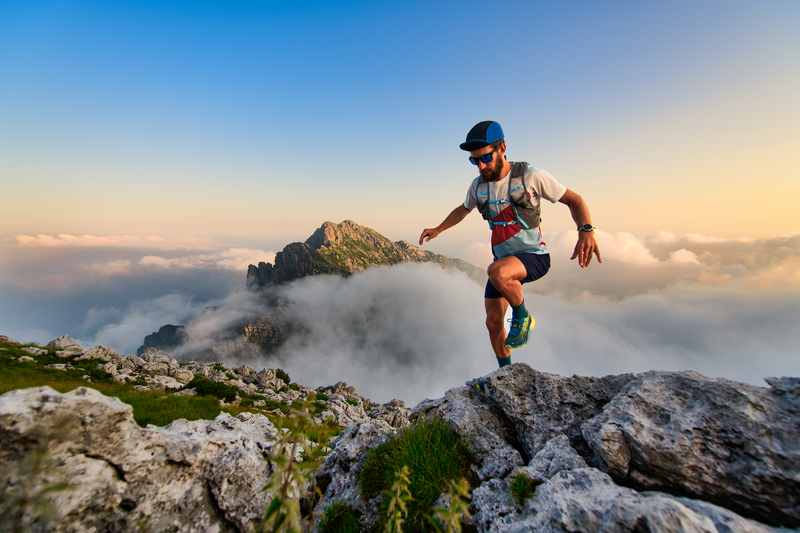
95% of researchers rate our articles as excellent or good
Learn more about the work of our research integrity team to safeguard the quality of each article we publish.
Find out more
REVIEW article
Front. Immunol. , 22 January 2025
Sec. Cancer Immunity and Immunotherapy
Volume 16 - 2025 | https://doi.org/10.3389/fimmu.2025.1518555
This article is part of the Research Topic Cancer Immunity and Radiotherapy View all 6 articles
Molecular imaging technologies have significantly transformed cancer research and clinical practice, offering valuable tools for visualizing and understanding the complex tumor immune microenvironment. These technologies allow for the non-invasive examination of key components within the tumor immune microenvironment, including immune cells, cytokines, and stromal cells, providing crucial insights into tumor biology and treatment responses. This paper reviews the latest advancements in molecular imaging, with a focus on its applications in assessing interactions within the tumor immune microenvironment. Additionally, the challenges faced by molecular imaging technologies are discussed, such as the need for highly sensitive and specific imaging agents, issues with data integration, and difficulties in clinical translation. The future outlook emphasizes the potential of molecular imaging to enhance personalized cancer treatment through the integration of artificial intelligence and the development of novel imaging probes. Addressing these challenges is essential to fully realizing the potential of molecular imaging in improving cancer diagnosis, treatment, and patient outcomes.
Tumor immunotherapy has transformed the way cancer is treated by utilizing the immune system’s capacity to identify and destroy cancerous cells. Immunotherapy functions by enhancing or reinstating the immune system’s capacity to combat cancer, in contrast to conventional treatments that specifically target cancer cells (1). This strategy encompasses multiple approaches, including cancer vaccines, adoptive cell transfer, and immune checkpoint inhibitors, each of which offers a distinct mechanism to improve anti-tumor immunity (2, 3). By preventing the inhibitory mechanisms that cancer cells employ to avoid immune detection, immune checkpoint inhibitors, such as PD-1/PD-L1 and CTLA-4 inhibitors, have shown extraordinary success in treating a variety of malignancies (4, 5). Patients with cancers that were previously incurable now have far higher survival rates thanks to these treatments. Furthermore, the efficiency of immunotherapy has been further increased by developments in biomaterials, such as nano-delivery systems, which improve the targeted distribution of therapeutic drugs, lowering systemic toxicity and enhancing patient outcomes (6). By combining these cutting-edge techniques, cancer treatment is being stretched to new heights, with the prospect of better patient outcomes and sustained remission. Tumor immunotherapy has the potential to revolutionize cancer treatment by offering new, less hazardous, and more efficient treatment choices as research into it advances.
Molecular imaging has the potential to revolutionize medical diagnosis and research by providing a means of seeing, characterizing, and quantifying biological processes occurring at the molecular and cellular levels in live creatures (7). In contrast to conventional imaging methods, molecular imaging offers valuable insights into the fundamental mechanisms of diseases, hence promoting timely identification, accurate diagnosis, and the assessment of therapeutic effectiveness. The creation of innovative molecular imaging methods and probes is one of the major developments in this area (8). Comparably, immune cell dynamics in cancer patients have been visualized by PET imaging with new radiotracers, which has aided in the noninvasive evaluation of treatment responses (9). Furthermore, the use of 124I-labeled antibodies has demonstrated potential in focusing on particular immune cells for PET imaging, allowing for a thorough view of activated T cells in vivo (10). These developments highlight the critical role molecular imaging plays in expanding our knowledge of disease mechanisms, increasing the precision of diagnostics, and developing individualized treatment plans.
Targeted tumor immunotherapy is advancing due to the incorporation of new molecular imaging agents and methodologies. These developments improve the accuracy and efficacy of therapeutic interventions and deepen our understanding of tumor-immune interactions. With enhanced monitoring of treatment responses, more precise diagnoses, and the creation of customized immunotherapy plans, molecular imaging technologies have the potential to revolutionize cancer treatment. This article will review the development of molecular imaging techniques in recent years, the role of molecular imaging in the tumor immune microenvironment, and its application in tumor immunotherapy. Additionally, it explores the challenges and future development trends of molecular imaging technology.
Optical imaging has become an essential tool in molecular imaging, providing a fine-grained understanding of biological processes at the molecular and cellular levels. This technology encompasses various techniques, including optical coherence tomography, bioluminescence imaging, and fluorescence imaging, each offering unique advantages for research and therapeutic applications. Fluorescence and bioluminescence imaging have garnered significant attention due to their increased sensitivity, non-radiative nature, and cost-effectiveness compared to traditional imaging modalities. These methods enable real-time monitoring of biological processes in living organisms, making them indispensable for tumor research and drug development (11). For instance, fluorescence molecular tomography (FMT) and bioluminescence tomography (BLT) facilitate three-dimensional imaging of molecular and cellular processes in vivo, enhancing our understanding of tumor biology and treatment efficacy (12). The diagnostic potential of optical imaging is enhanced when combined with other imaging modalities, such as nuclear medicine. Hybrid systems that integrate optical and nuclear imaging techniques, such as photonic-based nuclear medicine detectors and Cerenkov luminescence imaging, provide comprehensive insights into the molecular foundations of diseases (13). The resolution and sensitivity of optical imaging systems have also improved in recent years. High-resolution imaging techniques, such as optical coherence tomography and reflectance confocal microscopy, have significantly enhanced our ability to identify and diagnose diseases at the cellular and molecular levels (14).
The characterization and monitoring of various molecular events in vivo have been facilitated by the development of novel fluorescent protein-based genetically encoded probes and lipoprotein-based nanocarriers, aiding in the early diagnosis of tumors and the development of new drugs (15). Tansi et al. highlights the use of bispecific liposomes that target the tumor microenvironment, enabling effective tumor imaging and therapeutic applications. These liposomes, which target both fibroblast activation protein (FAP) and endoglin, facilitate enhanced tumor detection and selective drug delivery (16). Similarly, Li et al. developed engineered near-infrared fluorescent protein assemblies that improve both in vivo imaging and drug delivery in cancer treatment (17). Optical imaging enhances molecular imaging by matching the sensitivity performance of nuclear medicine techniques, particularly in visualizing biomarker expression levels (18). Additionally, near-infrared (NIR) optical imaging enables the noninvasive assessment of significant molecular events in vivo, including the imaging of cell surface receptors, proteases, and apoptosis in conditions such as rheumatoid arthritis, cancer, and cardiovascular disease (19). Optical imaging is a highly effective modality in molecular imaging due to its versatility, sensitivity, and high resolution. It continues to push the boundaries of clinical diagnostics and biomedical research, providing in-depth, up-to-date understanding of the intricate molecular mechanisms underpinning both health and disease.
Magnetic Resonance Imaging (MRI) is a non-invasive imaging technique that utilizes strong magnetic fields and radiofrequency waves to produce detailed images of internal body structures. It excels in differentiating various types of soft tissues, making it particularly valuable in neurological, musculoskeletal, cardiovascular, and oncological imaging. MRI operates by exploiting the magnetic properties of hydrogen atoms, which are abundant in the human body. When placed in a strong magnetic field, the nuclei of hydrogen atoms align with the field (19). Radiofrequency pulses are then used to disturb this alignment, and as the nuclei return to their original state, they emit signals that are detected and used to construct images. The resulting images have high spatial resolution and excellent contrast between different types of soft tissues. One of the significant advancements in MRI is the development of advanced imaging modalities such as multi-parametric MRI sequences, diffusion tensor imaging (DTI), and functional MRI (fMRI). These techniques allow for comprehensive characterization of complex conditions like glioblastomas, providing critical insights into tumor microenvironments and aiding in personalized therapy approaches (20). Additionally, fMRI and tractography are increasingly used to map brain functions and critical pathways, minimizing postsurgical neuro-deficits. MRI perfusion imaging has emerged as a powerful tool in assessing tumor microvascular structure and physiology. Techniques such as dynamic susceptibility contrast (DSC) and dynamic contrast enhancement (DCE) MRI provide quantitative imaging of tumor perfusion, which is crucial for treatment planning and monitoring (21). Hybrid imaging systems that combine MRI with PET or CT offer comprehensive diagnostic capabilities by providing both anatomical and functional information. This integration is particularly beneficial in oncology, where detailed imaging of tumor metabolism and structure is required for accurate diagnosis and treatment planning (22).Due to its unique imaging capabilities, MRI is extensively used in diagnosing and managing neurological, cardiovascular, and musculoskeletal diseases, as well as in oncology. It provides critical insights into the complex biological processes underlying various diseases.
Single Photon Emission Computed Tomography (SPECT) is an advanced nuclear imaging technology that provides three-dimensional images of functional processes within the body. By utilizing gamma-emitting radioisotopes and gamma cameras, SPECT offers valuable insights into various physiological and pathological conditions (23). When gamma rays are emitted from within the patient, they are detected by gamma cameras rotating around the patient. The collected data are then reconstructed into three-dimensional images, providing detailed information about the distribution and concentration of the radiopharmaceutical within the body. This enables high-specificity visualization of functional processes such as blood flow, metabolism, and receptor binding. SPECT/CT imaging combines the functional information provided by SPECT with the anatomical details from CT, resulting in more accurate localization and characterization of lesions (24). This hybrid imaging modality is particularly valuable in tumor imaging, lymphoma staging, and dosimetry calculations for radiotherapy (25). Additionally, SPECT has found applications in cardiovascular imaging, infection and inflammation imaging, and cerebral vasculitis. The combination of SPECT with CT, along with the development of new imaging techniques and radiopharmaceuticals, has enhanced its accuracy and utility across various medical fields, including oncology, neurology, and cardiology.
Positron Emission Tomography (PET) operates by detecting gamma rays indirectly emitted by positron-emitting radiotracers, the most commonly used being fluorodeoxyglucose (FDG), a glucose analog. When the radiotracer decays, it emits positrons that collide with electrons, resulting in the emission of gamma photons. These photons are detected by the PET scanner, which reconstructs the data into three-dimensional images, providing detailed information about metabolic activity and function. PET imaging plays a crucial role in monitoring responses to immunotherapy in various cancers, including lung adenocarcinoma, Hodgkin’s lymphoma, and breast cancer. It offers non-invasive whole-body imaging, aiding in the assessment of treatment response and tumor heterogeneity (26–28). Recent studies highlight the use of PET radiomics to visualize tumor-infiltrating CD8+ T cell exhaustion, optimizing radiotherapy and immunotherapy combinations in lung cancer models (29). This approach enhances understanding of tumor immune dynamics, aiding in the development of personalized treatment strategies. The latest developments in CD8-PET/CT imaging provide a non-invasive alternative to assess CD8+ tumor infiltration levels in patients undergoing immunotherapy for solid tumors, offering crucial insights for predicting immunotherapy response (30). Additionally, studies have found that Total Metabolic Tumor Volume (TMTV) on 18F-FDG PET/CT serves as an important prognostic biomarker in patients with extensive small cell lung cancer undergoing first-line chemo-immunotherapy, with strong predictive value for overall survival and progression-free survival (31). The development of immuno-PET imaging using tumor-specific Major Histocompatibility Complex II (tsMHC-II) to predict immunotherapy response, particularly checkpoint inhibitor therapy in melanoma, represents a significant advancement. Immuno-PET can provide valuable insights into the dynamic changes in tsMHC-II expression related to treatment response, aiding in predicting therapeutic outcomes (32). The integration of PET with immuno-oncology offers new insights into tumor-immune interactions and enhances the precision of therapeutic interventions. As technology advances, PET will continue to be a key tool in medical imaging, providing critical insights into the functional processes of various diseases.
Ultrasound imaging uses high-frequency sound waves to generate images of internal structures. The transducer emits sound waves that pass through the body and reflect off tissues and organs. These echoes are captured and converted into real-time images. Ultrasound is non-invasive, does not use ionizing radiation, and provides detailed information about soft tissue structures. Recent studies suggest that measuring tumor stiffness and perfusion using ultrasound imaging can predict responses to cancer immunotherapy. This method shows a strong linear correlation between tumor stiffness (elastic modulus) and perfusion parameters (33). Ultrasound imaging is also used to temporarily disrupt the blood-brain barrier and blood-tumor barrier, improving the delivery and efficacy of brain tumor immunotherapy by temporarily increasing the permeability of these barriers (34). Researchers have used CD93-targeted microbubbles in ultrasound imaging to predict the tumor immune microenvironment, aiding immunotherapy decision-making. This method provides a non-invasive approach to assess and monitor the tumor immune status (35). Ultrasound imaging can also monitor vascular changes after tumor radiotherapy, which are associated with immune cell infiltration. This technique offers a non-invasive way to track the effectiveness of tumor immunotherapy (36). With advancing technology, ultrasound imaging methods are evolving. For instance, a new contrast agent has been developed for synergistic chemophotothermal therapy and enhanced immunotherapy of liver cancer and its metastases, showing significant efficacy in preclinical studies (37). Researchers have developed a multifunctional high-intensity focused ultrasound (HIFU)-specific metal-organic framework nano system that effectively inhibits tumor growth and eliminates lung metastases in preclinical models by combining HIFU with hypoxia-activated chemoimmunotherapy, representing a new cancer treatment strategy (38). Focused ultrasound (FUS) in both thermal ablation and mechanical forms can enhance tumor immunotherapy by improving immune cell infiltration and treatment penetration. This method has shown promising results in various preclinical and clinical trials (39). With continuous innovations in imaging technologies and contrast agents, ultrasound imaging will remain a key tool in medical imaging, providing crucial insights into the functional processes of various diseases.
Multimodal imaging combines two or more imaging techniques to provide comprehensive information about tissue structure and function. Common combinations include CT, MRI, PET, ultrasound, and optical imaging. Each technology has its unique advantages; for example, MRI offers excellent soft tissue contrast, while PET provides functional and metabolic information. By integrating these technologies, multimodal imaging enhances diagnostic accuracy and provides detailed insights into disease processes.
Recent research has developed integrated multimodal imaging and synergistic therapeutic nanoplatforms for precise tumor nanomedicine (40, 41). These platforms enable enhanced tri-modal photothermal-chemotherapy-immunotherapy, demonstrating their potential in comprehensive tumor treatment and monitoring (42). Multifunctional nanoplatforms combining CAR-T cell immunotherapy and chemo-photothermal therapy have shown promise in multimodal imaging-guided tumor immunotherapy applications. These platforms enhance therapeutic efficacy by promoting lymphoid tissue angiogenesis and improving CAR-T cell infiltration in solid tumors (43). Additionally, studies indicate that the multifunctional theranostic agent TPA-BT-DPTQ can be used for multimodal imaging, including fluorescence imaging (FLI), photoacoustic imaging (PAI), and photothermal imaging (PTI). This agent combines photothermal therapy and immune effects, enhancing the efficacy of immunotherapy in inhibiting tumor growth and preventing cancer metastasis and recurrence (44). Advances in MRI imaging, combined with radiomics and deep learning, facilitate the assessment of tumor response to immunotherapy. This combination explores the biological characteristics of hepatocellular carcinoma (HCC), aiding in personalized treatment planning (40). The integration of various imaging technologies and the development of novel theranostic agents offer new insights into tumor-immune interactions and enhance the precision of therapeutic interventions (Figure 1).
Figure 1. Schematic representation of different techniques for real-time monitoring of immune cells and immune signaling molecules in the immune microenvironment through molecular imaging. The main techniques include optical imaging, positron emission tomography (PET), magnetic resonance imaging (MRI), computed tomography (CT), and ultrasound imaging.
T cells are a crucial component of the immune system and play a key role in tumor immunotherapy. Cytotoxic T lymphocytes (CTLs), also known as CD8+ T cells, are essential for directly killing cancer cells (45). They recognize tumor antigens presented by MHC class I molecules through their T cell receptors (TCRs). Once activated, CTLs release cytotoxic granules containing perforin and granzymes, which induce apoptosis in target cells. CTLs also produce cytokines such as IFN-γ and TNF-α, further enhancing the anti-tumor immune response (46). CD4+ T cells, or helper T cells, support anti-tumor immunity by coordinating immune responses. They assist in activating CTLs and B cells and regulate TME by secreting various cytokines. CD4+ T cells can differentiate into subsets, including Th1, Th2, Th17, and regulatory T cells (Tregs), each playing different roles in immune regulation. For example, Th1 cells promote CTL responses by producing IFN-γ, while Tregs maintain tolerance and prevent autoimmunity by suppressing immune responses through cytokines like IL-10 and TGF-β, and contact-dependent mechanisms (47, 48). Tregs are critical in maintaining immune homeostasis and preventing autoimmune reactions. They inhibit effective anti-tumor immune responses by suppressing CTLs and other effector immune cells (49, 50). Memory T cells provide long-lasting anti-tumor immunity by remembering previously encountered tumor antigens. Upon re-exposure to the same antigen, they rapidly mount a robust immune response, offering long-term protection against tumor recurrence (51). Natural killer T (NKT) cells exhibit properties of both T cells and natural killer (NK) cells, recognizing lipid antigens presented by CD1d molecules. They participate in tumor immunity by producing cytokines that activate other immune cells and directly killing tumor cells (52, 53).
Molecular imaging technologies have revolutionized the monitoring and evaluation of tumor immunotherapy. These non-invasive imaging methods allow visualization of biological processes at molecular and cellular levels, aiding in treatment assessment and optimization. PET imaging combined with radiolabeled antibodies is widely used to track T cells in vivo. For instance, 124I-Basiliximab has been developed for CD25-targeted immuno-PET imaging, showing promising results in distinguishing activated from non-activated human peripheral blood mononuclear cells (10, 54). Additionally, a humanized radiolabeled CD8-targeted minibody has been used to quantify tumor-infiltrating CD8+ T cells in brain tumors via PET imaging (55). Another study used PET imaging to monitor CD4+ T cell recovery during combined immunotherapy and chemotherapy in triple-negative breast cancer (56). A novel SPECT/CT imaging probe, 99mTc-sum IL-2, targets IL-2Rβ/IL-2Rγ receptors on tumor-infiltrating T cells, showing potential in predicting immune responses to anti-PD-L1 therapy and tracking adoptively transferred (ACT) T cells (57). Optical imaging techniques, including fluorescence and bioluminescence imaging, are used to study T cell behavior in real-time. Rare-earth nanoparticles have been developed for non-invasive in vivo imaging of tumor-infiltrating T cells, providing valuable information for early identification of non-responders and improved treatment outcomes (58). X-ray fluorescence imaging (XFI) has demonstrated potential in tracking T cells labeled with nanoparticles, offering a non-invasive method to monitor T cell distribution and activity within tumors, aiding in evaluating immunotherapy efficacy (59). Whole-body dynamic imaging and kinetic modeling of 18F-AraG have been used to study T cell distribution and activation in healthy individuals and cancer patients undergoing immunotherapy. This method provides detailed insights into immune cell activity and treatment response (60).
Chimeric Antigen Receptor (CAR) T cell therapy is a groundbreaking immunotherapeutic approach in which T cells are genetically engineered to express CARs that specifically recognize and target cancer cells (61). Recent studies have leveraged advanced imaging techniques to monitor the biodistribution and therapeutic effects of CAR-T cells. PET imaging using 89Zr-labeled CAR-T cells has facilitated real-time tracking of their distribution within tumors. For example, the study by Volpe et al. demonstrated the use of 89Zr-labeled CAR-T cells to assess their biodistribution and kinetics in vivo, revealing their accumulation in organs such as the liver and lungs shortly after infusion. This technique provides crucial insights into the effectiveness of CAR-T cell therapy and helps optimize treatment protocols (62).In addition, FDG-PET imaging has proven invaluable in assessing the response to CAR-T therapy, particularly in lymphoma patients. This imaging modality has been employed to monitor treatment efficacy and predict the onset of cytokine release syndrome (CRS) (63). These imaging techniques not only track the migration and persistence of CAR-T cells but also correlate with clinical outcomes, aiding in the management of therapeutic responses and potential toxicities.
Advances in molecular imaging significantly enhance our ability to visualize and understand T cell dynamics, offering new directions for optimizing cancer immunotherapy.
B cells function as antigen-presenting cells (APCs) by capturing and processing antigens, which are then presented to T cells through major histocompatibility complex (MHC) class II molecules. This interaction is crucial for activating CD4+ helper T cells, which in turn help activate CD8+ cytotoxic T cells, thereby enhancing the overall anti-tumor immune response (64, 65). B cells also differentiate into plasma cells that produce antibodies specific to tumor antigens. These antibodies can directly neutralize tumor cells, mark them for destruction by other immune cells through antibody-dependent cellular cytotoxicity (ADCC), or activate the complement system to induce tumor cell lysis. Tumor-infiltrating B cells (TIL-Bs) have been shown to play significant roles in tumor control by promoting anti-tumor immunity and interacting with other immune cells within the tumor microenvironment (TME). TIL-Bs facilitate the formation of tertiary lymphoid structures (TLSs) within tumors, which are associated with better prognosis and improved responses to immunotherapy (66). However, in some cases, B cells can exhibit immunosuppressive functions that may promote tumor progression. Regulatory B cells (Bregs) suppress effective anti-tumor immune responses by producing immunosuppressive cytokines such as IL-10, inhibiting T cell activity, and promoting the expansion of regulatory T cells (Tregs) (67).
PET and SPECT are advanced imaging technologies used to visualize and track B cells in vivo. These techniques allow non-invasive monitoring of B cell distribution, activation, and function within the TME, providing insights into their role during immunotherapy. For example, CD69 PET imaging has been used to monitor immune activation induced by immunotherapy, detecting CD69 expression on B cells and other immune cells with high sensitivity (68).Near-infrared fluorescence imaging using specific antibodies conjugated with fluorescent dyes, such as Miltuximab®-IRDye800, shows promise in targeting and visualizing B cells in tumors. This technology demonstrated high probe accumulation in urothelial carcinoma, indicating its potential for molecular imaging of bladder cancer (69). High-throughput spatial molecular imaging enables detailed characterization of TLSs and lymphoid aggregates in various solid tumors at the single-cell and subcellular levels. This approach provides comprehensive insights into the spatial organization and functional state of B cells within the TME (70). B cells play diverse and crucial roles in tumor immunity, from antigen presentation and antibody production to regulating immune responses in the TME (71). Advances in molecular imaging technologies have significantly enhanced our ability to visualize and understand B cell dynamics.
Tumor-associated macrophages (TAMs) play dual roles in the tumor microenvironment (TME), promoting both tumor growth and suppression. The polarization state of TAMs determines their function: M1 macrophages exhibit anti-tumor activity, while M2 macrophages support tumor growth and immune evasion (72). TAMs enhance tumor invasion and metastasis by secreting growth factors, angiogenic factors, and matrix metalloproteinases, which promote tumor cell proliferation, angiogenesis, and extracellular matrix degradation. For instance, in colorectal cancer, the polarization state of TAMs significantly affects tumor growth and metastasis (72). TAMs create an immunosuppressive environment in the TME by secreting cytokines such as IL-10 and TGF-β and expressing immune checkpoint molecules like PD-L1, which inhibit the functions of cytotoxic T cells and natural killer (NK) cells (72). Exosome secretion by TAMs also plays a key role in suppressing anti-tumor immunity. Exosomes carrying high levels of PD-L1 can inhibit CD8+ T cell proliferation and function (73).
A significant advancement in tumor molecular imaging is the development of nanoparticle contrast agents targeting TAMs. One notable example is SDIO nanoparticles, which show great potential in specifically detecting M2-polarized TAMs using MRI. This advancement is promising for assessing the localization and function of TAMs in cancer diagnosis and therapy. By providing clearer images of TAM distribution and activity within tumors, SDIO nanoparticles may improve the accuracy and effectiveness of cancer imaging and subsequent treatment (74). Similarly, 68Ga-labeled mannose-functionalized serum albumin nanoparticles have been developed for non-invasive imaging of TAMs. These nanoparticles not only help visualize TAMs but also closely monitor immune cell enrichment in the TME and response to anti-PD1 therapy, making them valuable tools for diagnostic imaging and therapeutic monitoring (75). Another significant advancement is the use of peptide-based imaging probes for TAM imaging. The 68Ga-labeled M2pep peptide has garnered attention for its rapid and targeted accumulation in tumors, making it an effective non-invasive TAM imaging probe (76). In PET imaging, probes such as 68Ga-NOTA-COG1410 have made significant strides. This radioligand targets TREM2 on TAMs, providing a specific and effective PET probe for diagnosing gastrointestinal tumors, thereby enhancing the specificity and sensitivity of PET imaging for these cancers (77). Additionally, the RP832c peptide targets CD206 expressed on M2-like macrophages. This peptide has therapeutic properties that reprogram TAMs to an anti-tumor phenotype and serves as a PET imaging agent in mouse cancer models (78). MRI susceptibility imaging has emerged as a promising non-invasive method for quantifying and phenotyping TAMs, particularly in glioblastoma, offering a way to assess TAM presence and activity without invasive procedures (79). Moreover, mass spectrometry and multiplex imaging technologies provide new techniques for studying the TME, revealing differences in the presence and behavior of TAMs, vasculature, and tumor cells in various tumor regions (80). These advancements highlight the importance of imaging technologies in understanding and targeting TAMs in the TME, paving the way for improved diagnostic and therapeutic strategies.
Natural killer (NK) cells are a vital component of the innate immune system, known for their ability to recognize and destroy aberrant or infected cells without prior sensitization. This potent cytotoxic activity and capacity to regulate immune responses make NK cells promising targets for cancer immunotherapy (81). NK cells can directly kill tumor cells by releasing cytotoxic granules containing perforin and granzymes, inducing apoptosis in target cells (82). They also express death receptor ligands, such as TRAIL and FasL, which trigger apoptosis in tumor cells expressing the corresponding receptors (83). NK cells produce cytokines such as IFN-γ and TNF-α, which enhance the anti-tumor activity of other immune cells, including dendritic cells (DCs) and T cells. NK cells also interact with DCs to promote the maturation and activation of adaptive immune responses, thereby bridging innate and adaptive immunity (84). NK cells play a crucial role in antibody-dependent cellular cytotoxicity (ADCC), a process where antibodies bound to tumor cells facilitate their destruction by NK cells. This is mediated by the binding of the antibody’s Fc region to CD16 (FcγRIII) on NK cells, leading to the release of cytotoxic granules and cytokines (85). Advances in genetic engineering, such as CRISPR/Cas9 technology, have enhanced NK cell cytotoxicity and resistance to the immunosuppressive tumor microenvironment (86). NK cell therapies, including CAR-NK cells and NK cell engagers (NKCEs), are actively being developed and tested in clinical trials. These therapies aim to harness NK cells’ natural cytotoxicity and enhance their ability to target and destroy tumor cells (87). Combination therapies with immune checkpoint inhibitors, such as anti-PD-1, have shown promising results in preclinical models, highlighting the potential of NK cells in cancer immunotherapy.
CAR-NK cells have shown promising therapeutic potential in treating various cancers, including aggressive ovarian cancer. Recent studies have monitored HER2-targeted CAR-NK cells using humanized PET reporter gene imaging. This non-invasive method allows real-time tracking of CAR-NK cells within patients, providing important insights into their distribution and activity in the tumor microenvironment, which helps optimize CAR-NK cell therapies. NK-92 cells were genetically modified to express a HER2-targeted CAR, the bioluminescence imaging reporter Antares, and the sodium iodide symporter (NIS), enabling detailed imaging (88). Additionally, researchers have used 89Zr-oxine-labeled NK cells for PET imaging to track their migration and distribution for up to seven days post-infusion. Real-time tracking is crucial for understanding NK cell dynamics and their interactions with tumor cells, enhancing the effectiveness of NK cell therapies (89). The molecular imaging agent [18F] AlF-mNOTA-GZP can detect changes in immune cell populations expressing granzyme B (such as GZB+ CD8+ T cells and GZB+ NK cells), further deepening our understanding of immune responses and treatment efficacy (90). Pham et al. utilized PET imaging to investigate the migration of 89Zr-labeled NK cells to HER2-positive breast tumors and assess the effects of trastuzumab treatment. The 89Zr-NK cells preserved their functional properties and predominantly accumulated in the liver and spleen. Treatment with trastuzumab increased the presence of NK cells within the tumor, demonstrating the effectiveness of this method for tracking NK cell migration (91).
A significant advancement in NK cell imaging is using MRI to track labeled NK cells. Researchers have used superparamagnetic iron oxide nanoparticles to label NK cells, enabling their visualization in vivo and clearly demonstrating NK cell migration to tumor sites. This method allows real-time monitoring of NK cell distribution and accumulation (92). Another study demonstrated the non-invasive detection and quantification of intratumoral NK cells using 19F MRI. This technology allows precise tracking of NK cell persistence and viability, highlighting its potential for real-time monitoring of NK cell therapies (93). Recent research has found that biomimetic nanoparticles can be used to label NK cells, facilitating effective MRI tracking. This approach not only improves NK cell visualization but also enhances their therapeutic efficacy by ensuring targeted delivery to tumor sites (94). Advances in molecular imaging technologies have significantly improved our understanding of NK cell dynamics, distribution, and interactions within the tumor microenvironment.
Dendritic cells (DCs) are a crucial component of the immune system, serving as potent antigen-presenting cells (APCs) that bridge innate and adaptive immunity. They play a key role in tumor immunity (95). DCs efficiently capture and process tumor antigens, presenting them to T cells via major histocompatibility complex (MHC) molecules. DC-mediated cross-priming is essential for generating anti-tumor CD8+ T cell immunity, which is the cornerstone of effective immunotherapy (96). Type 2 conventional dendritic cells (cDC2s) are particularly important in supporting cytotoxic T cell responses and helper T cell differentiation. They are involved in both anti-tumor and pro-tumor immune responses, making them critical targets for cancer vaccination and cDC2-targeted immunotherapy (97). DCs also play a significant role in immune regulation. Blocking immune checkpoints such as Tim-3 can enhance the anti-tumor immunity of the STING agonist ADU-S100 by regulating the release of CD4+ T cells from cDC2s (98). This highlights the potential of combining checkpoint inhibitors with DC-targeted therapies to enhance anti-tumor responses. As potent activators of T cells and efficient antigen-presenting cells, DCs play a central role in tumor immunity. Therapies targeting DCs, including vaccines and metabolic reprogramming, offer promising prospects for enhancing cancer immunotherapy effectiveness (99).
MRI has emerged as a powerful tool for tracking DC vaccines. This imaging modality allows for the non-invasive determination of the accuracy of therapeutic DC injections and their subsequent migration to lymph nodes. By providing real-time visualization, MRI tracking serves as an early biomarker, potentially predicting the efficacy of tumor vaccination before clinical outcomes become apparent. This method significantly improves our understanding of DC distribution and their role in initiating immune responses (100). Autologous dendritic cells have been labeled with clinical superparamagnetic iron oxide preparations or In-oxine and injected intranodally under ultrasound guidance in melanoma patients. In contrast to scintigraphy, MRI assesses the accuracy of dendritic cell delivery as well as cell migration patterns between and within nodes (101). The development of engineered DC vaccines enables non-invasive monitoring of their spatiotemporal fate using CT and NIRF imaging. This technology allows real-time visualization of vaccine accumulation in draining lymph nodes and subsequent potent T cell responses. Advances in this imaging technology facilitate personalized tumor imaging and enhance the efficacy of radioimmunotherapy (102, 103). Hypoxia imaging using 18F-FMISO PET has been employed to guide hypoxia-targeted treatments for unresponsive tumors. This technology improves responses to immune checkpoint therapy by leveraging immune cell populations, such as dendritic cells, within the tumor microenvironment. The ability to identify hypoxic and immunosuppressive regions within tumors aids in developing more effective treatment strategies (104). Combined bioluminescence imaging and I-124 PET provides insight into the biological behavior of dendritic cells in living organisms and can be a useful tool for optimizing DC-based immunotherapy regimens (105). The development of advanced imaging technologies has greatly enhanced our ability to track and understand the role of DCs in cancer immunotherapy.
Neutrophils are the most abundant granulocytes in the blood and a key component of the innate immune system. They play a crucial role in defending against infections and can exhibit both pro-tumor and anti-tumor functions in tumorigenesis (106). In the tumor microenvironment (TME), neutrophils can display either an anti-tumor (N1) or pro-tumor (N2) phenotype. N1 tumor-associated neutrophils (TANs) are generally considered anti-tumor, while N2 TANs promote tumor growth and metastasis (107). Neutrophils facilitate tumor progression by secreting factors that support angiogenesis, extracellular matrix remodeling, and cancer cell proliferation. They also release neutrophil extracellular traps (NETs) that capture circulating tumor cells, promoting their distant metastasis (108). Additionally, neutrophils can induce T cell anergy, characterized by a shift in lipid transport pathways, which inhibits T cell proliferation and activation (109). Neutrophils contribute to creating a tumor-supportive microenvironment. For example, sTLR9^+ neutrophils are heavily recruited to tumor inoculation sites, promoting a microenvironment that supports tumor growth (110). Moreover, immature neutrophils in the bone marrow naturally exhibit immunosuppressive and pro-tumor activities (111). Targeting neutrophils as a therapeutic strategy includes modulating the CXCLs-CXCR2 axis to regulate their recruitment and function in the TME (112). Neutrophils exhibit functional plasticity, significantly influencing tumor biology through interactions with other immune cells and the TME.
In imaging, researchers have developed an activatable polymer probe for fluorescence and photoacoustic imaging of tumor-associated neutrophils (TANs). This innovation provides a new tool for TAN imaging, facilitating cancer immunotherapy drug screening and enhancing our understanding of TANs’ roles in cancer immunotherapy (113). Additionally, a lipid-based molecular probe, TFML, combined with engineered neutrophils, has shown promise in targeted photoacoustic imaging of brain tumors. This approach offers potential for neutrophil-based nanotherapy and improved brain tumor imaging (114). Furthermore, researchers have used antibody-conjugated superparamagnetic iron oxide particles (SPIOs) in magnetic particle imaging (MPI) to non-invasively and sensitively track neutrophils. This method allows for radiation-free imaging of inflammatory cells, providing a safe and effective way to monitor neutrophil dynamics (115). Combining neutrophil targeting with magnetic targeting has also increased the accumulation of photothermal agents at tumor sites, observed through MR imaging to visualize tumor location. Philipp Spatz et al. developed and preliminarily characterized the first 18F-CXCR2 radiotracer for PET imaging targeting neutrophils. This tracer, designed based on a phthalimide template and obtained through indirect labeling, demonstrated specificity and diagnostic potential in neutrophil imaging (116).
Myeloid-derived suppressor cells (MDSCs) are a heterogeneous group of immune cells derived from myeloid progenitors that play a crucial role in tumor-induced immunosuppression (117). They exhibit strong immunosuppressive activity and promote tumor cell proliferation and invasion by secreting growth factors and enzymes, both directly and indirectly (118). Accumulating in the tumor microenvironment, MDSCs inhibit the function of anti-tumor effector cells such as T cells and NK cells, thereby weakening the body’s anti-tumor immune response. By suppressing the immune system, MDSCs enable tumor cells to evade immune detection and attack (119).
PET imaging has been used to study the mobilization of monocytic MDSCs (M-MDSCs) induced by liver transplant injury, which promotes tumor recurrence through the CXCL10/TLR4/MMP14 signaling pathway. This imaging method offers potential therapeutic interventions to reduce post-liver transplant tumor recurrence (120). Additionally, studies have found a strong correlation between [18F] DPA-714 uptake and the quantity and activation level of glioma-associated myeloid cells (GAMs). TSPO expression is primarily limited to HLA-DR+ activated GAMs, particularly tumor-infiltrating HLA-DR+ MDSCs and TAMs. The findings suggest that [18F] DPA-714-PET can be used for non-invasive imaging of the immunosuppressive tumor microenvironment in gliomas and to characterize the heterogeneity of myeloid cell infiltration at different disease stages (121). Moreover, researchers have designed a biomimetic manganese-based theranostic nanoplatform for multimodal imaging and dual immunotherapy of cancer. This platform utilizes a manganese dioxide coating and MDSC cell membrane camouflage to target the tumor microenvironment for photothermal imaging, photoacoustic imaging, and magnetic resonance imaging, enhancing anti-tumor immunity through chemodynamic therapy and photothermal therapy (122). Advances in imaging technology have significantly improved our understanding of MDSCs and their role in tumor biology (Figure 2; Table 1).
Figure 2. Schematic of the molecular images used to target immune cells, which include B cells, MDSC, NK cells, T cells, neutrophils, DCs and macrophages in TME.
Mesenchymal stem cells (MSCs) are adult stem cells with self-renewal and multipotent differentiation capabilities, initially isolated from bone marrow but also present in adipose tissue, umbilical cord, placenta, and dental pulp (123). MSCs can differentiate into various cell types, including osteocytes, chondrocytes, and adipocytes, making them highly valuable in tissue engineering and regenerative medicine (124). Additionally, MSCs possess potent immunomodulatory functions, inhibiting immune cell activity and inflammatory responses, and have potential applications in transplant medicine, such as preventing graft-versus-host disease (GVHD). In the tumor microenvironment, MSCs play a particularly notable role. They exhibit a natural tumor-tropic property, enabling them to migrate to tumor sites, making them ideal carriers for targeted delivery of anticancer drugs, genes, and nanomaterials (125). For instance, MSCs can carry the sodium/iodide symporter (NIS) gene, driven by the IL-6 promoter, significantly enhancing glioblastoma (GBM) uptake of radioactive tracers, thereby improving imaging diagnostics and therapeutic outcomes. Studies have shown that mice treated with IL-6-NIS-MSCs and subsequent 131I therapy experienced significant tumor growth delay and increased survival rates (126). MSCs also play a crucial role in regulating immune responses within the tumor microenvironment. They secrete various cytokines and growth factors that modulate immune responses, suppressing the activity of T cells, B cells, NK cells, and macrophages, and reducing inflammation. This characteristic makes MSCs highly promising for tumor therapy. However, despite their therapeutic potential, some studies indicate that MSCs might promote tumor growth and metastasis under certain conditions. By secreting growth factors and matrix metalloproteinases (MMPs), MSCs can enhance tumor cell proliferation, angiogenesis, and invasion (127). This mechanism is complex and depends on the tumor type, MSC source, and specific tumor microenvironment.
Furthermore, the combination of MSCs with nanotechnology has shown significant promise. For example, a conductive nanocomposite hydrogel composed of gold nanorods and synthetic silicate nanoplatelets (GNR@SN/Gel) has been developed for myocardial infarction (MI) treatment. This hydrogel provides electromechanical coupling and allows PET/CT imaging to monitor implantation sites. The use of MSCs with this hydrogel has shown protective effects on myocardial survival and cardiac function, indicating great potential in MI therapy (128). Another study used 89Zr-indium oxine labeling and PET-CT imaging to demonstrate the distribution of MSCTRAIL cell therapy in a lung cancer mouse model. This method enables non-invasive tracking of MSCTRAIL without affecting the MSC phenotype and therapeutic efficacy, offering a potential approach for evaluating novel cell therapies, understanding their mechanisms, migration dynamics, and patient variability (129). MSCs play complex and multifaceted roles in the tumor microenvironment, acting as effective therapeutic tools but also potentially promoting tumor progression in certain scenarios.
In the tumor microenvironment, stromal cells, particularly cancer-associated fibroblasts (CAFs), promote tumor progression and therapeutic resistance by producing extracellular matrix (ECM) components and secreting cytokines and growth factors (130). Stromal cells also interact with immune cells, influencing their function and fostering an immunosuppressive environment. Molecular imaging techniques, such as PET and MRI, can monitor these cells’ activities in real-time, providing critical diagnostic information. Radiolabeled imaging combined with radioligands can accurately pinpoint tumor areas, supporting personalized treatment (131). For example, 177Lu-PSMA-617 radioligand therapy is a targeted treatment for prostate cancer patients expressing prostate-specific membrane antigen (PSMA). The use of radiolabeled imaging techniques such as 177Lu-PSMA-617 helps identify PSMA-positive tumors, enabling clinicians to administer targeted therapy based on the specific characteristics of the tumor (132). High-throughput spatial molecular imaging technologies, such as CosMx™, can detect thousands of RNA species at the single-cell level, offering detailed spatial analysis of the complex functions of stromal cells (133). Overall, molecular imaging significantly enhances the effectiveness of tumor diagnosis and treatment by precisely locating and monitoring stromal cells (134).
Cancer-associated fibroblasts (CAFs) are a significant and dynamic component of the tumor microenvironment (TME), playing crucial roles in tumor progression, metastasis, immune regulation, and therapeutic resistance. CAFs originate from various sources, including resident fibroblasts, epithelial-mesenchymal transition (EMT), and bone marrow-derived mesenchymal stem cells (MSCs) (135). CAFs support tumor cell proliferation and invasion by secreting growth factors, cytokines, and ECM components while remodeling the ECM to create barriers that facilitate tumor cell migration and protect them from therapeutic agents (136). They also create an immunosuppressive microenvironment by secreting immunosuppressive cytokines and chemokines, inhibiting the activity of cytotoxic T cells and NK cells, and promoting immune evasion (137). Additionally, CAFs enhance cancer stem cell survival and proliferation, induce metabolic reprogramming in tumor cells, and increase their resistance to chemotherapy and radiotherapy (138). The heterogeneity and plasticity of CAFs enable them to adapt to various signals in the TME, exhibiting different functional states. Targeting CAFs is a potential cancer therapy strategy aimed at reducing tumor growth, enhancing efficacy, and overcoming therapeutic resistance (139).
In recent years, imaging technologies targeting CAFs have made significant advances, greatly improving cancer diagnosis and treatment capabilities. One such technology involves [99mTc] Tc-HYNIC-FAPI, a novel molecular probe specifically targeting CAFs by binding to fibroblast activation protein (FAP), demonstrating high specificity and sensitivity for SPECT/CT imaging (140). This technology excels in accurately locating and quantifying CAFs, aiding in understanding their role in tumor progression. IR-780 dye, a near-infrared dye, selectively accumulates in the mitochondria of cancer cells and CAFs, enhancing cancer immunotherapy by increasing T lymphocyte infiltration and serving imaging purposes (141). FAP-targeting ligands like PNT6555 deliver therapeutic agents directly to the tumor stroma by binding to FAP, increasing drug concentration and reducing side effects, offering both imaging and therapeutic functions (142). FAP-targeting radioligands such as FAP8-IP-DOTA exhibit high affinity and specificity, providing high-contrast tumor microenvironment images and prolonged retention in tumors, suitable for precise tumor localization and repeated treatment (143). Albumin-binding FAP radioligands like 68Ga-FSDD3I further enhance tumor uptake and retention time, offering high-quality imaging in PET scans (144). Researchers have studied the use of 68Ga-FAPI PET/CT imaging to monitor the effectiveness of combining a TGF-β receptor (TGF-βR) inhibitor with immunotherapy for treating metastatic colorectal cancer (CRC). The study showed that 68Ga-FAPI PET/CT imaging can accurately monitor the dynamic changes in CAFs and tumor immune responses. The TGF-βR inhibitor enhanced tumor-infiltrating T cells, increasing CRC sensitivity to KN046, a drug that blocks both PD-L1 and CTLA-4. This study supports the use of dual 68Ga-FAPI and 18F-FDG PET/CT imaging in managing CRC (145).These advancements provide new hope for early cancer detection, precise localization, and personalized treatment, promising to significantly improve cancer diagnosis and treatment outcomes (Figure 3).
Figure 3. Published studies of fibroblast activating protein inhibitor positron emission tomography (FAPI) in the diagnosis of various types of cancer.
Integrins are transmembrane receptors that facilitate cell adhesion to the ECM and play a crucial role in cell migration, invasion, survival, and immune responses. Their role in tumor immunity has been extensively studied, revealing their dual functions in promoting and inhibiting immune responses within TME (146). Integrins are essential for the migration and infiltration of immune cells. For example, integrin αLβ2 (LFA-1) on T cells interacts with ICAM-1 on endothelial cells, promoting T cell migration to tumors (147). Integrins also play a key role in the activation and function of immune cells. For instance, integrin αMβ2 (Mac-1) on macrophages mediates phagocytosis and antigen presentation. Furthermore, integrins are vital in recruiting and polarizing TAMs, which can either support or inhibit tumor growth. Integrins can also interact with immune checkpoint molecules, influencing their expression and function (148). Blocking integrin interactions can reduce PD-1 and CTLA-4 expression, thereby enhancing T cell activation and anti-tumor responses (149). Inhibitors or antibodies targeting integrins have emerged as promising strategies in cancer immunotherapy.
Integrins are transmembrane receptors that promote cell adhesion to the ECM, playing a critical role in tumor growth and metastasis. Researchers have developed various integrin-targeting imaging probes, validating their efficacy in preclinical and clinical studies. One such probe is Ga-68-Trivehexin, developed to target integrin αvβ6. This probe has shown high affinity and selectivity across multiple cancers, demonstrating high-specific uptake in metastatic PDAC and head and neck squamous cell carcinoma (HNSCC) in clinical PET/CT imaging. These findings suggest that Ga-68-Trivehexin holds great potential for imaging tumors expressing integrin αvβ6 (150). Another study developed a heterodimeric peptide targeting vascular endothelial growth factor receptor (VEGFR) and integrin αv, labeled with zirconium-89 (89Zr) for PET imaging of gliomas. This probe exhibited strong binding capability and clear tumor visualization in a mouse glioma xenograft model. Its dual-targeting characteristic provided superior imaging performance over single-targeting probes, showcasing the advantages of multi-target probes in tumor imaging (151). Additionally, the clinical evaluation of 68Ga-FAPI-RGD, a PET probe simultaneously targeting fibroblast activation protein (FAP) and integrin αvβ3, has demonstrated higher tumor uptake and tumor-to-background ratio (TBR) compared to traditional 18F-FDG PET/CT. This probe offers better lesion detection and tumor delineation, particularly in diagnosing lymph node and bone metastases, highlighting its potential as a new tool for cancer imaging (152). An exploratory study also assessed the effectiveness of 68Ga-FAPI-RGD in PET/CT imaging of patients with suspected malignant lung tumors. The results showed that 68Ga-FAPI-RGD had a higher primary lesion detection rate, increased tumor uptake, and better accuracy in evaluating mediastinal lymph nodes compared to 18F-FDG and single-target probes 68Ga-RGD and 68Ga-FAPI. This indicates that dual-targeting probes significantly improve lung cancer diagnosis and staging (153). These advancements highlight the critical role of integrins in cancer molecular imaging, providing new perspectives for future diagnostics and treatments. By developing novel imaging probes targeting integrins, scientists can more accurately detect and monitor tumors, optimize treatment plans, and ultimately improve patient outcomes.
Matrix metalloproteinases (MMPs) play a crucial role in tumor immunity by regulating ECM degradation, cell migration, and signal transduction within TME. MMPs degrade ECM components, promoting tumor cell invasion and metastasis, and release growth factors and cytokines stored in the ECM, altering the immune environment of the TME (154). MMP-2 and MMP-9 degrade type IV collagen, increasing the availability of angiogenic factors, and promoting tumor angiogenesis, which provides nutrients and oxygen to tumor cells. Additionally, MMPs affect the function and migration of immune cells (155). MMP-9 has been shown to regulate T cell migration and activation, influencing their infiltration and anti-tumor activity. TAMs also express high levels of MMPs, promoting the formation of an immunosuppressive TME and inhibiting anti-tumor immune responses (156). Targeted inhibition of MMPs can reactivate the anti-tumor functions of immune cells and enhance the effectiveness of immunotherapy.
Researchers have developed a dual-modality (PET/NIRF) imaging agent targeting MT1-MMP, which is overexpressed in high-grade sarcomas. This imaging agent, [89Zr] Zr-DFO-anti-MT1-MMP-IRDye800CW, demonstrates high specificity for MT1-MMP, allowing for preoperative planning and intraoperative guidance, significantly improving surgical outcomes in a sarcoma mouse model. This indicates its important application value in enhancing the success rate of sarcoma surgeries (157). Another study explored a fluorinated derivative of mangiferin (MF) as a potential PET radiopharmaceutical. They found that F-propyl-MF can form a stable complex with the catalytic zinc atom of MMP-9, demonstrating its potential as a PET imaging agent. This research provides a theoretical basis for the development of MMP-9-targeted PET imaging agents (158). Furthermore, a dual-modality imaging probe targeting MMP-14, overexpressed in glioma cells, was developed. This probe combines a PET radionuclide with near-infrared fluorescence (NIRF) dye, activating the fluorescent signal upon MMP-14 cleavage, improving the visualization and differentiation of glioma cells. In preclinical models, this probe significantly enhanced tumor imaging, offering a new tool for non-invasive imaging and intraoperative navigation (159). Additionally, a multifunctional PET/MRI nanoparticle was designed to image MMP-2 activity in atherosclerotic plaques. The nanoparticle, 64Cu-NOTA-IONP@MMP2c-PEG2K, showed high uptake and excellent imaging contrast in macrophage-rich plaques. This smart nanoparticle can non-invasively assess MMP-2 activity in vivo, providing detailed molecular imaging information through combined PET and MRI, aiding in early diagnosis and therapeutic intervention (160). By developing novel imaging probes targeting MMPs, scientists can more accurately detect and monitor tumors, optimize treatment plans, and ultimately improve patient outcomes.
Cytokines are small signaling proteins that play a crucial role in tumor development by regulating immune responses and cell-to-cell communication. Cytokines secreted by NK cells, such as IFN-γ and TNF-α, can enhance their anticancer functions (161). TAMs in ovarian cancer secrete cytokines like IL-6 and TGF-β, which promote tumor invasion and metastasis (162). In prostate cancer, the androgen receptor signaling pathway regulates cytokines, affecting immune-mediated antitumor responses (163). Neutrophil functions within the tumor microenvironment are influenced by cytokines, displaying both pro-tumor and anti-tumor roles (164). Radiolabeled anti-PD-L1 monoclonal antibodies used in PET imaging can non-invasively assess PD-L1 expression levels, predicting the efficacy of immunotherapy (165). In patients with schistosomiasis-related bladder cancer, levels of pro-inflammatory cytokines such as IL-1β, IL-6, and TNF-α are elevated (166). Cytokines also play a significant role in the interactions between cancer stem cells and the immune system (167). These studies demonstrate that cytokines secreted by immune cells are vital in modulating the tumor microenvironment, significantly impacting tumor growth, invasion, and therapeutic response.
In recent years, significant progress has been made in understanding the role of cytokines secreted by immune cells in the tumor microenvironment and their application in PET imaging. These studies have not only enhanced our understanding of cytokine functions but also provided new tools for precise cancer diagnosis and treatment. PD-L1 PET imaging has been used to assess PD-L1 expression in tumors, a key immunosuppressive cytokine secreted by tumor cells and tumor-infiltrating immune cells. By using radiolabeled anti-PD-L1 monoclonal antibodies (e.g., 89Zr-atezolizumab), researchers can non-invasively image PD-L1 expression levels and evaluate their relationship with immunotherapy response (168). Additionally, IL-2, another crucial cytokine primarily secreted by activated T cells, promotes the proliferation and activation of T cells and NK cells. Radiolabeled IL-2 (e.g., 18F-IL-2) has been used in PET imaging to monitor the activation state and distribution of immune cells, playing a vital role in evaluating cancer patients’ immune responses and optimizing immunotherapy strategies (169).
CXCL8 (also known as IL-8), a chemokine secreted by various cells including tumor and immune cells, primarily attracts neutrophils to tumor sites. PET tracers labeled with CXCL8 or its receptor can image neutrophil infiltration in tumors, which is crucial for studying tumor inflammation and the immunosuppressive microenvironment (170). CXCR3 is a chemokine receptor primarily expressed on activated T cells and natural killer cells, playing a role in inflammation and immune responses. A novel F-18-labeled small molecule PET tracer for CXCR3 imaging successfully detected CXCR3 expression in atherosclerotic mouse models (171). CXCR4, a chemokine receptor highly expressed in various tumor cells, is closely associated with tumor invasion and metastasis. In this study, researchers used a radiolabeled CXCR4 ligand for PET/CT imaging of nasopharyngeal carcinoma patients. The results showed that Ga-68-labeled CXCR4 PET/CT successfully localized and quantified CXCR4 expression levels in tumors, providing crucial information about tumor biology (172). CCR2 is critical for monocyte and macrophage migration. An 18F-labeled radiotracer targeting CCR2, used in PET imaging, revealed CCR2’s essential role in monocyte recruitment and inflammatory responses (173). CCR5, expressed on various immune cells including T cells, macrophages, and dendritic cells, is involved in HIV infection, inflammatory diseases, and cancer. Successful CCR5 PET imaging with 64Cu-DOTA-DAPTA-targeted nanoparticles effectively localized and quantified CCR5 expression (174). CMKLR1 regulates the activity of natural killer cells and dendritic cells under the mediation of the chemokine Chemerin. CMKLR1’s role in metabolic diseases, inflammation, and cancer makes it an emerging target for PET imaging. A PET tracer targeting CMKLR1 has been used to detect CMKLR1 expression in acute lung injury (175).The advancements in PET imaging technology for studying cytokines secreted by tumor immune cells have significantly deepened our understanding of the tumor microenvironment and opened new avenues for precise cancer treatment. Future research directions include developing more specific and sensitive PET tracers and combining PET with other imaging techniques for multimodal imaging.
In recent years, significant advancements in imaging technologies have enabled the simultaneous targeting of multiple regions within TME. These developments are crucial for understanding the complex interactions among tumor cells, the immune system, stromal cells, and blood vessels, all of which contribute to cancer progression and therapeutic resistance. The integration of different imaging modalities allows for more comprehensive and precise analysis of the TME, enhancing the ability to monitor tumor dynamics in real-time and guide targeted therapies effectively.
Researchers have developed hybrid imaging techniques by combining modalities such as CT, MRI, FLT. These multimodal imaging systems enable the simultaneous visualization of multiple components of the TME, including tumor vasculature, immune cells, and stromal elements. For example, combining CT and MRI with FLT improves biodistribution analysis and tumor characterization, offering superior soft tissue contrast that makes it easier to identify tumor boundaries and track therapeutic agents more effectively (176). Additionally, the integration of functional imaging modalities allows for the identification of tumor-associated changes, such as alterations in perfusion or metabolic activity. By targeting multiple regions of the TME simultaneously, these imaging techniques provide a more comprehensive view of the tumor’s biological environment.
Another significant advancement is the development of multiplexed imaging technologies, such as Imaging Mass Cytometry (IMC). This technique allows for the simultaneous assessment of multiple markers at the single-cell level, providing a deeper understanding of the cellular composition and spatial organization of the TME. Researchers have utilized IMC to analyze a variety of markers within the TME, revealing insights into the interactions between immune and stromal components (177). By characterizing the immune context and stromal features of the tumor, IMC offers strong evidence for studying tumor heterogeneity, which is critical for understanding treatment responses and identifying new therapeutic targets.
By simultaneously targeting and visualizing multiple components of the TME, clinicians can gain a more comprehensive understanding of tumor behavior, which aids in developing more personalized and effective treatment strategies.
Molecular imaging technologies are increasingly important in cancer diagnosis, treatment, and monitoring, especially in the context of immunotherapy. However, these technologies face several challenges that need to be addressed to enhance their clinical utility. Additionally, the future prospects of molecular imaging in tumor immunotherapy offer promising opportunities to improve patient outcomes. One of the main challenges of molecular imaging is the complexity and sensitivity of these technologies. Techniques such as PET, MRI, and SERS (surface-enhanced Raman spectroscopy) require high sensitivity and specificity to detect molecular changes at the cellular level. The complexity of tumor biology, including the heterogeneity of cancer cells and the dynamic nature of the tumor microenvironment, makes it difficult to develop imaging agents that can precisely target specific biomarkers without generating non-specific background signals. For instance, while SERS-based biosensors show promise, their application in the early diagnosis of cancers like pancreatic cancer still faces significant obstacles (178). Another notable challenge is the limited availability of multimodal imaging agents. These agents combine the advantages of various imaging modalities to provide comprehensive insights, such as integrating the high spatial resolution of MRI with the functional imaging capabilities of PET. However, creating stable and biocompatible imaging agents that work effectively across multiple modalities is a complex process. Recent research on pH-targeted molecular imaging techniques, such as pHLIP-anchored imaging agents, has shown potential in tumor diagnosis and therapy, but there remains a need for more versatile and efficient imaging agents in clinical settings (179, 180). Additionally, the integration and interpretation of the vast amounts of data generated by molecular imaging technologies present a significant challenge. The exponential growth of data requires robust, standardized frameworks for analysis and interpretation. Artificial intelligence (AI) and machine learning (ML) have been proposed as solutions, particularly for analyzing complex multimodal datasets. However, the clinical integration of AI tools, such as graph neural networks (GNNs), is still in its early stages (181, 182).
The future of molecular imaging is closely linked to advancements in AI and ML. These technologies are expected to play a critical role in improving image analysis and interpretation, helping to overcome challenges such as image noise, variability in imaging protocols, and the subjectivity of image interpretation. By training AI models on large datasets, predictive algorithms can be developed to assist clinicians in making more accurate diagnostic and treatment decisions. For example, deep learning technologies are being explored for the early detection of lung cancer and lung nodules, aiming to improve diagnostic accuracy and efficiency (183). Another promising research area is the development of novel imaging agents that target specific molecular pathways and provide real-time insights into tumor biology. These imaging agents must be highly specific, stable, and capable of delivering clear contrast in imaging studies. Activatable multimodal probes that respond to specific physiological changes in the tumor microenvironment, such as pH shifts or the presence of particular enzymes, are gaining attention for in vivo imaging and theranostics. These probes can be activated under specific conditions, providing more targeted and accurate imaging (184). The clinical translation and regulatory approval of molecular imaging technologies remain significant challenges. Bringing novel imaging agents and technologies from the lab to the clinic requires rigorous testing, including preclinical studies, clinical trials, and regulatory approvals. This process is often lengthy and costly, potentially delaying the availability of new imaging technologies for patients. Therefore, streamlining these processes is crucial for accelerating the clinical application of molecular imaging technologies. Molecular imaging plays a critical role in tumor immunotherapy, particularly in the management of immune checkpoint inhibitors (ICIs). PET/CT molecular imaging using [18F]-FDG has shown significant promise in evaluating the tumor microenvironment, detecting immune-related adverse events, assessing treatment efficacy, and predicting outcomes. This technology enables the non-invasive monitoring of immune responses and the identification of biomarkers that guide personalized immunotherapy strategies (185). Furthermore, novel molecular imaging tracers are making whole-body visualization through PET possible, allowing for the assessment of tumor and immune cell characteristics, which aids in making treatment decisions for tumor immunotherapy. These tracers provide insights into drug distribution and immune checkpoint molecules, which are crucial for optimizing treatment strategies and improving patient outcomes (186).
Advancements in imaging technologies have significantly enhanced the ability to monitor tumor responses to immunotherapy, facilitating more personalized treatment strategies. Among these, Molecular MRI has emerged as a key tool for non-invasively assessing tumor responses, detecting early signs of treatment failure, and providing insights into the biological effects of therapy. Additionally, this technique benefits from the integration of AI, which helps interpret complex imaging data and improves the accuracy of immunotherapy response assessments (187). Another promising approach is the use of non-immunogenic imaging techniques, such as murine sodium iodide symporter (mNIS) for SPECT imaging. This method provides a sensitive way to monitor tumor responses in immune-competent models, overcoming the immunogenicity issues commonly associated with traditional imaging techniques, thus enabling more accurate evaluations of immunotherapy efficacy (188). Furthermore, PET/CT imaging plays a crucial role in monitoring responses to immunotherapy, especially in breast cancer, where it helps assess tumor heterogeneity and track treatment efficacy. This imaging modality is essential for evaluating therapeutic responses and identifying patterns that can guide personalized treatment plans (28). Collectively, these advanced imaging technologies are critical for optimizing tumor immunotherapy, enhancing our ability to tailor treatments based on real-time, patient-specific tumor characteristics.
One of the major challenges in harnessing NK cells for cancer immunotherapy is their reduced numbers in the TME. Studies have shown that NK cells often undergo depletion in the TME, primarily due to immunosuppressive factors such as TGF-β, hypoxia, and suppressive immune cells like regulatory T cells (189). This poses a significant hurdle for effective NK cell-based therapies, as their reduced presence limits their ability to exert cytotoxic effects against tumor cells. For example, researchers have employed highly specific markers such as CD56 in combination with novel nanotechnology-based probes to enhance the detection of NK cells, even when their numbers are low. This approach significantly improves the sensitivity and precision of NK cell identification, making it feasible to study their dynamics in challenging conditions (190). In addition, strategies to expand NK cell populations in vivo are actively being explored to address the limitation of low cell numbers. These include cytokine-based therapies, such as IL-15 super-agonists, which promote NK cell proliferation and activation, as well as ex vivo expansion techniques prior to reinfusion, ensuring a robust population of functional NK cells for therapeutic applications (191, 192). Furthermore, advanced single-cell sequencing and high-throughput spatial molecular imaging technologies provide detailed insights into the functional states of NK cells within the ME. These methods not only facilitate the detection of NK cells but also enable a deeper understanding of their interactions within the TME (193). NK cells not only play a pivotal role in the treatment of solid tumors but also serve as a critical component in leukemia immunotherapy (189). For instance, the adoptive transfer of NK cells engineered to express the bone marrow homing receptor CXCR4R334X has enhanced the targeting of leukemia cells residing in the bone marrow, thereby improving therapeutic efficacy (194). Similarly, CAR-NK cells designed to target specific leukemia antigens, such as CD19 and CD33, have demonstrated greater cytotoxicity and specificity against acute myeloid leukemia (AML) cells (195). Furthermore, advancements in molecular imaging technologies provide crucial support for NK cell-based therapies by enabling precise monitoring of NK cell migration, persistence, and interactions within the tumor microenvironment (196).
Molecular imaging has become an indispensable tool in cancer research and treatment, particularly in understanding and visualizing the complex interactions within the tumor microenvironment. Technologies that enable the non-invasive monitoring of immune cells, cytokines, and matrix components have brought significant advancements to the field, allowing for more precise and personalized treatment approaches. However, several challenges remain, including the development of more sensitive and specific imaging agents, the effective integration and interpretation of large datasets, and the translation of laboratory discoveries into clinical practice. The future of molecular imaging lies in overcoming these challenges through advances in artificial intelligence, the creation of innovative imaging probes, and the integration of these technologies with personalized medicine. Continued research and development in this field are essential for improving cancer diagnosis, monitoring treatment efficacy, and ultimately enhancing patient outcomes.
XW: Writing – original draft. WS: Validation, Writing – original draft. LY: Validation, Visualization, Writing – original draft. CL: Validation, Writing – original draft. HY: Validation, Writing – review & editing. DG: Funding acquisition, Writing – review & editing.
The author(s) declare financial support was received for the research, authorship, and/or publication of this article. This study was supported by the National Natural Science Foundation of China (82303646 to DG). The funding agencies had no role in study design, data collection, and analysis, decision to publish, or preparation of the manuscript.
Figures in the article were drawn using BioRender.com.
The authors declare that the research was conducted in the absence of any commercial or financial relationships that could be construed as a potential conflict of interest.
The author(s) declare that no Generative AI was used in the creation of this manuscript.
All claims expressed in this article are solely those of the authors and do not necessarily represent those of their affiliated organizations, or those of the publisher, the editors and the reviewers. Any product that may be evaluated in this article, or claim that may be made by its manufacturer, is not guaranteed or endorsed by the publisher.
1. Park J, Hsueh PC, Li Z, Ho PC. Microenvironment-driven metabolic adaptations guiding CD8(+) T cell anti-tumor immunity. Immunity. (2023) 56:32–42. doi: 10.1016/j.immuni.2022.12.008
2. van Weverwijk A, de Visser KE. Mechanisms driving the immunoregulatory function of cancer cells. Nat Rev Cancer. (2023) 23:193–215. doi: 10.1038/s41568-022-00544-4
3. Zhao J, Dong Y, Zhang Y, Wang J, Wang Z. Biophysical heterogeneity of myeloid-derived microenvironment to regulate resistance to cancer immunotherapy. Advanced Drug delivery Rev. (2022) 191:114585. doi: 10.1016/j.addr.2022.114585
4. Wang DR, Wu XL, Sun YL. Therapeutic targets and biomarkers of tumor immunotherapy: response versus non-response. Signal transduction targeted Ther. (2022) 7:331. doi: 10.1038/s41392-022-01136-2
5. Yin N, Li X, Zhang X, Xue S, Cao Y, Niedermann G, et al. Development of pharmacological immunoregulatory anti-cancer therapeutics: current mechanistic studies and clinical opportunities. Signal transduction targeted Ther. (2024) 9:126. doi: 10.1038/s41392-024-01826-z
6. Xiao M, Tang Q, Zeng S, Yang Q, Yang X, Tong X, et al. Emerging biomaterials for tumor immunotherapy. Biomaterials Res. (2023) 27:47. doi: 10.1186/s40824-023-00369-8
7. Rowe SP, Pomper MG. Molecular imaging in oncology: Current impact and future directions. CA: Cancer J Clin. (2022) 72:333–52. doi: 10.3322/caac.21713
8. Schwenck J, Sonanini D, Cotton JM, Rammensee HG, la Fougère C, Zender L, et al. Advances in PET imaging of cancer. Nat Rev Cancer. (2023) 23:474–90. doi: 10.1038/s41568-023-00576-4
9. Quan Z, Han Z, Yang Y, Wang J, Wang H, Yang L, et al. Noninvasive monitoring of immunotherapy in lung cancer by lymphocyte activation gene 3 PET imaging of tumor-infiltrating lymphocytes. J Nucl med: Off publication Soc Nucl Med. (2024) 65:25–32. doi: 10.2967/jnumed.123.266002
10. Wang S, Liu F, Wang P, Wen L, Wang Z, Guo Q, et al. (124)I radiolabeled basiliximab for CD25-targeted immuno-PET imaging of activated T cells. Mol pharmaceutics. (2022) 19:2629–37. doi: 10.1021/acs.molpharmaceut.2c00330
11. Qin C, Zhu S, Tian J. New optical molecular imaging systems. J Curr Pharm Biotechnol. (2010) 11:620–7.
12. Tian J, Bai J, Yan XP, Bao S, Li Y, Liang W, et al. Multimodality molecular imaging. IEEE Eng Med Biol Mag. (2008) 27:48–57. doi: 10.1109/memb.2008.923962
13. Cen P, Zhou Y, Cui C, Wei Y, Cheng Z, Wu S, et al. Optical molecular imaging and theranostics in neurological diseases based on aggregation-induced emission luminogens. Eur J Nucl Med Mol Imaging. (2022) 49:4529–50. doi: 10.1007/s00259-022-05894-7
14. Valdés PA, Roberts DW, Lu FK, Golby A. Optical technologies for intraoperative neurosurgical guidance. Neurosurgical Focus. (2016) 40:E8. doi: 10.3171/2015.12.Focus15550
15. Waaijer SJH, Kok IC, Eisses B, Schröder CP, Jalving M, Brouwers AH, et al. Molecular imaging in cancer drug development. J Nucl Med. (2018) 59:726–32. doi: 10.2967/jnumed.116.188045
16. Tansi FL, Rüger R, Kollmeier AM, Rabenhold M, Steiniger F, Kontermann RE, et al. Targeting the tumor microenvironment with fluorescence-activatable bispecific endoglin/fibroblast activation protein targeting liposomes. Pharmaceutics. (2020) 12(4). doi: 10.3390/pharmaceutics12040370
17. Li J, Li B, Sun J, Ma C, Wan S, Li Y, et al. Engineered near-infrared fluorescent protein assemblies for robust bioimaging and therapeutic applications. Advanced materials (Deerfield Beach Fla). (2020) 32:e2000964. doi: 10.1002/adma.202000964
18. Kubeil M, Martínez IIS, Bachmann M, Kopka K, Tuck KL, Stephan H. Dual-labelling strategies for nuclear and fluorescence molecular imaging: current status and future perspectives. Pharm (Basel Switzerland). (2022) 15(4). doi: 10.3390/ph15040432
19. Liu N, Chen X, Kimm MA, Stechele M, Chen X, Zhang Z, et al. In vivo optical molecular imaging of inflammation and immunity. J Mol Med (Berlin Germany). (2021) 99:1385–98. doi: 10.1007/s00109-021-02115-w
20. Shukla G, Alexander GS, Bakas S, Nikam R, Talekar K, Palmer JD, et al. Advanced magnetic resonance imaging in glioblastoma: a review. Chin Clin Oncol. (2017) 6:40. doi: 10.21037/cco.2017.06.28
21. Jackson A, O'Connor J, Thompson G, Mills S. Magnetic resonance perfusion imaging in neuro-oncology. Cancer Imaging. (2008) 8:186–99. doi: 10.1102/1470-7330.2008.0019
22. Radue EW, Weigel M, Wiest R, Urbach H. Introduction to magnetic resonance imaging for neurologists. Continuum (Minneapolis Minn). (2016) 22:1379–98. doi: 10.1212/con.0000000000000391
23. Slomka PJ, Pan T, Berman DS, Germano G. Advances in SPECT and PET hardware. Prog Cardiovasc Dis. (2015) 57:566–78. doi: 10.1016/j.pcad.2015.02.002
24. Ritt P. Recent developments in SPECT/CT. Semin Nucl Med. (2022) 52:276–85. doi: 10.1053/j.semnuclmed.2022.01.004
25. Ljungberg M, Pretorius PH. SPECT/CT: an update on technological developments and clinical applications. Br J Radiol. (2018) 91:20160402. doi: 10.1259/bjr.20160402
26. Park C, Na KJ, Choi H, Ock CY, Ha S, Kim M, et al. Tumor immune profiles noninvasively estimated by FDG PET with deep learning correlate with immunotherapy response in lung adenocarcinoma. Theranostics. (2020) 10:10838–48. doi: 10.7150/thno.50283
27. Tutino F, Giovannini E, Chiola S, Giovacchini G, Ciarmiello A. Assessment of response to immunotherapy in patients with hodgkin lymphoma: towards quantifying changes in tumor burden using FDG-PET/CT. J Clin Med. (2023) 12:3498. doi: 10.3390/jcm12103498
28. Vaz SC, Graff SL, Ferreira AR, Debiasi M, de Geus-Oei LF. PET/CT in patients with breast cancer treated with immunotherapy. Cancers. (2023) 15:2620. doi: 10.3390/cancers15092620
29. Zhang Y, Hu HH, Zhou SH, Xia WY, Zhang Y, Zhang JP, et al. PET-based radiomics visualizes tumor-infiltrating CD8 T cell exhaustion to optimize radiotherapy/immunotherapy combination in mouse models of lung cancer. biomark Res. (2023) 11:10. doi: 10.1186/s40364-023-00454-z
30. Potdevin G, Ayx I, Dillon L, Mahieu E, Sade H, Schmidt G. A first assessment of CD8-PET/CT with 89Zr-Crefmirlimab as predictive biomarker for response to standard of care immunotherapy in patients with solid tumors. JCR. (2023) 83:3577–.
31. Grambow-Velilla J, Seban RD, Chouahnia K, Assié JB, Champion L, Girard N, et al. Total metabolic tumor volume on 18F-FDG PET/CT is a useful prognostic biomarker for patients with extensive small-cell lung cancer undergoing first-line chemo-immunotherapy. Cancers. (2023) 15(8). doi: 10.3390/cancers15082223
32. Yang Z, Li F, Huang Y, Yin N, Chu J, Ma Y, et al. Dynamic tumor-specific MHC-II immuno-PET predicts the efficacy of checkpoint inhibitor immunotherapy in melanoma. J Nucl med: Off publication Soc Nucl Med. (2022) 63:1708–14. doi: 10.2967/jnumed.121.263151
33. Voutouri C, Stylianopoulos T, Mpekris F, Panagi M, Michael C, Martin J. 108 Prediction of cancer immunotherapy response using ultrasound imaging of tumor stiffness and perfusion. Regular and Young Investigator Award Abstracts. (2022).
34. Sheybani ND. Emerging applications of image-guided therapeutic ultrasound for brain tumor-directed immunomodulation and immunotherapy. J Acoustical Soc America. (2022) 152:A154–A.
35. Wang D, Xing C, Liang Y, Wang C, Zhao P, Liang X, et al. Ultrasound imaging of tumor vascular CD93 with MMRN2 modified microbubbles for immune microenvironment prediction. Advanced materials (Deerfield Beach Fla). (2024) 36:e2310421. doi: 10.1002/adma.202310421
36. Martello SE, Xia J, Kusunose J, Hacker BC, Mayeaux MA, Lin EJ, et al. Ultrasound imaging enables longitudinal tracking of vascular changes that correlate with immune response after radiotherapy. Theranostics. (2024) 14:6883–96. doi: 10.1101/2023.08.04.552076
37. Qiu Y, Wu Z, Chen Y, Liao J, Zhang Q, Wang Q, et al. Nano ultrasound contrast agent for synergistic chemo-photothermal therapy and enhanced immunotherapy against liver cancer and metastasis. Advanced Sci (Weinheim Baden-Wurttemberg Germany). (2023) 10:e2300878. doi: 10.1002/advs.202300878
38. Sun T, Li J, Zhou Y, Zeng C, Luo C, Luo X, et al. Metal-organic framework-mediated synergistic hypoxia-activated chemo-immunotherapy induced by high intensity focused ultrasound for enhanced cancer theranostics. Small (Weinheim an der Bergstrasse Germany). (2024) 20:e2306338. doi: 10.1002/smll.202306338
39. Memari E, Khan D, Alkins R, Helfield B. Focused ultrasound-assisted delivery of immunomodulating agents in brain cancer. J Controlled release: Off J Controlled Release Soc. (2024) 367:283–99. doi: 10.1016/j.jconrel.2024.01.034
40. Chen Y, Yang C, Sheng L, Jiang H, Song B. The era of immunotherapy in hepatocellular carcinoma: the new mission and challenges of magnetic resonance imaging. Cancers. (2023) 15:4677. doi: 10.3390/cancers15194677
41. Zhang H, Chen K, Guo K, Tao J, Song L, Ren S, et al. Multimodal imaging-guided photoimmunotherapy of pancreatic cancer by organosilica nanomedicine. Advanced healthcare materials. (2024) 13:e2302195. doi: 10.1002/adhm.202302195
42. Gu M, Zhang L, Hao L, Wang K, Yang W, Liu Z, et al. Upconversion nanoplatform enables multimodal imaging and combinatorial immunotherapy for synergistic tumor treatment and monitoring. ACS Appl materials interfaces. (2023) 15:21766–80. doi: 10.1021/acsami.2c22420
43. Shi B, Li D, Yao W, Wang W, Jiang J, Wang R, et al. Multifunctional theranostic nanoparticles for multi-modal imaging-guided CAR-T immunotherapy and chemo-photothermal combinational therapy of non-Hodgkin's lymphoma. Biomaterials Sci. (2022) 10:2577–89. doi: 10.1039/d1bm01982a
44. Yan D, Wang M, Wu Q, Niu N, Li M, Song R, et al. Multimodal imaging-guided photothermal immunotherapy based on a versatile NIR-II aggregation-induced emission luminogen. Angewandte Chemie (International ed English). (2022) 61:e202202614. doi: 10.1002/anie.202202614
45. Sun L, Su Y, Jiao A, Wang X, Zhang B. T cells in health and disease. Signal transduction targeted Ther. (2023) 8:235. doi: 10.1038/s41392-023-01471-y
46. van der Leun AM, Thommen DS, Schumacher TN. CD8(+) T cell states in human cancer: insights from single-cell analysis. Nat Rev Cancer. (2020) 20:218–32. doi: 10.1038/s41568-019-0235-4
47. Oh DY, Fong L. Cytotoxic CD4(+) T cells in cancer: Expanding the immune effector toolbox. Immunity. (2021) 54:2701–11. doi: 10.1016/j.immuni.2021.11.015
48. Pereira MVA, Galvani RG, Gonçalves-Silva T, de Vasconcelo ZFM, Bonomo A. Tissue adaptation of CD4 T lymphocytes in homeostasis and cancer. Front Immunol. (2024) 15:1379376. doi: 10.3389/fimmu.2024.1379376
49. Xiong W, Gao X, Zhang T, Jiang B, Hu MM, Bu X, et al. USP8 inhibition reshapes an inflamed tumor microenvironment that potentiates the immunotherapy. Nat Commun. (2022) 13:1700. doi: 10.1038/s41467-022-29401-6
50. Tay C, Tanaka A, Sakaguchi S. Tumor-infiltrating regulatory T cells as targets of cancer immunotherapy. Cancer Cell. (2023) 41:450–65. doi: 10.1016/j.ccell.2023.02.014
51. Okła K, Farber DL, Zou W. Tissue-resident memory T cells in tumor immunity and immunotherapy. J Exp Med. (2021) 218:e20201605. doi: 10.1084/jem.20201605
52. Feng Y, Zhang H, Shao J, Du C, Zhou X, Guo X, et al. Research progress of nanomaterials acting on NK cells in tumor immunotherapy and imaging. Biology. (2024) 13:153. doi: 10.3390/biology13030153
53. Pan R, Ryan J, Pan D, Wucherpfennig KW, Letai A. Augmenting NK cell-based immunotherapy by targeting mitochondrial apoptosis. Cell. (2022) 185:1521–38.e18. doi: 10.1016/j.cell.2022.03.030
54. Lee JH, Jung KH, Kim M, Lee KH. Cysteine-specific (89)Zr-labeled anti-CD25 IgG allows immuno-PET imaging of interleukin-2 receptor-α on T cell lymphomas. Front Immunol. (2022) 13:1017132. doi: 10.3389/fimmu.2022.1017132
55. Nagle VL, Henry KE, Hertz CAJ, Graham MS, Campos C, Parada LF, et al. Imaging tumor-infiltrating lymphocytes in brain tumors with [(64)Cu]Cu-NOTA-anti-CD8 PET. Clin Cancer research: an Off J Am Assoc Cancer Res. (2021) 27:1958–66. doi: 10.1158/1078-0432.Ccr-20-3243
56. Napier TS, Lynch SE, Lu Y, Song PN, Burns AC, Sorace AG. Molecular imaging of oxygenation changes during immunotherapy in combination with paclitaxel in triple negative breast cancer. Biomedicines. (2023) 11:125. doi: 10.3390/biomedicines11010125
57. Gao Y, Luo Q, Sun Z, Gao H, Yu Y, Sun Y, et al. Implication of (99m)Tc-sum IL-2 SPECT/CT in immunotherapy by imaging of tumor-infiltrating T cells. J immunother Cancer. (2023) 11:e005925. doi: 10.1136/jitc-2022-005925
58. Kataria S, Qi J, Lin CW, Li Z, Dane EL, Iyer AM, et al. Noninvasive in vivo imaging of T-cells during cancer immunotherapy using rare-earth nanoparticles. ACS nano. (2023) 17:17908–19. doi: 10.1021/acsnano.3c03882
59. Kahl H, Staufer T, Körnig C, Schmutzler O, Rothkamm K, Grüner F. Feasibility of monitoring tumor response by tracking nanoparticle-labelled T cells using X-ray fluorescence imaging-A numerical study. Int J Mol Sci. (2021) 22:8736. doi: 10.3390/ijms22168736
60. Omidvari N, Levi J, Abdelhafez YG, Wang Y, Nardo L, Daly ME, et al. Total-body dynamic imaging and kinetic modeling of (18)F-araG in healthy individuals and a non-small cell lung cancer patient undergoing anti-PD-1 immunotherapy. medRxiv. (2023) 9:2329. doi: 10.1101/2023.09.22.23295860
61. Levstek L, Janžič L, Ihan A, Kopitar AN. Biomarkers for prediction of CAR T therapy outcomes: current and future perspectives. Front Immunol. (2024) 15:1378944. doi: 10.3389/fimmu.2024.1378944
62. Volpe A, Nagle VL, Lewis JS, Ponomarev V. Predicting CAR-T cell Immunotherapy Success through ImmunoPET. Clin Cancer research: an Off J Am Assoc Cancer Res. (2021) 27:911–2. doi: 10.1158/1078-0432.Ccr-20-4297
63. Casadei B, Paccagnella A, Farolfi A, Argnani L, Malizia C, Paolani G, et al. FDG-PET imaging and radiomics in response assessment of lymphoma patients undergoing car t-cell therapy. Hematological Oncology. (2021) 39(S2). doi: 10.1002/hon.188_2880
64. Laumont CM, Banville AC, Gilardi M, Hollern DP, Nelson BH. Tumour-infiltrating B cells: immunological mechanisms, clinical impact and therapeutic opportunities. Nat Rev Cancer. (2022) 22:414–30. doi: 10.1038/s41568-022-00466-1
65. Laumont CM, Nelson BH. B cells in the tumor microenvironment: Multi-faceted organizers, regulators, and effectors of anti-tumor immunity. Cancer Cell. (2023) 41:466–89. doi: 10.1016/j.ccell.2023.02.017
66. Zhou J, Zhou R, Zhu Y, Deng S, Muhuitijiang B, Li C, et al. Investigating the impact of regulatory B cells and regulatory B cell-related genes on bladder cancer progression and immunotherapeutic sensitivity. J Exp Clin Cancer research: CR. (2024) 43:101. doi: 10.1186/s13046-024-03017-8
67. Michaud D, Steward CR, Mirlekar B, Pylayeva-Gupta Y. Regulatory B cells in cancer. Immunol Rev. (2021) 299:74–92. doi: 10.1111/imr.12939
68. Edwards KJ, Chang B, Babazada H, Lohith K, Park DH, Farwell MD, et al. Using CD69 PET imaging to monitor immunotherapy-induced immune activation. Cancer Immunol Res. (2022) 10:1084–94. doi: 10.1158/2326-6066.Cir-21-0874
69. Polikarpov D, Campbell D, McRobb L, Zaslavsky A, Zvyagin A, Walsh B, et al. Abstract A08: Application of Miltuximab®-IRDye800 for near-infrared fluorescence imaging of urothelial carcinoma in vivo. Clin Cancer Res. (2020) 26:A08–A. doi: 10.1158/1557-3265.BLADDER19-A08
70. Liu Y, Zhang Y, Han G, Pei G, Cho KS, Dai E, et al. Abstract 1152: Pan-cancer single-cell and subcellular characterization of lymphoid aggregates and tertiary lymphoid structures using high-plex spatial molecular imaging. Cancer Res. (2024) 84:1152. doi: 10.1158/1538-7445.AM2024-115
71. Ingravallo G, Tamma R, Opinto G, Annese T, Gaudio F, Specchia G, et al. The effect of the tumor microenvironment on lymphoid neoplasms derived from B cells. Diagnostics (Basel Switzerland). (2022) 12:573. doi: 10.3390/diagnostics12030573
72. DeRyckere D, Huelse JM, Earp HS, Graham DK. TAM family kinases as therapeutic targets at the interface of cancer and immunity. Nat Rev Clin Oncol. (2023) 20:755–79. doi: 10.1038/s41571-023-00813-7
73. Zhong W, Lu Y, Han X, Yang J, Qin Z, Zhang W, et al. Upregulation of exosome secretion from tumor-associated macrophages plays a key role in the suppression of anti-tumor immunity. Cell Rep. (2023) 42:113224. doi: 10.1016/j.celrep.2023.113224
74. Zhou J, Meli VS, Yu-Tin Chen E, Kapre R, Nagalla R, Xiao W, et al. Magnetic resonance imaging of tumor-associated-macrophages (TAMs) with a nanoparticle contrast agent. RSC Adv. (2022) 12:7742–56. doi: 10.1039/d1ra08061j
75. Gu GJ, Chung H, Park JY, Yoo R, Im HJ, Choi H, et al. Mannosylated-serum albumin nanoparticle imaging to monitor tumor-associated macrophages under anti-PD1 treatment. J Nanobiotechnol. (2023) 21:31. doi: 10.1186/s12951-023-01791-9
76. Huang M, Wang R, Li M, Cai H, Tian R. Peptide-based [(68)Ga]Ga labeled PET tracer for tumor imaging by targeting tumor-associated macrophages. Pharmaceutics. (2022) 14:2511. doi: 10.3390/pharmaceutics14112511
77. Shi D, Si Z, Xu Z, Cheng Y, Lin Q, Fu Z, et al. Synthesis and evaluation of (68)Ga-NOTA-COG1410 targeting to TREM2 of TAMs as a specific PET probe for digestive tumor diagnosis. Analytical Chem. (2022) 94:3819–30. doi: 10.1021/acs.analchem.1c04701
78. Parker CC, Bin Salam A, Song PN, Gallegos C, Hunt A, Yates C, et al. Evaluation of a CD206-targeted peptide for PET imaging of macrophages in syngeneic mouse models of cancer. Mol pharmaceutics. (2023) 20:2415–25. doi: 10.1021/acs.molpharmaceut.2c00977
79. Nazem A, Guiry SC, Pourfathi M, Ware JB, Anderson H, Iyer SK, et al. MR susceptibility imaging for detection of tumor-associated macrophages in glioblastoma. J neuro-oncol. (2022) 156:645–53. doi: 10.1007/s11060-022-03947-3
80. Anand A, Harwood DS, Bishop C, Todd K, Ellis R, Ellis R, et al. P12.08.A Uncovering the glioblastoma tumor-microenvironment by high-end multiplexing with imaging mass cytometry. Neuro-Oncology. (2022) 24:ii78–ii. doi: 10.1093/neuonc/noac174.273
81. Zhou Y, Cheng L, Liu L, Li X. NK cells are never alone: crosstalk and communication in tumour microenvironments. Mol Cancer. (2023) 22:34. doi: 10.1186/s12943-023-01737-7
82. Wolf NK, Kissiov DU, Raulet DH. Roles of natural killer cells in immunity to cancer, and applications to immunotherapy. Nat Rev Immunol. (2023) 23:90–105. doi: 10.1038/s41577-022-00732-1
83. Yang YL, Yang F, Huang ZQ, Li YY, Shi HY, Sun Q, et al. T cells, NK cells, and tumor-associated macrophages in cancer immunotherapy and the current state of the art of drug delivery systems. Front Immunol. (2023) 14:1199173. doi: 10.3389/fimmu.2023.1199173
84. Kirchhammer N, Trefny MP, Natoli M, Brücher D, Smith SN, Werner F, et al. NK cells with tissue-resident traits shape response to immunotherapy by inducing adaptive antitumor immunity. Sci Trans Med. (2022) 14:eabm9043. doi: 10.1126/scitranslmed.abm9043
85. Page A, Chuvin N, Valladeau-Guilemond J, Depil S. Development of NK cell-based cancer immunotherapies through receptor engineering. Cell Mol Immunol. (2024) 21:315–31. doi: 10.1038/s41423-024-01145-x
86. Bexte T, Alzubi J, Reindl LM, Wendel P, Schubert R, Salzmann-Manrique E, et al. CRISPR-Cas9 based gene editing of the immune checkpoint NKG2A enhances NK cell mediated cytotoxicity against multiple myeloma. Oncoimmunology. (2022) 11:2081415. doi: 10.1080/2162402x.2022.2081415
87. Dagher OK, Posey AD Jr. Forks in the road for CAR T and CAR NK cell cancer therapies. Nat Immunol. (2023) 24:1994–2007. doi: 10.1038/s41590-023-01659-y
88. Shalaby N, Xia Y, Kelly JJ, Sanchez-Pupo R, Martinez F, Fox MS, et al. Imaging CAR-NK cells targeted to HER2 ovarian cancer with human sodium-iodide symporter-based positron emission tomography. Eur J Nucl Med Mol Imaging. (2024) 51:3176–90. doi: 10.1007/s00259-024-06722-w
89. Sato N, Szajek LP, Choyke PL. Tracking of NK cells by positron emission tomography using (89)Zr-oxine ex vivo cell labeling. Methods Mol Biol (Clifton NJ). (2022) 2463:153–61. doi: 10.1007/978-1-0716-2160-8_11
90. Goggi JL, Hartimath SV, Xuan TY, Khanapur S, Jieu B, Chin HX, et al. Granzyme B PET imaging of combined chemotherapy and immune checkpoint inhibitor therapy in colon cancer. Mol Imaging Biol. (2021) 23:714–23. doi: 10.1007/s11307-021-01596-y
91. Pham TT, Chenoweth A, Patel N, Banu A, Osborn G, Blower PJ, et al. In vivo PET imaging of (89)Zr-labeled natural killer cells and the modulating effects of a therapeutic antibody. J Nucl Med. (2024) 65:1035–42. doi: 10.2967/jnumed.124.267876
92. Burga RA, Khan DH, Agrawal N, Bollard CM, Fernandes R. Designing magnetically responsive biohybrids composed of cord blood-derived natural killer cells and iron oxide nanoparticles. Bioconjugate Chem. (2019) 30:552–60. doi: 10.1021/acs.bioconjchem.9b00048
93. Lechuga LM, Forsberg MH, Walker KL, Ludwig KD, Capitini CM, Fain SB. Detection and viability of murine NK cells in vivo in a lymphoma model using fluorine-19 MRI. NMR biomed. (2021) 34:e4600. doi: 10.1002/nbm.4600
94. Chong L, Jiang YW, Wang D, Chang P, Xu K, Li J. Targeting and repolarizing M2-like tumor-associated macrophage-mediated MR imaging and tumor immunotherapy by biomimetic nanoparticles. J nanobiotechnol. (2023) 21:401. doi: 10.1186/s12951-023-02122-8
95. Kvedaraite E, Ginhoux F. Human dendritic cells in cancer. Sci Immunol. (2022) 7:eabm9409. doi: 10.1126/sciimmunol.abm9409
96. Pittet MJ, Di Pilato M, Garris C, Mempel TR. Dendritic cells as shepherds of T cell immunity in cancer. Immunity. (2023) 56:2218–30. doi: 10.1016/j.immuni.2023.08.014
97. Saito Y, Komori S, Kotani T, Murata Y, Matozaki T. The role of type-2 conventional dendritic cells in the regulation of tumor immunity. Cancers. (2022) 14:1976. doi: 10.3390/cancers14081976
98. Luo J, Pang S, Hui Z, Zhao H, Xu S, Yu W, et al. Blocking Tim-3 enhances the anti-tumor immunity of STING agonist ADU-S100 by unleashing CD4(+) T cells through regulating type 2 conventional dendritic cells. Theranostics. (2023) 13:4836–57. doi: 10.7150/thno.86792
99. Wculek SK, Cueto FJ, Mujal AM, Melero I, Krummel MF, Sancho D. Dendritic cells in cancer immunology and immunotherapy. Nat Rev Immunol. (2020) 20:7–24. doi: 10.1038/s41577-019-0210-z
100. Bulte JWM, Shakeri-Zadeh A. In vivo MRI tracking of tumor vaccination and antigen presentation by dendritic cells. Mol Imaging Biol. (2022) 24:198–207. doi: 10.1007/s11307-021-01647-4
101. de Vries IJ, Lesterhuis WJ, Barentsz JO, Verdijk P, van Krieken JH, Boerman OC, et al. Magnetic resonance tracking of dendritic cells in melanoma patients for monitoring of cellular therapy. Nat Biotechnol. (2005) 23:1407–13. doi: 10.1038/nbt1154
102. Au-Yeung YMB, Zeng Z, Huang J. Abstract 6747: Engineering M13 filamentous phages to target dendritic cells and elicit anti-tumor immunity. Cancer Res. (2024) 84:6747–. doi: 10.1158/1538-7445.AM2024-6747
103. Yu H, Guo H, Zu H, Ding H, Lin S, Wang Y, et al. Therapeutic dendritic cell vaccines engineered with antigen-biomineralized Bi2S3 nanoparticles for personalized tumor radioimmunotherapy. Aggregate. (2022) 3:e194. doi: 10.1002/agt2.194
104. Reeves KM, Song PN, Angermeier A, Della Manna D, Li Y, Wang J, et al. (18)F-FMISO PET imaging identifies hypoxia and immunosuppressive tumor microenvironments and guides targeted evofosfamide therapy in tumors refractory to PD-1 and CTLA-4 inhibition. Clin Cancer Res. (2022) 28:327–37. doi: 10.1158/1078-0432.Ccr-21-2394
105. Ahn SB, Lee SB, Singh TD, Cho SJ, Kim SK, Lee IK, et al. Multimodality imaging of bone marrow-derived dendritic cell migration and antitumor immunity. Trans Oncol. (2017) 10:262–70. doi: 10.1016/j.tranon.2017.01.003
106. Hedrick CC, Malanchi I. Neutrophils in cancer: heterogeneous and multifaceted. Nat Rev Immunol. (2022) 22:173–87. doi: 10.1038/s41577-021-00571-6
107. Gong YT, Zhang LJ, Liu YC, Tang M, Lin JY, Chen XY, et al. Neutrophils as potential therapeutic targets for breast cancer. Pharmacol Res. (2023) 198:106996. doi: 10.1016/j.phrs.2023.106996
108. Papayannopoulos V. Neutrophil extracellular traps in immunity and disease. Nat Rev Immunol. (2018) 18:134–47. doi: 10.1038/nri.2017.105
109. Giridharan T, Suzuki S, Khan ANH, Stokolosa A, Long MD, Rosario SR, et al. Neutrophils in the tumor microenvironment (TME) induce T cell non-responsiveness characterized by a shift from proliferation and activation to lipid transport pathways. J Immunol. (2023) 210:169.11–.11. doi: 10.4049/jimmunol.210.Supp.169.11
110. Kou M, Lu W, Zhu M, Qu K, Wang L, Yu Y. Massively recruited sTLR9(+) neutrophils in rapidly formed nodules at the site of tumor cell inoculation and their contribution to a pro-tumor microenvironment. Cancer immunol immunother: CII. (2023) 72:2671–86. doi: 10.1007/s00262-023-03451-1
111. Li Y, Liu W, Che K, Lu C, Wei J. Identification of immunosuppressive and tumor-promoting neutrophils in bone marrow of human and human immune system mice. J Immunol. (2023) 210(1_Supplement):84.02. doi: 10.4049/jimmunol.210.Supp.84.02
112. Ji HZ, Liu B, Ren M, Li S, Zheng JF, Liu TY, et al. The CXCLs-CXCR2 axis modulates the cross-communication between tumor-associated neutrophils and tumor cells in cervical cancer. Expert Rev Clin Immunol. (2024) 20:559–69. doi: 10.1080/1744666x.2024.2305808
113. Zhang Y, He S, Xu C, Jiang Y, Miao Q, Pu K. An activatable polymeric nanoprobe for fluorescence and photoacoustic imaging of tumor-associated neutrophils in cancer immunotherapy. Angewandte Chemie (International ed English). (2022) 61:e202203184. doi: 10.1002/anie.202203184
114. Liu X, Duan Y, Hu D, Wu M, Chen C, Ghode PB, et al. Targeted photoacoustic imaging of brain tumor mediated by neutrophils engineered with lipid-based molecular probe. ACS Mater Lett. (2021) 3:1284–90.
115. Chandrasekharan P, Fung KLB, Zhou XY, Cui W, Colson C, Mai D, et al. Non-radioactive and sensitive tracking of neutrophils towards inflammation using antibody functionalized magnetic particle imaging tracers. Nanotheranostics. (2021) 5:240–55. doi: 10.7150/ntno.50721
116. Spatz P, Chen X, Reichau K, Huber ME, Mühlig S, Matsusaka Y, et al. Development and initial characterization of the first (18)F-CXCR2-targeting radiotracer for PET imaging of neutrophils. J medicinal Chem. (2024) 67:6327–43. doi: 10.1021/acs.jmedchem.3c02285
117. Lasser SA, Ozbay Kurt FG, Arkhypov I, Utikal J, Umansky V. Myeloid-derived suppressor cells in cancer and cancer therapy. Nat Rev Clin Oncol. (2024) 21:147–64. doi: 10.1038/s41571-023-00846-y
118. Barry ST, Gabrilovich DI, Sansom OJ, Campbell AD, Morton JP. Therapeutic targeting of tumour myeloid cells. Nat Rev Cancer. (2023) 23:216–37. doi: 10.1038/s41568-022-00546-2
119. Hegde S, Leader AM, Merad M. MDSC: Markers, development, states, and unaddressed complexity. Immunity. (2021) 54:875–84. doi: 10.1016/j.immuni.2021.04.004
120. Liu H, Ling CC, Yeung WHO, Pang L, Liu J, Zhou J, et al. Monocytic MDSC mobilization promotes tumor recurrence after liver transplantation via CXCL10/TLR4/MMP14 signaling. Cell Death Dis. (2021) 12:489. doi: 10.1038/s41419-021-03788-4
121. Zinnhardt B, Müther M, Roll W, Backhaus P, Jeibmann A, Foray C, et al. TSPO imaging-guided characterization of the immunosuppressive myeloid tumor microenvironment in patients with Malignant glioma. Neuro Oncol. (2020) 22:1030–43. doi: 10.1093/neuonc/noaa023
122. Zhao Y, Pan Y, Zou K, Lan Z, Cheng G, Mai Q, et al. Biomimetic manganese-based theranostic nanoplatform for cancer multimodal imaging and twofold immunotherapy. Bioactive materials. (2023) 19:237–50. doi: 10.1016/j.bioactmat.2022.04.011
123. Yan L, Li J, Zhang C. The role of MSCs and CAR-MSCs in cellular immunotherapy. Cell communication signaling: CCS. (2023) 21:187. doi: 10.1186/s12964-023-01191-4
124. Egan H, Treacy O, Lynch K, Leonard NA, O'Malley G, Reidy E, et al. Targeting stromal cell sialylation reverses T cell-mediated immunosuppression in the tumor microenvironment. Cell Rep. (2023) 42:112475. doi: 10.1016/j.celrep.2023.112475
125. Wu R, Fan X, Wang Y, Shen M, Zheng Y, Zhao S, et al. Mesenchymal stem cell-derived extracellular vesicles in liver immunity and therapy. Front Immunol. (2022) 13:833878. doi: 10.3389/fimmu.2022.833878
126. Kitzberger C, Shehzad K, Morath V, Spellerberg R, Ranke J, Steiger K, et al. Interleukin-6-controlled, mesenchymal stem cell-based sodium/iodide symporter gene therapy improves survival of glioblastoma-bearing mice. Mol Ther oncolytics. (2023) 30:238–53. doi: 10.1016/j.omto.2023.08.004
127. Lin Z, Wu Y, Xu Y, Li G, Li Z, Liu T. Mesenchymal stem cell-derived exosomes in cancer therapy resistance: recent advances and therapeutic potential. Mol Cancer. (2022) 21:179. doi: 10.1186/s12943-022-01650-5
128. Zhu K, Jiang D, Wang K, Zheng D, Zhu Z, Shao F, et al. Conductive nanocomposite hydrogel and mesenchymal stem cells for the treatment of myocardial infarction and non-invasive monitoring via PET/CT. J nanobiotechnol. (2022) 20:211. doi: 10.1186/s12951-022-01432-7
129. Patrick PS, Kolluri KK, Zaw Thin M, Edwards A, Sage EK, Sanderson T, et al. Lung delivery of MSCs expressing anti-cancer protein TRAIL visualised with (89)Zr-oxine PET-CT. Stem Cell Res Ther. (2020) 11:256. doi: 10.1186/s13287-020-01770-z
130. Gabai Y, Assouline B, Ben-Porath I. Senescent stromal cells: roles in the tumor microenvironment. Trends Cancer. (2023) 9:28–41. doi: 10.1016/j.trecan.2022.09.002
131. van der Heide CD, Dalm SU. Radionuclide imaging and therapy directed towards the tumor microenvironment: a multi-cancer approach for personalized medicine. Eur J Nucl Med Mol Imaging. (2022) 49:4616–41. doi: 10.1007/s00259-022-05870-1
132. Kratochwil C, Fendler WP, Eiber M, Hofman MS, Emmett L, Calais J, et al. Joint EANM/SNMMI procedure guideline for the use of (177)Lu-labeled PSMA-targeted radioligand-therapy ((177)Lu-PSMA-RLT). Eur J Nucl Med Mol Imaging. (2023) 50:2830–45. doi: 10.1007/s00259-023-06255-8
133. He S, Patrick M, Reeves JW, Danaher P, Preciado J, Phan J, et al. Abstract 5637: Path to the holy grail of spatial biology: Spatial single-cell whole transcriptomes using 6000-plex spatial molecular imaging on FFPE tissue. Cancer Res. (2023) 83:5637. doi: 10.1158/1538-7445.AM2023-5637
134. Wu J, Mayer AT, Li R. Integrated imaging and molecular analysis to decipher tumor microenvironment in the era of immunotherapy. Semin Cancer Biol. (2022) 84:310–28. doi: 10.1016/j.semcancer.2020.12.005
135. Lavie D, Ben-Shmuel A, Erez N, Scherz-Shouval R. Cancer-associated fibroblasts in the single-cell era. Nat Cancer. (2022) 3:793–807. doi: 10.1038/s43018-022-00411-z
136. Biffi G, Tuveson DA. Diversity and biology of cancer-associated fibroblasts. Physiol Rev. (2021) 101:147–76. doi: 10.1152/physrev.00048.2019
137. Kennel KB, Bozlar M, De Valk AF, Greten FR. Cancer-associated fibroblasts in inflammation and antitumor immunity. Clin Cancer Res. (2023) 29:1009–16. doi: 10.1158/1078-0432.Ccr-22-1031
138. Zhang H, Yue X, Chen Z, Liu C, Wu W, Zhang N, et al. Define cancer-associated fibroblasts (CAFs) in the tumor microenvironment: new opportunities in cancer immunotherapy and advances in clinical trials. Mol Cancer. (2023) 22:159. doi: 10.1186/s12943-023-01860-5
139. Jenkins L, Jungwirth U, Avgustinova A, Iravani M, Mills A, Haider S, et al. Cancer-associated fibroblasts suppress CD8+ T-cell infiltration and confer resistance to immune-checkpoint blockade. Cancer Res. (2022) 82:2904–17. doi: 10.1158/0008-5472.Can-21-4141
140. Jiang Y, Tian Y, Feng B, Zhao T, Du L, Yu X, et al. A novel molecular imaging probe [(99m)Tc]Tc-HYNIC-FAPI targeting cancer-associated fibroblasts. Sci Rep. (2023) 13:3700. doi: 10.1038/s41598-023-30806-6
141. Yang W, Chen Z, Qu L, Zhang C, Chen H, Zheng J, et al. IR-780 dye-based targeting of cancer-associated fibroblasts improves cancer immunotherapy by increasing intra-tumoral T lymphocytes infiltration. Curr Cancer Drug Targets. (2024) 24:642–53. doi: 10.2174/0115680096261142231018104854
142. Hallett RM, Poplawski SE, Dornan MH, Ahn SH, Pan S, Wengen W, et al. Abstract 3303: Pre-clinical characterization of the novel FAP targeting ligand PNT6555 for imaging and therapy of cancer. Cancer Res. (2022) 82:3303–. doi: 10.1158/1538-7445.AM2022-3303
143. Mukkamala R, Spencer LD, Daniel CJ, Tudi P, Kaine MN, Horner A, et al. Abstract 1119: Novel fibroblast activation protein targeting radioligand for imaging and radiotherapy of solid tumors. Cancer Res. (2024) 84:1119–. doi: 10.1158/1538-7445.AM2024-1119
144. Meng L, Fang J, Zhao L, Wang T, Yuan P, Zhao Z, et al. Rational design and pharmacomodulation of protein-binding theranostic radioligands for targeting the fibroblast activation protein. J medicinal Chem. (2022) 65:8245–57. doi: 10.1021/acs.jmedchem.1c02162
145. Li K, Liu W, Yu H, Chen J, Tang W, Wang J, et al. 68Ga-FAPI PET imaging monitors response to combined TGF-βR inhibition and immunotherapy in metastatic colorectal cancer. J Clin Invest. (2024) 134:e170490. doi: 10.1172/jci170490
146. Cooper J, Giancotti FG. Integrin signaling in cancer: mechanotransduction, stemness, epithelial plasticity, and therapeutic resistance. Cancer Cell. (2019) 35:347–67. doi: 10.1016/j.ccell.2019.01.007
147. Slack RJ, Macdonald SJF, Roper JA, Jenkins RG, Hatley RJD. Emerging therapeutic opportunities for integrin inhibitors. Nat Rev Drug Discovery. (2022) 21:60–78. doi: 10.1038/s41573-021-00284-4
148. Sparkenbaugh EM, Henderson MW, Miller-Awe M, Abrams C, Ilich A, Trebak F, et al. Factor XII contributes to thrombotic complications and vaso-occlusion in sickle cell disease. Blood. (2023) 141:1871–83. doi: 10.1182/blood.2022017074
149. Liu F, Wu Q, Dong Z, Liu K. Integrins in cancer: Emerging mechanisms and therapeutic opportunities. Pharmacol Ther. (2023) 247:108458. doi: 10.1016/j.pharmthera.2023.108458
150. Quigley NG, Steiger K, Hoberück S, Czech N, Zierke MA, Kossatz S, et al. PET/CT imaging of head-and-neck and pancreatic cancer in humans by targeting the "Cancer Integrin" αvβ6 with Ga-68-Trivehexin. Eur J Nucl Med Mol Imaging. (2022) 49:1136–47. doi: 10.1007/s00259-021-05559-x
151. Liu W, Ma H, Li F, Cai H, Liang R, Chen X, et al. PET imaging of VEGFR and integrins in glioma tumor xenografts using (89)Zr labelled heterodimeric peptide. Bioorganic medicinal Chem. (2022) 59:116677. doi: 10.1016/j.bmc.2022.116677
152. Zhao L, Wen X, Xu W, Pang Y, Sun L, Wu X, et al. Clinical evaluation of (68)Ga-FAPI-RGD for imaging of fibroblast activation protein and integrin α(v)β(3) in various cancer types. J Nucl Med. (2023) 64:1210–7. doi: 10.2967/jnumed.122.265383
153. Wang R, Jakobsson V, Wang J, Zhao T, Peng X, Li B, et al. Dual targeting PET tracer [(68)Ga]Ga-FAPI-RGD in patients with lung neoplasms: a pilot exploratory study. Theranostics. (2023) 13:2979–92. doi: 10.7150/thno.86007
154. de Almeida LGN, Thode H, Eslambolchi Y, Chopra S, Young D, Gill S, et al. Matrix metalloproteinases: from molecular mechanisms to physiology, pathophysiology, and pharmacology. Pharmacol Rev. (2022) 74:712–68. doi: 10.1124/pharmrev.121.000349
155. Wang M, Chen J, Zhao S, Zheng J, He K, Liu W, et al. Atrazine promotes breast cancer development by suppressing immune function and upregulating MMP expression. Ecotoxicol Environ Saf. (2023) 253:114691. doi: 10.1016/j.ecoenv.2023.114691
156. Kwon MJ. Matrix metalloproteinases as therapeutic targets in breast cancer. Front Oncol. (2022) 12:1108695. doi: 10.3389/fonc.2022.1108695
157. Pringle TA, Chan CD, Luli S, Blair HJ, Rankin KS, Knight JC. Synthesis and in vivo evaluation of a site-specifically labeled radioimmunoconjugate for dual-modal (PET/NIRF) imaging of MT1-MMP in sarcomas. Bioconjugate Chem. (2022) 33:1564–73. doi: 10.1021/acs.bioconjchem.2c00306
158. Gálvez-Rodríguez A, Ferino-Pérez A, Rodríguez-Riera Z, Guerra IR, Jáuregui-Haza UJ. In silico evaluation of new mangiferin-based Positron Emission Tomography radiopharmaceuticals through the inhibition of metalloproteinase-9. J Mol Graphics Model. (2023) 124:108569. doi: 10.1016/j.jmgm.2023.108569
159. Kasten BB, Jiang K, Cole D, Jani A, Udayakumar N, Gillespie GY, et al. Targeting MMP-14 for dual PET and fluorescence imaging of glioma in preclinical models. Eur J Nucl Med Mol Imaging. (2020) 47:1412–26. doi: 10.1007/s00259-019-04607-x
160. Tu Y, Ma X, Chen H, Fan Y, Jiang L, Zhang R, et al. Molecular imaging of matrix metalloproteinase-2 in atherosclerosis using a smart multifunctional PET/MRI nanoparticle. Int J nanomed. (2022) 17:6773–89. doi: 10.2147/ijn.S385679
161. Vivier E, Rebuffet L, Narni-Mancinelli E, Cornen S, Igarashi RY, Fantin VR. Natural killer cell therapies. Nature. (2024) 626:727–36. doi: 10.1038/s41586-023-06945-1
162. Cheng K, Cai N, Zhu J, Yang X, Liang H, Zhang W. Tumor-associated macrophages in liver cancer: From mechanisms to therapy. Cancer Commun (London England). (2022) 42:1112–40. doi: 10.1002/cac2.12345
163. Cheng B, Huang H. Expanding horizons in overcoming therapeutic resistance in castration-resistant prostate cancer: targeting the androgen receptor-regulated tumor immune microenvironment. Cancer Biol Med. (2023) 20:568–74. doi: 10.20892/j.issn.2095-3941.2023.0256
164. Souza FW, Miao EA. Neutrophils only die twice. Sci Adv. (2023) 9:eadm8715. doi: 10.1126/sciadv.adm8715
165. Chamoto K, Yaguchi T, Tajima M, Honjo T. Insights from a 30-year journey: function, regulation and therapeutic modulation of PD1. Nat Rev Immunol. (2023) 23:682–95. doi: 10.1038/s41577-023-00867-9
166. Mohammed SA, Hetta HF, Zahran AM, Tolba MEM, Attia RAH, Behnsawy HM, et al. T cell subsets, regulatory T, regulatory B cells and proinflammatory cytokine profile in Schistosoma haematobium associated bladder cancer: First report from Upper Egypt. PloS Negl Trop Dis. (2023) 17:e0011258. doi: 10.1371/journal.pntd.0011258
167. Wu B, Shi X, Jiang M, Liu H. Cross-talk between cancer stem cells and immune cells: potential therapeutic targets in the tumor immune microenvironment. Mol Cancer. (2023) 22:38. doi: 10.1186/s12943-023-01748-4
168. Mu W, Jiang L, Shi Y, Tunali I, Gray JE, Katsoulakis E, et al. Non-invasive measurement of PD-L1 status and prediction of immunotherapy response using deep learning of PET/CT images. J Immunother Cancer. (2021) 9:e002118. doi: 10.1136/jitc-2020-002118
169. Chan YH, Teo TH, Torres-Ruesta A, Hartimath SV, Chee RS, Khanapur S, et al. Longitudinal [18F]FB-IL-2 PET imaging to assess the immunopathogenicity of O'nyong-nyong virus infection. Front Immunol. (2020) 11:894. doi: 10.3389/fimmu.2020.00894
170. Kerekes G, Czókolyová M, Hamar A, Pusztai A, Tajti G, Katkó M, et al. Effects of 1-year tofacitinib therapy on angiogenic biomarkers in rheumatoid arthritis. Rheumatol (Oxford England). (2023) 62:Si304–si12. doi: 10.1093/rheumatology/kead502
171. Alluri SR, Higashi Y, Berendzen A, Grisanti LA, Watkinson LD, Singh K, et al. Synthesis and preclinical evaluation of a novel fluorine-18 labeled small-molecule PET radiotracer for imaging of CXCR3 receptor in mouse models of atherosclerosis. EJNMMI Res. (2023) 13:67. doi: 10.1186/s13550-023-01017-x
172. Ramachandran A, Krishnaraju VS, Kumar R, Shukla J, Laroya I. Ga-68 chemokine receptor-4 PET/CT imaging in schminke type of nasopharyngeal carcinoma. Indian J Nucl Med. (2022) 37:284–5. doi: 10.4103/ijnm.ijnm_184_21
173. Zhang X, Qiu L, Sultan DH, Luehmann HP, Yu Y, Zhang X, et al. Development of a CCR2 targeted (18)F-labeled radiotracer for atherosclerosis imaging with PET. Nucl Med Biol. (2024) 130-131:108893. doi: 10.1016/j.nucmedbio.2024.108893
174. Luehmann HP, Pressly ED, Detering L, Wang C, Pierce R, Woodard PK, et al. PET/CT imaging of chemokine receptor CCR5 in vascular injury model using targeted nanoparticle. J Nucl Med. (2014) 55:629–34. doi: 10.2967/jnumed.113.132001
175. Mannes PZ, Barnes CE, Biermann J, Latoche JD, Day KE, Zhu Q, et al. Molecular imaging of chemokine-like receptor 1 (CMKLR1) in experimental acute lung injury. Proc Natl Acad Sci United States America. (2023) 120:e2216458120. doi: 10.1073/pnas.2216458120
176. Schraven S, Brück R, Rosenhain S, Lemainque T, Heines D, Noormohammadian H, et al. CT- and MRI-aided fluorescence tomography reconstructions for biodistribution analysis. Invest Radiol. (2024) 59:504–12. doi: 10.1097/rli.0000000000001052
177. O'Neill B, Kala S, Lim S, Hupple C, Lane N, Sorensen RN, et al. Abstract 4625: A comprehensive guided workflow for highplex imaging, tissue segmentation, and multiplex cellular phenotyping for tumor microenvironment analysis. Cancer Res. (2023) 83:4625–. doi: 10.1158/1538-7445.AM2023-4625
178. Xu L, Xie Y, Lin J, Wu A, Jiang T. Advancements in SERS-based biological detection and its application and perspectives in pancreatic cancer. VIEW. (2024) 5:20230070. doi: 10.1002/VIW.20230070
179. Liu YC, Wang ZX, Pan JY, Wang LQ, Dai XY, Wu KF, et al. Recent advances in imaging agents anchored with pH (Low) insertion peptides for cancer theranostics. Molecules (Basel Switzerland). (2023) 28:2175. doi: 10.3390/molecules28052175
180. Hajfathalian M, Mossburg KJ, Radaic A, Woo KE, Jonnalagadda P, Kapila Y, et al. A review of recent advances in the use of complex metal nanostructures for biomedical applications from diagnosis to treatment. Wiley Interdiscip Rev Nanomed nanobiotechnol. (2024) 16:e1959. doi: 10.1002/wnan.1959
181. Gogoshin G, Rodin AS. Graph neural networks in cancer and oncology research: emerging and future trends. Cancers. (2023) 15:5858. doi: 10.3390/cancers15245858
182. Waqas A, Tripathi A, Ramachandran RP, Stewart PA, Rasool G. Multimodal data integration for oncology in the era of deep neural networks: a review. Front Artif Intell. (2024) 7:1408843. doi: 10.3389/frai.2024.1408843
183. Wang R, Dai W, Gong J, Huang M, Hu T, Li H, et al. Development of a novel combined nomogram model integrating deep learning-pathomics, radiomics and immunoscore to predict postoperative outcome of colorectal cancer lung metastasis patients. J Hematol Oncol. (2022) 15:11. doi: 10.1186/s13045-022-01225-3
184. Wang Y, Hu Y, Ye D. Activatable multimodal probes for in vivo imaging and theranostics. Angew. Chem. Int. (2022) 61. doi: 10.1002/anie.202209512
185. Gao Y, Wu C, Chen X, Ma L, Zhang X, Chen J, et al. PET/CT molecular imaging in the era of immune-checkpoint inhibitors therapy. Front Immunol. (2022) 13:1049043. doi: 10.3389/fimmu.2022.1049043
186. van de Donk PP, Oosting SF, Knapen DG, van der Wekken AJ, Brouwers AH, Lub-de Hooge MN, et al. Molecular imaging to support cancer immunotherapy. J immunother Cancer. (2022) 10:e004949. doi: 10.1136/jitc-2022-004949
187. Vladimirov N, Perlman O. Molecular MRI-based monitoring of cancer immunotherapy treatment response. Int J Mol Sci. (2023) 24:3151. doi: 10.3390/ijms24043151
188. Merrill JR, Inguscio A, Chung T, Demestichas B, Garcia LA, Habel J, et al. Sensitive, non-immunogenic in vivo imaging of cancer metastases and immunotherapy response. Cell Stress. (2023) 7:59–68. doi: 10.15698/cst2023.08.288
189. Wang D, Sun Z, Zhu X, Zheng X, Zhou Y, Lu Y, et al. GARP-mediated active TGF-β1 induces bone marrow NK cell dysfunction in AML patients with early relapse post-allo-HSCT. Blood. (2022) 140:2788–804. doi: 10.1182/blood.2022015474
190. Dannhauser D, Rossi D, Palatucci AT, Rubino V, Carriero F, Ruggiero G, et al. Non-invasive and label-free identification of human natural killer cell subclasses by biophysical single-cell features in microfluidic flow. Lab chip. (2021) 21:4144–54. doi: 10.1039/d1lc00651g
191. Ning J, Davis Z, Zhu S, Miller J, Chen C. SURG-09. Targeting a shared glioblastoma cell state through adaptive natural killer cells augmented with trispecific killer cell engager (TriKE) immune-modulator against CD276. Neuro-Oncology. (2023) 25:v263–v. doi: 10.1093/neuonc/noad179.1008
192. Park HS, Kim J, Cho MY, Cho YJ, Suh YD, Nam SH, et al. Effectual labeling of natural killer cells with upconverting nanoparticles by electroporation for in vivo tracking and biodistribution assessment. ACS Appl materials interfaces. (2020) 12:49362–70. doi: 10.1021/acsami.0c12849
193. Yoshida Y, Yoshio S, Yamazoe T, Mori T, Tsustui Y, Kawai H, et al. Phenotypic characterization by single-cell mass cytometry of human intrahepatic and peripheral NK cells in patients with hepatocellular carcinoma. Cells. (2021) 10:1495. doi: 10.3390/cells10061495
194. Segerberg F, Lambert M, Sanz-Ortega L, Andersson A, Childs RW, Carlsten M. Improved leukemia clearance after adoptive transfer of NK cells expressing the bone marrow homing receptor CXCR4(R334X). HemaSphere. (2023) 7:e974. doi: 10.1097/hs9.0000000000000974
195. Albinger N, Müller S, Kostyra J, Kuska J, Mertlitz S, Penack O, et al. Manufacturing of primary CAR-NK cells in an automated system for the treatment of acute myeloid leukemia. Bone marrow Transplant. (2024) 59:489–95. doi: 10.1038/s41409-023-02180-4
Keywords: molecular imaging, tumor microenvironment, tumor immune, immunotherapy, immune cells
Citation: Wang X, Shen W, Yao L, Li C, You H and Guo D (2025) Current status and future prospects of molecular imaging in targeting the tumor immune microenvironment. Front. Immunol. 16:1518555. doi: 10.3389/fimmu.2025.1518555
Received: 28 October 2024; Accepted: 02 January 2025;
Published: 22 January 2025.
Edited by:
Haibo Zhang, Zhejiang Provincial People’s Hospital, ChinaReviewed by:
Dongyao Wang, University of Science and Technology of China, ChinaCopyright © 2025 Wang, Shen, Yao, Li, You and Guo. This is an open-access article distributed under the terms of the Creative Commons Attribution License (CC BY). The use, distribution or reproduction in other forums is permitted, provided the original author(s) and the copyright owner(s) are credited and that the original publication in this journal is cited, in accordance with accepted academic practice. No use, distribution or reproduction is permitted which does not comply with these terms.
*Correspondence: Duancheng Guo, Z3VvZHVhbmNoZW5nQHNoY2Eub3JnLmNu; Huiming You, eW91aHVpbUAxNjMuY29t
Disclaimer: All claims expressed in this article are solely those of the authors and do not necessarily represent those of their affiliated organizations, or those of the publisher, the editors and the reviewers. Any product that may be evaluated in this article or claim that may be made by its manufacturer is not guaranteed or endorsed by the publisher.
Research integrity at Frontiers
Learn more about the work of our research integrity team to safeguard the quality of each article we publish.