 10.1016/j.radonc.2020.11.001
82. Hirose K, Sato M. Clinical results and prognostic factors in boron neutron capture therapy for recurrent squamous cell carcinoma of the head and neck under the Japan national health insurance system: A retrospective study of the initial 47 patients. Int J Radiat Oncol Biol Phys. (2024) 120:796–804. doi: 10.1016/j.ijrobp.2024.03.041
83. Sato M, Hirose K. Efficacy and safety of boron neutron capture therapy for Hypopharyngeal/Laryngeal cancer patients with previous head and neck irradiation. Radiother Oncol. (2024) 198:110382. doi: 10.1016/j.radonc.2024.110382
84. Wang LW, Chen YW, Ho CY, Hsueh Liu YW, Chou FI, Liu YH, et al. Fractionated BNCT for locally recurrent head and neck cancer: experience from a phase I/II clinical trial at Tsing Hua Open-Pool Reactor. Appl Radiat Isot. (2014) 88:23–7. doi: 10.1016/j.apradiso.2013.11.134
85. Wang LW, Chen YW, Ho CY, Hsueh Liu YW, Chou FI, Liu YH, et al. Fractionated boron neutron capture therapy in locally recurrent head and neck cancer: A prospective phase I/II trial. Int J Radiat Oncol Biol Phys. (2016) 95:396–403. doi: 10.1016/j.ijrobp.2016.02.028
86. Lee JC, Chuang KS, Hsueh Liu YW, Lin TY, Teng YC, Wang LW. A comparison of dose distributions in gross tumor volume between boron neutron capture therapy alone and combined boron neutron capture therapy plus intensity modulation radiation therapy for head and neck cancer. PloS One. (2019) 14:e0210626. doi: 10.1371/journal.pone.0210626
87. Wang LW, Liu YH, Chu PY, Liu HM, Peir JJ, Lin KH, et al. Boron neutron capture therapy followed by image-guided intensity-modulated radiotherapy for locally recurrent head and neck cancer: A prospective phase I/II trial. Cancers. (2023) 15:2762. doi: 10.3390/cancers15102762
88. Saidi D, Cheray M, Osman AM, Stratoulias V, Lindberg OR, Shen X, et al. Glioma-induced SIRT1-dependent activation of hMOF histone H4 lysine 16 acetyltransferase in microglia promotes a tumor supporting phenotype. Oncoimmunology. (2018) 7:e1382790. doi: 10.1080/2162402X.2017.1382790
89. Yang K, Wu Z, Zhang H, Zhang N, Wu W, Wang Z, et al. Glioma targeted therapy: insight into future of molecular approaches. Mol Cancer. (2022) 21:39. doi: 10.1186/s12943-022-01513-z
90. Weller M, Le Rhun E. How did lomustine become standard of care in recurrent glioblastoma? Cancer Treat Rev. (2020) 87:102029. doi: 10.1016/j.ctrv.2020.102029
91. Halperin EC, Burger PC, Bullard DE. The fallacy of the localized supratentorial Malignant glioma. Int J Radiat Oncol Biol Phys. (1988) 15:505–9. doi: 10.1016/S0360-3016(98)90036-0
92. Sullivan FJ, Herscher LL, Cook JA, Smith J, Steinberg SM, Epstein AH, et al. National Cancer Institute (phase II) study of high-grade glioma treated with accelerated hyperfractionated radiation and iododeoxyuridine: results in anaplastic astrocytoma. Int J Radiat Oncol Biol Phys. (1994) 30:583–90. doi: 10.1016/0360-3016(92)90944-D
93. Sweet WH. The uses of nuclear disintegration in the diagnosis and treatment of brain tumor. New Engl J Med. (1951) 245:875–8. doi: 10.1056/NEJM195112062452301
94. Farr LE, Sweet WH, Robertson JS, Foster CG, Locksley HB, Sutherland DL, et al. Neutron capture therapy with boron in the treatment of glioblastoma multiforme. Am J Roentgenol Radium Ther Nuclear Med. (1954) 71:279–93.
95. Slatkin DN, Stoner RD, Rosander KM, Kalef-Ezra JA, Laissue JA. Central nervous system radiation syndrome in mice from preferential 10B(n, alpha)7Li irradiation of brain vasculature. Proc Natl Acad Sci United States Am. (1988) 85:4020–4. doi: 10.1073/pnas.85.11.4020
96. Sweet WH. Early history of development of boron neutron capture therapy of tumors. J Neuro-oncol. (1997) 33:19–26. doi: 10.1023/A:1005752827194
97. Asbury AK, Ojemann RG, Nielsen SL, Sweet WH. Neuropathologic study of fourteen cases of Malignant brain tumor treated by boron-10 slow neutron capture radiation. J Neuropathol Exp Neurol. (1972) 31:278–303. doi: 10.1097/00005072-197204000-00005
98. Goodwin JT, Farr LE, Sweet WH, Robertson JS. Pathological study of eight patients with glioblastoma multiforme treated by neutron-capture therapy using boron 10. Cancer. (1955) 8:601–15. doi: 10.1002/1097-0142(1955)8:3<601::aid-cncr2820080326>3.0.co;2-r
99. Slatkin DN. A history of boron neutron capture therapy of brain tumours. Postulation of a brain radiation dose tolerance limit. Brain. (1991) 114:1609–29. doi: 10.1093/brain/114.4.1609
100. Hatanaka H. Clinical results of boron neutron capture therapy. Basic Life Sci. (1990) 54:15–21. doi: 10.1007/978-1-4684-5802-2_2
101. Hatanaka H, Nakagawa Y. Clinical results of long-surviving brain tumor patients who underwent boron neutron capture therapy. Int J Radiat Oncol Biol Phys. (1994) 28:1061–6. doi: 10.1016/0360-3016(94)90479-0
102. Stupp R, Mason WP, van den Bent MJ, Weller M, Fisher B, Taphoorn MJ, et al. Radiotherapy plus concomitant and adjuvant temozolomide for glioblastoma. New Engl J Med. (2005) 352:987–96. doi: 10.1056/NEJMoa043330
103. Hopewell JW, Gorlia T, Pellettieri L, Giusti V, H-Stenstam B, Sköld K. Boron neutron capture therapy for newly diagnosed glioblastoma multiforme: an assessment of clinical potential. Appl Radiat Isot. (2011) 69:1737–40. doi: 10.1016/j.apradiso.2011.03.022
104. Sander A, Wosniok W, Gabel D. Case numbers for a randomized clinical trial of boron neutron capture therapy for Glioblastoma multiforme. Appl Radiat Isot. (2014) 88:16–9. doi: 10.1016/j.apradiso.2013.11.092
105. He H, Li J, Jiang P, Tian S, Wang H, Fan R, et al. The basis and advances in clinical application of boron neutron capture therapy. Radiat Oncol (London England). (2021) 16:216. doi: 10.1186/s13014-021-01939-7
106. Yoon DK JJ, Suh TS. Application of proton boron fusion reaction to radiation therapy: A Monte Carlo simulation study. Appl Phys Lett. (2014) 105:223507. doi: 10.1063/1.4903345
107. Cirrone GAP, Manti L, Margarone D, Petringa G, Giuffrida L, Minopoli A, et al. First experimental proof of Proton Boron Capture Therapy (PBCT) to enhance protontherapy effectiveness. Sci Rep. (2018) 8:1141. doi: 10.1038/s41598-018-19258-5
108. Zavestovskaya IN, Popov AL, Kolmanovich DD, Tikhonowski GV, Pastukhov AI, Savinov MS, et al. Boron nanoparticle-enhanced proton therapy for cancer treatment. Nanomater (Basel Switzerland). (2023) 13:2167. doi: 10.3390/nano13152167
109. Jelínek Michaelidesová A, Kundrát P, Zahradníček O, Danilová I, Pachnerová Brabcová K, Vachelová J, et al. First independent validation of the proton-boron capture therapy concept. Sci Rep. (2024) 14:19264. doi: 10.1038/s41598-024-69370-y
110. Manandhar M, Bright SJ, Flint DB, Martinus DKJ, Kolachina RV, Ben Kacem M, et al. Effect of boron compounds on the biological effectiveness of proton therapy. Med Phys. (2022) 49:6098–109. doi: 10.1002/mp.15824
111. Durante M, Paganetti H. Nuclear physics in particle therapy: a review. Rep Prog Phys. (2016) 79:096702. doi: 10.1088/0034-4885/79/9/096702
112. Han Y, Geng C, Altieri S, Bortolussi S, Liu Y, Wahl N, et al. Combined BNCT-CIRT treatment planning for glioblastoma using the effect-based optimization. Phys Med Biol. (2023) 69. doi: 10.1088/1361-6560/ad120f
113. Shamsabadi R, Baghani HR. DNA-damage RBE assessment for combined boron and gadolinium neutron capture therapy. J Appl Clin Med Phys. (2024) 25:e14399. doi: 10.1002/acm2.14399
114. Bergs JW, Krawczyk PM, Borovski T, ten Cate R, Rodermond HM, Stap J, et al. Inhibition of homologous recombination by hyperthermia shunts early double strand break repair to non-homologous end-joining. DNA Repair. (2013) 12:38–45. doi: 10.1016/j.dnarep.2012.10.008
115. Zhu L, Altman MB, Laszlo A, Straube W, Zoberi I, Hallahan DE, et al. Ultrasound hyperthermia technology for radiosensitization. Ultrasound Med Biol. (2019) 45:1025–43. doi: 10.1016/j.ultrasmedbio.2018.12.007
116. Deng H, Cai Y, Feng Q, Wang X, Tian W, Qiu S, et al. Ultrasound-stimulated microbubbles enhance radiosensitization of nasopharyngeal carcinoma. Cell Physiol Biochem. (2018) 48:1530–42. doi: 10.1159/000492263
117. Ibsen S, Schutt CE, Esener S. Microbubble-mediated ultrasound therapy: a review of its potential in cancer treatment. Drug Design Dev Ther. (2013) 7:375–88. doi: 10.2147/DDDT.S31564
118. Hynynen K, McDannold N, Vykhodtseva N, Jolesz FA. Noninvasive MR imaging-guided focal opening of the blood-brain barrier in rabbits. Radiology. (2001) 220:640–6. doi: 10.1148/radiol.2202001804
119. Aryal M, Fischer K, Gentile C, Gitto S, Zhang YZ, McDannold N. Effects on P-glycoprotein expression after blood-brain barrier disruption using focused ultrasound and microbubbles. PloS One. (2017) 12:e0166061. doi: 10.1371/journal.pone.0166061
120. Przystupski D, Ussowicz M. Landscape of cellular bioeffects triggered by ultrasound-induced sonoporation. Int J Mol Sci. (2022) 23:11222. doi: 10.3390/ijms231911222
121. Zhou Z, Guan B, Xia H, Zheng R, Xu B. Particle radiotherapy in the era of radioimmunotherapy. Cancer Lett. (2023) 567:216268. doi: 10.1016/j.canlet.2023.216268
122. Smilowitz HM, Micca PL, Nawrocky MM, Slatkin DN, Tu W, Coderre JA. The combination of boron neutron-capture therapy and immunoprophylaxis for advanced intracerebral gliosarcomas in rats. J Neuro-oncol. (2000) 46:231–40. doi: 10.1023/A:1006409721365
123. Khan AA, Maitz C, Quanyu C, Hawthorne F. BNCT induced immunomodulatory effects contribute to mammary tumor inhibition. PloS One. (2019) 14:e0222022. doi: 10.1371/journal.pone.0222022
124. Chen J, Dai Q, Yang Q, Bao X, Zhou Y, Zhong H, et al. Therapeutic nucleus-access BNCT drug combined CD47-targeting gene editing in glioblastoma. J Nanobiotechnol. (2022) 20:102. doi: 10.1186/s12951-022-01304-0
125. Shi Y, Guo Z, Fu Q, Shen X, Zhang Z, Sun W, et al. Localized nuclear reaction breaks boron drug capsules loaded with immune adjuvants for cancer immunotherapy. Nat Commun. (2023) 14:1884. doi: 10.1038/s41467-023-37253-x
126. Kinashi Y, Takata T, Sakurai Y, Tanaka H. Carcinogenesis observed in the spleens of balb/c mice after head-neutron irradiation. Anticancer Res. (2023) 43:1455–61. doi: 10.21873/anticanres.16294
127. Chang CH, Chen CJ, Yu CF, Tsai HY, Chen FH, Chiang CS. Targeting M-MDSCs enhances the therapeutic effect of BNCT in the 4-NQO-induced murine head and neck squamous cell carcinoma model. Front Oncol. (2023) 13:1263873. doi: 10.3389/fonc.2023.1263873
128. Frydryk Benitez DN, Palmieri MA, Langle YV, Monti Hughes A, Pozzi ECC, Thorp SI, et al. Therapeutic efficacy, radiotoxicity and abscopal effect of BNCT at the RA-3 nuclear reactor employing oligo-fucoidan and glutamine as adjuvants in an ectopic colon cancer model in rats. Life (Basel Switzerland). (2023) 13:1538. doi: 10.3390/life13071538
129. Chiu YL, Fu WY, Huang WY, Hsu FT, Chen HW, Wang TW, et al. Enhancing cancer therapy: boron-rich polyboronate ester micelles for synergistic boron neutron capture therapy and PD-1/PD-L1 checkpoint blockade. Biomater Res. (2024) 28:0040. doi: 10.34133/bmr.0040
130. Lv L, Zhang J, Wang Y, Liang H, Liu Q, Hu F, et al. Boron neutron capture therapy-derived extracellular vesicles via DNA accumulation boost antitumor dendritic cell vaccine efficacy. Adv Sci (Weinheim Baden-Wurttemberg Germany). (2024) 11:e2405158. doi: 10.1002/advs.202405158
131. Fujimoto T, Yamasaki O, Kanehira N, Matsushita H, Sakurai Y, Kenmotsu N, et al. Overcoming immunotherapy resistance and inducing abscopal effects with boron neutron immunotherapy (B-NIT). Cancer Sci. (2024) 115:3231–47. doi: 10.1111/cas.v115.10
132. Marigo I, Dolcetti L, Serafini P, Zanovello P, Bronte V. Tumor-induced tolerance and immune suppression by myeloid derived suppressor cells. Immunol Rev. (2008) 222:162–79. doi: 10.1111/j.1600-065X.2008.00602.x
Keywords: boron neutron capture therapy, radiobiology, clinical trials, immunotherapy, combined treatments
Citation: Zhou Z, Chen Y, Wang Y, Hong Y, Guan H, Huang F, Fu F, Li X, Zheng R and Xu B (2025) ‘Cell knife’ for cancer: the clinician’s perspective. Front. Immunol. 16:1536355. doi: 10.3389/fimmu.2025.1536355
Received: 28 November 2024; Accepted: 31 March 2025;
Published: 17 April 2025.
Edited by:
Silvia Capuani, National Research Council (CNR), ItalyReviewed by:
Sunyoung Jang, The Pennsylvania State University, United StatesCopyright © 2025 Zhou, Chen, Wang, Hong, Guan, Huang, Fu, Li, Zheng and Xu. This is an open-access article distributed under the terms of the Creative Commons Attribution License (CC BY). The use, distribution or reproduction in other forums is permitted, provided the original author(s) and the copyright owner(s) are credited and that the original publication in this journal is cited, in accordance with accepted academic practice. No use, distribution or reproduction is permitted which does not comply with these terms.
*Correspondence: Xiaobo Li, bGl4aWFvYm8yMDA0QDEyNi5jb20=; Rong Zheng, emhlbmdycm9uZ0BvdXRsb29rLmNvbQ==; Benhua Xu, YmVuaHVheHVAc2luYS5jb20=
†These authors have contributed equally to this work
Disclaimer: All claims expressed in this article are solely those of the authors and do not necessarily represent those of their affiliated organizations, or those of the publisher, the editors and the reviewers. Any product that may be evaluated in this article or claim that may be made by its manufacturer is not guaranteed or endorsed by the publisher.
Research integrity at Frontiers
Learn more about the work of our research integrity team to safeguard the quality of each article we publish.