- Department of Oral Anatomy and Physiology, Jilin Provincial Key Laboratory of Oral Biomedical Engineering, Hospital of Stomatology, Jilin University, Changchun, China
Oral squamous cell carcinoma (OSCC) is a highly aggressive and malignant tumor of oral cavity with a poor prognosis and high mortality due to the limitations of existing therapies. The significant role of tumor microenvironment (TME) in the initiation, development, and progression of OSCC has been widely recognized. Various cells in TME, including tumor-associated macrophages (TAMs), cancer-associated fibroblasts (CAFs), T lymphocytes, tumor-associated neutrophils (TANs), myeloid-derived suppressor cells (MDSCs) and dendritic cells (DCs), form a complicated and important cellular network to modulate OSCC proliferation, invasion, migration, and angiogenesis by secreting RNAs, proteins, cytokines, and metabolites. Understanding the interactions among cells in TME provides the foundation for advanced clinical diagnosis and therapies. This review summarizes the current literature that describes the role of various cellular components and other TME factors in the progression of OSCC, hoping to provide new ideas for the novel OSCC treatment strategies targeting the complicated cellular network and factors that mediate the interactive loops among cells in TME.
1 Introduction
Oral squamous cell carcinoma (OSCC) is a highly aggressive and malignant tumor of oral cavity. The number of new cases and deaths in 2020 is 377713 and 117757 respectively (1). Smoking, alcohol abuse, betel nut chewing, and genetic factors are strongly associated with OSCC occurrence (2). The incidence of OSCC is also regional due to poor habits of the local population (1, 2). OSCC is usually treated with surgical resection combined with chemoradiotherapy, but the 5-year survival rate for patients with advanced OSCC remains low (3). The tumor microenvironment (TME) comprises immune cells, fibroblasts, blood vessels, and extracellular complexes that are closely associated with OSCC progression (Figure 1) (4). Cells in TME can form complex cellular networks by secreting RNAs, proteins, cytokines, and metabolites (5). Moreover, the interactions among cells in TME affect OSCC proliferation, invasion, migration, and angiogenesis (6).
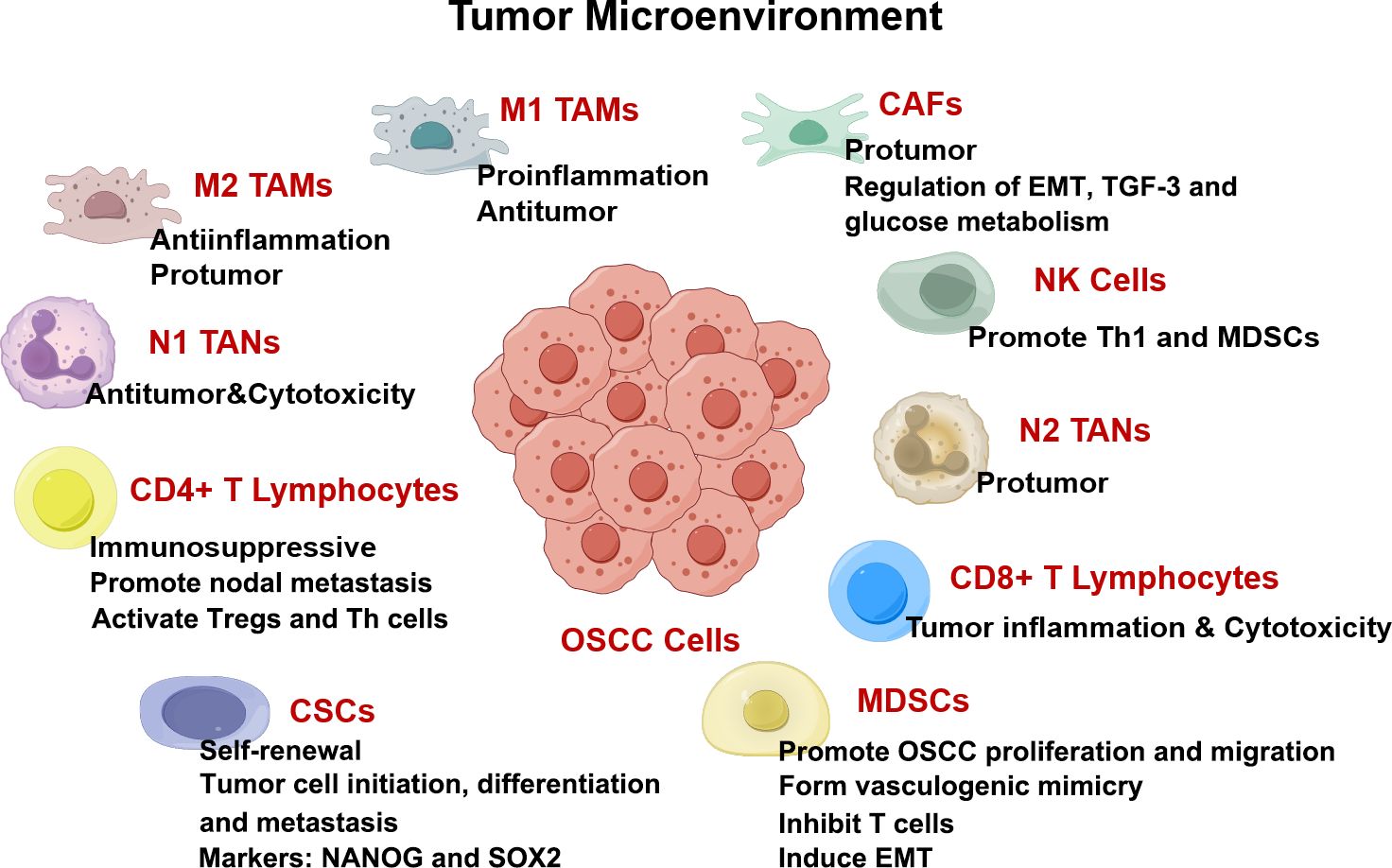
Figure 1. TME in OSCC drawn by Figdraw. Components in TME including cancer-associated fibroblasts (CAFs), myeloid-derived suppressor cells (MDSCs), T lymphocytes, tumor-associated neutrophils (TANs), tumor-associated macrophages (TAMs), and cytokines, are all intricately interconnected with the angiogenesis, proliferation, invasion, and migration of OSCC.
T lymphocytes constitute a crucial component within the immune microenvironment of OSCC. T lymphocytes can be classified into regulatory T-cells (Tregs), T helper (Th) cells, cytotoxic T lymphocytes (CTLs), mucosal-associated invariant T cells (MAIT), natural killer (NK) cells, γδ T cells et al. according to their different surface markers and functions (7). CTLs can control and kill tumor cells to exert an immune effect and Th cells can assist immune cells in removing pathogens (7). NK cells and cluster of differentiation (CD) 8+ T cells possess the capability to recognize and kill tumor cells (8). Tregs foster an immunosuppressive microenvironment, thereby advancing the progression of tumors. It is demonstrated that the downregulation of SLC3A2 regulates immune evasion and promotes metastasis by diminishing the immune capabilities of T lymphocytes in OSCC (9). TME suppresses the immune response by regulating signaling pathways, including VitD signaling pathway, regulating the expression of Tregs and Th cells (10). The functionality of CTLs and Th cells is impeded in OSCC.
The mutations of cancer-related gene, such as tumor suppressor gene P53 (TP53) and HRAS, exert significant influences on head and neck squamous cell carcinoma (HNSCC) as well as the immune mechanisms within TME (11). TP53 gain-of-function mutation TP53 decreases CD8+ T cell levels within TME by modulating cytokine expression, which in turn inhibits the infiltration of Tregs and M2 tumor-associated macrophages (TAMs), thereby facilitating immune escape and the progression of OSCC (12). This review summarizes the current literature that describes the role of various cellular components and other TME factors in the progression of OSCC, hoping to provide new ideas for the novel OSCC treatment strategies targeting the complicated cellular network and factors that mediate the interactive loops among cells in TME.
2 TAMs
2.1 Subtypes and polarization of TAMs
Macrophages can be polarized into classically activated macrophages (M1) and alternatively activated macrophages (M2) (Figure 2) (13). M1 TAMs polarization is driven by lipopolysaccharides (LPS), interferon-gamma (IFN-γ), tumor necrotic factor alpha (TNF-α), interleukin (IL)-12, and IL-18 (Figure 2). M1 TAMs exhibit pro-inflammatory and anti-tumor responses through secreted cytokines, recruitment of cluster of differentiation (CD) 8+ T cells and natural killer (NK) cells, and increased antigen presentation (Figure 2). IL-4, IL-10, IL-13, and transforming growth factor beta (TGF-β) promote the polarization of M2 TAMs that displays an anti-inflammatory response and promotes tumor growth, metastasis, and angiogenesis (Figure 2) (14, 15).
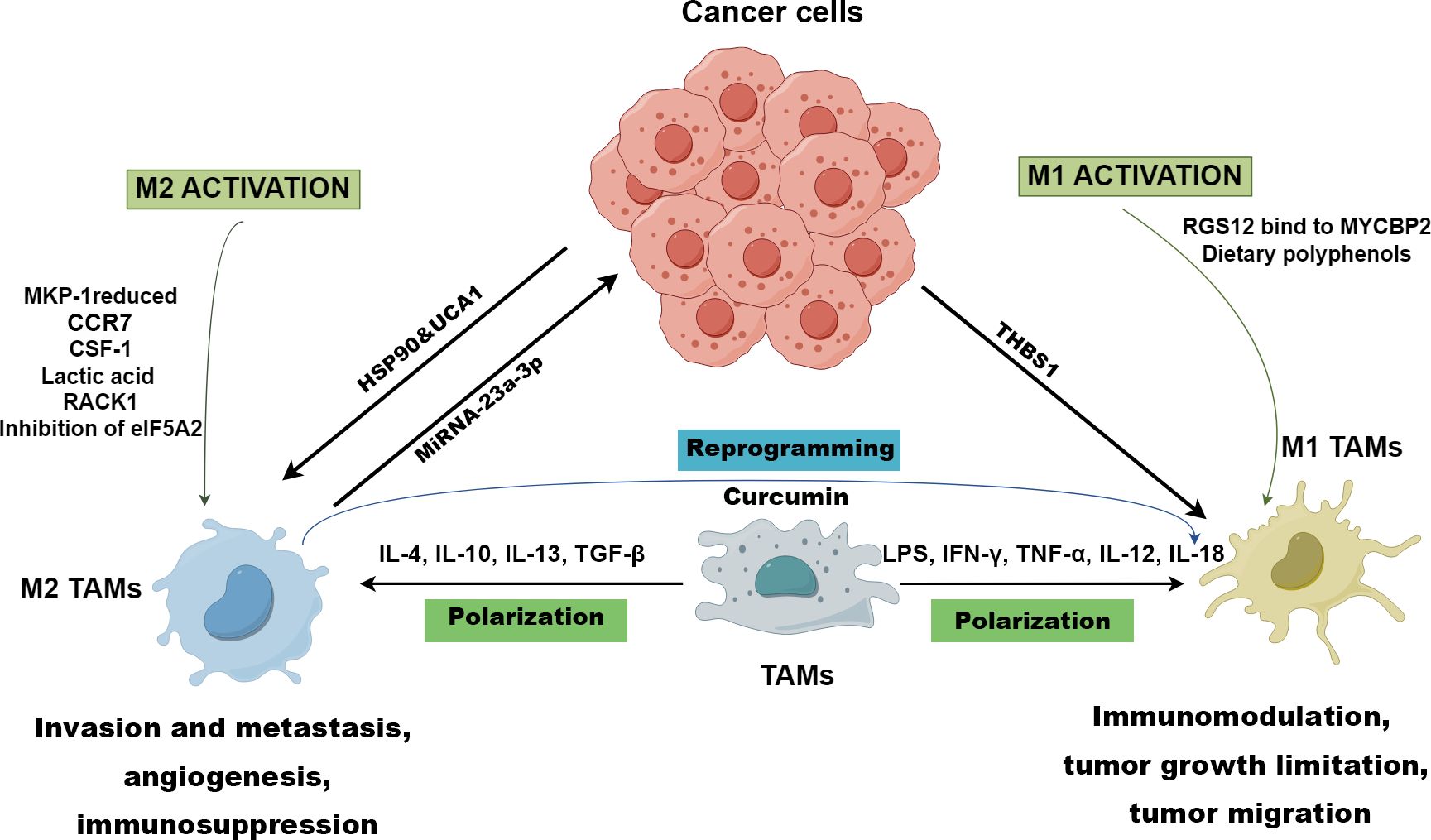
Figure 2. Polarization of TAMs drawn by Figdraw. M1 TAMs polarization is driven by LPS, IFN-γ, TNF-α, IL-12, and IL-18, while IL-4, IL-10, IL-13, and TGF-β promote macrophages towards M2 polarized state. RGS12 binds to MYCBP2 to degrade KIF2A in TAMs driving TAMs to polarize towards the M1 phenotype. However, CCR7, CSF-1, lactic acid, RACK1, reduced MKP-1, and inhibition of eIF5A2 contribute to the genesis of M2 TAMs. M1 TAMs are conducive to immunomodulation, tumor growth limitation, and tumor migration, while M2 TAMs foster invasion and metastasis, angiogenesis, and immunosuppression in OSCC. The TME prompts the polarization of M1 TAMs through cancer-derived exosomal THBS1, while exosomal UCA1 and HSP-90 guide TAMs towards the M2 phenotype polarization. Exosomal miRNA-23a-3p derived from M2 TAMs promotes the proliferation and invasion of OSCC cells and inhibits cancer cell apoptosis. In immunotherapies, and curcumin can reprogram M2 TAMs to M1 TAMs to inhibit OSCC.
OSCC cells contributes to the genesis of M2 TAMs through the p38/MKP-1 signaling axis (Figure 2) (16). Ono et al. found that heat shock protein 90 (HSP-90)-enriched metastatic oral cancer-derived extracellular vesicles (MEV) can also tune TAMs to M2 phenotype (Figure 2) (17). Plasminogen activator inhibitor-1 (PAI-1) and IL-8 derived from OSCC cells have been proved to conduce to the production of CD206+ TAMs (18). Zhou et al. reported that CCR7 in OSCC cells facilitate M2 polarization of macrophages by increasing production of CCL19 and CCL21 (Figure 2) (19). Moreover, stromal cells (SCs), verrucous OSCC−associated SCs, and ED−type OSCC-associated SCs are conducive to the differentiation towards M2 phenotype (20). OSCC cells-derived HMGB1and macrophage endogenous HMGB1 facilitate the polarization of M1 macrophages via TLR4/NF-κB signaling (21). Chen et al. demonstrated that OSCC cells-derived THBS1 in exosome promotes M1 macrophages polarization (22). These studies indicate that OSCC cells-derived proteins, enzymes, cytokines and chemokines in TME can induce M2 or M1 polarization of macrophages. However, there are few studies on macrophage polarization induced by other cells besides OSCC cells, and the mechanism has not been fully elucidated. In addition, the studies involved in the regulation of TAMs by HMGB1 secreted during cell death is still controversial.
2.2 Role of TAMs in OSCC invasion and migration
2.2.1 M2 TAMs
M2 macrophage-derived exosomal miRNA-23a-3p promotes the proliferation and invasion of OSCC cells and inhibits apoptosis by inhibiting the expression of phosphatase and tensin homolog (PTEN) in vitro and in vivo (Figure 2, Table 1) (23). Wang et al. found that TAMs facilitated OSCC migration and invasion via the MIF/NLRP3/IL-1β pathway, which could be disrupted through melatonin (Table 1) (24). Increasing colony stimulating factor-1 (CSF-1) levels causes increased infiltration of M2 TAMs, which has an influence on the development and progression of OSCC (Table 1; Figure 2) (25). Lactic acid-induced M2 TAMs promote OSCC migration and invasion via GPNMB/CD44 pathway (Figure 2; Table 1) (26). M2 TAMs enhance OSCC migration and metastasis via CCL13 upregulated by Stress Granule (Table 1) (27).
2.2.2 M1 TAMs
IL-6 derived from High mobility group box 1 (HMGB1)-activated M1 TAMs enhances OSCC migration and invasion (Table 1) (28). M1 TAMs polarized by exosomal thrombospondin 1 (THBS1) from OSCC cells can promote the EMT process and induce cancer stem cells (CSCs) through the IL6/Stat3/THBS1 feedback loop (Figure 2; Table 1) (29).
2.2.3 TAMs
TAMs activation and polarization induced by overexpression of TWIST1 and CSF1 in OSCC cells facilitate OSCC invasion (Table 1) (30). TAM-derived HMGB1 promotes OSCC invasion via IL-6/NF-κB/MMP-9 pathway (Table 1) (21). Lin et al. found that alpha-enolase (ENO1) and lactic acid derived from tumor cells promotes OSCC migration and invasion by coordinating IL-6 release from TAMs (34).
Although it is widely acknowledged that M2 TAMs promote OSCC invasion and migration through complex signaling pathways involving the secretion of bioactive molecules, M1 TAMs have also been shown to contribute to the progression of OSCC metastasis. The regulation of TAMs polarization and cytokine secretion by lactic acid has gradually attracted the attention of researchers. In particular, TAM-derived IL-6 can significantly promote the invasion and migration of OSCC, which is expected to become a potential therapeutic target. However, the characterization of TAMs phenotype remains inadequate in some studies, and the detailed intracellular signaling pathways related to TAMs polarization and cytokine secretion needs further elucidation.
2.3 Role of TAMs in OSCC angiogenesis
M2c and M2d TAMs can elevate glycolysis and initiate angiogenesis with secreted chemokines, fibronectins, and growth factors (35). CSCs, endothelial cells (ECs), and TAMs constitute interrelation through the exchange of cytokine mediators, such as vascular endothelial growth factor (VEGF), IL-6, IL-8, and CSF-1, leading to microvessel density improvement and progression of OSCC (36). ALDH3A1 overexpression in OSCC cells suppresses CRI regulated by TAMs, resulting in inhibition of angiogenesis (37). TAMs play an important role in angiogenesis by producing pro-angiogenic factors. CSCs, ECs, and TAMs construct a sophisticated cellular network, utilizing mediators to regulate angiogenesis in OSCC. Targeting TAMs is an effective way to disrupt the formation of tumor vascular networks and thus inhibit the progression of OSCC. However, the detailed signaling pathways related to the production of pro-angiogenic factors in TAMs and targeted drug interventions need to be further investigated.
2.4 Role of TAMs in OSCC immunosuppression
M2 TAMs promote the immune escape of OSCC through upregulating the checkpoint programmed death-ligand 1 (PD-L1) expression (Table 1) (31). OSCC cells-derived GM-CSF modulates the level of PD-L1 expression in TAMs via JAK2/STAT3 signaling cascade to promote immune escape (Table 1) (32). Endogenous HMGB1 in TAMs promotes immunosuppression and immune escape via the IL-6/STAT3/PD-L1 pathway (Table 1) (21). M2 TAMs induced by cancer stem cell-derived UCA1 targeting LAMC2 promotes immunosuppression by suppressing CD4+ T-cell proliferation and IFN-γ production (Figure 2, Table 1) (33). A high level of Perilipin 2 (PLIN2) derived from TAMs induces less CD8+ T cells but more TAMs and Foxp3+ Tregs, inducing immunosuppression in OSCC (38). Foxp3+ Tregs contribute to the evasion of immune surveillance through the secretion of cytokines by M2 TAMs in TME (39).
M2 TAMs promote the immunosuppression of OSCC by inducing the expression of PD-L1 and directly inhibiting CD4+ and CD8+ T cells. Inhibition of M2 macrophages polarization can be used as a long-term therapeutic strategy to control or reverse immunosuppression and immune escape. However, the correlation between the density of specific subtypes of TAMs and immune escape remains controversial. The surface receptors and signaling pathways of TAMs that mediate M2 TAMs polarization as well as TAMs-secreted mediators associated with immunosuppression are the focus of further research. Meanwhile, it is necessary to verify whether HMGB1 and PLIN2 can be used as potential therapeutic directions for OSCC.
2.5 Immunotherapies targeting TAMs
Currently, immunotherapies targeting TAMs mainly concentrate on three aspects: hindering macrophages from polarizing, removing M2 TAMs from the TME, and repolarizing M2 TAMs towards M1 TAMs (39, 40). Myeloid-based Dusp1-deficiency can reduce the accumulation and polarization of TAMs, which may be a novel target to reprogram TAMs and inhibit the progression of OSCC (41). Targeted inhibition of eukaryotic initiation factor 5A2 (eIF5A2) impedes tumor growth and polarization towards M2 TAMs, which is correlated with the function of modulating cell proliferation, CSC properties, and EMT in oral cancer (Figure 2) (42). PY314, an antibody against TREM2, can exhaust TREM2+ TAMs to decrease exhausted CD8+ T cells and the method has already been applied in clinic (43). Regulator of G protein signaling 12 (RGS12) suppresses OSCC by driving M1 TAMs polarization via MYCBP2/KIF2A signaling pathway (Figure 2) (44). Dietary polyphenols can promote polarization towards M1 TAMs and curcumin can reprogram M2 TAMs to M1 TAMs to inhibit OSCC (Figure 2) (45, 46). Receptor for activated C kinase 1 (RACK1) fosters the polarization towards M2 TAMs improving the M2/M1 TAMs ratio by means of regulating NF-κB, which can be used as a potential therapeutic target (Figure 2) (47).
A significant breakthrough has been made in cancer immunotherapy through advancements in nanomedicine. Nanomedicines targeting TAMs plays a very important role in anti-tumor immunotherapy, mainly focusing on inhibiting the recruitment of TAMs, killing TAMs, reprogramming the TAMs phenotype, and promoting the immune function of TAMs (48, 49). Mannose-modified hyaluronic acid nanocapsules targeting TAMs enable precise drug delivery and reprogramming of M2 TAMs (50).
Given the high infiltration of TAMs in OSCC and their intimate involvement in OSCC progression, targeting TAMs has emerged as a promising area of research for therapeutic intervention. However, the diverse subtypes of TAMs and complexity of TME have significantly limited the advance of immunotherapy specifically targeting TAMs in OSCC. Further elucidation of the regulatory mechanism for TAMs polarization and mediator secretion, and the development of TAMs targeting drugs using nanomedicine has become the focus of further research.
3 CAFs
3.1 Activation of fibroblasts into CAFs
CAFs are activated fibroblasts with mesenchymal cell lineage as well as heterogeneity of phenotype and function in TME (51, 52). CAFs secrete a variety of active factors that are involved in tumor biologic behaviors such as formation and maintenance of tumor mass, generation and maintenance of cancer cell stemness, immune regulation, angiogenesis, metabolic response, ECM remodeling, homeostasis, and treatment resistance (53).
TGF-β activates fibroblasts into CAFs, and lncRNA PVT1 expression further stimulates CAFs proliferation by increasing infiltration of TGF-β (54). Exosomes released from HNSCC cells can convert normal fibroblasts into CAFs (55). Numerous evidences showed that OSCC cells-derived extracellular vesicles (EVs) can activate fibroblasts into CAFs, although EVs vary in content and may include RNAs, proteins and TGFβ (56). CAFs can activate OSCC cells and suppress immune cells, which is related to their secretion of RNAs, proteins, TGF-β and their own regulation of glucose metabolism (Figure 3).
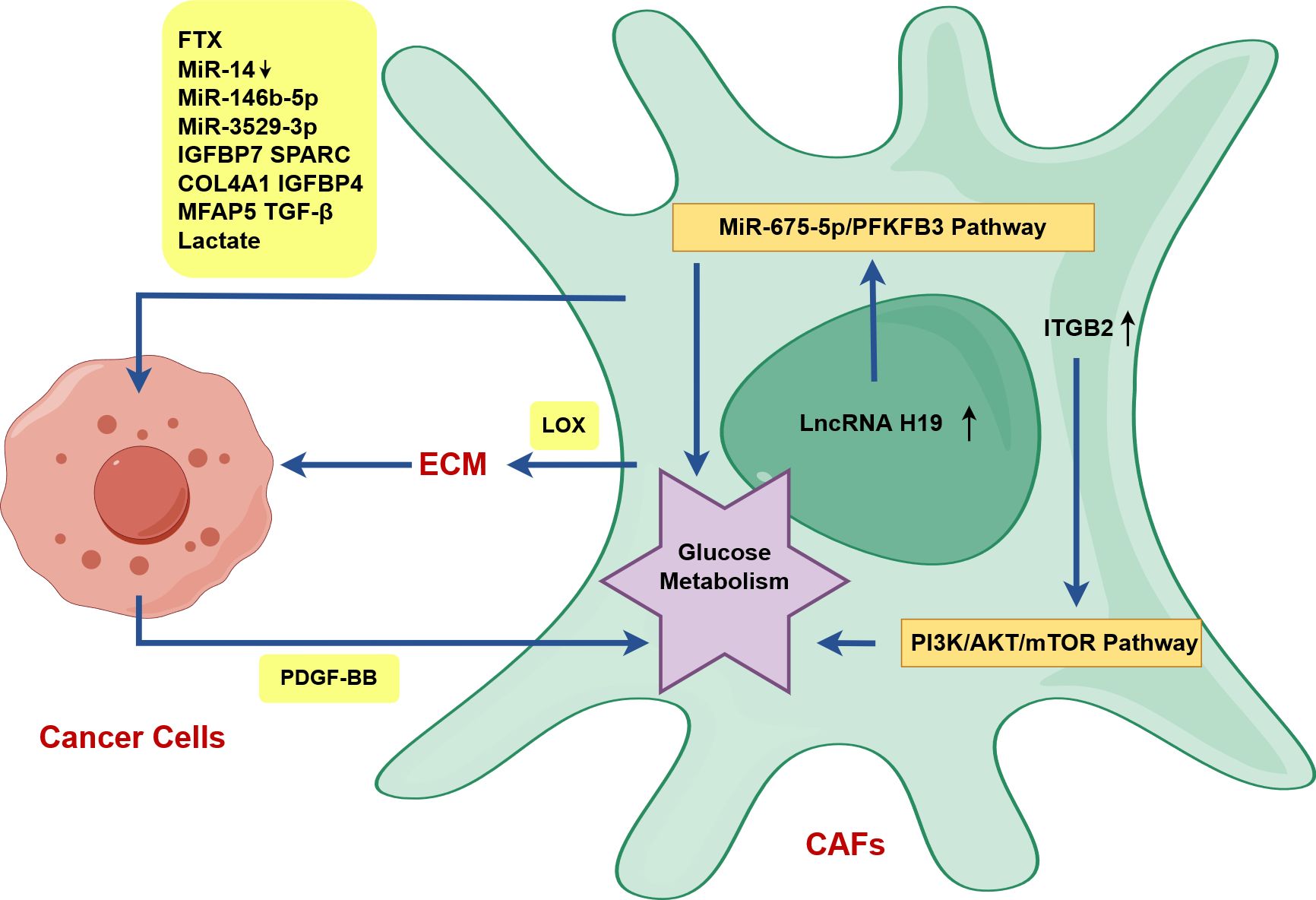
Figure 3. CAFs activate OSCC cells in OSCC drawn by Figdraw. ITGB2 expression in CAFs can improve the glycolysis ability of CAFs by activating the PI3K/AKT/mTOR axis, and upregulated lncRNA H19 in CAFs can promote glucose metabolism via the lncRNA H19/miR-675-5p/PFKFB3 signaling cascade. PDGF-BB-induced glucose metabolism reprogramming in CAFs promotes the proliferation, invasion and metastasis of tongue squamous cell carcinoma (TSCC) via the secretion of lactates. CAFs can directly activate OSCC cells and promote OSCC progression by secreting RNAs, proteins, TGF-β and lactate (FTX, decreased miR-14, miR-146b-5p, miR-3529-3p, IGFBP7, SPARC, COL4A1, IGFBP4, MFAP5, TGF-β, lactate). In particular, CAFs indirectly activate OSCC cells via LOX-mediated the remodeling of stromal collagen microenvironment.
3.2 CAFs activate OSCC cells
3.2.1 CAFs-derived RNAs activate OSCC cells
The podoplanin (PDPN)+ CAFs secrete exosomal lncRNA FTX to increase the migratory capability of OSCC by suppressing ferroptosis via FTX/FEN1/ACSL4 pathway (Figure 3) (57). The overexpression of lncRNA TIRY in CAFs can lead to decreased expression of miR-14 in CAFs-derived exosomes, which facilitates EMT, invasion and metastasis of OSCC cells via Wnt/β-catenin pathway (Figure 3) (58). CAFs-derived exosomal miR-146b-5p facilitates OSCC proliferation and metastasis through downregulating homeodomain-interacting protein kinase 3 (HIPK3) expression (Figure 3) (59). PDGF-BB-induced CAFs-derived exosomal miR-3529-3p promotes OSCC proliferation, migration, and invasion as well as inhibits apoptosis of cancer cells (Figure 3) (60).
3.2.2 CAFs-derived proteins activate OSCC cells
FERM domain containing kindlin 2 (FERMT2) upregulation in CAFs promotes EMT of OSCC by modulating the secretion of insulin-like growth factor binding protein 7 (IGFBP7), secreted protein acidic and rich in cysteine (SPARC), collagen type IV alpha 1 chain (COL4A1), and insulin-like growth factor binding protein 4 (IGFBP4) in oral CAFs (Figure 3) (61). CAFs located at the invasive tumor front (CAFs-F), characterized by higher expression of the fibroblast activation marker SMA, were found to secrete elevated levels of microfibril-associated protein 5 (MFAP5) in comparison to NFs and the superficial tumor (CAFs-S) (62). That’s why the degree of EMT and the impact on the invasion and metastasis of OSCC are greater in CAFs-F (62).
Lysyloxidase (LOX) highly expressed in CAFs can remodel the matrix collagen microenvironment, enhance matrix stiffness, and accumulate nuclear β-catenin giving rise to EMT and tumor invasion through the FAK phosphorylation pathway (Figure 3) (63). Stromal nicotinamide N-methyltransferase (NNMT), a specific biomarker of CAFs, can regulate type I collagen deposition through epigenetically regulating the transcription of LOX via the NNMT-LOX-FAK axis by reducing the enrichment of H3K27me3, which can foster OSCC stemness by maintaining FAK signaling (Figure 3) (64). Mediated by integrin α2β1, CAFs-derived LOX-rich EVs mediate collagen crosslinking through fibronectin, periostin, and bone morphogenetic protein-1, and promote EMT via p-FAK/p-paxillin/YAP signaling pathway (65).
3.2.3 CAFs-derived TGF-β activates OSCC cells
TGF-β can activate OSCC cells via classical SMAD pathway. TGF-β secreted by CAFs in invasive cancer nests could increase the level of sex-determining region Y (SRY)-box9 (SOX9) expression by activating SMAD pathway, which was conducive to OSCC invasion and migration (66). Single-cell analysis showed that CAFs could promote OSCC invasion by secreting TGF-β through SMAD pathway (67).
3.2.4 CAFs-derived lactate activates OSCC cells
Higher integrin beta 2 (ITGB2)-mediated lactate release in CAFs promotes OSCC proliferation by oxidation of NADH in mitochondrial oxidative phosphorylation system (Figure 3) (68). LncRNA H19/miR-675-5p/PFKFB3 axis-mediated glycolysis reprogramming in CAFs enhances OSCC growth through lactate secretion (Figure 3) (69). PDGF-BB-induced glucose metabolism reprogramming in CAFs promotes the proliferation, invasion and metastasis of tongue squamous cell carcinoma (TSCC) via the secretion of lactates (70).
CAFs can directly activate OSCC cells and promote OSCC progression by secreting RNAs, proteins, TGF-β and lactate. In particular, CAFs indirectly activate OSCC cells via LOX-mediated the remodeling of stromal collagen microenvironment. CAFs-derived exosomal lncRNA and miRNA promote OSCC proliferation, migration, and invasion, but relevant studies on circ RNA are lacking. The mechanism of lactate-related glycolysis reprogramming in CAFs has gradually become a research hotspot. In addition, the mediators secreted by CAFs to activate OSCC cells in some relevant studies have not been clarified, which needs to be further investigated (71).
3.3 CAFs suppress immune cells
CAFs-derived exosomal immune-related genes (hsa-miR-139-5p, ACTR2 and EIF6) induce immunosuppression and promote OSCC proliferation by regulating the expression of PIGR, CD81, UACA, and PTTG1IP in cancer cells (72). CAF-derived ACLP suppresses CD8+ T cell migration and facilitates OSCC progression (73). Zhao et al. reported that the low number of CD68+ CAFs, a novel subtype of CAFs, exhibited a negative association with the proportion of Tregs (74).
CAFs can indirectly or directly inhibit immune cells to promote the progression of OSCC. The indirect inhibition effect of CAFs on immune cells is achieved by inducing immunosuppressive microenvironment. Genome sequencing and mRNA-miRNA interaction network analysis provide evidence for the correlation between CAFs-derived exosomal immune-related genes and immunosuppression. However, their detailed regulatory mechanism in OSCC has not been fully clarified due to the lack of comprehensive molecular biological analysis. The next step is to further elucidate the specific mechanism of CAFs inhibiting immune cells through in vitro and in vivo experiments, while focusing on the regulation and inhibition of CAFs on T lymphocytes.
4 T lymphocytes
4.1 Role of Tregs in OSCC
Inflammatory cells within TME secrete chemokines like C-X-C motif chemokine (CXCL)12 and CCL17 to recruit Tregs (10, 75, 76). Alternatively, in non-inflammatory conditions, mutations in genes such as epidermal growth factor receptor (EGFR) can modulate signaling pathways, leading tumor cells to produce chemokines like CCL22 that enhance Tregs infiltration (77). Furthermore, the level of Tregs in the TME is also intricately linked to tumor-draining lymph nodes (TDLNs) (78).
Forkhead box P3 (FOXP3) serves as a significant identifier for the presence and function of regulatory T cells (Tregs), which are essential modulators for the immune system (79). Chatzopoulos et al. found that Foxp3+ T cells took part in the immunosuppressive microenvironment in TSCC (80). Hayashi reported that Foxp3+ T cell expression might play a role in nodal metastasis and exhibited a significant correlation with the weak immune response, onset of OSCC, and enhancement of tumor malignancy (81). However, Koike et al. reported that Foxp3+ T cells might not suppress immune response but might perform site-specific anti-tumor response in OSCC (82). The Foxp3+ T cell density at the invasive front exhibits a positive correlation with overall survival (OS), disease-specific survival (DFS), recurrence-free survival, and metastasis-free survival, which means Foxp3+ T cells may be a novel prognostic factor for OSCC (82). IL-23R- Tregs display a more immunosuppressive profile compared to IL-23R+ Tregs, which is regulated by IL-23 secreted by other cells in TME (83).
4.2 Role of CTLs in OSCC
The expansion of CD4+ cytotoxic T lymphocytes with specific gene expression profiles may inhibit the tumor immunity in OSCC (84). Increased PD-1 levels and glycolysis in CD4+ T cells are positively associated with lymph node metastasis of OSCC (Figure 4) (85). Statistical analysis revealed that the level of CD8+ T cells in TSCC exhibited a crucial correlation with the tumor inflammation signature and cytotoxicity signature (80). CD8+ T cells with the overexpression of PD-1 or TOX in OSCC have low cytotoxicity and weak ability to proliferate, which impairs the function of immunosuppression (Figure 4) (79). Exhausted T cells are characterized by upregulated immunosuppressive checkpoints including PD-1, TIM-3, and CTLA-4 (Figure 4) (86). Exhausted CD4+ T cells which appear earlier and more in number than exhausted CD8+ T cells foster the development of oral cancer (86).
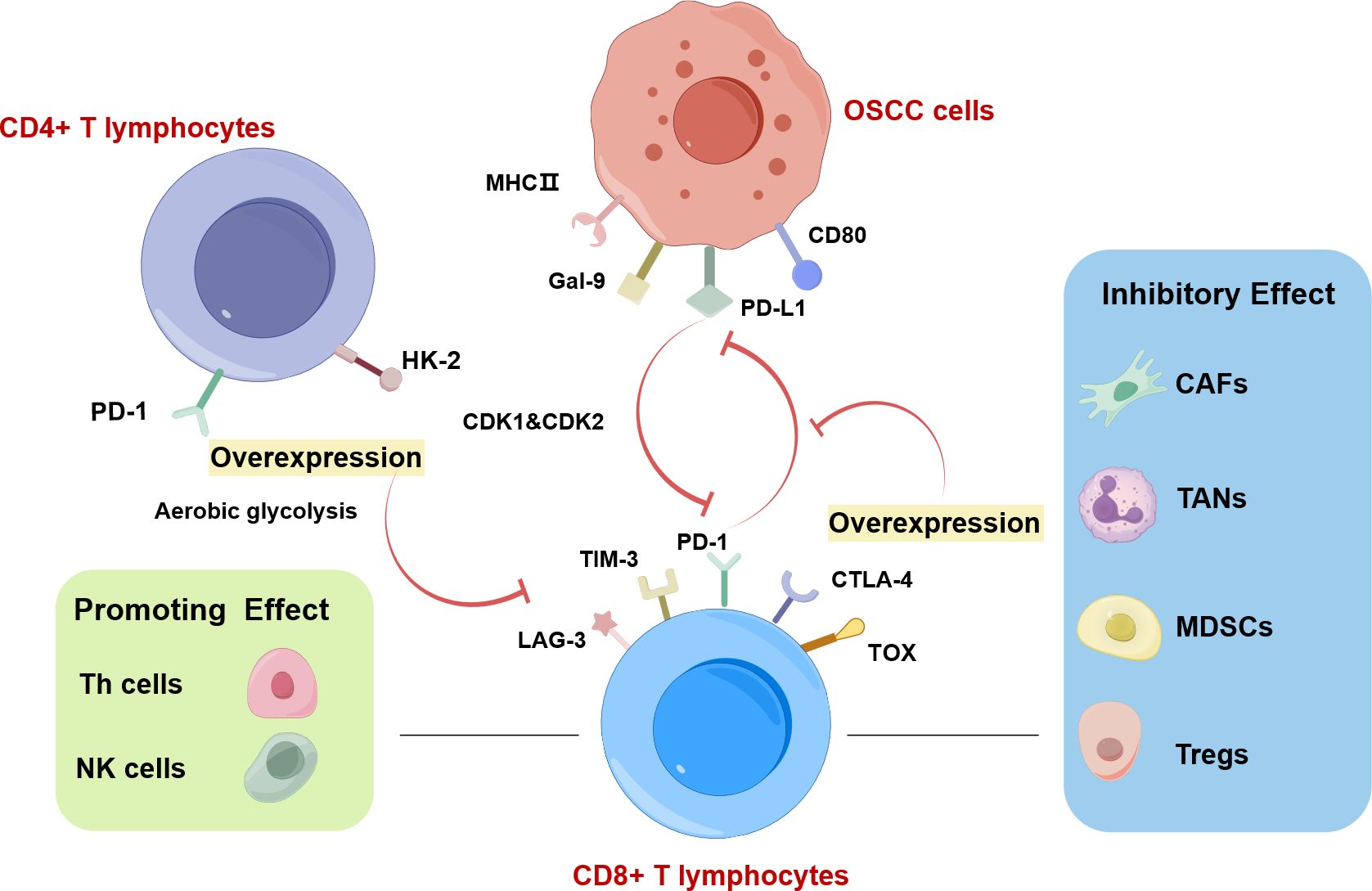
Figure 4. CD8+ T lymphocytes in OSCC drawn by Figdraw. Increased PD-1 levels and glycolysis in CD4+ T cells are positively associated with lymph node metastasis of OSCC. The interaction between CD8+ T cells and cancer cells is regulated by immune cells in TME. Among them, CAFs, TANs, MDSCs, and Tregs exhibit inhibitory effects on CD8+ T lymphocytes. Conversely, NK cells and Th cells play a supportive role in promoting CD8+ T lymphocyte function. The highly expressed T lymphocyte proliferation regulator-related genes (RANs) (CDK1 and CDK2) in malignant cells and T cells, can inhibit the function of CD8+ T cells. CD8+ T cells overexpressing PD-1 or TOX in OSCC have low cytotoxicity and weak ability to proliferate, which is associated with aerobic glycolysis. Exhausted CD8+ T cells are characterized by upregulated immunosuppressive checkpoints including PD-1, TIM-3, and CTLA-4.
4.3 Role of other T lymphocytes in OSCC
A high ratio of CD45RO-expressing tumor-infiltrating lymphocytes (TILs) was associated with a disease-free/overall survival improvement in OSCC patients, suggesting that CD45RO-expressing TILs are useful biomarkers for OSCC (87). Caspase activation in TILs is associated with lymph node metastasis in OSCC (88). malignant cells and T cells overexpress T lymphocyte proliferation regulator-related genes (RANs) to maintain the immunosuppressive microenvironment by impeding the functionality of plasmacytoid dendritic cells (pDCs) and CD8+ T cells but enhancing the activity of Th2 cells and Th cells (Figure 4) (89).
In conclusion, Tregs exhibit immunosuppressive properties, yet there are also reports indicating their anti-tumor effects. CTLs possess the capability to eliminate tumor cells, thereby inhibiting OSCC and being linked to a positive prognosis. The role of Tregs in OSCC is intricate, and their impact on the prognosis of OSCC patients remains contentious. Given this complexity, there is a pressing need for more comprehensive and in-depth research into Tregs.
5 TANs
Tumor-associated neutrophils (TANs) are a kind of tumor-associated myeloid cells derived from blood neutrophils in TME (90). Due to their plasticity, TANs can polarize into distinct phenotypes upon stimulation by various cytokines and growth factors, which can be broadly categorized as N1 TANs and N2 TANs (91, 92). N1 TANs are related to the process against tumor proliferation with direct cytotoxicity, while N2 TANs can promote tumor progression (91). The overexpression of chemerin, a kind of effective chemoattractant protein derived from tumor cells, can improve the number of TANs in TME (93). TANs-derived small extracellular vesicles (sEVs) can also promote as well as inhibit tumor growth (94).
The pro-tumor functions of TANs include the promotion of proliferation and anti-apoptosis, EMT, invasion, metastasis, nodal spread, tumor vascularization, and immunosuppression (91, 95). B-cell activating factor (BAFF) secreted by TANs promotes proliferation of OSCC cells and hinders their apoptosis (96). TANs contribute to OSCC proliferation, invasion, and migration via JAK2/STAT3 signaling pathway and EMT with the help of chemerin (93). Lonardi et al. revealed that a high proportion of CD66+ TANs in TDLNs had been found in OSCC patients with worse prognosis which indicated TANs might promote nodal spread through lymphatic vessels (97). TANs also play a role in OSCC through the network with other myeloid immune cells to affect prognosis (98). The upregulation of ALDH3A1 serves to impede the polarization of neutrophils towards the N2 phenotype and also hinders their migration by inhibiting IL-8 secretion to deactivate the PI3K/AKT/NF-κB pathway, which is conducive to inhibiting inflammation and halting oral cancer growth (99).
Interactions between OSCC cells and TANs modulate the behavior of TANs and reprogram them to mediate pro-inflammatory or immunosuppressive responses. OSCC cells- and TANs-derived sEVs carry complex mediators which finally are delivered to respective recipient cells in TME, constructing the complete positive feedback interactive loops. Although the details of complex bidirectional crosstalk remain unknown, a better understanding of the interactive loops might provide new treatment strategies for OSCC in the future.
6 MDSCs
Hematopoietic precursor cells in bone marrow make up MDSCs, which can be classified into polymorphonuclear MDSCs (PMN-MDSCs) and monocytic MDSCs (M-MDSCs) (100, 101). OSCC cells can facilitate the process of transforming to MDSCs with immunosuppressive phenotype (102). OSCC-derived MDSCs can foster the proliferation, migration, and poor prognosis of cancer cells (102). MDSCs can also induce EMT in OSCC cells, which is conducive to vasculogenic mimicry formation (102). Kouketsu et al. reported that MDSCs had an inhibitory effect on T cells leading to the reconstitution of TME, which was associated with the oncogenesis of OSCC (103). Moreover, Jiang et al. reported that the elevated proportion of CD11b+ MDSCs could promote the generation of tumors (104). The presence of CD11b+ MDSCs in both the core and invasive margins of OSCC exhibits a strong association with the lower OS and DFS of OSCC, indicating their potential as a prognostic factor (104). At present, most studies on the role of MDSCs in OSCC remain in the correlation aspect, and there are few studies involved in the detailed mediators, receptors and intracellular signaling pathways. Further studies should focus on the molecular mechanism of MDSCs regulation in OSCC, which is helpful to provide potential targets for intervention.
7 Cytokines
7.1 Role of TNF in OSCC
TNF-α and IL-8 are prominently overexpressed in OSCC and OPMDs, significantly contributing to the advancement of malignant transformation (Table 2) (105). Gupta et al. reported that both TNF-α and TNF-β took an active part in the progression of precancerous lesions and OSCC in the North India population, but the influence of TNF-α was stronger (Table 2) (106). OSCC patients have abundant rare in situ cytokine-secreting T cell populations such as CD4+ Th cells secreting TNF-α and CD8+ T cells secreting IFN-γ (Table 2) (107). The antigen-reactive memory T cells promote tumor immune control through the secreted type I cytokines, especially TNF-α (Table 2) (107).
7.2 Role of TGF in OSCC
TGF-α has been linked to facilitating cell proliferation, and metastasis, suppressing immune responses, and promoting angiogenesis (Table 2) (108). TNF-α promotes TGF-β-induced endothelial-to-mesenchymal transition (EndMT) via TGF-β signal augmentation in OSCC (Table 2) (109). Furthermore, Maldonado et al. demonstrated that suppressing the secretion of TGF-β in TME would interfere with the polarization of M2 TAMs, inhibiting invasion and chemotaxis of OSCC cells (Table 2) (110).
7.3 Role of interleukin in OSCC
Lopez-Labady et al. have reported that the level of IL-1β and IL-8 in OSCC was comparatively less than that found in normal mucosal tissue, indicating that the chemotactic activity and anti-tumor function are decreased in OSCC (111). IL-6 can contribute to tumor growth by up-regulating anti-apoptotic genes and promoting cell proliferation via a STAT-dependent pathway (Table 2) (112). IL-6 can facilitate the migration ability of OSCC cells undergoing EMT through the interaction of IL-6-ATATT3 axis and Rac1 axis (Table 2) (113). IL-6 and IL-8 may alter TME by recruiting inflammatory cells and reducing TIMP-1 production, and may serve as potential prognostic biomarkers for OSCC (Table 2) (114). OSCC-derived EVs can promote tumor proliferation and invasion by altering the levels of inflammatory cytokines in TME, and activating the IL-17/TRAF6/AP-1 signaling pathway (Table 2) (115).
7.4 Role of VEGF in OSCC
In OSCC, overexpressed VEGF participates in angiogenesis through the VEGF/VEGFR pathway, which is related to the occurrence of lymph node and distant metastasis and poor prognosis of OSCC (Table 2) (116).
Cytokines, including IL-8, TNF-α, TNF-β, CXCL9, CXCL10, CCL5, CCL17, CCL22, and IL-10, exhibit close correlations with the progression of OPMDs to OSCC by fostering inflammation, angiogenesis, and immune modulation. Similarly, TGF, IL, TNF, and VEGF play pivotal roles in OSCC development by stimulating the proliferation and migration of OSCC cells, modulating the immune response and angiogenesis, and influencing bone resorption. As a result, these cytokines hold promise as potential novel prognostic biomarkers for clinical diagnosis and treatment of OSCC. However, the precise role of various cytokines in oral squamous cell carcinoma (OSCC) remains incompletely understood. Therefore, future research is necessary to delve deeper into the specific mechanisms and effects of these cytokines in OSCC, with the aim of identifying potential targets for immunotherapy.
8 Hypoxia
8.1 Role of hypoxia in OSCC
Hypoxia biomarkers exhibit an association with early dysplastic epithelial changes, which indicates that hypoxia has a potential effect on oral carcinogenesis (117). Hypoxia exhibits a significant association with immune cell infiltration and gene mutations in OSCC (118). Hypoxic TME induces changes to histone acetylation and methylation in OSCC (119). Under hypoxia, the upregulated Tumor protein D52 (TPD52) expression at the center of OSCC is beneficial to cell viability and proliferation by inhibiting autophagic signal transduction (120). Overexpression of special AT-rich sequence-binding protein 2 (SATB2) in SCC9 cells induced by hypoxia increases the stemness of SCC9 cells and accelerates autophagy by affecting the level of Beclin-1 and the conversion of LC3-I (121). The level of regulated in development and DNA damage responses 1 (REDD1) that induces hypoxia considerably increases in OSCC leading to rising microvessel density and lymphatic metastasis (122). Suprabasin elevates the invasion, migration, and angiogenic ability of OSCC in hypoxia (123). Carbonic anhydrase 9 (CA9) is frequently expressed in TSCC due to hypoxia, which can regulate oncogenes and signaling pathways such as apoptosis, hypoxia, PI3K/AKR/mTOR signaling (124). The expression of Phosphoglycerate kinase 1 (PGK1) is also increased because of hypoxia, which engages the AKT signaling pathway to foster glycolysis, facilitate cell growth, and contribute to metastasis by promoting EMT (125). Vacuolar ATPase (V-ATPase), an enzyme that influences cellular glycolysis, primarily regulates potential of hydrogen (pH) within TME, and autophagy triggered by V-ATPase may contribute to the development of chemotherapy resistance in OSCC (126). Additionally, the inhibitory effect of odecylmethylaminoethyl methacrylate (DMAEM) on OSCC is enhanced by the low pH in hypoxia conditions (127).
8.2 Role of HIF-1α in OSCC
The positive correlation among the expression of CD44, HIF1α, and Snail in advancing grades of OSCC and their metastatic lymph suggests that there is a dynamic interaction between CSCs, hypoxia, and EMT, which facilitates the formation and maintenance of oral CSCs (128). Bharti et al. found the overexpression of HIF-1α in T1-staged OSCC (129). HIF-1α exhibits a crucial correlation with the deterioration of precancerous lesions and the poor prognosis of OSCC (130). Swartz et al. demonstrated the level of HIF-1α expression in OSCC cells is notably higher than that in laryngeal squamous cell carcinoma (LSCC) and oropharyngeal squamous cell carcinoma (OPSCC) (131). They found that HIF-1α had a positive correlation with better OS and DFS which is different from the finding in LSCC and OPSCC (131). There exists an important statistical correlation between the overexpression of HIF-1α and enlargement in tumor size, which means HIF-1α has a potential diagnostic role and maybe a novel auxiliary diagnostic indicator (132).
Hypoxia improves the sensitivity of HSC-2 to lactoferrin (LF) which is an iron-binding glycoprotein causing HIF-1α, HIF-2α, and p53 protein expression upregulated (133). Expression of HIF-1α can modulate the level of Par3 and TJs expression in OSCC, and the crosstalk between partitioning-defective protein 3 (Par3) and tight junctions (TJs) is disrupted during hypoxia, facilitating the metastasis of OSCC by upregulating MMP activity (134). The PER1/HIF-1α negative feedback loop facilitates ferroptosis and suppresses OSCC progression (135). MicroRNA-18a performs a detrimental function in the migration and invasive capabilities of OSCC cells by downregulating the level of HIF-1α (136). HIF-1α serves as a crucial hypoxia indicator that impacts the prognosis of OSCC by regulating TME and the function of OSCC cells. However, the influence of HIF-1α on prognosis remains controversial, necessitating an investigation into the pathway through which HIF-1α operates within the TME, ultimately targeting HIF-1α as a pivotal therapeutic focus.
8.3 Role of hypoxia-relative genes in OSCC
Lan et al. reported that hypoxia-relative genes contributed to the increase of CD68+/CD163+ TAMs and Albumin Human Serum-coated perfluorocarbon-carrying oxygen was an effective way to alleviate hypoxia (137). Hypoxia-related genes, aldolase A (ALDOA), prolyl 4-hydroxylase subunit alpha-1 (P4HA1), PGK1, and VEGFA, exhibit a significant correlation with OS in OSCC, which can predict the prognosis of patients (138). Lu and Liu found a positive correlation between phosphofructokinase, platelet (PFKP) and serpin peptidase inhibitor, clade E, member 1 (SERPINE1), which could also lead to poor prognosis (139). Furthermore, Han et al. revealed that the mutation of nuclear receptor binding SET domain protein 1 (NSD1) also indicated a high hypoxia potential index in OSCC (118). Hypoxia leads to elevated levels of miR-1825 in OSCC cells, promoting angiogenesis via the exosomal miR-1825/TSC2/mTOR signaling pathway (140). Piwi-interacting RNA-39980 inhibits the proliferation and migration of TSCC by suppressing the oncogene farnesyl-diphosphate farnesyltransferase 1 (FDFT1), disrupting ROS production, fostering a hypoxic TME, elevating levels of damaged DNA, and ultimately triggering cell death (141). In hypoxic conditions, hsa_circRNA_101036 boosts endoplasmic reticulum (ER) stress via the miR-21-3p/transmembrane and tetratricopeptide repeat containing 1 (TMTC1) pathway, ultimately causing cell apoptosis and generating ROS that can be removed by peroxiredoxin 5 (142, 143).
In hypoxic TME, hypoxia-relative genes facilitate cell death by modulating ROS levels, resulting in the inhibition the proliferation and migration. Meanwhile, Hypoxia promoting angiogenesis via the increased exosomal miR-1825. Conversely, the upregulation of numerous hypoxia-relative genes leads to an increase in microvascular density or enhances the serum’s oxygen-carrying capacity, thereby providing adequate nourishment and oxygen to OSCC.
9 Conclusion
TME has a significant impact on the regulation of OSCC initiation, development, and progression. Various cells in TME form an immunosuppressive environment to promote OSCC angiogenesis, proliferation, invasion, and migration through the cellular network with excretive secreting RNAs, proteins, cytokines, and metabolites. Therefore, it is pivotal to explore the interaction between cancer cells and the immune system in TME for achieving effective treatment of OSCC.A profound understanding of the crosstalk between tumor cells and various cell types in TME may allow the development of novel treatment strategies to relieve immunosuppression in OSCC. It has been reported that numerous immunotherapies targeting TME have been investigated for colon, breast, gastric, and lung cancer, while few have looked at therapies relevant to OSCC. Thus, more investigation needs to be advanced to offer new perspectives on treatment strategies targeting TME, including the complicated cellular network and factors that mediate the dynamic loop.
Author contributions
CL: Writing – original draft. XD: Writing – review & editing. BL: Writing – review & editing.
Funding
The author(s) declare financial support was received for the research, authorship, and/or publication of this article. This research was funded by Natural Science Foundation of Jilin Province (YDZJ202401228ZYTS).
Conflict of interest
The authors declare that the research was conducted in the absence of any commercial or financial relationships that could be construed as a potential conflict of interest.
Publisher’s note
All claims expressed in this article are solely those of the authors and do not necessarily represent those of their affiliated organizations, or those of the publisher, the editors and the reviewers. Any product that may be evaluated in this article, or claim that may be made by its manufacturer, is not guaranteed or endorsed by the publisher.
References
1. Sung H, Ferlay J, Siegel RL, Laversanne M, Soerjomataram I, Jemal A, et al. Global cancer statistics 2020: GLOBOCAN estimates of incidence and mortality worldwide for 36 cancers in 185 countries. CA Cancer J Clin. (2021) 71:209–49. doi: 10.3322/caac.21660
2. Johnson DE, Burtness B, Leemans CR, Lui VWY, Bauman JE, Grandis JR. Head and neck squamous cell carcinoma. Nat Rev Dis Primers. (2020) 6:92. doi: 10.1038/s41572-020-00224-3
3. Shen Y, Chen Y, Lin Y, Li Y, Liu P, Zhang B, et al. CDK5RAP2 is a Wnt target gene and promotes stemness and progression of oral squamous cell carcinoma. Cell Death Dis. (2023) 14:107. doi: 10.1038/s41419-023-05652-z
4. Chandralekha Selvakumar S, Auxzilia Preethi K, Sekar D. MicroRNAs and cancer-associated fibroblasts in the tumour microenvironment of oral squamous cell carcinoma (OSCC). Oral Oncol. (2022) 134:106124. doi: 10.1016/j.oraloncology.2022.106124
5. Chen SH, Hsiao SY, Chang KY, Chang JY. New insights into oral squamous cell carcinoma: from clinical aspects to molecular tumorigenesis. Int J Mol Sci. (2021) 22:2252. doi: 10.3390/ijms22052252
6. Biffi G, Tuveson DA. Diversity and biology of cancer-associated fibroblasts. Physiol Rev. (2021) 101:147–76. doi: 10.1152/physrev.00048.2019
7. Wu Y, Yuan M, Wang C, Chen Y, Zhang Y, Zhang J. T lymphocyte cell: A pivotal player in lung cancer. Front Immunol. (2023) 14:1102778. doi: 10.3389/fimmu.2023.1102778
8. John S, Joseph AP, Pillai VBR, Ramani P, Jayanthi P, Ramalingam K. Evaluation of cytotoxic T lymphocytes and natural killer cell distribution in oral squamous cell carcinoma and oral epithelial dysplasia: an immunohistochemical study. Cureus J Med Sci. (2024) 16:7. doi: 10.7759/cureus.56323
9. Wu JE, Li Y, Hou J. Downregulation of SLC3A2 mediates immune evasion and accelerates metastasis in oral squamous cell carcinoma. J Cell Mol Med. (2023) 28:e18010. doi: 10.1111/jcmm.18010
10. Fraga M, Yáñez M, Sherman M, Llerena F, Hernandez M, Nourdin G, et al. Immunomodulation of T helper cells by tumor microenvironment in oral cancer is associated with CCR8 expression and rapid membrane vitamin D signaling pathway. Front Immunol. (2021) 12:643298. doi: 10.3389/fimmu.2021.643298
11. Lyu H, Li M, Jiang Z, Liu Z, Wang X. Correlate the TP53 mutation and the HRAS mutation with immune signatures in head and neck squamous cell cancer. Comput Struct Biotechnol J. (2019) 17:1020–30. doi: 10.1016/j.csbj.2019.07.009
12. Shi Y, Ren X, Cao S, Chen X, Yuan B, Brasil da Costa FH, et al. TP53 gain-of-function mutation modulates the immunosuppressive microenvironment in non-HPV-associated oral squamous cell carcinoma. J Immunother Cancer. (2023) 11:15. doi: 10.1136/jitc-2023-006666
13. Gao J, Liang Y, Wang L. Shaping polarization of tumor-associated macrophages in cancer immunotherapy. Front Immunol. (2022) 13:888713. doi: 10.3389/fimmu.2022.888713
14. Nadella V, Garg M, Kapoor S, Barwal TS, Jain A, Prakash H. Emerging neo adjuvants for harnessing therapeutic potential of M1 tumor associated macrophages (TAM) against solid tumors: Enusage of plasticity. Ann Transl Med. (2020) 8:1029. doi: 10.21037/atm-20-695
15. Anderson NR, Minutolo NG, Gill S, Klichinsky M. Macrophage-based approaches for cancer immunotherapy. Cancer Res. (2021) 81:1201–8. doi: 10.1158/0008-5472.CAN-20-2990
16. Li Z, Liu FY, Kirkwood KL. The p38/MKP-1 signaling axis in oral cancer: Impact of tumor-associated macrophages. Oral Oncol. (2020) 103:104591. doi: 10.1016/j.oraloncology.2020.104591
17. Ono K, Sogawa C, Kawai H, Tran MT, Taha EA, Lu Y, et al. Triple knockdown of CDC37, HSP90-alpha and HSP90-beta diminishes extracellular vesicles-driven Malignancy events and macrophage M2 polarization in oral cancer. J Extracell Vesicles. (2020) 9:1769373. doi: 10.1080/20013078.2020.1769373
18. Kai K, Moriyama M, Haque A, Hattori T, Chinju A, Hu C, et al. Oral squamous cell carcinoma contributes to differentiation of monocyte-derived tumor-associated macrophages via PAI-1 and IL-8 production. Int J Mol Sci. (2021) 22:9475. doi: 10.3390/ijms22179475
19. Zhou WH, Wang Y, Yan C, Du WD, Al-Aroomi MA, Zheng L, et al. CC chemokine receptor 7 promotes macrophage recruitment and induces M2-polarization through CC chemokine ligand 19&21 in oral squamous cell carcinoma. Discovery Oncol. (2022) 13:67. doi: 10.1007/s12672-022-00533-x
20. Shan Q, Takabatake K, Kawai H, Oo MW, Sukegawa S, Fujii M, et al. Crosstalk between cancer and different cancer stroma subtypes promotes the infiltration of tumor−associated macrophages into the tumor microenvironment of oral squamous cell carcinoma. Int J Oncol. (2022) 60:78. doi: 10.3892/ijo.2022.5368
21. Wen JL, Yin PP, Su Y, Gao F, Wu YL, Zhang WB, et al. Knockdown of HMGB1 inhibits the crosstalk between oral squamous cell carcinoma cells and tumor-associated macrophages. Int Immunopharmacol. (2023) 119:110259. doi: 10.1016/j.intimp.2023.110259
22. Xiao M, Zhang J, Chen W, Chen W. M1-like tumor-associated macrophages activated by exosome-transferred THBS1 promote Malignant migration in oral squamous cell carcinoma. J Exp Clin Canc Res. (2018) 37:143. doi: 10.1186/s13046-018-0815-2
23. Li J, Bao Y, Peng S, Jiang C, Zhu L, Zou S, et al. M2 macrophages-derived exosomal miRNA-23a-3p promotes the progression of oral squamous cell carcinoma by targeting PTEN. Curr Issues Mol Biol. (2023) 45:4936–47. doi: 10.3390/cimb45060314
24. Wang L, Wang C, Tao Z, Zhu W, Su Y, Choi WS. Tumor-associated macrophages facilitate oral squamous cell carcinomas migration and invasion by MIF/NLRP3/IL-1β circuit: A crosstalk interrupted by melatonin. Biochim Biophys Acta Mol Basis Dis. (2023) 1869:166695. doi: 10.1016/j.bbadis.2023.166695
25. Guo XY, Zhang JY, Shi XZ, Wang Q, Shen WL, Zhu WW, et al. Upregulation of CSF-1 is correlated with elevated TAM infiltration and poor prognosis in oral squamous cell carcinoma. Am J Transl Res. (2020) 12:6235–49.
26. Lin Y, Qi Y, Jiang M, Huang W, Li B. Lactic acid-induced M2-like macrophages facilitate tumor cell migration and invasion via the GPNMB/CD44 axis in oral squamous cell carcinoma. Int Immunopharmacol. (2023) 124:110972. doi: 10.1016/j.intimp.2023.110972
27. Liu Z, Rui T, Lin Z, Xie S, Zhou B, Fu M, et al. Tumor-associated macrophages promote metastasis of oral squamous cell carcinoma via CCL13 regulated by stress granule. Cancers (Basel). (2022) 14:5081. doi: 10.3390/cancers14205081
28. Jiang M, Liu L, Huang W, Qi Y, Li Y, Li B. HMGB1-activated tumor-associated macrophages promote migration and invasion via NF-κB/IL-6 signaling in oral squamous cell carcinoma. Int Immunopharmacol. (2024) 126:111200. doi: 10.1016/j.intimp.2023.111200
29. You Y, Tian Z, Du Z, Wu K, Xu G, Dai M, et al. M1-like tumor-associated macrophages cascade a mesenchymal/stem-like phenotype of oral squamous cell carcinoma via the IL6/Stat3/THBS1 feedback loop. J Exp Clin Cancer Res. (2022) 41:10. doi: 10.1186/s13046-021-02222-z
30. da Silva SD, Marchi FA, Su J, Yang L, Valverde L, Hier J, et al. Co-overexpression of TWIST1-CSF1 is a common event in metastatic oral cancer and drives biologically aggressive phenotype. Cancers (Basel). (2021) 13:153. doi: 10.3390/cancers13010153
31. Suárez-Sánchez FJ, Lequerica-Fernández P, Suárez-Canto J, Rodrigo JP, Rodriguez-Santamarta T, Domínguez-Iglesias F, et al. Macrophages in oral carcinomas: relationship with cancer stem cell markers and PD-L1 expression. Cancers (Basel). (2020) 12:1764. doi: 10.3390/cancers12071764
32. Wang P, Tao L, Yu Y, Wang Q, Ye P, Sun Y, et al. Oral squamous cell carcinoma cell-derived GM-CSF regulates PD-L1 expression in tumor-associated macrophages through the JAK2/STAT3 signaling pathway. Am J Cancer Res. (2023) 13:589–601.
33. Wu L, Ye S, Yao Y, Zhang C, Liu W. Oral cancer stem cell-derived small extracellular vesicles promote M2 macrophage polarization and suppress CD4(+) T-cell activity by transferring UCA1 and targeting LAMC2. Stem Cells Int. (2022) 2022:5817684. doi: 10.1155/2022/5817684
34. Lin Y, Zhang W, Liu L, Li W, Li Y, Li B. ENO1 promotes OSCC migration and invasion by orchestrating IL-6 secretion from macrophages via a positive feedback loop. Int J Mol Sci. (2023) 24:737. doi: 10.3390/ijms24010737
35. Anders CB, Smith H, Garret J, Ammons MCB. Metabolic immunomodulation, transcriptional regulation, and signal protein expression define the metabotype and effector functions of five polarized macrophage phenotypes. bioRxiv. (2020). doi: 10.1101/2020.03.10.985788
36. Polverini PJ, Nör F, Nör JE. Crosstalk between cancer stem cells and the tumor microenvironment drives progression of premalignant oral epithelium. Front Oral Health. (2022) 3:1095842. doi: 10.3389/froh.2022.1095842
37. Wang B, He Y, Wang B, Li J, Qin L. ALDH3A1 overexpression in OSCC inhibits inflammation via phospho-Ser727 at STAT3 in tumor-associated macrophages. Oral Dis. (2023) 29:1513–24. doi: 10.1111/odi.14161
38. He Y, Dong Y, Zhang X, Ding Z, Song Y, Huang X, et al. Lipid droplet-related PLIN2 in CD68(+) tumor-associated macrophage of oral squamous cell carcinoma: implications for cancer prognosis and immunotherapy. Front Oncol. (2022) 12:824235. doi: 10.3389/fonc.2022.824235
39. Kalogirou EM, Tosios KI, Christopoulos PF. The role of macrophages in oral squamous cell carcinoma. Front Oncol. (2021) 11:611115. doi: 10.3389/fonc.2021.611115
40. Dallavalasa S, Beeraka NM, Basavaraju CG, Tulimilli SV, Sadhu SP, Rajesh K, et al. The role of tumor associated macrophages (TAMs) in cancer progression, chemoresistance, angiogenesis and metastasis - current status. Curr Med Chem. (2021) 28:8203–36. doi: 10.2174/0929867328666210720143721
41. Li Z, Zhang L, Liu FY, Li P, He J, Kirkwood CL, et al. MKP-1 is required to limit myeloid-cell mediated oral squamous cell carcinoma progression and regional extension. Oral Oncol. (2021) 120:105401. doi: 10.1016/j.oraloncology.2021.105401
42. Zeng J, Ye Z, Shi S, Liang Y, Meng Q, Zhang Q, et al. Targeted inhibition of eIF5A(hpu) suppresses tumor growth and polarization of M2-like tumor-associated macrophages in oral cancer. Cell Death Dis. (2023) 14:579. doi: 10.1038/s41419-023-06109-z
43. Bruna F, Scodeller P. Pro-tumorigenic macrophage infiltration in oral squamous cell carcinoma and possible macrophage-aimed therapeutic interventions. Front Oncol. (2021) 11:675664. doi: 10.3389/fonc.2021.675664
44. Yuan G, Yang S, Yang S. RGS12 represses oral squamous cell carcinoma by driving M1 polarization of tumor-associated macrophages via controlling ciliary MYCBP2/KIF2A signaling. Int J Oral Sci. (2023) 15:11. doi: 10.1038/s41368-023-00216-5
45. Jiang M, Qi Y, Huang W, Lin Y, Li B. Curcumin Reprograms TAMs from a Protumor Phenotype towards an Antitumor Phenotype via Inhibiting MAO-A/STAT6 Pathway. Cells. (2022) 11:3473. doi: 10.3390/cells11213473
46. Huang X, Wang Y, Yang W, Dong J, Li L. Regulation of dietary polyphenols on cancer cell pyroptosis and the tumor immune microenvironment. Front Nutr. (2022) 9:974896. doi: 10.3389/fnut.2022.974896
47. Dan H, Liu S, Liu J, Liu D, Yin F, Wei Z, et al. RACK1 promotes cancer progression by increasing the M2/M1 macrophage ratio via the NF-κB pathway in oral squamous cell carcinoma. Mol Oncol. (2020) 14:795–807. doi: 10.1002/1878-0261.12644
48. Cheng Y, Song S, Wu P, Lyu B, Qin M, Sun Y, et al. Tumor associated macrophages and TAMs-based anti-tumor nanomedicines. Adv Healthc Mater. (2021) 10:e2100590. doi: 10.1002/adhm.202100590
49. Wei Y, Li R, Wang Y, Fu J, Liu J, Ma X. Nanomedicines targeting tumor cells or tumor-associated macrophages for combinatorial cancer photodynamic therapy and immunotherapy: strategies and influencing factors. Int J Nanomed. (2024) 19:10129–44. doi: 10.2147/IJN.S466315
50. Fernández-Mariño I, Anfray C, Crecente-Campo J, Maeda A, Ummarino A, Teijeiro-Valiño C, et al. Mannose-modified hyaluronic acid nanocapsules for the targeting of tumor-associated macrophages. Drug Delivery Transl Re. (2023) 13:1896–911. doi: 10.1007/s13346-022-01265-9
51. Wang Z, Yang Q, Tan Y, Tang Y, Ye J, Yuan B, et al. Cancer-associated fibroblasts suppress cancer development: the other side of the coin. Front Cell Dev Biol. (2021) 9:613534. doi: 10.3389/fcell.2021.613534
52. Arebro J, Lee CM, Bennewith KL, Garnis C. Cancer-associated fibroblast heterogeneity in Malignancy with focus on oral squamous cell carcinoma. Int J Mol Sci. (2024) 25:1300. doi: 10.3390/ijms25021300
53. Shoucair I, Mello FW, Jabalee J, Maleki S, Garnis C. The role of cancer-associated fibroblasts and extracellular vesicles in tumorigenesis. Int J Mol Sci. (2020) 21:6837. doi: 10.3390/ijms21186837
54. Meng Z, Li TJ, Li J, Ding SX, Liu YJ, Zhao GL, et al. LncRNAPVT1 is associated with cancer-associated fibroblasts proliferation through regulating TGF-βin oral squamous cell carcinoma. Immunol Invest. (2024) 53:1250–63. doi: 10.1080/08820139.2024.2395874
55. Mito I, Takahashi H, Kawabata-Iwakawa R, Horikawa M, Ida S, Tada H, et al. Tumor-derived exosomes elicit cancer-associated fibroblasts shaping inflammatory tumor microenvironment in head and neck squamous cell carcinoma. Oral Oncol. (2023) 136:106270. doi: 10.1016/j.oraloncology.2022.106270
56. Arebro J, Towle R, Lee CM, Bennewith KL, Garnis C. Extracellular vesicles promote activation of pro-inflammatory cancer-associated fibroblasts in oral cancer. Front Cell Dev Biol. (2023) 11:1240159. doi: 10.3389/fcell.2023.1240159
57. Li Y, Ma Z, Li W, Xu X, Shen P, Zhang SE, et al. PDPN(+) CAFs facilitate the motility of OSCC cells by inhibiting ferroptosis via transferring exosomal lncRNA FTX. Cell Death Dis. (2023) 14:759. doi: 10.1038/s41419-023-06280-3
58. Jin N, Jin N, Bu W, Li X, Liu L, Wang Z, et al. Long non-coding RNA TIRY promotes tumor metastasis by enhancing epithelial-to-mesenchymal transition in oral cancer. Exp Biol Med (Maywood). (2020) 245:585–96. doi: 10.1177/1535370220903673
59. He L, Guo J, Fan Z, Yang S, Zhang C, Cheng B, et al. Exosomal miR-146b-5p derived from cancer-associated fibroblasts promotes progression of oral squamous cell carcinoma by downregulating HIPK3. Cell Signal. (2023) 106:110635. doi: 10.1016/j.cellsig.2023.110635
60. You D, Wang Y, Xu J, Yang R, Wang W, Wang X, et al. MiR-3529-3p from PDGF-BB-induced cancer-associated fibroblast-derived exosomes promotes the Malignancy of oral squamous cell carcinoma. Discovery Oncol. (2023) 14:166. doi: 10.1007/s12672-023-00753-9
61. Ma X, Zhao D, Liu S, Zuo J, Wang W, Wang F, et al. FERMT2 upregulation in CAFs enhances EMT of OSCC and M2 macrophage polarization. Oral Dis. (2024) 30:991–1003. doi: 10.1111/odi.14610
62. Wang Y, Wang R, Li B, Huang Z, Zhao S, Chen S, et al. Cancer-associated fibroblasts in the invasive tumour front promote the metastasis of oral squamous cell carcinoma through MFAP5 upregulation. Gene. (2023) 876:147504. doi: 10.1016/j.gene.2023.147504
63. Zhang JY, Zhu WW, Wang MY, Zhai RD, Wang Q, Shen WL, et al. Cancer-associated fibroblasts promote oral squamous cell carcinoma progression through LOX-mediated matrix stiffness. J Transl Med. (2021) 19:513. doi: 10.1186/s12967-021-03181-x
64. Zhao H, Li R, Chen Y, Yang X, Shang Z. Stromal nicotinamide N-methyltransferase orchestrates the crosstalk between fibroblasts and tumour cells in oral squamous cell carcinoma: evidence from patient-derived assembled organoids. Oncogene. (2023) 42:1166–80. doi: 10.1038/s41388-023-02642-5
65. Liu X, Li J, Yang X, Li X, Kong J, Qi D, et al. Carcinoma-associated fibroblast-derived lysyl oxidase-rich extracellular vesicles mediate collagen crosslinking and promote epithelial-mesenchymal transition via p-FAK/p-paxillin/YAP signaling. Int J Oral Sci. (2023) 15:32. doi: 10.1038/s41368-023-00236-1
66. Haga K, Yamazaki M, Maruyama S, Kawaharada M, Suzuki A, Hoshikawa E, et al. Crosstalk between oral squamous cell carcinoma cells and cancer-associated fibroblasts via the TGF-β/SOX9 axis in cancer progression. Transl Oncol. (2021) 14:101236. doi: 10.1016/j.tranon.2021.101236
67. Yang W, Zhang S, Li T, Zhou Z, Pan J. Single-cell analysis reveals that cancer-associated fibroblasts stimulate oral squamous cell carcinoma invasion via the TGF-β/Smad pathway. Acta Biochim Biophys Sin (Shanghai). (2022) 55:262–73. doi: 10.3724/abbs.2022132
68. Zhang X, Dong Y, Zhao M, Ding L, Yang X, Jing Y, et al. ITGB2-mediated metabolic switch in CAFs promotes OSCC proliferation by oxidation of NADH in mitochondrial oxidative phosphorylation system. Theranostics. (2020) 10:12044–59. doi: 10.7150/thno.47901
69. Yang J, Shi X, Yang M, Luo J, Gao Q, Wang X, et al. Glycolysis reprogramming in cancer-associated fibroblasts promotes the growth of oral cancer through the lncRNA H19/miR-675-5p/PFKFB3 signaling pathway. Int J Oral Sci. (2021) 13:12. doi: 10.1038/s41368-021-00115-7
70. Xu J, Bian L, You D, Li Z, Wang T, Li Y, et al. PDGF-BB accelerates TSCC via fibroblast lactates limiting miR-26a-5p and boosting mitophagy. Cancer Cell Int. (2024) 24:5. doi: 10.1186/s12935-023-03172-6
71. Kayamori K, Katsube KI, Hirai H, Harada H, Ikeda T. Role of stromal fibroblast-induced WNT7A associated with cancer cell migration through the AKT/CLDN1 signaling axis in oral squamous cell carcinoma. Lab Invest. (2023) 103:100228. doi: 10.1016/j.labinv.2023.100228
72. Wang WZ, Cao X, Bian L, Gao Y, Yu M, Li YT, et al. Analysis of mRNA-miRNA interaction network reveals the role of CAFs-derived exosomes in the immune regulation of oral squamous cell carcinoma. BMC Cancer. (2023) 23:591. doi: 10.1186/s12885-023-11028-5
73. Sekiguchi S, Yorozu A, Okazaki F, Niinuma T, Takasawa A, Yamamoto E, et al. ACLP activates cancer-associated fibroblasts and inhibits CD8+ T-cell infiltration in oral squamous cell carcinoma. Cancers (Basel). (2023) 15:4303. doi: 10.3390/cancers15174303
74. Zhao X, Ding L, Lu Z, Huang X, Jing Y, Yang Y, et al. Diminished CD68(+) cancer-associated fibroblast subset induces regulatory T-cell (Treg) infiltration and predicts poor prognosis of oral squamous cell carcinoma patients. Am J Pathol. (2020) 190:886–99. doi: 10.1016/j.ajpath.2019.12.007
75. Maeda S, Nakazawa M, Uchida M, Yoshitake R, Nakagawa T, Nishimura R, et al. Foxp3+ Regulatory T cells associated with CCL17/CCR4 expression in carcinomas of dogs. Vet Pathol. (2020) 57:497–506. doi: 10.1177/0300985820921535
76. Liu ZQ, Zhang Z, Zhang Y, Zhou WK, Zhang X, Peng CB, et al. Spatial transcriptomics reveals that metabolic characteristics define the tumor immunosuppression microenvironment via iCAF transformation in oral squamous cell carcinoma. Int J Oral Sci. (2024) 16:12. doi: 10.1038/s41368-023-00267-8
77. Sugiyama E, Togashi Y, Takeuchi Y, Shinya S, Tada Y, Kataoka K, et al. Blockade of EGFR improves responsiveness to PD-1 blockade in EGFR-mutated non-small cell lung cancer. Sci Immunol. (2020) 5:13. doi: 10.1126/sciimmunol.aav3937
78. Piersiala K, da Silva PFN, Lagebro V, Kolev A, Starkhammar M, Elliot A, et al. Tumour-draining lymph nodes in head and neck cancer are characterized by accumulation of CTLA-4 and PD-1 expressing Treg cells. Trans Oncol. (2022) 23:10. doi: 10.1016/j.tranon.2022.101469
79. Chen J, Yang J, Li H, Yang Z, Zhang X, Li X, et al. Single-cell transcriptomics reveal the intratumoral landscape of infiltrated T-cell subpopulations in oral squamous cell carcinoma. Mol Oncol. (2021) 15:866–86. doi: 10.1002/1878-0261.12910
80. Chatzopoulos K, Sotiriou S, Collins AR, Kartsidis P, Schmitt AC, Chen X, et al. Transcriptomic and immunophenotypic characterization of tumor immune microenvironment in squamous cell carcinoma of the oral tongue. Head Neck Pathol. (2021) 15:509–22. doi: 10.1007/s12105-020-01229-w
81. Hayashi T, Yoshikawa K, Suzuki S, Gosho M, Ueda R, Kazaoka Y. Tumor-infiltrating FoxP3+ T cells are associated with poor prognosis in oral squamous cell carcinoma. Clin Exp Dent Res. (2022) 8:152–9. doi: 10.1002/cre2.v8.1
82. Koike K, Dehari H, Ogi K, Shimizu S, Nishiyama K, Sonoda T, et al. Prognostic value of FoxP3 and CTLA-4 expression in patients with oral squamous cell carcinoma. PloS One. (2020) 15:e0237465. doi: 10.1371/journal.pone.0237465
83. Li W, An N, Wang M, Liu X, Mei Z. Interleukin-23 receptor defines T helper 1-like regulatory T cells in oral squamous cell carcinoma. Immun Inflammation Dis. (2022) 10:e746. doi: 10.1002/iid3.v10.12
84. Chen H, Sameshima J, Yokomizo S, Sueyoshi T, Nagano H, Miyahara Y, et al. Expansion of CD4+ cytotoxic T lymphocytes with specific gene expression patterns may contribute to suppression of tumor immunity in oral squamous cell carcinoma: single-cell analysis and in vitro experiments. Front Immunol. (2023) 14:1305783. doi: 10.3389/fimmu.2023.1305783
85. Wu K, Han N, Mao Y, Li Y. Increased levels of PD1 and glycolysis in CD4(+) T cells are positively associated with lymph node metastasis in OSCC. BMC Oral Health. (2023) 23:356. doi: 10.1186/s12903-023-03043-6
86. Dong Y, Wang Z, Mao F, Cai L, Dan H, Jiang L, et al. PD-1 blockade prevents the progression of oral carcinogenesis. Carcinogenesis. (2021) 42:891–902. doi: 10.1093/carcin/bgab035
87. Ito N, Yamasaki S, Shintani T, Matsui K, Obayashi F, Koizumi K, et al. Tumor-infiltrating CD45RO(+) memory cells are associated with favorable prognosis in oral squamous cell carcinoma patients. Cancers (Basel). (2023) 15:2221. doi: 10.3390/cancers15082221
88. Bhosale PG, Kennedy RA, Watt FM. Caspase activation in tumour-infiltrating lymphocytes is associated with lymph node metastasis in oral squamous cell carcinoma. J Pathol. (2023) 261:43–54. doi: 10.1002/path.v261.1
89. Cui Y, Cheng Y, Huang W, Liu J, Zhang X, Bu M, et al. A novel T-cell proliferation-associated gene predicts prognosis and reveals immune infiltration in patients with oral squamous cell carcinoma. Arch Oral Biol. (2023) 152:105719. doi: 10.1016/j.archoralbio.2023.105719
90. Taucher E, Taucher V, Fink-Neuboeck N, Lindenmann J, Smolle-Juettner FM. Role of tumor-associated neutrophils in the molecular carcinogenesis of the lung. Cancers (Basel). (2021) 13:5972. doi: 10.3390/cancers13235972
91. Wu L, Zhang XH. Tumor-associated neutrophils and macrophages-heterogenous but not chaotic. Front Immunol. (2020) 11:553967. doi: 10.3389/fimmu.2020.553967
92. Mantovani A, Marchesi F, Jaillon S, Garlanda C, Allavena P. Tumor-associated myeloid cells: diversity and therapeutic targeting. Cell Mol Immunol. (2021) 18:566–78. doi: 10.1038/s41423-020-00613-4
93. Hu X, Xiang F, Feng Y, Gao F, Ge S, Wang C, et al. Neutrophils promote tumor progression in oral squamous cell carcinoma by regulating EMT and JAK2/STAT3 signaling through chemerin. Front Oncol. (2022) 12:812044. doi: 10.3389/fonc.2022.812044
94. Rubenich DS, Omizzollo N, Szczepański MJ, Reichert TE, Whiteside TL, Ludwig N, et al. Small extracellular vesicle-mediated bidirectional crosstalk between neutrophils and tumor cells. Cytokine Growth Factor Rev. (2021) 61:16–26. doi: 10.1016/j.cytogfr.2021.08.002
95. Domnich M, Riedesel J, Pylaeva E, Kürten CHL, Buer J, Lang S, et al. Oral neutrophils: underestimated players in oral cancer. Front Immunol. (2020) 11:565683. doi: 10.3389/fimmu.2020.565683
96. Jablonska E, Iwaniuk A, Ratajczak-Wrona W, Grubczak K, Dziemianczyk-Pakiela D, Moniuszko M, et al. The promoting effect of neutrophil-derived BAFF molecule on the proliferation and life span of CAL-27 oral squamous carcinoma cells. Immunobiology. (2022) 227:152247. doi: 10.1016/j.imbio.2022.152247
97. Lonardi S, Missale F, Calza S, Bugatti M, Vescovi R, Debora B, et al. Tumor-associated neutrophils (TANs) in human carcinoma-draining lymph nodes: a novel TAN compartment. Clin Transl Immunol. (2021) 10:e1252. doi: 10.1002/cti2.v10.2
98. Hadjigol S, Shah BA, O’Brien-Simpson NM. The ‘Danse macabre’-neutrophils the interactive partner affecting oral cancer outcomes. Front Immunol. (2022) 13:894021. doi: 10.3389/fimmu.2022.894021
99. He Y, Qu Y, Jin S, Zhang Y, Qin L. ALDH3A1 upregulation inhibits neutrophils N2 polarization and halts oral cancer growth. Oral Dis. (2024) 30:4231–42. doi: 10.1111/odi.14863
100. Veglia F, Perego M, Gabrilovich D. Myeloid-derived suppressor cells coming of age. Nat Immunol. (2018) 19:108–19. doi: 10.1038/s41590-017-0022-x
101. Ma T, Renz BW, Ilmer M, Koch D, Yang Y, Werner J, et al. Myeloid-derived suppressor cells in solid tumors. Cells. (2022) 11:310. doi: 10.3390/cells11020310
102. Pang X, Fan HY, Tang YL, Wang SS, Cao MX, Wang HF, et al. Myeloid derived suppressor cells contribute to the Malignant progression of oral squamous cell carcinoma. PloS One. (2020) 15:e0229089. doi: 10.1371/journal.pone.0229089
103. Kouketsu A, Haruka S, Kuroda K, Hitoshi M, Kensuke Y, Tsuyoshi S, et al. Myeloid-derived suppressor cells and plasmacytoid dendritic cells are associated with oncogenesis of oral squamous cell carcinoma. J Oral Pathol Med. (2023) 52:9–19. doi: 10.1111/jop.13386
104. Jiang Y, Wang C, Wang Y, Zhang W, Liu L, Cheng J. Prognostic role of CD11b(+) myeloid-derived suppressor cells in oral squamous cell carcinoma. Arch Med Sci. (2023) 19:171–9. doi: 10.5114/aoms/116683
105. Babiuch K, Kuśnierz-Cabala B, Kęsek B, Okoń K, Darczuk D, Chomyszyn-Gajewska M. Evaluation of proinflammatory, NF-kappaB dependent cytokines: IL-1α, IL-6, IL-8, and TNF-α in tissue specimens and saliva of patients with oral squamous cell carcinoma and oral potentially Malignant disorders. J Clin Med. (2020) 9:867. doi: 10.3390/jcm9030867
106. Gupta S, Nigam K, Srivastav RK, Ahmad MK, Mahdi AA, Sanyal S. Genetic polymorphism of tumor necrosis factor alpha (TNF-α) and tumor necrosis factor beta (TNF-β) genes and risk of oral pre-cancer and cancer in North Indian population. Oral Maxillofac Surg. (2022) 26:33–43. doi: 10.1007/s10006-020-00929-5
107. Stamova S, Ott-Rötzer B, Smetak H, Schäffler K, Eder R, Fink I, et al. Characterization and ex vivo expansion of rare in situ cytokine secreting T cell populations from tumor tissue and blood of oral squamous cell carcinoma patients. J Immunol Methods. (2021) 496:113086. doi: 10.1016/j.jim.2021.113086
108. Ferreira KDM, Tramontani Ramos R, Stofela Sodré C, Nivoloni Tannure P, Armada L, de Souza Gonçalves L, et al. Pro-and anti-inflammatory biomarkers as potential prognosis markers in oral squamous cell carcinoma. Int J odontostomatol. (2022) 16:258–66. doi: 10.4067/S0718-381X2022000200258
109. Yoshimatsu Y, Wakabayashi I, Kimuro S, Takahashi N, Takahashi K, Kobayashi M, et al. TNF-α enhances TGF-β-induced endothelial-to-mesenchymal transition via TGF-β signal augmentation. Cancer Sci. (2020) 111:2385–99. doi: 10.1111/cas.v111.7
110. Maldonado LAG, Nascimento CR, Rodrigues Fernandes NA, Silva ALP, D’Silva NJ, Rossa C Jr. Influence of tumor cell-derived TGF-β on macrophage phenotype and macrophage-mediated tumor cell invasion. Int J Biochem Cell Biol. (2022) 153:106330. doi: 10.1016/j.biocel.2022.106330
111. Lopez-Labady J, Bologna-Molina R, Villarroel-Dorrego M. Expression of interleukin-1ß and interleukin-8 in oral potentially Malignant disorders and carcinomas. Front Oral Health. (2021) 2:649406. doi: 10.3389/froh.2021.649406
112. Wei LY, Lin HC, Tsai FC, Ko JY, Kok SH, Cheng SJ, et al. Effects of Interleukin-6 on STAT3-regulated signaling in oral cancer and as a prognosticator of patient survival. Oral Oncol. (2022) 124:105665. doi: 10.1016/j.oraloncology.2021.105665
113. Alves A, Diel L, Ramos G, Pinto A, Bernardi L, Yates J 3rd, et al. Tumor microenvironment and Oral Squamous Cell Carcinoma: A crosstalk between the inflammatory state and tumor cell migration. Oral Oncol. (2021) 112:105038. doi: 10.1016/j.oraloncology.2020.105038
114. Radulescu R, Totan AR, Imre MM, Miricescu D, Didilescu A, Greabu M. Mediators of extracellular matrix degradation and inflammation: A new team of possible biomarkers for oral squamous cell carcinoma stage. Exp Ther Med. (2021) 22:877. doi: 10.3892/etm.2021.10309
115. Li R, Zhou Y, Zhang M, Xie R, Duan N, Liu H, et al. Oral squamous cell carcinoma-derived EVs promote tumor progression by regulating inflammatory cytokines and the IL-17A-induced signaling pathway. Int Immunopharmacol. (2023) 118:110094. doi: 10.1016/j.intimp.2023.110094
116. Niklander S, Bordagaray MJ, Fernández A, Hernández M. Vascular endothelial growth factor: A translational view in oral non-communicable diseases. Biomolecules. (2021) 11:85. doi: 10.3390/biom11010085
117. Kujan O, Siddiqui I, Lee C, Idrees M, Shearston K, Farah CS. Automated immunohistochemical quantification of hypoxia biomarkers shows correlation with dysplastic epithelial changes. J Oral Pathol Med. (2023) 52:504–13. doi: 10.1111/jop.13427
118. Han Y, Wang X, Xia K, Su T. A novel defined hypoxia-related gene signature to predict the prognosis of oral squamous cell carcinoma. Ann Transl Med. (2021) 9:1565. doi: 10.21037/atm-21-4990
119. Khan T, Iftikhar F, Akhlaq R, Musharraf SG, Ali A. Acidotic and hypoxic tumor microenvironment induces changes to histone acetylation and methylation in oral squamous cell carcinoma. BioMed Chromatogr. (2023) 37:e5616. doi: 10.1002/bmc.v37.6
120. Abe Y, Mukudai Y, Kurihara M, Houri A, Chikuda J, Yaso A, et al. Tumor protein D52 is upregulated in oral squamous carcinoma cells under hypoxia in a hypoxia-inducible-factor-independent manner and is involved in cell death resistance. Cell Biosci. (2021) 11:122. doi: 10.1186/s13578-021-00634-0
121. Dong W, Chen Y, Qian N, Sima G, Zhang J, Guo Z, et al. SATB2 knockdown decreases hypoxia-induced autophagy and stemness in oral squamous cell carcinoma. Oncol Lett. (2020) 20:794–802. doi: 10.3892/ol.2020.11589
122. Feng Y, Song K, Shang W, Chen L, Wang C, Pang B, et al. REDD1 overexpression in oral squamous cell carcinoma may predict poor prognosis and correlates with high microvessel density. Oncol Lett. (2020) 19:431–41. doi: 10.3892/ol.2019.11070
123. Houri A, Mukudai Y, Abe Y, Watanabe M, Nara M, Miyamoto S, et al. Suprabasin enhances the invasion, migration, and angiogenic ability of oral squamous cell carcinoma cells under hypoxic conditions. Oncol Rep. (2023) 49:83. doi: 10.3892/or.2023.8520
124. Guan C, Ouyang D, Qiao Y, Li K, Zheng G, Lao X, et al. CA9 transcriptional expression determines prognosis and tumour grade in tongue squamous cell carcinoma patients. J Cell Mol Med. (2020) 24:5832–41. doi: 10.1111/jcmm.v24.10
125. Zhang Y, Cai H, Liao Y, Zhu Y, Wang F, Hou J. Activation of PGK1 under hypoxic conditions promotes glycolysis and increases stem cell−like properties and the epithelial−mesenchymal transition in oral squamous cell carcinoma cells via the AKT signalling pathway. Int J Oncol. (2020) 57:743–55. doi: 10.3892/ijo.2020.5083
126. Lagzian A, Askari M, Haeri MS, Sheikhi N, Banihashemi S, Nabi-Afjadi M, et al. Increased V-ATPase activity can lead to chemo-resistance in oral squamous cell carcinoma via autophagy induction: new insights. Med Oncol. (2024) 41:13. doi: 10.1007/s12032-024-02313-9
127. Liu SY, Chen J, Zhou XD, Hao Y, Zong YW, Shi YY, et al. A pH-sensitive smart monomer prevents oral cancer progression. Adv NanoBiomed Res. (2024) 4:2300119. doi: 10.1002/anbr.202300119
128. Varsha VK, Savita JK, Girish HC, Shyamala K. Role of hypoxia and epithelial-mesenchymal transition in the formation and maintenance of oral cancer stem cells in oral squamous cell carcinomas and metastatic lymph node: An immunohistochemical analysis. J Oral Maxillofac Pathol. (2023) 27:307–14. doi: 10.4103/jomfp.jomfp_368_22
129. Bharti A, Urs AB, Kumar P. Significance of HIF-1α Expression and LOXL-2 localization in progression of oral squamous cell carcinoma. Asian Pac J Cancer Prev. (2021) 22:341–7. doi: 10.31557/APJCP.2021.22.2.341
130. Eckert AW, Kappler M, Große I, Wickenhauser C, Seliger B. Current understanding of the HIF-1-dependent metabolism in oral squamous cell carcinoma. Int J Mol Sci. (2020) 21:6083. doi: 10.3390/ijms21176083
131. Swartz JE, Wegner I, Noorlag R, van Kempen PMW, van Es RJJ, de Bree R, et al. HIF-1a expression and differential effects on survival in patients with oral cavity, larynx, and oropharynx squamous cell carcinomas. Head Neck. (2021) 43:745–56. doi: 10.1002/hed.26530
132. Sumera S, Ali A, Yousafzai YM, Durrani Z, Alorini M, Aleem B, et al. Overexpression of hypoxia-inducible factor-1α and its relation with aggressiveness and grade of oral squamous cell carcinoma. Diagn (Basel). (2023) 13:451. doi: 10.3390/diagnostics13030451
133. Kosim MY, Fukazawa T, Miyauchi M, Hirohashi N, Tanimoto K. p53 status modifies cytotoxic activity of lactoferrin under hypoxic conditions. Front Pharmacol. (2022) 13:988335. doi: 10.3389/fphar.2022.988335
134. Kim S, Park S, Moon EH, Kim GJ, Choi J. Hypoxia disrupt tight junctions and promote metastasis of oral squamous cell carcinoma via loss of par3. Cancer Cell Int. (2023) 23:79. doi: 10.1186/s12935-023-02924-8
135. Yang YX, Tang H, Zheng JW, Yang K. The PER1/HIF-1alpha negative feedback loop promotes ferroptosis and inhibits tumor progression in oral squamous cell carcinoma. Trans Oncol. (2022) 18:101360. doi: 10.1016/j.tranon.2022.101360
136. Kim S, Park S, Oh JH, Lee SS, Lee Y, Choi J. MicroRNA-18a regulates the metastatic properties of oral squamous cell carcinoma cells via HIF-1α expression. BMC Oral Health. (2022) 22:378. doi: 10.1186/s12903-022-02425-6
137. Lan Z, Zou KL, Cui H, Chen H, Zhao YY, Yu GT. PFC@O(2) targets HIF-1α to reverse the immunosuppressive TME in OSCC. J Clin Med. (2023) 12:560. doi: 10.3390/jcm12020560
138. Zhao C, Zhou Y, Ma H, Wang J, Guo H, Liu H. A four-hypoxia-genes-based prognostic signature for oral squamous cell carcinoma. BMC Oral Health. (2021) 21:232. doi: 10.1186/s12903-021-01587-z
139. Lu X, Liu X, Jain DK. Establishment and analysis of hypoxia-related model in oral squamous cell carcinoma. J Healthc Eng. (2022) 2022:1–11. doi: 10.1155/2022/6602648
140. Capik O, Gumus R, Karatas OF. Hypoxia-induced tumor exosomes promote angiogenesis through miR-1825/TSC2/mTOR axis in oral squamous cell carcinoma. Head Neck. (2023) 45:2259–73. doi: 10.1002/hed.27460
141. Chattopadhyay T, Mallick B. FDFT1 repression by piR-39980 prevents oncogenesis by regulating proliferation and apoptosis through hypoxia in tongue squamous cell carcinoma. Life Sci. (2023) 329:19. doi: 10.1016/j.lfs.2023.121954
142. Deng W, Fu J, Lin SG, Wen QT, Fu LB, Chen XZ. Hsa_circRNA_101036 aggravates hypoxic-induced endoplasmic reticulum stress via the miR-21-3p/TMTC1 axis in oral squamous cell carcinoma. Heliyon. (2024) 10:14. doi: 10.1016/j.heliyon.2024.e32969
Keywords: oral squamous cell carcinoma, tumor microenvironment, tumor-associated macrophages, interaction, cellular network
Citation: Li C, Dong X and Li B (2024) Tumor microenvironment in oral squamous cell carcinoma. Front. Immunol. 15:1485174. doi: 10.3389/fimmu.2024.1485174
Received: 23 August 2024; Accepted: 02 December 2024;
Published: 18 December 2024.
Edited by:
Eyad Elkord, Xi’an Jiaotong-Liverpool University, ChinaReviewed by:
Remya Raja, Mayo Clinic Arizona, United StatesRoberto Rangel, University of Texas MD Anderson Cancer Center, United States
Copyright © 2024 Li, Dong and Li. This is an open-access article distributed under the terms of the Creative Commons Attribution License (CC BY). The use, distribution or reproduction in other forums is permitted, provided the original author(s) and the copyright owner(s) are credited and that the original publication in this journal is cited, in accordance with accepted academic practice. No use, distribution or reproduction is permitted which does not comply with these terms.
*Correspondence: Bo Li, bGJvQGpsdS5lZHUuY24=