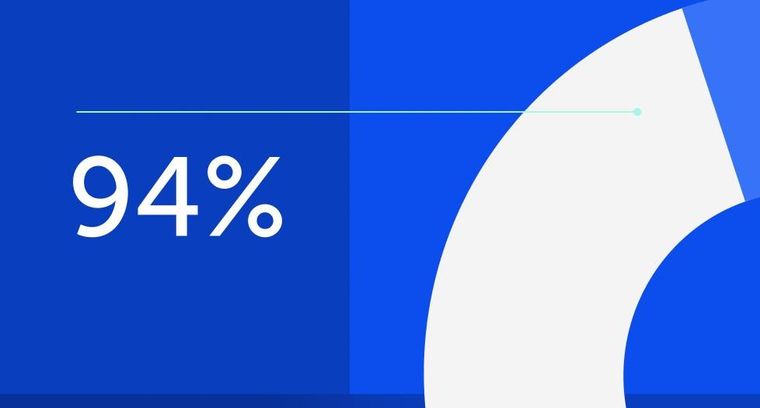
94% of researchers rate our articles as excellent or good
Learn more about the work of our research integrity team to safeguard the quality of each article we publish.
Find out more
REVIEW article
Front. Immunol., 08 April 2025
Sec. Inflammation
Volume 16 - 2025 | https://doi.org/10.3389/fimmu.2025.1480534
This article is part of the Research TopicThe Role of Inflammation in Organ InjuryView all 14 articles
Diabetic neuropathic pain (DNP) is one of the most prevalent complications of diabetes, characterized by a high global prevalence and a substantial affected population with limited effective therapeutic options. Although DNP is closely associated with hyperglycemia, an increasing body of research suggests that elevated blood glucose levels are not the sole inducers of DNP. The pathogenesis of DNP is intricate, involving the release of inflammatory mediators, alterations in synaptic plasticity, demyelination of nerve fibers, and ectopic impulse generation, yet the precise mechanisms remain to be elucidated. The spinal dorsal horn coordinates dynamic interactions between peripheral and central pain pathways, wherein dorsal horn neurons, microglia, and astrocytes synergize with Schwann cell-derived signals to process nociceptive information flow. Abnormally activated neurons can alter signal transduction by modifying the local microenvironment, compromising myelin integrity, and diminishing trophic support, leading to neuronal sensitization and an amplifying effect on peripheral pain signals, which in turn triggers neuropathic pain. Ion channels play a pivotal role in signal conduction, with the modulation of sodium, potassium, and calcium channels being particularly crucial for the regulation of pain signals. In light of the rising incidence of diabetes and the current scarcity of effective DNP treatments, a thorough investigation into the interactions between neurons and glial cells, especially the mechanisms of ion channel function in DNP, is imperative for identifying potential drug targets, developing novel therapeutic strategies, and thereby enhancing the prospects for DNP management.
The global rise in the number of diabetes patients has propelled the study of diabetes-related complications to the forefront of medical research. As the third leading cause of mortality worldwide (1), following cancer and cardiovascular diseases, diabetes presents a significant threat to global public health. In 2021, diabetes was diagnosed in 10.5% of the world’s population, with an anticipated increase to a 12.2% prevalence rate by mid-century, affecting an estimated 780 million individuals (2). China, notably, is home to over 110 million adults with diabetes, constituting 24% of the global diabetic population and ranking as the country with the highest number of diabetic patients (3). However, a considerable proportion, approximately one-third to one-half of patients, remain unaware of their condition (4), often receiving a diagnosis only upon the emergence of severe complications or during routine health check-ups. This scenario poses a substantial challenge to the early detection and management of diabetes. Moreover, it amplifies the healthcare burden, particularly in low- and middle-income nations where investment in diabetes care is critically lacking (5).
Diabetes imposes numerous challenges on daily living, with inadequate blood sugar management leading to detrimental effects on various bodily systems, including the heart, blood vessels, nerves, kidneys, and eyes, potentially resulting in irreversible damage (6–10). Conditions such as diabetic retinopathy, diabetic nephropathy, diabetic cardiomyopathy, and diabetic neuropathic pain exemplify the disease’s role as the fourth leading cause of disability globally, following cancer, cardiovascular diseases, and trauma (1). Notably, diabetic neuropathic pain stands out as a prevalent complication, with nerve damage often manifesting early in the disease’s progression. Consequently, the chronic pain associated with nerve injury significantly diminishes patients’ quality of life. The etiology of neuropathic pain remains elusive, encompassing factors like inflammation, microvascular disease, metabolic stress, and neurotransmitter imbalances (11–14), with many theories yet to achieve scholarly consensus (Figure 1). Notably, the dysregulation of ion channel homeostasis in neuronal cells may serve as a critical molecular hub underlying aberrant nociceptive signal transmission.
Figure 1. Pathogenic mechanisms of diabetic neuropathic pain. Diabetic neuropathic pain involves complex and multifactorial pathogenic mechanisms, including hyperglycemia, neuroglial activation, synaptic plasticity abnormalities, microvascular dysfunction, neurotrophic deficiency, oxidative stress, ion channel dysregulation, and inflammatory pathways, among others.
Spinal dorsal horn is a pivotal center for the relay of peripheral pain signals (15, 16), densely populated with neurons and glial cells that are essential for the propagation of electrical impulses (17). Upon abnormal activation of these cells, Upon abnormal activation of these cells, substantial release of inflammatory mediators and chemokines initiates a pathological cascade. This coordinated dysregulation not only exacerbates oxidative stress but consequently propagates neural conduction disorders, amplifies aberrant neuronal firing, and potentiates maladaptive synaptic plasticity through feedforward mechanisms (18–20). In clinical practice, strategies for managing diabetic neuropathic pain (DNP) commonly include glycemic control (21), neurotrophic support (22), and anti-inflammatory interventions (23). Although these treatments may provide relief in the early stages of DNP, pain and discomfort often persist even when blood glucose levels are well-managed in the later stages. This persistent pain can erode patients’ adherence to treatment and potentially lead to co-morbid psychiatric conditions (24). This article reviews the roles of neurons and supporting cells, such as astrocytes, microglia, and Schwann cells, in DNP. It delves into the cellular mechanisms by which these cells mediate neuropathic pain and examines how alterations in ion channels influence the progression of DNP. The aim is to offer novel perspectives for the mechanistic understanding and therapeutic development of DNP.
The spinal dorsal horn, situated within the posterior gray matter of the spinal cord, serves as a pivotal relay station for the processing of sensory information from the limbs, with a particular focus on pain. This region is replete with diverse neuronal and glial cell types, integral to the integration and modulation of pain signals (Figure 2). The locus coeruleus-norepinephrine system (LC: NE) acts as a significant feedback mechanism, inhibiting the ascending transmission of pain within the spinal cord. Research has demonstrated that dampening the LC: NE pathway can mitigate inflammatory responses and alleviate pain by curbing the activity of astrocytes and microglia (25). In a mouse model of spontaneous encephalomyelitis, Ding et al. (26) observed early astrocyte activation. The anion channel LRRC8A on astrocytes, when activated, facilitates glutamate release, which is intimately linked to neuropathic pain (NP). Recent studies have reaffirmed that the activation of the JAK2/STAT3 signaling pathway drives the conversion of astrocytes and microglia into pro-inflammatory phenotypes, and targeting the upstream regulator IL-6 of this pathway can markedly attenuate DNP (27). The GABAB receptor, abundant in the spinal dorsal horn, exerts a crucial regulatory effect on synaptic transmission and inflammatory processes (28). Stimulation of GABAB receptors can ameliorate DNP by suppressing the TLR4/Myd88/NF-κB signaling cascade (29). While neuronal activation within the spinal dorsal horn can initiate pain via various mechanisms, the temporal dynamics, magnitude, and precise functions of these neurons in DNP exhibit variability. Moreover, the interplay between different cell types amplifies the intricacy of developing targeted therapeutic approaches (Table 1).
Figure 2. Spinal dorsal horn and spinal nerve neuronal cells in mediating diabetic neuropathic pain. Microglia, astrocytes, and Schwann cells synergistically mediate neuroinflammatory signaling and neuronal hyperexcitability in diabetic neuropathy. Activated microglia release pro-inflammatory cytokines (e.g., TNF-α, IL-1β), amplifying nociceptive transmission. Astrocytes sustain neuroinflammation by propagating cytokine cascades and disrupting glutamate homeostasis, leading to central sensitization. Schwann cells, through peripheral nerve damage, secrete nerve growth factor (NGF), which sensitizes nociceptive neurons and enhances pain signaling to the spinal cord. Neuronal circuits in the spinal dorsal horn integrate these inputs, with neurotrophic factors further promoting synaptic plasticity and chronic pain.
Microglia, as the primary immune cells of the central nervous system (CNS), are pivotal in maintaining CNS homeostasis, facilitating post-injury repair, modulating immune responses, and participating in neuroinflammatory processes (52, 53). Emerging evidence highlights the pivotal role of microglial activation in mediating pathophysiological processes underlying diabetes mellitus and its complications. Under diabetic conditions, elevated glucose levels are known to drive microglial polarization from the anti-inflammatory M2 phenotype to the pro-inflammatory M1 phenotype, thereby augmenting systemic inflammatory responses, and Levetiracetam was utilized to suppress microglial activation, which consequently reduced the secretion of pro-inflammatory cytokines such as TNF-α, IL-1β, and IL-6, and ameliorated cognitive deficits in a murine model (54). Similar observations regarding microglial activation and functional alterations have been reported in diabetic retinopathy (55). Microglia’s role in pain processing has been well-established in pain management and pharmacological research (56). However, the specific activation status of microglia in a hyperglycemia peripheral nerve environment and their subsequent pain-mediating actions remain elusive. Hyperglycemia not only triggers microglial activation but also induces dynamic morphological remodeling, as evidenced by enhanced Iba1 immunoreactivity observed in the medial region of the spinal dorsal horn, exhibiting characteristic cellular hypertrophy (57). Furthermore, heightened phosphorylation of the N-methyl-D-aspartate receptor (NMDAR) NR1 subunit (pNR1) on microglia has been linked to the development of mechanical hyperalgesia in diabetic mice (58).
DNP predominantly arising from peripheral nerve damage, is also significantly modulated by the central nervous system’s regulatory dysfunction. This central dysregulation in turn induces abnormalities in peripheral sensory neurons (59). Microglia are instrumental in the etiology of DNP and engage in immune regulation, inflammatory responses, neurotransmitter imbalances, and receptor activation, such as the inhibition of P2X4 and Axl receptors (30, 60). These processes can precipitate abnormal neuronal firing and the phagocytosis of myelin debris, as well as the release of insulin-like growth factors (61, 62). Even in the early stages of diabetes, microglia are activated by elevated blood glucose levels, leading to a notable proliferation within the spinal cord’s gray and white matter. Activated microglia exhibit ultrastructural changes, including an abundance of endoplasmic reticulum and lysosomal fragments and an increase in nuclear-associated actin filaments. Functionally, they demonstrate heightened phagocytic activity, evident by the presence of myelin and axonal debris within their cytoplasm (63). Bullatine A demonstrates consistent analgesic efficacy across preclinical neuropathic pain models, encompassing spinal nerve ligation, cancer-induced pain, and diabetic neuropathic pain (DNP). This pharmacological action is primarily mediated through microglial activation within the superficial dorsal horn laminae, triggering subsequent dynorphin A release. Notably, Bullatine A’s analgesic effects are abolished by κ-opioid receptor antagonism or dynorphin A neutralization (37, 64). Sun et al. (65) have validated that C18-diterpene alkaloids primarily target microglia over neurons and astrocytes to mitigate pain hypersensitivity. Morevermicroglia-derived C-class chemokine XCL1 plays a pathogenic role in the neuroimmune pathogenesis of diabetic neuropathic pain (DNP) (40).
Astrocyte activation within the spinal cord is a pivotal factor in the development of diabetic neuropathic pain (DNP). Recent findings through single-cell sequencing and spatial transcriptomics have revealed co-localization of C4b with astrocytes and GABAergic neurons in mice subjected to a high-fat diet (66). It has been established that astrocyte activity is markedly diminished in hyperglycemia conditions (67, 68). Ge et al. (47) has demonstrated that dihydromyricetin mitigates the deleterious effects of hyperglycemia on astrocytes and decreases the expression of inflammatory cytokines such as TNF-α and IL-1β by targeting the purinergic receptor P2X7. Astrocyte activation not only elevates inflammatory cytokine levels but also has a direct link to neuropathic pain, as evidenced by the phosphorylation of c-Jun N-terminal kinase (pJNK) in astrocyte (46).
The identity of the cell types in the spinal cord dorsal horn and peripheral nerves that mediate DNP remains a contentious issue across various studies. Liao et al. (69) discovered that the administration of l-α-aminoadipate, an astrocyte-specific inhibitor, significantly reduced mechanical allodynia in type 2 diabetic mice, whereas minocycline, a microglia-specific inhibitor, did not alleviate this symptom. This finding underscores the importance of astrocyte activation in the spinal cord in the pathogenesis of DNP. Activated astrocytes are posited to propagate pain signals by increasing IL-1β levels and NMDA receptor phosphorylation in dorsal horn neurons (70). In contrast to research on spinal glial cells, some researchers propose that alterations in neurotransmitter and receptor expression within the ventrolateral periaqueductal gray (vlPAG) are crucial for endogenous pain modulation (71, 72). Studies have indicated that astrocyte activation in the vlPAG is closely associated with the mechanical withdrawal threshold (MWT) in DNP rats, and treatment with fluorocitrate (FC), a selective astrocyte activation inhibitor, or neurotropin analgesics can ameliorate MWT (41). Additionally, Lan et al. confirmed that vlPAG astrocytes regulate diabetes-associated neuropathic pain and related anxiety-like behaviors (45). The most recent research (42) employs chemogenetic methods to show that the Designer Receptors Exclusively Activated by Designer Drugs (DREADD) can bidirectionally regulate astrocyte activation in the basolateral amygdala (BLA), with activation alleviating and inhibition exacerbating mechanical allodynia in rats.
Schwann cells, the principal glial cells of the peripheral nervous system, perform critical functions such as providing trophic support and nutrition to neurons, ensheathing them to form myelin, thereby enhancing nerve impulse propagation, fostering axonal regeneration, and engaging in immune modulation (73–75). In the clinical context of DNP, initial structural and functional impairments encompass axonal defects termed axonopathy and pathological changes within Schwann cells, referred to as Schwann cell disease. These cells are pivotal in the pathogenesis of DNP, underscoring their essential role in peripheral nerve support. In 2013, Zenker (76) put forth a notable hypothesis regarding the neurochemical mechanisms underlying DNP, positing that Schwann cells, along with dorsal root ganglion neurons and spinal oligodendrocytes, are integral mediators of neuropathic pain.
Peripheral nerve damage is a fundamental pathological underpinning of dDNP (77). Prolonged hyperglycemia in diabetes engenders oxidative stress and amplifies inflammatory responses, precipitating aberrant peripheral nerve conduction and pain sensation (78). Hyperglycemia impedes Schwann cell secretion of nerve growth factor (NGF) and neurotrophin 3 (NT3), curtailing the neurotrophic support essential for axonal health and impeding regeneration (79). Zheng et al. (80) have validated that hyperglycemia-induced Schwann cell pyroptosis potentially contributes significantly to neuropathy, and modulation of systemic inflammatory factors can avert this pyroptosis, safeguarding neuronal integrity. Recent studies have indicated that bupropion, an antidepressant medication, is associated with a diminished prevalence of neuropathy among diabetics; experimental models in mice have corroborated that bupropion mitigates Schwann cell mortality and upholds myelin integrity (81). Consequently, maintaining the integrity of peripheral nerves is of utmost importance in alleviating and preventing DNP. This involves focusing on reducing abnormal nerve signal conduction, which is crucial for minimizing the development and progression of the condition.
The dorsal root ganglion (DRG) serves as the sensory nexus for spinal nerves, harboring pseudo-unipolar neurons that are pivotal for conveying sensory information from the periphery to the central nervous system (82). Pathophysiological alterations within the DRG encompass neuronal hyperactivity, neurotransmitter dysregulation, and escalated pain signal conduction—factors intimately associated with hyperalgesia and chronic pain conditions (83). Research indicates that DRG neurons in diabetic patients are frequently subjected to metabolic stress, rendering them more vulnerable to the detrimental impacts of hyperglycemia (84). Notably, the large, myelinated A-fiber neurons within the DRG, particularly Aβ fibers, are instrumental in pain signal transmission (85, 86). Elevated plasma osmotic pressure in diabetic patients, a consequence of hyperglycemia, can result in diminished peak amplitude of the ascending compound action potential (CAP) in A fibers. This heightened osmotic pressure can precipitate paresthesia and chronic pain by disrupting signal transduction within A fibers. Xu et al. (87) have discovered that toll-like receptor 5 (TLR5) co-localizes with A-fiber neurons in the DRG, and local anesthetic agents can impede TLR5-mediated sodium currents. This inhibition is achieved by activating TLR5 and its ligand, flagellin, thus mitigating diabetic neuropathy. Current research further validates the significant role of Aβ low-threshold mechanoreceptors (Aβ-LTMRs) in mechanical hyperalgesia, with varying degrees of receptor activation and deactivation dictating the facilitation or suppression of mechanical hyperalgesia (88).
The transient receptor potential vanilloid type 1 (TRPV1) is an ion channel belonging to the transient receptor potential (TRP) superfamily of cation channels (89). It is notably expressed in the DRG, where its blockade can effectively reduce the transmission of pain signals. TRPV1 also exerts regulatory roles in the central nervous system, implicating it in a spectrum of diseases including neurodegeneration, obesity, and diabetes (90–92). Notably, it is particularly abundant in the spinal cord’s dorsal horn, where it modulates peripheral pain signal transmission. An abundance of research has documented elevated expression levels of TRPM8 (transient receptor potential melastatin 8) in the spinal cord and DRG of neuropathic pain animal models, positing TRPM8 as a promising therapeutic target (93, 94). Adnan Khan et al. reported a significant upregulation of spinal TRPV1/TRPM8 proteins in a streptozotocin-induced diabetic model. Treatment with Aju-I markedly attenuated the expression of these proteins in the spinal cord of mice. These findings align with previous studies, suggesting that the pharmacological targeting of TRPV1/TRPM8 could mitigate hyperalgesia in models of diabetic neuropathy (95–97).
Research has confirmed that the activation of transient receptor potential vanilloid 1 (TRPV1) on A-fiber neurons plays a critical role in the mechanism of pain amplification (98). Recent investigations have identified that GPR177 is predominantly expressed in large-caliber A-fiber DRG neurons (99). It has been elucidated that GPR177 facilitates the secretion of WNT5a into the cerebrospinal fluid (CSF) by A-fiber DRG neurons, a process imperative for sustaining DNP. In vitro experimentation has corroborated that the extracellular application of WNT5a elicits rapid currents in both heterologous cells that express TRPV1 and in nociceptive DRG neurons (99). Administration of Aju-I has been shown to significantly diminish the expression levels of TRPV1 and TRPM8. Furthermore, Aju-I augments antioxidant capacity and curbs the secretion of inflammatory cytokines. Collectively, this research delineates that Aju-I mitigates pain behaviors in the streptozotocin (STZ)-induced diabetic neuropathy model by modulating the Nrf2/Keap-1/HO-1 signaling pathway and the activity of TRPV1/TRPM8 nociceptors (100).
Sodium channels are a primary therapeutic target for the development of novel analgesics aimed at neuropathic pain management (101). Nerve injury can modulate the expression of sodium channels, which may lead to heightened peripheral nerve excitability and the generation of ectopic discharges at the nerve, dorsal root ganglion, or site of injury (102). These channels consist of an α subunit that forms the pore, associated with one or two β subunits of a regulatory nature. The α subunits are encoded by nine genes, namely Nav1.1 through Nav1.9 (103, 104). Sodium channel inhibitors, such as amitriptyline and mexiletine, among other anticonvulsant medications, have clinically demonstrated efficacy in alleviating neuropathic pain by promoting the stabilization of the inactivated state of sodium channels (105).
Differential alterations in sodium channels within small nociceptive C-fiber DRG neurons are implicated in the pathogenesis of diabetic neuropathy (106, 107). Research has established that early-stage diabetic conditions are characterized by modifications in nanochannels of large DRG neurons and myelinated A-fibers (108). In diabetic mice, the expression of Na(v)1.2, Na(v)1.3, Na(v)1.7, and Na(v)1.9 in DRG neurons was increased, accompanied by a significant rise in sodium currents and the mean peak current density of the slow ramp current. Conversely, at the sodium channel-rich nodes of Ranvier, the signal intensity of Na(v)1.6 was reduced, and the quantity of Na(v)1.8 was significantly decreased. Therefore, the differential expression of sodium channels in large DRG neurons and A-fibers may be a key factor mediating diabetic neuropathic changes (108). Additionally, alterations in sodium channel proteins in medium or small DRG neurons can also contribute to abnormal sensory conduction in the early stages of diabetes (109). Another study (110) found that in diabetic rats, the expression of tetrodotoxin-sensitive (TTX-S) sodium channels Na(v)1.3 and Na(v)1.7 was significantly increased, while the expression of Na(v)1.6 (TTX-S) and Na(v)1.8 (tetrodotoxin-resistant, TTX-R) was decreased. Phosphorylation of sodium channels can enhance sodium current levels (111). Consequently, the study further revealed that serine/threonine phosphorylation levels of Na(v)1.6 and Na(v)1.8 were elevated by more than 60%. Simultaneously, the anti-phosphotyrosine immunoreactivity levels of Na(v)1.6 and Na(v)1.7 increased by approximately 80%. These findings suggest that not only differential expression of sodium channels but also their differential phosphorylation at serine/threonine and tyrosine residues plays a significant role in the etiology of diabetic painful neuropathy. Phosphorylation of Na(v)1.8 is associated with increased excitability and pain sensitization in DRG neurons of diabetic rats. Rapamycin can inhibit mTOR and Na(v)1.8 phosphorylation, thereby reducing current density and voltage thresholds and alleviating hyperalgesia (112). A novel pyridine derivative has shifted the traditional frequency-dependent inhibition of currents to a voltage-dependent inhibition of TTX-R currents, providing relief from tactile allodynia (113) (Table 2).
Under hyperglycemia conditions, the capsaicin-induced calcium (Ca2+) influx in DRG neurons is diminished, potentially a significant contributor to the accumulation of reactive oxygen species (ROS) within these cells (127). The precise role of TRPV1 in mediating neural injury is still under investigation. Previous research has indicated that capsaicin can trigger a reduction in MitoTracker Red fluorescence, an elevation in cytosolic cytochrome c, and the activation of caspase 3, all of which are indicative of heightened oxidative stress (128). Utilizing TRPV1 antagonists, the expression of mu-calpain and the heightened calpain activity observed in DRG neurons of diabetic rats were effectively mitigated. It is thought that the activation of TRPV1 receptor on neuropathy relatively early leads to the preference of large DRG neurons over neuronal stress at the early stages of neuropathy (128). Cao et al. have demonstrated that diabetic rats exhibit high-voltage-activated calcium channel (HVA) currents in larger neurons, as well as T-type currents in medium to large neurons and small DRG neurons (129). There was a notable increase in the expression of Cav3.2 mRNA and the proportion of its corresponding elements. Antagonizing the M4 receptor can significantly attenuate the calcium inflow current, offering pain relief.
Voltage-dependent potassium channels (KCNQ) are extensively expressed in dorsal root ganglia and play a pivotal role in modulating neuronal excitability (Figure 3). Studies have established that the inhibition of the KCNQ pathway can significantly curtail the influx of potassium ions, attenuate signal transmission, and provide substantial relief from peripheral nerve damage and the activation of inflammatory mediators. KCNQ channels are instrumental in generating a slow, non-inactivating potassium current, commonly referred to as the M current (IM). They become active within the subthreshold range of membrane potential and influence various facets of neuronal excitability (130). In diabetic rat models, there is a notable reduction in the expression levels of KCNQ2/3/5 channel proteins in DRG neurons, along with a decrease in IM density. Concurrently, recordings under current clamp conditions have documented depolarization of the resting membrane potential (RMP), a reduction in action potential (AP) threshold, and an increase in AP frequency, indicative of heightened neuronal excitability. This increase in excitability is thought to augment mechanical allodynia and thermal hyperalgesia associated with diabetic neuropathic pain (120). However, not all neurons exhibit altered potassium channel expression; it has been confirmed that Kv7.5 predominantly diminishes immunoreactivity in small to medium-sized nerve fibers of less than 40μm, while Kv7.2 mainly reduces immunoreactivity in neurons less than 30μm. The downregulation of potassium ion channel proteins is believed to contribute to the over-excitability mechanism of neurons (131). Leish Juchri et al. has identified the activation of the Kv7 pathway as a significant biological target for the retigabine (ezogabine)-mediated reduction of mechanical hypersensitivity in diabetic peripheral neuropathy (DPN) mouse models, and when the Kv7/M channel is obstructed, the analgesic effect of ezogabine is significantly diminished (132).
Figure 3. Ion channels’ role in the pathogenesis of diabetic neuropathic pain. Extracellular calcium ions (Ca²⁺) activate TRPM7 and TRPV4 channels, leading to an increase in intracellular calcium levels, which in turn activates caspase 8 and caspase 9, promoting cell apoptosis. Intracellular reactive oxygen species (ROS) regulate the opening of ion channels, exacerbating oxidative stress and promoting the transcription and release of cytokines, thereby intensifying pain. Following Yoda1 activation of the mechanosensitive Piezo1 channel, ATP release exacerbates K⁺ efflux and P2X receptor-mediated Na⁺/Ca²⁺ influx, leading to inhibition of glutamate transporter function and amplification of nociceptive signaling. Concurrently, the PLC-IP3 pathway triggers endoplasmic reticulum Ca²⁺ release via hydrolysis of PIP₂, enhancing spontaneous slow inward currents (SICs) and inducing central sensitization and mechanical allodynia. BDNF alleviates hyperalgesia by activating the TrkB receptor and inhibiting potassium ion efflux.
Following nerve injury, the current densities of various types of potassium currents, including sustained current (I(K)), transient current (I(A)), and I(D), were significantly reduced in medium and large DRG neurons. Additionally, the mRNA levels of I(A) subunits, including Kv1.1, Kv1.2, Kv1.3, and Kv1.4, were decreased by approximately 35-80% (133, 134). Potassium channels are positioned as promising therapeutic targets for the treatment of neuropathic pain. Voltage-gated potassium channel α-subunits are critical targets mediating central sensitization and pain regulation mechanisms (135). Studies have shown that the miR-17-92 cluster is widely expressed in DRG neurons following injury. The miR-17-92 cluster reduces outward potassium currents, particularly A-type currents, by targeting and suppressing the expression of α-subunits, ultimately leading to altered neuronal excitability. Furthermore, the administration of potassium channel modulators (flupirtine and NS5806) effectively alleviated mechanical hyperalgesia induced by miR-17-92 overexpression (136). In diabetic rats, the total voltage-gated potassium channel (Kv) current density, A-type current (I(A)), and delayed rectifier potassium current (I(K)) peak density were significantly reduced in medium- and large-diameter DRG neurons, while no significant differences were observed in small-diameter neurons (121). Concurrently, the mRNA levels of I(A)-related subunits (including Kv1.4, Kv3.4, Kv4.2, and Kv4.3) in DRGs of diabetic rats were approximately halved compared to the control group (121). Therefore, diabetes may primarily induce peripheral pain abnormalities by impairing Kv channel function in medium- and large-diameter DRG neurons.
Alterations in astrocytic ion channels within the spinal cord play a pivotal role in mediating neuropathic pain. Ligand-gated cationic channels such as P2X receptors permit transmembrane flux of Na⁺, Ca²⁺, and K⁺. As non-selective cation channels, P2X receptors mediate ATP-dependent Na⁺/Ca²⁺ influx and K⁺ efflux, inducing membrane depolarization and enhancing neuronal excitability, thereby contributing to peripheral and central sensitization in chronic pain (137). The normal function of glutamate transporters on astrocytes relies on Na⁺ influx, with glutamate transporter currents reflecting electrogenic glutamate uptake. Reduced glutamate transporter currents indicate synaptic glutamate accumulation, amplifying pain signal transmission (138). Lundborg et al. (139) demonstrated that glial cell line-derived neurotrophic factor (GDNF) induces intracellular Ca²⁺ transients in astrocytes via receptor binding by using the Ca²⁺-sensitive fluorescent probe fura-2 AM, and Ca²⁺ transients may disrupt astrocytic function, exacerbating neuroinflammation and pain. The inwardly rectifying potassium channel protein 4.1 (Kir4.1) is specifically expressed in astrocytes, conditional knockout of spinal astrocytic Kir4.1 induces hyperalgesia, while its overexpression alleviates pain (140). Kir4.1 downregulation not only enhances neuronal excitability but also promotes glutamate release from both astrocytes and neurons, further amplifying nociceptive signaling (141). GABA release modulates neuronal excitability, influencing sensory processing and pain transmission. Studies reveal that glutamate binding to astrocytic receptors elevates intracellular Ca²⁺, triggering GABA release—a process blocked by Ca²⁺ chelation in astrocytes (142). Orai1, a core component of calcium release-activated calcium (CRAC) channels, mediates Ca²⁺ entry into astrocytes (143). Birla et al. (144) found that Toll-like receptor 4 (TLR4) upregulates astrocytic Orai1 expression, enhancing Ca²⁺ influx. Notably, Orai1 knockout astrocytes exhibit >50% reduction in inflammatory cytokines, positioning Orai1 as a therapeutic target for chronic pain. In neuropathic pain models, astrocytic KATP channel subunits (SUR1, SUR2, Kir6.1) show marked protein downregulation. Pharmacological activation of KATP channels with cromakalim dose-dependently prevents or suppresses hyperalgesia and allodynia in model rats, concurrently inhibiting NMDA receptor hyperactivation, Ca²⁺-dependent signaling pathways, and modulating astrocytic connexin 43 expression to alleviate neuropathic pain (145). Furthermore, astrocyte-neuron crosstalk manifests as Ca²⁺-elevation-induced slow inward currents (SICs) in lamina II neurons, with increased spontaneous SICs correlating with hyperalgesia and mechanical allodynia progression (146). Thus, astrocytic ion channels directly or indirectly regulate pain pathways, and their precise roles in DNP require further investigation.
Intrathecal administration of the sodium channel activator BmK I in rats upregulated P2X7R expression on microglia in the spinal dorsal horn, accompanied by elevated pro-inflammatory cytokine levels, and pharmacological blockade of P2X7R using brilliant blue G (BBG, a P2X7R antagonist) significantly alleviated spontaneous pain behaviors and thermal hyperalgesia (147). Although this study did not directly assess sodium flux changes induced by BmK I, prior reports indicate that BBG exhibits sodium channel inhibitory activity. N-type voltage-dependent calcium channels (VDCCs), particularly Cav2.2, are critical regulators in the initiation and maintenance of neuropathic pain (148). Japanese researchers (149) observed that conditional Cav2.2 knockdown mice showed markedly reduced tactile allodynia following spinal nerve ligation (SNL) injury, though thermal hyperalgesia remained unaffected. Additionally, Cav2.2 knockdown reduced microglial proliferation in the spinal cord. Upregulation of P2X4 receptors in spinal microglia is implicated in neuropathic pain development. Taspine attenuates neuropathic pain by inhibiting P2X4 receptor activity, impairing microglial function, and reversing the enhancement of ATP-induced calcium signaling by ivermectin (a P2X4 receptor positive allosteric modulator). Mechanistically, Taspine suppresses microglial inflammatory responses via P2X4 receptor inhibition. The Ca²⁺-activated potassium channel KCa3.1 regulates microglial physiological functions by facilitating K⁺ efflux, which induces membrane hyperpolarization, paradoxically promoting Ca²⁺ influx and sustaining elevated intracellular Ca²⁺ concentrations (150). Administration of the KCa3.1 channel inhibitor senicapoc significantly reversed mechanical hyperalgesia in rats with peripheral nerve injury while suppressing microglial activation and inflammatory factor release (151). BK channels, another class of Ca²⁺-activated potassium channels, are activated by increased intracellular Ca²⁺ in microglia following nerve injury, promoting K⁺ efflux to modulate cellular excitability and neurotransmitter release. In neurons, BK channels inhibit action potential generation via membrane hyperpolarization, thereby reducing neuronal excitability. However, specific knockdown of the Ca²⁺-activated potassium channel β3 auxiliary subunit (KCNMB3) suppresses BK channel activation in microglia, attenuating microglial activation and pain-related molecule release, ultimately alleviating hyperalgesia (152). Thus, BK channel activation in microglia enhances pain signaling not through hyperpolarization-mediated excitability suppression but by promoting microglial activation and pain-enhancing molecule release. Ketamine, a clinically used analgesic for both acute and chronic pain, exhibits distinct mechanisms, its acute analgesic effects primarily arise from NMDA receptor inhibition in neurons (153), whereas its chronic pain-modulating mechanisms remain controversial. Lin et al (154). demonstrated that S-ketamine suppresses BK channel currents in microglia, reducing pathological microglial activation, inflammatory cytokine release, P2X4 receptor expression, and BDNF synthesis, thereby mitigating chronic neuropathic pain.
Piezo1 and Piezo2 are mechanosensitive ion channels (MSCs) widely expressed throughout the body. Recent studies reveal that Piezo1 is highly expressed not only in neurons but also in Schwann cells. Under stimulation by the Piezo1 agonist Yoda1, Schwann cells exhibit increased intracellular calcium concentration ([Ca²⁺]i), suggesting a critical role for Schwann cell Piezo1 in mechanosensation and pain transduction (155). In a separate investigation, researchers explored Piezo2 regulation in Schwann cells, demonstrating its predominant expression in differentiated Schwann cells. Piezo2 mediates mechanical stimulus detection (e.g., cellular swelling) to trigger Ca²⁺ influx, thereby regulating cell volume and neurotrophic factor release, and Piezo2 deficiency paradoxically enhances TRPV4 channel activity, exacerbating calcium overload (156). Human tonsil-derived mesenchymal stem cells can differentiate into Schwann-like cells (NRPCs), which may alleviate hyperalgesia by secreting neurotrophic factors such as BDNF and glial cell line-derived neurotrophic factor (GDNF). These factors not only promote neural regeneration but also modulate TRPV1 expression in DRG neurons (51). Schwann cells express diverse potassium channels, including A-type (KA), delayed rectifier (KD), and inwardly rectifying (KIR) subtypes. Delayed rectifier potassium currents are generated by heteromeric complexes of Kv channel α-subunits. Broad-spectrum potassium channel blockers (e.g., quinine, 4-aminopyridine, quinidine) effectively inhibit Schwann cell proliferation, whereas selective Kv1.1, Kv1.2, Kv1.3, and Kv1.6 channel toxins like dendrotoxin I (DTX) show no such inhibitory effects (157). Schwann cells are classified into myelinating and non-myelinating subtypes, with the latter being crucial for maintaining neural functionality. Notably, Kir4.1 is specifically expressed and functionally active in non-myelinating Schwann cells, where it regulates potassium uptake to modulate membrane potential and neural signaling. This mechanism may contribute to pain perception and related pathologies, offering novel directions for therapeutic exploration (158).
The Kv4 channel complex consists of pore-forming subunits (Kv4.1-Kv4.3) and two regulatory subunits (KChIP1-4 and DPP6/DPP10). Previous studies confirmed that Kv4.3 knockout selectively induces mechanical hypersensitivity (159). Guo et al. (160) demonstrated that knockdown of any component of the Kv4 complex (Kv4.3, KChIP1, KChIP2, or DPP10) reduces the expression of other subunits, induces mechanical hypersensitivity, and enhances excitability in IB4⁺ nociceptors. Regulatory subunits of Kv4 channels represent promising therapeutic targets for precision treatment of neuropathic pain. In contrast to other ion channels, Kir7.1 is predominantly localized to neuronal membranes rather than glial cells in rats. Co-immunoprecipitation revealed that spinal Kir7.1 channels undergo SUMO-1 modification but not SUMO-2/3 conjugation, with SUMOylation upregulated following spared nerve injury (SNI). Furthermore, pharmacological inhibition of SUMOylation using ginkgolic acid (GA, an E1 inhibitor) or 2-D08 (a UBC9 inhibitor) increases surface expression of Kir7.1 in the spinal cord (161), highlighting Kir channels as potential therapeutic targets for pain management (162). Nav1.8, a voltage-gated sodium channel selectively expressed in peripheral nociceptors, is enriched in DRG neurons (163). In a Phase III clinical trial involving 2,447 participants, suzetrigine significantly alleviated moderate-to-severe acute pain by selectively inhibiting Nav1.8-mediated pain signaling in the peripheral nervous system (164). Similarly, Nav1.7, another voltage-gated sodium channel essential for initiating C-fiber action potentials (APs), confers pain insensitivity in Nav1.7 knockout (KO) mice or upon pharmacological inhibition (165). Huang et al (166). reported that the Nav1.7 I234T mutation depolarizes the resting membrane potential (RMP) in a subset of DRG neurons, rendering them electrically silent and incapable of generating APs, thereby causing congenital pain insensitivity. Conversely, milder RMP depolarization in other neurons increases neuronal excitability, driving pain phenotypes. Intracellular calcium concentration ([Ca²⁺]i) in DRG neurons is critically associated with neuropathic pain. Lamotrigine elevates [Ca²⁺]i by activating the PLC-IP3R/RyR signaling pathway and stimulating calmodulin-dependent kinase II (CaMKII). This [Ca²⁺]i increase primarily originates from intracellular Ca²⁺ release rather than extracellular Ca²⁺ influx (167).
The etiology of DNP is intricate, and there exists a dearth of efficacious therapeutic agents and modalities (168). DNP significantly impairs patients’ quality of life, with studies indicating that persistent chronic pain adversely affects mental well-being, further impacting their daily routines (169, 170). Despite extensive research highlighting that DNP involves a complex interplay of factors in aberrant signal transduction (171, 172), the pathophysiological intricacies present a formidable challenge in the development of potent medications. Presently, the US FDA has approved three medications for DNP treatment: pregabalin, duloxetine, and the 8% capsaicin patch (173–175). Moreover, amitriptyline, and select opioid analgesics are also utilized clinically to alleviate DNP symptoms (176–178). In severe cases, potent opioids such as fentanyl may be prescribed (179), necessitating a delicate balance between effective pain management and the risks of addiction to prevent patients from succumbing to substance dependence (180).
Additionally, herbal extracts and compound formulations derived from traditional Chinese medicine offer novel avenues for the alleviation of DNP, contributing fresh perspectives for the development of DNP therapeutics and the refinement of treatment protocols (181, 182) (Table 3). Jinmaitong, a traditional Chinese medicinal prescription, Zhang and his colleagues has been demonstrated that Jinmaitong, a traditional Chinese medicinal prescription, exhibits efficacy in mitigating microglial activation induced by hyperglycemia curb the activation of inflammatory factors and chemokines, and bolster the expression of anti-inflammatory mediators within the body (183). Quercetin has been shown to suppress the expression of the synaptic protein PSD-95 and the synaptic plasticity of neurons in the dorsal horn, thereby diminishing the propagation of aberrant signals and reducing hyperalgesia (184). Curcumin, with its antioxidant and anti-inflammatory properties, has also proven effective in curbing the release of inflammatory mediators. Korean researchers (46) have confirmed in DNP models that curcumin can alleviate neuropathic pain by suppressing the heightened expression of PJN on astrocytes and neurons. Furthermore, other botanical extracts, such as Emodin, Nerunjil, and Emblica, have been identified to significantly contribute to the inhibition of inflammatory factor release, oxidative stress, and the mitigation of DNP (199, 201).
Neuropathic pain is associated with various risk factors, including but not limited to age, geographic location, smoking, alcohol consumption, gender, and disease duration (202–204). Among these, gender is an important factor that should not be overlooked in personalized clinical pharmacotherapy. Cardinez et al (205). investigated long-term diabetic patients in Canada and found that over one-third of these patients experienced neuropathic pain, with 42% being female patients, compared to 27% of male patients. Notably, the association of female gender as a risk factor for diabetic neuropathic pain (DNP) remained robust regardless of whether patients had concomitant neuropathy. Alon Abraham reported that women are more sensitive to pain intensity than men, with a 28.3% higher frequency of pain occurrence in females. Additionally, women reported higher pain intensity, with a greater prevalence of severe pain compared to men (206). A genome-wide association study (207) conducted in the UK confirmed that diabetic neuropathic pain is a heritable trait and identified gender-related genetic loci, specifically Chr1p35.1 (ZSCAN20-TLR12P) in males and Chr8p23.1 (HMGB1P46) in females. This represents the first genetic evidence of gender differences in DNP (208), providing a foundation for gender-based pharmacological research and therapeutic strategies for DNP.
Despite the discovery of gender differences in diabetic neuropathic pain (DNP) from clinical and genetic perspectives, the underlying biological mechanisms remain unclear. One hypothesis suggests that the interaction between the immune and nervous systems may be one of the possible mechanisms leading to chronic pain. Adaptive immunity could be a primary factor mediating these gender differences. Previous studies have confirmed that in DNP patients, there is an increased proportion of CD4+ central memory T cells, along with a rise in the absolute numbers of monocytes and natural killer cells, which indicate an inflammatory response (209). Although researchers have not differentiated the relative differences in T cells by gender, this suggests alterations in the adaptive immune response related to DNP. Further research is needed to clarify the changes in immune cell composition and clinical features in DNP patients. Sorge et al (210). revealed that mechanical hypersensitivity in female mice primarily relies on adaptive immune cells, whereas male mice depend on glial cells for pain perception. However, this does not imply that glial cells are uninvolved in the pain processes in female mice; such a phenomenon typically occurs when female mice lack adaptive immune cells, possibly due to the significantly higher presence of peripheral resident T cells in female mice compared to their male counterparts (211). Kuhn et al (212). similarly found that when colony-stimulating factor 1 (CSF1) was injected intrathecally into mice, the activation of microglia in male mice was enhanced, while the activation of microglia was less pronounced in female mice who showed a significant increase in regulatory T cells (Tregs). Subsequent research confirmed that Tregs, as inflammation suppressors, alleviate pain hypersensitivity in mice by inhibiting microglial activation. Interestingly, when CD4+ T cells are absent (excluding CD8+ T cells), female mice require 2 to 3 times the analgesic dose compared to male mice. Additionally, the activation of astrocytes plays a crucial role in neuropathic pain. Recent studies have shown that IL-16 expression levels are elevated in a spinal nerve ligation model involving female mice. Knocking down IL-16 or administering FGF22-IN-1 (a CD4 inhibitor) alleviated pain hypersensitivity in these mice. The interaction of IL-16 with CD4 on CD3+ T cells mediates astrocyte activation, representing an important mechanism of neuropathic pain (213). The gender differences in the perception of neuropathic pain involve complex interactions between the immune and nervous systems (214). The adaptive immune system plays a key role in these sex differences, and the roles of glial cells also vary between genders. More research is needed to explore these mechanisms in depth, to enhance our understanding and treatment of DNP.
Gender differences in neuropathic pain may arise from ion channel mechanisms. Orai1, a core component of calcium release-activated calcium (CRAC) channels, serves as a critical regulator of calcium influx (215). Recent studies demonstrate that conditional knockout of microglial Orai1 in male mice suppresses microglial proliferation, reduces spinal levels of cytokines including TNFα, IL-6, IL-1β, and BDNF, and inhibits excitatory synaptic transmission in dorsal horn neurons, whereas female mice exhibit no significant alterations in these phenotypes (216). In males, microglia-derived BDNF drives neuronal hyperexcitability and mechanical allodynia through KCC2 downregulation, whereas chloride imbalance in females predominantly involves adaptive immune cell pathways rather than microglial mechanisms (210). Yang et al. (217) revealed that TRPA1 and TRPV1 activation induces pain hypersensitivity via TRPM8-dependent release of inflammatory neuropeptides (CGRP and substance P), with notable sexual dimorphism, CGRP exclusively mediates aberrant pain in female mice, and CGRP receptor antagonists specifically reverse cold hypersensitivity in neuroinflammatory female models. Conversely, male mice cold allodynia operates through TLR4 signaling, with TLR4 inhibition providing male-specific analgesia. Furthermore, the “Sex Hormone-ThermoTRP Axis” proposed by Cabañero’s team delineates how sex hormones differentially regulate ion channel functionality and immune signaling networks to mediate pain dimorphism, establishing a molecular framework for gender-specific analgesic strategies (218). Sexual dimorphism in pain represents a frequently overlooked biological determinant in mechanistic research and therapeutic development. Elucidating the dynamic interactions among hormones, ion channels, and immune cell characteristics will advance our understanding of sex-specific pain pathophysiology and facilitate the development of precision-targeted therapeutic interventions.
Diabetes, characterized by chronically elevated blood sugar levels, is emerging as a significant global health concern due to its rising prevalence (219). Over recent decades, the advancement of public health education and the dissemination of fundamental diabetes knowledge have led to an increased societal understanding of the condition. Adherence to a proper lifestyle and consistent medication use can effectively maintain blood sugar levels within a normal and stable range (220),and this has fortified the practical foundation for patient-centered diabetes management strategies that emphasize prevention and control (221, 222). Nonetheless, despite well-managed blood sugar levels, diabetic patients remain susceptible to complications that can severely diminish their quality of life. In particular, DNP may develop early in the course of the disease (223, 224) and persist even when blood sugar levels are stable, manifesting as symptoms such as limb paresthesia, spontaneous pain, and hyperalgesia (225). This indicates that the pathogenesis of DNP is not solely attributable to hyperglycemia and underscores the need for a deeper investigation into its underlying mechanisms to address clinical challenges (226). In clinical practice, some patients lack a comprehensive understanding of DNP and are even unaware of its connection to diabetes (227). Consequently, when faced with painful clinical manifestations, they may exhibit a dismissive attitude, which can dampen their engagement in DNP treatment.
Recent studies on the mechanistic connections between existing analgesic drugs and ion channel-targeted therapeutic strategies have demonstrated that the analgesic effects of conventional medications extend beyond classical neurotransmitter modulation, involving intricate interactions with ion channels. Pregabalin, a first-line treatment for diabetic neuropathic pain (DNP), exerts its primary action by binding to the α2δ subunit of voltage-gated calcium channels (VGCCs), thereby reducing calcium influx, suppressing presynaptic neurotransmitter release, and alleviating neuronal hyperexcitability (228, 229). Research from South Korean scientists revealed that intrathecal co-administration of pregabalin with P2X3/7 inhibitors and κ-opioid receptor agonists enhances analgesic efficacy in a combinatorial manner, suggesting that multi-targeted modulation of ion channels represents a promising strategy for pain management (230). Similarly, mirogabalin, which shares pregabalin’s mechanism of targeting the α2δ subunit but exhibits distinct ligand selectivity and slower dissociation kinetics, has demonstrated superior analgesic potency, enhanced safety profiles, and significantly reduced adverse effects in clinical trials compared to pregabalin (231). Additionally, synergistic analgesic effects have been observed when combining pregabalin with Kv7 potassium channel openers (flupirtine and retigabine), achieved through dual regulation of voltage-gated calcium and potassium channels. These findings underscore the potential of multi-mechanistic ion channel modulation as a refined approach for developing next-generation analgesics (232). Duloxetine, a serotonin-norepinephrine reuptake inhibitor, exerts its analgesic effects not only through inhibition of neurotransmitter reuptake but also via multimodal modulation of ion channels. It suppresses peripheral nociceptive signaling by blocking transient currents of Nav1.7 sodium channels (233) and further regulates TRP channel families, specifically inhibiting TRPC1/TRPC5 and TRPC4 channel activity to reduce calcium influx while decreasing current densities of TRPM2 and TRPV1 channels (234), thereby mitigating calcium overload-induced oxidative stress and apoptosis (235). Notably, although co-administration with minocycline improves mechanical allodynia by suppressing microglial activation, it exerts no significant alleviation of cold allodynia (236), underscoring the pathway-specific nature of duloxetine’s analgesic actions. A meta-analysis (237) demonstrated that 8% capsaicin effectively alleviates pain in patients with painful diabetic peripheral neuropathy, with fewer central adverse effects compared to oral analgesics. Capsaicin exerts its analgesic effects primarily by agonizing the TRPV1, triggering calcium influx that induces transient release followed by depletion of neuropeptides such as substance P from nerve terminals, thereby reducing nociceptive sensitization (238). Hagenacker et al (238). further revealed that capsaicin differentially modulates L-type, N-type, and T-type voltage-activated calcium channel currents in DRG neurons, with significant suppression of calcium channel activity in small-diameter neurons, potentially influencing neuronal excitability and neurotransmitter release. Additionally, capsaicin enhances intracellular sodium concentration ([Na⁺]i) in DRG neurons via TRPV1 activation, which indirectly suppresses voltage-gated sodium channel currents, attenuating neuronal excitability and action potential propagation. Intriguingly, high-concentration capsaicin selectively inhibits the sustained potassium current (IK), inducing hyperactivation of nociceptive neurons, which may contribute to its paradoxical hyperalgesic effects (239). These findings provide novel insights into the dual pro-nociceptive and analgesic mechanisms of capsaicin, highlighting its complex modulation of ion channel networks in pain pathways.
Regulating blood sugar levels stands as the fundamental approach in the management of DNP. GLP-1 receptor agonists, exemplified by semaglutide, have demonstrated significant efficacy in stimulating insulin secretion and reducing blood glucose levels effectively (240, 241). Semaglutide’s extended dosing interval enhances patient adherence to medication regimens, offering renewed optimism for the chronic management of diabetes. Presently, innovative diabetes treatments are under clinical investigation, including bimagrumab, orforglipron, and umbilical cord-derived mesenchymal stromal cells (242–244). These novel therapies are set to expand the horizons of diabetes care. Nonetheless, the proliferation of these treatments has intensified the focus on diabetes-related complications. In contrast to the diverse array of new hyperglycemia medications, DNP treatment options remain limited. Moreover, the effectiveness of current drugs can be variable among individuals and may entail certain side effects (245). Consequently, investigating the etiology of DNP, identifying novel therapeutic targets, and formulating new pharmaceuticals are crucial for easing patient symptoms and enhancing their quality of life. The application of herbal medicine, including traditional Chinese medicine, has a millennia-long history in disease treatment. Notable examples include drugs like artemisinin (246), arsenic trioxide (247) (a primary component of arsenic), and vincristine (248), which, following rigorous scientific validation, have been globally recognized for their potent effects against malaria and various cancers, including leukemia. Therefore, investigating the monomeric constituents and composite formulations within traditional Chinese medicines, in conjunction with contemporary pharmacological techniques, may offer novel insights and pathways for the research and development of DNP therapeutics.
The pathogenesis of diabetic neuropathic pain (DNP) involves complex interactions between neurons and glial cells (microglia, astrocytes, and Schwann cells), with dysregulation of ion channels playing a central role in abnormal nociceptive signaling. Neuronal hyperexcitability arises from imbalanced sodium (Nav1.7/1.8 overactivation), potassium (KCNQ functional suppression), and calcium channels (TRPV1/TRPM8-mediated calcium overload), while glial cells amplify pain through pro-inflammatory cytokine release, synaptic plasticity modulation, and neurotrophic dysregulation. Ion channel-targeted therapies (Nav1.8 inhibitors, Kv7 activators, TRP modulators) show promise by reducing neuronal excitability and glial inflammation. However, attention should be paid to the impact of gender differences on the function of ion channels. When developing new drugs targeting ion channels, gender-specific mechanisms should be incorporated to optimize clinical efficacy.
JW: Conceptualization, Formal Analysis, Investigation, Methodology, Project administration, Writing – original draft, Writing – review & editing, Supervision. HH: Conceptualization, Formal Analysis, Investigation, Project administration, Supervision, Writing – original draft, Writing – review & editing. XL: Conceptualization, Methodology, Project administration, Resources, Supervision, Writing – original draft, Writing – review & editing.
The author(s) declare that no financial support was received for the research and/or publication of this article.
Figures were created with biorender.com.
The authors declare that the research was conducted in the absence of any commercial or financial relationships that could be construed as a potential conflict of interest.
All claims expressed in this article are solely those of the authors and do not necessarily represent those of their affiliated organizations, or those of the publisher, the editors and the reviewers. Any product that may be evaluated in this article, or claim that may be made by its manufacturer, is not guaranteed or endorsed by the publisher.
1. GBD 2017 Disease and Injury Incidence and Prevalence Collaborators. Global, regional, and national incidence, prevalence, and years lived with disability for 354 diseases and injuries for 195 countries and territories, 1990-2017: a systematic analysis for the Global Burden of Disease Study 2017. Lancet (London England). (2018) 392:1789–858. doi: 10.1016/S0140-6736(18)32279-7
2. Sun H, Saeedi P, Karuranga S, Pinkepank M, Ogurtsova K, Duncan BB, et al. IDF Diabetes Atlas: Global, regional and country-level diabetes prevalence estimates for 2021 and projections for 2045. Diabetes Res Clin Pract. (2022) 183:109119. doi: 10.1016/j.diabres.2021.109119
3. Li Y, Teng D, Shi X, Qin G, Qin Y, Quan H, et al. Prevalence of diabetes recorded in mainland China using 2018 diagnostic criteria from the American Diabetes Association: national cross sectional study. BMJ. (2020) 369:m997. doi: 10.1136/bmj.m997
4. Ceriello A, deValk HW, Guerci B, Haak T, Owens D, Canobbio M, et al. The burden of type 2 diabetes in Europe: Current and future aspects of insulin treatment from patient and healthcare spending perspectives. Diabetes Res Clin Pract. (2020) 161:108053. doi: 10.1016/j.diabres.2020.108053
5. Kehlenbrink S, Smith J, Ansbro É, Fuhr DC, Cheung A, Ratnayake R, et al. The burden of diabetes and use of diabetes care in humanitarian crises in low-income and middle-income countries. Lancet Diabetes Endocrinol. (2019) 7:638–47. doi: 10.1016/S2213-8587(19)30082-8
6. Ritchie RH, Abel ED. Basic mechanisms of diabetic heart disease. Circ Res. (2020) 126:1501–25. doi: 10.1161/CIRCRESAHA.120.315913
7. Zhang X, Sivaprasad S, Ting DSW. Editorial: Ocular complications associated with diabetes mellitus. Front Endocrinol (Lausanne). (2023) 14:1193522. doi: 10.3389/fendo.2023.1193522
8. Shi Y, Vanhoutte PM. Macro- and microvascular endothelial dysfunction in diabetes. J Diabetes. (2017) 9:434–49. doi: 10.1111/1753-0407.12521
9. Li X, Lu L, Hou W, Huang T, Chen X, Qi J, et al. Epigenetics in the pathogenesis of diabetic nephropathy. Acta Biochim Et Biophys Sinica. (2022) 54:163–72. doi: 10.3724/abbs.2021016
10. Rosenberger DC, Blechschmidt V, Timmerman H, Wolff A, Treede R-D. Challenges of neuropathic pain: focus on diabetic neuropathy. J Neural Transm (Vienna Austria: 1996). (2020) 127:589–624. doi: 10.1007/s00702-020-02145-7
11. Fang X-X, Wang H, Song H-L, Wang J, Zhang Z-J. Neuroinflammation involved in diabetes-related pain and itch. Front In Pharmacol. (2022) 13:921612. doi: 10.3389/fphar.2022.921612
12. Ved N, Da Vitoria Lobo ME, Bestall SM, L Vidueira C, Beazley-Long N, Ballmer-Hofer K, et al. Diabetes-induced microvascular complications at the level of the spinal cord: a contributing factor in diabetic neuropathic pain. J Physiol. (2018) 596:3675–93. doi: 10.1113/JP275067
13. Dong J, Zuo Z, Yan W, Liu W, Zheng Q, Liu X. Berberine ameliorates diabetic neuropathic pain in a rat model: involvement of oxidative stress, inflammation, and μ-opioid receptors. Naunyn Schmiedebergs Arch Pharmacol. (2019) 392:1141–9. doi: 10.1007/s00210-019-01659-6
14. Bagues A, Hu J, Alshanqiti I, Chung M-K. Neurobiological mechanisms of botulinum neurotoxin-induced analgesia for neuropathic pain. Pharmacol Ther. (2024) 259:108668. doi: 10.1016/j.pharmthera.2024.108668
15. Todd AJ. Neuronal circuitry for pain processing in the dorsal horn. Nat Rev Neurosci. (2010) 11:823–36. doi: 10.1038/nrn2947
16. Li D, Kim W, Shin D, Jung Y, Bae H, Kim SK. Preventive effects of bee venom derived phospholipase A(2) on oxaliplatin-induced neuropathic pain in mice. Toxins (Basel). (2016) 8(1):27. doi: 10.3390/toxins8010027
17. Tsuda M, Koga K, Chen T, Zhuo M. Neuronal and microglial mechanisms for neuropathic pain in the spinal dorsal horn and anterior cingulate cortex. J Neurochemistry. (2017) 141:486–98. doi: 10.1111/jnc.14001
18. Tan AM, Samad OA, Fischer TZ, Zhao P, Persson A-K, Waxman SG. Maladaptive dendritic spine remodeling contributes to diabetic neuropathic pain. J Neuroscience: Off J Soc For Neurosci. (2012) 32:6795–807. doi: 10.1523/JNEUROSCI.1017-12.2012
19. Schuelert N, Gorodetskaya N, Just S, Doods H, Corradini L. Electrophysiological characterization of spinal neurons in different models of diabetes type 1- and type 2-induced neuropathy in rats. Neuroscience. (2015) 291:146–54. doi: 10.1016/j.neuroscience.2015.02.003
20. Dohrn MF, Dumke C, Hornemann T, Nikolin S, Lampert A, Espenkott V, et al. Deoxy-sphingolipids, oxidative stress, and vitamin C correlate with qualitative and quantitative patterns of small fiber dysfunction and degeneration. Pain. (2022) 163:1800–11. doi: 10.1097/j.pain.0000000000002580
21. Ghavami H, Radfar M, Soheily S, Shamsi SA, Khalkhali HR. Effect of lifestyle interventions on diabetic peripheral neuropathy in patients with type 2 diabetes, result of a randomized clinical trial. Agri. (2018) 30:165–70. doi: 10.5505/agri.2018.45477
22. Christofides EA, Valentine V. L-methylfolate in diabetic peripheral neuropathy: A narrative review. Endocr Pract. (2023) 29:663–9. doi: 10.1016/j.eprac.2023.04.005
23. Pabreja K, Dua K, Sharma S, Padi SSV, Kulkarni SK. Minocycline attenuates the development of diabetic neuropathic pain: possible anti-inflammatory and anti-oxidant mechanisms. Eur J Pharmacol. (2011) 661:15–21. doi: 10.1016/j.ejphar.2011.04.014
24. Naranjo C, Del Reguero L, Moratalla G, Hercberg M, Valenzuela M, Failde I. Anxiety, depression and sleep disorders in patients with diabetic neuropathic pain: a systematic review. Expert Rev Neurother. (2019) 19:1201–9. doi: 10.1080/14737175.2019.1653760
25. Li J, Wei Y, Zhou J, Zou H, Ma L, Liu C, et al. Activation of locus coeruleus-spinal cord noradrenergic neurons alleviates neuropathic pain in mice via reducing neuroinflammation from astrocytes and microglia in spinal dorsal horn. J Neuroinflammation. (2022) 19:123. doi: 10.1186/s12974-022-02489-9
26. Ding Y, Hu L, Wang X, Sun Q, Hu T, Liu J, et al. The contribution of spinal dorsal horn astrocytes in neuropathic pain at the early stage of EAE. Neurobiol Disease. (2022) 175:105914. doi: 10.1016/j.nbd.2022.105914
27. Lee JY, Park CS, Seo KJ, Kim IY, Han S, Youn I, et al. IL-6/JAK2/STAT3 axis mediates neuropathic pain by regulating astrocyte and microglia activation after spinal cord injury. Exp Neurology. (2023) 370:114576. doi: 10.1016/j.expneurol.2023.114576
28. Malcangio M. GABAB receptors and pain. Neuropharmacology. (2018) 136:102–5. doi: 10.1016/j.neuropharm.2017.05.012
29. Liu P, Yuan H-B, Zhao S, Liu F-F, Jiang Y-Q, Guo Y-X, et al. Activation of GABAB receptor suppresses diabetic neuropathic pain through toll-like receptor 4 signaling pathway in the spinal dorsal horn. Mediators Inflammation. (2018) 2018:6016272. doi: 10.1155/2018/6016272
30. Qu S-Y, Wang H-Z, Hu Q-Q, Ma Y-Q, Kang Y-R, Ma L-Q, et al. Electroacupuncture may alleviate diabetic neuropathic pain by inhibiting the microglia P2X4R and neuroinflammation. Purinergic Signalling. (2023). doi: 10.1007/s11302-023-09972-9
31. Zhang Q, Li Q, Liu S, Zheng H, Ji L, Yi N, et al. Glucagon-like peptide-1 receptor agonist attenuates diabetic neuropathic pain via inhibition of NOD-like receptor protein 3 inflammasome in brain microglia. Diabetes Res Clin Pract. (2022) 186:109806. doi: 10.1016/j.diabres.2022.109806
32. Song ZH, Song XJ, Yang CL, Cao P, Mao Y, Jin Y, et al. Up-regulation of microglial chemokine CXCL12 in anterior cingulate cortex mediates neuropathic pain in diabetic mice. Acta Pharmacol Sin. (2023) 44:1337–49. doi: 10.1038/s41401-022-01046-7
33. Lee JY, Choi HY, Park CS, Pyo MK, Yune TY, Kim GW, et al. GS-KG9 ameliorates diabetic neuropathic pain induced by streptozotocin in rats. J Ginseng Res. (2019) 43:58–67. doi: 10.1016/j.jgr.2017.08.004
34. Jin G-L, Hong L-M, Liu H-P, Yue R-C, Shen Z-C, Yang J, et al. Koumine modulates spinal microglial M1 polarization and the inflammatory response through the Notch-RBP-Jκ signaling pathway, ameliorating diabetic neuropathic pain in rats. Phytomedicine. (2021) 90:153640. doi: 10.1016/j.phymed.2021.153640
35. Shen C-L, Wang R, Santos JM, Elmassry MM, Stephens E, Kim N, et al. Ginger alleviates mechanical hypersensitivity and anxio-depressive behavior in rats with diabetic neuropathy through beneficial actions on gut microbiome composition, mitochondria, and neuroimmune cells of colon and spinal cord. Nutr Res. (2024) 124:73–84. doi: 10.1016/j.nutres.2024.01.014
36. Kuthati Y, Rao VN, Huang W-H, Busa P, Wong C-S. Teneligliptin co-infusion alleviates morphine tolerance by inhibition of spinal microglial cell activation in streptozotocin-induced diabetic rats. Antioxidants (Basel Switzerland). (2023) 12(7):1478. doi: 10.3390/antiox12071478
37. Huang Q, Mao X-F, Wu H-Y, Li T-F, Sun M-L, Liu H, et al. Bullatine A stimulates spinal microglial dynorphin A expression to produce anti-hypersensitivity in a variety of rat pain models. J Neuroinflammation. (2016) 13:214. doi: 10.1186/s12974-016-0696-2
38. Zhang T-T, Xue R, Fan S-Y, Fan Q-Y, An L, Li J, et al. Ammoxetine attenuates diabetic neuropathic pain through inhibiting microglial activation and neuroinflammation in the spinal cord. J Neuroinflammation. (2018) 15:176. doi: 10.1186/s12974-018-1216-3
39. Hwang S-M, Rahman MM, Go EJ, Kim YH, Park C-K. Specific transcription factors Ascl1 and Lhx6 attenuate diabetic neuropathic pain by modulating spinal neuroinflammation and microglial activation in mice. Biomedicine Pharmacotherapy = Biomedecine Pharmacotherapie. (2024) 173:116392. doi: 10.1016/j.biopha.2024.116392
40. Zychowska M, Rojewska E, Piotrowska A, Kreiner G, Mika J. Microglial inhibition influences XCL1/XCR1 expression and causes analgesic effects in a mouse model of diabetic neuropathy. Anesthesiology. (2016) 125:573–89. doi: 10.1097/ALN.0000000000001219
41. Liu X, He J, Gao J, Xiao Z. Fluorocitrate and neurotropin confer analgesic effects on neuropathic pain in diabetic rats via inhibition of astrocyte activation in the periaqueductal gray. Neurosci Letters. (2022) 768:136378. doi: 10.1016/j.neulet.2021.136378
42. Lu J-S, Yang L, Chen J, Xiong F-F, Cai P, Wang X-Y, et al. Basolateral amygdala astrocytes modulate diabetic neuropathic pain and may be a potential therapeutic target for koumine. Br J Pharmacol. (2023) 180:1408–28. doi: 10.1111/bph.16011
43. Lu J, Yang L, Xu Y, Ai L, Chen J, Xiong F, et al. The modulatory effect of motor cortex astrocytes on diabetic neuropathic pain. J Neuroscience: Off J Soc For Neurosci. (2021) 41:5287–302. doi: 10.1523/JNEUROSCI.2566-20.2021
44. Deng XT, Wu MZ, Xu N, Ma PC, Song XJ. Activation of ephrinB-EphB receptor signalling in rat spinal cord contributes to maintenance of diabetic neuropathic pain. Eur J Pain. (2017) 21:278–88. doi: 10.1002/ejp.922
45. Yang L, Lu J, Guo J, Chen J, Xiong F, Wang X, et al. Ventrolateral periaqueductal gray astrocytes regulate nociceptive sensation and emotional motivation in diabetic neuropathic pain. J Neuroscience: Off J Soc For Neurosci. (2022) 42:8184–99. doi: 10.1523/JNEUROSCI.0920-22.2022
46. Park H, Lee J-H, Sim JH, Park J, Choi S-S, Leem JG. Effects of curcumin treatment in a diabetic neuropathic pain model of rats: involvement of c-jun N-terminal kinase located in the astrocytes and neurons of the dorsal root ganglion. Pain Res Manage. (2021) 2021:8787231. doi: 10.1155/2021/8787231
47. Ge H, Sun M, Wei X, Zhang M, Tu H, Hao Y, et al. Protective effects of dihydromyricetin on primary hippocampal astrocytes from cytotoxicity induced by comorbid diabetic neuropathic pain and depression. Purinergic Signalling. (2020) 16:585–99. doi: 10.1007/s11302-020-09752-9
48. Chang L-L, Wang H-C, Tseng K-Y, Su M-P, Wang J-Y, Chuang Y-T, et al. Upregulation of miR-133a-3p in the sciatic nerve contributes to neuropathic pain development. Mol Neurobiology. (2020) 57:3931–42. doi: 10.1007/s12035-020-01999-y
49. Olukman M, Önal A, Celenk FG, Uyanıkgil Y, Cavuşoğlu T, Düzenli N, et al. Treatment with NADPH oxidase inhibitor apocynin alleviates diabetic neuropathic pain in rats. Neural Regener Res. (2018) 13:1657–64. doi: 10.4103/1673-5374.232530
50. Gao N, Ma B, Jia H, Hao C, Jin T, Liu X. Translocator protein alleviates allodynia and improves Schwann cell function against diabetic peripheral neuropathy via activation of the Nrf2-dependent antioxidant system and promoting autophagy. Diabetes Med. (2023) 40:e15090. doi: 10.1111/dme.15090
51. Yum Y, Park S, Nam YH, Yoon J, Song H, Kim HJ, et al. Therapeutic effect of schwann cell-like cells differentiated from human tonsil-derived mesenchymal stem cells on diabetic neuropathy in db/db mice. Tissue Eng Regener Med. (2024) 21:761–76. doi: 10.1007/s13770-024-00638-0
52. Prinz M, Jung S, Priller J. Microglia biology: one century of evolving concepts. Cell. (2019) 179:292–311. doi: 10.1016/j.cell.2019.08.053
53. Borst K, Dumas AA, Prinz M. Microglia: Immune and non-immune functions. Immunity. (2021) 54:2194–208. doi: 10.1016/j.immuni.2021.09.014
54. Zhang Y-Y, Wang L, Guo H, Han T-T, Chang Y-H, Cui X-C. Levetiracetam attenuates diabetes-associated cognitive impairment and microglia polarization by suppressing neuroinflammation. Front In Pharmacol. (2023) 14:1145819. doi: 10.3389/fphar.2023.1145819
55. Pfeifer CW, Walsh JT, Santeford A, Lin JB, Beatty WL, Terao R, et al. Dysregulated CD200-CD200R signaling in early diabetes modulates microglia-mediated retinopathy. Proc Natl Acad Sci United States America. (2023) 120:e2308214120. doi: 10.1073/pnas.2308214120
56. Tansley S, Gu N, Guzmán AU, Cai W, Wong C, Lister KC, et al. Microglia-mediated degradation of perineuronal nets promotes pain. Sci (New York NY). (2022) 377:80–6. doi: 10.1126/science.abl6773
57. Tsuda M, Ueno H, Kataoka A, Tozaki-Saitoh H, Inoue K. Activation of dorsal horn microglia contributes to diabetes-induced tactile allodynia via extracellular signal-regulated protein kinase signaling. Glia. (2008) 56:378–86. doi: 10.1002/glia.20623
58. Daulhac L, Maffre V, Mallet C, Etienne M, Privat A-M, Kowalski-Chauvel A, et al. Phosphorylation of spinal N-methyl-d-aspartate receptor NR1 subunits by extracellular signal-regulated kinase in dorsal horn neurons and microglia contributes to diabetes-induced painful neuropathy. Acta Histochem. (2011) 113(6):677–6. doi: 10.1016/j.ejpain.2010.06.003
59. Zychowska M, Rojewska E, Przewlocka B, Mika J. Mechanisms and pharmacology of diabetic neuropathy - experimental and clinical studies. Pharmacol Rep. (2013) 65:1601–10. doi: 10.1016/S1734-1140(13)71521-4
60. Wang Q, Xie Y, Ma S, Luo H, Qiu Y. Role of microglia in diabetic neuropathic pain. Front Cell Dev Biol. (2024) 12:1421191. doi: 10.3389/fcell.2024.1421191
61. Talbot S, Couture R. Emerging role of microglial kinin B1 receptor in diabetic pain neuropathy. Exp Neurology. (2012) 234:373–81. doi: 10.1016/j.expneurol.2011.11.032
62. Chen X, Le Y, Tang S-Q, He W-Y, He J, Wang Y-H, et al. Painful diabetic neuropathy is associated with compromised microglial IGF-1 signaling which can be rescued by green tea polyphenol EGCG in mice. Oxid Med Cell Longevity. (2022) 2022:6773662. doi: 10.1155/2022/6773662
63. Lanlua P, Prommahom A, Sricharoenvej S. Increased number of activated microglia in rat spinal cord during early stage of diabetic induction. Folia Morphol (Warsz). (2020) 79:662–71. doi: 10.5603/FM.a2019.0136
64. Li T-F, Fan H, Wang Y-X. Aconitum-derived bulleyaconitine A exhibits antihypersensitivity through direct stimulating dynorphin A expression in spinal microglia. J Pain. (2016) 17:530–48. doi: 10.1016/j.jpain.2015.12.015
65. Sun M-L, Ao J-P, Wang Y-R, Huang Q, Li T-F, Li X-Y, et al. Lappaconitine, a C18-diterpenoid alkaloid, exhibits antihypersensitivity in chronic pain through stimulation of spinal dynorphin A expression. Psychopharmacol (Berl). (2018) 235:2559–71. doi: 10.1007/s00213-018-4948-y
66. Lin L, Basu R, Chatterjee D, Templin AT, Flak JN, Johnson TS. Disease-associated astrocytes and microglia markers are upregulated in mice fed high fat diet. Sci Rep. (2023) 13:12919. doi: 10.1038/s41598-023-39890-0
67. Zhao Y, Pu D, Sun Y, Chen J, Luo C, Wang M, et al. High glucose-induced defective thrombospondin-1 release from astrocytes via TLR9 activation contributes to the synaptic protein loss. Exp Cell Res. (2018) 363:171–8. doi: 10.1016/j.yexcr.2017.12.030
68. Bahniwal M, Little JP, Klegeris A. High glucose enhances neurotoxicity and inflammatory cytokine secretion by stimulated human astrocytes. Curr Alzheimer Res. (2017) 14:731–41. doi: 10.2174/1567205014666170117104053
69. Liao Y-H, Zhang G-H, Jia D, Wang P, Qian N-S, He F, et al. Spinal astrocytic activation contributes to mechanical allodynia in a mouse model of type 2 diabetes. Brain Res. (2011) 1368:324–35. doi: 10.1016/j.brainres.2010.10.044
70. Zhang R-X, Li A, Liu B, Wang L, Ren K, Zhang H, et al. IL-1ra alleviates inflammatory hyperalgesia through preventing phosphorylation of NMDA receptor NR-1 subunit in rats. Pain. (2008) 135:232–9. doi: 10.1016/j.pain.2007.05.023
71. Sun Y, Wang J, Liang S-H, Ge J, Lu Y-C, Li J-N, et al. Involvement of the ventrolateral periaqueductal gray matter-central medial thalamic nucleus-basolateral amygdala pathway in neuropathic pain regulation of rats. Front Neuroanat. (2020) 14:32. doi: 10.3389/fnana.2020.00032
72. Guo M, Jiang Z, Chen Y, Wang F, Wang Z. Inflammatory cytokines in midbrain periaqueductal gray contribute to diabetic induced pain hypersensitivity through phosphoinositide 3-kinase/protein kinase B/mammalian target of rapamycin signaling pathway. Korean J Pain. (2021) 34:176–84. doi: 10.3344/kjp.2021.34.2.176
73. Zhang W-J, Wu C-L, Liu J-P. Schwann cells as a target cell for the treatment of cancer pain. Glia. (2023) 71:2309–22. doi: 10.1002/glia.24391
74. Salzer JL. Schwann cell myelination. Cold Spring Harbor Perspect In Biol. (2015) 7:a020529. doi: 10.1101/cshperspect.a020529
75. Ciotu CI, Kistner K, Kaindl U, Millesi F, Weiss T, Radtke C, et al. Schwann cell stimulation induces functional and structural changes in peripheral nerves. Glia. (2023) 71:945–56. doi: 10.1002/glia.24316
76. Zenker J, Ziegler D, Chrast R. Novel pathogenic pathways in diabetic neuropathy. Trends In Neurosciences. (2013) 36:439–49. doi: 10.1016/j.tins.2013.04.008
77. Poitras TM, Munchrath E, Zochodne DW. Neurobiological opportunities in diabetic polyneuropathy. Neurotherapeutics. (2021) 18:2303–23. doi: 10.1007/s13311-021-01138-y
78. Weinberg Sibony R, Segev O, Dor S, Raz I. Overview of oxidative stress and inflammation in diabetes. J Diabetes. (2024) 16:e70014. doi: 10.1111/1753-0407.70014
79. Dey I, Midha N, Singh G, Forsyth A, Walsh SK, Singh B, et al. Diabetic Schwann cells suffer from nerve growth factor and neurotrophin-3 underproduction and poor associability with axons. Glia. (2013) 61:1990–9. doi: 10.1002/glia.22570
80. Cheng Y-C, Chu L-W, Chen J-Y, Hsieh S-L, Chang Y-C, Dai Z-K, et al. Loganin attenuates high glucose-induced schwann cells pyroptosis by inhibiting ROS generation and NLRP3 inflammasome activation. Cells. (2020) 9(9):1948. doi: 10.3390/cells9091948
81. Majd H, Amin S, Ghazizadeh Z, Cesiulis A, Arroyo E, Lankford K, et al. Deriving Schwann cells from hPSCs enables disease modeling and drug discovery for diabetic peripheral neuropathy. Cell Stem Cell. (2023) 30(5):632–47.e10. doi: 10.1016/j.stem.2023.04.006
82. Esposito MF, Malayil R, Hanes M, Deer T. Unique characteristics of the dorsal root ganglion as a target for neuromodulation. Pain Med. (2019) 20:S23–30. doi: 10.1093/pm/pnz012
83. Berta T, Qadri Y, Tan P-H, Ji R-R. Targeting dorsal root ganglia and primary sensory neurons for the treatment of chronic pain. Expert Opin On Ther Targets. (2017) 21:695–703. doi: 10.1080/14728222.2017.1328057
84. Feldman EL, Nave K-A, Jensen TS, Bennett DLH. New horizons in diabetic neuropathy: mechanisms, bioenergetics, and pain. Neuron. (2017) 93:1296–313. doi: 10.1016/j.neuron.2017.02.005
85. Graham RD, Bruns TM, Duan B, Lempka SF. Dorsal root ganglion stimulation for chronic pain modulates Aβ-fiber activity but not C-fiber activity: A computational modeling study. Clin Neurophysiol. (2019) 130:941–51. doi: 10.1016/j.clinph.2019.02.016
86. Lempka SF, Patil PG. Innovations in spinal cord stimulation for pain. Curr Opin BioMed Eng. (2018) 8:51–60. doi: 10.1016/j.cobme.2018.10.005
87. Xu Z-Z, Kim YH, Bang S, Zhang Y, Berta T, Wang F, et al. Inhibition of mechanical allodynia in neuropathic pain by TLR5-mediated A-fiber blockade. Nat Med. (2015) 21:1326–31. doi: 10.1038/nm.3978
88. Gautam M, Yamada A, Yamada AI, Wu Q, Kridsada K, Ling J, et al. Distinct local and global functions of mouse Aβ low-threshold mechanoreceptors in mechanical nociception. Nat Commun. (2024) 15:2911. doi: 10.1038/s41467-024-47245-0
89. Moran MM. TRP channels as potential drug targets. Annu Rev Pharmacol Toxicol. (2018) 58:309–30. doi: 10.1146/annurev-pharmtox-010617-052832
90. Lu J, Wu K, Sha X, Lin J, Chen H, Yu Z. TRPV1 alleviates APOE4-dependent microglial antigen presentation and T cell infiltration in Alzheimer’s disease. Transl Neurodegener. (2024) 13:52. doi: 10.1186/s40035-024-00445-6
91. Li L, Ma L, Luo Z, Wei X, Zhao Y, Zhou C, et al. Lack of TRPV1 aggravates obesity-associated hypertension through the disturbance of mitochondrial Ca2+ homeostasis in brown adipose tissue. Hypertens Res. (2022) 45:789–801. doi: 10.1038/s41440-021-00842-8
92. Shen Y-R, Cheng L, Zhang D-F. TRPV1: A novel target for the therapy of diabetes and diabetic complications. Eur J Pharmacol. (2024) 984:177021. doi: 10.1016/j.ejphar.2024.177021
93. Cao S, Li Q, Hou J, Li Z, Cao X, Liu X, et al. Intrathecal TRPM8 blocking attenuates cold hyperalgesia via PKC and NF-κB signaling in the dorsal root ganglion of rats with neuropathic pain. J Pain Res. (2019) 12:1287–96. doi: 10.2147/JPR.S197168
94. Du L, Zhu J, Liu S, Yang W, Hu X, Zhang W, et al. Transient receptor potential melastatin 8 contributes to the interleukin-33-mediated cold allodynia in a mouse model of neuropathic pain. Pain. (2025) 166:347–59. doi: 10.1097/j.pain.0000000000003346
95. Abdelkader NF, Ibrahim SM, Moustafa PE, Elbaset MA. Inosine mitigated diabetic peripheral neuropathy via modulating GLO1/AGEs/RAGE/NF-κB/Nrf2 and TGF-β/PKC/TRPV1 signaling pathways. Biomedicine Pharmacotherapy = Biomedecine Pharmacotherapie. (2022) 145:112395. doi: 10.1016/j.biopha.2021.112395
96. Pabbidi MR, Premkumar LS. Role of transient receptor potential channels trpv1 and trpm8 in diabetic peripheral neuropathy. J Diabetes Treat. (2017) 2017(4):029.
97. Zan Y, Kuai C-X, Qiu Z-X, Huang F. Berberine ameliorates diabetic neuropathy: TRPV1 modulation by PKC pathway. Am J Chin Med. (2017) 45:1709–23. doi: 10.1142/S0192415X17500926
98. Henrich F, Magerl W, Klein T, Greffrath W, Treede R-D. Capsaicin-sensitive C- and A-fibre nociceptors control long-term potentiation-like pain amplification in humans. Brain. (2015) 138:2505–20. doi: 10.1093/brain/awv108
99. Xie Y-K, Luo H, Zhang S-X, Chen X-Y, Guo R, Qiu X-Y, et al. GPR177 in A-fiber sensory neurons drives diabetic neuropathic pain via WNT-mediated TRPV1 activation. Sci Trans Med. (2022) 14:eabh2557. doi: 10.1126/scitranslmed.abh2557
100. Khan A, Wang F, Shal B, Khan AU, Zahra SS, Haq IU, et al. Anti-neuropathic pain activity of Ajugarin-I via activation of Nrf2 signaling and inhibition of TRPV1/TRPM8 nociceptors in STZ-induced diabetic neuropathy. Pharmacol Res. (2022) 183:106392. doi: 10.1016/j.phrs.2022.106392
101. Nguyen PT, Yarov-Yarovoy V. Towards structure-guided development of pain therapeutics targeting voltage-gated sodium channels. Front In Pharmacol. (2022) 13:842032. doi: 10.3389/fphar.2022.842032
102. Ma RSY, Kayani K, Whyte-Oshodi D, Whyte-Oshodi A, Nachiappan N, Gnanarajah S, et al. Voltage gated sodium channels as therapeutic targets for chronic pain. J Pain Res. (2019) 12:2709–22. doi: 10.2147/JPR.S207610
103. Bennett DL, Clark AJ, Huang J, Waxman SG, Dib-Hajj SD. The role of voltage-gated sodium channels in pain signaling. Physiol Rev. (2019) 99:1079–151. doi: 10.1152/physrev.00052.2017
104. Wood JN, Iseppon F. Sodium channels. Brain Neurosci Adv. (2018) 2:2398212818810684. doi: 10.1177/2398212818810684
105. Suter MR, Kirschmann G, Laedermann CJ, Abriel H, Decosterd I. Rufinamide attenuates mechanical allodynia in a model of neuropathic pain in the mouse and stabilizes voltage-gated sodium channel inactivated state. Anesthesiology. (2013) 118:160–72. doi: 10.1097/ALN.0b013e318278cade
106. Sun W, Miao B, Wang X-C, Duan J-H, Wang W-T, Kuang F, et al. Reduced conduction failure of the main axon of polymodal nociceptive C-fibres contributes to painful diabetic neuropathy in rats. Brain. (2012) 135:359–75. doi: 10.1093/brain/awr345
107. Chen X, Levine JD. Hyper-responsivity in a subset of C-fiber nociceptors in a model of painful diabetic neuropathy in the rat. Neuroscience. (2001) 102:185–92. doi: 10.1016/S0306-4522(00)00454-1
108. Hong S, Wiley JW. Altered expression and function of sodium channels in large DRG neurons and myelinated A-fibers in early diabetic neuropathy in the rat. Biochem Biophys Res Commun. (2006) 339:652–60. doi: 10.1016/j.bbrc.2005.11.057
109. Lai J, Porreca F, Hunter JC, Gold MS. Voltage-gated sodium channels and hyperalgesia. Annu Rev Pharmacol Toxicol. (2004) 44:371–97. doi: 10.1146/annurev.pharmtox.44.101802.121627
110. Hong S, Morrow TJ, Paulson PE, Isom LL, Wiley JW. Early painful diabetic neuropathy is associated with differential changes in tetrodotoxin-sensitive and -resistant sodium channels in dorsal root ganglion neurons in the rat. J Biol Chem. (2004) 279:29341–50. doi: 10.1074/jbc.M404167200
111. Hudmon A, Choi J-S, Tyrrell L, Black JA, Rush AM, Waxman SG, et al. Phosphorylation of sodium channel Na(v)1.8 by p38 mitogen-activated protein kinase increases current density in dorsal root ganglion neurons. J Neuroscience: Off J Soc For Neurosci. (2008) 28:3190–201. doi: 10.1523/JNEUROSCI.4403-07.2008
112. He W-Y, Zhang B, Xiong Q-M, Yang C-X, Zhao W-C, He J, et al. Intrathecal administration of rapamycin inhibits the phosphorylation of DRG Nav1.8 and attenuates STZ-induced painful diabetic neuropathy in rats. Neurosci Lett. (2016) 619:21–8. doi: 10.1016/j.neulet.2016.02.064
113. Zhang X-F, Shieh C-C, Chapman ML, Matulenko MA, Hakeem AH, Atkinson RN, et al. A-887826 is a structurally novel, potent and voltage-dependent Na(v)1.8 sodium channel blocker that attenuates neuropathic tactile allodynia in rats. Neuropharmacology. (2010) 59:201–7. doi: 10.1016/j.neuropharm.2010.05.009
114. de Macedo FHP, Aires RD, Fonseca EG, Ferreira RCM, MaChado DPD, Chen L, et al. TNF-α mediated upregulation of NaV1.7 currents in rat dorsal root ganglion neurons is independent of CRMP2 SUMOylation. Mol Brain. (2019) 12:117. doi: 10.1186/s13041-019-0538-0
115. Meng B, Shen L-L, Shi X-T, Gong Y-S, Fan X-F, Li J, et al. Effects of curcumin on TTX-R sodium currents of dorsal root ganglion neurons in type 2 diabetic rats with diabetic neuropathic pain. Neurosci Letters. (2015) 605:59–64. doi: 10.1016/j.neulet.2015.08.011
116. Ren Y-S, Qian N-S, Tang Y, Liao Y-H, Yang Y-L, Dou K-F, et al. Sodium channel Nav1.6 is up-regulated in the dorsal root ganglia in a mouse model of type 2 diabetes. Brain Res Bull. (2012) 87:244–9. doi: 10.1016/j.brainresbull.2011.10.015
117. Tan AM, Samad OA, Dib-Hajj SD, Waxman SG. Virus-mediated knockdown of nav1.3 in dorsal root ganglia of STZ-induced diabetic rats alleviates tactile allodynia. Mol Med. (2015) 21:544–52. doi: 10.2119/molmed.2015.00063
118. Zhou Z-Y, Wang J-Y, Li Z-X, Zheng H-L, Zhou Y-N, Huang L-N, et al. Branched-chain amino acids deficiency promotes diabetic neuropathic pain through upregulating LAT1 and inhibiting kv1. 2 Channel. Adv Sci (Weinh). (2024) 11:e2402086. doi: 10.1002/advs.202402086
119. Wang X-C, Wang S, Zhang M, Gao F, Yin C, Li H, et al. [amp]]Alpha;-Dendrotoxin-sensitive Kv1 channels contribute to conduction failure of polymodal nociceptive C-fibers from rat coccygeal nerve. J Neurophysiol. (2016) 115:947–57. doi: 10.1152/jn.00786.2014
120. Yu T, Li L, Liu H, Li H, Liu Z, Li Z. KCNQ2/3/5 channels in dorsal root ganglion neurons can be therapeutic targets of neuropathic pain in diabetic rats. Mol Pain. (2018) 14:1744806918793229. doi: 10.1177/1744806918793229
121. Cao X-H, Byun H-S, Chen S-R, Cai Y-Q, Pan H-L. Reduction in voltage-gated K+ channel activity in primary sensory neurons in painful diabetic neuropathy: role of brain-derived neurotrophic factor. J Neurochemistry. (2010) 114:1460–75. doi: 10.1111/j.1471-4159.2010.06863.x
122. Chen L, Wang H, Xing J, Shi X, Huang H, Huang J, et al. Silencing P2X7R alleviates diabetic neuropathic pain involving TRPV1 via PKCϵ/P38MAPK/NF-κB signaling pathway in rats. Int J Mol Sci. (2022) 23(22):14141. doi: 10.3390/ijms232214141
123. Aydın B, Nazıroğlu M. Involvement of TRPM7 channel on the induction of diabetic neuropathic pain in mice: protective role of selenium and curcumin. Biol Trace Elem Res. (2023) 201:2377–95. doi: 10.1007/s12011-022-03518-7
124. Osmanlıoğlu HÖ, Nazıroğlu M. Resveratrol modulates diabetes-induced neuropathic pain, apoptosis, and oxidative neurotoxicity in mice through TRPV4 channel inhibition. Mol Neurobiology. (2024) 61:7269–86. doi: 10.1007/s12035-024-04311-4
125. Yazğan B, Yazğan Y, Nazıroğlu M. Alpha-lipoic acid modulates the diabetes mellitus-mediated neuropathic pain via inhibition of the TRPV1 channel, apoptosis, and oxidative stress in rats. J Bioenerg Biomembr. (2023) 55:179–93. doi: 10.1007/s10863-023-09971-w
126. Kahya MC, Nazıroğlu M, Övey İS. Modulation of diabetes-induced oxidative stress, apoptosis, and ca2+ Entry through TRPM2 and TRPV1 channels in dorsal root ganglion and hippocampus of diabetic rats by melatonin and selenium. Mol Neurobiology. (2017) 54:2345–60. doi: 10.1007/s12035-016-9727-3
127. Chen X, Duan Y, Riley AM, Welch MA, White FA, Grant MB, et al. Long-term diabetic microenvironment augments the decay rate of capsaicin-induced currents in mouse dorsal root ganglion neurons. Molecules (Basel Switzerland). (2019) 24:775. doi: 10.3390/molecules24040775
128. Hong S, Agresta L, Guo C, Wiley JW. The TRPV1 receptor is associated with preferential stress in large dorsal root ganglion neurons in early diabetic sensory neuropathy. J Neurochemistry. (2008) 105:1212–22. doi: 10.1111/j.1471-4159.2008.05220.x
129. Cao X-H, Byun HS, Chen S-R, Pan H-L. Diabetic neuropathy enhances voltage-activated Ca2+ channel activity and its control by M4 muscarinic receptors in primary sensory neurons. J Neurochemistry. (2011) 119:594–603. doi: 10.1111/j.1471-4159.2011.07456.x
130. Greene DL, Hoshi N. Modulation of Kv7 channels and excitability in the brain. Cell Mol Life Sciences: CMLS. (2017) 74:495–508. doi: 10.1007/s00018-016-2359-y
131. Djouhri L, Zeidan A, Abd-El-Aleem SA. Changes in expression of Kv7.5 and Kv7.2 channels in dorsal root ganglion neurons in the streptozotocin rat model of painful diabetic neuropathy. Neurosci Lett. (2020) 736:135277. doi: 10.1016/j.neulet.2020.135277
132. Djouhri L, Malki MI, Zeidan A, Nagi K, Smith T. Activation of Kv7 channels with the anticonvulsant retigabine alleviates neuropathic pain behaviour in the streptozotocin rat model of diabetic neuropathy. J Drug Targeting. (2019) 27:1118–26. doi: 10.1080/1061186X.2019.1608552
133. Everill B, Kocsis JD. Reduction in potassium currents in identified cutaneous afferent dorsal root ganglion neurons after axotomy. J Neurophysiol. (1999) 82:700–8. doi: 10.1152/jn.1999.82.2.700
134. Yang EK, Takimoto K, Hayashi Y, de Groat WC, Yoshimura N. Altered expression of potassium channel subunit mRNA and alpha-dendrotoxin sensitivity of potassium currents in rat dorsal root ganglion neurons after axotomy. Neuroscience. (2004) 123:867–74. doi: 10.1016/j.neuroscience.2003.11.014
135. Huang H-Y, Cheng J-K, Shih Y-H, Chen P-H, Wang C-L, Tsaur M-L. Expression of A-type K channel alpha subunits Kv 4.2 and Kv 4.3 in rat spinal lamina II excitatory interneurons and colocalization with pain-modulating molecules. Eur J Neurosci. (2005) 22:1149–57. doi: 10.1111/j.1460-9568.2005.04283.x
136. Sakai A, Saitow F, Maruyama M, Miyake N, Miyake K, Shimada T, et al. MicroRNA cluster miR-17-92 regulates multiple functionally related voltage-gated potassium channels in chronic neuropathic pain. Nat Commun. (2017) 8:16079. doi: 10.1038/ncomms16079
137. Illes P. P2X7 receptors amplify CNS damage in neurodegenerative diseases. Int J Mol Sci. (2020) 21(17):5996. doi: 10.3390/ijms21175996
138. Zhang H, Xin W, Dougherty PM. Synaptically evoked glutamate transporter currents in Spinal Dorsal Horn Astrocytes. Mol Pain. (2009) 5:36. doi: 10.1186/1744-8069-5-36
139. Lundborg C, Westerlund A, Björklund U, Biber B, Hansson E. Ifenprodil restores GDNF-evoked Ca(2+) signalling and Na(+)/K(+) -ATPase expression in inflammation-pretreated astrocytes. J Neurochemistry. (2011) 119:686–96. doi: 10.1111/j.1471-4159.2011.07465.x
140. Ou M, Chen Y, Liu J, Zhang D, Yang Y, Shen J, et al. Spinal astrocytic MeCP2 regulates Kir4.1 for the maintenance of chronic hyperalgesia in neuropathic pain. Prog In Neurobiol. (2023) 224:102436. doi: 10.1016/j.pneurobio.2023.102436
141. Ohno Y, Kinboshi M, Shimizu S. Inwardly rectifying potassium channel kir4.1 as a novel modulator of BDNF expression in astrocytes. Int J Mol Sci. (2018) 19(11):3313. doi: 10.3390/ijms19113313
142. Ju YH, Cho J, Park J-Y, Kim H, Hong E-B, Park KD, et al. Tonic excitation by astrocytic GABA causes neuropathic pain by augmenting neuronal activity and glucose metabolism. Exp Mol Med. (2024) 56:1193–205. doi: 10.1038/s12276-024-01232-z
143. Toth AB, Hori K, Novakovic MM, Bernstein NG, Lambot L, Prakriya M. CRAC channels regulate astrocyte Ca2+ signaling and gliotransmitter release to modulate hippocampal GABAergic transmission. Sci Signaling. (2019) 12(582):eaaw5450. doi: 10.1126/scisignal.aaw5450
144. Birla H, Xia J, Gao X, Zhao H, Wang F, Patel S, et al. Toll-like receptor 4 activation enhances Orai1-mediated calcium signal promoting cytokine production in spinal astrocytes. Cell Calcium. (2022) 105:102619. doi: 10.1016/j.ceca.2022.102619
145. Wu X-F, Liu W-T, Liu Y-P, Huang Z-J, Zhang Y-K, Song X-J. Reopening of ATP-sensitive potassium channels reduces neuropathic pain and regulates astroglial gap junctions in the rat spinal cord. Pain. (2011) 152:2605–15. doi: 10.1016/j.pain.2011.08.003
146. Bardoni R, Ghirri A, Zonta M, Betelli C, Vitale G, Ruggieri V, et al. Glutamate-mediated astrocyte-to-neuron signalling in the rat dorsal horn. J Physiol. (2010) 588:831–46. doi: 10.1113/jphysiol.2009.180570
147. Zhou J, Zhang X, Zhou Y, Wu B, Tan Z-Y. Up-regulation of P2X7 receptors contributes to spinal microglial activation and the development of pain induced by bmK-I. Neurosci Bull. (2019) 35:624–36. doi: 10.1007/s12264-019-00345-0
148. Saegusa H, Kurihara T, Zong S, Kazuno A, Matsuda Y, Nonaka T, et al. Suppression of inflammatory and neuropathic pain symptoms in mice lacking the N-type Ca2+ channel. EMBO J. (2001) 20:2349–56. doi: 10.1093/emboj/20.10.2349
149. Saegusa H, Tanabe T. N-type voltage-dependent Ca2+ channel in non-excitable microglial cells in mice is involved in the pathophysiology of neuropathic pain. Biochem Biophys Res Commun. (2014) 450:142–7. doi: 10.1016/j.bbrc.2014.05.103
150. Nadzirin IB, Fortuny-Gomez A, Ngum N, Richards D, Ali S, Searcey M, et al. Taspine is a natural product that suppresses P2X4 receptor activity via phosphoinositide 3-kinase inhibition. Br J Pharmacol. (2021) 178:4859–72. doi: 10.1111/bph.15663
151. Staal RGW, Khayrullina T, Zhang H, Davis S, Fallon SM, Cajina M, et al. Inhibition of the potassium channel KCa3.1 by senicapoc reverses tactile allodynia in rats with peripheral nerve injury. Eur J Pharmacol. (2017) 795:1–7. doi: 10.1016/j.ejphar.2016.11.031
152. Imari K, Harada Y, Zhang J, Mori Y, Hayashi Y. KCNMB3 in spinal microglia contributes to the generation and maintenance of neuropathic pain in mice. Int J Mol Med. (2019) 44:1585–93. doi: 10.3892/ijmm.2019.4279
153. Petrenko AB, Yamakura T, Askalany AR, Kohno T, Sakimura K, Baba H. Effects of ketamine on acute somatic nociception in wild-type and N-methyl-D-aspartate (NMDA) receptor epsilon1 subunit knockout mice. Neuropharmacology. (2006) 50:741–7. doi: 10.1016/j.neuropharm.2005.11.019
154. Hayashi Y, Kawaji K, Sun L, Zhang X, Koyano K, Yokoyama T, et al. Microglial Ca(2+)-activated K(+) channels are possible molecular targets for the analgesic effects of S-ketamine on neuropathic pain. J Neuroscience: Off J Soc For Neurosci. (2011) 31:17370–82. doi: 10.1523/JNEUROSCI.4152-11.2011
155. Shin SM, Itson-Zoske B, Fan F, Gani U, Rahman M, Hogan QH, et al. Peripheral sensory neurons and non-neuronal cells express functional Piezo1 channels. Mol Pain. (2023) 19:17448069231174315. doi: 10.1177/17448069231174315
156. Suttinont C, Maeno K, Yano M, Sato-Numata K, Numata T, Tsutsumi M. Role of piezo2 in schwann cell volume regulation and its impact on neurotrophic release regulation. Cell Physiol Biochemistry: Int J Exp Cell Physiology Biochemistry Pharmacol. (2024) 58:292–310. doi: 10.33594/000000713
157. Sobko A, Peretz A, Shirihai O, Etkin S, Cherepanova V, Dagan D, et al. Heteromultimeric delayed-rectifier K+ channels in schwann cells: developmental expression and role in cell proliferation. J Neuroscience: Off J Soc For Neurosci. (1998) 18:10398–408. doi: 10.1523/JNEUROSCI.18-24-10398.1998
158. Procacci NM, Hastings RL, Aziz AA, Christiansen NM, Zhao J, DeAngeli C, et al. Kir4.1 is specifically expressed and active in non-myelinating Schwann cells. Glia. (2023) 71:926–44. doi: 10.1002/glia.24315
159. Zemel BM, Ritter DM, Covarrubias M, Muqeem T. A-type KV channels in dorsal root ganglion neurons: diversity, function, and dysfunction. Front In Mol Neurosci. (2018) 11:253. doi: 10.3389/fnmol.2018.00253
160. Kuo Y-L, Cheng J-K, Hou W-H, Chang Y-C, Du P-H, Jian J-J, et al. K+ Channel modulatory subunits KChIP and DPP participate in kv4-mediated mechanical pain control. J Neuroscience: Off J Soc For Neurosci. (2017) 37:4391–404. doi: 10.1523/JNEUROSCI.1619-16.2017
161. Lv Y-Y, Wang H, Fan H-T, Xu T, Xin W-J, Guo R-X. SUMOylation of Kir7.1 participates in neuropathic pain through regulating its membrane expression in spinal cord neurons. CNS Neurosci Ther. (2022) 28:1259–67. doi: 10.1111/cns.13871
162. Walsh KB. Screening technologies for inward rectifier potassium channels: discovery of new blockers and activators. SLAS Discovery. (2020) 25:420–33. doi: 10.1177/2472555220905558
163. Hameed S. Nav1.7 and Nav1.8: Role in the pathophysiology of pain. Mol Pain. (2019) 15:1744806919858801. doi: 10.1177/1744806919858801
164. Osteen JD, Immani S, Tapley TL, Indersmitten T, Hurst NW, Healey T, et al. Pharmacology and mechanism of action of suzetrigine, a potent and selective naV1.8 pain signal inhibitor for the treatment of moderate to severe pain. Pain Ther. (2025) 14(2):655–74. doi: 10.1007/s40122-024-00697-0
165. Deng L, Dourado M, Reese RM, Huang K, Shields SD, Stark KL, et al. Nav1.7 is essential for nociceptor action potentials in the mouse in a manner independent of endogenous opioids. Neuron. (2023) 111(17):2642–59.e13. doi: 10.1016/j.neuron.2023.05.024
166. Huang J, Mis MA, Tanaka B, Adi T, Estacion M, Liu S, et al. Atypical changes in DRG neuron excitability and complex pain phenotype associated with a Nav1.7 mutation that massively hyperpolarizes activation. Sci Rep. (2018) 8:1811. doi: 10.1038/s41598-018-20221-7
167. Lee ES, Ryu JH, Kim EJ, Kim GT, Cho YW, Park HJ, et al. Lamotrigine increases intracellular Ca(2+) levels and Ca(2+)/calmodulin-dependent kinase II activation in mouse dorsal root ganglion neurones. Acta Physiol (Oxf). (2013) 207:397–404. doi: 10.1111/apha.12034
168. Chang MC, Yang S. Diabetic peripheral neuropathy essentials: a narrative review. Ann Palliat Med. (2023) 12:390–8. doi: 10.21037/apm-22-693
169. Zhang R, Lai M, Wang D. Psychologic impacts on diabetic neuropathic pain. Curr Pain Headache Rep. (2022) 26:423–7. doi: 10.1007/s11916-022-01040-y
170. Rohde C, Finnerup NB, Schmitz N, Jensen TS, Thomsen RW, Østergaard SD. Is diabetic neuropathy associated with increased risk of developing mental disorders? Eur J Endocrinol. (2022) 186:K39–43. doi: 10.1530/EJE-21-1168
171. Dewanjee S, Das S, Das AK, Bhattacharjee N, Dihingia A, Dua TK, et al. Molecular mechanism of diabetic neuropathy and its pharmacotherapeutic targets. Eur J Pharmacol. (2018) 833:472–523. doi: 10.1016/j.ejphar.2018.06.034
172. Miyashita A, Kobayashi M, Yokota T, Zochodne DW. Diabetic polyneuropathy: new strategies to target sensory neurons in dorsal root ganglia. Int J Mol Sci. (2023) 24(6):5977. doi: 10.3390/ijms24065977
173. Freeman R. New and developing drugs for the treatment of neuropathic pain in diabetes. Curr Diabetes Rep. (2013) 13:500–8. doi: 10.1007/s11892-013-0396-6
174. Yang M, Qian C, Liu Y. Suboptimal treatment of diabetic peripheral neuropathic pain in the United States. Pain Med. (2015) 16:2075–83. doi: 10.1111/pme.12845
175. Blair HA. Capsaicin 8% Dermal patch: A review in peripheral neuropathic pain. Drugs. (2018) 78:1489–500. doi: 10.1007/s40265-018-0982-7
176. Tesfaye S, Sloan G, Petrie J, White D, Bradburn M, Julious S, et al. Comparison of amitriptyline supplemented with pregabalin, pregabalin supplemented with amitriptyline, and duloxetine supplemented with pregabalin for the treatment of diabetic peripheral neuropathic pain (OPTION-DM): a multicentre, double-blind, randomised crossover trial. Lancet (London England). (2022) 400:680–90. doi: 10.1016/S0140-6736(22)01472-6
177. Gaskell H, Derry S, Stannard C, Moore RA. Oxycodone for neuropathic pain in adults. Cochrane Database Systematic Rev. (2016) 7:CD010692. doi: 10.1002/14651858.CD010692.pub3
178. Sommer C, Klose P, Welsch P, Petzke F, Häuser W. Opioids for chronic non-cancer neuropathic pain. An updated systematic review and meta-analysis of efficacy, tolerability and safety in randomized placebo-controlled studies of at least 4 weeks duration. Eur J Pain. (2020) 24(1):3–18. doi: 10.1002/ejp.1494
179. Pesa J, Meyer R, Quock TP, Rattana SK, Mody SH. Opioid utilization patterns among medicare patients with diabetic peripheral neuropathy. Am Health Drug Benefits. (2013) 6:188–96.
180. Scharnagel R, Kaiser U, Schütze A, Heineck R, Gossrau G, Sabatowski R. Chronic non-cancer-related pain. Long-term treatment with rapid-release and short-acting opioids in the context of misuse and dependency. Schmerz. (2013) 27(1):7–19. doi: 10.1007/s00482-012-1278-6
181. Kim SE, Chung G, Kim SK. Phytochemical-based therapeutics from traditional eastern medicine: analgesic effects and ion channel modulation. Front Pain Res (Lausanne). (2025) 6:1537154. doi: 10.3389/fpain.2025.1537154
182. Kim N, Chung G, Son SR, Park JH, Lee YH, Park KT, et al. Magnolin inhibits paclitaxel-induced cold allodynia and ERK1/2 activation in mice. Plants (Basel). (2023) 12(12):2283. doi: 10.3390/plants12122283
183. Wang S, Taledaohan A, Tuohan M, Zhang J, Li Y, Song W, et al. Jinmaitong alleviates diabetic neuropathic pain by inhibiting JAK2/STAT3 signaling in microglia of diabetic rats. J Ethnopharmacol. (2024) 333:118442. doi: 10.1016/j.jep.2024.118442
184. Wang R, Qiu Z, Wang G, Hu Q, Shi N, Zhang Z, et al. Quercetin attenuates diabetic neuropathic pain by inhibiting mTOR/p70S6K pathway-mediated changes of synaptic morphology and synaptic protein levels in spinal dorsal horn of db/db mice. Eur J Pharmacol. (2020) 882:173266. doi: 10.1016/j.ejphar.2020.173266
185. Guan S, Shen Y, Ge H, Xiong W, He L, Liu L, et al. Dihydromyricetin alleviates diabetic neuropathic pain and depression comorbidity symptoms by inhibiting P2X7 receptor. Front In Psychiatry. (2019) 10:770. doi: 10.3389/fpsyt.2019.00770
186. Sharma S, Chopra K, Kulkarni SK. Effect of insulin and its combination with resveratrol or curcumin in attenuation of diabetic neuropathic pain: participation of nitric oxide and TNF-alpha. Phytother Res. (2007) 21:278–83. doi: 10.1002/ptr.v21:3
187. Jia T, Rao J, Zou L, Zhao S, Yi Z, Wu B, et al. Nanoparticle-encapsulated curcumin inhibits diabetic neuropathic pain involving the P2Y12 receptor in the dorsal root ganglia. Front In Neurosci. (2017) 11:755. doi: 10.3389/fnins.2017.00755
188. Zhao X, Li X-L, Liu X, Wang C, Zhou D-S, Ma Q, et al. Antinociceptive effects of fisetin against diabetic neuropathic pain in mice: Engagement of antioxidant mechanisms and spinal GABAA receptors. Pharmacol Res. (2015) 102:286–97. doi: 10.1016/j.phrs.2015.10.007
189. Jesus CHA, Redivo DDB, Gasparin AT, Sotomaior BB, de Carvalho MC, Genaro K, et al. Cannabidiol attenuates mechanical allodynia in streptozotocin-induced diabetic rats via serotonergic system activation through 5-HT1A receptors. Brain Res. (2019) 1715:156–64. doi: 10.1016/j.brainres.2019.03.014
190. Li Z, Gan Y, Kang T, Zhao Y, Huang T, Chen Y, et al. Camphor attenuates hyperalgesia in neuropathic pain models in mice. J Pain Res. (2023) 16:785–95. doi: 10.2147/JPR.S398607
191. Hu P, Mei Q-Y, Ma L, Cui W-G, Zhou W-H, Zhou D-S, et al. Secoisolariciresinol diglycoside, a flaxseed lignan, exerts analgesic effects in a mouse model of type 1 diabetes: Engagement of antioxidant mechanism. Eur J Pharmacol. (2015) 767:183–92. doi: 10.1016/j.ejphar.2015.10.024
192. Tiwari V, Kuhad A, Chopra K. Emblica officinalis corrects functional, biochemical and molecular deficits in experimental diabetic neuropathy by targeting the oxido-nitrosative stress mediated inflammatory cascade. Phytother Res. (2011) 25:1527–36. doi: 10.1002/ptr.3440
193. Kandhare AD, Raygude KS, Ghosh P, Ghule AE, Bodhankar SL. Neuroprotective effect of naringin by modulation of endogenous biomarkers in streptozotocin induced painful diabetic neuropathy. Fitoterapia. (2012) 83:650–9. doi: 10.1016/j.fitote.2012.01.010
194. Kajal A, Singh R. Coriandrum sativum improve neuronal function via inhibition of oxidative/nitrosative stress and TNF-α in diabetic neuropathic rats. J Ethnopharmacol. (2020) 263:112959. doi: 10.1016/j.jep.2020.112959
195. Kishore L, Kaur N, Singh R. Effect of Kaempferol isolated from seeds of Eruca sativa on changes of pain sensitivity in Streptozotocin-induced diabetic neuropathy. Inflammopharmacology. (2018) 26(4):993–1003. doi: 10.1007/s10787-017-0416-2
196. Kaeidi A, Esmaeili-Mahani S, Sheibani V, Abbasnejad M, Rasoulian B, Hajializadeh Z, et al. Olive (Olea europaea L.) leaf extract attenuates early diabetic neuropathic pain through prevention of high glucose-induced apoptosis: in vitro and in vivo studies. J Ethnopharmacol. (2011) 136:188–96. doi: 10.1016/j.jep.2011.04.038
197. Hajializadeh Z, Nasri S, Kaeidi A, Sheibani V, Rasoulian B, Esmaeili-Mahani S. Inhibitory effect of Thymus caramanicus Jalas on hyperglycemia-induced apoptosis in in vitro and in vivo models of diabetic neuropathic pain. J Ethnopharmacol. (2014) 153:596–603. doi: 10.1016/j.jep.2014.02.049
198. Zhao W-C, Zhang B, Liao M-J, Zhang W-X, He W-Y, Wang H-B, et al. Curcumin ameliorated diabetic neuropathy partially by inhibition of NADPH oxidase mediating oxidative stress in the spinal cord. Neurosci Letters. (2014) 560:81–5. doi: 10.1016/j.neulet.2013.12.019
199. Ranjithkumar R, Prathab Balaji S, Balaji B, Ramesh RV, Ramanathan M. Standardized Aqueous Tribulus terristris (nerunjil) extract attenuates hyperalgesia in experimentally induced diabetic neuropathic pain model: role of oxidative stress and inflammatory mediators. Phytother Res. (2013) 27:1646–57. doi: 10.1002/ptr.4915
200. Shivam, Gupta AK. Neuroprotective effects of isolated mangiferin from swertia chirayita leaves regulating oxidative pathway on streptozotocin-induced diabetic neuropathy in experimental rats. Cent Nerv Syst Agents Med Chem. (2024) 24:182–95. doi: 10.2174/0118715249255977231213053039
201. Li L, Sheng X, Zhao S, Zou L, Han X, Gong Y, et al. Nanoparticle-encapsulated emodin decreases diabetic neuropathic pain probably via a mechanism involving P2X3 receptor in the dorsal root ganglia. Purinergic Signalling. (2017) 13:559–68. doi: 10.1007/s11302-017-9583-2
202. Chahbi Z, Lahmar B, Hadri SE, Abainou L, Kaddouri S, Qacif H, et al. The prevalence of painful diabetic neuropathy in 300 Moroccan diabetics. Pan Afr Med J. (2018) 31:158. doi: 10.11604/pamj.2018.31.158.14687
203. Christensen DH, Knudsen ST, Gylfadottir SS, Christensen LB, Nielsen JS, Beck-Nielsen H, et al. Metabolic factors, lifestyle habits, and possible polyneuropathy in early type 2 diabetes: A nationwide study of 5,249 patients in the danish centre for strategic research in type 2 diabetes (DD2) cohort. Diabetes Care. (2020) 43:1266–75. doi: 10.2337/dc19-2277
204. Ponirakis G, Elhadd T, Al Ozairi E, Brema I, Chinnaiyan S, Taghadom E, et al. Prevalence and risk factors for diabetic peripheral neuropathy, neuropathic pain and foot ulceration in the Arabian Gulf region. J Diabetes Investig. (2022) 13:1551–9. doi: 10.1111/jdi.13815
205. Cardinez N, Lovblom LE, Bai J-W, Lewis E, Abraham A, Scarr D, et al. Sex differences in neuropathic pain in longstanding diabetes: Results from the Canadian Study of Longevity in Type 1 Diabetes. J Diabetes Complications. (2018) 32:660–4. doi: 10.1016/j.jdiacomp.2018.05.001
206. Abraham A, Barnett C, Katzberg HD, Lovblom LE, Perkins BA, Bril V. Sex differences in neuropathic pain intensity in diabetes. J Neurological Sci. (2018) 388:103–6. doi: 10.1016/j.jns.2018.03.008
207. Meng W, Deshmukh HA, Donnelly LA, Torrance N, Colhoun HM, Palmer CNA, et al. A genome-wide association study provides evidence of sex-specific involvement of chr1p35.1 (ZSCAN20-TLR12P) and chr8p23.1 (HMGB1P46) with diabetic neuropathic pain. EBioMedicine. (2015) 2:1386–93. doi: 10.1016/j.ebiom.2015.08.001
208. Belfer I. Sex-specific genetic control of diabetic neuropathic pain suggests subsequent development of men-only and women-only analgesic strategies. EBioMedicine. (2015) 2:1280. doi: 10.1016/j.ebiom.2015.08.038
209. O’Brien JA, McGuire HM, Shinko D, Fazekas de St Groth B, Russo MA, Bailey D, et al. T lymphocyte and monocyte subsets are dysregulated in type 1 diabetes patients with peripheral neuropathic pain. Brain Behav Immun Health. (2021) 15:100283. doi: 10.1016/j.bbih.2021.100283
210. Sorge RE, Mapplebeck JCS, Rosen S, Beggs S, Taves S, Alexander JK, et al. Different immune cells mediate mechanical pain hypersensitivity in male and female mice. Nat Neurosci. (2015) 18:1081–3. doi: 10.1038/nn.4053
211. Scotland RS, Stables MJ, Madalli S, Watson P, Gilroy DW. Sex differences in resident immune cell phenotype underlie more efficient acute inflammatory responses in female mice. Blood. (2011) 118:5918–27. doi: 10.1182/blood-2011-03-340281
212. Kuhn JA, Vainchtein ID, Braz J, Hamel K, Bernstein M, Craik V, et al. Regulatory T-cells inhibit microglia-induced pain hypersensitivity in female mice. ELife. (2021) 10:e69056. doi: 10.7554/eLife.69056
213. Zhu X, Li X, Liu S, Zhao YH, Liu XR, Liu XY, et al. Enhanced interleukin-16-CD4 signaling in CD3 T cell mediates neuropathic pain via activating astrocytes in female mice. Neuropharmacology. (2024) 259:110115. doi: 10.1016/j.neuropharm.2024.110115
214. Coraggio V, Guida F, Boccella S, Scafuro M, Paino S, Romano D, et al. Neuroimmune-driven neuropathic pain establishment: A focus on gender differences. Int J Mol Sci. (2018) 19(1):281. doi: 10.3390/ijms19010281
215. Stauderman KA. CRAC channels as targets for drug discovery and development. Cell Calcium. (2018) 74:147–59. doi: 10.1016/j.ceca.2018.07.005
216. Tsujikawa S, DeMeulenaere KE, Centeno MV, Ghazisaeidi S, Martin ME, Tapies MR, et al. Regulation of neuropathic pain by microglial Orai1 channels. Sci Adv. (2023) 9:eade7002. doi: 10.1126/sciadv.ade7002
217. Yang C, Yamaki S, Jung T, Kim B, Huyhn R, McKemy DD. Endogenous inflammatory mediators produced by injury activate TRPV1 and TRPA1 nociceptors to induce sexually dimorphic cold pain that is dependent on TRPM8 and GFRα3. J Neuroscience: Off J Soc For Neurosci. (2023) 43:2803–14. doi: 10.1523/JNEUROSCI.2303-22.2023
218. Cabañero D, Villalba-Riquelme E, Fernández-Ballester G, Fernández-Carvajal A, Ferrer-Montiel A. ThermoTRP channels in pain sexual dimorphism: new insights for drug intervention. Pharmacol Ther. (2022) 240:108297. doi: 10.1016/j.pharmthera.2022.108297
219. Lin J, Thompson TJ, Cheng YJ, Zhuo X, Zhang P, Gregg E, et al. Projection of the future diabetes burden in the United States through 2060. Popul Health Metr. (2018) 16:9. doi: 10.1186/s12963-018-0166-4
220. Lo DF, Gawash A, Shah KP, Emanuel J, Goodwin B, Shamilov DD, et al. Voices of wisdom: geriatric interviews on self-management of type 2 diabetes in the United States-A systematic review and metasynthesis. J Diabetes Res. (2024) 2024:2673742. doi: 10.1155/2024/2673742
221. Inzucchi SE, Bergenstal RM, Buse JB, Diamant M, Ferrannini E, Nauck M, et al. Management of hyperglycemia in type 2 diabetes: a patient-centered approach: position statement of the American Diabetes Association (ADA) and the European Association for the Study of Diabetes (EASD). Diabetes Care. (2012) 35:1364–79. doi: 10.2337/dc12-0413
222. Papakonstantinou E, Oikonomou C, Nychas G, Dimitriadis GD. Effects of diet, lifestyle, chrononutrition and alternative dietary interventions on postprandial glycemia and insulin resistance. Nutrients. (2022) 14(4):823. doi: 10.3390/nu14040823
223. Dohrn MF, Othman A, Hirshman SK, Bode H, Alecu I, Fähndrich E, et al. Elevation of plasma 1-deoxy-sphingolipids in type 2 diabetes mellitus: a susceptibility to neuropathy? Eur J Neurol. (2015) 22(5):806–e55. doi: 10.1111/ene.12663
224. Sharma S, Vas P, Rayman G. Small fiber neuropathy in diabetes polyneuropathy: is it time to change? J Diabetes Sci Technol. (2022) 16:321–31. doi: 10.1177/1932296821996434
225. Calcutt NA. Diabetic neuropathy and neuropathic pain: a (con)fusion of pathogenic mechanisms? Pain. (2020) 161:S65–86. doi: 10.1097/j.pain.0000000000001922
226. Sloan G, Selvarajah D, Tesfaye S. Pathogenesis, diagnosis and clinical management of diabetic sensorimotor peripheral neuropathy. Nat Rev Endocrinology. (2021) 17:400–20. doi: 10.1038/s41574-021-00496-z
227. Boulton AJM. Diabetic neuropathy: is pain God’s greatest gift to mankind? Semin Vasc Surg. (2012) 25:61–5. doi: 10.1053/j.semvascsurg.2012.04.009
228. Guo S-J, Shi Y-Q, Zheng Y-N, Liu H, Zheng Y-L. The voltage-gated calcium channel α2δ Subunit in neuropathic pain. Mol Neurobiology. (2025) 62:2561–72. doi: 10.1007/s12035-024-04424-w
229. Joshi I, Taylor CP. Pregabalin action at a model synapse: binding to presynaptic calcium channel alpha2-delta subunit reduces neurotransmission in mice. Eur J Pharmacol. (2006) 553:82–8. doi: 10.1016/j.ejphar.2006.09.019
230. Jung Y-H, Kim YO, Han JH, Kim Y-C, Yoon MH. Isobolographic analysis of drug combinations with intrathecal BRL52537 (κ-opioid agonist), pregabalin (Calcium channel modulator), AF 353 (P2X3 receptor antagonist), and A804598 (P2X7 receptor antagonist) in neuropathic rats. Anesth Analgesia. (2017) 125:670–7. doi: 10.1213/ANE.0000000000001883
231. Zajączkowska R, Mika J, Leppert W, Kocot-Kępska M, Malec-Milewska M, Wordliczek J. Mirogabalin-A novel selective ligand for the α2δ Calcium channel subunit. Pharm (Basel). (2021) 14(2):112. doi: 10.3390/ph14020112
232. Huang C-N, Chen Y-M, Xiao X-Y, Zhou H-L, Zhu J, Qin H-M, et al. Pregabalin can interact synergistically with Kv7 channel openers to exert antinociception in mice. Eur J Pharmacol. (2023) 954:175870. doi: 10.1016/j.ejphar.2023.175870
233. Wang S-Y, Calderon J, Kuo Wang G. Block of neuronal Na+ channels by antidepressant duloxetine in a state-dependent manner. Anesthesiology. (2010) 113:655–65. doi: 10.1097/ALN.0b013e3181e89a93
234. Zimova L, Ptakova A, Mitro M, Krusek J, Vlachova V. Activity dependent inhibition of TRPC1/4/5 channels by duloxetine involves voltage sensor-like domain. Biomedicine Pharmacotherapy = Biomedecine Pharmacotherapie. (2022) 152:113262. doi: 10.1016/j.biopha.2022.113262
235. Demirdaş A, Nazıroğlu M, Övey İS. Duloxetine reduces oxidative stress, apoptosis, and ca2+ Entry through modulation of TRPM2 and TRPV1 channels in the hippocampus and dorsal root ganglion of rats. Mol Neurobiology. (2017) 54:4683–95. doi: 10.1007/s12035-016-9992-1
236. Sałat K, Furgała-Wojas A, Sałat R. The microglial activation inhibitor minocycline, used alone and in combination with duloxetine, attenuates pain caused by oxaliplatin in mice. Molecules (Basel Switzerland). (2021) 26(12):3577. doi: 10.3390/molecules26123577
237. van Nooten F, Treur M, Pantiri K, Stoker M, Charokopou M. Capsaicin 8% Patch versus oral neuropathic pain medications for the treatment of painful diabetic peripheral neuropathy: A systematic literature review and network meta-analysis. Clin Ther. (2017) 39(4):787–803.e18. doi: 10.1016/j.clinthera.2017.02.010
238. Burness CB, McCormack PL. Capsaicin 8% Patch: A review in peripheral neuropathic pain. Drugs. (2016) 76:123–34. doi: 10.1007/s40265-015-0520-9
239. Yang R, Xiong Z, Liu C, Liu L. Inhibitory effects of capsaicin on voltage-gated potassium channels by TRPV1-independent pathway. Cell Mol Neurobiology. (2014) 34:565–76. doi: 10.1007/s10571-014-0041-1
240. Nauck MA, Quast DR, Wefers J, Meier JJ. GLP-1 receptor agonists in the treatment of type 2 diabetes - state-of-the-art. Mol Metab. (2021) 46:101102. doi: 10.1016/j.molmet.2020.101102
241. Pratley RE, Aroda VR, Lingvay I, Lüdemann J, Andreassen C, Navarria A, et al. Semaglutide versus dulaglutide once weekly in patients with type 2 diabetes (SUSTAIN 7): a randomised, open-label, phase 3b trial. Lancet Diabetes Endocrinol. (2018) 6:275–86. doi: 10.1016/S2213-8587(18)30024-X
242. Heymsfield SB, Coleman LA, Miller R, Rooks DS, Laurent D, Petricoul O, et al. Effect of bimagrumab vs placebo on body fat mass among adults with type 2 diabetes and obesity: A phase 2 randomized clinical trial. JAMA Netw Open. (2021) 4:e2033457. doi: 10.1001/jamanetworkopen.2020.33457
243. Pratt E, Ma X, Liu R, Robins D, Coskun T, Sloop KW, et al. Orforglipron (LY3502970), a novel, oral non-peptide glucagon-like peptide-1 receptor agonist: A Phase 1b, multicentre, blinded, placebo-controlled, randomized, multiple-ascending-dose study in people with type 2 diabetes. Diabetes Obes Metab. (2023) 25:2642–9. doi: 10.1111/dom.15150
244. Carlsson P-O, Espes D, Sisay S, Davies LC, Smith CIE, Svahn MG. Umbilical cord-derived mesenchymal stromal cells preserve endogenous insulin production in type 1 diabetes: a Phase I/II randomised double-blind placebo-controlled trial. Diabetologia. (2023) 66:1431–41. doi: 10.1007/s00125-023-05934-3
245. Iqbal Z, Azmi S, Yadav R, Ferdousi M, Kumar M, Cuthbertson DJ, et al. Diabetic peripheral neuropathy: epidemiology, diagnosis, and pharmacotherapy. Clin Ther. (2018) 40:828–49. doi: 10.1016/j.clinthera.2018.04.001
246. Tu Y. Artemisinin-A gift from traditional chinese medicine to the world (Nobel lecture). Angew Chem Int Ed Engl. (2016) 55:10210–26. doi: 10.1002/anie.201601967
247. Lengfelder E, Hofmann WK, Nowak D. Impact of arsenic trioxide in the treatment of acute promyelocytic leukemia. Leukemia. (2012) 26:433–42. doi: 10.1038/leu.2011.245
Keywords: diabetic neuropathic pain, microglia, astrocytes, Schwann cells, ion channels, spinal dorsal horn
Citation: Wu J, Hu H and Li X (2025) Spinal neuron-glial crosstalk and ion channel dysregulation in diabetic neuropathic pain. Front. Immunol. 16:1480534. doi: 10.3389/fimmu.2025.1480534
Received: 14 August 2024; Accepted: 19 March 2025;
Published: 08 April 2025.
Edited by:
Sun Kwang Kim, Kyung Hee University, Republic of KoreaReviewed by:
Livio Luongo, University of Campania Luigi Vanvitelli, ItalyCopyright © 2025 Wu, Hu and Li. This is an open-access article distributed under the terms of the Creative Commons Attribution License (CC BY). The use, distribution or reproduction in other forums is permitted, provided the original author(s) and the copyright owner(s) are credited and that the original publication in this journal is cited, in accordance with accepted academic practice. No use, distribution or reproduction is permitted which does not comply with these terms.
*Correspondence: Xi Li, MTUxODAxNzEwNDBAMTYzLmNvbQ==
†These authors have contributed equally to this work and share first authorship
Disclaimer: All claims expressed in this article are solely those of the authors and do not necessarily represent those of their affiliated organizations, or those of the publisher, the editors and the reviewers. Any product that may be evaluated in this article or claim that may be made by its manufacturer is not guaranteed or endorsed by the publisher.
Research integrity at Frontiers
Learn more about the work of our research integrity team to safeguard the quality of each article we publish.