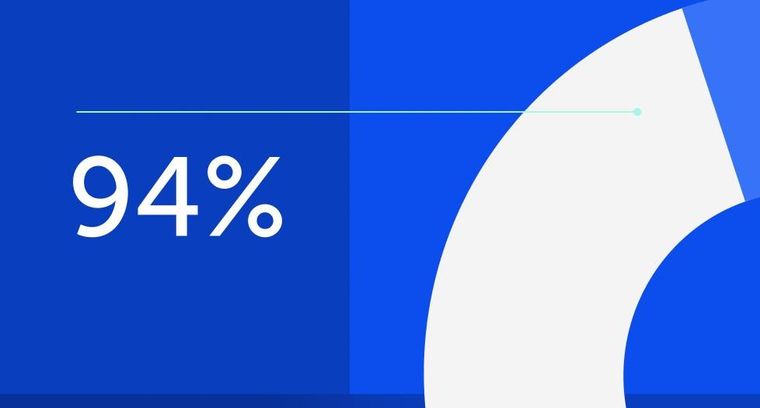
94% of researchers rate our articles as excellent or good
Learn more about the work of our research integrity team to safeguard the quality of each article we publish.
Find out more
REVIEW article
Front. Immunol., 14 April 2025
Sec. Parasite Immunology
Volume 16 - 2025 | https://doi.org/10.3389/fimmu.2025.1484686
Colorectal cancer is one of the most lethal tumors, posing a financial and healthcare burden. This study investigates how helminths and pre-existing diseases such as colitis, obesity, diabetes, and gut microbiota issues influence colon cancer development and prognosis. The immune system’s protective immunosuppressive response to helminth invasion minimizes inflammation-induced cell damage and DNA mutations, lowering the risk of colorectal cancer precursor lesions. Helminth infection-mediated immunosuppression can hasten colorectal cancer growth and metastasis, which is detrimental to patient outcomes. Some helminth derivatives can activate immune cells to attack cancer cells, making them potentially useful as colorectal cancer vaccines or therapies. This review also covers gene editing approaches. We discovered that using CRISPR/Cas9 to inhibit live helminths modulates miRNA, which limits tumor growth. We propose more multicenter studies into helminth therapy’s long-term effects and immune regulation pathways. We hope to treat colorectal cancer patients with helminth therapy and conventional cancer treatments in an integrative setting.
Graphical Abstract. Graphical summary. The “Helminth infection” section demonstrates that the presence of helminths in the host's body leads to activating a sequence of immunological responses. This encompasses an elevation in pro-inflammatory molecules (IFN-α, IL-1β, IL-12) and the control of anti-inflammatory molecules (TGF-β, IFN-10). A well-regulated immune response can decrease cellular harm and DNA alterations resulting from inflammation, diminishing the likelihood of developing colorectal cancer precursor lesions. Nevertheless, the helminth infection's immunosuppressive environment may also facilitate the growth and spread of cancer, adversely affecting patient outcomes. The "Parasite antigens" section illustrates the mechanisms by which dendritic cells (DCs) internalize and digest parasite antigens, presenting them to CD8+ T lymphocytes. Activated T cells secrete cytotoxic chemicals such as PFN and GzmB, as well as cytokines like IFN-γ and TNF-α, which cause cancer cells to die. This approach proposes that antigens produced from helminths have the potential to be utilized as vaccines or therapies for colorectal cancer. This would include utilizing the immune system to specifically target and eliminate cancer cells. The section on “CRISPR/Cas9 gene editing helminth” focuses on the progress made in gene editing technologies, specifically CRISPR/Cas9, that enable accurate alterations to the helminth genome. Researchers can reduce the harmfulness of live helminths by selectively focusing on particular sequences in the genomic DNA. These genetically altered parasitic helminths can be employed to control the levels of microRNA, decrease the expression of cancer-promoting microRNAs, and stabilize the messenger RNA of tumor suppressor genes, so effectively impeding the formation of tumors.The “Helminth infection” section demonstrates that the presence of helminths in the host’s body leads to activating a sequence of immunological responses. This encompasses an elevation in pro-inflammatory molecules (IFN-α, IL-1β, IL-12) and the control of anti-inflammatory molecules (TGF-β, IFN-10). A well-regulated immune response can decrease cellular harm and DNA alterations resulting from inflammation, diminishing the likelihood of developing colorectal cancer precursor lesions. Nevertheless, the helminth infection’s immunosuppressive environment may also facilitate the growth and spread of cancer, adversely affecting patient outcomes.The “Parasite antigens” section illustrates the mechanisms by which dendritic cells (DCs) internalize and digest parasite antigens, presenting them to CD8+ T lymphocytes. Activated T cells secrete cytotoxic chemicals such as PFN and GzmB, as well as cytokines like IFN-γ and TNF-α, which cause cancer cells to die. This approach proposes that antigens produced from helminths have the potential to be utilized as vaccines or therapies for colorectal cancer. This would include utilizing the immune system to specifically target and eliminate cancer cells.The section on “CRISPR/Cas9 gene editing helminth” focuses on the progress made in gene editing technologies, specifically CRISPR/Cas9, that enable accurate alterations to the helminth genome. Researchers can reduce the harmfulness of live helminths by selectively focusing on particular sequences in the genomic DNA. These genetically altered parasitic helminths can be employed to control the levels of microRNA, decrease the expression of cancer-promoting microRNAs, and stabilize the messenger RNA of tumor suppressor genes, so effectively impeding the formation of tumors.
Poor hygienic conditions mainly cause helminth infections, particularly in less developed socioeconomic and industrialized areas (1). A common feature of these helminths is their ability to survive long-term within the host (2). This requires helminths to possess efficient strategies to evade the host’s immune system attacks. Regardless of the type of helminth, they are rapidly detected by the immune system, triggering responses from stromal cells such as epithelial cells and keratinocytes, which secrete alarm protein molecules such as thymic stromal lymphopoietin, IL-25, and IL-33 (3). These proteins impact type 2 innate lymphoid cells (ILCs), which release cytokines including IL-5 and IL-13. In particular, IL-13 can interact with dendritic cells (DCs) to assist them in polarizing naïve T cells into a Th2 phenotype (4), suppressing pro-inflammatory reactions.
Colorectal cancer (CRC) is one of the leading tumors worldwide and, along with lung cancer, prostate cancer, and breast cancer, is considered a major human killer (5). According to the World Health Organization, there were 1.8 million new CRC cases diagnosed globally in 2018, with 862,000 deaths attributed to CRC (6). The public health concerns associated with CRC are more serious due to its growing prevalence worldwide. This underscores the pressing need to enhance preventive, early detection, and therapeutic approaches to mitigate their adverse impact on global health and socioeconomic circumstances (7). However, conventional treatments for CRC have demonstrated slight effectiveness, underscoring the pressing need for novel therapeutic strategies.
Some species of helminths have been proposed as potential treatments for diseases such as inflammatory bowel disease, celiac disease, atherosclerosis, non-alcoholic fatty liver disease, and multiple sclerosis (8–12). Many studies have also indicated that helminths can potentially treat cancer (13–17). This review aims to investigate the potential of helminths to enhance risk factors associated with the development of CRC, prevent the occurrence of CRC, and analyze the correlation between helminths and the development and prognosis of CRC.
Specific genetic mutations in oncogenes, tumor suppressor genes, and genes associated with DNA repair pathways are the cause of CRC. It starts from polyps and abnormal crypts, progresses to early adenomas, then to advanced adenomas (Figure 1A), and eventually results in CRC. About 70% of CRC cases follow this mutation sequence. While genetic and environmental factors both influence CRC development, most cases are sporadic, with only around 25% having a family history, indicating that acquired factors are key contributors (18).
Figure 1. Mechanisms of intestinal inflammation, metabolic dysfunction, and CRC development. (A) Progression from normal epithelium to CRC, including hyperplasia, formation of small and large adenomatous polyps, progression to severe dysplasia, adenocarcinoma, and ultimately cancer. (B) Role of metabolic dysfunction in CRC. Adipose tissue releases leptin and insulin, activating the PI3K/AKT/mTOR signaling pathway in intestinal cells. This activation promotes tumor cell survival and proliferation, leading to CRC development. (C) Impact of inflammatory bowel disease (IBD) on CRC incidence. Inflammation induces activation of p53 and other key genes related to polyp formation. Chronic inflammation disrupts the balance between proinflammatory and anti-inflammatory substances, promoting DNA damage and resistance to apoptosis. (D) Changes in gut microbiota associated with IBD and metabolic diseases. IBD is associated with increased Bacteroides and Proteobacteria, while metabolic diseases like obesity and diabetes are linked to changes in Firmicutes, Bacteroides, Roseburia, and Faecalibacterium populations. These changes enhance pro-inflammatory conditions, reduce butyrate production, and facilitate the formation of a cancer-prone environment.
Obesity and diabetes significantly increase CRC risk. Obesity, linked to leptin production by adipose tissue, activates macrophage growth, migration, and cytokine production and stimulates multiple signaling pathways such as JAKs/STATs and PI3K/AKT, which promote cancer cell proliferation and metastasis (Figure 1B) (19, 20). Similarly, diabetes accelerates CRC development via insulin resistance and IGF system alterations, with elevated IGF-I levels promoting cell proliferation and cancer growth (21).
Patients with IBD have a 2–6 times higher CRC risk due to complex carcinogenic mechanisms (22). Inflammation causes oxidative stress and DNA damage, triggering a sequence from inflammation to dysplasia to cancer (Figure 1C) (23, 24). This process activates oncogenes and deactivates tumor suppressor genes like p53 (25, 26). IBD also induces chromosomal instability and disrupts intestinal ecology, promoting CRC through the production of carcinogens and the breakdown of epithelial barriers (27, 28). The indirect carcinogenic pathway involves cytokines released by inflammatory and epithelial cells, with IL-6 playing a crucial role in CRC pathogenesis through JAK-STAT3 activation. Tumor-associated macrophages and leukocytes further drive chronic inflammation and carcinogenesis (29, 30). In IBD patients, these mechanisms significantly increase CRC risk, highlighting the importance of early diagnosis and effective treatment to reduce the risk of progression.
Alterations in gut microbiota composition also contribute to CRC risk. IBD and metabolic diseases disrupt the microbial balance, increasing CRC incidence. IBD patients have a lower microbial gene count, with Bacteroides and Proteobacteria increased, while healthy individuals predominantly have Actinobacteria and Verrucomicrobia (Figure 1D) (31). In obese patients, Firmicutes increase, while Bacteroides decrease, leading to increased intestinal permeability and inflammation. This dysbiosis promotes the transition from adenoma to invasive cancer. The loss of butyrate-producing bacteria in obesity and type 2 diabetes further promotes inflammation and tumorigenesis (32, 33).
Moreover, reduced microbial diversity is strongly linked to CRC development, as it activates NF-κB signaling and triggers inflammatory processes, which contribute to carcinogenesis (34, 35). Therefore, regulating microbial composition may offer a promising approach to CRC prevention and treatment.
Specific cytokines, such as FOXP3, TNF-α, and IFN-γ, regulate tumor immunity, often with elevated expression in CRC. FOXP3 activation in cancer cells leads to cytokine secretion (TGF-β, IL-10), suppressing immunity, and is linked to poor prognosis (36–38). TNF-α, produced by macrophages, promotes epithelial-mesenchymal transition, aiding metastasis; high TNF-α expression predicts tumor deterioration (39, 40). IFN-γ activates macrophages, with its deficiency promoting CRC development. In contrast, specific IFN-γ expression enhances innate immunity and suppresses tumors, correlating with better prognosis (41–43).
Several interleukins contribute to CRC progression. IL-1β, a pro-inflammatory cytokine, boosts cell proliferation and increases CRC risk (44, 45). IL-17 from CD4+ T cells supports tumor development and angiogenesis by stimulating VEGF production in cancer cells (46, 47). Elevated levels of IFN-γ, IL-12, IL-15, and IL-18 are linked to favorable CRC outcomes, while IL-4, IL-6, IL-17, TNF, TGF-β, and VEGF indicate tumor progression (48–52).
The intricate and significant subject of helminth infection’s influence on tumor growth. Some helminth infections are indeed associated with cancer, such as clonorchiasis and Opisthorchis viverrini (pathogens of cholangiocarcinoma) and Schistosoma japonicum (a risk factor for liver and CRC) (53, 54). The regulation of immune responses by parasitic infections is also an important aspect. For instance, the generated Th1 immune response can suppress tumor growth and be essential in the early stages of Eimeria granulosa infection to eliminate cancer cells (55, 56); however, as the infection progresses, the activation of the Th2 immune response may promote tumor progression and metastasis. This phase-dependent change in immune response requires further research to understand its impact on tumor development (57, 58). Additionally, the anti-cancer activity induced by parasitic infection is limited by the virulence of the live parasite and the morbidity induced. Although attempts to use live vaccination strategies have garnered significant attention, their effectiveness is not perfect. Therefore, consideration is being given to using parasite-derived products as new therapeutic agents (59–61).
Helminth infections often mediate immune responses that lean towards immunosuppression. For example, through a TGF-β-dependent mechanism, the excretory/secretory (E/S) products of Echinococcus multilocularis (E. multilocularis) larvae induce the transformation of CD4(+) T cells into Foxp3(+) Tregs in vitro. When T cells come into contact with E/S products, they release more of the immunosuppressive cytokine IL-10 (62). Helminth-generated extracellular vesicles (EVs), similar to exosomes, also play a crucial role in parasite-host interactions. For example, EVs from Nippostrongylus brasiliensis protect mice from intestinal inflammation when administered via a single intraperitoneal injection. Important inflammatory cytokines linked to colitis pathophysiology, including IL-6, IL-1β, IFNγ, and IL-17a, were markedly suppressed in the colon tissues of mice given EVs (63).
CD4+ T cell subsets play a key role in the host’s protective response against expelling helminths while also regulating many inflammation and immune parameters associated with parasite expulsion (64) Th1 cells facilitate the control of intracellular infections by generating cytokines such as IFN-γ, IL-2, and IL-12. However, in most cases, the host’s immune response to helminth infections is primarily driven by Th2-like T helper cell responses, significantly producing IL-4, IL-5, IL-10, IL-13, IL-25, and IL-31 (65–68), to facilitate protective settlement against helminths (69). Epithelial cells are among the first cell types to encounter intestinal helminths (70). Therefore, when stimulated, damaged, or dying, they release cytokines, including IL-25 and IL-33, which are crucial in inducing innate immune responses and promoting type 2 inflammation processes (71). The interaction of these immune factors leads to the further development of immune responses, including the recruitment of eosinophils, B cells, and M2 macrophage activation (72); helminth infections can also increase mucus production by intestinal goblet cells, promoting beneficial bacterial growth; inhibiting harmful bacteria, and exerting anti-inflammatory effects (Figure 2) (73, 74).
Figure 2. Intestinal immune response process induced by parasitic infection. Recognition and dissemination: (A) Parasitic antigens are recognized and captured by antigen-presenting cells (APCs) in the lamina propria. (B) Induction: APCs activate T cells in the mesenteric lymph nodes. TGF-β and IL-10 promote the differentiation of regulatory T cells (Tregs), while IL-4 promotes the differentiation of Th2 cells. Th2 cells secrete IL-4 and IL-13, further stimulating plasma cells to produce IgA, IgM, and IgE antibodies. Meanwhile, Th17 and Th1 cell responses are inhibited, reducing the production of IFN-γ and TNF-α. (C) Clearance and resolution: In the lamina propria, ILC2 cells secrete IL-4, IL-13, and IL-22, recruiting and activating eosinophils and M2 macrophages to promote anti-parasitic immune responses. Monocytes also participate in the resolution of inflammation under the influence of IL-10 and TGF-β. Through multiple pathways, the inflammatory response is regulated, restoring intestinal homeostasis.
Current cancer immunotherapy strategies aim to stimulate host immune cells to target tumor-associated antigens (TAAs), either directly or indirectly, to attack cancer cells (75). Vaccination remains a promising and significant approach to cancer immunotherapy. However, clinical barriers, such as immune tolerance to TAAs, limited immunogenicity of TAAs, and active immune evasion mechanisms employed by advanced cancers, hinder its success (76). Overcoming these barriers may require non-toxic immune modulators or adjuvants to boost both innate and adaptive tumor-specific immune responses, forming the basis for successful vaccine formulations (77).Parasite - derived antigens may hold the key to overcoming these obstacles and improving the effectiveness of cancer vaccines.
Although a variety of tumor-specific antigens have been identified and utilized, most do not trigger strong and appropriate immune responses (78). Tumor - associated glycoproteins, such as sialyl - Tn, TF, and Tn, are often used in cancer diagnostics and prognosis and are mainly found on cancer cells (79). These glycoproteins have also been found in the adult and larval stages of Schistosoma mansoni and in patients with cystic echinococcosis (CE) (80). There is evidence that hydatid cyst antigens have anticancer effects against various types of cancer in vitro and mouse models, including CRC (81–84). Some anti-parasitic drugs can inhibit tumor growth, suggesting that there may be shared antigenic epitopes between certain parasites and cancer cells (85). Given the presence of these antigens in both parasites and cancer cells, it is not surprising that there is an immunological cross-reactivity between the two. Tn and sTn antigens linked to cancer have been identified in both the larval and adult stages of E.granulosus (80), as well as TF antigens linked to CRC (86). It has been confirmed that there is immunological cross-reactivity between the sera of cancer patients and parasite antigens (87), which means that the host immune system can recognize these common antigens and attack them. While antigens from cancer cells usually have weak immunogenicity, those from microbes often have strong immunogenicity (88, 89). Therefore, parasite - derived antigens offer a way to overcome the immunogenicity limitations of cancer antigens and may enhance immune responses against cancer cells (81). Immunizing with parasite antigens could change the immunosuppressive tumor microenvironment into an immune-supportive one, amplifying anticancer immune responses and opening up new possibilities for CRC treatments.
In the exploration of prospective vaccine targets, numerous significant antigens/proteins from helminths have demonstrated considerable promise. Heligmosomoides polygyrus antigens modulate macrophage activities, enhancing immune regulation within the tumor microenvironment and inhibiting breast cancer cell growth (90). Likewise, the E. granulosus antigen B component promotes M1/M2 macrophage polarization, hence augmenting inflammation and facilitating pathogen clearance (91). Specific glycans generated from parasites, including IL-33 modulators, enhance the activation of antigen-presenting cells such as dendritic cells, highlighting their potential in cancer immunotherapy (92). E. granulosus antigens, in both crude hydatid cyst forms and Tn-like peptides, elicit significant IFN-γ production in immunized mice, signifying Th1 polarization (93). Antigens from Trypanosoma cruzi, employed in CRC-specific anticancer vaccines, have demonstrated considerable efficacy in inhibiting tumor growth in the colon in many investigations (13, 15). Additionally, a new recombinant protein from Toxoplasma gondii, rGRA6Nt, has demonstrated the ability to limit tumor growth in murine colorectal cancer models while simultaneously tripling the density of CD8+ T cells within the tumors (94). These findings highlight the revolutionary potential of parasite antigens in the advancement of successful cancer immunotherapies.
Parasite antigens, sharing immunomodulatory pathways with viral antigens, have demonstrated the ability to upregulate immune-activating genes and enhance T cell activity in HTLV-1 virus models, echoing mechanisms observed in tumor immunotherapy (95). These antigens also exhibit potential in managing immune toxicities, such as CAR-T therapy-related neurotoxic syndromes (96). Their broad immunological and biological activities position them as valuable tools for the next generation of cancer immunotherapy.
The regulation of CRC immune factors by helminth infections is a complex process worthy of in-depth exploration. Changes in cytokines can reflect the impact of helminth infections on the immune system, where pro-carcinogenic factors such as IL-10, TGF-β, and IL-35 show an increased risk, while anti-carcinogenic factors like IL-6, IL-1β, IFNγ, and IL-17a exhibit a downward trend. This phenomenon suggests that helminth infections may primarily promote carcinogenesis. The International Agency for Research on Cancer has categorized Schistosoma haematobium (S. haematobium) as a Group 1 carcinogen (97), closely associated with the incidence of bladder squamous cell carcinoma. S. mansoni, on the other hand, is classified as Group 3, with insufficient evidence currently confirming its carcinogenicity in humans. Nonetheless, new findings from studies using cell cultures and animal models indicate that S. haematobium may promote the development of liver and CRC (98). Recent studies also indicate that certain parasitic infections can lead to downregulation of tumor suppressor genes; for instance, Theileria annulata infection promotes p53 suppression and genomic instability (99). Similarly, Toxocara canis (T. canis)infection may create an immunosuppressive tumor microenvironment, increasing tumor size and weight and potentially increasing the risk of breast cancer by reducing P53 gene expression (100–102). The evidence linking helminth infections to carcinogenesis is substantial (Table 1), suggesting that despite various interacting factors contributing to CRC, direct use of live helminth infections as a therapeutic agent for CRC is not advisable. Without sufficient clinical trial support, we cannot definitively determine how helminth infections affect immune responses. Therefore, a more profound understanding of the mechanisms of helminth infections is needed to develop more effective strategies for CRC prevention and treatment.
When exploring the impact of helminth infections on immune regulation in CRC, it is essential to consider the risk factors in epidemiology and think about how to mitigate them to prevent CRC. Inflammation is a common characteristic among these risk factors. Therefore, controlling inflammatory immune responses becomes a key strategy in reducing risk.
Helminth infections positively impact the treatment of IBD (118, 119). Research primarily focuses on two regulatory factors: IL-10 and TGF-β (120–122) IL-10 and TGF-β can inhibit the production of inflammatory mediators. Engineered lactobacilli expressing IL-10 successfully prevented the development of colitis, showing potential as a treatment for IBD (123, 124). Studies have confirmed that Heligmosomoides polygyrus (H. polygyrus) can relieve colitis symptoms in animals lacking IL-10 (125) and that schistosome eggs protect mice against colitis produced by TNBS (126). Increasing TGF-β activity has also shown potential value in treating inflammatory bowel disease (127, 128). Helminth infections can induce the production of the Th2 cytokine IL-4, further activating and driving the output of TGF-β (129, 130). This plays a key role in inhibiting the autoreactive Th1 and Th17 responses that mediate autoimmune diseases (131).
A meta-analysis showed that some parasitic infections benefit human metabolism, such as lowering fasting blood glucose and HbA1c levels and reducing the prevalence of metabolic syndrome and type 2 diabetes (132). In managing obesity and diabetes, studies have found that various parasitic helminths positively impact the immune response to obesity or malnutrition by regulating Th2 immune responses (133). Helminth infections significantly reduced insulin resistance, liver fat accumulation, and fatty acid synthase gene expression in mice, possibly due to the upregulation of Th2 factors promoting the production of alternatively activated macrophages, which secrete IL-10 to inhibit inflammation (134). Research also indicates that Omega-1, a substance from Schistosoma mansoni(S. mansoni) eggs, effectively induces Th2 cell responses in mice and improves obesity caused by a high-fat diet (135–137). Studies have found that various soil-transmitted helminths, such as Ascaris spp and Ancylostoma duodenale, can improve BMI, enhance tissue insulin sensitivity, and reduce the risk of metabolic syndrome (138). Additionally, mice infected with H. polygyrus and their offspring showed significantly reduced weight gain on a high-fat diet, possibly due to changes in gut microbiota and increased short-chain fatty acid levels (139). These results suggest that helminth infections not only inhibit obesity but also potentially positively affect the treatment of diabetes (140).
The preservation of human health depends on gut bacteria (141). The interaction between parasites and the gut microbiota also significantly impacts host health (142). The gut microbiome’s diversity and abundance change when helminths are present (143). According to studies, mice infected with nematodes that resemble hookworms had far lower fasting blood glucose levels, with a marked increase in Lactobacillus in their gut microbiota (144), a genus known for its benefits in T2D (145). Research has also found that helminth infections can indirectly regulate NE concentration to inhibit obesity by altering the gut microbiota (146). Because the gut microbiotas of mice and humans differ significantly, more research is required to determine the precise effects of helminths on the gut microbiota, even though these findings highlight the positive impact of helminths on the host gut microbiota, particularly in improving metabolic health and resisting metabolic-related diseases.
Although helminth infections may promote cancer development, certain derivatives of these helminths show anticancer potential. For example, high-pressure sterilized S.mansoni antigens have exhibited anticancer protective effects in DMH-induced Colorectal cancer mice (147). Similarly, antigens from T.canis can stimulate immune responses and suppress cancer cells at high concentrations (148). It has been demonstrated that T.canis extracts stimulate human leukocytes to produce Th1 and regulatory cytokines. Their secreted proteins are highly similar to anticancer drugs in computer analyses (148). Additionally, antigens from E.granulosus have demonstrated anticancer effects in vitro and animal models; for instance, EgKI-1 can induce anticancer effects in tumor tissues, suggesting potential development as a cancer vaccine (78). Recent studies have further shown that molecules derived from the helminth Taenia crassiceps (T. crassiceps) can enhance the effectiveness of the chemotherapeutic agent 5-fluorouracil (5FU) in treating CRC. T. crassiceps modulates inflammatory cytokines, alters the tumor microenvironment, and promotes the recruitment of immune cells such as NK cells and CD8+ T cells, thereby increasing tumor cell apoptosis and reducing tumor growth (149).
Research on helminth-derived compounds for anticancer purposes is not limited to these examples alone. Many in vitro and animal experiments have shown that helminth derivatives have inhibitory effects on cancer, including studies on CRC (Table 2). These findings provide new perspectives on the complex relationship between helminth infections and cancer and open new possibilities for developing therapeutic strategies based on helminth-derived compounds.
Helminth therapy may become an option for the prevention and treatment of CRC. Despite some helminths having carcinogenic potential, antigens derived from helminths are being studied for their potent anti-cancer activity while minimizing side effects as much as possible (93). To generate helminth antigens at high concentrations, researchers should concentrate on creating standardized and controlled helminth antigen compounds by carefully identifying and extracting crucial immunogenic components (158). Antigen composition and structure can be precisely manipulated to increase antigen safety and effectiveness. Engineered recombinant antigens allow for fusion and modification to achieve maximum anti-cancer efficacy. These antigen characteristics include enhancing immunity, reducing side effects, or improving tissue targeting (159). When used in combination with conventional therapies, helminth therapy may produce unexpected results. Immune system-focused treatments, including immune checkpoint inhibitors or adoptive cell therapy, may benefit more from helminth-induced immune regulation (Figure 3A) (160).
Figure 3. Overview of helminth-based colorectal cancer (CRC) therapy approaches. (A) Combination Therapy: Integration of helminth therapy with conventional cancer treatments (chemotherapy, hormone therapy, and targeted therapy) enhances therapeutic outcomes. Helminth therapy modulates the tumor microenvironment, improves immune responses, and increases the sensitivity of cancer cells to cytotoxic treatments. (B) CRISPR/Cas9 Gene Editing and miRNA Modulation: Engineering helminths via CRISPR/Cas9 enables precise genetic modifications, such as reducing carcinogenic risks and tailoring parasites for therapeutic purposes. This technology enhances the safety of live vaccines and improves their anti-cancer effects. Additionally, helminth-derived molecules and attenuated parasites can regulate host miRNAs, particularly oncomiRs like miR-21, restoring tumor suppressor gene expression and reducing tumor progression. (C) Helminth Delivery System: Nematodes, such as Anisakis simplex, are coated with hydrophilic polymers to bypass immune detection, enabling targeted delivery of therapeutic molecules to tumors. This novel delivery system shows promise for improving drug precision and effectiveness.
Depending on the stage of cancer, for inflammatory cancers, helminth therapy can enhance the regulatory and anti-inflammatory milieu it induces, hence improving immune responses to malignancies (161). Additionally, helminth therapy may sensitize cancer cells to chemotherapy, making them more susceptible to the cytotoxic effects of chemotherapy. Helminth therapy can reduce the amount of time and dose required for treatment while increasing the effectiveness of chemotherapy by altering the tumor microenvironment and boosting immune responses (162).
Live parasitic infections may exacerbate symptoms of CRC and other cancers; hence, utilizing gene editing technology is a promising approach when considering live vaccines. With the rapid advancement of biotechnology, next-generation CRISPR gene editing technologies such as CRISPR-Cas13 (163) can be employed to engineer parasites with specific traits: short lifecycle, inability to proliferate, or sensitivity to particular drugs. Recent genetic engineering tools like CRISPR/Cas9 allow for gene knockout/deletion in parasitic helminths, with potential for future exploration in gene knock-in/insertion within parasite genomes (Figure 3B) (164). Current research indicates that CRISPR/Cas9 gene editing can enhance the safety of parasite eggs (165), particularly those of blood flukes, affirming the feasibility of developing and applying S. mansoni, a carcinogenic parasite, for CRC therapy. CRISPR and CRISPR-based alternative technologies will continue to thrive, potentially aiding the development of new strategies involving parasite antigens to tame indigenous parasitic helminths (166), thereby integrating more carcinogenic parasites into helminth therapy shortly.
A class of molecules known as small non-coding RNAs (miRNAs) that regulate gene expression (167) is thought to be important for cancer treatment. They participate in tumorigenesis by modulating signaling pathways critical for processes such as proliferation, apoptosis, and migration of cancer cells (168–170). Genome analysis studies have revealed dysregulation of multiple miRNAs in cancer, with those overexpressed targeting tumor suppressor genes and stimulating cell proliferation, angiogenesis, and metastasis termed as oncomiRs (171). miR-21 is a widely reported oncomiR, upregulated in CRC (172, 173), suggesting that modulating its expression levels could be a therapeutic approach to inhibit tumor development.
Parasites also manipulate host immune responses by releasing miRNAs to establish chronic infections (174–176). Some parasites release exosomes containing miRNAs that can be absorbed by host cells and affect gene expression (177), especially taken up by immune cells such as dendritic, macrophage, and monocytes (178). Subsequent enzymatic and protein interaction cascades mediate gene expression in eukaryotes (179). According to related studies, after infection with attenuated Leishmania donovani, expression of miR-21 significantly decreases in macrophages and DCs. In contrast, cells infected with wild-type parasites show higher miR-21 expression (Figure 3B). We observe that attenuated parasites demonstrate anticancer potential in this regard, whereas untreated parasites not only lack anticancer effects but further promote oncomiR upregulation (180). Therefore, using live attenuated parasite vaccines or other therapeutic approaches may contribute to cancer treatment.
Wildan Mubarok and colleagues have developed an innovative method for cancer treatment using Anisakis simplex. These helminths are immersed in a phenol polymer, forming a hydrophilic coating that protects them from the immune system. In vitro experiments have demonstrated successful drug delivery and cytotoxicity (Figure 3C). This technique is not limited to Anisakis simplex; it can also be applied to other nematode species, with functional molecules being replaceable as needed. In the future, nematodes could be used to deliver functional “cargo” to a range of specific targets, playing a significant role in cancer treatment. Utilizing a helminth delivery system for cancer therapy is an innovative and promising research direction (181).
By protecting nematodes from immune system attacks and leveraging their natural affinity for cancer cells and delivery capabilities, this method shows significant potential for improving the precision and effectiveness of anticancer drug delivery (181). However, the hydrogel sheath cannot completely maintain internal pH levels against external influences, meaning that the activity of enzymes and nematodes is still affected by pH fluctuations. Applying this technology to colorectal cancer (CRC) treatment remains a challenge. With further research and multicenter trials, this technique could potentially be used in clinical settings, offering new treatment options for cancer patients.
As a potential therapeutic approach, helminth therapy shows promise in treating conditions like inflammatory bowel disease. However, its safety concerns also warrant attention. Implanting attenuated live helminth vaccines may pose various safety risks, such as direct induction of CRC, uncontrolled infections, organ migration, and potential adverse reactions. Comprehensive assessment and analysis are necessary when selecting helminths to understand their infectivity and potential side effects on human health, ensuring treatment safety and efficacy (182). Currently, the lack of standardized treatment protocols for helminth therapy increases uncertainty in the treatment process. Establishing standardized treatment protocols is crucial to safeguard the safety and effectiveness of treatment (183). Preclinical research and limited clinical trials are essential for evaluating the safety and efficacy of helminth therapy. Sufficient experimentation and validation are needed to advance helminth therapy development and ensure its safety.
With the advancement of modern medicine and information technology, research on parasitic therapy is gradually deepening. We can utilize modern detection techniques to monitor the real-time effects of live vaccines in patients, such as the Helminth Egg AutoDetection (HEAD) system (184)、Kato-Katz method (185)、Mini-FLOTAC technique (186)、qPCR (187) and other latest monitoring technologies. These enable patients to monitor parasite quantity and activity, ensuring infections remain manageable.
Translating these discoveries from murine models to clinical applications is a formidable undertaking. A significant problem stems from the variety of parasitic worm species and the intricacy of their life cycles. This raises issues regarding safety and tolerance, as the introduction of live helminths or their components may elicit unforeseen side effects, including heightened vulnerability to additional infections (188, 189). Moreover, the optimal dosage or duration of parasite infections required to provide protection in humans remains largely unclear, and apprehensions about the possible adverse effects of these infections have impeded clinical research. The analysis of clinical data on experimental helminth infections is further complicated by significant immunological heterogeneity among diverse genetic backgrounds. Indeed, not all research has shown an effect of helminth infections or deworming on allergic inflammation (190, 191), aligning with the absence of therapeutic benefits of helminth infections in human autoimmune allergic inflammation (192–194).
Consequently, the selection of suitable helminth species and the determination of the ideal therapy dosage and duration continue to be important unresolved issues in scientific research and clinical practice. While proteins produced from helminths exhibit potential in modifying immune responses and mitigating inflammation in conditions such as inflammatory bowel disease and asthma, the exact mechanisms of their actions remain inadequately elucidated (195, 196). The control of pro-inflammatory cytokines and the activation of anti-inflammatory responses have been noted; however, these effects are typically localized and lack consistent replication at the systemic level (196, 197). Moreover, elements such as host genetics, food, and environmental variables influence variability in treatment responses, complicating the uniformity of therapy regimens (61). Species-specific variations among helminths and the development of suitable dosage regimens are critical elements that necessitate more research to enhance treatment results. Similar to other biological therapies, helminthic treatments also pose the danger of resistance development, as the host immune system may progressively establish tolerance, hence diminishing therapeutic efficacy over time (198). The clinical efficacy of helminth therapy may be considerably influenced by the formulation and storage conditions of the therapeutic helminths. Experimental investigations indicate that inappropriate pH levels during storage can diminish the therapeutic efficacy of Trichuris suis eggs (199). The advancement of helminth-derived biopharmaceuticals encounters additional obstacles concerning the pharmacokinetics and molecular characteristics of these substances (200).
The notion of employing parasites as a therapeutic strategy frequently encounters skepticism and apprehension from the public, which constitutes a significant obstacle to the acceptance and implementation of helminth therapy (201). Moreover, cultural and societal conceptions of helminths vary considerably. In Western societies, where personal hygiene and environmental sanitation are emphasized, the concept of putting helminths into the human body is especially difficult to comprehend. This cultural aversion may hinder the acceptability of helminth therapy and its incorporation into conventional medical practice (11, 202).
Helminth therapy encounters substantial regulatory obstacles owing to the intricate and rigorous approval processes for biological therapeutics. Moreover, comprehensive clinical trials are necessary to establish both safety and efficacy, which can be costly and time-consuming (203). Moreover, the economic obstacles linked to the development and commercialization of helminth-based medicines introduce an additional level of complexity. The expenses associated with research, development, and regulatory approval might be too costly, especially for small enterprises or research groups (203).
CRC continues to be a significant global health concern, requiring the investigation of novel treatment approaches. This review examines multiple facets of helminths in relation to colorectal cancer. Helminth therapy has considerable potential for treating precursor illnesses of colorectal cancer, such as colitis, obesity, diabetes, and changes in gut flora. By inhibiting the immune system’s reaction to helminth invasion, it is possible to decrease cell damage resulting from inflammation and DNA changes, thereby reducing the likelihood of developing precancerous lesions. However, the possible hazards and therapeutic benefits of helminths are highlighted. Helminth infections can exacerbate cancer progression by weakening the immune system, which is a notable risk factor. On the other hand, antigens derived from helminths exhibit encouraging anti-cancer characteristics and have the potential to be employed as novel constituents in vaccines or therapeutic agents.
Gene editing tools, like CRISPR/Cas9, can mitigate the adverse consequences of live helminth infections while preserving their beneficial therapeutic benefits. One potential approach is to explore the use of helminths to modulate oncomiRs associated with colorectal cancer, to mitigate the abnormal expression of tumor suppressor genes. In addition, helminth delivery systems provide a promising therapeutic strategy by utilizing helminths to transport anticancer medications to specific locations in the colon for more efficient treatment of colorectal cancer.
Future research should give priority to the advancement of standardized helminth antigen compounds, with a particular focus on enhancing their immunogenicity and ensuring their safety. Utilizing helminth antigens to stimulate anticancer immune responses can result in immunotargeted therapy, which, when paired with conventional cancer treatments, can yield remarkably potent outcomes. It is essential to develop uniform standards and monitoring systems for the clinical use of helminth treatment. Using sophisticated detection methods to control helminth infections in controlled situations can improve the safety of helminth therapy. We anticipate that future studies will yield additional evidence substantiating the clinical effectiveness of this therapy, thereby providing a cost-effective and efficient treatment alternative for CRC patients globally.
HYL: Conceptualization, Funding acquisition, Methodology, Writing – original draft, Writing – review & editing. CS: Data curation, Formal Analysis, Software, Visualization, Writing – original draft, Writing – review & editing. YHZ: Investigation, Project administration, Resources, Supervision, Validation, Writing – review & editing. XY: Investigation, Methodology, Writing – review & editing. LL: Methodology, Writing – review & editing. XZ: Writing – review & editing, Methodology, Supervision. YQ: Methodology, Writing – review & editing. YW: Methodology, Writing – review & editing. JX: Methodology, Supervision, Writing – review & editing. YJZ: Conceptualization, Supervision, Writing – review & editing. HRL: Methodology, Supervision, Writing – review & editing. LZ: Formal Analysis, Methodology, Writing – review & editing. KC: Conceptualization, Supervision, Writing – review & editing.
The author(s) declare that financial support was received for the research and/or publication of this article. This research was funded by the National Natural Science Foundation of China (Grant No: 32000293); Guangxi Natural Science Foundation (Grant Nos: 2020JJA130077 and 2018JJB140423); and Zhejiang Shuren University Basic Scientific Research Special Funds (Grant No: 2024XZ014).
Parts of the materials used in the images within this article originate from Biorender, which we have further modified and enhanced. We hereby express our gratitude.
The authors declare that the research was conducted in the absence of any commercial or financial relationships that could be construed as a potential conflict of interest.
All claims expressed in this article are solely those of the authors and do not necessarily represent those of their affiliated organizations, or those of the publisher, the editors and the reviewers. Any product that may be evaluated in this article, or claim that may be made by its manufacturer, is not guaranteed or endorsed by the publisher.
1. Braseth AL, Elliott DE, Ince MN. Parasitic infections of the gastrointestinal track and liver. Gastroenterol Clinics. (2021) 50:361–81. doi: 10.1016/j.gtc.2021.02.011
2. Paily K, Hoti S, Das P. A review of the complexity of biology of lymphatic filarial parasites. J Parasitic Dis. (2009) 33:3–12. doi: 10.1007/s12639-009-0005-4
3. Wiedemann M, Voehringer D. Immunomodulation and immune escape strategies of gastrointestinal helminths and schistosomes. Front Immunol. (2020) 11:572865. doi: 10.3389/fimmu.2020.572865
4. Maazi H, Akbari O. Type two innate lymphoid cells: the janus cells in health and disease. Immunol Rev. (2017) 278:192–206. doi: 10.1111/imr.2017.278.issue-1
5. Labianca R, Beretta GD, Kildani B, Milesi L, Merlin F, Mosconi S, et al. Colon cancer. Crit Rev oncology/hematology. (2010) 74:106–33. doi: 10.1016/j.critrevonc.2010.01.010
6. Siegel RL, Miller KD, Jemal A. Cancer statistics, 2019. CA: Cancer J Clin. (2019) 69:7–34. doi: 10.3322/caac.21551
7. Yu D, Ou Z, Zhang W, He H, Li Y, He W, et al. Global and national trends in years of life lost and years lived with disability caused by three common gastrointestinal cancers from 1990 to 2019. BMC Gastroenterol. (2022) 22:493. doi: 10.1186/s12876-022-02567-5
8. Atagozli T, Elliott DE, Ince MN. Helminth lessons in inflammatory bowel diseases (Ibd). Biomedicines. (2023) 11(4):1200. doi: 10.3390/biomedicines11041200
9. Croese J, Miller GC, Marquart L, Llewellyn S, Gupta R, Becker L, et al. Randomized, placebo controlled trial of experimental hookworm infection for improving gluten tolerance in celiac disease. Clin Transl Gastroenterol. (2020) 11:e00274. doi: 10.14309/ctg.0000000000000274
10. Yang Y, Ding X, Chen F, Wu X, Chen Y, Zhang Q, et al. Inhibition Effects of Nippostrongylus Brasiliensis and Its Derivatives against Atherosclerosis in Apoe(-/-) Mice through Anti-Inflammatory Response. Pathogens. (2022) 11(10):1208. doi: 10.3390/pathogens11101208
11. Liu X, Jiang Y, Ye J, Wang X. Helminth infection and helminth-derived products: A novel therapeutic option for non-alcoholic fatty liver disease. Front Immunol. (2022) 13:999412. doi: 10.3389/fimmu.2022.999412
12. Tanasescu R, Tench CR, Constantinescu CS, Telford G, Singh S, Frakich N, et al. Hookworm treatment for relapsing multiple sclerosis: A randomized double-blinded placebo-controlled trial. JAMA Neurol. (2020) 77:1089–98. doi: 10.1001/jamaneurol.2020.1118
13. Ubillos L, Freire T, Berriel E, Chiribao ML, Chiale C, Festari MF, et al. Trypanosoma cruzi extracts elicit protective immune response against chemically induced colon and mammary cancers. Int J Cancer. (2016) 138:1719–31. doi: 10.1002/ijc.v138.7
14. Borges BC, Uehara IA, dos Santos MA, Martins FA, de Souza FC, Junior ÁF, et al. The recombinant protein based on trypanosoma cruzi P21 interacts with cxcr4 receptor and abrogates the invasive phenotype of human breast cancer cells. Front Cell Dev Biol. (2020) 8. doi: 10.3389/fcell.2020.569729
15. Freire T, Landeira M, Giacomini C, Festari MF, Pittini Á, Cardozo V, et al. Trypanosoma cruzi-derived molecules induce anti-tumour protection by favouring both innate and adaptive immune responses. Int J Mol Sci. (2022) 23:15032. doi: 10.3390/ijms232315032
16. Yue T-T, Zhang N, Li J-H, Lu X-Y, Wang X-C, Li X, et al. Anti-osteosarcoma effect of antiserum against cross antigen tpd52 between osteosarcoma and trichinella spiralis. Parasites Vectors. (2021) 14:1–13. doi: 10.1186/s13071-021-05008-6
17. Luo J, Yu L, Xie G, Li D, Su M, Zhao X, et al. Study on the mitochondrial apoptosis pathways of small cell lung cancer H446 cells induced by trichinella spiralis muscle larvae esps. Parasitology. (2017) 144:793–800. doi: 10.1017/S0031182016002535
18. Nguyen LH, Goel A, Chung DC. Pathways of colorectal carcinogenesis. Gastroenterology. (2020) 158:291–302. doi: 10.1053/j.gastro.2019.08.059
19. Soltani G, Poursheikhani A, Yassi M, Hayatbakhsh A, Kerachian M, Kerachian MA. Obesity, diabetes and the risk of colorectal adenoma and cancer. BMC endocrine Disord. (2019) 19:1–10. doi: 10.1186/s12902-019-0444-6
20. Hidayat K, Yang CM, Shi BM. Body fatness at an early age and risk of colorectal cancer. Int J Cancer. (2018) 142:729–40. doi: 10.1002/ijc.v142.4
21. Cirillo F, Catellani C, Sartori C, Lazzeroni P, Amarri S, Street ME. Obesity, insulin resistance, and colorectal cancer: could mirna dysregulation play a role? Int J Mol Sci. (2019) 20:2922. doi: 10.3390/ijms20122922
22. Mattar MC, Lough D, Pishvaian MJ, Charabaty A. Current management of inflammatory bowel disease and colorectal cancer. Gastrointest Cancer Res. (2011) 4(2):53–61.
23. Baker KT, Salk JJ, Brentnall TA, Risques RA. Precancer in ulcerative colitis: the role of the field effect and its clinical implications. Carcinogenesis. (2018) 39:11–20. doi: 10.1093/carcin/bgx117
24. Pino MS, Chung DC. The chromosomal instability pathway in colon cancer. Gastroenterology. (2010) 138:2059–72. doi: 10.1053/j.gastro.2009.12.065
25. Itzkowitz SH, Yio X. Inflammation and cancer iv. Colorectal cancer in inflammatory bowel disease: the role of inflammation. Am J physiology-gastrointestinal liver Physiol. (2004) 287:G7–G17. doi: 10.1152/ajpgi.00079.2004
26. Dirisina R, Katzman RB, Goretsky T, Managlia E, Mittal N, Williams DB, et al. P53 and puma independently regulate apoptosis of intestinal epithelial cells in patients and mice with colitis. Gastroenterology. (2011) 141:1036–45. doi: 10.1053/j.gastro.2011.05.032
27. Kay J, Thadhani E, Samson L, Engelward B. Inflammation-induced DNA damage, mutations and cancer. DNA Repair. (2019) 83:102673. doi: 10.1016/j.dnarep.2019.102673
28. Arthur JC, Jobin C. The struggle within: microbial influences on colorectal cancer. Inflammatory bowel Dis. (2011) 17:396–409. doi: 10.1002/ibd.21354
29. Feagins LA. Role of transforming growth factor-r in inflammatory bowel disease and colitis-associated colon cancer. Inflammatory bowel Dis. (2010) 16:1963–8. doi: 10.1002/ibd.21281
30. Ren W, Shen S, Sun Z, Shu P, Shen X, Bu C, et al. Jak-stat3 pathway triggers dicer1 for proteasomal degradation by ubiquitin ligase complex of cul4adcaf1 to promote colon cancer development. Cancer Lett. (2016) 375:209–20. doi: 10.1016/j.canlet.2016.02.055
31. Ghosh S, Pramanik S. Structural diversity, functional aspects and future therapeutic applications of human gut microbiome. Arch Microbiol. (2021) 203:5281–308. doi: 10.1007/s00203-021-02516-y
32. Yang J, Yu J. The association of diet, gut microbiota and colorectal cancer: what we eat may imply what we get. Protein Cell. (2018) 9:474–87. doi: 10.1007/s13238-018-0543-6
33. Pauli JR, Ropelle ER, Cintra DE, Carvalho-Filho MA, Moraes JC, De Souza CT, et al. Acute physical exercise reverses seNitrosation of the insulin receptor, insulin receptor substrate 1 and protein kinase B/akt in diettenteon. Obese wistar rats. J Physiol. (2008) 586:659–71. doi: 10.1113/jphysiol.2007.142414
34. Han S, Zhuang J, Wu Y, Wu W, Yang X. Progress in research on colorectal cancer-related microorganisms and metabolites. Cancer Manage Res. (2020) 8703–20. doi: 10.2147/CMAR.S268943
35. Ganesan K, Jayachandran M, Xu B. Diet-derived phytochemicals targeting colon cancer stem cells and microbiota in colorectal cancer. Int J Mol Sci. (2020) 21:3976. doi: 10.3390/ijms21113976
36. Grimmig T, Kim M, Germer C-T, Gasser M, Maria Waaga-Gasser A. The role of foxp3 in disease progression in colorectal cancer patients. Oncoimmunology. (2013) 2:e24521. doi: 10.4161/onci.24521
37. Liu Y, Lan Q, Lu L, Chen M, Xia Z, Ma J, et al. Phenotypic and functional characteristic of a newly identified cd8+ Foxp3– cd103+ Regulatory T cells. J Mol Cell Biol. (2014) 6:81–92. doi: 10.1093/jmcb/mjt026
38. Kim M, Grimmig T, Grimm M, Lazariotou M, Meier E, Rosenwald A, et al. Expression of foxp3 in colorectal cancer but not in treg cells correlates with disease progression in patients with colorectal cancer. PloS One. (2013) 8:e53630. doi: 10.1371/journal.pone.0053630
39. Al Obeed OA, Alkhayal KA, Al Sheikh A, Zubaidi AM, Vaali-Mohammed M-A, Boushey R, et al. Increased expression of tumor necrosis factor-s is associated with advanced colorectal cancer stages. World J Gastroenterology: WJG. (2014) 20:18390. doi: 10.3748/wjg.v20.i48.18390
40. Stanilov N, Miteva L, Dobreva Z, Stanilova S. Colorectal cancer severity and survival in correlation with tumour necrosis factor-alpha. Biotechnol Biotechnol Equip. (2014) 28:911–7. doi: 10.1080/13102818.2014.965047
41. Kosmidis C, Sapalidis K, Koletsa T, Kosmidou M, Efthimiadis C, Anthimidis G, et al. Interferon-s and colorectal cancer: an up-to date. J Cancer. (2018) 9:232. doi: 10.7150/jca.22962
42. Wang L, Wang Y, Song Z, Chu J, Qu X. Deficiency of interferon-gamma or its receptor promotes colorectal cancer development. J Interferon Cytokine Res. (2015) 35:273–80. doi: 10.1089/jir.2014.0132
43. Liu S, Yu X, Wang Q, Liu Z, Xiao Q, Hou P, et al. Specific expression of interferon-0 induced by synergistic activation mediator-derived systems activates innate immunity and inhibits tumorigenesis. J Microbiol Biotechnol. (2017) 27:1855–66. doi: 10.4014/jmb.1705.05081
44. Cohen I, Rider P, Carmi Y, Braiman A, Dotan S, White MR, et al. Differential release of chromatin-bound il-1m discriminates between necrotic and apoptotic cell death by the ability to induce sterile inflammation. Proc Natl Acad Sci. (2010) 107:2574–9. doi: 10.1073/pnas.0915018107
45. Rider P, Carmi Y, Guttman O, Braiman A, Cohen I, Voronov E, et al. Il-1n and il-1n recruit different myeloid cells and promote different stages of sterile inflammation. J Immunol. (2011) 187:4835–43. doi: 10.4049/jimmunol.1102048
46. Chae W-J, Gibson TF, Zelterman D, Hao L, Henegariu O, Bothwell AL. Ablation of il-17a abrogates progression of spontaneous intestinal tumorigenesis. Proc Natl Acad Sci. (2010) 107:5540–4. doi: 10.1073/pnas.0912675107
47. Liu J, Duan Y, Cheng X, Chen X, Xie W, Long H, et al. Il-17 is associated with poor prognosis and promotes angiogenesis via stimulating vegf production of cancer cells in colorectal carcinoma. Biochem Biophys Res Commun. (2011) 407:348–54. doi: 10.1016/j.bbrc.2011.03.021
48. Carson WE, Dierksheide JE, Jabbour S, Anghelina M, Bouchard P, Ku G, et al. Coadministration of interleukin-18 and interleukin-12 induces a fatal inflammatory response in mice: critical role of natural killer cell interferon-y production and stat-mediated signal transduction. Blood J Am Soc Hematol. (2000) 96:1465–73. doi: 10.1182/blood.v96.4.1465.h8001465_1465_1473
49. Chaisavaneeyakorn S, Othoro C, Shi YP, Otieno J, Chaiyaroj SC, Lal AA, et al. Relationship between plasma interleukin-12 (Il-12) and il-18 levels and severe malarial anemia in an area of holoendemicity in western Kenya. Clin Vaccine Immunol. (2003) 10:362–6. doi: 10.1128/CDLI.10.3.362-366.2003
50. Feng X, Zhang Z, Sun P, Song G, Wang L, Sun Z, et al. Interleukin-18 is a prognostic marker and plays a tumor suppressive role in colon cancer. Dis Markers. (2020) 2020:1–9. doi: 10.1155/2020/6439614
51. Braummaumv H, Mauerer B, Andris J, Berlin C, Wieder T, Kesselring R. The cytokine network in colorectal cancer: implications for new treatment strategies. Cells. (2022) 12:138. doi: 10.3390/cells12010138
52. Briukhovetska D, Dörr J, Endres S, Libby P, Dinarello CA, Kobold S. Interleukins in cancer: from biology to therapy. Nat Rev Cancer. (2021) 21:481–99. doi: 10.1038/s41568-021-00363-z
53. Feng M, Cheng X. Parasite-associated cancers (Blood flukes/liver flukes). Infect Agents Associated Cancers: Epidemiol Mol Biol. (2017) 193–205. doi: 10.1007/978-981-10-5765-6_12
54. Hamid HK. Schistosoma japonicum–aponicumma colorectal cancer: A review. Am J Trop Med hygiene. (2019) 100:501. doi: 10.4269/ajtmh.18-0807
55. Wegner N, Wait R, Sroka A, Eick S, Nguyen KA, Lundberg K, et al. Peptidylarginine deiminase from porphyromonas gingivalis citrullinates human fibrinogen and A-drinogen implications for autoimmunity in rheumatoid arthritis. Arthritis Rheumatism. (2010) 62:2662–72. doi: 10.1002/art.27552
56. Scher JU, Sczesnak A, Longman RS, Segata N, Ubeda C, Bielski C, et al. Expansion of intestinal prevotella copri correlates with enhanced susceptibility to arthritis. elife. (2013) 2:e01202. doi: 10.7554/eLife.01202
57. Langdon K, Phie J, Thapa CB, Biros E, Loukas A, Haleagrahara N. Helminth-based therapies for rheumatoid arthritis: A systematic review and meta-analysis. Int Immunopharmacol. (2019) 66:366–72. doi: 10.1016/j.intimp.2018.11.034
58. Allin KH, Nielsen T, Pedersen O. Mechanisms in endocrinology: gut microbiota in patients with type 2 diabetes mellitus. Eur J Endocrinol. (2015) 172:R167–R77. doi: 10.1530/EJE-14-0874
59. Darani HY, Yousefi M. Parasites and cancers: parasite antigens as possible targets for cancer immunotherapy. Future Oncol. (2012) 8:1529–35. doi: 10.2217/fon.12.155
60. Kang Y-J, Jo J-O, Cho M-K, Yu H-S, Leem S-H, Song KS, et al. Trichinella spiralis infection reduces tumor growth and metastasis of B16-F10 melanoma cells. Veterinary Parasitol. (2013) 196:106–13. doi: 10.1016/j.vetpar.2013.02.021
61. Venkatakrishnan A, Sarafian JT, Jirků-Pomajbnrish K, Parker W. Socio-medical studies of individuals self-treating with helminths provide insight into clinical trial design for assessing helminth therapy. Parasitol Int. (2022) 87:102488. doi: 10.1016/j.parint.2021.102488
62. Nono JK, Lutz MB, Brehm K. Expansion of host regulatory T cells by secreted products of the tapeworm echinococcus multilocularis. Front Immunol. (2020) 11:798. doi: 10.3389/fimmu.2020.00798
63. Ryan S, Jones L, Eichenberger RM, Buitrago G, Polster R, de Oca MM, et al. Hookworm secreted extracellular vesicles interact with host cells and prevent inducible colitis in mice. Front Immunol. (2018) 9:850. doi: 10.3389/fimmu.2018.00850
64. Kidd P. Th1/th2 balance: the hypothesis, its limitations, and implications for health and disease. Altern Med Rev. (2003) 8:223–46.
65. Fort MM, Cheung J, Yen D, Li J, Zurawski SM, Lo S, et al. Il-25 induces il-4, il-5, and il-13 and th2-associated pathologies in vivo. Immunity. (2001) 15:985–95. doi: 10.1016/S1074-7613(01)00243-6
66. Harnett W, Harnett M. Molecular basis of wormsulartt5 immunomodulation. Parasite Immunol. (2006) 28:535–43. doi: 10.1111/j.1365-3024.2006.00893.x
67. Dillon SR, Sprecher C, Hammond A, Bilsborough J, Rosenfeld-Franklin M, Presnell SR, et al. Interleukin 31, a cytokine produced by activated T cells, induces dermatitis in mice. Nat Immunol. (2004) 5:752–60. doi: 10.1038/ni1084
68. Finkelman FD, Shea-heaelma T, Morris SC, Gildea L, Strait R, Madden KB, et al. Interleukinannkli interleukinannklinnrnst host protection against intestinal nematode parasites. Immunol Rev. (2004) 201:139–55. doi: 10.1111/j.0105-2896.2004.00192.x
69. Maizels RM, Balic A, Gomez-Escobar N, Nair M, Taylor MD, Allen JE. Helminth parasites--masters of regulation. Immunol Rev. (2004) 201:89–116. doi: 10.1111/j.0105-2896.2004.00191.x
70. Sorobetea D, Svensson-Frej M, Grencis R. Immunity to gastrointestinal nematode infections. Mucosal Immunol. (2018) 11:304–15. doi: 10.1038/mi.2017.113
71. Hammad H, Lambrecht BN. Barrier epithelial cells and the control of type 2 immunity. Immunity. (2015) 43:29–40. doi: 10.1016/j.immuni.2015.07.007
72. Maizels RM, McSorley HJ. Regulation of the host immune system by helminth parasites. J Allergy Clin Immunol. (2016) 138:666–75. doi: 10.1016/j.jaci.2016.07.007
73. Ramanan D, Bowcutt R, Lee SC, Tang MS, Kurtz ZD, Ding Y, et al. Helminth infection promotes colonization resistance via type 2 immunity. Science. (2016) 352:608–12. doi: 10.1126/science.aaf3229
74. Shute A, Callejas BE, Li S, Wang A, Jayme TS, Ohland C, et al. Cooperation between host immunity and the gut bacteria is essential for helminth-evoked suppression of colitis. Microbiome. (2021) 9:1–18. doi: 10.1186/s40168-021-01146-2
75. Topalian SL, Weiner GJ, Pardoll DM. Cancer immunotherapy comes of age. J Clin Oncol. (2011) 29:4828–36. doi: 10.1200/JCO.2011.38.0899
76. Mellman I, Coukos G, Dranoff G. Cancer immunotherapy comes of age. Nature. (2011) 480:480–9. doi: 10.1038/nature10673
77. Aly HA. Cancer therapy and vaccination. J Immunol Methods. (2012) 382:1–23. doi: 10.1016/j.jim.2012.05.014
78. Buonaguro L, Petrizzo A, Tornesello ML, Buonaguro FM. Translating tumor antigens into cancer vaccines. Clin Vaccine Immunol. (2011) 18:23–34. doi: 10.1128/CVI.00286-10
79. Kudelka MR, Ju T, Heimburg-Molinaro J, Cummings RD. Simple sugars to complex diseases-molinaro0 O-glycans in cancer. Adv Cancer Res. (2015) 126:53–135. doi: 10.1016/bs.acr.2014.11.002
80. Errico DA, Medeiros A, Mıguez M, Casaravilla C, Malgor R, Carmona C, et al. O-glycosylation in echinococcus granulosus: identification and characterization of the carcinoma-associated tn antigen. Exp Parasitol. (2001) 98:100–9. doi: 10.1006/expr.2001.4620
81. Aref N, Shirzad H, Yousefi M, Darani H. Effect of different hydatid cyst molecules on hela and vero cell lines growth in vitro. J Immunodefic Disord. (2013) 2(01). doi: 10.4172/2324-853X.1000105
82. Darani HY, Sharafi SM, Mokarian F, Yousefi M, Sharafi SA, Jafari R. Therapeutic effect of hydatid cyst liquid on melanoma tumor growth in mouse model. Br J Med Med Res. (2016) 18:1–7. doi: 10.9734/bjmmr/2016/27220
83. Rostami rad S, Daneshpour S, Mofid MR, Andalib A, Eskandariyan A, Darani HY. Effect of hydatid cyst antigens on inhibition of melanoma cancer growth in mouse model. Cell Mol Biol. (2018) 64(12):1–5. doi: 10.14715/cmb/2018.64.12.1
84. Berriel E, Russo S, Monin L, Festari MF, Berois N, Fernisili G, et al. Antitumor activity of human hydatid cyst fluid in a murine model of colon cancer. Sci World J. (2013) 2013(1). doi: 10.1155/2013/230176
85. Li Y-Q, Zheng Z, Liu Q-X, Lu X, Zhou D, Zhang J, et al. Repositioning of antiparasitic drugs for tumor treatment. Front Oncol. (2021) 11:670804. doi: 10.3389/fonc.2021.670804
86. Casaravilla C, Malgor R, Carmona C. Characterization of carbohydrates of adult echinococcus granulosus by lectin-binding analysis. J Parasitol. (2003) 89:57–61. doi: 10.1645/0022-3395(2003)089[0057:COCOAE]2.0.CO;2
87. Daneshpour S, Bahadoran M, Hejazi SH, Eskandarian AA, Mahmoudzadeh M, Darani HY. Common antigens between hydatid cyst and cancers. Advanced Biomed Res. (2016) 5:9. doi: 10.4103/2277-9175.175242
88. Herberman RB. Immunogenicity of tumor antigens. Biochim Biophys Acta (BBA) - Rev Cancer. (1977) 473:93–119. doi: 10.1016/0304-419X(77)90002-6
89. Wang S, Guo J, Bai Y, Sun C, Wu Y, Liu Z, et al. Bacterial outer membrane vesicles as a candidate tumor vaccine platform. Front Immunol. (2022) 13:987419. doi: 10.3389/fimmu.2022.987419
90. Firmanty P, Doligalska M, Krol M, Taciak B. Deciphering the dual role of heligmosomoides polygyrus antigens in macrophage modulation and breast cancer cell growth. Veterinary Sci. (2024) 11:69. doi: 10.3390/vetsci11020069
91. Qian Y-Y, Huang F-F, Chen S-Y, Zhang W-X, Wang Y, Du P-F, et al. Therapeutic effect of recombinant echinococcus granulosus antigen B subunit 2 protein on sepsis in a mouse model. Parasites Vectors. (2024) 17:467. doi: 10.1186/s13071-024-06540-x
92. Inclan-Rico JM, Napuri CM, Lin C, Hung L-Y, Ferguson AA, Liu X, et al. Mrgpra3 Neurons Drive Cutaneous Immunity against Helminths through Selective Control of Myeloid-Derived Il-33. Nat Immunol. (2024) 25:2068–84. doi: 10.1038/s41590-024-01982-y
93. Noya V, Bay S, Festari MF, Garcar EP, Rodriguez E, Chiale C, et al. Mucin-like peptides from echinococcus granulosus induce antitumor activity. Int J Oncol. (2013) 43:775–84. doi: 10.3892/ijo.2013.2000
94. Mani R, Martin CG, Balu KE, Wang Q, Rychahou P, Izumi T, et al. A novel protozoa parasite-derived protein adjuvant is effective in immunization with cancer cells to activate the cancer-specific protective immunity and inhibit the cancer growth in a murine model of colorectal cancer. Cells. (2024) 13:111. doi: 10.3390/cells13020111
95. Bahavar A, Moradi A, Mohammadi H, Norouzi M, Khodayar S, Mozhgani H, et al. Possible trace of htlv-1 virus in modulation of cbl-B, itch, and pp2a suppressor genes. Int J Mol Cell Med (IJMCM). (2024) 14:472–82. doi: 10.22088/IJMCM.BUMS.14.1.472
96. Al Zaki A, Sutton M, Rehman A, Le CC, Gammon S, Napoli A, et al. Imaging activated innate immune myeloid cells in immune effector cell-associated neurotoxicity syndrome (Icans) following car T-cell therapy. Blood. (2024) 144:3428. doi: 10.1182/blood-2024-211204
97. Van Tong H, Brindley PJ, Meyer CG, Velavan TP. Parasite infection, carcinogenesis and human Malignancy. EBioMedicine. (2017) 15:12–23. doi: 10.1016/j.ebiom.2016.11.034
98. von Bvonv V, Lichtenberger J, Grevelding CG, Falcone FH, Roeb E, Roderfeld M. Does schistosoma mansoni facilitate carcinogenesis? Cells. (2021) 10:1982. doi: 10.3390/cells10081982
99. Sharma P, Dandesena D, Suresh A, Budhwar R, Godwin J, Singh S, et al. Theileria annulata infection promotes P53 suppression, genomic instability and DNA deaminase apobec3h upregulation leading to cancer-like phenotype in host cells. bioRxiv. (2024). doi: 10.1101/2024.02.20.581323
100. Maizels RM. Toxocara canis: molecular basis of immune recognition and evasion. Veterinary Parasitol. (2013) 193:365–74. doi: 10.1016/j.vetpar.2012.12.032
101. Ruiz-Manzano RA, Palacios-Arreola MI, Hernández-Cervantes R, Del Río-Araiza VH, Nava-Castro KE, Ostoa-Saloma P, et al. Potential novel risk factor for breast cancer: toxocara canis infection increases tumor size due to modulation of the tumor immune microenvironment. Front Oncol. (2020) 10. doi: 10.3389/fonc.2020.00736
102. Kazemi F, Moradi-Sardareh H, Arjmand R, Tavalla M, Amari A, Cheraghian B. Toxocara canis increases the potential of breast cancer by reducing the expression of the P53 protein. Curr Mol Med. (2024) 24:335–43. doi: 10.2174/1566524023666230320103506
103. Herbort CP, Tuaillon N, Buggage RR, Egwuagu CE, Chan C-C. Detection of toxoplasma gondii DNA in primary intraocular B-cell lymphoma. Modern Pathol. (2001) 14:995–9. doi: 10.1038/modpathol.3880424
104. Turhan N, Esendagli G, Ozkayar O, Tunali G, Sokmensuer C, Abbasoglu O. Cobasoglurn9 of E chinococcus granulosus infection and cancer metastasis in the liver correlates with reduced th1 immune responses. Parasite Immunol. (2015) 37:16–22. doi: 10.1111/pim.2014.37.issue-1
105. Abdou AG, Harba NM, Afifi AF, Elnaidany NF. Assessment of cryptosporidium parvum infection in immunocompetent and immunocompromised mice and its role in triggering intestinal dysplasia. Int J Infect Dis. (2013) 17:e593–600. doi: 10.1016/j.ijid.2012.11.023
106. Benamrouz S, Conseil V, Chabe M, Praet M, Audebert C, Blervaque R, et al. Cryptosporidium parvum-induced ileo-caecal adenocarcinoma and wnt signaling in a mouse model. Dis Models Mech. (2014) 7:693–700. doi: 10.1242/dmm.013292
107. Sawant M, Baydoun M, Creusy C, Chabs M, Viscogliosi E, Certad G, et al. Cryptosporidium and colon cancer: cause or consequence? Microorganisms. (2020) 8:1665. doi: 10.3390/microorganisms8111665
108. Seo AN, Goo Y-K, Chung D-I, Hong Y, Kwon O, Bae H-I. Comorbid gastric adenocarcinoma and gastric and duodenal strongyloides stercoralis infection: A case report. Korean J Parasitol. (2015) 53:95. doi: 10.3347/kjp.2015.53.1.95
109. Bahrami S, Esmaeilzadeh S, Oryan A. Role of oxidative stress in concomitant occurrence of fasciola gigantica and leiomyoma in cattle. Veterinary Parasitol. (2014) 203:43–50. doi: 10.1016/j.vetpar.2014.02.047
110. Rainey JJ, Mwanda WO, Wairiumu P, Moormann AM, Wilson ML, Rochford R. Spatial distribution of burkittut lymphoma in Kenya and association with malaria risk. Trop Med Int Health. (2007) 12:936–43. doi: 10.1111/j.1365-3156.2007.01875.x
111. Medjkane S, Perichon M, Marsolier J, Dairou J, Weitzman J. Theileria induces oxidative stress and hif1s activation that are essential for host leukocyte transformation. Oncogene. (2014) 33:1809–17. doi: 10.1038/onc.2013.134
112. Cock-Rada AM, Medjkane S, Janski N, Yousfi N, Perichon M, Chaussepied M, et al. Smyd3 promotes cancer invasion by epigenetic upregulation of the metalloproteinase mmp-9. Cancer Res. (2012) 72:810–20. doi: 10.1158/0008-5472.CAN-11-1052
113. Twu O, Dessí D, Vu A, Mercer F, Stevens GC, De Miguel N, et al. Trichomonas vaginalis homolog of macrophage migration inhibitory factor induces prostate cell growth, invasiveness, and inflammatory responses. Proc Natl Acad Sci. (2014) 111:8179–84. doi: 10.1073/pnas.1321884111
114. Pastille E, Frede A, McSorley HJ, Gräb J, Adamczyk A, Kollenda S, et al. Intestinal helminth infection drives carcinogenesis in colitis-associated colon cancer. PloS Pathog. (2017) 13:e1006649. doi: 10.1371/journal.ppat.1006649
115. Hayes KS, Cliffe LJ, Bancroft AJ, Forman SP, Thompson S, Booth C, et al. Chronic trichuris muris infection causes neoplastic change in the intestine and exacerbates tumour formation in apc min/+ Mice. PloS Negl Trop Dis. (2017) 11:e0005708. doi: 10.1371/journal.pntd.0005708
116. Sulżyc-Bielicka V, Kołodziejczyk L, Adamska M, Skotarczak B, Jaczewska S, Safranow K, et al. Colorectal cancer and blastocystis sp. Infection. Parasites Vectors. (2021) 14:1–9. doi: 10.1186/s13071-021-04681-x
117. Kim T-S, Pak JH, Kim J-B, Bahk YY. Clonorchis sinensis, an oriental liver fluke, as a human biological agent of cholangiocarcinoma: A brief review. BMB Rep. (2016) 49:590. doi: 10.5483/BMBRep.2016.49.11.109
118. Liu T-C, Stappenbeck TS. Genetics and pathogenesis of inflammatory bowel disease. Annu Rev Pathology: Mech Dis. (2016) 11:127–48. doi: 10.1146/annurev-pathol-012615-044152
119. McGovern DP, Kugathasan S, Cho JH. Genetics of inflammatory bowel diseases. Gastroenterology. (2015) 149:1163–76.e2. doi: 10.1053/j.gastro.2015.08.001
120. Hunter MM, Wang A, Hirota CL, McKay DM. Neutralizing anti-il-10 antibody blocks the protective effect of tapeworm infection in a murine model of chemically induced colitis. J Immunol. (2005) 174:7368–75. doi: 10.4049/jimmunol.174.11.7368
121. Smith P, Mangan NE, Walsh CM, Fallon RE, McKenzie AN, van Rooijen N, et al. Infection with a helminth parasite prevents experimental colitis via a macrophage-mediated mechanism. J Immunol. (2007) 178:4557–66. doi: 10.4049/jimmunol.178.7.4557
122. Ince MN, Elliott DE, Setiawan T, Metwali A, Blum A, Hl C, et al. Role of T cell tgfla signaling in intestinal cytokine responses and helminthic immune modulation. Eur J Immunol. (2009) 39:1870–8. doi: 10.1002/eji.200838956
123. Braat H, Rottiers P, Hommes DW, Huyghebaert N, Remaut E, Remon JP, et al. A phase I trial with transgenic bacteria expressing interleukin-10 in crohnle disease. Clin Gastroenterol Hepatol. (2006) 4:754–9. doi: 10.1016/j.cgh.2006.03.028
124. Steidler L, Hans W, Schotte L, Neirynck S, Obermeier F, Falk W, et al. Treatment of murine colitis by lactococcus lactis secreting interleukin-10. Science. (2000) 289:1352–5. doi: 10.1126/science.289.5483.1352
125. Elliott DE, Setiawan T, Metwali A, Blum A, Urban JF Jr., Weinstock JV. Heligmosomoides polygyrus inhibits established colitis in illitisshedides mice. Eur J Immunol. (2004) 34:2690–8. doi: 10.1002/eji.200324833
126. Elliott DE, Li J, Blum A, Metwali A, Qadir K, Urban JF Jr., et al. Exposure to schistosome eggs protects mice from tnbs-induced colitis. Am J Physiology-Gastrointestinal Liver Physiol. (2003) 284:G385–G91. doi: 10.1152/ajpgi.00049.2002
127. Fantini M, Becker C, Tubbe I, Nikolaev A, Lehr HA, Galle P, et al. Transforming growth factor beta induced foxp3+ Regulatory T cells suppress th1 mediated experimental colitis. Gut. (2006) 55:671–80. doi: 10.1136/gut.2005.072801
128. Sedda S, Marafini I, Dinallo V, Di Fusco D, Monteleone G. The tgf-elSmad system in ibd pathogenesis. Inflammatory Bowel Dis. (2015) 21:2921–5. doi: 10.1097/MIB.0000000000000542
129. Li Y, Guan X, Liu W, Chen H-L, Truscott J, Beyatli S, et al. Helminth-induced production of tgf-u and suppression of graft-versus-host disease is dependent on il-4 production by host cells. J Immunol. (2018) 201:2910–22. doi: 10.4049/jimmunol.1700638
130. Sun X-M, Guo K, Hao C-Y, Zhan B, Huang J-J, Zhu X. Trichinella spiralis excretorylamunol.17 products stimulate host regulatory T cell differentiation through activating dendritic cells. Cells. (2019) 8:1404. doi: 10.3390/cells8111404
131. Walsh KP, Brady MT, Finlay CM, Boon L, Mills KHG. Infection with a helminth parasite attenuates autoimmunity through tgf-ughunityio suppression of th17 and th1 responses1. J Immunol. (2009) 183:1577–86. doi: 10.4049/jimmunol.0803803
132. Rennie C, Fernandez R, Donnelly S, McGrath KC. The impact of helminth infection on the incidence of metabolic syndrome: A systematic review and meta-analysis. Front Endocrinol (Lausanne). (2021) 12:728396. doi: 10.3389/fendo.2021.728396
133. Schmidt V, Hogan AE, Fallon PG, Schwartz C. Obesity-mediated immune modulation: one step forward,(Th) 2 steps back. Front Immunol. (2022) 13:932893. doi: 10.3389/fimmu.2022.932893
134. Morimoto M, Azuma N, Kadowaki H, Abe T, Suto Y. Regulation of type 2 diabetes by helminth-induced th2 immune response. J Veterinary Med Sci. (2016) 78:1855–64. doi: 10.1292/jvms.16-0183
135. Hams E, Bermingham R, Wurlod FA, Hogan AE, O'Shea D, Preston RJ, et al. The helminth T2 RNase ω1 promotes metabolic homeostasis in an IL-33- and group 2 innate lymphoid cell-dependent mechanism. FASEB J. (2016) 30(2):824–35. doi: 10.1096/fj.15-277822
136. Zande HJP, Gonzalez MA, de Ruiter K, Wilbers RH, García-Tardón N, van Huizen M, et al. The helminth glycoprotein omega-1 improves metabolic homeostasis in obese mice through type 2 immunity-independent inhibition of food intake. FASEB J. (2021) 35(2). doi: 10.1096/fj.202001973r
137. Su CW, Chen C-Y, Jiao L, Long SR, Mao T, Ji Q, et al. Helminth-induced and th2-dependent alterations of the gut microbiota attenuate obesity caused by high-fat diet. Cell Mol Gastroenterol Hepatol. (2020) 10:763–78. doi: 10.1016/j.jcmgh.2020.06.010
138. Wiria AE, Hamid F, Wammes LJ, Prasetyani MA, Dekkers OM, May L, et al. Infection with soil-transmitted helminths is associated with increased insulin sensitivity. PloS One. (2015) 10:e0127746. doi: 10.1371/journal.pone.0127746
139. Su C-W, Chen C-Y, Mao T, Chen N, Steudel N, Jiao L, et al. Maternal helminth infection protects offspring from high-fat-diet-induced obesity through altered microbiota and scfas. Cell Mol Immunol. (2023) 20:389–403. doi: 10.1038/s41423-023-00979-1
140. Duan Q, Xiong L, Liao C, Liu Z, Xiao Y, Huang R, et al. Population based and animal study on the effects of schistosoma japonicum infection in the regulation of host glucose homeostasis. Acta Tropica. (2018) 180:33–41. doi: 10.1016/j.actatropica.2018.01.002
141. Rinninella E, Raoul P, Cintoni M, Franceschi F, Miggiano GAD, Gasbarrini A, et al. What is the healthy gut microbiota composition? A changing ecosystem across age, environment, diet, and diseases. Microorganisms. (2019) 7:14. doi: 10.3390/microorganisms7010014
142. Beyhan YE, Yıldız MR. Microbiota and parasite relationship. Diagn Microbiol Infect Dis. (2023) 106:115954. doi: 10.1016/j.diagmicrobio.2023.115954
143. LlinnL-Caballero K, Caraballo L. Helminths and bacterial microbiota: the interactions of two of humansction friendsti. Int J Mol Sci. (2022) 23:13358. doi: 10.3390/ijms232113358
144. Khudhair Z, Alhallaf R, Eichenberger RM, Whan J, Kupz A, Field M, et al. Gastrointestinal helminth infection improves insulin sensitivity, decreases systemic inflammation, and alters the composition of gut microbiota in distinct mouse models of type 2 diabetes. Front Endocrinol. (2021) 11:606530. doi: 10.3389/fendo.2020.606530
145. Pintarič M, Langerholc T. Probiotic mechanisms affecting glucose homeostasis: A scoping review. Life. (2022) 12:1187. doi: 10.3390/life12081187
146. Shimokawa C, Obi S, Shibata M, Olia A, Imai T, Suzue K, et al. Suppression of obesity by an intestinal helminth through interactions with intestinal microbiota. Infect Immun. (2019) 87:10.1128/iai.00042-19. doi: 10.1128/iai.00042-19
147. Eissa MM, Ismail CA, El-Azzouni MZ, Ghazy AA, Hadi MA. Immuno-therapeutic potential of schistosoma mansoni and trichinella spiralis antigens in a murine model of colon cancer. Investigational New Drugs. (2019) 37:47–56. doi: 10.1007/s10637-018-0609-6
148. Bahadory S, Sadraei J, Zibaei M, Pirestani M, Dalimi A. In vitro anti-gastrointestinal cancer activity of toxocara canis-derived peptide: analyzing the expression level of factors related to cell proliferation and tumor growth. Front Pharmacol. (2022) 13. doi: 10.3389/fphar.2022.878724
149. Mendoza-Rodríguez MG, Medina-Reyes D, Sánchez-Barrera CA, Fernández-Muñoz KV, García-Castillo V, Ledesma-Torres JL, et al. Helminth-derived molecules improve 5-fluorouracil treatment on experimental colon tumorigenesis. Biomedicine Pharmacotherapy. (2024) 175:116628. doi: 10.1016/j.biopha.2024.116628
150. Eissa MM, Gaafar MR, Younis LK, Ismail CA, El Skhawy N. Prophylactic antineoplastic activity of toxoplasma gondii rh derived antigen against ehrlich solid carcinoma with evidence of shared antigens by comparative immunoblotting. Infect Agents Cancer. (2023) 18:21. doi: 10.1186/s13027-023-00500-3
151. Baird JR, Byrne KT, Lizotte PH, Toraya-Brown S, Scarlett UK, Alexander MP, et al. Immune-mediated regression of established B16f10 melanoma by intratumoral injection of attenuated toxoplasma gondii protects against rechallenge. J Immunol. (2013) 190:469–78. doi: 10.4049/jimmunol.1201209
152. Ismail CA, Eissa MM, Gaafar MR, Younis LK, El Skhawy N. Toxoplasma gondii-derived antigen modifies tumor microenvironment of ehrlich solid carcinoma murine model and enhances immunotherapeutic activity of cyclophosphamide. Med Oncol. (2023) 40:136. doi: 10.1007/s12032-023-01994-y
153. Hafez EN, Moawed FSM, Abdel-Hamid GR, Elbakary NM. Gamma radiation-attenuated toxoplasma gondii provokes apoptosis in ehrlich ascites carcinoma-bearing mice generating long-lasting immunity. Technol Cancer Res Treat. (2020) 19:1533033820926593. doi: 10.1177/1533033820926593
154. Sosoniuk-Roche E, Cruz P, Maldonado I, Duaso L, Pesce B, Michalak M, et al. In vitro treatment of a murine mammary adenocarcinoma cell line with recombinant trypanosoma cruzi calreticulin promotes immunogenicity and phagocytosis. Mol Immunol. (2020) 124:51–60. doi: 10.1016/j.molimm.2020.05.013
155. Atayde VD, Jasiulionis MG, Cortez M, Yoshida N. A recombinant protein based on trypanosoma cruzi surface molecule gp82 induces apoptotic cell death in melanoma cells. Melanoma Res. (2008) 18(3):172–83. doi: 10.1097/CMR.0b013e3282feeaab
156. Ding J, Liu X, Tang B, Bai X, Wang Y, Li S, et al. Trichinella spiralis esp inhibits tumor cell growth by regulating the immune response and inducing apoptosis. Acta Tropica. (2021) 225:106172. doi: 10.21203/rs.3.rs-257172/v1
157. Ding J, Tang B, Liu X, Bai X, Wang Y, Li S, et al. Excretory-secretory product of trichinella spiralis inhibits tumor cell growth by regulating the immune response and inducing apoptosis. Acta Tropica. (2022) 225:106172. doi: 10.1016/j.actatropica.2021.106172
158. Bolhassani A, Safaiyan S, Rafati S. Improvement of different vaccine delivery systems for cancer therapy. Mol Cancer. (2011) 10:1–20. doi: 10.1186/1476-4598-10-3
159. Saylor K, Gillam F, Lohneis T, Zhang C. Designs of antigen structure and composition for improved protein-based vaccine efficacy. Front Immunol. (2020) 11:283. doi: 10.3389/fimmu.2020.00283
160. Thuru X, Magnez R, El-Bouazzati H, Vergoten G, Quesnel B, Bailly C. Drug repurposing to enhance antitumor response to pd-1/pd-L1 immune checkpoint inhibitors. Cancers. (2022) 14:3368. doi: 10.3390/cancers14143368
161. Crinier A, Vivier E, Blery M. Helper-like innate lymphoid cells and cancer immunotherapy. Semin Immunol. (2019) 41:101–274. doi: 10.1016/j.smim.2019.04.002
162. Wei G, Wang Y, Yang G, Wang Y, Ju R. Recent progress in nanomedicine for enhanced cancer chemotherapy. Theranostics. (2021) 11:6370. doi: 10.7150/thno.57828
163. Quansah E, Chen Y, Yang S, Wang J, Sun D, Zhao Y, et al. Crispr-cas13 in malaria parasite: diagnosis and prospective gene function identification. Front Microbiol. (2023) 14:1076947. doi: 10.3389/fmicb.2023.1076947
164. Lalawmpuii K, Lalrinkima H. Genetic manipulations in helminth parasites. J Parasit Dis. (2023) 47:203–14. doi: 10.1007/s12639-023-01567-w
165. Gupta D, Bhattacharjee O, Mandal D, Sen MK, Dey D, Dasgupta A, et al. Crispr-cas9 system: A new-fangled dawn in gene editing. Life Sci. (2019) 232:116636. doi: 10.1016/j.lfs.2019.116636
166. Pal S, Dam S. Crispr-cas9: taming protozoan parasites with bacterial scissor. J Parasit Dis. (2022) 46:1204–12. doi: 10.1007/s12639-022-01534-x
167. Ambros V, Horvitz HR. The lin-14 locus of caenorhabditis elegans controls the time of expression of specific postembryonic developmental events. Genes Dev. (1987) 1:398–414. doi: 10.1101/gad.1.4.398
168. Duffy J, Padovani F, Brunetti G, Noy P, Certa U, Hegner M. Towards personalised rapid label free mirna detection for cancer and liver injury diagnostics in cell lysates and blood based samples. Nanoscale. (2018) 10:12797–804. doi: 10.1039/C8NR03604G
169. Kutwin P, Konecki T, Borkowska EM, Traczyk-Borszyńska M, Jabłonowski Z. Urine mirna as a potential biomarker for bladder cancer detectionor meta-analysis. Cent Eur J Urol. (2018) 71:177. doi: 10.5173/ceju.2018.1605
170. Jiang M, Li X, Quan X, Li X, Zhou B. Clinically correlated micrornas in the diagnosis of non-small cell lung cancer: A systematic review and meta-analysis. BioMed Res Int. (2018) 2018:1–14. doi: 10.1155/2018/5930951
171. Svoronos AA, Engelman DM, Slack FJ. Oncomir or tumor suppressor? The duplicity of micrornas in cancer. Cancer Res. (2016) 76:3666–70. doi: 10.1158/0008-5472.CAN-16-0359
172. Si M, Zhu S, Wu H, Lu Z, Wu F, Mo Y. Mir-21-mediated tumor growth. Oncogene. (2007) 26:2799–803. doi: 10.1038/sj.onc.1210083
173. Toiyama Y, Takahashi M, Hur K, Nagasaka T, Tanaka K, Inoue Y, et al. Serum mir-21 as a diagnostic and prognostic biomarker in colorectal cancer. J Natl Cancer institute. (2013) 105:849–59. doi: 10.1093/jnci/djt101
174. Buck AH, Coakley G, Simbari F, McSorley HJ, Quintana JF, Le Bihan T, et al. Exosomes secreted by nematode parasites transfer small rnas to mammalian cells and modulate innate immunity. Nat Commun. (2014) 5:5488. doi: 10.1038/ncomms6488
175. Britton C, Winter AD, Gillan V, Devaney E. Micrornas of parasitic helminthstonns…2-01534- characterization and potential as drug targets. Int J Parasitology: Drugs Drug Resistance. (2014) 4:85–94. doi: 10.1016/j.ijpddr.2014.03.001
176. Tran N, Ricafrente A, To J, Lund M, Marques TM, Gama-Carvalho M, et al. Fasciola hepatica hijacks host macrophage mirna machinery to modulate early innate immune responses. Sci Rep. (2021) 11:6712. doi: 10.1038/s41598-021-86125-1
177. Tkach M, Thach C. Communication by extracellular vesicles: where we are and where we need to go. Cell. (2016) 164:1226–32. doi: 10.1016/j.cell.2016.01.043
178. Dagenais M, Gerlach JQ, Wendt GR, Collins JJ III, Atkinson LE, Mousley A, et al. Analysis of schistosoma mansoni extracellular vesicles surface glycans reveals potential immune evasion mechanism and new insights on their origins of biogenesis. Pathogens. (2021) 10:1401. doi: 10.3390/pathogens10111401
179. Chowdhury S, Sais D, Donnelly S, Tran N. The knowns and unknowns of helminthryhur mirna cross-kingdom communication. Trends Parasitol. (2023) 40(2):176–91. doi: 10.1016/j.pt.2023.12.003
180. Gannavaram S, Bhattacharya P, Siddiqui A, Ismail N, Madhavan S, Nakhasi HL. Mir-21 expression determines the early vaccine immunity induced by ldcen–/– immunization. Front Immunol. (2019) 10:481605. doi: 10.3389/fimmu.2019.02273
181. Mubarok W, Nakahata M, Kojima M, Sakai S. Nematode surface functionalization with hydrogel sheaths tailored in situ. Materials Today Bio. (2022) 15:100328. doi: 10.1016/j.mtbio.2022.100328
182. Weinstock JV, Elliott DE. Helminths and the ibd hygiene hypothesis. Inflammatory bowel Dis. (2009) 15:128–33. doi: 10.1002/ibd.20633
183. Cheng AM, Jaint D, Thomas S, Wilson J, Parker W. Overcoming evolutionary mismatch by self-treatment with helminths: current practices and experience. J Evol Med. (2015) 3:1–22. doi: 10.4303/jem/235910
184. JiméJim B, Maya C, Velasquez G, Barrios JA, Perez M, Romri A. Helminth egg automatic detector (Head): improvements in development for digital identification and quantification of helminth eggs and its application online. MethodsX. (2020) 7:101158. doi: 10.1016/j.mex.2020.101158
185. Zendejas-Heredia PA, Colella V, Hii SF, Traub RJ. Comparison of the egg recovery rates and limit of detection for soil-transmitted helminths using the kato-katz thick smear, faecal flotation and quantitative real-time pcr in human stool. PloS Negl Trop Dis. (2021) 15:e0009395. doi: 10.1371/journal.pntd.0009395
186. Lobos-Ovalle D, Navarrete C, Navedo JG, Peña-Espinoza M, Verdugo C. Improving the sensitivity of gastrointestinal helminth detection using the mini-flotac technique in wild birds. Parasitol Res. (2021) 120:3319–24. doi: 10.1007/s00436-021-07267-9
187. Dunn JC, Papaiakovou M, Han KT, Chooneea D, Bettis AA, Wyine NY, et al. The increased sensitivity of qpcr in comparison to kato-katz is required for the accurate assessment of the prevalence of soil-transmitted helminth infection in settings that have received multiple rounds of mass drug administration. Parasit Vectors. (2020) 13:324. doi: 10.1186/s13071-020-04197-w
188. Bohnacker S, Troisi F, de los Reyes Jimesik M, Esser-von Bieren J. What can parasites tell us about the pathogenesis and treatment of asthma and allergic diseases. Front Immunol. (2020) 11:2106. doi: 10.3389/fimmu.2020.02106
189. Sipahi AM, Baptista DM. Helminths as an alternative therapy for intestinal diseases. World J Gastroenterol. (2017) 23:6009. doi: 10.3748/wjg.v23.i33.6009
190. Alcantara-Neves NM, Veiga RV, Dattoli VCC, Fiaccone RL, Esquivel R, Cruz ÁA, et al. The effect of single and multiple infections on atopy and wheezing in children. J Allergy Clin Immunol. (2012) 129:359–67. doi: 10.1016/j.jaci.2011.09.015
191. Cooper PJ, Chico ME, Vaca MG, Moncayo A-L, Bland JM, Mafla E, et al. Effect of albendazole treatments on the prevalence of atopy in children living in communities endemic for geohelminth parasites: A cluster-randomised trial. Lancet. (2006) 367:1598–603. doi: 10.1016/S0140-6736(06)68697-2
192. Bager P, Arnved J, Rønborg S, Wohlfahrt J, Poulsen LK, Westergaard T, et al. Trichuris suis ova therapy for allergic rhinitis: A randomized, double-blind, placebo-controlled clinical trial. J Allergy Clin Immunol. (2010) 125:123–30. doi: 10.1016/j.jaci.2009.08.006
193. Feary J, Venn A, Brown A, Hooi D, Falcone FH, Mortimer K, et al. Safety of hookworm infection in individuals with measurable airway responsiveness: A randomized placebozedness:led feasibility study. Clin Exp Allergy. (2009) 39:1060–8. doi: 10.1111/j.1365-2222.2009.03187.x
194. Feary J, Venn A, Mortimer K, Brown A, Hooi D, Falcone F, et al. Experimental hookworm infection: A randomized placebozedal.s:led trial in asthma. Clin Exp Allergy. (2010) 40:299–306. doi: 10.1111/j.1365-2222.2009.03433.x
195. Elliott DE, Weinstock JV. Helminthic therapy: using worms to treat immune-mediated disease. Pathogen-Derived Immunomodulatory Molecules. (2009) 666:157–66. doi: 10.1007/978-1-4419-1601-3_12
196. Heylen M, Ruyssers NE, De Man JG, Timmermans J-P, Pelckmans PA, Moreels TG, et al. Worm proteins of schistosoma mansoni reduce the severity of experimental chronic colitis in mice by suppressing colonic proinflammatory immune responses. PloS One. (2014) 9:e110002. doi: 10.1371/journal.pone.0110002
197. Maruszewska-Cheruiyot M, Donskow-Łysoniewska K, Doligalska M. Helminth therapy: advances in the use of parasitic worms against inflammatory bowel diseases and its challenges. Helminthologia. (2018) 55:1–11. doi: 10.1515/helm-2017-0048
198. Sutherland I, Moen I, Leathwick D. Increased burdens of drug-resistant nematodes due to anthelmintic treatment. Parasitology. (2002) 125:375–81. doi: 10.1017/S0031182002002184
199. Parker W. Not infection with parasitic worms, but rather colonization with therapeutic helminths. Immunol Lett. (2017) 192:104–5. doi: 10.1016/j.imlet.2017.07.008
200. Coghlan A, Partridge FA, Duque-Correa MA, Rinaldi G, Clare S, Seymour L, et al. A drug repurposing screen for whipworms informed by comparative genomics. PloS Negl Trop Dis. (2023) 17:e0011205. doi: 10.1371/journal.pntd.0011205
201. Bizhani N. Herbal therapy and treatment of worm infections, emphasizing taenia solium. Iran J Public Health. (2015) 44:1555–6.
202. Finkelman FD. Worming their way into the pharmacy: use of worms and worm products to treat inflammatory diseases. Arthritis Rheum. (2012) 64:3068–71. doi: 10.1002/art.34635
Keywords: colorectal cancer, precursors of colorectal cancer, helminth therapy, helminth-derived products, immune modulation, combination therapy
Citation: Li H, Shan C, Zhu Y, Yao X, Lin L, Zhang X, Qian Y, Wang Y, Xu J, Zhang Y, Li H, Zhao L and Chen K (2025) Helminth-induced immune modulation in colorectal cancer: exploring therapeutic applications. Front. Immunol. 16:1484686. doi: 10.3389/fimmu.2025.1484686
Received: 22 August 2024; Accepted: 24 March 2025;
Published: 14 April 2025.
Edited by:
Jason Paul Gigley, University of Wyoming, United StatesReviewed by:
Namrata Anand, University of Chicago Medical Center, United StatesCopyright © 2025 Li, Shan, Zhu, Yao, Lin, Zhang, Qian, Wang, Xu, Zhang, Li, Zhao and Chen. This is an open-access article distributed under the terms of the Creative Commons Attribution License (CC BY). The use, distribution or reproduction in other forums is permitted, provided the original author(s) and the copyright owner(s) are credited and that the original publication in this journal is cited, in accordance with accepted academic practice. No use, distribution or reproduction is permitted which does not comply with these terms.
*Correspondence: Hongyu Li, aG9uZ3l1ODg5MjZAempzcnUuZWR1LmNu; Keda Chen, Y2hlbmtkQHpqc3J1LmVkdS5jbg==
†These authors have contributed equally to this work and share first authorship
Disclaimer: All claims expressed in this article are solely those of the authors and do not necessarily represent those of their affiliated organizations, or those of the publisher, the editors and the reviewers. Any product that may be evaluated in this article or claim that may be made by its manufacturer is not guaranteed or endorsed by the publisher.
Research integrity at Frontiers
Learn more about the work of our research integrity team to safeguard the quality of each article we publish.