- 1Gansu University of Traditional Chinese Medicine, Lanzhou, China
- 2Wenzhou Medical University, Wenzhou, China
- 3Affiliated Hospital of Gansu University of Traditional Chinese Medicine, Lanzhou, China
Postmenopausal osteoporosis (PMOP) is a metabolic bone disease driven by estrogen deficiency, primarily manifesting as reduced bone mass and heightened fracture risk. Its development is intricately linked to the balance between Th17 and Treg cells. Recent studies have highlighted the significant role of gut homeostasis in PMOP. The gut microbiota profoundly impacts bone health by modulating the host’s immune system, metabolic pathways, and endocrine functions. In particular, the regulation of Th17 and Treg cell balance by gut homeostasis plays a pivotal role in the onset and progression of PMOP. Th17 cells secrete pro-inflammatory cytokines that stimulate osteoclast activity, accelerating bone resorption, while Treg cells counteract this process through anti-inflammatory mechanisms, preserving bone mass. The gut microbiota and its metabolites can influence Th17/Treg equilibrium, thereby modulating bone metabolism. Furthermore, the integrity of the gut barrier is critical for systemic immune stability, and its disruption can lead to immune dysregulation and metabolic imbalances. Thus, targeting gut homeostasis to restore Th17/Treg balance offers a novel therapeutic avenue for the prevention and treatment of PMOP.
1 Introduction
PMOP is a metabolic bone disease primarily characterized by bone loss driven by estrogen deficiency. It progresses silently in postmenopausal women and is typically diagnosed only after the occurrence of fragility fractures (1). In China, approximately 20.6% of women over 40 years old are affected by osteoporosis (2). Gut homeostasis, a crucial regulator of bone metabolism, is strongly associated with the onset and progression of PMOP and has emerged as a key area of research (3). Gut homeostasis involves the stability of the gut microbiota, its metabolic functions, and the integrity of the gut’s physical and immune barriers (4). The gut microbiota, a vast and complex microbial community residing in the gastrointestinal tract, plays a pivotal role in bone metabolism and health by interacting with the host’s immune, metabolic, and endocrine systems (5).
The gut barrier consists of multiple layers (6): the outer layer, comprising mucus, symbiotic microbes, antimicrobial proteins, and secretory immunoglobulin A; the middle layer, formed by intestinal epithelial cells; and the innermost layer, which includes innate and adaptive immune cells (7). The gut mucosal immune system executes diverse immune defense functions through immune cells located at induction and effector sites (8). Within the gut, immune cells such as dendritic cells, macrophages, and T cells recognize and respond to microbiota and their metabolites, thereby regulating both local and systemic immune responses (9). Disruption of the gut barrier can result in epithelial cell apoptosis, promoting a pro-inflammatory environment that drives the differentiation of helper T cells 17 (Th17) and regulatory T cells (Treg) (10). Alterations in the gut microbiota are closely associated with changes in bone mass, particularly through their role in bone immunology and the gut-bone axis (11, 12). Previous studies have investigated the role of the gut microbiota in PMOP (13). Thus, elucidating the mechanisms by which gut homeostasis regulates the Th17/Treg balance could provide valuable insights into the pathogenesis of PMOP and inform the development of novel therapeutic strategies.
2 Pathological pathways of PMOP
PMOP is primarily caused by estrogen deficiency, which leads to decreased bone mass, deterioration of microarchitecture, and impaired bone metabolism. A hallmark pathological feature of PMOP is the imbalance between bone resorption and formation, with accelerated resorption outpacing bone formation (14). Recent advances in osteoimmunology have revealed that estrogen deficiency induces a chronic low-grade inflammatory state, significantly contributing to disease progression. This inflammatory response is mediated through various immune pathways, driving the pathological mechanisms underlying PMOP. System is shown in Figure 1.
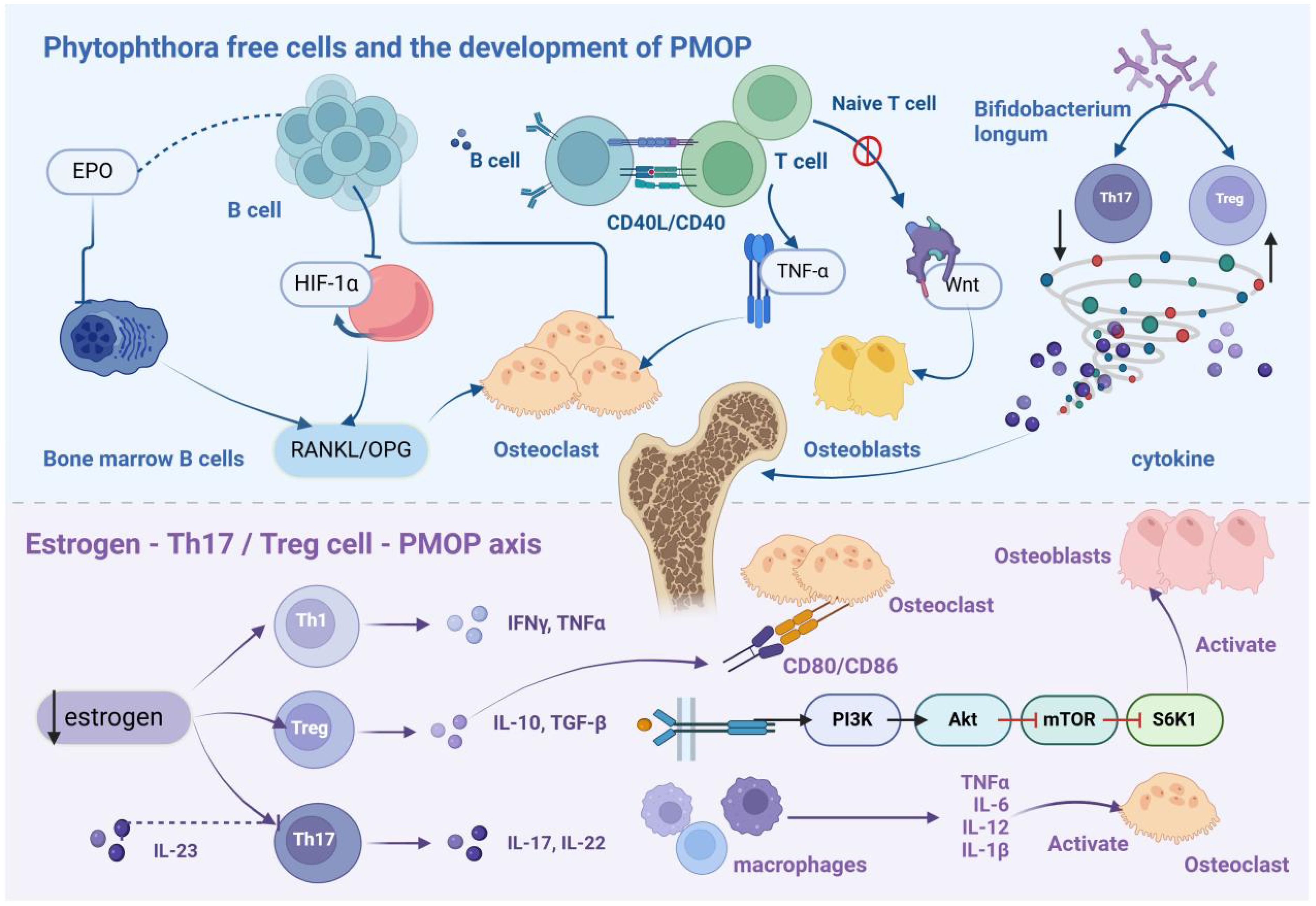
Figure 1. Immunopathological Mechanism of PMOP. B cells differentiate into osteoclasts, leading to bone loss. In the estrogen-deficient state, hypoxia-inducible factor-1α signaling is activated in B cells, enhancing RANKL expression and promoting osteoclastogenesis, thereby inducing PMOP. T cells normally exhibit bone-protective functions in basal bone metabolism. However, following ovariectomy, CD4+ and CD8+ T cells become activated and secrete RANKL and other osteoclastic factors. Treg cells secrete IL-10, TGF-β, and other anti-resorptive cytokines, whereas Th17 cells produce IL-17, which stimulates osteoclastogenesis. Additionally, IL-17 triggers mesenchymal stem cells to release osteoclast differentiation factors. Thus, estrogen modulates bone metabolism by regulating the balance between Th17 and Treg cells.
2.1 Immune cells and the development of PMOP
B cells contribute to humoral immunity through antibody production and regulate bone metabolism. Studies have highlighted their osteoclastogenic potential, particularly under erythropoietin stimulation, where bone marrow B cells express receptor activator of nuclear factor kappa-Β ligand (RANKL), modulating bone metabolism via the RANKL/osteoprotegerin axis. High erythropoietin concentrations induce B cell differentiation into osteoclasts, resulting in bone loss (15). Additionally, estrogen downregulates hypoxia-inducible factor-1α expression by upregulating heat shock protein 70 production. In ovariectomized mice, elevated hypoxia-inducible factor-1α levels activate downstream pathways, upregulating RANKL gene expression in B cells and promoting osteoclastogenesis, thus accelerating PMOP progression (16).
T cells, originating from bone marrow lymphoid stem cells, mature in the thymus before circulating through systemic immune organs and tissues. Under normal conditions, T cells maintain bone homeostasis via multiple mechanisms. Estrogen presence facilitates T cell-derived CD40 ligand interactions with B cell surface CD40, enhancing osteoprotegerin mRNA expression and protecting bone (17). However, in PMOP, this protective mechanism is disrupted. Following ovariectomy, activated CD4+ and CD8+ T cells increase Dickkopf-1 production, inhibiting osteoblast Wnt signaling via paracrine effects (18). Simultaneously, these T cells secrete tumor necrosis factor-α (TNF-α) and RANKL, accelerating bone resorption (19). PMOP patients also exhibit elevated LIGHT expression in circulating monocytes and T cells, which enhances osteoclastogenesis by modulating TNF and RANKL expression (20).
Treg cells and Th17 cells, key subsets of T cells, exhibit differentiation plasticity but serve opposing functions. Treg cells suppress osteoclast differentiation by inhibiting IL-17 expression, whereas Th17 cells promote osteoclastogenesis via RANKL signaling (21). Maintaining the balance between these populations is critical for preserving normal bone mass (22). Emerging research demonstrates that gut microbiota significantly influences Treg and Th17 cell functions. For example, Bifidobacterium longum modulates Breg cell expression, establishing a Breg-Treg-Th17 axis that enhances Treg cell function and suppresses Th17 activity. This modulation reduces pro-inflammatory cytokines, such asInterleukin-6 (IL-6), Interleukin-17 (IL-17), and TNF-α, while increasing anti-inflammatory factors, including Interleukin-10 (IL-10) and IFN-γ, thereby protecting bone and alleviating PMOP symptoms (23).
2.2 The estrogen-Th17/Treg cell-PMOP axis
Chronic inflammation-induced bone metabolic dysregulation is a fundamental pathological feature of PMOP, with the Th17/Treg cell balance and associated cytokine networks orchestrating bone remodeling. Treg cells inhibit bone resorption through two mechanisms: secretion of anti-resorptive factors such as IL-10 and transforming growth factor-β (TGF-β), and interaction of their surface cytotoxic T-lymphocyte-associated protein 4 with CD80/CD86 on osteoclast precursors, which activates indoleamine-2,3-dioxygenase. This activation triggers tryptophan catabolism, inducing precursor cell apoptosis (24). Conversely, Th17 cells enhance osteoclastogenesis by expressing RANKL and secreting IL-17, which stimulates macrophages to produce pro-inflammatory mediators, including TNF-α and IL-6, further upregulating RANKL expression in osteoclast-supporting cells (22).
Estrogen deficiency disrupts the Th17/Treg balance. In estrogen-depleted conditions, heightened Th17 cell activity increases IL-17 production, promoting bone marrow mesenchymal stem cell proliferation and osteogenic differentiation while inducing macrophage colony-stimulating factor and RANKL secretion, thereby accelerating osteoclastogenesis (22, 25). Clinical studies report significantly elevated IL-17 levels in postmenopausal osteoporotic vertebral compression fracture patients (26). Additionally, Interleukin-23 exacerbates bone loss through two mechanisms: enhancing Th17 cell activity and inducing T cell RANKL expression. Targeting these pathways offers therapeutic potential, as anti-IL-17 antibodies promote bone regeneration via forkhead box o1 and activating transcription factor 4 activation (27), while anti-Interleukin-23 antibodies prevent estrogen deficiency-induced bone loss (28). Recent studies show that B10 cell adoptive transfer reduces Th17 cell populations and inhibits alveolar bone osteoporosis in ovariectomized mice (29). At the molecular level, estrogen stimulates Treg cells to produce IL-10 and TGF-β1, suppressing osteoclast differentiation and bone resorption. TGF-β, a key bone repair regulator, balances osteoblast differentiation and osteoclast formation (30). Molecular studies reveal that TGF-β1 enhances osteoblast survival, differentiation, and migration by activating the phosphatidylinositol 3-kinase/protein kinase B/mechanistic target of rapamycin/ribosomal protein S6 kinase beta-1 signaling pathway (31). These findings suggest that targeting Treg cell activity may provide effective therapeutic strategies for bone protection in inflammatory conditions.
3 Intestinal homeostasis affects the development of PMOP
PMOP is the most common form of primary osteoporosis and is characterized by its complex pathogenesis. Recent research has underscored the critical role of the gut microbiota in the development and progression of this condition. PMOP is associated with significant alterations in gut microbiota composition, including an inverse relationship between the abundance of proteobacteria and bone mass. Additionally, the relative abundances of bacteroides, parabacteroides, and lactobacillus are significantly increased. These microbial changes are thought to aggravate bone loss by influencing bone metabolism and promoting inflammatory responses, thus accelerating disease progression.The gut microbiota is a critical regulator of immune system homeostasis, modulating immune cell activity and cytokine networks (32–35). Accumulating evidence indicates that gut microbiota dysbiosis significantly contributes to the pathogenesis of PMOP, primarily by disrupting the Th17/Treg cell balance. This immunological equilibrium is precisely governed by the gut microbiota and its metabolic products (36, 37).
The gut microbiota influences PMOP through two distinct immunological mechanisms. In adaptive immunity, specific bacterial populations exhibit unique immunomodulatory functions. For instance, segmented filamentous bacteria (SFB) promote intestinal Th17 cell development through defined pathways, with SFB-mediated Th17 responses enhancing barrier integrity by inhibiting bacterial translocation in constitutively myosin light chain kinase-activated mouse models (38). Notably, intestinal Th17 cells display significant functional heterogeneity: SFB-induced Th17 cells maintain gut homeostasis, while citrobacter-induced Th17 cells exhibit pro-inflammatory characteristics (39). Additionally, clostridium species produce immunomodulatory metabolites that stimulate Treg cell development, thereby establishing immune tolerance (40–42).
In innate immunity, gut-associated lymphoid tissue-resident cells serve as the primary defense against exogenous antigens. Disruption of gut microecological homeostasis abnormally activates innate immune cells, increasing pro-inflammatory mediators such as IL-12, Interleukin-23, and type I interferons and reducing anti-inflammatory factors such as TGF-β and IL-10 (36). This dysregulation enhances antigen presentation to CD4+ T cells by dendritic cells and macrophages, promoting differentiation into inflammatory T cell subsets (43). Under specific microenvironmental conditions, CD4+ T cells can alternatively differentiate into immunosuppressive Treg cells, forming complex regulatory networks (44, 45). System is shown in Figure 2.
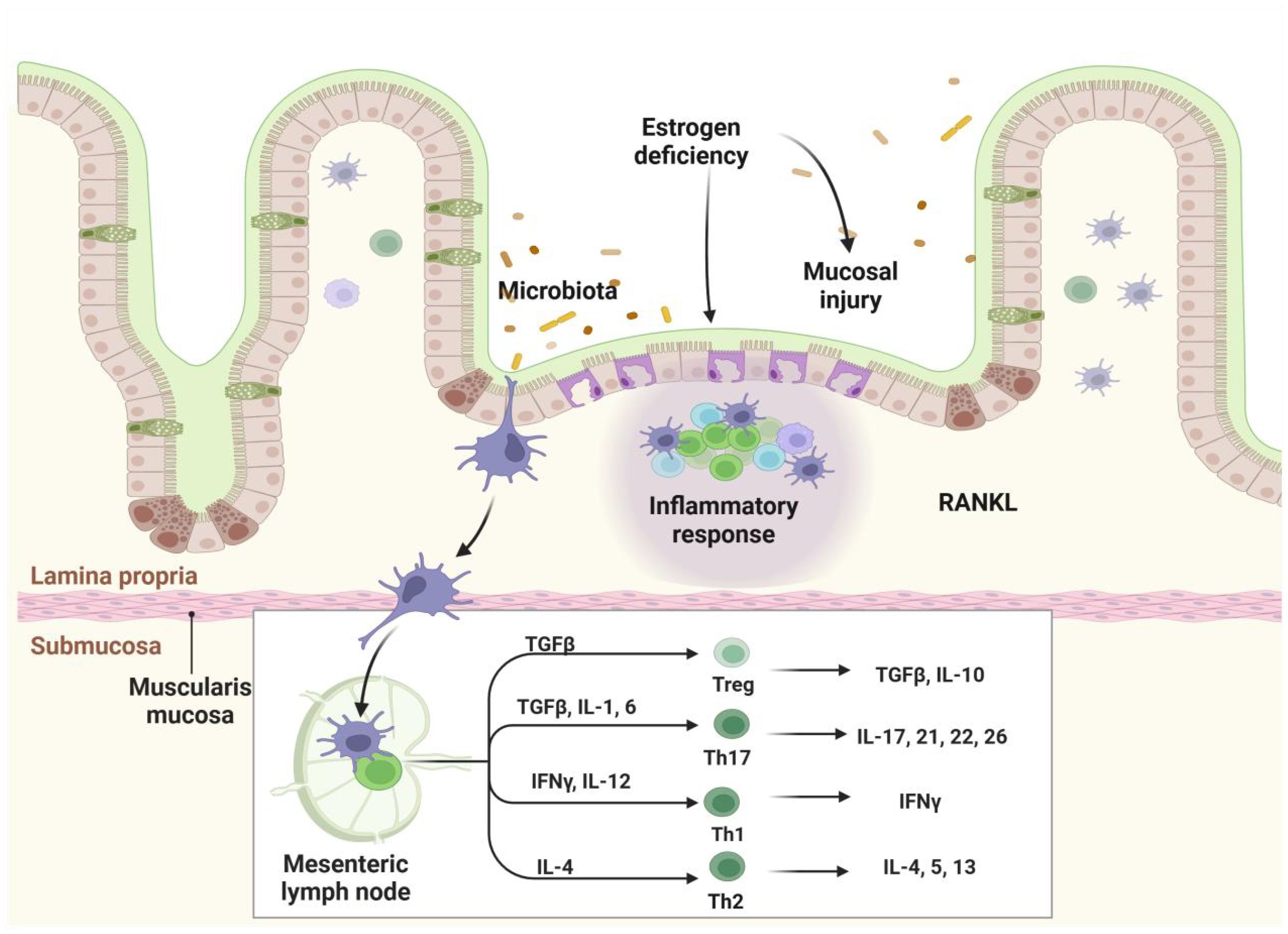
Figure 2. Gut Homeostasis and Immune Regulation. Gut homeostasis plays a pivotal role in maintaining immune equilibrium. Disruption of the gut microbiota and damage to the intestinal barrier can lead to immune dysregulation, characterized by the abnormal activation of innate immune cells, including dendritic cells and macrophages. This activation induces the production of elevated levels of pro-inflammatory cytokines, such as IL-12, IL-23, and type I interferons, while anti-inflammatory cytokines, including TGF-β and IL-10, are suppressed. Activated antigen-presenting cells, like dendritic cells and macrophages, present microbial antigens to CD4+ T helper cells, driving their differentiation into various pro-inflammatory T cell subsets, including Th1, Th2, and Th17.
The gut microbiota also regulates bone metabolism through endocrine signaling pathways. Intestinal epithelial cells activate mitogen-activated protein kinase signaling via estrogen receptors (46), triggering nitric oxide/cyclic guanosine monophosphate cascades that enhance osteoblast differentiation and osteoprotegerin secretion while inhibiting osteoclastogenesis (47). Furthermore, clostridium species maintain estrogen homeostasis through β-glucuronidase-mediated enterohepatic circulation (48), directly linking estrogen levels to microbiota composition. During PMOP progression, the gut microbiota modulates disease development by influencing estrogen deficiency-induced inflammation. Decreased estrogen levels impair intestinal barrier integrity, enhancing Th17 cell differentiation. These activated Th17 cells produce pro-inflammatory mediators such as TNF-α, RANKL, and IL-17, creating a positive feedback loop that promotes osteoclastogenesis and bone loss (49). These mechanisms highlight the therapeutic potential of targeting the gut microbiota-immune-endocrine axis in PMOP treatment.
4 Mechanisms by which gut homeostasis regulates the Th17/Treg cell balance and its impact on PMOP
Gut homeostasis is a multi-layered defense system comprising the gut microbiota, mucus layer, single-layer epithelium, and immune cells within the lamina propria (50). The mucus layer and epithelial cells form a physical barrier that prevents bacterial adhesion (51). The lamina propria and submucosa play critical roles in immune responses, protecting the host from both commensal and pathogenic microbial invasion (52). Epithelial cells regulate trans-epithelial permeability through tight junctions, thereby maintaining epithelial barrier integrity (53, 54). Disruption of gut homeostasis in PMOP is primarily mediated by the immune-modulating effects of microbial metabolites (55, 56). When gut homeostasis is compromised, pathogenic microbes proliferate, and excessive production of metabolites such as lipopolysaccharides (LPS) damages the intestinal barrier. This increases mucosal permeability and exacerbates both intestinal and systemic inflammatory responses. Under these conditions, Th17 cell activity is elevated while Treg cell function is suppressed, creating an imbalance that accelerates osteoclast formation and bone resorption. This process ultimately contributes to bone loss and PMOP progression. Figure 3 illustrates the impact of gut homeostasis on the Th17/Treg balance in PMOP.
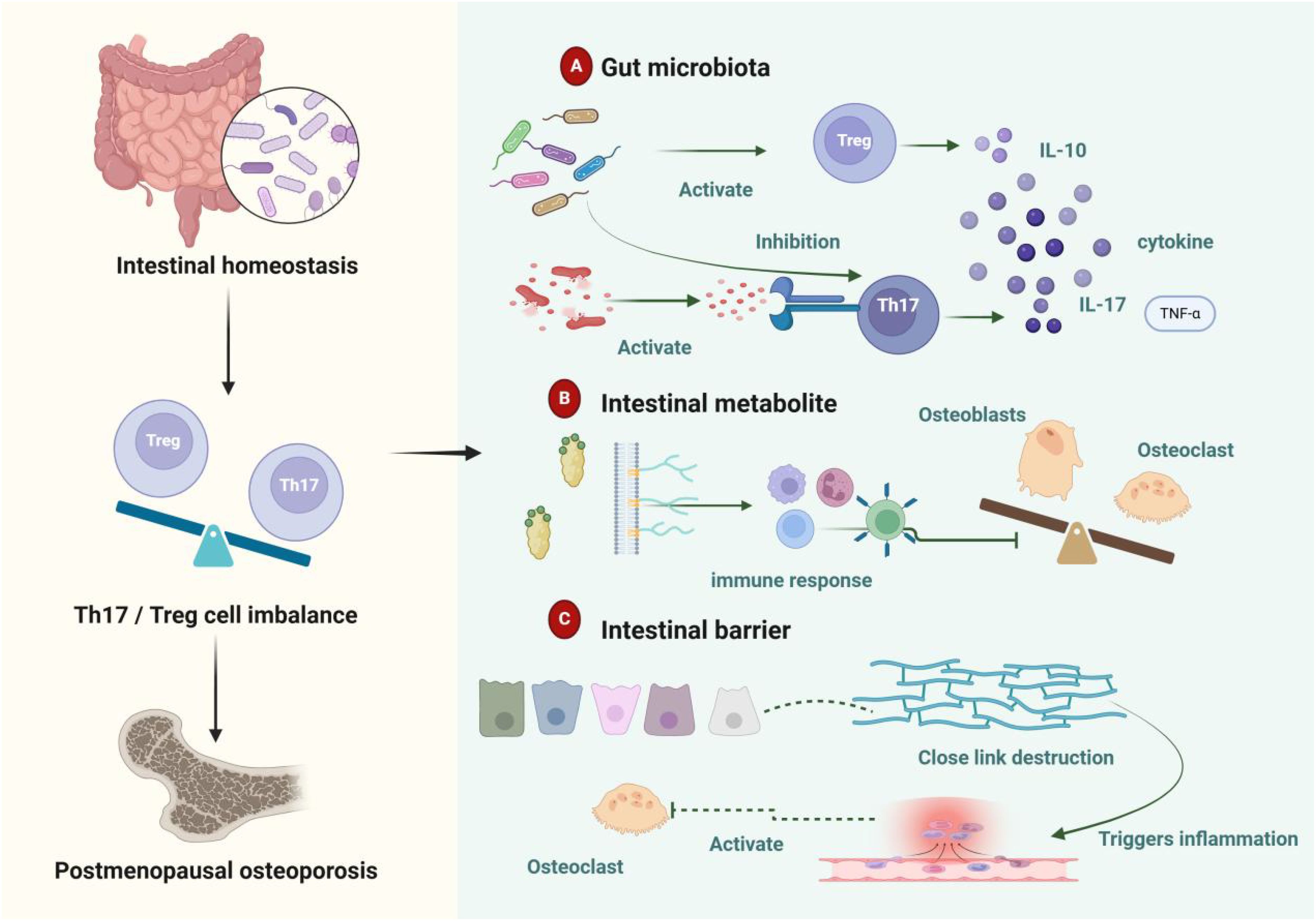
Figure 3. Gut Homeostasis Regulates Th17/Treg Balance and Influences PMOP. (A) The gut microbiota modulates the balance between Th17 and Treg cells, as well as cytokine levels, affecting the development and progression of PMOP. (B) Microbial-derived metabolites from the gut regulate multiple immune cells, causing an imbalance between osteoblasts and osteoclasts, which contributes to PMOP. (C) Intestinal barrier damage increases permeability and disrupts tight junctions, triggering inflammation and promoting osteoclast differentiation, thereby accelerating the onset and progression of PMOP.
4.1 The gut microbiota-Th17/Treg-PMOP axis
In patients with PMOP, an increased abundance of clostridium species has been observed, with its relative abundance negatively correlated with bone mineral density (BMD) (57). Research suggests that clostridium activates the protein kinase B beta signaling pathway, promoting M1 macrophage production, thereby triggering inflammation and accelerating osteoporosis progression (58, 59). In a randomized trial involving postmenopausal Japanese women, participants treated with the probiotic Bacillus subtilis showed a significant increase in total hip BMD compared to the placebo group (60). After 12 and 24 weeks of Bacillus subtilis treatment, the abundance of clostridium species significantly decreased, potentially improving BMD by reducing pro-resorptive cytokines (60). Additionally, lactobacillus reuteri (american type culture collection PTA-6475) has been shown to reduce bone loss in elderly women with low BMD (61).
Lactobacillus acidophilus mitigates bone loss and enhances bone heterogeneity in osteoporotic mice by modulating the Treg-Th17 cell balance (62). Similarly, lactobacillus rhamnosus reduces bone loss and preserves bone health in ovariectomized mice (63). Probiotics such as Bifidobacterium and clostridium species promote Treg cell differentiation, thereby inhibiting excessive bone resorption. Intervention with Bifidobacterium longum significantly increases Breg cell proportions and the levels of IL-10 and interferon-gamma while decreasing the production of TNF-α, IL-6, and IL-17. Clostridium-stimulated Bregs also significantly enhance Treg cell proportions and IL-10 expression while reducing Th17 cells and IL-17 levels, indicating a potent regulatory role in Th17/Treg differentiation. Pathogenic bacteria such as clostridium and Ruminococcus are associated with Th17 cell activation, exacerbating osteoclastogenesis and bone resorption (64). Toxins produced by Bacteroides fragilis activate intestinal Th17 cell recruitment via the JAK-STAT3 pathway (65–67). Thus, alterations in gut microbiota composition disrupt the Th17/Treg balance, affecting bone metabolism and contributing to osteoporosis progression (68).
4.2 The gut metabolites-Th17/Treg-PMOP axis
Gut microbiota-derived metabolites play a pivotal role in regulating immune cell development and function, including both adaptive and innate immune responses. IL-10, produced by effector T cells, acts as a key self-regulatory mechanism for maintaining immune homeostasis (68). Short-chain fatty acids (SCFAs) enhance IL-10 production in differentiated Th1 cells via a G-protein-coupled receptor 43-dependent pathway and induce IL-10 during Th1 and Th17 cell differentiation by inhibiting histone deacetylase activity (69). SCFAs and other metabolites directly influence osteoblast and osteoclast differentiation and activation, with butyrate playing a crucial role in osteoclast metabolism regulation (33). Butyrate-treated dendritic cells induce the expression of immunosuppressive enzymes, such as indoleamine 2,3-dioxygenase 1 and aldehyde dehydrogenase 1 family member A2, in a solute carrier family 5 member 8-dependent manner. This promotes Treg differentiation while inhibiting Th1 differentiation (70). Microbial tryptophan metabolites, such as indole and its derivatives, bind to aryl hydrocarbon receptors, influencing B cell development (71), differentiation (72), and cytokine regulation (73) via aryl hydrocarbon receptor signaling. LPS, components of Gram-negative bacteria, trigger cytokine cascades driving T cell-mediated inflammation (74). LPS also inhibits osteoblast maturation and activates osteoclasts, enhancing bone resorption and exacerbating PMOP progression. Additionally, bile acids and their metabolites regulate host immune responses by modulating the Th17/Treg cell balance (75). These metabolites provide mechanistic insights into the interplay between gut homeostasis and immune regulation.
4.3 The gut barrier-Th17/Treg-PMOP axis
Disruption of gut barrier function can lead to leakage of intestinal contents, creating a pro-inflammatory environment (76). In mouse models, elevated zonulin levels degrade essential tight junction proteins and increase the expression of claudin-2 and claudin-15, compromising tight junction integrity and severely impairing gut barrier function Increased intestinal permeability triggers T cell-mediated mucosal inflammation and facilitates the migration of autoreactive T cells, such as Th1 and Th17 cells, from the gut to other sites like joints, potentially contributing to PMOP onset (77). Estrogen plays a key role in maintaining intestinal epithelial barrier function, and its deficiency increases gut permeability (78). This enhanced permeability triggers inflammatory responses that promote osteoclast formation, ultimately leading to bone loss (79, 80). Sufficient estrogen levels activate Tregs, inhibit osteoclastogenesis, and prevent osteoblast destruction, contributing to the maintenance of bone mass (79, 81, 82). Tight junctions between intestinal epithelial cells are essential for preserving gut barrier integrity. Lactobacillus rhamnosus supports epithelial integrity through carbohydrate transport and metabolism (83). Specifically, lactobacillus rhamnosus GG regulates both the gut microbiota and gut barrier, modulating the Th17/Treg balance and alleviating osteoporosis induced by estrogen deficiency.
5 PMOP treatment strategies based on gut homeostasis regulation
Current clinical treatments for PMOP, including calcium supplements, calcitonin, bisphosphonates, and estrogen, often have limited efficacy and are associated with significant side effects. Consequently, there is a pressing need for safe, effective, and low-risk therapeutic options for PMOP patients. In recent years, increasing research into the relationship between gut microbiota and bone metabolism has highlighted the potential of strategies targeting gut homeostasis for preventing and treating PMOP. The interaction between gut microbiota and bone metabolism offers a novel approach to osteoporosis management. By modulating the host’s immune, metabolic, and endocrine systems, the gut microbiota can directly or indirectly regulate bone homeostasis, presenting new possibilities for the comprehensive treatment of PMOP.
5.1 Gut microbiota modulators
Probiotics have demonstrated potential to enhance the growth and metabolic activity of beneficial bacteria, offering significant benefits for bone health (16). They produce metabolites and genetic products that directly interact with epithelial and immune cells, improving gut function, reducing inflammation, lowering intestinal pH to promote calcium absorption, and preventing the colonization of harmful bacteria. Collectively, these effects support osteoblast activity and contribute to the maintenance of bone health (84, 85). Probiotic supplementation has been shown to increase bone density and promote fracture healing. Animal studies have further demonstrated that probiotics enhance bone mass by inhibiting CD4+ T cell proliferation in the bone marrow and reducing the expression of pro-inflammatory cytokines such as TNF-α (11, 86).
Additionally, the gut microbiota regulates immune balance and microbial stability through tryptophan and its metabolites, including indole and serotonin (87). Exogenous supplementation of tryptophan metabolites, such as indole acetic acid and indole-3-propionic acid, effectively restores intestinal barrier integrity in ovariectomy -induced PMOP mouse models and alleviates osteoporosis. This process critically depends on the activation of the aryl hydrocarbon receptor. Mechanistically, tryptophan metabolites, particularly indole acetic acid, activate intestinal AhR, which, in turn, stimulates the Wnt/β-catenin signaling pathway to restore intestinal barrier function. indole acetic acid and indole-3-propionic acid supplementation also enhances M2 macrophage secretion of IL-10, which diffuses from the intestinal lamina propria to the bone marrow, promoting osteogenesis while suppressing osteoclast formation. Notably, the therapeutic effects of tryptophan metabolites on intestinal homeostasis and osteoporosis symptoms are significantly reduced in ovariectomy mice lacking intestinal AhR. These findings highlight gut microbial tryptophan metabolites as promising therapeutic candidates for osteoporosis by modulating the AhR-mediated gut-bone axis.
By modulating the immune system, the gut microbiota influences bone metabolism, potentially affecting bone density and turnover. This highlights the gut microbiota as a novel therapeutic target for osteoporosis treatment and fracture prevention (5). For example, Lactobacillus casei modulates gut microbiota composition, reduces pro-inflammatory cytokines such as IL-17, interleukin-1β, IL-6, and TNF-α, and adjusts the Th1/Th17 ratio, thereby inhibiting the onset and progression of PMOP (88). Another byproduct of colonic microbial fermentation, hydrogen gas, is produced in significant amounts by certain strains of clostridium and may have potential effects on gut and bone health (89–91).
5.2 Fecal microbiota transplantation
Fecal microbiota transplantation (FMT) has been shown to reshape gut microbiota and mitigate bone loss in ovariectomy-induced osteoporotic mice. The mechanisms underlying these effects include correcting gut microbiota dysbiosis, elevating SCFAs levels, improving gut permeability, and inhibiting the release of pro-osteoclastogenic factors, collectively suppressing excessive osteoclast formation (92). The circulatory system serves as a bridge connecting osteoclastogenic factors, transgenic cells, SCFAs, and the skeletal system. FMT effectively prevents ovariectomy-induced bone loss by limiting osteoclast overactivity (93). Compared to ovariectomized controls, FMT-treated mice exhibited increased expression of tight junction proteins such as occludin and reduced secretion of pro-osteoclastogenic factors, including TNF-α and interleukin-1β (94). Furthermore, FMT optimized gut microbiota composition and abundance, while increasing fecal SCFAs levels, particularly acetate and propionate (95). Consequently, FMT represents a promising alternative therapy and a potential strategy for preventing and treating PMOP in the future.
5.3 Maintaining gut barrier integrity
The integrity of the gut barrier is essential for the prevention and treatment of PMOP. An intact mucosal barrier prevents harmful substances, such as bacterial LPS, from entering systemic circulation (94). This, in turn, suppresses activation of the toll-like receptor 4 receptor signaling pathway in macrophages, thereby reducing the release of pro-inflammatory cytokines like TNF-α and inhibiting osteoclast differentiation and activation, which would otherwise accelerate bone resorption (96, 97). Additionally, a healthy gut microbiota produces beneficial metabolites, including SCFAs, that regulate bone metabolism. SCFAs inhibit osteoclast activity by downregulating key molecules such as TNF receptor-associated factor 6 and nuclear factor of activated T-cells 1, while simultaneously promoting bone formation by upregulating osteoblast differentiation-related genes (61, 98). Butyrate, a major SCFAs, promotes the differentiation of bone-protective Tregs, which, in turn, induce CD8+ T cells to release Wnt10b, activating the Wnt signaling pathway in osteoblasts (99, 100). Lucas et al. demonstrated that SCFAs supplementation or a high-fiber diet can prevent menopause and inflammation-induced bone loss, significantly increasing bone mass (70). Vegetarians and individuals adhering to a mediterranean diet tend to have higher SCFA levels, which are associated with improved bone health (101). Dietary supplementation with oligosaccharides enhances SCFA production, contributing to increased BMD (102). In antibiotic-treated mice, SCFA supplementation reduced bone loss without affecting bone turnover rates (103). Thus, maintaining gut barrier integrity and regulating gut microbial metabolites are critical for inhibiting bone resorption and promoting bone formation effectively.
6 Conclusion
PMOP is a complex metabolic bone disorder characterized by estrogen deficiency, which leads to bone loss and structural deterioration through disruptions in the immune-endocrine network. Recent research highlights the central role of intestinal homeostasis in PMOP development by regulating immune and endocrine systems. Dysregulation of the gut microbiota-Th17/Treg-bone metabolism axis has emerged as a key pathogenic mechanism. Disrupted microbial balance alters Th17/Treg homeostasis, stimulates osteoclast formation, and increases bone resorption, ultimately compromising bone integrity. Microbial metabolites influence bone cell function through immune activation and Th17/Treg modulation, driving disease progression. Impaired gut barrier function further initiates inflammation, accelerating bone loss.
These findings suggest that therapeutic strategies targeting gut microbiota and barrier function could regulate metabolic and immune systems, offering new opportunities for PMOP treatment. However, the specific mechanisms through which microbial species or metabolites regulate signaling pathways and immune responses in PMOP remain poorly understood, and their clinical potential requires further validation. Future research should prioritize clinical studies exploring the efficacy and mechanisms of gut microbiota regulation in PMOP prevention and treatment. Investigating the roles of specific probiotics or metabolites, developing novel gut microbiota-targeted therapies, and combining traditional pharmacological treatments with lifestyle interventions could provide innovative approaches for the comprehensive management of PMOP. As the field of osteomicrobiology advances, future studies should also examine the interactions between gut microbiota and drug metabolism in PMOP patients, as well as the potential of gut homeostasis regulation in PMOP therapy. These efforts could lead to novel therapeutic strategies and targets, better addressing patient needs, improving treatment outcomes, and enhancing quality of life.
Author contributions
PQ: Investigation, Writing – original draft, Writing – review & editing. RX: Writing – review & editing, Supervision, Conceptualization, Validation, Investigation, Resources. HL: Writing – review & editing. ZZ: Writing – review & editing. YC: Writing – review & editing. JM: Writing – review & editing. KW: Writing – review & editing. XX: Methodology, Resources, Writing – original draft, Writing – review & editing.
Funding
The author(s) declare financial support was received for the research, authorship, and/or publication of this article. This research was supported by the National Nature Fund Regional Project (82160911, 81860864); Central University Basic Scientific Research Business Expenses Project (31920210041); The special project of science and technology development under the guidance of the central government (YDZX20206200002356).
Acknowledgments
The authors acknowledge the use of Biorender to create schematic representations in Figures 1–3. The agreement numbers associated with this use are ZN27AVF0IQ、UE27AVF81Y、ON27AVFD2P.
Conflict of interest
The authors declare that the research was conducted in the absence of any commercial or financial relationships that could be construed as a potential conflict of interest.
Publisher’s note
All claims expressed in this article are solely those of the authors and do not necessarily represent those of their affiliated organizations, or those of the publisher, the editors and the reviewers. Any product that may be evaluated in this article, or claim that may be made by its manufacturer, is not guaranteed or endorsed by the publisher.
Abbreviations
PMOP, Postmenopausal osteoporosis; Treg, Regulatory T cells; Th17, Helper T cells 17; SCFAs, Short-chain fatty acids; IL-10, Interleukin-10; LPS, Lipopolysaccharide; SFB, Segmented filamentous bacteria; RANKL, Receptor Activator of Nuclear Factor Kappa-Β Ligand; TNF-α, tumor necrosis factor-α; TGF-β, transforming growth factor-β; BMD, bone mineral density ; FMT, Fecal microbiota transplantation; IL-6, Interleukin-6; IL-17, Interleukin-17.
References
1. Watts NB, Bilezikian JP, Camacho PM, Greenspan SL, Harris ST, Hodgson SF, et al. American Association of Clinical Endocrinologists Medical Guidelines for Clinical Practice for the diagnosis and treatment of postmenopausal osteoporosis. Endocr Pract Off J Am Coll Endocrinol Am Assoc Clin Endocrinol. (2010) 16 Suppl 3:1–37. doi: 10.4158/ep.16.s3.1
2. Wang L, Yu W, Yin X, Cui L, Tang S, Jiang N, et al. Prevalence of osteoporosis and fracture in China: the China osteoporosis prevalence study. JAMA Netw Open. (2021) 4:e2121106. doi: 10.1001/jamanetworkopen.2021.21106
3. Ji J, Gu Z, Li N, Dong X, Wang X, Yao Q, et al. Gut microbiota alterations in postmenopausal women with osteoporosis and osteopenia from Shanghai, China. PeerJ. (2024) 12:e17416. doi: 10.7717/peerj.17416
4. Qi P, Lv J, Yan X, Bai L, Zhang L. Microfluidics: insights into intestinal microorganisms. Microorganisms. (2023) 11:1134. doi: 10.3390/microorganisms11051134
5. Ohlsson C, Sjögren K. Effects of the gut microbiota on bone mass. Trends Endocrinol Metab TEM. (2015) 26:69–74. doi: 10.1016/j.tem.2014.11.004
6. Di Tommaso N, Gasbarrini A, Ponziani FR. Intestinal barrier in human health and disease. Int J Environ Res Public Health. (2021) 18:12836. doi: 10.3390/ijerph182312836
7. Camilleri M, Madsen K, Spiller R, Greenwood-Van Meerveld B, Verne GN. Intestinal barrier function in health and gastrointestinal disease. Neurogastroenterol Motil. (2012) 24:503–12. doi: 10.1111/j.1365-2982.2012.01921.x
8. Helgeland L, Vaage JT, Rolstad B, Midtvedt T, Brandtzaeg P. Microbial colonization influences composition and T-cell receptor V beta repertoire of intraepithelial lymphocytes in rat intestine. Immunology. (1996) 89:494–501. doi: 10.1046/j.1365-2567.1996.d01-783.x
9. Li Y, Zhang S-X, Yin X-F, Zhang M-X, Qiao J, Xin X-H, et al. The gut microbiota and its relevance to peripheral lymphocyte subpopulations and cytokines in patients with rheumatoid arthritis. J Immunol Res. (2021) 2021:6665563. doi: 10.1155/2021/6665563
10. Zhao T, Wei Y, Zhu Y, Xie Z, Hai Q, Li Z, et al. Gut microbiota and rheumatoid arthritis: From pathogenesis to novel therapeutic opportunities. Front Immunol. (2022) 13:1007165. doi: 10.3389/fimmu.2022.1007165
11. McCabe LR, Irwin R, Schaefer L, Britton RA. Probiotic use decreases intestinal inflammation and increases bone density in healthy male but not female mice. J Cell Physiol. (2013) 228:1793–8. doi: 10.1002/jcp.24340
12. Guss JD, Horsfield MW, Fontenele FF, Sandoval TN, Luna M, Apoorva F, et al. Alterations to the gut microbiome impair bone strength and tissue material properties. J Bone Miner Res Off J Am Soc Bone Miner Res. (2017) 32:1343–53. doi: 10.1002/jbmr.3114
13. Eastell R, O’Neill TW, Hofbauer LC, Langdahl B, Reid IR, Gold DT, et al. Postmenopausal osteoporosis. Nat Rev Dis Primer. (2016) 2:16069. doi: 10.1038/nrdp.2016.69
14. Black DM, Rosen CJ. Clinical practice. Postmenopausal osteoporosis. N Engl J Med. (2016) 374:254–62. doi: 10.1056/NEJMcp1513724
15. Deshet-Unger N, Kolomansky A, Ben-Califa N, Hiram-Bab S, Gilboa D, Liron T, et al. Erythropoietin receptor in B cells plays a role in bone remodeling in mice. Theranostics. (2020) 10:8744–56. doi: 10.7150/thno.45845
16. Meng X, Lin Z, Cao S, Janowska I, Sonomoto K, Andreev D, et al. Estrogen-mediated downregulation of HIF-1α signaling in B lymphocytes influences postmenopausal bone loss. Bone Res. (2022) 10:15. doi: 10.1038/s41413-022-00189-x
17. Alankus B, Ecker V, Vahl N, Braun M, Weichert W, Macher-Göppinger S, et al. Pathological RANK signaling in B cells drives autoimmunity and chronic lymphocytic leukemia. J Exp Med. (2021) 218:e20200517. doi: 10.1084/jem.20200517
18. Lehmann J, Thiele S, Baschant U, Rachner TD, Niehrs C, Hofbauer LC, et al. Mice lacking DKK1 in T cells exhibit high bone mass and are protected from estrogen-deficiency-induced bone loss. iScience. (2021) 24:102224. doi: 10.1016/j.isci.2021.102224
19. Li J, Wang Q, Yang R, Zhang J, Li X, Zhou X, et al. BMI-1 mediates estrogen-deficiency-induced bone loss by inhibiting reactive oxygen species accumulation and T cell activation. J Bone Miner Res Off J Am Soc Bone Miner Res. (2017) 32:962–73. doi: 10.1002/jbmr.3059
20. Brunetti G, Storlino G, Oranger A, Colaianni G, Faienza MF, Ingravallo G, et al. LIGHT/TNFSF14 regulates estrogen deficiency-induced bone loss. J Pathol. (2020) 250:440–51. doi: 10.1002/path.5385
21. Raphael I, Nalawade S, Eagar TN, Forsthuber TG. T cell subsets and their signature cytokines in autoimmune and inflammatory diseases. Cytokine. (2015) 74:5–17. doi: 10.1016/j.cyto.2014.09.011
22. Bhadricha H, Patel V, Singh AK, Savardekar L, Patil A, Surve S, et al. Increased frequency of Th17 cells and IL-17 levels are associated with low bone mineral density in postmenopausal women. Sci Rep. (2021) 11:16155. doi: 10.1038/s41598-021-95640-0
23. Sapra L, Shokeen N, Porwal K, Saini C, Bhardwaj A, Mathew M, et al. Bifidobacterium longum Ameliorates Ovariectomy-Induced Bone Loss via Enhancing Anti-Osteoclastogenic and Immunomodulatory Potential of Regulatory B Cells (Bregs). Front Immunol. (2022) 13:875788. doi: 10.3389/fimmu.2022.875788
24. Fischer L, Herkner C, Kitte R, Dohnke S, Riewaldt J, Kretschmer K, et al. Foxp3+ Regulatory T cells in bone and hematopoietic homeostasis. Front Endocrinol. (2019) 10:578. doi: 10.3389/fendo.2019.00578
25. Singh KB, Rai R, Khanka S, Singh D. Discontinuation of PTH therapy amplifies bone loss by increasing oxidative stress: An event ameliorated by sequential IL-17 neutralizing antibody therapy. BioMed Pharmacother Biomedecine Pharmacother. (2022) 145:112390. doi: 10.1016/j.biopha.2021.112390
26. Lu L, Liu Y, Nazierhan S, Sun Z, Aikeremu D, Alimasi W, et al. Expression changes of IL-17 in zoledronic acid combined with PVP technology in the treatment of postmenopausal osteoporotic vertebral compression fracture and its predictive value of relapse. J Musculoskelet Neuronal Interact. (2020) 20:563–9.
27. Dixit M, Singh KB, Prakash R, Singh D. Functional block of IL-17 cytokine promotes bone healing by augmenting FOXO1 and ATF4 activity in cortical bone defect model. Osteoporos Int J Establ Result Coop Eur Found Osteoporos Natl Osteoporos Found USA. (2017) 28:2207–20. doi: 10.1007/s00198-017-4012-5
28. Shukla P, Mansoori MN, Singh D. Efficacy of anti-IL-23 monotherapy versus combination therapy with anti-IL-17 in estrogen deficiency induced bone loss conditions. Bone. (2018) 110:84–95. doi: 10.1016/j.bone.2018.01.027
29. Wang Y, Zhang W, Lim S-M, Xu L, Jin J-O. Interleukin-10-producing B cells help suppress ovariectomy-mediated osteoporosis. Immune Netw. (2020) 20:e50. doi: 10.4110/in.2020.20.e50
30. Jann J, Gascon S, Roux S, Faucheux N. Influence of the TGF-β Superfamily on osteoclasts/osteoblasts balance in physiological and pathological bone conditions. Int J Mol Sci. (2020) 21:7597. doi: 10.3390/ijms21207597
31. Zhang Z, Zhang X, Zhao D, Liu B, Wang B, Yu W, et al. TGF−β1 promotes the osteoinduction of human osteoblasts via the PI3K/AKT/mTOR/S6K1 signalling pathway. Mol Med Rep. (2019) 19:3505–18. doi: 10.3892/mmr.2019.10051
32. Noverr MC, Huffnagle GB. Does the microbiota regulate immune responses outside the gut? Trends Microbiol. (2004) 12:562–8. doi: 10.1016/j.tim.2004.10.008
33. Yang W, Cong Y. Gut microbiota-derived metabolites in the regulation of host immune responses and immune-related inflammatory diseases. Cell Mol Immunol. (2021) 18:866–77. doi: 10.1038/s41423-021-00661-4
34. Benveniste J, Lespinats G, Salomon J. Serum and secretory IgA in axenic and holoxenic mice. J Immunol Baltim Md 1950. (1971) 107:1656–62. doi: 10.4049/jimmunol.107.6.1656
35. Macpherson AJ, Harris NL. Interactions between commensal intestinal bacteria and the immune system. Nat Rev Immunol. (2004) 4:478–85. doi: 10.1038/nri1373
36. Zhang X, Chen B, Zhao L-D, Li H. The gut microbiota: emerging evidence in autoimmune diseases. Trends Mol Med. (2020) 26:862–73. doi: 10.1016/j.molmed.2020.04.001
37. Cheng H, Guan X, Chen D, Ma W. The th17/treg cell balance: A gut microbiota-modulated story. Microorganisms. (2019) 7:583. doi: 10.3390/microorganisms7120583
38. Edelblum KL, Sharon G, Singh G, Odenwald MA, Sailer A, Cao S, et al. The microbiome activates CD4 T-cell-mediated immunity to compensate for increased intestinal permeability. Cell Mol Gastroenterol Hepatol. (2017) 4:285–97. doi: 10.1016/j.jcmgh.2017.06.001
39. Omenetti S, Bussi C, Metidji A, Iseppon A, Lee S, Tolaini M, et al. The intestine harbors functionally distinct homeostatic tissue-resident and inflammatory Th17 cells. Immunity. (2019) 51:77–89.e6. doi: 10.1016/j.immuni.2019.05.004
40. Ivanov II, Atarashi K, Manel N, Brodie EL, Shima T, Karaoz U, et al. Induction of intestinal Th17 cells by segmented filamentous bacteria. Cell. (2009) 139:485–98. doi: 10.1016/j.cell.2009.09.033
41. Atarashi K, Tanoue T, Shima T, Imaoka A, Kuwahara T, Momose Y, et al. Induction of colonic regulatory T cells by indigenous Clostridium species. Science. (2011) 331:337–41. doi: 10.1126/science.1198469
42. Atarashi K, Tanoue T, Oshima K, Suda W, Nagano Y, Nishikawa H, et al. Treg induction by a rationally selected mixture of Clostridia strains from the human microbiota. Nature. (2013) 500:232–6. doi: 10.1038/nature12331
43. Wang Y, Yin Y, Chen X, Zhao Y, Wu Y, Li Y, et al. Induction of intestinal Th17 cells by flagellins from segmented filamentous bacteria. Front Immunol. (2019) 10:2750. doi: 10.3389/fimmu.2019.02750
44. Lina C, Conghua W, Nan L, Ping Z. Combined treatment of etanercept and MTX reverses Th1/Th2, Th17/Treg imbalance in patients with rheumatoid arthritis. J Clin Immunol. (2011) 31:596–605. doi: 10.1007/s10875-011-9542-6
45. Chen X, Oppenheim JJ. Th17 cells and Tregs: unlikely allies. J Leukoc Biol. (2014) 95:723–31. doi: 10.1189/jlb.1213633
46. Qin B, Dong L, Guo X, Jiang J, He Y, Wang X, et al. Expression of G protein-coupled estrogen receptor in irritable bowel syndrome and its clinical significance. Int J Clin Exp Pathol. (2014) 7:2238–46.
47. Zhang C, Peng J, Wu S, Jin Y, Xia F, Wang C, et al. Dioscin promotes osteoblastic proliferation and differentiation via Lrp5 and ER pathway in mouse and human osteoblast-like cell lines. J BioMed Sci. (2014) 21:30. doi: 10.1186/1423-0127-21-30
48. Vemuri R, Sylvia KE, Klein SL, Forster SC, Plebanski M, Eri R, et al. The microgenderome revealed: sex differences in bidirectional interactions between the microbiota, hormones, immunity and disease susceptibility. Semin Immunopathol. (2019) 41:265–75. doi: 10.1007/s00281-018-0716-7
49. Hernandez CJ, Guss JD, Luna M, Goldring SR. Links between the microbiome and bone. J Bone Miner Res Off J Am Soc Bone Miner Res. (2016) 31:1638–46. doi: 10.1002/jbmr.2887
50. Untersmayr E, Brandt A, Koidl L, Bergheim I. The intestinal barrier dysfunction as driving factor of inflammaging. Nutrients. (2022) 14:949. doi: 10.3390/nu14050949
51. Majka G, Więcek G, Śróttek M, Śpiewak K, Brindell M, Koziel J, et al. The impact of lactoferrin with different levels of metal saturation on the intestinal epithelial barrier function and mucosal inflammation. Biometals Int J Role Met Ions Biol Biochem Med. (2016) 29:1019–33. doi: 10.1007/s10534-016-9973-x
52. Muñoz M, Heimesaat MM, Danker K, Struck D, Lohmann U, Plickert R, et al. Interleukin (IL)-23 mediates Toxoplasma gondii-induced immunopathology in the gut via matrixmetalloproteinase-2 and IL-22 but independent of IL-17. J Exp Med. (2009) 206:3047–59. doi: 10.1084/jem.20090900
53. Otani T, Furuse M. Tight junction structure and function revisited. Trends Cell Biol. (2020) 30:805–17. doi: 10.1016/j.tcb.2020.08.004
54. Keita AV, Söderholm JD. The intestinal barrier and its regulation by neuroimmune factors. Neurogastroenterol Motil. (2010) 22(7):718–33. doi: 10.1111/j.1365-2982.2010.01498.x
55. He J, Chu Y, Li J, Meng Q, Liu Y, Jin J, et al. Intestinal butyrate-metabolizing species contribute to autoantibody production and bone erosion in rheumatoid arthritis. Sci Adv. (2022) 8:eabm1511. doi: 10.1126/sciadv.abm1511
56. Yu D, Du J, Pu X, Zheng L, Chen S, Wang N, et al. The gut microbiome and metabolites are altered and interrelated in patients with rheumatoid arthritis. Front Cell Infect Microbiol. (2022) 11:763507. doi: 10.3389/fcimb.2021.763507
57. Greenbaum J, Lin X, Su K-J, Gong R, Shen H, Shen J, et al. Integration of the human gut microbiome and serum metabolome reveals novel biological factors involved in the regulation of bone mineral density. Front Cell Infect Microbiol. (2022) 12:853499. doi: 10.3389/fcimb.2022.853499
58. Liu L, Liang L, Liang H, Wang M, Lu B, Xue M, et al. Fusobacterium nucleatum Aggravates the Progression of Colitis by Regulating M1 Macrophage Polarization via AKT2 Pathway. Front Immunol. (2019) 10:1324. doi: 10.3389/fimmu.2019.01324
59. Yang D-H, Yang M-Y. The role of macrophage in the pathogenesis of osteoporosis. Int J Mol Sci. (2019) 20:2093. doi: 10.3390/ijms20092093
60. Cronin O, Lanham-New SA, Corfe BM, Gregson CL, Darling AL, Ahmadi KR, et al. Role of the microbiome in regulating bone metabolism and susceptibility to osteoporosis. Calcif Tissue Int. (2022) 110:273–84. doi: 10.1007/s00223-021-00924-2
61. Li P, Ji B, Luo H, Sundh D, Lorentzon M, Nielsen J. One-year supplementation with Lactobacillus reuteri ATCC PTA 6475 counteracts a degradation of gut microbiota in older women with low bone mineral density. NPJ Biofilms Microbiomes. (2022) 8:84. doi: 10.1038/s41522-022-00348-2
62. Dar HY, Shukla P, Mishra PK, Anupam R, Mondal RK, Tomar GB, et al. Lactobacillus acidophilus inhibits bone loss and increases bone heterogeneity in osteoporotic mice via modulating Treg-Th17 cell balance. Bone Rep. (2018) 8:46–56. doi: 10.1016/j.bonr.2018.02.001
63. Sapra L, Dar HY, Bhardwaj A, Pandey A, Kumari S, Azam Z, et al. Lactobacillus rhamnosus attenuates bone loss and maintains bone health by skewing Treg-Th17 cell balance in Ovx mice. Sci Rep. (2021) 11:1807. doi: 10.1038/s41598-020-80536-2
64. Zhu L, Hua F, Ding W, Ding K, Zhang Y, Xu C. The correlation between the Th17/Treg cell balance and bone health. Immun Ageing A. (2020) 17:30. doi: 10.1186/s12979-020-00202-z
65. Yu M, Malik Tyagi A, Li J-Y, Adams J, Denning TL, Weitzmann MN, et al. PTH induces bone loss via microbial-dependent expansion of intestinal TNF+ T cells and Th17 cells. Nat Commun. (2020) 11:468. doi: 10.1038/s41467-019-14148-4
66. Schnupf P, Gaboriau-Routhiau V, Sansonetti PJ, Cerf-Bensussan N. Segmented filamentous bacteria, Th17 inducers and helpers in a hostile world. Curr Opin Microbiol. (2017) 35:100–9. doi: 10.1016/j.mib.2017.03.004
67. Chen B, Ye D, Luo L, Liu W, Peng K, Shu X, et al. Adhesive bacteria in the terminal ileum of children correlates with increasing Th17 cell activation. Front Pharmacol. (2020) 11:588560. doi: 10.3389/fphar.2020.588560
68. Durant L, Watford WT, Ramos HL, Laurence A, Vahedi G, Wei L, et al. Diverse targets of the transcription factor STAT3 contribute to T cell pathogenicity and homeostasis. Immunity. (2010) 32:605–15. doi: 10.1016/j.immuni.2010.05.003
69. Kalliolias GD, Papavassiliou AG. Stabilizing the integrity of intestinal barrier to control arthritis. Arthritis Res Ther. (2024) 26:135. doi: 10.1186/s13075-024-03378-7
70. Lucas S, Omata Y, Hofmann J, Böttcher M, Iljazovic A, Sarter K, et al. Short-chain fatty acids regulate systemic bone mass and protect from pathological bone loss. Nat Commun. (2018) 9:55. doi: 10.1038/s41467-017-02490-4
71. Rosser EC, Piper CJM, Matei DE, Blair PA, Rendeiro AF, Orford M, et al. Microbiota-derived metabolites suppress arthritis by amplifying aryl-hydrocarbon receptor activation in regulatory B cells. Cell Metab. (2020) 31:837–51. doi: 10.1016/j.cmet.2020.03.003
72. Li J, Bhattacharya S, Zhou J, Phadnis-Moghe AS, Crawford RB, Kaminski NE. Aryl hydrocarbon receptor activation suppresses EBF1 and PAX5 and impairs human B lymphopoiesis. J Immunol Baltim Md. (2017) 199:3504–15. doi: 10.4049/jimmunol.1700289
73. Vaidyanathan B, Chaudhry A, Yewdell WT, Angeletti D, Yen W-F, Wheatley AK, et al. The aryl hydrocarbon receptor controls cell-fate decisions in B cells. J Exp Med. (2017) 214:197–208. doi: 10.1084/jem.20160789
74. Piper CJM, Rosser EC, Oleinika K, Nistala K, Krausgruber T, Rendeiro AF, et al. Aryl hydrocarbon receptor contributes to the transcriptional program of IL-10-producing regulatory B cells. Cell Rep. (2019) 29:1878–1892.e7. doi: 10.1016/j.celrep.2019.10.018
75. Lin L, Zhang K, Xiong Q, Zhang J, Cai B, Huang Z, et al. Gut microbiota in pre-clinical rheumatoid arthritis: From pathogenesis to preventing progression. J Autoimmun. (2023) 141:103001. doi: 10.1016/j.jaut.2023.103001
76. Xu X, Wang M, Wang Z, Chen Q, Chen X, Xu Y, et al. The bridge of the gut–joint axis: Gut microbial metabolites in rheumatoid arthritis. Front Immunol. (2022) 13:1007610 PMID: 36275747. doi: 10.3389/fimmu.2022.1007610
77. Kinashi Y, Hase K. Partners in leaky gut syndrome: intestinal dysbiosis and autoimmunity. Front Immunol. (2021) 12:673708 PMID: 33968085. doi: 10.3389/fimmu.2021.673708
78. Van Itallie CM, Fanning AS, Bridges A, Anderson JM. ZO-1 stabilizes the tight junction solute barrier through coupling to the perijunctional cytoskeleton. Mol Biol Cell. (2009) 20:3930–40. doi: 10.1091/mbc.e09-04-0320
79. Xu X, Jia X, Mo L, Liu C, Zheng L, Yuan Q, et al. Intestinal microbiota: a potential target for the treatment of postmenopausal osteoporosis. Bone Res. (2017) 5:17046. doi: 10.1038/boneres.2017.46
80. Hass MA, Nichol P, Lee L, Levin RM. Estrogen modulates permeability and prostaglandin levels in the rabbit urinary bladder. Prostaglandins Leukot Essent Fatty Acids. (2009) 80:125–9. doi: 10.1016/j.plefa.2008.11.010
81. Kim YG, Lee C-K, Nah S-S, Mun SH, Yoo B, Moon H-B. Human CD4+CD25+ regulatory T cells inhibit the differentiation of osteoclasts from peripheral blood mononuclear cells. Biochem Biophys Res Commun. (2007) 357:1046–52. doi: 10.1016/j.bbrc.2007.04.042
82. Zaiss MM, Axmann R, Zwerina J, Polzer K, Gückel E, Skapenko A, et al. Treg cells suppress osteoclast formation: a new link between the immune system and bone. Arthritis Rheumatol. (2007) 56:4104–12. doi: 10.1002/art.23138
83. Locantore P, Del Gatto V, Gelli S, Paragliola RM, Pontecorvi A. The interplay between immune system and microbiota in osteoporosis. Mediators Inflamm. (2020) 2020:3686749. doi: 10.1155/2020/3686749
84. Wieërs G, Belkhir L, Enaud R, Leclercq S, Philippart de Foy J-M, Dequenne I, et al. How probiotics affect the microbiota. Front Cell Infect Microbiol. (2019) 9:454. doi: 10.3389/fcimb.2019.00454
85. Hill C, Guarner F, Reid G, Gibson GR, Merenstein DJ, Pot B, et al. Expert consensus document. The International Scientific Association for Probiotics and Prebiotics consensus statement on the scope and appropriate use of the term probiotic. Nat Rev Gastroenterol Hepatol. (2014) 11:506–14. doi: 10.1038/nrgastro.2014.66
86. Liu J-H, Yue T, Luo Z-W, Cao J, Yan Z-Q, Jin L, et al. Akkermansia muciniphila promotes type H vessel formation and bone fracture healing by reducing gut permeability and inflammation. Dis Model Mech. (2020) 13:dmm043620. doi: 10.1242/dmm.043620
87. Gao J, Xu K, Liu H, Liu G, Bai M, Peng C, et al. Impact of the gut microbiota on intestinal immunity mediated by tryptophan metabolism. Front Cell Infect Microbiol. (2018) 8:13. doi: 10.3389/fcimb.2018.00013
88. Xiao H, Wang Y, Chen Y, Chen R, Yang C, Geng B, et al. Gut-bone axis research: unveiling the impact of gut microbiota on postmenopausal osteoporosis and osteoclasts through Mendelian randomization. Front Endocrinol. (2024) 15:1419566. doi: 10.3389/fendo.2024.1419566
89. Miller TL, Wolin MJ. Formation of hydrogen and formate by Ruminococcus albus. J Bacteriol. (1973) 116:836–46. doi: 10.1128/jb.116.2.836-846.1973
90. Carbonero F, Benefiel AC, Gaskins HR. Contributions of the microbial hydrogen economy to colonic homeostasis. Nat Rev Gastroenterol Hepatol. (2012) 9:504–18. doi: 10.1038/nrgastro.2012.85
91. Duncan SH, Hold GL, Barcenilla A, Stewart CS, Flint HJ. Roseburia intestinalis sp. nov., a novel saccharolytic, butyrate-producing bacterium from human faeces. Int J Syst Evol Microbiol. (2002) 52:1615–20. doi: 10.1099/00207713-52-5-1615
92. Ooijevaar RE, Terveer EM, Verspaget HW, Kuijper EJ, Keller JJ. Clinical application and potential of fecal microbiota transplantation. Annu Rev Med. (2019) 70:335–51. doi: 10.1146/annurev-med-111717-122956
93. Macdonald TT, Monteleone G. Immunity, inflammation, and allergy in the gut. Science. (2005) 307:1920–5. doi: 10.1126/science.1106442
94. Schoultz I, Keita ÅV. The intestinal barrier and current techniques for the assessment of gut permeability. Cells. (2020) 9:1909. doi: 10.3390/cells9081909
95. Zhang Y-W, Cao M-M, Li Y-J, Lu P-P, Dai G-C, Zhang M, et al. Fecal microbiota transplantation ameliorates bone loss in mice with ovariectomy-induced osteoporosis via modulating gut microbiota and metabolic function. J Orthop Transl. (2022) 37:46–60. doi: 10.1016/j.jot.2022.08.003
96. AlQranei MS, Senbanjo LT, Aljohani H, Hamza T, Chellaiah MA. Lipopolysaccharide- TLR-4 Axis regulates Osteoclastogenesis independent of RANKL/RANK signaling. BMC Immunol. (2021) 22:23. doi: 10.1186/s12865-021-00409-9
97. Schepper JD, Collins F, Rios-Arce ND, Kang HJ, Schaefer L, Gardinier JD, et al. Involvement of the gut microbiota and barrier function in glucocorticoid-induced osteoporosis. J Bone Miner Res Off J Am Soc Bone Miner Res. (2020) 35:801–20. doi: 10.1002/jbmr.3947
98. Kondo T, Chiba T, Tousen Y. Short-chain fatty acids, acetate and propionate, directly upregulate osteoblastic differentiation. Int J Food Sci Nutr. (2022) 73:800–8. doi: 10.1080/09637486.2022.2078285
99. Furusawa Y, Obata Y, Fukuda S, Endo TA, Nakato G, Takahashi D, et al. Commensal microbe-derived butyrate induces the differentiation of colonic regulatory T cells. Nature. (2013) 504:446–50. doi: 10.1038/nature12721
100. Khosla S. The microbiome adds to the complexity of parathyroid hormone action on bone. J Clin Invest. (2020) 130:1615–7. doi: 10.1172/JCI135712
101. Nestares T, Martín-Masot R, de Teresa C, Bonillo R, Maldonado J, Flor-Alemany M, et al. Influence of mediterranean diet adherence and physical activity on bone health in celiac children on a gluten-free diet. Nutrients. (2021) 13:1636. doi: 10.3390/nu13051636
102. Weaver CM, Martin BR, Nakatsu CH, Armstrong AP, Clavijo A, McCabe LD, et al. Galactooligosaccharides improve mineral absorption and bone properties in growing rats through gut fermentation. J Agric Food Chem. (2011) 59:6501–10. doi: 10.1021/jf2009777
Keywords: gut homeostasis, Th17/Treg cell balance, PMOP, mechanism study, gut microbiome
Citation: Qi P, Xie R, Liu H, Zhang Z, Cheng Y, Ma J, Wan K and Xie X (2024) Mechanisms of gut homeostasis regulating Th17/Treg cell balance in PMOP. Front. Immunol. 15:1497311. doi: 10.3389/fimmu.2024.1497311
Received: 16 September 2024; Accepted: 25 November 2024;
Published: 13 December 2024.
Edited by:
Xuefeng Wang, Soochow University, ChinaReviewed by:
Hamid Ahmadi, University of Pécs, HungaryJiajia Ni, Guangzhou National Laboratory, China
Copyright © 2024 Qi, Xie, Liu, Zhang, Cheng, Ma, Wan and Xie. This is an open-access article distributed under the terms of the Creative Commons Attribution License (CC BY). The use, distribution or reproduction in other forums is permitted, provided the original author(s) and the copyright owner(s) are credited and that the original publication in this journal is cited, in accordance with accepted academic practice. No use, distribution or reproduction is permitted which does not comply with these terms.
*Correspondence: XingWen Xie, cTEzMzA5MzI5OTQ5QDE2My5jb20=
†These authors have contributed equally to this work