- 1The First Clinical College, Gannan Medical University, Ganzhou, Jiangxi, China
- 2Department of Urology, The First Affiliated hospital of Gannan Medical University, Ganzhou, Jiangxi, China
- 3Key Laboratory of Urology and Andrology of Ganzhou, Ganzhou, Jiangxi, China
- 4Department of Graduate, The First Affiliated Hospital of Gannan Medical University, Jiangxi, Jiangxi, China
Urologic malignancies, characterized by their high aggressiveness and metastatic potential, pose a significant public health challenge globally. Ferroptosis, a novel mode of cell death, typically arises from intracellular iron ion overload and the accumulation of lipid peroxides. This process has been shown to play a crucial regulatory role in various pathological conditions, particularly in cancer, including urologic cancers. However, the comprehensive regulatory mechanisms underlying ferroptosis remain poorly understood, which somewhat limits its broader application in cancer therapy. Non-coding RNAs (ncRNAs), which encompass microRNAs (miRNAs), long non-coding RNAs (lncRNAs), and circular RNAs (circRNAs), are non-coding transcripts that play pivotal roles in various physiological processes, such as proliferation, differentiation, apoptosis, and cell cycle regulation, by modulating the expression of target genes. The biological functions and potential regulatory mechanisms of ncRNAs in the context of cancer-related ferroptosis have been partially elucidated. Research indicates that ncRNAs can influence the progression of urologic cancers by affecting cell proliferation, migration, and drug resistance through the regulation of ferroptosis. Consequently, this review aims to clarify the functions and mechanisms of the ncRNA-ferroptosis axis in urologic cancers and to evaluate the clinical significance of ferroptosis-related ncRNAs, thereby providing new insights into cancer biology and therapeutic strategies that may ultimately benefit a diverse range of cancer patients.
1 Introduction
Urologic malignancies, including renal cancer, bladder cancer, and prostate cancer, have emerged as critical global public health concerns, with both incidence and mortality rates on the rise. According to the GLOBOCAN 2022 data report, the number of newly diagnosed urologic tumor cases increased from approximately 2.4 million in 2020 to 2.667 million in 2022, accounting for 13.35% of the total global cancer cases (1, 2). Global deaths attributed to urologic malignancies rose from 767,208 to 825,953, corresponding to an 8.4% increase in the share of all cancer-related deaths (1, 2). Prostate cancer ranks as the second most common cancer among men worldwide, following lung cancer, and is the fifth leading cause of cancer-related deaths (1). Bladder cancer, the second most prevalent urogenital malignancy, was the tenth most common cancer globally in 2020 (1, 2). Despite its relatively lower global incidence rate, renal cancer (including renal pelvis cancer) accounted for approximately 553,000 new cases and 208,953 deaths in 2022, underscoring its significance as a major type of urologic cancer (1). Given the trends of global population growth and aging, the incidence of urologic cancers is projected to rise further, posing significant challenges to public health and imposing considerable economic burdens on healthcare systems (3–5).
In recent years, significant advancements have been made in the diagnosis and treatment of diseases affecting the urinary system. However, urologic tumors, particularly those in advanced stages or with metastases, continue to be the leading cause of mortality among affected patients. For individuals with early-stage disease, radical surgery remains the preferred treatment option; nevertheless, challenges in preventing tumor metastasis and recurrence persist. In patients with advanced, unresectable tumors, prognosis is often poor due to their high resistance to chemotherapy and radiotherapy. Therefore, a comprehensive exploration of the molecular mechanisms underlying the initiation and progression of urologic tumors is essential for enhancing prevention efforts and optimizing clinical management strategies.
Ferroptosis, a novel form of iron-dependent programmed cell death, is characterized by its reliance on iron and the accumulation of lipid peroxides, which ultimately lead to cellular membrane rupture and cell death (6). Since its introduction by Dr. Brent R. Stockwell and colleagues in 2012, in their efforts to develop drugs targeting RAS proto-oncogene GTPase (RAS)-mutated cancer cells, dysregulation of the ferroptosis regulatory system has been linked to various physiological and pathological conditions (7, 8). Increasing evidence underscores the significant role of ferroptosis in multiple cancers, including urologic malignancies, thereby positioning it as a potential therapeutic target for these diseases (9–11). In contrast, non-coding RNAs (ncRNAs) are RNA molecules that do not directly participate in protein synthesis. Although they do not encode proteins, ncRNAs perform essential regulatory functions within cells and are involved in various biological processes, including development, proliferation, transcription, post-transcriptional modification, apoptosis, and cellular metabolism (12). NcRNAs are primarily categorized into three types: circular RNAs (circRNAs), long non-coding RNAs (lncRNAs), and microRNAs (miRNAs) (13). These ncRNA molecules exhibit tissue- and disease-specific expression patterns, indicating their potential as biomarkers for disease assessment (14). Recent studies have uncovered abnormal expression patterns of ncRNAs in various diseases, particularly in cancers, including urologic malignancies (15–18). In some instances, ncRNAs influence tumor progression by modulating ferroptosis pathways, highlighting their potential as therapeutic targets for regulating these pathways in cancer cells and thus providing new opportunities to enhance patient prognosis (19–22).
In summary, this review aims to explore the critical roles of ncRNAs and ferroptosis in the progression of urologic malignancies and their potential utility as biomarkers and targets for therapeutic intervention. By systematically evaluating and integrating recent research findings in related fields, this review aims to advance the understanding of the complex roles of ncRNAs in regulating ferroptosis-related pathways. Ultimately, this review aims to provide novel insights and strategies for the prevention, diagnosis, and treatment of urologic malignancies.
2 The current status of ferroptosis regulation mechanisms
Programmed cell death (PCD) is a complex and finely regulated biological process. It represents an active mechanism of cell death governed by genetic factors, intricately linked to the maintenance of organismal homeostasis and the onset of disease. PCD encompasses several distinct forms, including apoptosis, pyroptosis, necrosis, autophagy, and ferroptosis (23, 24). Ferroptosis, a unique form of cell death, was first identified in 2003 during experiments designed to screen small molecules for selective tumor cell killing and was formally designated by Stockwell et al. in 2012 (7, 25). Its core mechanism involves the disruption of intracellular redox homeostasis, particularly the depletion of glutathione (GSH) and the reduction of glutathione peroxidase 4 (GPX4) activity. This disruption impairs GPX4’s ability to effectively metabolize lipid peroxides, thereby triggering Fe²+-mediated lipid peroxidation reactions. This cascade ultimately leads to increased levels of reactive oxygen species (ROS), which are essential for the induction of ferroptosis (26). As a distinct mode of cell death, ferroptosis holds substantial scientific value in cell biology research and therapeutic applications. Further investigation into its mechanisms promises to provide new insights into prevention and treatment strategies for related diseases (Figure 1).
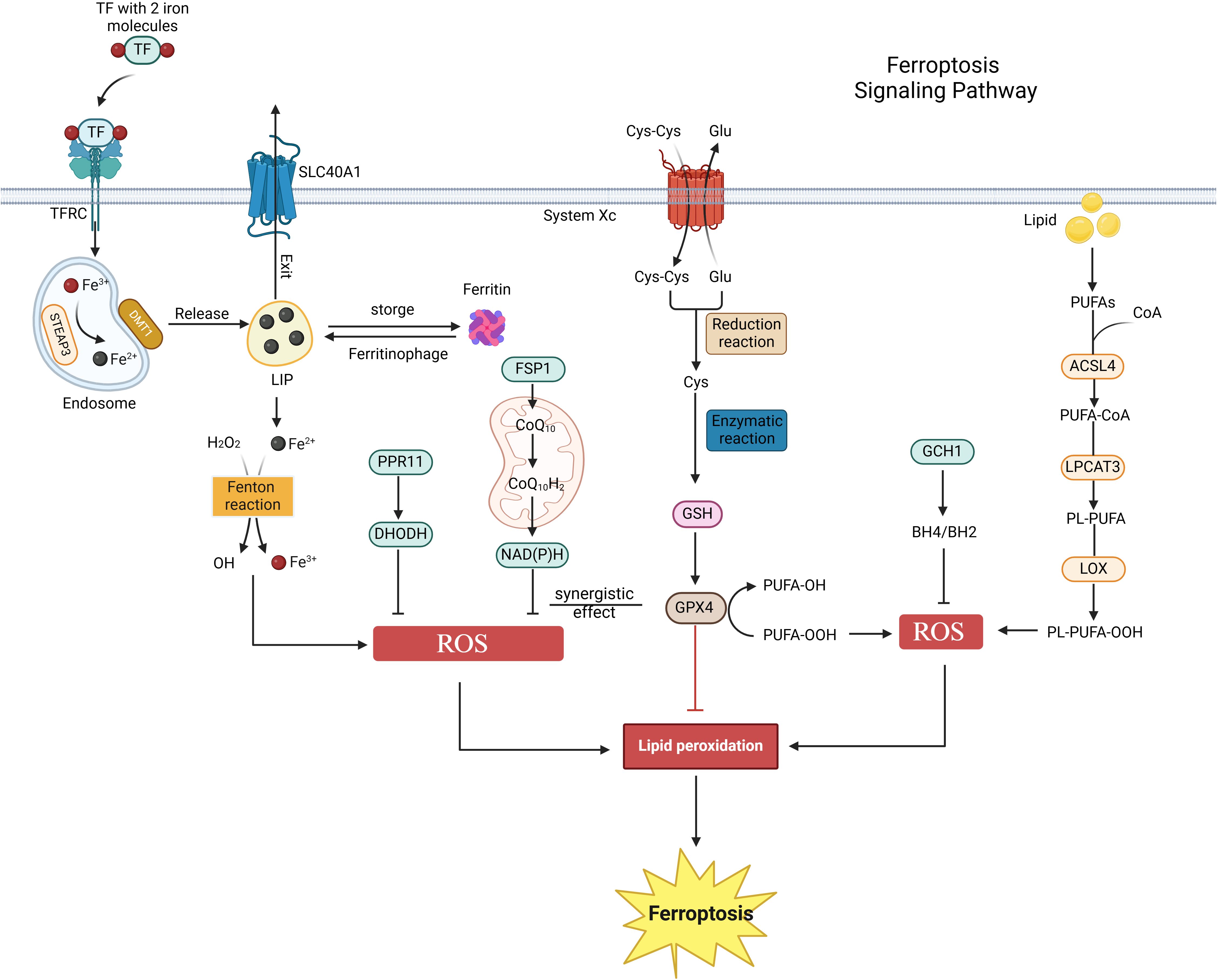
Figure 1. Ferroptosis signaling pathway. Ferroptosis is primarily regulated by iron metabolism, amino acid metabolism, and lipid metabolism. Initially, extracellular iron ions bind to TF/TFRC, subsequently entering the cytoplasm to form endosomes. These ions then enter the labile iron pool (LIP) via the actions of STEAP3 metal reductase and DMT1. Ferritin regulates the content of the LIP through autophagy or storage, whereas excess iron ions are expelled from cells via SLC40A1. The Fenton reaction involving Fe²+ and hydrogen peroxide generates reactive oxygen species (ROS), which promote ferroptosis. Secondly, cystine enters the cell via System Xc- and is converted to glutathione (GSH) through reduction and enzymatic reactions. The GSH-GPX4 axis functions as an antioxidant defense system in vivo, inhibiting ferroptosis. Lastly, polyunsaturated fatty acids (PUFA) in lipids are enzymatically converted into PL-PUFA-OOH, promoting ferroptosis. Additionally, the FSP1-CoQ10-NAD(P)H, PPR11-DHODH, and GCH1-BH4 pathways also regulate ferroptosis.
2.1 Iron metabolism and ferroptosis
A strong connection exists between iron metabolism homeostasis and ferroptosis. Within cells, hydrogen peroxide (H2O2) and iron ions (Fe²+) react through the Fenton reaction under acidic conditions, generating hydroxyl radicals (OH-), which are among the most destructive oxidants in reactive oxygen species (ROS) (27). These highly reactive ROS can initiate a series of oxidative reactions, including lipid peroxidation, DNA damage, and protein oxidation, ultimately leading to cell death (28). In the plasma, iron enters cells as Fe³+, bound to transferrin and its receptor (TF/TFRC), and is subsequently reduced to Fe²+ by the action of STEAP3 metalloreductase (29). Excess Fe²+ is expelled from cells via solute carrier family 40 member 1 (SLC40A1) and is oxidized back to Fe³+ during this process (30). Iron ions (Fe²+/Fe³+), as primary inducers of ferroptosis, can promote ROS generation through both enzymatic and non-enzymatic reactions (31). Studies have demonstrated that iron chelators can reduce free iron levels, inhibit lipid peroxidation, and thus suppress ferroptosis (32). Consequently, the regulation of iron uptake, utilization, storage, and efflux in cells and tissues can significantly influence susceptibility to ferroptosis (33).
2.2 Amino acid metabolism and ferroptosis
The glutathione-GPX4 axis in amino acid metabolism plays a crucial role in maintaining cellular homeostasis. System Xc-, composed of the light chain SLC7A11 and the heavy chain SLC3A2, mediates the reverse transport of intracellular glutamate and extracellular cystine at a 1:1 ratio. In this process, cystine is reduced to cysteine, which is a vital precursor for the synthesis of GSH (25). GSH serves as a non-enzymatic antioxidant within cells, essential for protecting against oxidative stress damage. The glutathione peroxidase family (GPX) consists of a group of antioxidant enzymes widely distributed in mammals, primarily comprising eight members, namely GPX1 through GPX8 (34). A common characteristic of these enzymes is their ability to catalyze the conversion of organic hydroperoxides (ROOH) or H2O2 into water and corresponding alcohols, thereby effectively eliminating intracellular reactive oxygen species (ROS) or lipid peroxides and safeguarding cells from oxidative stress damage (34, 35). Although all members of the GPX family contribute to the anti-oxidative stress process, GPX4 plays an irreplaceable role in ferroptosis, a specific form of cell death. GPX4 utilizes GSH as a reducing agent to directly act on lipid hydroperoxides present in the cell membrane, transforming them into innocuous alcohols, thereby inhibiting lipid peroxidation and preserving the integrity of the cell membrane (36). Consequently, the glutathione-GPX4 axis represents a critical antioxidant defense system in the body. Classical ferroptosis inducers, such as Erastin and Ras-selective lethal small molecule 3 (RSL3), specifically target System Xc- and GPX4, respectively, to exert their effects (37–39). Erastin binds to SLC7A11, blocking its transport function, which leads to impaired cystine transport and reduced GSH production. This results in the accumulation of lipid peroxides, inducing ferroptosis (40). RSL3 forms a covalent bond with GPX4, rendering it inactive, disrupting the cellular redox balance, promoting lipid peroxidation, and inducing ferroptosis (41).
2.3 Lipid metabolism and ferroptosis
Furthermore, lipid metabolism is intricately linked to ferroptosis. Lipid peroxidation predominantly occurs in membrane phospholipids that are rich in polyunsaturated fatty acids (PUFAs) (42, 43). Specific PUFAs, particularly those found in phosphatidylethanolamine (PE) and phosphatidylcholine (PC), serve as principal targets in ferroptosis (7, 43). ROS or lipoxygenases (LOXs) oxidize non-toxic PUFAs (PL-PUFAs) into toxic lipid peroxides (PL-PUFA-OOH), thereby inducing ferroptosis (32). Key enzymes in the lipid metabolism pathway, such as ACSL4 and LPCAT3, play a crucial role in regulating cellular lipid composition and sensitivity to ferroptosis (42, 44, 45). ACSL4 catalyzes the condensation of free PUFA with coenzyme A (CoA) to form derivatives, which are subsequently esterified into membrane phospholipids by LPCAT3. This process facilitates the onset of ferroptosis (46, 47). Therefore, supplementing PL-PUFA or inhibiting enzymes such as ACSL4 and LPCAT3 can modulate cellular sensitivity to ferroptosis (48–50).
2.4 Other parallel pathways and ferroptosis
Moreover, recent studies have identified several parallel pathways involved in ferroptosis. FSP1 is a novel ferroptosis resistance gene that operates under conditions of GPX4 deficiency or RSL3 inhibition. It is recruited to the plasma membrane through N-myristoylation, functioning as an NAD(P)H-dependent reductase that converts coenzyme Q10 to ubiquinol-10, thereby preventing the accumulation of lipid peroxides (51). The FSP1-CoQ10-NAD(P)H pathway acts as an independent parallel system that collaborates with GPX4 and glutathione to inhibit phospholipid peroxidation and ferroptosis (52). Conversely, DHODH, an enzyme that catalyzes the conversion of dihydroorotic acid to orotic acid, operates parallel to mitochondrial GPX4, inhibiting ferroptosis of the mitochondrial inner membrane by reducing ubiquinone (CoQ) to panalcohol (CoQH) (53). Recent studies have demonstrated that the PRR11-DHODH axis plays a crucial role in regulating ferroptosis in tumor cells. Miao et al. reported that PRR11 maintains the stability of DHODH by inhibiting its polyubiquitination-mediated degradation, thereby driving ferroptosis and drug resistance in glioblastoma (54). Additionally, genome-wide activation screening has identified a group of genes that can counteract ferroptosis, including GCH1 and its metabolites BH4/BH2 (55). GCH1-expressing cells synthesize BH4/BH2 through lipid remodeling to prevent phospholipid depletion and inhibit ferroptosis, a mechanism that operates independently of the GPX4/glutathione system (55). In conclusion, the regulatory mechanisms of ferroptosis remain to be fully elucidated, and their potential impacts on pathophysiological processes, particularly in urologic malignancies, warrant further investigation.
3 The role of ferroptosis in urological cancers
In recent years, ferroptosis has garnered significant attention in the field of cancer research, primarily due to its unique mechanisms and morphological characteristics (56). As research has progressed, the relationship between ferroptosis and malignant urinary system tumors has increasingly become a focal point of investigation. Relevant studies from the past year are summarized in Table 1. In urologic malignancies, various cancer-related signaling pathways regulate ferroptosis in tumor cells (57, 58). For instance, the phosphatidylinositol 3-kinase (PI3K)-AKT signaling pathway influences cell migration and invasion. In renal cell carcinoma, abnormal activation of this pathway promotes cancer cell migration and invasion, thereby accelerating tumor metastasis (59, 60). Hao et al. demonstrated that the combination of the fatty acid amide hydrolase (FAAH) inhibitor URB597 with the ferroptosis inducer RSL3 effectively modulates the PI3K-AKT signaling pathway, enhancing RCC cell sensitivity to ferroptosis and significantly inhibiting tumor growth and metastasis (61). Furthermore, dipeptidyl peptidase 9 (DPP9) competes with NRF2 for binding to KEAP1 in an enzyme-independent manner, thereby disrupting the KEAP1-NRF2 signaling pathway and influencing tumorigenesis and drug resistance in clear cell renal carcinoma (ccRCC) (11). The interaction between ferroptosis and tumor suppressor genes during tumor development serves as a natural defense mechanism (62). Conversely, ferroptosis escape mechanisms mediated by oncogenes or specific signaling pathways contribute to tumor initiation, progression, metastasis, and drug resistance (63–65). As a crucial tumor suppressor gene, P53 plays a key role in regulating the cell cycle, DNA repair, apoptosis, and metabolic pathways (66). In bladder cancer, P53 inhibits the activity of SLC7A11 (62), a critical component of System Xc- on the cell membrane, which is closely associated with ferroptosis (25). Inhibition of SLC7A11 activates ALOX15B lipoxygenase, which subsequently induces ferroptosis in BC cells (62).
It is noteworthy that certain tumor cells exhibit intrinsic sensitivity to ferroptosis due to their unique metabolic characteristics, elevated levels of ROS, and specific gene mutations. This sensitivity reveals potential therapeutic vulnerabilities in particular cancer types (67, 68). Therefore, ferroptosis is recognized as one of the key mechanisms of cell death induced by various cancer therapies, including radiotherapy, immunotherapy, chemotherapy, and targeted therapies (69–72). Multiple studies have demonstrated that ferroptosis can enhance the sensitivity of urinary system cancers to chemotherapy through the modulation of associated pathways (73–75). For instance, Wang et al. found that absent in melanoma 2 (AIM2) can promote the progression of renal cell carcinoma and sunitinib resistance independently of the inflammasome (73). Mechanistic studies revealed that AIM2 facilitates the phosphorylation and proteasomal degradation of FOXO3a, subsequently inhibiting the transcriptional activity of ACSL4. This inhibition of ACSL4 contributes to ferroptosis resistance and sunitinib resistance in kidney cancer. Interestingly, the ferroptosis inducer RSL3 demonstrated a synergistic effect with sunitinib, suggesting that targeting ferroptosis may represent a novel approach for treating sunitinib-resistant kidney cancer (73). Additionally, docetaxel resistance in prostate cancer and cisplatin resistance in bladder cancer are also closely linked to ferroptosis (74, 75). Therefore, an in-depth exploration of the molecular mechanisms underlying ferroptosis and its intricate regulatory network is essential for developing effective treatment strategies for malignant urinary system tumors.
4 Non-coding RNA targeted regulation of ferroptosis
Non-coding RNA (ncRNA), a distinct class of RNA molecules that do not directly encode proteins, plays a crucial role in biological processes at the RNA level and is extensively involved in regulating gene expression (94). Among these ncRNAs, microRNA (miRNA), circular RNA (circRNA), and long non-coding RNA (lncRNA) are key regulatory molecules currently under extensive investigation. These molecules influence gene expression through various mechanisms, including transcriptional regulation, post-transcriptional regulation, and epigenetic modifications (95).
MiRNAs are a class of non-coding, single-stranded RNA molecules approximately 21-23 nucleotides in length, encoded by endogenous genes (96, 97). They induce cleavage, degradation, or translation inhibition of target mRNA through complementary binding to the 3’-UTR region of the target mRNA, thereby inhibiting the expression of target genes (97, 98). Recent studies have demonstrated that various miRNAs can regulate ferroptosis by targeting ferroptosis-related genes. For instance, miR-545 suppresses its expression by binding to TF mRNA, which reduces iron uptake and subsequently inhibits ferroptosis while promoting CRC cell survival (99). Conversely, miR-30b-5p and miR-124 diminish iron excretion by inhibiting the expression of FPN1 (an iron export protein), thereby promoting ferroptosis (100, 101). LncRNAs are a class of non-coding RNA molecules that exceed 200 nucleotides in length (102). They regulate gene expression at the epigenetic level through various mechanisms, including chromatin remodeling, transcriptional regulation, translation regulation, and post-translational modification (103). For instance, Wang et al. found that lncRNA-LINC00618 reduces SLC7A11 transcription in leukemia, thereby regulating ferroptosis at the transcriptional level (104). Additionally, lncRNAs can function as competitive endogenous RNAs (ceRNAs). They act as “sponges” for microRNAs (miRNAs), absorbing miRNAs and reducing their inhibitory effects on target mRNAs, thereby indirectly upregulating target gene expression (105, 106). For example, lncRNA-NEAT1 enhances MIOX expression by competitively binding to miR-362-3p, thereby increasing ferroptosis and offering a potential strategy for improving chemotherapy sensitivity in hepatocellular carcinoma (HCC) patients (107). CircRNAs are another class of non-coding RNA molecules characterized by a closed ring structure, lacking a 5’-terminal cap and a 3’-terminal poly(A) tail (108, 109). Current studies indicate that circRNAs primarily regulate ferroptosis by modulating downstream gene expression as miRNA sponges. Wang et al. reported that circ_0067934 attenuates ferroptosis and promotes cell proliferation by targeting the miR-545-3p/SLC7A11 signaling pathway in thyroid cancer cells (110). CircIL4R binds to miR-541-3p, inhibiting miR-541-3p and enhancing GPX4, thereby suppressing ferroptosis in hepatocellular carcinoma (111). Numerous studies have confirmed that circRNAs can function as ceRNAs to regulate ferroptosis (112–114).
Existing studies have demonstrated that ncRNAs regulate ferroptosis by targeting metabolic mechanisms associated with this process, with recent reviews summarizing this area of research (115–118). Overall, ferroptosis is influenced by iron metabolism, lipid metabolism, and amino acid metabolism. Numerous proteins, particularly transcription factors, modulate ferroptosis either directly or indirectly through the regulation of these metabolic pathways. For instance, in the iron metabolism pathway, iron uptake is mediated through interactions between transferrin (TF) and transferrin receptor (TFRC), as well as the reduction of STEAP3. Ferritin, heat shock protein β-1 (HSPB1), and iron response element binding protein 2 (IREB2) facilitate iron utilization and export via iron transporter 1 (FPN, also known as SLC40A1) (119–123). Overall, ferroptosis is influenced by iron metabolism, lipid metabolism, and amino acid metabolism. Numerous proteins, particularly transcription factors, modulate ferroptosis either directly or indirectly through the regulation of these metabolic pathways. For instance, in the iron metabolism pathway, iron uptake is mediated through interactions between transferrin (TF) and transferrin receptor (TFRC), as well as the reduction of STEAP3. Ferritin, heat shock protein β-1 (HSPB1), and iron response element binding protein 2 (IREB2) facilitate iron utilization and export via iron transporter 1 (FPN, also known as SLC40A1) (42, 44, 45, 124, 125). NcRNAs primarily regulate ferroptosis through interactions with key enzymes involved in these enzymatic reactions. Amino acid metabolism is critically dependent on the stable function of System Xc-. SLC7A11 and SLC3A2 are crucial components of System Xc-, responsible for transporting glutathione (GSH). GPX4 utilizes GSH as a reducing agent and serves as a key protective enzyme in the antioxidant defense system (36). Currently, most ncRNAs predominantly target SLC7A11 and GPX4 mRNA to modulate amino acid metabolism, thereby influencing ferroptosis. Additionally, ncRNAs can affect other regulators of ferroptosis, such as NRF2, p53, and FSP1 (126–128).
In conclusion, ncRNAs possess significant potential in modulating ferroptosis, with mechanisms of action that are both intricate and varied. As research progresses, it is anticipated that the detailed roles and mechanisms of ncRNAs in ferroptosis will be elucidated, potentially leading to novel strategies for the prevention and treatment of related diseases. Table 2 presents information regarding the regulation of ferroptosis by ncRNAs, thereby enhancing our understanding of the research advancements in this domain.
5 Non-coding RNAs target ferroptosis in urologic cancers
5.1 MiRNA-ferroptosis axis in urologic tumors
In the complex pathophysiological mechanisms underlying urinary system cancers, the interplay between miRNAs and ferroptosis has increasingly emerged as a focal point of scientific investigation. MiRNAs, a class of short, non-coding RNAs, play a significant role in regulating cellular physiological and pathological processes through the precise modulation of target gene expression (146). Ferroptosis, an emerging mode of cell death, is characterized by the regulation of intracellular iron metabolism and lipid peroxidation; its role in cancer has been progressively elucidated. In the context of urinary system cancers, the relationship between miRNAs and ferroptosis is particularly noteworthy. Studies have demonstrated that several miRNAs act as key regulators of ferroptosis-related genes, thereby influencing the survival and proliferation of cancer cells by modulating the ferroptosis process. For example, Huang et al. identified that miR-217, derived from bladder cancer (BC) tissue exosomes, plays a crucial role in inhibiting ferroptosis in BC. Functionally, the introduction of miR-217 into cells resulted in a reduction of malondialdehyde (MDA), lactate dehydrogenase (LDH), and Fe²+ levels, while simultaneously increasing the level of superoxide dismutase (SOD), thus significantly inhibiting both ferroptosis and apoptosis (147). This finding elucidates the potential mechanisms by which miRNAs regulate ferroptosis in urinary system tumors.
Further exploration reveals that the mechanistic role of the miRNA-ferroptosis axis in urinary system tumors operates at multiple levels. On one hand, miRNAs can directly target crucial genes in the ferroptosis pathway, such as ferritin heavy chain 1 (FTH1) and solute carrier family 40 member 1 (SLC40A1), which are essential for maintaining intracellular redox balance and iron homeostasis (148, 149). The significant role of NRF2 and its target genes in preventing ferroptosis positions this pathway as a promising target for cancer treatment. The NRF2/FTH1 axis is critical for maintaining cellular iron metabolism balance (150). In prostate cancer, miR-29a-5p modulates ferroptosis by influencing the levels and accumulation of intracellular Fe2+ and MDA via the NRF2/FTH1 axis (148). Knocking down or inhibiting miR-29a-5p can activate ferroptosis, thereby suppressing the proliferation of PC cells both in vitro and in vivo, providing new insights for subsequent cancer treatment (148). Similarly, miR-4735-3p impedes the growth of clear ccRCC cells by binding to the 3’-UTR of SLC40A1 and downregulating its expression, which resultsing in iron overload and ferroptosis (149). Conversely, miRNAs can mediate interactions between tumor cells and macrophages, thereby indirectly regulating ferroptosis (151). Xiao et al. found that tumor-associated macrophages secrete taurine to inhibit ferroptosis by activating the LXRα/SCD1 axis in PC. Mechanistically, LXRα promotes ferroptosis through its downstream target, stearoyl-CoA desaturase 1 (SCD1), which is recognized as a ferroptosis suppressor gene; its overexpression inhibits ferroptosis. Concurrently, LXRα can upregulate the expression of miR-181a-5p and the RNA-binding protein FUS, promoting the enrichment of miR-181a-5p in tumor-derived exosomes. These miR-181a-5p-enriched exosomes can subsequently modulate macrophage M2 polarization and upregulate the expression of the taurine transporter gene TauT by targeting the Hippo-YAP pathway. This process increases taurine secretion and further inhibits ferroptosis in PC. In summary, the study by Xiao et al. demonstrates that miR-181a-5p can shuttle from tumor cells to macrophages, enhancing taurine secretion as a feedback mechanism and thereby facilitating crosstalk between macrophages and tumor cells (151). This finding provides a novel perspective on the role of miRNAs in mediating interactions between tumor cells and immune cells.
The miRNA-ferroptosis axis demonstrates significant potential in the treatment of urinary system tumors. Acquired chemotherapy resistance frequently occurs in advanced tumors, complicating treatment efforts. Since ferroptosis acts as a tumor suppressor in cancer development, its activation may represent an effective anticancer strategy (152). Research has shown that cancer-associated fibroblast (CAF)-derived exosomes can impede ROS accumulation in prostate cancer cells and reduce mitochondrial damage, thereby inhibiting iron-induced ferroptosis in these cells and promoting acquired chemotherapy resistance (153). Zhao et al. found that miR-432-5p within CAF-derived exosomes inhibits the ferroptosis process by targeting CHAC1 expression, thereby enhancing the resistance of prostate cancer cells to docetaxel. Notably, the knockdown of miR-432-5p expression in CAFs significantly increases tumor sensitivity to chemotherapy (153). Consequently, a comprehensive investigation of the miR-432-5p/CHAC1 axis may provide a promising strategy to counteract acquired chemotherapy resistance in advanced tumors. Moreover, certain anti-tumor compounds have been shown to impact the miRNA-ferroptosis axis. Icariin II (ICS II), an active flavonoid derived from the traditional Chinese medicine Epimedium, exhibits anti-tumor activity across various cancers (154, 155). Yu et al. demonstrated that ICS II inhibits the proliferation, migration, and invasion of RCC cells (156). Functionally, ICS II promotes the accumulation of Fe2+, MDA, and ROS in RCC cells while reducing glutathione (GSH) levels, thereby inducing ferroptosis. Mechanistically, ICS II downregulates GPX4 expression through miR-324-3p, and this pathway operates independently of p53. Consequently, ICS II may serve as a promising therapeutic agent for RCC by inducing ferroptosis in RCC cells via its action on the miR-324-3p/GPX4 axis (156). Further research has indicated that the combined application of icariin (ICA) and curcumin produces a synergistic effect in inducing apoptosis and ferroptosis, significantly inhibiting the viability and proliferation of PC cells (157). Both compounds affect lipid metabolism in PC by modulating the miR-7/mTOR/SREBP1 pathway, thereby influencing autophagy and ferroptosis (157).
In the progression of urinary system tumors, miRNAs modulate ferroptosis by targeting ferroptosis-related genes, thereby influencing tumor cell proliferation, invasion, and immune modulation (Figure 2). The aforementioned studies have identified promising avenues for the development of novel ferroptosis-regulating targets, including the miRNA-217, miR-29a-5p/NRF2/FTH1 axis, miR-4735-3p/SLC40A1 axis, and the miR-181a-5p/Hippo-YAP signaling pathway. These targets have the potential to impede the progression of urinary system tumors. In the realm of cancer therapy, precise control of ferroptosis through pathways such as miR-432-5p/CHAC1, miR-324-3p/GPX4, and miR-7/mTOR/SREBP1 presents a promising strategy to enhance the efficacy of drug treatments. This approach not only supports ongoing research into therapeutic interventions but also lays the groundwork for the development of innovative anticancer therapies. Consequently, there is an urgent need to further explore the crucial role of the miRNA-ferroptosis axis in urinary system cancers, as it may enrich our understanding of tumorigenesis and unlock the potential to transform cancer treatment, ultimately enhancing patient outcomes.
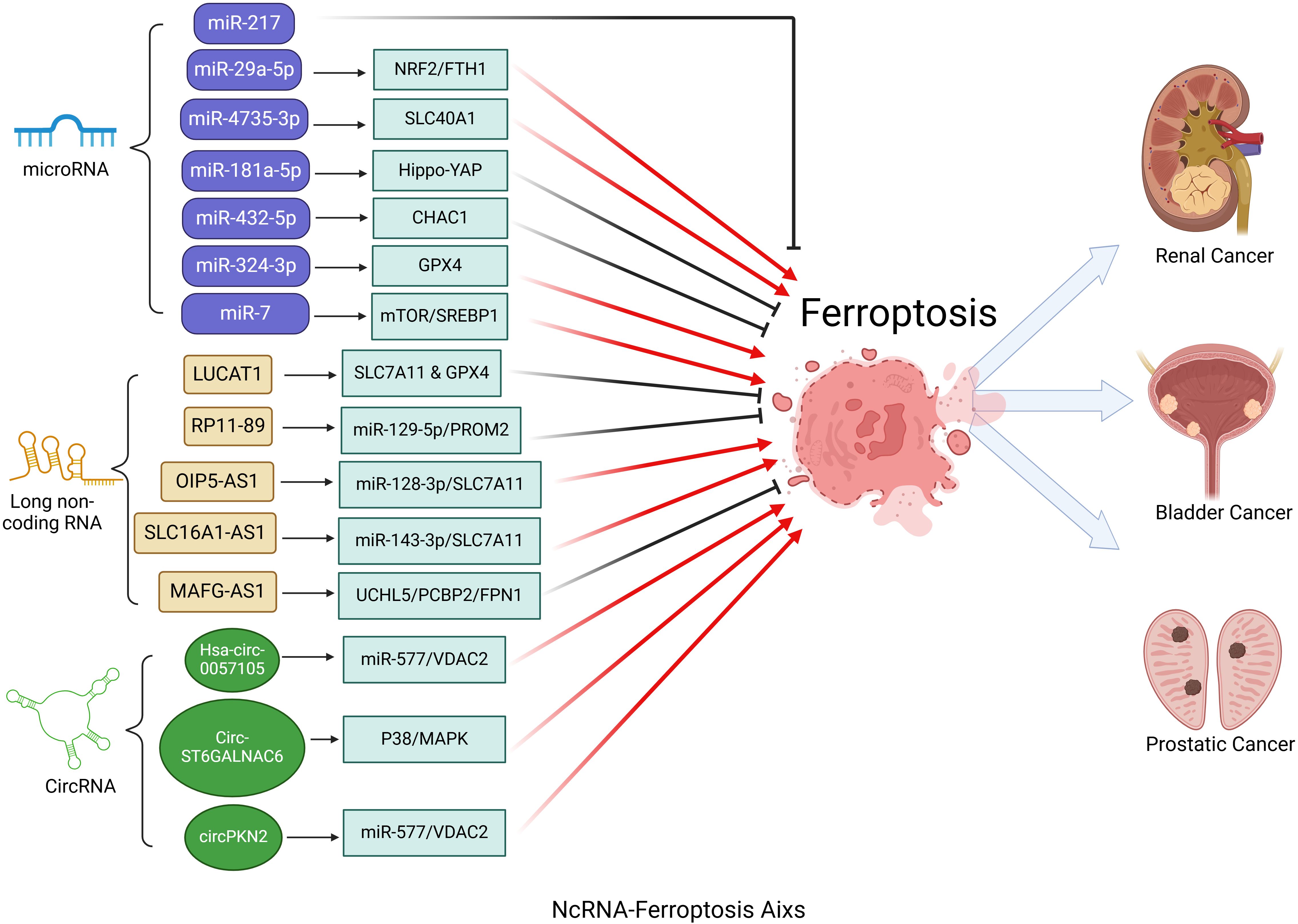
Figure 2. Critical role of the ncRNA-ferroptosis axis in urologic cancers. MicroRNAs (miRNAs) influence ferroptosis in urologic cancers by regulating genes associated with ferroptosis. Long non-coding RNAs (lncRNAs) and circular RNAs (circRNAs) regulate ferroptosis primarily by targeting specific microRNAs (miRNAs) through a sponge effect.
5.2 LncRNA-ferroptosis axis in urologic tumors
Given the ability of long non-coding RNAs (lncRNAs) to regulate gene expression at multiple levels—including chromatin remodeling, transcription, translation, and post-translational regulation—the lncRNA-ferroptosis axis in urinary system cancers has been the subject of extensive research (103). Primarily, lncRNAs regulate ferroptosis in urinary system tumors through gene transcription. For instance, the high expression of lncRNA-LUCAT1 significantly inhibits ferroptosis in bladder cancer (BC) (158). Cao et al. reported that LUCAT1 binds to the m6A reader IGF2BP1 to form a LUCAT1-IGF2BP1 complex, which stabilizes STAT3 mRNA and inhibits the expression of ferroptosis-related genes, including down-regulation of COX2, ACSL4, and NOX1, as well as up-regulation of SLC7A11 and GPX4. This mechanism promotes the migration and invasion of BC cells (158). Additionally, lncRNA MAFG-AS1 also inhibits ferroptosis in bladder cancer by modulating iron metabolism-related proteins (159). Further investigations have shown that high expression of MAFG-AS1 correlates with poor prognosis in bladder cancer patients, while those with low expression exhibit greater sensitivity to adjuvant chemotherapy involving cisplatin and gemcitabine. In conclusion, this study highlights the critical role of the MAFG-AS1/UCHL5/PCBP2/FPN1 axis in cisplatin resistance in bladder cancer, suggesting that inhibition of MAFG-AS1 may promote ferroptosis and enhance cellular sensitivity to cisplatin treatment (159). Second, lncRNA functions as a ceRNA that regulates ferroptosis in urinary system cancers (160–162). LncRNA can exert a “sponge effect” on miRNA, thereby promoting the upregulation of related target gene expression. An illustrative example is lncRNA RP11-89 in BC, which upregulates the expression of PROM2 by sponging miR-129-5p, consequently activating iron output and inhibiting ferroptosis in BC. This finding elucidates the novel role of RP11-89 as an oncogene and suggests the potential for treating BC by modulating the miR-129-5p/PROM2 axis (160). Similarly, lncRNA OIP5-AS1 and SLC16A1-AS1 regulate ferroptosis through the miR-128-3p/SLC7A11 and miR-143-3p/SLC7A11 signaling axes in prostate cancer and kidney cancer, respectively, further confirming the extensive role of lncRNA in regulating ferroptosis in urinary system cancers (161, 162).
As research on urinary system cancers deepens, an expanding body of evidence indicates that ncRNAs, particularly ferroptosis-associated lncRNAs (FRLs), play a significant role in the occurrence, development, and prognosis of these malignancies. Bioinformatics analyses further underscore the considerable potential of FRLs as prognostic markers for urological cancers.
In 2021, Xing et al. first identified that three FRLs—DUXAP8, LUCAT1, and LINC02609—were significantly associated with the overall survival (OS) of renal clear cell carcinoma (163). The risk assessment model developed using these FRLs can accurately predict ccRCC prognosis and offers novel approaches for cancer prognosis screening. This finding not only enhances our understanding of ccRCC prognosis but also provides a critical foundation for the development of personalized treatment options. In a subsequent study, Lai et al. integrated data from the TCGA and FerrDb databases, screening a total of 433 FRLs. They conducted univariate Cox regression, Lasso regression, and multivariate Cox regression analyses, identifying eight FALs (LINC00460, AC124854.1, AC084876.1, IGFL2-AS1, LINC00551, AC083967.1, AC073487.1, and LINC02446) as prognostic features of ccRCC (164). They developed a risk assessment model, FLPS, using these eight FALs. This model not only demonstrates independent predictive value for ccRCC but also elucidates how lncRNAs involved in FLPS influence the progression and survival outcomes of ccRCC. Furthermore, they found that FLPS was closely related to immune infiltration, suggesting the potential therapeutic value of immune checkpoint inhibitors for high-risk ccRCC patients. This discovery provides a new strategy and direction for immunotherapy in ccRCC (164). In renal papillary cell carcinoma (KIRP), Wu et al.’s study also revealed the potential of FRLs as biomarkers (165). They identified a specific group of FRLs associated with both the occurrence and progression of KIRP. CASC19, AC090197.1, AC099850.3, AL033397.2, LINC00462, and B3GALT1-AS1 were significantly upregulated in high-risk groups, suggesting their potential role as oncogenic factors. Conversely, LNCTAM34A and AC024022.1 were significantly upregulated in the low-risk group, indicating their potential function as tumor suppressor factors in KIRP. The differential expression patterns of these FRLs in high-risk and low-risk groups imply that they may serve as either tumor-promoting or tumor-suppressing factors. These findings provide a crucial reference for the early diagnosis, prognosis evaluation, and treatment selection of KIRP.
Studies have demonstrated that a variety of functional RNA molecules (FRLs) serve as prognostic markers not only in renal cell carcinoma but also in prostate and bladder cancers (166–168). FRLs influence tumor cell proliferation and apoptosis directly, while also modulating immune cell infiltration and function by regulating the tumor immune microenvironment. This dual role contributes significantly to tumor development and progression. Therefore, the critical importance of FRLs in urinary system cancers must be acknowledged. Identifying FRLs associated with urinary system tumors and developing a prognostic risk assessment model based on these FRLs will facilitate more accurate prognosis predictions and enable the creation of personalized treatment and monitoring plans. This approach not only enhances the relevance and effectiveness of cancer treatment but also improves patients’ quality of life and extends their survival.
The aforementioned studies have illuminated two critical aspects of the lncRNA-ferroptosis axis in urinary system tumors. Firstly, lncRNAs significantly influence tumor progression and treatment response by modulating ferroptosis. Specifically, lncRNAs such as LUCAT1, MAFG-AS1, RP11-89, OIP5-AS1, and SLC16A1-AS1 exert their effects by regulating ferroptosis-related genes, thereby impacting the development and management of urinary system cancers. Notably, the role of lncRNAs as miRNA sponges adds an additional layer of complexity to this regulatory network, underscoring the necessity for a comprehensive understanding of their interactions. Elucidating the precise mechanisms by which these lncRNA-mediated ferroptotic processes contribute to tumorigenesis is essential for enhancing our understanding of cancer development. Secondly, FRLs identified through bioinformatic methods underscore their strong association with patient prognosis. With an anticipated continuous increase in the identification of FRLs, the establishment of more accurate and sensitive prognostic models based on these biomarkers represents a significant advancement for urinary system cancers.
5.3 CircRNA-ferroptosis aixs in urologic tumors
Similar to lncRNAs, circRNAs can regulate the expression of downstream genes by acting as miRNA sponges, thereby influencing the process of ferroptosis in tumors. Although relatively few studies have focused on circRNAs in urinary system cancers, existing research has highlighted their significant potential in this area. Cen et al. demonstrated that circRNA (Has-circ-0057105) functions as a sponge for miR-577 in renal cell carcinoma, modulating the expression of the COL1A1 and VDAC2 genes at both mRNA and protein levels (169). VDAC2 is a nuclear-encoded mitochondrial protein involved in ferroptosis, responsible for regulating the exchange of ions and metabolites between the cytosol and mitochondria (170). Notably, Overexpression of VDAC2 is closely associated with sensitivity to ferroptosis (40, 170). VDAC2 overexpression did not directly induce ferroptosis in their experiments, nor did it influence cell proliferation or levels of intercellular iron, MDA, and GSH. However, when the ferroptosis inducer Erastin was administered to RCC cells, a more pronounced ferroptosis effect was observed in the VDAC2 overexpression group, characterized by iron accumulation, MDA overproduction, and GSH depletion (169). In summary, this study illustrates that Hsa-circ-0057105 regulates ferroptosis sensitivity in RCC through the miR-577/VDAC2 axis, providing a novel target for RCC treatment. Additionally, circRNAs can bind to functional proteins, influence downstream signaling pathways, and regulate ferroptosis. Wang et al. elucidated the mechanism by which circST6GALNAC6 regulates ferroptosis in BC (171). CircST6GALNAC6 interacts with HSBP1, inhibiting its phosphorylation at serine-15, and thereby activating the P38/MAPK signaling pathway to promote ferroptosis (171). Recently, a novel circRNA, circPKN2, has been identified as significantly down-regulated in bladder cancer and closely associated with patient prognosis (172). Subsequent studies have demonstrated that circPKN2 promotes the ubiquitination of SCD1 and inhibits the WNT pathway by recruiting STUB1 in bladder cancer, thereby enhancing ferroptosis in BC cells and inhibiting tumor growth and metastasis (172).
Therefore, the circRNA-ferroptosis axis plays a crucial role in the development of urinary system cancers (Figure 2). A detailed investigation into the specific mechanisms of these circRNAs in ferroptosis may pave the way for novel therapeutic strategies in the treatment of urinary system cancers.
6 Limitations and future perspectives
To the best of our knowledge, this is a review that summarizes the evidence connecting ncRNAs and ferroptosis in urological oncology. As illustrated in Figure 2, ncRNA-mediated ferroptosis may play a crucial role in the malignant transformation of urinary system cancers, significantly impacting cancer cell proliferation, cell cycle progression, migration, invasion, and angiogenesis. Regarding the miRNA-ferroptosis axis, ferroptosis mediated by miR-27, miR-29a-5p, miR-181a-5p, and miR-4735-3p regulates urinary system tumors by influencing cancer cell proliferation. Concurrently, ferroptosis mediated by miR-432-5p, miR-7, and miR-324-3p contributes to cancer therapy by altering tumor sensitivity to drug treatments. However, to date, no miRNA-ferroptosis axis specifically targeting bladder cancer treatment has been identified. For the lncRNA-ferroptosis axis, lncRNAs such as LUCAT1 and MAFG-AS1 directly target ferroptosis-related genes to regulate ferroptosis, thereby influencing bladder cancer development and drug resistance. Conversely, lncRNAs RP11-89, OIP5-AS1, and SLC16A1-AS1 function as miRNA sponges, modulating ferroptosis in the urinary system by targeting corresponding miRNAs. Notably, numerous FRLs have been identified through bioinformatic analyses, demonstrating potential as independent prognostic factors. Prognostic risk models based on these FRLs hold significant clinical value, assisting physicians in accurately assessing patient conditions and formulating personalized treatment plans. Although research on the circRNA-ferroptosis axis remains limited, studies have revealed that ferroptosis mediated by Hsa_circ_0057105, CircST6GALNAC6, and circPKN2 plays important roles in renal and bladder cancers. Future investigations into the circRNA-ferroptosis axis in prostate cancer are anticipated. In light of these findings, targeting ncRNAs for the modulation of ferroptosis may emerge as reliable diagnostic and prognostic biomarkers, as well as promising therapeutic strategies in urological oncology.
When interpreting this comprehensive review, it is essential to acknowledge certain inherent limitations. First, due to the scarcity of clinical studies, further research is necessary to thoroughly elucidate the diagnostic, therapeutic, and prognostic value of ncRNAs and ferroptosis in urinary system cancers. Second, the molecular mechanisms underlying ncRNA-mediated ferroptosis and urinary system tumorigenesis require further clarification and investigation. In addition to targeting genes and corresponding signaling pathways, other factors, such as the tumor microenvironment and tumor immunity, may also play critical roles in the pathogenesis and progression of urinary system cancers. Finally, it is important to note that ncRNAs exhibit dual roles in cancer, acting as both tumor suppressors and promoters in specific urinary system tumors. Consequently, future studies should focus on exploring the specific mechanisms of ncRNAs within ferroptosis signaling pathways, particularly their interactions with vital biological processes such as iron metabolism, lipid peroxidation, and the antioxidant system. By unraveling these interactions, we can gain a deeper understanding of the biological functions of ncRNAs in the progression of urinary system cancers, including cell proliferation, epithelial-mesenchymal transition, and the induction of chemotherapy resistance. Based on the regulatory mechanisms of the ncRNA-ferroptosis axis, novel ferroptosis-inducing therapies are anticipated in the future.
7 Conclusion
This review highlights that ncRNA-mediated ferroptosis is a significant molecular mechanism involved in the onset and progression of urinary system tumors, such as prostate cancer, bladder cancer, and renal cancer. The regulation of ncRNAs, whether through upregulation or downregulation, can either activate or inhibit ferroptosis in these malignancies. By precisely modulating the expression of genes related to ferroptosis, ncRNAs are instrumental in this process, as they influence the metabolic balance of intracellular iron ions, lipid peroxidation reactions, and antioxidant defense systems.
Author contributions
WL: Conceptualization, Writing – original draft, Writing – review & editing. LZ: Data curation, Writing – original draft, Writing – review & editing. PL: Formal analysis, Writing – original draft, Writing – review & editing. TC: Funding acquisition, Writing – original draft, Writing – review & editing. ZJ: Investigation, Writing – original draft, Writing – review & editing. QC: Methodology, Writing – original draft, Writing – review & editing. LC: Project administration, Writing – original draft, Writing – review & editing. LG: Project administration, Writing – original draft, Writing – review & editing. FZ: Software, Writing – original draft, Writing – review & editing. BQ: Conceptualization, Funding acquisition, Supervision, Writing – original draft, Writing – review & editing.
Funding
The author(s) declare that financial support was received for the research, authorship, and/or publication of this article. This study is supported by The Scientific Research Project of National Health Care Commission (HDSL202001057), the Research Project of Jiangxi Provincial Health and Health Commission (SKJP20203656) and the Jiangxi Province 2023 Postgraduate Innovation Special Fund Project (YC2023-S954).
Acknowledgments
All figures are created with BioRender.com.
Conflict of interest
The authors declare that the research was conducted in the absence of any commercial or financial relationships that could be construed as a potential conflict of interest.
Publisher’s note
All claims expressed in this article are solely those of the authors and do not necessarily represent those of their affiliated organizations, or those of the publisher, the editors and the reviewers. Any product that may be evaluated in this article, or claim that may be made by its manufacturer, is not guaranteed or endorsed by the publisher.
References
1. Bray F, Laversanne M, Sung H, Ferlay J, Siegel RL, Soerjomataram I, et al. Global cancer statistics 2022: GLOBOCAN estimates of incidence and mortality worldwide for 36 cancers in 185 countries. CA Cancer J Clin. (2024) 74:229–63. doi: 10.3322/caac.21834
2. Sung H, Ferlay J, Siegel RL, Laversanne M, Soerjomataram I, Jemal A, et al. Global cancer statistics 2020: GLOBOCAN estimates of incidence and mortality worldwide for 36 cancers in 185 countries. CA Cancer J Clin. (2021) 71:209–49. doi: 10.3322/caac.21660
3. Liu J, Dong L, Zhu Y, Dong B, Sha J, Zhu HH, et al. Prostate cancer treatment - China’s perspective. Cancer Lett. (2022) 550:215927. doi: 10.1016/j.canlet.2022.215927
4. Jubber I, Ong S, Bukavina L, Black PC, Compérat E, Kamat AM, et al. Epidemiology of bladder cancer in 2023: A systematic review of risk factors. Eur Urol. (2023) 84:176–90. doi: 10.1016/j.eururo.2023.03.029
5. Siegel RL, Giaquinto AN, Jemal A. Cancer statistics, 2024. CA Cancer J Clin. (2024) 74:12–49. doi: 10.3322/caac.21820
6. Xie Y, Hou W, Song X, Yu Y, Huang J, Sun X, et al. Ferroptosis: process and function. Cell Death Differ. (2016) 23:369–79. doi: 10.1038/cdd.2015.158
7. Dixon SJ, Lemberg KM, Lamprecht MR, Skouta R, Zaitsev EM, Gleason CE, et al. Ferroptosis: an iron-dependent form of nonapoptotic cell death. Cell. (2012) 149:1060–72. doi: 10.1016/j.cell.2012.03.042
8. Dai E, Chen X, Linkermann A, Jiang X, Kang R, Kagan VE, et al. A guideline on the molecular ecosystem regulating ferroptosis. Nat Cell Biol. (2024) 26(9):1447–57. doi: 10.1038/s41556-024-01360-8
9. Bebber CM, Thomas ES, Stroh J, Chen Z, Androulidaki A, Schmitt A, et al. Ferroptosis response segregates small cell lung cancer (SCLC) neuroendocrine subtypes. Nat Commun. (2021) 12:2048. doi: 10.1038/s41467-021-22336-4
10. Ding Y, Chen X, Liu C, Ge W, Wang Q, Hao X, et al. Identification of a small molecule as inducer of ferroptosis and apoptosis through ubiquitination of GPX4 in triple negative breast cancer cells. J Hematol Oncol. (2021) 14:19. doi: 10.1186/s13045-020-01016-8
11. Chang K, Chen Y, Zhang X, Zhang W, Xu N, Zeng B, et al. DPP9 stabilizes NRF2 to suppress ferroptosis and induce sorafenib resistance in clear cell renal cell carcinoma. Cancer Res. (2023) 83:3940–55. doi: 10.1158/0008-5472.Can-22-4001
12. Qu Z, Adelson DL. Evolutionary conservation and functional roles of ncRNA. Front Genet. (2012) 3:205. doi: 10.3389/fgene.2012.00205
13. Ling H, Vincent K, Pichler M, Fodde R, Berindan-Neagoe I, Slack FJ, et al. Junk DNA and the long non-coding RNA twist in cancer genetics. Oncogene. (2015) 34:5003–11. doi: 10.1038/onc.2014.456
14. Kim T, Reitmair A. Non-coding RNAs: functional aspects and diagnostic utility in oncology. Int J Mol Sci. (2013) 14:4934–68. doi: 10.3390/ijms14034934
15. Loganathan T, Doss CG. Non-coding RNAs in human health and disease: potential function as biomarkers and therapeutic targets. Funct Integr Genomics. (2023) 23:33. doi: 10.1007/s10142-022-00947-4
16. Sarkar FH, Li Y, Wang Z, Kong D, Ali S. Implication of microRNAs in drug resistance for designing novel cancer therapy. Drug Resist Updat. (2010) 13:57–66. doi: 10.1016/j.drup.2010.02.001
17. Eptaminitaki GC, Stellas D, Bonavida B, Baritaki S. Long non-coding RNAs (lncRNAs) signaling in cancer chemoresistance: From prediction to druggability. Drug Resist Updat. (2022) 65:100866. doi: 10.1016/j.drup.2022.100866
18. Shen M, Li X, Qian B, Wang Q, Lin S, Wu W, et al. Crucial roles of microRNA-mediated autophagy in urologic Malignancies. Int J Biol Sci. (2021) 17:3356–68. doi: 10.7150/ijbs.61175
19. Jiang W, Xia J, Xie S, Zou R, Pan S, Wang ZW, et al. Long non-coding RNAs as a determinant of cancer drug resistance: Towards the overcoming of chemoresistance via modulation of lncRNAs. Drug Resist Updat. (2020) 50:100683. doi: 10.1016/j.drup.2020.100683
20. Chai B, Ma Z, Wang X, Xu L, Li Y. Functions of non-coding RNAs in regulating cancer drug targets. Acta Biochim Biophys Sin (Shanghai). (2022) 54:279–91. doi: 10.3724/abbs.2022006
21. Matsui M, Corey DR. Non-coding RNAs as drug targets. Nat Rev Drug Discovery. (2017) 16:167–79. doi: 10.1038/nrd.2016.117
22. Wang J, Zhang Z, Zhuang J, Kang D, Song W. CircCOL5A1 is involved in proliferation, invasion, and inhibition of ferroptosis of colorectal cancer cells via miR-1287-5p/SLC7A11. J Biochem Mol Toxicol. (2024) 38:e23772. doi: 10.1002/jbt.23772
23. Liu L, Li H, Hu D, Wang Y, Shao W, Zhong J, et al. Insights into N6-methyladenosine and programmed cell death in cancer. Mol Cancer. (2022) 21:32. doi: 10.1186/s12943-022-01508-w
24. Liu T, Zhu C, Chen X, Guan G, Zou C, Shen S, et al. Ferroptosis, as the most enriched programmed cell death process in glioma, induces immunosuppression and immunotherapy resistance. Neuro Oncol. (2022) 24:1113–25. doi: 10.1093/neuonc/noac033
25. Dolma S, Lessnick SL, Hahn WC, Stockwell BR. Identification of genotype-selective antitumor agents using synthetic lethal chemical screening in engineered human tumor cells. Cancer Cell. (2003) 3:285–96. doi: 10.1016/s1535-6108(03)00050-3
26. Jiang X, Peng Q, Peng M, Oyang L, Wang H, Liu Q, et al. Cellular metabolism: A key player in cancer ferroptosis. Cancer Commun (Lond). (2024) 44:185–204. doi: 10.1002/cac2.12519
27. Henning Y, Blind US, Larafa S, Matschke J, Fandrey J. Hypoxia aggravates ferroptosis in RPE cells by promoting the Fenton reaction. Cell Death Dis. (2022) 13:662. doi: 10.1038/s41419-022-05121-z
28. Graf E, Mahoney JR, Bryant RG, Eaton JW. Iron-catalyzed hydroxyl radical formation. Stringent requirement for free iron coordination site. J Biol Chem. (1984) 259:3620–4. doi: 10.1016/S0021-9258(17)43139-5
29. Lambe T, Simpson RJ, Dawson S, Bouriez-Jones T, Crockford TL, Lepherd M, et al. Identification of a Steap3 endosomal targeting motif essential for normal iron metabolism. Blood. (2009) 113:1805–8. doi: 10.1182/blood-2007-11-120402
30. Li J, Kang R, Tang D. Monitoring autophagy-dependent ferroptosis. Methods Cell Biol. (2021) 165:163–76. doi: 10.1016/bs.mcb.2020.10.012
31. Xu M, Zha H, Han R, Cheng Y, Chen J, Yue L, et al. Cyclodextrin-derived ROS-generating nanomedicine with pH-modulated degradability to enhance tumor ferroptosis therapy and chemotherapy. Small. (2022) 18:e2200330. doi: 10.1002/smll.202200330
32. Stockwell BR, Friedmann Angeli JP, Bayir H, Bush AI, Conrad M, Dixon SJ, et al. Ferroptosis: A regulated cell death nexus linking metabolism, redox biology, and disease. Cell. (2017) 171:273–85. doi: 10.1016/j.cell.2017.09.021
33. Zhang DD. Ironing out the details of ferroptosis. Nat Cell Biol. (2024) 26(9):1386–93. doi: 10.1038/s41556-024-01361-7
34. Brigelius-Flohé R, Maiorino M. Glutathione peroxidases. Biochim Biophys Acta. (2013) 1830:3289–303. doi: 10.1016/j.bbagen.2012.11.020
35. Zhao L, Zong W, Zhang H, Liu R. Kidney toxicity and response of selenium containing protein-glutathione peroxidase (Gpx3) to cdTe QDs on different levels. Toxicol Sci. (2019) 168:201–8. doi: 10.1093/toxsci/kfy297
36. Xie Y, Kang R, Klionsky DJ, Tang D. GPX4 in cell death, autophagy, and disease. Autophagy. (2023) 19:2621–38. doi: 10.1080/15548627.2023.2218764
37. Chen L, Li X, Liu L, Yu B, Xue Y, Liu Y. Erastin sensitizes glioblastoma cells to temozolomide by restraining xCT and cystathionine-γ-lyase function. Oncol Rep. (2015) 33:1465–74. doi: 10.3892/or.2015.3712
38. Yang WS, SriRamaratnam R, Welsch ME, Shimada K, Skouta R, Viswanathan VS, et al. Regulation of ferroptotic cancer cell death by GPX4. Cell. (2014) 156:317–31. doi: 10.1016/j.cell.2013.12.010
39. Liu Y, Wang Y, Liu J, Kang R, Tang D. Interplay between MTOR and GPX4 signaling modulates autophagy-dependent ferroptotic cancer cell death. Cancer Gene Ther. (2021) 28:55–63. doi: 10.1038/s41417-020-0182-y
40. Yagoda N, von Rechenberg M, Zaganjor E, Bauer AJ, Yang WS, Fridman DJ, et al. RAS-RAF-MEK-dependent oxidative cell death involving voltage-dependent anion channels. Nature. (2007) 447:864–8. doi: 10.1038/nature05859
41. Xie Y, Zhu S, Song X, Sun X, Fan Y, Liu J, et al. The tumor suppressor p53 limits ferroptosis by blocking DPP4 activity. Cell Rep. (2017) 20:1692–704. doi: 10.1016/j.celrep.2017.07.055
42. Doll S, Proneth B, Tyurina YY, Panzilius E, Kobayashi S, Ingold I, et al. ACSL4 dictates ferroptosis sensitivity by shaping cellular lipid composition. Nat Chem Biol. (2017) 13:91–8. doi: 10.1038/nchembio.2239
43. Kagan VE, Mao G, Qu F, Angeli JP, Doll S, Croix CS, et al. Oxidized arachidonic and adrenic PEs navigate cells to ferroptosis. Nat Chem Biol. (2017) 13:81–90. doi: 10.1038/nchembio.2238
44. Chen F, Kang R, Liu J, Tang D. The ACSL4 network regulates cell death and autophagy in diseases. Biol (Basel). (2023) 12(6):864. doi: 10.3390/biology12060864
45. Yuan H, Li X, Zhang X, Kang R, Tang D. Identification of ACSL4 as a biomarker and contributor of ferroptosis. Biochem Biophys Res Commun. (2016) 478:1338–43. doi: 10.1016/j.bbrc.2016.08.124
46. Dixon SJ, Winter GE, Musavi LS, Lee ED, Snijder B, Rebsamen M, et al. Human haploid cell genetics reveals roles for lipid metabolism genes in nonapoptotic cell death. ACS Chem Biol. (2015) 10:1604–9. doi: 10.1021/acschembio.5b00245
47. Feng H, Stockwell BR. Unsolved mysteries: How does lipid peroxidation cause ferroptosis? PloS Biol. (2018) 16:e2006203. doi: 10.1371/journal.pbio.2006203
48. Zhang X, Liu Z, Li Z, Qi L, Huang T, Li F, et al. Ferroptosis pathways: Unveiling the neuroprotective power of cistache deserticola phenylethanoid glycosides. J Ethnopharmacol. (2024) 333:118465. doi: 10.1016/j.jep.2024.118465
49. Ryan F, Blex C, Ngo TD, Kopp MA, Michalke B, Venkataramani V, et al. Ferroptosis inhibitor improves outcome after early and delayed treatment in mild spinal cord injury. Acta Neuropathol. (2024) 147:106. doi: 10.1007/s00401-024-02758-2
50. Zhang Q, Zhou J, Zhai D, Jiang Q, Yang M, Zhou M. Gut microbiota regulates the ALK5/NOX1 axis by altering glutamine metabolism to inhibit ferroptosis of intrahepatic cholangiocarcinoma cells. Biochim Biophys Acta Mol Basis Dis. (2024) 1870:167152. doi: 10.1016/j.bbadis.2024.167152
51. Bersuker K, Hendricks JM, Li Z, Magtanong L, Ford B, Tang PH, et al. The CoQ oxidoreductase FSP1 acts parallel to GPX4 to inhibit ferroptosis. Nature. (2019) 575:688–92. doi: 10.1038/s41586-019-1705-2
52. Doll S, Freitas FP, Shah R, Aldrovandi M, da Silva MC, Ingold I, et al. FSP1 is a glutathione-independent ferroptosis suppressor. Nature. (2019) 575:693–8. doi: 10.1038/s41586-019-1707-0
53. Mao C, Liu X, Zhang Y, Lei G, Yan Y, Lee H, et al. DHODH-mediated ferroptosis defence is a targetable vulnerability in cancer. Nature. (2021) 593:586–90. doi: 10.1038/s41586-021-03539-7
54. Miao Z, Xu L, Gu W, Ren Y, Li R, Zhang S, et al. A targetable PRR11-DHODH axis drives ferroptosis- and temozolomide-resistance in glioblastoma. Redox Biol. (2024) 73:103220. doi: 10.1016/j.redox.2024.103220
55. Kraft VAN, Bezjian CT, Pfeiffer S, Ringelstetter L, Müller C, Zandkarimi F, et al. GTP cyclohydrolase 1/tetrahydrobiopterin counteract ferroptosis through lipid remodeling. ACS Cent Sci. (2020) 6:41–53. doi: 10.1021/acscentsci.9b01063
56. Lei G, Zhuang L, Gan B. Targeting ferroptosis as a vulnerability in cancer. Nat Rev Cancer. (2022) 22:381–96. doi: 10.1038/s41568-022-00459-0
57. Hassannia B, Vandenabeele P, Vanden Berghe T. Targeting ferroptosis to iron out cancer. Cancer Cell. (2019) 35:830–49. doi: 10.1016/j.ccell.2019.04.002
58. Yang WH, Ding CC, Sun T, Rupprecht G, Lin CC, Hsu D, et al. The hippo pathway effector TAZ regulates ferroptosis in renal cell carcinoma. Cell Rep. (2019) 28:2501–8.e4. doi: 10.1016/j.celrep.2019.07.107
59. Lee H, Zhuang L, Gan B. VHL governs m6A modification and PIK3R3 mRNA stability in clear cell renal cell carcinomas. J Clin Invest. (2024) 134(8):e179560. doi: 10.1172/jci179560
60. Lu K, Zhao Y, Li Y, Fu Z, Chen Y, Kong Y, et al. IFI16 promotes the progression of clear cell renal cell carcinoma through the IL6/PI3K/AKT axis. J Transl Med. (2024) 22:533. doi: 10.1186/s12967-024-05354-w
61. Hao J, Chen Q, Feng Y, Jiang Q, Sun H, Deng B, et al. Combination treatment with FAAH inhibitors/URB597 and ferroptosis inducers significantly decreases the growth and metastasis of renal cell carcinoma cells via the PI3K-AKT signaling pathway. Cell Death Dis. (2023) 14:247. doi: 10.1038/s41419-023-05779-z
62. Li X, Xiong W, Wang Y, Li Y, Cheng X, Liu W. p53 activates the lipoxygenase activity of ALOX15B via inhibiting SLC7A11 to induce ferroptosis in bladder cancer cells. Lab Invest. (2023) 103:100058. doi: 10.1016/j.labinv.2022.100058
63. Yi J, Zhu J, Wu J, Thompson CB, Jiang X. Oncogenic activation of PI3K-AKT-mTOR signaling suppresses ferroptosis via SREBP-mediated lipogenesis. Proc Natl Acad Sci U S A. (2020) 117:31189–97. doi: 10.1073/pnas.2017152117
64. Ubellacker JM, Tasdogan A, Ramesh V, Shen B, Mitchell EC, Martin-Sandoval MS, et al. Lymph protects metastasizing melanoma cells from ferroptosis. Nature. (2020) 585:113–8. doi: 10.1038/s41586-020-2623-z
65. Friedmann Angeli JP, Krysko DV, Conrad M. Ferroptosis at the crossroads of cancer-acquired drug resistance and immune evasion. Nat Rev Cancer. (2019) 19:405–14. doi: 10.1038/s41568-019-0149-1
66. Efe G, Rustgi AK, Prives C. p53 at the crossroads of tumor immunity. Nat Cancer. (2024) 5:983–95. doi: 10.1038/s43018-024-00796-z
67. Wu J, Minikes AM, Gao M, Bian H, Li Y, Stockwell BR, et al. Intercellular interaction dictates cancer cell ferroptosis via NF2-YAP signalling. Nature. (2019) 572:402–6. doi: 10.1038/s41586-019-1426-6
68. Hangauer MJ, Viswanathan VS, Ryan MJ, Bole D, Eaton JK, Matov A, et al. Drug-tolerant persister cancer cells are vulnerable to GPX4 inhibition. Nature. (2017) 551:247–50. doi: 10.1038/nature24297
69. Lei G, Zhang Y, Koppula P, Liu X, Zhang J, Lin SH, et al. The role of ferroptosis in ionizing radiation-induced cell death and tumor suppression. Cell Res. (2020) 30:146–62. doi: 10.1038/s41422-019-0263-3
70. Guo J, Xu B, Han Q, Zhou H, Xia Y, Gong C, et al. Ferroptosis: A novel anti-tumor action for cisplatin. Cancer Res Treat. (2018) 50:445–60. doi: 10.4143/crt.2016.572
71. Sun X, Ou Z, Chen R, Niu X, Chen D, Kang R, et al. Activation of the p62-Keap1-NRF2 pathway protects against ferroptosis in hepatocellular carcinoma cells. Hepatology. (2016) 63:173–84. doi: 10.1002/hep.28251
72. Wang W, Green M, Choi JE, Gijón M, Kennedy PD, Johnson JK, et al. CD8(+) T cells regulate tumour ferroptosis during cancer immunotherapy. Nature. (2019) 569:270–4. doi: 10.1038/s41586-019-1170-y
73. Wang Q, Gao S, Shou Y, Jia Y, Wei Z, Liu Y, et al. AIM2 promotes renal cell carcinoma progression and sunitinib resistance through FOXO3a-ACSL4 axis-regulated ferroptosis. Int J Biol Sci. (2023) 19:1266–83. doi: 10.7150/ijbs.79853
74. Zhao Y, Ren P, Yang Z, Wang L, Hu C. Inhibition of SND1 overcomes chemoresistance in bladder cancer cells by promoting ferroptosis. Oncol Rep. (2023) 49(1):16. doi: 10.3892/or.2022.8453
75. Chen F, Wu S, Kuang N, Zeng Y, Li M, Xu C. ABCB1-mediated docetaxel resistance reversed by erastin in prostate cancer. FEBS J. (2024) 291:3249–66. doi: 10.1111/febs.17135
76. Shrestha RK, Nassar ZD, Hanson AR, Iggo R, Townley SL, Dehairs J, et al. ACSM1 and ACSM3 regulate fatty acid metabolism to support prostate cancer growth and constrain ferroptosis. Cancer Res. (2024) 84:2313–32. doi: 10.1158/0008-5472.Can-23-1489
77. Zhang H, Zhou Y, Feng Y, Hou W, Chen Y, Xing Z, et al. Cyclin-dependent kinase 12 deficiency reprogrammes cellular metabolism to alleviate ferroptosis potential and promote the progression of castration-resistant prostate cancer. Clin Transl Med. (2024) 14:e1678. doi: 10.1002/ctm2.1678
78. Zou P, Chen Z, He Q, Zhuo Y. Polyphyllin I induces ferroptosis in castration-resistant prostate cancer cells through the ERK/DNMT1/ACSL4 axis. Prostate. (2024) 84:64–73. doi: 10.1002/pros.24626
79. Shi J, Ma C, Zheng Z, Zhang T, Li Z, Sun X, et al. Low-dose antimony exposure promotes prostate cancer proliferation by inhibiting ferroptosis via activation of the Nrf2-SLC7A11-GPX4 pathway. Chemosphere. (2023) 339:139716. doi: 10.1016/j.chemosphere.2023.139716
80. Sun R, Yan B, Li H, Ding D, Wang L, Pang J, et al. Androgen receptor variants confer castration resistance in prostate cancer by counteracting antiandrogen-induced ferroptosis. Cancer Res. (2023) 83:3192–204. doi: 10.1158/0008-5472.Can-23-0285
81. Nie J, Zhang P, Liang C, Yu Y, Wang X. ASCL1-mediated ferroptosis resistance enhances the progress of castration-resistant prostate cancer to neurosecretory prostate cancer. Free Radic Biol Med. (2023) 205:318–31. doi: 10.1016/j.freeradbiomed.2023.06.006
82. Wang ME, Chen J, Lu Y, Bawcom AR, Wu J, Ou J, et al. RB1-deficient prostate tumor growth and metastasis are vulnerable to ferroptosis induction via the E2F/ACSL4 axis. J Clin Invest. (2023) 133(10):e166647. doi: 10.1172/jci166647
83. Chen WJ, Pan XW, Song X, Liu ZC, Xu D, Chen JX, et al. Preoperative neoadjuvant targeted therapy remodels intra-tumoral heterogeneity of clear-cell renal cell carcinoma and ferroptosis inhibition induces resistance progression. Cancer Lett. (2024) 593:216963. doi: 10.1016/j.canlet.2024.216963
84. Xue W, Jian W, Meng Y, Wang T, Cai L, Yu Y, et al. Knockdown of SETD2 promotes erastin-induced ferroptosis in ccRCC. Cell Death Dis. (2023) 14:539. doi: 10.1038/s41419-023-06057-8
85. Vokshi BH, Davidson G, Tawanaie Pour Sedehi N, Helleux A, Rippinger M, Haller AR, et al. SMARCB1 regulates a TFCP2L1-MYC transcriptional switch promoting renal medullary carcinoma transformation and ferroptosis resistance. Nat Commun. (2023) 14:3034. doi: 10.1038/s41467-023-38472-y
86. Ni X, Ye C, Yu X, Zhang Y, Hou Y, Zheng Q, et al. Overcoming the compensatory increase in NRF2 induced by NPL4 inhibition enhances disulfiram/copper-induced oxidative stress and ferroptosis in renal cell carcinoma. Eur J Pharmacol. (2023) 960:176110. doi: 10.1016/j.ejphar.2023.176110
87. Kang L, Wang D, Shen T, Liu X, Dai B, Zhou D, et al. PDIA4 confers resistance to ferroptosis via induction of ATF4/SLC7A11 in renal cell carcinoma. Cell Death Dis. (2023) 14:193. doi: 10.1038/s41419-023-05719-x
88. Liu T, Xu X, Li J, Bai M, Zhu W, Liu Y, et al. ALOX5 deficiency contributes to bladder cancer progression by mediating ferroptosis escape. Cell Death Dis. (2023) 14:800. doi: 10.1038/s41419-023-06333-7
89. Qin J, Li Z, Su L, Wen X, Tang X, Huang M, et al. Expression of transferrin receptor/TFRC protein in bladder cancer cell T24 and its role in inducing iron death in bladder cancer. Int J Biol Macromol. (2024) 274(Pt 1):133323. doi: 10.1016/j.ijbiomac.2024.133323
90. Yu X, He Z, Wang Z, Ke S, Wang H, Wang Q, et al. Brusatol hinders the progression of bladder cancer by Chac1/Nrf2/SLC7A11 pathway. Exp Cell Res. (2024) 438:114053. doi: 10.1016/j.yexcr.2024.114053
91. Li W, Shen Y, Yang C, Ye F, Liang Y, Cheng Z, et al. Identification of a novel ferroptosis-inducing micropeptide in bladder cancer. Cancer Lett. (2024) 582:216515. doi: 10.1016/j.canlet.2023.216515
92. Xia Y, Xiang L, Yao M, Ai Z, Yang W, Guo J, et al. Proteomics, transcriptomics, and phosphoproteomics reveal the mechanism of talaroconvolutin-A suppressing bladder cancer via blocking cell cycle and triggering ferroptosis. Mol Cell Proteomics. (2023) 22:100672. doi: 10.1016/j.mcpro.2023.100672
93. Luo Y, Zhang Y, Pang S, Min J, Wang T, Wu D, et al. PCBP1 protects bladder cancer cells from mitochondria injury and ferroptosis by inducing LACTB mRNA degradation. Mol Carcinog. (2023) 62:907–19. doi: 10.1002/mc.23533
94. Djebali S, Davis CA, Merkel A, Dobin A, Lassmann T, Mortazavi A, et al. Landscape of transcription in human cells. Nature. (2012) 489:101–8. doi: 10.1038/nature11233
95. Esteller M. Non-coding RNAs in human disease. Nat Rev Genet. (2011) 12:861–74. doi: 10.1038/nrg3074
96. Bartel DP. MicroRNAs: target recognition and regulatory functions. Cell. (2009) 136:215–33. doi: 10.1016/j.cell.2009.01.002
98. Fabian MR, Sonenberg N, Filipowicz W. Regulation of mRNA translation and stability by microRNAs. Annu Rev Biochem. (2010) 79:351–79. doi: 10.1146/annurev-biochem-060308-103103
99. Zheng S, Hu L, Song Q, Shan Y, Yin G, Zhu H, et al. miR-545 promotes colorectal cancer by inhibiting transferring in the non-normal ferroptosis signaling. Aging (Albany NY). (2021) 13:26137–47. doi: 10.18632/aging.203801
100. Bao WD, Zhou XT, Zhou LT, Wang F, Yin X, Lu Y, et al. Targeting miR-124/Ferroportin signaling ameliorated neuronal cell death through inhibiting apoptosis and ferroptosis in aged intracerebral hemorrhage murine model. Aging Cell. (2020) 19:e13235. doi: 10.1111/acel.13235
101. Zhang H, He Y, Wang JX, Chen MH, Xu JJ, Jiang MH, et al. miR-30-5p-mediated ferroptosis of trophoblasts is implicated in the pathogenesis of preeclampsia. Redox Biol. (2020) 29:101402. doi: 10.1016/j.redox.2019.101402
102. Mattick JS, Amaral PP, Carninci P, Carpenter S, Chang HY, Chen LL, et al. Long non-coding RNAs: definitions, functions, challenges and recommendations. Nat Rev Mol Cell Biol. (2023) 24:430–47. doi: 10.1038/s41580-022-00566-8
103. Statello L, Guo CJ, Chen LL, Huarte M. Gene regulation by long non-coding RNAs and its biological functions. Nat Rev Mol Cell Biol. (2021) 22:96–118. doi: 10.1038/s41580-020-00315-9
104. Wang Z, Chen X, Liu N, Shi Y, Liu Y, Ouyang L, et al. A nuclear long non-coding RNA LINC00618 accelerates ferroptosis in a manner dependent upon apoptosis. Mol Ther. (2021) 29:263–74. doi: 10.1016/j.ymthe.2020.09.024
105. Zhang J, Xu T, Liu L, Zhang W, Zhao C, Li S, et al. LMSM: A modular approach for identifying lncRNA related miRNA sponge modules in breast cancer. PloS Comput Biol. (2020) 16:e1007851. doi: 10.1371/journal.pcbi.1007851
106. López-Urrutia E, Bustamante Montes LP, Ladrón de Guevara Cervantes D, Pérez-Plasencia C, Campos-Parra AD. Crosstalk between long non-coding RNAs, micro-RNAs and mRNAs: deciphering molecular mechanisms of master regulators in cancer. Front Oncol. (2019) 9:669. doi: 10.3389/fonc.2019.00669
107. Zhang Y, Luo M, Cui X, O’Connell D, Yang Y. Long noncoding RNA NEAT1 promotes ferroptosis by modulating the miR-362-3p/MIOX axis as a ceRNA. Cell Death Differ. (2022) 29:1850–63. doi: 10.1038/s41418-022-00970-9
108. Dawoud A, Ihab Zakaria Z, Hisham Rashwan H, Braoudaki M, Youness RA. Circular RNAs: New layer of complexity evading breast cancer heterogeneity. Noncoding RNA Res. (2023) 8:60–74. doi: 10.1016/j.ncrna.2022.09.011
109. Kristensen LS, Andersen MS, Stagsted LVW, Ebbesen KK, Hansen TB, Kjems J. The biogenesis, biology and characterization of circular RNAs. Nat Rev Genet. (2019) 20:675–91. doi: 10.1038/s41576-019-0158-7
110. Xu Q, Zhou L, Yang G, Meng F, Wan Y, Wang L, et al. CircIL4R facilitates the tumorigenesis and inhibits ferroptosis in hepatocellular carcinoma by regulating the miR-541-3p/GPX4 axis. Cell Biol Int. (2020) 44:2344–56. doi: 10.1002/cbin.11444
111. Wang HH, Ma JN, Zhan XR. Circular RNA circ_0067934 attenuates ferroptosis of thyroid cancer cells by miR-545-3p/SLC7A11 signaling. Front Endocrinol (Lausanne). (2021) 12:670031. doi: 10.3389/fendo.2021.670031
112. Lyu N, Zeng Y, Kong Y, Chen Q, Deng H, Ou S, et al. Ferroptosis is involved in the progression of hepatocellular carcinoma through the circ0097009/miR-1261/SLC7A11 axis. Ann Transl Med. (2021) 9:675. doi: 10.21037/atm-21-997
113. Liu Y, Li L, Yang Z, Wen D, Hu Z. Circular RNA circACAP2 Suppresses Ferroptosis of Cervical Cancer during Malignant Progression by miR-193a-5p/GPX4. J Oncol. (2022) 2022:5228874. doi: 10.1155/2022/5228874
114. Ou R, Lu S, Wang L, Wang Y, Lv M, Li T, et al. Circular RNA circLMO1 Suppresses Cervical Cancer Growth and Metastasis by Triggering miR-4291/ACSL4-Mediated Ferroptosis. Front Oncol. (2022) 12:858598. doi: 10.3389/fonc.2022.858598
115. Shan C, Liang Y, Wang K, Li P. Noncoding RNAs in cancer ferroptosis: From biology to clinical opportunity. BioMed Pharmacother. (2023) 165:115053. doi: 10.1016/j.biopha.2023.115053
116. Zheng X, Zhang C. The regulation of ferroptosis by noncoding RNAs. Int J Mol Sci. (2023) 24(17):13336. doi: 10.3390/ijms241713336
117. Zuo YB, Zhang YF, Zhang R, Tian JW, Lv XB, Li R, et al. Ferroptosis in cancer progression: role of noncoding RNAs. Int J Biol Sci. (2022) 18:1829–43. doi: 10.7150/ijbs.66917
118. Nejadi Orang F, Abdoli Shadbad M. Competing endogenous RNA networks and ferroptosis in cancer: novel therapeutic targets. Cell Death Dis. (2024) 15:357. doi: 10.1038/s41419-024-06732-4
119. Babu KR, Muckenthaler MU. miR-148a regulates expression of the transferrin receptor 1 in hepatocellular carcinoma. Sci Rep. (2019) 9:1518. doi: 10.1038/s41598-018-35947-7
120. Ohgami RS, Campagna DR, Greer EL, Antiochos B, McDonald A, Chen J, et al. Identification of a ferrireductase required for efficient transferrin-dependent iron uptake in erythroid cells. Nat Genet. (2005) 37:1264–9. doi: 10.1038/ng1658
121. Sun X, Ou Z, Xie M, Kang R, Fan Y, Niu X, et al. HSPB1 as a novel regulator of ferroptotic cancer cell death. Oncogene. (2015) 34:5617–25. doi: 10.1038/onc.2015.32
122. Meyron-Holtz EG, Ghosh MC, Iwai K, LaVaute T, Brazzolotto X, Berger UV, et al. Genetic ablations of iron regulatory proteins 1 and 2 reveal why iron regulatory protein 2 dominates iron homeostasis. EMBO J. (2004) 23:386–95. doi: 10.1038/sj.emboj.7600041
123. Terzi EM, Sviderskiy VO, Alvarez SW, Whiten GC, Possemato R. Iron-sulfur cluster deficiency can be sensed by IRP2 and regulates iron homeostasis and sensitivity to ferroptosis independent of IRP1 and FBXL5. Sci Adv. (2021) 7(22):eabg4302. doi: 10.1126/sciadv.abg4302
124. Cai W, Liu L, Shi X, Liu Y, Wang J, Fang X, et al. Alox15/15-hpETE aggravates myocardial ischemia-reperfusion injury by promoting cardiomyocyte ferroptosis. Circulation. (2023) 147:1444–60. doi: 10.1161/circulationaha.122.060257
125. Yang WS, Kim KJ, Gaschler MM, Patel M, Shchepinov MS, Stockwell BR. Peroxidation of polyunsaturated fatty acids by lipoxygenases drives ferroptosis. Proc Natl Acad Sci U S A. (2016) 113:E4966–75. doi: 10.1073/pnas.1603244113
126. Yuan J, Lv T, Yang J, Wu Z, Yan L, Yang J, et al. HDLBP-stabilized lncFAL inhibits ferroptosis vulnerability by diminishing Trim69-dependent FSP1 degradation in hepatocellular carcinoma. Redox Biol. (2022) 58:102546. doi: 10.1016/j.redox.2022.102546
127. Zhang Y, Koppula P, Gan B. Regulation of H2A ubiquitination and SLC7A11 expression by BAP1 and PRC1. Cell Cycle. (2019) 18:773–83. doi: 10.1080/15384101.2019.1597506
128. Chen D, Tavana O, Chu B, Erber L, Chen Y, Baer R, et al. NRF2 is a major target of ARF in p53-independent tumor suppression. Mol Cell. (2017) 68:224–32.e4. doi: 10.1016/j.molcel.2017.09.009
129. Zhang Y, Qian J, Fu Y, Wang Z, Hu W, Zhang J, et al. Inhibition of DDR1 promotes ferroptosis and overcomes gefitinib resistance in non-small cell lung cancer. Biochim Biophys Acta Mol Basis Dis. (2024) 1870:167447. doi: 10.1016/j.bbadis.2024.167447
130. Fu R, You Y, Wang Y, Wang J, Lu Y, Gao R, et al. Sanggenol L induces ferroptosis in non-small cell lung cancer cells via regulating the miR-26a-1-3p/MDM2/p53 signaling pathway. Biochem Pharmacol. (2024) 226:116345. doi: 10.1016/j.bcp.2024.116345
131. Zhang L, Xu Y, Cheng Z, Zhao J, Wang M, Sun Y, et al. The EGR1/miR-139/NRF2 axis orchestrates radiosensitivity of non-small-cell lung cancer via ferroptosis. Cancer Lett. (2024) 595:217000. doi: 10.1016/j.canlet.2024.217000
132. Yin C, Zhang MM, Wang GL, Deng XY, Tu Z, Jiang SS, et al. Loss of ADAR1 induces ferroptosis of breast cancer cells. Cell Signal. (2024) 121:111258. doi: 10.1016/j.cellsig.2024.111258
133. Wang W, Wang T, Zhang Y, Deng T, Zhang H, Ba YI. Gastric cancer secreted miR-214-3p inhibits the anti-angiogenesis effect of apatinib by suppressing ferroptosis in vascular endothelial cells. Oncol Res. (2024) 32:489–502. doi: 10.32604/or.2023.046676
134. Kindrat I, Tryndyak V, de Conti A, Shpyleva S, Mudalige TK, Kobets T, et al. MicroRNA-152-mediated dysregulation of hepatic transferrin receptor 1 in liver carcinogenesis. Oncotarget. (2016) 7:1276–87. doi: 10.18632/oncotarget.6004
135. Sui X, Hu N, Zhang Z, Wang Y, Wang P, Xiu G. ASMTL-AS1 impedes the Malignant progression of lung adenocarcinoma by regulating SAT1 to promote ferroptosis. Pathol Int. (2021) 71:741–51. doi: 10.1111/pin.13158
136. Tong X, Yu Z, Xing J, Liu H, Zhou S, Huang Y, et al. LncRNA HCP5-encoded protein regulates ferroptosis to promote the progression of triple-negative breast cancer. Cancers (Basel). (2023) 15(6):1880. doi: 10.3390/cancers15061880
137. Zong K, Lin C, Luo K, Deng Y, Wang H, Hu J, et al. Ferroptosis-related lncRNA NRAV affects the prognosis of hepatocellular carcinoma via the miR-375-3P/SLC7A11 axis. BMC Cancer. (2024) 24:496. doi: 10.1186/s12885-024-12265-y
138. Xiong Y, Kong X, Tu S, Xin W, Wei Y, Yi S, et al. LINC02086 inhibits ferroptosis and promotes Malignant phenotypes of pancreatic cancer via miR-342-3p/CA9 axis. Funct Integr Genomics. (2024) 24:49. doi: 10.1007/s10142-024-01329-8
139. Zhang R, Pan T, Xiang Y, Zhang M, Xie H, Liang Z, et al. Curcumenol triggered ferroptosis in lung cancer cells via lncRNA H19/miR-19b-3p/FTH1 axis. Bioact Mater. (2022) 13:23–36. doi: 10.1016/j.bioactmat.2021.11.013
140. Wang P, Hu Z, Yu S, Su S, Wu R, Chen C, et al. A novel protein encoded by circFOXP1 enhances ferroptosis and inhibits tumor recurrence in intrahepatic cholangiocarcinoma. Cancer Lett. (2024) 598:217092. doi: 10.1016/j.canlet.2024.217092
141. Liu B, Ma H, Liu X, Xing W. CircSCN8A suppresses Malignant progression and induces ferroptosis in non-small cell lung cancer by regulating miR-1290/ACSL4 axis. Cell Cycle. (2023) 22:758–76. doi: 10.1080/15384101.2022.2154543
142. Xi Y, Shen Y, Wu D, Zhang J, Lin C, Wang L, et al. CircBCAR3 accelerates esophageal cancer tumorigenesis and metastasis via sponging miR-27a-3p. Mol Cancer. (2022) 21:145. doi: 10.1186/s12943-022-01615-8
143. Shanshan W, Hongying M, Jingjing F, Yiming Y, Yu R, Rui Y. CircDTL functions as an oncogene and regulates both apoptosis and ferroptosis in non-small cell lung cancer cells. Front Genet. (2021) 12:743505. doi: 10.3389/fgene.2021.743505
144. Peng D, Liang M, Li L, Yang H, Fang D, Chen L, et al. Circ_BBS9 as an early diagnostic biomarker for lung adenocarcinoma: direct interaction with IFIT3 in the modulation of tumor immune microenvironment. Front Immunol. (2024) 15:1344954. doi: 10.3389/fimmu.2024.1344954
145. Zhang XY, Li SS, Gu YR, Xiao LX, Ma XY, Chen XR, et al. CircPIAS1 promotes hepatocellular carcinoma progression by inhibiting ferroptosis via the miR-455-3p/NUPR1/FTH1 axis. Mol Cancer. (2024) 23:113. doi: 10.1186/s12943-024-02030-x
146. Saliminejad K, Khorram Khorshid HR, Soleymani Fard S, Ghaffari SH. An overview of microRNAs: Biology, functions, therapeutics, and analysis methods. J Cell Physiol. (2019) 234:5451–65. doi: 10.1002/jcp.27486
147. Huang ZM, Wang H, Ji ZG. Bladder cancer tissue-derived exosomes suppress ferroptosis of T24 bladder cancer cells by transporting miR-217. Environ Mol Mutagen. (2023) 64:39–49. doi: 10.1002/em.22520
148. Yang G, Pan Q, Lu Y, Zhu J, Gou X. miR-29a-5p modulates ferroptosis by targeting ferritin heavy chain FTH1 in prostate cancer. Biochem Biophys Res Commun. (2023) 652:6–13. doi: 10.1016/j.bbrc.2023.02.030
149. Zhu C, Song Z, Chen Z, Lin T, Lin H, Xu Z, et al. MicroRNA-4735-3p facilitates ferroptosis in clear cell renal cell carcinoma by targeting SLC40A1. Anal Cell Pathol (Amst). (2022) 2022:4213401. doi: 10.1155/2022/4213401
150. Torti FM, Torti SV. Regulation of ferritin genes and protein. Blood. (2002) 99:3505–16. doi: 10.1182/blood.v99.10.3505
151. Xiao H, Du X, Tao Z, Jing N, Bao S, Gao WQ, et al. Taurine inhibits ferroptosis mediated by the crosstalk between tumor cells and tumor-associated macrophages in prostate cancer. Adv Sci (Weinh). (2024) 11:e2303894. doi: 10.1002/advs.202303894
152. Zhao Y, Li Y, Zhang R, Wang F, Wang T, Jiao Y. The role of erastin in ferroptosis and its prospects in cancer therapy. Onco Targets Ther. (2020) 13:5429–41. doi: 10.2147/ott.S254995
153. Zhao J, Shen J, Mao L, Yang T, Liu J, Hongbin S. Cancer associated fibroblast secreted miR-432-5p targets CHAC1 to inhibit ferroptosis and promote acquired chemoresistance in prostate cancer. Oncogene. (2024) 43:2104–14. doi: 10.1038/s41388-024-03057-6
154. Shi CJ, Li SY, Shen CH, Pan FF, Deng LQ, Fu WM, et al. Icariside II suppressed tumorigenesis by epigenetically regulating the circβ-catenin-Wnt/β-catenin axis in colorectal cancer. Bioorg Chem. (2022) 124:105800. doi: 10.1016/j.bioorg.2022.105800
155. Tang Z, Du W, Xu F, Sun X, Chen W, Cui J, et al. Icariside II enhances cisplatin-induced apoptosis by promoting endoplasmic reticulum stress signalling in non-small cell lung cancer cells. Int J Biol Sci. (2022) 18:2060–74. doi: 10.7150/ijbs.66630
156. Yu R, Zhou Y, Shi S, Wang X, Huang S, Ren Y. Icariside II induces ferroptosis in renal cell carcinoma cells by regulating the miR-324-3p/GPX4 axis. Phytomedicine. (2022) 102:154182. doi: 10.1016/j.phymed.2022.154182
157. Xu W, Ding J, Li B, Sun T, You X, He Q, et al. Effects of icariin and curcumol on autophagy, ferroptosis, and lipid metabolism based on miR-7/m-TOR/SREBP1 pathway on prostate cancer. Biofactors. (2023) 49:438–56. doi: 10.1002/biof.1927
158. Cao Y, Zou Z, Wu X, Li W, Lu Z, Hu J, et al. LUCAT1 inhibits ferroptosis in bladder cancer by regulating the mRNA stability of STAT3. Gene. (2024) 894:147974. doi: 10.1016/j.gene.2023.147974
159. Xiang L, Zeng Q, Liu J, Xiao M, He D, Zhang Q, et al. MAFG-AS1/MAFG positive feedback loop contributes to cisplatin resistance in bladder urothelial carcinoma through antagonistic ferroptosis. Sci Bull (Beijing). (2021) 66:1773–88. doi: 10.1016/j.scib.2021.01.027
160. Luo W, Wang J, Xu W, Ma C, Wan F, Huang Y, et al. LncRNA RP11-89 facilitates tumorigenesis and ferroptosis resistance through PROM2-activated iron export by sponging miR-129-5p in bladder cancer. Cell Death Dis. (2021) 12:1043. doi: 10.1038/s41419-021-04296-1
161. Zhang Y, Guo S, Wang S, Li X, Hou D, Li H, et al. LncRNA OIP5-AS1 inhibits ferroptosis in prostate cancer with long-term cadmium exposure through miR-128-3p/SLC7A11 signaling. Ecotoxicol Environ Saf. (2021) 220:112376. doi: 10.1016/j.ecoenv.2021.112376
162. Li YZ, Zhu HC, Du Y, Zhao HC, Wang L. Silencing lncRNA SLC16A1-AS1 Induced Ferroptosis in Renal Cell Carcinoma Through miR-143-3p/SLC7A11 Signaling. Technol Cancer Res Treat. (2022) 21:15330338221077803. doi: 10.1177/15330338221077803
163. Xing XL, Yao ZY, Ou J, Xing C, Li F. Development and validation of ferroptosis-related lncRNAs prognosis signatures in kidney renal clear cell carcinoma. Cancer Cell Int. (2021) 21:591. doi: 10.1186/s12935-021-02284-1
164. Lai J, Miao S, Ran L. Ferroptosis-associated lncRNA prognostic signature predicts prognosis and immune response in clear cell renal cell carcinoma. Sci Rep. (2023) 13:2114. doi: 10.1038/s41598-023-29305-5
165. Wu Z, Huang X, Cai M, Huang P. Potential biomarkers for predicting the overall survival outcome of kidney renal papillary cell carcinoma: an analysis of ferroptosis-related LNCRNAs. BMC Urol. (2022) 22:152. doi: 10.1186/s12894-022-01037-0
166. Liu C, Gao Y, Ni J, Chen S, Hu Q, Wang C, et al. The ferroptosis-related long non-coding RNAs signature predicts biochemical recurrence and immune cell infiltration in prostate cancer. BMC Cancer. (2022) 22:788. doi: 10.1186/s12885-022-09876-8
167. Hou J, Lu Z, Cheng X, Dong R, Jiang Y, Wu G, et al. Ferroptosis-related long non-coding RNA signature predicts the prognosis of bladder cancer. BMC Cancer. (2022) 22:719. doi: 10.1186/s12885-022-09805-9
168. Liu J, Zhang Z, Liu X, Zhang W, Meng L, Wang J, et al. Predictive role of ferroptosis-related long non-coding RNAs in bladder cancer and their association with immune microenvironment and immunotherapy response. World J Surg Oncol. (2022) 20:47. doi: 10.1186/s12957-022-02514-4
169. Cen J, Liang Y, Feng Z, Chen X, Chen J, Wang Y, et al. Hsa_circ_0057105 modulates a balance of epithelial-mesenchymal transition and ferroptosis vulnerability in renal cell carcinoma. Clin Transl Med. (2023) 13:e1339. doi: 10.1002/ctm2.1339
170. Yang Y, Luo M, Zhang K, Zhang J, Gao T, Connell DO, et al. Nedd4 ubiquitylates VDAC2/3 to suppress erastin-induced ferroptosis in melanoma. Nat Commun. (2020) 11:433. doi: 10.1038/s41467-020-14324-x
171. Wang L, Wu S, He H, Ai K, Xu R, Zhang L, et al. CircRNA-ST6GALNAC6 increases the sensitivity of bladder cancer cells to erastin-induced ferroptosis by regulating the HSPB1/P38 axis. Lab Invest. (2022) 102:1323–34. doi: 10.1038/s41374-022-00826-3
Keywords: urologic malignancy, ferroptosis, miRNA, lncRNA, circRNA, molecular mechanism
Citation: Li W, Zheng L, Luo P, Chen T, Zou J, Chen Q, Cheng L, Gan L, Zhang F and Qian B (2024) Critical role of non-coding RNA-mediated ferroptosis in urologic malignancies. Front. Immunol. 15:1486229. doi: 10.3389/fimmu.2024.1486229
Received: 27 August 2024; Accepted: 11 October 2024;
Published: 31 October 2024.
Edited by:
Zhihao Wang, Wuhan University, ChinaReviewed by:
Huizhi Liang, Albert Einstein College of Medicine, United StatesYuqi Zhang, AbbVie, United States
Yuer Wu, University of California, Davis, United States
Haochen Jiang, University of Nebraska Medical Center, United States
Copyright © 2024 Li, Zheng, Luo, Chen, Zou, Chen, Cheng, Gan, Zhang and Qian. This is an open-access article distributed under the terms of the Creative Commons Attribution License (CC BY). The use, distribution or reproduction in other forums is permitted, provided the original author(s) and the copyright owner(s) are credited and that the original publication in this journal is cited, in accordance with accepted academic practice. No use, distribution or reproduction is permitted which does not comply with these terms.
*Correspondence: Biao Qian, MjA2NTM1NzkyMUBxcS5jb20=