- 1Department of Critical Care Medicine, Affiliated Hospital of Inner Mongolia Medical University, Hohhot, Inner Mongolia Autonomous Region, China
- 2Department of Orthopaedics, Inner Mongolia Autonomous Region People’s Hospital, Hohhot, Inner Mongolia Autonomous Region, China
- 3Pathology Department, Inner Mongolia Autonomous Region People’s Hospital, Hohhot, Inner Mongolia Autonomous Region, China
- 4Department of Surgery ICU, Affiliated Hospital of Inner Mongolia Medical University, Hohhot, Inner Mongolia Autonomous Region, China
- 5Physical Examination Center, Affiliated Hospital of Inner Mongolia Medical University, Hohhot, Inner Mongolia Autonomous Region, China
Organ transplantation is a vital intervention for end-stage organ failure; however, ischemia-reperfusion injury is a complication of transplantation, affecting the prognosis and survival of transplant recipients. As a complex ecosystem, recent research has highlighted the role of the intestinal microecology in transplantation, revealing its significant interplay with ischemia-reperfusion injury. This review explores the interaction between ischemia-reperfusion injury and intestinal microecology, with a special focus on how ischemia-reperfusion injury affects intestinal microecology and how these microecological changes contribute to complications after organ transplantation, such as infection and rejection. Based on a comprehensive analysis of current research advances, this study proposes potential strategies to improve transplant outcomes, offering guidance for future research and clinical practice.
1 Introduction
Organ transplantation is an effective treatment option for end-stage organ failure. However, due to the inevitable ischemia-reperfusion injury in the process of donor organ acquisition, preservation, and transplantation as well as the function and survival of transplanted organs face challenges (1). Ischemia-reperfusion injury (IRI) is caused by cell hypoxia and the accumulation of metabolites during ischemia, followed by oxidative stress and inflammation during reperfusion in the donor organ, which not only affects the primary function of the transplanted organ but may also lead to long-term complications (2). Intestinal microecology is closely related to the occurrence and development of various diseases, including metabolic, immune, and infectious diseases (3). In recent years, increasing evidence has shown that the intestinal microecology plays an important regulatory role in solid organ transplantation. Therefore, understanding and regulating the impact of IRI on intestinal microecology has become an important research focus, aimed at improving the transplant success rates and patient survival quality. Starting from the mechanism of action of IRI in organ transplantation and intestinal microecology, this paper will deeply explore the interaction between them and analyze the main research progress and application prospects in this field.
2 Mechanisms of IRI
IRI is a serious pathological condition that poses a major challenge during organ transplantation, resulting in substantial cellular damage and death (4). It is a major cause of primary graft dysfunction, a delayed graft function, chronic graft dysfunction, graft rejection, and other post-transplant complications and contributes to increased morbidity and mortality among transplant recipients (5).
2.1 Pathophysiological changes during ischemia
At the cellular level, ischemia leads to hypoxia, which disrupts cellular metabolism and reduces cellular bioenergetics by inhibiting the function of the mitochondrial electron transport chain (6). Low oxygen levels deplete ATP in the mitochondria and shift to anaerobic metabolism, which impairs the function of energy-dependent sodium-potassium pumps at the cell membrane (7). Reduced Na+ -K+ pump activity leads to intracellular Na+ accumulation and further inhibits Na+-H+ pump activity (8). Disruption of ionic homeostasis and maintenance of the membrane potential lead to intracellular Ca2+ overload through inhibition of the Na+-Ca2+ pump (9). The hypertonic state of cells occurs due to elevated intracellular Na+, H+, and Ca2+ concentrations, resulting in water influx into the cytoplasm to maintain osmotic balance, leading to cellular edema (10). The accumulation of H+ in cells and the production of lactic acid by glycolysis can reduce the cellular PH, leading to metabolic acidosis and conformational changes in proteins (1) (Figure 1). In addition, Ca2+ overload can lead to cell damage via excessive production of reactive oxygen species (ROS). ROS accumulation impairs protein folding in the endoplasmic reticulum and activates a range of protein complexes, such as mitogen-activated protein kinase, calcium/calmodulin-dependent protein kinase, protein kinase C, and receptor-interacting protein kinase, which trigger a pro-inflammatory cascade (11). Studies have shown that in the process of ischemia, succinate accumulation leads to ROS accumulation, and pyruvate dehydrogenase kinase 4, a mitochondrial enzyme, can mitigate IRI-induced kidney injury by inhibiting ROS generation (12).
2.2 Pathophysiological changes during reperfusion
Reperfusion refers to the process of restoring blood supply. Although this process provides much-needed oxygen and nutrients, it may exacerbate ischemic injury due to the presence of other pathological processes, such as increased production of ROS, excessive inflammatory response, and programmed cell death (13). Reintroduction of excess oxygen increases ROS production, which disrupts the antioxidant defense system of the cell and results in oxidative stress on the cell membrane (14) (Figure 1). This imbalance between the overproduction of ROS and the cell’s antioxidant capacity is termed oxidative stress.
In a study of mouse heart transplantation, knocking down the mediators of oxidative stress induced excessive activation of ROS and further aggravated the degree of cardiac tissue damage (4). Excessive ROS not only promotes inflammation but also contributes to endothelial cell injury. Oxidative stress caused by ROS induces the release of pro-inflammatory cytokines through the activation of nuclear factor-κB, whereas endothelial cell activation induces endothelial-leukocyte adhesion and endothelial-platelet aggregation, which destroys cellular structure, eventually leading to cell death and aggravating ischemic injury (15). This process stimulates the innate immune response, which in turn enhances the activation of the adaptive immune response and promotes graft allogeneic identification.
IRI significantly affects the prognosis of organ transplantation via a series of complex pathophysiological changes. Regardless of whether it is a metabolic disorder and cell damage during the ischemia phase or oxidative stress and inflammatory response during the reperfusion phase, IRI has profound effects on cells and tissues, potentially leading to organ dysfunction. The next focus will be on how this damage further exacerbates the occurrence of complications after transplantation by disturbing intestinal microecology.
3 Effects of IRI on intestinal microecology
The intestinal microecology is composed of intestinal flora, intestinal epithelial cells, and the intestinal mucosal immune system 9 (16). Under normal circumstances, the quantity and distribution of intestinal microorganisms are relatively constant, and the microbial flora is relatively balanced and stable. However, diseases, immunity, stress, and other factors may affect the quantity, activity, or location of intestinal flora, resulting in an imbalance in intestinal flora and affecting the function of other distant organs (17). Intestinal microecology not only maintains intestinal homeostasis through competition and cooperative relationships but also protects host health through multiple intestinal barrier mechanisms. During organ transplantation, the inflammatory response and tissue damage caused by IRI can directly impair the intestinal barrier, disrupt the structure and function of the intestinal microbial community, and affect the clinical outcome after transplantation.
3.1 Mechanical barrier failure
The intestinal mechanical barrier is composed of intestinal epithelial cells and tight junctions, which are the first lines of defense to prevent the invasion of pathogenic microorganisms and toxins (18). Tight junctions are composed of membrane proteins, such as claudin, occludin, and junctional adhesion molecules, along with peripheral cytoplasmic proteins, such as zonula occludens (ZO), whose expression is inversely correlated with intestinal permeability (19) (Figure 2). During liver transplantation, intestinal blood flow is slowed due to clamping of the inferior vena cava and portal vein, and extensive intestinal mucosa is damaged by ischemia and hypoxia (20). The results of orthotopic liver transplantation male rats study showed that indicators representing an impaired intestinal barrier function (including endotoxin, diamine oxidase, and D-lactate levels) were significantly increased during IRI. At the same time, IRI after liver transplantation leads to the downregulation of Occludin and ZO-1 protein expression and increases epithelial cellular gaps in the rat intestine, which increases the risk of translocation of toxins and microorganisms through the paracellular pathway (21). Further studies have shown that intestinal epithelial cells are susceptible to the effects of IRI and then undergo apoptosis during transplantation. The expression of caspase-3 and apoptosis of intestinal epithelial cells increase significantly within 8 h of reperfusion after transplantation, indicating that the early stage of reperfusion is a critical period for intestinal barrier dysfunction (22). This phenomenon has also been verified in a kidney IRI model (23). In addition, the high level of endotoxins in the serum induces a host inflammatory response, which further exacerbates intestinal mucosal permeability (24).
3.2 Chemical barrier damage
The intestinal chemical barrier is mainly composed of a mucus layer secreted by intestinal mucosal epithelial cells, which can neutralize the acidic environment, prevent adhesion of pathogenic bacteria, and inhibit their growth (25) (Figure 2). The structure of the intestinal mucus layer is significantly altered during IRI, which may be related to ROS accumulation and inflammatory responses in the host (26). IRI stimulates the inflammatory response and oxidative stress by activating neutrophils, which release inflammatory cytokines and free radicals (27). Heme oxygenase (HO) is a specialized enzyme that degrades heme and is assembled from biliverdin, carbon monoxide, and free iron (28). HO-1 is normally expressed in the mucosal layer of the gastrointestinal tract and protects against stress-related tissue damage (29). In the intestinal transplantation and liver transplantation mouse model, compared with the control group, pretreatment with HO-1 or biliverdin can significantly inhibit leukocyte aggregation, thereby reducing the expression of proinflammatory cytokines and chemokines and ultimately diminishing systemic inflammation and oxidative stress (26, 30).
Regarding oxidative stress induced by IRI, researchers have found that glutamine and hydrogen can effectively reduce the oxidative stress response and even alleviate other organ complications in recipients by increasing endogenous cellular antioxidants (31). Hydrogen can play an antioxidant role by selectively neutralizing hydroxyl radicals. Because of its high diffusivity, it can reach mitochondria, which produce a large amount of ROS during IRI, and nuclear subcellular compartments, where ROS accumulation causes DNA damage (32). In addition, insufficient ATP synthesis caused by hypoxia reduces the frequency of ciliary oscillation, slows down intestinal peristalsis, and inhibits intestinal self-cleaning, which may cause accumulation of pathogenic bacteria in the intestine (33). These factors collectively contribute to impairment of the intestinal chemical barrier during organ transplantation, which in turn may trigger bacterial translocation and systemic inflammatory responses.
3.3 Immune barrier weakening
The immune barrier is an important part of the intestinal microecology and the body’s immune defense function, which protects the host from pathogenic antigen attack through both humoral and cellular immunity (34). Secretory immunoglobulin A (sIgA), the main immunoglobulin in intestinal secretions, is produced by lymphocytes and plasma cells throughout the intestinal mucosa and is a major defense mechanism against pathogen invasion by binding and neutralizing pathogens and preventing them from adhering to the surface of the intestinal mucosa (35) (Figure 2). Both the response to pathogens under normal conditions, such as harmless food antigens or commensal bacteria, and the induction of tolerance are the dual functions of sIgA in maintaining intestinal mucosal homeostasis. This process can be achieved by directly identifying the receptor binding domain of the pathogen to prevent the pathogen from interacting with epithelial cells and altering bacterial viability or pathogenicity (36). Specifically, sIgA promotes the health of intestinal epithelial cells and supports the expression of tight junction proteins, thereby maintaining the integrity of the intestinal barrier. In addition, sIgA may also indirectly enhance host immune defense by affecting the composition of gut microbiota. In the study by Lin et al., PBS-pretreated infected mice treated with probiotics exhibited higher sIgA levels and showed a more similar gut microbiota to healthy mice, as shown by a decrease in Proteobacteria and an increase in Bacteroidobacteria, along with a significant decrease in intestinal permeability and LPS levels. This suggests that sIgA can further effectively reduce the incidence of intestinal barrier damage by controlling intestinal microbiota homeostasis (37). Ischemic preconditioning before organ transplantation can effectively reduce fecal sIgA levels (38). In the mouse intestinal ischaemia/reperfusion model, IgA mRNA expression in the intestinal mucosa, sIgA concentration in the lavage fluid, and the percentage of bacteria coated with IgA in the feces of the injury group was lower than that of the sham-operated group 2 h after reperfusion, indicating that intestinal IRI not only reduced sIgA production but also impaired the bacterial binding ability of sIgA after reperfusion (39). These alterations set the stage for subsequent pathogen penetration into the intestinal barrier, facilitating bacterial translocation.
3.4 Biological barrier
The biological barrier is composed of a bacterial membrane layer formed by the attachment of deep intestinal microorganisms, such as Bifidobacterium and Lactobacillus, to the intestinal mucosa, which can maintain the balance of intestinal flora and inhibit the invasion and proliferation of pathogenic bacteria (Figure 2). Intestinal bacteria play a crucial role in forming biological barriers, hindering the entry of harmful bacteria and promoting the growth and maturation of the immune system by producing various enzymes (40). For example, some genera of Firmicutes can penetrate the mucus layer, which can stimulate intestinal epithelial cells to produce large amounts of antimicrobial proteins and limit the colonization of pathogenic microorganisms in the gut (41). In contrast, IRI can lead to a significant decrease in the diversity of the intestinal microbiota, manifested as a decrease in beneficial bacteria and an increase in harmful bacteria. For example, multiple liver transplantation studies have found that IRI leads to a significant decrease in beneficial bacteria, such as Lactobacillus and Bifidobacterium, while opportunistic pathogens, such as Enterococcus and Enterobacteriaceae, are increased (42). Simultaneously, the inflammatory response and oxidative stress caused by IRI also damage the intestinal mechanical, chemical, and immune barriers, thereby promoting bacterial colonization and translocation and further increasing the risk of enterogenous infection (39). For example, increased intestinal permeability, bacterial translocation, and endotoxemia can be clearly observed in a liver ischemia-reperfusion model (43).
In addition, researchers have found that an increase in Enterobacteriaceae and a decrease in Lactobacillus and Ruminococcus are markers of kidney IRI-induced ecological dysregulation and are associated with a decline in short-chain fatty acid (SCFA) levels (44). SCFAs are the main products of dietary fiber fermentation by intestinal flora, which play a crucial role in maintaining the intestinal barrier function, regulating immune responses, and preventing inflammation (45). Additional supplementation with SCFAs can significantly protect intestinal villi from IRI (46).
In conclusion, disruption of any intestinal defense barrier during organ transplant IRI can lead to intestinal microecological disorders. Once these intestinal defense barriers fail, the intestinal microecological balance is disrupted, causing intestinal damage and potentially triggering or exacerbating a series of post-transplantation complications. Understanding and intervening in these injury mechanisms are important for improving the success rate of organ transplantation and the prognosis of patients after transplantation.
4 Influence of intestinal microecology on complications after transplantation
The destruction of tight junction proteins and the inflammatory response caused by IRI can trigger intestinal flora imbalance, which increases the risk of infection, acute rejection, and graft death after transplantation.
4.1 Early postoperative infection
As one of the most common complications of solid organ transplantation, infection can significantly increase morbidity and mortality among patients (47) (Table 1). In 2018, Haak et al. indicated that a lack of butyrate-producing bacteria in the fecal microbiota was associated with an increased susceptibility to respiratory infections in allogeneic hematopoietic stem cell transplant recipients, at the same time, an abundance of butyrate producing bacteria >1% was associated with a 5-fold reduction in the development of future lower respiratory tract viral infections (48). Based on this, Lee et al. conducted a study involving 168 kidney transplant recipients and showed that the relative abundance of fecal butyrate-producing intestinal bacteria in the stool was >1% had a significantly lower risk of respiratory viral infections and influenza at 6 months, 1 year, and 2 years after transplantation than those with lower levels of these bacterias. The study also noted that high levels of butyrate-producing gut bacteria were associated with a reduced risk of cytomegalovirus viremia one year after kidney transplantation (49). Simultaneously, a decreased abundance of Firmicutes and Faecalibacterium prausnitzii and an increased proportion of Bacteroidetes and Proteobacteria were found in the intestines of kidney transplant recipients, which was closely related to the occurrence of infection after transplantation (52). Due to the anatomical connectivity of portal circulation, the liver is constantly exposed to bacterial products of intestinal microbiome origin, and Kato et al. found that loss of intestinal flora diversity is highly associated with the occurrence of bloodstream infections after liver transplantation (50). Antimicrobial therapy, which is commonly used to prevent or treat infections after transplantation, can lead to the colonization of multidrug-resistant pathogens, such as Clostridium difficile, further exacerbating ecological dysbiosis. Bruminhent et al. showed that Clostridium difficile infection (CDI) is an independent risk factor for mortality in the heart transplant population and is more common in patients undergoing retransplantation than the general population. Studies have shown that CDI has the highest frequency within one month of heart transplantation (53). Similarly, in the liver transplant population, colonization of multi-drug-resistant strains was significantly associated with increased levels of Enterococcus and Klebsiella and decreased levels of Bacteroides and Lachnospira in the gut microbiota (51). In addition, the authors suggested that the reduced alpha diversity of the intestinal microbiota before transplantation appears to be a marker of colonization by carbapenem-resistant Enterobacteriaceae following liver transplantation (51).
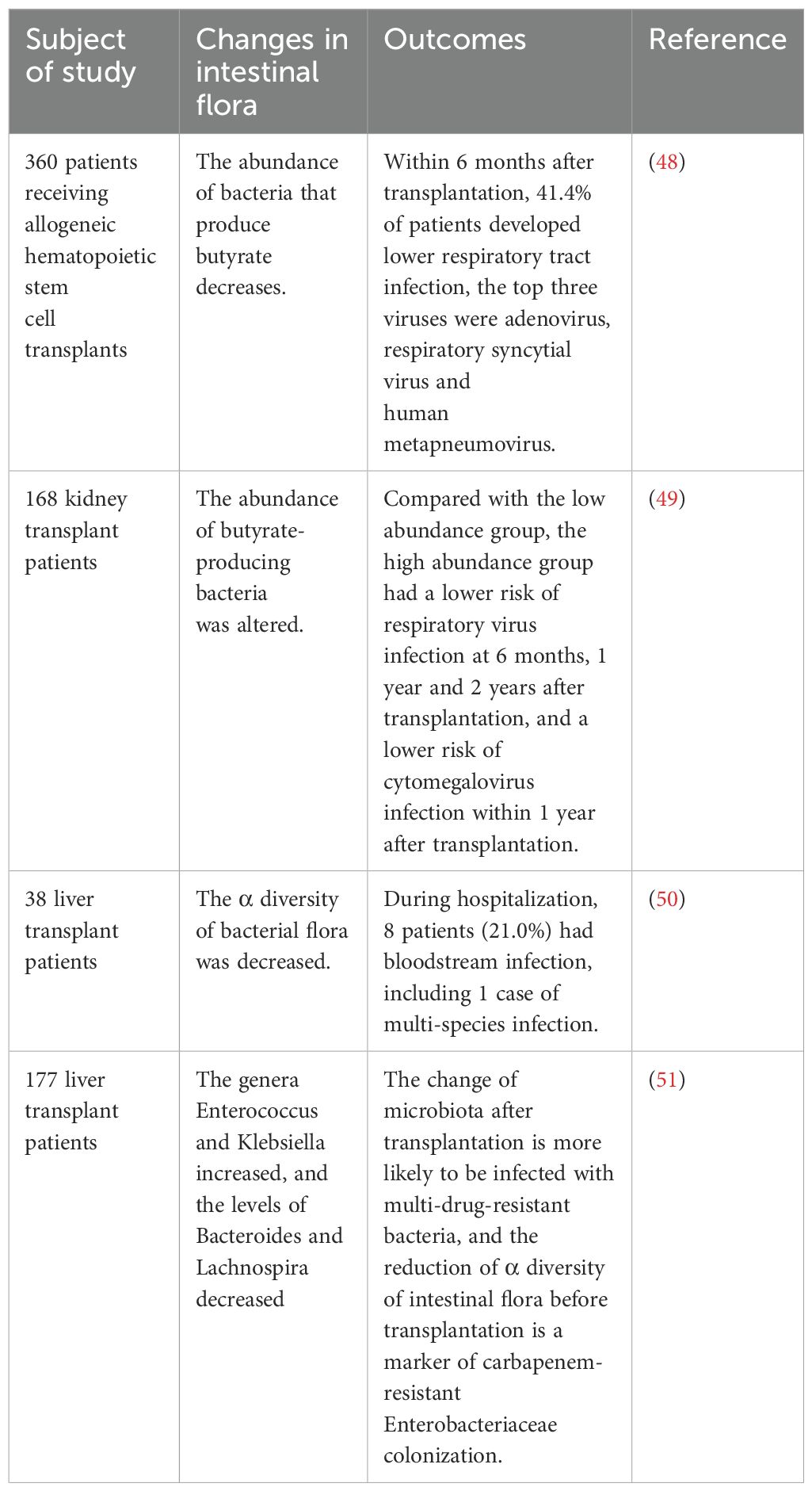
Table 1. Evidence of the association between intestinal microecology and infection in transplant recipients.
4.2 Acute rejection
Acute rejection remains the leading cause of graft dysfunction after organ transplantation (54). Previous studies suggested that postoperative intestinal bacterial translocation plays a key role in the development of immune rejection (Table 2). Lei et al. showed that both germ-free and antibiotic-pre-treated mice exhibited a significant reduction in alloimmune responses and increased the graft survival after skin grafting. However, when these germ-free mice were transplanted with intestinal bacteria from conventional mice, skin graft rejection was accelerated, indicating that intestinal bacteria have an important impact on the process of rejection (55). Similarly, Wang et al. found that patients with decreased abundances of Firmicutes and Bacteroidetes and increased abundances of Proteobacteria, Actinobacteria, Lactobacillus, Fusobacteria, and Faecalibacterium in the intestinal flora after kidney transplantation were more likely to have graft rejection than those with a more balanced microbial composition or those who maintained higher levels of Firmicutes and Bacteroidetes (56). This was further supported by Kato et al., who reported that liver transplant recipients with rejection had a significant increase in Bacteroidaceae, Enterobacteriaceae, Streptococcaceae, and Bifidobacteriaceae, along with a significant decrease in Enterococcaceae, Lactobacillaceae, Clostridiaceae, Ruminococcaceae, and Peptostreptococcaceae in their intestines compared to healthy recipients (50). In animal models, mice with reduced levels of Enterococcaceae and Lactobacillaceae and increased levels of Clostridiaceae in the gut showed higher rejection (60). Similar phenomena have been observed in other organ transplants. For example, heart transplant mice treated with endogenous Bifidobacterium pseudolongum derived from the feces of pregnant mice showed lower rejection than those treated with conventional antibiotics or untreated controls (57). Using a multi-omics analysis, Wu et al. discovered that a species of the genus Bacteroides significantly reduced by 75% when it increased the acute rejection of lung transplantation (58). In patients undergoing small intestine transplantation, an increased Proteobacteria/Firmicutes ratio can be identified as a sensitive and specific indicator of rejection (59).
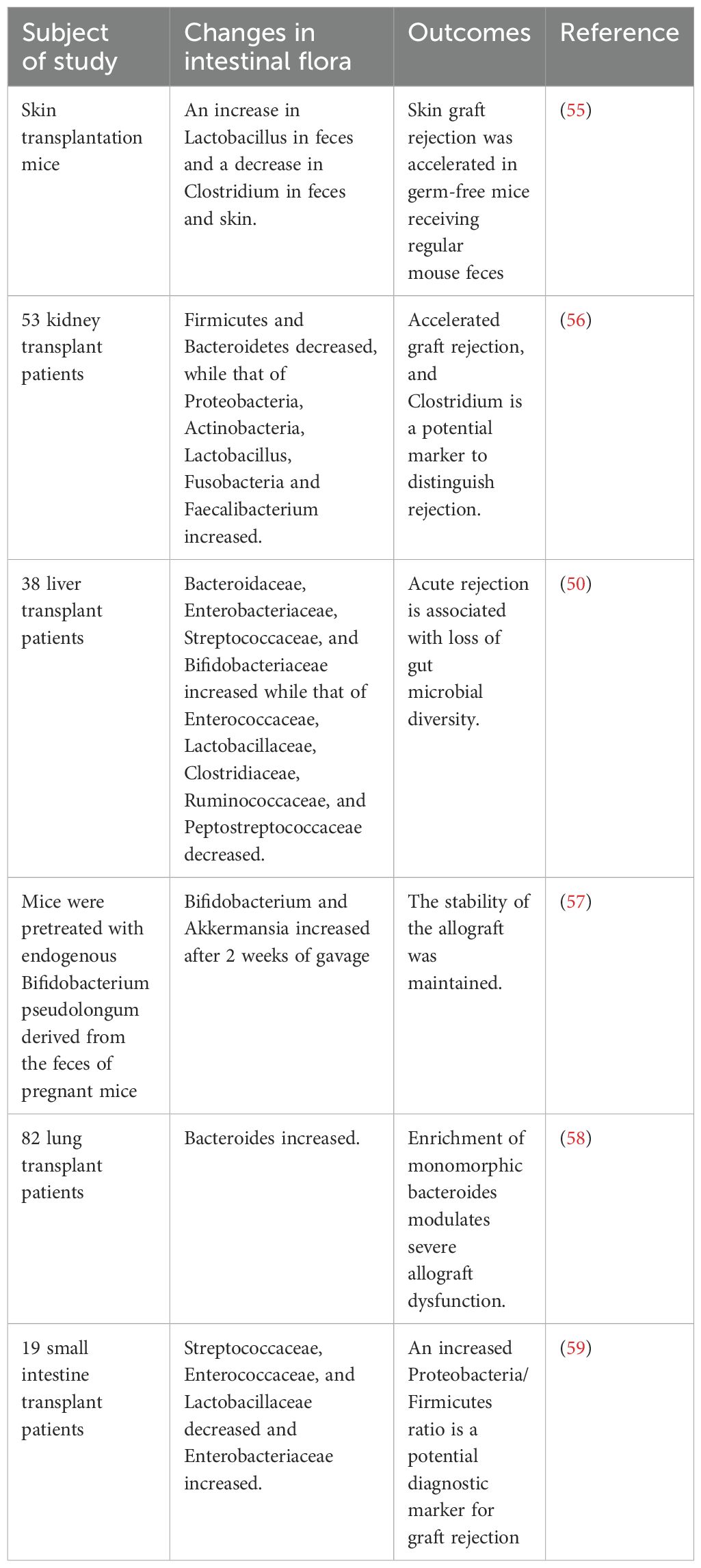
Table 2. Evidence of the association between intestinal microecology and acute rejection in transplant recipients.
4.3 Graft survival rate
Recent studies have established a strong link between the intestinal microecology and improved graft survival outcomes after transplantation. First, Peled et al. demonstrated that a pattern of microbiota disruption, characterized by loss of diversity and dominance of a single taxon, was observed in allogeneic hematopoietic stem cell transplantation and then proposed an association between lower intestinal diversity and the risk of transplantation-related death, as well as death due to graft-versus-host disease (61). Specifically, a higher abundance of Gammaproteobacteria, including Enterobacteriaceae, was associated with higher mortality, whereas a higher abundance of Lachnospiraceae and Actinomycetaceae was associated with a better prognosis (62). Swarte et al. conducted a shotgun metagenomics analysis to investigate the impact of microbial dysbiosis on recipient survival following liver and kidney transplantation. Their study revealed that as the severity of microbial dysbiosis increased, the overall survival period of recipients significantly decreased. Specifically, by comparing the Shannon diversity index and Aitchison distance, they found that for every unit decrease in microbial diversity at the time of liver transplantation, the overall risk of mortality increased by 45%. Additionally, a smaller Aitchison distance was associated with a lower likelihood of mortality after liver transplantation, indicating a close relationship between microbial community similarity and recipient prognosis. This finding was also applicable to kidney transplantation (63). In addition, Willner et al. Found that the emergence of new Pseudomonas species after transplantation was associated with the most severe complications of lung transplantation (64). Based on this research, several specific taxa, including Atopobium, Coprobacillus, Megamonas, Subdoligranulum, and Enterococcaceae, were found to be significantly related to the risk of mortality following liver transplantation (63). In basic experiments, the use of mice from different suppliers confirmed that Alistipes can significantly affect the survival time of skin grafts (65). The intestinal microecology plays an important role in the development of complications after transplantation. Changes in intestinal microecology caused by ischemia-reperfusion injury not only increase the risk of early postoperative infection but are also closely related to acute rejection and graft mortality. A deeper understanding of how intestinal microecology impacts post-transplant complications is helpful in exploring new treatment strategies, thereby improving the success rate and long-term prognosis of patients after transplantation.
5 Application prospects of microbial therapy
In recent years, as our understanding of organ transplantation-related injuries has deepened, microbial therapy has emerged as a promising therapeutic strategy for preventing IRI (Table 3). This approach leverages manipulation of the gut microbiota to potentially mitigate the adverse effects of IRI, offering a novel strategy to improve outcomes in organ transplantation. Previous studies have shown that the recovery of intestinal barrier damage after IRI is closely related to the timing of intervention. According to the study of Kato et al., intestinal microecological intervention in the early stage after liver transplantation (within 72 hours after surgery) can significantly reduce the incidence of postoperative infection (52).
5.1 Probiotics and prebiotics
The International Scientific Society for Probiotics and Prebiotics defines probiotics as “living microorganisms that, when given in sufficient quantities, can exert beneficial effects on the health of the host” (73). The beneficial effects of probiotics are mainly achieved through the following mechanisms: inhibition of intestinal epithelial cell apoptosis, stimulation of mucus secretion, downregulation of pro-inflammatory mediators, upregulation of anti-inflammatory responses, interference with pathogen adhesion and invasion, and activation of innate and adaptive immune responses (74).
Most studies on the application of probiotics in organ transplantation have focused on animal models. Studies have shown that supplementation with probiotics containing Blautia products can reverse vancomycin-resistant Enterococcus infection in germ-free mice (69). Similar results have also been observed in clinical studies, in which the incidence of bacterial infections after liver and kidney transplantation was significantly reduced in patients treated with Lactobacillus plantarum (67, 75). The study by Grat et al. further confirmed that post-transplant infection rates were significantly reduced in liver transplant recipients supplemented with probiotics, along with improvements in biochemical markers of allograft function, such as bilirubin concentration and transaminase activity (68). The role of prebiotics in organ transplant complications is as important as that of a food source that promotes the growth of beneficial gut microbes. In kidney transplant recipients with gastrointestinal symptoms, significant suppression of infection and gastrointestinal symptoms was observed after seven weeks of treatment with prebiotic powder suspension (69).
5.2 Fecal microbial transplantation
FMT is the transfer of feces from healthy donors to the colon of patients with diseases caused by altered microbiota, with the aim of restoring the normal microbiota to cure the disease (Figure 3). The earliest application of FMT was for the treatment of recurrent CDI (76). The first successful FMT procedure was performed in 2012 in an immunocompromised allogeneic stem cell transplant recipient with severe CDI (77). Subsequently, a multicenter, retrospective study demonstrated the safety and efficacy of FMT in the treatment of severe or explosive CDI after organ transplantation (70). In a 2016 case report, FMT was administered to patients with acute rejection after liver transplantation, and clinical symptoms improved and intestinal mucosal repair appeared within 24 hours after transplantation (78).
In addition, FMT can effectively improve microbial diversity (79), rebuild the composition of intestinal microbiota, and protect the graft from multi-drug-resistant bacterial damage (71). However, although FMT effectively prevents the development of CDI after transplantation by reestablishing microbiota complexity, it is still largely hindered by undefined microbiome-coding genes and gene clusters critical for resistance to CDI.
Bacteriophage therapy (BT) has been proposed as a new strategy to prevent multi-drug-resistant bacterial infections. Among the 3 lung transplant recipients with life-threatening multi-drug-resistant bacterial infections caused by Pseudomonas aeruginosa (n=2) and Burkholderia dolosa (n=1), 2 were successfully discharged from the hospital off ventilator support after BT treatment, and no adverse events related to BT were found in any of the 3 cases (72).
Microbial therapy has shown great potential in organ transplantation. These therapies reduce organ transplantation-related complications by regulating the intestinal microecology (Table 4). Despite their promising prospects, the safety, efficacy, and standardized application of these techniques still face many challenges. Further clinical studies are needed to validate and optimize them to promote their widespread application in organ transplantation.
6 Summary
In summary, IRI, as a key factor affecting the success of organ transplantation, not only directly damages the graft function but also leads to a series of postoperative complications by destroying the intestinal barrier and microecological balance. The intestinal microecology plays an important role in the recovery process after transplantation, and its imbalance is closely related to infection, acute rejection, and increased graft mortality. Therefore, maintaining and reconstructing the stability of the intestinal microecology is of great significance in reducing the incidence of complications after transplantation. Microbial therapies, such as probiotics and fecal microbial transplantation, have shown great potential in improving the transplant prognosis. Future studies should further reveal the mechanistic link between intestinal microbiota and organ transplantation and explore individualized microbial therapy strategies to optimize the effects of organ transplantation and improve the long-term prognosis of patients. In-depth study and regulation of the intestinal microecology will provide a new therapeutic perspective and method for organ transplantation.
Author contributions
YL: Writing – original draft, Writing – review & editing. PL: Writing – review & editing. YG: Writing – review & editing. TY: Writing – review & editing. YL: Writing – review & editing. ZL: Writing – review & editing. SZ: Writing – review & editing.
Funding
The author(s) declare financial support was received for the research, authorship, and/or publication of this article. Supported by National Natural Science Foundation of China (61865014) and Inner Mongolia Autonomous Region science and technology program (2021GG0129).
Conflict of interest
The authors declare that this study was conducted in the absence of any commercial or financial relationship that could be interpreted as a potential conflict of interest.
Publisher’s note
All claims expressed in this article are solely those of the authors and do not necessarily represent those of their affiliated organizations, or those of the publisher, the editors and the reviewers. Any product that may be evaluated in this article, or claim that may be made by its manufacturer, is not guaranteed or endorsed by the publisher.
References
1. Fernández AR, Sánchez-Tarjuelo R, Cravedi P, Ochando J, López-Hoyos M. Review: Ischemia Reperfusion Injury-A Translational Perspective In Organ Transplantation. Int J Mol Sci. (2020) 21:8549. doi: 10.3390/ijms21228549
2. Dery KJ, Yao S, Cheng B, Kupiec-Weglinski JW. New Therapeutic Concepts Against Ischemia-Reperfusion Injury In Organ Transplantation. Expert Rev Clin Immunol. (2023) 19:1205–24. doi: 10.1080/1744666X.2023.2240516
3. Ahearn-Ford S, Je B, Stewart CJ. Development Of The Gut Microbiome In Early Life. Exp Physiol. (2022) 107:415–21. doi: 10.1113/eph.v107.5
4. Fei Q, Liu J, Qiao L, Zhang M, Xia H, Lu D, et al. Mst1 Attenuates Myocardial Ischemia/Reperfusion Injury Following Heterotopic Heart Transplantation In Mice Through Regulating Keap1/Nrf2 Axis. Biochem Biophys Res Commun. (2023) 644:140–8. doi: 10.1016/j.bbrc.2022.12.087
5. Wang M, Pan W, Xu Y, Zhang J, Wan J, Jiang H. Microglia-Mediated Neuroinflammation: A Potential Target For The Treatment Of Cardiovascular Diseases. J Inflammation Res. (2022) 15:3083–94. doi: 10.2147/JIR.S350109
6. Kowalczyk P, Sulejczak D, Kleczkowska P, Bukowska-Ośko I, Kucia M, Popiel M, et al. Mitochondrial Oxidative Stress-A Causative Factor And Therapeutic Target In Many Diseases. Int J Mol Sci. (2021) 22:13384. doi: 10.3390/ijms222413384
7. Chen W, Wang L, Liang P, Mast J, Mathis C, Liu CY, et al. Reducing Ischemic Kidney Injury Through Application Of A Synchronization Modulation Electric Field To Maintain Na(+)/K(+)-Atpase Functions. Sci Transl Med. (2022) 14:eabj4906. doi: 10.1126/scitranslmed.abj4906
8. Abdulsalam TM, Hasanin AH, Mohamed RH, El Sayed Badawy A. Angiotensin Receptor-Neprilysin Inhibitior (Thiorphan/Irbesartan) Decreased Ischemia-Reperfusion Induced Ventricular Arrhythmias In Rat; In Vivo Study. Eur J Pharmacol. (2020) 882:173295. doi: 10.1016/j.ejphar.2020.173295
9. Zhao R, Xie E, Yang X, Gong B. Alliin Alleviates Myocardial Ischemia-Reperfusion Injury By Promoting Autophagy. Biochem Biophys Res Commun. (2019) 512:236–43. doi: 10.1016/j.bbrc.2019.03.046
10. Sumida TS. Hyperosmotic Stress Response Regulates Interstitial Homeostasis And Pathogenic Inflammation. J Biochem. (2023) 173:159–66. doi: 10.1093/jb/mvad009
11. Movahed M, Brockie S, Hong J, Fehlings MG. Transcriptomic Hallmarks Of Ischemia-Reperfusion Injury. Cells. (2021) 10:1838. doi: 10.3390/cells10071838
12. Oh CJ, Kim MJ, Lee JM, Kim DH, Kim IY, Park S, et al. Inhibition Of Pyruvate Dehydrogenase Kinase 4 Ameliorates Kidney Ischemia-Reperfusion Injury By Reducing Succinate Accumulation During Ischemia And Preserving Mitochondrial Function During Reperfusion. Kidney Int. (2023) 104:724–39. doi: 10.1016/j.kint.2023.06.022
13. Tang Q, Dong C, Sun Q. Immune Response Associated With Ischemia And Reperfusion Injury During Organ Transplantation. Inflammation Res. (2022) 71:1463–76. doi: 10.1007/s00011-022-01651-6
14. Carcy R, Cougnon M, Poet M, Durandy M, Sicard A, Counillon L, et al. Targeting Oxidative Stress, A Crucial Challenge In Renal Transplantation Outcome. Free Radic Biol Med. (2021) 169:258–70. doi: 10.1016/j.freeradbiomed.2021.04.023
15. Zhang S, Shaw-Boden J, Banz Y, Bongoni AK, Taddeo A, Spirig R, et al. Effects Of C1 Inhibitor On Endothelial Cell Activation In A Rat Hind Limb Ischemia-Reperfusion Injury Model. J Vasc Surg. (2018) 68:209s–221s.E2. doi: 10.1016/j.jvs.2017.10.072
16. Wiertsema SP, Van Bergenhenegouwen J, Garssen J, Knippels LMJ. The Interplay Between The Gut Microbiome And The Immune System In The Context Of Infectious Diseases Throughout Life And The Role Of Nutrition In Optimizing Treatment Strategies. Nutrients. (2021) 13:886. doi: 10.3390/nu13030886
17. Afzaal M, Saeed F, Shah YA, Hussain M, Rabail R, Socol CT, et al. Human Gut Microbiota In Health And Disease: Unveiling The Relationship. Front Microbiol. (2022) 13:999001. doi: 10.3389/fmicb.2022.999001
18. Assimakopoulos SF, Eleftheriotis G, Lagadinou M, Karamouzos V, Dousdampanis P, Siakallis G, et al. Sars Cov-2-Induced Viral Sepsis: The Role Of Gut Barrier Dysfunction. Microorganisms. (2022) 10:1050. doi: 10.3390/microorganisms10051050
19. Allam-Ndoul B, Castonguay-Paradis S, Veilleux A. Gut Microbiota And Intestinal Trans-Epithelial Permeability. Int J Mol Sci. (2020) 21:6402. doi: 10.3390/ijms21176402
20. Woodhouse CA, Patel VC, Singanayagam A, Shawcross DL. Review Article: The Gut Microbiome As A Therapeutic Target In The Pathogenesis And Treatment Of Chronic Liver Disease. Aliment Pharmacol Ther. (2018) 47:192–202. doi: 10.1111/apt.2018.47.issue-2
21. Lv P, Chen T, Liu P, Zheng L, Tian J, Tan F, et al. Dexmedetomidine Attenuates Orthotopic Liver Transplantation-Induced Acute Gut Injury Via α 2-Adrenergic Receptor-Dependent Suppression Of Oxidative Stress. Oxid Med Cell Longev. (2019) 2019:9426368. doi: 10.1155/2019/9426368
22. Yuan DD, Chi XJ, Jin Y, Li X, Ge M, Gao WL, et al. Intestinal Injury Following Liver Transplantation Was Mediated By Tlr4/Nf-κb Activation-Induced Cell Apoptosis. Mol Med Rep. (2016) 13:1525–32. doi: 10.3892/mmr.2015.4719
23. Yang J, Ji GE, Park MS, Seong YJ, Go YS, Lee HY, et al. Probiotics Partially Attenuate The Severity Of Acute Kidney Injury Through An Immunomodulatory Effect. Kidney Res Clin Pract. (2021) 40:620–33. doi: 10.23876/j.krcp.20.265
24. Ding C, Han F, Xiang H, Wang Y, Li Y, Zheng J, et al. Probiotics Ameliorate Renal Ischemia-Reperfusion Injury By Modulating The Phenotype Of Macrophages Through The Il-10/Gsk-3β/Pten Signaling Pathway. Pflugers Arch. (2019) 471:573–81. doi: 10.1007/s00424-018-2213-1
25. Gao Y, Meng L, Liu H, Wang J, Zheng N. The Compromised Intestinal Barrier Induced By Mycotoxins. Toxins (Basel). (2020) 12:619. doi: 10.3390/toxins12100619
26. Nojima T, Obara T, Yamamoto H, Yumoto T, Igawa T, Aokage T, et al. Luminal Administration Of Biliverdin Ameliorates Ischemia-Reperfusion Injury Following Intestinal Transplant In Rats. Surgery. (2022) 172:1522–8. doi: 10.1016/j.surg.2022.07.021
27. Aboelez MO, Ezelarab H, Alotaibi G, Abouzed DEE. Inflammatory Setting, Therapeutic Strategies Targeting Some Pro-Inflammatory Cytokines And Pathways In Mitigating Ischemia/Reperfusion-Induced Hepatic Injury: A Comprehensive Review. Naunyn Schmiedebergs Arch Pharmacol. (2024) 397(9):6299–315. doi: 10.1007/s00210-024-03074-y
28. Dery KJ, Nakamura K, Kadono K, Et al. Human Antigen R. (Hur): A Regulator Of Heme Oxygenase-1 Cytoprotection In Mouse And Human Liver Transplant Injury. Hepatology. (2020) 72:1056–72. doi: 10.1002/hep.31093
29. Corona D, Ekser B, Gioco R, Caruso M, Schipa C, Veroux P, et al. Heme-Oxygenase And Kidney Transplantation: A Potential For Target Therapy. Biomolecules. (2020) 10:840. doi: 10.3390/biom10060840
30. Chi X, Yao W, Xia H, Jin Y, Li X, Cai J, et al. Elevation Of Ho-1 Expression Mitigates Intestinal Ischemia-Reperfusion Injury And Restores Tight Junction Function In A Rat Liver Transplantation Model. Oxid Med Cell Longev. (2015) 2015:986075. doi: 10.1155/2015/986075
31. Shu X, Zhang J, Wang Q, Xu Z, Yu T. Glutamine Decreases Intestinal Mucosal Injury In A Rat Model Of Intestinal Ischemia-Reperfusion By Downregulating Hmgb1 And Inflammatory Cytokine Expression. Exp Ther Med. (2016) 12:1367–72. doi: 10.3892/etm.2016.3468
32. Buchholz BM, Kaczorowski DJ, Sugimoto R, Yang R, Wang Y, Billiar TR, et al. Hydrogen Inhalation Ameliorates Oxidative Stress In Transplantation Induced Intestinal Graft Injury. Am J Transplant. (2008) 8:2015–24. doi: 10.1111/j.1600-6143.2008.02359.x
33. Luo H, Guo P, Zhou Q. Role Of Tlr4/Nf-κb In Damage To Intestinal Mucosa Barrier Function And Bacterial Translocation In Rats Exposed To Hypoxia. PloS One. (2012) 7:e46291. doi: 10.1371/journal.pone.0046291
34. Di Sabatino A, Santacroce G, Rossi CM, Broglio G, Lenti MV. Role Of Mucosal Immunity And Epithelial-Vascular Barrier In Modulating Gut Homeostasis. Intern Emerg Med. (2023) 18:1635–46. doi: 10.1007/s11739-023-03329-1
35. Long C, Zhou X, Xia F, Zhou B. Intestinal Barrier Dysfunction And Gut Microbiota In Non-Alcoholic Fatty Liver Disease: Assessment, Mechanisms, And Therapeutic Considerations. Biol (Basel). (2024) 13:243. doi: 10.3390/biology13040243
36. Li Y, Jin L, Chen T. The Effects Of Secretory Iga In The Mucosal Immune System. BioMed Res Int. (2020) 2020:2032057. doi: 10.1155/2020/2032057
37. Lin S, Mukherjee S, Li J, Hou W, Pan C, Liu J. Mucosal Immunity-Mediated Modulation Of The Gut Microbiome By Oral Delivery Of Probiotics Into Peyer’s Patches. Sci Adv. (2021) 7:eabf0677. doi: 10.1126/sciadv.abf0677
38. Ren Z, Cui G, Lu H, Chen X, Jiang J, Liu H, et al. Liver Ischemic Preconditioning (Ipc) Improves Intestinal Microbiota Following Liver Transplantation In Rats Through 16s Rdna-Based Analysis Of Microbial Structure Shift. PloS One. (2013) 8:e75950. doi: 10.1371/journal.pone.0075950
39. Zhang XY, Liu ZM, Zhang HF, Li YS, Wen SH, Shen JT, et al. Tgf-β1 Improves Mucosal Iga Dysfunction And Dysbiosis Following Intestinal Ischaemia-Reperfusion In Mice. J Cell Mol Med. (2016) 20:1014–23. doi: 10.1111/jcmm.2016.20.issue-6
40. Barathan M, Ng SL, Lokanathan Y, Ng MH, Law JX. The Profound Influence Of Gut Microbiome And Extracellular Vesicles On Animal Health And Disease. . Int J Mol Sci. (2024) 25:4024. doi: 10.3390/ijms25074024
41. Huang P, Cao J, Chen J, Luo Y, Gong X, Wu C, et al. Crosstalk Between Gut Microbiota And Renal Ischemia/Reperfusion Injury. Front Cell Infect Microbiol. (2022) 12:1015825. doi: 10.3389/fcimb.2022.1015825
42. Assimakopoulos SF, Bhagani S, Aggeletopoulou I, Tsounis EP, Tsochatzis EA. The Role Of Gut Barrier Dysfunction In Postoperative Complications In Liver Transplantation: Pathophysiological And Therapeutic Considerations. Infection. (2024) 52:723–36. doi: 10.1007/s15010-024-02182-4
43. Nastos C, Kalimeris K, Papoutsidakis N, Tasoulis MK, Lykoudis PM, Theodoraki K , et al. Global Consequences Of Liver Ischemia/Reperfusion Injury. Oxid Med Cell Longev. (2014) 2014:906965. doi: 10.1155/2014/906965
44. Yang J, Kim CJ, Go YS, Lee HY, Kim MG, Oh SW, et al. Intestinal Microbiota Control Acute Kidney Injury Severity By Immune Modulation. Kidney Int. (2020) 98:932–46. doi: 10.1016/j.kint.2020.04.048
45. Wei L, Singh R, Ro S, Ghoshal UC. Gut Microbiota Dysbiosis In Functional Gastrointestinal Disorders: Underpinning The Symptoms And Pathophysiology. Jgh Open. (2021) 5:976–87. doi: 10.1002/jgh3.12528
46. Schofield ZV, Wu M, Hansbro PM, Cooper MA, Woodruff TM. Acetate Protects Against Intestinal Ischemia-Reperfusion Injury Independent Of Its Cognate Free Fatty Acid 2 Receptor. FASEB J. (2020) 34:10418–30. doi: 10.1096/fj.202000960R
47. Adelman MW, Connor AA, Hsu E, Saharia A, Mobley CM, Victor DW 3rd, et al. Bloodstream Infections After Solid Organ Transplantation: Clinical Epidemiology And Antimicrobial Resistance (2016-21). Jac Antimicrob Resist. (2024) 6:Dlad158. doi: 10.1093/jacamr/dlad158
48. Haak BW, Littmann ER, Chaubard JL, Pickard AJ, Fontana E, Adhi F, et al. Impact Of Gut Colonization With Butyrate-Producing Microbiota On Respiratory Viral Infection Following Allo-Hct. Blood. (2018) 131:2978–86. doi: 10.1182/blood-2018-01-828996
49. Lee JR, Huang J, Magruder M, Zhang LT, Gong C, Sholi AN, et al. Butyrate-Producing Gut Bacteria And Viral Infections In Kidney Transplant Recipients: A Pilot Study. Transpl Infect Dis. (2019) 21:E13180. doi: 10.1111/tid.13180
50. Kato K, Nagao M, Miyamoto K, Oka K, Takahashi M, Yamamoto M, et al. Longitudinal Analysis Of The Intestinal Microbiota In Liver Transplantation. Transplant Direct. (2017) 3:E144. doi: 10.1097/TXD.0000000000000661
51. Annavajhala MK, Gomez-Simmonds A, Macesic N, Sullivan SB, Kress A, Khan SD, et al. Colonizing Multidrug-Resistant Bacteria And The Longitudinal Evolution Of The Intestinal Microbiome After Liver Transplantation. Nat Commun. (2019) 10:4715. doi: 10.1038/s41467-019-12633-4
52. Ye J, Yao J, He F, Sun J, Zhao Z, Wang Y. Regulation Of Gut Microbiota: A Novel Pretreatment For Complications In Patients Who Have Undergone Kidney Transplantation. Front Cell Infect Microbiol. (2023) 13:1169500. doi: 10.3389/fcimb.2023.1169500
53. Nanayakkara D, Nanda N. Clostridium Difficile Infection In Solid Organ Transplant Recipients. Curr Opin Organ Transplant. (2017) 22:314–9. doi: 10.1097/MOT.0000000000000430
54. Lan X, Zhang J, Ren S, Wang H, Shao B, Qin Y, et al. Oxymatrine Combined With Rapamycin To Attenuate Acute Cardiac Allograft Rejection. Heliyon. (2024) 10:E29448. doi: 10.1016/j.heliyon.2024.e29448
55. Lei YM, Chen L, Wang Y, Stefka AT, Molinero LL, Theriault B, et al. The Composition Of The Microbiota Modulates Allograft Rejection. J Clin Invest. (2016) 126:2736–44. doi: 10.1172/JCI85295
56. Wang J, Li X, Wu X, Wang Z, Zhang C, Cao G, et al. Gut Microbiota Alterations Associated With Antibody-Mediated Rejection After Kidney Transplantation. Appl Microbiol Biotechnol. (2021) 105:2473–84. doi: 10.1007/s00253-020-11069-x
57. Gavzy SJ, Kensiski A, Saxena V, Lakhan R, Hittle L, Wu L, et al. Early Immunomodulatory Program Triggered By Protolerogenic Bifidobacterium Pseudolongum Drives Cardiac Transplant Outcomes. Transplantation. (2024) 108:E91–E105. doi: 10.1097/TP.0000000000004939
58. Wu J, Li C, Gao P, Zhang C, Zhang P, Zhang L, et al. Intestinal Microbiota Links To Allograft Stability After Lung Transplantation: A Prospective Cohort Study. Signal Transduct Target Ther. (2023) 8:326. doi: 10.1038/s41392-023-01515-3
59. Oh PL, Martínez I, Sun Y, Walter J, Peterson DA, Mercer DF. Characterization Of The Ileal Microbiota In Rejecting And Nonrejecting Recipients Of Small Bowel Transplants. Am J Transplant. (2012) 12:753–62. doi: 10.1111/j.1600-6143.2011.03860.x
60. Duong N, Bajaj JS. The Impact Of The Gut Microbiome On Liver Transplantation. Curr Opin Organ Transplant. (2021) 26:587–94. doi: 10.1097/MOT.0000000000000930
61. Peled JU, Gomes A, Devlin SM, Littmann ER, Taur Y, Sung AD, et al. Microbiota As Predictor Of Mortality In Allogeneic Hematopoietic-Cell Transplantation. N Engl J Med. (2020) 382:822–34. doi: 10.1056/NEJMoa1900623
62. Shono Y, Van Den Brink M. Gut Microbiota Injury In Allogeneic Haematopoietic Stem Cell Transplantation. Nat Rev Cancer. (2018) 18:283–95. doi: 10.1038/nrc.2018.10
63. Swarte JC, Li Y, Hu S, Björk JR, Gacesa R, Vich Vila A, et al. Gut Microbiome Dysbiosis Is Associated With Increased Mortality After Solid Organ Transplantation. Sci Transl Med. (2022) 14:eabn7566. doi: 10.1126/scitranslmed.abn7566
64. Willner DL, Hugenholtz P, Yerkovich ST, Tan ME, Daly JN, Lachner N, et al. Reestablishment Of Recipient-Associated Microbiota In The Lung Allograft Is Linked To Reduced Risk Of Bronchiolitis Obliterans Syndrome. Am J Respir Crit Care Med. (2013) 187:640–7. doi: 10.1164/rccm.201209-1680OC
65. Mcintosh CM, Chen L, Shaiber A, Eren AM, Alegre ML. Gut Microbes Contribute To Variation In Solid Organ Transplant Outcomes In Mice. Microbiome. (2018) 6:96. doi: 10.1186/s40168-018-0474-8
66. Kim SG, Becattini S, Moody TU, Shliaha PV, Littmann ER, Seok R, et al. Microbiota-Derived Lantibiotic Restores Resistance Against Vancomycin-Resistant Enterococcus. Nature. (2019) 572:665–9. doi: 10.1038/s41586-019-1501-z
67. Rayes N, Seehofer D, Theruvath T, Schiller RA, Langrehr JM, Jonas S, et al. Supply Of Pre- And Probiotics Reduces Bacterial Infection Rates After Liver Transplantation–A Randomized, Double-Blind Trial. Am J Transplant. (2005) 5:125–30. doi: 10.1111/j.1600-6143.2004.00649.x
68. Grąt M, Wronka KM, Lewandowski Z, Grąt K, Krasnodębski M, Stypułkowski J, et al. Effects Of Continuous Use Of Probiotics Before Liver Transplantation: A Randomized, Double-Blind, Placebo-Controlled Trial. Clin Nutr. (2017) 36:1530–9. doi: 10.1016/j.clnu.2017.04.021
69. Chan S, Hawley CM, Pascoe EM, Cao C, Campbell SB, Campbell KL, et al. Prebiotic Supplementation In Kidney Transplant Recipients For Preventing Infections And Gastrointestinal Upset: A Randomized Controlled Feasibility Study. J Ren Nutr. (2022) 32:718–25. doi: 10.1053/j.jrn.2022.02.006
70. Cheng YW, Phelps E, Ganapini V, Khan N, Ouyang F, Xu H, et al. Fecal Microbiota Transplantation For The Treatment Of Recurrent And Severe Clostridium Difficile Infection In Solid Organ Transplant Recipients: A Multicenter Experience. Am J Transplant. (2019) 19:501–11. doi: 10.1111/ajt.15058
71. Stripling J, Kumar R, Baddley JW, Nellore A, Dixon P, Howard D, et al. Loss Of Vancomycin-Resistant Enterococcus Fecal Dominance In An Organ Transplant Patient With Clostridium Difficile Colitis After Fecal Microbiota Transplant. Open Forum Infect Dis. (2015) 2:Ofv078. doi: 10.1093/ofid/ofv078
72. Aslam S, Courtwright AM, Koval C, Lehman SM, Morales S, Furr CL, et al. Early Clinical Experience Of Bacteriophage Therapy In 3 Lung Transplant Recipients. Am J Transplant. (2019) 19:2631–9. doi: 10.1111/ajt.15503
73. Hill C, Guarner F, Reid G, Gibson GR, Merenstein DJ, Pot B, et al. Expert Consensus Document. The International Scientific Association For Probiotics And Prebiotics Consensus Statement On The Scope And Appropriate Use Of The Term Probiotic. Nat Rev Gastroenterol Hepatol. (2014) 11:506–14. doi: 10.1038/nrgastro.2014.66
74. Doycheva I, Leise MD, Watt KD. The Intestinal Microbiome And The Liver Transplant Recipient: What We Know And What We Need To Know. Transplantation. (2016) 100:61–8. doi: 10.1097/TP.0000000000001008
75. Dudzicz S, Kujawa-Szewieczek A, Kwiecień K, Więcek A, Adamczak M. Lactobacillus Plantarum 299v Reduces The Incidence Of Clostridium Difficile Infection In Nephrology And Transplantation Ward-Results Of One Year Extended Study. Nutrients. (2018) 10:1574. doi: 10.3390/nu10111574
76. Wang JW, Kuo CH, Kuo FC, Wang YK, Hsu WH, Yu FJ, et al. Fecal Microbiota Transplantation: Review And Update. J Formos Med Assoc. (2019) 118 Suppl 1:S23–31. doi: 10.1016/j.jfma.2018.08.011
77. Neemann K, Eichele DD, Smith PW, Bociek R, Akhtari M, Freifeld A. Fecal Microbiota Transplantation For Fulminant Clostridium Difficile Infection In An Allogeneic Stem Cell Transplant Patient. Transpl Infect Dis. (2012) 14:E161–165. doi: 10.1111/tid.2012.14.issue-6
78. Schneider KM, Wirtz TH, Kroy D, Albers S, Neumann UP, Strowig T, et al. Successful Fecal Microbiota Transplantation In A Patient With Severe Complicated Clostridium Difficile Infection After Liver Transplantation. Case Rep Gastroenterol. (2018) 12:76–84. doi: 10.1159/000481937
Keywords: organ transplantation, ischemia-reperfusion injury, intestinal microecology, intestinal barrier, microbial therapy
Citation: Lian Y-q, Li P-f, Guo Y, Tao Y-l, Liu Y-n, Liang Z-y and Zhu S-f (2024) Interaction between ischemia-reperfusion injury and intestinal microecology in organ transplantation and its therapeutic prospects. Front. Immunol. 15:1495394. doi: 10.3389/fimmu.2024.1495394
Received: 12 September 2024; Accepted: 15 November 2024;
Published: 06 December 2024.
Edited by:
Shao-wei Li, Taizhou Hospital Affiliated to Wenzhou Medical University, ChinaReviewed by:
Weitao Que, Shanghai General Hospital, ChinaJohanna Wagner, University Hospital Wuerzburg, Germany
Copyright © 2024 Lian, Li, Guo, Tao, Liu, Liang and Zhu. This is an open-access article distributed under the terms of the Creative Commons Attribution License (CC BY). The use, distribution or reproduction in other forums is permitted, provided the original author(s) and the copyright owner(s) are credited and that the original publication in this journal is cited, in accordance with accepted academic practice. No use, distribution or reproduction is permitted which does not comply with these terms.
*Correspondence: Shu-fen Zhu, NjE1MzU0NTM0QHFxLmNvbQ==