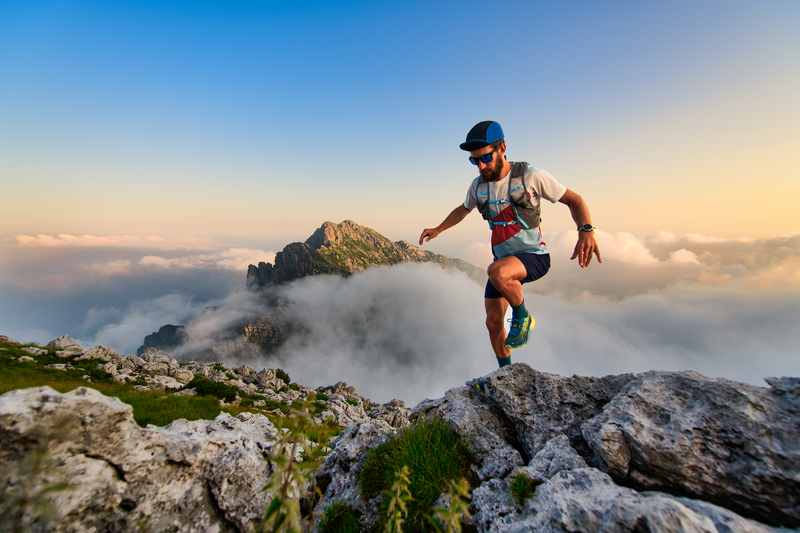
95% of researchers rate our articles as excellent or good
Learn more about the work of our research integrity team to safeguard the quality of each article we publish.
Find out more
REVIEW article
Front. Immunol. , 23 January 2025
Sec. Cancer Immunity and Immunotherapy
Volume 15 - 2024 | https://doi.org/10.3389/fimmu.2024.1498781
This article is part of the Research Topic Role of ncRNAs in Immunogenic Cell Death of Cancer View all 5 articles
Cancer immunotherapy has shown significant potential in treating several malignancies by stimulating the host immune system to recognize and attack cancer cells. Immunogenic cell death (ICD) can amplify the antitumor immune responses and reverse the immunosuppressive tumor microenvironment, thus increasing the sensitivity of cancer immunotherapy. In recent years, noncoding RNAs (ncRNAs) have emerged as key regulatory factors in ICD and oncologic immunity. Accordingly, ICD-related ncRNAs hold promise as novel therapeutic targets for optimizing the efficacy of cancer immunotherapy. However, the immunomodulatory properties of ICD-related ncRNAs have not yet been comprehensively summarized. Hence, we summarize the current knowledge on ncRNAs involved in ICD and their potential roles in cancer immunotherapy in this review. It deepens our understanding of ncRNAs associated with ICD and provides a new strategy to enhance cancer immunotherapy by specifically targeting the ICD-related ncRNAs.
Over the past few decades, there has been a prospective development in cancer treatment, mainly oriented towards immunotherapy, replacing or combining with classical treatment regimens (1). Cancer immunotherapies mainly include immune checkpoint inhibitors, therapeutic vaccines, and adoptive cell therapies, all of which have been successfully implemented in clinical settings (2–6). However, only a small subset of patients benefit from cancer immunotherapies based on these strategies, while other patients experience low response rates or suffer from immunotherapy-related toxicities (7, 8). Therefore, advanced strategies to break the existing bottleneck of cancer immunotherapy are urgently required.
Immunogenic cell death (ICD) is a modality of regulated cell death triggered by cytotoxic treatments, which serves as the initial signal for the antitumor immune responses in immunocompetent hosts (9). It involves the release of damage-associated molecular patterns (DAMPs) and tumor-associated antigens (TAAs) from stressed or dying cells, which enhances antitumor immune responses (10). In cancer immunotherapy, ICD not only eliminate cancer cells directly but also transforms dying tumor cells into a source of antigens, thereby activating dendritic cells and cytotoxic T-cell responses (11, 12). Furthermore, ICD promotes long-lasting antitumor immune responses by reversing the immunosuppressive tumor microenvironment and synergizing with immune checkpoint inhibitors, making it a cornerstone for developing next-generation cancer immunotherapies (9). Accordingly, the concept of ICD reveals the immunogenic capacity of cancer cells and introduces a new paradigm in cancer immunotherapy (13).
To date, shreds of evidence have manifested that noncoding RNAs (ncRNAs) are the hubs of ICD-related molecular communication networks (14, 15). NcRNAs refer to those RNA molecules that do not participate in protein-coding and have been revealed to regulate various cellular processes in both developmental and pathological conditions (16). NcRNAs are categorized into long noncoding RNAs (lncRNAs), microRNAs (miRNAs), circular RNAs (circRNAs), small interfering RNAs (siRNAs), and Piwi-interacting RNAs (piRNAs) based on their length, size, shape, and cellular functions (17). Among these, miRNA, lncRNA and circRNA are the main types of ncRNA that exert multiple effects on the complex regulation of ICD and antitumor immune response (Figure 1) (16). Mature miRNAs are single-stranded ncRNA with a length of approximately 22 nucleotides and participate in antigen presentation, immune cell infiltration, and endoplasmic reticulum stress-mediated ICD amplification (18). LncRNAs, a subset of regulatory ncRNAs with a length of more than 200 nt, serve as hinges bridging ICD and antitumor immunity, and can serve as prognostic biomarkers in hepatocellular carcinoma and lung adenocarcinoma (19–21). CircRNAs are endogenous ncRNAs characterized by a covalently closed loop structure, lacking the 3′poly(A) tail and the 5′-cap, which modulate metabolic reprogramming and reshape the immune landscape (22). In cancer immunotherapy, ICD-related ncRNAs regulate important pathways involved in ICD, including the release of DAMPs, the recruitment of immune cells, and the reprogram of the tumor immune microenvironment (18, 23). Therefore, targeting ICD-related ncRNAs can enhance the immunogenicity of tumor cells, overcome resistance to conventional therapies, and improve the effectiveness of classical immunotherapies such as anti-PD-1 immunotherapy. This innovative strategy offers a promising opportunity for developing personalized and effective cancer immunotherapies.
Figure 1. Biosynthesis of ncRNAs. (miRNA, MicroRNA; lncRNA, Long non-coding RNA; circRNA, Circular RNA).
Taken together, emerging evidence reveals the untapped potential of ncRNAs in inducing ICD and triggering antitumor immune responses. Nevertheless, there is a lack of systematic elaboration on the immunomodulatory properties of ICD-related ncRNAs. Consequently, this review aims to systematically summarize ICD-related ncRNAs, elaborate the regulatory network underlying ncRNA involvement in ICD, and explore their prospective functions in cancer immunotherapy. We hope this review will deepen our understanding of ICD-related ncRNAs and provide new strategies to maximize the efficiency of cancer immunotherapy by specifically targeting the ICD-related ncRNAs.
Stressed or dying cells release cytokines and damage-associated molecules that interact with organisms to indicate the immunogenicity of cell death (24). In the absence of reactive epitopes, these signaling molecules may drive apoptosis rather than adaptive immunity; however, in the case of malignant cells with sufficient antigenicity, they execute an immunogenic death mode, leading to cytotoxic lymphocyte-mediated immune response. This suggests that ICD inducers provide new opportunities for cancer immunotherapy. In cancer immunotherapy, ICD inducers stimulate tumor cells to release signals that activate resident immune cells and recruit inflammatory cells, thereby enhancing the antitumor immune response (Figure 2) (25). Simultaneously, numerous DAMP signals (such as calreticulin and high mobility group protein 1), inflammatory factors (such as interferons and interleukins) and tumor antigens are present in a spatiotemporally dependent manner, corresponding to the specific antitumor immune response (26).
Figure 2. Mechanism underlying ICD in cancer immunotherapy. ICD inducers induce dying tumor cells release damage-associated molecular patterns (DAMPs), such as adenosine triphosphate (ATP), and high-mobility group box 1 (HMGB1), which activate dendritic cells (DCs), T cells and other immune cells, promoting antigen presentation and antitumor immune response. TCR, T cell receptor; MHC, major histocompatibility complex class I.
The ability to initiate ICD is contingent upon the capacity to induce endoplasmic reticulum (ER) stress, as the exposure of ER chaperones to the outer plasma membrane is a fundamental event in ICD induction (24). Type I ICD inducers (e.g., chemotherapy) initiate ICD through non-ER pathways but prompt ICD-related immunoreactivity through secondary or “side” effects of ER stress; type II ICD inducers (e.g., photodynamic therapy) selectively target ER components to induce ICD by directly mediating ER stress (27). Under the process of ICD inducers, damage-associated molecular patterns (DAMPs) promote conversion in the tumor microenvironment from a non-inflammatory “cold” immune state to an inflammatory “hot” immune state by coordinating elaborate information transmission between cancer cells and immune cells (26).
According to prior research, DAMPs exposure trigger ICD by functioning as “find-me,” “eat-me,” and “danger” signals (24). (1) “find-me” signal: it mainly refers to adenosine triphosphate (ATP) released by tumor cells, which recruits macrophages, etc., to seek out damage sites, leading to the secretion of pro-inflammatory cytokines and activation of subsequent adaptive immunity; (2) “eat-me” signal: it mainly refers to the exposure of the ER molecular chaperone calreticulin on the surface of dying cells, which increases the phagocytosis and cross-presentation of tumor antigens in the participation of major histocompatibility complex class I (MHC-I) to activate antigen-specific T cell responses; (3) “danger” signals: it mainly refers to high mobility group protein 1 (HMGB1) released by tumor cells, which increases the binding affinity of transcription factors and DNA by bending or twisting the DNA double helix, regulates the immunogenicity of tumor cells, and promotes the subsequent inflammatory response and ICD. In addition, recent studies suggest that mitochondrial DNA (mtDNA) functions as a DAMP signal, connecting cellular stress to ICD-mediated antitumor immunity (28, 29). Under conditions of cellular stress, mtDNA is released into the cytoplasm and induces ICD through several pathways, including the cGAS-STING signaling pathway, toll-like receptor 9 (TLR9), and the NOD-like receptor family pyrin domain-containing 3 (NLRP3) inflammasome, thereby enhancing the immune response (30; Wang et al., 2023). In short, the DAMPs series signals released by tumor cells are crucial for initiating and expanding the ICD cascade.
The first event of the ICD cascade is the exposure of calmodulin to the surface of dying cells, which is commonly located in the lumen of ER and translocates within hours of ICD inducer stimulation (31). Subsequently, another hallmark of ICD that can be observed after calmodulin exposure is the translocation of heat shock proteins (HSPs), which triggers the activation and maturation of DCs (32). After 12 hours of exposure to calmodulin, the second event of the ICD cascade started. HMGB1 is released into the extracellular space and binds to Toll-like receptors (TLRs), ensuring the optimal processing and presentation of tumor antigens by DCs (33). The final event in the process of ICD is the secretion of ATP into the extracellular space, which serves as a “find-me” signal (24). Dying cells mark their presence with “find-me” signals so that phagocytes can quickly recognize and efficiently eliminate them. Consequently, this immunological cascade specifically activates cytotoxic T cells to induce immunogenic death against cancer cells and reprograms the “immune infiltration” microenvironment, which improves the clinical effect of cancer immunotherapy (11).
Emerging evidence suggests that ncRNAs with immunogenic properties are involved in multiple processes of T cell-dependent ICD (34). ICD-related ncRNAs can recruit cytotoxic T cells to the tumor site and participate in the reprogramming of the “immune infiltration” microenvironment (35). Therefore, ICD-related ncRNAs possess the capability to optimize the clinical efficacy of cancer immunotherapy by reversing the immunosuppressive system and amplifying immunogenic effects.
DAMPs are messenger molecules emitted by stressed dying cells, fulfilling the role of “danger signal” or immune adjuvant (36). While undergoing ICD, the released DAMPs efficiently enhance the immunogenicity of cells and are recognized by innate pattern recognition receptors (PRRs), thus facilitating the recruitment and maturation of antigen-presenting cells and activating adaptive immune responses against neoplastic antigens (11). With the broadening of the research on DAMPs, the molecular types of DAMPs are constantly expanded. At present, the molecules that have been encompassed in the category of DAMPs include calreticulin, heat-shock protein (HSP), HMGB1, ATP, type I interferons (IFNs) and mitochondrial DNA (mtDNA), all of which are closely related to ICD (36). In this section, we provide an overview of only a few DAMPs that have been extensively studied and discuss the related ncRNAs (Figure 3).
Figure 3. DAMPs (calreticulin, HSP, HMGB1, ATP, type I IFNs and mtDNA) in the ICD process and their related ncRNAs.
Calreticulin is an evolutionarily conserved DAMP that resides in the lumen of the ER (37). As an essential ER molecular chaperone, it is closely related to Ca2+ homeostasis, assembly of MHC-I molecules, antigen loading and immunogenic cell death (38). When cells are exposed to stress factors such as ICD inducers, calreticulin is transferred to the cell membrane surface, where it acts as an “eat me” signal to interact with CD91 and TLR4 for promoting phagocytosis mediated by antigen-presenting cells (APCs), ultimately triggering the cross-presentation of antigens and the initiation of T cell-dependent antitumor immune response (13). It has been confirmed that calreticulin exposure is a prerequisite for ICD and the subsequent specific oncologic immunity, suggesting that calmodulin-related ncRNAs are potential targets for cancer immunotherapy.
Truxova et al. found that calreticulin exposure increases the aggregation of CD11c+CD14+ marrow cell populations in tumor microenvironment, thereby enhancing the phagocytic and cytotoxic effects of natural killer (NK) cells on acute myeloid leukemia cells (39). From the viewpoint of epigenetic mechanisms, many ncRNAs (including miR-115, miR-378, miR-146a, miR-27a-5p, miR-30c, miR-29b, miR-15/16, miR-362-5p, lnc-CD56 and lnc-Rroid loci) are involved in the process of NK cells-mediated immune response (40). According to these observations, a probable signaling overlap between ncRNAs and calreticulin- mediated ICD is implied.
HSPs (mainly HSP70 and HSP90) are also typical DAMPs and are considered to be important signals that mediates the ICD of cancer cells (41). HSPs are exposed on the cell surface in the form of a high-immunogenicity complex at the early stage of ICD and dictates the immunogenicity of cancer cells (42). Donatella et al. found that the high expression of HSP under stress promotes the cross-presentation function of APCs, thus enhancing immunogenicity (43). Furthermore, HSP plays a chemokine-like role in recruiting immune cells (44). It promotes DCs to secrete pro-inflammatory cytokines such as IL-6, IL-12, and granulocyte-macrophage colony-stimulating factors, further strengthening the ICD-induced immune response (44).
Multiple lines of evidence indicate that ncRNAs are relevant to HSP, revealing the importance of ncRNAs in immunogenicity and anti-tumor immune response (45). Regarding HSP-related miRNAs: miR-29a and miR-214 are positively correlated with HSP90; miR-134-5p, miR-223, and miR-361-3p are negatively correlated with HSP90. These results indicate that HSP-related ncRNAs fulfill a multifaceted role in ICD, suggesting that targeted strategies should be designed according to actual clinical conditions. On the subject of lncRNAs, LIMIT (lncRNA inducing MHC-I and immunogenicity of tumor) disrupts the effects of HSP and leads to the subsequent transcription of the MHC-I machinery, so it is claimed to have a cancer immunogenic function (46). Accordingly, LIMIT and other HSP-related ncRNAs conceptually show therapeutic promise in ICD-mediated cancer immunotherapy.
HMGB1 is a widely expressed non-histone nuclear protein that participates in maintaining nucleosome structure and regulating genetic processes (47). What’s more, studies have shown that the release of HMGB1 from the nucleus into the extracellular fluid of pre-apoptotic or dying cells is one of the prominent attributes of ICD (48). During the ICD process, the translocated HMGB1 couples with TLR4 on the surface of DCs to improve the efficient processing and cross-presentation of tumor-associating antigens before apoptosis, thereby activating specific T-cell immune responses (49). In cancer immunotherapy models, knocking out HMGB1 in tumor cells or neutralizing HMGB1 with specific antibodies can notably reduce the tumor-suppressing capacity (50). From a clinical perspective, interfering with the functions of HMGB1 renders breast cancer patients with TLR4 allele loss more susceptible to relapse after treatment (51).
Considering the significant role of HMGB1 in the process of ICD, HMGB1-related ncRNAs are regarded as potential targets for inducing oncologic immunogenicity. For example, lncRNA MALAT1, lncRNA TUG1, lncRNA ZEB2-AS1, lncRNA PCAT1, miR-372-3p, miR-577 and miR–142-3p are upstream targets of HMGB1, suggesting that these ncRNAs are associated with ICD (52). Among these, miR-142-3p, which regulates HMGB1 formation, has been shown to fulfill an important immunomodulatory role in various cancers, such as breast cancer, lung cancer, colorectal cancer and gastric cancer (53). LncRNA OIP5-AS1 was demonstrated to reduce inflammation by upregulating HMGB1, and lncRNA ZEB2-AS1 depletion was demonstrated to inhibit the invasion of pancreatic cancer cells by suppressing HMGB1 (54, 55). These results point to new possibilities: (1) ncRNAs associated with HMGB1 and ICD can serve as therapeutic targets for tumors; (2) cascade amplification of ICD effects can be achieved by targeting ncRNAs, thereby expanding tumor immunotherapy.
When ICD occurs, ATP is secreted from dying cells in an autophagy-dependent and pannexin-1-mediated manner (56). The secreted ATP is an important indicator that mediates ICD and serves as an “eat-me” signal to swiftly recruit DCs and macrophages to the neoplastic region (57). In detail, ATP binds to purinergic receptor P2Y2 (P2RY2) on the cellular surface, promoting the infiltration of tumor-infiltrating myeloid cells into the neoplastic area (57). In addition, the released ATP by dying tumor cells fosters the formation of caspase-1-dependent NLRP3 inflammasome in DCs and promotes the secretion of IL-1β, further stimulating antitumor immune responses (13). It is worth noting that although extracellular ATP plays an essential role in the ICD process, non-immunogenic cell death patterns also lead to the release of ATP. Hence, the release of ATP cannot be used as the exclusive indicator of ICD, and it needs to be combined with other DAMPs to monitor the occurrence of ICD more accurately.
ATP is an essential substance in cells, and the molecular regulatory network related to ATP is extremely complex (58). Consequently, ncRNAs are extensively implicated in the multifaceted network of ATP. For example, mitochondrial ncRNAs such as miR-5787, miR-2392, lncRNA MDL1, LIPCAR are directly involved in the production of ATP in mitochondria; lncRNA kcnq1ot1 and miR-378a regulate ATP synthase through the competing endogenous RNAs (ceRNA) axis in type 2 diabetic heart; and lncRNA glycoLINC enhances glycolytic flux to increase ATP production (59–61). It is speculated that ATP-related ncRNAs may exert immunostimulatory effects in cancer immunotherapy through the DAMP-ICD axis. Disconsolately, there is no convincing evidence to straightforwardly indicate that ncRNAs induce ICD by ATP-mediated immunogenicity, and further research is needed to explain this issue.
Tumor cells undergoing ICD secrete type I IFNs through the activation of TLR3 by cyclic guanosine monophosphate-adenosine monophosphate synthase/stimulator of interferon genes (cGAS/STING) signaling pathway (62). The secreted type I IFNs can couple with homodimeric or heterodimeric receptors expressed on the surface of immune cells and exert immunostimulatory effects (63). In addition to direct immunostimulatory effects, type I IFNs can couple with IFN-α and IFN-β receptors on the surface of tumor cells, triggering the recruitment of T cells in the tumor by autocrine and paracrine signaling pathways (64). Moreover, studies have found that the induction of ICD in tumors driven by anthracyclines and radiotherapy are forcefully dependent on type I IFNs (65). Therefore, type I IFNs and their relevant indicators can be utilized to evaluate the ICD of tumor cells and predict the clinical response of anthracycline-based chemotherapy.
Type I IFNs have been evidenced to have functional interactions with miR-218, miR-744, miR-155, linc00513, Lnc10, and NR_033736, suggesting that these ncRNAs are involved in the induction of ICD in tumors (66). Given that type I IFNs are considered notable ICD inducers for anthracycline-based chemotherapy, type I IFNs-related ncRNAs have received widespread attention as sensitizing or prognostic molecules for anthracycline-based chemotherapy.
Mitochondria are specialized cellular organelles that contain one or more copies of their own genome (mtDNA) (67). When mitochondria are damaged or stressed, mtDNA can be released from mitochondria into the cytoplasm and participates in ICD activation as DAMP (28). The main mechanisms by which mtDNA induces ICD in tumor immunotherapy involve the cGAS-STING signaling pathway, toll-like receptor 9, and NLRP3 inflammasome (68). Specifically, (1) mtDNA is recognized by cGAS in the cytoplasm to activate the STING pathway, which then mediates the production of type I IFNs; (2) mtDNA with CpG-rich islands can be recognized by TLR9 to trigger the NF-κB signaling pathway, leading to the release of proinflammatory cytokines such as TNFα and IL-6; (3) mtDNA directly activates the NLRP3 inflammasome to induce the activation of caspase-1, which in turn to promote the release of proinflammatory cytokines (28, 69). Taken together, mtDNA functions as a critical immunoregulatory factor by activating ICD through multiple pathways in response to cellular stress and damage.
In particular, ncRNAs involved in the epigenetic regulation of mtDNA can be encoded by the mitochondrial genome (70). For example, MDL1, which mostly covers the entire mitochondrial D-loop region of the human mtDNA, and MDL1AS, which is the antisense transcript of MDL1, play noteworthy roles in mito-nuclear crosstalk and mtDNA regulation (71). Based on the above background, we support that mtDNA is an important feature of the ICD-corresponded immunogenicity and speculate that mtDNA-related ncRNAs are potential triggers of ICD.
The tumor microenvironment can provide a supportive environment for the occurrence of ICD and is a determinant of tumor eradication or survival (72). As a complex and heterogeneous milieu, the tumor microenvironment is composed of surrounding blood vessels, the extracellular matrix (ECM), cancer-associated cells, immune cells, and multiple signaling molecules (73). Among them, the dynamic interaction between ncRNAs and immune cells provides the prerequisite signal for ICD of tumor cells. Hence, this section intends to provide an overview of ncRNAs in the context of the tumor microenvironment and respectively summarize the contributions of ncRNAs in the crosstalk between immune cells and tumor cells, illustrating the relationship of ncRNAs with ICD.
Originally, miRNA molecules are transcribed by RNA polymerase II to primary miRNA (pri-miRNA) with a typical stem-loop structure, and then this pri-miRNA is cleaved to a precursor miRNA (pre-miRNA) of approximately 70 bases by Drosha in the nucleus (74). Then, the pre-miRNA is transported into the cytoplasm by exportin5 (Exp5)-ras-related nuclear protein (RAN)-guanosine-5’-triphosphate (GTP) complex and processed to double-stranded miRNA by Dicer (74). One strand of the double-stranded miRNA is degraded, and the remaining “mature” strand assembles with Argonaute to form the RNA-induced silencing complex (RISC) (75). Consequently, the miRNA-RISC is pulled towards complementary sites in the 5’ or 3’ untranslated regions of target mRNAs, resulting in mRNA degradation or translational repression (76). In addition to fulfilling the role of mRNA decoys, miRNAs can also serve as agonists for TLRs, thereby activating downstream effects such as ICD (77).
In the immune microenvironment, NK cells are a type of cytotoxic lymphocyte in the innate immune system that promptly takes action upon identifying “danger” signals (78). NK cells not only have direct cytotoxicity against tumor cells, but also secrete cytokines and chemokines to evoke anti-tumor immune response (79). Furthermore, Kübler et al. have demonstrated that NK cells eradicate ER-stressed cells by calreticulin and receptor NKp46, suggesting that NK cells have a synergistic effect on ICD (80). Studies based on miRNA have shown that miR-30e regulates the cytotoxicity of type I IFN-activated NK cells by targeting perforin (81); highly expressed miR-155 enhances the activity of NK cells and induces the secretion of the immunostimulatory cytokine interferon-γ by regulating hematopoietic-specific inositol phosphatase 1 (82). It is noteworthy that miR-155 is the most-studied miRNA in the immune microenvironment and has been shown to be aberrantly expressed in a variety of tumors, as shown in Figure 4 (83). In addition to the NK cells mentioned above, miRNA can also regulate macrophages to regain their tumor-killing ability (84). That said, these existing studies of miRNAs in TLRs, immune cells, and the tumor microenvironment at least suggest their therapeutic promise in ICD-mediated cancer immunotherapy.
The best-described function of lncRNAs in the tumorigenesis and development of tumors is to regulate gene expression through multiple mechanisms, such as reshaping chromatin and genome architecture, epigenetic regulation, and posttranscriptional processing (85). Moreover, recent studies have shown that lncRNAs not only regulate the recruitment and function of immune cells, such as macrophage polarization and T cell activation, but also regulate cytokines and related pathways, further remodeling the tumor immune microenvironment and activating anti-tumor immune responses (35). For example, lincRNA-Cox2 regulates the expression of many immune-related genes in response to activation of toll-like receptor signaling (86); lnc-DC in the tumor microenvironment regulates the differentiation of DCs and stimulates T cell activation during antitumor immune response (87); overexpression of lncRNA GAS5 amplifies the killing effect of NK cells on liver cancer by regulating miR-544/RUNX3 (88). Based on the above, one possible area for cancer immunotherapy is targeting immunogenic lncRNAs in the tumor microenvironment to initiate ICD.
Li et al. identify the first immunogenic lncRNA in cancer, establishing a direct link between lncRNAs and ICD for the first time (46). In this study, Li et al. divided melanoma lesions into “hot tumors” with a considerable number of T cell infiltrations and “cold tumors” with only a minor amount of T cell infiltrations, and studied the expression differences of lncRNAs in the two groups. Through functional analysis, one of the lncRNAs enriched in hot tumors was found to have the function of regulating MHC-I expression and tumor immunogenicity, and it was named LIMIT (lncRNA inducing MHC-I and immunogenicity of tumor). Furthermore, clinical analysis showed that the expression level of LIMIT was positively correlated with T cell infiltration, MHC-I antigen presentation, and IFNγ signaling pathway in cancers; patients with high levels of LIMIT expression had improved survival rates and response rates to immunotherapy. In terms of molecular mechanism, LIMIT cis-activates the guanylate binding protein (GBP) gene cluster to bind to HSP90, a type of DAMP, thereby initiating the downstream ICD effect (Figure 5). Taken together, the discovery of immunogenic lncRNAs supports the elucidation of the complex ICD mechanism and guides ICD-related lncRNAs to illuminate cancer immunotherapy.
Figure 5. LIMIT mediates ICD as an immunogenic lncRNA in the tumor immune microenvironment. Stimulated by IFNγ, LIMIT cis-activates the guanylate-binding protein (GBP) gene cluster, leading to the subsequent interaction of GBPs with the HSP90, which releases heat shock factor-1 (HSF1) in the cytoplasm and initiates the downstream ICD effect.
The evolutionary conservation of circRNAs generally suggests that they perform certain essential functions. Current studies have uncovered that circRNAs serve a regulatory function in physiological and pathological processes through various pathways, mainly including acting as miRNA sponges, interacting with proteins, coupled with regulating transcription, splicing and translation (89). In the tumor microenvironment, circRNAs can intervene in the activity of immune-related cells or the expression of programmed death-ligand 1(PD-L1) to mediate tumor immune surveillance (90). For example, cancer cell-derived exosomal circUHRF1 exerted tumor immunosuppression by inducing NK cell exhaustion and reducing sensitivity to programmed cell death protein 1 (PD-1) blockade immunotherapy in hepatocellular carcinoma (HCC) (91); increased circFAT1 coordinately controlled cancer stemness and immune evasion through promoting signal transducer and activator of transcription 3 (STAT3) activation and inhibiting CD8+ T cell infiltration into the tumor microenvironment (92). Thus, the evidence above suggests that targeting immune-related cells and the PD-1/PD-L1 pathway through circRNAs could be a prospective orientation for cancer immunotherapy.
Furthermore, immunogenic circRNAs can act as tumor antigens or be mediated to express tumor-specific antigens for ICD-related immune responses. CircFam53b is a tumor-specific circular RNA that encodes a highly immunogenic cryptic antigen with a strong affinity for human leukocyte antigen (HLA), which causes massive infiltration of CD8+ T cells and induces ICD-related immune response (93). Vaccines based on circFam53b or its encoded cryptic antigen can promote antigen presentation and motivate ICD-related immunity, thereby effectively controlling the progression of breast cancer and melanoma. Accordingly, vaccines based on tumor-specific circRNAs possess potent ICD effects, and further studies are required to evaluate the potential of immunogenic circRNAs in enhancing clinical outcomes.
In this section, we review the pros and cons of the therapeutic loss-of-function strategies based on ICD-related ncRNAs. In particular, we focus on antisense oligonucleotides, which have emerged as a leading candidate in ncRNA therapeutics due to their favorable pharmacological properties and programmable design. We also describe two competing drug modalities: small interfering RNAs (siRNAs) and miRNAs.
Antisense oligonucleotides represent the initial technology in using oligonucleotides for disease treatment and have been the most successful approach to gene therapy (94). They complementarily pair with messenger RNA through single-stranded nucleotides, blocking specific gene expression and protein synthesis (95). By designing different antisense oligonucleotides, it is possible to inhibit the functions of various messenger RNAs, thereby achieving the goal of disease treatment. The first antisense oligonucleotide drug approved is Fomivirsen, which was approved by the food and drug administration (FDA) in 1998 for the treatment of cytomegalovirus retinitis in acquired immune deficiency syndrome (AIDS) patients (96). In cancer immunotherapy, antisense oligonucleotides have the potential to directly target ncRNAs by designing precise molecules that reduce or inhibit the expression of specific ICD-related ncRNAs, thereby impacting immunogenicity.
siRNAs are double-stranded RNA molecules with 20-25 nucleotides in length, containing a guide strand and a passenger strand (97). The functional mechanism of siRNA drugs mainly involves the RNA interference (RNAi) pathway, which identifies and degrades complementary RNA, thereby silencing the expression of related proteins (98). At present, significant advances in the formulation of siRNAs have enabled long-term effectiveness in vivo, allowing dose adjustments every 6 months (99). In this context, siRNA offers the potential to target ICD-related ncRNAs directly, thereby impacting cancer immunotherapy. This involves designing precise molecules to decrease the expression of ncRNAs associated with ICD negatively and enhance the ICD effect.
Therapeutic strategies based on miRNA are mainly divided into two categories: miRNA antagonists and miRNA mimics (74). MiRNA antagonists are chemically synthesized miRNA passenger strands, also known as anti-miR or antagomiR (76). MiRNA antagonists can complement and pair with the active strand of miRNA with high affinity to form new double-stranded miRNA, which inhibits the function of miRNA through the RISC degradation pathway (76). The first miRNA antagonist to undergo clinical research is Miravirsen, an inhibitor that complements the 5’ end of miR-122 (100). Phase I and Phase II clinical trials have demonstrated the efficacy of Miravirsen in treating hepatitis C without serious side effects (101). According to this, we can design miRNA antagonists that target ICD-related ncRNAs to expand cancer immunotherapy by amplifying the immunogenicity of tumor cells.
In general, siRNA and antisense oligonucleotides have different characteristics in ncRNAs-mediated therapy, and it is difficult to foresee which one will become the dominant technology. The selection of each strategy should consider the unique biological characteristics and mode of action of the ICD-mediated ncRNAs comprehensively. Antisense oligonucleotides and siRNAs have the potential to directly target ncRNAs to reduce or inhibit the expression of specific ICD-related ncRNAs, thereby impacting the ICD effect. On the other hand, miRNA antagonists offer an alternative therapeutic approach by disrupting the interaction between ICD-related ncRNAs and their molecular partners or directly intervening in the structure of ICD-related ncRNAs.
Although some therapeutic ncRNAs have been approved for disease treatment, the clinical application of ncRNAs still faces the following challenges: (1) the naked single-stranded RNA molecules are easily degraded by nucleases in the physiological environment; (2) the low delivery efficiency and off-target effects are limitations of ICD-related ncRNAs; (3) ncRNAs are biological macromolecules with negatively charged, making it difficult to penetrate the anionic lipid bilayer and enter cells. In addition, it is worthy of attention to explore how to rationally harness the immunogenicity of ncRNAs in clinical applications to expand the efficacy of ICD. Therefore, it is necessary to design appropriate strategies for delivering ICD-related ncRNAs to the tumor microenvironment to fully realize the potential for cancer immunotherapy.
Exosomes are nanoscale vesicles secreted by cells to facilitate intercellular communication through delivering specific “cargo”, such as proteins, lipids, and nucleic acids (102). Properties of exosomes, such as excellent biocompatibility, minimal cytotoxicity, and high stability in circulation, make them attractive as ideal delivery systems for cancer therapy (103). For example, engineered exosomes can effectively encapsulate miR-140 and deliver it to chondrocytes, which has been shown to alleviate the progression of osteoarthritis (104). MiR-140 functions as an immune mediator that contributes to the suppressive effect of tumors and serves as a potential molecular target in cancer immunotherapy (105). Mechanistically, the immunomodulatory effects of miR-140 are associated with increased infiltration of cytotoxic T cells and decreased infiltration of myeloid-derived suppressor cells, suggesting that miR-140 is an ICD-related ncRNA (106). Accordingly, delivering ICD-related ncRNAs via engineered exosomes is theoretically feasible and is expected to inhibit tumor development through enhancing the immunogenicity of tumor cells. In addition, engineered exosomes have been shown to specifically induce ICD in drug-resistant tumors, thereby bypassing the apoptotic pathway to eliminate tumor-resistant cells efficiently (107). Therefore, ICD-related ncRNAs combined with exosome delivery vesicles have the potential to overcome the challenges and dilemmas of cancer immunotherapy.
Lipid-based ncRNAs delivery systems include anionic liposomes, neutral liposomes, cationic liposomes, and lipid particles (108). Encapsulating ncRNAs in anionic or neutral liposomes can prevent ncRNAs from nucleases-mediated degradation while remarkably improving their biocompatibility and pharmacokinetic properties (109). Although cationic liposomes have lower biocompatibility compared to other liposomes, they can enhance the enrichment of ncRNAs in the tumor microenvironment and facilitate the uptake of tumor cells (108). The current focus of liposome-delivered ncRNAs is primarily on miRNAs. For example, miRNA-29b, miRNA-101, miRNA-122, miR-181a, and miR-2000 are ncRNAs that have been successfully encapsulated in liposomes and have been shown to exert anti-tumor activity in lung cancer, hepatocellular carcinoma, and blood malignancies (110). Moreover, lipid particles-encapsulated siRNA targeting the cell-cycle proteins polo-like kinase 1 (PLK1) have been used in phase I and II clinical trials for patients with advanced solid tumors (111).
When it comes to lipid delivery systems, researchers are focusing on their adaptability and modifiability. Researchers anticipate that the engineered liposomes will not be selective for the encapsulated ncRNAs and will transport different ncRNAs with equal efficiency. In this context, ICD-related ncRNAs are expected to be efficiently delivered by liposomes to optimize cancer immunotherapy.
Polymer nanocarriers are biodegradable colloidal delivery systems widely used for the delivery of drug and ncRNAs (112). For example, CALLA-01, a water-soluble polycationic nanocarrier containing siRNA based on cyclodextrin polymer, is the first polymer nanoparticle used in clinical trials for tumor-targeted siRNA delivery (113). Engineered polymer nanoparticles function as immune adjuvants to enhance anti-tumor immune responses and have been demonstrated in tumor models (114). Using polymer nanoparticles to deliver ICD-related ncRNAs may achieve high encapsulation efficiency, sustained release behavior, and broaden the therapeutic effect of ICD.
The nucleic acid-conjugate delivery systems directly couple ncRNAs to the delivery material for obtaining single-component drug delivery systems with unequivocal properties (115). Given its inherent immunogenicity, tumor antigens offer an optional carrier for co-delivering ICD-related ncRNAs to create customized agonists that target anti-tumor immune responses.
In addition, although chemical modification cannot directly serve as a delivery system for ncRNAs, it can obviously improve the properties of the ncRNAs (116). Reasonable chemical modification can enhance the physiological stability and targeting of ncRNAs (117). Importantly, chemical modifications can improve the immunogenicity of ncRNAs, which is beneficial for stimulating anti-tumor immune response. Therefore, modifying ICD-related ncRNAs to amplify the immunogenic effect is a novel avenue to induce ICD and enhance cancer immunotherapy.
Utilizing gene therapy based on ICD-related ncRNAs can enhance cancer immunotherapy by strengthening immunogenic effects. However, due to the limited immunogenicity of monotherapy, it is necessary to combine it with other methods to induce ICD and further enhance the effectiveness of cancer immunotherapy. To date, studies have shown that various physical stimuli (such as photodynamic therapy, ionizing radiation, near-infrared photoimmunotherapy, high pressure, and hyperthermia) can induce the immunogenic death of tumor cells, which represents a new direction in exploring cancer immunotherapy for the future (24). Therefore, ICD-related ncRNAs combined with other pathways that induce immunogenic death of tumor cells could boost immunogenicity to expand anti-tumor immune responses, thereby improving the immunotherapeutic effect of tumor patients.
Conventional chemotherapy drugs are tremendously cytotoxic and concentrate on eliminating dividing tumor cells (118). The therapeutic strategy of inducing cell death appears reasonable, as inhibiting tumor development through programmed cell death (e.g., apoptosis, necroptosis, autophagy, ferroptosis, pyroptosis, etc.) has been extensively researched (119). However, the therapeutic strategy for modulating ICD in cancer immunotherapy differs from conventional strategies of triggering tumor cell death. The therapeutic strategy targeting ICD-related ncRNAs regulates multiple DMAPs in the tumor microenvironment to indirectly eliminate tumor cells, indicating that it could be utilized as a combined approach for cascade-enhanced tumor suppression. Hence, multi-modal therapeutic strategies based on ICD and other programmed cell death could eliminate tumor cells in a multipronged manner and effectively prevent tumor progression.
Emerging research in cancer immunotherapy attempts to reveal how ncRNAs and ICD synergistically enhance tumor immunogenicity and induce antitumor immune responses. However, much remains to be discovered about unknown ncRNAs, particularly ICD-related ncRNAs and their functional mechanisms. Even for the widely known ncRNAs, their roles in ICD and executed mechanisms could be altered with spatial-temporal adjustment. Therefore, efforts still need to analyze the expression levels and spatiotemporal regulation of ICD-related ncRNAs. With the continuous progress of sequencing technology, we expect that single-cell RNA sequencing will be integrated with new high-throughput techniques, such as spatial transcriptomics, to identify specific ICD-related ncRNAs with high sensitivity and precision. Moreover, existing research suggests that ICD plays a multifaceted role in the immune system, partially elucidating the ambiguity of ICD in cancer immunotherapy (120). This again underscores the requirement for further studies on the context-dependent role of ICD-related ncRNAs in cancer immunotherapy. In addition, evidence straightly supporting the effect of the ICD-related ncRNAs on cancer immunotherapy is currently unavailable but is theoretically reasonable. Accordingly, in vivo experiments and prospective clinical research are urgently needed to evaluate the clinical significance of ICD-related ncRNAs in cancer immunotherapy.
Immunogenic death is one of the modes of tumor cell death that can also occur throughout the body (121). Therefore, the systemic effects on the host immune system should be cautiously considered when conducting cancer immunotherapy based on ICD-related ncRNAs. Inspired by drug delivery systems, engineered ncRNAs with specific and efficient targeting capabilities are more optimized therapeutic tools. Furthermore, ICD is different from apoptosis induced by conventional chemotherapeutic drugs, indicating that ICD-related ncRNAs could be utilized as a combination strategy to improve the effectiveness of tumor eradication. Hence, precise identification of patients most likely to benefit from ICD-related ncRNAs-mediated cancer immunotherapy and enhanced tumor eradication through single or multimodal therapeutic strategies represent opportunities for antitumor therapy.
While ncRNAs have been shown to enhance ICD and improve the effectiveness of cancer immunotherapies, recent research suggests that some ncRNAs may confer resistance to ICD induction and chemotherapy (122, 123). Here, we provide a summary of how ncRNAs could contribute to such resistance: (1) suppressing the expression and/or release of DAMPs, thereby reducing ICD and contributing to resistance against cancer therapies; (2) inhibiting the expression of drug efflux pumps (e.g., ATP-binding cassette transporters) and anti-apoptotic proteins (e.g., Bcl-2), thereby reducing the susceptibility of tumor cells to chemotherapy and ICD; (3) constructing an immunosuppressive tumor microenvironment, thereby reducing the efficacy of ICD and chemotherapy.
Taken together, the future development of ICD-related ncRNAs will focus on the following aspects: (1) decoding more information about ncRNAs and identifying key ncRNAs involved in the ICD process; (2) establishing standardized ICD evaluation criteria to detect the effects of ICD-related ncRNAs; (3) analyzing the crosstalk between ncRNAs and ICD and devising personalized therapeutic strategies based on ICD-related ncRNAs; (4) developing the design, synthesis, modification and delivery technologies of ncRNAs to reduce off-target effects and improve the performance of ICD-related ncRNAs; (5) combining with other therapeutic strategies to improve the antitumor immune response and the effectiveness of tumor eradication; (6) classifying tumor patients according to genetic mapping and assessing the suitability of ICD-related ncRNAs for individual patients; (7) constructing an algorithm and/or ncRNA network to predict prognostic and therapeutic responses.
In summary, ICD is now recognized as a complex cell death program that serves as a cornerstone of cancer immunotherapy. ICD-related ncRNAs present therapeutic potential as immunoregulation signals, providing opportunities to enhance antitumor immune responses in difficult-to-treat malignancies. Furthermore, in-depth research of ICD-related ncRNAs is advantageous in monitoring and evaluating the antitumor immune response. In the future, targeting ICD-related ncRNAs through single or combined pathways should be considered as a new paradigm for cancer immunotherapy and a complement to precision medicine, which may improve the outcomes of current cancer treatments.
GS: Investigation, Visualization, Writing – original draft, Writing – review & editing. LH: Conceptualization, Supervision, Writing – review & editing.
The author(s) declare that no financial support was received for the research, authorship, and/or publication of this article.
The authors declare that the research was conducted in the absence of any commercial or financial relationships that could be construed as a potential conflict of interest.
All claims expressed in this article are solely those of the authors and do not necessarily represent those of their affiliated organizations, or those of the publisher, the editors and the reviewers. Any product that may be evaluated in this article, or claim that may be made by its manufacturer, is not guaranteed or endorsed by the publisher.
1. Ganesh K, Stadler ZK, Cercek A, Mendelsohn RB, Shia J, Segal NH, et al. Immunotherapy in colorectal cancer: rationale, challenges and potential. Nat Rev Gastroenterol Hepatol. (2019) 16:361–75. doi: 10.1038/s41575-019-0126-x
2. June CH, O’Connor RS, Kawalekar OU, Ghassemi S, Milone MC. CAR T cell immunotherapy for human cancer. Sci (New York N.Y.). (2018) 359:1361–5. doi: 10.1126/science.aar6711
3. Ribas A, Wolchok JD. Cancer immunotherapy using checkpoint blockade. Sci (New York N.Y.). (2018) 359:1350–5. doi: 10.1126/science.aar4060
4. Zhang Y, Zhang Z. The history and advances in cancer immunotherapy: understanding the characteristics of tumor-infiltrating immune cells and their therapeutic implications. Cell Mol Immunol. (2020) 17:807–21. doi: 10.1038/s41423-020-0488-6
5. Lin MJ, Svensson-Arvelund J, Lubitz GS, Marabelle A, Melero I, Brown BD, et al. Cancer vaccines: the next immunotherapy frontier. Nat Cancer. (2022) 3:911–26. doi: 10.1038/s43018-022-00418-6
6. Dagher OK, Schwab RD, Brookens SK, Posey AD. Advances in cancer immunotherapies. Cell. (2023) 186. doi: 10.1016/j.cell.2023.02.039
7. Das S, Johnson DB. Immune-related adverse events and anti-tumor efficacy of immune checkpoint inhibitors. J For Immunotherapy Cancer. (2019) 7:306. doi: 10.1186/s40425-019-0805-8
8. Kennedy LB, Salama AKS. A review of cancer immunotherapy toxicity. CA: Cancer J For Clin. (2020) 70:86–104. doi: 10.3322/caac.21596
9. Galluzzi L, Guilbaud E, Schmidt D, Kroemer G, Marincola FM. Targeting immunogenic cell stress and death for cancer therapy. Nat Rev Drug Discovery. (2024) 23:445–60. doi: 10.1038/s41573-024-00920-9
10. Kroemer G., Galassi C., Zitvogel L, Galluzzi L.. Immunogenic cell stress and death. Nat Immunol. (2022) 23(4):487–500. doi: 10.1038/s41590-022-01132-2
11. Krysko DV, Garg AD, Kaczmarek A, Krysko O, Agostinis P, Vandenabeele P. Immunogenic cell death and DAMPs in cancer therapy. Nat Rev Cancer. (2012) 12:860–75. doi: 10.1038/nrc3380
12. Galluzzi L, Buqué A, Kepp O, Zitvogel L, Kroemer G. Immunogenic cell death in cancer and infectious disease. Nat Rev Immunol. (2017) 17:97–111. doi: 10.1038/nri.2016.107
13. Ahmed A, Tait SWG. Targeting immunogenic cell death in cancer. Mol Oncol. (2020) 14:2994–3006. doi: 10.1002/1878-0261.12851
14. Cai C, Shu K, Chen W, Ding J, Guo Z, Wei Y, et al. Construction and validation of a model based on immunogenic cell death-associated lncRNAs to predict prognosis and direct therapy for kidney renal clear cell carcinoma. Aging. (2023) 15:5304–38. doi: 10.18632/aging.204741
15. Sun D, Zhang C. Immunogenic cell death-related long noncoding RNA influences immunotherapy against lung adenocarcinoma. Oncol Res. (2023) 31:753–67. doi: 10.32604/or.2023.029287
16. Zhang Y, Mao Q, Xia Q, Cheng J, Huang Z, Li Y, et al. Noncoding RNAs link metabolic reprogramming to immune microenvironment in cancers. J Hematol Oncol. (2021) 14:169. doi: 10.1186/s13045-021-01179-y
17. Hombach S, Kretz M. Non-coding RNAs: classification, biology and functioning. Adv In Exp Med Biol. (2016) 937:3–17. doi: 10.1007/978-3-319-42059-2_1
18. Azizi M, Salehi-Mazandarani S, Nikpour P, Andalib A, Rezaei M. The role of unfolded protein response-associated miRNAs in immunogenic cell death amplification: A literature review and bioinformatics analysis. Life Sci. (2023) 314:121341. doi: 10.1016/j.lfs.2022.121341
19. He M, Gu W, Gao Y, Liu Y, Liu J, Li Z. Molecular subtypes and a prognostic model for hepatocellular carcinoma based on immune- and immunogenic cell death-related lncRNAs. Front In Immunol. (2022) 13:1043827. doi: 10.3389/fimmu.2022.1043827
20. Chen W, Shu K, Cai C, Ding J, Zhang X, Zhang W, et al. Prognostic value and immune landscapes of immunogenic cell death-related lncRNAs in hepatocellular carcinoma. Bioscience Rep. (2023) 43. doi: 10.1042/BSR20230634
21. Shu K, Cai C, Chen W, Ding J, Guo Z, Wei Y, et al. Prognostic value and immune landscapes of immunogenic cell death-associated lncRNAs in lung adenocarcinoma. Sci Rep. (2023) 13:19151. doi: 10.1038/s41598-023-46669-w
22. Li C, Wang X, Chen T, Li W, Zhou X, Wang L, et al. Huaier induces immunogenic cell death via circCLASP1/PKR/eIF2α Signaling pathway in triple negative breast cancer. Front In Cell Dev Biol. (2022) 10:913824. doi: 10.3389/fcell.2022.913824
23. Wang Y, Yue T, He Q. Comprehensive analysis of ICD-related lncRNAs in predicting risk stratification, clinical prognosis and immune response for breast cancer. Aging. (2023) 15:8833–50. doi: 10.18632/aging.205002
24. Troitskaya OS, Novak DD, Richter VA, Koval OA. Immunogenic cell death in cancer therapy. Acta Naturae. (2022) 14:40–53. doi: 10.32607/actanaturae.11523
25. Zhou J, Wang G, Chen Y, Wang H, Hua Y, Cai Z. Immunogenic cell death in cancer therapy: Present and emerging inducers. J Cell Mol Med. (2019) 23:4854–65. doi: 10.1111/jcmm.14356
26. Ti D, Yan X, Wei J, Wu Z, Wang Y, Han W. Inducing immunogenic cell death in immuno-oncological therapies. Chin J Cancer Res = Chung-kuo Yen Cheng Yen Chiu. (2022) 34:1–10. doi: 10.21147/j.issn.1000-9604.2022.01.01
27. Krysko O, Løve Aaes T, Bachert C, Vandenabeele P, Krysko DV. Many faces of DAMPs in cancer therapy. Cell Death Dis. (2013) 4:e631. doi: 10.1038/cddis.2013.156
28. West AP, Shadel GS. Mitochondrial DNA in innate immune responses and inflammatory pathology. Nat Rev Immunol. (2017) 17:363–75. doi: 10.1038/nri.2017.21
29. Yin M, Dong J, Sun C, Liu X, Liu Z, Liu L, et al. Raddeanin A enhances mitochondrial DNA-cGAS/STING axis-mediated antitumor immunity by targeting transactive responsive DNA-binding protein 43. Advanced Sci (Weinheim Baden-Wurttemberg Germany). (2023) 10:e2206737. doi: 10.1002/advs.202206737
30. Heilig R, Lee J, Tait SWG. Mitochondrial DNA in cell death and inflammation. Biochem Soc Trans. (2023) 51:457–72. doi: 10.1042/BST20221525
31. Obeid M, Tesniere A, Ghiringhelli F, Fimia GM, Apetoh L, Perfettini J-L, et al. Calreticulin exposure dictates the immunogenicity of cancer cell death. Nat Med. (2007) 13:54–61. doi: 10.1038/nm1523
32. Pawaria S, Binder RJ. CD91-dependent programming of T-helper cell responses following heat shock protein immunization. Nat Commun. (2011) 2:521. doi: 10.1038/ncomms1524
33. Zitvogel L, Apetoh L, Ghiringhelli F, Kroemer G. Immunological aspects of cancer chemotherapy. Nat Rev Immunol. (2008) 8:59–73. doi: 10.1038/nri2216
34. Zhang W, Xu C, Yang Z, Zhou J, Peng W, Zhang X, et al. Circular RNAs in tumor immunity and immunotherapy. Mol Cancer. (2024) 23:171. doi: 10.1186/s12943-024-02082-z
35. Park E-G, Pyo S-J, Cui Y, Yoon S-H, Nam J-W. Tumor immune microenvironment lncRNAs. Briefings In Bioinf. (2022) 23:1–25. doi: 10.1093/bib/bbab504
36. Ma M, Jiang W, Zhou R. DAMPs and DAMP-sensing receptors in inflammation and diseases. Immunity. (2024) 57:752–71. doi: 10.1016/j.immuni.2024.03.002
37. Martins I, Kepp O, Galluzzi L, Senovilla L, Schlemmer F, Adjemian S, et al. Surface-exposed calreticulin in the interaction between dying cells and phagocytes. Ann New York Acad Sci. (2010) 1209:77–82. doi: 10.1111/j.1749-6632.2010.05740.x
38. Obeid M, Tesniere A, Ghiringhelli F, Fimia GM, Apetoh L, Perfettini J-L, et al. Calreticulin exposure dictates the immunogenicity of cancer cell death. Nat Med. (2007) 13:54–61. doi: 10.1038/nm1523
39. Truxova I, Kasikova L, Salek C, Hensler M, Lysak D, Holicek P, et al. Calreticulin exposure on Malignant blasts correlates with improved natural killer cell-mediated cytotoxicity in acute myeloid leukemia patients. Haematologica. (2020) 105:1868–78. doi: 10.3324/haematol.2019.223933
40. Yang C, Shen C, Feng T, Li H. Noncoding RNA in NK cells. J Leukocyte Biol. (2019) 105:63–71. doi: 10.1002/JLB.1RU0518-197RR
41. Stricher F, Macri C, Ruff M, Muller S. HSPA8/HSC70 chaperone protein: structure, function, and chemical targeting. Autophagy. (2013) 9:1937–54. doi: 10.4161/auto.26448
42. Milani V, Noessner E, Ghose S, Kuppner M, Ahrens B, Scharner A, et al. Heat shock protein 70: role in antigen presentation and immune stimulation. Int J Hyperthermia: Off J Eur Soc For Hyperthermic Oncology North Am Hyperthermia Group. (2002) 18:563–75. doi: 10.1080/02656730210166140
43. D’Eliseo D, Manzi L, Velotti F. Capsaicin as an inducer of damage-associated molecular patterns (DAMPs) of immunogenic cell death (ICD) in human bladder cancer cells. Cell Stress Chaperones. (2013) 18:801–8. doi: 10.1007/s12192-013-0422-2
44. Zininga T, Ramatsui L, Shonhai A. Heat shock proteins as immunomodulants. Molecules (Basel Switzerland). (2018) 23. doi: 10.3390/molecules23112846
45. Mattoo S, Gupta A, Chauhan M, Agrawal A, Pore SK. Prospects and challenges of noncoding-RNA-mediated inhibition of heat shock protein 90 for cancer therapy. Biochim Et Biophys Acta Gene Regul Mech. (2024) 1867:195006. doi: 10.1016/j.bbagrm.2024.195006
46. Li G, Kryczek I, Nam J, Li X, Li S, Li J, et al. LIMIT is an immunogenic lncRNA in cancer immunity and immunotherapy. Nat Cell Biol. (2021) 23:526–37. doi: 10.1038/s41556-021-00672-3
47. Xue J, Suarez JS, Minaai M, Li S, Gaudino G, Pass HI, et al. HMGB1 as a therapeutic target in disease. J Cell Physiol. (2021) 236:3406–19. doi: 10.1002/jcp.30125
48. Kepp O, Senovilla L, Vitale I, Vacchelli E, Adjemian S, Agostinis P, et al. Consensus guidelines for the detection of immunogenic cell death. Oncoimmunology. (2014) 3:e955691. doi: 10.4161/21624011.2014.955691
49. Troitskaya O, Golubitskaya E, Biryukov M, Varlamov M, Gugin P, Milakhina E, et al. Non-thermal plasma application in tumor-bearing mice induces increase of serum HMGB1. Int J Mol Sci. (2020) 21. doi: 10.3390/ijms21145128
50. Liu P, Zhao L, Loos F, Iribarren K, Lachkar S, Zhou H, et al. Identification of pharmacological agents that induce HMGB1 release. Sci Rep. (2017) 7:14915. doi: 10.1038/s41598-017-14848-1
51. Apetoh L, Ghiringhelli F, Tesniere A, Obeid M, Ortiz C, Criollo A, et al. Toll-like receptor 4-dependent contribution of the immune system to anticancer chemotherapy and radiotherapy. Nat Med. (2007) 13:1050–9. doi: 10.1038/nm1622
52. Wang T, Gan X. Emerging roles of HMGB1-related lncRNA: from molecular biology to clinical application. Am J Physiol Cell Physiol. (2022) 323:C1149–60. doi: 10.1152/ajpcell.00296.2022
53. Zareifar P, Ahmed HM, Ghaderi P, Farahmand Y, Rahnama N, Esbati R, et al. miR-142-3p/5p role in cancer: From epigenetic regulation to immunomodulation. Cell Biochem Funct. (2024) 42:e3931. doi: 10.1002/cbf.3931
54. Song W, Wang K, Yang X, Dai W, Fan Z. Long non−coding RNA BANCR mediates esophageal squamous cell carcinoma progression by regulating the IGF1R/Raf/MEK/ERK pathway via miR−338−3p. Int J Mol Med. (2020) 46:1377–88. doi: 10.3892/ijmm.2020.4687
55. Zheng Z, Zhang G, Liang X, Li T. LncRNA OIP5-AS1 facilitates ox-LDL-induced endothelial cell injury through the miR-98-5p/HMGB1 axis. Mol Cell Biochem. (2021) 476:443–55. doi: 10.1007/s11010-020-03921-5
56. Follo C, Cheng Y, Richards WG, Bueno R, Broaddus VC. Autophagy facilitates the release of immunogenic signals following chemotherapy in 3D models of mesothelioma. Mol Carcinogenesis. (2019) 58:1754–69. doi: 10.1002/mc.23050
57. Fucikova J, Kepp O, Kasikova L, Petroni G, Yamazaki T, Liu P, et al. Detection of immunogenic cell death and its relevance for cancer therapy. Cell Death Dis. (2020) 11:1013. doi: 10.1038/s41419-020-03221-2
58. Bonora M, Patergnani S, Rimessi A, De Marchi E, Suski JM, Bononi A, et al. ATP synthesis and storage. Purinergic Signalling. (2012) 8:343–57. doi: 10.1007/s11302-012-9305-8
59. Liang H, Liu J, Su S, Zhao Q. Mitochondrial noncoding RNAs: new wine in an old bottle. RNA Biol. (2021) 18:2168–82. doi: 10.1080/15476286.2021.1935572
60. Durr AJ, Hathaway QA, Kunovac A, Taylor AD, Pinti MV, Rizwan S, et al. Manipulation of the miR-378a/mt-ATP6 regulatory axis rescues ATP synthase in the diabetic heart and offers a novel role for lncRNA Kcnq1ot1. Am J Physiol Cell Physiol. (2022) 322:C482–95. doi: 10.1152/ajpcell.00446.2021
61. Zhu Y, Jin L, Shi R, Li J, Wang Y, Zhang L, et al. The long noncoding RNA glycoLINC assembles a lower glycolytic metabolon to promote glycolysis. Mol Cell. (2022) 82:542–54. doi: 10.1016/j.molcel.2021.11.017
62. Motwani M, Pesiridis S, Fitzgerald KA. DNA sensing by the cGAS-STING pathway in health and disease. Nat Rev Genet. (2019) 20:657–74. doi: 10.1038/s41576-019-0151-1
63. Fucikova J, Moserova I, Urbanova L, Bezu L, Kepp O, Cremer I, et al. Prognostic and predictive value of DAMPs and DAMP-associated processes in cancer. Front In Immunol. (2015) 6:402. doi: 10.3389/fimmu.2015.00402
64. Allard B, Allard D, Buisseret L, Stagg J. The adenosine pathway in immuno-oncology. Nat Rev Clin Oncol. (2020) 17:611–29. doi: 10.1038/s41571-020-0382-2
65. Musella M, Galassi C, Manduca N, Sistigu A. The yin and yang of type I IFNs in cancer promotion and immune activation. Biology. (2021) 10. doi: 10.3390/biology10090856
66. Ghafouri-Fard S, Poornajaf Y, Dashti F, Hussen BM, Taheri M, Jamali E. Interaction between non-coding RNAs and interferons: with an especial focus on type I interferons. Front In Immunol. (2022) 13:877243. doi: 10.3389/fimmu.2022.877243
67. Yan C, Duanmu X, Zeng L, Liu B, Song Z. Mitochondrial DNA: distribution, mutations, and elimination. Cells. (2019) 8. doi: 10.3390/cells8040379
68. Hu M-M, Shu H-B. Mitochondrial DNA-triggered innate immune response: mechanisms and diseases. Cell Mol Immunol. (2023) 20:1403–12. doi: 10.1038/s41423-023-01086-x
69. Guo Y, Gu R, Gan D, Hu F, Li G, Xu G. Mitochondrial DNA drives noncanonical inflammation activation via cGAS-STING signaling pathway in retinal microvascular endothelial cells. Cell Communication Signaling: CCS. (2020) 18:172. doi: 10.1186/s12964-020-00637-3
70. Sharma N, Pasala MS, Prakash A. Mitochondrial DNA: epigenetics and environment. Environ Mol Mutagenesis. (2019) 60:668–82. doi: 10.1002/em.22319
71. Gao S, Tian X, Chang H, Sun Y, Wu Z, Cheng Z, et al. Two novel lncRNAs discovered in human mitochondrial DNA using PacBio full-length transcriptome data. Mitochondrion. (2018) 38:41–7. doi: 10.1016/j.mito.2017.08.002
72. Pitt JM, Marabelle A, Eggermont A, Soria JC, Kroemer G, Zitvogel L. Targeting the tumor microenvironment: removing obstruction to anticancer immune responses and immunotherapy. Ann Oncology: Off J Eur Soc For Med Oncol. (2016) 27:1482–92. doi: 10.1093/annonc/mdw168
73. Meurette O, Mehlen P. Notch signaling in the tumor microenvironment. Cancer Cell. (2018) 34:536–48. doi: 10.1016/j.ccell.2018.07.009
74. Diener C, Keller A, Meese E. Emerging concepts of miRNA therapeutics: from cells to clinic. Trends In Genetics: TIG. (2022) 38:613–26. doi: 10.1016/j.tig.2022.02.006
75. Fabian MR, Sonenberg N. The mechanics of miRNA-mediated gene silencing: a look under the hood of miRISC. Nat Struct Mol Biol. (2012) 19:586–93. doi: 10.1038/nsmb.2296
76. Lu TX, Rothenberg ME. MicroRNA. J Allergy Clin Immunol. (2018) 141:1202–7. doi: 10.1016/j.jaci.2017.08.034
77. Fabbri M. TLRs as miRNA receptors. Cancer Res. (2012) 72:6333–7. doi: 10.1158/0008-5472.CAN-12-3229
78. Wu S-Y, Fu T, Jiang Y-Z, Shao Z-M. Natural killer cells in cancer biology and therapy. Mol Cancer. (2020) 19:120. doi: 10.1186/s12943-020-01238-x
79. Minetto P, Guolo F, Pesce S, Greppi M, Obino V, Ferretti E, et al. Harnessing NK cells for cancer treatment. Front In Immunol. (2019) 10:2836. doi: 10.3389/fimmu.2019.02836
80. Kübler K, tho Pesch C, Gehrke N, Riemann S, Dassler J, Coch C, et al. Immunogenic cell death of human ovarian cancer cells induced by cytosolic poly(I:C) leads to myeloid cell maturation and activates NK cells. Eur J Immunol. (2011) 41:3028–39. doi: 10.1002/eji.201141555
81. Wang P, Gu Y, Zhang Q, Han Y, Hou J, Lin L, et al. Identification of resting and type I IFN-activated human NK cell miRNomes reveals microRNA-378 and microRNA-30e as negative regulators of NK cell cytotoxicity. J Immunol (Baltimore Md.: 1950). (2012) 189:211–21. doi: 10.4049/jimmunol.1200609
82. Trotta R, Chen L, Ciarlariello D, Josyula S, Mao C, Costinean S, et al. miR-155 regulates IFN-γ production in natural killer cells. Blood. (2012) 119:3478–85. doi: 10.1182/blood-2011-12-398099
83. Michaille J-J, Awad H, Fortman EC, Efanov AA, Tili E. miR-155 expression in antitumor immunity: The higher the better? Genes Chromosomes Cancer. (2019) 58:208–18. doi: 10.1002/gcc.22698
84. Corrêa LH, Corrêa R, Farinasso CM, de Sant’Ana Dourado LP, Magalhães KG. Adipocytes and macrophages interplay in the orchestration of tumor microenvironment: new implications in cancer progression. Front In Immunol. (2017) 8:1129. doi: 10.3389/fimmu.2017.01129
85. Ransohoff JD, Wei Y, Khavari PA. The functions and unique features of long intergenic non-coding RNA. Nat Rev Mol Cell Biol. (2018) 19:143–57. doi: 10.1038/nrm.2017.104
86. Hu G, Gong A-Y, Wang Y, Ma S, Chen X, Chen J, et al. LincRNA-cox2 promotes late inflammatory gene transcription in macrophages through modulating SWI/SNF-mediated chromatin remodeling. J Immunol (Baltimore Md.: 1950). (2016) 196:2799–808. doi: 10.4049/jimmunol.1502146
87. Zhang W, Yang M, Yu L, Hu Y, Deng Y, Liu Y, et al. Long non-coding RNA lnc-DC in dendritic cells regulates trophoblast invasion via p-STAT3-mediated TIMP/MMP expression. Am J Reprod Immunol (New York N.Y.: 1989). (2020) 83:e13239. doi: 10.1111/aji.13239
88. Fang P, Xiang L, Chen W, Li S, Huang S, Li J, et al. LncRNA GAS5 enhanced the killing effect of NK cell on liver cancer through regulating miR-544/RUNX3. Innate Immun. (2019) 25:99–109. doi: 10.1177/1753425919827632
89. Zhou W-Y, Cai Z-R, Liu J, Wang D-S, Ju H-Q, Xu R-H. Circular RNA: metabolism, functions and interactions with proteins. Mol Cancer. (2020) 19:172. doi: 10.1186/s12943-020-01286-3
90. Zhang Q, Wang W, Zhou Q, Chen C, Yuan W, Liu J, et al. Roles of circRNAs in the tumour microenvironment. Mol Cancer. (2020) 19:14. doi: 10.1186/s12943-019-1125-9
91. Zhang P-F, Gao C, Huang X-Y, Lu J-C, Guo X-J, Shi G-M, et al. Cancer cell-derived exosomal circUHRF1 induces natural killer cell exhaustion and may cause resistance to anti-PD1 therapy in hepatocellular carcinoma. Mol Cancer. (2020) 19:110. doi: 10.1186/s12943-020-01222-5
92. Jia L, Wang Y, Wang C-Y. circFAT1 promotes cancer stemness and immune evasion by promoting STAT3 activation. Advanced Sci (Weinheim Baden-Wurttemberg Germany). (2021) 8:2003376. doi: 10.1002/advs.202003376
93. Huang D, Zhu X, Ye S, Zhang J, Liao J, Zhang N, et al. Tumour circular RNAs elicit anti-tumour immunity by encoding cryptic peptides. Nature. (2024) 625:593–602. doi: 10.1038/s41586-023-06834-7
94. Bennett CF. Therapeutic antisense oligonucleotides are coming of age. Annu Rev Med. (2019) 70:307–21. doi: 10.1146/annurev-med-041217-010829
95. Egli M, Manoharan M. Chemistry, structure and function of approved oligonucleotide therapeutics. Nucleic Acids Res. (2023) 51:2529–73. doi: 10.1093/nar/gkad067
96. McClorey G, Wood MJ. An overview of the clinical application of antisense oligonucleotides for RNA-targeting therapies. Curr Opin In Pharmacol. (2015) 24:52–8. doi: 10.1016/j.coph.2015.07.005
97. Carthew RW, Sontheimer EJ. Origins and Mechanisms of miRNAs and siRNAs. Cell. (2009) 136:642–55. doi: 10.1016/j.cell.2009.01.035
98. Alshaer W, Zureigat H, Al Karaki A, Al-Kadash A, Gharaibeh L, Hatmal M, et al. siRNA: Mechanism of action, challenges, and therapeutic approaches. Eur J Pharmacol. (2021) 905:174178. doi: 10.1016/j.ejphar.2021.174178
99. Ahn I, Kang CS, Han J. Where should siRNAs go: applicable organs for siRNA drugs. Exp Mol Med. (2023) 55:1283–92. doi: 10.1038/s12276-023-00998-y
100. Lindow M, Kauppinen S. Discovering the first microRNA-targeted drug. J Cell Biol. (2012) 199:407–12. doi: 10.1083/jcb.201208082
101. Janssen HLA, Reesink HW, Lawitz EJ, Zeuzem S, Rodriguez-Torres M, Patel K, et al. Treatment of HCV infection by targeting microRNA. New Engl J Med. (2013) 368:1685–94. doi: 10.1056/NEJMoa1209026
102. Pegtel DM, Gould SJ. Exosomes. Annu Rev Biochem. (2019) 88:487–514. doi: 10.1146/annurev-biochem-013118-111902
103. Tenchov R, Sasso JM, Wang X, Liaw W-S, Chen C-A, Zhou QA. Exosomes─Nature’s lipid nanoparticles, a rising star in drug delivery and diagnostics. ACS Nano. (2022) 16:17802–46. doi: 10.1021/acsnano.2c08774
104. Liang Y, Xu X, Li X, Xiong J, Li B, Duan L, et al. Chondrocyte-targeted microRNA delivery by engineered exosomes toward a cell-free osteoarthritis therapy. ACS Appl Materials Interfaces. (2020) 12:36938–47. doi: 10.1021/acsami.0c10458
105. Ghafouri-Fard S, Bahroudi Z, Shoorei H, Abak A, Ahin M, Taheri M. microRNA-140: A miRNA with diverse roles in human diseases. Biomedicine Pharmacotherapy = Biomedecine Pharmacotherapie. (2021) 135:111256. doi: 10.1016/j.biopha.2021.111256
106. Ji X, Wang E, Tian F. MicroRNA-140 suppresses osteosarcoma tumor growth by enhancing anti-tumor immune response and blocking mTOR signaling. Biochem Biophys Res Commun. (2018) 495:1342–8. doi: 10.1016/j.bbrc.2017.11.120
107. Wu X, Ban C, Deng W, Bao X, Tang N, Wu Y, et al. Unveiling the PDK4-centered rituximab-resistant mechanism in DLBCL: the potential of the “Smart” exosome nanoparticle therapy. Mol Cancer. (2024) 23:144. doi: 10.1186/s12943-024-02057-0
108. Zhao Y, Huang L. Lipid nanoparticles for gene delivery. Adv In Genet. (2014) 88:13–36. doi: 10.1016/B978-0-12-800148-6.00002-X
109. Mendonça MCP, Kont A, Kowalski PS, O’Driscoll CM. Design of lipid-based nanoparticles for delivery of therapeutic nucleic acids. Drug Discovery Today. (2023) 28:103505. doi: 10.1016/j.drudis.2023.103505
110. Scheideler M, Vidakovic I, Prassl R. Lipid nanocarriers for microRNA delivery. Chem Phys Lipids. (2020) 226:104837. doi: 10.1016/j.chemphyslip.2019.104837
111. Judge AD, Robbins M, Tavakoli I, Levi J, Hu L, Fronda A, et al. Confirming the RNAi-mediated mechanism of action of siRNA-based cancer therapeutics in mice. J Clin Invest. (2009) 119:661–73. doi: 10.1172/JCI37515
112. Bordat A, Boissenot T, Nicolas J, Tsapis N. Thermoresponsive polymer nanocarriers for biomedical applications. Advanced Drug Delivery Rev. (2019) 138:167–92. doi: 10.1016/j.addr.2018.10.005
113. Davis ME. The first targeted delivery of siRNA in humans via a self-assembling, cyclodextrin polymer-based nanoparticle: from concept to clinic. Mol Pharmaceutics. (2009) 6:659–68. doi: 10.1021/mp900015y
114. Danhier F, Ansorena E, Silva JM, Coco R, Le Breton A, Préat V. PLGA-based nanoparticles: an overview of biomedical applications. J Controlled Release: Off J Controlled Release Soc. (2012) 161:505–22. doi: 10.1016/j.jconrel.2012.01.043
115. Folini M, Bandiera R, Millo E, Gandellini P, Sozzi G, Gasparini P, et al. Photochemically enhanced delivery of a cell-penetrating peptide nucleic acid conjugate targeting human telomerase reverse transcriptase: effects on telomere status and proliferative potential of human prostate cancer cells. Cell Proliferation. (2007) 40:905–20. doi: 10.1111/j.1365-2184.2007.00470.x
116. Grünweller A, Hartmann RK. Chemical modification of nucleic acids as a key technology for the development of RNA-based therapeutics. Die Pharmazie. (2016) 71:8–16. doi: 10.1691/ph.2016.5738
117. Adachi H, Hengesbach M, Yu Y-T, Morais P. From antisense RNA to RNA modification: therapeutic potential of RNA-based technologies. Biomedicines. (2021) 9. doi: 10.3390/biomedicines9050550
118. Wei G, Wang Y, Yang G, Wang Y, Ju R. Recent progress in nanomedicine for enhanced cancer chemotherapy. Theranostics. (2021) 11:6370–92. doi: 10.7150/thno.57828
119. Peng F, Liao M, Qin R, Zhu S, Peng C, Fu L, et al. Regulated cell death (RCD) in cancer: key pathways and targeted therapies. Signal Transduction Targeted Ther. (2022) 7:286. doi: 10.1038/s41392-022-01110-y
120. Dai E, Zhu Z, Wahed S, Qu Z, Storkus WJ, Guo ZS. Epigenetic modulation of antitumor immunity for improved cancer immunotherapy. Mol Cancer. (2021) 20:171. doi: 10.1186/s12943-021-01464-x
121. Arimoto K-I, Miyauchi S, Liu M, Zhang D-E. Emerging role of immunogenic cell death in cancer immunotherapy. Front In Immunol. (2024) 15:1390263. doi: 10.3389/fimmu.2024.1390263
122. Pucci P, Rescigno P, Sumanasuriya S, de Bono J, Crea F. Hypoxia and noncoding RNAs in taxane resistance. Trends In Pharmacol Sci. (2018) 39:695–709. doi: 10.1016/j.tips.2018.05.002
Keywords: ncRNAs, DAMPs, immunogenic cell death, antitumor immune responses, cancer immunotherapy
Citation: Sun G and He L (2025) A new paradigm for cancer immunotherapy: targeting immunogenic cell death-related noncoding RNA. Front. Immunol. 15:1498781. doi: 10.3389/fimmu.2024.1498781
Received: 19 September 2024; Accepted: 30 December 2024;
Published: 23 January 2025.
Edited by:
Neng Wang, Guangzhou University of Chinese Medicine, ChinaCopyright © 2025 Sun and He. This is an open-access article distributed under the terms of the Creative Commons Attribution License (CC BY). The use, distribution or reproduction in other forums is permitted, provided the original author(s) and the copyright owner(s) are credited and that the original publication in this journal is cited, in accordance with accepted academic practice. No use, distribution or reproduction is permitted which does not comply with these terms.
*Correspondence: Ling He, aGVsaW5naGRAMTYzLmNvbQ==
Disclaimer: All claims expressed in this article are solely those of the authors and do not necessarily represent those of their affiliated organizations, or those of the publisher, the editors and the reviewers. Any product that may be evaluated in this article or claim that may be made by its manufacturer is not guaranteed or endorsed by the publisher.
Research integrity at Frontiers
Learn more about the work of our research integrity team to safeguard the quality of each article we publish.