- 1Department of Surgery, University of Florida, Gainesville, FL, United States
- 2Division of Pulmonary and Critical Care Medicine, Department of Medicine, University of Florida, Gainesville, FL, United States
- 3Department of Pharmacology and Therapeutics, University of Florida, Gainesville, FL, United States
Lung ischemia-reperfusion injury (IRI), a common complication after lung transplantation (LTx), plays a crucial role in both primary graft dysfunction (PGD) and chronic lung allograft dysfunction (CLAD) thereby adversely impacting the clinical outcomes in these patient cohorts. Lung IRI is characterized by several molecular events including immune cell infiltration, reactive oxygen species (ROS) generation, calcium overload, inflammation and various forms of cell death pathways. Currently, no therapeutic agents are available to clinically prevent lung IRI. While animal and cell culture models are highly valuable in understanding the pathophysiology of lung IRI, they may not completely recapitulate the complexity of human lung tissue pathology. This limitation necessitates the requirement for developing innovative preclinical human research tools that can supplement available scientific modalities. Emerging evidence suggests that precision-cut lung slices (PCLS) have become an indispensable tool in scientific research to study lung biology in an ex vivo tissue system. Recent studies using PCLS have investigated lung diseases including asthma, chronic obstructive pulmonary disease (COPD), and lung cancer. Although PCLS can be successfully employed to determine the deleterious events in the pathogenesis of lung IRI, including cell-cell interactions as well as hallmarks of inflammation and oxidative stress-dependent pathways, detailed studies employing PCLS to decipher these molecular events in post-LTx injury are currently limited. This review focuses on the applicability and unexplored potential of PCLS as a powerful tool in lung IRI research for understanding the pathophysiology and consequent development of new therapeutic modalities.
1 Introduction
Respiratory diseases rank among the leading causes of morbidity and mortality worldwide, and the extremely high disability rate imposes a huge financial burden on the families of patients (1). As they affect millions worldwide, these conditions are critical areas of medical research. Lung diseases can be categorized broadly into chronic and acute. Chronic respiratory diseases are a group of different conditions affecting the airways, which all impair lung function over time. These include asthma and rhinitis, chronic obstructive pulmonary disease (COPD), occupational lung diseases, sleep-disordered breathing (SDB), pulmonary hypertension and pulmonary interstitial diseases (2). Acute respiratory diseases are conditions that affect the respiratory system (airways, lungs) and have a sudden onset, typically lasting a short period (a few days to a few weeks). These include acute lung injury (ALI), acute respiratory distress syndrome (ARDS) and COVID-19-associated ARDS (3). Lung ischemia-reperfusion injury (IRI) is a form of acute lung injury (ALI), commonly occurring after lung transplantation (LTx) (4). In-depth molecular and cellular signaling pathway analysis of allograft tolerance or rejection requires reliable and reproducible in vitro and in vivo models of primary graft dysfunction (PGD) and chronic lung allograft dysfunction (CLAD). Recent advances in ex vivo models include precision cut lung slices (PCLS) that represent uniform tissue slices generated from animal or human lungs, and have proven to be a valuable tool to facilitate high-throughput studies in basic lung biology (5). In this review, we explore the applications of PCLS in lung IRI, highlighting the importance and utility of PCLS as a valuable tool in investigating post-LTx injury.
2 Lung ischemia reperfusion injury
LTx is a potentially life-prolonging therapy for patients with end-stage lung disease; however, potential benefits are limited due to several complications that result in chronic rejection of the allograft tissue (6). Despite advances in surgical management and immunosuppression, survival rates following LTx remain significantly lower compared to other solid organ transplants, with a current median survival of 5.8 years (7). While restoring blood flow is essential for recovery, reperfusion itself causes further damage, triggering acute, sterile inflammation. IRI is therefore defined by two phases of organ damage: the initial phase, caused by the interruption of blood supply (during organ procurement), and the second phase, occurring when blood flow is restored (reperfusion phase during post-LTx) (8). Clinically, lung IRI can lead to pulmonary dysfunction, decreased PO2, edema, increased pulmonary vascular resistance and enhanced vascular permeability causing immune cell infiltration and inflammation. Lung tissue oxygenation is compromised as ventilation/perfusion (V/Q) mismatch occurs due to pulmonary edema and elevated peak airway pressures that leads to inadequate ventilation (9).
At the cellular level, lung IRI is characterized by exacerbation of cellular dysfunction and cell death. During IRI, a rapid accumulation of reactive oxygen species (ROS) occurs shortly after reperfusion, accompanied by increased activity of ROS-generating enzymes (10). Intracellular calcium overload is another major event in the initial steps of lung IRI (11). Calcium overload and excessive ROS generation trigger both apoptosis and necrosis causing a further release of ROS and proinflammatory cytokines. These multifaceted processes activate resident lung macrophages, an early and critical source of pro-inflammatory mediators that orchestrate the IRI response involving immune as well as parenchymal cells such as alveolar type II (ATII) epithelial cells (12). Furthermore, chemokine secretion, such as C-X-C motif chemokine ligand 1 (CXCL1) by ATII cells, and increased endothelial permeability leads to infiltration of neutrophils, which plays an important role in pathophysiology of lung IRI (13). These inflammatory pathways and mechanisms contribute to increased pulmonary vascular resistance and microvascular permeability, endothelial cell dysfunction, resulting in pulmonary edema and impaired gas exchange, that are established clinical hallmarks of PGD (12, 14). Emerging evidence also shows that IRI induced tissue/cell injury involves regulated cell death pathways such as autophagy, apoptosis, necroptosis, and ferroptosis (15). Taken together, the inflammatory milieu in the post-LTx tissue microenvironment involves a synchronized crosstalk between immune cells such as alveolar macrophages and neutrophils, as well as parenchymal cells such as ATII cells and endothelial cells, which culminate in IRI thereby leading to acute rejection.
2.1 Models of lung IRI
Preclinical models are essential to address knowledge gaps in three of the most vexing and serious LTx complications: PGD, acute rejection (AR), and CLAD. Traditional animal models and ex vivo/in vitro models are the common experimental models used in studying lung IRI.
2.1.1 In vivo models
Orthotopic LTx, which is more commonly performed using the left lung, has been used to study PGD and recapitulate the various steps in clinical LTx such as cold and warm ischemia as well as immunosuppression using murine models (16–18). This model has the advantage that it simulates the surgical procedure of human LTx. Orthotopic LTx is a technically demanding procedure but is more ideal for studying immune response following LTx (19). Researchers have explored various anti-inflammatory and immune regulatory therapies targeting different pathways in this small animal LTx model (20, 21).
Another commonly used model to study lung IRI is the hilar ligation model (21). This is an efficient, accelerated proxy for lung IRI, eliciting the same pathophysiological changes in a shorter timeframe that recapitulates the preclinical framework of ALI (22). This murine model involves mechanical ventilation, a thoracotomy to expose the left lung hilum, and occlusion of all hilar structures with a microvascular clamp for a variable period of warm ischemia followed by unclamping allowing for reperfusion (23). This model showed extensive pulmonary tissue injury evident by increased neutrophil infiltration, apoptosis and pulmonary dysfunction (21, 22, 24). Another variation of the hilar clamp model involves isolating and clamping only the pulmonary artery while preserving gas exchange, known as the nonhypoxic lung ischemia model (25). An advantage of the hilar ligation model for studying IRI component of PGD is that it is less technically challenging than orthotopic single LTx models, but the limitations include lack of recapitulation of cold storage, donor-dependent characteristics, and absence of immunosuppression. Overall, these murine models make them an appropriate platform for testing therapeutic interventions, evaluating rehabilitative potential, and identifying biomarkers predictive of PGD (21, 23, 24, 26).
2.1.2 Ex vivo lung perfusion
Ex vivo lung perfusion (EVLP) has developed as a novel method to maintain donation after cardiac death (DCD) lungs in physiologically protective conditions outside the body and allows accurate evaluation of lung function, as well as providing a new platform for therapeutic treatment and repair of damaged donor lungs before transplantation (27). During EVLP, the donor lungs are subjected to a perfusion apparatus that offers a controlled environment, allowing for the delivery of oxygen and nutrients while removing carbon dioxide and other waste products. EVLP has been used to study several molecular events and therapeutic agents in lung IRI, including interleukin-10 (IL-10), mesenchymal stem cell-derived extracellular vesicles, β-adrenoreceptor agonists, and sevoflurane, yielding promising results in preconditioning of donor lungs to increase the donor pool and with the goal to mitigate post-LTx IRI (14). Several preclinical studies using EVLP in porcine LTx models have shown the relevance of temperature regulation of donor organ preservation with normothermic and hypothermic conditions to enhance the suitability of DCD organs and attenuate post-LTx IRI, that can be effectively studied using this technique (28–33).
2.1.3 In vitro models
Detailed cellular level analysis facilitated by in vitro models of tissue injury and induction of IRI have been successfully reported in cell lines of kidney, liver, heart and lung (34). To closely mimic the process of ischemia and subsequent reperfusion, previous studies have used in vitro hypoxia-reoxygenation (HR) as a model in lung cells. In this model, cells are cultured in a controlled environment with reduced oxygen levels (typically 1–5% O2) or in the complete absence of oxygen. After the hypoxia period, the cells are re-exposed to normal oxygen levels (20–21% O2) to simulate reperfusion that lead to substantial production of oxidative stress and inflammation (21, 35, 36). The use of co-cultures and transwell membranes using different cell types also facilitates cell-cell interaction that is a key hallmark of lung IRI.
While animal models have significantly advanced our understanding of disease mechanisms and are crucial for studying in vivo processes, they do not necessarily mimic the nature and complexity of native human tissue and genome. Additionally, in some cases, such as with species-specific infections, especially in pulmonary diseases, animals may not entirely recapitulate the immunogenic response of human disease. Moreover, animal models are also limited to provide high-throughput analysis of drugs or pathogen isolates (37, 38). Furthermore, in vitro models encompassing cells in culture may not accurately simulate the in vivo environment experienced by lung cells during transplantation. Since the cell culture systems do not replicate the complex structure of pulmonary tissue, the ability of in vitro test results has inherent limitations as well (39, 40). Hence, there is a need for improved, scalable models, which also will enable reduction and refinement of animal research (37). Thus, recent evidence suggests that PCLS has emerged as a highly relevant ex vivo model for studying injury and repair responses, as well as for the development of novel therapies.
3 Precision cut lung slices
PCLS are thin and uniform lung slices made from human or animal lung tissues that have attracted increasing attention (1). PCLS can be prepared from naïve, genetically modified, or diseased animals as well as from human surgical samples and lung explants, and can be even cryopreserved for future use (41). The ex vivo tissue obtained from PCLS maintains the homogeneity and architecture of the primary tissue and retains resident cell content and morphology of the lung, including smooth muscle cells, epithelial cells, and fibroblasts. These cells maintain their intercellular interactions and cell-to-matrix relationships within the complex structures of the lung. PCLS are now widely utilized to assess integrated cellular responses triggered by inflammatory, fibrotic, or infectious stimuli, as well as for investigating lung damage and regeneration (42). Studies have compared in vivo exposed lungs and PCLS behavior and sensitivity to a wide panel of stimuli (physical, mechanical, chemical, pharmacological). Collectively, these studies conclude and suggest that PCLS respond in a manner similar to in vivo lung tissue (43). The development of PCLS technique, with improved methods for maintaining viability and function in prolonged culture, has resulted in numerous applications in pulmonary research. PCLS have been used in lung anatomy studies also been used in numerous toxicology studies to assess the efficacy and safety of new therapeutic targets (44). Further, PCLS have proven to be useful for studying a variety of conditions including asthma, allergic responses, vascular responses, early fibrosis, COPD, alveologenesis, lung cancer and respiratory infections, among other applications (37, 43, 44).
3.1 Preparation and maintenance of PCLS
The history of PCLS can be traced back to the general development of techniques for preparing and studying tissue slices in general. The development of tissue-slicing techniques began in the late 19th and early 20th centuries when researchers used specialized instruments for cutting thin sections from various tissues for microscopic examination (45). The development of vibratome/microtome allowed for the production of tissue slices with greater precision and consistent thickness (46). A significant breakthrough in PCLS was achieved in 1980s, where in Placke and Fisher infused airways of rat and hamster lungs with heated liquid agarose. This agarose solidifies at temperatures below 25°C, helps in maintaining the inflated state of the lung and prevents the collapse of the airways and delicate alveoli during the slicing process (47). This method was further developed and first applied to human lung tissue in 1994 (48). Since then, various adaptations have been made and detailed step‐by‐step protocols for generating PCLS from human and mouse tissue have been reported in the literature (49, 50).
Central to all protocols in the preparation of PCLS is that the freshly retrieved whole lungs or individual lobes are infused with warm, low-melting agarose dissolved in buffer or tissue culture medium, at a concentration ranging from 0.5% to 3% (38) (Figure 1). The most common and easiest way to deliver the agarose to the lung is by cannulating the trachea in small animals (42). In human PCLS, lung sections are filled via the main bronchus with low melting point agarose solution (51). However, human lung samples are often received as partial lobes from surgical resections. If the airways cannot be identified, multiple small injections of agarose with a fine needle can be made directly into the alveolar tissue to inflate the lung (52). The lungs are then cooled to below 25°C by immersing the tissue in cold buffer to solidify the agarose and stiffen the parenchyma before slicing. After solidification, the tissue is sliced using a vibratome/compresstome to obtain PCLS of uniform thickness between 150-500 µm (38). Protocols for culturing PCLS vary in terms of culture media conditions and duration, depending on the specific outcomes being studied. Serum is either absent or added in very low quantities to prevent cell proliferation and changes to the native cellular diversity (52). For incubation, 50 µg/mL gentamicin and 0.25 µg/mL amphotericin B are added to serum-free minimal essential medium (MEM) and then placed in a 5% CO2 incubator at 37°C. The medium is changed every 30 min for the first 2 hours, once per hour for the next 2 hours, and then switched to 24 hour intervals to remove the residual agarose and cell debris (1). Most studies using PCLS have been conducted under short-term culturing conditions (≤72 h), but there are reports showing that PCLS can remain viable for up to 28 days (53).
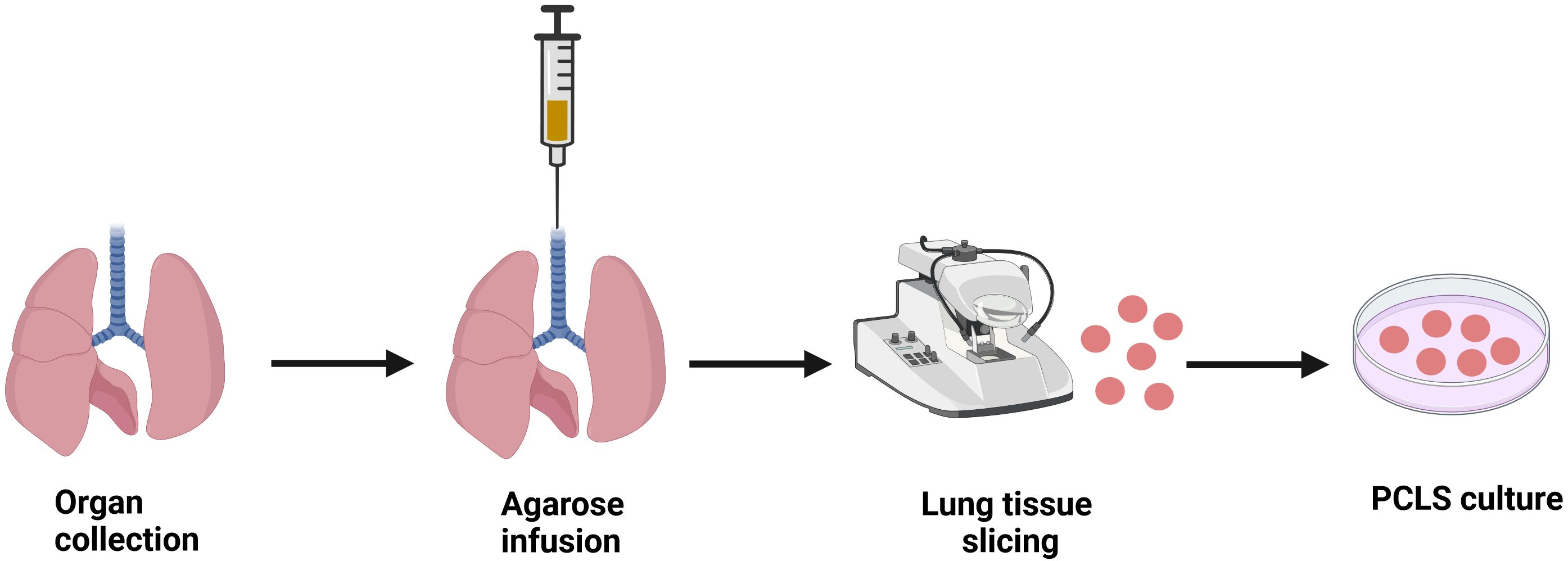
Figure 1. Schematic diagram illustrating the procedure to generate precision cut lung slices (PCLS). Lung tissue is harvested and PCLS is prepared using a specialized tissue slicer following agarose infusion into the lungs. Ex vivo PCLS can be then cultured in appropriate conditions for studying respective pathological milieu. Image created using biorender.com.
3.2 Application of PCLS in LTx research
The number of studies utilizing PCLS in LTx research is limited (54, 55). Hence, the application of PCLS in lung IRI is an underexplored area that could play a significant role in advancing LTx research. PCLS can be used to investigate many of the pathological events during lung IRI, making it a powerful tool for studying this condition. Here, we describe the deleterious molecular events in lung IRI that can be effectively studied by employing PCLS.
3.2.1 PCLS as a research tool for oxidative stress and inflammation
The robust oxidative stress and sterile inflammation occurring upon reperfusion is a key driver of PGD leading to inferior short- and long-term survival in LTx (4, 12). Baste et al. (54) demonstrated that PCLS can be used to study lung IRI by employing a hybrid model, with an in vivo ischemic period followed by ex vivo reoxygenation (54). In this study, following in vivo ischemia, lungs excised, PCLS were prepared and transferred to medium for ex vivo reoxygenation. This study indicated that there was induction of oxidative stress, as evidenced by changes in antioxidant enzyme activities, representing the ability of PCLS tissue to mimic a pivotal cellular process of lung IRI. Histological analysis indicated bronchiolar epithelial hyperplasia and necrotic changes in the lung slices, mainly after longer ischemic durations, suggesting the presence of inflammatory responses in this pulmonary tissue (54, 56). In a recent study, PCLS prepared from human or porcine lungs were used to model acute rejection (55). Peripheral blood mononuclear cells, isolated from blood type-matched donors to mimic transplantation, were introduced into the tissue to measure immune responses. The results showed that PCLS exhibited histological signs of acute rejection, such as increased immune infiltration and alveolar wall thickening (55). In another study, PCLS were employed to detect changes in cytokine levels, oxidative stress markers and mitochondrial activity in response to nanomaterials (40). The study found increased levels of cytokines in response to a number of nanomaterials (tumor necrosis factor-alpha (TNF-α), Interleukin-8 (IL-8), macrophage colony-stimulating factor (M-CSF), and osteopontin (OPN)). These cytokines reflect different stages and aspects of inflammation, indicating the model’s ability to capture inflammatory responses. The study also showed significant alterations in reduced glutathione (GSH) levels, indicating induction of oxidative stress and confirming that PCLS can be used to determine inflammation and oxidative stress due to nanomaterial toxicity (40). Similarly, PCLS were able to produce pro-inflammatory cytokines upon lipopolysaccharide (LPS) stimulation. Cultured PCLS were also shown to be capable of generating re-call immune responses, characterized by cytokine production, against antigens commonly found in routine vaccinations against influenza virus and tetanus toxoid. This suggests that PCLS have the potential to exhibit immunologic memory, allowing them to respond to previously encountered antigens (57). In another study employing human PCLS, the initial response to LPS exposure was marked by a coordinated release of early release cytokines such as interleukin-1 beta (IL-1β), macrophage inflammatory protein-1 beta (MIP-1β), and Interferon-gamma (IFN-γ). These cytokines are produced mainly by macrophages, epithelial cells, fibroblasts, and/or endothelial cells, and they play a significant role in activating epithelial or airway smooth muscle cells. Interestingly, the study also showed that cytokine production in human lung slices and human BAL fluid from in vivo studies after treatment with LPS are correlated (58), reinforcing the applicability of PCLS in investigating the molecular pathways of pulmonary inflammation. PCLS has also been used to study the behavior and activation of macrophages in the lung environment (59). The use of PCLS as an ex vivo model to delineate lung tissue inflammatory responses in respiratory diseases has been reported recently (1).
Endothelial cell dysfunction and disruption of the endothelial barrier are hallmarks of lung IRI and contribute greatly to PGD. Depolarization of endothelial cell membranes induces ROS production and subsequent inflammation (4). PCLS has been employed to study the effects of cigarette smoke on endothelial functions. The findings showed impaired vasorelaxation and increased sensitivity to vasoconstriction, which are indicative of endothelial dysfunction (60). In a recent study, PCLS exposed to nitrogen mustard (a cytotoxic agent), showed evidence of oxidative stress as shown by reduced total and oxidized glutathione levels and increased catalase levels (61). Further, oxidative stress was elevated in PCLS exposed gasoline exhaust particles (62). PCLS exposed to organophosphorus pesticides exhibited reduced GSH levels and increased oxidized glutathione levels (63). Furthermore, PCLS subjected to hypoxia exhibited increased ROS levels (64). Altogether, PCLS represents a valuable tool for investigating inflammation and oxidative stress, the major deleterious molecular events occurring during IRI, making it a useful model for studying IRI mechanisms and testing various therapeutic modalities.
3.2.2 Application of PCLS to measure calcium overload
Intracellular calcium overload is one of the main pathological mechanism of lung IRI (65). A recent study has shown that PCLS can be used to evaluate intracellular calcium signaling (66). Employing Oregon green to measure intracellular Ca2+ levels in lung slices, a previous study showed that Ca2+ oscillations are essential for maintaining the contractile state of the airway smooth muscle cells and, thus, the airway contraction (67). It is shown that the inhibition of calcium activated potassium channels increased cytoplasmic Ca2+ in PCLS (68). Furthermore, the correlation of airway contraction with smooth muscle cell Ca2+ signaling has been possible by combining the PCLS with real-time confocal or two-photon microscopy (67, 69). These reports suggest the applicability of using ex vivo PCLS to decipher the relevance of calcium and ion-mediated signaling in post-LTx IRI.
3.2.3 Application of PCLS to study cell death pathways
Various cell death pathways have been observed in lung IRI, including apoptosis, necrosis, pyroptosis and ferroptosis (70). PCLS treated with hyperoxia exhibited increased DNA fragmentation, a hallmark sign of apoptosis and necrosis as revealed by TdT-mediated dUTP-biotin nick end labeling (TUNEL) staining (71). Furthermore, PCLS treated with nanoparticles exhibited increased apoptosis indicated by significant increase in caspase-3 staining (72, 73). In another study, whole diesel exhaust induced a pro- inflammatory and apoptotic response in PCLS which were reduced by exhaust filtration (74). A recent study has shown that PCLS can be used to investigate ferroptosis (75). Together, these studies suggest that PCLS can be effectively employed to delineate various cell death pathways that have been reported in post-LTx injury.
4 Summary, limitations and future perspectives
4.1 Summary
Lung IRI is the main risk factor for primary graft dysfunction and mortality following LTx. While extensive research has been conducted on lung IRI using other established animal and cell culture models, the underlying mechanisms and signal transduction pathways remain elusive, and a specific, effective treatment is still unavailable. PCLS can make a huge advancement in lung IRI research by bridging the gap between in vitro and in vivo models. PCLS reflects the complex cellular composition and the matrix effects of a diseased human lung, and biological diversity underlying differential treatment response in patients may well be studied in PCLS from different patients (76). The integration of agarose stabilization, precise mechanical cutting, and advanced imaging techniques has transformed PCLS into a powerful tool for studying lung physiology in both health and disease (52). Although many of the molecular events associated with lung IRI, such as oxidative stress, inflammation, calcium overload, and cell death pathways, can be studied using PCLS, only a few studies have employed this model so far. Recent evidence and previous studies, as summarized above, demonstrate that PCLS can be a valuable tool for investigating the pathogenesis and delineating the mechanistic potential of therapeutic targets in the treatment of lung IRI (Figure 2). Thus, PCLS offers a robust, cutting-edge platform that can drive significant advances in the study and treatment of lung IRI.
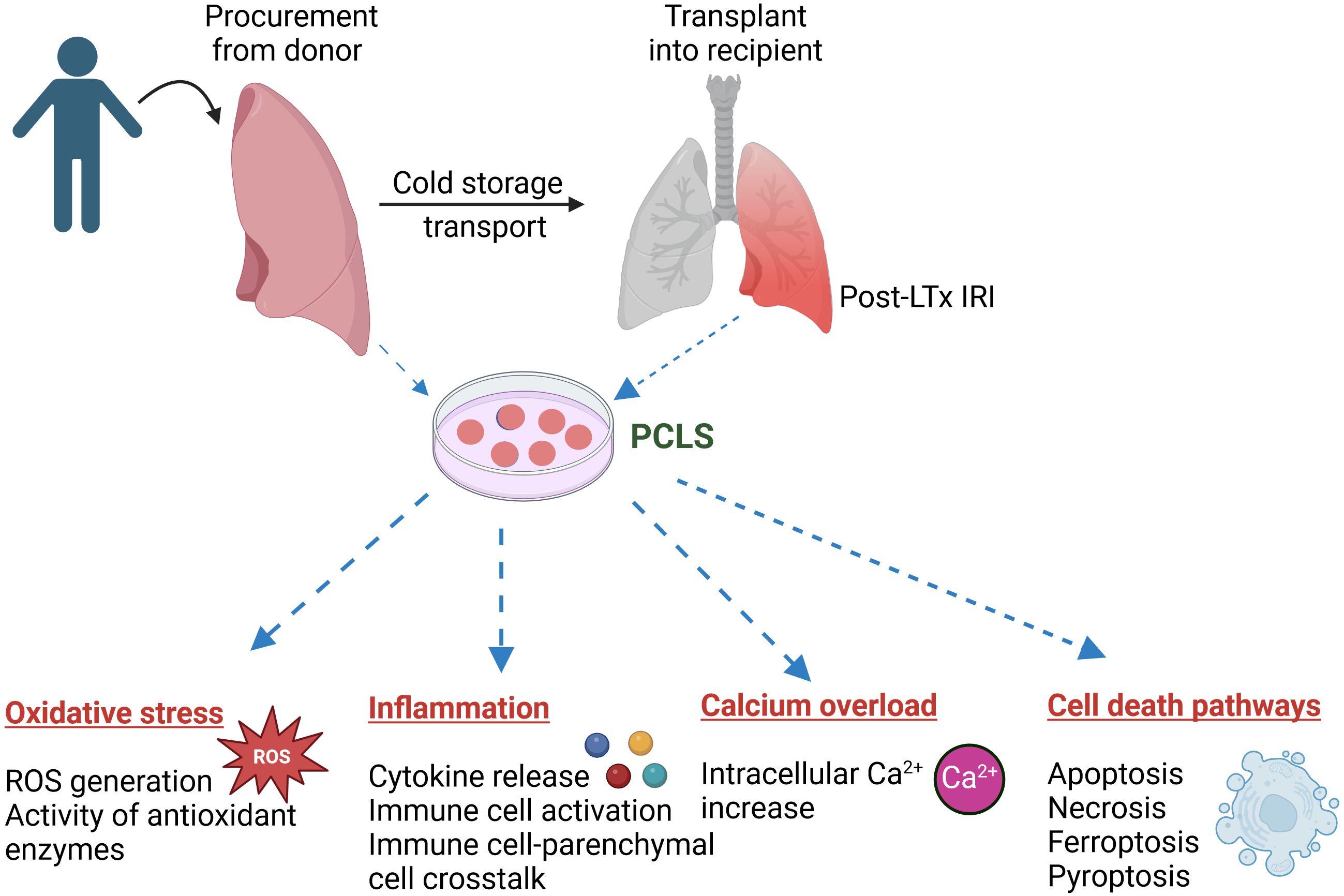
Figure 2. Application of precision cut lung slices (PCLS) in investigating the pathogenesis of lung ischemia reperfusion injury (IRI) following lung transplantation (LTx). PCLS tissue can be generated from donor or recipient lungs to study a myriad of biological processes, such as oxidative stress, inflammation, Ca2+ overload and various cell death pathways, involved in lung IRI. Image created using biorender.com.
4.2 Limitations of using PCLS in LTx research
Several potential tissue-related challenges arise when using PCLS such as cellular changes induced by agarose instillation into the lung and pre-contraction of airways (51). Issues with agarose instillation can result in problems such as mushy lungs when cutting sections and shredded tissue (77). Further, the vast amount of agarose in PCLS impedes RNA extraction by standard procedures (78). Additionally, PCLS currently lack a standardized cell culture medium, and an optimal method for medium optimization has yet to be established (1). Further, human lung tissue suitable for slicing is not available at many research institutions, and obtaining such tissue is challenging due to varying clinical and regulatory frameworks (37). Additionally, the effects of breathing and air-liquid interface cannot be studied employing PCLS (76). Furthermore, PCLS are isolated from the circulatory system in the body, making it difficult for researchers to evaluate the migration of cells between the lung, blood, and lymph nodes and preventing the replication of cellular trafficking of inflammatory cells and humoral factors, as well as antigen presentation that primes the immunogenic response in the in vivo environment. These limitations dampen the ability to evaluate adaptive immune responses and therefore needs further refining of this technique (1, 79).
4.3 Future perspectives
PCLS offers a versatile platform for investigating a wide range of lung diseases. However, optimizing and refining PCLS methods is essential to unlock its full potential as a research tool. Enhancing the longevity, structural integrity, and functional relevance of PCLS is essential to develop it into a model that more accurately reflects in vivo conditions. The short duration of PCLS culture viability is a major drawback in the field. Although attempts have been made to extend it, further attention is needed to improve both the longevity and functionality of PCLS. Additionally, PCLS should be combined with advanced imaging techniques to visualize three-dimensional cell-cell interactions, overcoming the limitations of two-dimensional imaging. Genetic manipulation or omics approaches should be applied to PCLS to gain deeper understanding of lung health and disease. As discussed in this review, there is significant potential for employing PCLS in LTx research. Likewise, the use of PCLS in lung research should be expanded to study complex lung diseases and drug responses that remain unexplored. In summary, utilizing PCLS to further our understanding of molecular events and explore various therapeutic options for post-LTx lung IRI represents a promising avenue for future research in this field. By bridging gaps between in vitro studies and clinical applications, PCLS present valuable potential for translational advancements in pulmonary research.
Author contributions
DK: Formal Analysis, Investigation, Methodology, Writing – original draft, Writing – review & editing. AS: Conceptualization, Formal Analysis, Funding acquisition, Investigation, Project administration, Resources, Supervision, Visualization, Writing – original draft, Writing – review & editing.
Funding
The author(s) declare that financial support was received for the research, authorship, and/or publication of this article. This work was supported by Department of Surgery, University of Florida.
Conflict of interest
The authors declare that the research was conducted in the absence of any commercial or financial relationships that could be construed as a potential conflict of interest.
Generative AI statement
The authors declare that no Generative AI was used in the creation of this manuscript.
Publisher’s note
All claims expressed in this article are solely those of the authors and do not necessarily represent those of their affiliated organizations, or those of the publisher, the editors and the reviewers. Any product that may be evaluated in this article, or claim that may be made by its manufacturer, is not guaranteed or endorsed by the publisher.
References
1. Liu Y, Wu P, Wang Y, Yang H, Zhou G, Wu X, et al. Application of precision-cut lung slices as an in vitro model for research of inflammatory respiratory diseases. Bioengineering (Basel). (2022) 9:767. doi: 10.3390/bioengineering9120767
2. Bousquet J, Kiley J, Bateman ED, Viegi G, Cruz AA, Khaltaev N, et al. Prioritised research agenda for prevention and control of chronic respiratory diseases. Eur Respir J. (2010) 36:995–1001. doi: 10.1183/09031936.00012610
3. Matthay MA, Zemans RL, Zimmerman GA, Arabi YM, Beitler JR, Mercat A, et al. Acute respiratory distress syndrome. Nat Rev Dis Primers. (2019) 5:18. doi: 10.1038/s41572-019-0069-0
4. Laubach VE, Sharma AK. Mechanisms of lung ischemia-reperfusion injury. Curr Opin Organ Transplant. (2016) 21:246–52. doi: 10.1097/MOT.0000000000000304
5. Rosmark O, Ibáñez-Fonseca A, Thorsson J, Dellgren G, Hallgren O, Larsson Callerfelt AK, et al. A tunable physiomimetic stretch system evaluated with precision cut lung slices and recellularized human lung scaffolds. Front Bioeng Biotechnol. (2022) 10:995460. doi: 10.3389/fbioe.2022.995460
6. Calabrese DR, Aminian E, Mallavia B, Liu F, Cleary SJ, Aguilar OA, et al. Natural killer cells activated through NKG2D mediate lung ischemia-reperfusion injury. J Clin Invest. (2021) 131:e137047. doi: 10.1172/JCI137047
7. Singh TP, Cherikh WS, Hsich E, Lewis A, Perch M, Kian S, et al. Graft survival in primary thoracic organ transplant recipients: A special report from the International Thoracic Organ Transplant Registry of the International Society for Heart and Lung Transplantation. J Heart Lung Transplant. (2023) 42:1321–33. doi: 10.1016/j.healun.2023.07.017
8. Fitridge R, Thompson M eds. Mechanisms of Vascular Disease: A Reference Book for Vascular Specialists. University of Adelaide Press (2011). Available online at: http://www.jstor.org/stable/10.20851/j.ctt1sq5w94
9. Weyker PD, Webb CA, Kiamanesh D, Flynn BC. Lung ischemia reperfusion injury: a bench-to-bedside review. Semin Cardiothorac Vasc Anesth. (2013) 17:28–43. doi: 10.1177/1089253212458329
10. Chen-Yoshikawa TF. Ischemia-reperfusion injury in lung transplantation. Cells. (2021) 10:1333. doi: 10.3390/cells10061333
11. Ferrari RS, Andrade CF. Oxidative stress and lung ischemia-reperfusion injury. Oxid Med Cell Longev. (2015) 2015:590987. doi: 10.1155/2015/590987
12. Roesel MJ, Sharma NS, Schroeter A, Matsunaga T, Xiao Y, Zhou H, et al. Primary graft dysfunction: the role of aging in lung ischemia-reperfusion injury. Front Immunol. (2022) 13:891564. doi: 10.3389/fimmu.2022.891564
13. Liao F, Scozzi D, Zhou D, Maksimos M, Diedrich C, Cano M, et al. Nanoparticle targeting of neutrophil glycolysis prevents lung ischemia-reperfusion injury. Am J Transplant. (2024) 24:1382–94. doi: 10.1016/j.ajt.2024.03.028
14. Talaie T, DiChiacchio L, Prasad NK, Pasrija C, Julliard W, Kaczorowski DJ, et al. Ischemia-reperfusion injury in the transplanted lung: A literature review. Transplant Direct. (2021) 7:e652. doi: 10.1097/TXD.0000000000001104
15. Wang Y, Dong Z, Zhang Z, Yang K, Li X. Postconditioning with irisin attenuates lung ischemia/reperfusion injury by suppressing ferroptosis via induction of the nrf2/HO-1 signal axis. Oxid Med Cell Longev. (2022) 2022:9911167. doi: 10.1155/2022/9911167
16. Patel KJ, Cheng Q, Stephenson S, Allen DP, Li C, Kilkenny J, et al. Emphysema-associated autoreactive antibodies exacerbate post-lung transplant ischemia-reperfusion injury. Am J Respir Cell Mol Biol. (2019) 60:678–86. doi: 10.1165/rcmb.2018-0224OC
17. Li C, Patel K, Tu Z, Yang X, Kulik L, Alawieh A, et al. A novel injury site-natural antibody targeted complement inhibitor protects against lung transplant injury. Am J Transplant. (2021) 21:2067–78. doi: 10.1111/ajt.16404
18. Bribriesco AC, Li W, Nava RG, Spahn JH, Kreisel D. Experimental models of lung transplantation. Front Biosci (Elite Ed). (2013) 5:266–72. doi: 10.2741/E614
19. Yamada Y, Windirsch K, Dubs L, Kenkel D, Jang JH, Inci I, et al. Chronic airway fibrosis in orthotopic mouse lung transplantation models-an experimental reappraisal. Transplantation. (2018) 102:e49–58. doi: 10.1097/TP.0000000000001917
20. Jin X, Kaes J, Van Slambrouck J, Inci I, Arni S, Geudens V, et al. A comprehensive review on the surgical aspect of lung transplant models in mice and rats. Cells. (2022) 11:480. doi: 10.3390/cells11030480
21. Leroy V, Cai J, Tu Z, McQuiston A, Sharma S, Emtiazjoo A, et al. Resolution of post-lung transplant ischemia-reperfusion injury is modulated via Resolvin D1-FPR2 and Maresin 1-LGR6 signaling. J Heart Lung Transplant. (2023) 42:562–74. doi: 10.1016/j.healun.2022.12.013
22. Gielis JF, Jungraithmayr W, Boulet GA, Bogers JP, Weder W, Cos P, et al. A murine model of lung ischemia and reperfusion injury: tricks of the trade. J Surg Res. (2015) 194:659–66. doi: 10.1016/j.jss.2014.12.008
23. Lama VN, Belperio JA, Christie JD, El-Chemaly S, Fishbein MC, Gelman AE, et al. Models of Lung Transplant Research: a consensus statement from the National Heart, Lung, and Blood Institute workshop. JCI Insight. (2017) 2:e93121. doi: 10.1172/jci.insight.93121
24. Leroy V, Manual Kollareth DJ, Tu Z, Valisno JAC, Woolet-Stockton M, Saha BK, et al. MerTK-dependent efferocytosis by monocytic-MDSCs mediates resolution of post-lung transplant ischemia-reperfusion injury. JCI Insight. (2024) 9:e179876. doi: 10.1101/2024.01.18.576261
25. Matot I, Manevich Y, Al-Mehdi AB, Song C, Fisher AB. Fluorescence imaging of lipid peroxidation in isolated rat lungs during nonhypoxic lung ischemia. Free Radic Biol Med. (2003) 34:785–90. doi: 10.1016/S0891-5849(02)01435-1
26. Cai J, Gehrau R, Tu Z, Leroy V, Su G, Shang J, et al. MicroRNA-206 antagomiR−enriched extracellular vesicles attenuate lung ischemia−reperfusion injury through CXCL1 regulation in alveolar epithelial cells. J Heart Lung Transplant. (2020) 39:1476–90. doi: 10.1016/j.healun.2020.09.012
27. Mehaffey JH, Charles EJ, Narahari AK, Schubert S, Laubach VE, Teman NR, et al. Increasing circulating sphingosine-1-phosphate attenuates lung injury during ex vivo lung perfusion. J Thorac Cardiovasc Surg. (2018) 156:910–7. doi: 10.1016/j.jtcvs.2018.02.090
28. Wagner CE, Pope NH, Charles EJ, Huerter ME, Sharma AK, Salmon MD, et al. Ex vivo lung perfusion with adenosine A2A receptor agonist allows prolonged cold preservation of lungs donated after cardiac death. J Thorac Cardiovasc Surg. (2016) 151:538–45. doi: 10.1016/j.jtcvs.2015.07.075
29. Mehaffey JH, Charles EJ, Sharma AK, Salmon M, Money D, Schubert S, et al. Ex vivo lung perfusion rehabilitates sepsis-induced lung injury. Ann Thorac Surg. (2017) 103:1723–9. doi: 10.1016/j.athoracsur.2017.01.018
30. Ali A, Nykanen A, Brambate E, Mariscal A, Chen M, Wannberg B, et al. Successful transplantation of porcine lungs following 3 days of preservation using a modified cold static method paired with intermittent normothermic ex vivo lung perfusion (EVLP). J Heart Lung Transplant. (2020) 39:S353. doi: 10.1016/j.healun.2020.01.413
31. Lin H, Chen M, Tian F, Tikkanen J, Ding L, Andrew Cheung HY, et al. [amp]]alpha;(1)-Anti-trypsin improves function of porcine donor lungs during ex-vivo lung perfusion. J Heart Lung Transplant. (2018) 37:656–66. doi: 10.1016/j.healun.2017.09.019
32. Huang L, Hough O, Vellanki RN, Takahashi M, Zhu Z, Xiang YY, et al. L-alanyl-L-glutamine modified perfusate improves human lung cell functions and extend porcine ex vivo lung perfusion. J Heart Lung Transplant. (2023) 42:183–95. doi: 10.1016/j.healun.2022.10.022
33. Tavasoli F, Liu M, Machuca T, Bonato R, Grant DR, Cypel M, et al. Increased arginase expression and decreased nitric oxide in pig donor lungs after normothermic ex vivo lung perfusion. Biomolecules. (2020) 10:300. doi: 10.3390/biom10020300
34. Hofmann J, Pühringer M, Steinkellner S, Holl AS, Meszaros AT, Schneeberger S, et al. Novel, innovative models to study ischemia/reperfusion-related redox damage in organ transplantation. Antioxidants (Basel). (2022) 12:31. doi: 10.3390/antiox12010031
35. Lv JL, Shi LN, Zhai CY, Wang GJ, Qu Y. Bag-1L Protects against Cell Apoptosis in an In Vitro Model of Lung Ischemia-Reperfusion Injury through the C-Terminal “Bag” Domain. BioMed Res Int. (2021) 2021:8822807. doi: 10.1155/2021/8822807
36. Ilie M, Hofman V, Zangari J, Chiche J, Mouroux J, Mazure NM, et al. Response of CAIX and CAXII to in vitro re-oxygenation and clinical significance of the combined expression in NSCLC patients. Lung Cancer. (2013) 82:16–23. doi: 10.1016/j.lungcan.2013.07.005
37. Alsafadi HN, Uhl FE, Pineda RH, Bailey KE, Rojas M, Wagner DE, et al. Applications and approaches for three-dimensional precision-cut lung slices. Disease modeling and drug discovery. Am J Respir Cell Mol Biol. (2020) 62:681–91. doi: 10.1165/rcmb.2019-0276TR
38. Viana F, O’Kane CM, Schroeder GN. Precision-cut lung slices: A powerful ex vivo model to investigate respiratory infectious diseases. Mol Microbiol. (2022) 117:578–88. doi: 10.1111/mmi.v117.3
39. Nel A, Xia T, Meng H, Wang X, Lin S, Ji Z, et al. Nanomaterial toxicity testing in the 21st century: use of a predictive toxicological approach and high-throughput screening. Acc Chem Res. (2013) 46:607–21. doi: 10.1021/ar300022h
40. Sauer UG, Vogel S, Aumann A, Hess A, Kolle SN, Ma-Hock L, et al. Applicability of rat precision-cut lung slices in evaluating nanomaterial cytotoxicity, apoptosis, oxidative stress, and inflammation. Toxicol Appl Pharmacol. (2014) 276:1–20. doi: 10.1016/j.taap.2013.12.017
41. Li G, Cohen JA, Martines C, Ram-Mohan S, Brain JD, Krishnan R, et al. Preserving airway smooth muscle contraction in precision-cut lung slices. Sci Rep. (2020) 10:6480. doi: 10.1038/s41598-020-63225-y
42. Lam M, Lamanna E, Organ L, Donovan C, Bourke JE. Perspectives on precision cut lung slices-powerful tools for investigation of mechanisms and therapeutic targets in lung diseases. Front Pharmacol. (2023) 14:1162889. doi: 10.3389/fphar.2023.1162889
43. Morin JP, Baste JM, Gay A, Crochemore C, Corbière C, Monteil C. Precision cut lung slices as an efficient tool for in vitro lung physio-pharmacotoxicology studies. Xenobiotica. (2013) 43:63–72. doi: 10.3109/00498254.2012.727043
44. Michalaki C, Dean C, Johansson C. The use of precision-cut lung slices for studying innate immunity to viral infections. Curr Protoc. (2022) 2:e505. doi: 10.1002/cpz1.v2.8
45. Koziol-White C, Gebski E, Cao G, Panettieri RA Jr. Precision cut lung slices: an integrated ex vivo model for studying lung physiology, pharmacology, disease pathogenesis and drug discovery. Respir Res. (2024) 25:231. doi: 10.1186/s12931-024-02855-6
46. Krumdieck CL, dos Santos JE, Ho KJ. A new instrument for the rapid preparation of tissue slices. Anal Biochem. (1980) 104:118–23. doi: 10.1016/0003-2697(80)90284-5
47. Placke ME, Fisher GL. Adult peripheral lung organ culture–a model for respiratory tract toxicology. Toxicol Appl Pharmacol. (1987) 90:284–98. doi: 10.1016/0041-008X(87)90336-X
48. Fisher RL, Smith MS, Hasal SJ, Hasal KS, Gandolfi AJ, Brendel K. The use of human lung slices in toxicology. Hum Exp Toxicol. (1994) 13:466–71. doi: 10.1177/096032719401300703
49. Lyons-Cohen MR, Thomas SY, Cook DN, Nakano H. Precision-cut mouse lung slices to visualize live pulmonary dendritic cells. J Vis Exp. (2017) (122):55465. doi: 10.3791/55465
50. Neuhaus V, Schaudien D, Golovina T, Temann UA, Thompson C, Lippmann T, et al. Assessment of long-term cultivated human precision-cut lung slices as an ex vivo system for evaluation of chronic cytotoxicity and functionality. J Occup Med Toxicol. (2017) 12:13. doi: 10.1186/s12995-017-0158-5
51. Wohlsen A, Martin C, Vollmer E, Branscheid D, Magnussen H, Becker WM, et al. The early allergic response in small airways of human precision-cut lung slices. Eur Respir J. (2003) 21:1024–32. doi: 10.1183/09031936.03.00027502
52. Sanderson MJ. Exploring lung physiology in health and disease with lung slices. Pulm Pharmacol Ther. (2011) 24:452–65. doi: 10.1016/j.pupt.2011.05.001
53. Patel V, Amin K, Allen D, Ukishima L, Wahab A, Grodi C, et al. Comparison of long-term human precision-cut lung slice culture methodology and response to challenge: an argument for standardisation. Altern Lab Anim. (2021) 49:209–22. doi: 10.1177/02611929211061884
54. Baste JM, Gay A, Smail H, Noël R, Bubenheim M, Begueret H, et al. Organotypic lung culture: A new model for studying ischemia and ex vivo perfusion in lung transplantation. Exp Lung Res. (2015) 41:564–75. doi: 10.3109/01902148.2015.1123328
55. Niroomand A, Hirdman G, Mittendorfer M, Bolukbas D, Olm F, Lindstedt S. An ex vivo model of acute rejection using precision cut lung slices. J Heart Lung Transplant. (2023) 42:s176. doi: 10.1016/j.healun.2023.02.1675
56. Wang GF, Lai MD, Yang RR, Chen PH, Su YY, Lv BJ, et al. Histological types and significance of bronchial epithelial dysplasia. Mod Pathol. (2006) 19:429–37. doi: 10.1038/modpathol.3800553
57. Temann A, Golovina T, Neuhaus V, Thompson C, Chichester JA, Braun A, et al. Evaluation of inflammatory and immune responses in long-term cultured human precision-cut lung slices. Hum Vaccin Immunother. (2017) 13:351–8. doi: 10.1080/21645515.2017.1264794
58. Switalla S, Lauenstein L, Prenzler F, Knothe S, Förster C, Fieguth HG, et al. Natural innate cytokine response to immunomodulators and adjuvants in human precision-cut lung slices. Toxicol Appl Pharmacol. (2010) 246:107–15. doi: 10.1016/j.taap.2010.04.010
59. Karger A, Mansouri S, Leisegang MS, Weigert A, Günther S, Kuenne C, et al. ADPGK-AS1 long noncoding RNA switches macrophage metabolic and phenotypic state to promote lung cancer growth. EMBO J. (2023) 42:e111620. doi: 10.15252/embj.2022111620
60. Wright JL, Churg A. Short-term exposure to cigarette smoke induces endothelial dysfunction in small intrapulmonary arteries: analysis using Guinea pig precision cut lung slices. J Appl Physiol. (1985) 104:1462–9. doi: 10.1152/japplphysiol.00520.2007
61. Bellomo A, Herbert J, Kudlak MJ, Laskin JD, Gow AJ, Laskin DL. Identification of early events in nitrogen mustard pulmonary toxicity that are independent of infiltrating inflammatory cells using precision cut lung slices. Toxicol Appl Pharmacol. (2024) 486:116941. doi: 10.1016/j.taap.2024.116941
62. Maikawa CL, Zimmerman N, Rais K, Shah M, Hawley B, Pant P, et al. Murine precision-cut lung slices exhibit acute responses following exposure to gasoline direct injection engine emissions. Sci Total Environ. (2016) 568:1102–9. doi: 10.1016/j.scitotenv.2016.06.173
63. Tigges J, Worek F, Thiermann H, Wille T. Organophosphorus pesticides exhibit compound specific effects in rat precision-cut lung slices (PCLS): mechanisms involved in airway response, cytotoxicity, inflammatory activation and antioxidative defense. Arch Toxicol. (2022) 96:321–34. doi: 10.1007/s00204-021-03186-x
64. Desireddi JR, Farrow KN, Marks JD, Waypa GB, Schumacker PT. Hypoxia increases ROS signaling and cytosolic Ca(2+) in pulmonary artery smooth muscle cells of mouse lungs slices. Antioxid Redox Signal. (2010) 12:595–602. doi: 10.1089/ars.2009.2862
65. Wu W, Meng F, Zhang H, Tian H, Zhang X. Neutrophil PPIF exacerbates lung ischemia-reperfusion injury after lung transplantation by promoting calcium overload-induced neutrophil extracellular traps formation. Int Immunopharmacol. (2024) 142:113051. doi: 10.1016/j.intimp.2024.113051
66. Bai Y, Ai X. Utilizing the precision-cut lung slice to study the contractile regulation of airway and intrapulmonary arterial smooth muscle. J Vis Exp. (2022) (183):10.3791/63932. doi: 10.3791/63932-v
67. Bergner A, Sanderson MJ. Acetylcholine-induced calcium signaling and contraction of airway smooth muscle cells in lung slices. J Gen Physiol. (2002) 119:187–98. doi: 10.1085/jgp.119.2.187
68. Milara J, Ballester B, Morell A, Ortiz JL, Escrivá J, Fernández E, et al. JAK2 mediates lung fibrosis, pulmonary vascular remodelling and hypertension in idiopathic pulmonary fibrosis: an experimental study. Thorax. (2018) 73:519–29. doi: 10.1136/thoraxjnl-2017-210728
69. Perez JF, Sanderson MJ. The frequency of calcium oscillations induced by 5-HT, ACH, and KCl determine the contraction of smooth muscle cells of intrapulmonary bronchioles. J Gen Physiol. (2005) 125:535–53. doi: 10.1085/jgp.200409216
70. Capuzzimati M, Hough O, Liu M. Cell death and ischemia-reperfusion injury in lung transplantation. J Heart Lung Transplant. (2022) 41:1003–13. doi: 10.1016/j.healun.2022.05.013
71. Ruigrok MJR, Tomar J, Frijlink HW, Melgert BN, Hinrichs WLJ, Olinga P. The effects of oxygen concentration on cell death, anti-oxidant transcription, acute inflammation, and cell proliferation in precision-cut lung slices. Sci Rep. (2019) 9:16239. doi: 10.1038/s41598-019-52813-2
72. Dong M, Philippi C, Loretz B, Nafee N, Schaefer UF, Friedel G, et al. Tissue slice model of human lung cancer to investigate telomerase inhibition by nanoparticle delivery of antisense 2’-O-methyl-RNA. Int J Pharm. (2011) 419:33–42. doi: 10.1016/j.ijpharm.2011.07.009
73. van Rijt SH, Bölükbas DA, Argyo C, Datz S, Lindner M, Eickelberg O, et al. Protease-mediated release of chemotherapeutics from mesoporous silica nanoparticles to ex vivo human and mouse lung tumors. ACS Nano. (2015) 9:2377–89. doi: 10.1021/nn5070343
74. Le Prieur E, Vaz E, Bion A, Dionnet F, Morin JP. Toxicity of diesel engine exhausts in an in vitro model of lung slices in biphasic organotypic culture: induction of a proinflammatory and apoptotic response. Arch Toxicol. (2000) 74:460–6. doi: 10.1007/s002040000138
75. Schwab A, Rao Z, Zhang J, Gollowitzer A, Siebenkäs K, Bindel N, et al. Zeb1 mediates EMT/plasticity-associated ferroptosis sensitivity in cancer cells by regulating lipogenic enzyme expression and phospholipid composition. Nat Cell Biol. (2024) 26:1470–81. doi: 10.1038/s41556-024-01464-1
76. Bonniaud P, Fabre A, Frossard N, Guignabert C, Inman M, Kuebler WM, et al. Optimising experimental research in respiratory diseases: an ERS statement. Eur Respir J. (2018) 51:1702133. doi: 10.1183/13993003.02133-2017
77. Rosales Gerpe MC, van Vloten JP, Santry LA, de Jong J, Mould RC, Pelin A, et al. Use of precision-cut lung slices as an ex vivo tool for evaluating viruses and viral vectors for gene and oncolytic therapy. Mol Ther Methods Clin Dev. (2018) 10:245–56. doi: 10.1016/j.omtm.2018.07.010
78. Niehof M, Reamon-Buettner SM, Danov O, Hansen T, Sewald K. A modified protocol for successful miRNA profiling in human precision-cut lung slices (PCLS). BMC Res Note. (2021) 14:255. doi: 10.1186/s13104-021-05674-w
Keywords: precision cut lung slices, lung ischemia reperfusion injury, lung transplantation, primary graft dysfunction, inflammation, oxidative stress
Citation: Kollareth DJM and Sharma AK (2024) Precision cut lung slices: an innovative tool for lung transplant research. Front. Immunol. 15:1504421. doi: 10.3389/fimmu.2024.1504421
Received: 30 September 2024; Accepted: 11 November 2024;
Published: 28 November 2024.
Edited by:
Ilker Iskender, University Hospital Zürich, SwitzerlandReviewed by:
Pranali Nitin Shah, Brigham and Women’s Hospital and Harvard Medical School, United StatesPedro Augusto Reck Dos Santos, Mayo Clinic Arizona, United States
Copyright © 2024 Kollareth and Sharma. This is an open-access article distributed under the terms of the Creative Commons Attribution License (CC BY). The use, distribution or reproduction in other forums is permitted, provided the original author(s) and the copyright owner(s) are credited and that the original publication in this journal is cited, in accordance with accepted academic practice. No use, distribution or reproduction is permitted which does not comply with these terms.
*Correspondence: Ashish K. Sharma, YXNoaXNoLnNoYXJtYUBzdXJnZXJ5LnVmbC5lZHU=