- 1Faculty of Health and Medical Sciences, University of Surrey, Guildford, United Kingdom
- 2Department of Virology, Animal and Plant Health Agency, Addlestone, United Kingdom
The type I interferons (IFNs) are a group of key cytokines of the vertebrate innate immune system that induce an antiviral state in uninfected cells. Experimental in-vitro and in-vivo data have proven the fundamental role these cytokines possess in the protective response to a wide variety of pathogens, including herpesviruses. In a clinical setting, IFNs have been an important treatment in humans for several decades and increasing evidence demonstrates their potential in controlling viral haemorrhagic fevers when administered early in disease. In juvenile Asian elephants, elephant endotheliotropic herpesvirus haemorrhagic disease (EEHV-HD) often proves fatal when an effective adaptive immune response cannot be mounted in time, suggesting that an enhancement of the innate immune response could provide protection. This study sequenced six members of the Asian elephant type I IFNs, most closely related to sequences from the African elephant and Florida manatee. Subsequently, recombinant Asian elephant IFNα and IFNβ proteins were expressed and assessed for bioactivity in-vitro, relative to recombinant human IFNs, using a novel infection model incorporating primary Asian elephant fibroblasts and bovine alphaherpesvirus 1 (BoHV-1) as a surrogate for EEHV. In a dose-dependent manner, both Asian elephant IFNs and human IFNα2a protected cells from BoHV-1 infection in this proof-of-concept study, even if applied up to 24 hours post-infection in-vitro.
1 Introduction
Interferons (IFNs) are a conserved family of pleiotropic cytokines, identified across all vertebrate species (1, 2). Type I IFNs (IFN-I) have been identified through their antiviral effects, but alongside their immunomodulatory activities (3–5), they provide a crucial stimulatory bridge between the innate and adaptive immune responses (6–10). IFNα is a multigene cluster in mammals, whereas only one IFNβ gene exists in humans, mice and horses (11, 12). Once IFN-I bind to their receptor, IFN signalling via the JAK/STAT pathway results in the transcription of hundreds of interferon-stimulated genes, [ISGs; (13–16)], proven to be vital in the immune response to invading pathogens, including herpesviruses (17, 18).
Elephant endotheliotropic herpesviruses (EEHVs), also known as Elephantid betaherpesviruses, are a group of potentially fatal viruses in the genus Proboscivirus, within the Betaherpesvirinae (19, 20). Since the initial cases, over 100 fatalities associated with EEHV haemorrhagic disease (EEHV-HD) have been reported in both ex-situ (captive) and in-situ (both wild and captive in range countries) juvenile Asian elephants (Elephas maximus) between the ages of 1 and 8 years old (21–27). Since their initial identification in 1995 (28), the EEHV-HD mortality rate in captive Asian elephants has been reported to be as high as 90% (29). Additionally, as no anti-viral compound has yet proven effective against EEHVs, these viruses remain among the highest threats to the sustainability of captive populations of this endangered species (30–34).
Although the clinical disease associated with EEHVs is often acute, clinical signs are preceded by a period of subclinical infection (35–37) before an exponential increase in blood EEHV load and disease manifestation (38, 39). Therefore, the application of veterinary antiviral compounds at a sufficiently early stage of infection may prove beneficial in limiting the development of EEHV-HD.
The exogenous administration of IFNs has been widely applied to viral diseases of humans, establishing them as important therapeutics, most notably in relation to hepatitis B and C (40–45). Additionally, IFNs have emerged as a crucial part of the innate response to viral haemorrhagic fevers, with an early and sustained response correlated with survival (46–50). Therefore, the clinical application of IFN-I against EEHV infections warrants further investigation. However, the lack of elephant IFN-I interferons, and the ongoing inability to culture EEHVs in-vitro, has hindered more targeted research.
This study aimed to characterise Asian elephant type I IFNs, including their gene sequences, expression of the associated proteins, and evaluation of their in-vitro antiviral bioactivity, relative to human IFNs, using an in-vitro herpesviral infection model. The preliminary results of this study may provide additional interventional strategies to boost the Asian elephant innate immune system in the early stages of EEHV infections to prevent progression to clinical disease.
2 Materials and methods
2.1 Animals and sample collection
The single Asian elephant used in this study was a seven-year-old captive-born female housed at a zoological collection within the United Kingdom. The animal was clinically healthy at the time of sample collection. Blood samples were collected under trained behaviour into ethylenediaminetetraacetic acid (EDTA) blood tubes for the purpose of routine EEHV monitoring and/or diagnostics according to routine veterinary practices. Veterinary interventions such as blood sample collection for the purpose of clinical health screening fall under the Veterinary Surgeons Act 1966 and do not require further ethical approval, as they are intended solely to maintain animal welfare. Samples were sent at ambient temperature overnight to the laboratory and refrigerated at 4-8°C until being processed within 2 hours.
2.2 Extraction of genomic DNA (gDNA)
Extraction of gDNA from 140 µL aliquots of whole EDTA blood was performed using the QIAamp Viral RNA Mini Kit (Qiagen, Manchester, UK) according to the manufacturer’s instructions and samples were eluted into a final volume of 60 μL of buffer AVE.
2.3 Identification of Asian elephant IFN genes
The published sequences from six Asian elephant genomes [NCBI SRA database https://www.ncbi.nlm.nih.gov/sra; accession numbers SRS927124, SRS927123, SRS927126, SRP065915, SRS1158889, ERS365881 and ERS365882; (51, 52)] were used in combination with the online NCBI BLAST tool (https://blast.ncbi.nlm.nih.gov/Blast.cgi) for the assembly of predicted Asian elephant IFNα and IFNβ genes. The published corresponding gene sequences of the horse (Equus caballus; accession numbers NM_001099441, NM_001099440), and predicted corresponding gene sequences of the African elephant (Loxodonta africana; accession numbers XM_003407658.2, XM_003407655.2, XM_023545333.1, XM_003407652.2, XM_003407651.2, XM_003407333.2, XM_023545332.1, XM_010587902.2, XM_003407336.2) were used as respective templates in predicting IFNs of Asian elephants. The 5’ and 3’ untranslated regions (UTRs) of the IFN genes were also included in their assemblies. Primers were designed within the UTRs either side of the 5’ and the 3’ ends of the predicted IFN genes to verify entire coding sequences through polymerase chain reaction (PCR) and Sanger sequencing.
Primers used in this study were synthesised by Eurofins Genomics (Ebersburg, Germany; Table 1). PCRs were performed in a Veriti Thermal Cycler (Life Technologies, Paisley, UK) and, as target genes were predicted to be intronless, gDNA was used as a template. Each PCR contained 10 μl of Fast Cycling PCR Master Mix (Qiagen), 2 pmol each of forward and reverse primer, 2 μl of gDNA and nuclease-free water to a final reaction volume of 20 μl. Thermocycling was performed for five minutes at 95°C, followed by 40 cycles of 95°C for 15 seconds, 55°C for 30 seconds and 72°C for one minute.
Aliquots of each PCR product were mixed at a ratio of 5:1 with 6x Blue/Orange Loading Dye (Promega UK Ltd.), separated through electrophoresis in a 2% agarose gel containing SYBR® Safe DNA Gel stain (Life Technologies) and visualised under ultraviolet light using a Universal Hood II transilluminator (Bio-Rad, Watford, UK). DNA bands of the predicted size were cut from the gel and purified using the MinElute® Gel Extraction Kit (Qiagen) according to the manufacturer’s instructions.
Purified DNA amplicons were subjected to a single run of Sanger sequencing at the APHA Central Sequencing Unit. The nucleotide (NT) and deduced amino acid (AA) sequences were analysed via NCBI BLAST and the Protein Homology/Analogy Recognition Engine V 2.0 [Phyre2; http://www.sbg.bio.ic.ac.uk/phyre2/html/page.cgi?id=index; (53)] respectively. All NT and AA sequence alignments were performed using ClustalW in MegAlign 15.0 (DNASTAR).
2.4 Cloning Asian elephant IFN genes
Amino acid sequences of the IFNα (EleIFNα1) and IFNβ (EleIFNβ) genes were analysed for signalling peptides using the SignalP v 4.0 online tool [http://www.cbs.dtu.dk/services/SignalP/index.php; (54)]. The signalling peptide NT sequences and the stop codon were then excluded from each gene sequence. The IFNα and IFNβ sequences were each codon-optimised for expression in Escherichia coli (E.coli) using Eurofins Genomics online software (https://www.eurofinsgenomics.eu/en/home), synthesised, and sub-cloned into Invitrogen™ Champion™ pET303CT-His plasmid vectors (Fischer Scientific, Loughborough, UK).
2.5 Bacterial expression and purification of IFN proteins
The pET303CT-His plasmid vectors were transformed into BL21(DE3) Competent Cells (New England Biolabs, Hitchin, UK) according to the manufacturer’s instructions. Up to 100 µL of each transformation reaction was spread over LB agar plates infused with 100 μg/mL ampicillin, and single colonies were picked the following day to inoculate a 10 mL starter culture of selective LB broth (containing 100 μg/mL ampicillin).
Starter cultures were screened after 24 hours for gene inserts using T7 sequencing primers (Table 1) in a PCR as described above but only running for 35 cycles. Amplicons were verified by visualisation of the predicted size DNA band following agarose gel electrophoresis. Aliquots of each starter culture were then added to an expression culture of selective LB broth. Expression cultures were incubated at 37°C and approximately 250 rpm until the optical density, at a wavelength of 600 nm, reached 0.5 to 0.8. Protein expression was then induced with isopropyl β-D-1-thiogalactopyranoside (IPTG) at a final concentration of 400 µM for between three and five hours at 37°C. Bacteria were harvested by centrifugation at 5,000 x g for 15 minutes at 4°C, the supernatant was discarded, and individual cell pellets were weighed before storage at -20°C.
Bacterial cell pellets were thawed at room temperature before 10 mL B-PER™ Complete Bacterial Protein Extraction Reagent and 100 μL 10x Halt™ Protease Inhibitor Cocktail (both Fisher Scientific) were added per gram of cell pellet. Cell pellets were resuspended by vortexing and incubated for 20 minutes at room temperature in a bench-top shaker at 150 rpm.
Solubilisation of bacterial inclusion bodies was performed using a combination of sodium lauroyl sarcosinate (sarkosyl), Triton X-100 and 3-[(3-cholamidopropyl)dimethylammonio]-1-propanesulfonate (CHAPS) detergents similar to previous reports (55, 56). Sarkosyl (Sigma Aldrich, Gillingham, UK) was added to a final concentration of 2% and incubated at room temperature in a bench-top shaker at 150 rpm for at least two hours. This was followed by centrifugation at 20,000 x g for 20 minutes at 4°C and the supernatant was transferred to a new tube and stored. The process was repeated with the insoluble pellet but sarkosyl was added to a final concentration of 10%. The supernatants were combined, and Triton X-100 and CHAPS (both Sigma Aldrich) were added to final concentrations of 4% and 40 mM, respectively.
Purification of the His-tagged Asian elephant IFN proteins was performed using 1 mL tubes of the Thermo Scientific™ HisPur™ Ni-NTA Purification Kit (Fisher Scientific) according to the manufacturer’s protocol. Purified protein preparations were concentrated using Amicon® Ultra-15 10K Centrifugal Filter Devices (Millipore Corporation, Watford, UK) at 4,000 x g for 20 minutes.
Residual imidazole was removed from the soluble protein preparations using Zeba™ Spin Desalting Columns (5 mL size) (Fisher Scientific) according to the manufacturer’s instructions. Final protein preparations in sterile PBS were divided into 50 µL aliquots and stored until further use at -80°C.
Dilutions of these protein preparations were made in sterile PBS and quantified using the Thermo Scientific™ Coomassie Plus (Bradford) Assay Kit (Fisher Scientific) according to the manufacturer’s “microplate procedures” protocol. All standards and samples were measured in triplicate. The absorbance of each sample at 595 nm was measured using an Infinite® M Plex plate reader (Tecan UK Ltd., Reading, UK).
2.6 SDS-PAGE and Western blot
Aliquots of protein preparations were added to 5 µL of 4x Bolt™ Lithium Dodecyl Sulphate (LDS) Sample Buffer, 2 µL of 10x Bolt ™ Reducing Agent and sterile deionised water to a final volume of 20 µL. The samples were heated for 10 minutes at 70°C and loaded onto a Bis-Tris Plus 4-12% gradient polyacrylamide gel in 2-(N-morpholino) ethanesulfonic acid (MES) SDS buffer containing antioxidant (all Fisher Scientific). Invitrogen™ iBright™ Prestained Protein Ladder (11-250 kDa; Fisher Scientific) and BLUeye™ Prestained Protein Ladder (10-245 kDa; Geneflow Ltd., Lichfield, UK) were also loaded onto the gel to monitor protein separation. Alternatively, SeeBlue® Pre-Stained Protein Standard (3-198 kDa; Fisher Scientific) replaced the BLUeye™ Protein Ladder. Electrophoresis was carried out at 200 volts for 25 minutes.
For Coomassie staining, gels were washed in sterile deionised water and immersed in Coomassie Brilliant Blue R-250 staining solution (Bio-Rad) for one hour. The staining solution was replaced with de-staining solution (40% methanol, 10% glacial acetic acid, 50% distilled water) until background staining had been removed and the bands of the protein ladder and/or sample proteins were clearly visible. The gels were visualised using an Azure c280 imaging system (Cambridge Bioscience, Cambridge, UK).
For Western blots, protein bands were transferred to nitrocellulose membranes using the Invitrogen™ iBlot™ 2 Dry blotting system (Fisher Scientific) according to the manufacturer’s instructions. The membrane was then washed in sterile deionised water and immersed in 5 mL of 1x iBind™ solution (Fisher Scientific). Membrane blocking and antibody binding were performed using the iBind™ Western System (Fisher Scientific) according to the manufacturer’s instructions. A primary monoclonal mouse anti-His tag antibody (Sigma Aldrich; Clone 6AT18) was diluted 1:1,000 and the secondary polyclonal rabbit anti-mouse IgG – horseradish peroxidase antibody (Sigma Aldrich) was diluted 1:16,000. After incubations, the membrane was washed twice in 20 mL of sterile distilled water for two minutes and then immersed in 7.5 mL of Thermo Scientific™ SuperSignal™ West Pico PLUS Chemiluminescent Substrate working solution (Fisher Scientific) at room temperature for five minutes. The membrane was placed in a clear plastic wrap and imaged using an Azure c280 imaging system.
2.7 Primary Asian elephant fibroblast cell culture
Primary Asian elephant preputial fibroblast (EF) cells were cultured in a 75 cm2 cell culture flask containing EF medium consisting of: Gibco™ Minimum essential medium (Fisher Scientific), 10% FBS, Penicillin-Streptomycin (10,000 units penicillin/mL, 10,000 µg streptomycin/mL) and Nystatin (10,000 units/mL; Fisher Scientific). Upon reaching 75 to 80% confluency, cells were passaged or seeded into 24-well plates at 50% confluency per well.
2.8 Herpesviral infection of elephant fibroblasts
As attempts to culture EEHVs have so far been unsuccessful (57–59), infections of primary Asian elephant fibroblasts were trialled with two other herpesviruses, to ascertain whether either was capable of inducing a measurable cytopathic effect (CPE) in this cell type. Serial tenfold dilutions (10-1 to 10-8) were made in Dulbecco’s Modified Eagle Medium (DMEM) of BoHV-1 Oxford strain with a titre of 107.5 PfU/mL and Equine alphaherpesvirus 1 (EHV-1) strain V592. These viruses were chosen in part for their availability during this proof-of-concept study, but also based on practical considerations during infection assays, compared to the phylogenetically more closely related human cytomegalovirus (HCMV) or human herpesvirus 6 (HHV-6). A 100 µL aliquot of each virus preparation or 100 µL of DMEM for no-virus-controls was added to each well. Plates were sealed and incubated at 37°C and 5% CO2 for one hour. Each well was subsequently washed three times with 1.5 mL DMEM medium before 1.5 mL EF medium was applied, the plate was re-sealed, and incubated for up to seven days.
EF cells were fixed with neutral buffered 10% formalin (Sigma Aldrich) for at least 40 minutes. Formalin was then replaced with 250 µL of 2.3% Crystal Violet solution (Sigma Aldrich). Plates were incubated at room temperature for five minutes, washed and air-dried. Assessment of cell monolayers was performed using a Leica DM IL LED microscope (Leica Microsystems UK Ltd., Milton Keynes, UK). Over the course of the study, BoHV-1 infection of Asian elephant fibroblasts (without the addition of IFNs) was investigated in five experimental replicates.
2.9 Effect of IFN on herpesviral infections of elephant fibroblasts
Initial investigations of the bioactivity of human and Asian elephant IFNs were carried out using 1:100 dilutions of unquantified Asian elephant recombinant IFNα1 and β preparations (rEleIFNα1, rEleIFNβ) alongside a control preparation from the insert-less pET303CT-His plasmid vector. Similarly, dilutions were made of recombinant human interferon alpha 2a (hIFNα2a) and human interferon beta 1a (hIFNβ1a; both Immunotools, Friesoythe, Germany) to produce preparations of 1,000 international units (IU)/mL. All preparations were produced in DMEM medium.
In three experimental replicates, EF medium was removed from each well and 1 mL aliquots of unquantified IFN solutions were added 8 hours prior to infection with BoHV-1.
For a subsequent investigation of the time point at which IFNs no longer protect EF cells from BoHV-1 infection, serial 1:10 dilutions were made of quantified rEleIFNα1, rEleIFNβ, hIFNα2a, and recombinant human interferon beta 1b (hIFNβ1b; Miltenyi Biotec., Bisley, UK). All dilutions were produced in DMEM medium and were made with equal masses of rEleIFNs and the corresponding human protein (IFNα: 500 ng/mL - 5 pg/mL; IFNβ: 1.67 µg/mL and 333.33 ng/mL - 30 pg/mL). Based on IU/ng data available for the hIFN preparations, these dilutions equated to 100,000-1 IU/ml. In two experimental replicates, EF cells were infected with BoHV-1 and at 0-, 8-, 12-, 16-, 24-, 48- and 72-hours post-infection (hpi), the EF medium was removed and replaced with 1 mL of the respective IFN solutions. Incubation in IFNs was continued for 24 hours before preparations were replaced once more with 1.5 mL EF medium. Plates were fixed, stained and assessed as described above.
3 Results
3.1 Identification of Asian elephant IFN genes and proteins
Using predicted sequences from the African elephant and published sequences from the horse as templates, 13 distinct intronless IFNα-like sequences and a single IFNβ-like sequence were retrieved from the Asian elephant sequence assemblies available at the time. PCR-based amplification and sequencing identified four putatively functional Asian elephant IFNα genes, annotated in order of identification (EleIFNα1, IFNα2, IFNα3, IFNα4; GenBank accession numbers OR450024, OR450025, OR450026, OR450027). The EleIFNα1, IFNα2, IFNα3 and IFNα4 genes were each 591 base pairs in length and translated to proteins of 196 AA with predicted molecular weights of approximately 22.5 kDa. Upon alignment, the IFNα were 94.4-97.5% identical at the AA level. The fifth IFNα gene amplified was 600 base pairs in length, with a premature stop codon at position 107 of the AA sequence, demonstrating the identification of an Asian elephant IFNα pseudogene (GenBank accession number OR450028; EleIFNαPG; Figure 1). The 564-bp EleIFNβ gene identified encoded a putatively functional protein of 187 AA with predicted molecular weight of 21.6 kDa (GenBank accession number OR450029).
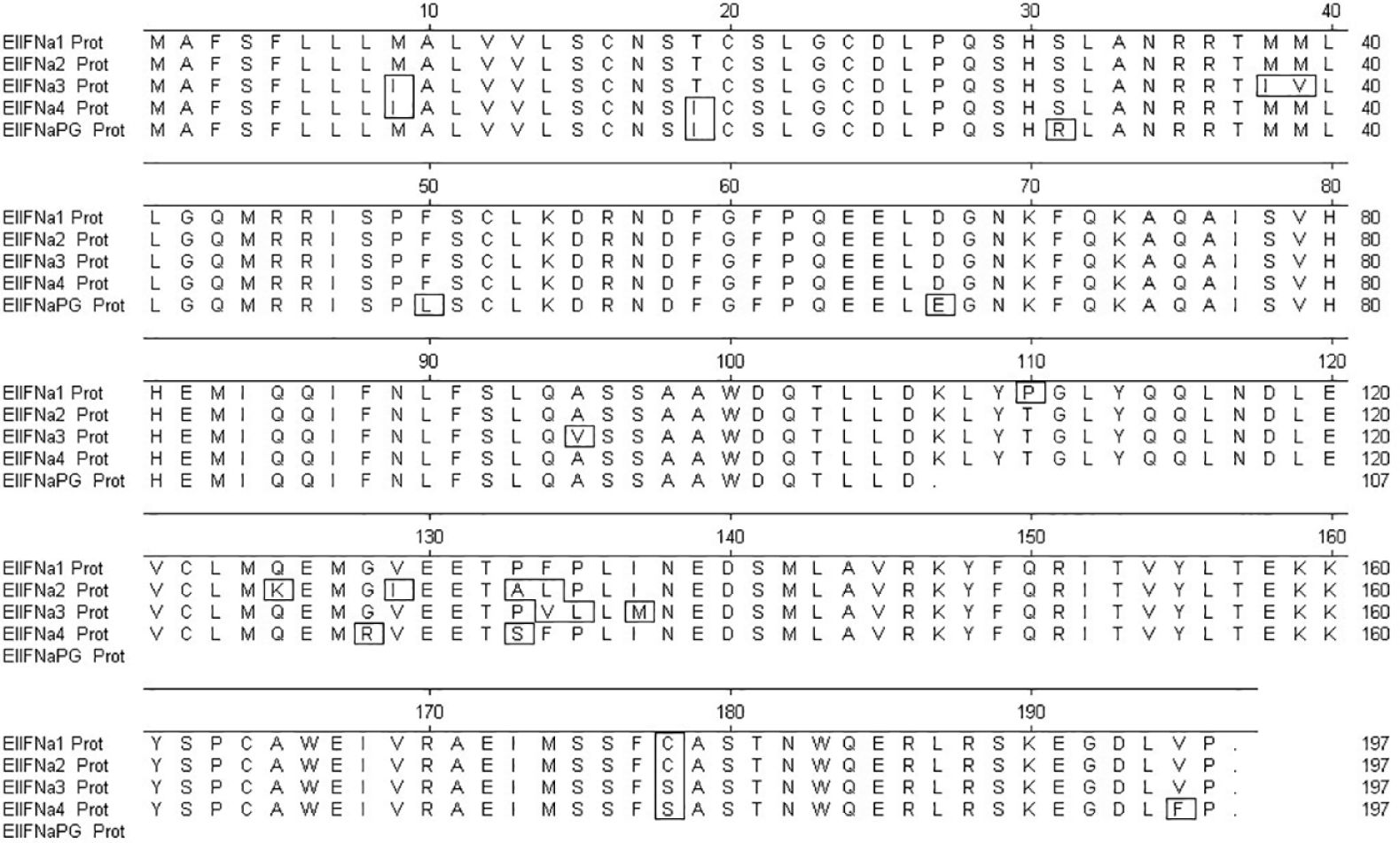
Figure 1. Multiple alignment of the amplified Asian elephant IFNα amino acid (AA) sequences using MegAlign software. The first 23 AA at the N-terminus of each protein are the predicted signal sequences. The stop codon within the nucleotide sequence of the IFNα pseudogene (IFNαPG) is at position 107. Boxed AAs are those that differ from the consensus.
Using NCBI BLAST, the coding regions of the Asian elephant IFNα sequences had the highest homology with that of the African elephant with 91-99% and 86-98% identity at the NT and AA levels, respectively. Of similar immunogenetic relation were the Florida manatee (Trichechus manatus latirostris) sequences with 86-94% NT and 82-90% AA identity, whilst other mammalian species, including humans, were of significantly lower homology (53-78% identity at the AA level). Although the Asian and African elephant IFNβ AA sequences were 98.9% identical, the identity level dropped to 60% for human IFNβ (Table 2).
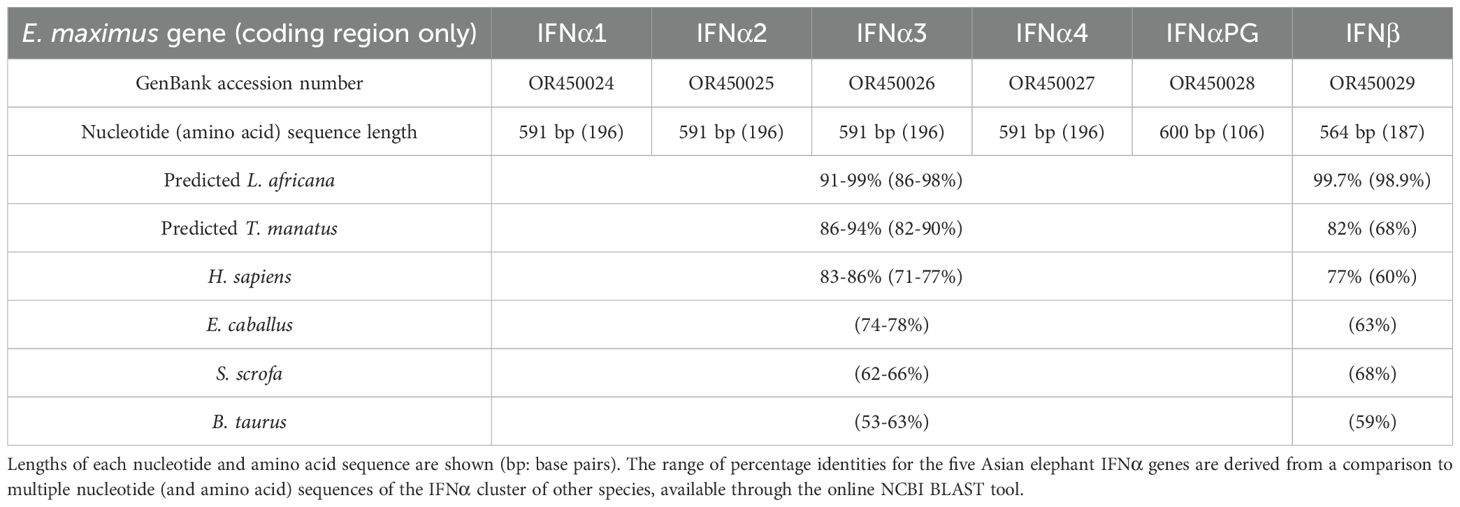
Table 2. Asian elephant IFN sequences obtained in this study and their percentage homology with selected mammalian species.
3.2 Expression cloning of recombinant Asian elephant IFN
rEleIFNα1 and rEleIFNβ were successfully expressed, demonstrated by the presence of strong protein bands corresponding to the predicted sizes of 20.1 kDa and 19.2 kDa (Figure 2). Although co-purified contaminants were present, rEleIFNα1 and rEleIFNβ represented the substantial majority of proteins in each preparation, judged by the visible density of the corresponding protein bands in the Coomassie stained gels. Western blot analysis similarly confirmed expression of His-tagged rEleIFNα1 and rEleIFNβ. Whilst only a single protein band was visualised for rEleIFNβ, additional bands were observed in the rEleIFNα1 preparations, but at approximately double and triple the predicted molecular weight of the monomeric protein, possibly representing oligomerization. No chemiluminescent signal was recorded for control preparations.
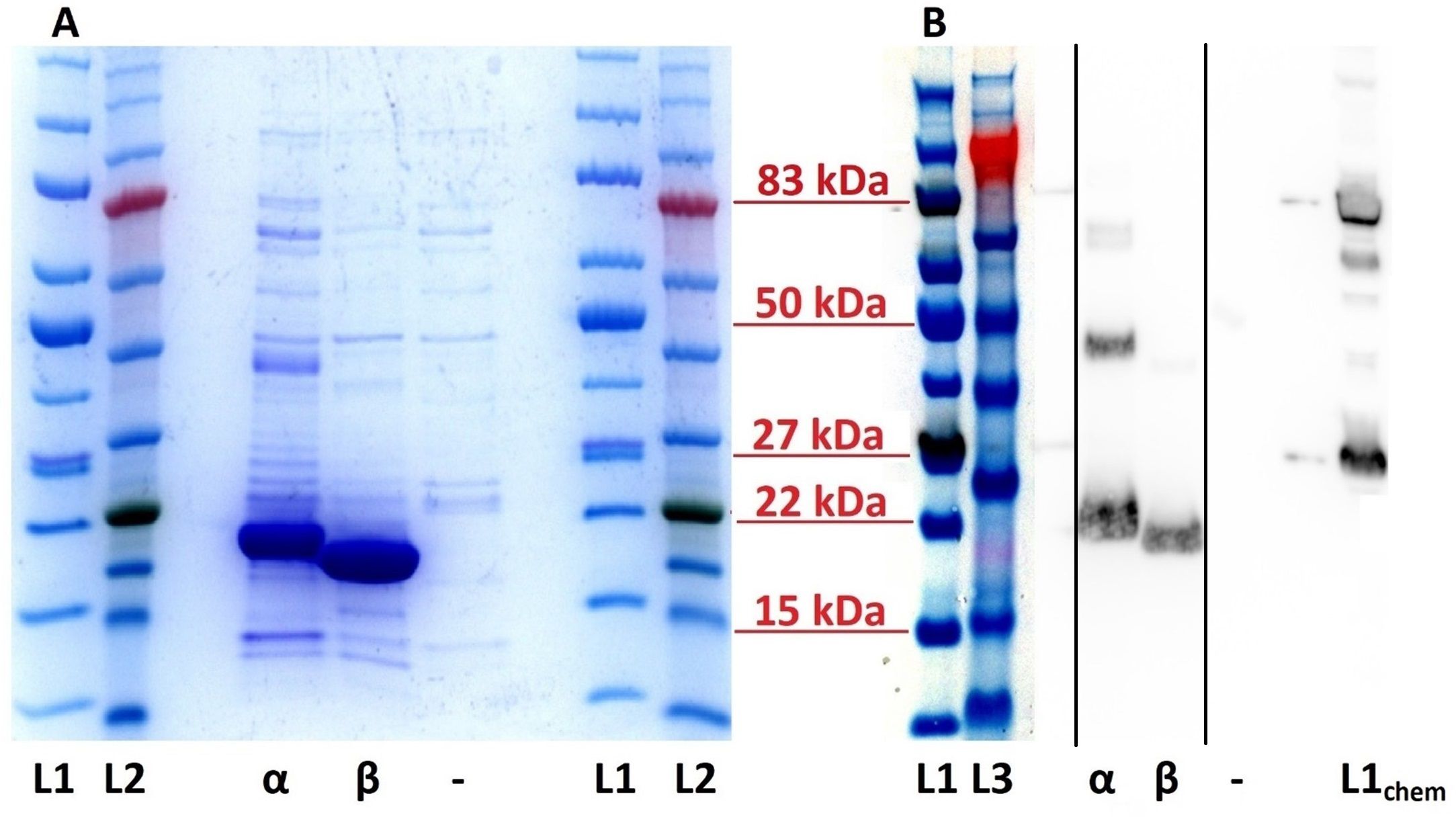
Figure 2. SDS-PAGE (A) and Western blot (B) of purified recombinant Asian elephant IFN proteins. (A) Bands of approximately 20 kDa and 19 kDa represent the rEleIFNα1 (α) and rEleIFNβ (β) proteins in the respective lanes. No significant corresponding protein expression could be seen in the control (–) preparations (pET303CT-His plasmid vector without IFN gene insert). (B) Using a monoclonal mouse anti-His tag antibody, positive chemiluminescent signal was observed for both rEleIFNα1 (α) and rEleIFNβ (β) at the expected respective sizes but not for control (–) preparations. Protein ladders are present on either side of each gel with relevant molecular weights indicated in red. L1: iBright™ Prestained Protein Ladder, L2: BLUeye™ Prestained Protein Ladder, L3: SeeBlue™ Pre-stained Protein Standard (all under visible light). L1chem: iBright™ Prestained Protein Ladder (under chemiluminescence). This figure is a combination of two separate gels, the original images of which have been submitted as Supplementary Material. Solid black lines in panel (B) indicate where sections of the original blot image have been spliced.
3.3 Bovine herpesvirus infection model of EF cells
A surrogate system was required to investigate the antiviral activity of recombinant IFNs as no in-vitro EEHV infection model has yet been established (57–59). To do so, monolayers of EF cells were inoculated with either BoHV-1 or EHV-1 and the cells were fixed and stained 7 dpi to visualise CPE. Monolayers of EF cells were consistently and effectively destroyed by a 10-3 dilution of BoHV-1, but no CPE was observed in cells inoculated with a 10-1 dilution of EHV-1 (data not shown). To determine the kinetics of BoHV-1-in EF cells, quadruplicate wells were fixed and stained at 24-hour intervals from 2 to 7 dpi (Figure 3). Viral plaques were consistently visible at 3 dpi and destruction of the EF cell monolayer was evident within five days. Accordingly, a 10-3 dilution of BoHV-1 was used in all subsequent experiments to determine the antiviral bioactivity of IFNs in this study.
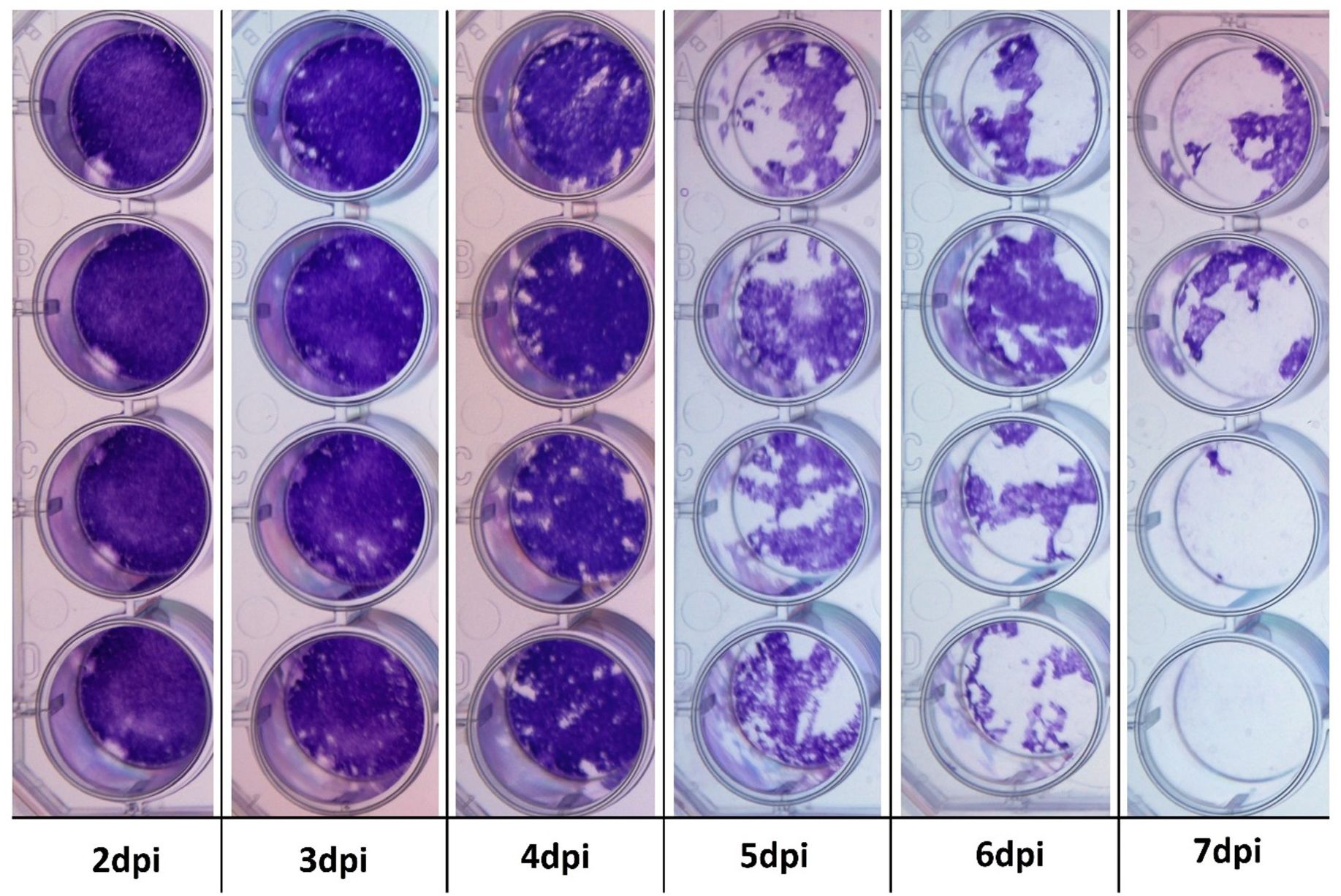
Figure 3. Cytopathogenic effect in BoHV-1-infected primary Asian elephant fibroblasts. Quadruplicate wells were infected with BoHV-1 and subsequently fixed and stained to evaluate for any cytopathogenic effects from 2 to 7 dpi. Viral plaques are evident at 3 dpi and monolayer integrity is lost beyond 5 dpi.
3.4 Bioactivity of human and Asian elephant IFNs in EF cells
Bioactivity of recombinant human and Asian elephant IFNs was initially demonstrated in an experiment using 1,000 IU/mL of rhIFNα2a or rhIFNβ1a or 1:100 dilutions of unquantified preparations of rEleIFNα1 or rEleIFNβ. EF cells were incubated with the IFNs for 8 hours prior to infection with BoHV-1. rEleIFNα1 and rEleIFNβ both protected cells from BoHV-1 induced CPE, whereas monolayers in control preparations were destroyed (Figure 4). Monolayers also remained intact following pre-exposure to 1,000 IU of hIFNα2a but were destroyed in wells pre-exposed to 1,000 IU of hIFNβ1a. No CPE was evident in uninfected cells. Considering the outcome of this experiment, the hIFNβ supplier was changed.
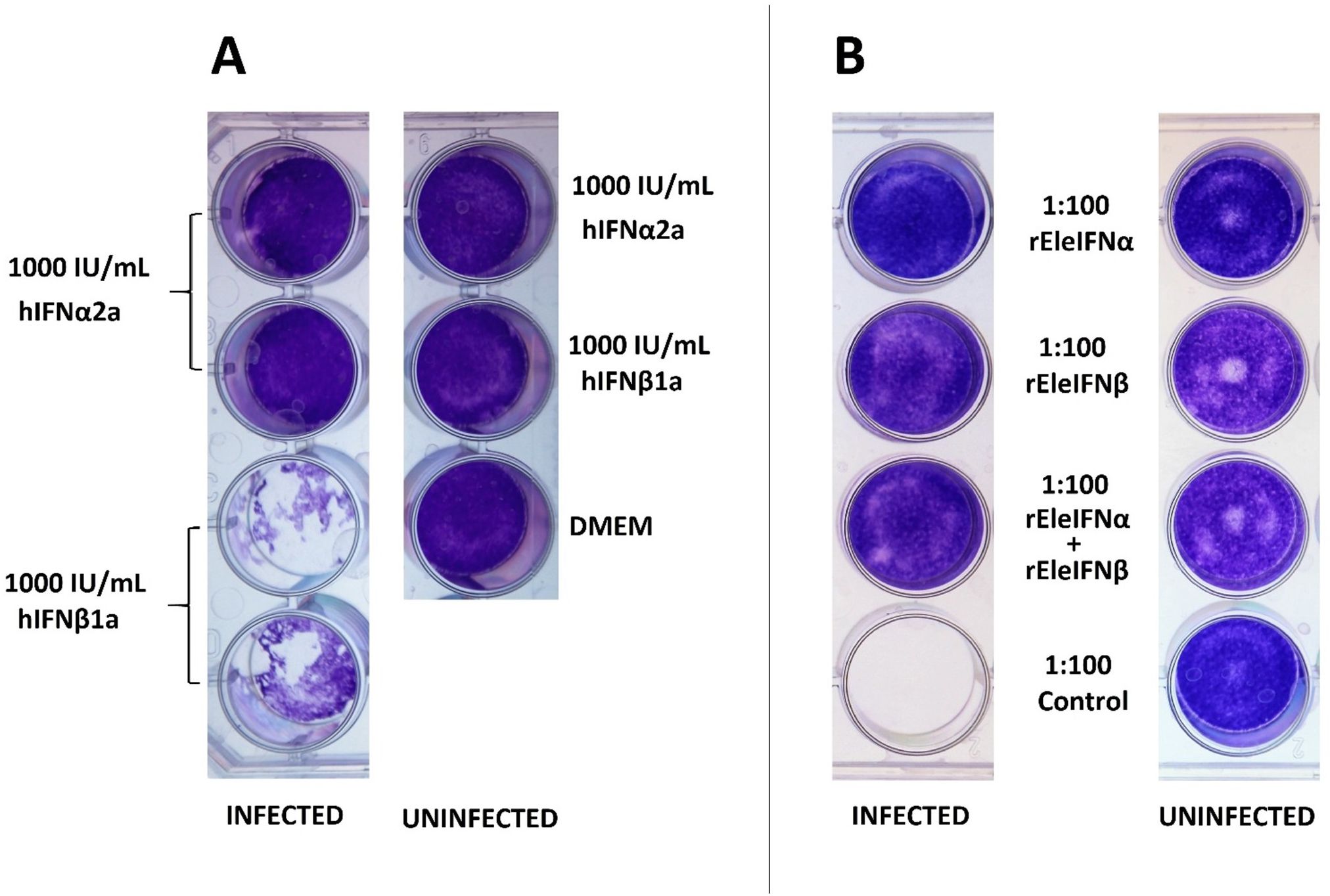
Figure 4. Anti-viral activity of recombinant human (A) and Asian elephant (B) IFNs in primary Asian elephant fibroblasts. EF cells were incubated in 1 mL dilutions of hIFNs or rEleIFNs for 8 hours prior to infection with BoHV-1 and cell monolayers were assessed 7 dpi. At the dilutions investigated, no protein preparations were cytotoxic in themselves and hIFNα2a, rEleIFNα1 and rEleIFNβ offered full protection from CPE. No protection was provided by hIFNβ1a or control preparations (protein preparations produced from pET303CT-His plasmid vectors without IFN gene insert).
IFNs require a certain timeframe to exert their antiviral effects, and for rapidly growing viruses, pre-incubation of cells with IFN is essential for anti-viral protection. A further experiment was conducted to ascertain the timepoint at which IFN no longer protects EF cell monolayers and to compare relative bioactivities of recombinant human and Asian elephant IFNs. To do so, serial dilutions of IFNα (500 ng/mL - 5 pg/mL) and IFNβ (1.67 µg/mL and 333.33 ng/mL - 30 pg/mL) were applied at 0, 8, 12, 16, 24, 48 and 72 hpi. Based on data available for the rhIFN preparations, the dilutions tested ranged between 100,000 IU to 1 IU of IFN. Pre-exposure to at least 1,000 IU (5 ng) of hIFNα2a provided EF cells with full protection from BoHV-1 infection when administered up to 48 hpi (Figure 5). In contrast, 500 ng of rEleIFNα1 was required to consistently prevent CPE and protection was achievable only when applied up to 24 hpi. Cell monolayers were protected from BoHV-1 infection with only 33.3 ng of rEleIFNβ but the protective effect was also largely lost if applied beyond 24 hpi. No significant protective effect was observed using hIFNβ1b, indicating a difference in cross-species reactivity between human IFN alpha and beta. No protection from BoHV-1 infection was observed when IFNs were applied beyond 48 hpi.
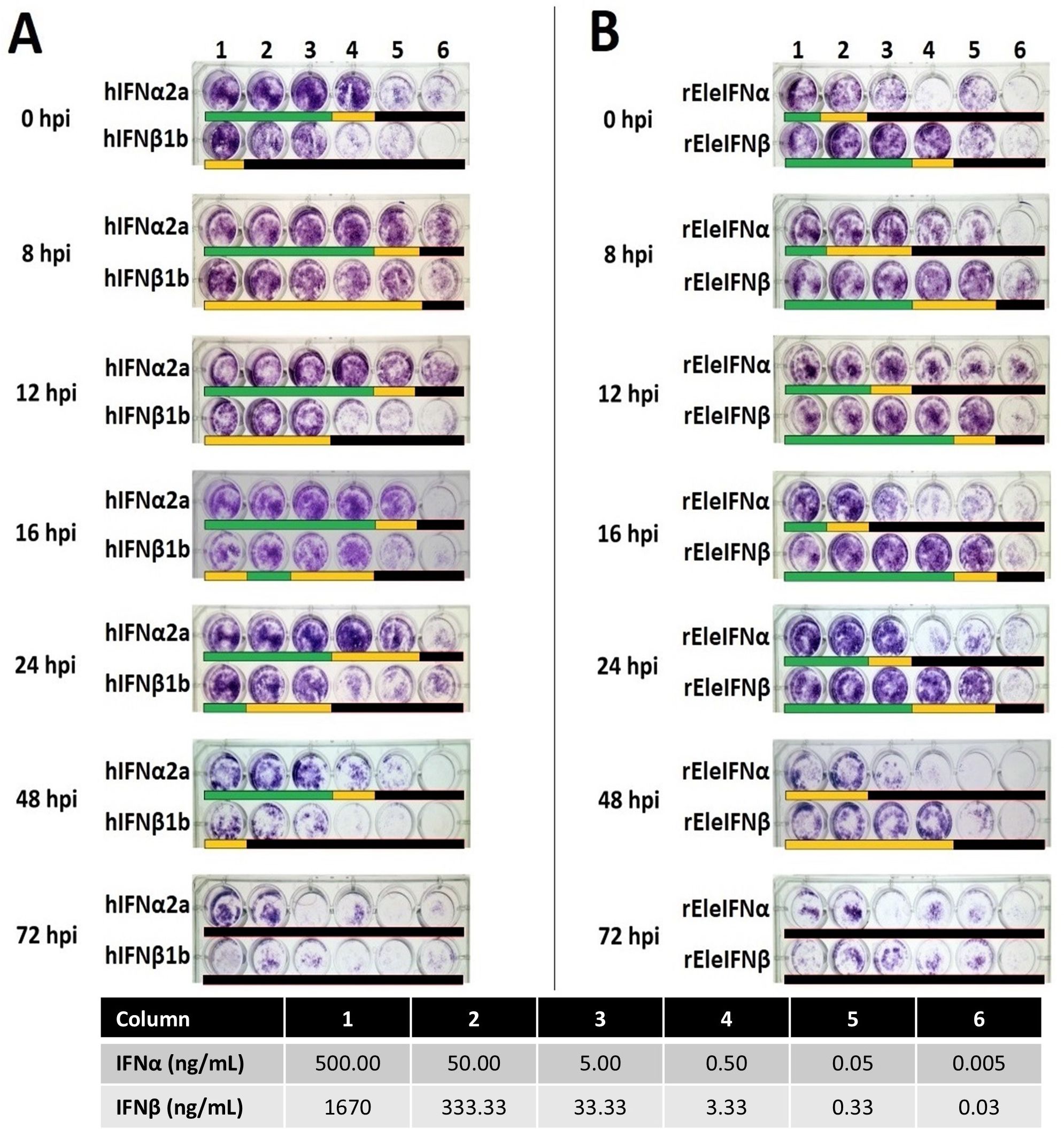
Figure 5. Kinetics of recombinant human (A) and Asian elephant (B) IFN bioactivities. EF cells infected with BoHV-1 were incubated in six dilutions of IFNs (Columns 1-6) for 24 hours at seven timepoints (0-72h) post-infection. Cell monolayers were assessed microscopically at 7 dpi. Beneath each well, a lack of CPE is denoted by a green bar, occasional (<5) viral plaques by a yellow bar and monolayer destruction by a black bar. Antiviral activity was observed with the application of hIFNα up to 48 hpi, with a minimum effective dose of 5 ng providing full protection from BoHV-1 infection. Up to 24 hpi, 33.3 ng of rEleIFNβ demonstrated potent antiviral activity. No antiviral activity was recorded for any IFN when applied beyond 48 hpi. Poor growth of EF cells was differentiated from CPE upon microscopic examination. Infected control wells (n=1 on every plate; not shown) demonstrated significant changes in morphology prior to death (e.g. shrinkage and clumping) whereas all uninfected control wells (n=1 on every plate; not shown) contained a population of healthy fibroblasts.
4 Discussion
Here we identified five Asian elephant IFNα genes alongside a single IFNβ gene. Unsurprisingly, the Asian elephant gene sequences had the highest identity to those predicted from their closest relatives, the African elephant (Loxodonta africana) and Florida manatee (Trichechus manatus latirostris). Sequence diversity within the Asian elephant IFNα genes resembled those reported previously for other species, including humans (60–63). The repertoire of IFNα subtypes is most likely attributed to gene duplication events that have occurred throughout the evolution of vertebrate species, highlighting the vital role of these cytokines for their survival (1, 64). Gene duplication events may mitigate against replication errors but can also lead to the creation of pseudogenes, as identified here, and previously in both human and mouse IFN loci (11, 61, 65, 66).
Expression of recombinant proteins employed in this study was similar to previously published protocols (67–72). However, the lengthy dialysis in ever-decreasing concentrations of urea and guanidine hydrochloride was replaced by solubilisation of inclusion bodies with sarkosyl, Triton X-100 and CHAPS, as a more efficient method of recovering recombinant proteins (55, 56, 73). In the current study, a final concentration of 10% sarkosyl was necessary to obtain a sufficient amount of both His-tagged rEleIFNs that could be purified directly from the resulting solutions. Interestingly, both SDS-PAGE and Western blot analyses suggested the presence of homodimers of rEleIFNα1 and to a lesser extent, rEleIFNβ. Such a phenomenon has been documented for human IFNs, with as yet unknown biological consequences (74–77).
Subtypes of IFNα are known to possess distinct biological potencies (78–80), but here only EleIFNα1 was chosen for expression and purification as a proof-of-concept endeavour. As binding affinities of all subtypes of the human IFNα family have been demonstrated to be relatively comparable (81, 82), the expression of further rEleIFNα subtypes was not deemed a priority.
As IFNα family proteins are known to cross-react between animal species (83–86), it was considered prudent to compare bioactivity of the rEleIFNs to human IFN proteins in EF cells. Additionally, given the impact of EEHVs in this species, it was decided that an infection model using a herpesvirus was most pertinent to evaluate the antiviral bioactivity of the IFNs, rather than using the standard Vesicular Stomatitis Virus (VSV) model (87–89). The selection of BoHV-1 as a surrogate for EEHV was primarily due to the ongoing inability to culture the latter in-vitro (57–59). BoHV-1 and EHV-1 are known to induce a lytic infection in a variety of cell lines from different species (90–95). However, only BoHV-1 demonstrated a visible CPE in EF cells. Given that earlier studies have demonstrated a seroconversion of Asian elephants against BoHV-1 (96, 97) this result may not be as surprising as it initially appears. However, further work is needed to explore the lack of replication observed in relation to EHV-1.
Both recombinant Asian elephant IFNα and IFNβ proteins demonstrated significant antiviral activity against BoHV-1 infection of EF cells, when applied eight hours prior, and up to 24 hours post infection. The latter is perhaps not overly surprising, given that the development of a CPE was evident in untreated EF cells only after 72 hours.
As human IFNs are known to exert immune effects beyond the native species (50, 98–101), the bioactivity of human IFNα2a on EF cells was anticipated. However, the observation that human IFNβ had minimal (hIFNβ1b) or no (hIFNβ1a) bioactivity was surprising. This may be explained by the relatively distinct AA sequences of human IFNα and IFNβ, despite exerting their effects via the same receptor. Additionally, human and Asian elephant IFNα genes shared 71-77% sequence identity, whilst sequence identity for IFNβ gene was 60% (Table 2).
Interestingly, the protective effect of IFNs continued for the entire seven-day BoHV-1 study period, even after removal of IFNs, suggesting that IFN promoted a “head start immunity”, as proposed recently (102). Therefore, interferon signalling and ISG induction had occurred by, or continued beyond, the point of infection. The longevity of this effect is likely to be explained by the ongoing presence of the original virus inoculum and virus progeny, providing sustained interaction between the pathogen recognition receptors (PRRs) within the EF cells and the viral pathogen-associated molecular patterns (PAMPs). Indeed, recent work has demonstrated the expression of multiple anti-viral mediator genes, including IFNs, in Asian elephant white blood cells in response to the in-vitro administration of authorised PAMP-based veterinary medicinal products (103). A similar innate immune response, and the expression of innate immune mediators, is expected following exposure of EF cells to BoHV-1, but more crucially, following the exogenous administration of IFNs.
Recombinant IFNs displayed a positive correlation between the administered dose and the level of protection against BoHV-1. These preliminary dose-dependent responses were largely unaffected whether the IFNs were applied at the point of infection (0 hpi) or over the following 24 hours (8-24 hpi). The observed lack of protection at 48 hpi indicates that IFN signalling pathways need to have activated ISGs and anti-viral mediators before this point, whereafter certain viral proteins are expected to drive the immune-evasion of the IFN system (7, 104–107).
Since this study was conducted, a draft Asian elephant genome has been published (GenBank Bioproject PRJNA861314; assembly GCA_024166365.1, July 2022). However, although this assembly is not yet complete, it does contain some noteworthy information in relation to this study. Whilst the IFN locus cannot be described at this stage, all predicted IFN-I genes are located in chromosome 9 (of 27 autosomes). Of the 11 predicted IFNα genes, two are annotated as pseudogenes, with one having the pre-mature stop codon described here. Of the nine predicted functional IFNα genes, eight are annotated as IFNalpha-5-like, and one (XP_049750889.1) as IFNalpha-6-like. Importantly, the latter sequence has low identity score (<20%) and is shorter (182 AA) than all other predicted IFNα proteins, questioning the accuracy of its annotation. Whilst our annotation of IFNα1-4 is based on the chronological order of discovery, it is meaningful to highlight the fact that they are considered to represent four unique bioactive IFNα proteins. Notably, only the IFNα3, IFNαPG and IFNβ sequences described here are 99.5-100% identical to the draft genome, with a single mutation in IFNα3 likely representing a single nucleotide polymorphism. However, IFNα1, IFNα2 and IFNα4 are only 95-96% identical to the nearest match from the draft genome. It is unlikely that such differences are due to inter-animal variability and instead these IFNα sequences may represent duplications of IFNα not yet annotated in the draft genome, demonstrating the challenges associated with annotating genome areas with gene duplications. Additionally, since the current number of identified IFNα genes (eight or nine functional genes) is considerably lower than those in mammalian livestock species, humans, or mice, it is reasonable to assume that the IFNα1, IFNα2 and IFNα4 sequences identified in this study may represent additional genes to be added to the Asian elephant genome annotation.
Regarding the relative bioactivity of the two rEleIFNs, rEleIFNβ consistently provided the same level of protection as 15 times the same mass of rEleIFNα1. Although based on the previously mentioned assumption of an equal concentration of bioactive protein across the two preparations, this suggests that a similar hierarchy of affinity for the type I IFN receptor exists in Asian elephants as seen in humans and mice, where IFNβ demonstrates approximately 20 times greater affinity for the type I IFN receptor than IFNα (78, 79, 82). This would appear to make rEleIFNβ a preferred choice for clinical application. However, the preliminary demonstration of bioactivity of recombinant human IFNα in Asian elephant fibroblasts is an important consideration in relation to possible in-vivo applications, since pharmaceutical grade products, authorised for use in humans, are already available for veterinary prescription and administration.
The successful cloning and characterisation of rEleIFNs in this study, in combination with the development of a herpesviral infection model, provides an important preliminary means of demonstrating antiviral activity in Asian elephants. Work adding resolution to the Asian elephant genome (108) will aid in the description of the IFN-I cluster genome locus. Future work can accordingly document the remaining IFN genes, providing the basis to study their respective functions further, as documented in humans (78–80).
This study was designed as a preliminary investigation into whether the clinical application of rIFNs could contribute to the control of EEHV-HD, thereby maintaining the welfare of the captive population of Asian elephants worldwide, and aiding efforts for their conservation. In a recent case report, the clinical application of rhIFNα, along with a DNA immunostimulant, were considered to significantly contribute to controlling EEHV1A-HD in a juvenile Asian elephant, despite an exponential rise in viraemia (38). Importantly, the calf survived with no adverse effects. The same treatment protocol, but excluding IFNs or veterinary immunostimulants, had proven unsuccessful in a separate case of EEHV1A-HD at the same zoological collection less than 6 months earlier, highlighting the potential contribution of such biological products to disease control.
Data availability statement
The datasets presented in this study can be found in online repositories. The names of the repository/repositories and accession number(s) can be found in the article/Supplementary Material.
Ethics statement
The animal studies were approved by the APHA Animal Welfare Ethical Review Body. The studies were conducted in accordance with the local legislation and institutional requirements. Written informed consent was obtained from the owners for the participation of their animals in this study.
Author contributions
JH: Conceptualization, Data curation, Formal Analysis, Investigation, Methodology, Project administration, Writing – original draft, Writing – review & editing. TM: Methodology, Supervision, Writing – review & editing. AD: Conceptualization, Funding acquisition, Methodology, Project administration, Resources, Supervision, Writing – review & editing. FS: Conceptualization, Funding acquisition, Methodology, Project administration, Resources, Supervision, Visualization, Writing – review & editing.
Funding
The author(s) declare that financial support was received for the research and/or publication of this article. Funding for this work was provided by the Zoological Society of London, Chester Zoo and Rotterdam Zoo.
Acknowledgments
The authors would like to thank veterinary and zookeeping staff at Zoological Society London Whipsnade Zoo for providing blood samples and Professor Julian Chantrey and Dr. Ben McDermott at the University of Liverpool for kindly donating the primary Asian elephant fibroblast cells. The authors are grateful to Hannah Davies at the APHA for help with sample archiving and extraction. JH was partly supported through the Chester Zoo Conservation and Science Scholar Programme. The content of this manuscript is also presented online, in the thesis of JH (109).
Conflict of interest
The authors declare that the research was conducted in the absence of any commercial or financial relationships that could be construed as a potential conflict of interest.
The author(s) declared that they were an editorial board member of Frontiers, at the time of submission. This had no impact on the peer review process and the final decision.
Generative AI statement
The author(s) declare that no Generative AI was used in the creation of this manuscript.
Publisher’s note
All claims expressed in this article are solely those of the authors and do not necessarily represent those of their affiliated organizations, or those of the publisher, the editors and the reviewers. Any product that may be evaluated in this article, or claim that may be made by its manufacturer, is not guaranteed or endorsed by the publisher.
Supplementary material
The Supplementary Material for this article can be found online at: https://www.frontiersin.org/articles/10.3389/fimmu.2025.1533038/full#supplementary-material
Supplementary Figure 1 | A full scan of the entire original SDS-PAGE gel, shown in Figure 2A, of purified recombinant Asian elephant IFN proteins. Bands of approximately 20 kDa and 19 kDa represent the rEleIFNα (lane 4) and rEleIFNβ (lane 5) proteins in the respective lanes. No significant corresponding protein expression could be seen in the control preparations (pET303CT-His plasmid vector without IFN gene insert; lane 6). Protein ladders are present on either side of the gel: iBright™ Prestained Protein Ladder (lanes 1 and 8), BLUeye™ Prestained Protein Ladder (lanes 2 and 9).
Supplementary Figure 2 | An uncropped and unedited visible light image of the entire original Western blot gel shown in Figure 2B. Protein ladders are present on either side of the gel: iBright™ Prestained Protein Ladder (lanes 1 and 11), SeeBlue™ Pre-stained Protein Standard (lanes 2 and 12).
Supplementary Figure 3 | An uncropped and unedited chemiluminescence image of the entire original Western blot gel shown in Figure 2B. Using a monoclonal mouse anti-His tag antibody, positive chemiluminescent signal was observed for both rEleIFNα (lanes 4 and 5) and rEleIFNβ (lanes 6 and 7) at the expected respective sizes but not for control preparations (lanes 8 and 9). Protein ladders are visible on either side of the gel: iBright™ Prestained Protein Ladder (lanes 1 and 11).
References
1. Secombes CJ, Zou J. Evolution of interferons and interferon receptors. Front Immunol. (2017) 8:2–11. doi: 10.3389/fimmu.2017.00209
2. Schultz U, Kaspers B, Staeheli P. The interferon system of non-mammalian vertebrates. Dev Comp Immunol. (2004) 28:499–508. doi: 10.1016/j.dci.2003.09.009
3. Wilson V, Jeffreys AJ, Barrie PA, Boseley PG, Slocombe PM, Easton A, et al. A comparison of vertebrate interferon gene families detected by hybridization with human interferon DNA. J Mol Biol. (1983) 166:457–75. doi: 10.1016/S0022-2836(83)80281-2
4. Merigan TC, Winget CA, Dixon CB. Purification and characterization of vertebrate interferons. J Mol Biol. (1965) 13:679–91. doi: 10.1016/S0022-2836(65)80135-8
5. Redmond AK, Zou J, Secombes CJ, MacQueen DJ, Dooley H. Discovery of all three types in cartilaginous fishes enables phylogenetic resolution of the origins and evolution of interferons. Front Immunol. (2019) 10. doi: 10.3389/fimmu.2019.01558
6. Goodbourn S, Didcock L, Randall RE. Interferons: Cell signalling, immune modulation, antiviral responses and virus countermeasures. J Gen Virol. (2000) 81:2341–64. doi: 10.1099/0022-1317-81-10-2341
7. Randall RE, Goodbourn S. Interferons and viruses: An interplay between induction, signalling, antiviral responses and virus countermeasures. J Gen Virol. (2008) 89:1–47. doi: 10.1099/vir.0.83391-0
8. Iwasaki A, Medzhitov R. Control of adaptive immunity by the innate immune system. Nat Immunol. (2015) 16:343–53. doi: 10.1038/ni.3123
9. Hoebe K, Janssen E, Beutler B. The interface between innate and adaptive immunity. Nat Immunol. (2004) 5:971–4. doi: 10.1038/ni1004-971
10. Le Bon A, Tough DF. Links between innate and adaptive immunity via type I interferon. Curr Opin Immunol. (2002) 14:432–6. doi: 10.1016/S0952-7915(02)00354-0
11. Pestka S, Krause CD, Walter MR. Interferons, interferon-like cytokines, and their receptors. Immunol Rev. (2004) 202:8–32. doi: 10.1111/j.0105-2896.2004.00204.x
12. Adolf G, Traxler E, Maurer-Fogy I. Recombinant equine interferon-beta1: purification and preliminary characterisation. J Interferon Res. (1990) 10:255–67. doi: 10.1089/jir.1990.10.255
13. Der SD, Zhou A, Williams BRG, Silverman RH. Identification of genes differentially regulated by interferon α, β, or γ using oligonucleotide arrays. Proc Natl Acad Sci U S A. (1998) 95:15623–8. doi: 10.1073/pnas.95.26.15623
14. Platanias LC. Mechanisms of type-I- and type-II-interferon-mediated signalling. Nat Rev Immunol. (2005) 5:375–86. doi: 10.1038/nri1604
15. Kroczynska B, Mehrotra S, Arslan AD, Kaur S, Platanias LC. Regulation of interferon-dependent mRNA translation of target genes. J Interf Cytokine Res. (2014) 34:289–96. doi: 10.1089/jir.2013.0148
16. Lee AJ, Ashkar AA. The dual nature of type I and type II interferons. Front Immunol. (2018) 9:1–10. doi: 10.3389/fimmu.2018.02061
17. Al-Khatib K, Williams BRG, Silverman RH, Halford W, Carr DJJ. The murine double-stranded RNA-dependent protein kinase PKR and the murine 2′,5′-oligoadenylate synthetase-dependent RNase L are required for IFN-β-mediated resistance against herpes simplex virus type 1 in primary trigeminal ganglion culture. Virology. (2003) 313:126–35. doi: 10.1016/S0042-6822(03)00298-8
18. Lenschow DJ, Lai C, Frias-Staheli N, Giannakopoulos NV, Lutz A, Wolff T, et al. IFN-stimulated gene 15 functions as a critical antiviral molecule against influenza, herpes, and Sindbis viruses. PNAS. (2007) 104:1371–6. doi: 10.1073/pnas.0607038104
19. Ehlers B, Burkhardt S, Goltz M, Bergmann V, Ochs A, Weiler H, et al. Genetic and ultrastructural characterization of a European isolate of the fatal endotheliotropic elephant herpesvirus. J Gen Virol. (2001) 82:475–82. doi: 10.1099/0022-1317-82-3-475
20. Davison AJ, Eberle R, Ehlers B, Hayward GS, McGeoch DJ, Minson AC, et al. The order herpesvirales. Arch Virol. (2009) 154:171–7. doi: 10.1007/s00705-008-0278-4
21. Long SY, Latimer EM, Hayward GS. Review of elephant endotheliotropic herpesviruses and acute hemorrhagic disease. ILAR J. (2015) 56:283–96. doi: 10.1093/ilar/ilv041
22. Pavulraj S, Eschke K, Prahl A, Flügger M, Trimpert J, Van Den Doel PB, et al. Fatal elephant endotheliotropic herpesvirus infection of two young asian elephants. Microorganisms. (2019) 7:1–17. doi: 10.3390/microorganisms7100396
23. Oo ZM, Aung YH, Aung TT, San N, Tun ZM, Hayward GS, et al. Elephant endotheliotropic herpesvirus hemorrhagic disease in Asian elephant calves in logging camps, Myanmar. Emerg Infect Dis. (2020) 26:63–9. doi: 10.3201/eid2601.190159
24. Mahato G, Sarma KK, Pathak DC, Barman NN, Gogoi P, Dutta M, et al. Endotheliotropic herpesvirus infection in Asian elephants (Elephas maximus) of Assam, India. Vet World. (2019) 12:1790–6. doi: 10.14202/vetworld.
25. Crawley JAH, Lahdenperä M, Min Oo Z, Htut W, Nandar H, Lummaa V. Taming age mortality in semi-captive Asian elephants. Sci Rep. (2020) 10:1–8. doi: 10.1038/s41598-020-58590-7
26. Lee MH, Nathan SKSS, Benedict L, Nagalingam P, Latimer E, Hughes T, et al. The first reported cases of elephant endotheliotropic herpesvirus infectious haemorrhagic disease in Malaysia: case report. Virol J. (2021) 18:1–8. doi: 10.1186/s12985-021-01694-x
27. Yang N, Bao M, Zhu B, Shen Q, Guo X, Li W, et al. Elephant endotheliotropic herpesvirus 1, 4 and 5 in China: occurrence in multiple sample types and implications for wild and captive population surveillance. Viruses. (2022) 14:1–17. doi: 10.3390/v14020411
28. Richman LK, Montali RJ, Garber RL, Kennedy MA, Lehnhardt J, Hildebrandt T, et al. Novel endotheliotropic herpesviruses fatal for Asian and African elephants. Science. (1999) 283:1171–6. doi: 10.1126/science.283.5405.1171
29. Zong J, Latimer E, Heaggans SY, Richman LK, Hayward GS. (2007). Pathogenesis and molecular epidemiology of fatal elephant endotheliotropic disease associated with the expanding proboscivirus genus of the betaherpesvirinae, in: Proceedings of the International Elephant Conservation and Research Symposium, Florida. pp. 22–35.
30. Wiese RJ. Asian elephants are not self-sustaining in North America. Zoo Biol. (2000) 19:299–309. doi: 10.1002/1098-2361(2000)19:5<299::AID-ZOO2>3.0.CO;2-Z
31. Clubb R, Rowcliffe M, Lee P, Mar KU, Moss C, Mason GJ. Compromised survivorship in zoo elephants. Science. (2008) 322:1649. doi: 10.1126/science.1164298
32. Kendall R, Howard L, Masters N, Grant R. The impact of elephant endotheliotropic herpesvirus on the captive asian elephant (Elephas maximus) population of the United Kingdom and Ireland (1995–2013). J Zoo Wildl Med. (2016) 47:405–18. doi: 10.1638/2015-0217.1
33. Hoornweg TE, Schaftenaar W, Maurer G, van den Doel PB, Molenaar FM, Chamouard-Galante A, et al. Elephant endotheliotropic herpesvirus is omnipresent in elephants in european zoos and an asian elephant range country. Viruses. (2021) 13:1–15. doi: 10.3390/v13020283
34. Mills G. Finding a solution to a deadly virus in elephants. Vet Rec. (2022) 190:148–9. doi: 10.1002/vetr.v190.4
35. Wissink-Argilaga N, Dastjerdi A, Molenaar FM. Using in-house hematology to direct decision-making in the successful treatment and monitoring of a clinical and subsequently subclinical case of elephant endotheliotropic herpesvirus 1B. J Zoo Wildl Med. (2019) 50:498–502. doi: 10.1638/2018-0096
36. Fuery A, Tan J, Peng R, Flanagan JP, Tocidlowski ME, Howard LL, et al. Clinical infection of two captive asian elephants (Elephas maximus) with elephant endotheliotropic herpesvirus 1B. J Zoo Wildl Med. (2016) 47:319–24. doi: 10.1638/2015-0074.1
37. Stanton JJ, Zong J-C, Eng C, Howard L, Flanagan J, Stevens M, et al. Kinetics of viral loads and genotypic analysis of elephant endotheliotropic herpesvirus-1 infection in captive asian elephants (Elephas maximus). J Zoo Wildl Med. (2013) 44:42–54. doi: 10.1638/1042-7260-44.1.42
38. Drake GJ, Haycock J, Dastjerdi A, Davies H, Lopez FJ. Use of immunostimulants in the successful treatment of a clinical EEHV1A infection in an Asian elephant (Elephas maximus). Vet Rec Case Rep. (2020) 8. doi: 10.1136/vetreccr-2020-001158
39. Dastjerdi A, Seilern-Moy K, Darpel K, Steinbach F, Molenaar F. Surviving and fatal Elephant Endotheliotropic Herpesvirus-1A infections in juvenile Asian elephants - lessons learned and recommendations on anti-herpesviral therapy. BMC Vet Res. (2016) 12:1–11. doi: 10.1186/s12917-016-0806-5
40. Manns MP, Wedemeyer H, Cornberg M. Treating viral hepatitis C: Efficacy, side effects, and complications. Gut. (2006) 55:1350–9. doi: 10.1136/gut.2005.076646
41. Foster GR. Pegylated interferons for the treatment of chronic hepatitis C: Pharmacological and clinical differences between peginterferon-α-2a and peginterferon-α-2b. Drugs. (2010) 70:147–65. doi: 10.2165/11531990-000000000-00000
42. El-Baky NA, Redwan EM. Therapeutic alpha-interferons protein: Structure, production, and biosimilar. Prep Biochem Biotechnol. (2015) 45:109–27. doi: 10.1080/10826068.2014.907175
43. Tao Y, Wu D, Zhou L, Chen E, Liu C, Tang X, et al. Present and future therapies for chronic hepatits B. In: Tang H, editor. Hepatitis B Virus Inection Molecular Virology to Antiviral Drugs Advances in Experimental Medicine and Biology, vol. 1179 . Singapore: Springer Singapore (2020). p. 137–86.
44. Phillips S, Mistry S, Riva A, Cooksley H, Hadzhiolova-Lebeau T, Plavova S, et al. Peg-interferon lambda treatment induces robust innate and adaptive immunity in chronic hepatitis B patients. Front Immunol. (2017) 8. doi: 10.3389/fimmu.2017.00621
45. Gull I, Aslam MS, Tipu I, Mushtaq R, Ali TZ, Athar MA. Development of latent Interferon alpha 2b as a safe therapeutic for treatment of Hepatitis C virus infection. Sci Rep. (2019) 9:1–12. doi: 10.1038/s41598-019-47074-y
46. Bray M. The role of the type I interferon response in the resistance of mice of filovirus infection. J Gen Virol. (2001) 82:1365–73. doi: 10.1099/0022-1317-82-6-1365
47. Mahanty S, Gupta M, Paragas J, Bray M, Ahmed R, Rollin PE. Protection from lethal infection is determined by innate immune responses in a mouse model of Ebola virus infection. Virology. (2003) 312:415–24. doi: 10.1016/S0042-6822(03)00233-2
48. Hutchinson KL, Rollin PE. Cytokine and chemokine expression in humans infected with Sudan ebola virus. J Infect Dis. (2007) 196:S357–63. doi: 10.1086/520611
49. Konde MK, Baker DP, Traore FA, Sow MS, Camara A, Barry AA, et al. Interferon β-1a for the treatment of Ebola virus disease: A historically controlled, single-arm proof-of-concept trial. PloS One. (2017) 12:1–13. doi: 10.1371/journal.pone.0169255
50. Smith LM, Hensley LE, Geisbert TW, Johnson J, Stossel A, Honko A, et al. Interferon- therapy prolongs survival in rhesus macaque models of ebola and marburg hemorrhagic fever. J Infect Dis. (2013) 208:310–8. doi: 10.1093/infdis/jis921
51. Dastjerdi A, Robert C, Watson M. Low coverage sequencing of two Asian elephant (Elephas maximus) genomes. Gigascience. (2014) 3:1–3. doi: 10.1186/2047-217X-3-12
52. Reddy PC, Sinha I, Kelkar A, Habib F, Pradhan SJ, Sukumar R, et al. Comparative sequence analyses of genome and transcriptome reveal novel transcripts and variants in the Asian elephant Elephas maximus. J Biosci. (2015) 40:891–907. doi: 10.1007/s12038-015-9580-y
53. Kelley LA, Mezulis S, Yates CM, Wass MN, Sternberg MJE. The Phyre2 web portal for protein modeling, prediciton and analysis. Nat Protoc. (2015) 10:845–58. doi: 10.1038/nprot.2015.053
54. Petersen TN, Brunak S, Von Heijne G, Nielsen H. SignalP 4.0: Discriminating signal peptides from transmembrane regions. Nat Methods. (2011) 8:785–6. doi: 10.1038/nmeth.1701
55. Tao H, Liu W, Simmons BN, Harris HK, Cox C, Massiah MA. Purifying natively folded proteins from inclusion bodies using sarkosyl, Triton X-100, and CHAPS. Bio Tech. (2010) 48:61–4. doi: 10.2144/000113304
56. Massiah MA, Wright KM, Du H. Obtaining soluble folded proteins from inclusion bodies using sarkosyl, triton X-100, and CHAPS: Application to LB and M9 minimal media. Curr Protoc Protein Sci. (2016) 84:6.13.1–6.13.24. doi: 10.1002/0471140864.2016.84.issue-1
57. Seilern-Moy K. Pathogenic characteristics of elephant Endotheliotropic Herpesvirus Infection in Asian Elephants. United Kingdom: University of Surrey (2017).
58. Bennett L. Epidemiology and molecular biology of Elephant Endotheliotropic Herpesvirus 1 in the Asian elephant Elephas maximus - PhD thesis. United Kingdom: University of Nottingham (2018).
59. Photichai K, Guntawang T, Sittisak T, Kochagul V, Chuammitri P, Thitaram C, et al. Attempt to isolate elephant endotheliotropic herpesvirus (EEHV) using a continuous cell culture system. Animals. (2020) 10. doi: 10.3390/ani10122328
60. Pfeffer LM, Dinarello CA, Herberman RB, Williams BRG, Borden EC, Bordens R, et al. Biological properties of recombinant α-interferons: 40th Anniversary of the discovery of interferons. Cancer Res. (1998) 58:2489–99.
61. Diaz MO, Pomykala HM, Bohlander SK, Maltepe E, Malik K, Brownstein B, et al. Structure of the human type-I interferon gene cluster determined from a YAC clone contig. Genomics. (1994) 22:540–52. doi: 10.1006/geno.1994.1427
62. Zwarthoff EC, Mooren ATA, Trapman J. Organization, structure and expression of murine interferon alpha genes. Nucleic Acids Res. (1985) 13:791–804. doi: 10.1093/nar/13.3.791
63. Velan B, Cohen S, Grosfeld H, Leitner M, Shafferman A. Bovine interferon alpha genes. Structure and expression. J Biol Chem. (1985) 260:5498–504. doi: 10.1016/S0021-9258(18)89050-0
64. Woelk CH, Frost SDW, Richman DD, Higley PE, Kosakovsky Pond SL. Evolution of the interferon alpha gene family in eutherian mammals. Gene. (2007) 397:38–50. doi: 10.1016/j.gene.2007.03.018
65. Henco K, Brosius J, Fujisawa A, Fujisawa JI, Haynes JR, Hochstadt J, et al. Structural relationship of human interferon alpha genes and pseudogenes. J Mol Biol. (1985) 185:227–60. doi: 10.1016/0022-2836(85)90401-2
66. van Pesch V, Lanaya H, Renauld J-C, Michiels T. Characterization of the murine alpha interferon gene family. J Virol. (2004) 78:8219–28. doi: 10.1128/JVI.78.15.8219-8228.2004
67. Steinbach F, Mauel S, Beier I. Recombinant equine interferons: expression cloning and biological activity. Vet Immunol Immunopathol. (2002) 84:83–95. doi: 10.1016/S0165-2427(01)00396-8
68. Sreekumar E, Janki MBV, Arathy DS, Hariharan R, Premraj CA, Rasool TJ. Molecular characterization and expression of Interferon-γ of Asian elephant (Elephas maximus). Vet Immunol Immunopathol. (2007) 118:75–83. doi: 10.1016/j.vetimm.2007.04.012
69. Srivastava P, Bhattacharaya P, Pandey G, Mukherjee KJ. Overexpression and purification of recombinant human interferon alpha2b in Escherichia coli. Protein Expr Purif. (2005) 41:313–22. doi: 10.1016/j.pep.2004.12.018
70. Li M, He S. Purification and characterization of recombinant human interleukin-29 expressed in Escherichia coli. J Biotechnol. (2006) 112:334–40. doi: 10.1016/j.jbiotec.2005.11.019
71. Meng J, Yan Z, Xue X, Hao Q, Wan Y, Qin X, et al. High-yield expression, purification and characterization of tumor-targeted IFN-α2a. Cytotherapy. (2007) 9:60–8. doi: 10.1080/14653240601094322
72. Morowvat MH, Babaeipour V, Rajabi-Memari H, Vahidi H, Maghsoudi N. Overexpression of recombinant human beta interferon (rhINF-β) in periplasmic space of Escherichia coli. Iran J Pharm Res. (2014) 13:151–60.
73. Singh A, Upadhyay V, Upadhyay AK, Singh SM, Panda AK. Protein recovery from inclusion bodies of Escherichia coli using mild solubilization process. Microb Cell Fact. (2015) 14:1–10. doi: 10.1186/s12934-015-0222-8
74. Nagabhushan TL, Reichert P, Walter MR, Murgolo NJ. Type I interferon structures: Possible scaffolds for the interferon-alpha receptor complex. Can J Chem. (2002) 80:1166–73. doi: 10.1139/v02-158
75. Radhakrishnan R, Walter LJ, Hruza A, Reichert P, Trotta PP, Nagabhushan TL, et al. Zinc mediated dimer of human interferon-α(2b) revealed by X-ray crystallography. Structure. (1996) 4:1453–63. doi: 10.1016/S0969-2126(96)00152-9
76. Beldarraín A, Cruz Y, Cruz O, Navarro M, Gil M. Purification and conformational properties of a human interferon α2b produced in Escherichia coli. Biotechnol Appl Biochem. (2001) 33:173. doi: 10.1042/ba20010001
77. Karpusas M, Nolte M, Benton CB, Meier W, Lipscomb WN, Goelz S. The crystal structure of human interferon β at 2.2-Å resolution. Proc Natl Acad Sci U.S.A. (1997) 94:11813–8. doi: 10.1073/pnas.94.22.11813
78. Thomas C, Moraga I, Levin D, Krutzik PO, Podoplelova Y, Trejo A, et al. Structural linkage between ligand discrimination and receptor activation by Type I interferons. Cell. (2011) 146:621–32. doi: 10.1016/j.cell.2011.06.048
79. Génin P, Vaccaro A, Civas A. The role of differential expression of human interferon-A genes in antiviral immunity. Cytokine Growth Factor Rev. (2009) 20:283–95. doi: 10.1016/j.cytogfr.2009.07.005
80. Lavoie TB, Kalie E, Crisafulli-Cabatu S, Abramovich R, DiGioia G, Moolchan K, et al. Binding and activity of all human alpha interferon subtypes. Cytokine. (2011) 56:282–9. doi: 10.1016/j.cyto.2011.07.019
81. Piehler J, Thomas C, Christopher Garcia K, Schreiber G. Structural and dynamic determinants of type I interferon receptor assembly and their functional interpretation. Immunol Rev. (2012) 250:317–34. doi: 10.1111/imr.2012.250.issue-1
82. Schreiber G. The molecular basis for differential type I interferon signaling. J Biol Chem. (2017) 292:7285–94. doi: 10.1074/jbc.R116.774562
83. Horisberger MA, De Staritzky K. A recombinant human interferon-α B/D hybrid with a broad host-range. J Gen Virol. (1987) 68:945–8. doi: 10.1099/0022-1317-68-3-945
84. Ghaffar A, Mayer EP, Barnhart DC, Pyo S, Szymaniec S, Hochkeppel H -K, et al. Cross-species antiviral activity of a recombinant human alpha-interferon hybrid. Ann N Y Acad Sci. (1992) 653:314–22. doi: 10.1111/j.1749-6632.1992.tb19658.x
85. Namangala B, Inoue N, Kohara J, Kuboki N, Sakurai T, Hayashida K, et al. Evidence for the immunostimulatory effects of low-dose orally delivered human IFN-α in cattle. J Interf Cytokine Res. (2006) 26:675–81. doi: 10.1089/jir.2006.26.675
86. Bridgman R, Rossi CR, Campos M. The sensitivity of domestic animal cell lines to eight recombinant human interferons. J Interferon Res. (1988) 8:1–4. doi: 10.1089/jir.1988.8.1
87. Rubinstein S, Familletti PC, Pestka S. Convenient assay for interferons. J Virol. (1981) 37:755–8. doi: 10.1128/jvi.37.2.755-758.1981
88. Baer A, Kehn-Hall K. Viral concentration determination through plaque assays: Using traditional and novel overlay systems. J Vis Exp. (2014) 93):1–10. doi: 10.3791/52065
89. Meager A, Gaines Das R, Zoon K, Mire-Sluis A. Establishment of new and replacement World Health Organization International Biological Standards for human interferon alpha and omega. J Immunol Methods. (2001) 257:17–33. doi: 10.1016/S0022-1759(01)00460-4
90. Muylkens B, Thiry J, Kirten P, Schynts F, Thiry E. Bovine herpesvirus 1 infection and infectious bovine rhinotracheitis. Vet Res. (2007) 38:181–209. doi: 10.1051/vetres:2006059
91. Ma G, Azab W, Osterrieder N. Equine herpesviruses type 1 (EHV-1) and 4 (EHV-4)-Masters of co-evolution and a constant threat to equids and beyond. Vet Microbiol. (2013) 167:123–34. doi: 10.1016/j.vetmic.2013.06.018
92. Whalley JM, Ruitenberg KM, Sullivan K, Seshadri L, Hansen K, Birch D, et al. Host cell tropism of equine herpesviruses: Glycoprotein D of EHV-1 enables EHV-4 to infect a non-permissive cell line. Arch Virol. (2007) 152:717–25. doi: 10.1007/s00705-006-0885-x
93. Abdelgawad A, Azab W, Damiani AM, Baumgartner K, Will H, Osterrieder N, et al. Zebra-borne equine herpesvirus type 1 (EHV-1) infection in non-African captive mammals. Vet Microbiol. (2014) 169:102–6. doi: 10.1016/j.vetmic.2013.12.011
94. Wohlsein P, Lehmbecker A, Spitzbarth I, Algermissen D, Baumgärtner W, Böer M, et al. Fatal epizootic equine herpesvirus 1 infections in new and unnatural hosts. Vet Microbiol. (2011) 149:456–60. doi: 10.1016/j.vetmic.2010.11.024
95. Biswas S, Bandyopadhyay S, Dimri U, H. Patra P. Bovine herpesvirus-1 (BHV-1) - a re-emerging concern in livestock: A revisit to its biology, epidemiology, diagnosis, and prophylaxis. Vet Q. (2013) 33:68–81. doi: 10.1080/01652176.2013.799301
96. Metzler AE, Ossent P, Guscetti F, Rubel A, Lang EM. Serological evidence of herpesvirus infection in captive Asian elephants (Elephas maximus). J Wildl Dis. (1990) 26:41–9. doi: 10.7589/0090-3558-26.1.41
97. Bhat MN, Manickam R, Kumanan K. Serological evidence of bovine herpesviruses 1 and 2 in Asian elephants. J Wildl Dis. (1997) 33:919–20. doi: 10.7589/0090-3558-33.4.919
98. Cummins JM, Tompkins MB, Olsen RG, Tompkins WA, Lewis MG. Oral use of human alpha interferon in cats. J Biol Response Mod. (1988) 7:513–23.
99. Fish EN, Banerjee K, Levine HL, Stebbing N. Antiherpetic effects of a human alpha interferon analog, IFN-αCon1, in hamsters. Antimicrob Agents Chemother. (1986) 30:52–6. doi: 10.1128/AAC.30.1.52
100. Roney CS, Rossi CR, Smith PC, Lauerman LC, Spano JS, Hanrahan LA, et al. Effect of human leukocyte A interferon on prevention of infectious bovine rhinotracheitis virus infection of cattle. Am J Vet Res. (1985) 46:1251–5. doi: 10.2460/ajvr.1985.46.06.1251
101. Brunda MJ, Rosenbaum D. Modulation of murine natural killer cell activity in vitro and in vivo by recombinant human interferons. Cancer Res. (1984) 44:597–601.
102. McCarthy RR, Everett HE, Graham SP, Steinbach F, Crooke HR. Head start immunity: characterizing the early protection of C strain vaccine against subsequent classical swine fever virus infection. Front Immunol. (2019) 10:1–11. doi: 10.3389/fimmu.2019.01584
103. Haycock J, Maehr T, Dastjerdi A, Steinbach F. Immunostimulation of Asian elephant (Elephas maximus) blood cells by parapoxvirus ovis and CpG motif-containing bacterial plasmid DNA upregulates innate immune gene expression. Front Immunol. (2024) 15:1–13. doi: 10.3389/fimmu.2024.1329820
104. Haig DM. Subversion and piracy: DNA viruses and immune evasion. Res Vet Sci. (2001) 70:205–19. doi: 10.1053/rvsc.2001.0462
105. Levy DE, García-Sastre A. The virus battles: IFN induction of the antiviral state and mechanisms of viral evasion. Cytokine Growth Factor Rev. (2001) 12:143–56. doi: 10.1016/S1359-6101(00)00027-7
106. Melchjorsen J, Matikainen S, Paludan SR. Activation and evasion of innate antiviral immunity by herpes simplex virus. Viruses. (2009) 1:737–59. doi: 10.3390/v1030737
107. Katze MG, He Y, Gale M. Viruses and interferon: A fight for supremacy. Nat Rev Immunol. (2002) 2:675–87. doi: 10.1038/nri888
108. Prado NA, Campana MG, Brown JL, Maldonado JE, Latimer E, Pearson VR. Investigating disease susceptibility to elephant endotheliotropic herpesviruses in North American elephants using a genome-wide approach. In: Proceedings of the 2019 North American EEHV Workshop (2019) Houston, Texas: EEHV Advisory Group & Houston Zoo. p. 28.
Keywords: EEHV, elephant, innate immunity, interferon, herpesvirus
Citation: Haycock J, Maehr T, Dastjerdi A and Steinbach F (2025) Asian elephant interferons alpha and beta and their anti-herpes viral activity. Front. Immunol. 16:1533038. doi: 10.3389/fimmu.2025.1533038
Received: 22 November 2024; Accepted: 05 March 2025;
Published: 25 March 2025.
Edited by:
Jiong Chen, Ningbo University, ChinaReviewed by:
Avinash Premraj, Management of Scientific Centers & Presidential Camels, United Arab EmiratesRebecca L. Tallmadge, Cornell University, United States
Luke Jones, University of Oxford, United Kingdom
Devika Gautam, Deenbandhu Chhotu Ram University of Science and Technology, India
Copyright © 2025 Haycock, Maehr, Dastjerdi and Steinbach. This is an open-access article distributed under the terms of the Creative Commons Attribution License (CC BY). The use, distribution or reproduction in other forums is permitted, provided the original author(s) and the copyright owner(s) are credited and that the original publication in this journal is cited, in accordance with accepted academic practice. No use, distribution or reproduction is permitted which does not comply with these terms.
*Correspondence: Falko Steinbach, Zi5zdGVpbmJhY2hAc3VycmV5LmFjLnVr