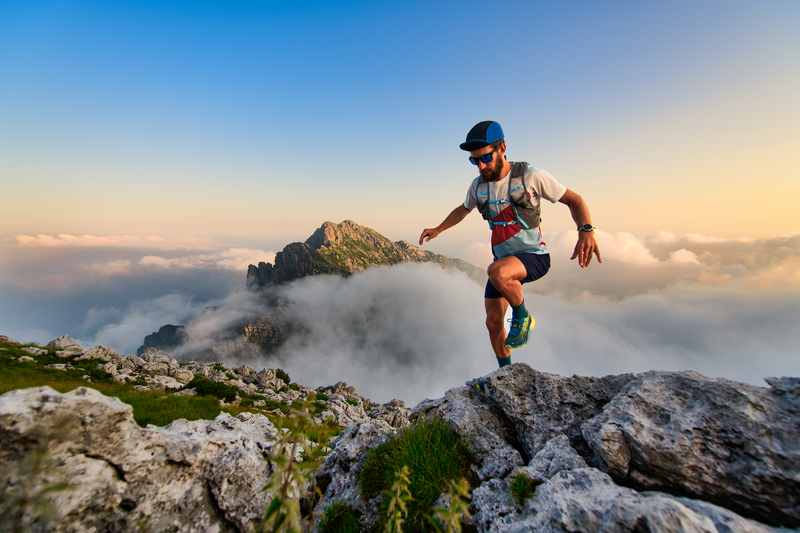
95% of researchers rate our articles as excellent or good
Learn more about the work of our research integrity team to safeguard the quality of each article we publish.
Find out more
REVIEW article
Front. Immunol. , 28 January 2025
Sec. Inflammation
Volume 16 - 2025 | https://doi.org/10.3389/fimmu.2025.1489015
This article is part of the Research Topic Cholesterol, inflammation and immunity View all 4 articles
As an immune and metabolic organ, the liver affects the progression and prognosis of sepsis. Despite the severe adverse effects of sepsis liver injury on the body, treatment options remain limited. Sphingosine-1-phosphate (S1P) is a widely distributed lipid signaling molecule that binds to five sphingosine-1-phosphate receptors (S1PR) to regulate downstream signaling pathways involved in the pathophysiological processes of sepsis, including endothelial permeability, cytokine release, and vascular tone. This review summarizes current research on the role of S1P in normal liver biology and describes the mechanisms by which changes in S1P/S1PR affect the development of liver-related diseases. At the same time, the pathological processes underlying liver injury, as evidenced by clinical manifestations during sepsis, were comprehensively reviewed. This paper focused on the mechanistic pathways through which S1P and its receptors modulate immunity, bile acid metabolism, and liver-intestinal circulation in septic liver injury. Finally, the relationships between S1P and its receptors with liver inflammation and metabolism and the use of related drugs for the treatment of liver injury were examined. By elucidating the role of S1P and its receptor in the pathogenesis of sepsis liver injury, this review established a molecular targeting framework, providing novel insights into clinical and drug development.
Sepsis is a life-threatening condition hallmarked by organ dysfunction caused by a dysregulated host response to infection (1, 2). A 2016 systematic review estimated the current epidemiology of sepsis at approximately 30 million exacerbations, resulting in 6 million deaths annually worldwide (3). Owing to its special anatomical location, the liver is one of the most critically affected organs by sepsis (4). According to epidemiological studies, the average incidence of liver dysfunction in patients with sepsis is 39.9%, with the mortality rate of septic patients with liver insufficiency or failure as high as 54%-68% (5). Notably, a significant relationship was identified between the occurrence or deterioration of liver dysfunction and mortality during septic shock (6). The pathogenesis of sepsis-induced liver injury is intricate and is associated with an imbalance between pro-inflammatory and anti-inflammatory responses caused by the activation of inflammatory factors, the generation of oxygen free radicals and subsequent peroxide reaction, the obstruction of bile acid secretion and excretion, the vicious circle involving the gut-liver axis, hypoperfusion-induced microcirculation disturbances, hepatocyte autophagy, direct or indirect hepatocyte apoptosis caused by endotoxins, and so on (7, 8). Thus, liver dysfunction can severely compromise the outcome of sepsis and has been identified as a strong independent predictor of mortality in the intensive care unit (9).
Sphingosine-1-phosphate (S1P) is a signaling lipid that is ubiquitous in human body fluids, tissues, and cells (10). S1P biosynthesis occurs in the endoplasmic reticulum, where L-serine and palmitoyl-CoA undergo a series of reduction, transformation, and modification reactions to form ceramide, which is deacylated into sphingosine and finally phosphorylated by two sphingosine kinases, namely SphK1 and SphK2, to form S1P (11, 12). The cytosolic level of S1P is regulated by sphingosine-1-phosphate phosphatase and sphingosine-1-phosphate lyase (13). Specifically, S1P can be dephosphorylated by the former or irreversibly degraded to phosphoethanolamine and hexadecenal by the latter (13). S1P is predominantly distributed in the serum and lymph in the human body and is primarily transported by concentration gradients (14). Moreover, it is exported from cells and can act as an autocrine and paracrine signaling molecule in the surrounding environment or in distant tissues (15).
S1P can regulate various pathophysiological processes in sepsis, including endothelial permeability, cytokine release, and vascular tone (16). Furthermore, it exerts a significant influence on the physiological and pathological changes in the immune system, lung tissue, and liver tissue by binding to different G protein-coupled receptors (GPCRS), namely S1PR1-5 (17, 18). S1P and its receptors can further alleviate hepatocyte injury and liver metabolic disorders by mitigating the inflammatory response and improving vascular permeability in sepsis (19, 20). In clinical trials, serum S1P concentration was lower in patients with sepsis compared to normal controls, with the loss of serum S1P being correlated with disease severity (21, 22). The distribution and action of S1P can affect the degree of liver injury in sepsis (23). For instance, in mouse models of lipopolysaccharide (LPS)-induced liver injury and inflammation, LPS-induced inflammation up-regulates hepatic S1PR1 and S1PR3 expression (24, 25). Pretreatment of mice with 4-deoxypyridoxine (DOP) or the sphingosine analog FTY720 attenuated the disruption in pulmonary and hepatic vascular barriers after the development of sepsis (26). While existing evidence indicates that S1P and its receptors may play a key role in mediating immunity and metabolism in sepsis-induced liver injury, a systematic review of the literature is lacking. Therefore, this article reviewed the recent research progress on S1P and its receptors as potential therapeutic targets in the immunoregulation and metabolism of sepsis-induced liver injury.
As is well documented, the liver is a vital organ that regulates S1P levels in the blood (27, 28). Indeed, hepatectomy has been demonstrated to reduce plasma S1P levels, suggesting that the liver may be involved in regulating plasma S1P levels in vivo (29).
Extracellular vesicles (EVs) are small lipid bilayer-enclosed nanovesicles released by various cell types and play a key role in intercellular communication, including the transmission of inflammatory signals, promotion of angiogenesis and tissue remodeling (30). The EV membrane is rich in cholesterol and sphingomyelin (31). In lipotoxicity-induced liver inflammation, EVs secreted by hepatocytes are rich in S1P and mediate the chemotaxis of macrophages through the S1P1 receptor (32). Studies have shown that hepatocyte-derived exosomes may be potential biomarkers of liver disease (33). In addition, exosome-induced hepatocyte proliferation depends on the intracellular production of S1P (33).
S1P is released from primary synthetic cells, and during transportation, approximately 60% of S1P is bound to apolipoprotein M (ApoM) in high-density lipoprotein (HDL), whilst the remaining 40% is bound to albumin (34). However, ApoM is typically synthesized in the liver (35). Plasma S1P levels were reduced by 50% in apoM-deficient mice, demonstrating a direct correlation between circulating S1P levels and ApoM (36). The same connection exists in humans (37). In the liver, S1PR1-5 receptor subtypes are present (38, 39). Among them, S1PR1, S1PR2, and S1PR3 are all highly expressed in the liver. In contrast, the levels of S1PR4 and S1PR5 expression are too low to be detectable (40).
S1P is closely related to inflammatory markers (41). In circulation, S1P bound to HDL exerts an effective anti-inflammatory effect on smooth muscle cells by inhibiting the expression of inflammatory genes stimulated by TNF-α (42). However, surprisingly, S1P exhibits completely opposite effects when binding to different receptors. The SPHK1/S1P/S1PR1 signaling pathway plays a crucial role in sphingolipid metabolism and signal transduction (25). Studies have shown that the activation of SPHK1 further triggers downstream molecules such as TRAF-2, NFκB, Ras, and PLC, promoting the production of inflammatory factors and affecting cell survival, proliferation, migration, and apoptosis (25). Additionally, S1P promotes the generation of TNF-α, IL-1, IL-6, and monocyte chemoattractant proteins through S1PR2, and S1PR2 can also activate the RhoA/ROCK signaling pathway, leading to pyroptosis of THP-1 cells (43, 44). Moreover, in macrophages, S1P is catalyzed by SphK1 and binds to S1PR3 to regulate aerobic glycolysis and influence macrophage polarization, mediating the inflammatory response in sepsis (45). S1PR4 expression is downregulated in LPS/IFN-γ-induced primary M1 macrophages, but no significant changes are observed in M2 macrophages (46). Subsequent studies have shown that the deficiency of S1PR4 promotes the activation of pro-inflammatory macrophages (46). In the research of chronic obstructive pulmonary disease, it was found that the increased expression of S1PR5 may lead to macrophage dysfunction (47).
S1P plays a decisive role in liver physiology and metabolism (48). S1PR2 can be activated by taurocholate (TCA) and other conjugated bile acids, which in turn regulates hepatic lipid and sterol metabolism by up-regulating the expression and activity of SphK2, increasing the gene expression of nuclear S1P, inhibiting specific histone deacetylases (HDAC), and increasing histone acetylation (49, 50). In addition to the promoting role shown in lipid metabolism, S1P also plays a pivotal role in governing glucose metabolism, glycogen deposition, and lipid accumulation in hepatocytes (51). For example, ApoM/S1P exerts protective effects against insulin resistance by activating insulin signaling pathways, such as AKT and AMPK pathways, to enhance mitochondrial function (52). Additionally, bile acid-activated S1PR2 displays insulin-like activity in hepatic glucose regulation, whereas S1PR2 antagonists prevent palmitic acid-mediated insulin resistance (53). Therefore, the determination of S1P levels in bile and its target organs, such as the liver, bile ducts, and intestine, is essential to further elucidate the role of S1P in the pathophysiology of hepatobiliary diseases (54).
The liver is considered to be one of the major organs of the immune system and is principally subdivided into parenchymal and nonparenchymal cells (55). Hepatic parenchymal cells are hepatocytes (HCS) and cholangiocytes, which account for 60% to 80% of liver tissue and function as part of the “hepatic immune system” (56). Nonparenchymal cells, namely liver sinusoidal endothelial cells (LSECs), liver satellite cells, Kupffer cells, neutrophils, monocytes, T and B lymphocytes, natural killer (NK) cells, and NKT cells, also possess immune functions (57). Collectively, these cells constitute liver physiological functions, including nutrient metabolism, drug detoxification, and immune response (58). Of note, immune cells make up a large proportion of the liver composition (59). For example, macrophages are abundant in healthy rodent livers, with 20 to 40 macrophages per 100 hepatocytes (55). A diverse range of S1PR expression patterns was noted in the immune system (Table 1).
Recent studies have reported that the S1P/S1PR axis also plays an integral role in acute liver failure, metabolic syndrome, non-alcoholic fatty liver disease, liver fibrosis, and liver cancer (60). For example, S1P plays a key role in acetaminophen-induced acute liver injury by promoting endoplasmic reticulum stress and mitochondrial permeability transition (61) and also affects cancer progression by regulating histone deacetylase (HDAC1/2) and NF-κB pathways (62). The S1P/S1PR1 axis delays the development of alcoholic liver disease by preventing the migration of Th17 (helper T cells) and their accumulation in the liver and intestine (63). Meanwhile, SphKs and its product S1P have been described to regulate hepatocyte apoptosis and survival (39). In summary, sphingosine-1-phosphate (S1P) regulates pathophysiological processes in the liver, encompassing liver regeneration, endothelial barrier, and immune response (64).
In a previous study, S1P levels measured by HPLC were elevated in human fibrotic livers, accompanied by an increased expression level of S1PR1 and S1PR3 in myofibroblasts (65, 66). This may be ascribed to the upregulation of S1PR1 and S1PR3, facilitating the differentiation of bone marrow mesenchymal stem cells (BMSC) into myofibroblasts (67). S1PR1/3 antagonists can attenuate the degree of liver fibrosis by suppressing the up-regulation of angiogenesis markers in the damaged liver (65). In addition, S1PR2-siRNA can alleviate liver fibrosis and inflammation by inhibiting the formation of neutrophil extracellular traps (NET) in fatty liver model mice (68). During the early stages of chronic liver disease, S1PR2-mediated activation of neutrophils is implicated in the progression of liver injury and may potentially serve as a novel therapeutic target for cholestatic liver disease (69). Inflammation driven by the NLRP3 inflammasome is a key trigger of liver fibrosis during cholestatic liver injury (70). S1P promotes the activation of the NLRP3 inflammasome and the secretion of pro-inflammatory cytokines (IL-1β and IL-18) through the S1PR2/Gα pathway (71). Treatment with JTE-013, an S1PR2 inhibitor, significantly limited the initiation and activation of the NLRP3 inflammasome in cholestatic liver injury (40, 71). In conclusion, different S1P/S1PR pathways exert distinct effects at various stages of liver fibrosis.
The development of Nonalcoholic steatohepatitis (NASH) is linked to endoplasmic reticulum (ER) stress and necessitates the co-localization of Caspase-2 with S1P (72). Thus, the interrelationship between lipid accumulation, cytokines, and lysophospholipids plays a central role in the pathogenesis of NASH (72). In a mouse model of NASH, liver S1P levels and Sphk1 levels were increased (73). Reem Rida et al. pointed out using HepG2 cells that FTY720P binds to S1PR3 and sequentially activates Gq and phosphatidylinositol 3-kinase (PI3K), which can promote lipid accumulation (73). In an experimental NASH animal model, S1PR1 knockdown inhibited saturated fat-induced NF-κB signaling and decreased the mRNA expression level of TNFα and monocyte chemoattractant protein 1 (MCP1) in HepG2 cells (74). The role of S1P in steatohepatitis is highlighted by the fact that the administration of S1P antagonists to experimental NASH animals eradicates the disease (75). Taken together, these data establish a mechanistic link between sphingolipid signaling and hepatic lipid accumulation and inflammation in NASH, providing valuable insights for the treatment of this disease.
S1P has been shown to promote cell proliferation, migration, invasion, and epithelial-mesenchymal transition (EMT) in Hepatocellular carcinoma (HCC) (60). In an in vitro experiment, the HCC cell line HepG2 was found to secrete S1P, thereby preventing cell proliferation (76). FTY720 not only exerts potent antiangiogenic effects but is also cytotoxic to cancer cells and has been found to effectively inhibit the proliferation of numerous cancer cells (77). At present, FTY720 is regarded as a novel immunosuppressant with potent anticancer properties (78) In the MCA-RH7777 hepatocellular carcinoma cell line model, FTY720 reduced intrahepatic and pulmonary metastases as well as tumorigenesis, possibly through its function as an S1PR1 modulator (79). FTY720 also induced apoptosis in HCC cells by activating protein kinase Cδ signaling (80). In addition, FTY720 inhibited the invasion and proliferation of HCC by down-regulating S1PR1 expression and inhibited the recurrence of HCC after liver transplantation (81). Lastly, Fty720 significantly prolonged the life span of rats with cancerous liver and non-cancerous liver transplantation, highlighting its role in lowering the risk of recurrence and prolonging survival in cancer patients following liver transplantation (81).
Cholestasis is generally associated with sepsis (5). Early liver dysfunction (plasma bilirubin >2 mg/dL) was observed in 11% of critically ill patients in a large prospective multicenter study and was identified as a strong independent risk factor for late mortality (82). During sepsis, the liver is attacked by pathogens, toxins, or inflammatory mediators, and pathological changes can progress from hepatocyte dysfunction to liver injury, eventually culminating in liver failure (83). Liver dysfunction frequently occurs during the early phase of sepsis, primarily due to inflammation and hypoperfusion (84, 85). At the same time, inflammation also triggers the formation of ROS, leading to oxidative stress (86). Septic liver injury is characterized by the following clinical features: hypoxic hepatitis, sepsis-related cholestasis, and hyperbilirubinemia (7). On the one hand, persistent hepatic ischemia, hypoxia and inflammation cause oxygen metabolism within liver cells (87). On the other hand, energy deficiency, endotoxin, or proinflammatory cytokines may cause cholestatic hepatocyte injury by disrupting the outflow and excretion of bile (88). Besides, the hepatobiliary excretion mechanism is particularly sensitive to inflammation (Figure 1), which increases vulnerability to disruption in bile metabolism post-infection. A vicious cycle may be formed upon endotoxin-induced cholestasis, leading to a decrease in bile acid concentration in the small intestine, thereby driving bacterial translocation and endotoxin absorption and further aggravating cholestasis (89).
Figure 1. During sepsis, liver macrophages (Kupffer cells) in hepatic sinusoids synthesize large amounts of inflammatory cytokines (TNF-α, IL-6, IL-1β) through the TLR4-MyD88/NF-κB pathway. Moreover, stellate cells produce chemokine (CCL2), which attracts other immune cells and signals hepatocytes to synthesize acute-phase proteins APPs, amplifying the inflammatory effect and resulting in an inflammatory storm. When an inappropriate immune response or overwhelming inflammation occurs, high levels of DAMP and proinflammatory cytokines are generated in the liver, leading to significant hepatocyte damage, macrophage autophagy, and apoptosis. These cytokines also mediate damage to hepatocyte bile transport system (NTCP; OATP; BSEP; MRP2), which disrupts tight junctions and limits intracellular biotransformation, leading to bile metabolism disorders. In addition to impacting metabolism, inflammation and ischemia lead to bile duct structure damage, with bile flow defects further exacerbating cholestasis. TNF-α, tumour necrosis factor-alpha;IL-6, interleukin-6; IL-1β, interleukin 1 beta; TLR4, Toll-like receptor 4; MyD88,Myeloid Differentiation Primary Response Protein 88; NF-κB, nuclear factor κB; CCL2, C-C motif chemokine ligand 2; DAMP, damage-associated molecular pattern; NTCP, basolateral sodium taurocholate cotransporter; OATP, organic anion transporter; BSEP, tubular bile salt outlet pump; MRP2, tubular conjugate export pump.
Recent studies have concluded that S1P is associated with certain models of acute liver injury (90). Nevertheless, although S1P has been documented to contribute to liver injury in the majority of studies, it may exert protective effects in others. For instance, S1P participates in the recruitment and activation of immune cells during liver injury (71). However, in vitro studies have revealed that S1P protects hepatocytes from apoptotic injury (91).
Hyperactivation of the immune system is a common cause of acute organ injury, including the liver, wherein various liver-resident immune cells, such as Kupffer cells (KCS), liver natural killer T (NKT), and dendritic cells, play an instrumental role (92). This inflammatory cascade not only eliminates bacteria but also damages the liver (93). In an in situ perfusion experiment in piglets, endotoxins caused only pulmonary hypertension and neutropenia, while the levels of tumor necrosis factor α (TNF-α), interleukin (IL) -6, and nitric oxide (NO) were elevated when perfused into the lungs alone, without causing pulmonary edema (94). However, when the liver was included in the perfusion, the endotoxins caused marked hypoxemia and pulmonary edema with significant increases in the levels of TNF-α, IL-6, and NO (94). These findings conjointly suggest that the liver is a major source of cytokine and NO production following exposure to endotoxins (5).
S1P is a chief component in regulating the transport and activation of several immune cells (23). As an important immune organ in the body, the S1P signal transduction pathway is also involved in inflammatory liver injury (23). Indeed, in LPS/D-galactosamine (D-Gal)-induced liver failure, S1P levels were elevated, whilst the expression of SphK1, S1PR1, and S1PR3 was upregulated in the liver, and the levels of serum markers of liver failure, AST and ALT, and the serum cytokines TNF-α, IL-1, and IL-6 were increased (25). We speculate that SphK1 may play up-regulate the expression of S1P in peripheral blood monocytes, Kupffer cells, and liver resident macrophages (95). Treatment with the pan-SPHK inhibitor N, N-dimethylsphingosine reduced mortality in mice with sepsis-induced liver injury, as well as decreased liver inflammation and cell death (96). Furthermore, the Spns2/S1P signaling pathway has been shown to play a regulatory role at different stages of the inflammatory response. Specifically, the Spns2/S1P signaling pathway regulates the intensity of macrophage-mediated inflammation via the lactate-ROS axis during the early stages of sepsis (97). At later stages, it maintains the antimicrobial response of macrophages. Consequently, enhancing this pathway may restore the balance in immune responses during sepsis and prevent early hyperinflammation and late-stage immunosuppression (97). A previous study indicated that down-regulating the SPHK1/S1P/S1PR1 pathway can inhibit inflammation and oxidative stress, thereby relieving LPS-induced acute liver injury (25). Another study postulated that S1PR2 deficiency mediates the type 2 immune response and alleviates sepsis-induced lung injury by promoting IL-33 release from macrophages (98). Meanwhile, both S1PR2 gene deletion and pharmacological inhibition of S1PR2 significantly limited bacterial load, enhanced macrophage phagocytosis, and improved the survival rate of septic mice (99). It is worthwhile emphasizing that S1P promotes the activation of the NLRP3 inflammasome and contributes to the secretion of IL-1β and IL-18 in bone marrow-derived macrophages through S1PR2 (100). Conversely, S1PR2 knockdown inhibits the activation of the NLRP3 inflammasome in bone marrow-derived macrophages and alleviates liver inflammation (71, 100). Inhibition of S1PR3 can inhibit ATP-induced NLRP3 inflammasome activation in macrophages through TWIK2-mediated potassium efflux (101). Therefore, different S1PRs may operate together to optimize innate immunity and manage infection during sepsis.
In addition to macrophages, NK cells have been extensively studied. They constitute an important innate immune cell population in the liver, accounting for 30%-40% and 10%-20% of total intrahepatic lymphocytes in humans and mice, respectively (102). Importantly, NK cells play a critical role in the first-line innate defense and immune surveillance of the liver by eliminating invading pathogens, toxins, and circulating tumor cells. Moreover, they also mediate liver injury by interacting with cytokines such as IFN-γ and TNF-α (103). Mouse liver Kupffer cells are stimulated by bacterial superantigens to synthesize IL-12 and IL-18, which activate NK cells and NK1+ T cells to produce IFN-g (104). IL-12/LPS-activated NK1 T cells exhibited potent cytotoxicity against homologous liver cell lines in vitro (105). Originating from the bone marrow (BM), NK cells are released into the circulation and are abundant in the spleen and liver (106). S1PR5 is a key chemokine receptor in NK cells that regulates the distribution of NK cells in vivo and their trafficking to lesion sites (107). In mice deficient in this receptor, NK cell distribution is altered, with decreased NK cell numbers in the blood and spleen and increased NK cell numbers in the lymph nodes (LN) and BM (108). Noteworthily, S1PR5 knockdown has been shown to reduce the number of circulating NK cells in the liver, spleen, and lungs of mice (109). S1PR5 is required for NK cell mobilization to inflammatory organs (108). Poly (I: C) is a compound that stimulates NK cells and induces the recruitment of NK cells from the spleen to the liver. After treatment with poly (I: C), wild-type NK cells accumulated in the liver (four to five-fold increase) and decreased in the spleen. At the same time, serum ALT/AST levels were marginally elevated, accompanied by mild inflammation and focal necrosis in the liver. In contrast, poly (I:C) treatment failed to cause significant changes in NK cell abundance in spleen or liver of S1PR5-deficient mice (110). PolyI: C induction also significantly up-regulated the expression of S1PR5 in the liver (111). Dong et al. demonstrated that the depletion of NK cells significantly attenuated PolyI: C-induced liver injury (112). Overall, these findings indicate that modulating the distribution of NK cells in the liver by regulating the expression of S1PR5 may be a new therapeutic approach for the treatment of sepsis-induced liver injury.
Bile formation is an active osmotic process that involves the passive movement of organic and inorganic molecules across the tubular membrane and water and also necessitates the involvement of integrated membrane proteins, namely the intact cytoskeleton, tight junctions, and intracellular signaling (88). Bile acids are synthesized from cholesterol in hepatocytes and, after binding to glycine or taurine, are actively transported into the biliary system via ATP-binding cassette (ABC) transporters (113). Likewise, S1P can also be transported to the extracellular space by ATP-binding cassette (ABC) transporters (114). Hepatocytes secrete bile acids through bile salt export proteins (BSEP, ABCB11), ABCB4, and ABCG5/ABCG8 (115). An increasing number of in vitro studies have unveiled that LPS-induced proinflammatory mediators (including TNF-α) impair the downstream expression and functional integrity of hepatocyte transporters, such as BSEP and multidrug resistance-associated protein 2 (mrp-2) (116). Among them, the most prominent transport is the energy-dependent tubular ATP-binding cassette (ABC) transporter required for normal bile secretion, which underlies sepsis-associated cholestasis (117). This inflammatory shock may lead to permanent hepatic excretory dysfunction (85). ABCA1 and ABCG1 play a central role in cholesterol efflux from macrophages during atherosclerosis (118). In an earlier study, S1P and S1PR3 receptors were identified as modulators of ABCA1-mediated cholesterol efflux (118). The fact that S1PR3-deficient macrophages have lower ABCA1 gene expression and reduced cholesterol efflux suggests that sustained S1PR3 signaling is required to maintain basal cholesterol efflux (119). This finding led us to hypothesize the role of S1P and S1PR in the regulation of bile acid secretion in hepatocytes.
In sepsis, inflammatory factors may mediate sepsis-related excretory liver dysfunction through the PI3K pathway (85). Considering that bile acid-induced inflammasome activation has a synergistic effect with LPS, cholestasis further aggravates LPS-induced sepsis (120). It has also been found that in inflammatory cholangiopathies, proinflammatory cytokines stimulate biliary epithelium to produce NO through inducible nitric oxide synthase (NOS2) induction. In turn, NO causes ductal cholestasis through reactive nitrogen oxides (RNOS)-mediated inhibition of adenylate cyclase (AC) and cyclic adenosine monophosphate (cAMP)-dependent HCO3- and Cl- secretory mechanisms (116, 121). The use of JTE-013, a specific inhibitor of S1PR2, may improve cholestatic liver injury induced by common bile duct ligation by reducing hepatitis response and apoptosis (122). Nonetheless, the mechanism by which JTE-013 ameliorates liver injury remains elusive, and its relationship with the PI3K pathway and NO warrants further exploration.
Ascribed to anatomical connections, the theory of hepato-enteric circulation is widely accepted, whereby the gut and liver communicate bi-directly through the biliary tract, portal vein, and systemic circulation (123). At present, existing evidence suggests that the enterohepatic crosstalk between the gut and the liver through bacterial translocation and liver-derived molecules, such as bile acids, play a crucial role in the pathogenesis of sepsis (123). Due to bacterial invasion during sepsis, intestinal barrier disruption and intestinal microbiota dysregulation result in the translocation of intestinal pathogen-associated molecular patterns (PAMP) and damage-associated molecular patterns (DAMP) to the liver and systemic circulation (9). Under physiological conditions, the liver regulates immune defense through mechanisms such as bacterial clearance, cytokine production, acute-phase protein release, and regulation of inflammatory metabolism (124). However, during systemic infections, these inflammatory responses may become excessive, leading to impaired pathogen clearance and impaired hepatic metabolism, which can lead to further damage to the gut barrier (89).
The gut barrier consists of a single-cell layer of epithelium, the local immune system, and the microbiome (125). This barrier includes a mechanical component, namely the biological structure composed of intestinal epithelial cells (IEC) and vascular endothelial cells (EC) (126). Various tight junctions (TJS) are present between cells, which comprise various tight junction proteins and cadherins, such as including ZO-1, occludin, cadherin, claudin, and β-catenin. These junctions can resist the invasion of pathogens and harmful substances from infiltrating the intestinal mucosa and prevent the exudation of substances in the intercellular space (127). From the pathophysiology of intestinal injury, cellular alterations and bacterial toxins can induce intestinal edema and intestinal dysfunction by disrupting normal intercellular junctions, reducing vascular endothelial cadherins and TJS, and increasing vascular permeability (128). In preclinical models of sepsis, changes in tight junctions were observed after 1 hour of sepsis onset, with intestinal hyperpermeability persisting for at least 48 hours (125). LPS, a component of the outer wall of Gram-negative bacteria, alters TJ protein assembly, leading to small intestinal leakage, and regulates the inflammatory response through TLR4, further aggravating the changes in TJ (129).
S1P has been described as a barrier-enhancing molecule (130). In a clinical study, plasma S1P levels were reduced, and VE-cadherin levels were increased in patients with septic shock (22). S1P can up-regulate the expression of cell junction-related proteins such as E-cadherin and ZO-1 and promote VE-cadherin translocation, thereby strengthening the intestinal epithelial barrier and reducing the permeability of the intestinal epithelial layer, reducing sepsis-related intestinal injury, and improving the survival rate in sepsis (131, 132). In addition, a significantly negative correlation was noted between the severity of endothelial dysfunction and plasma S1P concentration in sepsis (133). Recent studies have hypothesized that S1P regulates EC biology via various molecular mechanisms, including vascular development and angiogenesis, inflammation, permeability, and the production of reactive oxygen species (ROS), nitric oxide (NO), and hydrogen sulfide (H2S) (134). During sepsis, S1P interacts with the receptors S1PR1 on EC to reduce vascular permeability and maintain the EC barrier through the aforementioned mechanisms (135). Therefore, we posit that S1P plays a major role in intestinal barrier injury during sepsis. In an earlier study investigating alcoholic liver disease, treatment with citrate-ethanol-derived red rice seed skin extract (RRA) increased SPHK2 and S1P levels, improved gut microbiota composition, restored the integrity of the intestinal barrier, reduced plasma and liver LPS levels, and inhibited the activation of the Toll-like receptor 4 (TLR4)/nuclear factor κB (NF-κB) pathway, as well as alleviated liver pathological damage and oxidative stress, reduced inflammation and apoptosis, and enhanced liver function (136). Consequently, alleviating damage to the intestinal barrier function may attenuate hepatic injury (Figure 2). However, not all S1P receptors contribute to barrier enhancement (137). For example, intravascular administration of S1P can prevent inflammatory damage associated with acute lung injury. Conversely, elevated S1P concentrations in vitro may lead to severe disruption of the endothelial cell barrier, potentially through engagement of S1PRs other than S1PR1 (137).
Figure 2. During sepsis, bacterial inflammation leads to the destruction of intercellular junctions in the intestinal barrier, including AJ, TJ, and GJ. In addition, MLCK is associated with inflammatory activation. As a result, the gut’s primitive microbial flora is dysregulated, and bacteria are translocated into the blood. Two injury patterns, PAMP and DAMP, reach the liver via the portal vein and hepatic artery. As the first line of defense, the liver is also damaged by the imbalance between pro-inflammatory and anti-inflammatory factors, the peroxidation cascade, and microcirculation disorders, resulting in inflammatory storms, bile acid metabolism disorders, and impaired bacterial clearance. Among them, elevated bile acid levels exacerbate inflammation and oxidative stress and lead to hepatocyte apoptosis or necrosis. Interestingly, the gut-liver axis further amplifies these effects. Increased intestinal permeability leads to increased systemic inflammation through a positive feedback loop. S1P can enhance liver function and concurrently alleviate liver injury by protecting intestinal barrier function, inhibiting inflammation, minimizing bacterial translocation, and improving bile excretion. TNF, tumor necrosis factor; AJ, adherens junctions; TJ, tight junctions; GJ, gap junctions; MLCK, myosin light chain kinase; PAMP, pathogen-associated molecular pattern; DAMP, damage-associated molecular pattern.
S1P plays a significant role in inflammatory responses, immune regulation, and the maintenance of the intestinal mucosal barrier, and is considered a potential therapeutic biomarker for sepsis (134). In the S1P transduction pathway, some drugs can alter the physiological function of S1P in various diseases by targeting S1P receptors. In addition, sphingosine kinase (SPHK) and S1P lyase (S1PL) are key enzymes that affect the physiological functions of S1P. Increasing or decreasing the activity of these corresponding enzymes to regulate the concentration of S1P in different cells represents a promising treatment for diseases (138).
Fingolimod (FTY-720) is an oral immunomodulatory drug (18) that can competitively bind to S1PR1, leading to receptor internalization and inhibiting the egression of lymphocytes from secondary lymphoid organs, thereby exerting immunosuppressive effects (139). After oral administration, the plasma concentration of FTY720 gradually rises to its peak approximately 12 to 24 hours post-dose (140). Recent research suggests that it is also effective for the treatment of ulcerative colitis (141). In a previous study, FTY-720 reduced S1PR1 levels, inhibited the NF-κb/IL-6/STAT3 cascade, and delayed the progression of colitis-related tumors (142). Furthermore, FTY-720 ameliorates diabetes-induced liver injury by inhibiting oxidative stress and inflammation (143). In preclinical animal models of hepatopulmonary syndrome, FTY-720 reduced systemic inflammation, portal pressure and liver fibrosis, significantly improving the survival rate of the animals (144). Additionally, preliminary studies suggest that FTY-720 may have potential antibacterial effects (145). The above-mentioned results signal that FTY-720 may modulate the regulation of the NF-κB pathway, and its use in sepsis-induced liver injury may inhibit inflammation and oxidative stress. Therefore, FTY-720, as an S1P agonist, holds promise as a candidate hepatoprotective agent.
With the deepening of clinical research, it was found that FTY-720 has low receptor selectivity (146). While activating S1PR1, it also activates S1PR3, leading to the activation of G protein-gated inward rectifier potassium channels in the myocardium, thereby causing adverse reactions such as bradycardia and atrioventricular block. In addition, other side effects have been reported, including headache, flu-like symptoms, diarrhea, back pain and cough, with an incidence rate of 10% or higher; other less common adverse events include elevated liver enzyme levels (8%), macular edema (0.4%) and hypertension (6.5%), which greatly limit its application (147). However, clinical studies have shown that the concurrent use of silymarin can reduce liver complications caused by FTY-720 (148). The effects of FTY-720 on multiple S1P receptor subtypes explain its known and some unexpected adverse reactions. Moreover, new-generation drugs have also been proposed. Siponimod (BAF312) is a dual agonist of S1PR1 and S1PR5, with a selectivity for S1PR1 and S1PR5 about 1000 times higher than that for S1PR2-4, and can significantly and persistently induce the internalization of S1PR1 (18). Current data show that BAF312 has been approved for use in patients with active secondary progressive multiple sclerosis (149). Due to the weak activation of S1PR3 by BAF312, cardiac adverse reactions are less (150). However, other adverse reactions still exist, such as hypertension, lymphopenia, cystoid macular edema and convulsions (150).
Alternatively, Ozamomod is a sphingosine-1-phosphate (S1P) receptor modulator with high affinity for S1P isoforms 1 and 5 (S1P1 and S1P5) (151). In a phase III trial, Ozanimod outperformed placebo as an induction and maintenance therapy in patients with moderately to severely active ulcerative colitis (152). Currently, Ozamomod is approved by the US Food and Drug Administration (FDA) and the European Medicines Agency for the treatment of UC. Notably, S1PR1 agonists can improve barrier function by restoring TJ proteins and inhibiting IEC cell apoptosis (153). Moreover, it can inhibit bacterial translocation by alleviating intestinal barrier damage during sepsis and the liver immune response. Nonetheless, its effects on S1PR5 and in reducing the number of NK cells in the liver to alleviate inflammation remain elusive.
S1P and its receptors play key roles in the immune and metabolic processes of the body and are closely related to various inflammatory responses, oxidative stress, and lipid deposition diseases. As an integral organ for immunity and metabolism, the liver not only protects against the primary attack of bacterial toxins during sepsis but also regulates the metabolism of deposited substances after the attack. S1P and S1PR (1–3) are highly expressed in the liver and can act through various signaling pathways. For instance, the Spns2/S1P signaling pathway plays an inhibitory role during the early stage of the inflammatory response to prevent inflammatory storms and plays a promoting role at later stages to reduce damage caused by immune suppression. Additionally, S1P likely plays an essential role in the regulation of bile acid metabolism, which is particularly relevant in the context of liver injury. Further research on the mechanism of mutual regulation of liver injury, immunity, and metabolism may assist in the discovery of novel therapeutic targets. At present, S1PR drugs and other modulators have shown efficacy in regulating immunity. Thus, future studies should explore new metabolic targets to advance treatment strategies for inflammatory and metabolic disturbances linked to sepsis-induced liver injury.
To date, S1P production has mainly relied on chemical synthesis or blood extraction, but these methods suffer from the limitations of complexity and high cost. To address this problem, a novel approach has been proposed: S1P production using Saccharomyces cerevisiae strains (154). This not only provides new ideas for the genetic manipulation of sphingolipid derivatives, but also demonstrates the potential of biosynthesis in this field. Accurate quantification of S1P, a membrane phospholipid metabolite, has been a challenge. To this end, liquid chromatography-tandem mass spectrometry (LC-MS/MS) was introduced to enable a comprehensive analysis of these lipids and can be used for S1P analysis in plasma and extracellular vesicle components such as macrophages-derived vesicles (155). Furthermore, we observed that S1P concentration changes in blood are different from those in organ tissues after the onset of sepsis. This difference may be due to changes in the total amount or redistribution of S1P in vivo, but the specific regulatory mechanism remains unclear. Future studies are needed to investigate whether S1P regulation of different organs is influenced by the S1PR expression in the organ itself, especially those S1PR that are difficult to measure with existing techniques (such as S1PR4/5 in the liver). These receptors may play an important role through pathway amplification effects rather than solely dependent on their own expression levels. Finally, the determination of S1P levels in bile and its target organs, such as liver, bile ducts, and intestine, under physiological conditions is essential for a deeper understanding of the pathological changes of S1P in hepatobiliary and digestive diseases. It should be noted that there are differences in animal and human metabolic levels and therefore should be taken into account in studies. As more and more scholars focus on the role of S1P, it is believed that these problems will be solved in the future.
BW: Writing – original draft, Conceptualization, Data curation, Writing – review & editing. XW: Investigation, Software, Writing – review & editing. JC: Data curation, Formal analysis, Writing – review & editing. JY: Methodology, Supervision, Writing – review & editing. HZ: Data curation, Supervision, Writing – review & editing. XL: Methodology, Supervision, Writing – review & editing.
The author(s) declare financial support was received for the research, authorship, and/or publication of this article. This work was supported by the Jiangxi Provincial Health Commission science and Technology project (No. 202410342), The Doctoral Startup Project of the First Affiliated Hospital of Gannan Medical University(No. QD202336),and the Jiangxi Province Gannan Medical University Key Topics Special Fund Project (No. ZD201918).
All figures are created with Figraw. We thank Home for Researchers editorial team (www.home-for-researchers.com) for language editing service.
The authors declare that the research was conducted in the absence of any commercial or financial relationships that could be construed as a potential conflict of interest.
All claims expressed in this article are solely those of the authors and do not necessarily represent those of their affiliated organizations, or those of the publisher, the editors and the reviewers. Any product that may be evaluated in this article, or claim that may be made by its manufacturer, is not guaranteed or endorsed by the publisher.
1. Gotts JE, Matthay MA. Sepsis: pathophysiology and clinical management. BMJ. (2016) 353:i1585. doi: 10.1136/bmj.i1585
2. Raith EP, Udy AA, Bailey M, McGloughlin S, MacIsaac C, Bellomo R, et al. Prognostic accuracy of the SOFA score, SIRS criteria, and qSOFA score for in-hospital mortality among adults with suspected infection admitted to the intensive care unit. JAMA. (2017) 317:290–300. doi: 10.1001/jama.2016.20328
3. Fleischmann C, Scherag A, Adhikari NKJ, Hartog CS, Tsaganos T, Schlattmann P, et al. Assessment of global incidence and mortality of hospital-treated sepsis. Current estimates and limitations. Am J Respir Crit Care Med. (2016) 193:259–72. doi: 10.1164/rccm.201504-0781OC
4. Saini K, Bolia R, Bhat NK. Incidence, predictors and outcome of sepsis-associated liver injury in children: a prospective observational study. Eur J Pediatr. (2022) 181:1699–707. doi: 10.1007/s00431-022-04374-2
5. Yan J, Li S, Li S. The role of the liver in sepsis. Int Rev Immunol. (2014) 33:498–510. doi: 10.3109/08830185.2014.889129
6. Nesseler N, Launey Y, Aninat C, White J, Corlu A, Pieper K, et al. Liver dysfunction is associated with long-term mortality in septic shock. Am J Respir Crit Care Med. (2016) 193:335–7. doi: 10.1164/rccm.201508-1660LE
7. Woźnica EA, Inglot M, Woźnica RK, Łysenko L. Liver dysfunction in sepsis. Adv Clin Exp Med. (2018) 27:547–51. doi: 10.17219/acem/68363
8. Jenniskens M, Langouche L, Vanwijngaerden Y-M, Mesotten D, Van den Berghe G. Cholestatic liver (dys)function during sepsis and other critical illnesses. Intensive Care Med. (2016) 42:16–27. doi: 10.1007/s00134-015-4054-0
9. Zhang X, Liu H, Hashimoto K, Yuan S, Zhang J. The gut-liver axis in sepsis: interaction mechanisms and therapeutic potential. Crit Care. (2022) 26:213. doi: 10.1186/s13054-022-04090-1
10. Obinata H, Hla T. Sphingosine 1-phosphate and inflammation. Int Immunol. (2019) 31:617–25. doi: 10.1093/intimm/dxz037
11. Gault CR, Obeid LM, Hannun YA. An overview of sphingolipid metabolism: from synthesis to breakdown. Adv Exp Med Biol. (2010) 688:1–23. doi: 10.1007/978-1-4419-6741-1_1
12. Weigel C, Bellaci J, Spiegel S. Sphingosine-1-phosphate and its receptors in vascular endothelial and lymphatic barrier function. J Biol Chem. (2023) 299:104775. doi: 10.1016/j.jbc.2023.104775
13. Jozefczuk E, Guzik TJ, Siedlinski M. Significance of sphingosine-1-phosphate in cardiovascular physiology and pathology. Pharmacol Res. (2020) 156:104793. doi: 10.1016/j.phrs.2020.104793
14. Baeyens AAL, Schwab SR. Finding a way out: S1P signaling and immune cell migration. Annu Rev Immunol. (2020) 38:759–84. doi: 10.1146/annurev-immunol-081519-083952
15. Hisano Y, Nishi T, Kawahara A. The functional roles of S1P in immunity. J Biochem. (2012) 152:305–11. doi: 10.1093/jb/mvs090
16. Winkler MS, Nierhaus A, Holzmann M, Mudersbach E, Bauer A, Robbe L, et al. Decreased serum concentrations of sphingosine-1-phosphate in sepsis. Crit Care. (2015) 19:372. doi: 10.1186/s13054-015-1089-0
17. Chen H, Wang J, Zhang C, Ding P, Tian S, Chen J, et al. Sphingosine 1-phosphate receptor, a new therapeutic direction in different diseases. BioMed Pharmacother. (2022) 153:113341. doi: 10.1016/j.biopha.2022.113341
18. Bravo GÁ, Cedeño RR, Casadevall MP, Ramió-Torrentà L. Sphingosine-1-phosphate (S1P) and S1P signaling pathway modulators, from current insights to future perspectives. Cells. (2022) 11(13):2058. doi: 10.3390/cells11132058
19. Gräler MH. The role of sphingosine 1-phosphate in immunity and sepsis. Am J Clin Exp Immunol. (2012) 1(2):90–100.
20. Kurano M, Tsuneyama K, Morimoto Y, Shimizu T, Jona M, Kassai H, et al. Apolipoprotein M protects lipopolysaccharide-treated mice from death and organ injury. Thromb Haemost. (2018) 118:1021–35. doi: 10.1055/s-0038-1641750
21. Winkler MS, Nierhaus A, Poppe A, Greiwe G, Gräler MH, Daum G. Sphingosine-1-phosphate: A potential biomarker and therapeutic target for endothelial dysfunction and sepsis? Shock. (2017) 47:666–72. doi: 10.1097/SHK.0000000000000814
22. Piotti A, Novelli D, Meessen JMTA, Ferlicca D, Coppolecchia S, Marino A, et al. Endothelial damage in septic shock patients as evidenced by circulating syndecan-1, sphingosine-1-phosphate and soluble VE-cadherin: a substudy of ALBIOS. Crit Care. (2021) 25:113. doi: 10.1186/s13054-021-03545-1
23. Frej C, Linder A, Happonen KE, Taylor FB, Lupu F, Dahlbäck B. Sphingosine 1-phosphate and its carrier apolipoprotein M in human sepsis and in Escherichia coli sepsis in baboons. J Cell Mol Med. (2016) 20:1170–81. doi: 10.1111/jcmm.12831
24. Rosenberg AJ, Liu H, Jin H, Yue X, Riley S, Brown SJ, et al. Design, synthesis, and in vitro and in vivo evaluation of an (18)F-labeled sphingosine 1-phosphate receptor 1 (S1P1) PET tracer. J Med Chem. (2016) 59:6201–20. doi: 10.1021/acs.jmedchem.6b00390
25. Zeng M, Feng A, Wang L, Li K, Zhou J. Aralia saponin A isolated from Achyranthes bidentata Bl. ameliorates LPS/D-GalN induced acute liver injury via SPHK1/S1P/S1PR1 pathway in vivo and in vitro. Int Immunopharmacol. (2023) 124:110912. doi: 10.1016/j.intimp.2023.110912
26. Hemdan NYA, Weigel C, Reimann C-M, Gräler MH. Modulating sphingosine 1-phosphate signaling with DOP or FTY720 alleviates vascular and immune defects in mouse sepsis. Eur J Immunol. (2016) 46:2767–77. doi: 10.1002/eji.201646417
27. Rohrbach T, Maceyka M, Spiegel S. Sphingosine kinase and sphingosine-1-phosphate in liver pathobiology. Crit Rev Biochem Mol Biol. (2017) 52:543–53. doi: 10.1080/10409238.2017.1337706
28. Aoki M, Aoki H, Ramanathan R, Hait NC, Takabe K. Sphingosine-1-phosphate signaling in immune cells and inflammation: roles and therapeutic potential. Mediators Inflamm. (2016) 2016:8606878. doi: 10.1155/2016/8606878
29. Xiong Y, Hla T. S1P control of endothelial integrity. Curr Top Microbiol Immunol. (2014) 378:85–105. doi: 10.1007/978-3-319-05879-5_4
30. Tkach M, Théry C. Communication by extracellular vesicles: where we are and where we need to go. Cell. (2016) 164:1226–32. doi: 10.1016/j.cell.2016.01.043
31. Yáñez-Mó M, Siljander PRM, Andreu Z, Zavec AB, Borràs FE, Buzas EI, et al. Biological properties of extracellular vesicles and their physiological functions. J Extracell Vesicles. (2015) 4:27066. doi: 10.3402/jev.v4.27066
32. Liao C-Y, Song MJ, Gao Y, Mauer AS, Revzin A, Malhi H. Hepatocyte-derived lipotoxic extracellular vesicle sphingosine 1-phosphate induces macrophage chemotaxis. Front Immunol. (2018) 9:2980. doi: 10.3389/fimmu.2018.02980
33. Nojima H, Freeman CM, Schuster RM, Japtok L, Kleuser B, Edwards MJ, et al. Hepatocyte exosomes mediate liver repair and regeneration via sphingosine-1-phosphate. J Hepatol. (2016) 64:60–8. doi: 10.1016/j.jhep.2015.07.030
34. Christoffersen C, Obinata H, Kumaraswamy SB, Galvani S, Ahnström J, Sevvana M, et al. Endothelium-protective sphingosine-1-phosphate provided by HDL-associated apolipoprotein M. Proc Natl Acad Sci U S A. (2011) 108:9613–8. doi: 10.1073/pnas.1103187108
35. Hajny S, Christoffersen C. A novel perspective on the apoM-S1P axis, highlighting the metabolism of apoM and its role in liver fibrosis and neuroinflammation. Int J Mol Sci. (2017) 18(8):1636. doi: 10.3390/ijms18081636
36. Liu M, Allegood J, Zhu X, Seo J, Gebre AK, Boudyguina E, et al. Uncleaved ApoM signal peptide is required for formation of large ApoM/sphingosine 1-phosphate (S1P)-enriched HDL particles. J Biol Chem. (2015) 290:7861–70. doi: 10.1074/jbc.M114.631101
37. Velagapudi S, Rohrer L, Poti F, Feuerborn R, Perisa D, Wang D, et al. Apolipoprotein M and sphingosine-1-phosphate receptor 1 promote the transendothelial transport of high-density lipoprotein. Arterioscler Thromb Vasc Biol. (2021) 41:e468–e79. doi: 10.1161/ATVBAHA.121.316725
38. Wang C, Mao J, Redfield S, Mo Y, Lage JM, Zhou X. Systemic distribution, subcellular localization and differential expression of sphingosine-1-phosphate receptors in benign and Malignant human tissues. Exp Mol Pathol. (2014) 97:259–65. doi: 10.1016/j.yexmp.2014.07.013
39. Karimian G, Buist-Homan M, Schmidt M, Tietge UJF, de Boer JF, Klappe K, et al. Sphingosine kinase-1 inhibition protects primary rat hepatocytes against bile salt-induced apoptosis. Biochim Biophys Acta. (2013) 1832:1922–9. doi: 10.1016/j.bbadis.2013.06.011
40. Wang Y, Aoki H, Yang J, Peng K, Liu R, Li X, et al. The role of sphingosine 1-phosphate receptor 2 in bile-acid-induced cholangiocyte proliferation and cholestasis-induced liver injury in mice. Hepatology. (2017) 65:2005–18. doi: 10.1002/hep.29076
41. Askanase AD, D'Cruz D, Kalunian K, Merrill JT, Navarra SV, Cahuzac C, et al. Cenerimod, a sphingosine-1-phosphate receptor modulator, versus placebo in patients with moderate-to-severe systemic lupus erythematosus (CARE): an international, double-blind, randomised, placebo-controlled, phase 2 trial. Lancet Rheumatol. (2025) 7:e21–32. doi: 10.1016/S2665-9913(24)00246-7
42. Juhász L, Lőrincz H, Szentpéteri A, Nádró B, Varga É, Paragh G, et al. Sphingosine 1-phosphate and apolipoprotein M levels and their correlations with inflammatory biomarkers in patients with untreated familial hypercholesterolemia. Int J Mol Sci. (2022) 23(22):14065. doi: 10.3390/ijms232214065
43. Ahmed T, Suzuki T, Terao R, Yamagishi R, Fujino R, Azuma K, et al. Roles of sphingosine kinase and sphingosine-1-phosphate receptor 2 in endotoxin-induced acute retinal inflammation. Ocul Immunol Inflamm. (2024) 32:1633–47. doi: 10.1080/09273948.2023.2273963
44. Lin T, Peng M, Zhu Q, Pan X. S1PR2 participates in intestinal injury in severe acute pancreatitis by regulating macrophage pyroptosis. Front Immunol. (2024) 15:1405622. doi: 10.3389/fimmu.2024.1405622
45. Wang D, Bi X, Zhao L, Xiang S, Xi W, Yang S, et al. Targeting SphK1/S1PR3 axis ameliorates sepsis-induced multiple organ injury via orchestration of macrophage polarization and glycolysis. Biochim Biophys Acta Mol Cell Res. (2025) 1872:119877. doi: 10.1016/j.bbamcr.2024.119877
46. Wang S, Tian Z, Lu Y, Huang Z, Fan Y, Li B, et al. Sphingosine-1-phosphate receptor 4 attenuates neutrophilic airway inflammation in experimental asthma via repressing proinflammatory macrophage activation. Int J Biol Sci. (2023) 19:1597–615. doi: 10.7150/ijbs.80256
47. Barnawi J, Tran HB, Roscioli E, Hodge G, Jersmann H, Haberberger R, et al. Pro-phagocytic effects of thymoquinone on cigarette smoke-exposed macrophages occur by modulation of the sphingosine-1-phosphate signalling system. COPD. (2016) 13:653–61. doi: 10.3109/15412555.2016.1153614
48. Chakrabarty S, Bui Q, Badeanlou L, Hester K, Chun J, Ruf W, et al. S1P/S1PR3 signalling axis protects against obesity-induced metabolic dysfunction. Adipocyte. (2022) 11:69–83. doi: 10.1080/21623945.2021.2021700
49. Sarkar J, Aoki H, Wu R, Aoki M, Hylemon P, Zhou H, et al. Conjugated bile acids accelerate progression of pancreatic cancer metastasis via S1PR2 signaling in cholestasis. Ann Surg Oncol. (2023) 30:1630–41. doi: 10.1245/s10434-022-12806-4
50. Miao R-R, Zhan S, Cui S-X, Qu X-J. Intestinal aberrant sphingolipid metabolism shaped-gut microbiome and bile acids metabolome in the development of hepatic steatosis. FASEB J. (2022) 36:e22398. doi: 10.1096/fj.202200148RR
51. Osawa Y, Seki E, Kodama Y, Suetsugu A, Miura K, Adachi M, et al. Acid sphingomyelinase regulates glucose and lipid metabolism in hepatocytes through AKT activation and AMP-activated protein kinase suppression. FASEB J. (2011) 25:1133–44. doi: 10.1096/fj.10-168351
52. Kurano M, Tsukamoto K, Shimizu T, Kassai H, Nakao K, Aiba A, et al. Protection against insulin resistance by apolipoprotein M/sphingosine-1-phosphate. Diabetes. (2020) 69:867–81. doi: 10.2337/db19-0811
53. Ravichandran S, Finlin BS, Kern PA, Özcan S. Sphk2-/- mice are protected from obesity and insulin resistance. Biochim Biophys Acta Mol Basis Dis. (2019) 1865:570–6. doi: 10.1016/j.bbadis.2018.12.012
54. Nagahashi M, Yuza K, Hirose Y, Nakajima M, Ramanathan R, Hait NC, et al. The roles of bile acids and sphingosine-1-phosphate signaling in the hepatobiliary diseases. J Lipid Res. (2016) 57:1636–43. doi: 10.1194/jlr.R069286
55. Kubes P, Jenne C. Immune responses in the liver. Annu Rev Immunol. (2018) 36:247–77. doi: 10.1146/annurev-immunol-051116-052415
56. Bogdanos DP, Gao B, Gershwin ME. Liver immunology. Compr Physiol. (2013) 3:567–98. doi: 10.1002/cphy.c120011
57. Parlar YE, Ayar SN, Cagdas D, Balaban YH. Liver immunity, autoimmunity, and inborn errors of immunity. World J Hepatol. (2023) 15:52–67. doi: 10.4254/wjh.v15.i1.52
58. Aizarani N, Saviano A, Sagar, Mailly L, Durand S, Herman JS, et al. A human liver cell atlas reveals heterogeneity and epithelial progenitors. Nature. (2019) 572:199–204. doi: 10.1038/s41586-019-1373-2
59. Yao X, Liu Y, Mao M, Yang L, Zhan Q, Xiao J. Calorie restriction mimetic, resveratrol, attenuates hepatic ischemia and reperfusion injury through enhancing efferocytosis of macrophages via AMPK/STAT3/S1PR1 pathway. J Nutr Biochem. (2024) 126:109587. doi: 10.1016/j.jnutbio.2024.109587
60. Paul B, Lewinska M, Andersen JB. Lipid alterations in chronic liver disease and liver cancer. JHEP Rep. (2022) 4:100479. doi: 10.1016/j.jhepr.2022.100479
61. Li L, Wang H, Zhang J, Sha Y, Wu F, Wen S, et al. SPHK1 deficiency protects mice from acetaminophen-induced ER stress and mitochondrial permeability transition. Cell Death Differ. (2020) 27:1924–37. doi: 10.1038/s41418-019-0471-x
62. Jin L, Liu W-R, Tian M-X, Fan J, Shi Y-H. The SphKs/S1P/S1PR1 axis in immunity and cancer: more ore to be mined. World J Surg Oncol. (2016) 14:131. doi: 10.1186/s12957-016-0884-7
63. Chu S, Sun R, Gu X, Chen L, Liu M, Guo H, et al. Inhibition of sphingosine-1-phosphate-induced th17 cells ameliorates alcohol-associated steatohepatitis in mice. Hepatology. (2021) 73:952–67. doi: 10.1002/hep.31321
64. Sano N, Tamura T, Toriyabe N, Nowatari T, Nakayama K, Tanoi T, et al. New drug delivery system for liver sinusoidal endothelial cells for ischemia-reperfusion injury. World J Gastroenterol. (2015) 21:12778–86. doi: 10.3748/wjg.v21.i45.12778
65. Yang L, Yue S, Yang L, Liu X, Han Z, Zhang Y, et al. Sphingosine kinase/sphingosine 1-phosphate (S1P)/S1P receptor axis is involved in liver fibrosis-associated angiogenesis. J Hepatol. (2013) 59:114–23. doi: 10.1016/j.jhep.2013.02.021
66. King A, Houlihan DD, Kavanagh D, Haldar D, Luu N, Owen A, et al. Sphingosine-1-phosphate prevents egress of hematopoietic stem cells from liver to reduce fibrosis. Gastroenterology. (2017) 153:233–248.e16. doi: 10.1053/j.gastro.2017.03.022
67. Li C, Zheng S, You H, Liu X, Lin M, Yang L, et al. Sphingosine 1-phosphate (S1P)/S1P receptors are involved in human liver fibrosis by action on hepatic myofibroblasts motility. J Hepatol. (2011) 54:1205–13. doi: 10.1016/j.jhep.2010.08.028
68. Zhao X, Yang L, Chang N, Hou L, Zhou X, Yang L, et al. Neutrophils undergo switch of apoptosis to NETosis during murine fatty liver injury via S1P receptor 2 signaling. Cell Death Dis. (2020) 11:379. doi: 10.1038/s41419-020-2582-1
69. Zhao X, Yang L, Chang N, Hou L, Zhou X, Dong C, et al. Neutrophil recruitment mediated by sphingosine 1-phosphate (S1P)/S1P receptors during chronic liver injury. Cell Immunol. (2021) 359:104243. doi: 10.1016/j.cellimm.2020.104243
70. Sayaf K, Battistella S, Russo FP. NLRP3 inflammasome in acute and chronic liver diseases. Int J Mol Sci. (2024) 25(8):4537. doi: 10.3390/ijms25084537
71. Hou L, Zhang Z, Yang L, Chang N, Zhao X, Zhou X, et al. NLRP3 inflammasome priming and activation in cholestatic liver injury via the sphingosine 1-phosphate/S1P receptor 2/Gα(12/13)/MAPK signaling pathway. J Mol Med (Berl). (2021) 99:273–88. doi: 10.1007/s00109-020-02032-4
72. Mauer AS, Hirsova P, Maiers JL, Shah VH, Malhi H. Inhibition of sphingosine 1-phosphate signaling ameliorates murine nonalcoholic steatohepatitis. Am J Physiol Gastrointest Liver Physiol. (2017) 312:G300–G13. doi: 10.1152/ajpgi.00222.2016
73. Rida R, Kreydiyyeh S. Effect of FTY720P on lipid accumulation in HEPG2 cells. Sci Rep. (2023) 13:19716. doi: 10.1038/s41598-023-46011-4
74. Geng T, Sutter A, Harland MD, Law BA, Ross JS, Lewin D, et al. SphK1 mediates hepatic inflammation in a mouse model of NASH induced by high saturated fat feeding and initiates proinflammatory signaling in hepatocytes. J Lipid Res. (2015) 56:2359–71. doi: 10.1194/jlr.M063511
75. Rachakonda VP, Reeves VL, Aljammal J, Wills RC, Trybula JS, DeLany JP, et al. Serum autotaxin is independently associated with hepatic steatosis in women with severe obesity. Obes (Silver Spring). (2015) 23:965–72. doi: 10.1002/oby.20960
76. Bao M, Chen Z, Xu Y, Zhao Y, Zha R, Huang S, et al. Sphingosine kinase 1 promotes tumour cell migration and invasion via the S1P/EDG1 axis in hepatocellular carcinoma. Liver Int. (2012) 32:331–8. doi: 10.1111/j.1478-3231.2011.02666.x
77. Ho JWY, Man K, Sun CK, Lee TK, Poon RTP, Fan ST. Effects of a novel immunomodulating agent, FTY720, on tumor growth and angiogenesis in hepatocellular carcinoma. Mol Cancer Ther. (2005) 4:1430–8. doi: 10.1158/1535-7163.MCT-05-0021
78. Wu J-Y, Wang Z-X, Zhang G, Lu X, Qiang G-H, Hu W, et al. Targeted co-delivery of Beclin 1 siRNA and FTY720 to hepatocellular carcinoma by calcium phosphate nanoparticles for enhanced anticancer efficacy. Int J Nanomedicine. (2018) 13:1265–80. doi: 10.2147/IJN.S156328
79. Li CX, Shao Y, Ng KTP, Liu XB, Ling CC, Ma YY, et al. FTY720 suppresses liver tumor metastasis by reducing the population of circulating endothelial progenitor cells. PloS One. (2012) 7:e32380. doi: 10.1371/journal.pone.0032380
80. Hung J-H, Lu Y-S, Wang Y-C, Ma Y-H, Wang D-S, Kulp SK, et al. FTY720 induces apoptosis in hepatocellular carcinoma cells through activation of protein kinase C delta signaling. Cancer Res. (2008) 68:1204–12. doi: 10.1158/0008-5472.CAN-07-2621
81. Ushitora Y, Tashiro H, Ogawa T, Tanimoto Y, Kuroda S, Kobayashi T, et al. Suppression of hepatocellular carcinoma recurrence after rat liver transplantation by FTY720, a sphingosine-1-phosphate analog. Transplantation. (2009) 88:980–6. doi: 10.1097/TP.0b013e3181b9ca69
82. Kramer L, Jordan B, Druml W, Bauer P, Metnitz PGH. Incidence and prognosis of early hepatic dysfunction in critically ill patients–a prospective multicenter study. Crit Care Med. (2007) 35:1099–104. doi: 10.1097/01.CCM.0000259462.97164.A0
83. Kim TS, Choi DH. Liver dysfunction in sepsis. Korean J Gastroenterol. (2020) 75:182–7. doi: 10.4166/kjg.2020.75.4.182
84. Lu H. Inflammatory liver diseases and susceptibility to sepsis. Clin Sci (Lond). (2024) 138:435–87. doi: 10.1042/CS20230522
85. Recknagel P, Gonnert FA, Westermann M, Lambeck S, Lupp A, Rudiger A, et al. Liver dysfunction and phosphatidylinositol-3-kinase signalling in early sepsis: experimental studies in rodent models of peritonitis. PloS Med. (2012) 9:e1001338. doi: 10.1371/journal.pmed.1001338
86. Xie T, Xin Q, Cao X, Chen R, Ren H, Liu C, et al. Clinical characteristics and construction of a predictive model for patients with sepsis related liver injury. Clin Chim Acta. (2022) 537:80–6. doi: 10.1016/j.cca.2022.10.004
87. Spapen H. Liver perfusion in sepsis, septic shock, and multiorgan failure. Anat Rec (Hoboken). (2008) 291:714–20. doi: 10.1002/ar.20646
88. Nesseler N, Launey Y, Aninat C, Morel F, Mallédant Y, Seguin P. Clinical review: The liver in sepsis. Crit Care. (2012) 16:235. doi: 10.1186/cc11381
89. Sun J, Zhang J, Wang X, Ji F, Ronco C, Tian J, et al. Gut-liver crosstalk in sepsis-induced liver injury. Crit Care. (2020) 24:614. doi: 10.1186/s13054-020-03327-1
90. Svetlov SI, Sautin YY, Crawford JM. EDG receptors and hepatic pathophysiology of LPA and S1P: EDG-ology of liver injury. Biochim Biophys Acta. (2002) 1582:251–6. doi: 10.1016/S1388-1981(02)00178-6
91. Park SW, Kim M, Chen SWC, Brown KM, D'Agati VD, Lee HT. Sphinganine-1-phosphate protects kidney and liver after hepatic ischemia and reperfusion in mice through S1P1 receptor activation. Lab Invest. (2010) 90:1209–24. doi: 10.1038/labinvest.2010.102
92. Robinson MW, Harmon C, O'Farrelly C. Liver immunology and its role in inflammation and homeostasis. Cell Mol Immunol. (2016) 13:267–76. doi: 10.1038/cmi.2016.3
93. Wang D, Yin Y, Yao Y. Advances in sepsis-associated liver dysfunction. Burns Trauma. (2014) 2(3):97–105. doi: 10.4103/2321-3868.132689
94. Siore AM, Parker RE, Stecenko AA, Cuppels C, McKean M, Christman BW, et al. Endotoxin-induced acute lung injury requires interaction with the liver. Am J Physiol Lung Cell Mol Physiol. (2005) 289:L769–L76. doi: 10.1152/ajplung.00137.2005
95. Lei Y-C, Yang L-L, Li W, Luo P, Zheng P-F. Inhibition of sphingosine kinase 1 ameliorates acute liver failure by reducing high-mobility group box 1 cytoplasmic translocation in liver cells. World J Gastroenterol. (2015) 21:13055–63. doi: 10.3748/wjg.v21.i46.13055
96. Lei Y-C, Yang L-L, Li W, Luo P. Sphingosine kinase 1 dependent protein kinase C-δ activation plays an important role in acute liver failure in mice. World J Gastroenterol. (2015) 21:13438–46. doi: 10.3748/wjg.v21.i48.13438
97. Fang C, Ren P, Bian G, Wang J, Bai J, Huang J, et al. Enhancing Spns2/S1P in macrophages alleviates hyperinflammation and prevents immunosuppression in sepsis. EMBO Rep. (2023) 24:e56635. doi: 10.15252/embr.202256635
98. Gong C, Jin Y, Wang X, Mao J, Wang D, Yu X, et al. Lack of S1PR2 in macrophage ameliorates sepsis-associated lung injury through inducing IL-33-mediated type 2 immunity. Am J Respir Cell Mol Biol. (2024) 70:215–25. doi: 10.1165/rcmb.2023-0075OC
99. Hou J, Chen Q, Zhang K, Cheng B, Xie G, Wu X, et al. Sphingosine 1-phosphate receptor 2 signaling suppresses macrophage phagocytosis and impairs host defense against sepsis. Anesthesiology. (2015) 123:409–22. doi: 10.1097/ALN.0000000000000725
100. Hou L, Yang L, Chang N, Zhao X, Zhou X, Dong C, et al. Macrophage sphingosine 1-phosphate receptor 2 blockade attenuates liver inflammation and fibrogenesis triggered by NLRP3 inflammasome. Front Immunol. (2020) 11:1149. doi: 10.3389/fimmu.2020.01149
101. Wang Y, Wang C, He Q, Chen G, Yu J, Cang J, et al. Inhibition of sphingosine-1-phosphate receptor 3 suppresses ATP-induced NLRP3 inflammasome activation in macrophages via TWIK2-mediated potassium efflux. Front Immunol. (2023) 14:1090202. doi: 10.3389/fimmu.2023.1090202
102. Hou X, Zhou R, Wei H, Sun R, Tian Z. NKG2D-retinoic acid early inducible-1 recognition between natural killer cells and Kupffer cells in a novel murine natural killer cell-dependent fulminant hepatitis. Hepatology. (2009) 49:940–9. doi: 10.1002/hep.22725
103. Zhang X, Lou J, Bai L, Chen Y, Zheng S, Duan Z. Immune regulation of intrahepatic regulatory T cells in fibrotic livers of mice. Med Sci Monit. (2017) 23:1009–16. doi: 10.12659/MSM.899725
104. Ami K, Ohkawa T, Koike Y, Sato K, Habu Y, Iwai T, et al. Activation of human T cells with NK cell markers by staphylococcal enterotoxin A via IL-12 but not via IL-18. Clin Exp Immunol. (2002) 128:453–9. doi: 10.1046/j.1365-2249.2002.01854.x
105. Abdi K, Laky K, Abshari M, Hill EM, Lantz L, Singh NJ, et al. Dendritic cells Trigger IFN-γ secretion by NK cells independent of IL-12 and IL-18. Eur J Immunol. (2022) 52:1431–40. doi: 10.1002/eji.202149733
106. Tang Y, Peitzsch C, Charoudeh HN, Cheng M, Chaves P, Jacobsen SEW, et al. Emergence of NK-cell progenitors and functionally competent NK-cell lineage subsets in the early mouse embryo. Blood. (2012) 120:63–75. doi: 10.1182/blood-2011-02-337980
107. Dorsam G, Graeler MH, Seroogy C, Kong Y, Voice JK, Goetzl EJ. Transduction of multiple effects of sphingosine 1-phosphate (S1P) on T cell functions by the S1P1 G protein-coupled receptor. J Immunol. (2003) 171:3500–7. doi: 10.4049/jimmunol.171.7.3500
108. Jenne CN, Enders A, Rivera R, Watson SR, Bankovich AJ, Pereira JP, et al. T-bet-dependent S1P5 expression in NK cells promotes egress from lymph nodes and bone marrow. J Exp Med. (2009) 206:2469–81. doi: 10.1084/jem.20090525
109. Zhang J, Dunk CE, Lye SJ. Sphingosine signalling regulates decidual NK cell angiogenic phenotype and trophoblast migration. Hum Reprod. (2013) 28:3026–37. doi: 10.1093/humrep/det339
110. Walzer T, Chiossone L, Chaix J, Calver A, Carozzo C, Garrigue-Antar L, et al. Natural killer cell trafficking in vivo requires a dedicated sphingosine 1-phosphate receptor. Nat Immunol. (2007) 8:1337–44. doi: 10.1038/ni1523
111. Qu M, Cui J, Zhu J, Ma Y, Yuan X, Shi J, et al. Bone marrow-derived mesenchymal stem cells suppress NK cell recruitment and activation in PolyI:C-induced liver injury. Biochem Biophys Res Commun. (2015) 466:173–9. doi: 10.1016/j.bbrc.2015.08.125
112. Dong Z, Wei H, Sun R, Hu Z, Gao B, Tian Z. Involvement of natural killer cells in PolyI:C-induced liver injury. J Hepatol. (2004) 41:966–73. doi: 10.1016/j.jhep.2004.08.021
113. Bertolini A, Fiorotto R, Strazzabosco M. Bile acids and their receptors: modulators and therapeutic targets in liver inflammation. Semin Immunopathol. (2022) 44:547–64. doi: 10.1007/s00281-022-00935-7
114. Spiegel S, Maczis MA, Maceyka M, Milstien S. New insights into functions of the sphingosine-1-phosphate transporter SPNS2. J Lipid Res. (2019) 60:484–9. doi: 10.1194/jlr.S091959
115. Linton KJ. Lipid flopping in the liver. Biochem Soc Trans. (2015) 43:1003–10. doi: 10.1042/BST20150132
116. Spirlì C, Fabris L, Duner E, Fiorotto R, Ballardini G, Roskams T, et al. Cytokine-stimulated nitric oxide production inhibits adenylyl cyclase and cAMP-dependent secretion in cholangiocytes. Gastroenterology. (2003) 124:737–53. doi: 10.1053/gast.2003.50100
117. Moseley RH. Sepsis and cholestasis. Clin Liver Dis. (2004) 8:83–94. doi: 10.1016/S1089-3261(03)00134-X
118. Keul P, Peters S, von Wnuck Lipinski K, Schröder NH, Nowak MK, Duse DA, et al. Sphingosine-1-phosphate (S1P) lyase inhibition aggravates atherosclerosis and induces plaque rupture in apoE-/-mice. Int J Mol Sci. (2022) 23(17):9606. doi: 10.3390/ijms23179606
119. Vaidya M, Jentsch JA, Peters S, Keul P, Weske S, Gräler MH, et al. Regulation of ABCA1-mediated cholesterol efflux by sphingosine-1-phosphate signaling in macrophages. J Lipid Res. (2019) 60:506–15. doi: 10.1194/jlr.M088443
120. Hao H, Cao L, Jiang C, Che Y, Zhang S, Takahashi S, et al. Farnesoid X receptor regulation of the NLRP3 inflammasome underlies cholestasis-associated sepsis. Cell Metab. (2017) 25(4):856–67.e5. doi: 10.1016/j.cmet.2017.03.007
121. Spirlì C, Nathanson MH, Fiorotto R, Duner E, Denson LA, Sanz JM, et al. Proinflammatory cytokines inhibit secretion in rat bile duct epithelium. Gastroenterology. (2001) 121:156–69. doi: 10.1053/gast.2001.25516
122. Cao H, Chen L, Zeng Z, Wu X, Lei Y, Jia W, et al. Reversal of cholestatic liver disease by the inhibition of sphingosine 1-phosphate receptor 2 signaling. PeerJ. (2024) 12:e16744. doi: 10.7717/peerj.16744
123. Schnabl B, Brenner DA. Interactions between the intestinal microbiome and liver diseases. Gastroenterology. (2014) 146:1513–24. doi: 10.1053/j.gastro.2014.01.020
124. Strnad P, Tacke F, Koch A, Trautwein C. Liver - guardian, modifier and target of sepsis. Nat Rev Gastroenterol Hepatol. (2017) 14:55–66. doi: 10.1038/nrgastro.2016.168
125. Fay KT, Ford ML, Coopersmith CM. The intestinal microenvironment in sepsis. Biochim Biophys Acta Mol Basis Dis. (2017) 1863:2574–83. doi: 10.1016/j.bbadis.2017.03.005
126. Assimakopoulos SF, Triantos C, Thomopoulos K, Fligou F, Maroulis I, Marangos M, et al. Gut-origin sepsis in the critically ill patient: pathophysiology and treatment. Infection. (2018) 46:751–60. doi: 10.1007/s15010-018-1178-5
127. Kaminsky LW, Al-Sadi R, Ma TY. IL-1β and the intestinal epithelial tight junction barrier. Front Immunol. (2021) 12:767456. doi: 10.3389/fimmu.2021.767456
128. Haussner F, Chakraborty S, Halbgebauer R, Huber-Lang M. Challenge to the intestinal mucosa during sepsis. Front Immunol. (2019) 10:891. doi: 10.3389/fimmu.2019.00891
129. Stephens M, von der Weid P-Y. Lipopolysaccharides modulate intestinal epithelial permeability and inflammation in a species-specific manner. Gut Microbes. (2020) 11:421–32. doi: 10.1080/19490976.2019.1629235
130. Wang Z, Qi Y, Wang F, Zhang B, Jianguo T. Circulating sepsis-related metabolite sphinganine could protect against intestinal damage during sepsis. Front Immunol. (2023) 14:1151728. doi: 10.3389/fimmu.2023.1151728
131. Zou F, Wang S, Xu M, Wu Z, Deng F. The role of sphingosine-1-phosphate in the gut mucosal microenvironment and inflammatory bowel diseases. Front Physiol. (2023) 14:1235656. doi: 10.3389/fphys.2023.1235656
132. Ziegler AC, Gräler MH. Barrier maintenance by S1P during inflammation and sepsis. Tissue Barriers. (2021) 9:1940069. doi: 10.1080/21688370.2021.1940069
133. Fan Y, Chen J, Liu D, Li W, Wang H, Huang Y, et al. HDL-S1P protects endothelial function and reduces lung injury during sepsis in vivo and in vitro. Int J Biochem Cell Biol. (2020) 126:105819. doi: 10.1016/j.biocel.2020.105819
134. Qiu Y, Shen J, Jiang W, Yang Y, Liu X, Zeng Y. Sphingosine 1-phosphate and its regulatory role in vascular endothelial cells. Histol Histopathol. (2022) 37:213–25. doi: 10.14670/HH-18-428
135. Jacobson JR, Garcia JGN. Novel therapies for microvascular permeability in sepsis. Curr Drug Targets. (2007) 8:509–14. doi: 10.2174/138945007780362719
136. Chen Y, Zhao Z, Guo S, Li Y, Yin H, Tian L, et al. Red rice seed coat targeting SPHK2 ameliorated alcoholic liver disease via restored intestinal barrier and improved gut microbiota in mice. Nutrients. (2023) 15(19):4176. doi: 10.3390/nu15194176
137. Sammani S, Moreno-Vinasco L, Mirzapoiazova T, Singleton PA, Chiang ET, Evenoski CL, et al. Differential effects of sphingosine 1-phosphate receptors on airway and vascular barrier function in the murine lung. Am J Respir Cell Mol Biol. (2010) 43:394–402. doi: 10.1165/rcmb.2009-0223OC
138. Bai J, Hu J-p. The drug development based on sphingosine-1-phosphate signaling pathway. Yao Xue Xue Bao. (2016) 51:1822–8.
139. Chaudhry BZ, Cohen JA, Conway DS. Sphingosine 1-phosphate receptor modulators for the treatment of multiple sclerosis. Neurotherapeutics. (2017) 14:859–73. doi: 10.1007/s13311-017-0565-4
140. Kovarik JM, Schmouder RL, Serra D, Wang Y, Wiegand H, Dilzer SC, et al. FTY720 pharmacokinetics in mild to moderate hepatic impairment. J Clin Pharmacol. (2005) 45:446–52. doi: 10.1177/0091270004274288
141. Ladrón Abia P, Alcalá Vicente C, Martínez Delgado S, Bastida Paz G. Fingolimod-induced remission in a patient with ulcerative colitis and multiple sclerosis. Gastroenterol Hepatol. (2021) 44:156–7. doi: 10.1016/j.gastrohep.2020.07.020
142. Verstockt B, Vetrano S, Salas A, Nayeri S, Duijvestein M, Vande Casteele N. Sphingosine 1-phosphate modulation and immune cell trafficking in inflammatory bowel disease. Nat Rev Gastroenterol Hepatol. (2022) 19:351–66. doi: 10.1038/s41575-021-00574-7
143. Ye H, Sun M, Jin Z, Yuan Y, Weng H. FTY-720 alleviates diabetes-induced liver injury by inhibiting oxidative stress and inflammation. Fundam Clin Pharmacol. (2023) 37:960–70. doi: 10.1111/fcp.12897
144. Baweja S, Kumari A, Negi P, Tomar A, Tripathi DM, Mourya AK, et al. Hepatopulmonary syndrome is associated with low sphingosine-1-phosphate levels and can be ameliorated by the functional agonist fingolimod. J Hepatol. (2023) 79:167–80. doi: 10.1016/j.jhep.2023.03.018
145. He X, Kang K, Pan D, Sun Y, Chang B. FTY720 attenuates APAP−induced liver injury via the JAK2/STAT3 signaling pathway. Int J Mol Med. (2022) 49(5):67. doi: 10.3892/ijmm.2022.5123
146. Aguiar C, Batista S, Pacheco R. Cardiovascular effects of fingolimod: Relevance, detection and approach. Rev Port Cardiol. (2015) 34:279–85. doi: 10.1016/j.repc.2014.11.012
147. Alhubaishi AA, Alsharekh LM, Almoharb HF, Alqarni FA, Alqahtani AM, Alghazwni MK, et al. Assessment of clinician adherence to Fingolimod instructions and its effect on patient safety. Neurosci (Riyadh). (2024) 29:184–9. doi: 10.17712/nsj.2024.3.20240040
148. Ghiasian M, Nafisi H, Ranjbar A, Mohammadi Y, Ataei S. Antioxidative effects of silymarin on the reduction of liver complications of fingolimod in patients with relapsing-remitting multiple sclerosis: A clinical trial study. J Biochem Mol Toxicol. (2021) 35:e22800. doi: 10.1002/jbt.22800
149. Coyle PK, Freedman MS, Cohen BA, Cree BAC, Markowitz CE. Sphingosine 1-phosphate receptor modulators in multiple sclerosis treatment: A practical review. Ann Clin Transl Neurol. (2024) 11:842–55. doi: 10.1002/acn3.52017
150. Wunsch F, Nguyen TN, Wolber G, Bermudez M. Structural determinants of sphingosine-1-phosphate receptor selectivity. Arch Pharm (Weinheim). (2023) 356:e2300387. doi: 10.1002/ardp.202300387
151. Paik J. Ozanimod: A review in ulcerative colitis. Drugs. (2022) 82:1303–13. doi: 10.1007/s40265-022-01762-8
152. Sandborn WJ, Feagan BG, D'Haens G, Wolf DC, Jovanovic I, Hanauer SB, et al. Ozanimod as induction and maintenance therapy for ulcerative colitis. N Engl J Med. (2021) 385:1280–91. doi: 10.1056/NEJMoa2033617
153. Martín-Hernández D, Muñoz-López M, Tendilla-Beltrán H, Caso JR, García-Bueno B, Menchén L, et al. Immune system and brain/intestinal barrier functions in psychiatric diseases: is sphingosine-1-phosphate at the helm? Int J Mol Sci. (2023) 24(16):12634. doi: 10.3390/ijms241612634
154. Jang I-S, Lee SJ, Bahn Y-S, Baek S-H, Yu BJ. Engineering of Saccharomyces cerevisiae as a platform strain for microbial production of sphingosine-1-phosphate. Microb Cell Fact. (2024) 23:310. doi: 10.1186/s12934-024-02579-8
155. Felippe TVD, Toro DM, de Carvalho JCS, Nobre-Azevedo P, Rodrigues LFM, Oliveira BTM, et al. High-resolution targeted mass spectrometry for comprehensive quantification of sphingolipids: clinical applications and characterization of extracellular vesicles. Anal Biochem. (2024) 698:115732. doi: 10.1016/j.ab.2024.115732
156. Zhang M, Hei R, Zhou Z, Xiao W, Liu X, Chen Y. Macrophage polarization involved the inflammation of chronic obstructive pulmonary disease by S1P/HDAC1 signaling. Am J Cancer Res. (2023) 13:4478–89.
157. Lan YY, De Creus A, Colvin BL, Abe M, Brinkmann V, Coates PTH, et al. The sphingosine-1-phosphate receptor agonist FTY720 modulates dendritic cell trafficking in vivo. Am J Transplant. (2005) 5:2649–59. doi: 10.1111/j.1600-6143.2005.01085.x
158. Maeda Y, Matsuyuki H, Shimano K, Kataoka H, Sugahara K, Chiba K. Migration of CD4 T cells and dendritic cells toward sphingosine 1-phosphate (S1P) is mediated by different receptor subtypes: S1P regulates the functions of murine mature dendritic cells via S1P receptor type 3. J Immunol. (2007) 178:3437–46. doi: 10.4049/jimmunol.178.6.3437
159. Dillmann C, Mora J, Olesch C, Brüne B, Weigert A. S1PR4 is required for plasmacytoid dendritic cell differentiation. Biol Chem. (2015) 396:775–82. doi: 10.1515/hsz-2014-0271
160. Wang F, Zhao S, Gu Z, Zhao X, Yang N, Guan L, et al. S1PR5 regulates NK cell responses in preventing graft-versus-host disease while preserving graft-versus-tumour activity in a murine allogeneic haematopoietic stem cell transplantation model. Hematol Oncol. (2020) 38(1):89–102. doi: 10.1002/hon.2669
161. Matloubian M, Lo CG, Cinamon G, Lesneski MJ, Xu Y, Brinkmann V, et al. Lymphocyte egress from thymus and peripheral lymphoid organs is dependent on S1P receptor 1. Nature. (2004) 427:355–60. doi: 10.1038/nature02284
162. Matsuyuki H, Maeda Y, Yano K, Sugahara K, Chiba K, Kohno T, et al. Involvement of sphingosine 1-phosphate (S1P) receptor type 1 and type 4 in migratory response of mouse T cells toward S1P. Cell Mol Immunol. (2006) 3:429–37.
163. Dixit D, Hallisey VM, Zhu EY, Okuniewska M, Cadwell K, Chipuk JE, et al. S1PR1 inhibition induces proapoptotic signaling in T cells and limits humoral responses within lymph nodes. J Clin Invest. (2024) 134:e174984. doi: 10.1172/JCI174984
164. Wang W, Graeler MH, Goetzl EJ. Type 4 sphingosine 1-phosphate G protein-coupled receptor (S1P4) transduces S1P effects on T cell proliferation and cytokine secretion without signaling migration. FASEB J. (2005) 19:1731–3. doi: 10.1096/fj.05-3730fje
165. Schlicher L, Kulig P, von Münchow A, Murphy MJ, Keller MP. In vitro characterization of sphingosine 1-phosphate receptor 1 (S1P1) expression and mediated migration of primary human T and B cells in the context of cenerimod, a novel, selective S1P1 receptor modulator. Int J Mol Sci. (2022) 23(3):1191. doi: 10.3390/ijms23031191
166. Blumenfeld S, Staun-Ram E, Miller A. Fingolimod therapy modulates circulating B cell composition, increases B regulatory subsets and production of IL-10 and TGFβ in patients with Multiple Sclerosis. J Autoimmun. (2016) 70:40–51. doi: 10.1016/j.jaut.2016.03.012
167. Green JA, Suzuki K, Cho B, Willison LD, Palmer D, Allen CDC, et al. The sphingosine 1-phosphate receptor S1P2 maintains the homeostasis of germinal center B cells and promotes niche confinement. Nat Immunol. (2011) 12:672–80. doi: 10.1038/ni.2047
Keywords: S1P, S1PRs, sepsis, immune, bile acid metabolism, liver-intestinal circulation
Citation: Wang B, Wu X, Cheng J, Ye J, Zhu H and Liu X (2025) Regulatory role of S1P and its receptors in sepsis-induced liver injury. Front. Immunol. 16:1489015. doi: 10.3389/fimmu.2025.1489015
Received: 31 August 2024; Accepted: 13 January 2025;
Published: 28 January 2025.
Edited by:
Antonio La Cava, University of California, Los Angeles, United StatesReviewed by:
Andy Ruiz, National Institute of Respiratory Diseases-Mexico (INER), MexicoCopyright © 2025 Wang, Wu, Cheng, Ye, Zhu and Liu. This is an open-access article distributed under the terms of the Creative Commons Attribution License (CC BY). The use, distribution or reproduction in other forums is permitted, provided the original author(s) and the copyright owner(s) are credited and that the original publication in this journal is cited, in accordance with accepted academic practice. No use, distribution or reproduction is permitted which does not comply with these terms.
*Correspondence: Xiaofeng Liu, bGl1eGlhb2ZlbmczOTEzQDE2My5jb20=
Disclaimer: All claims expressed in this article are solely those of the authors and do not necessarily represent those of their affiliated organizations, or those of the publisher, the editors and the reviewers. Any product that may be evaluated in this article or claim that may be made by its manufacturer is not guaranteed or endorsed by the publisher.
Research integrity at Frontiers
Learn more about the work of our research integrity team to safeguard the quality of each article we publish.