- 1BIOCEV, First Faculty of Medicine, Charles University, Vestec, Czechia
- 2Department of Paediatrics and Inherited Metabolic Disorders, First Faculty of Medicine, Charles University and General University Hospital, Prague, Czechia
- 3Section on Medical Neuroendocrinology, Eunice Kennedy Shriver National Institute of Child Health and Human Development, National Institutes of Health, Bethesda, MD, United States
- 4Department of Pathological Physiology, Faculty of Medicine, Masaryk University, Brno, Czechia
- 5Department of Physiology, Faculty of Medicine, Masaryk University, Brno, Czechia
Despite enormous progress, advanced cancers are still one of the most serious medical problems in current society. Although various agents and therapeutic strategies with anticancer activity are known and used, they often fail to achieve satisfactory long-term patient outcomes and survival. Recently, immunotherapy has shown success in patients by harnessing important interactions between the immune system and cancer. However, many of these therapies lead to frequent side effects when administered systemically, prompting treatment modifications or discontinuation or, in severe cases, fatalities. New therapeutic approaches like intratumoral immunotherapy, characterized by reduced side effects, cost, and systemic toxicity, offer promising prospects for future applications in clinical oncology. In the context of locally advanced or metastatic cancer, combining diverse immunotherapeutic and other treatment strategies targeting multiple cancer hallmarks appears crucial. Such combination therapies hold promise for improving patient outcomes and survival and for promoting a sustained systemic response. This review aims to provide a current overview of immunotherapeutic approaches, specifically focusing on the intratumoral administration of drugs in patients with locally advanced and metastatic cancers. It also explores the integration of intratumoral administration with other modalities to maximize therapeutic response. Additionally, the review summarizes recent advances in intratumoral immunotherapy and discusses novel therapeutic approaches, outlining future directions in the field.
1 Introduction
Today, cancer ranks as the second leading cause of mortality worldwide (1) with predicted incidence expected to reach 28.4 million cases by 2040 (2). Locally advanced tumors (those that have significantly progressed in size or are often inoperable due to locoregional spread) and metastatic tumors (those that have spread to distant parts of the body) (3) are the primary causes of cancer-related death (4). According to the Surveillance, Epidemiology, and End Results database (2014–2020), poor 5-year survival rates are documented for metastatic and regional diseases, such as 99.6% for localized, 86.7% for regional, and 31.9% for distant female breast cancer (5).
One of the main challenges in cancer treatment is finding effective modalities to combat both primary and distant metastatic tumors, as well as addressing post-treatment minimal residual disease (MRD), which includes small cancer cell clusters, micrometastasis, or even single cancer cells. While advances in liquid biopsy for detecting MRD are promising in hematological malignancies, they remain challenging for many solid tumors (6, 7). The unpredictable development of metastasis, proximity to vital organs or vessels, as well as large tumor size often complicate the treatment of advanced cancers (3). Generally, the size of a lesion, the number of affected lymph nodes, and the extent of metastasis correlate with a worse prognosis, also known as the tumor, node, and metastasis (TNM) staging system (8).
Current treatment options for locally advanced and metastatic cancer include systemic and local therapies or their combinations, depending on the type, localization, and stage of cancer progression (9–14). According to the National Cancer Institute, Bethesda, Maryland, systemic cancer treatment nonspecifically travels through the bloodstream and affects both cancerous and healthy cells (15), while local therapies target cancer cells with reduced toxicity to nearby and distant healthy cells (16). Systemic treatment options include chemotherapy, immunotherapy, hormonal, and specifically targeted therapies (17), such as epigenetic drugs, tyrosine kinase inhibitors, or anti-angiogenesis drugs (18). Local treatments comprise surgery, radiotherapy (9, 13, 19–21), photodynamic (22), and ablation therapies (9, 13, 21).
Although side effects are observed with both treatment types (23, 24), systemic therapies are often the leading cause of bone marrow suppression, gastrointestinal dysfunction, endocrine abnormalities (25), and immune-related adverse events (26). Given these challenges, there is an urgent need for new treatment strategies and combinations that lower side effects, achieve synergistic or additive activity, and increase efficacy for treating locally advanced and metastatic cancer (27). This review provides an up-to-date overview of current immunotherapeutic and other treatment approaches, focusing on intratumoral administration for the treatment of locally advanced and metastatic cancer. We emphasize combination therapy, where intratumoral administration is paired with other modalities, to improve the overall therapeutic response. By examining the latest advancements, particularly in immunotherapy, we also discuss novel, promising therapeutic approaches for the near future.
2 Cancer immunotherapy
The involvement of the immune system in tumor development was first proposed in 1909. This idea was further studied about 50 years later by F. M. Burnet and L. Thomas. They hypothesized that tumor neoantigens could trigger T cell immune response to eliminate cancer cells (28). This hypothesis was validated in the 1990s by numerous experiments, extending to the interplay between innate and adaptive immunity necessary for efficient tumor eradication (29–31) and the generation of immunological memory to prevent disease recurrence (32).
Cancer immunotherapy is a promising approach that enhances tumor immunogenicity and stimulates the immune response against cancer cells (33, 34). For decades, researchers have explored ways to stimulate or inhibit immune response to fight cancer. Currently, several types of immunotherapies are recognized, including cancer vaccines (e.g., dendritic cell, peptide/protein, gene (35), viral (36), oncolytic viral (37), or repurposed viral vaccines) (35), monoclonal antibodies and checkpoint inhibitors (ICIs) (38), adoptive cell therapies (39, 40), and immunomodulators (e.g., cytokines, pattern recognition receptor (PRR) and stimulator of interferon genes (STING) agonists, and vaccine adjuvants) (41). Based on their modulation of the immune system, they can be categorized as either active or passive, although many immunotherapies exhibit overlaps. Active immunotherapy is based on a patient’s immunization with pathogen vaccines, tumor cells or their parts, ICIs, or cytokines, with a subsequent generation of various immune mediators and cells to destroy a tumor lesion, ultimately through effector T cells. Passive immunotherapy, on the other hand, includes the administration of ex vivo stimulated or modified immune cells, such as T cells, natural killer (NK) cells, or chimeric antigen receptor T cell therapy (CAR-T), as well as the administration of specific monoclonal antibodies, without the necessity to initiate the production of own immune mediators and cells to fight cancer (42, 43). Depending on their immunostimulatory activity, monoclonal antibodies can be considered either targeted therapy (44) or immunotherapy (45).
To date, numerous systemic immunotherapies have been approved for the treatment of advanced cancers (National Library of Medicine Database, European Medicine Agency Database). Systemic administration is essential for effectively managing metastatic disease (46), as it is both practically feasible (47) and ensures a broad distribution of therapeutic agents throughout the body (46). These primarily include ICIs and their combinations with chemotherapy, targeted therapy (e.g., vascular endothelial growth factor (VEGF), kinase, and epidermal growth factor receptor (EGFR) inhibitors), or additional ICI molecules. Other treatment options include, for instance, sipuleucel-T (FDA, viral therapy), aldesleukin (FDA, cytokine therapy), lifileucel (FDA, adoptive cell therapy), or enfortumab (FDA/EMA, anti-nektin-4 antibody). Although these therapies have been approved for managing advanced cancers, they are not combined with other treatment modalities.
3 Intratumoral immunotherapy
The beginning of intentional local treatments can be dated back to 1700 – 1800 AD (48), almost 5,000 years after the first cases were documented in old papyruses and treated (49). In the case of metastatic disease, the earliest evidence is from 1200 BC (50) (Figure 1). As with any of the standard cancer treatments, the introduction of immunotherapy, including the intratumoral approach, was a gradual process developing with new-gained knowledge and ever-changing discoveries in the biological field. Significant advances in cancer research and treatments began to emerge with the foundation of the first hospital for cancer patients, Sloan Kettering Institute (currently Memorial Sloan Kettering Cancer Center), in 1884 (51) (Figure 1). For instance, several cases describing tumor eradication after infections were reported by F. Fehleisen, W. Bush (52), and W. Coley (52–54). Although not well understood at that time, Coley’s work illuminated the potential of direct tumor injection to stimulate the immune system to fight both primary tumors and metastases (38). Furthermore, the “seed and soil” hypothesis of organ-specific metastatic dissemination was proposed (55) (Figure 1). A few years after the postulation that the immune system could reduce tumor growth (28), sterile procedures (56, 57) were introduced, leaving W. Coley misunderstood and forgotten. Additionally, the first metastatic mouse melanoma model was established (58). With further scientific advances, hallmarks of cancer (59) and the neoadjuvant approach were introduced (60), immunotherapy was named the Breakthrough of 2013 (61), and itRECIST criteria, i.e., recommendations for the assessment of intratumoral immunotherapy clinical trials, was proposed (in lorange) (62) (Figure 1). The induction of systemic immune response against distal untreated lesions, better known as abscopal or anesthetic effects, was sometimes reported during better-defined radiotherapy (63, 64). For a long time, only systemic treatment was considered necessary to reach all metastatic lesions. However, to specifically target cancer cells, reduce off-target toxicities, and increase treatment efficacy, a focus has shifted again towards local, particularly intratumoral immunotherapies (47, 65). The first approved systemic monoclonal antibody and cell-based therapy for advanced cancer were ipilimumab (FDA/EMA) (66) and lifileucel (FDA) (67), respectively (in purple). However, to this day, the only FDA/EMA-approved intratumoral immunotherapy is Talimogene Laherparepvec (T-VEC) (68), which is used for the treatment of unresectable melanoma lesions (in green) (Figure 1). Herpes virus G47∆ has been approved particularly by the Japan Ministry of Health, Labour and Welfare for the intratumoral treatment of malignant glioma (69) and adenovirus H101 in China for advanced nasopharyngeal cancer (70).
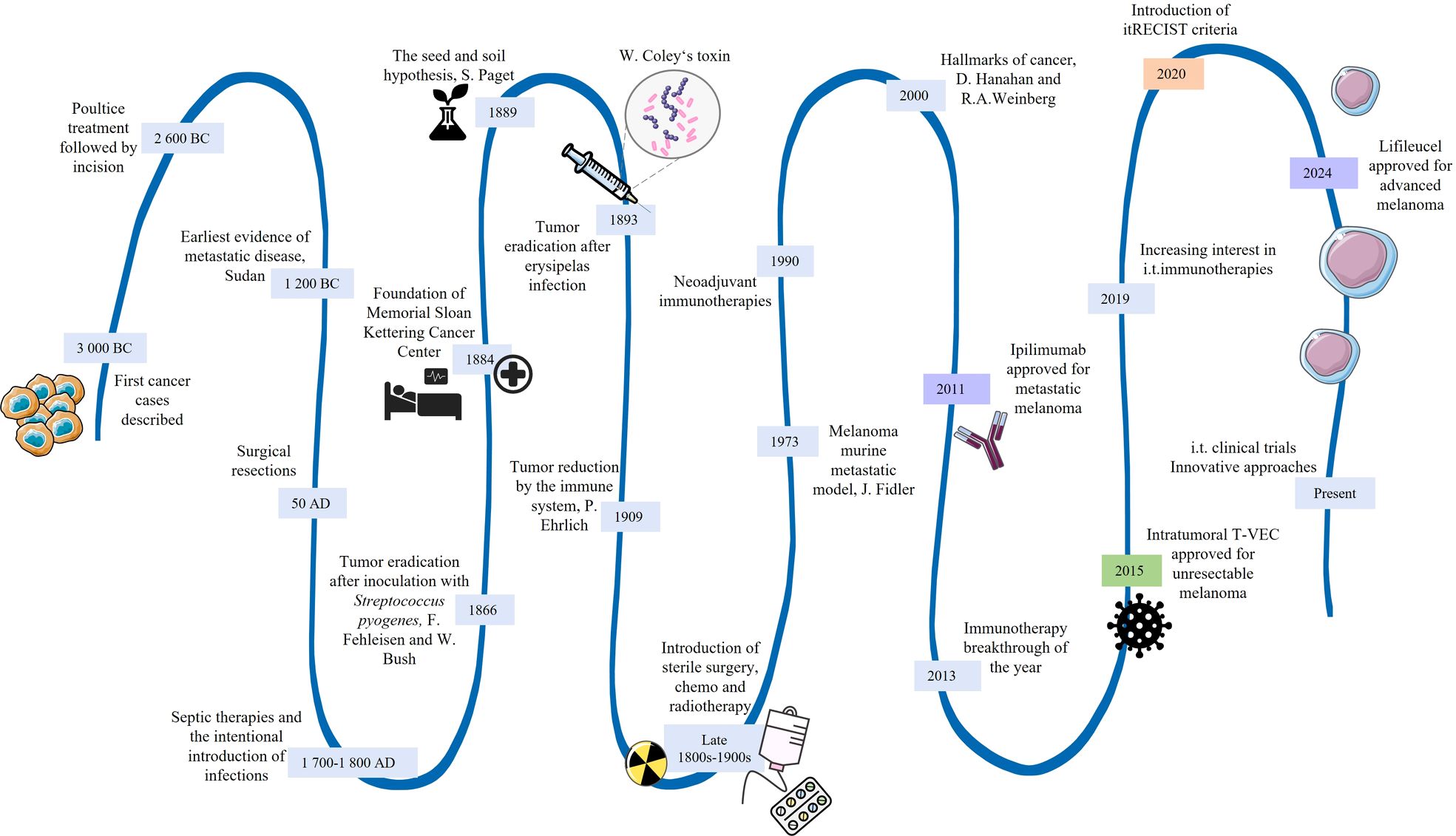
Figure 1. Historical milestones of the intratumoral approach, including the key events for advanced cancers. i.t. intratumoral.
Intratumoral immunotherapy is based on the principle of in situ immunization, where the immune response is primed directly within the tumor microenvironment. This can involve the stimulation of pre-existing anti-tumor immunity or the initiation of the new, often tumor-specific immune responses (65, 71, 72). The specific mechanisms activated depend on several factors, including the type of immunotherapeutic agent used, its biological activity, timing, and combination with other therapies (47, 65, 71). One of the common mechanisms is the activation of dendritic cells within the tumor through intratumoral injection of immunotherapeutic agents. These activated dendritic cells present tumor antigens to T cells, initiating a robust anti-tumor immune response (73, 74). Another mechanism involves the injection of agents such as cytokines or cytokine-inducing compounds into the tumor, which modulate the local immune environment and promote the recruitment and activation of immune cells (75, 76). Additionally, intratumoral administration of checkpoint inhibitors can block inhibitory signals within the tumor microenvironment, enhancing the activity of T cells against cancer cells (77, 78). Oncolytic viruses, which selectively infect and kill tumor cells, also play a role by releasing tumor antigens and stimulating a broader immune response (79). Approved or investigational systemic immunotherapies can be intended for local administration if accessible tumor lesions (i.e., primary tumors or metastases) are present for direct injection via skin, surgery, or any of the endoscopic procedures, and pending their potential future approval for use in local treatment settings (71).
Intratumoral administration exerts several advantages over the traditional systemic approach: (a) It significantly reduces the exposure of immunotherapeutic drugs to healthy tissues, thereby lowering the likelihood of side effects (47, 65, 72, 80). (b) It enables combinations of drugs that may be excessively toxic if administered systemically (65, 80). (c) It is easier to achieve high intratumoral bioavailability (47, 65, 72). (d) It allows for lower doses, reducing costs while maintaining therapeutic efficacy (65, 80). However, intratumoral immunotherapy also has its disadvantages. Not all tumors are accessible for direct injection, limiting the applicability of this approach to certain cancer types and locations (81, 82). Local treatments can cause reactions at the injection site, including undesired pain, swelling, and inflammation (83–85). The variability within the tumor microenvironment can also affect the uniformity and effectiveness of the treatment (86). Moreover, the administration of intratumoral immunotherapies requires precise delivery techniques, such as image-guided injections, which can complicate the process (47, 81, 87). Contrary to systemic therapies, intratumorally administered agents may not reach undetected lesions (88–90). Finally, while strong local immune activation is an advantage, it may not always result in a sufficiently robust systemic response to control metastatic disease (91). This risk of insufficient systemic response remains a challenge in effectively managing cancer with intratumoral immunotherapy.
4 Rationale for combination therapy in the treatment of advanced cancer
In the last two decades, the introduction of concepts such as the “Hallmarks of Cancer” and the “Cancer-Immunity Cycle” has profoundly influenced the understanding and treatment of cancer. Despite the natural ability of the innate and adaptive immune system to recognize and eliminate cancer, malignant/metastatic cells have developed numerous evading mechanisms. These hallmarks were described by Hanahan and Weinberg to conceptualize cancer as a complex tissue of cells communicating with each other. Currently, fourteen hallmarks of cancer have been introduced, including (1): evading growth suppressors (2), non-mutational epigenetic reprogramming (3), genome instability and mutations (4), avoiding immune destruction (5), inducing or accessing vasculature (6), resisting cell death (7), activating invasion and metastasis (8), tumor-promoting inflammation (9), enabling replicative immortality (10), polymorphic microbiomes (11), senescent cells (12), unlocking phenotypic plasticity (13), deregulating cellular metabolism, and (14) sustaining proliferative signaling (Table 1) (59, 146, 147).
In 2013, Chen and Mellman introduced a novel concept, the “Cancer-Immunity Cycle”, which describes crucial steps for an efficient anti-tumor immune response (148). The critical step of the cycle is the induction of local disruption of tumor cells, followed by the release of specific tumor motives such as tumor antigens, damage-associated molecular patterns (DAMPs), pathogen-associated molecular patterns (PAMPs), and various pro-inflammatory cytokines (148, 149). These released tumor motives consequently attract the cells of innate immunity (e.g., macrophages or dendritic cells (DCs)) that start to engulf and present tumor antigens with major histocompatibility complex (MHC) molecules. Stimulated antigen-presenting cells (APCs) then migrate to draining lymph nodes where they present tumor antigen-MHC complexes to naïve T cells, activating them in an antigen-specific manner. This leads to the infiltration of the primary tumor by activated adaptive immunity cells, which subsequently destroy tumor cells. This process further triggers the release of tumor motives and pro-inflammatory signals (148). Tumor cells in distant lesions, i.e., metastases, may be eradicated under certain conditions (Figure 2) (150, 151).
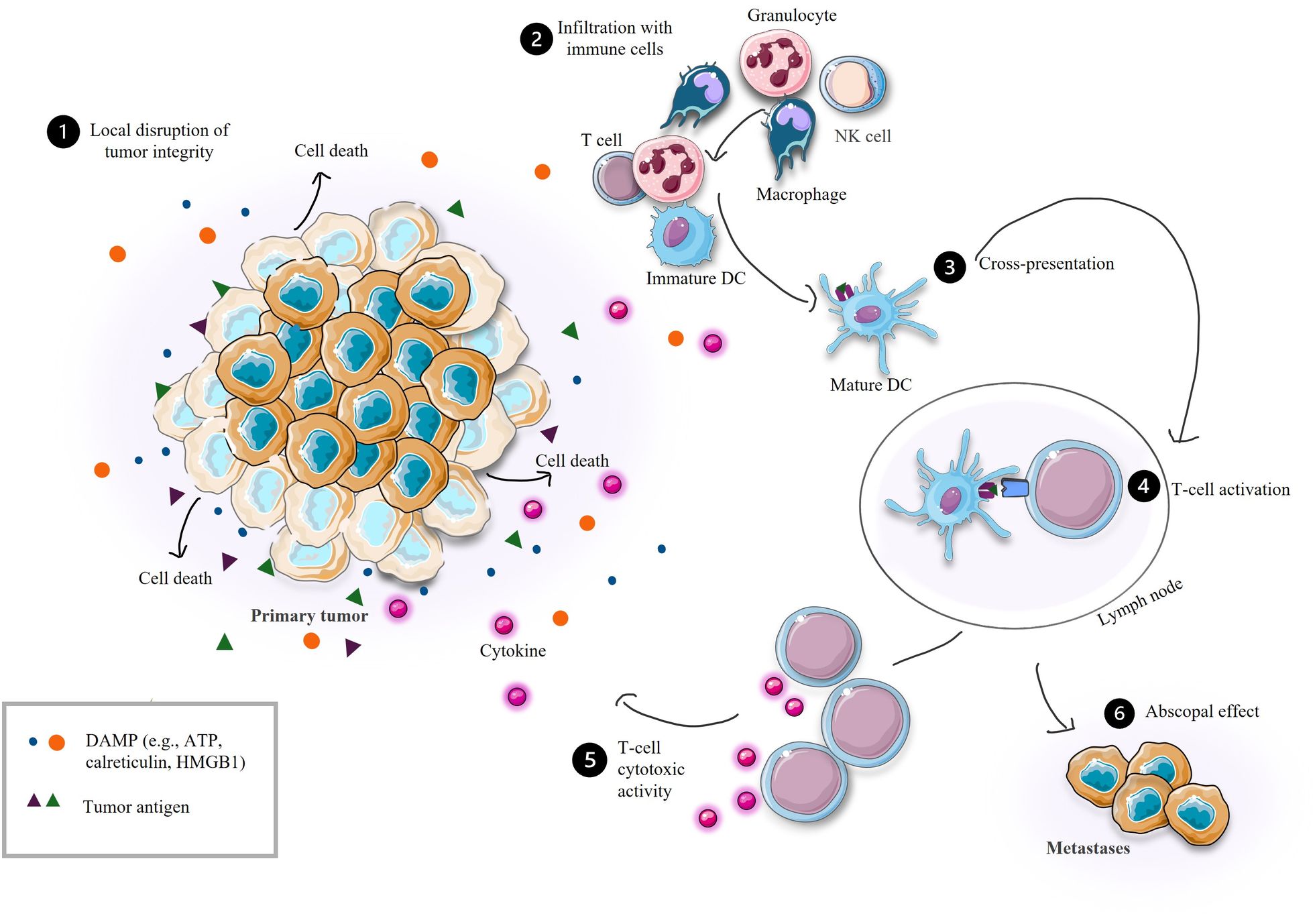
Figure 2. Efficient anti-tumor immune response. DC, dendritic cell; NK, natural killer; DAMP, damage-associated molecular pattern; ATP, adenosine triphosphate; HMGB1, high mobility group box 1 protein.
Taking these concepts in mind, cancer treatment for advanced and metastatic tumors should target two or more “hallmarks of cancer” (152–154) while incorporating at least one approach that stimulates immunogenic cell death (ICD) to enhance efficacy and increase the immunogenicity of cancer cells (155). ICD is a regulated form of cell death characterized by membrane rupture, the release of molecules such as adenosine triphosphate (ATP), high mobility group box 1 protein (HMGB1), annexin 1, heat shock protein (HSP), type I interferon, cytosolic DNA/RNA, tumor antigens, and the translocation of calreticulin to the cell membrane (156, 157). Several types of ICD have been identified, including immunogenic apoptosis, necrosis, and pyroptosis (155), as well as recently proposed ferroptosis, parthanatos, immunogenic entotic or netotic cell death, lysosome- and autophagy-dependent cell death, and alkaliptosis (158). ICD has also been described for immunotherapeutic approaches. Examples of therapies inducing ICD are summarized in Table 1. While it may be reasonable to expect ICIs or cell therapies to indirectly induce ICD via immune cell mediators, studies on the release of DAMPs have primarily focused on established ICD inducers without examining the potential of ICIs or cell therapies alone (159, 160).
5 Combinations of intratumoral immunotherapies with other treatment modalities
5.1 Intratumoral cancer vaccines
Cancer vaccines are a diverse group of therapies that include dendritic cells, peptide/protein, gene, viral, oncolytic viral or repurposed viral vaccines (35) (Table 2). They aim to stimulate both the innate and adaptive arms of the immune system (35, 164) and are typically combined with immune adjuvants to enhance the immune response (35, 164). Despite the development of numerous cancer vaccines and their modification, their efficacy is often reduced in advanced and metastatic cancers (165, 166). Therefore, combining them with other therapeutic approaches is essential (167).
5.1.1 Viral repurposed and viral oncolytic vaccines
A promising approach in cancer vaccines is drug repurposing, which involves identifying new therapeutic uses for existing medications. Existing knowledge of safety profiles, pharmacokinetics, and manufacturing processes can expedite the introduction of new treatments to the market (168). Repurposed vaccines, such as diphtheria, influenza, measles, smallpox, and yellow fever vaccines, have been explored for their potential anticancer properties (169). For example, intratumoral administration of the influenza vaccine in a murine metastatic breast cancer model induced acute inflammation, reduced tumor size, and reversed resistance to systemic ICIs, leading to reduced metastases (170). There is also a clinical trial underway to evaluate the safety of influenza vaccination among breast cancer patients receiving chemotherapy in the neoadjuvant setting (NCT06229392). In phase I of this study (NCT06229392), 2 doses of seasonal flu vaccine will be administered to breast cancer tissue, and the tumor and whole body response will be studied. Similarly, in a bilateral colorectal murine model, intratumoral administration of the yellow fever vaccine combined with systemic administration of anti-programmed cell death protein 1 (anti-PD-1) and anti-CD137 reduced tumor growth. Interestingly, mice that were preimmunized with the same vaccine demonstrated enhanced local and distant antitumor immunity (171), highlighting the potential of widespread vaccine deployment.
In addition to repurposing vaccines, researchers investigated the concurrent intratumoral administration of various oncolytic viruses and bacteria alongside other therapies to amplify the antitumor effects in various preclinical models (172) and clinical studies targeting advanced disease (Supplementary Table 2). Numerous oncolytic viruses, such as coxsackievirus (NCT02307149), dengue virus (NCT03990493), and vaccinia virus (NCT05859074), are currently used for the in situ immune system activation in clinical trials (173) (Supplementary Table 1). However, results from clinical trials using oncolytic viruses alone as cancer vaccines often yielded disappointing outcomes (174). Therefore, combination strategies are preferred to enhance the effectiveness of oncolytic immunotherapies (173). Combining oncolytic virus therapy with ICIs in preclinical models of several cancers has shown significantly prolonged survival compared to untreated mice or those receiving either therapy alone (175–177). Given that ICIs are extensively employed in treating locally advanced and metastatic cancers, most clinical trials focus on combining oncolytic immunotherapies with the systemic administration of ICIs (Supplementary Table 1). Furthermore, intratumorally administered oncolytic adenovirus VCN-01 has shown encouraging biological and clinical activity when administered with chemotherapy to patients with pancreatic adenocarcinoma (PDAC) (NCT02045589). VCN-01 was administered by endoscopic ultrasound guidance to the primary lesion. In all treated patients, the injected lesion remained stable or decreased in size. Of the seven evaluable patients, five experienced progression at 4 months, one at 8 months, and one at 31 months after treatment. Progression in all patients was due to the appearance of new lesions or the growth of distant, non-injected metastatic lesions (178). In 2023, the FDA granted Fast Track designation to intravenously administered VCN-01 for treating metastatic PDAC (NCT05673811), following promising results from previous studies combining intravenous VCN-01 with chemotherapy for PDAC treatment (NCT02045602) (179).
Advances in genetic engineering have led to the development of recombinant viruses that attract immune cells to infiltrate the tumor and deliver additional tumor antigens and immunomodulators. Consequently, their activity triggers T cell activation and enhances the anti-tumor immune response. T-VEC, the first FDA/EMA-approved oncolytic virus for intratumoral application, is an example of a genetically engineered herpes virus with an additional gene for human granulocyte-macrophage colony-stimulating factor (GM-CSF), showing encouraging outcomes for several advanced tumors (180, 181). In clinical trial NCT02509507, T-VEC was injected intratumorally in 21-day cycles with intravenous pembrolizumab, and a feasible and tolerable combination was demonstrated to continue further investigation (182). Besides incorporating the gene for GM-CSF into viral vectors in different oncolytic viruses (NCT02562755, NCT05162118, NCT04050436), viruses expressing cytokines such as IL-12 and IL-15 or ICIs are currently tested (NCT06008925, NCT05081492, NCT04370587, NCT04735978, NCT06124001).
In clinical trials, virotherapies are typically administered through either intratumoral or intravenous routes. While intratumoral administration is expected to result in fewer adverse events and limited accumulation of viral particles within metastatic lesions, intravenous administration faces challenges such as restricted penetration of viral particles through dense tumor stroma and an immunosuppressive microenvironment, along with rapid serum degradation by the immune system (183). However, a few studies have reported comparable results between local and systemic administration (184). In the case of repurposed viral vaccines, intratumoral administration may offer an advantage by preventing the rapid elimination of viral particles due to neutralizing antibodies and memory cells (183). The limited number of clinical trials directly comparing the safety and efficacy of intratumoral versus systemic administration highlights an urgent need for more comprehensive studies to fill this critical gap in research.
5.1.2 Peptide/protein and dendritic cell vaccines
Intratumoral peptide/protein and dendritic cell vaccines represent promising strategies for the treatment of advanced cancers (185, 186). They involve the administration of tumor-specific antigens, i.e., neoantigens or peptides and ex vivo primed dendritic cells, respectively, to stimulate the adaptive arm of the immune system to combat cancer (36). However, in the advanced disease setting, combining other treatment modalities is essential for effective treatment (186, 187).
Dendritic cancer vaccines are often investigated in combination with systemic checkpoint inhibitors (e.g., NCT03942328, NCT03546361, NCT03707808). Several clinical trials also examined the efficacy of intratumorally delivered DCs with a kinase inhibitor for the treatment of metastatic renal cell carcinoma (NCT02432846, 2014-004510-28). For instance, the trial NCT02432846 reported that local delivery of allogeneic DCs combined with the tyrosine kinase inhibitor sunitinib resulted in a partial response in 30.8% of patients with metastatic renal cell carcinoma. Further, phase II of clinical trial NCT04796194 examines the intratumoral administration of LTX-315, an oncolytic peptide, with systemic anti-PD-1. In phase I, this combination demonstrated an acceptable safety profile and substantial volume reduction in 29% of the patients, and 86% of biopsies had an increase in intralesional CD8+ T cells posttreatment (NCT04796194) (188). However, direct comparisons between systemic and localized treatment regimens are still lacking. In the case of peptide vaccines, there are only a few clinical trials investigating the intratumoral administration of peptide/protein vaccines, emphasizing the need for such studies.
While most clinical studies prioritize systemic administration routes for both protein/peptide and DC vaccines, intratumoral delivery has shown a potential to mitigate the immunosuppressive tumor microenvironment. Preclinical evidence supports this approach. A study investigating antigen-pulsed DCs demonstrated that intratumoral administration, when combined with subcutaneous delivery, led to a reduction in regulatory T cell (Treg) populations, decreased TGFβ expression, and increased T cell infiltration within a glioblastoma mouse model. This dual administration strategy exhibited superior results compared to subcutaneous injection alone (189). While the preclinical studies demonstrate promising results, additional clinical investigation is necessary.
5.2 Intratumoral immunomodulators
Immunomodulators are a form of immunotherapy that regulate the immune system’s response and encompass cytokine therapy, pattern recognition receptor (PRR) and stimulator of interferon genes (STING) agonists, and vaccine adjuvants (190). STING or PRR agonists like Pam2Cys (toll-like receptor (TLR2), Poly(I:C) or Poly-ICLC (TLR3), monophosphoryl lipid A (TLR4), ADU-S100 (STING), and resiquimod or imiquimod (TLR7/8) when interacting with their respective receptors on tumor or immune cells, can stimulate APCs, macrophages, B and T cells, and the production of various cytokines and chemokines (191, 192). In preclinical studies, intratumorally administered STING agonists have shown promising results across different cancer types (193). In a murine model of metastatic sarcoma, intratumoral administration of STING agonist DMXAA resulted in a 60% reduction in tumor size and prolonged survival. Additionally, the systemic anti-tumor immune response was observed in this metastatic model, resulting in approximately 50% reduction of primary lesions and lung metastases (194). Based on promising preclinical results, several clinical trials involving intratumoral STING agonists were initiated, preferably combined with ICI therapy (Supplementary Table 2). For example, when STING agonist MK-1454 was administered intratumorally as monotherapy, complete (CR) or partial responses (PR) in clinical trials for advanced solid tumors were not achieved. However, when combined with ICI, a PR of 24% (6/25) was observed, with reductions in both injected and non-injected lesion sizes (195) (NCT03010176). In a follow-up study involving patients with advanced or metastatic head and neck squamous cell carcinoma, an overall response rate of 50% was noted with this combination treatment (NCT04220866). Additionally, newer STING agonists like BMS-986301, MK-2118, ONM-501, and BMS-986301 hold the potential to enhance our understanding of STING agonists’ role in cancer immunotherapy (Supplementary Table 1).
PRR agonists are a significant focus of the clinical trials under discussion here and elsewhere (Supplementary Table 2) (196). The lack of tumor specificity and dose-limiting systemic toxicities upon intravenous administration increased interest in intratumoral administration as an alternative approach (197, 198). Various TLR9 activators, including CMP-001, SD-101, tilsotolimod, MGN1703, and CpG, have been investigated primarily in conjunction with ICI therapies to assess their safety, tolerability, and ability to stimulate immune responses within the tumor microenvironment (199). For instance, early data on SD-101 with PD-1 blockade showed increased clinical efficacy with minimal additional toxicity relative to PD-1 blockade alone in advanced melanoma (200). Efficacy of SD-101 is currently being evaluated in combination with PD-1 blockade and radiation therapy in patients with metastatic pancreatic cancer (NCT04050085) and prostate cancer (NCT03007732); and in combination with anti-OX40 antibody in patients with advanced solid tumors (NCT03831295). CMP-001, combined with PD-1 blockade therapy, entered phase II/III study for patients with unresectable or metastatic melanoma (NCT04695977). However, the study was terminated due to business decisions. CMP-001 is being further evaluated in various locally advanced and metastatic cancers (Supplementary Table 1). The results of another phase I trial demonstrated that the combination of CMP-001 with PD-1 inhibitor pembrolizumab elicited the best objective response rate (ORR) per RECIST v1.1 criteria of 23.5% (95% CI, 15.5%-33.1%) Moreover, post-progression responders achieved the best ORR of 27.6% (95% CI, 19.0%-37.6%) (NCT02680184) (201). Optimizing the mixture of carefully selected immunomodulators and therapeutics for direct intratumoral combined administration may increase vaccine efficacy. For instance, in a murine model of pancreatic adenocarcinoma, a combination of intratumorally administered TLR agonists (Poly (I:C), R-848, LTA), mannan-BAM, and agonistic anti-CD40 antibodies resulted in a notable 67% decrease in tumor size (202). Likewise, in the bilateral murine colon cancer model, the synergistic effects of this treatment induced systemic immune response, resulting in tumor growth delay and complete tumor regression in a subset of untreated representative metastatic tumors (203).
Besides STING and PRR agonists, bacteria-based therapies are examined in the advanced disease setting. For instance, attenuated Clostridium novyi, depleted of its lethal toxin gene, is currently tested for intratumoral administration in clinical trials with systemic ICI treatment (NCT03435952). This vaccine administration has previously demonstrated tumor-specific T-cell induction and decreased tumor size (204) (NCT01924689), supporting the integration of bacterial vaccines amongst potential immunotherapeutic strategies for advanced diseases. Another clinical study with T3P-Y058-739, a genetically modified, live attenuated strain of the bacterium Yersinia enterocolitica, alongside ICI treatment, will be evaluated in patients with advanced solid tumors (NCT05120596).
Additionally, various cytokines such as IL-2, IL-12, interferon (IFN)-α, and GM-CSF can trigger anti-tumor immunity or inhibit angiogenesis (75, 76). Their short half-life, low biodistribution, and toxicity limit their practical systemic application (76, 205). However, directly injecting the immunomodulators into the tumor site could optimize their efficacy. In murine melanoma and colorectal cancer models, intratumoral administration of mRNA encoding IFN-α, IL-12, IL-15, and GM-CSF enhanced by systemic administration of anti-PD-1, reduced tumor growth of both primary tumors and metastases (206). Several methods to deliver cytokines directly in the tumors alongside systemic therapy (mostly ICIs) for patients with advanced diseases have been tested, including mRNA vaccines (NCT06249048), adenoviral vector encoding IL-12 (NCT04050085, NCT04006119, NCT02423902), recombinant fusion proteins (NCT06284590), cytokines (NCT01480323, NCT01672450), or plasmids (NCT02493361, NCT04526730).
5.3 Intratumoral adoptive cell therapies
Adoptive cell therapies enhance the immune system’s ability to fight cancer by administering genetically engineered or expanded patient immune cells that can specifically target and destroy cancer cells. This approach includes CAR-T therapy, tumor-infiltrating lymphocyte (TIL), NK cell (39, 40), and γδ-T cell therapy, and CAR therapy for cells of innate immunity (e.g., CAR-NK, CAR-NKT, or CAR macrophage). Despite their promising potential, these therapies can face challenges such as antigen escape (207), low infiltration of transferred cells into tumor lesions, and the presence of immunosuppressive mechanisms, including a hostile tumor environment and immunosuppressive cells (208). Furthermore, one of the limitations is their short half-life and cytokine release syndrome upon intravenous administration (209, 210). Local administration may facilitate the infiltration of adoptively transferred cells (40, 208) and mitigate systemic toxicity (211), highlighting additional advantages of intratumoral administration for such cell therapies.
For treating advanced cancers in both clinical and preclinical settings, cell therapies are often combined with approaches that induce oncolysis. These combinations includes an intratumoral administration of zoledronate-pulsed dendritic cells with intravenous T lymphocytes and gemcitabine (212), chemotherapy (213) (NCT02018458), low-dose cisplatin and 5-fluorouracil (214), photodynamic therapy (215), oncolytic viral therapy (216), or intratumoral CD4⁺ Th1 memory cells and cryoablation (NCT00861107). The repeated intratumoral application of CD1c myeloid DC alongside ICI and synthetic saponin-based adjuvant ASO1b, together with systemic low-dose ICI, has demonstrated encouraging results in treating refractory advanced melanoma (217) (NCT03707808). In this phase I trial, 4 patients (50%) obtained complete response (CR) in the injected lesions. Of these, 2 patients obtained an overall CR, and one patient PR. Median progression-free survival (PFS) and overall survival (OS) were 24.1 and 41.9 weeks, respectively (217). Furthermore, autologous DCs injected with an adjuvant booster (Prevnar vaccine) are being studied in patients with unresectable intrahepatic cholangiocarcinoma after standard high-dose external beam radiotherapy (NCT03942328). This approach has shown a favorable safety profile and encouraging signs of efficacy and induction of tumor-specific immunity. Early response data from the five subjects who have completed the protocol showed ORR of 60% (n=3, all partial response) (218). Phase II of the study NCT03942328 will focus on combinations with ICI that could further enhance immunotherapy outcomes. The promising results of integrating cell-based vaccines with other treatments warrant additional clinical investigation to broaden the range of available immunotherapy options.
5.4 Intratumoral immune checkpoint inhibitors and monoclonal antibodies
Checkpoint molecules, such as cytotoxic T-lymphocyte associated protein 4 (CTLA-4) and programmed cell death protein 1/programmed cell death ligand 1 (PD-1/L1), maintain self-tolerance and prevent any autoimmune reactions by modulating the activity of T cells (78). Nevertheless, tumor cells can exploit checkpoint molecules to evade immune responses. Thus, blocking these checkpoints can immunity against cancer. For example, an antagonistic monoclonal antibody anti-CTLA-4 releases the inhibition of APC activity mediated by the interaction between CTLA-4 on Tregs and CD80/86 on APC. Additionally, anti-PD-1 or anti-PD-L1 antibodies reverse the negative interaction between tumor cells and T cells, thereby stimulating their activity (77, 78). Successful checkpoint inhibitor therapy depends on the presence of pre-existing anti-tumor immunity (65). Some studies report poor treatment responses to checkpoint blockade due to impaired antigen presentation, loss of neoantigens, insufficient T cell infiltration, or inhibition of T cell killing activity in an immunosuppressive tumor microenvironment (TME) (219). Furthermore, treatment with systemic monoclonal antibodies, including checkpoint inhibitors, is accompanied by immune-related adverse events (220, 221)(e.g., NCT01844505, NCT02142738, NCT02477826) due to their long serum half-life enabling the interaction with various cells. High molecular weight also mitigates the intratumoral bioavailability (197, 221). Some of these obstacles, particularly immunosuppressive TME, can be targeted with intratumoral administration (222). Additionally, combinations of checkpoint inhibitors with other immunomodulatory approaches to overcome immune evasion mechanisms, or with cytostatic drugs targeting cancer cell growth, immortality, angiogenesis, or genome instability, have been introduced (223).
Current clinical trials focus on a combination of in situ vaccination and systemic treatment, mainly targeting immune checkpoints. The reason is a synergy observed between local immunostimulatory therapies and systemic checkpoint inhibitors (224) and approval of numerous checkpoint inhibibtors for systemic administration, such as antagonistic CTLA-4 (e.g., ipilimumab and tremelimumab) and anti-PD-1/L1 antibodies (e.g., pembrolizumab, atezolizumab, nivolumab, cemiplimab, durvalumab, avelumab), alone or in combination with other therapeutic interventions. Among novel checkpoint molecules, such as lymphocyte activation gene 3 protein (LAG-3) and T cell immunoglobulin mucin-3 (78, 225), systemic dual inhibitor of PD-1 and LAG-3, Opdualag, is currently the only one authorized by the FDA for clinical use for unresectable or metastatic melanoma. To date, intratumoral checkpoint blockade has been combined with approaches that stimulate the formation of cytotoxic T cells, such as anti-CD40 monoclonal antibody, and hypofractionated radiotherapy (226); oncolytic viral therapy (227, 228) (NCT04725331); and chemotherapy (229). Furthermore, to counteract the resistance that may develop against immune checkpoint inhibitors (230–232), combination therapies with immunostimulatory agents such as BO-112 (233), CMP-001 (CMP-001-001; NCT02680184), SD-101 (234), and bacteria Fusobacterium nucleatum (235), or targeted therapy such as VEGF (236) and CDK4/6 inhibitor (237), have been introduced, underscoring the necessity for continued immunostimulation. Additionally, CD40 is a promising target for immune checkpoint therapies. CD40 agonistic monoclonal antibodies stimulate cells of both the innate and adaptive immune systems, including macrophages, neutrophils, and DCs (238). Currently, several clinical trials in various stages are investigating anti-CD40 therapies in combination with irreversible electroporation (NCT06205849) and pembrolizumab (NCT02706353, NCT02988960). One completed trial examined the combination of anti-CD40 with a TLR agonist (NCT03831295).
Currently, no checkpoint inhibitor has been approved by either the FDA or EMA for intratumoral administration in the advanced disease setting. However, clinical studies on intratumoral administration of checkpoint inhibitors in advanced tumors, especially with combination therapies, are ongoing (e.g., NCT03707808). For instance, intratumoral checkpoint blockade has been combined with oncolytic viral therapy (227) and chemotherapy (229). While studies support the preference for combined therapies to enhance immune checkpoint blockade efficacy, the outcomes of intratumoral administration combined with cytotoxic therapies for patients with advanced and metastatic disease are yet to be fully explored. Current evidence regarding potential combinations for both intratumoral and systemic administration could expedite further research.
6 Timing of intratumoral immunotherapies combined with other treatment modalities
Understanding and optimizing the timing of intratumoral immunotherapy administration alongside other therapeutic approaches is essential for maximizing therapeutic efficacy and improving patient outcomes. Chemotherapy and radiotherapy can significantly affect the viability and function of immune cells, thus, the ideal timing for each combination should be studied thoroughly (239, 240). It should also be noted that many patients have undergone previous treatments, which may impact the function of therapeutic combination. While the timing for some systemic combination therapies for the treatment of advanced cancers has been explored (Supplementary Table 2), the timing for intratumorally administered therapies may differ significantly from systemic administration. For instance, there may be leakage of therapeutics into nearby tissues or systemic circulation following local injection (241). However, localized delivery of chemotherapeutics can potentially reduce systemic adverse effects often connected with higher therapeutic dose. Leakage can be mitigated through specific injection techniques or by injection of various sites of a tumor, as well as by a needle type (81, 242, 243). This allows for more flexibility in timing for combined therapies.
ICIs are often combined with prior chemotherapeutic intervention, while targeted therapies typically precede chemotherapy or radiotherapy (Supplementary Table 1). Although administering checkpoint inhibitors before chemotherapy regimens may be unconventional and not fully aligned with the potential induction of ICD by chemotherapy (104–107, 109, 244), there are promising results. For instance, results reported by Szabados et al. demonstrated the benefits of this schedule. Patients with metastatic urothelial carcinoma who received initial treatment with ICIs followed by chemotherapy had a better response rate (64%) compared to those who received chemotherapy before ICI treatment (21%) (245). However, studies on lung cancer and advanced esophageal squamous cell carcinoma point to improved treatment efficacy when immunotherapy is applied several days after chemotherapy (246). Additionally, recent studies have explored the role of circadian signals in cancer development, immune system recognition, and the effectiveness of immunotherapies (247, 248). For example, early systemic administration of checkpoint inhibitors has been shown to extend patient survival four times longer than a late-day administration (249, 250).
In the case of cell-based therapies, chemotherapy-induced lymphodepletion prior to cell therapies like CAR-T has been shown to enhance CAR-T proliferation. This approach not only facilitates an early treatment option for solid tumors but also addresses the prolonged development and infusion timelines associated with CAR-T therapy (251). In the context of targeted therapy and chemotherapy combinations, approved treatments typically involve administering monoclonal antibodies, such as those targeting EGF/EGFR, tyrosine-protein kinase erbB-2 receptor, or VEGF/VEGFR, prior to cytotoxic therapy. However, in patients with non-small cell lung cancer, pretreatment with an anti-VEGF antibody (bevacizumab) has been found to hinder the delivery of chemotherapeutic drugs (252).
These findings underscore the variability in treatment outcomes across different cancer types and highlight the critical need for additional research in this area. Determining the optimal timing for administering these therapies is crucial for integrating them into standard care. This decision must account for factors such as the time required for the immune system to develop a specific response to the antigen, the necessity of multiple doses, and potential interactions with concurrent therapies. Furthermore, time schedules are often not reported in clinical trials or not fully described when treatments are administered on the same day (e.g., NCT01672450, NCT02493361, NCT04220866) (Supplementary Table 2). It cannot be implied whether concurrent or non-simultaneous administration of combination therapies is necessary. Additionally, there are currently no clinical trials examining different time schedules of the same treatment for advanced cancers emphasizing the urgent need for such studies.
7 Clinical trials
The landscape of cancer treatment has undergone a significant transformation with the introduction of intratumoral immunotherapy, particularly for locally advanced and metastatic cancers. Numerous clinical trials examining the safety and efficacy of combinations of intratumoral immunotherapy with other treatment modalities have been conducted to date. These mainly include intratumoral oncolytic viral therapy or PRR agonists in combination with systemic checkpoint inhibitors (Supplementary Table 1).
Designing clinical trials can be highly complex, often serving as a final treatment option when other therapies have failed, particularly in advanced disease cases. The FDA’s approval of metastasis-free survival (MFS) as an endpoint for non-metastatic prostate cancer marks a significant advancement (253). This decision reflects shortcomings in using PFS as an endpoint for metastatic disease, as PFS fails to provide insights into metastatic activity, which is the primary cause of death (254). Recently, numerous clinical trials have adopted MFS as either a primary or secondary outcome for prostate (NCT05352178, NCT04641078, NCT03569241, NCT01341652), nasopharyngeal (NCT03290820), colorectal (NCT00643877), and breast cancer (NCT04278469, NCT02448576), and melanoma (NCT06157099). However, there are currently no trials utilizing MFS outcomes for the advanced disease setting.
Most studies on the combination of intratumoral immunotherapy with other therapies are currently in phases I/II (Supplementary Table 1). NCT04695977 entered phase II/III but has been recently terminated due to business decisions. In this study, vaccinia virus Pexa-Vec was administered as 3 bi-weekly intratumoral injections, followed by protein kinase inhibitor sorafenib at week 6. The median TTP was 2.0 months (95% CI: 1.77, 2.96) and 4.2 months (95% CI: 2.92, 4.63); ORR was 19.2% (45 patients) and 20.9% (47 patients); and DCR was 50.0% (117 patients) and 57.3% (129 patients) in the Pexa-vec plus sorafenib and sorafenib arms, respectively. The median OS was 12.7 months (95% CI: 9.89, 14.95) in the Pexa-vec plus sorafenib arm and 14.0 months (95% CI: 11.01, 18.00) in the sorafenib arm, which led to early termination of the study (255). This underscores how essential it is to thoroughly evaluate the safety, specificity, and efficacy to successfully navigate this innovative path in immunotherapy. The primary goal of these comprehensive strategies is to choose a treatment regimen that enhances effective, long-lasting, and tumor-specific immunity in cancer patients, thereby extending their survival. Moreover, ongoing advancements allow for investigating potential new therapeutic approaches, as outlined in the final chapter.
8 Future directions in the treatment of advanced cancer
8.1 Photoactivated therapy
Photoactivated therapy, also known as photodynamic therapy (PDT), represents a promising approach in the targeted treatment of advanced cancers. This therapy utilizes specific photosensitizing agents that preferentially accumulate in tumor tissues and, upon exposure to light of a particular wavelength, generate reactive oxygen species (ROS). These ROS lead to localized tumor cell destruction, minimizing damage to surrounding healthy tissue and resulting in fewer side effects (256, 257). Furthermore, PDT can induce immunogenic cell death, thereby activating the immune system to recognize and target residual cancer cells and lesions, which may enhance antitumor immunity (258, 259).
The targeted approach of PDT makes it particularly suitable for localized treatment of multidrug-resistant and clinically challenging tumors (260), as well as advanced and metastatic tumors (261), especially when combined with other modalities (262). In future scenarios, PDT could be effectively integrated with intratumoral immunotherapy to strengthen local immune responses and improve patient outcomes in cases of locally advanced and metastatic cancer.
As technological advancements enable deeper tissue penetration and more precise light delivery, PDT could play a crucial role in enhancing targeted drug delivery. These developments align with current efforts in optimizing intratumoral immunotherapy for challenging malignancies, highlighting PDT’s potential as a complementary therapeutic modality in the management of advanced cancer.
8.2 Neoadjuvant setting
Therapeutic options for cancer diseases have undergone significant transformation in recent years, particularly neoadjuvant immune checkpoint inhibitors. Neoadjuvant cancer therapy, administered usually before surgery, traditionally aims to shrink tumors to facilitate surgical resection (263). However, the innovative application of ICIs in the neoadjuvant setting has introduced a paradigm shift, offering improved resectability and enhanced systemic anti-tumor immunity. This dual approach leverages the primary tumor as a source of antigens, thereby inducing an immune response capable of targeting and eliminating dormant tumor cells or distal micrometastases, which are often connected with post-surgical relapse (264).
Recent clinical trials have shown promising advancements in using neoadjuvant ICIs across various cancers. In advanced melanoma, the SWOG S1801 trial demonstrated that event-free survival at 2 years was 72% in the neoadjuvant-adjuvant pembrolizumab group compared to 49% adjuvant therapy, establishing neoadjuvant immunotherapy as a new standard of care (265). For non-small cell lung cancer, results from clinical trials have shown favorable pathologic response rates with minimal adverse events when using ICIs like nivolumab in a neoadjuvant setting (266). Neoadjuvant ICIs in breast cancer have also demonstrated significant improvements in pathologic complete response rate and event-free survival in clinical trials (267). Similarly, neoadjuvant immunotherapy with nivolumab and ipilimumab in locally advanced mismatch repair-deficient colon cancer has shown groundbreaking results (268). Additionally, the intratumoral neoadjuvant treatment consisting of CpG, a TLR9 agonist, and anti-OX40 achieved low toxicity and enhanced systemic response in a murine model of metastatic colorectal and breast cancer. A short break between the intratumoral immunotherapy and surgical resection was essential for the treatment efficacy (Figure 3) (269). These examples of clinical trials highlight the potential of neoadjuvant immunotherapy to improve surgical outcomes and survival rates across different cancer types. Several neoadjuvant intratumoral immunotherapy trials have been underway as well, including virotherapy approaches, TLR agonists, gene therapies, or cell-based vaccines (80).
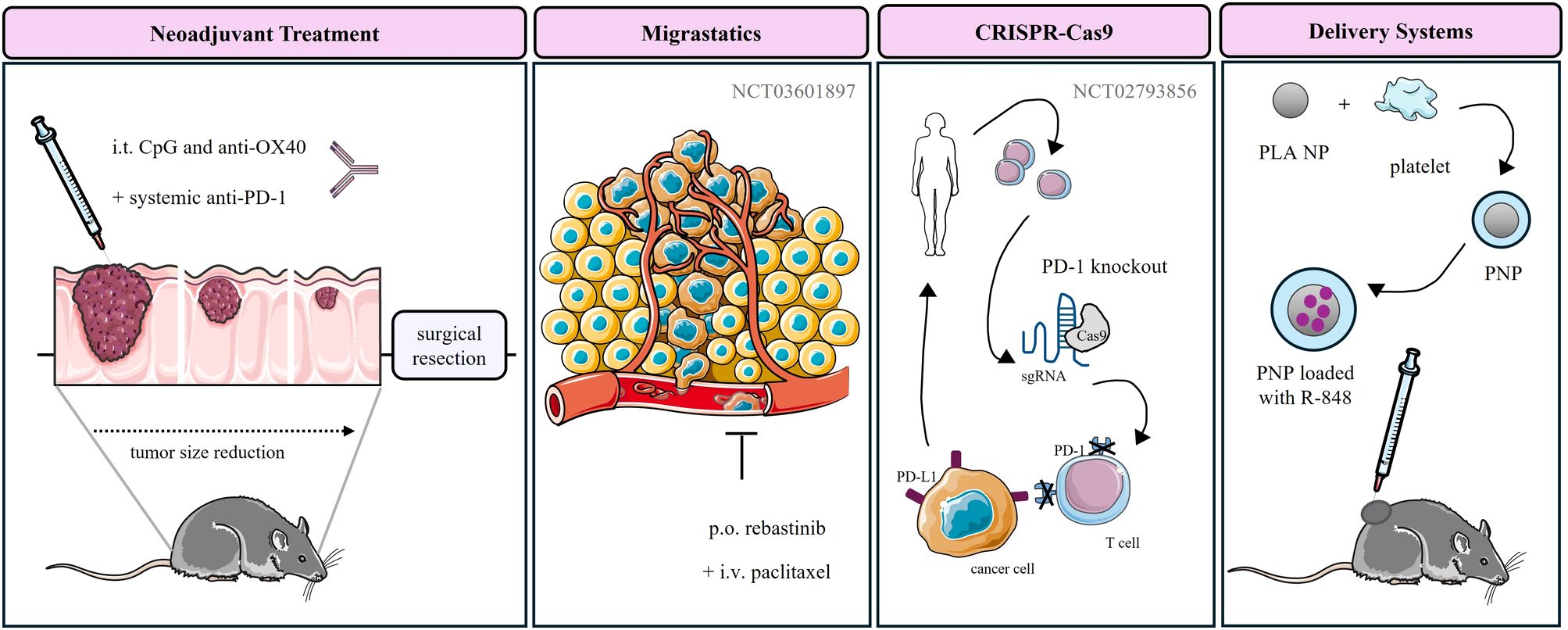
Figure 3. Novel therapeutic approaches for the treatment of advanced cancers. Up to date, intratumoral neoadjuvant immunotherapy combined with other treatment approaches has only been studied preclinically. Agents with anti-migratory activity, such as rebastinib, are currently being investigated in clinical trials through systemic administration. Additionally, the CRISPR-Cas9 gene editing tool is being utilized to knock out the PD-1 gene in patients’ T cells. However, no clinical trials have yet examined a combination approach. Novel delivery systems, such as platelet membrane-coated nanoparticles, have only been studied in animal models. CpG, cytosine-phosphate-guanine; PD-1, programmed cell death protein 1; PD-L1, programmed death ligand 1; sgRNA, single guide RNA; Cas9, Clustered regularly interspaced short palindromic repeats-associated protein 9; NP, nanoparticle; PLA, polylactic acid; PNP, platelet coated nanoparticle; R-848, resiquimod 848.
The future of neoadjuvant immunotherapy has promising research and ongoing clinical trials, which may uncover innovative treatment combinations and strategies. With such knowledge of tumor immunology and immune checkpoint pathways, we anticipate even more effective and personalized neoadjuvant therapies, leading to better surgical outcomes, reduced relapse rates, and improved overall survival for cancer patients.
8.3 Migrastatics
Although the research focuses mainly on targeting the proliferative capacity of cancer cells and generating antiproliferative and cytostatic drugs, the inhibition of cancer cell motility is gaining increased interest as the presence of metastasis represents a significant challenge for today’s oncology (147). Migrastatics, a term first proposed by Gandalovicova et al., 2017, represent a group of drugs that aim to inhibit the dissemination of cancerous cells to distant sites. Cytostatic drugs exert high cytotoxic stress on cancer cells, often leading to the selection of resistant cell populations over time. Since migrastatics do not kill cancer cells directly but rather inhibit their ability to migrate and spread, there is less selective pressure for the development of drug-resistant mutations. Although drugs with anti-migratory activity do not aim to reduce primary lesions, the inhibition of cancer cell motility lowers the number of resistant cells within the tumor (133).
To date several candidates targeting actin polymerization, actomyosin contractility, tropomyosin, myosin, and cAMP-dependent, cGMP-dependent, and protein kinase C (AGC) kinases or a stabilization/destabilization of actin cytoskeleton have been identified (133), including novel and repurposed drug targets. These include rebastinib (Figure 3) (270), paclitaxel, docetaxel, metformin, tamoxifen, mitotam, and voloxicimab (271). Furthermore, the inhibition of epithelial-mesenchymal (EMT) or mesenchymal-epithelial transition (MET) (271–273), matrix metalloproteinases (MMP), cell adhesion (134), or the SDHB subunit of oxidative phosphorylation complex II has been described to reduce cancer cell invasiveness and metastasis (274).
In the case of advanced cancers, migrastatics in combination with antiproliferative drugs are believed to achieve great efficacy (133). Currently, there are two completed clinical trials (NCT03717415; NCT03601897) and one clinical trial (NCT02824575) terminated by a pharmaceutical company, examining the efficacy of rebastinib in combination with chemotherapy for the treatment of metastatic disease. Although intratumoral administration of migrastatics may reduce the side effects upon continuous treatments (65), including the mitigation of motility or cytokinesis of healthy cells (133), lower therapeutic doses of combination therapy (65), and increase sensitivity to mitosis-targeted drugs (133), it can be speculated that circulating tumor cells may be targeted with a systemic approach. A combination of drugs with anti-migratory activity and reduced selective pressure for drug-resistant mutations, along with other treatments, requires further clinical investigation in both systemic and intratumoral settings.
8.4 CRISPR-Cas9
CRISPR-Cas9, clustered regularly interspaced short palindromic repeats/associated protein 9, is a gene editing tool with a potential application in CAR-T and TIL adoptive cell therapies, the generation of cancer animal models, and drug screening (275, 276). The development of CAR-T therapy from each patient is time-consuming, excluding those patients with metastatic disease. By incorporating CRISPR-Cas9 to generate CAR-T cell therapy from healthy donors, i.e., shifting the therapy from autologous to allogeneic CAR-T cells, a shortened and better quality manufacturing period, lower treatment cost, and a higher number of T cells could be achieved (277). Besides the genetic modification of CAR specificity, additional alterations, such as a deletion of PD-1 (278) or LAG-3 (279), or the repair of KRAS oncogenic mutations (280) can be introduced.
To date, several clinical trials have examined the safety and efficacy of CRISPR-Cas9-engineered CAR-T cells for the treatment of advanced cancer. For instance, a phase I clinical trial examined the safety of CAR-T PD-1 knockout therapy in patients with metastatic non-small cell lung cancer after chemotherapy treatment (NCT02793856) (Figure 3). The majority of clinical trials examine the systemic administration, including NCT04417764, NCT04976218, and NCT05812326, although the development of autoimmune reactions against donor TCR and HLA molecules, such as host versus graft response and graft versus host disease can be expected (281). Intratumoral administration of CRISPR-Cas9-modified cell-based therapies is scarce, including the injection of mRNA-transfected c-Met-CAR T cells in the treatment of metastatic breast cancer (NCT01837602).
Although CRISPR-Cas9 is a promising gene-editing tool for oncologic application, local administration in both early stage and advanced disease settings requires further examination.
8.5 Delivery systems
Besides the local administration of therapeutic agents into tumor lesions, approaches to enhance drug localization and distribution specifically to tumor sites have also been investigated. Ongoing advancements in biomaterial development focus on enhancing physicochemical properties and particle size to improve drug retention, ensure uniform distribution within lesions, regulate drug release, and enhance drug solubility (282–284). To date, several drug nano-delivery systems have been developed and include organic nanoparticles (e.g., polymersomes, polymeric nanoparticles and micelles, liposomes, and lipid nanoparticles) (283, 285) and inorganic nanoparticles (e.g., gold and iron particles, hydrogels, and silica), peptide and antibody-drug conjugates (285, 286), extracellular vesicles (e.g., apoptotic bodies, exosomes, and microvesicles) (287), targeted protein degradation systems (e.g., LYTAC and PROTACS) (288), cell or cell-membrane coated nanoparticles (e.g., erythrocytes, platelets, macrophages, neutrophils, leukocytes or tumor cells) (289, 290) and oncolytic virus-based delivery systems (285, 291). Additionally, novel transdermal patches (292), hydrogels (292), or sprayable gels (293) are under investigation.
Particularly noteworthy are peptide and antibody-drug conjugates, which ensure reduced toxicity and precise tumor targeting (285), and cell-based delivery systems utilizing organic and inorganic nanoparticles coated with cell membranes to evade immune recognition (289, 290). To date, several delivery systems have been approved for the systemic treatment of advanced cancers, including, Myocet (liposome-encapsulated doxorubicin), Abraxane (albumin-bound paclitaxel), Onivyde (liposomal topoisomerase inhibitor), Kadcyla (trastuzumab-DM1 conjugate), Enhertu (trastuzumab-deruxtecan conjugate), Padcev (enfortumab-vedotin conjugate) or Trodelvy (sacituzumab-govitecan conjugate). Systemic toxicities associated with these treatments can be mitigated by local administration, which lowers systemic exposure, increases drug concentration, and prevents drug leakage into the bloodstream. Factors such as particle size, charge, and injection rate significantly influence nanoparticle distribution within the tissue (294).
Although no intratumoral delivery system for intratumoral application has been approved yet, several studies examined the encapsulation of immunotherapies for local administration. For instance, local administration of CpG and anti-PD1 antibody DNA nano-cocoon after resection of primary tumor into tumor bed inhibited the disease recurrence and metastasis generation (295). Similarly, silica-zinc oxide micro-rosettes loaded with doxorubicin and Poly(I:C) were reported to reduce primary tumor and metastases growth (296). Furthermore, inhibition of the growth of primary tumors and metastases has been achieved upon intratumoral treatment with platelet membrane-coated nanoparticles loaded with R848 (297) (Figure 3), and polymeric nanoparticles loaded with antigen peptides (298). In conclusion, continued research and development in targeted drug delivery systems are essential to improve the efficacy and safety of cancer treatments.
9 Conclusion
Despite significant advancements, locally advanced and metastatic cancers remain a critical medical challenge. Conventional anticancer treatments and therapeutic approaches, though effective in certain instances, frequently do not adequately enhance overall patient outcomes and survival rates for those with advanced disease. Intratumoral immunotherapy offers a promising alternative with fewer side effects, lower costs, and reduced toxicity. T-VEC monotherapy is the only intratumoral immunotherapy approved for the treatment of unresectable melanoma lesions. The future of cancer treatment lies in the development of combination therapies that induce immunogenic cell death and target multiple hallmarks of cancer. These approaches can potentially enhance the therapeutic response and reduce the likelihood of resistance. Emerging strategies such as migrastatics, CRISPR-Cas9- modified cell therapies, and advanced drug delivery systems represent promising avenues for the treatment of advanced cancers.
Future research should focus on optimizing these innovative treatments and integrating them into standard care. This includes further investigation into the timing and sequencing of combination therapies, exploring new targets and mechanisms of action, and improving drug delivery systems to enhance specificity and reduce toxicity. Additionally, personalized medicine approaches, leveraging genomic and molecular profiling, will be crucial in tailoring treatments to individual patients, maximizing efficacy, and minimizing adverse effects. By addressing the limitations of current therapies and exploring new frontiers, we can move closer to achieving effective, long-term control of locally advanced and metastatic cancers, providing new hope for fighting cancer.
Author contributions
MS: Visualization, Writing – original draft, Writing – review & editing. KV: Conceptualization, Writing – review & editing. OU: Conceptualization, Writing – review & editing. JF: Conceptualization, Writing – review & editing. KPe: Conceptualization, Writing – review & editing. MM: Supervision, Writing – review & editing, Funding acquisition. ZK: Supervision, Writing – review & editing. PM: Supervision, Writing – review & editing. KPa: Supervision, Writing – review & editing, Conceptualization, Funding acquisition. MJ: Supervision, Writing – review & editing, Conceptualization, Funding acquisition.
Funding
The author(s) declare financial support was received for the research, authorship, and/or publication of this article. This work was supported by projects of Charles University in Prague (SVV260637; UNCE 24/MED/022; Progres LF1 Q38 and Q27, Cooperatio ONCO) and by the project of Masaryk University in Brno, Faculty of Medicine (MUNI/A/1587/2023). Moreover, the work was supported by the Ministry of Education, Youth, and Sports grant no. LM2023053 (EATRIS-CZ), the Technology Agency of the Czech Republic within project TN02000109 and FW10010306, and the Ministry of Health grants nos. NU22-D-136. We are also grateful for the support from project National Institute for Cancer Research (Programme EXCELES, ID Project No. LX22NPO5102) - funded by the European Union - Next Generation EU. The work was also supported by the National Institute for Neurological Research (Programme EXCELES, ID Project No. LX22NPO5107) funded by the European Union - Next Generation EU. This study was funded by the National Institutes of Health (grant number Z1AHD008735) awarded to KPa. This work was supported by the Intramural Research Program of the National Institutes of Health, Eunice Kennedy Shriver National Institute of Child Health and Human Development. This work was supported by the Ministry of Education, Youth, and Sports of the Czech Republic, program Inter-Excellence II, Inter-Action-LUAUS24, grant number LUAUS24120. This work was also supported by the _METROFOOD_-_CZ_ research infrastructure_project_. (_MEYS Grant No_: _LM2023064_) including access to its facilities.
Acknowledgments
All figures were drawn using images from Servier Medical Art and PowerPoint (Microsoft 365, version 2406). Servier Medical Art by Servier is licensed under a Creative Commons Attribution 4.0 Unported License (https://creativecommons.org/licenses/by/4.0/).
Conflict of interest
The authors declare that the research was conducted in the absence of any commercial or financial relationships that could be construed as a potential conflict of interest.
The author(s) declared that they were an editorial board member of Frontiers, at the time of submission. This had no impact on the peer review process and the final decision.
Publisher’s note
All claims expressed in this article are solely those of the authors and do not necessarily represent those of their affiliated organizations, or those of the publisher, the editors and the reviewers. Any product that may be evaluated in this article, or claim that may be made by its manufacturer, is not guaranteed or endorsed by the publisher.
Supplementary material
The Supplementary Material for this article can be found online at: https://www.frontiersin.org/articles/10.3389/fimmu.2024.1479483/full#supplementary-material
References
1. Bray F, Laversanne M, Weiderpass E, Soerjomataram I. The ever-increasing importance of cancer as a leading cause of premature death worldwide. Cancer. (2021) 127:3029–30. doi: 10.1002/cncr.33587
2. Sung H, Ferlay J, Siegel RL, Laversanne M, Soerjomataram I, Jemal A, et al. Global cancer statistics 2020: GLOBOCAN estimates of incidence and mortality worldwide for 36 cancers in 185 countries. CA Cancer J Clin. (2021) 71:209–49. doi: 10.3322/caac.21660
3. Society AC. What are advanced and metastatic cancers? (2024). Available online at: https://www.cancer.org/cancer/managing-cancer/advanced-cancer/what-is.html:~:text=But%20other%20locally%20advanced%20cancers,cured%20or%20controlled%20with%20treatment (Accessed August 4, 2024).
4. Fares J, Fares MY, Khachfe HH, Salhab HA, Fares Y. Molecular principles of metastasis: a hallmark of cancer revisited. Signal transduction targeted Ther. (2020) 5:28. doi: 10.1038/s41392-020-0134-x
5. Surveillance E, and results program, in: Cancer stat facts: female breast cancer . Available online at: https://seer.cancer.gov/statfacts/html/breast.html (Accessed November 16, 2024). National Cancer Institute.
6. Parker AL, Benguigui M, Fornetti J, Goddard E, Lucotti S, Insua-Rodriguez J, et al. Current challenges in metastasis research and future innovation for clinical translation. Clin Exp Metastasis. (2022) 39:263–77. doi: 10.1007/s10585-021-10144-5
7. Parisi C, Tagliamento M, Belcaid L, Aldea M, Bayle A, Remon-Masip J, et al. Circulating tumor DNA in clinical trials for solid tumors: Challenges and current applications. J Liquid Biopsy. (2023) 100007. doi: 10.1016/j.jlb.2023.100007
8. Institute NC. Cancer staging (2022). Available online at: https://www.cancer.gov/about-cancer/diagnosis-staging/staging (Accessed July 19, 2024).
9. Gennari A, André F, Barrios C, Cortes J, de Azambuja E, DeMichele A, et al. ESMO Clinical Practice Guideline for the diagnosis, staging and treatment of patients with metastatic breast cancer☆. Ann Oncol. (2021) 32:1475–95. doi: 10.1016/j.annonc.2021.09.019
10. Dingemans A-M, Früh M, Ardizzoni A, Besse B, Faivre-Finn C, Hendriks L, et al. Small-cell lung cancer: ESMO Clinical Practice Guidelines for diagnosis, treatment and follow-up☆. Ann Oncol. (2021) 32:839–53. doi: 10.1016/j.annonc.2021.03.207
11. Postmus PE, Kerr KM, Oudkerk M, Senan S, Waller DA, Vansteenkiste J, et al. Early and locally advanced non-small-cell lung cancer (NSCLC): ESMO Clinical Practice Guidelines for diagnosis, treatment and follow-up. Ann Oncol. (2017) 28:iv1–iv21. doi: 10.1093/annonc/mdx222
12. Michielin O, van Akkooi ACJ, Ascierto PA, Dummer R, Keilholz U.Y2xpbmljYWxndWlkZWxpbmVzQGVzbW8ub3JnEGCEa. Cutaneous melanoma: ESMO Clinical Practice Guidelines for diagnosis, treatment and follow-updagger. Ann Oncol. (2019) 30:1884–901. doi: 10.1093/annonc/mdz411
13. Cervantes A, Adam R, Roselló S, Arnold D, Normanno N, Taïeb J, et al. Metastatic colorectal cancer: ESMO Clinical Practice Guideline for diagnosis, treatment and follow-up☆. Ann Oncol. (2023) 34:10–32. doi: 10.1016/j.annonc.2022.10.003
14. Parker C, Castro E, Fizazi K, Heidenreich A, Ost P, Procopio G, et al. Prostate cancer: ESMO Clinical Practice Guidelines for diagnosis, treatment and follow-up. Ann Oncol. (2020) 31:1119–34. doi: 10.1016/j.annonc.2020.06.011
15. Institute NC. Systemic therapy . Available online at: https://www.cancer.gov/publications/dictionaries/cancer-terms/def/systemic-therapy (Accessed August 4, 2024).
16. Institute NC. Local therapy . Available online at: https://www.cancer.gov/publications/dictionaries/cancer-terms/def/local-therapy (Accessed August 4, 2024).
17. Palumbo MO, Kavan P, Miller WH Jr., Panasci L, Assouline S, Johnson N, et al. Systemic cancer therapy: achievements and challenges that lie ahead. Front Pharmacol. (2013) 4:57. doi: 10.3389/fphar.2013.00057
18. Min HY, Lee HY. Molecular targeted therapy for anticancer treatment. Exp Mol Med. (2022) 54:1670–94. doi: 10.1038/s12276-022-00864-3
19. Ganesh K, Massague J. Targeting metastatic cancer. Nat Med. (2021) 27:34–44. doi: 10.1038/s41591-020-01195-4
20. Hendriks LE, Kerr KM, Menis J, Mok TS, Nestle U, Passaro A, et al. Oncogene-addicted metastatic non-small-cell lung cancer: ESMO Clinical Practice Guideline for diagnosis, treatment and follow-up. Ann Oncol. (2023) 34:339–57. doi: 10.1016/j.annonc.2022.12.009
21. Fassnacht M, Assie G, Baudin E, Eisenhofer G, de la Fouchardiere C, Haak HR, et al. Adrenocortical carcinomas and Malignant phaeochromocytomas: ESMO-EURACAN Clinical Practice Guidelines for diagnosis, treatment and follow-up. Ann Oncol. (2020) 31:1476–90. doi: 10.1016/j.annonc.2020.08.2099
22. Agency EM. Foscan (2016). Available online at: https://www.ema.europa.eu/en/medicines/human/EPAR/foscan (Accessed August 4, 2024).
23. Gabriele N, Burnet NG, Shankar S, WM LA, Hegi-Johnson F. Radiotherapy toxicity (Primer). Nat Reviews: Dis Primers. (2019) 5. doi: 10.1038/s41572-019-0064-5
24. Dharmarajan KV, Rich SE, Johnstone CA, Hertan LM, Wei R, Colbert LE, et al. Top 10 tips palliative care clinicians should know about radiation oncology. J palliative Med. (2018) 21:383–8. doi: 10.1089/jpm.2018.0009
25. Carr C, Ng J, Wigmore T. The side effects of chemotherapeutic agents. Curr Anaesthesia Crit Care. (2008) 19:70–9. doi: 10.1016/j.cacc.2008.01.004
26. Conroy M, Naidoo J. Immune-related adverse events and the balancing act of immunotherapy. Nat Commun. (2022) 13:392. doi: 10.1038/s41467-022-27960-2
27. Mokhtari RB, Homayouni TS, Baluch N, Morgatskaya E, Kumar S, Das B, et al. Combination therapy in combating cancer. Oncotarget. (2017) 8:38022. doi: 10.18632/oncotarget.16723
28. Dunn GP, Old LJ, Schreiber RD. The immunobiology of cancer immunosurveillance and immunoediting. Immunity. (2004) 21:137–48. doi: 10.1016/j.immuni.2004.07.017
29. Dunn GP, Old LJ, Schreiber RD. The three Es of cancer immunoediting. Annu Rev Immunol. (2004) 22:329–60. doi: 10.1146/annurev.immunol.22.012703.104803
30. Dunn GP, Bruce AT, Ikeda H, Old LJ, Schreiber RD. Cancer immunoediting: from immunosurveillance to tumor escape. Nat Immunol. (2002) 3:991–8. doi: 10.1038/ni1102-991
31. Smyth MJ, Godfrey DI, Trapani JA. A fresh look at tumor immunosurveillance and immunotherapy. Nat Immunol. (2001) 2:293–9. doi: 10.1038/86297
32. Finn OJ. Immuno-oncology: understanding the function and dysfunction of the immune system in cancer. . Ann Oncol. (2012) 23 Suppl 8:viii6–9. doi: 10.1093/annonc/mds256
33. Zhou Z, Ni K, Deng H, Chen X. Dancing with reactive oxygen species generation and elimination in nanotheranostics for disease treatment. Advanced Drug delivery Rev. (2020) 158:73–90. doi: 10.1016/j.addr.2020.06.006
34. Institute NC. Immunotherapy . Available online at: https://www.cancer.gov/publications/dictionaries/cancer-terms/def/immunotherapy (Accessed August 4, 2024).
35. Lopes A, Vandermeulen G, Preat V. Cancer DNA vaccines: current preclinical and clinical developments and future perspectives. J Exp Clin Cancer Res. (2019) 38:146. doi: 10.1186/s13046-019-1154-7
36. Vishweshwaraiah YL, Dokholyan NV. mRNA vaccines for cancer immunotherapy. Front Immunol. (2022) 13:1029069. doi: 10.3389/fimmu.2022.1029069
37. Bartlett DL, Liu Z, Sathaiah M, Ravindranathan R, Guo Z, He Y, et al. Oncolytic viruses as therapeutic cancer vaccines. Mol cancer. (2013) 12:1–16. doi: 10.1186/1476-4598-12-103
38. Zhang Y, Zhang Z. The history and advances in cancer immunotherapy: understanding the characteristics of tumor-infiltrating immune cells and their therapeutic implications. Cell Mol Immunol. (2020) 17:807–21. doi: 10.1038/s41423-020-0488-6
39. Zhang P, Zhang G, Wan X. Challenges and new technologies in adoptive cell therapy. J Hematol Oncol. (2023) 16:97. doi: 10.1186/s13045-023-01492-8
40. Du S, Yan J, Xue Y, Zhong Y, Dong Y. Adoptive cell therapy for cancer treatment. In: Exploration. Wiley Online Library (2023) 3(4):20210058. doi: 10.1002/EXP.20210058
41. Immunomodulators CRI. Checkpoint inhibitors, cytokines, agonists, and adjuvants . Available online at: https://www.cancerresearch.org/treatment-types/immunomodulators (Accessed November 16, 2024).
42. Baxter D. Active and passive immunization for cancer. Hum Vaccin Immunother. (2014) 10:2123–9. doi: 10.4161/hv.29604
43. Liu R, Peng L, Zhou L, Huang Z, Zhou C, Huang C. Oxidative stress in cancer immunotherapy: molecular mechanisms and potential applications. Antioxidants (Basel). (2022) 11. doi: 10.3390/antiox11050853
44. Institute NC. Targeted therapy to treat cancer (2022). Available online at: https://www.cancer.gov/about-cancer/treatment/types/targeted-therapies (Accessed August 4, 2024).
45. Institute NC. Monoclonal antibodies (2019). Available online at: https://www.cancer.gov/about-cancer/treatment/types/immunotherapy/monoclonal-antibodies:~:text=They%20are%20a%20type%20of,the%20immune%20system%20against%20cancer (Accessed August 4, 2024).
46. Gerstberger S, Jiang Q, Ganesh K. Metastasis. Cell. (2023) 186:1564–79. doi: 10.1016/j.cell.2023.03.003
47. Melero I, Castanon E, Alvarez M, Champiat S, Marabelle A. Intratumoural administration and tumour tissue targeting of cancer immunotherapies. Nat Rev Clin Oncol. (2021) 18:558–76. doi: 10.1038/s41571-021-00507-y
48. Cann SH, Van Netten J, Van Netten C, Glover D. Spontaneous regression: a hidden treasure buried in time. Med hypotheses. (2002) 58:115–9. doi: 10.1054/mehy.2001.1469
49. Hajdu SI. A note from history: landmarks in history of cancer, part 1. Cancer. (2011) 117:1097–102. doi: 10.1002/cncr.25553
50. Binder M, Roberts C, Spencer N, Antoine D, Cartwright C. On the antiquity of cancer: evidence for metastatic carcinoma in a young man from ancient Nubia (c. 1200 BC). PloS One. (2014) 9:e90924. doi: 10.1371/journal.pone.0090924
51. Center MSKC. Historical timeline . Available online at: https://www.mskcc.org/about/history-milestones/historical-timeline (Accessed August 4, 2024).
52. Oelschlaeger TA. Bacteria as tumor therapeutics? Bioengineered bugs. (2010) 1:146–7. doi: 10.4161/bbug.1.2.11248
53. Coley WB. II. Contribution to the knowledge of sarcoma. Ann Surg. (1891) 14:199–220. doi: 10.1097/00000658-189112000-00015
54. Coley WB. The treatment of Malignant tumors by repeated inoculations of erysipelas. With a report of ten original cases. 1893. Clin Orthop Relat Res. (1991) 262):3–11.
55. Akhtar M, Haider A, Rashid S, Al-Nabet ADM. Paget’s “seed and soil” theory of cancer metastasis: an idea whose time has come. Adv anatomic pathology. (2019) 26:69–74. doi: 10.1097/PAP.0000000000000219
56. Hajdu SI. A note from history: landmarks in history of cancer, part 4. Cancer. (2012) 118:4914–28. doi: 10.1002/cncr.27509
57. Kardamakis D, Baatout S, Bourguignon M, Foray N, Socol Y. History of radiation biology. In: Baatout S, editor. Radiobiology textbook, vol. p . Springer International Publishing, Cham (2023). p. 1–24.
58. Giacobbe A, Abate-Shen C. Modeling metastasis in mice: a closer look. Trends cancer. (2021) 7:916–29. doi: 10.1016/j.trecan.2021.06.010
59. Hanahan D, Weinberg RA. The hallmarks of cancer. cell. (2000) 100:57–70. doi: 10.1016/S0092-8674(00)81683-9
60. Jiang S, Liu Y, Zheng H, Zhang L, Zhao H, Sang X, et al. Evolutionary patterns and research frontiers in neoadjuvant immunotherapy: a bibliometric analysis. Int J Surgery. (2023) 109:2774–83. doi: 10.1097/JS9.0000000000000492
61. Couzin-Frankel J. Breakthrough of the year 2013. Cancer Immunother Science. (2013) 342:1432–3. doi: 10.1126/science.342.6165.1432
62. Goldmacher GV, Khilnani AD, Andtbacka RH, Luke JJ, Hodi FS, Marabelle A, et al. Response criteria for intratumoral immunotherapy in solid tumors: itRECIST. J Clin Oncol. (2020) 38:2667–76. doi: 10.1200/JCO.19.02985
63. Prise KM, O'Sullivan JM. Radiation-induced bystander signalling in cancer therapy. Nat Rev Cancer. (2009) 9:351–60. doi: 10.1038/nrc2603
64. Kingsley D. An interesting case of possible abscopal effect in Malignant melanoma. Br J radiology. (1975) 48:863–6. doi: 10.1259/0007-1285-48-574-863
65. Marabelle A, Tselikas L, De Baere T, Houot R. Intratumoral immunotherapy: using the tumor as the remedy. Ann Oncol. (2017) 28:xii33–43. doi: 10.1093/annonc/mdx683
66. DAILYMED. YERVOY- ipilimumab injection (2023). Available online at: https://dailymed.nlm.nih.gov/dailymed/drugInfo.cfm?setid=2265ef30-253e-11df-8a39-0800200c9a66 (Accessed August 4, 2024).
67. DAILYMED. AMTAGVI- lifileucel suspension (2024). Available online at: https://dailymed.nlm.nih.gov/dailymed/drugInfo.cfm?setid=91b0c63a-9a7e-46d0-a562-dc0d7d7867f3 (Accessed August 5, 2024).
68. DAILYMED. IMLYGIC- talimogene laherparepvec injection, suspension (2023). Available online at: https://dailymed.nlm.nih.gov/dailymed/drugInfo.cfm?setid=64ffb680-ea8c-42fc-9649-9e8c0eb77ddb (Accessed August 5, 2024).
69. Todo T, Ito H, Ino Y, Ohtsu H, Ota Y, Shibahara J, et al. Intratumoral oncolytic herpes virus G47∆ for residual or recurrent glioblastoma: a phase 2 trial. Nat Med. (2022) 28:1630–9. doi: 10.1038/s41591-022-01897-x
70. Yi L, Ning Z, Xu L, Shen Y, Zhu X, Yu W, et al. The combination treatment of oncolytic adenovirus H101 with nivolumab for refractory advanced hepatocellular carcinoma: An open-label, single-arm, pilot study. ESMO Open. (2024) 9:102239. doi: 10.1016/j.esmoop.2024.102239
71. Marabelle A, Andtbacka R, Harrington K, Melero I, Leidner R, de Baere T, et al. Starting the fight in the tumor: expert recommendations for the development of human intratumoral immunotherapy (HIT-IT). Ann Oncol. (2018) 29:2163–74. doi: 10.1093/annonc/mdy423
72. Champiat S, Tselikas L, Farhane S, Raoult T, Texier M, Lanoy E, et al. Intratumoral immunotherapy: from trial design to clinical practice. Clin Cancer Res. (2021) 27:665–79. doi: 10.1158/1078-0432.CCR-20-0473
73. Mullins SR, Vasilakos JP, Deschler K, Grigsby I, Gillis P, John J, et al. Intratumoral immunotherapy with TLR7/8 agonist MEDI9197 modulates the tumor microenvironment leading to enhanced activity when combined with other immunotherapies. J immunotherapy cancer. (2019) 7:1–18. doi: 10.1186/s40425-019-0724-8
74. Osorio JC, Knorr DA, Weitzenfeld P, Yao N, Baez M, DiLillo M, et al. Intratumoral Fc-optimized agonistic CD40 antibody induces tumor rejection and systemic antitumor immunity in patients with metastatic cancer. Res Sq. (2024). doi: 10.21203/rs.3.rs-4244833/v1
75. Floros T, Tarhini AA. Anticancer cytokines: biology and clinical effects of interferon-α2, interleukin (IL)-2, IL-15, IL-21, and IL-12. In: Seminars in oncology. Elsevier (2015) 42(4):539–48. doi: 10.1053/j.seminoncol.2015.05.015
76. Berraondo P, Sanmamed MF, Ochoa MC, Etxeberria I, Aznar MA, Pérez-Gracia JL, et al. Cytokines in clinical cancer immunotherapy. Br J cancer. (2019) 120:6–15. doi: 10.1038/s41416-018-0328-y
77. He X, Xu C. Immune checkpoint signaling and cancer immunotherapy. Cell Res. (2020) 30:660–9. doi: 10.1038/s41422-020-0343-4
78. Pardoll DM. The blockade of immune checkpoints in cancer immunotherapy. Nat Rev Cancer. (2012) 12:252–64. doi: 10.1038/nrc3239
79. Wu Y-Y, Sun T-K, Chen M-S, Munir M, Liu H-J. Oncolytic viruses-modulated immunogenic cell death, apoptosis and autophagy linking to virotherapy and cancer immune response. Front Cell Infection Microbiol. (2023) 13:1142172. doi: 10.3389/fcimb.2023.1142172
80. Hong WX, Haebe S, Lee AS, Westphalen CB, Norton JA, Jiang W, et al. Intratumoral immunotherapy for early-stage solid tumors. Clin Cancer Res. (2020) 26:3091–9. doi: 10.1158/1078-0432.CCR-19-3642
81. Brito-Orama S, Sheth RA. The contemporary landscape and future directions of intratumoral immunotherapy. J Immunotherapy Precis Oncol. (2023) 6:84–90. doi: 10.36401/JIPO-22-8
82. Ghosn M, Tselikas L, Champiat S, Deschamps F, Bonnet B, Carre É, et al. Intratumoral immunotherapy: is it ready for prime time? Curr Oncol Rep. (2023) 25:857–67. doi: 10.1007/s11912-023-01422-4
83. Janku F, Fu S, Murthy R, Karp D, Hong D, Tsimberidou A, et al. 383 First-in-man clinical trial of intratumoral injection of clostridium Novyi-NT spores in combination with pembrolizumab in patients with treatment-refractory advanced solid tumors. J ImmunoTherapy Cancer. (2020) 8:A233–A. doi: 10.1136/jitc-2020-SITC2020.0383
84. Papa S, Adami A, Metoudi M, Beatson R, George MS, Achkova D, et al. Intratumoral pan-ErbB targeted CAR-T for head and neck squamous cell carcinoma: interim analysis of the T4 immunotherapy study. J Immunother Cancer. (2023) 11:e007162. doi: 10.1136/jitc-2023-007162
85. Zawit M, Swami U, Awada H, Arnouk J, Milhem M, Zakharia Y. Current status of intralesional agents in treatment of Malignant melanoma. Ann Trans Med. (2021) 9. doi: 10.21037/atm-21-491
86. El-Sayes N, Vito A, Mossman K. Tumor heterogeneity: a great barrier in the age of cancer immunotherapy. Cancers. (2021) 13:806. doi: 10.3390/cancers13040806
87. Som A, Rosenboom JG, Chandler A, Sheth RA, Wehrenberg-Klee E. Image-guided intratumoral immunotherapy: Developing a clinically practical technology. Adv Drug Delivery Rev. (2022) 189:114505. doi: 10.1016/j.addr.2022.114505
88. Pohl H, Robertson DJ. Colorectal cancers detected after colonoscopy frequently result from missed lesions. Clin Gastroenterol Hepatology. (2010) 8:858–64. doi: 10.1016/j.cgh.2010.06.028
89. Hovda T, Larsen M, Romundstad L, Sahlberg KK, Hofvind S. Breast cancer missed at screening; hindsight or mistakes? Eur J Radiol. (2023) 165:110913. doi: 10.1016/j.ejrad.2023.110913
90. Shimauchi A, Jansen SA, Abe H, Jaskowiak N, Schmidt RA, Newstead GM. Breast cancers not detected at MRI: review of false-negative lesions. Am J Roentgenology. (2010) 194:1674–9. doi: 10.2214/AJR.09.3568
91. Xia Y, Yang R, Zhu J, Wang H, Li Y, Fan J, et al. Engineered nanomaterials trigger abscopal effect in immunotherapy of metastatic cancers. Front Bioengineering Biotechnol. (2022) 10:890257. doi: 10.3389/fbioe.2022.890257
92. Aznar MA, Planelles L, Perez-Olivares M, Molina C, Garasa S, Etxeberría I, et al. Immunotherapeutic effects of intratumoral nanoplexed poly I:C. J Immunother Cancer. (2019) 7:116. doi: 10.1186/s40425-019-0568-2
93. Duewell P, Steger A, Lohr H, Bourhis H, Hoelz H, Kirchleitner S, et al. RIG-I-like helicases induce immunogenic cell death of pancreatic cancer cells and sensitize tumors toward killing by CD8+ T cells. Cell Death Differentiation. (2014) 21:1825–37. doi: 10.1038/cdd.2014.96
94. Shieh J-J, Huang S-W. The TLR7 agonist imiquimod triggers immunogenic cell death in cancer cells. Ann Oncol. (2017) 28:ix102. doi: 10.1093/annonc/mdx621.031
95. See WA, Zhang G, Chen F, Cao Y, Langenstroer P, Sandlow J. Bacille-Calmette Guerin induces caspase-independent cell death in urothelial carcinoma cells together with release of the necrosis-associated chemokine high molecular group box protein 1. BJU Int. (2009) 103:1714–20. doi: 10.1111/j.1464-410X.2008.08274.x
96. Bommareddy PK, Zloza A, Rabkin SD, Kaufman HL. Oncolytic virus immunotherapy induces immunogenic cell death and overcomes STING deficiency in melanoma. Oncoimmunology. (2019) 8:e1591875. doi: 10.1080/2162402X.2019.1591875
97. Araki H, Tazawa H, Kanaya N, Kajiwara Y, Yamada M, Hashimoto M, et al. Oncolytic virus-mediated p53 overexpression promotes immunogenic cell death and efficacy of PD-1 blockade in pancreatic cancer. Mol Therapy-Oncolytics. (2022) 27:3–13. doi: 10.1016/j.omto.2022.09.003
98. Annels NE, Simpson GR, Denyer M, Arif M, Coffey M, Melcher A, et al. Oncolytic reovirus-mediated recruitment of early innate immune responses reverses immunotherapy resistance in prostate tumors. Mol Therapy-Oncolytics. (2021) 20:434–46. doi: 10.1016/j.omto.2020.09.010
99. Koks CA, Garg AD, Ehrhardt M, Riva M, Vandenberk L, Boon L, et al. Newcastle disease virotherapy induces long-term survival and tumor-specific immune memory in orthotopic glioma through the induction of immunogenic cell death. Int J cancer. (2015) 136:E313–E25. doi: 10.1002/ijc.29202
100. Wang M, Duan Y, Yang M, Guo Y, Li F, Wang J, et al. The analysis of immunogenic cell death induced by ablation at different temperatures in hepatocellular carcinoma cells. Front Cell Dev Biol. (2023) 11:1146195. doi: 10.3389/fcell.2023.1146195
101. Yu Z, Geng J, Zhang M, Zhou Y, Fan Q, Chen J. Treatment of osteosarcoma with microwave thermal ablation to induce immunogenic cell death. Oncotarget. (2014) 5:6526. doi: 10.18632/oncotarget.2310
102. Korbelik M, Zhang W, Merchant S. Involvement of damage-associated molecular patterns in tumor response to photodynamic therapy: surface expression of calreticulin and high-mobility group box-1 release. Cancer Immunology Immunother. (2011) 60:1431–7. doi: 10.1007/s00262-011-1047-x
103. Ringel-Scaia VM, Beitel-White N, Lorenzo MF, Brock RM, Huie KE, Coutermarsh-Ott S, et al. High-frequency irreversible electroporation is an effective tumor ablation strategy that induces immunologic cell death and promotes systemic anti-tumor immunity. EBioMedicine. (2019) 44:112–25. doi: 10.1016/j.ebiom.2019.05.036
104. Bugaut H, Bruchard M, Berger H, Derangère V, Odoul L, Euvrard R, et al. Bleomycin exerts ambivalent antitumor immune effect by triggering both immunogenic cell death and proliferation of regulatory T cells. PloS One. (2013) 8:e65181. doi: 10.1371/journal.pone.0065181
105. Novosiadly R, Schaer D, Amaladas N, Rasmussen E, Lu ZH, Sonyi A, et al. Abstract 4549: Pemetrexed enhances anti-tumor efficacy of PD1 pathway blockade by promoting intra tumor immune response via immunogenic tumor cell death and T cell intrinsic mechanisms. Cancer Res. (2018) 78:4549–. doi: 10.1158/1538-7445.Am2018-4549
106. Tesniere A, Schlemmer F, Boige V, Kepp O, Martins I, Ghiringhelli F, et al. Immunogenic death of colon cancer cells treated with oxaliplatin. Oncogene. (2010) 29:482–91. doi: 10.1038/onc.2009.356
107. Li C, Sun H, Wei W, Liu Q, Wang Y, Zhang Y, et al. Mitoxantrone triggers immunogenic prostate cancer cell death via p53-dependent PERK expression. Cell Oncol (Dordr). (2020) 43:1099–116. doi: 10.1007/s13402-020-00544-2
108. Lau TS, Chan LKY, Man GCW, Wong CH, Lee JHS, Yim SF, et al. Paclitaxel induces immunogenic cell death in ovarian cancer via TLR4/IKK2/SNARE-dependent exocytosis. Cancer Immunol Res. (2020) 8:1099–111. doi: 10.1158/2326-6066.CIR-19-0616
109. Lau T-S, Chan LK-Y, Man GC-W, Kwong J. Abstract 1232: Paclitaxel induces immunogenic cell death in ovarian cancer via TLR4-independent and dependent pathways. Cancer Res. (2019) 79:1232. doi: 10.1158/1538-7445.Am2019-1232
110. Gulla A, Morelli E, Samur MK, Botta C, Hideshima T, Bianchi G, et al. Bortezomib induces anti–multiple myeloma immune response mediated by cGAS/STING pathway activation. Blood Cancer discovery. (2021) 2:468–83. doi: 10.1158/2643-3230.BCD-21-0047
111. Pozzi C, Cuomo A, Spadoni I, Magni E, Silvola A, Conte A, et al. The EGFR-specific antibody cetuximab combined with chemotherapy triggers immunogenic cell death. Nat Med. (2016) 22:624–31. doi: 10.1038/nm.4078
112. Hossain DMS, Javaid S, Cai M, Zhang C, Sawant A, Hinton M, et al. Dinaciclib induces immunogenic cell death and enhances anti-PD1–mediated tumor suppression. J Clin Invest. (2018) 128:644–54. doi: 10.1172/JCI94586
113. Liu P, Zhao L, Pol J, Levesque S, Petrazzuolo A, Pfirschke C, et al. Crizotinib-induced immunogenic cell death in non-small cell lung cancer. Nat Commun. (2019) 10:1486. doi: 10.1038/s41467-019-09415-3
114. Jarauta V, Jaime P, Gonzalo O, de Miguel D, Ramírez-Labrada A, Martínez-Lostao L, et al. Inhibition of autophagy with chloroquine potentiates carfilzomib-induced apoptosis in myeloma cells in vitro and in vivo. Cancer Lett. (2016) 382:1–10. doi: 10.1016/j.canlet.2016.08.019
115. Golden EB, Frances D, Pellicciotta I, Demaria S, Helen Barcellos-Hoff M, Formenti SC. Radiation fosters dose-dependent and chemotherapy-induced immunogenic cell death. Oncoimmunology. (2014) 3:e28518. doi: 10.4161/onci.28518
116. Sequeira GR, Sahores A, Dalotto-Moreno T, Perrotta RM, Pataccini G, Vanzulli SI, et al. Enhanced antitumor immunity via endocrine therapy prevents mammary tumor relapse and increases immune checkpoint blockade sensitivity. Cancer Res. (2021) 81:1375–87. doi: 10.1158/0008-5472.CAN-20-1441
117. Chen M, Linstra R, van Vugt MA. Genomic instability, inflammatory signaling and response to cancer immunotherapy. Biochim Biophys Acta (BBA)-Reviews Cancer. (2022) 1877:188661. doi: 10.1016/j.bbcan.2021.188661
118. Wang B, Li R, Wu S, Liu X, Ren J, Li J, et al. Breast cancer resistance to cyclin-dependent kinases 4/6 inhibitors: intricacy of the molecular mechanisms. Front Oncol. (2021) 11:651541. doi: 10.3389/fonc.2021.651541
119. Zhang M, Zhang L, Hei R, Li X, Cai H, Wu X, et al. CDK inhibitors in cancer therapy, an overview of recent development. Am J Cancer Res. (2021) 11:1913–35.
120. Feehley T, O’Donnell CW, Mendlein J, Karande M, McCauley T. Drugging the epigenome in the age of precision medicine. Clin Epigenetics. (2023) 15:6. doi: 10.1186/s13148-022-01419-z
121. Costa P, Sales SLA, Pinheiro DP, Pontes LQ, Maranhão SSA, Pessoa CdÓ, et al. Epigenetic reprogramming in cancer: From diagnosis to treatment. Front Cell Dev Biol. (2023) 11:1116805. doi: 10.3389/fcell.2023.1116805
122. Slade D. PARP and PARG inhibitors in cancer treatment. Genes Dev. (2020) 34:360–94. doi: 10.1101/gad.334516.119
123. Bai Y, Wang W, Wang J. Targeting DNA repair pathways: Mechanisms and potential applications in cancer therapy. Genome Instability Disease. (2020) 1:318–38. doi: 10.1007/s42764-020-00026-7
124. Ferguson LR, Chen H, Collins AR, Connell M, Damia G, Dasgupta S, et al. Genomic instability in human cancer: Molecular insights and opportunities for therapeutic attack and prevention through diet and nutrition. Semin Cancer Biol. (2015) 35:S5–S24. doi: 10.1016/j.semcancer.2015.03.005
125. Garrett MD, Collins I. Anticancer therapy with checkpoint inhibitors: what, where and when? Trends Pharmacol Sci. (2011) 32:308–16. doi: 10.1016/j.tips.2011.02.014
126. Li T, Yang Y, Qi H, Cui W, Zhang L, Fu X, et al. CRISPR/Cas9 therapeutics: progress and prospects. Signal Transduct Target Ther. (2023) 8:36. doi: 10.1038/s41392-023-01309-7
127. Eso Y, Shimizu T, Takeda H, Takai A, Marusawa H. Microsatellite instability and immune checkpoint inhibitors: toward precision medicine against gastrointestinal and hepatobiliary cancers. J gastroenterology. (2020) 55:15–26. doi: 10.1007/s00535-019-01620-7
128. Yang M, Olaoba OT, Zhang C, Kimchi ET, Staveley-O’Carroll KF, Li G. Cancer immunotherapy and delivery system: an update. Pharmaceutics. (2022) 14:1630. doi: 10.3390/pharmaceutics14081630
129. Xie N, Shen G, Gao W, Huang Z, Huang C, Fu L. Neoantigens: promising targets for cancer therapy. Signal Transduction Targeted Ther. (2023) 8:9. doi: 10.1038/s41392-022-01270-x
130. Cao Y, Langer R, Ferrara N. Targeting angiogenesis in oncology, ophthalmology and beyond. Nat Rev Drug Discovery. (2023) 22:476–95. doi: 10.1038/s41573-023-00671-z
131. Carneiro BA, El-Deiry WS. Targeting apoptosis in cancer therapy. Nat Rev Clin Oncol. (2020) 17:395–417. doi: 10.1038/s41571-020-0341-y
132. Diepstraten ST, Anderson MA, Czabotar PE, Lessene G, Strasser A, Kelly GL. The manipulation of apoptosis for cancer therapy using BH3-mimetic drugs. Nat Rev Cancer. (2022) 22:45–64. doi: 10.1038/s41568-021-00407-4
133. Gandalovičová A, Rosel D, Fernandes M, Veselý P, Heneberg P, Čermák V, et al. Migrastatics—anti-metastatic and anti-invasion drugs: promises and challenges. Trends cancer. (2017) 3:391–406. doi: 10.1016/j.trecan.2017.04.008
134. Raudenska M, Petrlakova K, Jurinakova T, Leischner Fialova J, Fojtu M, Jakubek M, et al. Engine shutdown: migrastatic strategies and prevention of metastases. Trends Cancer. (2023) 9:293–308. doi: 10.1016/j.trecan.2023.01.001
135. Hamidi H, Ivaska J. Every step of the way: integrins in cancer progression and metastasis. Nat Rev Cancer. (2018) 18:533–48. doi: 10.1038/s41568-018-0038-z
136. Hou J, Karin M, Sun B. Targeting cancer-promoting inflammation—have anti-inflammatory therapies come of age? Nat Rev Clin Oncol. (2021) 18:261–79. doi: 10.1038/s41571-020-00459-9
137. Gao J, Pickett HA. Targeting telomeres: advances in telomere maintenance mechanism-specific cancer therapies. Nat Rev Cancer. (2022) 22:515–32. doi: 10.1038/s41568-022-00490-1
138. Keith WN, Bilsland A, Hardie M, Evans TJ. Drug Insight: cancer cell immortality—telomerase as a target for novel cancer gene therapies. Nat Clin Pract Oncol. (2004) 1:88–96. doi: 10.1038/ncponc0044
139. Lythgoe MP, Mullish BH, Frampton AE, Krell J. Polymorphic microbes: a new emerging hallmark of cancer. Trends Microbiol. (2022) 30:1131–4. doi: 10.1016/j.tim.2022.08.004
140. Schmitt CA, Wang B, Demaria M. Senescence and cancer - role and therapeutic opportunities. Nat Rev Clin Oncol. (2022) 19:619–36. doi: 10.1038/s41571-022-00668-4
141. Fanelli GN, Naccarato AG, Scatena C. Recent advances in cancer plasticity: cellular mechanisms, surveillance strategies, and therapeutic optimization. Front Oncol. (2020) 10:569. doi: 10.3389/fonc.2020.00569
142. Gu Y, Zhang Z, Ten Dijke P. Harnessing epithelial-mesenchymal plasticity to boost cancer immunotherapy. Cell Mol Immunol. (2023) 20:318–40. doi: 10.1038/s41423-023-00980-8
143. Bhat GR, Sethi I, Sadida HQ, Rah B, Mir R, Algehainy N, et al. Cancer cell plasticity: From cellular, molecular, and genetic mechanisms to tumor heterogeneity and drug resistance. Cancer Metastasis Rev. (2024) 43:197–228. doi: 10.1007/s10555-024-10172-z
144. Stine ZE, Schug ZT, Salvino JM, Dang CV. Targeting cancer metabolism in the era of precision oncology. Nat Rev Drug Discovery. (2022) 21:141–62. doi: 10.1038/s41573-021-00339-6
145. Pandey P, Khan F, Upadhyay TK, Seungjoon M, Park MN, Kim B. New insights about the PDGF/PDGFR signaling pathway as a promising target to develop cancer therapeutic strategies. Biomedicine Pharmacotherapy. (2023) 161:114491. doi: 10.1016/j.biopha.2023.114491
146. Hanahan D, Weinberg RA. Hallmarks of cancer: the next generation. cell. (2011) 144:646–74. doi: 10.1016/j.cell.2011.02.013
147. Hanahan D. Hallmarks of cancer: new dimensions. Cancer discovery. (2022) 12:31–46. doi: 10.1158/2159-8290.CD-21-1059
148. Chen DS, Mellman I. Oncology meets immunology: the cancer-immunity cycle. immunity. (2013) 39:1–10. doi: 10.1016/j.immuni.2013.07.012
149. Senders ZJ, Martin RCG 2nd. Intratumoral immunotherapy and tumor ablation: A local approach with broad potential. Cancers (Basel). (2022) 14:1754. doi: 10.3390/cancers14071754
150. Gu C, Wang X, Wang K, Xie F, Chen L, Ji H, et al. Cryoablation triggers type I interferon-dependent antitumor immunity and potentiates immunotherapy efficacy in lung cancer. J Immunotherapy Cancer. (2024) 12:e008386. doi: 10.1136/jitc-2023-008386
151. Carbone C, Piro G, Agostini A, Delfino P, De Sanctis F, Nasca V, et al. Intratumoral injection of TLR9 agonist promotes an immunopermissive microenvironment transition and causes cooperative antitumor activity in combination with anti-PD1 in pancreatic cancer. J Immunother Cancer. (2021) 9:e002876. doi: 10.1136/jitc-2021-002876
152. Yap TA, Omlin A, De Bono JS. Development of therapeutic combinations targeting major cancer signaling pathways. J Clin Oncol. (2013) 31:1592–605. doi: 10.1200/JCO.2011.37.6418
153. Sochacka-Ćwikła A, Mączyński M, Regiec A. FDA-approved small molecule compounds as drugs for solid cancers from early 2011 to the end of 2021. Molecules. (2022) 27:2259. doi: 10.3390/molecules27072259
154. Zappasodi R, Merghoub T, Wolchok JD. Emerging concepts for immune checkpoint blockade-based combination therapies. Cancer Cell. (2018) 33:581–98. doi: 10.1016/j.ccell.2018.03.005
155. Guo ZS, Liu Z, Bartlett DL. Oncolytic immunotherapy: dying the right way is a key to eliciting potent antitumor immunity. Front Oncol. (2014) 4:74. doi: 10.3389/fonc.2014.00074
156. Galluzzi L, Vitale I, Warren S, Adjemian S, Agostinis P, Martinez AB, et al. Consensus guidelines for the definition, detection and interpretation of immunogenic cell death. J Immunother Cancer. (2020) 8:e000337. doi: 10.1136/jitc-2019-000337
157. Fucikova J, Kepp O, Kasikova L, Petroni G, Yamazaki T, Liu P, et al. Detection of immunogenic cell death and its relevance for cancer therapy. Cell Death disease. (2020) 11:1013. doi: 10.1038/s41419-020-03221-2
158. Tang D, Kang R, Berghe TV, Vandenabeele P, Kroemer G. The molecular machinery of regulated cell death. Cell Res. (2019) 29:347–64. doi: 10.1038/s41422-019-0164-5
159. Shi F, Huang X, Hong Z, Lu N, Huang X, Liu L, et al. Improvement strategy for immune checkpoint blockade: A focus on the combination with immunogenic cell death inducers. Cancer Lett. (2023) 562:216167. doi: 10.1016/j.canlet.2023.216167
160. Calvillo-Rodríguez KM, Lorenzo-Anota HY, Rodríguez-Padilla C, Martínez-Torres AC, Scott-Algara D. Immunotherapies inducing immunogenic cell death in cancer: insight of the innate immune system. Front Immunol. (2023) 14:1294434. doi: 10.3389/fimmu.2023.1294434
161. Kepp O, Marabelle A, Zitvogel L, Kroemer G. Oncolysis without viruses—inducing systemic anticancer immune responses with local therapies. Nat Rev Clin Oncol. (2020) 17:49–64. doi: 10.1038/s41571-019-0272-7
162. Gujar S, Pol JG, Kroemer G. Heating it up: Oncolytic viruses make tumors ‘hot’and suitable for checkpoint blockade immunotherapies. Taylor Francis;. (2018) p:e1442169. doi: 10.1080/2162402X.2018.1442169
163. Melero I, Gato M, Shekarian T, Aznar A, Valsesia-Wittmann S, Caux C, et al. Repurposing infectious disease vaccines for intratumoral immunotherapy. J Immunotherapy Cancer. (2020) 8:e000443. doi: 10.1136/jitc-2019-000443
164. Herrada AA, Rojas-Colonelli N, González-Figueroa P, Roco J, Oyarce C, Ligtenberg MA, et al. Harnessing DNA-induced immune responses for improving cancer vaccines. Hum Vaccines immunotherapeutics. (2012) 8:1682–93. doi: 10.4161/hv.22345
165. Kaczmarek M, Poznańska J, Fechner F, Michalska N, Paszkowska S, Napierała A, et al. Cancer vaccine therapeutics: limitations and effectiveness—A literature review. Cells. (2023) 12:2159. doi: 10.3390/cells12172159
166. Janes ME, Gottlieb AP, Park KS, Zhao Z, Mitragotri S. Cancer vaccines in the clinic. Bioengineering Trans Med. (2024) 9:e10588. doi: 10.1002/btm2.10588
167. Fan T, Zhang M, Yang J, Zhu Z, Cao W, Dong C. Therapeutic cancer vaccines: advancements, challenges, and prospects. Signal Transduction Targeted Ther. (2023) 8:450. doi: 10.1038/s41392-023-01674-3
168. Xia Y, Sun M, Huang H, Jin W-L. Drug repurposing for cancer therapy. Signal Transduction Targeted Ther. (2024) 9:92. doi: 10.1038/s41392-024-01808-1
169. Vandeborne L, Pantziarka P, Van Nuffel AMT, Bouche G. Repurposing infectious diseases vaccines against cancer. Front Oncol. (2021) 11:688755. doi: 10.3389/fonc.2021.688755
170. Newman JH, Chesson CB, Herzog NL, Bommareddy PK, Aspromonte SM, Pepe R, et al. Intratumoral injection of the seasonal flu shot converts immunologically cold tumors to hot and serves as an immunotherapy for cancer. Proc Natl Acad Sci U S A. (2020) 117:1119–28. doi: 10.1073/pnas.1904022116
171. Aznar MA, Molina C, Teijeira A, Rodriguez I, Azpilikueta A, Garasa S, et al. Repurposing the yellow fever vaccine for intratumoral immunotherapy. EMBO Mol Med. (2020) 12:e10375. doi: 10.15252/emmm.201910375
172. Fu R, Qi R, Xiong H, Lei X, Jiang Y, He J, et al. Combination therapy with oncolytic virus and T cells or mRNA vaccine amplifies antitumor effects. Signal Transduction Targeted Ther. (2024) 9:118. doi: 10.1038/s41392-024-01824-1
173. Yan Z, Zhang Z, Chen Y, Xu J, Wang J, Wang Z. Enhancing cancer therapy: the integration of oncolytic virus therapy with diverse treatments. Cancer Cell Int. (2024) 24:242. doi: 10.1186/s12935-024-03424-z
174. Guo ZS, Lu B, Guo Z, Giehl E, Feist M, Dai E, et al. Vaccinia virus-mediated cancer immunotherapy: cancer vaccines and oncolytics. J immunotherapy cancer. (2019) 7:1–21. doi: 10.1186/s40425-018-0495-7
175. Liu Z, Ravindranathan R, Kalinski P, Guo ZS, Bartlett DL. Rational combination of oncolytic vaccinia virus and PD-L1 blockade works synergistically to enhance therapeutic efficacy. Nat Commun. (2017) 8:14754. doi: 10.1038/ncomms14754
176. Chen C-Y, Wang P-Y, Hutzen B, Sprague L, Swain HM, Love JK, et al. Cooperation of oncolytic herpes virotherapy and PD-1 blockade in murine rhabdomyosarcoma models. Sci Rep. (2017) 7:2396. doi: 10.1038/s41598-017-02503-8
177. Wang G, Kang X, Chen KS, Jehng T, Jones L, Chen J, et al. An engineered oncolytic virus expressing PD-L1 inhibitors activates tumor neoantigen-specific T cell responses. Nat Commun. (2020) 11:1395. doi: 10.1038/s41467-020-15229-5
178. Bazan-Peregrino M, Garcia-Carbonero R, Laquente B, Álvarez R, Mato-Berciano A, Gimenez-Alejandre M, et al. VCN-01 disrupts pancreatic cancer stroma and exerts antitumor effects. J Immunother Cancer. (2021) 9:e003254. doi: 10.1136/jitc-2021-003254
179. Garcia-Carbonero R, Bazan-Peregrino M, Gil-Martín M, Álvarez R, Macarulla T, Riesco-Martinez MC, et al. Phase I, multicenter, open-label study of intravenous VCN-01 oncolytic adenovirus with or without nab-paclitaxel plus gemcitabine in patients with advanced solid tumors. J Immunother Cancer. (2022) 10:e003255. doi: 10.1136/jitc-2021-003255
180. Perez MC, Miura JT, Naqvi SMH, Kim Y, Holstein A, Lee D, et al. Talimogene laherparepvec (TVEC) for the treatment of advanced melanoma: A single-institution experience. Ann Surg Oncol. (2018) 25:3960–5. doi: 10.1245/s10434-018-6803-0
181. Soliman H, Hogue D, Han H, Mooney B, Costa R, Lee MC, et al. Oncolytic T-VEC virotherapy plus neoadjuvant chemotherapy in nonmetastatic triple-negative breast cancer: a phase 2 trial. Nat Med. (2023) 29:450–7. doi: 10.1038/s41591-023-02210-0
182. Hecht JR, Pless M, Cubillo A, Calvo A, Chon HJ, Liu C, et al. Early safety from a phase I, multicenter, open-label clinical trial of talimogene laherparepvec (T-VEC) injected (inj) into liver tumors in combination with pembrolizumab (pem). J Clin Oncol. (2020) 38:3015. doi: 10.1200/JCO.2020.38.15_suppl.3015
183. Zheng M, Huang J, Tong A, Yang H. Oncolytic viruses for cancer therapy: barriers and recent advances. Mol Therapy-Oncolytics. (2019) 15:234–47. doi: 10.1016/j.omto.2019.10.007
184. Garcia-Carbonero R, Salazar R, Duran I, Osman-Garcia I, Paz-Ares L, Bozada JM, et al. Phase 1 study of intravenous administration of the chimeric adenovirus enadenotucirev in patients undergoing primary tumor resection. J immunotherapy cancer. (2017) 5:1–13. doi: 10.1186/s40425-017-0277-7
185. Anguille S, Smits EL, Lion E, van Tendeloo VF, Berneman ZN. Clinical use of dendritic cells for cancer therapy. Lancet Oncol. (2014) 15:e257–67. doi: 10.1016/s1470-2045(13)70585-0
186. Abd-Aziz N, Poh CL. Development of peptide-based vaccines for cancer. J Oncol. (2022) 2022:9749363. doi: 10.1155/2022/9749363
187. Najafi S, Mortezaee K. Advances in dendritic cell vaccination therapy of cancer. Biomedicine Pharmacotherapy. (2023) 164:114954. doi: 10.1016/j.biopha.2023.114954
188. Spicer J, Marabelle A, Baurain JF, Jebsen NL, Jøssang DE, Awada A, et al. Safety, antitumor activity, and T-cell responses in a dose-ranging phase I trial of the oncolytic peptide LTX-315 in patients with solid tumors. Clin Cancer Res. (2021) 27:2755–63. doi: 10.1158/1078-0432.Ccr-20-3435
189. Pellegatta S, Poliani PL, Stucchi E, Corno D, Colombo CA, Orzan F, et al. Intra-tumoral dendritic cells increase efficacy of peripheral vaccination by modulation of glioma microenvironment. Neuro-Oncology. (2010) 12:377–88. doi: 10.1093/neuonc/nop024
190. Institute CR. Immunomodulators: checkpoint inhibitors, cytokines, agonists, and adjuvants . Available online at: https://www.cancerresearch.org/treatment-types/immunomodulators (Accessed July 15, 2024).
191. Owen AM, Fults JB, Patil NK, Hernandez A, Bohannon JK. TLR agonists as mediators of trained immunity: mechanistic insight and immunotherapeutic potential to combat infection. Front Immunol. (2021) 11:622614. doi: 10.3389/fimmu.2020.622614
192. Shekarian T, Valsesia-Wittmann S, Brody J, Michallet MC, Depil S, Caux C, et al. Pattern recognition receptors: immune targets to enhance cancer immunotherapy. Ann Oncol. (2017) 28:1756–66. doi: 10.1093/annonc/mdx179
193. Le Naour J, Zitvogel L, Galluzzi L, Vacchelli E, Kroemer G. Trial watch: STING agonists in cancer therapy. Oncoimmunology. (2020) 9:1777624. doi: 10.1080/2162402X.2020.1777624
194. Marritt KL, Hildebrand KM, Hildebrand KN, Singla AK, Zemp FJ, Mahoney DJ, et al. Intratumoral STING activation causes durable immunogenic tumor eradication in the KP soft tissue sarcoma model. Front Immunol. (2022) 13:1087991. doi: 10.3389/fimmu.2022.1087991
195. Harrington K, Brody J, Ingham M, Strauss J, Cemerski S, Wang M, et al. Preliminary results of the first-in-human (FIH) study of MK-1454, an agonist of stimulator of interferon genes (STING), as monotherapy or in combination with pembrolizumab (pembro) in patients with advanced solid tumors or lymphomas. Ann Oncol. (2018) 29:viii712. doi: 10.1093/annonc/mdy424.015
196. Rolfo C, Giovannetti E, Martinez P, McCue S, Naing A. Applications and clinical trial landscape using Toll-like receptor agonists to reduce the toll of cancer. NPJ Precis Oncol. (2023) 7:26. doi: 10.1038/s41698-023-00364-1
197. Liu B, Zhou H, Tan L, Siu KTH, Guan X-Y. Exploring treatment options in cancer: tumor treatment strategies. Signal Transduction Targeted Ther. (2024) 9:175. doi: 10.1038/s41392-024-01856-7
198. Adams S. Toll-like receptor agonists in cancer therapy. Immunotherapy. (2009) 1:949–64. doi: 10.2217/imt.09.70
199. Karapetyan L, Luke JJ, Davar D. Toll-like receptor 9 agonists in cancer. OncoTargets Ther. (2020) 13:10039–60. doi: 10.2147/OTT.S247050
200. Ribas A, Medina T, Kummar S, Amin A, Kalbasi A, Drabick JJ, et al. SD-101 in combination with pembrolizumab in advanced melanoma: results of a phase ib, multicenter study. Cancer Discovery. (2018) 8:1250–7. doi: 10.1158/2159-8290.CD-18-0280
201. Milhem M, Zakharia Y, Davar D, Buchbinder E, Medina T, Daud A, et al. 304 Intratumoral injection of CMP-001, a toll-like receptor 9 (TLR9) agonist, in combination with pembrolizumab reversed programmed death receptor 1 (PD-1) blockade resistance in advanced melanoma. BMJ Specialist Journals. (2020) 8. doi: 10.1136/jitc-2020-SITC2020.0304
202. Uher O, Caisova V, Padoukova L, Kvardova K, Masakova K, Lencova R, et al. and anti-CD40 immunotherapy in established murine pancreatic adenocarcinoma: understanding therapeutic potentials and limitations. Cancer Immunology Immunother. (2021) 70:3303–12. doi: 10.1007/s00262-021-02920-9
203. Medina R, Wang H, Caisová V, Cui J, Indig IH, Uher O, et al. Induction of immune response against metastatic tumors via vaccination of mannan-BAM, TLR ligands and anti-CD40 antibody (MBTA). Adv Ther (Weinh). (2020) 3. doi: 10.1002/adtp.202000044
204. Janku F, Zhang HH, Pezeshki A, Goel S, Murthy R, Wang-Gillam A, et al. Intratumoral injection of clostridium novyi-NT spores in patients with treatment-refractory advanced solid tumors. Clin Cancer Res. (2021) 27:96–106. doi: 10.1158/1078-0432.Ccr-20-2065
205. Fu Y, Tang R, Zhao X. Engineering cytokines for cancer immunotherapy: a systematic review. Front Immunol. (2023) 14:1218082. doi: 10.3389/fimmu.2023.1218082
206. Hotz C, Wagenaar TR, Gieseke F, Bangari DS, Callahan M, Cao H, et al. Local delivery of mRNA-encoded cytokines promotes antitumor immunity and tumor eradication across multiple preclinical tumor models. Sci Transl Med. (2021) 13:eabc7804. doi: 10.1126/scitranslmed.abc7804
207. Neo SY, Xu S, Chong J, Lam K-P, Wu J. Harnessing novel strategies and cell types to overcome immune tolerance during adoptive cell therapy in cancer. J Immunotherapy Cancer. (2023) 11:e006434. doi: 10.1136/jitc-2022-006434
208. Sterner RC, Sterner RM. CAR-T cell therapy: current limitations and potential strategies. Blood Cancer J. (2021) 11:69. doi: 10.1038/s41408-021-00459-7
209. Peng L, Sferruzza G, Yang L, Zhou L, Chen S. CAR-T and CAR-NK as cellular cancer immunotherapy for solid tumors. Cell Mol Immunol. (2024) 21:1089–108. doi: 10.1038/s41423-024-01207-0
210. Loureiro LR, Feldmann A, Bergmann R, Koristka S, Berndt N, Máthé D, et al. Extended half-life target module for sustainable UniCAR T-cell treatment of STn-expressing cancers. J Exp Clin Cancer Res. (2020) 39:77. doi: 10.1186/s13046-020-01572-4
211. Gu X, Zhang Y, Zhou W, Wang F, Yan F, Gao H, et al. Infusion and delivery strategies to maximize the efficacy of CAR-T cell immunotherapy for cancers. Exp Hematol Oncol. (2024) 13:70. doi: 10.1186/s40164-024-00542-2
212. Hirooka Y, Kawashima H, Ohno E, Ishikawa T, Kamigaki T, Goto S, et al. Comprehensive immunotherapy combined with intratumoral injection of zoledronate-pulsed dendritic cells, intravenous adoptive activated T lymphocyte and gemcitabine in unresectable locally advanced pancreatic carcinoma: a phase I/II trial. Oncotarget. (2018) 9:2838–47. doi: 10.18632/oncotarget.22974
213. Yu B, Kusmartsev S, Cheng F, Paolini M, Nefedova Y, Sotomayor E, et al. Effective combination of chemotherapy and dendritic cell administration for the treatment of advanced-stage experimental breast cancer. Clin Cancer Res. (2003) 9:285–94.
214. Tanaka F, Yamaguchi H, Ohta M, Mashino K, Sonoda H, Sadanaga N, et al. Intratumoral injection of dendritic cells after treatment of anticancer drugs induces tumor-specific antitumor effect in vivo. Int J Cancer. (2002) 101:265–9. doi: 10.1002/ijc.10597
215. Saji H, Song W, Furumoto K, Kato H, Engleman EG. Systemic antitumor effect of intratumoral injection of dendritic cells in combination with local photodynamic therapy. Clin Cancer Res. (2006) 12:2568–74. doi: 10.1158/1078-0432.Ccr-05-1986
216. Schwarze JK, Tijtgat J, Awada G, Cras L, Vasaturo A, Bagnall C, et al. Intratumoral administration of CD1c (BDCA-1)(+) and CD141 (BDCA-3)(+) myeloid dendritic cells in combination with talimogene laherparepvec in immune checkpoint blockade refractory advanced melanoma patients: a phase I clinical trial. J Immunother Cancer. (2022) 10:e005141. doi: 10.1136/jitc-2022-005141
217. Tijtgat J, Geeraerts X, Boisson A, Stevens L, Vounckx M, Dirven I, et al. Intratumoral administration of the immunologic adjuvant AS01(B) in combination with autologous CD1c (BDCA-1)(+)/CD141 (BDCA-3)(+) myeloid dendritic cells plus ipilimumab and intravenous nivolumab in patients with refractory advanced melanoma. J Immunother Cancer. (2024) 12:e008148. doi: 10.1136/jitc-2023-008148
218. Fonkoua LK, Wang P, Hallemeier C, Atwell T, Tran N, Mahipal A, et al. Preliminary results of a pilot study of intratumoral injection of autologous dendritic cells after high-dose conformal external beam radiotherapy in unreseltable primary liver cancers. BMJ Specialist Journals. (2022) 10. doi: 10.1136/jitc-2022-SITC2022.0689
219. Zhou X, Ni Y, Liang X, Lin Y, An B, He X, et al. Mechanisms of tumor resistance to immune checkpoint blockade and combination strategies to overcome resistance. Front Immunol. (2022) 13:915094. doi: 10.3389/fimmu.2022.915094
220. Johnson DB, Nebhan CA, Moslehi JJ, Balko JM. Immune-checkpoint inhibitors: long-term implications of toxicity. Nat Rev Clin Oncol. (2022) 19:254–67. doi: 10.1038/s41571-022-00600-w
221. Martins F, Sofiya L, Sykiotis GP, Lamine F, Maillard M, Fraga M, et al. Adverse effects of immune-checkpoint inhibitors: epidemiology, management and surveillance. Nat Rev Clin Oncol. (2019) 16:563–80. doi: 10.1038/s41571-019-0218-0
222. Jin Y, Huang Y, Ren H, Huang H, Lai C, Wang W, et al. Nano-enhanced immunotherapy: Targeting the immunosuppressive tumor microenvironment. Biomaterials. (2024) 122463. doi: 10.1016/j.biomaterials.2023.122463
223. Walsh RJ, Sundar R, Lim JS. Immune checkpoint inhibitor combinations—current and emerging strategies. Br J Cancer. (2023) 128:1415–7. doi: 10.1038/s41416-023-02181-6
224. De Lombaerde E, De Wever O, De Geest BG. Delivery routes matter: Safety and efficacy of intratumoral immunotherapy. Biochim Biophys Acta (BBA) - Rev Cancer. (2021) 1875:188526. doi: 10.1016/j.bbcan.2021.188526
225. Wolf Y, Anderson AC, Kuchroo VK. TIM3 comes of age as an inhibitory receptor. Nat Rev Immunol. (2020) 20:173–85. doi: 10.1038/s41577-019-0224-6
226. Liu HC, Viswanath DI, Pesaresi F, Xu Y, Zhang L, Di Trani N, et al. Potentiating antitumor efficacy through radiation and sustained intratumoral delivery of anti-CD40 and anti-PDL1. Int J Radiat Oncol Biol Phys. (2021) 110:492–506. doi: 10.1016/j.ijrobp.2020.07.2326
227. Yuan Y, Zhang J, Kessler J, Rand J, Modi B, Chaurasiya S, et al. Phase I study of intratumoral administration of CF33-HNIS-antiPDL1 in patients with metastatic triple negative breast cancer. J Clin Oncol. (2022) 40:e13070–e. doi: 10.1200/JCO.2022.40.16_suppl.e13070
228. Jhawar SR, Wang S-J, Thandoni A, Bommareddy PK, Newman JH, Giurini EF, et al. Combination oncolytic virus, radiation therapy, and immune checkpoint inhibitor treatment in anti-PD-1-refractory cancer. J immunotherapy Cancer. (2023) 11:e006780. doi: 10.1136/jitc-2023-006780
229. Banstola A, Poudel K, Emami F, Ku SK, Jeong JH, Kim JO, et al. Localized therapy using anti-PD-L1 anchored and NIR-responsive hollow gold nanoshell (HGNS) loaded with doxorubicin (DOX) for the treatment of locally advanced melanoma. Nanomedicine. (2021) 33:102349. doi: 10.1016/j.nano.2020.102349
230. Zaretsky JM, Garcia-Diaz A, Shin DS, Escuin-Ordinas H, Hugo W, Hu-Lieskovan S, et al. Mutations associated with acquired resistance to PD-1 blockade in melanoma. New Engl J Med. (2016) 375:819–29. doi: 10.1056/NEJMoa1604958
231. Karasarides M, Cogdill AP, Robbins PB, Bowden M, Burton EM, Butterfield LH, et al. Hallmarks of resistance to immune-checkpoint inhibitors. Cancer Immunol Res. (2022) 10:372–83. doi: 10.1158/2326-6066.CIR-20-0586
232. Weber R, Fleming V, Hu X, Nagibin V, Groth C, Altevogt P, et al. Myeloid-derived suppressor cells hinder the anti-cancer activity of immune checkpoint inhibitors. Front Immunol. (2018) 9:1310. doi: 10.3389/fimmu.2018.01310
233. Márquez-Rodas I, Longo F, Rodriguez-Ruiz ME, Calles A, Ponce S, Jove M, et al. Intratumoral nanoplexed poly I:C BO-112 in combination with systemic anti-PD-1 for patients with anti-PD-1-refractory tumors. . Sci Transl Med. (2020) 12:eabb0391. doi: 10.1126/scitranslmed.abb0391
234. Wang S, Campos J, Gallotta M, Gong M, Crain C, Naik E, et al. Intratumoral injection of a CpG oligonucleotide reverts resistance to PD-1 blockade by expanding multifunctional CD8+ T cells. Proc Natl Acad Sci. (2016) 113:E7240–E9. doi: 10.1073/pnas.1608555113
235. Gao Y, Bi D, Xie R, Li M, Guo J, Liu H, et al. Fusobacterium nucleatum enhances the efficacy of PD-L1 blockade in colorectal cancer. Signal Transduct Target Ther. (2021) 6:398. doi: 10.1038/s41392-021-00795-x
236. Chen J-S, Hsieh Y-C, Chou C-H, Wu Y-H, Yang M-H, Chu S-H, et al. Chidamide plus tyrosine kinase inhibitor remodel the tumor immune microenvironment and reduce tumor progression when combined with immune checkpoint inhibitor in naïve and anti-PD-1 resistant CT26-bearing mice. Int J Mol Sci. (2022) 23:10677. doi: 10.3390/ijms231810677
237. Nayyar N, de Sauvage MA, Chuprin J, Sullivan EM, Singh M, Torrini C, et al. CDK4/6 inhibition sensitizes intracranial tumors to PD-1 blockade in preclinical models of brain metastasis. Clin Cancer Res. (2024) 30:420–35. doi: 10.1158/1078-0432.CCR-23-0433
238. Jian C-Z, Lin L, Hsu C-L, Chen Y-H, Hsu C, Tan C-T, et al. A potential novel cancer immunotherapy: agonistic anti-CD40 antibodies. Drug Discovery Today. (2024) 103893. doi: 10.1016/j.drudis.2024.103893
239. Van Meir H, Nout R, Welters M, Loof N, De Kam M, Van Ham J, et al. Impact of (chemo) radiotherapy on immune cell composition and function in cervical cancer patients. Oncoimmunology. (2017) 6:e1267095. doi: 10.1080/2162402X.2016.1267095
240. McMahon RA, D'Souza C, Neeson PJ, Siva S. Innate immunity: Looking beyond T-cells in radiation and immunotherapy combinations. Neoplasia. (2023) 46:100940. doi: 10.1016/j.neo.2023.100940
241. Mikhail AS, Morhard R, Mauda-Havakuk M, Kassin M, Arrichiello A, Wood BJ. Hydrogel drug delivery systems for minimally invasive local immunotherapy of cancer. Adv Drug Delivery Rev. (2023) 202:115083. doi: 10.1016/j.addr.2023.115083
242. Munoz NM, Williams M, Dixon K, Dupuis C, McWatters A, Avritscher R, et al. Influence of injection technique, drug formulation and tumor microenvironment on intratumoral immunotherapy delivery and efficacy. J Immunother Cancer. (2021) 9. doi: 10.1136/jitc-2020-001800
243. Sheth RA, Murthy R, Hong DS, Patel S, Overman MJ, Diab A, et al. Assessment of image-guided intratumoral delivery of immunotherapeutics in patients with cancer. JAMA Netw Open. (2020) 3:e207911. doi: 10.1001/jamanetworkopen.2020.7911
244. Lau T-S, Chan LK-Y, Man GC-W, Kwong J. Paclitaxel induces immunogenic cell death in ovarian cancer via TLR4-independent and dependent pathways. Cancer Res. (2019) 79:1232. doi: 10.1158/2326-6066.CIR-19-0616
245. Szabados B, van Dijk N, Tang YZ, van der Heijden MS, Wimalasingham A, de Liano AG, et al. Response rate to chemotherapy after immune checkpoint inhibition in metastatic urothelial cancer. Eur urology. (2018) 73:149–52. doi: 10.1016/j.eururo.2017.08.022
246. Yao W, Zhao X, Gong Y, Zhang M, Zhang L, Wu Q, et al. Impact of the combined timing of PD-1/PD-L1 inhibitors and chemotherapy on the outcomes in patients with refractory lung cancer. ESMO Open. (2021) 6:100094. doi: 10.1016/j.esmoop.2021.100094
247. Cash E, Sephton S, Woolley C, Elbehi AM, Ri A, Ekine-Afolabi B, et al. The role of the circadian clock in cancer hallmark acquisition and immune-based cancer therapeutics. J Exp Clin Cancer Res. (2021) 40:1–14. doi: 10.1186/s13046-021-01919-5
248. Wang C, Barnoud C, Cenerenti M, Sun M, Caffa I, Kizil B, et al. Dendritic cells direct circadian anti-tumour immune responses. Nature. (2023) 614:136–43. doi: 10.1038/s41586-022-05605-0
249. Karaboué A, Innominato PF, Wreglesworth NI, Duchemann B, Adam R, Lévi FA. Why does circadian timing of administration matter for immune checkpoint inhibitors’ efficacy? Br J Cancer. (2024) 131:1–14. doi: 10.1038/s41416-024-02704-9
250. Landré T, Karaboué A, Buchwald Z, Innominato P, Qian D, Assié J, et al. Effect of immunotherapy-infusion time of day on survival of patients with advanced cancers: a study-level meta-analysis. ESMO Open. (2024) 9:102220. doi: 10.1016/j.esmoop.2023.102220
251. Wang AX, Ong XJ, D’Souza C, Neeson PJ, Zhu JJ. Combining chemotherapy with CAR-T cell therapy in treating solid tumors. Front Immunol. (2023) 14:1140541. doi: 10.3389/fimmu.2023.1140541
252. Van der Veldt AA, Lubberink M, Bahce I, Walraven M, de Boer MP, Greuter HN, et al. Rapid decrease in delivery of chemotherapy to tumors after anti-VEGF therapy: implications for scheduling of anti-angiogenic drugs. Cancer Cell. (2012) 21:82–91. doi: 10.1016/j.ccr.2011.11.023
253. Beaver JA, Kluetz PG, Pazdur R. Metastasis-free survival—a new end point in prostate cancer trials. New Engl J Med. (2018) 378:2458–60. doi: 10.1056/NEJMp1805966
254. Booth CM, Eisenhauer EA, Gyawali B, Tannock IF. Progression-free survival should not be used as a primary end point for registration of anticancer drugs. J Clin Oncol. (2023) 41:4968–72. doi: 10.1200/JCO.23.01423
255. Abou-Alfa GK, Galle PR, Chao Y, Erinjeri J, Heo J, Borad MJ, et al. PHOCUS: A phase 3, randomized, open-label study of sequential treatment with pexa-vec (JX-594) and sorafenib in patients with advanced hepatocellular carcinoma. Liver Cancer. (2024) 13:248–64. doi: 10.1159/000533650
256. Li X, Lovell JF, Yoon J, Chen X. Clinical development and potential of photothermal and photodynamic therapies for cancer. Nat Rev Clin Oncol. (2020) 17:657–74. doi: 10.1038/s41571-020-0410-2
257. Kejík Z, Hajduch J, Abramenko N, Vellieux F, Veselá K, Fialová JL, et al. Cyanine dyes in the mitochondria-targeting photodynamic and photothermal therapy. Commun Chem. (2024) 7:180. doi: 10.1038/s42004-024-01256-6
258. Alzeibak R, Mishchenko TA, Shilyagina NY, Balalaeva IV, Vedunova MV, Krysko DV. Targeting immunogenic cancer cell death by photodynamic therapy: past, present and future. J Immunother Cancer. (2021) 9. doi: 10.1136/jitc-2020-001926
259. Castano AP, Mroz P, Hamblin MR. Photodynamic therapy and anti-tumour immunity. Nat Rev Cancer. (2006) 6:535–45. doi: 10.1038/nrc1894
260. Zhou H, Tang D, Yu Y, Zhang L, Wang B, Karges J, et al. Theranostic imaging and multimodal photodynamic therapy and immunotherapy using the mTOR signaling pathway. Nat Commun. (2023) 14:5350. doi: 10.1038/s41467-023-40826-5
261. Liang X, Chen M, Bhattarai P, Hameed S, Dai Z. Perfluorocarbon@Porphyrin nanoparticles for tumor hypoxia relief to enhance photodynamic therapy against liver metastasis of colon cancer. ACS Nano. (2020) 14:13569–83. doi: 10.1021/acsnano.0c05617
262. Lima-Sousa R, Melo BL, Alves CG, Moreira AF, Mendonça AG, Correia IJ, et al. Combining photothermal-photodynamic therapy mediated by nanomaterials with immune checkpoint blockade for metastatic cancer treatment and creation of immune memory. Advanced Funct Materials. (2021) 31:2010777. doi: 10.1002/adfm.202010777
263. Institute NC. Neoadjuvant therapy . Available online at: https://www.cancer.gov/publications/dictionaries/cancer-terms/def/neoadjuvant-therapy (Accessed July 25, 2024).
264. Topalian SL, Taube JM, Pardoll DM. Neoadjuvant checkpoint blockade for cancer immunotherapy. Science. (2020) 367:eaax0182. doi: 10.1126/science.aax0182
265. Patel SP, Othus M, Chen Y, Wright GP Jr., Yost KJ, Hyngstrom JR, et al. Neoadjuvant-adjuvant or adjuvant-only pembrolizumab in advanced melanoma. N Engl J Med. (2023) 388:813–23. doi: 10.1056/NEJMoa2211437
266. Yotsukura M, Nakagawa K, Suzuki K, Takamochi K, Ito H, Okami J, et al. Recent advances and future perspectives in adjuvant and neoadjuvant immunotherapies for lung cancer. Japanese J Clin Oncol. (2021) 51:28–36. doi: 10.1093/jjco/hyaa187
267. Fountzila E, Ignatiadis M. Neoadjuvant immunotherapy in breast cancer: a paradigm shift? Ecancermedicalscience. (2020) 14. doi: 10.3332/ecancer.2020.1147
268. Chalabi M, Verschoor YL, Tan PB, Balduzzi S, Van Lent AU, Grootscholten C, et al. Neoadjuvant immunotherapy in locally advanced mismatch repair–deficient colon cancer. New Engl J Med. (2024) 390:1949–58. doi: 10.1056/NEJMoa2400634
269. Hong WX, Sagiv-Barfi I, Czerwinski DK, Sallets A, Levy R. Neoadjuvant intratumoral immunotherapy with TLR9 activation and anti-OX40 antibody eradicates metastatic cancer. Cancer Res. (2022) 82:1396–408. doi: 10.1158/0008-5472.Can-21-1382
270. Solomon J, Raskova M, Rosel D, Brabek J, Gil-Henn H. Are we ready for migrastatics? Cells. (2021) 10:1845. doi: 10.3390/cells10081845
271. Ramesh V, Brabletz T, Ceppi P. Targeting EMT in cancer with repurposed metabolic inhibitors. Trends Cancer. (2020) 6:942–50. doi: 10.1016/j.trecan.2020.06.005
272. Huang Y, Hong W, Wei X. The molecular mechanisms and therapeutic strategies of EMT in tumor progression and metastasis. J Hematol Oncol. (2022) 15:129. doi: 10.1186/s13045-022-01347-8
273. Wang H, Guo S, Kim S-J, Shao F, Ho JWK, Wong KU, et al. Cisplatin prevents breast cancer metastasis through blocking early EMT and retards cancer growth together with paclitaxel. Theranostics. (2021) 11:2442. doi: 10.7150/thno.46460
274. Caino MC, Ghosh JC, Chae YC, Vaira V, Rivadeneira DB, Faversani A, et al. PI3K therapy reprograms mitochondrial trafficking to fuel tumor cell invasion. Proc Natl Acad Sci. (2015) 112:8638–43. doi: 10.1073/pnas.1500722112
275. Zhang H, Qin C, An C, Zheng X, Wen S, Chen W, et al. Application of the CRISPR/Cas9-based gene editing technique in basic research, diagnosis, and therapy of cancer. Mol cancer. (2021) 20:1–22. doi: 10.1186/s12943-021-01431-6
276. Wang S-W, Gao C, Zheng Y-M, Yi L, Lu J-C, Huang X-Y, et al. Current applications and future perspective of CRISPR/Cas9 gene editing in cancer. Mol cancer. (2022) 21:57. doi: 10.1186/s12943-022-01518-8
277. Torikai H, Cooper LJ. Translational implications for off-the-shelf immune cells expressing chimeric antigen receptors. Mol Ther. (2016) 24:1178–86. doi: 10.1038/mt.2016.106
278. Su S, Hu B, Shao J, Shen B, Du J, Du Y, et al. CRISPR-Cas9 mediated efficient PD-1 disruption on human primary T cells from cancer patients. Sci Rep. (2016) 6:20070. doi: 10.1038/srep20070
279. Zhang Y, Zhang X, Cheng C, Mu W, Liu X, Li N, et al. CRISPR-Cas9 mediated LAG-3 disruption in CAR-T cells. Front Med. (2017) 11:554–62. doi: 10.1007/s11684-017-0543-6
280. Jang G, Kweon J, Kim Y. CRISPR prime editing for unconstrained correction of oncogenic KRAS variants. Commun Biol. (2023) 6:681. doi: 10.1038/s42003-023-05052-1
281. Depil S, Duchateau P, Grupp S, Mufti G, Poirot L. [amp]]lsquo;Off-the-shelf’allogeneic CAR T cells: development and challenges. Nat Rev Drug discovery. (2020) 19:185–99. doi: 10.1038/s41573-019-0051-2
282. Hossen S, Hossain MK, Basher M, Mia M, Rahman M, Uddin MJ. Smart nanocarrier-based drug delivery systems for cancer therapy and toxicity studies: A review. J advanced Res. (2019) 15:1–18. doi: 10.1016/j.jare.2018.06.005
283. Chen D, Liu X, Lu X, Tian J. Nanoparticle drug delivery systems for synergistic delivery of tumor therapy. Front Pharmacol. (2023) 14:1111991. doi: 10.3389/fphar.2023.1111991
284. Xu X, Ho W, Zhang X, Bertrand N, Farokhzad O. Cancer nanomedicine: from targeted delivery to combination therapy. Trends Mol Med. (2015) 21:223–32. doi: 10.1016/j.molmed.2015.01.001
285. Liu X, Cheng Y, Mu Y, Zhang Z, Tian D, Liu Y, et al. Diverse drug delivery systems for the enhancement of cancer immunotherapy: an overview. Front Immunol. (2024) 15:1328145. doi: 10.3389/fimmu.2024.1328145
286. Hoppenz P, Els-Heindl S, Beck-Sickinger AG. Peptide-drug conjugates and their targets in advanced cancer therapies. Front Chem. (2020) 8:571. doi: 10.3389/fchem.2020.00571
287. Herrmann IK, Wood MJA, Fuhrmann G. Extracellular vesicles as a next-generation drug delivery platform. Nat nanotechnology. (2021) 16:748–59. doi: 10.1038/s41565-021-00931-2
288. Zhao L, Zhao J, Zhong K, Tong A, Jia D. Targeted protein degradation: mechanisms, strategies and application. Signal transduction targeted Ther. (2022) 7:113. doi: 10.1038/s41392-022-00966-4
289. Zhao J, Ruan J, Lv G, Shan Q, Fan Z, Wang H, et al. Cell membrane-based biomimetic nanosystems for advanced drug delivery in cancer therapy: A comprehensive review. Colloids Surfaces B: Biointerfaces. (2022) 215:112503. doi: 10.1016/j.colsurfb.2022.112503
290. Tan S, Wu T, Zhang D, Zhang Z. Cell or cell membrane-based drug delivery systems. Theranostics. (2015) 5:863–81. doi: 10.7150/thno.11852
291. Schroeder A, Heller DA, Winslow MM, Dahlman JE, Pratt GW, Langer R, et al. Treating metastatic cancer with nanotechnology. Nat Rev Cancer. (2012) 12:39–50. doi: 10.1038/nrc3180
292. Zhu W, Wei T, Xu Y, Jin Q, Chao Y, Lu J, et al. Non-invasive transdermal delivery of biomacromolecules with fluorocarbon-modified chitosan for melanoma immunotherapy and viral vaccines. Nat Commun. (2024) 15:820. doi: 10.1038/s41467-024-45158-6
293. Chen Q, Wang C, Zhang X, Chen G, Hu Q, Li H, et al. In situ sprayed bioresponsive immunotherapeutic gel for post-surgical cancer treatment. Nat nanotechnology. (2019) 14:89–97. doi: 10.1038/s41565-018-0319-4
294. Yun WS, Kim J, Lim D-K, Kim D-H, Jeon SI, Kim K. Recent studies and progress in the intratumoral administration of nano-sized drug delivery systems. Nanomaterials. (2023) 13:2225. doi: 10.3390/nano13152225
295. Wang C, Sun W, Wright G, Wang A, Gu Z. Inflammation-triggered cancer immunotherapy by programmed delivery of CpG and anti-PD1 antibody. Advanced materials (Deerfield Beach Fla). (2016) 28:8912. doi: 10.1002/adma.201506312
296. Qian G, Wang X, Li X, Ito A, Sogo Y, Ye J. An immuno-potentiating vehicle made of mesoporous silica-zinc oxide micro-rosettes with enhanced doxorubicin loading for combined chemoimmunotherapy. Chem Commun. (2019) 55:961–4. doi: 10.1039/C8CC09044K
297. Bahmani B, Gong H, Luk BT, Haushalter KJ, DeTeresa E, Previti M, et al. Intratumoral immunotherapy using platelet-cloaked nanoparticles enhances antitumor immunity in solid tumors. Nat Commun. (2021) 12:1999. doi: 10.1038/s41467-021-22311-z
Keywords: cancer, immunotherapy, intratumoral, combination therapy, advanced and metastatic cancer
Citation: Skalickova M, Hadrava Vanova K, Uher O, Leischner Fialova J, Petrlakova K, Masarik M, Kejík Z, Martasek P, Pacak K and Jakubek M (2025) Injecting hope: the potential of intratumoral immunotherapy for locally advanced and metastatic cancer. Front. Immunol. 15:1479483. doi: 10.3389/fimmu.2024.1479483
Received: 12 August 2024; Accepted: 17 December 2024;
Published: 09 January 2025.
Edited by:
Elena Voronov, Ben-Gurion University of the Negev, IsraelReviewed by:
Namrata Gautam, Moffitt Cancer Center, United StatesTommaso Virgilio, Institute for Research in Biomedicine (IRB), Switzerland
Copyright © 2025 Skalickova, Hadrava Vanova, Uher, Leischner Fialova, Petrlakova, Masarik, Kejík, Martasek, Pacak and Jakubek. This is an open-access article distributed under the terms of the Creative Commons Attribution License (CC BY). The use, distribution or reproduction in other forums is permitted, provided the original author(s) and the copyright owner(s) are credited and that the original publication in this journal is cited, in accordance with accepted academic practice. No use, distribution or reproduction is permitted which does not comply with these terms.
*Correspondence: Milan Jakubek, TWlsYW4uSmFrdWJla0BsZjEuY3VuaS5jeg==