- 1Department of Medicine, McMaster University, Hamilton, ON, Canada
- 2McMaster Immunology Research Center, Michael G. DeGroote Center for Learning and Discovery, McMaster University, Hamilton, ON, Canada
Introduction: Clinically, a dysbiotic vaginal microbiota (VMB) colonized with anaerobic species such as Gardnerella vaginalis has been linked to increased susceptibility to viral sexually transmitted infections (STIs) such as Herpes Simplex Virus Type 2 (HSV-2). The mechanism is poorly understood due to the lack of small animal models.
Methods: Mice were inoculated with 107 CFU of the eubiotic bacteria Lactobacillus crispatus, the dysbiotic bacteria G. vaginalis, or PBS as a negative control every 48 h for ten days. On day ten, mice were inoculated with 105 PFU WT HSV-2 333 and survival, pathology, and viral titers were assessed. To elucidate changes in the vaginal microenvironment following bacterial inoculations, vaginal tissue and washes were collected following ten days of inoculations. To assess barrier integrity, tissue was fixed and stained for the barrier protein Desmoglein-1 (DSG-1). To evaluate the immune microenvironment, tissue was processed for flow cytometry to examine tissue-resident T cells and cytokine production by T cells. Vaginal washes were used for multiplex cytokine/chemokine analysis.
Results: G. vaginalis inoculated mice infected with HSV-2 had significantly decreased survival rates, increased pathology, and higher viral titers than PBS and L. crispatus inoculated mice. The vaginal epithelium of G. vaginalis inoculated mice showed decreased DSG-1 staining compared to other groups, indicating compromised barrier function. Decreased total numbers of CD4+ and CD8+ T cells expressing activated mucosal immune markers CD44, CD69, and CD103 were observed in the vaginal tract of G. vaginalis inoculated mice. They also showed increased proportions of T cells expressing inflammatory cytokines TNF-α and IFN-γ, while L. crispatus inoculated mice had increased proportions and absolute counts of T cells expressing the regulatory cytokine IL-10. In the multiplex assay, vaginal washes from G. vaginalis mice had increased inflammatory cytokines and chemokines compared to L. crispatus and PBS groups.
Discussion: These results suggest G. vaginalis inoculation may be increasing HSV-2 infection by disrupting the epithelial barrier, decreasing protective immune responses and increasing tissue inflammation in the vaginal tract.
Introduction
The female genital tract is colonized by an endogenous collection of microbes, termed the vaginal microbiota (VMB), which plays a critical role in female reproductive health (1). Studies have characterized a eubiotic VMB to be composed of Lactobacillus species, with Lactobacillus crispatus as the dominant species correlated with positive reproductive health outcomes and decreased risk of sexually transmitted infections (STIs) (2–4). A dysbiotic VMB is distinguished by a diverse community structure comprised of anaerobic bacteria such as Gardnerella vaginalis, a bacterial species that is commonly seen in individuals with the clinical condition bacterial vaginosis (BV) (2). A diverse VMB composed of various anaerobes is characteristic of dysbiosis, but as G. vaginalis is the most common bacteria seen in BV patients (5), it is the bacteria that is focused on in this study. Crucially, women with BV are at an increased risk of acquiring STIs. It is essential to understand the underlying mechanisms to design prevention strategies for at-risk populations.
Herpes Simplex Virus Type 2 (HSV-2) is a viral STI that poses a massive global health burden (6). In 2016, an estimated 491.5 million (13%) people aged 15–49 years were infected with HSV-2, with the highest number of new infections in adolescent women (6, 7). Heterosexual transmission is more efficient from men to women, where one study found HSV-2 is transmitted to women almost twice as often as to men (8). While studies have shown that behavioural factors such as the use of progestin-based contraceptives (9), having multiple sexual partners (10), and douching (11) can enhance HSV-2 risk, it is important to study biological factors contributing to why women are more susceptible to prevent the spread of new infections to vulnerable populations.
As previously mentioned, the distinct VMB of the female reproductive tract has been shown to play a role in increased susceptibility to STIs in women. There is a great deal of clinical and epidemiological evidence to support this claim (12–14). One group showed that a high Nugent score, typically seen in BV, is associated with a 2-fold increased risk of HSV-2 infection (12). In another study, the enrichment of dysbiotic species G. vaginalis and Lactobacillus iners was associated with an increased likelihood of HSV-2 in both male and female sexual partners (13). This same group also found that there are increased HSV-2 lesions and ulcers in BV-positive women (14). Overall, this clinical evidence suggests a dysbiotic VMB is linked to increased susceptibility to HSV-2.
The exact physiological mechanisms of how eubiotic and dysbiotic VMB alter the vaginal microenvironment are unclear. An L. crispatus dominant VMB may decrease susceptibility to STIs by decreasing vaginal pH through the secretion of hydrogen peroxide and acid metabolites such as lactic acid (2, 15). G. vaginalis may increase susceptibility to HSV-2 by reducing the mucus barrier (16), decreasing epithelial barrier integrity (17), and reducing immune function in the vaginal mucosa (18). However, while in vitro and clinical studies can provide correlative data, it is difficult to elucidate cause-effect mechanisms due to the lack of human VMB-associated mouse models. Recent in vivo models that harbour eubiotic and dysbiotic VMB provide a physiological system to experimentally elucidate how VMB composition may lead to changes in the vaginal mucosa, and the outcome this has on STI infections (19, 20). In the context of Human Immunodeficiency Virus (HIV), dysbiotic bacteria were found to increase the number of activated CD4+ cells in the female genital tract of germ-free mice, which are the primary target cells of HIV (21). Similar correlations to explain the increased susceptibility to HSV-2 in the context of a dysbiotic VMB have not been well investigated in vivo.
We have recently published a study describing a VMB-associated mouse model temporarily colonized with the eubiotic bacteria L. crispatus and the dysbiotic bacteria G. vaginalis (19). In this study, we found estrogen aided in the successful colonization of mice with eubiotic and dysbiotic species, potentially through a glycogen-mediated mechanism (19). This model was utilized in the current study to assess the role of the VMB on HSV-2 susceptibility in vivo. We found dysbiotic G. vaginalis inoculation to decrease survival rates and increase HSV-2 pathology and viral titers compared to the eubiotic bacteria L. crispatus and the PBS-negative control groups, validating that our model recapitulates clinical observations. As previously mentioned, two postulated mechanisms as to how G. vaginalis may increase susceptibility to HSV-2 are by disrupting the epithelial barrier and by altering the immune microenvironment in the vaginal tract. In this study, G. vaginalis decreased the expression of Desmoglein-1 (DSG-1), a junctional protein that binds vaginal epithelial cells together (22, 23). This indicates a disruption in the epithelium, which may be associated with the increased susceptibility to HSV-2. G. vaginalis was also associated with decreased mucosal resident T cells and an inflammatory signature, which can also be linked to less protective immune responses and increased pathology in the vaginal mucosa, leading to lower survival rates in G. vaginalis inoculated mice. Using our novel VMB-associated mouse models, this study demonstrates for the first time cause-effect mechanisms as to how a dysbiotic VMB may increase susceptibility to HSV-2.
Materials and methods
Mice
8-10-week-old female conventional C57BL/6 mice were obtained from Charles River Laboratories (Saint-Constant, QC, Canada). All mice were maintained under specific pathogen-free and standard temperature-controlled conditions that followed a 12-hour light/dark cycle at the Central Animal Facility at McMaster University. Mice were allowed one week after arrival to acclimate prior to experimental use. All mouse studies performed were approved by and complied with the Animal Research Ethics Board (AREB) at McMaster University (AUP 22-03-07).
Colonization of mice
Bacteria were prepared as per previously published protocols (19). Briefly, Lactobacillus crispatus SJ-3C-US was grown in American Type Culture Collection (ATCC) medium 416 (Lactobacillus MRS broth) at 37°C in anaerobic conditions for 24 hours. Gardnerella vaginalis ATCC 14019 was grown in ATCC medium 1685 (NYC III medium) at 37°C in anaerobic conditions for 24 hours. Bacteria were then washed once with Phosphate-buffered saline (PBS) and resuspended in 25 µL PBS at 107 colony-forming units (CFU) per mouse. To colonize the mice, mice were inoculated intravaginally with 25 µL of the bacteria and then held facedown for one minute to allow for the bacteria to persist in the vaginal canal. To evaluate colonization, vaginal washes were collected from the mice and quantitative plating assays were performed as described previously using the Miles and Misra technique (19, 24). To maintain consistent colonization in the mice, L. crispatus and G. vaginalis were inoculated every 48 hours for ten days.
Estrus cycle staging
10 µL of vaginal washes were pipetted onto a glass slide and viewed under a microscope. The cells were observed and compared to images of vaginal washes from mice under different stages of the estrus cycle (25). Briefly, vaginal washes from mice in the estrus stage of their cycle were primarily composed of keratinized epithelial cells and vaginal washes from mice in the diestrus stage were composed primarily of leukocytes.
HSV-2 infection in bacteria colonized mice
Mice were colonized with bacteria for ten days before infection. After bacterial administration, if the mice were in diestrus, they were anesthetized with ketamine (150 mg ketamine/kg) and xylazine (10 mg of xylazine/kg). Mice were intravaginally inoculated with 105 PFU of wildtype HSV-2 333 and left on their backs on a heating pad for approximately 45 minutes to one hour to allow the virus to infect. Vaginal washes were collected daily for seven days post-infection, and genital pathology was monitored. Mice were euthanized when they reached a pathology of 4 or 5 (see next section).
Genital pathology scoring
As per endpoint monitoring protocols approved by AREB, mice were monitored every day for seven days post-infection and the pathology in the genital area was recorded following a 5-point scale as previously reported (26). The scale is no infection (0), slight genital redness and inflammation (1), genital swelling and redness (2), genital and surrounding area swelling, redness and hair loss (3), genital ulceration with hair loss (4), and ulceration and hair loss in surrounding areas followed by hind limb paralysis (5). Mice were euthanized when they reached high pathology scores of 4 or 5.
Collection of vaginal washes
30 μL of PBS was pipetted in and out of the vagina seven to eight times. A total of 50-60 μL/mouse was collected by repeating this twice. Vaginal washes were collected for up to seven days post HSV-2 infection and were stored at −80°C until required.
Vero plaque assay
Vero cells were seeded in a 12-well plate and left for 24 h to grow to confluency in α-Minimum Essential Medium (α-MEM), supplemented with 5% fetal bovine serum (FBS), 1% penicillin-streptomycin, 1% L-glutamate and 1% HEPES. Vaginal washes were diluted from 10-1 to 10-6 in serum-free α-MEM and added to the Vero cells. Plates were incubated for 2 h and were rocked every 15 minutes to allow the virus to distribute evenly when infecting cells and to prevent the cells from drying out. After the 2 h incubation, α-MEM with FBS was added on top of the monolayers to stop new viral adsorption. The plates were placed in a 37°C incubator for 48 h. After 48 h, the media was aspirated out of each well and the cells were fixed and stained with crystal violet for approximately 15-20 minutes. The plates were then rinsed in water and left to dry overnight. Plaques were counted in the well that had 30-300 plaques under a light microscope and the plaque forming units (PFU) per mL was calculated (27).
Immunohistochemistry of vaginal tissue
Mouse vaginal tissue was collected, placed in cassettes, and fixed in methacarn (60% methanol, 30% chloroform and 10% glacial acetic acid) for 72 h. Cassettes were transferred to 70% ethanol and samples were taken to McMaster Immunology Research Center (MIRC) Histology Core Facility for processing. Briefly, the tissue was embedded in paraffin, and slides were cut and mounted on microscope slides. Slides were then de-paraffinized and stained with a rabbit polyclonal Desmoglein-1 (DSG-1) antibody (Life Technologies, Cat #BS-6725R) as the primary antibody and a goat anti-rabbit IgG Alexa Fluor™ 488 secondary antibody (Invitrogen, Cat #A-11008) with a DAPI counterstain. An IgG isotype control (Santa Cruz Biotechnology, Cat #sc-2027) and a diluent negative control were also included. All samples were imaged on an inverted confocal laser-scanning microscope (Nikon Eclipse Ti2) at 20X magnification. For each experiment, confocal microscope settings for image acquisition and processing were identical between controls and bacteria-inoculated tissue samples. Three randomized images per sample were obtained for analysis using ImageJ software.
Tissue processing
After inoculation with VMB species for 10 days, mice were euthanized by cervical dislocation and the vaginal tissue, spleen and iliac lymph nodes (iLN) were collected in cold Roswell Park Memorial Institute (RPMI) media (Invitrogen, Burlington, Canada). Vaginal tissue was cut open with scissors to expose the interior and then cut into smaller pieces. Collagenase A (Cedarlane, Cat #11088793001) was added to 15 mL of RPMI at a mass of 0.00157 g/mL to digest the tissue. The vaginal tissue suspension was put in 50 mL Falcon tubes and placed in a 225 RPM shaker at 37°C for one hour. The supernatant was filtered through a 40 μm filter and collected in a fresh 50 mL Falcon tube on ice. The tissue suspension was again mixed with collagenase and fresh media and digested for another hour. The suspension was then filtered through a 40 μm filter, where the remaining tissue was also pushed through the filter using the back of a syringe. All samples were centrifuged at 1500 RPM at 4°C for 10 minutes. The supernatant was discarded, and the pellet was resuspended in 200 μL of RPMI media. For spleen and iLN analysis, 40 μm filters were placed in a 6-well plate and 6 mL of RPMI media was added. The spleen or iLN was added to the filter and the back of a syringe was used to break apart the tissue. The spleen or iLN suspension was collected in a 15 mL Falcon tube and the well was washed with another 6 mL media and added to the Falcon tube. The samples were centrifuged for 5 minutes at 1500 RPMs at 4°C. The iLN pellets were resuspended in 500 μL of RPMI. Only the spleen samples were further treated with 2mL Ammonium-Chloride-Potassium lysis buffer for 4 minutes at room temperature to lyse red blood cells. Samples were washed with 10 mL PBS and placed into the centrifuge for 5 minutes at 1500 RPMs at 4°C to obtain a pellet of cells. The spleen pellets were resuspended in 6 mL of RPMI media. Cells from all samples were then counted using a hemocytometer.
Flow cytometric analysis
Staining was done on 1x106 iLN and spleen cells and all cells from the vaginal tissue due to lower yields in 200 μL volumes. Cells were stimulated with a cell stimulation cocktail of ionomycin, PMA, brefeldin A, and monensin (eBioscience™ Cell Stimulation Cocktail, Thermo Fisher Scientific, Cat #00-4975-03) overnight for 16 h as recommended by the manufacturer’s protocol. Samples were incubated with Fc block (rat anti-mouse CD16/32, BD Biosciences, Cat #553142) for 20 minutes to decrease nonspecific Fc receptor binding. Cells were then stained for cell surface markers at 4°C using the following antibodies at 1:100 dilutions for vaginal samples and 1:200 dilutions for spleen and lymph node samples: CD3 PE-CF594 (Invitrogen), CD4 PerCP Cy5.5 (Invitrogen), CD8 BV786 (Invitrogen), CD44 AF700 (BD Bioscience), CD69 PE-Cy7 (Invitrogen), and CD103 PE (Invitrogen). Cells were incubated with these antibodies for 30 minutes and then washed with PBS. Cells were then stained with the Fixable Viability Dye eFluor™ 780 (Invitrogen) at 1:1000 for 20 minutes at 4°C. To prepare for intracellular staining, cells were permeabilized and fixed using the BD Cytofix/Cytoperm™ fixation/permeabilization kit (BD Biosciences, Cat#555028) according to the manufacturer’s protocol. Cells were then stained for intracellular markers at 4°C using the following antibodies at 1:100 dilutions for vaginal samples and 1:200 dilutions for spleen and lymph node samples: IFN-γ BV421 (Invitrogen), TNF-α FITC (Invitrogen), and IL-10 BV510 (BD Biosciences). The validity of staining was verified by fluorescence minus one (FMO) control. Data were collected by flow cytometric analysis using a Cytoflex flow cytometer system (Beckman Coulter Life Sciences, Indianapolis, USA). 50 μL of cell suspensions for iLN and spleen samples and the entire 200 μL was run for vaginal tissue samples. Results were analyzed using FlowJo software (Tree Star, Ashland, USA). For the vaginal tissue, the absolute count was reported as # cells/VAG (vaginal tract) and was calculated as the direct count reported by the flow cytometer, since the entire vaginal tissue was stained and run on the flow cytometer. For the iLN and the spleen, the absolute count was reported as # cells/mL and was calculated by dividing the count from the flow cytometer by 0.05 mL to get the per mL cell count (the volume of cell suspension that was run on the machine).
Multiplex cytokine and chemokine assay
Vaginal washes were collected from mice ten days after inoculation with bacteria. Washes were analyzed for cytokines and chemokines using the 31-Plex Mouse Cytokine/Chemokine Discovery Luminex Assay from Eve Technologies (Calgary, Canada), as per the manufacturer’s protocol.
Statistical analysis
All statistical analysis was done using GraphPad Prism version 9.4.1 (GraphPad Software, San Diego, USA). Two-way ANOVA with Tukey’s multiple comparisons was used to determine statistical significance for quantitative plating assays and plaque assays. HSV-2 survival data was analyzed using a Log-rank (Mantel-Cox) test. A one-way ANOVA with Tukey’s multiple comparisons was used to analyze mean fluorescence intensity from histology, all flow cytometry experiments, and multiplex experiments.
Results
Mice inoculated every 48 h with L. crispatus and G. vaginalis maintained consistent colonization
To elucidate the cause-effect changes eubiotic and dysbiotic bacteria have on the vaginal microenvironment and susceptibility to HSV-2, a small animal model that can consistently be colonized with bacteria for a short period was required. In a previous study, we reported that L. crispatus and G. vaginalis were able to persist in the mouse vaginal tract for on average 2.6 and 1.75 days, respectively (19). Since these values are close to 48 h and 72 h, these inoculation intervals were used to determine if consistent colonization could be maintained in the model. Mice were inoculated twice 48 h or 72 h apart with the eubiotic bacteria L. crispatus, the dysbiotic bacteria G. vaginalis, or PBS as a negative control. L. crispatus and G. vaginalis loads were evaluated with quantitative plating assays, a method which we have previously validated (19).
When mice were inoculated with PBS every 48 h (Figure 1A) or every 72 h (Figure 1B), no exogenous bacteria were detected, as expected. All mice inoculated with exogenous bacteria L. crispatus or G. vaginalis had detectable bacterial counts in the quantitative plating assays, indicating successful colonization (Figures 1C–E). Mice inoculated with L. crispatus every 48h had a high number of bacteria between the first and second inoculation, with none of the mice showing a period with low exogenous bacterial load, indicating consistent colonization (Figure 1C). In mice inoculated with G. vaginalis every 48 h, all except one showed a high exogenous bacterial count 24 h after the first inoculation (Figure 1E). 3/5 successfully colonized mice in this group showed declining G. vaginalis load at 48 h post-inoculation (Figure 1E). One mouse did not show any exogenous bacterial counts after the first inoculation, indicating unsuccessful colonization, which aligns with the lower rates of successful G. vaginalis colonization we have reported before (19). When L. crispatus was inoculated every 72 h, there was notably less consistency in colonization, and three mice had a period greater than 24 h with no colonization before the second inoculation (Figure 1D). Similarly, when mice were inoculated every 72 h with G. vaginalis, of the 5/6 mice colonized after the first inoculation, 3/5 mice had a period of more than 24 h with no bacteria detected (Figure 1F). Given the increased consistency in colonization when mice were inoculated every 48 h compared to 72 h, we proceeded with an inoculation regimen of every 48 h for this study. In this study, mice needed to be colonized with bacteria for a long enough duration of time to elicit a change in the vaginal microenvironment. Therefore, we maintained colonization for ten days (five inoculations every 48 hours). When mice were inoculated for ten days following this regimen, they consistently showed high counts of exogenous bacteria indicating short-term colonization (Supplementary Figure S1).
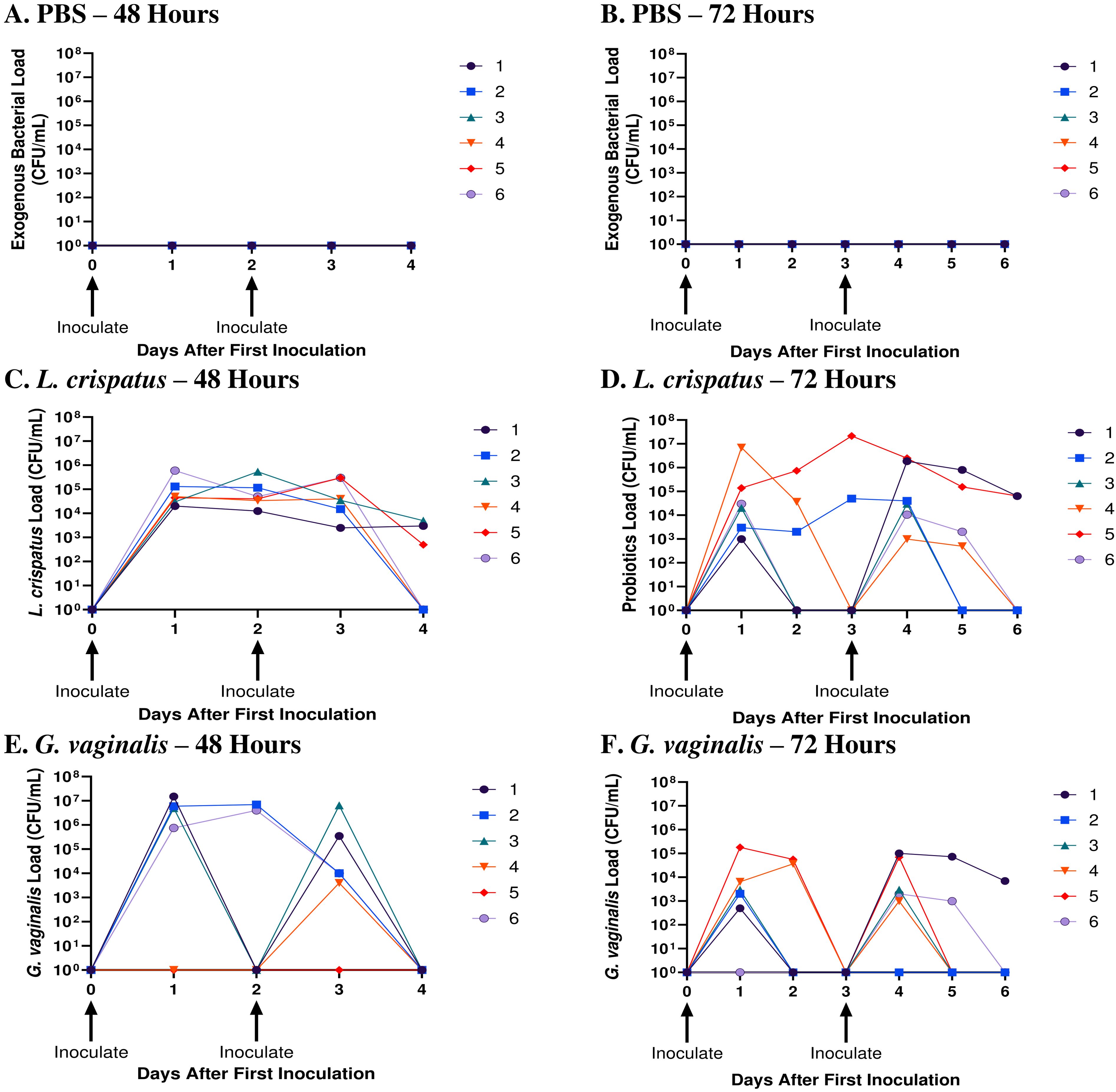
Figure 1. L. crispatus and G. vaginalis were consistently colonized when inoculated every 48 h. Female mice were inoculated twice 48 h or 72 h apart with 107 CFU L. crispatus, G. vaginalis, or PBS as a negative control. Data are from n = 6 per group from one experiment representative of 3 independent experiments with similar results. Vaginal washes were collected post-inoculation for up to 48 h or 72 h after the second inoculation and assessed using quantitative plating assays. Bacterial colonies of the inoculated species types were counted in PBS inoculated 48 h apart (A), PBS inoculated 72 h apart (B), L. crispatus inoculated 48 h apart (C), L. crispatus inoculated 72 h apart (D), G. vaginalis inoculated 48 h apart (E), and G. vaginalis inoculated 72 hours apart (F) groups. Different coloured points denote different mice. The data was analyzed using a two-way ANOVA with Tukey’s multiple comparisons, but no significance was found.
G. vaginalis inoculated mice had significantly decreased survival rates, increased pathology, and high viral titers when infected with HSV-2
After determining the best inoculation regimen in the human microbiota-associated mouse models, mice were infected with HSV-2 following colonization with L. crispatus or G. vaginalis to determine if differential outcomes were seen in these mice. Clinically, a dysbiotic VMB colonized with BV-associated bacteria such as G. vaginalis has been shown to increase susceptibility to HSV-2 in women (13). To elucidate if this could be recapitulated in our model, mice were inoculated with L. crispatus, G. vaginalis, or PBS every 48 h for ten days (five inoculations) and on day ten, infected with a sublethal dose of wildtype HSV-2 333 at 105 PFU. Administration of depot-medroxyprogesterone acetate (DMPA), a progestin-based contraceptive, before HSV-2 infection is a common practice to thin the vaginal epithelium to make mice more susceptible to HSV-2 (28), however, mice were not colonized with exogenous bacteria following DMPA administration (data not shown). Due to this, mice were staged to be in diestrus on the day of infection, the period when the epithelial barrier is thin during the normal mouse reproductive cycle (29). While not as successful as DMPA pre-treatment, this model of HSV-2 infection has previously been validated (30). The presence of bacteria following HSV-2 infection was quantitated with plating assays 24 h and five days post-infection. No bacteria were detected in the vaginal tract 24 h post-infection, indicating the virus was detrimental to vaginal bacterial growth (Supplementary Figure S2). At five days post-infection, no endogenous bacteria were detected, indicating the infection may be disrupting the ability for recolonization as well (Supplementary Figure S2).
Survival and pathology were recorded, and vaginal washes were collected up to seven days post-infection to determine viral titers. Cumulative data from three independent experiments showed that G. vaginalis inoculated mice had significantly decreased survival rates compared to L. crispatus and PBS groups (Figure 2A). All mice survived in the PBS group, 1/17 mice did not survive in the L. crispatus group, and 9/19 mice succumbed to infection in the G. vaginalis group (Figure 2A). From the cumulative data, G. vaginalis inoculated mice also had significantly higher viral titers at two days post-infection compared to other groups (Figure 2B). When looking at the cumulative pathology, G. vaginalis inoculated mice also had increased scores compared to other groups (Table 1). Individual mouse data for pathology (Figure 2C) and viral titers (Figure 2D) from one independent experiment representative of the three experiments are also included. In this experiment, 4/7 mice in the G. vaginalis group developed severe lesions and pathology, whereas no mice in the L. crispatus and PBS-negative control groups developed pathology over 7 days of monitoring (Figure 2C). When viral titers were examined in the vaginal washes, all mice in the G. vaginalis group showed high viral shedding, whereas only 3/7 mice in the PBS group and 2/6 mice in the L. crispatus group had high viral titers (Figure 2D). All measures indicated G. vaginalis was associated with increased HSV-2 infection compared to PBS and L. crispatus inoculated groups. L. crispatus did not appear to increase or decrease susceptibility to HSV-2 in vivo compared to PBS controls.
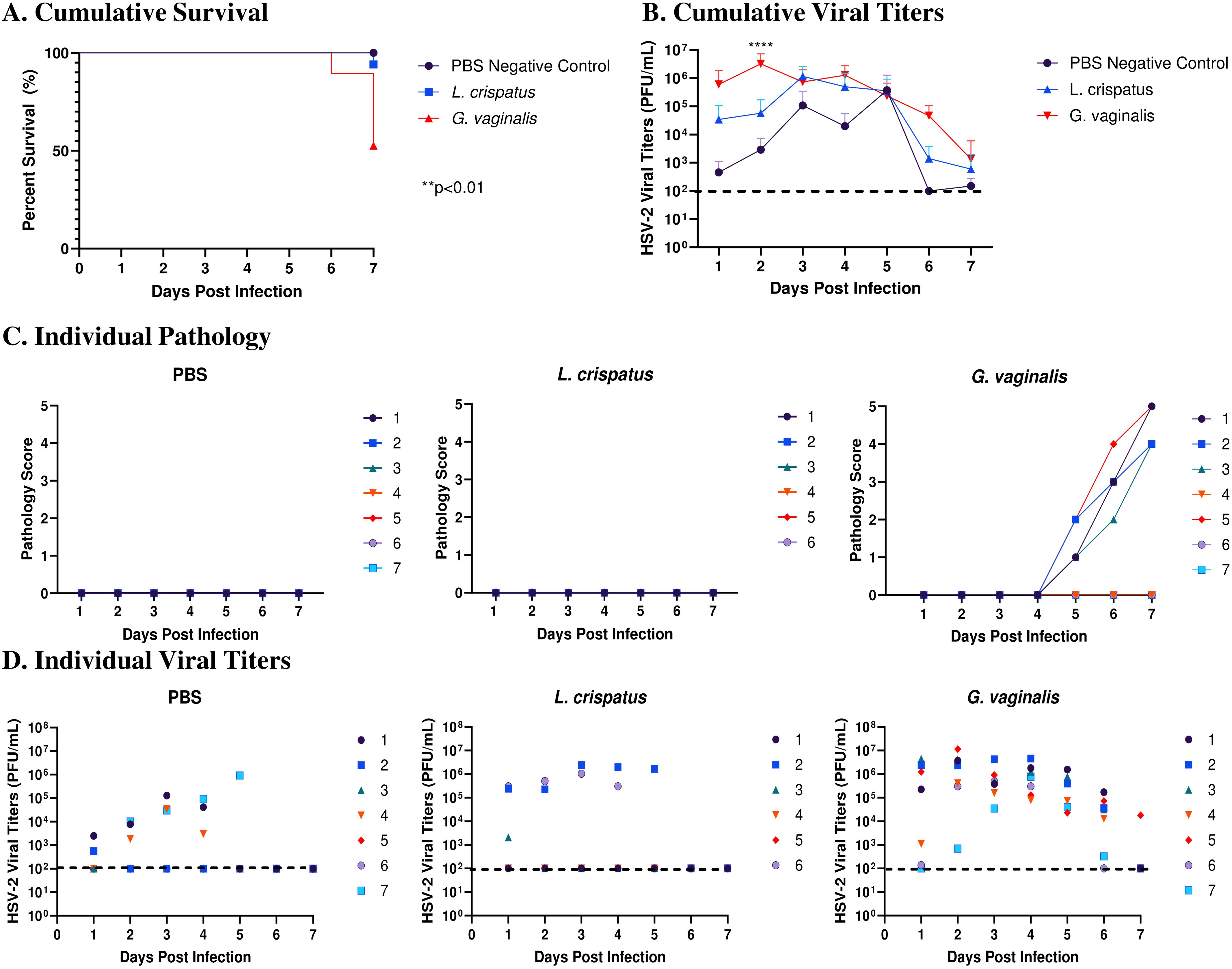
Figure 2. G. vaginalis inoculated mice showed decreased survival rates, increased pathology, and higher viral titers when infected with HSV-2 compared to L. crispatus and PBS-inoculated mice. Mice were administered 107 CFU L. crispatus, G. vaginalis, or PBS as a no-exogenous bacteria-negative control every 48 h for 10 days. 24 h after the most recent inoculation, mice in diestrus were infected with 105 PFU wildtype HSV-2 333. Data in panels (A, B) include cumulative data from three independent experiments (n=18 in the PBS group, n=17 in the L. crispatus group, and n=19 in the G. vaginalis group). Mice were euthanized when they reached a pathology score of 4 or 5, and survival was graphed in panel (A). Vaginal washes were collected up to 7 days post-infection and viral titers were determined with a Vero plaque assay (B). Vaginal pathology was recorded up to 7 days post-infection. Individual mouse data from one independent experiment representative of the three experiments was plotted for pathology (C) and viral titers (D) (n=7 per group, except L. crispatus n=6). Different coloured points denote different mice in panels (C, D). Survival data was analyzed using a Log-rank (Mantel-Cox) test (**p<0.01). Cumulative viral titers were analyzed with a two-way ANOVA with Tukey’s multiple comparisons (****p<0.0001).
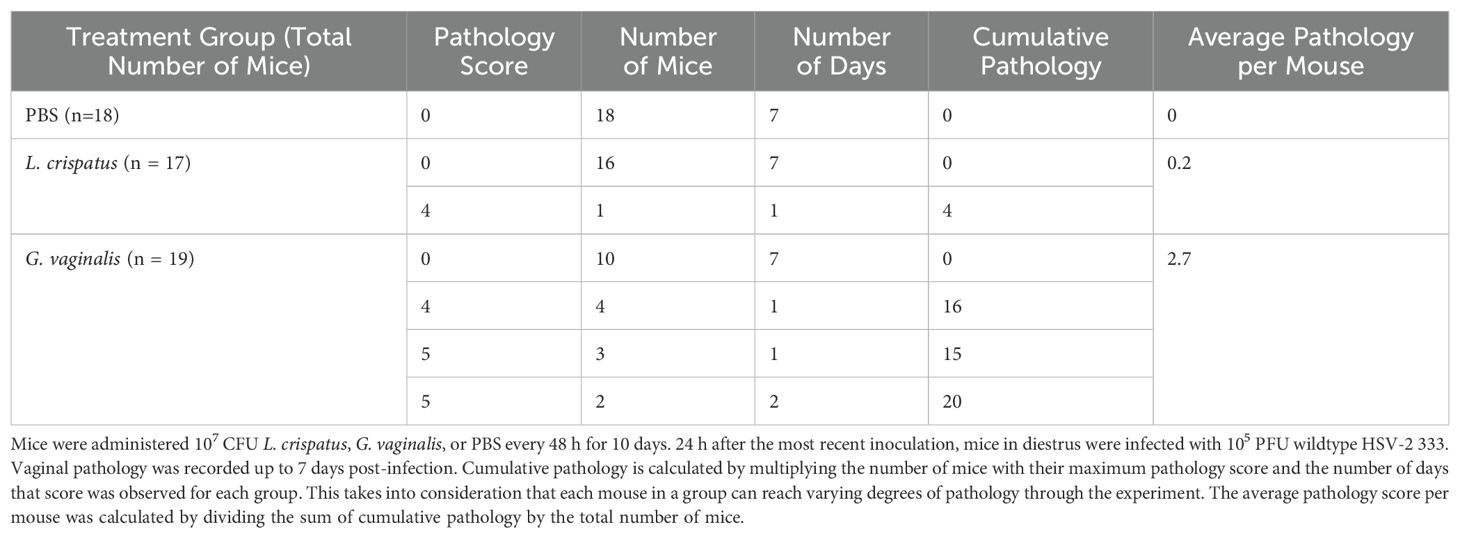
Table 1. G. vaginalis-inoculated mice showed increased cumulative pathology scores compared to L. crispatus- and PBS-inoculated mice.
G. vaginalis inoculated mice had decreased Desmoglein-1 expression compared to L. crispatus and PBS groups
After determining that G. vaginalis increased susceptibility to HSV-2 in the model, potential mechanisms as to why this is the case were explored. As the epithelium plays a vital role in susceptibility to HSV-2 (29), changes in the expression of an important epithelial barrier protein, Desmoglein-1 (DSG-1), were investigated. DSG-1 is expressed in vaginal tissue and decreased expression of this protein has previously been implicated with increased HSV-2 infection in mice (31). To determine if the VMB plays a role in DSG-1 expression, mice were inoculated every 48 h with L. crispatus, G. vaginalis, or PBS as a negative control for ten days (five inoculations). The vaginal tissue was collected on day ten and fixed and stained for DSG-1. When comparing the groups, G. vaginalis inoculated mice had significantly decreased expression of DSG-1 compared to L. crispatus and PBS groups (Figure 3). This was demonstrated by the decreased green fluorescent staining for DSG-1 in the G. vaginalis group compared to the L. crispatus and PBS groups (Figure 3A). When the mean fluorescence intensity was analyzed, there was significantly decreased expression in the G. vaginalis group compared to L. crispatus and PBS groups (Figure 3B). L. crispatus did not alter the expression of DSG-1 compared to the PBS control (Figures 3A, B).
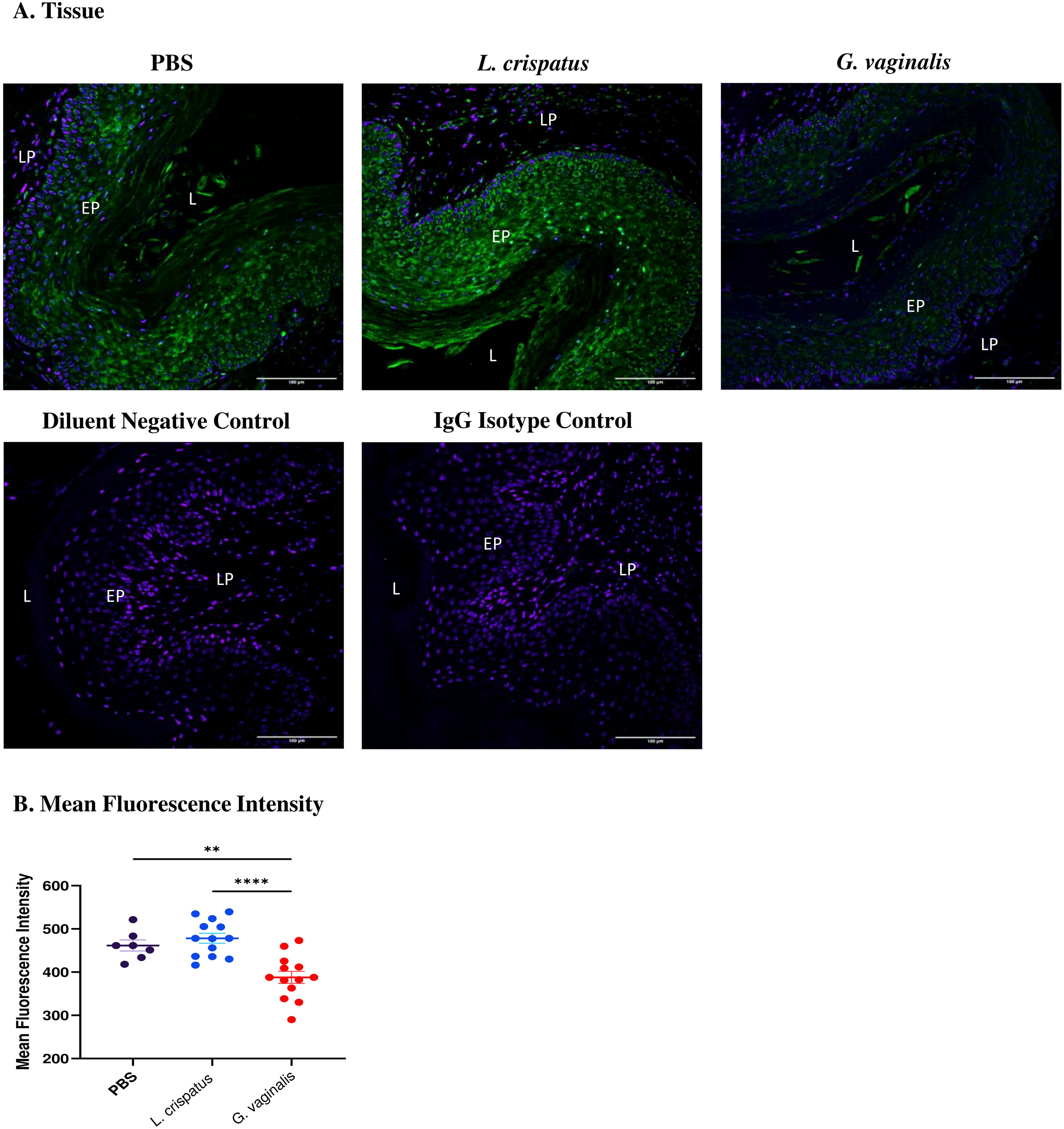
Figure 3. G. vaginalis inoculated mice had decreased Desmoglein-1 expression in the vaginal epithelium compared to L. crispatus and PBS groups. Mice were administered 107 CFU L. crispatus, G. vaginalis, or PBS as a no-exogenous bacteria-negative control every 48 h for 10 days. Vaginal tissue was collected from the mice and fixed in methacarn. The tissue was paraffin-embedded, deparaffinized, and stained for Desmoglein-1 (green) with a DAPI counterstain (blue). Images were taken using a confocal microscope at 20X magnification (A). The lumen (L), epithelium (EP), and lamina propria (LP) are indicated on all images. Mean fluorescence intensity was evaluated using three representative images per sample with ImageJ software (B). Data is representative of two independent experiments (n=13 per group, except PBS n=7). The data was analyzed using a one-way ANOVA with Tukey’s multiple comparisons (**p<0.01 and ****p<0.0001).
G. vaginalis inoculated mice had significantly decreased numbers of tissue-resident T cells compared to L. crispatus inoculated mice
To further elucidate why G. vaginalis may be increasing susceptibility to HSV-2 in vivo, changes in the immune microenvironment were evaluated through flow cytometry analysis. Mice were inoculated with L. crispatus, G. vaginalis, or PBS every 48 h for ten days (five inoculations). The vaginal tissue, spleen and iliac lymph nodes, which drain the reproductive tract, were collected from mice on day ten after inoculations and processed and stained for flow cytometry. The gating strategy is depicted in Supplementary Figure S3. No significant differences were seen in the cells from the spleen or the lymph nodes, indicating that the effect of vaginal bacterial colonization was site-specific and limited to local tissues (Supplementary Figure S4).
Since T cells have been shown to play a critical role in protection against HSV-2, our analysis was focused on these cells (32, 33). Vaginal cells were first gated on CD4+CD3+ T cells. Inoculation with L. crispatus or G. vaginalis did not significantly alter the proportions or absolute number of CD4+ T cells (Figure 4A), indicating these bacteria did not affect the overall numbers of CD4+ T cells in the vaginal mucosa. Next, the quality of T cell response in the vaginal tract following inoculation with different bacteria was evaluated by examining CD4+ T cells expressing the tissue-resident marker CD103 (34), the tissue-resident and activation marker CD69 (35), and the tissue-resident and adhesion marker CD44 (36). G. vaginalis inoculated mice had significantly decreased absolute numbers of CD44+CD4+ T cells (Figure 4B), CD69+CD4+ T cells (Figure 4C), and CD103+CD4+ T cells (Figure 4D) compared to L. crispatus inoculated mice. L. crispatus inoculated mice had significantly increased proportions of CD69+CD4+ T cells compared to the PBS group. All of these markers are mucosal tissue residency markers, signifying there was a decrease in tissue-resident helper T cells in the vaginal immune environment in the G. vaginalis inoculated mice compared to L. crispatus. Decreased numbers of resident CD4+ T cells in the vaginal tissue could be linked to the increased susceptibility to HSV-2 in the G. vaginalis inoculated mice.
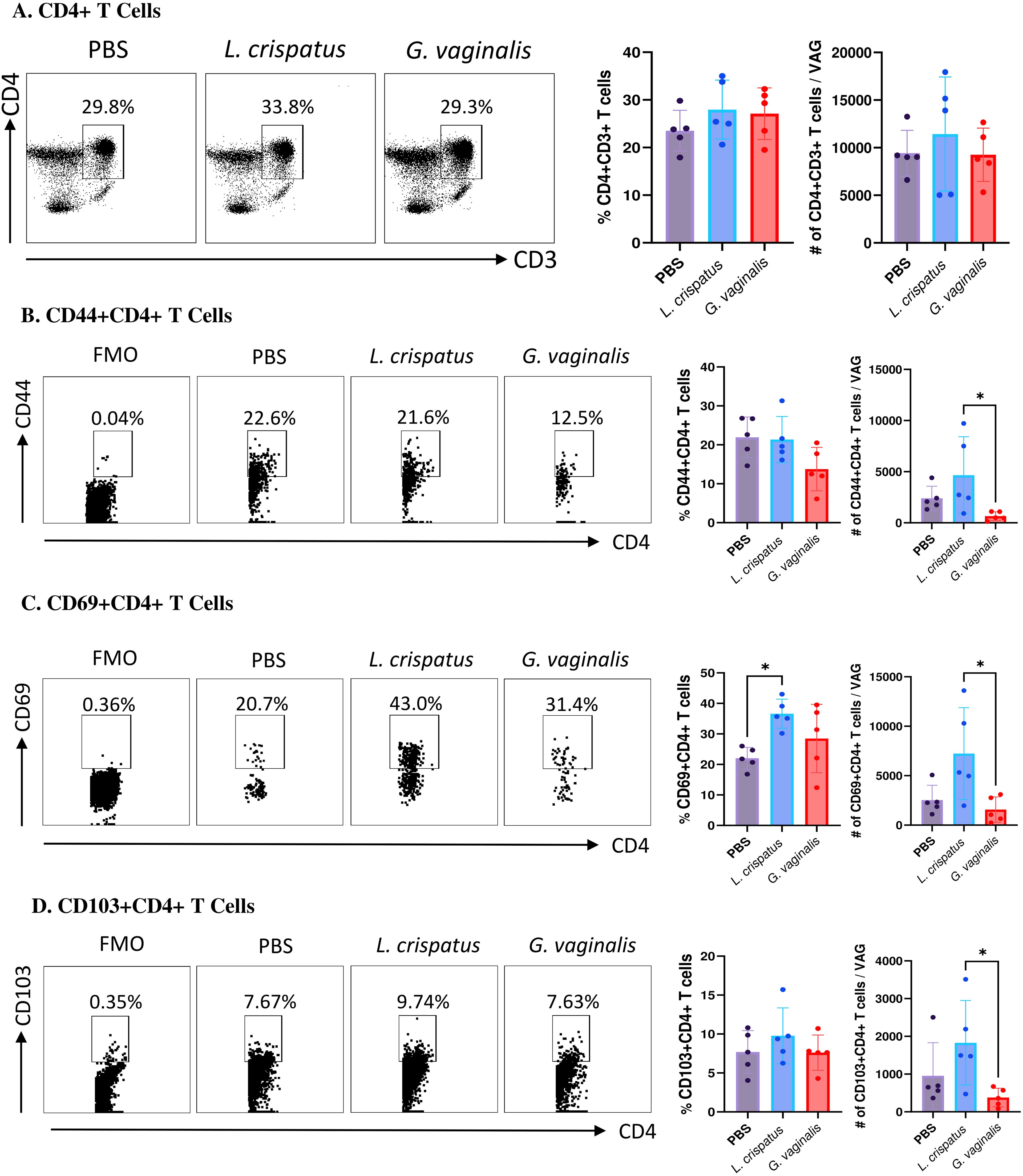
Figure 4. G. vaginalis inoculated mice had decreased activated and mucosal CD4+ T cells in the vaginal tract compared to L. crispatus inoculated mice. Female mice were intravaginally inoculated with 107 CFU L. crispatus, G. vaginalis, or PBS as a no-exogenous bacteria-negative control every 48 h for 10 days. On day 10 of the experiment, the vaginal tissue (VAG) was collected, processed, and stimulated for 16 h. Cells were stained for Live/Dead staining, CD3, CD4, CD44, CD69, and CD103 and ran on the Cytoflex flow cytometer and analyzed using FloJo software. The percent population and absolute count of CD4+ T cells are shown in panel (A). The percent population and absolute number of CD44+CD4+ T cells (B), CD69+CD4+ T cells (C), and CD103+CD4+ T cells (D) are depicted as well. Data are from n=5 per group, from one experiment representing three independent experiments. Data was analysed using a one-way ANOVA with Tukey’s multiple comparisons (*p<0.05).
Next, the CD8+CD3+ T cell compartment was examined (Figure 5A). Similar to the CD4+ T cells, there was not a significant change in the CD8+ T cells in the G. vaginalis or L. crispatus inoculated mice compared to the PBS group, indicating the bacterial inoculations did not significantly alter the total amount of CD8+ T cells in the vaginal mucosa. Once again, cells were further gated for mucosal markers including CD44+CD8+ T cells, CD69+CD8+ T cells, and CD103+CD8+ T cells. When compared to the PBS group, G. vaginalis inoculated mice had significantly decreased percentages and trends towards decreased absolute numbers of CD44+CD8+ T cells (Figure 5B). There was also significantly decreased absolute counts of CD69+CD8+ T cells (Figure 5C). There were trends toward decreased absolute counts of CD103+CD8+ T cells in the G. vaginalis group (Figure 5D). Although not as strong as the data for CD4+ T cells, there was a decrease in mucosal CD8+ T cells in G. vaginalis inoculated mice. This could also contribute to the increased susceptibility to HSV-2 in the G. vaginalis group.
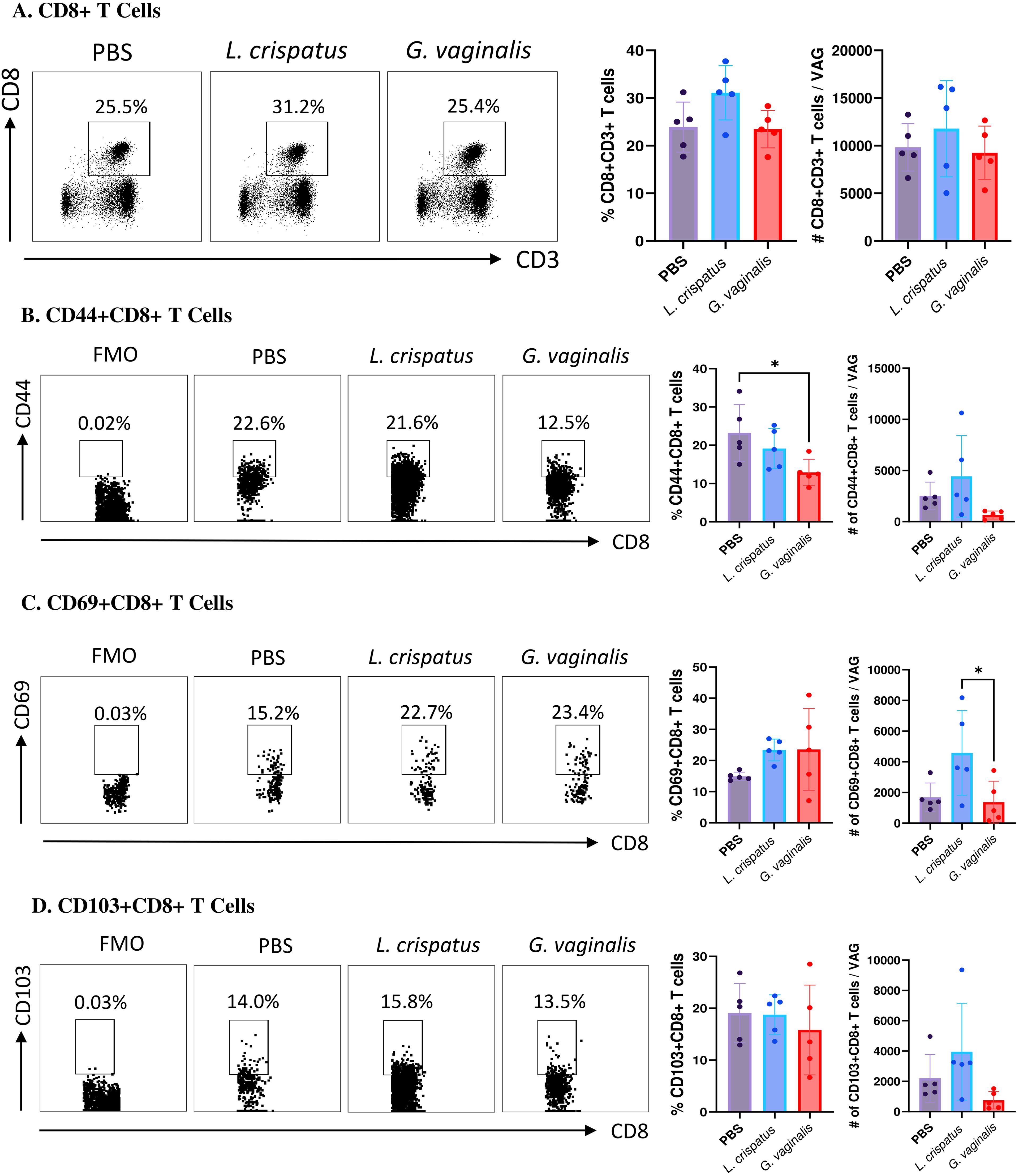
Figure 5. G. vaginalis inoculated mice had decreased activated and mucosal CD8+ T cells in the vaginal tract compared to L. crispatus inoculated mice. Female mice were intravaginally inoculated with 107 CFU L. crispatus, G. vaginalis, or PBS as a no-exogenous bacteria-negative control every 48 h for 10 days. On day 10 of the experiment, the vaginal tissue (VAG) was collected, processed, and stimulated for 16 h. Cells were stained for Live/Dead staining, CD3, CD8, CD44, CD69, and CD103, and ran on the Cytoflex flow cytometer and analyzed using FloJo software. The percent population and absolute number of CD8+ T cells are shown in panel (A). The percent population and absolute number of CD44+CD8+ T cells (B), CD69+CD8+ T cells (C), and CD103+CD8+ T cells (D) are depicted as well. Data are from n=5 per group, from one experiment representing three independent experiments. Data was analyzed using a one-way ANOVA with Tukey’s multiple comparisons (*p<0.05).
G. vaginalis inoculated mice had significantly increased levels of T cells expressing TNF-α and IFN-γ while L. crispatus inoculation enhanced IL-10 expression in T cells
In conjunction with mucosal cell surface markers, cytokine production by T cells was also assessed using flow cytometry to determine functional T cell populations in VMB-administered mice. Mice were inoculated with L. crispatus, G. vaginalis, or PBS as a negative control for ten days (five inoculations every 48 h). Following bacterial inoculation, the vaginal tissue, spleen and iliac lymph nodes were collected from mice on day ten after inoculations and processed and stained for flow cytometry. Cells were gated for CD4+ and CD8+ T cells and then further gated for the proinflammatory cytokines TNF-α and IFN-γ and the regulatory cytokine IL-10. Once again, no significant differences were noted in the cytokine expression by T cells from the spleen or the lymph nodes (Supplementary Figure S5), indicating that the effect of bacterial inoculation was site-specific and limited to the vaginal tract.
G. vaginalis inoculated mice had significantly increased proportions of TNF-α+CD4+ T cells compared to PBS and L. crispatus; however, the absolute numbers were not significantly different, indicating a possible selective enrichment of TNF-α producing CD4+ T cells (Figure 6A). There was no significant difference in the TNF-α+CD8+ T cells (Figure 6A). G. vaginalis inoculated mice had significantly increased proportions of IFN-γ+CD4+ and IFN-γ+CD8+ T cells compared to the PBS group (Figure 6B), but no difference in the absolute counts, once again indicating the possibility of selective enrichment. Lastly, the L. crispatus inoculated group has significantly increased proportions of IL-10+CD4+ T cells compared to PBS and significantly increased absolute counts of IL-10+CD4+ T cells compared to G. vaginalis (Figure 6C). L. crispatus inoculated mice also had significantly increased proportions of IL-10+CD8+ T cells compared to PBS and G. vaginalis groups and significantly increased absolute counts compared to G. vaginalis (Figure 6C). Overall, these results suggest G. vaginalis inoculation was associated with an inflammatory signature in vaginal T cells while L. crispatus inoculation was associated with regulatory cytokine production by vaginal T cells.
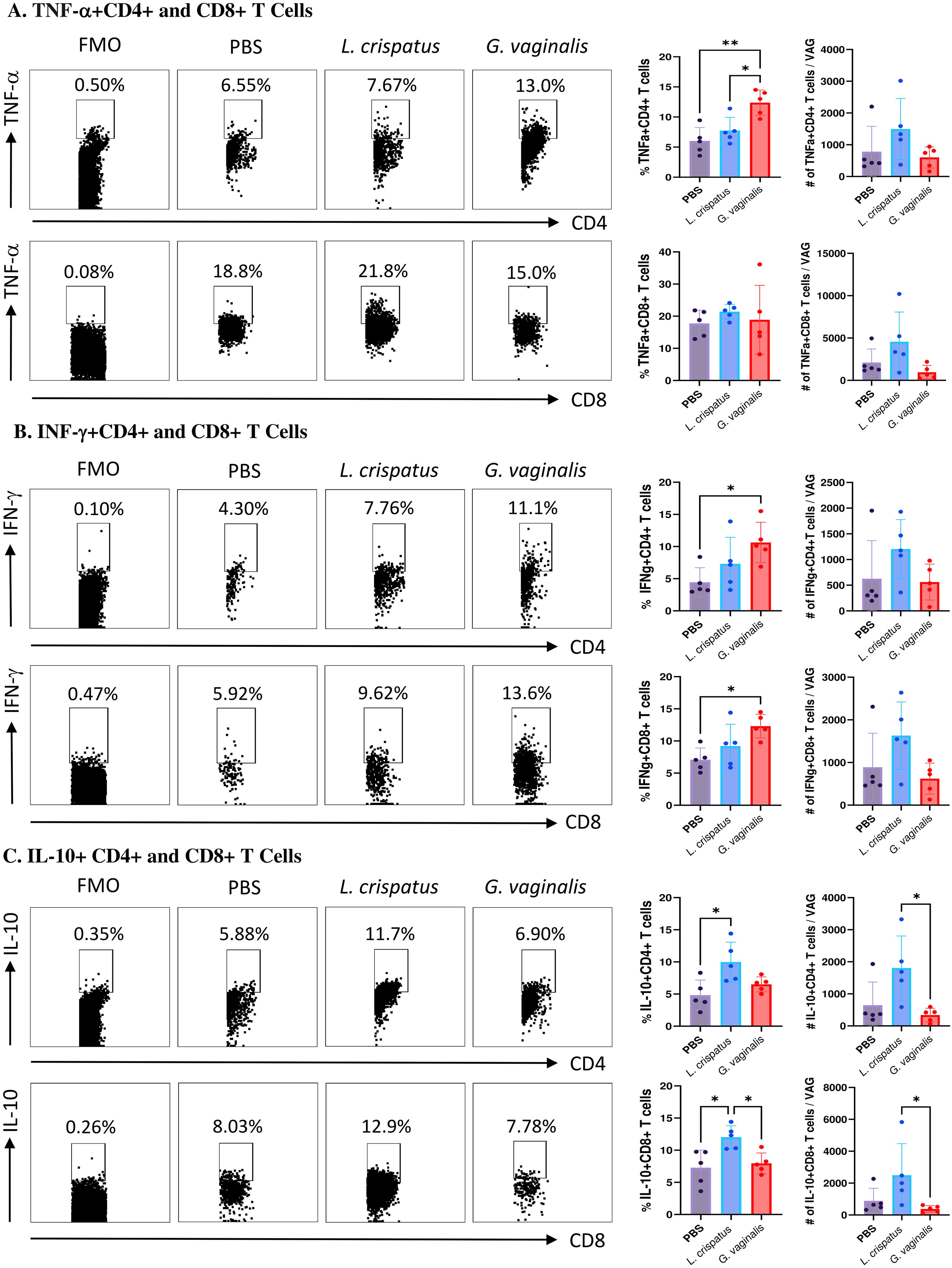
Figure 6. G. vaginalis inoculated mice had increased T cells expressing inflammatory cytokines and L. crispatus treated mice had increased T cells expressing regulatory cytokines in the vaginal tract. Female mice were intravaginally inoculated with 107 CFU L. crispatus, G. vaginalis, or PBS as a no-exogenous bacteria-negative control every 48 h for 10 days. On day 10 of the experiment, the vaginal tissue (VAG) was collected, processed, and stimulated for 16 h. Cells were stained for Live/Dead staining, CD3, CD4, CD8, TNF-α, IFN-γ, and IL-10, and ran on the Cytoflex flow cytometer and analyzed using FloJo software. CD4+ and CD8+ T cells expressing TNF-α (A), IFN-γ (B), and IL-10 (C) are depicted. Data are from n=5 per group, from one experiment representing three independent experiments. Data was analyzed using a one-way ANOVA with Tukey’s multiple comparisons (*p<0.05 and **p<0.01).
G. vaginalis inoculated mice had increased inflammatory cytokine and chemokine production compared to L. crispatus
Since the flow cytometric analysis indicated functional differences in T cell populations, we next examined if there were changes in the cytokine levels in the vaginal immune milieu following colonization with different bacteria. A multiplex assay was used to assess cytokine and chemokine levels after bacterial inoculation. Mice were inoculated for ten days (five inoculations 48 h apart) with L. crispatus, G. vaginalis, or PBS as a negative control. On day ten, vaginal washes were collected from mice and a 31-Plex mouse cytokine/chemokine assay was conducted to analyze cytokine levels in the vaginal tract. While the majority of the 31 cytokines and chemokines examined did not show any significant changes following colonization with different bacteria, some key cytokines and chemokines were altered. Overall G. vaginalis was associated with an inflammatory signature in the vaginal canal compared to L. crispatus. In congruence with the flow cytometry experiments, G. vaginalis inoculated mice had significantly increased TNF-α levels in the vaginal secretions compared to L. crispatus (Figure 7A). There were also trends towards increased IFN-γ, IL-1α, and IL-1β levels in the G. vaginalis group. L. crispatus had significantly decreased levels of IL-12p70 expression compared to PBS (Figure 7A). IL-10 levels were not significantly affected in bacteria-inoculated mice (Figure 7A). When the expression of chemokines was examined, G. vaginalis also had increased expression of chemokines that attract inflammatory cells, including a significant increase in LIX, a chemokine known to attract neutrophils (37), and a trend towards increased expression of MIP-1α and MIP-1β (Figure 7B). Other cytokines and chemokines from the 31-Plex assay did not show any trends or significance (data not shown).
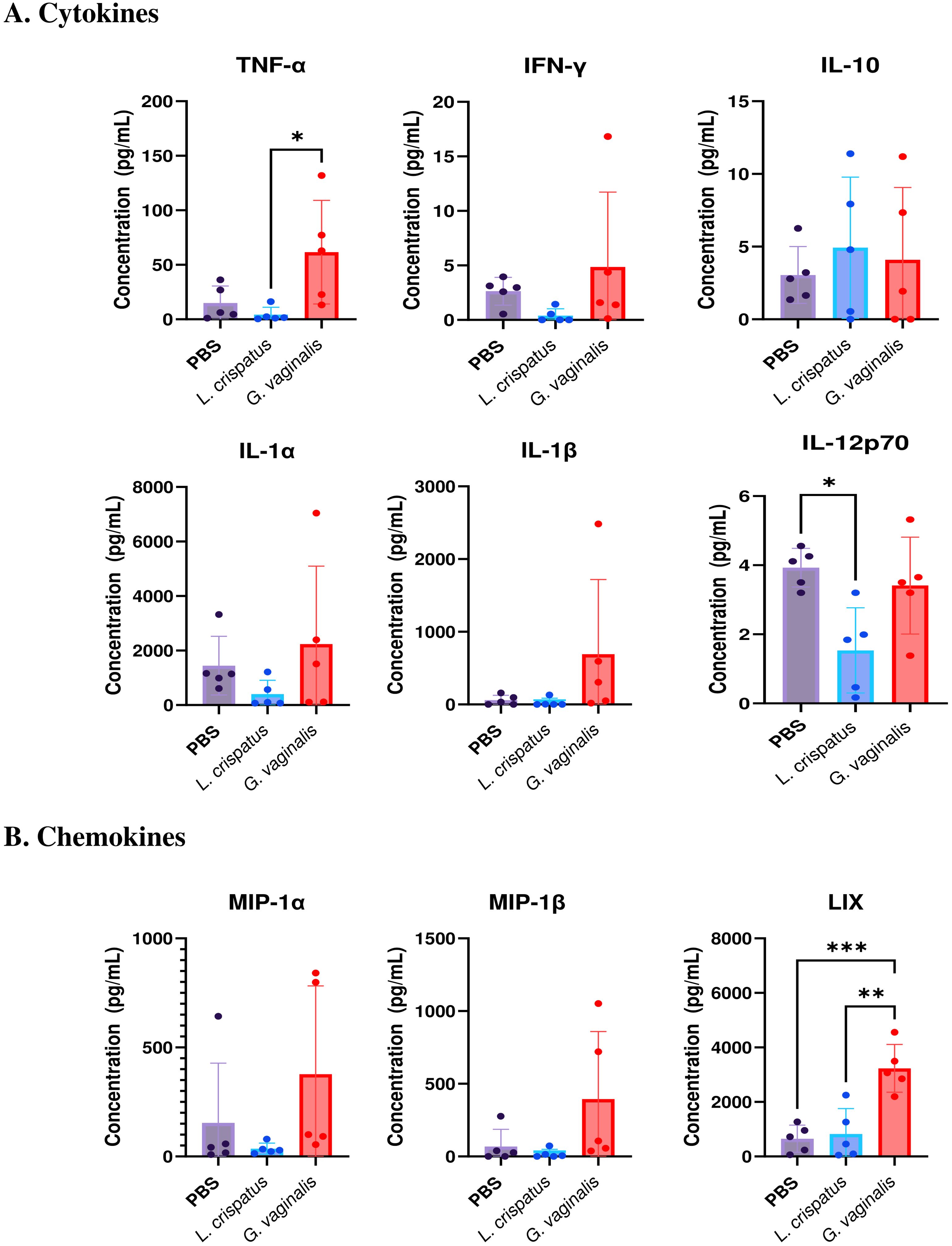
Figure 7. G. vaginalis inoculated mice had increased inflammatory cytokine and chemokine protein levels in the vaginal tract. Female mice were intravaginally inoculated with 107 CFU L. crispatus, G. vaginalis, or PBS as a no-exogenous bacteria-negative control every 48 h for 10 days. On day 10 of the experiment, vaginal washes were collected and sent to Eve Technologies to perform a 31-Plex mouse cytokine/chemokine assay. All significant cytokine (A) and chemokine (B) data are shown above. Data are from n=5 per group, from one independent experiment. Data was analyzed using a one-way ANOVA with Tukey’s multiple comparisons (*p<0.05, **p<0.01, and ***p<0.001).
Discussion
To prevent the spread of STIs in vulnerable populations, it is important to understand why women are disproportionately susceptible to viral STIs such as HSV-2. The distinct VMB of the female reproductive tract is thought to play a role in this susceptibility bias, which is supported by clinical and epidemiological evidence (12–14). However, epidemiological and in vitro studies are limited in their ability to elucidate cause-effect mechanisms of how VMB composition affects HSV-2 susceptibility. In vitro studies do not fully mimic a physiological system, and epidemiological studies are usually retrospective and typically demonstrate correlation rather than cause-effect relationships. Using human microbiota-associated (HMA) mouse models that harbour human VMB species can address some of the shortcomings of other studies and provide a physiologically relevant model that can be experimentally manipulated. There have been attempts to colonize mice with Lactobacillus species and G. vaginalis to assess physiological outcomes in the vaginal microenvironment, however, these models have not examined the outcomes of common sexually transmitted viruses, like HSV-2, in the presence of distinct bacteria present in the human VMB (20, 38–44). There is clinical evidence to suggest a dysbiotic VMB is associated with increased susceptibility to HSV-2 in women (12–14), however, biological mechanisms are lacking in the literature. In this study, we used our novel HMA models that were previously reported (19) to elucidate mechanisms as to how the VMB may affect susceptibility to HSV-2.
We first tested our models under HSV-2 infection to determine if inoculation with the dysbiotic bacteria G. vaginalis would mimic clinical evidence and increase susceptibility to HSV-2 in vivo. In the VMB associated mouse models, there was decreased survival, increased pathology, and higher viral titers in mice inoculated with G. vaginalis compared to L. crispatus and PBS mice. Normal mice that are at different stages of the reproductive cycle are typically not susceptible to HSV-2 unless they are experimentally manipulated to enable thinning of the vaginal epithelium (29, 30). We have previously reported when normal mice are infected with HSV-2 at a dose of 105 PFU, mice do not show pathology and survive, similar to the results from PBS mice in this study (29). No significant increase in susceptibility was seen for L. crispatus inoculated mice. However, we did observe significantly enhanced susceptibility in the G. vaginalis inoculated group compared to the PBS and L. crispatus groups. We have previously reported that the mouse VMB has a finite niche, and colonization with exogenous bacteria displaces the endogenous mouse microbiota (19). Due to the low levels of endogenous species, we posit that the change in susceptibility was due to the exogenous VMB species we inoculated. Importantly, no live bacteria could be detected in the vaginal tract following HSV-2 infection, indicating that any differences in susceptibility were due to changes that occurred in the vaginal microenvironment before exposure to the virus. The mice which developed pathology and did not survive followed the typical course of in vivo infection which has previously been described in several studies and published extensively (26, 28, 30, 31, 45, 46). Similar to other published studies, high viral titers were detected in the vaginal tract for 3-5 days post-infection at a time when the virus is known to replicate in the vaginal epithelium (30). During this time, the innate and early adaptive antiviral immune responses have been shown to be activated in order to contain the viral replication (47, 48). At the sublethal dose of challenge, in the L. crispatus and PBS groups, we would posit that the immune system was able to control the spread of the virus throughout the epithelium, which is why the viral titers began to decrease at around 4-5 days post-infection. In the G. vaginalis group, however, some mice rapidly developed pathology after five days of infection; we would predict that the virus had evaded the immune system, successfully obliterated the epithelium and spread to the paracervical ganglia and the central nervous system (28). By day 6-7 of infection, the decrease in vaginal viral titers even in mice that developed pathology is likely because the virus is no longer replicating in the vaginal epithelium and has entered the nervous system (30). Taken together, our results show that G. vaginalis inoculation shaped the vaginal microenvironment to be conducive to successful infection with the sublethal dose of HSV-2 prior to infection, ultimately leading to the pathology and lower survival rates in this group.
While this is the first study to report the role of the VMB on HSV-2 infection in vivo, other groups have also reported inoculation of G. vaginalis or other dysbiotic species to be associated with negative health outcomes. G. vaginalis has been implicated in adverse pregnancy outcomes in multiple models. Studies have shown that rabbits administered G. vaginalis to the upper reproductive tract have significantly lower live birth rates, lower fetal weights, and neural pathology in the fetus (49, 50). Mice inoculated intravaginally with G. vaginalis have been shown to have increased inflammation, soluble E-cadherin, and cervical remodelling at earlier time points of gestation. While cervical remodelling is a normal part of parturition, earlier indications can be implicated with pre-term birth (39). Studies have also connected dysbiotic bacteria and urinary tract infections. One in vivo study found bladder exposure to G. vaginalis triggered Escherichia coli egress from latent bladder reservoirs. G. vaginalis exposure was also sufficient to cause epithelial apoptosis in the bladder and to induce kidney injury via an IL-1 receptor pathway (51). Perhaps most relevant to this study, there are several mouse studies implicating a dysbiotic vaginal microbiota with an increased risk of bacterial STIs and HIV. One study found that infection with Group B Streptococcal species decreased the stability of the mouse VMB, and endogenous Staphylococcal abundance in the mouse VMB correlated with increased infection outcomes (52). Another study found that humanized mice administered MPA had increased VMB diversity, which resulted in enhanced HIV susceptibility (23). It was also found that the endogenous VMB of BALB/c mice consists mainly of Pseudomonas and Janthinobacterium, and that infection with HSV-2 shifted the vaginal microbiota of these mice to contain more dysbiotic species Staphylococcus and Escherichia (53). While these studies all implicate G. vaginalis and other dysbiotic bacteria with negative health outcomes, there are very few studies in the literature reporting the use of in vivo models to elucidate the underlying mechanisms to explain how the vaginal bacteria may affect susceptibility to infections. To our knowledge, this is the first study that administered eubiotic and dysbiotic human VMB species to mice to elucidate the effect these bacteria have on HSV-2 infection outcomes in vivo.
As previously mentioned, the main purpose of HMA models is to determine cause-effect mechanisms of how the VMB may affect susceptibility to infections such as HSV-2. In this study, we first determined the role of the VMB on barrier integrity, as the vaginal epithelial barrier is essential to HSV-2 infection (28, 54). Previous studies have shown that the vaginal epithelium plays a critical role in enabling HSV-2 infection since thin and/or disrupted epithelium allows the virus to rapidly gain entry into the lamina propria of the mucosa and establish infection (31, 55). Given the nature of how HSV-2 infects, we wanted to examine if human VMB species have an effect on the vaginal epithelium, which can help explain why G. vaginalis was associated with increased susceptibility to HSV-2 in our mice. We found G. vaginalis inoculation resulted in decreased Desmoglein-1 staining, which is a desmosomal adhesion protein found in the vaginal epithelium of humans and mice (22, 56). Desmosomes are a major intracellular adhesion junction at the basolateral membrane (57). The reduction of this protein in the G. vaginalis group provides a potential mechanism for the HSV-2 virus to breach the epithelium and infect the central nervous system, leading to increased HSV-2 pathology in this group. Another study has also found inoculation of mice with G. vaginalis decreases the expression of junctional proteins such as DSG-1 expression in the vaginal tract of BALB/c mice (58). G. vaginalis has also been shown in other clinical studies to disrupt the barrier by degrading mucin through the production of sialidase (59) and promoting epithelial cell shedding (60). Overall, our results align with previously published data on G. vaginalis and epithelial barrier disruption and provide a mechanism for how this dysbiotic bacteria may increase HSV-2 infection outcomes.
Another important factor in HSV-2 infection is the immune response to the virus. The composition of the VMB is known to alter immune responses in the vaginal mucosa, which can affect susceptibility to HSV-2 (61, 62). In our study, we found G. vaginalis was associated with decreased overall numbers of tissue-resident T cells. We saw a decrease in the numbers of T cells expressing CD69, CD103, and CD44 in G. vaginalis mice compared to L. crispatus inoculated mice. The presence of these markers can be used to identify activated mature mucosal immune cells that are available locally in the mucosal tissue to fight infection (34–36). G. vaginalis inoculated mice had significantly decreased numbers of activated CD69+ T cells, and T cells expressing mucosal homing markers CD103 and CD44 compared to L. crispatus mice in both the CD4 and CD8 compartments. Since the overall number of total CD4+ and CD8+ T cells was not changed in the vaginal tract of these mice, this could indicate a selective reduction in mature T cells that can readily fight HSV-2 upon infection. Whilst out of the scope of this study, other groups have investigated how G. vaginalis may alter T cell responses. In one study, G. vaginalis was found to possess slight immune stimulating activity against T cells. At high doses, G. vaginalis significantly increased proliferation and cytokine secretion in lymphocytes (63). This leads to a defective inflammatory response and atypical low-grade inflammation. Importantly, this atypical cytokine production also plays an important role in T cell activation and chemotaxis, which could play a role in the reduced activation markers seen in G. vaginalis inoculated mice (64).
We also found G. vaginalis inoculated mice to be associated with increased inflammatory cytokine and chemokine production. In HSV-2 infection, an influx of immune cells is a part of a productive immune response (65). However, having a state of constant non-specific inflammation can be associated with an increased risk of sexually transmitted infections such as HIV (66). Chronic inflammation can also damage healthy cells and tissues (67). Pro-inflammatory cytokines have also been attributed to a disruption of the epithelial barrier (68). G. vaginalis has been implicated with inflammation in multiple clinical and in vitro studies (69–74). Some in vivo studies have also indicated this. In one study, G. vaginalis has been shown to increase transcript levels of cytokines IL-8, IL-1β, and IL-10 in the cervices of mice and protein levels of IL-6 in the cervicovaginal fluid (39). Another group has also reported increased protein levels of pro-inflammatory cytokines TNF-α, IL-1β, and IL-6 in the vaginal tissue of G. vaginalis inoculated mice compared to control mice (40–42). In addition, the authors reported a higher number of inflammatory markers myeloperoxidase, iNOS, and COX-2 levels in the vaginal tissues of G. vaginalis inoculated mice. Additionally, when histology was performed on vaginal tissue, there appeared to be substantial edema and immune cell infiltration into the superficial mucosal layers (75). In our study, we also saw an increase in classic inflammatory cytokines TNF-α, IL-1α and IL-1β in G. vaginalis inoculated mice, similar to what has previously been reported. We also saw increased amounts of MIP-1α, MIP-1β, and LIX, which are potent recruiters of immune cells such as neutrophils (37). In our study, we also saw an increase of IL-10+ T cells in L. crispatus inoculated mice, but not G. vaginalis. IL-10 is a regulatory cytokine that plays a role in preventing damage to the host and maintaining normal tissue homeostasis (76), so, consistently, the eubiotic bacteria L. crispatus had increased IL-10+ T cells. In the multiplex assay, IL-10 protein levels were however unaffected by bacteria inoculation. The multiplex assay used vaginal washes, and all of the flow cytometry data was from whole tissue homogenates, so these differences may be due to the difference in sample types. We also saw a significant decrease in pro-inflammatory cytokine IL-12p70 protein levels in L. crispatus inoculated mice, further indicating a potential regulatory role for L. crispatus in vivo.
In summary, this study provides new and important insights into the mechanism behind increased HSV-2 infection due to a dysbiotic VMB using an in vivo model. While clinical studies have provided significant insights regarding the correlation between colonization by dysbiotic species such as G. vaginalis with increased susceptibility to HSV-2, mechanistic studies examining cause-effect relationships have not been possible due to the lack of animal models. In this study, we identified the disruption of the vaginal epithelial barrier and the reduction of protective immune responses in G. vaginalis inoculated mice, which likely contributed to the increased HSV-2 infection outcomes seen in these mice. The current model can be translated to humanized mice as well as germ-free mice to study the role of the VMB on HIV susceptibility and to further understand the immune development associated with the VMB. Moving forward, mouse models that harbour human vaginal microbiota species can play a critical role in better understanding the roles of the VMB in female reproductive health.
Data availability statement
The raw data supporting the conclusions of this article will be made available by the authors, without undue reservation.
Ethics statement
The animal study was approved by McMaster Animal Research Ethics Board. The study was conducted in accordance with the local legislation and institutional requirements.
Author contributions
NR: Conceptualization, Data curation, Formal analysis, Investigation, Methodology, Software, Visualization, Writing – original draft, Writing – review & editing. MM: Investigation, Methodology, Writing – review & editing. CH: Investigation, Writing – review & editing. AN: Investigation, Methodology, Writing – review & editing. CK: Conceptualization, Funding acquisition, Methodology, Project administration, Resources, Supervision, Validation, Writing – review & editing.
Funding
The author(s) declare that financial support was received for the research, authorship, and/or publication of this article. This research was supported by grants from the Canadian Institutes of Health Research (CIHR Operating Grant FRN#126019 (CK); NR received funding support from the Ontario Graduate Scholarship (OGS).
Acknowledgments
The authors acklowledge the help of Ravneet Hansi and Elizabeth Ball in technical support for mouse tissue processing.
Conflict of interest
The authors declare that the research was conducted in the absence of any commercial or financial relationships that could be construed as a potential conflict of interest.
Publisher’s note
All claims expressed in this article are solely those of the authors and do not necessarily represent those of their affiliated organizations, or those of the publisher, the editors and the reviewers. Any product that may be evaluated in this article, or claim that may be made by its manufacturer, is not guaranteed or endorsed by the publisher.
Supplementary material
The Supplementary Material for this article can be found online at: https://www.frontiersin.org/articles/10.3389/fimmu.2024.1487726/full#supplementary-material
Supplementary Figure 1 | Mice in estrus were able to stay colonized with exogenous bacteria. Female mice were inoculated five times every 48 h with 107 CFU L. crispatus or G. vaginalis. Data are from n = 4 per group from one independent experiment. Vaginal washes were collected every day until 24 h after the fifth inoculation (day 9) and assessed using quantitative plating assays. Bacterial colonies of the inoculated species types were counted in L. crispatus inoculated (A) and G. vaginalis inoculated (B) mice. Different coloured points denote different mice. Individual mouse data is plotted beside the cumulative graphs. Red dots in these graphs denote the mouse was in estrus and black dots denote the mouse was in diestrus on that day. The arrows indicate the day mice were inoculated with bacteria. The data was analyzed using a two-way ANOVA with Tukey’s multiple comparisons, but no significance was found.
Supplementary Figure 2 | No bacteria was detected in the vaginal tract following HSV-2 infection. Female mice were inoculated five times every 48 h with 107 CFU L. crispatus, G. vaginalis, or PBS as a no-exogenous bacteria negative control. Vaginal washes were collected 24 h before intravaginal infection with 105 PFU wildtype HSV-2, as well as one- and five-days post infection. Bacterial load was assessed using quantitative plating assays. Bacterial colonies were counted in n=14 mice and plotted. Different dots denote different mice. The data was analyzed with a one-way ANOVA with Tukey’s multiple comparisons (*p<0.05).
Supplementary Figure 3 | Gating Strategy for Flow Cytometry. Female mice were intravaginally inoculated with 107 CFU L. crispatus, G. vaginalis, or PBS as a no-exogenous bacteria-negative control every 48 h for 10 days. On day 10 of the experiment, the vaginal tissue was collected, processed, and stimulated for 16 h. Cells were stained for Live/Dead staining, CD3, CD4, and CD8 (other markers for tissue-resident and functional T cells were also included). The gating strategy up to CD4+CD3+ and CD8+CD3+ T cells is depicted above.
Supplementary Figure 4 | There were no differences in T cell populations in the spleen and lymph nodes of VMB-inoculated mice. Female mice were intravaginally inoculated with 107 CFU, L. crispatus, G. vaginalis, or PBS as a no-exogenous bacteria-negative control every 48 h for 10 days. On day 10 of the experiment, the spleen and iliac lymph nodes (LN) were collected, processed, and stimulated for 16 h. Cells were stained for Live/Dead staining, CD3, CD4, CD8, CD44, CD103, and CD69, ran on the Cytoflex flow cytometer, and analyzed using FloJo software. The gating strategy is shown in panel (A). The percent population and absolute count of CD44+CD4+ T cells in the spleen (B) and LN (C), CD44+CD8+ T cells in the spleen (D) and LN (E), CD69+CD4+ T cells in the spleen (F) and LN (G), CD69+CD8+ T cells in the spleen (H) and LN (I), CD103+CD4+ T cells in the spleen (J) and LN (K), and CD103+CD8+ T cells in the spleen (L) and LN (M), are depicted above. Data are from n=7 per group, from one experiment representing three independent experiments. Data was analyzed using a one-way ANOVA with Tukey’s multiple comparisons, but no significance was found.
Supplementary Figure 5 | There were no differences in cytokine-producing T cells in the spleen and lymph nodes of VMB-inoculated mice. Female mice were intravaginally inoculated with 107 CFU L. crispatus, G. vaginalis, or PBS as a no-exogenous bacteria-negative control every 48 h for 10 days. On day 10 of the experiment, the spleen and iliac lymph nodes (LN) were collected, processed, and stimulated for 16 h. Cells were stained for Live/Dead staining, CD3, CD4, CD8, TNF-α, IFN-γ, and IL-10, ran on the Cytoflex flow cytometer, and analyzed using FloJo software. The gating strategy is shown in panel (A). The percent population and absolute count of TNF-α+CD4+ T cells in the spleen (B) and LN (C), TNF-α+CD8+ T cells in the spleen (D) and LN (E), IFN-γ+CD4+ T cells in the spleen (F) and LN (G), IFN-γ+CD8+ T cells in the spleen (H) and LN (I), IL-10+CD4+ T cells in the spleen (J) and LN (K), and IL-10+CD8+ T cells in the spleen (L) and LN (M), are depicted above. Data are from n=7 per group, from one experiment representing three independent experiments. Data was analyzed using a one-way ANOVA with Tukey’s multiple comparisons, but no significance was found.
References
1. Peric A, Weiss J, Vulliemoz N, Baud D, Stojanov M. Bacterial colonization of the female upper genital tract. Int J Mol Sci. (2019) 20:3405. doi: 10.3390/ijms20143405
2. Ravel J, Gajer P, Abdo Z, Schneider GM, Koenig SSK, McCulle SL, et al. Vaginal microbiome of reproductive-age women. Proc Natl Acad Sci. (2011) 108:4680–7. doi: 10.1073/pnas.1002611107
3. Chee WJY, Chew SY, Than LTL. Vaginal microbiota and the potential of lactobacillus derivatives in maintaining vaginal health. Microbial Cell Factories. (2020) 19:203. doi: 10.1186/s12934-020-01464-4
4. Ghartey JP, Smith BC, Chen Z, Buckley N, Lo Y, Ratner AJ, et al. Lactobacillus crispatus dominant vaginal microbiome is associated with inhibitory activity of female genital tract secretions against escherichia coli. PloS One. (2014) 9:e96659. doi: 10.1371/journal.pone.0096659
5. Chen X, Lu Y, Chen T, Li R. The female vaginal microbiome in health and bacterial vaginosis. Front Cell Infect Microbiol. (2021) 11. doi: 10.3389/fcimb.2021.631972
6. WHO. Herpes Simplex Virus (2020). Available online at: https://www.who.int/news-room/fact-sheets/detail/herpes-simplex-virus (accessed July 3, 2024).
7. James C, Harfouche M, Welton NJ, Turner KM, Abu-Raddad LJ, Gottlieb SL, et al. Herpes simplex virus: global infection prevalence and incidence estimates, 2016. Bull World Health Organ. (2020) 98:315–29. doi: 10.2471/blt.19.237149
8. Wald A, Langenberg AG, Link K, Izu AE, Ashley R, Warren T, et al. Effect of condoms on reducing the transmission of herpes simplex virus type 2 from men to women. Jama. (2001) 285:3100–6. doi: 10.1001/jama.285.24.3100
9. Grabowski MK, Gray RH, Makumbi F, Kagaayi J, Redd AD, Kigozi G, et al. Use of injectable hormonal contraception and women’s risk of herpes simplex virus type 2 acquisition: A prospective study of couples in Rakai, Uganda. Lancet Glob Health. (2015) 3:e478–e86. doi: 10.1016/s2214-109x(15)00086-8
10. Dickson N, van Roode T, Herbison P, Taylor J, Cunningham A, Paul C. Risk of herpes simplex virus type 2 acquisition increases over early adulthood: evidence from a cohort study. Sex Transm Infect. (2007) 83:87–90. doi: 10.1136/sti.2006.020883
11. Cherpes TL, Meyn LA, Krohn MA, Hillier SL. Risk factors for infection with herpes simplex virus type 2: role of smoking, douching, uncircumcised males, and vaginal flora. Sex Transm Dis. (2003) 30:405–10. doi: 10.1097/00007435-200305000-00006
12. Cherpes TL, Meyn LA, Krohn MA, Lurie JG, Hillier SL. Association between acquisition of herpes simplex virus type 2 in women and bacterial vaginosis. Clin Infect Dis. (2003) 37:319–25. doi: 10.1086/375819
13. Mehta SD, Nandi D, Agingu W, Green SJ, Bhaumik DK, Bailey RC, et al. Vaginal and penile microbiome associations with herpes simplex virus type 2 in women and their male sex partners. J Infect Dis. (2020) 226:644–54. doi: 10.1093/infdis/jiaa529
14. Mehta SD, Pradhan AK, Green SJ, Naqib A, Odoyo-June E, Gaydos CA, et al. Microbial diversity of genital ulcers of hsv-2 seropositive women. Sci Rep. (2017) 7:15475. doi: 10.1038/s41598-017-15554-8
15. Nardini P, Ñahui Palomino RA, Parolin C, Laghi L, Foschi C, Cevenini R, et al. Lactobacillus crispatus inhibits the infectivity of chlamydia trachomatis elementary bodies, in vitro study. Sci Rep. (2016) 6:29024. doi: 10.1038/srep29024
16. Lewis WG, Robinson LS, Gilbert NM, Perry JC, Lewis AL. Degradation, foraging, and depletion of mucus sialoglycans by the vagina-adapted actinobacterium gardnerella vaginalis. J Biol Chem. (2013) 288:12067–79. doi: 10.1074/jbc.m113.453654
17. Roselletti E, Sabbatini S, Perito S, Mencacci A, Vecchiarelli A, Monari C. Apoptosis of vaginal epithelial cells in clinical samples from women with diagnosed bacterial vaginosis. Sci Rep. (2020) 10:1978. doi: 10.1038/s41598-020-58862-2
18. Amabebe E, Anumba DOC. Mechanistic insights into immune suppression and evasion in bacterial vaginosis. Curr Microbiol. (2022) 79:84. doi: 10.1007/s00284-022-02771-2
19. Rahman N, Mian MF, Nazli A, Kaushic C. Human vaginal microbiota colonization is regulated by female sex hormones in a mouse model. Front Cell Infection Microbiol. (2023) 13:1307451. doi: 10.3389/fcimb.2023.1307451
20. Morrill S, Gilbert NM, Lewis AL. Gardnerella vaginalis as a cause of bacterial vaginosis: appraisal of the evidence from in vivo models. Front Cell Infect Microbiol. (2020) 10. doi: 10.3389/fcimb.2020.00168
21. Gosmann C, Anahtar MN, Handley SA, Farcasanu M, Abu-Ali G, Bowman BA, et al. Lactobacillus-deficient cervicovaginal bacterial communities are associated with increased hiv acquisition in young South African women. Immunity. (2017) 46:29–37. doi: 10.1016/j.immuni.2016.12.013
22. Wessels JM, Nguyen PV, Vitali D, Mueller K, Vahedi F, Felker AM, et al. Depot medroxyprogesterone acetate (Dmpa) enhances susceptibility and increases the window of vulnerability to hiv-1 in humanized mice. Sci Rep. (2021) 11:3894. doi: 10.1038/s41598-021-83242-9
23. Wessels JM, Lajoie J, Cooper MIJH, Omollo K, Felker AM, Vitali D, et al. Medroxyprogesterone acetate alters the vaginal microbiota and microenvironment in a Kenyan sex worker cohort and is also associated with increased susceptibility to hiv-1 in humanized mice. Dis Models Mech. (2019) 12:dmm039669. doi: 10.1242/dmm.039669
24. Miles AA, Misra SS, Irwin JO. The estimation of the bactericidal power of the blood. J Hyg (Lond). (1938) 38:732–49. doi: 10.1017/s002217240001158x
25. Ajayi AF, Akhigbe RE. Staging of the estrous cycle and induction of estrus in experimental rodents: an update. Fertility Res Pract. (2020) 6:5. doi: 10.1186/s40738-020-00074-3
26. Bagri P, Ghasemi R, McGrath JJC, Thayaparan D, Yu E, Brooks AG, et al. Estradiol enhances antiviral cd4+ Tissue-resident memory T cell responses following mucosal herpes simplex virus 2 vaccination through an il-17-mediated pathway. J Virol. (2020) 95. doi: 10.1128/jvi.01206-20
27. Baer A, Kehn-Hall K. Viral concentration determination through plaque assays: using traditional and novel overlay systems. J Vis Exp. (2014) 93):e52065. doi: 10.3791/52065
28. Iversen MB, Paludan SR, Holm CK. Vaginal hsv-2 infection and tissue analysis. Bio Protoc. (2017) 7:e2383. doi: 10.21769/BioProtoc.2383
29. Kaushic C, Ashkar AA, Reid LA, Rosenthal KL. Progesterone increases susceptibility and decreases immune responses to genital herpes infection. J Virol. (2003) 77:4558–65. doi: 10.1128/jvi.77.8.4558-4565.2003
30. Gillgrass AE, Fernandez SA, Rosenthal KL, Kaushic C. Estradiol regulates susceptibility following primary exposure to genital herpes simplex virus type 2, while progesterone induces inflammation. J Virol. (2005) 79:3107–16. doi: 10.1128/jvi.79.5.3107-3116.2005
31. Quispe Calla N, Vicetti Miguel R, Boyaka P, Hall-Stoodley L, Kaur B, Trout W, et al. Medroxyprogesterone acetate and levonorgestrel increase genital mucosal permeability and enhance susceptibility to genital herpes simplex virus type 2 infection. Mucosal Immunol. (2016) 9(6):1571–83. doi: 10.1038/mi.2016.22
32. Nguyen PV, Kafka JK, Ferreira VH, Roth K, Kaushic C. Innate and adaptive immune responses in male and female reproductive tracts in homeostasis and following hiv infection. Cell Mol Immunol. (2014) 11:410–27. doi: 10.1038/cmi.2014.41
33. O’Neil TR, Hu K, Truong NR, Arshad S, Shacklett BL, Cunningham AL, et al. The role of tissue resident memory cd4 T cells in herpes simplex viral and hiv infection. Viruses. (2021) 13:359. doi: 10.3390/v13030359
34. Annacker O, Coombes JL, Malmstrom V, Uhlig HH, Bourne T, Johansson-Lindbom B, et al. Essential role for cd103 in the T cell–mediated regulation of experimental colitis. J Exp Med. (2005) 202:1051–61. doi: 10.1084/jem.20040662
35. Cibrián D, Sánchez-Madrid F. Cd69: from activation marker to metabolic gatekeeper. Eur J Immunol. (2017) 47:946–53. doi: 10.1002/eji.201646837
36. Jordan AR, Racine RR, Hennig MJ, Lokeshwar VB. The role of cd44 in disease pathophysiology and targeted treatment. Front Immunol. (2015) 6:182. doi: 10.3389/fimmu.2015.00182
37. Rovai LE, Herschman HR, Smith JB. The murine neutrophil-chemoattractant chemokines lix, kc, and mip-2 have distinct induction kinetics, tissue distributions, and tissue-specific sensitivities to glucocorticoid regulation in endotoxemia. J Leukoc Biol. (1998) 64:494–502. doi: 10.1002/jlb.64.4.494
38. Gilbert NM, Lewis WG, Lewis AL. Clinical features of bacterial vaginosis in a murine model of vaginal infection with gardnerella vaginalis. PloS One. (2013) 8:e59539. doi: 10.1371/journal.pone.0059539
39. Sierra L-J, Brown AG, Barilá GO, Anton L, Barnum CE, Shetye SS, et al. Colonization of the cervicovaginal space with gardnerella vaginalis leads to local inflammation and cervical remodeling in pregnant mice. PloS One. (2018) 13:e0191524. doi: 10.1371/journal.pone.0191524
40. Joo HM, Hyun WJ, Myoung KS, Ahn YT, Lee JH, Huh CS, et al. Lactobacillus johnsonii hy7042 ameliorates gardnerella vaginalis-induced vaginosis by killing gardnerella vaginalis and inhibiting nf-Kb activation. Int Immunopharmacol. (2011) 11:1758-65. doi: 10.1016/j.intimp.2011.07.002
41. Jang S-E, Jeong J-J, Choi S-Y, Kim H, Han M, Kim D-H. Lactobacillus rhamnosus hn001 and lactobacillus acidophilus la-14 attenuate gardnerella vaginalis-infected bacterial vaginosis in mice. Nutrients. (2017) 9:531. doi: 10.3390/nu9060531
42. Kim DE, Kim JK, Han SK, Jang SE, Han MJ, Kim DH. Lactobacillus plantarum nk3 and bifidobacterium longum nk49 alleviate bacterial vaginosis and osteoporosis in mice by suppressing nf-Kb-linked tnf-A Expression. J Med Food. (2019) 10:1022–31. doi: 10.1089/jmf.2019.4419
43. Wolfarth AA, Smith TM, Vaninsberghe D, Dunlop AL, Neish AS, Corwin EJ, et al. A human microbiota-associated murine model for assessing the impact of the vaginal microbiota on pregnancy outcomes. Front Cell Infection Microbiol. (2020) 10:570025. doi: 10.3389/fcimb.2020.570025
44. Choi S-I, Won G, Kim Y, Kang C-H, Kim G-H. Lactobacilli strain mixture alleviates bacterial vaginosis through antibacterial and antagonistic activity in gardnerella vaginalis-infected C57bl/6 mice. Microorganisms. (2022) 10:471. doi: 10.3390/microorganisms10020471
45. Quispe Calla N, Vicetti Miguel R, Torres A, Trout W, Gabriel J, Hatfield A, et al. Norethisterone enanthate increases mouse susceptibility to genital infection with herpes simplex virus type 2 and hiv type 1. ImmunoHorizons. (2020) 4:72–81. doi: 10.4049/immunohorizons.1900077
46. Vitali D, Bagri P, Wessels JM, Arora M, Ganugula R, Parikh A, et al. Curcumin can decrease tissue inflammation and the severity of hsv-2 infection in the female reproductive mucosa. Int J Mol Sci. (2020) 21:337. doi: 10.3390/ijms21010337
47. Bagri P, Yu E, Kaushic C. Chapter 13 - Mucosal Immunology of the Female Reproductive Tract and Its Regulation by Female Sex Hormones. In: Mor G, editor. Reprod Immunol. Cambridge, USA: Academic Press (2021). p. 253–76.
48. Kaushic C, Nguyen P. Immunology of the female reproductive mucosa. Encyclopedia Immunol. (2016) 5:63–72. doi: 10.1016/B978-0-12-374279-7.19014-9
49. Field NT, Newton ER, Kagan-Hallet K, Peairs WA. Perinatal effects of gardnerella vaginalis deciduitis in the rabbit. Am J Obstet Gynecol. (1993) 168:988–94. doi: 10.1016/S0002-9378(12)90858-3
50. McDuffie RS, Kunze M, Barr J, Wolf D, Sze C-I, Shikes R, et al. Chronic intrauterine and fetal infection with gardnerella vaginalis. Am J Obstet Gynecol. (2002) 187:1263–6. doi: 10.1067/mob.2002.127129
51. Gilbert NM, O’Brien VP, Lewis AL. Transient microbiota exposures activate dormant escherichia coli infection in the bladder and drive severe outcomes of recurrent disease. PloS Pathog. (2017) 13:e1006238. doi: 10.1371/journal.ppat.1006238
52. Vrbanac A, Riestra AM, Coady A, Knight R, Nizet V, Patras KA. The murine vaginal microbiota and its perturbation by the human pathogen group B streptococcus. BMC Microbiol. (2018) 18:197. doi: 10.1186/s12866-018-1341-2
53. Guerrero-Beltrán C, Garcia-Heredia I, Ceña-Diez R, Rodriguez-Izquierdo I, Serramía MJ, Martinez-Hernandez F, et al. Cationic dendrimer G2-S16 inhibits herpes simplex type 2 infection and protects mice vaginal microbiome. Pharmaceutics. (2020) 12:515. doi: 10.3390/pharmaceutics12060515
54. Parr MB, Parr EL. Intravaginal administration of herpes simplex virus type 2 to mice leads to infection of several neural and extraneural sites. J NeuroVirol. (2003) 9:594–602. doi: 10.1080/13550280390246499
55. Wessels JM, Felker AM, Dupont HA, Kaushic C. The relationship between sex hormones, the vaginal microbiome and immunity in hiv-1 susceptibility in women. Dis Model Mech. (2018) 11(9):035147. doi: 10.1242/dmm.035147
56. Blaskewicz CD, Pudney J, Anderson DJ. Structure and function of intercellular junctions in human cervical and vaginal mucosal epithelia. Biol Reprod. (2011) 85:97–104. doi: 10.1095/biolreprod.110.090423
57. Huber O. Structure and function of desmosomal proteins and their role in development and disease. Cell Mol Life Sci. (2003) 60:1872–90. doi: 10.1007/s00018-003-3050-7
58. Costa-Fujishima M, Yazdanpanah A, Horne S, Lamont A, Lopez P, Farr Zuend C, et al. Nonoptimal bacteria species induce neutrophil-driven inflammation and barrier disruption in the female genital tract. Mucosal Immunol. (2023) 16:341–56. doi: 10.1016/j.mucimm.2023.04.001
59. Cauci S, Culhane JF, Di Santolo M, McCollum K. Among pregnant women with bacterial vaginosis, the hydrolytic enzymes sialidase and prolidase are positively associated with interleukin-1beta. Am J Obstet Gynecol. (2008) 198:132.e1–7. doi: 10.1016/j.ajog.2007.05.035
60. Zevin AS, Xie IY, Birse K, Arnold K, Romas L, Westmacott G, et al. Microbiome composition and function drives wound-healing impairment in the female genital tract. PloS Pathog. (2016) 12:e1005889. doi: 10.1371/journal.ppat.1005889
61. Orfanelli T, Jayaram A, Doulaveris G, Forney LJ, Ledger WJ, Witkin SS. Human epididymis protein 4 and secretory leukocyte protease inhibitor in vaginal fluid. Reprod Sci. (2014) 21:538–42. doi: 10.1177/1933719113503416
62. Adapen C, Réot L, Menu E. Role of the human vaginal microbiota in the regulation of inflammation and sexually transmitted infection acquisition: contribution of the non-human primate model to a better understanding? Front Reprod Health. (2022) 4:992176. doi: 10.3389/frph.2022.992176
63. Bertran T, Brachet P, Vareille-Delarbre M, Falenta J, Dosgilbert A, Vasson MP, et al. Slight pro-inflammatory immunomodulation properties of dendritic cells by gardnerella vaginalis: the “Invisible man” of bacterial vaginosis? J Immunol Res. (2016) 2016:9747480. doi: 10.1155/2016/9747480
64. Garcia Erin M, Kraskauskiene V, Koblinski Jennifer E, Jefferson Kimberly K. Interaction of gardnerella vaginalis and vaginolysin with the apical versus basolateral face of a three-dimensional model of vaginal epithelium. Infection Immun. (2019) 87(4):e00646–18. doi: 10.1128/iai.00646-18
65. Chew T, Taylor KE, Mossman KL. Innate and adaptive immune responses to herpes simplex virus. Viruses. (2009) 1:979–1002. doi: 10.3390/v1030979
66. Mwatelah R, McKinnon LR, Baxter C, Abdool Karim Q, Abdool Karim SS. Mechanisms of sexually transmitted infection-induced inflammation in women: implications for hiv risk. J Int AIDS Soc. (2019) 22 Suppl 6:e25346. doi: 10.1002/jia2.25346
67. Furman D, Campisi J, Verdin E, Carrera-Bastos P, Targ S, Franceschi C, et al. Chronic inflammation in the etiology of disease across the life span. Nat Med. (2019) 25:1822–32. doi: 10.1038/s41591-019-0675-0
68. Schwecht I, Nazli A, Gill B, Kaushic C. Lactic acid enhances vaginal epithelial barrier integrity and ameliorates inflammatory effects of dysbiotic short chain fatty acids and hiv-1. Sci Rep. (2023) 13:20065. doi: 10.1038/s41598-023-47172-y
69. Lennard K, Dabee S, Barnabas SL, Havyarimana E, Blakney A, Jaumdally SZ, et al. Microbial composition predicts genital tract inflammation and persistent bacterial vaginosis in South African adolescent females. Infection Immun. (2017) 86(1):e00410–17. doi: 10.1128/iai.00410-17
70. Anahtar MN, Byrne EH, Doherty KE, Bowman BA, Yamamoto HS, Soumillon M, et al. Cervicovaginal bacteria are a major modulator of host inflammatory responses in the female genital tract. Immunity. (2015) 42:965–76. doi: 10.1016/j.immuni.2015.04.019
71. Gautam R, Borgdorff H, Jespers V, Francis SC, Verhelst R, Mwaura M, et al. Correlates of the molecular vaginal microbiota composition of african women. BMC Infect Dis. (2015) 15:86. doi: 10.1186/s12879-015-0831-1
72. Sabo MC, Lehman DA, Wang B, Richardson BA, Srinivasan S, Osborn L, et al. Associations between vaginal bacteria implicated in hiv acquisition risk and proinflammatory cytokines and chemokines. Sex Transmit Infect. (2020) 96:3–9. doi: 10.1136/sextrans-2018-053949
73. Masson L, Barnabas S, Deese J, Lennard K, Dabee S, Gamieldien H, et al. Inflammatory cytokine biomarkers of asymptomatic sexually transmitted infections and vaginal dysbiosis: A multicentre validation study. Sex Transmit Infect. (2019) 95:5–12. doi: 10.1136/sextrans-2017-053506
74. Manhanzva MT, Abrahams AG, Gamieldien H, Froissart R, Jaspan H, Jaumdally SZ, et al. Inflammatory and antimicrobial properties differ between vaginal lactobacillus isolates from South African women with non-optimal versus optimal microbiota. Sci Rep. (2020) 10:6196. doi: 10.1038/s41598-020-62184-8
75. Trinh HT, Lee IA, Hyun YJ, Kim DH. Artemisia princeps pamp. Essential oil and its constituents eucalyptol and A-terpineol ameliorate bacterial vaginosis and vulvovaginal candidiasis in mice by inhibiting bacterial growth and nf-Kb activation. Planta Med. (2011) 77:1996–2002. doi: 10.1055/s-0031-1280094
Keywords: vaginal microbiota (VMB), Lactobacillus, bacterial vaginosis, herpes simplex virus, inflammation, barrier integrity, mouse models, female reproductive health
Citation: Rahman N, Mian MF, Hayes CL, Nazli A and Kaushic C (2024) G. vaginalis increases HSV-2 infection by decreasing vaginal barrier integrity and increasing inflammation in vivo. Front. Immunol. 15:1487726. doi: 10.3389/fimmu.2024.1487726
Received: 28 August 2024; Accepted: 06 November 2024;
Published: 22 November 2024.
Edited by:
Rosangela Salerno-Goncalves, University of Maryland, United StatesReviewed by:
Scott Michael Baliban, University of Maryland, United StatesZohreh Izadifar, Boston Children’s Hospital and Harvard Medical School, United States
Copyright © 2024 Rahman, Mian, Hayes, Nazli and Kaushic. This is an open-access article distributed under the terms of the Creative Commons Attribution License (CC BY). The use, distribution or reproduction in other forums is permitted, provided the original author(s) and the copyright owner(s) are credited and that the original publication in this journal is cited, in accordance with accepted academic practice. No use, distribution or reproduction is permitted which does not comply with these terms.
*Correspondence: Charu Kaushic, a2F1c2hpY0BtY21hc3Rlci5jYQ==