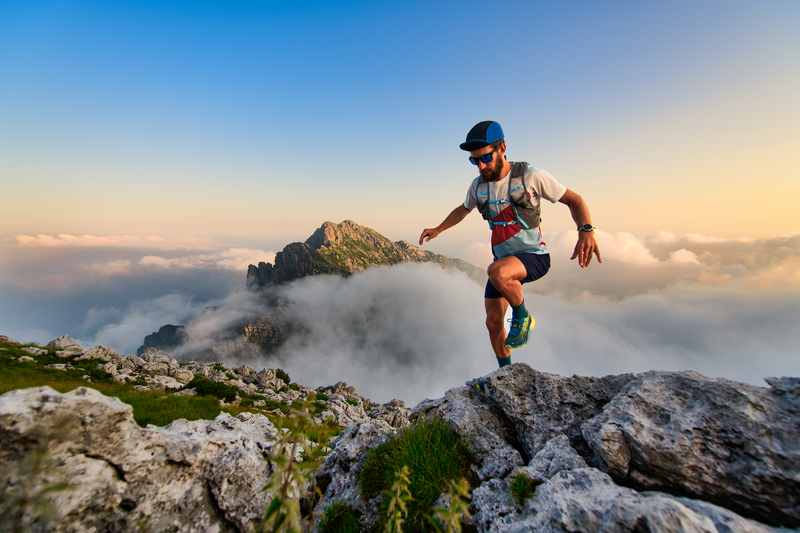
95% of researchers rate our articles as excellent or good
Learn more about the work of our research integrity team to safeguard the quality of each article we publish.
Find out more
REVIEW article
Front. Immunol. , 20 March 2025
Sec. Cancer Immunity and Immunotherapy
Volume 16 - 2025 | https://doi.org/10.3389/fimmu.2025.1526368
ETS-1, a key member of the Erythroblast Transformation-Specific (ETS) transcription factor family, plays an important role in cell biology and medical research due to its wide expression profile and strong transcriptional regulation ability. It regulates fundamental biological processes, including cell proliferation, differentiation, and apoptosis, and is involved in tumorigenesis and metastasis, promoting malignant behaviors such as angiogenesis, matrix degradation, and cell migration. Given the association between ETS-1 overexpression and the aggressive characteristics of multiple malignancies, it represents a promising therapeutic target in cancer treatment. This study aims to systematically analyze the role of ETS-1 within the tumor immune microenvironment, elucidating its mechanisms in cancer initiation, progression, and metastasis. It also investigates the differential expression of ETS-1 across tumor tissues and adjacent normal tissues, exploring its potential as a molecular marker for tumor diagnosis and prognosis.
The Erythroblast Transformation-Specific (ETS) transcription factor family consists of proteins characterized by a highly conserved ETS domain, which is involved in key biological processes, including cell proliferation, differentiation, apoptosis, and organogenesis (1, 2). This family has numerous members with coding genes distributed across various genomic loci, regulating downstream gene expression through specific DNA sequence recognition and binding to gene promoters or enhancers (3–5). ETS transcription factors are important in embryonic development, tissue homeostasis, and disease progression, making them one of the hot spots in biological and medical research (6).
Among the ETS family, ETS-1 is characterized by its broad expression and potent transcriptional regulation, making it a key research subject (7). ETS-1 participates in lymphocyte development and differentiation and significantly influences the proliferation, migration, and invasion of various cell types (8, 9). Notably, aberrant ETS-1 expression in various malignancies is strongly associated with tumor progression, including angiogenesis, extracellular matrix degradation, and metastasis—crucial steps in cancer invasion (10–13). Therefore, ETS-1 is considered a promising therapeutic target in cancer treatment, and its comprehensive study holds significant scientific and clinical importance.
Research in tumor immunity is a prominent focus in biomedicine, aiming to elucidate the mechanisms of interaction between tumors and the immune system and to identify effective immunotherapy strategies. Advances in understanding the tumor immune microenvironment have highlighted the immune system’s vital role in tumorigenesis, progression, and metastasis. Immune cells not only inhibit tumor growth through direct cytotoxicity but also modulate the tumor microenvironment via cytokine secretion (14). Therefore, a detailed investigation into the roles of key molecules such as ETS-1 in tumor immunity is of great significance for developing novel immunotherapeutic approaches.
This review systematically examines the expression and function of ETS-1 within the tumor immune microenvironment, aiming to elucidate its mechanisms in tumor initiation, progression, and metastasis. To thoroughly understand ETS-1’s role, we compare its expression in various tumor types and adjacent normal tissues. Evaluating the differential expression of ETS-1 across cancer subtypes may facilitate the identification of novel molecular markers for tumor diagnosis and prognosis. Moreover, this study explores the relationship between the molecular structure of ETS-1 and its functional roles. As a transcription factor, ETS-1’s activity is determined by key domains, including the ETS domain, transcriptional activation domain, and protein interaction domain. This review analyzes the binding mechanisms of ETS-1 to target gene promoter DNA using molecular biology and biochemical methods, thereby revealing the molecular basis of its transcriptional regulation. It also explores the interactions between ETS-1 and other transcription factors or signaling molecules to clarify its involvement in complex networks of tumor immune regulation.
Overall, this review aims to provide a comprehensive analysis of ETS-1’s mechanisms in tumor immunity, offering novel theoretical and experimental insights for tumor diagnosis, treatment, and prognosis assessment.
The ETS transcription factor family is a large and diverse gene family that is present in humans and various other species (1). The family members are further subdivided into multiple subfamilies based on sequence similarity and functional differences of the ETS domain (15–17). As genomics research advances, more ETS family members continue to be identified. The classification criteria include the conserved sequences within the ETS domain, structural protein features, combinations of functional domains, and the types of target genes they regulate.
Each ETS subfamily has unique structural and functional characteristics. For instance, the Polymoma Virus Enhancer-3 (PEA3) subfamily (e.g., PEA3, ETS Variant Transcription Factor 4 (E1AF), and Erythromycin Resistance Methylases (ERM)) is involved in processes such as cell migration, invasion, and tumor progression (18–20). The ETS Related Gene (ERG) subfamily is closely associated with heart and vascular development (21), while the ETV6 (or TEL) subfamily (e.g., TEL and ETS variant 6 (ETV6)) plays a role in hematopoietic system development and tumorigenesis (22). Furthermore, other subfamilies, such as ETS variant (ETV), friend leukemia virus integration (FLI), and GA binding protein transcription factor subunit alpha (GABPA), are implicated in a wide range of biological functions, including cell proliferation, differentiation, metabolism, and immune regulation (23–25) (Table 1).
The ETS domain is the core functional region of ETS transcription factors, showing a significant degree of conservation across different family members, particularly at the DNA-binding interface (26). This conservation enables ETS proteins to recognize and bind specific DNA sequences, thereby regulating the expression of downstream target genes. However, subtle variations in the sequence, domain length, and combination with other functional domains result in diverse DNA-binding specificities and transcriptional regulation capabilities among different ETS proteins (27).
The interaction between ETS proteins and DNA is a complex process involving multiple molecular interactions. The ETS domain typically recognizes and binds to the ETS binding site, usually a GGAA/T core sequence, located in the promoter or enhancer regions of target genes, through an α-helix-turn-α-helix motif (28–30). During this binding process, specific amino acid residues in the ETS domain form hydrogen bonds and hydrophobic interactions with DNA bases, stabilizing the protein-DNA complex (31, 32). Moreover, ETS proteins may interact with other transcription factors, further influencing transcriptional regulation (33, 34).
The activity of ETS family proteins is not only regulated by external signals and other transcription factors but also by intrinsic molecular mechanisms. Post-translational modifications such as autophosphorylation and ubiquitination influence ETS protein stability, subcellular localization, and transcriptional activity, with these modifications catalyzed by specific enzymes and finely controlled by intracellular and extracellular signals (26, 35, 36).
ETS proteins also engage in combined regulation with other transcription factors. In complex gene expression networks, they often form transcription factor complexes that co-regulate target gene expression, enhancing regulatory specificity and efficiency. This coordinated regulation allows for fine-tuned transcriptional responses to different external signals and cellular states (37). Furthermore, ETS protein activity is modulated by growth factors and signaling pathways. For instance, epidermal growth factor (EGF) and fibroblast growth factor (FGF) activate the mitogen-activated protein kinase (MAPK) pathway, leading to phosphorylation and activation of ETS proteins (38, 39). Further, ETS-1 can inhibit transforming growth factor alpha (TGF-α) expression, thereby restoring tumor cell proliferation in vivo and promoting autonomous growth in culture (40). Other pathways, including Phosphoinositide 3-kinase (PI3K)/Protein Kinase B (Akt) and Janus kinase and signal transducer and activator of transcription (JAK-STAT), also contribute to ETS protein regulation by modulating phosphorylation, stability, and interactions with other molecules (41, 42).
ETS-1, a key member of the ETS transcription factor family, has unique and complex structural characteristics. Its amino acid sequence is highly conserved, particularly in the ETS domain, which is essential for recognizing and binding DNA target sequences. In addition to the ETS domain, ETS-1 contains other functional regions, including a transcriptional activation domain, inhibition domain, nuclear localization signal (NLS), and potential phosphorylation sites. These domains collectively determine the transcriptional regulation activity and intracellular localization of ETS-1 (1, 123).
The ETS domain is the core structure of ETS-1, having a unique α-helix-turn-α-helix DNA-binding motif (Figure 1). This domain relies on hydrogen bonds and hydrophobic interactions to perform its function (124). The high conservation of the ETS domain ensures the precise recognition and binding to specific ETS binding sites, such as the GGAA/T core sequence, thereby regulating the expression of downstream target genes (125).
Figure 1. ETS protein structure. The ETS1 Pointed domain, the acidic transactivation domain, the autoinhibitory domains, and the Ets DNA binding domain, are indicated. Also shown is the conserved MAP kinase phosphorylation site (T38) found at the N-terminus of ETS1 protein.
ETS-1 plays a crucial regulatory role in cell proliferation, differentiation, and migration (126). It influences gene expression by binding directly to the promoter or enhancer regions of target genes, thus affecting cell growth and differentiation (127). For example, ETS-1 can up-regulate cyclin expression, accelerating the transition from the G1 to S phase, thereby promoting cell proliferation (128). Moreover, ETS-1 is also involved in cytoskeleton remodeling and regulates the expression of intercellular adhesion molecules, impacting cell migration and invasion (129).
ETS-1 also modulates apoptosis and angiogenesis (130, 131). It regulates apoptosis by influencing the expression of genes such as Bcl-2 family members and caspases (132). Furthermore, ETS-1 is involved in regulating the expression of angiogenic factors, including vascular endothelial growth factor (VEGF) and fibroblast growth factor (FGF), which are critical for angiogenesis and tumor-related vascular development (133, 134). Therefore, abnormal ETS-1 expression is associated with various diseases characterized by dysregulated angiogenesis, such as cancer and diabetic retinopathy (44, 127, 130).
The expression of ETS-1 is both widespread and tissue-specific. While it is expressed to varying degrees in most normal tissues and organs, its levels are highly regulated temporally and spatially. During embryonic development, ETS-1 is essential for the formation of key organs, such as the heart, blood vessels, and nervous system (130, 135). In adult tissues, its expression is associated with physiological processes, including cell proliferation, differentiation, and immune responses (51, 132, 136). Under pathological conditions, such as in tumor tissues, ETS-1 expression is often abnormally elevated, correlating with its role in tumorigenesis, progression, and metastasis (47, 137).
The expression of ETS-1 varies across different tumor types, with notable overexpression in several solid tumors, including breast cancer (11), prostate cancer (138), lung cancer (137), and colorectal cancer (45). Its expression levels also show significant changes in hematological malignancies, such as leukemia (139) and lymphoma (140). These variations may be attributed to the genetic background, the microenvironment of different tumors, and the functional specificity of ETS-1 in various cell types.
ETS-1 contributes to tumorigenesis and progression through multiple molecular mechanisms. It can directly regulate genes involved in cell proliferation, apoptosis, migration, and invasion, thereby promoting the malignant transformation and invasiveness of tumor cells (141, 142). The up-regulation of Ets-1 expression is intimately linked to tumour invasion and metastasis. It triggers the expression of a series of genes that have a crucial role in extracellular matrix remodeling and angiogenesis through transcription, and takes part in numerous invasion and metastasis processes such as extracellular matrix degradation and cell migration (142). Furthermore, ETS-1 may maintain the sustained growth and metastasis of tumor tissues by modulating the self-renewal and differentiation of cancer stem cells (143).
ETS-1 plays a crucial role in shaping the tumor immune microenvironment by regulating immune cell infiltration, activation, and the expression of immunosuppressive factors. It influences the migration and infiltration of immune cells into tumor tissues by modulating chemokines (e.g., CXCL12) and adhesion molecules (e.g., ICAM-1) (144), and affects immune cell activation and anti-tumor responses by regulating the expression and function of immune cell surface receptors (145). Furthermore, ETS-1 promotes tumor immune escape by directly regulating the expression of multiple immune checkpoint molecules. While the exact role of ETS-1 in the upregulation of PD-L1, CTLA-4, LAG-3, and TIM-3 requires further confirmation, studies suggest that ETS-1 may contribute to the regulation of these molecules, which are known to suppress T cell activation and function, thus enabling tumor cells to evade immune recognition and clearance (123, 146, 147). Consequently, the pivotal role of ETS-1 in tumor immune evasion highlights its potential as a promising target for immunotherapy.
As a multifunctional transcription factor, ETS-1 regulates genes associated with immunosuppression, thereby facilitating tumor immune escape. For example, ETS-1 exerts a pivotal influence in renal epithelial cells, particularly in the context of TGF-β1 stimulation. It primarily suppresses the downstream effects of the TGF-β signaling pathway, which is indicative of its significant regulatory role in extracellular matrix remodeling and fibrosis. This regulation is likely mediated through its impact on key molecules within the TGF-β signaling cascade. Additionally, ETS-1 plays a crucial role in shaping the tumor immune microenvironment by regulating the expression of immunosuppressive factors, such as TGF-β. TGF-β is known to inhibit T cell activation and proliferation, while enhancing the function of regulatory T cells (Tregs) and myeloid-derived suppressor cells (MDSCs), thus contributing to immune evasion. While the exact mechanism of ETS-1 activation of TGF-β expression requires further validation, studies suggest that ETS-1 may regulate TGF-β and its associated immune responses, underscoring its complex role in both fibrosis and immune modulation (148–150). ETS-1 also regulates interleukin-10 (IL-10), a cytokine that suppresses immune cell function by inhibiting antigen presentation by macrophages and dendritic cells and reducing the production of Th1 cytokines (151, 152). These factors contribute to immune escape through complex signaling pathways and cell-cell interactions, allowing tumor cells to evade the recognition and clearance of the immune system.
ETS-1 plays a pivotal role in the tumour immune microenvironment by modulating antigen presentation and cytokine networks through multiple mechanisms. Research has demonstrated that ETS-1 regulates the expression of MHC Class I molecules in tumour cells, thereby influencing the recognition of tumour cells by cytotoxic T cells (153). Additionally, ETS-1 modulates the expression of cell adhesion molecules, such as ICAM-1, which affects the infiltration of immune cells into the tumour microenvironment (154). These actions not only enhance the immune evasion capabilities of tumour cells but also weaken antitumor immune responses by influencing the adhesion and migration of immune cells. Furthermore, ETS-1 is involved in the regulation of various cytokines, including TGF-β and IL-10, which inhibit antigen presentation and reduce the production of Th1 cytokines, thereby further suppressing immune responses (151). Collectively, these studies highlight the multifaceted role of ETS-1 in promoting tumour immune evasion and progression by regulating antigen presentation and cytokine networks.
In addition to its direct effects on immune cells, ETS-1 influences the tumor microenvironment by regulating the extracellular matrix (ECM) composition and structure. The ECM plays a critical role in tumor growth, invasion, and metastasis, and ETS-1 contributes to ECM remodeling by up-regulating matrix metalloproteinases (MMPs) (155–157). MMPs are a class of enzymes that degrade various extracellular matrix components, and they play a key role in the migration and invasion of tumor cells (158). ETS-1 up-regulates the expression of MMPs, which not only helps tumor cells break through the matrix barrier and infiltrate and metastasize to surrounding tissues but also affects the infiltration and distribution of immune cells by changing the physical structure of the tumor microenvironment (159). For example, ECM degradation by MMPs may enhance vascular permeability, allowing more immune cells and inflammatory factors to enter the tumor microenvironment (160, 161). Structural changes in the ECM can also impact immune cell movement and distribution patterns, influencing anti-tumor immune responses (162–164).
ETS-1 plays a significant regulatory role in various biological processes of tumors, with its function being influenced by multiple signalling pathways, particularly the MAPK/ERK, PI3K/Akt, and TGF-β pathways. Firstly, ETS-1 is a key target of the MAPK/ERK (Mitogen-Activated Protein Kinase/Extracellular Signal-Regulated Kinase) pathway, which is crucial for cell proliferation, differentiation, survival, and response to external stimuli. Secondly, the expression and activity of ETS-1 are also regulated by the PI3K/Akt (Phosphoinositide 3-Kinase/Protein Kinase B) pathway. This pathway, essential for cell survival and metabolism, stabilizes ETS-1 protein and promotes its nuclear accumulation, thereby enhancing its transcriptional function. Additionally, the TGF-β (Transforming Growth Factor Beta) pathway, under the regulation of ETS-1, affects the remodeling of the extracellular matrix and the infiltration of immune cells. TGF-β signalling promotes ETS-1 expression, influencing the immune response within the tumour microenvironment and the potential for tumour metastasis, thereby supporting the aggressiveness of tumors (Figure 2).
Figure 2. The regulatory effect of ETS-1 related signaling pathway on tumor biological processes. This figure shows the role of ETS-1 in cell proliferation, cell migration, immune escape and angiogenesis through the three main signaling pathways of MAPK/ERK, PI3K/Akt and TGF-β.
In breast cancer, high expression of ETS-1 is closely associated with poor prognosis, increased malignancy, and invasiveness. Studies indicate that elevated ETS-1 levels correlate with higher tumor grade, greater risk of lymph node metastasis, and shorter patient survival (11, 127, 165). Thus, ETS-1 expression may serve as a valuable prognostic biomarker for breast cancer.
ETS-1 plays a key role in regulating breast cancer cell proliferation and metastasis. It acts as a transcription factor by binding to the promoter regions of cell cycle-related genes, such as cyclin D1 and c-myc. Cyclin D1, a key regulator in the cyclin family, is crucial for the transition from the G1 to S phase during cell division. ETS-1 directly activates cyclin D1 transcription by binding to its promoter, resulting in increased Cyclin D1 protein levels, which accelerate the progression through the G1/S checkpoint and increase breast cancer cell proliferation. Similarly, ETS-1 up-regulates the proto-oncogene c-myc, a transcription factor involved in cell proliferation, differentiation, and apoptosis. By increasing c-myc gene transcription, ETS-1 increases the accumulation of c-Myc protein, a nuclear-phosphorylated protein with transcription factor activity, thereby activating downstream targets such as ribosomal biosynthesis and cell cycle regulatory genes. This creates a positive feedback loop that further promotes cancer cell proliferation (166–169).
ETS-1 plays a significant role in the development and progression of gastric cancer. Research indicates that ETS-1 expression is significantly elevated in gastric cancer tissues compared to normal gastric mucosa and is strongly associated with the pathological stage, lymph node metastasis, and patient prognosis (170). ETS-1 contributes to gastric cancer progression by promoting cell proliferation, inhibiting apoptosis, and facilitating migration and invasion (171). An important mechanism in gastric cancer progression is immune escape, which enables tumor cells to evade immune surveillance and destruction. Inhibiting ETS-1 expression or function may help disrupt the immune escape process, potentially restoring the anti-tumor activity of the immune system (56, 142).
ETS-1 plays a significant regulatory role in colorectal cancer, where its high expression is strongly associated with increased malignancy, lymph node metastasis, and distant metastasis (166, 172). It promotes the occurrence and development of colorectal cancer by regulating cell proliferation, migration, and invasion (173). Furthermore, ETS-1 can induce epithelial-mesenchymal transition (EMT), increasing the invasive and migratory capabilities of colorectal cancer cells, thereby facilitating metastasis (174). ETS-1’s interaction with immune cells and matrix components in the tumor microenvironment affects local inflammation and immune evasion, further contributing to colorectal cancer progression. Thus, ETS-1 serves as both a key regulator in colorectal cancer biology and a potential therapeutic target. Targeting ETS-1 could provide new treatment options and improve clinical outcomes for patients.
In prostate cancer, ETS-1 expression is often higher compared to normal prostate tissue, correlating with disease progression and prognosis (175). ETS-1 may interact with multiple signaling pathways, including the androgen receptor (AR) pathway, which is important in androgen-dependent prostate cancer (176). Moreover, ETS-1 may interact with other transcription factors, such as Nuclear Factor Kappa B (NF-κB), modulating inflammation and the tumor microenvironment (177).
In addition, ETS1 can reduce the mRNA levels of Twist1, a gene involved in tumor cell motility and dissemination, thereby decreasing invasion and increasing cell growth to mitigate lung tumor metastasis. Downregulation of the ETS1 gene can inhibit cell proliferation and migration in non-small cell lung cancer (NSCLC) cells, slowing the progression of lung cancer (51, 178). In chronic myeloid leukemia (CML), ETS1 is associated with the BCR-ABL signaling pathway, regulates granulocyte differentiation, and is downregulated during tyrosine kinase inhibitor (TKI) treatment (179). In diffuse large B-cell lymphoma (DLBCL), ETS1 modulates B-cell signaling, differentiation, and immune-related genes, with FCMR identified as a novel target that promotes lymphomagenesis. In adult T-cell leukemia/lymphoma (ATLL), ETS1 is highly expressed in patients of North American descent and promotes tumor growth by regulating CCR4, making it a potential therapeutic target (180, 181).
Overall, ETS-1 plays a key regulatory role across various tumor types, with its expression and functional activity closely associated with tumor occurrence, development, prognosis, and response to immunotherapy. Therefore, understanding the mechanisms of ETS-1 in cancer is of great significance for developing novel anti-tumor strategies (Figure 3).
Figure 3. The expression of ETS-1 in different tumors. The map contains the role of ETS1 in breast cancer, gastric cancer, colorectal cancer, prostate cancer, kidney cancer, liver cancer, cervical cancer, pancreatic cancer and nasopharyngeal carcinoma.
With an in-depth understanding of the mechanism of action of ETS-1 in tumors, the development of inhibitors for ETS-1 has become a research focus. Current approaches to inhibiting ETS-1 include small molecule compounds, antisense oligonucleotides (ASOs), and CRISPR/Cas9-based gene editing tools. Small molecule compounds inhibit ETS-1’s transcriptional activity by binding to its DNA-binding or transcriptional activation domains (10). ASOs (antisense oligonucleotides) may interfere with the expression of ETS-1 by binding to its mRNA, thereby blocking its translation. Although the precise mechanism is not fully established in the current literature, the role of antisense oligonucleotides in regulating the expression of transcription factors has been widely studied, suggesting that they may influence the regulation of ETS-1 (182, 183). CRISPR/Cas9-based gene editing can knock out the ETS-1 gene, effectively eliminating its expression (164, 184). While their mechanisms differ, these inhibitors share a common goal of reducing ETS-1 expression and activity in tumor cells.
Chimeric antigen receptor T cell (CAR-T) therapy, an emerging immunotherapeutic approach, utilizes genetically engineered T cells to target and destroy tumour cells (185–187). ETS-1, an intracellular transcription factor, is a key player in tumorigenesis, making it a potential target for CAR-T therapies. However, targeting intracellular proteins like ETS-1 is challenging due to the traditional focus of CAR-T therapies on cell surface antigens. Recent advances, such as CRISPR-Cas9 gene editing and novel vector systems, have enabled the design of CAR-T cells capable of targeting specific intracellular molecules (188, 189). Additionally, research is exploring adapter molecules and logic gate designs to enhance CAR-T specificity and activity (190). While these innovations offer potential for targeting ETS-1, further optimization in design, production, and clinical application is needed. Future directions may include developing CAR structures that can penetrate the cell membrane or modulating ETS-1 expression through pharmacological and genetic approaches to enhance therapy efficacy.
Although ETS-1 inhibitors remain in preclinical development, preliminary data suggest their potential therapeutic value. In animal models, ETS-1 inhibitors have demonstrated significant suppression of tumor growth and metastasis, along with enhancement of anti-tumor immune responses (10, 52). However, clinical translation faces challenges, including issues of specificity, efficacy, safety, and optimal administration routes. Future clinical trials must focus on evaluating the safety, tolerability, and efficacy of ETS-1 inhibitors in humans to assess their potential as viable cancer therapeutics.
This review highlights the pivotal role of ETS-1 in tumor immunity, detailing its aberrant expression across various tumor types and its significant influence on tumorigenesis, progression, immune evasion, and sensitivity to immunotherapy. ETS-1 promotes tumor advancement not only by regulating tumor cell proliferation, migration, and invasion but also by contributing to immune escape mechanisms through modulation of immune cell infiltration, activation, and immune checkpoint molecule expression within the tumor microenvironment. These findings offer a new perspective on the molecular basis of tumor immunity and present ETS-1 as a promising target for developing novel anti-cancer therapies.
The clinical potential of ETS-1 in tumor immunotherapy is vast. As research into the functional mechanisms of ETS-1 progresses and therapeutic technologies advance, ETS-1 could emerge as a key target for cancer immunotherapy. In clinical settings, assessing ETS-1 expression levels may provide a means to evaluate patient prognosis and guide personalized treatment decisions. Moreover, developing targeted therapies and immunotherapeutic strategies directed at ETS-1 could offer new therapeutic options and improve outcomes for cancer patients.
To fully realize the potential of ETS-1 as a therapeutic target and facilitate its clinical translation, future research should prioritize the following areas: First, a deeper investigation into the molecular mechanisms of ETS-1 in tumorigenesis, including its interactions with key molecules and signaling pathways, is essential. Second, efforts should be directed toward developing more specific and efficacious ETS-1 inhibitors while minimizing potential toxicities. Third, exploring the combined use of ETS-1-targeted therapies with other immunotherapeutic strategies, such as immune checkpoint inhibitors or CAR-T cell therapy, could achieve synergistic therapeutic effects. Fourth, attention must be given to understanding and overcoming drug resistance mechanisms that may arise during ETS-1-targeted treatment. Finally, conducting large-scale clinical trials is crucial to validate the efficacy and safety of ETS-1-targeted therapies, thus providing robust evidence for clinical application.
SW: Writing – original draft. LW: Conceptualization, Funding acquisition, Resources, Supervision, Writing – review & editing. XZ: Conceptualization, Resources, Supervision, Writing – review & editing. HF: Data curation, Writing – original draft. MZ: Supervision, Validation, Writing – original draft. FL: Methodology, Supervision, Writing – original draft. DY: Investigation, Software, Writing – original draft.
The author(s) declare that financial support was received for the research and/or publication of this article. This research was funded by National Natural Science Foundation of China General Program, grant number 82274501.
The authors declare that the research was conducted in the absence of any commercial or financial relationships that could be construed as a potential conflict of interest.
The author(s) declare that no Generative AI was used in the creation of this manuscript.
All claims expressed in this article are solely those of the authors and do not necessarily represent those of their affiliated organizations, or those of the publisher, the editors and the reviewers. Any product that may be evaluated in this article, or claim that may be made by its manufacturer, is not guaranteed or endorsed by the publisher.
1. Sizemore GM, Pitarresi JR, Balakrishnan S, Ostrowski MC. The ETS family of oncogenic transcription factors in solid tumours. Nat Rev Cancer. (2017) 17:337–51. doi: 10.1038/nrc.2017.20
2. Wang YF, Huang Z, Sun MY, Huang WJ, Xia LM. ETS transcription factors: Multifaceted players from cancer progression to tumor immunity. Biochim Biophys Acta Rev Cancer. (2023) 1878:188872. doi: 10.1016/j.bbcan.2023.188872
3. Evans EL, Saxton J, Shelton SJ, Begitt A, Holliday ND, Hipskind RA, et al. Dimer formation and conformational flexibility ensure cytoplasmic stability and nuclear accumulation of Elk-1. Nucleic Acids Res. (2011) 39:6390–402. doi: 10.1093/nar/gkr266
4. Zhang TY, Wang YJ, Xie M, Ji XY, Luo XY, Chen XP, et al. HGF-mediated elevation of ETV1 facilitates hepatocellular carcinoma metastasis through upregulating PTK2 and c-MET. J Exp Clin Cancer Res. (2022) 41:275. doi: 10.1186/s13046-022-02475-2
5. Plotnik JP, Hollenhorst PC. Interaction with ZMYND11 mediates opposing roles of Ras-responsive transcription factors ETS1 and ETS2. Nucleic Acids Res. (2017) 45:4452–62. doi: 10.1093/nar/gkx039
6. Mundorf J, Donohoe CD, McClure CD, Southall TD, Uhlirova M. Ets21c governs tissue renewal, stress tolerance, and aging in the drosophila intestine. Cell Rep. (2019) 27:3019–33. doi: 10.1016/j.celrep.2019.05.025
7. Sheng KQ, Wu YC, Lin HB, Fang MH, Xue CR, Lin X, et al. Transcriptional regulation of Siglec-15 by ETS-1 and ETS-2 in hepatocellular carcinoma cells. Int J Mol Sci. (2023) 24:792. doi: 10.3390/ijms24010792
8. Koskela K, Lassila O. Single-cell analysis of Ets-1 transcription factor expression during lymphocyte activation and apoptosis. Scand J Immunol. (2003) 57:56–61. doi: 10.1046/j.1365-3083.2003.01192.x
9. Shin SS, Park SS, Hwang B, Kim WT, Choi YH, Kim WJ, et al. MicroRNA-106a suppresses proliferation, migration, and invasion of bladder cancer cells by modulating MAPK signaling, cell cycle regulators, and Ets-1-mediated MMP-2 expression. Oncol Rep. (2016) 36:2421–9. doi: 10.3892/or.2016.5015
10. Jie YM, Liu GJ, E M, Li Y, Xu G, Guo JJ, et al. Novel small molecule inhibitors of the transcription factor ETS-1 and their antitumor activity against hepatocellular carcinoma. Eur J Pharmacol. (2021) 906:174214. doi: 10.1016/j.ejphar.2021.174214
11. Furlan A, Vercamer C, Heliot L, Wernert N, Desbiens X, Pourtier A. Ets-1 drives breast cancer cell angiogenic potential and interactions between breast cancer and endothelial cells. Int J Oncol. (2019) 54:29–40. doi: 10.3892/ijo.2018.4605
12. Hashiya N, Jo N, Aoki M, Matsumoto K, Nakamura T, Sato Y, et al. In vivo evidence of angiogenesis induced by transcription factor Ets-1: Ets-1 is located upstream of angiogenesis cascade. Circulation. (2004) 109:3035–41. doi: 10.1161/01.CIR.0000130643.41587.DB
13. Zhang X, Wu D, Aldarouish M, Yin XD, Li CY, Wang CL. ETS-1: A potential target of glycolysis for metabolic therapy by regulating glucose metabolism in pancreatic cancer. Int J Oncol. (2017) 50:232–40. doi: 10.3892/ijo.2016.3770
14. Vu BT, Tan Le D, Van Pham P. Synergistic effect of chimeric antigen receptors and cytokineinduced killer cells: An innovative combination for cancer therapy. Biomed Res Ther. (2016) 3:653–65. doi: 10.15419/bmrat.v3i06.99
15. Zeng GD, Wang T, Zhang JY, Kang YJ, Feng L. FLI1 mediates the selective expression of hypoxia-inducible factor 1 target genes in endothelial cells under hypoxic conditions. FEBS Open Bio. (2021) 11:2174–85. doi: 10.1002/2211-5463.13220
16. Bose R, Karthaus WR, Armenia J, Abida W, Iaquinta PJ, Zhang Z, et al. ERF mutations reveal a balance of ETS factors controlling prostate oncogenesis. Nature. (2017) 546:671–5. doi: 10.1038/nature22820
17. Kerr N, Pintzas A, Holmes F, Hobson SA, Pope R, Wallace M, et al. The expression of ELK transcription factors in adult DRG: Novel isoforms, antisense transcripts and upregulation by nerve damage. Mol Cell Neurosci. (2010) 44:165–77. doi: 10.1016/j.mcn.2010.03.005
18. Kandemir B, Kurnaz IA. The role of Pea3 transcription factor subfamily in the nervous system. Mol Neurobiol. (2024) 62:3293–304. doi: 10.1007/s12035-024-04432-w
19. Park J, Jang JH, Oh S, Kim M, Shin C, Jeong M, et al. LPA-induced migration of ovarian cancer cells requires activation of ERM proteins via LPA1 and LPA2. Cell Signal. (2018) 44:138–47. doi: 10.1016/j.cellsig.2018.01.007
20. Takahashi A, Higashino F, Aoyagi M, Yoshida K, Itoh M, Kobayashi M, et al. E1AF degradation by a ubiquitin–proteasome pathway. Biochem Biophys Res Commun. (2005) 327:575–80. doi: 10.1016/j.bbrc.2004.12.045
21. Zhang X, Hu C, Yuan YP, Song P, Kong CY, Wu HM, et al. Endothelial ERG alleviates cardiac fibrosis via blocking endothelin-1-dependent paracrine mechanism. Cell Biol Toxicol. (2021) 37:873–90. doi: 10.1007/s10565-021-09581-5
22. Carella C, Potter M, Bonten J, Rehg JE, Neale G, Grosveld GC. The ETS factor TEL2 is a hematopoietic oncoprotein. Blood. (2006) 107:1124–32. doi: 10.1182/blood-2005-03-1196
23. Van Pham P, Vu NB, Nguyen HT, Dao TTT, Le HTN, Phi LT, et al. ETV-2 activated proliferation of endothelial cells and attenuated acute hindlimb ischemia in mice. In Vitro Cell Dev Biol Anim. (2017) 53:616–25. doi: 10.1007/s11626-017-0151-4
24. Park SW, Do HJ, Choi W, Kim JH. Fli-1 promotes proliferation and upregulates NANOGP8 expression in T-lymphocyte leukemia cells. Biochimie. (2020) 168:1–9. doi: 10.1016/j.biochi.2019.10.005
25. Guo YX, Yuan XT, Li KL, Dai MK, Zhang L, Wu YJ, et al. GABPA is a master regulator of luminal identity and restrains aggressive diseases in bladder cancer. Cell Death Differ. (2020) 27:1862–77. doi: 10.1038/s41418-019-0466-7
26. Ducker C, Shaw PE. Ubiquitin-mediated control of ETS transcription factors: Roles in cancer and development. Int J Mol Sci. (2021) 22:5119. doi: 10.3390/ijms22105119
27. Gerak CAN, Zhang SM, Balgi AD, Sadowski IJ, Sessions RB, McIntosh LP, et al. A multipronged screening approach targeting inhibition of ETV6 PNT domain polymerization. SLAS Discovery. (2021) 26:698–711. doi: 10.1177/2472555220979599
28. Lapinskas EJ, Svobodova S, Davis ID, Cebon J, Hertzog PJ, Pritchard MA. The Ets transcription factor ELF5 functions as a tumor suppressor in the kidney. Twin Res Hum Genet. (2011) 14:316–22. doi: 10.1375/twin.14.4.316
29. de Ruyck J, Brysbaert G, Villeret V, Aumercier M, Lensink MF. Computational characterization of the binding mode between oncoprotein Ets-1 and DNA-repair enzymes. Proteins. (2018) 86:1055–63. doi: 10.1002/prot.v86.10
30. Al-Hawary SIS, Pallathadka H, Hjazi A, Zhumanov ZE, Alazbjee AAA, Imad S, et al. ETS transcription factor ELK3 in human cancers: An emerging therapeutic target. Pathol Res Pract. (2023) 248:154728. doi: 10.1016/j.prp.2023.154728
31. Desjardins G, Okon M, Graves BJ, McIntosh LP. Conformational dynamics and the binding of specific and nonspecific DNA by the autoinhibited transcription factor Ets-1. Biochemistry. (2016) 55:4105–18. doi: 10.1021/acs.biochem.6b00460
32. Kamberaj H, van der Vaart A. Correlated motions and interactions at the onset of the DNA-induced partial unfolding of Ets-1. Biophys J. (2009) 96:1307–17. doi: 10.1016/j.bpj.2008.11.019
33. Esaki S, Evich MG, Erlitzki N, Germann MW, Poon GMK. Multiple DNA-binding modes for the ETS family transcription factor PU.1. J Biol Chem. (2017) 292:16044–54. doi: 10.1074/jbc.M117.798207
34. Wang S, Linde MH, Munde M, Carvalho VD, Wilson WD, Poon GMK. Mechanistic heterogeneity in site recognition by the structurally homologous DNA-binding domains of the ETS family transcription factors Ets-1 and PU.1. J Biol Chem. (2014) 289:21605–16. doi: 10.1074/jbc.M114.575340
35. Leight ER, Murphy JT, Fantz DA, Pepin D, Schneider DL, Ratliff TM, et al. Conversion of the LIN-1 ETS protein of Caenorhabditis elegans from a SUMOylated transcriptional repressor to a phosphorylated transcriptional activator. Genetics. (2015) 199:761–75. doi: 10.1534/genetics.114.172668
36. Nelson ML, Kang HS, Lee GM, Blaszczak AG, Lau DKW, McIntosh LP, et al. Ras signaling requires dynamic properties of Ets1 for phosphorylation-enhanced binding to coactivator CBP. Proc Natl Acad Sci U S A. (2010) 107:10026–31. doi: 10.1073/pnas.0915137107
37. Yang YX, Han X, Sun LJ, Shao FY, Yin Y, Zhang WZ. ETS transcription factors in immune cells and immune-related diseases. Int J Mol Sci. (2024) 25:10004. doi: 10.3390/ijms251810004
38. Mut M, Lule S, Demir O, Kurnaz IA, Vural I. Both mitogen-activated protein kinase (MAPK)/extracellular-signal-regulated kinases (ERK) 1/2 and phosphatidylinositide-3-OH kinase (PI3K)/Akt pathways regulate activation of E-twenty-six (ETS)-like transcription factor 1 (Elk-1) in U138 glioblastoma cells. Int J Biochem Cell Biol. (2012) 44:302–10. doi: 10.1016/j.biocel.2011.10.025
39. Znosko WA, Yu SB, Thomas K, Molina GA, Li CJ, Tsang W, et al. Overlapping functions of Pea3 ETS transcription factors in FGF signaling during zebrafish development. Dev Biol. (2010) 342:11–25. doi: 10.1016/j.ydbio.2010.03.011
40. Holterman CE, Franovic A, Payette J, Lee S. ETS-1 oncogenic activity mediated by transforming growth factor alpha. Cancer Res. (2010) 70:730–40. doi: 10.1158/0008-5472.CAN-09-2090
41. Chen HM, Chen WH, Zhang XJ, Hu L, Tang GJ, Kong J, et al. E26 transformation (ETS)−specific related transcription factor−3 (ELF3) orchestrates a positive feedback loop that constitutively activates the MAPK/Erk pathway to drive thyroid cancer. Oncol Rep. (2019) 41:570–8. doi: 10.3892/or.2018.6807
42. Gu WF, Qi GT, Chen L. SPIB knockdown inhibits the immune escape of ovarian cancer cells by reducing PD-L1 (CD274) expression and inactivating the JAK/STAT pathway. Iran J Immunol. (2023) 20:335–47. doi: 10.22034/iji.2023.98236.2559
43. Wu M, Liu XG, Jin WH, Li YB, Li Y, Hu QL, et al. Targeting ETS1 with RNAi-based supramolecular nanoassemblies for multidrug-resistant breast cancer therapy. J Control Release. (2017) 253:110–21. doi: 10.1016/j.jconrel.2017.03.011
44. Tang JF, Li YL, Liu BX, Liang W, Hu SB, Shi ML, et al. Uncovering a key role of ETS1 on vascular abnormality in glioblastoma. Pathol Oncol Res. (2021) 27:1609997. doi: 10.3389/pore.2021.1609997
45. Cao YY, Guo AN, Li MX, Ma XH, Bian XF, Chen YR, et al. ETS1 deficiency in macrophages suppresses colorectal cancer progression by reducing the F4/80+TIM4+ macrophage population. Carcinogenesis. (2024) 45:745–58. doi: 10.1093/carcin/bgae058
46. Yang XQ, Zhang YY, Fan HY. Downregulation of SBF2-AS1 functions as a tumor suppressor in clear cell renal cell carcinoma by inhibiting miR-338-3p-targeted ETS1. Cancer Gene Ther. (2021) 28:813–27. doi: 10.1038/s41417-020-0197-4
47. Tomar S, Plotnik JP, Haley J, Scantland J, Dasari S, Sheikh Z, et al. ETS1 induction by the microenvironment promotes ovarian cancer metastasis through focal adhesion kinase. Cancer Lett. (2018) 414:190–204. doi: 10.1016/j.canlet.2017.11.012
48. Tomihara H, Yamada D, Eguchi H, Iwagami Y, Noda T, Asaoka T, et al. MicroRNA-181b-5p, ETS1, and the c-Met pathway exacerbate the prognosis of pancreatic ductal adenocarcinoma after radiation therapy. Cancer Sci. (2017) 108:398–407. doi: 10.1111/cas.2017.108.issue-3
49. Ning ML, Qin SJ, Tian J, Wang YC, Liu QY. LncRNA AFAP-AS1 promotes anaplastic thyroid cancer progression by sponging miR-155-5p through ETS1/ERK pathway. Bioengineered. (2021) 12:1543–54. doi: 10.1080/21655979.2021.1918537
50. Guo JR, He KY, Yuan JL, An W, Yin WT, Li QT, et al. HMGA1 sensitizes esophageal squamous cell carcinoma to mTOR inhibitors through the ETS1-FKBP12 axis. Int J Biol Sci. (2024) 20:2640–57. doi: 10.7150/ijbs.95595
51. Cao B, Tan S, Tang HJ, Chen YH, Shu P. miR−512−5p suppresses proliferation, migration and invasion, and induces apoptosis in non−small cell lung cancer cells by targeting ETS1. Mol Med Rep. (2019) 19:3604–14. doi: 10.3892/mmr.2019.10022
52. Gan ai Wang HB, Chu F, Li ZJ, Bi Q, Li LX, Zhuang YL, et al. MTBP enhances the activation of transcription factor ETS-1 and promotes the proliferation of hepatocellular carcinoma cells. Front Oncol. (2022) 12:985082. doi: 10.3389/fonc.2022.985082
53. Zhu JL, Xu CX, Ruan LM, Wu JP, Li Y, Zhang XG. MicroRNA-146b overexpression promotes human bladder cancer invasion via enhancing ETS2-mediated mmp2 mRNA transcription. Mol Ther Nucleic Acids. (2019) 16:531–42. doi: 10.1016/j.omtn.2019.04.007
54. Liu XJ, Zhang CQ, Zhang ZH, Zhang ZP, Ji W, Cao SD, et al. E26 transformation-specific transcription factor ETS2 as an oncogene promotes the progression of hypopharyngeal cancer. Cancer Biother Radiopharm. (2017) 32:327–34. doi: 10.1089/cbr.2017.2296
55. Torres A, Alshalalfa M, Davicioni E, Gupta A, Yegnasubramanian S, Wheelan SJ, et al. ETS2 is a prostate basal cell marker and is highly expressed in prostate cancers aberrantly expressing p63. Prostate. (2018) 78:896–904. doi: 10.1002/pros.v78.12
56. Das L, Kokate SB, Rath S, Rout N, Singh SP, Crowe SE, et al. ETS2 and Twist1 promote invasiveness of Helicobacter pylori-infected gastric cancer cells by inducing Siah2. Biochem J. (2016) 473:1629–40. doi: 10.1042/BCJ20160187
57. Munera J, Cecena G, Jedlicka P, Wankell M, Oshima RG. Ets2 regulates colonic stem cells and sensitivity to tumorigenesis. Stem Cells. (2011) 29:430–9. doi: 10.1002/stem.599
58. Vazquez-Cabrera G, Skandik M, Roncier N, Real Oualit F, Cruz De Los Santos M, Baleviciute A, et al. ID2-ETS2 axis regulates the transcriptional acquisition of pro-tumoral microglia phenotype in glioma. Cell Death Dis. (2024) 15:512. doi: 10.1038/s41419-024-06903-3
59. Zhang ZH, Chen F, Li S, Guo HY, Xi HL, Deng J, et al. ERG the modulates Warburg effect and tumor progression in cervical cancer. Biochem Biophys Res Commun. (2020) 522:191–7. doi: 10.1016/j.bbrc.2019.11.079
60. Hashmi AA, Khan EY, Irfan M, Ali R, Asif H, Naeem M, et al. ERG oncoprotein expression in prostatic acinar adenocarcinoma; clinicopathologic significance. BMC Res Notes. (2019) 12:35. doi: 10.1186/s13104-019-4090-x
61. Sun ZP, Tan ZG, Peng C, Yi WM. LncRNA SNHG3 facilitates the Malignant phenotype of cholangiocarcinoma cells via the miR-3173–5p/ERG axis. J Gastrointest Surg. (2022) 26:802–12. doi: 10.1007/s11605-021-05160-5
62. Wang WL, Patel NR, Caragea M, Hogendoorn PCW, Lopez-Terrada D, Hornick JL, et al. Expression of ERG, an Ets family transcription factor, identifies ERG-rearranged Ewing sarcoma. Mod Pathol. (2012) 25:1378–83. doi: 10.1038/modpathol.2012.97
63. Rahim S, Beauchamp EM, Kong Y, Brown ML, Toretsky JA, Üren A. YK-4-279 inhibits ERG and ETV1 mediated prostate cancer cell invasion. PloS One. (2011) 6:e19343. doi: 10.1371/journal.pone.0019343
64. Sashida G, Bazzoli E, Menendez S, Stephen DN. The oncogenic role of the ETS transcription factors MEF and ERG. Cell Cycle. (2014) 9:3457–9. doi: 10.4161/cc.9.17.13000
65. Guneri-Sozeri PY, Ozden-Yilmaz G, Kisim A, Cakiroglu E, Eray A, Uzuner H, et al. FLI1 and FRA1 transcription factors drive the transcriptional regulatory networks characterizing muscle invasive bladder cancer. Commun Biol. (2023) 6:199. doi: 10.1038/s42003-023-04561-3
66. Chen NF, Zhao G, Yan X, Lv Z, Yin HM, Zhang SL, et al. A novel FLI1 exonic circular RNA promotes metastasis in breast cancer by coordinately regulating TET1 and DNMT1. Genome Biol. (2018) 19:218. doi: 10.1186/s13059-018-1594-y
67. Hughes CJ, Fields KM, Danis EP, Hsu JY, Neelakantan D, Vincent MY, et al. SIX1 and EWS/FLI1 co-regulate an anti-metastatic gene network in Ewing Sarcoma. Nat Commun. (2023) 14:4357. doi: 10.1038/s41467-023-39945-w
68. Chen E, Huang JJ, Chen M, Wu JW, Ouyang PY, Wang XN, et al. FLI1 regulates radiotherapy resistance in nasopharyngeal carcinoma through TIE1-mediated PI3K/AKT signaling pathway. J Transl Med. (2023) 21:134. doi: 10.1186/s12967-023-03986-y
69. Zhang X, Yang LS, Liu JN, Wang TL, Wang Z, Liu C. FEV-mediated WNT2 transcription is involved in the progression of colorectal cancer via the Wnt signaling. Cytotechnology. (2024) 76:683–96. doi: 10.1007/s10616-024-00643-0
70. Anderson PM, Manning LM, Rubin B, Chan TA, Kilpatrick SE, Hanna R, et al. Nested set information derived from fusion genes in Ewing sarcoma and other cancers. J Clin Oncol. (2022) 40:e23521. doi: 10.1200/JCO.2022.40.16_suppl.e23521
71. Cannon-Albright LA, Teerlink CC, Stevens J, Huang FW, Sipeky C, Schleutker J, et al. A rare variant in ERF (rs144812092) predisposes to prostate and bladder cancers in an extended pedigree. Cancers (Basel). (2021) 13:2399. doi: 10.3390/cancers13102399
72. Jomrich G, Maroske F, Stieger J, Preusser M, Ilhan-Mutlu A, Winkler D, et al. MK2 and ETV1 are prognostic factors in esophageal adenocarcinomas. J Cancer. (2018) 9:460–8. doi: 10.7150/jca.22310
73. Oh S, Shin S, Song H, Grande JP, Janknecht R. Relationship between ETS transcription factor ETV1 and TGF-beta-regulated SMAD proteins in prostate cancer. Sci Rep. (2019) 9:8186. doi: 10.1038/s41598-019-44685-3
74. Lin X, Zhi Y. CircPRELID2 functions as a promoter of renal cell carcinoma through the miR-22-3p/ETV1 cascade. BMC Urol. (2024) 24:104. doi: 10.1186/s12894-024-01490-z
75. Oh S, Shin S, Janknecht R. ETV1, 4 and 5: An oncogenic subfamily of ETS transcription factors. Biochim Biophys Acta. (2012) 1826:1–12. doi: 10.1016/j.bbcan.2012.02.002
76. Xu XL, Wang BS, Liu Y, Jing TT, Xu GQ, Zhang L, et al. ETV4 potentiates nuclear YAP retention and activities to enhance the progression of hepatocellular carcinoma. Cancer Lett. (2022) 537:215640. doi: 10.1016/j.canlet.2022.215640
77. Su HM, Shu SH, Tang WQ, Zheng CQ, Zhao LY, Fan H. ETV4 facilitates angiogenesis in hepatocellular carcinoma by upregulating MMP14 expression. Biochem Biophys Res Commun. (2023) 684:149137. doi: 10.1016/j.bbrc.2023.149137
78. Rodriguez AC, Vahrenkamp JM, Berrett KC, Clark KA, Guillen KP, Scherer SD, et al. ETV4 is necessary for estrogen signaling and growth in endometrial cancer cells. Cancer Res. (2020) 80:1234–45. doi: 10.1158/0008-5472.CAN-19-1382
79. Sun MC, Fang K, Li ZX, Chu Y, Xu AP, Zhao ZY, et al. ETV5 overexpression promotes progression of esophageal squamous cell carcinoma by upregulating SKA1 and TRPV2. Int J Med Sci. (2022) 19:1072–81. doi: 10.7150/ijms.71892
80. Meng DD, Li ZF, Ma XJ, Wu LN, Fu LJ, Qin GJ. ETV5 overexpression contributes to tumor growth and progression of thyroid cancer through PIK3CA. Life Sci. (2020) 253:117693. doi: 10.1016/j.lfs.2020.117693
81. Wang MX, Meng JW, Wang HY, Hu HJ, Hong Y. Atractylodes macrocephala III suppresses EMT in cervical cancer by regulating IGF2BP3 through ETV5. J Cell Mol Med. (2024) 28:e18081. doi: 10.1111/jcmm.18081
82. Monge M, Colas E, Doll A, Gonzalez M, Gil-Moreno A, Planaguma J, et al. ERM/ETV5 up-regulation plays a role during myometrial infiltration through matrix metalloproteinase-2 activation in endometrial cancer. Cancer Res. (2007) 67:6753–9. doi: 10.1158/0008-5472.CAN-06-4487
83. Yan QY, Lou GH, Qian Y, Qin B, Xu XP, Wang YN, et al. SPAG9 is involved in hepatocarcinoma cell migration and invasion via modulation of ELK1 expression. Onco Targets Ther. (2016) 9:1067–75. doi: 10.2147/OTT.S98727
84. Wei ST, Yu ZC, Shi R, An LF, Zhang Q, Zhang Q, et al. GPX4 suppresses ferroptosis to promote Malignant progression of endometrial carcinoma via transcriptional activation by ELK1. BMC Cancer. (2022) 22:881. doi: 10.1186/s12885-022-09986-3
85. Kong YK, Yin JJ, Fu Y, Chen YF, Zhou YH, Geng XQ. Suppression of Elk1 inhibits thyroid cancer progression by mediating PTEN expression. Oncol Rep. (2018) 40:1769–76. doi: 10.3892/or.2018.6554
86. Zhang WM, Zhang SP. Downregulation of circRNA_0000285 suppresses cervical cancer development by regulating miR197-3p–ELK1 axis. Cancer Manage Res. (2020) 12:8663–74. doi: 10.2147/CMAR.S253174
87. Xu H, Zhang L, Gao J, Wang JJ, Wang YH, Xiao DD, et al. Molecular and clinical features of a potential immunotherapy target ELK3 in glioma. Medicine. (2022) 101:e29544. doi: 10.1097/MD.0000000000029544
88. Lee JH, Hur W, Hong SW, Kim JH, Kim SM, Lee EB, et al. ELK3 promotes the migration and invasion of liver cancer stem cells by targeting HIF-1α. Oncol Rep. (2017) 37:813–22. doi: 10.3892/or.2016.5293
89. Zhang JL, Song HB, Chen C, Chen LP, Dai Y, Sun PH, et al. Methyltransferase-like protein 11A promotes migration of cervical cancer cells via up-regulating ELK3. Pharmacol Res. (2021) 172:105814. doi: 10.1016/j.phrs.2021.105814
90. Gao FX, Wang CX, Bai X, Ji JH, Huang XR. ELK4 promotes cell cycle progression and stem cell-like characteristics in HPV-associated cervical cancer by regulating the FBXO22/PTEN axis. Balkan Med J. (2023) 40:409–14. doi: 10.4274/balkanmedj.galenos.2023.2023-4-66
91. Lee HS, Jung W, Lee E, Chang H, Choi JH, Kim HG, et al. SIRT7, H3K18ac, and ELK4 immunohistochemical expression in hepatocellular carcinoma. J Pathol Transl Med. (2016) 50:337–44. doi: 10.4132/jptm.2016.05.20
92. Simmonds P, Loomis E, Curry E. DNA methylation-based chromatin compartments and ChIP-seq profiles reveal transcriptional drivers of prostate carcinogenesis. Genome Med. (2017) 9:54. doi: 10.1186/s13073-017-0443-z
93. Biswas A, Rajesh Y, Mitra P, Mandal M. ETV6 gene aberrations in non-haematological Malignancies: A review highlighting ETV6 associated fusion genes in solid tumors. Biochim Biophys Acta Rev Cancer. (2020) 1874:188389. doi: 10.1016/j.bbcan.2020.188389
94. Biswas A, Rajesh Y, Das S, Banerjee I, Kapoor N, Mitra P, et al. Therapeutic targeting of RBPJ, an upstream regulator of ETV6 gene, abrogates ETV6-NTRK3 fusion gene transformations in glioblastoma. Cancer Lett. (2022) 544:215811. doi: 10.1016/j.canlet.2022.215811
95. Meškytė EM, Pezzè L, Bartolomei L, Forcato M, Bocci IA, Bertalot G, et al. ETV7 reduces inflammatory responses in breast cancer cells by repressing the TNFR1/NF-κB axis. Cell Death Dis. (2023) 14:263. doi: 10.1038/s41419-023-05718-y
96. Liu H, Liu L, Liu Q, He FJ, Zhu H. LncRNA HOXD-AS1 affects proliferation and apoptosis of cervical cancer cells by promoting FRRS1 expression via transcription factor ELF1. Cell Cycle. (2022) 21:416–26. doi: 10.1080/15384101.2021.2020962
97. Wang L. ELF1-activated FOXD3-AS1 promotes the migration, invasion and EMT of osteosarcoma cells via sponging miR-296-5p to upregulate ZCCHC3. J Bone Oncol. (2020) 26:100335. doi: 10.1016/j.jbo.2020.100335
98. Lawrenson K, Song F, Hazelett DJ, Kar SP, Tyrer J, Phelan CM, et al. Genome-wide association studies identify susceptibility loci for epithelial ovarian cancer in east Asian women. Gynecol Oncol. (2019) 153:343–55. doi: 10.1016/j.ygyno.2019.02.023
99. Budka JA, Ferris MW, Capone MJ, Hollenhorst PC. Common ELF1 deletion in prostate cancer bolsters oncogenic ETS function, inhibits senescence and promotes docetaxel resistance. Genes Cancer. (2018) 17:198–214. doi: 10.18632/genesandcancer.v9i5-6
100. Ma XJ, Li Y. Circ_0028007 aggravates the Malignancy of nasopharyngeal carcinoma by regulating miR-656-3p/ELF2 axis. Biochem Genet. (2022) 60:2069–86. doi: 10.1007/s10528-022-10205-8
101. Li BY, Yao B, Guo XR, Wang ZY, Xie W, Wu XN, et al. c-Myc-induced long noncoding RNA MIRE cooperates with hnRNPK to stabilize ELF2 mRNA and promotes clear cell renal cell carcinogenesis. Cancer Gene Ther. (2023) 30:1215–26. doi: 10.1038/s41417-023-00631-0
102. Qi X, Chen DJ, Yu WC, Wang LM, Liu L, Tao XL. Long non-coding RNA PRNCR1 promotes ovarian cancer cell proliferation, migration and invasion by targeting the miR-653-5p/ELF2 axis. Mol Cell Biochem. (2022) 477:1463–75. doi: 10.1007/s11010-022-04371-x
103. Gong JM, Kong XD, Qi JH, Lu JK, Yuan SF, Wu M. CircRNA_104565 promoted cell proliferation in papillary thyroid carcinoma by sponging miR-134. Int J Gen Med. (2021) 14:179–85. doi: 10.2147/IJGM.S288360
104. Lu J, Zhang QQ, Mo LC, Chen WY, Mao LH. Comprehensive analysis of E47−like factors and verification of ELF4 in clear cell renal cell carcinoma. Oncol Lett. (2023) 26:395. doi: 10.3892/ol.2023.13981
105. Zhuang ZW, Zhang CY, Tan YQ, Zhang J, Zhong CL. ELF4 was a prognostic biomarker and related to immune infiltrates in glioma. J Cancer. (2024) 15:5101–17. doi: 10.7150/jca.96886
106. Xu AP, Sun MC, Li ZX, Chu Y, Fang K, Zhang YW, et al. ELF4 contributes to esophageal squamous cell carcinoma growth and metastasis by augmenting cancer stemness via FUT9. Acta Biochim Biophys Sin. (2023) 56:129–39. doi: 10.3724/abbs.2023225
107. Sze KMF, Ho DWH, Chiu YT, Tsui YM, Chan LK, Lee JMF, et al. Hepatitis B virus–telomerase reverse transcriptase promoter integration harnesses host ELF4, resulting in telomerase reverse transcriptase gene transcription in hepatocellular carcinoma. Hepatology. (2020) 73:23–40. doi: 10.1002/hep.3123
108. Yang J, Liu CJ, Guan JL, Wang Y, Su JW, Wang YX, et al. SPI1 mediates transcriptional activation of TPX2 and RNF2 to regulate the radiosensitivity of lung squamous cell carcinoma. Arch Biochem Biophys. (2022) 730:109425. doi: 10.1016/j.abb.2022.109425
109. Feng HY, Wang T, Ye JL, Yang Y, Huang X, Lai D, et al. SPI1 is a prognostic biomarker of immune infiltration and immunotherapy efficacy in clear cell renal cell carcinoma. Discovery Oncol. (2022) 13:134. doi: 10.1007/s12672-022-00592-0
110. Tao L, Wang XY, Zhou Q. Long noncoding RNA SNHG16 promotes the tumorigenicity of cervical cancer cells by recruiting transcriptional factor SPI1 to upregulate PARP9. Cell Biol Int. (2020) 44:773–84. doi: 10.1002/cbin.11272
111. Ho YJ, Lin YM, Huang YC, Yeh KT, Lin LI, Lu JW. Tissue microarray-based study of hepatocellular carcinoma validating SPIB as potential clinical prognostic marker. Acta Histochem. (2016) 118:38–45. doi: 10.1016/j.acthis.2015.11.005
112. Sun W, Wang ZY, Qin Y, Ji XY, Huang JP, Zhang F, et al. NEAT1_2/RRAD/EHF positive feedback loop facilitates aerobic glycolysis in papillary thyroid cancer cells. Endocrinology. (2023) 164:bqad085. doi: 10.1210/endocr/bqad085
113. Xu YY, Gao JW, Wang N, Zedenius J, Nilsson IL, Lui WO, et al. BRAF-induced EHF expression affects TERT in aggressive papillary thyroid cancer. J Clin Endocrinol Metab. (2024) 110(3):693–705. doi: 10.1210/clinem/dgae589
114. Luk IY, Reehorst CM, Mariadason JM. ELF3, ELF5, EHF and SPDEF transcription factors in tissue homeostasis and cancer. Molecules. (2018) 23:2191. doi: 10.3390/molecules23092191
115. Yang H, Yan HC. Expression of ELF5 in endometrial carcinoma tissues and its clinical significance. Oncol Lett. (2018) 16:3473–80. doi: 10.3892/ol.2018.9093
116. Li TS, Xu LJ, Wei Z, Zhang SM, Liu XY, Yang YZ, et al. ELF5 drives angiogenesis suppression though stabilizing WDTC1 in renal cell carcinoma. Mol Cancer. (2023) 22:184. doi: 10.1186/s12943-023-01871-2
117. Yan HC, Qiu LL, Xie XL, Yang H, Liu YL, Lin XM, et al. ELF5 in epithelial ovarian carcinoma tissues and biological behavior in ovarian carcinoma cells. Oncol Rep. (2017) 37:1412–8. doi: 10.3892/or.2017.5418
118. Wang YT, Ren XY, Li WY, Cao RY, Liu SY, Jiang LB, et al. SPDEF suppresses head and neck squamous cell carcinoma progression by transcriptionally activating NR4A1. Int J Oral Sci. (2021) 13:33. doi: 10.1038/s41368-021-00138-0
119. Lo YH, Noah TK, Chen MS, Zou W, Borras E, Vilar E, et al. SPDEF induces quiescence of colorectal cancer cells by changing the transcriptional targets of beta-catenin. Gastroenterology. (2017) 153:205–218.e8. doi: 10.1053/j.gastro.2017.03.048
120. Guo JC, Yang YJ, Guo M, Zhang JQ, Zheng JF, Liu Z. Involvement of CDK11B-mediated SPDEF ubiquitination and SPDEF-mediated microRNA-448 activation in the oncogenicity and self-renewal of hepatocellular carcinoma stem cells. Cancer Gene Ther. (2021) 28:1136–49. doi: 10.1038/s41417-020-00261-w
121. Steffan JJ, Koul S, Meacham RB, Koul HK. The transcription factor SPDEF suppresses prostate tumor metastasis. J Biol Chem. (2012) 287:29968–78. doi: 10.1074/jbc.M112.379396
122. Ye T, Feng J, Wan X, Xie D, Liu JB. Double agent: SPDEF gene with both oncogenic and tumor-suppressor functions in breast cancer. Cancer Manage Res. (2020) 12:3891–902. doi: 10.2147/CMAR.S243748
123. Garrett-Sinha LA. Review of Ets1 structure, function, and roles in immunity. Cell Mol Life Sci. (2013) 70:3375–90. doi: 10.1007/s00018-012-1243-7
124. Babayeva ND, Baranovskaya OI, Tahirov TH. Structural basis of Ets1 cooperative binding to widely separated sites on promoter DNA. PloS One. (2012) 7:e33698. doi: 10.1371/journal.pone.0033698
125. Bosselut R, Levin J, Adjadj E, Ghysdael J. A single amino-acid substitution in the Ets domain alters core DNA binding specificity of Ets1 to that of the related transcription factors Elf1 and E74. Nucleic Acids Res. (1993) 21:5184–91. doi: 10.1093/nar/21.22.5184
126. Dittmer J. The biology of the Ets1 proto-oncogene. Mol Cancer. (2003) 2:29. doi: 10.1186/1476-4598-2-29
127. Furlan A, Vercamer C, Bouali F, Damour I, Chotteau-Lelievre A, Wernert N, et al. Ets-1 controls breast cancer cell balance between invasion and growth. Int J Cancer. (2014) 135:2317–28. doi: 10.1002/ijc.v135.10
128. Zhong JJ, Ding R, Jiang HM, Li LF, Wan JL, Feng XQ, et al. Single-cell RNA sequencing reveals the molecular features of peripheral blood immune cells in children, adults and centenarians. Front Immunol. (2022) 13:1081889. doi: 10.3389/fimmu.2022.1081889
129. Zadora PK, Chumduri C, Imami K, Berger H, Mi Y, Selbach M, et al. Integrated phosphoproteome and transcriptome analysis reveals Chlamydia-induced epithelial-to-mesenchymal transition in host cells. Cell Rep. (2019) 26:1286–1302.e8. doi: 10.1016/j.celrep.2019.01.006
130. Casie Chetty S, Sumanas S. Ets1 functions partially redundantly with Etv2 to promote embryonic vasculogenesis and angiogenesis in zebrafish. Dev Biol. (2020) 465:11–22. doi: 10.1016/j.ydbio.2020.06.007
131. Yang F, Liu YF, Wang P, Wang XY, Chu MX, Wang PQ. Mutation of the ETS1 3′UTR interacts with miR-216a-3p to regulate granulosa cell apoptosis in sheep. Theriogenology. (2023) 210:133–42. doi: 10.1016/j.theriogenology.2023.07.025
132. Zhao W, Zhang SQ, Wang XY, Ma XL, Huang BR, Chen H, et al. ETS1 targets RYBP transcription to inhibit tumor cell proliferation. Biochem Biophys Res Commun. (2019) 509:810–6. doi: 10.1016/j.bbrc.2019.01.006
133. Chen JH, Fu Y, Day DS, Sun Y, Wang SY, Liang XD, et al. VEGF amplifies transcription through ETS1 acetylation to enable angiogenesis. Nat Commun. (2017) 8:383. doi: 10.1038/s41467-017-00405-x
134. Bhattacharya R, Ray Chaudhuri S, Roy SS. FGF9-induced ovarian cancer cell invasion involves VEGF-A/VEGFR2 augmentation by virtue of ETS1 upregulation and metabolic reprogramming. J Cell Biochem. (2018) 119:8174–89. doi: 10.1002/jcb.v119.10
135. Gu M, Li XD, Wu RH, Cheng X, Zhou SL, Gu XS. The transcription factor Ets1 influences axonal growth via regulation of Lcn2. Mol Neurobiol. (2024) 61:971–81. doi: 10.1007/s12035-023-03616-0
136. Taveirne S, Wahlen S, Van Loocke W, Kiekens L, Persyn E, Van Ammel E, et al. The transcription factor ETS1 is an important regulator of human NK cell development and terminal differentiati006Fn. Blood. (2020) 136:288–98. doi: 10.1182/blood.2020005204
137. Zhou XS, Zhou R, Zhou HX, Li QW, Hong JX, Meng R, et al. ETS-1 induces endothelial-like differentiation and promotes metastasis in non-small cell lung cancer. Cell Physiol Biochem. (2018) 45:1827–39. doi: 10.1159/000487874
138. Adler D, Wernert N. ETS transcription factors and prostate cancer: the role of the family prototype ETS-1 (review). Int J Oncol. (2012) 40:1748–54. doi: 10.3892/ijo.2012.1380
139. Bade-Döding C, Göttmann W, Baigger A, Farren M, Lee KP, Blasczyk R, et al. Autocrine GM-CSF transcription in the leukemic progenitor cell line KG1a is mediated by the transcription factor ETS1 and is negatively regulated through SECTM1 mediated ligation of CD7. Biochim Biophys Acta. (2014) 1840:1004–13. doi: 10.1016/j.bbagen.2013.10.043
140. Watanabe M, Itoh K, Togano T, Kadin ME, Watanabe T, Higashihara M, et al. Ets-1 activates overexpression of JunB and CD30 in Hodgkin’s lymphoma and anaplastic large-cell lymphoma. Am J Pathol. (2012) 180:831–8. doi: 10.1016/j.ajpath.2011.10.007
141. Jung HH, Lee J, Kim JH, Ryu KJ, Kang SA, Park C, et al. STAT1 and Nmi are downstream targets of Ets-1 transcription factor in MCF-7 human breast cancer cell. FEBS Lett. (2005) 579:3941–6. doi: 10.1016/j.febslet.2005.06.011
142. Mei DL, Qi YL, Xia YY, Ma J, Hu H, Ai J, et al. Microarray profile analysis identifies ETS1 as potential biomarker regulated by miR-23b and modulates TCF4 in gastric cancer. World J Surg Oncol. (2021) 19:311. doi: 10.1186/s12957-021-02417-w
143. Koessinger D, Albrecht V, Faber F, Jaehnert I, Schichor C. ETS-1 expression is hypoxia-independent in glioblastoma-derived endothelial and mesenchymal stem-like cells. Anticancer Res. (2018) 38:3347–55. doi: 10.21873/anticanres.12601
144. Shiu YT, Jaimes EA. Transcription factor ETS-1 and reactive oxygen species: Role in vascular and renal injury. Antioxid (Basel). (2018) 7:84. doi: 10.3390/antiox7070084
145. He Q, Gao H, Chang YL, Wu XH, Lin RT, Li GF, et al. ETS-1 facilitates Th1 cell-mediated mucosal inflammation in inflammatory bowel diseases through upregulating CIRBP. J Autoimmun. (2022) 132:102872. doi: 10.1016/j.jaut.2022.102872
146. Wang X, Liu T, Li Y, Ding A, Zhang C, Gu Y, et al. A splicing isoform of PD-1 promotes tumor progression as a potential immune checkpoint. Nat Commun. (2024) 15:9114. doi: 10.1038/s41467-024-53561-2
147. Macek Jilkova Z, Hilleret MN, Gerster T, Sturm N, Mercey-Ressejac M, Zarski J-P, et al. Increased intrahepatic expression of immune checkpoint molecules in autoimmune liver disease. Cells. (2021) 10:2671. doi: 10.3390/cells10102671
148. Lee CR, Lee W, Cho SK, Park SG. Characterization of multiple cytokine combinations and TGF-beta on differentiation and functions of myeloid-derived suppressor cells. Int J Mol Sci. (2018) 19:869. doi: 10.3390/ijms19030869
149. Liu M, Li S, Li MO. TGF-beta control of adaptive immune tolerance: A break from treg cells. Bioessays. (2018) 40:e1800063. doi: 10.1002/bies.201800063
150. Okano K, Hibi A, Miyaoka T, Inoue T, Sugimoto H, Tsuchiya K, et al. Inhibitory effects of the transcription factor Ets-1 on the expression of type I collagen in TGF-β1-stimulated renal epithelial cells. Mol Cell Biochem. (2012) 369:247–54. doi: 10.1007/s11010-012-1388-6
151. Lee CG, Kwon HK, Sahoo A, Hwang W, So JS, Hwang JS, et al. Interaction of Ets-1 with HDAC1 represses IL-10 expression in Th1 cells. J Immunol. (2012) 188:2244–53. doi: 10.4049/jimmunol.1101614
152. Yin ZN, Bahtiyar G, Zhang N, Liu LZ, Zhu P, Robert ME, et al. IL-10 regulates murine lupus. J Immunol. (2002) 169:2148–55. doi: 10.4049/jimmunol.169.4.2148
153. Kitamura N, Yokoyama H, Yashiro T, Nakano N, Nishiyama M, Kanada S, et al. Role of PU.1 in MHC class II expression through transcriptional regulation of class II transactivator pI in dendritic cells. J Allergy Clin Immunol. (2012) 129:814–824 e6. doi: 10.1016/j.jaci.2011.10.019
154. De Launoit Y, Audette M, Pelczar H, Plaza S, Baert J-L. The transcription of the intercellular adhesion molecule-1 is regulated by Ets transcription factors. Oncogene. (1998) 16:2065–73. doi: 10.1038/sj.onc.1201726
155. Behrens P, Rothe M, Wellmann A, Krischler J, Wernert N. The Ets-1 transcription factor is up-regulated together with MMP 1 and MMP 9 in the stroma of pre-invasive breast cancer. J Pathol. (2001) 194:43–50. doi: 10.1002/(ISSN)1096-9896
156. Naito T, Tanihata Y, Nishimura H, Tanaka T, Higuchi C, Taguchi T, et al. Expression of matrix metalloproteinase-9 associated with ets-1 proto-oncogene in rat tubulointerstitial cells. Nephrol Dial Transplant. (2005) 20:2333–48. doi: 10.1093/ndt/gfi013
157. Heo SH, Choi YJ, Ryoo HM, Cho JY. Expression profiling of ETS and MMP factors in VEGF-activated endothelial cells: role of MMP-10 in VEGF-induced angiogenesis. J Cell Physiol. (2010) 224:734–42. doi: 10.1002/jcp.v224:3
158. Guo WF, Gao XN, Zhan RH, Zhao ZT, Xu KH, Tang B. Tricolor imaging of MMPs to investigate the promoting roles of inflammation on invasion and migration of tumor cells. Talanta. (2021) 222:121525. doi: 10.1016/j.talanta.2020.121525
159. Okuducu AF, Zils U, Michaelis SAM, Mawrin C, von Deimling A. Increased expression of avian erythroblastosis virus E26 oncogene homolog 1 in World Health Organization grade 1 meningiomas is associated with an elevated risk of recurrence and is correlated with the expression of its target genes matrix metalloproteinase-2 and MMP-9. Cancer. (2006) 107:1365–72. doi: 10.1002/cncr.v107:6
160. Shan L, Wang FL, Zhai DD, Meng XY, Liu JJ, Lv XW. Matrix metalloproteinases induce extracellular matrix degradation through various pathways to alleviate hepatic fibrosis. Biomed Pharmacother. (2023) 161:114472. doi: 10.1016/j.biopha.2023.114472
161. Berg G, Barchuk M, Miksztowicz V. Behavior of metalloproteinases in adipose tissue, liver and arterial wall: An update of extracellular matrix remodeling. Cells. (2019) 8:158. doi: 10.3390/cells8020158
162. Lv D, Fei YJ, Chen HL, Wang JF, Han WW, Cui BM, et al. Crosstalk between T lymphocyte and extracellular matrix in tumor microenvironment. Front Immunol. (2024) 15:1340702. doi: 10.3389/fimmu.2024.1340702
163. Nazemi M, Rainero E. Cross-talk between the tumor microenvironment, extracellular matrix, and cell metabolism in cancer. Front Oncol. (2020) 10:239. doi: 10.3389/fonc.2020.00239
164. Du W, Xia XM, Hu F, Yu JY. Extracellular matrix remodeling in the tumor immunity. Front Immunol. (2024) 14:1340634. doi: 10.3389/fimmu.2023.1340634
165. Morales A, Morimoto S, Vilchis F, Taniyama N, Bautista CJ, Robles C, et al. Molecular expression of vascular endothelial growth factor, prokineticin receptor-1 and other biomarkers in infiltrating canalicular carcinoma of the breast. Oncol Lett. (2016) 12(4):2720–7. doi: 10.3892/ol.2016.4961
166. Peng Y, Li HT, Wu MH, Wang XY, Fan SQ, Liu F, et al. NGX6 inhibits AP-1 and Ets-1 expression and down-regulates cyclin D1 in human colorectal cancer. Acta Biochim Biophys Sin (Shanghai). (2009) 41:504–14. doi: 10.1093/abbs/gmp039
167. Cheng JQ, Kabbout M, Dakhlallah D, Sharma S, Bronisz A, Srinivasan R, et al. MicroRNA 17-92 cluster mediates ETS1 and ETS2-dependent RAS-oncogenic transformation. PloS One. (2014) 9(6):e100693. doi: 10.1371/journal.pone.0100693
168. Wang CF, Yang Y, Zhang G, Li JX, Wu XN, Ma XL, et al. Long noncoding RNA EMS connects c-Myc to cell cycle control and tumorigenesis. Proc Natl Acad Sci U S A. (2019) 116:14620–9. doi: 10.1073/pnas.1903432116
169. Siraj AK, Parvathareddy SK, Annaiyappanaidu P, Ahmed SO, Siraj N, Tulbah A, et al. High expression of cyclin D1 is an independent marker for favorable prognosis in middle eastern breast cancer. Onco Targets Ther. (2021) 14:3309–18. doi: 10.2147/OTT.S309091
170. Ito M, Nakayama T, Naito S, Matsuu M, Shichijo K, Sekine I. Expression of Ets-1 transcription factor in relation to angiogenesis in the healing process of gastric ulcer. Biochem Biophys Res Commun. (1998) 246:123–7. doi: 10.1006/bbrc.1998.8585
171. Taniguchi H, Fujiwara Y, Doki Y, Sugita Y, Sohma I, Miyata H, et al. Gene therapy using ets-1 transcription factor decoy for peritoneal dissemination of gastric cancer. Int J Cancer. (2007) 121:1609–17. doi: 10.1002/ijc.v121:7
172. Wai PY, Mi ZY, Gao CJ, Guo HT, Marroquin C, Kuo PC. Ets-1 and runx2 regulate transcription of a metastatic gene, osteopontin, in murine colorectal cancer cells. J Biol Chem. (2006) 281:18973–82. doi: 10.1074/jbc.M511962200
173. Motalebzadeh J, Shabani S, Rezayati S, Shakournia N, Mirzaei R, Mahjoubi B, et al. Prognostic value of FBXO39 and ETS-1 but not BMI-1 in Iranian colorectal cancer patients. Asian Pac J Cancer Prev. (2018) 19:1357–62. doi: 10.22034/apjcp.2018.19.5.1357
174. Bellovin DI, Simpson KJ, Danilov T, Maynard E, Rimm DL, Oettgen P, et al. Reciprocal regulation of RhoA and RhoC characterizes the EMT and identifies RhoC as a prognostic marker of colon carcinoma. Oncogene. (2006) 25:6959–67. doi: 10.1038/sj.onc.1209682
175. Alipov G, Nakayama T, Ito M, Kawai K, Naito S, Nakashima M, et al. Overexpression of Ets-1 proto-oncogene in latent and clinical prostatic carcinomas. Histopathology. (2005) 46:202–8. doi: 10.1111/j.1365-2559.2005.02059.x
176. Massie CE, Adryan B, Barbosa-Morais NL, Lynch AG, Tran MG, Neal DE, et al. New androgen receptor genomic targets show an interaction with the ETS1 transcription factor. EMBO Rep. (2007) 8:871–8. doi: 10.1038/sj.embor.7401046
177. Li LR, Miao XJ, Ni RZ, Miao XB, Wang L, Gu XD, et al. Epithelial-specific ETS-1 (ESE1/ELF3) regulates apoptosis of intestinal epithelial cells in ulcerative colitis via accelerating NF-κB activation. Immunol Res. (2015) 62:198–212. doi: 10.1007/s12026-015-8651-3
178. Millien G, Cao Y, O’Hara CJ, Tagne J-B, Hinds A, Williams MC, et al. ETS1 regulates Twist1 transcription in a KrasG12D/Lkb1–/– metastatic lung tumor model of non-small cell lung cancer. Clin Exp Met Astasis. (2018) 35:149–65. doi: 10.1007/s10585-018-9912-z
179. Desterke C, Voldoire M, Sorel N, Bonnet ML, Pagliaro S, Bennaceur-Griscelli A, et al. ETS1 expression is upregulated in chronic myeloid leukemia (CML) and the ETS1 transcriptional program correlates with disease progression towards blast crisis. Blood. (2016) 128:442–2. doi: 10.1182/blood.V128.22.442.442
180. Priebe V, Sartori G, Napoli S, Chung EYL, Cascione L, Kwee I, et al. Role of ETS1 in the transcriptional network of diffuse large B cell lymphoma of the activated B cell-like type. Cancers. (2020) 12:1912. doi: 10.3390/cancers12071912
181. Lchtel RA, Zhao Y, Aggarwal RK, Pradhan K, Maqbool SB. ETS1 is a novel transcriptional regulator of adult T-cell leukemia/lymphoma of North American descent. Blood Adv. (2022) 6:5613–24. doi: 10.1182/bloodadvances.2022007725
182. Liang XH, Nichols JG, De Hoyos CL, Crooke ST. Some ASOs that bind in the coding region of mRNAs and induce RNase H1 cleavage can cause increases in the pre-mRNAs that may blunt total activity. Nucleic Acids Res. (2020) 48:9840–58. doi: 10.1093/nar/gkaa715
183. Hörberg J, Carlesso A, Reymer A. Mechanistic insights into ASO-RNA complexation: Advancing antisense oligonucleotide design strategies. Mol Ther Nucleic Acids. (2024) 35:102351. doi: 10.1016/j.omtn.2024.102351
184. Szlachta K, Kuscu C, Tufan T, Adair SJ, Shang S, Michaels AD, et al. CRISPR knockout screening identifies combinatorial drug targets in pancreatic cancer and models cellular drug response. Nat Commun. (2018) 9:4275. doi: 10.1038/s41467-018-06676-2
185. Pagotto S, Simeone P, Brocco D, Catitti G, De Bellis D, Vespa S, et al. CAR-T-derived extracellular vesicles: A promising development of CAR-T anti-tumor therapy. Cancers (Basel). (2023) 15:1052. doi: 10.3390/cancers15041052
186. Lu JR, Jiang G. The journey of CAR-T therapy in hematological Malignancies. Mol Cancer. (2022) 21:194. doi: 10.1186/s12943-022-01663-0
187. Safarzadeh Kozani P, Safarzadeh Kozani P, Rahbarizadeh F. CAR-T cell therapy in T-cell Malignancies: Is success a low-hanging fruit. Stem Cell Res Ther. (2021) 12:527. doi: 10.1186/s13287-021-02595-0
188. Bai Z, Feng B, McClory SE, de Oliveira BC, Diorio C, Gregoire C, et al. Single-cell CAR T atlas reveals type 2 function in 8-year leukaemia remission. Nature. (2024) 634:702–11. doi: 10.1038/s41586-024-07762-w
189. Brudno JN, Maus MV, Hinrichs CS. CAR T cells and T-cell therapies for cancer: A translational science review. JAMA. (2024) 332:1924–35. doi: 10.1001/jama.2024.19462
Keywords: ETS family, tumor immunology, ETS-1, biological functions, immune microenvironment, immunotherapy targets
Citation: Wang SY, Wan L, Zhang XJ, Fang HX, Zhang MY, Li F and Yan DW (2025) ETS-1 in tumor immunology: implications for novel anti-cancer strategies. Front. Immunol. 16:1526368. doi: 10.3389/fimmu.2025.1526368
Received: 11 November 2024; Accepted: 03 March 2025;
Published: 20 March 2025.
Edited by:
Wenxue Ma, University of California, San Diego, United StatesReviewed by:
Farah Alfatyan, MUSC Health, United StatesCopyright © 2025 Wang, Wan, Zhang, Fang, Zhang, Li and Yan. This is an open-access article distributed under the terms of the Creative Commons Attribution License (CC BY). The use, distribution or reproduction in other forums is permitted, provided the original author(s) and the copyright owner(s) are credited and that the original publication in this journal is cited, in accordance with accepted academic practice. No use, distribution or reproduction is permitted which does not comply with these terms.
*Correspondence: Lei Wan, eXh3YW5sZWlAMTYzLmNvbQ==
Disclaimer: All claims expressed in this article are solely those of the authors and do not necessarily represent those of their affiliated organizations, or those of the publisher, the editors and the reviewers. Any product that may be evaluated in this article or claim that may be made by its manufacturer is not guaranteed or endorsed by the publisher.
Research integrity at Frontiers
Learn more about the work of our research integrity team to safeguard the quality of each article we publish.