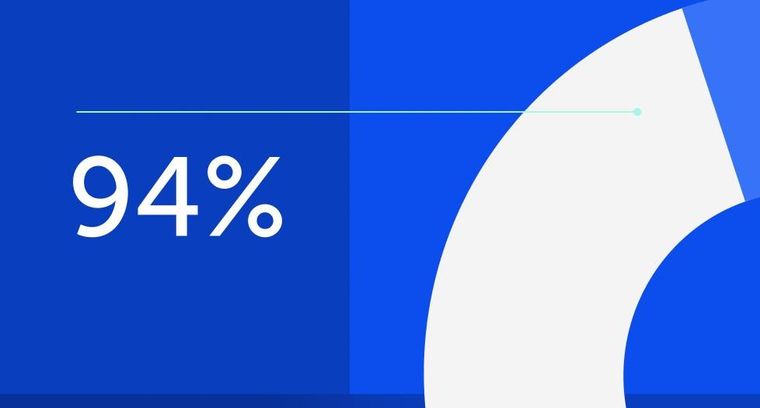
94% of researchers rate our articles as excellent or good
Learn more about the work of our research integrity team to safeguard the quality of each article we publish.
Find out more
REVIEW article
Front. Immunol., 17 January 2025
Sec. Molecular Innate Immunity
Volume 15 - 2024 | https://doi.org/10.3389/fimmu.2024.1479330
Macrophages are innate immune cells present in all tissues and play an important role in almost all aspects of the biology of living organisms. Extracellular vesicles (EVs) are released by cells and transport their contents (micro RNAs, mRNA, proteins, and long noncoding RNAs) to nearby or distant cells for cell-to-cell communication. Numerous studies have shown that macrophage-derived extracellular vesicles (M-EVs) and their contents play an important role in a variety of diseases and show great potential as biomarkers, therapeutics, and drug delivery vehicles for diseases. This article reviews the biological functions and mechanisms of M-EVs and their contents in chronic non-communicable diseases such as cardiovascular diseases, metabolic diseases, cancer, inflammatory diseases and bone-related diseases. In addition, the potential application of M-EVs as drug delivery systems for various diseases have been summarized.
Macrophages, including monocytes in the circulatory system and tissue-resident macrophages, are the most plastic immune cells in the hematopoietic system and are ubiquitous in all tissues. They play a crucial role in the entire spectrum of an organism’s biology, swiftly responding to various infections while also contributing to the development, homeostasis, initiation, maintenance, resolution of inflammation, tissue damage repair, and immunity (1–3). Tissue-resident macrophages are categorized based on their location and function, including microglia in the central nervous system, osteoclasts in bones, alveolar macrophages in the lungs and Kupffer cells in the liver (4, 5). Macrophages form different subtypes, such as M1 and M2, in different tissues depending on the environmental stimuli (6, 7), which represent the extremes of the polarization spectrum. Currently, an increasing number of studies have illuminated the existence of numerous intermediate polarization states within macrophages. M1 phenotypic macrophages are usually induced by interferon (IFN)-γ, lipopolysaccharide (LPS), granulocyte macrophage colony-stimulating factor, or tumor necrosis factor (TNF) alone or in combination in vitro. M1 polarization of macrophages alters the expression of a variety of cell surface markers such as cluster of differentiation (CD)80, CD86, CD36, inducible nitric oxide synthase (iNOS), and intercellular adhesion molecule 1. M1 phenotypic macrophages produce and secrete high levels of pro-inflammatory cytokines, which play an important role in the early stages of inflammation (6). In contrast, M2 phenotypic macrophages have anti-inflammatory effects. Depending on the activation stimulus, M2 macrophages can be further subdivided into different subtypes. M2 macrophages express a variety of cell surface markers, such as the mannose receptor (CD206), arginase-1, IL1R2, TLR-1, and TLR-8. Moreover, M2 phenotypic macrophages produce a variety of anti-inflammatory and pro-inflammatory cytokines (8). Functionally, M1 macrophages are able to eliminate infected cells (9), induce inflammatory responses, mediate tissue damage (10), impair tissue regeneration, and have antitumor activity (11, 12). M2 macrophages have a strong phagocytic ability, clear apoptotic cells, participate in the Th2 response and parasite clearance (13, 14), inhibit inflammation, and promote tissue repair, wound healing (10, 15, 16), angiogenesis, immunomodulation, and tumor formation and progression (17, 18). Macrophages play an important role in regulating the body’s diverse functions. Macrophage polarization is closely related to disease development, and polarization disorders may cause diseases. Macrophages with different phenotypes or responses to stimuli have been utilized for research purposes. However, macrophage-derived extracellular vesicles (EV) of different phenotypes exhibit behaviors similar to those of the parental cells, garnering widespread attention.
EVs are membrane-bound particles important for intercellular communication and with potential applications in diseases and therapeutics (19). Macrophage-derived extracellular vesicles (M-EVs) have garnered attention due to their similarities to macrophages. Frequently utilized primary macrophages include murine bone marrow-derived macrophages (BMDM) (20), peritoneal-derived macrophages (PCM) (21, 22), adipose tissue-derived macrophages, or monocytes isolated from human peripheral blood (23). Passage cell lines commonly used are RAW264.7 (24), mouse mononuclear macrophages (25), and the THP-1 cell line (26, 27). According to the latest guidelines of the International Society of Extracellular Vesicles and current research, M-EVs are classified as exosomes, microvesicles, and apoptotic bodies (28, 29). Exosomes originate from endosomes via endocytosis and are released outside the cell with a 50–150 nm diameter. Microvesicles (MV) originate from the cytoplasmic membrane with a diameter of 100–1000 nm (30). Apoptotic bodies, with a diameter of about 100–5000 nm, are formed during programmed cell death (31–33).
M-EVs are characterized by their morphology, size, and marker proteins. Commonly used techniques to characterize the morphology of EVs include scanning electron microscopy, transmission electron microscopy, cryo-electron microscopy, atomic force microscopy, and dynamic light scattering (34). Nanoparticle tracking analysis and tunable resistance pulse detection can be used to measure the concentration and particle size distribution of M-EVs (34, 35). Marker protein identification is critical and is usually performed using western blotting and enzyme-linked immunosorbent assay for routine analysis. Recommended positive marker proteins for characterizing M-EVs include transmembrane (CD9, CD63, and CD81) and cytosolic proteins (tumor susceptibility gene 101 and programmed cell death 6-interacting protein). The International Society of Extracellular Vesicles has identified proteins such as heat shock protein, actin, tubulin, and glyceraldehyde 3-phosphate dehydrogenase that do not meet EV-specific components (28). Notably, the identification of M-EVs should be analyzed in combination with the above aspects rather than relying on a single aspect.
The isolation and purification of EVs is the first step in subsequent studies. EVs separation can be achieved using methods such as differential ultracentrifugation, density gradient centrifugation, immunoaffinity, coprecipitation (36), size exclusion chromatography (37), asymmetric flow field fractionation (38), and a newly expanded method of microfluidic filtration (35). Currently, differential ultracentrifugation is the most commonly used and considered the gold standard for EV isolation (39). Each method has its own advantages and limitations, which are detailed in related reviews (35). Notably, the pretreatment method differs for different samples, and it is necessary to select an appropriate EV separation method according to the experimental purpose and requirements.
EVs transport various cargoes (RNA, proteins, lipids, and DNA) to nearby or distant recipient cells, influencing their function and intercellular communication. M-EVs contain numerous RNA, including microRNAs (miRNA), mRNA, circular RNAs (circRNA), long non-coding RNA (lncRNA), small interfering RNAs, small nucleolar RNAs, and transfer RNA (40). The mechanisms that control the specific loading of RNA species into exosomes rather complicated. Current research reports that miRNAs have sequence motifs, which control their localization into exosomes. The protein heterogeneous nuclear ribonucleoprotein A2B1 (hnRNPA2B1) specifically binds exosomal miRNAs through the recognition of these motifs and controls their loading into exosomes (41). The loading of miRNAs into exosomes can be modulated by mutagenesis of the identified motifs or changes in hnRNPA2B1 expression levels. Moreover, it has been reported that the YBX1 protein is intricately involved in the selective loading of specific microRNAs into exosomes (42).
Among EV inclusions, miRNAs are the most studied RNA in terms of function and biogenesis. They are a class of 17–24 nt short non-coding RNAs that bind to the 3 í-untranslated region of the target mRNA to regulate physiological and biochemical processes (43, 44). The levels of miRNA expression in EVs vary under various physiological and pathological conditions. For example, the expression of miR-142-3p in plasma exosomes of patients with idiopathic pulmonary fibrosis is significantly higher than in normal individuals (45). The miRNAs in M-EVs play a role in the progression of many diseases and have the potential to serve as diagnostic markers (46, 47). The mRNA delivered by M-EVs can be translated into recipient cells, serving as a protein source for regulation in recipient cells (48). As key competitive endogenous RNAs, lncRNAs act as sponges for miRNAs to negatively regulate miRNAs and thus exert their function (49, 50). Studies have confirmed that lncRNAs in M-EVs exert physiological effects in various diseases such as tumors, inflammatory diseases, and myocardial infarction (51–53). In M-EVs, circRNA also acts as an endogenous RNA that competitively binds to miRNA to affect the expression level of the target mRNA, thereby exerting physiological effects (54–56). M-EVs also influence disease progression by delivering functional proteins (57). Many studies have reported how EVs interact with recipient cells and deliver functional cargo (e.g., RNA, protein) via multiple EV uptake pathways including direct membrane fusion, clathrin-mediated endocytosis, macropinocytosis, caveolin-mediated uptake, phagocytosis, lipid raft-mediated internalization, and direct transfer via tunneling nanotubes (58, 59).
Atherosclerosis (AS) and myocardial infarction (MI) are prevalent cardiovascular conditions. AS is a chronic inflammatory disease of the large and medium-sized arteries with many known risk factors, including hypercholesterolemia, hypertension, diabetes, and smoking (60). AS is a chronic inflammatory process caused by the interaction of atherogenic lipoproteins with vascular wall cells (including endothelial cells, smooth muscle cells, and monocytes/macrophages) (61). Endothelial pro-inflammatory activation and endothelial cell dysfunction are important factors in the pathophysiology of AS (62). Recent studies have shown that M-EVs and their contents are involved in the pathogenesis of AS through a variety of pathways such as the regulation of glycolytic activity, endothelial vascular injury, inflammation, oxidative stress, hematopoiesis, neutrophil extracellular traps (NET), and macrophage infiltration (Table 1).
Oxidative low-density lipoprotein (oxLDL)-stimulated macrophage-derived extracellular vesicles (oxLDL-M-EVs) accelerate AS progression by mediating endothelial cell injury, macrophage accumulation, oxidative stress, and NETs (63–67). Liu et al. employed oxLDL to induce macrophage foam cells and release exosomes with high levels of miR-4532. This miRNA is delivered to endothelial cells via exosomes, targeting specific protein 1 to activate NF-κB p65 signaling, promoting vascular cell adhesion molecule-1 expression, and inducing endothelial dysfunction (63). Huang et al. showed that oxLDL-M-EVs attenuated endothelial cell growth and tube formation (64). The expression of miR-199a-5p decreases in oxLDL-M-EVs, and its downregulation promotes endothelial cell pyroptosis by enhancing SWI/SNF related, matrix associated, actin dependent regulator of chromatin, subfamily a, member 4 expression, further accelerating AS progression (65). The oxLDL-M-EVs reduce macrophage migration and promote the capture of macrophages in blood vessel walls; miR-146a may inhibit macrophage migration by targeting IGF2BP1 and human antigen R (66). Another study revealed significant upregulation of miRNA-146a in the serum-derived exosomes of patients with AS and oxLDL-M-EVs. MiR-146a could also induce oxidative stress to promote the formation of NETs by inhibiting superoxide dismutase 2 expression, further exacerbating the progression of AS (67). This suggests that the same miRNA can perform the same functions in multiple ways.
The inhibition of endothelial cell migration and proliferation associated with AS development. M-EVs inhibit endothelial cell migration by mediating the transport of integrin β1, thereby inhibiting the collagen-induced mitogen-activated protein kinase/extracellular signal-regulated kinase signaling pathway (68). MiR-185-3p and miR-503-5p are significantly upregulated in M-EVs and also inhibit endothelial cell proliferation and migration (69, 70). M2-EVs promote endothelial cell proliferation, and miR-221-3p-rich M2-EVs inhibit apoptosis and inflammation of HUVECs, and promote their proliferation; the mechanism may be that miR-221-3p targets inhibition of Grb10 expression, but further experiments are needed to confirm this (71). Another study showed that M-EVs promoted endothelial cell proliferation, migration, and tube formation in vitro (72).
Oxidative stress is closely related to inflammation, and M-EVs promote AS development by inducing oxidative stress and inflammation (67, 69, 73, 74). Macrophage-derived exosomes (M-Exo) miR-16-5p promotes the inflammatory response and oxidative stress in ApoE-/- mice, exacerbates pathological changes, and increases apoptosis by targeting Smad7 (73). Similarly, M1-EVs miR-185-3p accelerates AS progression by downregulating Smad7, aggravating pathology and endothelial cell adhesion in ApoE-/- mice, and enhancing lipid levels, oxidative stress, and inflammation (69). Yaker et al. reported that LPS stimulation of macrophage-derived extracellular vesicles (LPS-M1-EVs) could induce inflammation and oxidative stress in vascular smooth muscle cells (VSMCs), thus exacerbating vascular calcification (VC) (74). VC is a vascular complication commonly observed in patients with diseases such as AS and chronic kidney disease. High phosphate stimulation of M-EVs promotes VC in VSMCs (75). New et al. showed that macrophage-derived stromal vesicles enriched with S100A9 and annexin V contribute to atherosclerotic intimal microcalcification (76).
Atherosclerotic plaque formation is associated with VSMC migration and proliferation. Plasma EVs from patients with AS promote adhesion and migration of VSMCs. Macrophage foam cells release more EVs than normal macrophages, transporting integrins to VSMCs and activating the extracellular signal-regulated kinase (ERK) and Akt pathways, thus enhancing VSMC migration and adhesion (77). This indicates that macrophages in atherosclerotic lesions influence VSMCs via EVs, prompting their migration to and adhesion within the intima. Moreover, exosomes from nicotine-treated macrophages increased VSMC migration and proliferation by delivering miR-21-3p and targeting phosphatase and tensin homolog (PTEN), thereby accelerating AS (78).
Bouchareychas et al. investigated the effects of high glucose (HG)-BMDM-EVs on AS and found that they induced macrophage and myeloid progenitor cell proliferation, and regulated energy metabolism in vitro by increasing glycolytic activity. Infusion of HG-BMDM-EVs into ApoE-/- mice was found to enhance AS by increasing hematopoiesis, bone marrow cell supply, lesion macrophage content, and apoptotic cell number (79). IL-4-polarized bone marrow macrophage-derived exosomes (IL-4-BMDM-Exo) play a protective role by containing anti-inflammatory miRNAs (miRNA-99a/146b/378a), which inhibit inflammation by targeting NF-κB and TNF-α signaling. Infusion of IL-4-BMDM-Exos into ApoE-/- mice reduced transitional hematopoiesis in the bone marrow, thereby reducing the number of circulating myeloid cells and macrophages in aortic root lesions, resulting in reduced area of necrotic lesions in AS (80). This suggests that M-EVs are a promising treatment modality for AS.
Several studies have shown that M-EVs play an important role in mediating the immunomodulatory processes of MI and post-MI remodeling. In the MI microenvironment, mesenchymal cells effectively treat acute myocardial infarction (AMI) by influencing cell differentiation, migration, angiogenesis, and repair. Studies have shown that M1-Exo induced by hypoxia and serum deprivation (H/SD-M1-Exo) induce apoptosis in bone marrow mesenchymal stem cells (BMSCs) by delivering miR-222 (81). This affects the efficacy of BMSCs in AMI treatment. In a mouse model of MI, M1-BMDM-EVs increased CDC42 expression by carrying the lncRNA MALAT1 and competitively binding to miR-25-3p to activate the MEK/ERK pathway, thereby inhibiting angiogenesis and myocardial regeneration after MI (51). In the same model, M1-Exo miR-155 was transferred into endothelial cells (ECs), and miR-155 reduced the angiogenesis of ECs and exacerbated myocardial injury by simultaneously targeting the Rac family of small GTPases 1 (RAC1), p21 (RAC1)-activated kinase 2 (PAK2), sirtuin1 (Sirt1), and protein kinase AMP-activated catalytic subunit α2 (82). M1-Exo miR-155 can be transported not only to Ecs but also to cardiac fibroblasts, inhibiting fibroblast proliferation and promoting fibroblast inflammation by downregulating Sos1 expression, thereby impairing cardiac repair after MI (83). Interestingly, miR-155, an exosome derived from the adipose tissue macrophages in obese mice, was previously described to promotes obesity-induced insulin resistance. In distinct cell types, a single miRNA may bind to varying target genes, leading to contrasting biological effects. This phenomenon underscores the complexity and diversity of miRNA functions, which depend not only on miRNA expression levels but also on cell-specific regulation and interactions. Environmental conditions such as intracellular signaling pathway activity and epigenetic modification may also affect the mode of action of miRNAs (84). It may lead to difficulties in further clinical research. Studies have demonstrated that M2-EVs contribute to ventricular remodeling following MI. In a rat model of myocardial ischemia/reperfusion injury (MI/R), M2-Exos miR-148a are transported to cardiomyocytes and attenuates MI/R by downregulating thioredoxin-interacting protein (TXNIP) and inhibiting the TLR4/NF-κB/NLRP3 inflammasome signaling pathway (85). Another study has suggested that M2-EVs exert protective effects against ischemia-reperfusion cardiac injury. The main mechanism is that M2-EVs polarize heart-resident CC chemokine receptor 2 (CCR2) macrophages to the M2 phenotype, inhibit excessive glucose uptake and mtROS production by CCR2 macrophages, promote myocardial neovascularization, and facilitate cardiac repair after AMI, in which miR-181b-5p plays a key role, but further studies are needed to confirm this (86). In a mouse AMI model, M2-Exos carrying miR-1271-5p alleviated hypoxia-induced cardiomyocyte apoptosis and promoted cardiac repair of AMI by downregulating SOX6 expression (87). Wang et al. studied the effects of M2-EVs on cardiac fibroblasts and found that circUbe3a was overexpressed in M2-EVs, promoting the proliferation, migration, and phenotypic transformation of cardiac fibroblasts by directly targeting the miR-138-5p/RhoC axis, thus exacerbating myocardial fibrosis after MI (88). In summary, M-EV-mediated cell-to-cell communication plays an important role in the progression of AS and MI.
Obesity and type 2 diabetes mellitus (T2D) are common metabolic diseases, and a growing body of research suggests that M-EVs play an important role in these metabolic diseases by mediating inflammation and regulating insulin sensitivity, lipid metabolism, glucose uptake, and mitochondrial activity (Table 2).
Obesity-induced insulin resistance is a key factor in the pathogenesis of T2D (89). M-Exos from the adipose tissue of obese mice containing miR-155 promoted obesity-induced insulin resistance. In contrast, M-EVs from the adipose tissue of lean mice attenuated obesity-induced insulin resistance (90). MiRNAs encapsulated within M-EVs derived from obese mice constitute a sophisticated paracrine and endocrine signaling network, capable of transmitting molecular cues to insulin-responsive tissues. This system intricately modulates glucose tolerance by directly repressing specific target genes, thereby influencing metabolic regulation. MiR-29a and miR-210-5p are overexpressed in adipose tissue-derived exosomes and are delivered to adipocytes, muscle cells, and hepatocytes, mediating insulin signaling by targeting peroxisome proliferator-activated receptor-δ and SID1 transmembrane family member 2, respectively, thereby impairing glucose cellular uptake and inducing insulin resistance in vitro and in vivo (91, 92). However, there is a paucity of research on the role of specific miRNAs in lean adipose tissue-derived M-EVs in enhancing insulin sensitivity, warranting further exploration. Bone marrow macrophage-derived exosomes regulate insulin sensitivity. MiR-143-5p is significantly upregulated in M1-polarized bone marrow macrophage-derived exosomes (M1-BMDM-Exo) in high-fat-fed mice, promoting hepatocyte insulin resistance by targeting mitogen-activated protein kinase phosphatase-5 (MKP5), thereby reducing AKT and glycogen synthase kinase activation and glycogen production in hepatocytes (93). A separate study demonstrated that M2-polarized bone marrow macrophage-derived exosomes (M2-BMDM-Exo) improved insulin sensitivity. Administration of M2-BMDM-Exo to obese mice improved glucose tolerance and insulin sensitivity. MiR-690 is highly expressed in M2-BMDM-Exo and enhances insulin sensitivity in vivo and in vitro by targeting NAD⁺ kinase, suggesting its potential as a novel therapeutic insulin sensitizer (94). Metastasis-associated lung adenocarcinoma transcript 1 (MALAT1) and miR-210 are significantly upregulated in hyperglucose-induced macrophage-derived exosomes (HG-M-Exo), contributing to insulin resistance (95, 96). MALAT1, a conserved long noncoding RNA (lncRNA), plays an important role in diabetes-related complications. HG-M-Exo MALAT1 induces insulin resistance by inhibiting miR-150-5p expression, thereby upregulating resistin expression (95). MiR-210 directly binds to the mRNA sequence of NADH dehydrogenase ubiquinone 1α subcomplex 4 (NDUFA4), inhibiting its expression in 3T3-L1 adipocytes, impairing glucose uptake and mitochondrial complex IV activity, and promoting the pathogenesis of obesity and diabetes in mice (96). However, Phu et al. found that IL-4-polarized human macrophage-derived exosomes (IL-4-THP-1-Exo) promote browning during the differentiation of 3T3-L1 preadipocytes into adipocytes by inducing adipogenesis and improving mitochondrial activity, glucose metabolism, and insulin resistance in obese mice (97). M1-EVs have been shown to induce insulin resistance through inflammation-related signaling pathways. For example, M1-EVs contribute to obesity-induced insulin resistance by activating the NF-κB signaling pathway, reducing insulin signaling, and decreasing glucose uptake in human adipocytes (98). M1-Exo miR-27-3p contributes to the development of T2D in mice by targeting mitochondrial Rho GTPase 1 to promote mitophagy damage-mediated inflammation, macrophage activation of the inflammatory M1-like phenotype in vivo, and insulin resistance (99). Chronic low-grade inflammation is the main cause of obesity-induced insulin resistance (100), contributing to islet β cell dysfunction. MiR-212-5p is significantly up-regulated in M1-BMDM-Exo and islet-resident M-Exos in high-fat-fed mice, impairing insulin secretion in beta cells by targeting the sirtuin2 gene and inhibiting Akt/GSK-3β/β-catenin signaling pathway (101). Additionally, LPS-activated M-Exos upregulated inflammation-and carbohydrate catabolism-related genes in adipocytes (102). M-EVs affect not only the progression of obesity and diabetes but also the development of associated complications. Zhao et al. found that exosomes derived from the adipose tissue macrophages of obese mice induced ferroptosis by delivering miR-140-5p and targeting solute carrier family 7 member 11 (SLC7A11) to inhibit glutathione synthesis, contributing to obesity-induced myocardial dysfunction (103).
Diabetic nephropathy (DN) is one of the most common microvascular complications of diabetes. Inflammasome activation-mediated podocyte damage plays an important role in DN. Ding et al. analyzed the effect of HG-M-Exo on mouse podocyte cell lines and found that miR-21-5p increased the expression of inflammasome NLR family pyrin domain containing 3 (NLRP3), caspase-1, and IL-1β in podocytes by inhibiting anti-inflammatory factor A20, thus causing podocyte damage in diabetic nephropathy (104). Similarly, HG-M-Exos promote inflammatory responses and accelerate kidney injury in DN mice by promoting NLRP3 inflammasome activation and autophagy deficiency in mesangial cells (105). Mesangial cell dysfunction is also associated with DN pathophysiology. TGFβ1 mRNA is highly expressed in HG-M-Exo and is transferred to mesangial cells to activate the TGFβ1/Mothers against decapentaplegic homolog (Smad)3 pathway, leading to mesangial cell activation and proliferation and secretion of extracellular matrix and inflammatory cytokines, promoting mesangial expansion and renal fibrosis (106). In addition, HG-M-Exo miR-7002-5p reduces renal tubular epithelial autophagy by targeting autophagy related 9B, thereby inducing tubular dysfunction and inflammation and aggravating DN (107). M2-Exos attenuate HG-induced podocyte damage and miR-25-3p in M2-Exo activates autophagy in podocytes by inhibiting dual specificity phosphatase 1 (DUSP1) expression, thereby ameliorating HG-induced podocyte injury (108).
A prevalent complication of diabetes is impaired healing. Studies have shown that M-EVs aid in the healing of diabetic wounds and fractures. High-glucose-induced M1-polarized macrophage-derived exosomes (HG-M1-Exo) transport miR-503 into HUVECs, which targets insulin-like growth factor (IGF)1R expression, which results in HUVEC dysfunction and impedes wound healing in diabetic patients (109). Bone marrow M-Exos miR-144-5p in diabetic rats inhibits the osteogenic differentiation of BMSCs osteogenic differentiation by inhibition of Smad1 expression in vivo and in vitro, thereby impairing the fracture healing process (110). M-EVs also contribute to the restoration of diabetic wounds and fracture healing by significantly reducing pro-inflammatory cytokine secretion, inducing endothelial cell proliferation and migration, accelerating angiogenesis, and promoting wound repair (111). Xia et al. found that administering lean mouse adipose tissue M-Exos to diabetes-prone db/db mice enhances wound healing. Subsequent studies revealed that miR-222-3p expression is significantly upregulated in exosomes, which regulates macrophage polarization and promotes rapid healing of diabetic wounds by targeting the inhibition of Bcl-2-like protein 11 expression (112). Similarly, M-EVs have been shown to expedite diabetic fracture healing by modulating macrophage polarization. M2-Exos induce macrophage M2 polarization by activating the phosphoinositide 3-kinase (PI3K)/(protein kinase B) AKT pathway, thereby reducing the proportion of M1 macrophages, significantly regulating the bone immune microenvironment, and accelerating diabetic fracture healing (113).
In summary, EVs and their endocannabinoids derived from different phenotypes or sources of stimuli play important roles in the development of metabolic diseases and complications through multiple pathways.
Tumorigenesis depends, in part, on M-EV interactions with components of the tumor microenvironment (TME), and tumor-associated macrophages (TAM) are one of the most abundant immune cells in the TME and play an important role in tumorigenesis and progression (114). TAMs are divided into M1-like and M2-like phenotypes and have a special transition period from M1-like to M2-like phenotypes throughout tumor progression (115). M1 phenotypic TAMs exert pro-inflammatory and antitumor activity by secreting a variety of inflammatory cytokines (e.g. IL-6, TNF-α, IL-12, and ROS) and are associated with a favorable prognosis for cancer (116, 117). M2 phenotypic TAMs exert tumor promoting activity by inhibiting immune and inflammatory responses in cancer (117). Recent studies have shown that TAM-derived extracellular vesicles (TAM-EVs) play an important role in the TME by mediating tumor cell proliferation and invasion, angiogenesis, chronic inflammation, immunosuppression, and drug resistance (Table 3). The effects of EVs derived from TAMs with different phenotypic TAMs (M1/M2-EVs) on various cancers are reviewed below.
Liver cancer is the sixth most prevalent cancer worldwide. Hepatocellular carcinoma (HCC) comprises about 80% of primary liver cancers and is the major pathological type (118). Accumulating evidence suggests that M2-EVs and their components facilitate HCC progression. For example, M2-Exos transmit miR-27a-3p and miR-660-5p to HCC cells, enhancing their proliferation, migration, and invasion by downregulating TXNIP and Krüppel-like factor 3 (KLF3), respectively, while inducing cancer stemness. In vivo xenograft models in mice have demonstrated that both M2-Exo miR-27a-3P and miR-660-5p enhance the tumorigenicity of HCC cells (119, 120). M2-EVs promote HCC progression by mediating immunosuppression. In a mouse model of primary hepatocellular carcinoma, M2-EVs promote CD8 + T cell exhaustion via miR-21-5p, accelerating HCC progression. This mechanism involves miR-21-5p promoting CD8+ T cell depletion by targeting YOD1, which activates the YAP/β-catenin pathway (121). M2-EVs also promote HCC progression by promoting tumor angiogenesis. Lu et al. found that M2-Exos augment M2 macrophage recruitment and polarization by promoting CCL2 secretion in HCC cells. M2-Exo miR-23a-3p promotes HCC cell metastasis by targeting PTEN and TJP1 to enhance EMT, angiogenesis, and vascular permeability (122). Additionally, M-Exo miR-92a-2-5p within the tumor microenvironment enhances hepatoma cell invasion by modulating AR and PHLPP/p-AKT/β-catenin signaling (123). However, M-EVs also exhibit an inhibitory effect on HCC development. Aucher et al. showed that macrophages transfer specific endogenous miRNAs (miR-142 and miR-233) into HCC cells in a cell contact-dependent manner, thereby influencing the post-transcriptional regulation of proteins in HCC, decreasing the expression of stathmin-1 (STMN1) and insulin-like growth factor-1 receptor (IGF-1R), and inhibiting HCC cell proliferation (23).
Besides miRNAs, circRNAs secreted by M-Exos are involved in tumor progression. As competitive endogenous RNA, circRNAs competitively bind to miRNAs to affect the expression level of target mRNA, thereby exerting physiological effects. RBP-J modulates TLR-induced inflammatory macrophage polarization through Notch receptor to induce the expression of M1 macrophage-related genes (124). Zhang et al. investigated exosomes from macrophages overexpressing RBP-J and found that hsa_circ_0004658 was upregulated in exosomes, enhancing JAM3 expression by competitively binding to miR-499b-5p, thereby inhibiting HCC cell proliferation and inducing apoptosis (54). These findings suggest that M-EVs in the HCC tumor microenvironment can modulate HCC progression through multiple pathways. HCC is usually diagnosed at an advanced stage; treatment for advanced HCC is limited, and patients have poor prognosis. Thus, exploring M-EVs as potential early biomarkers for HCC is imperative.
Lung cancer (LC) ranks among the most prevalent malignancies globally, characterized by two primary subtypes: small cell lung cancer (SCLC) and non-small cell lung cancer (NSCLC) (125). Lung adenocarcinoma (LUAD) is the predominant variant, representing approximately 40% of all LC cases (126). Numerous studies have elucidated the role of M-Exos in the progression of LC. Specifically, M1-Exo is implicated in the promotion of tumor progression. Serine/threonine kinase 16 (STK16) promotes tumor cell viability and hinders apoptosis through the AKT1 signaling pathway. M1-Exo miR-181a-5p inhibits STK16 by targeting ETS1, thereby diminishing LUAD cells viability, fostering apoptosis, and retarding LUAD progression (127). Several studies have shown that M2-Exos accelerate LC progression by promoting cell migration, invasion, angiogenesis, and suppressing apoptosis (128–131). For example, M2-EV miR-942 promotes migration and invasion of LUAD and angiogenesis by targeting forkhead box (FOX)O1 and activating the Wnt/β-catenin pathway, thereby accelerating the progress of LUAD (130). M2-EV miR-22-3p has been reported to promote osteogenic differentiation of BMSCs by targeting PER2 to activate the Wnt/β-catenin signaling pathway (132). These results indicate that different miRNAs within M2-Exo from the same source act on different target genes and activate the same signaling pathway, thereby exerting physiological effects in different diseases. Another study showed that M2-EV miR-501-3p inhibits apoptosis by targeting WDR82 to promote the proliferation, migration, and invasion of lung cancer cells (129). Although WDR82 is typically underexpressed in tumor tissues, its overexpression in lung cancer cells has been observed to suppress the malignant transformation of these tumor cells. M2-Exo miR-155-3p also enhanced the growth of medulloblastoma cells by targeting WDR82, thus accelerating medulloblastoma progression (133). These results indicate that different miRNAs from the same source of M2-Exos can exert similar physiological effects by acting on the same target gene. The observation that different miRNAs exhibit similar anti-tumor effects on the same target gene suggests that Exos-miRNA primarily serves as an informational molecule. M2-Exos also promote tumor progression by mediating chemoresistance and radiation resistance. Apolipoprotein E (ApoE) secreted by M2-EV worsens the immunogenicity of tumor cells. ApoE inhibits tumor cell immunogenicity by directly binding to and inhibiting BiP function to reduce tumor MHC-I expression, thereby conferring resistance to immunotherapy (134). Wang et al. showed that M2-Exos delivered miR-3679-5p to LC cells and reduced NEDD4L-mediated c-Myc ubiquitination by inhibiting NEDD4L transcription, promoting aerobic glycolysis in LC cells, and inducing chemoresistance (135). These findings provide a basis for studying the mechanisms of drug resistance to improve lung cancer treatment. In addition, Zhang et al. found that lncRNA AGAP2-AS1 is overexpressed in M2-Exos and transferred to LC cells to promote radioresistance in LC cells by downregulating miR-296 and upregulating NOTCH2, the target gene of miR-296. Moreover, the expression levels of lncRNAs AGAP2-AS1 and NOTCH2 were increased in lung cancer cells and tissues, whereas miR-296 levels were diminished (136). This suggests that AGAP2-AS1 serves as a prognostic indicator in patients with LC.
Esophageal cancer (EC) is among the most lethal malignancies globally, ranking sixth in cancer mortality, primarily composed of squamous cell carcinoma (SCC) and adenocarcinoma (137). Gastric cancer (GC) ranks as the fifth most prevalent cancer and the third leading cause of cancer-related mortality (138). A burgeoning body of research has illuminated the association of M-Exos with tumorigenesis and progression. For example, lncRNA AFAP1-AS1 is highly expressed in M2-Exos and transferred to EC cells by downregulating miR-26a and upregulating ATF2 expression, thus promoting EC invasion and metastasis (139). In addition, M2-Exo LINC01592 accelerates the degradation of MHC-1 and promotes EC immune evasion by activating the E2F6/NBR1/MHC-I signaling pathway. Tumor cells can evade CTL immune attack owing to alterations or defects in MHC-I molecules on the surface of tumor cells (140). M-Exos also affect GC progression by mediating immune escape. M1-Exo deliver miR-16-5p to GC cells and activate the T cell immune response by targeting and inhibiting the expression of PD-L1, thereby reducing immune evasion of GC cells and inhibiting GC progression (141). However, M2-Exos promote the proliferation and migration of GC cells via p38/mitogen-activated protein kinase (MAPK) signaling pathways and achieve immune evasion by increasing the expression of PD-L1, thereby promoting GC progression (142). Additionally, M2-Exo miR-487a promotes GC proliferation by inhibiting the expression of the tumor suppressor gene T-lymphocyte antigen 1 (TIA1) (143). Multiple studies have shown that M2-Exos plays an important role in GC progression and chemoresistance (20, 144, 145). Chemotherapeutic agents such as cisplatin (DDP), doxorubicin (DOX), and paclitaxel (PTX) are used to treat various malignancies, including GC (146). Cui et al. showed that M2-Exo miR-588 contributes to DDP resistance in gastric cancer cells by partially targeting CYLD (20). Similarly, M2-Exo miR-21 is transferred to GC cells and downregulates PTEN to activate the PI3K/AKT signaling pathway, reducing chemosensitivity and apoptosis in GC cells and conferring DDP resistance in gastric cancer (144). In another study, M2-Exo delivered miR-223 to GC cells and induced DOX resistance in GC cells by targeting F-box and WD repeat domain-containing 7 (FBXW7). Moreover, the expression of plasma exosomal miR-223 is significantly increased in patients with GC with DOX-resistance, suggesting that miR-223 may be a potential indicator of DOX resistance in gastric cancer (145). Studies have shown that DCA-induced M-Exos indirectly affect GC progression. Xu et al. found that deoxycholic acid-stimulated M-Exos promoted the development of antispasmodic polypeptide-expressing metaplasia (SPEM) in mouse gastric organoids. SPEM is an important risk factor for gastric cancer (147). Similarly, deoxycholic acid-induced M-Exos were enriched with high levels of hsa-miR-30a-5p, and the overexpression of hsa-miR-30a-5p inhibited gastric epithelial cell proliferation and promoted intestinal metaplasia by targeting FOXD1. Gastrointestinal metaplasia is considered to be a risk factor for enteral gastric cancer (148). M2-Exos also exert physiological functions in GC by delivering proteins. For example, M2-Exos transfer functional ApoE into GC cells and promote GC cell migration by activating the PI3K-Akt signaling pathway (57).
Pancreatic cancer (PC) is a highly lethal malignancy that ranks seventh among cancer-related mortalities worldwide (149). Pancreatic ductal adenocarcinoma (PDAC) is the most common type of PC, accounting for >85% of all PC cases (150). M-Exo miRNAs and lncRNAs also affect PC progression. The lncRNA SBF1-AS2 is significantly upregulated in M2-Exos and upregulates the expression of XIAP by inhibiting the expression of miR-122-5p, thereby promoting the tumorigenicity of PC cells (151). Both miR-21a-5p and miR-501-3p are derived from M2-Exos and have been confirmed to promote PC cell migration and invasion (26, 152). The microRNA miR-21a-5p is up-regulated in M2-Exos and transferred to human pancreatic cancer cells (PaCa) to regulate the differentiation and activity of PaCa stem cells by directly targeting KLF3 (26). However, miR-501-3p promotes the migration, invasion, and tube formation of PDAC cells by targeting the TGFβR3 gene to activate the TGFβ signaling pathway, thus promoting the progression of PDAC (152). In addition, M2-Exo miR-155-5p and miR-221-5p have been confirmed to promote angiogenesis and subcutaneous tumor growth in PDAC by targeting E2F2 (153). M2-exo also influences PC development by mediating chemoresistance. Gemcitabine is the first-line chemotherapeutic drug for the treatment of PC; however, drug resistance limits the efficacy of chemotherapy. Guo et al. found that miR-222-3p increases cell proliferation and angiogenesis and decreases apoptosis in the tumor tissues of mice exposed to gemcitabine, mainly through the mechanism of miR-222-3p-induced gemcitabine resistance in PC progression by targeting TSC1 and activating the mTOR/AKT/PI3K pathway (27). Similarly, Binenbaum et al. found that M2-Exo miR-365 impairs gemcitabine activation by upregulating the nucleotide pool of triphosphates in cancer cells and inducing cytidine deaminase, thereby significantly reducing the sensitivity of PDAC cells to gemcitabine (154). Another study showed that M2-Exo miR-365 promoted the migration, invasion and tube formation of PDAC cells by targeting the TGFβR3 gene to activate the TGFβ signaling pathway, thus promoting the progression of PDAC (155). This suggests that the same miRNAs from the same source of M-Exos can exert similar physiological effects on diseases through different pathways. M2-Exo miR-3679-5p reduces NEDD4L-mediated c-Myc ubiquitination and promotes LC progression (135). Zhang et al. found that M2-Exo miR-193b-3p reduces TRIM62-mediated c-Myc ubiquitination by targeting TRIM62, and promotes the proliferation, migration, invasion, and glutamate uptake of PC cells in vivo and in vitro, thus promoting PC progression (152).
Colon cancer (CC) ranks among the most prevalent malignancies within the digestive tract and constitutes the third leading cause of cancer-related mortality globally (156). Many M2-EV miRNAs have been found to be involved in CC development. MiR-21-5p is a tumorigenic miRNA targeting KLF3, YOD1, and METTL3, thus promoting pancreatic, hepatocarcinoma, and thyroid cancer progression and inducing chemoresistance (26, 121, 157). Likewise, miR-155-5p is confirmed to be tumorigenic, targeting GREM1 and E2F2, thereby promoting intracranial aneurysms formation and angiogenesis in pancreatic ductal adenocarcinoma (153, 158). Lan et al. found that miR-21-5p and miR-155-5p are upregulated in M2-Exos and transferred to colorectal cancer cells, facilitating their migration and invasion by targeting and downregulating BRG1 expression (159). In addition, M2-Exo miR-155-5p enhances IL-6 expression by inhibiting ZC3H12B, thereby promoting immune escape and tumorigenesis in CC (160). This further illustrates that M2-Exo miR-21-5p and miR-155-5p have protumor activity and can promote the progression of multiple cancers. M2-Exo miR-501-3p promotes lung cancer and PDAC progression by targeting WDR82 and TGFβR3 (129, 161). Ding et al. found that miR-501-3p was transferred to CC cells via M2-EVs, targeting and downregulating SETD7, thereby upregulating DNMT1 and SOCS3, which enhances the malignant signature of CC cells in vitro and tumor growth in vivo (162). This suggests that identical miRNAs from common sources may exert analogous roles across diverse tumors. Studies have shown that M2-EV miRNAs can also promote CC progression by mediating inflammation-related signaling pathways. Both miR-186-5p and miR-183-5p are upregulated in M2-EVs and can promote the proliferation, invasion, and metastasis of CC cells by targeting DLC1 and THEM4, respectively, activating the β-catenin, PI3K/AKT, and NF-κB signaling pathways, thereby accelerating the progression of CC (163, 164). Another study reported that M2-EVs delivered miR-143-3p to colorectal cancer cells, where it promotes CC development by targeting ZC3H12A to upregulate C/EBP-β expression (165). Beyond miRNAs and lncRNAs, circRNAs secreted by M-EVs contribute to CC development. CircRNAs are a highly abundant and heterogeneous class of non-coding RNAs enriched in EVs. Fan et al. reported that M2-EVs enhance CC cell immune escape by delivering circRNACCDC66, inhibiting miR-342-3p expression and upregulating MTDH expression, thereby promoting CC development (55). Nevertheless, the physiological impacts of M-EV circRNAs in diseases remain underexplored.
Recent studies have shown that TAM-EVs are involved in the progression of some female-related cancers such as breast, cervical, ovarian, and endometrial cancers. Breast cancer (BC) is one of the most common malignancies in women. Studies have shown that miR-503-3p is overexpressed in TAM-EVs, activating the Wnt/β-catenin signaling pathway by targeting DACT2, promoting glycolysis and reducing mitochondrial oxidative phosphorylation in BC cells, thereby advancing BC development (166). Aberrant activation of the Wnt/β-catenin signaling pathway mediated by TAM-EVs has associated with several cancer types, including PDAC and CC (130, 163). BC metastasis is the leading cause of cancer-related death in women worldwide. Studies have shown that TAM-EV miRNAs promote the invasion and migration of BC cells. For example, miR-660 overexpressed in TAM-EVs, is transferred to BC cells, where it promotes invasion and migration by inhibiting KLHL21 and activating the NF-κB signaling pathway (167). In a similar study, M2-Exos shuttle miR-223 into BC cells and promotes BC cell invasion through the Mef2c-β-catenin pathway (168). These findings offer novel insights for developing treatments targeting breast cancer metastasis. Another study showed that miR-223 induces drug resistance in epithelial ovarian cancer (EOC) cells by targeting PTEN to activate the PI3K/AKT signaling pathway and trigger a chemoresistant phenotype (169). TAM-EV miR-21 also induces chemotherapy resistance in gastric cancer cells by targeting PTEN to activate the PI3K/AKT signaling pathway (144). These results indicate that different miRNAs can mediate the same signaling pathway by targeting the same target gene to exert similar physiological effects, further revealing that multiple miRNAs play a synergistic role in the development of diseases, rather than relying on a single miRNA. In addition to EOC and GC, TAM-EV-mediated aberrant activation of the PI3K/AKT pathway drives the progression of various cancers, including PC and CC (27, 164). In EOC, TAM-EV miR-221-3p exerts oncogenic effects by targeting CDKN1B, thereby regulating proliferation, migration, and G1/S transformation of EOC cells (170).
Ovarian cancer (OC) is a malignancy impacting the female reproductive tract. TAM-EVs facilitate OC progression by mediating immune escape and chemotherapy resistance. Chen et al. reported GATA-binding protein-3 (GATA3) expression in TAM-EVs. GATA3 is transferred into OC cells via TAM-EVs, inhibiting T-cell activity through the CD24/Siglec-10 pathway, thus promoting immune escape and chemoresistance (171). In addition, TAM-EV miR-29a-3p enhances PD-L1 expression by mediating the FOXO3-AKT/GSK3β axis, thereby promoting OC cell proliferation and immune escape in vitro and in vitro (172). These findings may provide novel therapeutic targets for the treatment of OC.
Cervical cancer is the leading malignant cancer in women worldwide, and studies have shown that TAM-EV lncRNAs are involved in the progression of cervical cancer. LRRC75A-AS1 promotes proliferation, migration, invasion, and EMT in cervical cancer by inhibiting miR-429 expression, upregulating SIX1 expression, and activating the signal transducer and activator of transcription (STAT)3/MMP-9 signaling axis (173). Endometrial cancer is a common gynecological malignancy that seriously threatens women’s health. Cyclin B1 is an important promoter that induces radioresistance in cancer cells by regulating the cell cycle. Studies have shown that hsa _circ_0001610 is overexpressed in M2-Exos and transferred to endometrial cancer cells, and negatively regulates the expression of miR-139-5p in endometrial cancer cells by directly binding to miR-139-5p, upregulating the expression of cyclin B1, thereby attenuating the radiosensitivity of endometrial cancer cells (56). Endometriosis (EMS) is a debilitating disease with a chronic inflammatory profile that resembles malignancy and includes progressive and aggressive growth (174). Studies have shown that peritoneal macrophage-derived exosomes (PCM-Exos) influence EMS progression PCM-Exo miR-22-3p promotes the proliferation, migration, and invasion of human ectopic endometrial stromal cells (eESCs) by targeting SIRT1 and activating the NF-κB pathway (22). Another study showed that PCM-Exos promoted the proliferation, migration, and invasion of eESCs by transporting lncRNA CHL1-AS1 into eESCs to downregulate miR-610 and upregulate MDM2 (21).
TAM-EVs also affect the progression of other types of cancer such as oral squamous cell carcinoma, head and neck squamous cell carcinoma, intracranial aneurysm, meningioma, glioma, and medulloblastoma. M1-Exo have inhibitory effects on tumors, including head squamous cell carcinoma (HNSCC), glioma, and human melanoma. The lncRNA HOTTIP is a key molecule in M1-Exo and activates the TLR5/NF-κB signaling pathway by competitively binding to miR-5a-19p and miR-3b-19p, thereby inhibiting the progression of HNSCC (52). M1-Exo miR-150 inhibits glioma progression by targeting MMP-16 to inhibit human glioma cells (175). However, M1-EV miR-29a-3p inhibits the proliferation of human melanoma cells and exerts antitumor effects (25). In oral squamous cell carcinoma (OSCC), M2-Exo miR-31-5p downregulates the expression of the tumor suppressor gene LATS2 by inhibiting the Hippo signaling pathway, thereby promoting OSCC progression (176). In addition, M-Exos attenuate the effects of chemotherapeutic agents on cell cycle regulation and apoptosis by activating the AKT/GSK-3β signaling pathway, thereby reducing the sensitivity of OSCC cells to the chemotherapeutic agents 5-fluorouracil (5-FU) and cis-diamine diachloroplatin (CDDP) (177). M2-EVs also mediate immunosuppression and chemoresistance in thyroid cancer. Ning et al. showed that M2-EV miR-21-5p downregulated METTL3 expression in thyroid cancer cells, thereby leading to demethylation and stabilization of CD70 mRNA in a YTHDF2-dependent manner and that upregulation of CD70 protein levels increases the abundance of immunosuppressive Tregs and terminal failure T cells, thus promoting immunosuppressive and anti-PD-1 therapy resistance (157). In addition, M2-EVs induce drug resistance in glioma cells. The expression of miR-27a-3p, miR-22-3p, and miR-221-3p is significantly upregulated in M2-EVs; these miRNAs promote neuromesenchymal transition (PMT) and radioresistance in glioma stem cells by targeting CHD7 to increase RelB-P50 and phosphorylated STAT3 (178). Yao et al. found that miR-15a and miR-92a blocks the PI3K/AKT/mTOR signaling pathway and inhibits glioma cell invasion and migration by binding to CCND1 and RAP1B, respectively. M2-Exos with insufficient miR-15a and miR-92a expression promote the migration and invasion of glioma cells (179). Another study showed that M2-Exos contribute to the development of meningioma by activating the TGFβ signaling pathway to promote the proliferation, migration, and invasion of meningioma cells (180). TAM-Exos can be absorbed by tumor cells as well as other cells, thereby regulating tumor progression. Studies have shown that miR-155-5p is upregulated in TAM-Exos and transported to smooth muscle cells (SMCs) to promote the proliferation and migration of SMCs by targeting GREM1, thereby promoting the formation of intracranial aneurysms and the activation and infiltration of TAMs (158).
EVs derived from macrophages of different phenotypes and their contents have different effects on the occurrence and development of inflammatory diseases, depending on their parental cell types and the cargo they carry (Table 4). In experimental autoimmune neuritis (EAN) animal models, M1 macrophage-derived exosomes (M1-Exo) directly regulate T cells, enhance Th1 cell differentiation (increase proportion) and effector function (increase IFN-γ intensity), and increase IFN-γ production in CD8+ T cells, promoting EAN progression (181). M2 macrophage-derived exosomes (M2-Exo) showed the potential to attenuate EAN. M-EVs lacking the endonuclease XPF-ERCC1 induce persistent DNA damage, promote cellular glucose uptake, and activate innate immune responses, leading to increased inflammation (182). In this procedure, Irreparable DNA damage triggers an exosome-based, metabolic reprogramming that leads to chronic inflammation and tissue pathology in nucleotide excision repair progeroid syndromes. Anti-inflammatory miRNAs (miR-146a, miR-146b, and miR-21-3p) in small EVs (sEVs) from LPS-stimulated RAW264.7 macrophages (LPS-M-sEV) downregulate pro-inflammatory target genes. In a mouse model of Freund’s-adjuvant-induced inflammatory pain, LPS-M-sEVs alleviated pain by modulating multiple genes (24). In the same animal model, M2-EVs miR-23a-3p modulated the histone deacetylase 2/nuclear factor erythroid 2–related factor 2 axis by targeting ubiquitin-specific proteinase 5 expression to reduce inflammatory pain (183). These results highlight the role of M-EVs in mediating inflammation and immune responses.
MiR-21a-5p is elevated in peritoneal M-Exo of dextran sulfate (DSS)-induced ulcerative colitis (UC) mice and promotes innate lymphoid type-2 cell activation by decreasing E-cadherin expression, leading to intestinal mucosal epithelium damage and worsened enteritis (184). In a DSS-induced inflammatory bowel disease model, LPS-stimulated bone marrow-derived M-Exo miR-223 accelerated colitis by inhibiting transmembrane and immunoglobulin domain-containing protein 1 to induce intestinal barrier dysfunction (185). Conversely, M2-Exo miR-590-3p suppressed pro-inflammatory cytokines by targeting large tumor suppressor kinase 1, activating yes-associated protein (YAP)/β-catenin-regulated transcription, thereby reducing inflammatory signaling, accelerating epithelial repair and wound healing in DSS-induced colitis (186). M2-EVs protect against intestinal inflammation; they upregulate maternally expressed gene 3 (MEG3) by delivering lncRNA MEG3 to colonic epithelial cells, then promoting the transcription of CAMP responsive element binding protein 1 via competitive binding to miR-20b-5p, thereby enhancing cell viability and mitigating inflammatory response in UC (53). The sEVs from tyrosine-protein phosphatase non-receptor type 1-knockout macrophages also alleviate intestinal inflammation, mainly by inducing macrophage M2 polarization and inhibiting TNF-α and nuclear factor kappa B (NF-κB) signaling via transporting mucin-containing cargo (80, 187).
In a mouse model of cancer-induced bone pain (CIBP), M2-Exo exerts antinociceptive effects by delivering miR-216a, which targets high mobility group box 1 expression to downregulate the TLR4-NF-κB signaling pathway (188). M2 macrophage-derived apoptotic bodies can prevent or alleviate osteoarthritis (OA) by reprogramming M1 to M2 macrophages, thus reducing chondrocyte damage (29). In a rat model of knee osteoarthritis (KOA), M2-EVs reduced cartilage inflammation by modulating the PI3K/AKT/mTOR pathway (189). Studies indicate that PI3K/AKT/mTOR pathway activation exerts anti-arthritic effects by enhancing chondrocyte proliferation and reducing apoptosis (190). In a rat model of spinal cord injury, peritoneal macrophage-derived exosomes (PCM-Exos) activated and promoted microglial autophagy by downregulating the PI3K/AKT/mTOR signaling pathway, thereby increasing anti-inflammatory microglial polarization, stimulating local microglial anti-inflammatory properties, and promoting post-spinal cord injury repair (191).
Abdominal aortic aneurysm (AAA) is a chronic inflammatory disease with no effective treatment currently available. Wang et al. demonstrated that M-Exos participate in AAA pathogenesis by activating the c-Jun N-terminal kinase (JNK) and p38 pathways to induce matrix metalloproteinase (MMP)-2 production (192). This suggests that M-Exos serve as a therapeutic target, warranting further investigation. Hemorrhagic shock (HS) predisposes patients to systemic inflammatory syndromes. In vivo and in vitro HS models have shown that the exosomes from HS-activated alveolar macrophages promote polymorphonuclear neutrophil (PMN) necroptosis by upregulating the production of nicotinamide adenine dinucleotide phosphate (NADPH) oxidase-derived ROS in PMNs, subsequently enhancing inflammation and tissue damage (193). Previous studies showed that M-EVs affect women’s health by modulating inflammatory factor expression. M-EVs enter the placenta via clathrin endocytosis, inducing pro-inflammatory responses and modulating placental cytokine production, thus potentially promotes responses to maternal inflammation and infection, thereby preventing fetal injury (194, 195). This may reveal a new way of transmitting information between the mother and placenta. M1-EVs are associated with pro-inflammatory and harmful effects but also have protective roles. M1-EVs suppress the migration and invasion of endometriosis-eutopic endometrial stroma cells (EM-ESCs) and hinder endometriosis development by reprogramming M2 to M1 macrophages, thereby reducing human umbilical vein endothelial cells (HUVEC) tube formation (196). These findings suggest a potential strategy for the treatment of endometriosis. Another study showed that M2-EVs contain specific miRNAs that improve ovarian function in elderly mice by downregulating the mTOR signaling pathway and improving the inflammatory microenvironment within the ovaries (197). These results suggest that M-EVs are crucial in inflammatory diseases, paving the way for clinical applications.
Macrophages are crucial for bone homeostasis (198, 199). Numerous studies indicate that M-EVs are pivotal in bone diseases like fractures, osteoarthritis, osteoporosis, and tendon injury. M-EVs mainly mediate osteoblast differentiation, angiogenesis, immune cell reprogramming, cell proliferation, and apoptosis (Table 5). Mesenchymal stem cell (MSC) osteogenic differentiation is a key factor in fracture healing (198). M1-Exos upregulate IL-6 and TNF-α in BMSCs, thus inhibiting their osteogenic differentiation through inflammatory mediation (200). Xia et al. investigated how different macrophage-derived exosomes (M0/M1/M2-Exos) affect BMMSC proliferation, osteogenesis, and adipose differentiation, finding that M1-Exos enhance BMMSC proliferation. M1-Exos have a more pronounced effect on BMMSC proliferation and osteogenic differentiation compared to M0-Exos or M2-Exos (201). Moreover, M1-Exos promote the osteogenic differentiation of BMSCs through microRNA-21a-5p during early inflammation (202). This seems to contradict previous results, but it is an interesting possibility that M-Exos of different phenotypes promote the osteogenic differentiation of BMSCs at different stages of the disease. This is consistent with the fact that M0 macrophages support osteogenesis during normal bone homeostasis, whereas M1 and M2 macrophages contribute to different stages of fracture healing (198). Angiogenesis is a prerequisite for osteogenesis during bone repair and regeneration. In vitro studies have shown that Mg2+-stimulated M-Exos inhibit angiogenesis in endothelial cells by down-regulating endothelial nitric oxide and vascular endothelial growth factor (203). However, exosomes derived from macrophages upon copper or cobalt ions promote angiogenesis (204, 205). M1-Exos also impact bone loss and osteoporosis. In a mouse model of postmenopausal osteoporosis, M1-Exo miR-98 exacerbated bone loss and osteoporosis by targeting DUSP1 and activating the JNK signaling pathway (205). In contrast, miR-690-enriched M2-Exos promote BMSC osteogenesis and reduce lipogenesis by upregulating IRS-1 and TZ levels, potentially offering a treatment for bone loss (207). M2-Exos also promote osteogenic differentiation of BMSCs via multiple pathways, exerting osteoprotective effects (48, 132, 208, 209). For example, M2-Exo miRNA-26a-5p promotes expression of osteogenic differentiation-related proteins ALP, RUNX-2, OPN, and Col-2, induces BMSCs osteogenic differentiation, and inhibits adipogenic differentiation (208). M2-Exo miR-22-3p promotes BMSCs osteogenic differentiation by targeting PER2 to activate the Wnt/β-catenin signaling pathway (132). In a mouse fracture model, M2-Exos inhibited the expression of salt-induced kinases 2 and 3 (SIK2 and SIK3) by delivering miR-5106 to promote osteogenic differentiation of BMSCs and accelerate fracture healing (209). The anti-inflammatory cytokine IL-10 is a key regulator in bone remodeling (198). M2-Exos deliver IL-10 mRNA directly to BMSCs and BMDMs, activating the cellular IL-10/IL-10R pathway, thereby promoting BMSC osteogenic differentiation and inhibiting BMDM formation (48). Mg2+ promotes osteogenesis and prevents inflammation, with Mg2+-induced M-Exos enhancing BMSC osteogenic differentiation by downregulating miR-381 (210). In bone tissue regeneration and repair, macrophage-derived small extracellular vesicles (ICM-M-sEV) treated with biomimetic intrafibrous mineralized collagen (IMC) promote osteogenic differentiation of BMSCs through the BMP2/Smad5 pathway (211). M2-Exos play a role in periodontal tissue regeneration and bone remodeling and enhance osteogenic differentiation of human periodontal ligament stem cells (PDLSC) (212). M2-Exos also induce healing of muscle and tendon injuries. The miR-501-enriched M2-Exo targeting YY1 promotes myoblast differentiation, myotube formation, and regeneration of damaged pubococcygeal muscle (213). M2-Exo miR-21-5p enhances tendon cell proliferation, migration, and fibrosis by suppressing Smad7, thus inducing peritendon fibrotic healing after tendon injury in vivo (214). Osteoarthritis (OA) is an inflammation-mediated patholytic disease closely linked to cartilage deformity severity. M1-EVs advance OA pathogenesis by activating atypical pyroptosis in chondrocytes and exacerbating chondrocyte catabolism and cartilage degradation (215).
Many studies have shown that M-EVs serve as drug delivery systems enhancing efficacy owing to their excellent stability, biosafety, compatibility and ability to cross the blood-brain barrier (BBB). M-EVs can be engineered by incorporating low-molecular-weight chemotherapeutic drugs, such as PTX and DOX, or therapeutic proteins, such as catalase and brain-derived neurotrophic factor, into EVs through method like co-incubation, electroporation, saponin permeabilization, freeze-thaw cycles, or sonication (216–218). Engineered M-EVs, as new drug delivery platforms, have several advantages over traditional delivery methods, including high biocompatibility, enhanced stability, precise targeting, and low immunogenicity (219). M1-EVs alone showed antitumor effects, as previously described (52, 127, 141). When M1-EVs serve as drug carriers alongside other drugs, they significantly improve the antitumor efficacy. For example, M1-EVs loaded with PTX, DOX, DDP, docetaxel (DTX), gemcitabine (GEM), and deferasirox (DFX) significantly improve the antitumor effects of these chemotherapeutic agents in vitro and in vivo by inhibiting tumor cell growth or inducing mitochondrial dysfunction, preventing M1 macrophages from repolarizing to the M2 phenotype, or increasing their sensitivity (216, 220–225). Genetic and chemical modifications can augment engineered M-EVs’ targeting and efficacy in tumor therapy. Kim et al. developed and optimized a formulation of PTX-loaded exosomes with an aminoethylanisamide-polyethylene glycol (AA-PEG) vector moiety to target the sigma receptor overexpressed in lung cancer cells. PTX-loaded AA-PEG-vectored exosomes (AA-PEG-exoPTX) have high loading capacity and targeting ability, enhancing antitumor efficacy upon systemic administration (226). Li et al. modified M-EVs with peptides targeting mesenchymal-epithelial transition factors, creating an M-EV-coated polylactic acid-glycolic acid copolymer nanoplatform that enhanced DOX’s cellular uptake and antitumor efficacy (227). Zhang et al. created a tri-modal synergistic therapy with exosome-based nanoplatforms. Utilizing exosomes as carriers, the UN@mSiO2-Ce6 (UC) probe was integrated into M1-Exos, forming the M1UC probe. The M1UC probe not only reprograms immunosuppressive M2 macrophages into antitumor M1 macrophages for immunotherapy but also catalyzes the endogenous production of nitric oxide (NO) by iNOS for gas therapy. Simultaneously, NO can react with ROS produced by the photosensitizer Ce6 to generate peroxynitrite, significantly enhancing the lethality of PDT in tumors (228). Engineered M-EVs mitigate inflammatory disease progression by targeting inflammation areas and enhancing anti-inflammatory effects. Studies have shown that loading the anti-inflammatory drug mycophenolic acid (MPA) into M-EVs as an immunomodulatory nanoplatform significantly enhanced the anti-inflammatory and antioxidant effects of MPA in vitro compared with those of free drugs (229). Simultaneously, loading melatonin into M-EVs specifically targets inflammatory areas and mediates immune reprogramming, thereby polarizing macrophages from M1 to M2 phenotypes (230). In addition, Wu et al. loaded 5-aminolevulinate hexyl hydrochloride (HAL) into M2-Exos via electroporation, leading to intrinsic heme biosynthesis and metabolism to produce anti-inflammatory carbon monoxide and bilirubin, enhancing anti-inflammatory effects (231). Moreover, Cheng et al. loaded stereoscopic Pd into M-EVs to construct a biomimetic nanoformulation (Pd-M-EVs). Pd-M-EVs inhibit macrophage M1 polarization and reduce neutrophil infiltration and recruitment by blocking mTORC1-HIF-1α-mediated glycolysis, thereby promoting colonic inflammation and remodeling of the immune microenvironment, improving the barrier function of the colonic mucosa, and effectively alleviating UC (232). Engineered M-EVs have also been used in fracture healing. Shou et al. conjugated 3WJ RNA nanoparticles with BMSC adapters to M2-Exos, creating aptamer-functionalization exosomes (3WJ-BMSCapt/M2-Exo) that target fracture sites, accelerating fracture healing in mice (233). The inability of most drugs to cross the BBB limits therapeutic applications for central nervous system diseases. Studies have shown that M-EVs cross the BBB and deliver therapeutic cargo to the brain as carriers of central nervous system drugs, such as the therapeutic protein brain-derived neurotrophic factor (BDNF) or baicalin (BA) (217, 234, 235). In a mouse model of Alzheimer’s disease (AD), encapsulation of silymarin (Slb) into M-EVs delivered more SIb to the brain and enhanced its brain-targeting ability, leading to better efficacy and alleviation of cognitive impairment (236). Li et al. showed that Edaravone (Edv)-loaded M-EVs improve the bioavailability of Edv and efficiently and specifically deliver Edv into the ischemic brain, thereby enhancing neuroprotection in a rat model of permanent middle cerebral artery occlusion (PMCAO) (237). Collectively, these findings suggest that M-EVs can serve as novel brain-targeted drug delivery systems.
Macrophages are innate immune cells distributed throughout the body that contribute to homeostasis and disease. M-EVs have the characteristics of parental cells, which play a wide role in the aforementioned diseases, mainly by mediating inflammation and immune responses, thus providing new ideas for the treatment of many diseases. M-EVs induce a pro-inflammatory microenvironment that favors a Th1 immune response, thereby inducing strong antigen-specific immune responses and making the immune system sensitive to cancer vaccines, demonstrating the potential of M-EVs as immunopotentiators for cancer vaccines (238). M-EVs have advantages, such as excellent stability, biosafety, compatibility, precise targeting, low immunogenicity, and the ability to cross the blood-brain barrier, making them highly effective drug carrier systems. This method of drug delivery selectively reduces non-specific uptake of the drug by normal cells, thereby reducing the risk of toxicity and adverse effects. The M-EVs derived from different types of macrophages have a significant ability to inhibit or promote inflammation, which will help to achieve a personalized and effective anti-cancer response.
However, there are still some challenges in their application, such as the low purity and yield of EVs, and further studies are needed to confirm their stability and safety. Notably, the internal environment of the body is very complex, and macrophages of different phenotypes exist simultaneously under different physiological and pathological conditions and jointly maintain physiological homeostasis. This suggests that M-EVs with different phenotypes coexist and work together.
Simultaneously, EVs contain a variety of bioactive substances including various proteins, lipids, and other biomolecules such as cytokines. These elements have the potential to influence the physiological state of recipient cells, thereby modulating their response to miRNAs. For instance, cytokines may alter miRNA expression patterns within recipient cells by activating distinct signal transduction pathways, consequently impacting the functional efficacy of miRNAs. However, most studies have focused on only one inclusion in a single phenotypic M-EV, such as only one or more miRNAs, or only one protein, mRNA, or lncRNA. A previous study found that the same miRNA from the same source of M-EVs can play a role in different diseases through different pathways, and different miRNAs can exert similar physiological effects by targeting the same target gene to regulate the same signaling pathway. Therefore, further research is required to confirm the use of a single miRNA as a potential biomarker for specific diseases. In forthcoming experimental designs, it may prove essential to rigorously regulate cytokine levels and cell state variables to facilitate an independent assessment of miRNA function. Employing high-throughput sequencing technologies could enable a comprehensive analysis of the phenotypic alterations in miRNAs and their target genes within recipient cells. This approach would be instrumental in elucidating the intricate interactions among various factors, thereby advancing our understanding of their collective impact. Further investigation into the transport mechanisms of miRNAs—encompassing their loading into and release from extracellular vesicles, as well as their interplay with cytokines—will significantly enhance our comprehension of the role that extracellular vesicles from macrophages play in disease pathogenesis. Such studies promise to elucidate the complex molecular dialogues that govern cellular communication and disease progression.
FL: Formal analysis, Investigation, Writing – original draft. HL: Methodology, Writing – review & editing. JW: Investigation, Writing – review & editing. QL: Project administration, Writing – review & editing. LZ: Funding acquisition, Writing – review & editing.
The author(s) declare financial support was received for the research, authorship, and/or publication of this article. This work was supported by grants from National Natural Science Foundation of China (NSFC) (No.82073532; No.82373554) and Natural Science Foundation of Guangdong Province, China. (No. 2023A1515011614).
The authors declare that the research was conducted in the absence of any commercial or financial relationships that could be construed as a potential conflict of interest.
All claims expressed in this article are solely those of the authors and do not necessarily represent those of their affiliated organizations, or those of the publisher, the editors and the reviewers. Any product that may be evaluated in this article, or claim that may be made by its manufacturer, is not guaranteed or endorsed by the publisher.
The Supplementary Material for this article can be found online at: https://www.frontiersin.org/articles/10.3389/fimmu.2024.1479330/full#supplementary-material
1. Wynn TA, Chawla A, Pollard JW. Macrophage biology in development, homeostasis and disease. Nature. (2013) 496:445–55. doi: 10.1038/nature12034
2. Wynn TA, Vannella KM. Macrophages in tissue repair, regeneration, and fibrosis. Immunity. (2016) 44:450–62. doi: 10.1016/j.immuni.2016.02.015
3. Gordon S, Martinez-Pomares L. Physiological roles of macrophages. Pflugers Arch. (2017) 469:365–74. doi: 10.1007/s00424-017-1945-7
4. Varol C, Mildner A, Jung S. Macrophages: development and tissue specialization. Annu Rev Immunol. (2015) 33:643–75. doi: 10.1146/annurev-immunol-032414-112220
5. Davies LC, Jenkins SJ, Allen JE, Taylor PR. Tissue-resident macrophages. Nat Immunol. (2013) 14:986–95. doi: 10.1038/ni.2705
6. Martinez FO, Gordon S. The M1 and M2 paradigm of macrophage activation: time for reassessment. F1000Prime Rep. (2014) 6:13. doi: 10.12703/P6-13
7. Yunna C, Mengru H, Lei W, Weidong C. Macrophage M1/M2 polarization. Eur J Pharmacol. (2020) 877:173090. doi: 10.1016/j.ejphar.2020.173090
8. Shapouri-Moghaddam A, Mohammadian S, Vazini H, Taghadosi M, Esmaeili SA, Mardani F, et al. Macrophage plasticity, polarization, and function in health and disease. J Cell Physiol. (2018) 233:6425–40. doi: 10.1002/jcp.v233.9
9. Hotamisligil GS. Inflammation and metabolic disorders. Nature. (2006) 444:860–7. doi: 10.1038/nature05485
10. Mantovani A, Biswas SK, Galdiero MR, Sica A, Locati M. Macrophage plasticity and polarization in tissue repair and remodelling. J Pathol. (2013) 229:176–85. doi: 10.1002/path.2013.229.issue-2
11. Mantovani A, Sozzani S, Locati M, Allavena P, Sica A. Macrophage polarization: tumor-associated macrophages as a paradigm for polarized M2 mononuclear phagocytes. Trends Immunol. (2002) 23:549–55. doi: 10.1016/S1471-4906(02)02302-5
12. Qian BZ, Pollard JW. Macrophage diversity enhances tumor progression and metastasis. Cell. (2010) 141:39–51. doi: 10.1016/j.cell.2010.03.014
13. Beschin A, De Baetselier P, Van Ginderachter JA. Contribution of myeloid cell subsets to liver fibrosis in parasite infection. J Pathol. (2013) 229:186–97. doi: 10.1002/path.2013.229.issue-2
14. Noël W, Raes G, Hassanzadeh GG, De Baetselier P, Beschin A. Alternatively activated macrophages during parasite infections. Trends Parasitol. (2004) 20:126–33. doi: 10.1016/j.pt.2004.01.004
15. Hinz B, Phan SH, Thannickal VJ, Prunotto M, Desmoulière A, Varga J, et al. Recent developments in myofibroblast biology: paradigms for connective tissue remodeling. Am J Pathol. (2012) 180:1340–55. doi: 10.1016/j.ajpath.2012.02.004
16. Wynn TA. Fibrotic disease and the T(H)1/T(H)2 paradigm. Nat Rev Immunol. (2004) 4:583–94. doi: 10.1038/nri1412
17. Biswas SK, Mantovani A. Macrophage plasticity and interaction with lymphocyte subsets: cancer as a paradigm. Nat Immunol. (2010) 11:889–96. doi: 10.1038/ni.1937
18. Squadrito ML, De Palma M. Macrophage regulation of tumor angiogenesis: implications for cancer therapy. Mol Aspects Med. (2011) 32:123–45. doi: 10.1016/j.mam.2011.04.005
19. van Niel G, Carter D, Clayton A, Lambert DW, Raposo G, Vader P. Challenges and directions in studying cell-cell communication by extracellular vesicles. Nat Rev Mol Cell Biol. (2022) 23:369–82. doi: 10.1038/s41580-022-00460-3
20. Cui HY, Rong JS, Chen J, Guo J, Zhu JQ, Ruan M, et al. Exosomal microRNA-588 from M2 polarized macrophages contributes to cisplatin resistance of gastric cancer cells. World J Gastroenterol. (2021) 27:6079–92. doi: 10.3748/wjg.v27.i36.6079
21. Liu T, Liu M, Zheng C, Zhang D, Li M, Zhang L. Exosomal lncRNA CHL1-AS1 Derived from Peritoneal Macrophages Promotes the Progression of Endometriosis via the miR-610/MDM2 Axis. Int J Nanomedicine. (2021) 16:5451–64. doi: 10.2147/IJN.S323671
22. Zhang L, Li HH, Yuan M, Li D, Wang GY. Exosomal miR-22-3p derived from peritoneal macrophages enhances proliferation, migration, and invasion of ectopic endometrial stromal cells through regulation of the SIRT1/NF-κB signaling pathway. Eur Rev Med Pharmacol Sci. (2020) 24:571–80. doi: 10.26355/eurrev_202001_20033
23. Aucher A, Rudnicka D, Davis DM. MicroRNAs transfer from human macrophages to hepato-carcinoma cells and inhibit proliferation. J Immunol. (2013) 191:6250–60. doi: 10.4049/jimmunol.1301728
24. Jean-Toussaint R, Lin Z, Tian Y, Gupta R, Pande R, Luo X, et al. Therapeutic and prophylactic effects of macrophage-derived small extracellular vesicles in the attenuation of inflammatory pain. Brain Behav Immun. (2021) 94:210–24. doi: 10.1016/j.bbi.2021.02.005
25. Saleh NA, Rode MP, Cisilotto J, Silva AH, Prigol AN, Da LEF, et al. MicroRNA-mediated antiproliferative effects of M1 macrophage-derived extracellular vesicles on melanoma cells. Immunol Invest. (2023), 1–20. doi: 10.1080/08820139.2023.2278774
26. Chang J, Li H, Zhu Z, Mei P, Hu W, Xiong X, et al. microRNA-21-5p from M2 macrophage-derived extracellular vesicles promotes the differentiation and activity of pancreatic cancer stem cells by mediating KLF3. Cell Biol Toxicol. (2022) 38:577–90. doi: 10.1007/s10565-021-09597-x
27. Guo Y, Wu H, Xiong J, Gou S, Cui J, Peng T. miR-222-3p-containing macrophage-derived extracellular vesicles confer gemcitabine resistance via TSC1-mediated mTOR/AKT/PI3K pathway in pancreatic cancer. Cell Biol Toxicol. (2022) 39:1203–14. doi: 10.1007/s10565-022-09736-y
28. Théry C, Witwer KW, Aikawa E, Alcaraz MJ, Anderson JD, Andriantsitohaina R, et al. Minimal information for studies of extracellular vesicles 2018 (MISEV2018): a position statement of the International Society for Extracellular Vesicles and update of the MISEV2014 guidelines. J Extracell Vesicles. (2018) 7:1535750. doi: 10.1080/20013078.2018.1535750
29. Qin L, Yang J, Su X, Xilan L, Lei Y, Dong L, et al. The miR-21-5p enriched in the apoptotic bodies of M2 macrophage-derived extracellular vesicles alleviates osteoarthritis by changing macrophage phenotype. Genes Dis. (2023) 10:1114–29. doi: 10.1016/j.gendis.2022.09.010
30. Maas S, Breakefield XO, Weaver AM. Extracellular vesicles: unique intercellular delivery vehicles. Trends Cell Biol. (2017) 27:172–88. doi: 10.1016/j.tcb.2016.11.003
31. Mathieu M, Martin-Jaular L, Lavieu G, Théry C. Specificities of secretion and uptake of exosomes and other extracellular vesicles for cell-to-cell communication. Nat Cell Biol. (2019) 21:9–17. doi: 10.1038/s41556-018-0250-9
32. Raposo G, Stoorvogel W. Extracellular vesicles: exosomes, microvesicles, and friends. J Cell Biol. (2013) 200:373–83. doi: 10.1083/jcb.201211138
33. Atkin-Smith GK, Tixeira R, Paone S, Mathivanan S, Collins C, Liem M, et al. A novel mechanism of generating extracellular vesicles during apoptosis via a beads-on-a-string membrane structure. Nat Commun. (2015) 6:7439. doi: 10.1038/ncomms8439
34. van der Pol E, Coumans FA, Grootemaat AE, Gardiner C, Sargent IL, Harrison P, et al. Particle size distribution of exosomes and microvesicles determined by transmission electron microscopy, flow cytometry, nanoparticle tracking analysis, and resistive pulse sensing. J Thromb Haemost. (2014) 12:1182–92. doi: 10.1111/jth.12602
35. Shao H, Im H, Castro CM, Breakefield X, Weissleder R, Lee H. New technologies for analysis of extracellular vesicles. Chem Rev. (2018) 118:1917–50. doi: 10.1021/acs.chemrev.7b00534
36. Alvarez ML, Khosroheidari M, Kanchi RR, DiStefano JK. Comparison of protein, microRNA, and mRNA yields using different methods of urinary exosome isolation for the discovery of kidney disease biomarkers. Kidney Int. (2012) 82:1024–32. doi: 10.1038/ki.2012.256
37. Nordin JZ, Lee Y, Vader P, Mäger I, Johansson HJ, Heusermann W, et al. Ultrafiltration with size-exclusion liquid chromatography for high yield isolation of extracellular vesicles preserving intact biophysical and functional properties. Nanomedicine. (2015) 11:879–83. doi: 10.1016/j.nano.2015.01.003
38. Sitar S, Kejžar A, Pahovnik D, Kogej K, Tušek-Žnidarič M, Lenassi M, et al. Size characterization and quantification of exosomes by asymmetrical-flow field-flow fractionation. Anal Chem. (2015) 87:9225–33. doi: 10.1021/acs.analchem.5b01636
39. Gardiner C, Di Vizio D, Sahoo S, Théry C, Witwer KW, Wauben M, et al. Techniques used for the isolation and characterization of extracellular vesicles: results of a worldwide survey. J Extracell Vesicles. (2016) 5:32945. doi: 10.3402/jev.v5.32945
40. O'Brien K, Breyne K, Ughetto S, Laurent LC, Breakefield XO. RNA delivery by extracellular vesicles in mammalian cells and its applications. Nat Rev Mol Cell Biol. (2020) 21:585–606. doi: 10.1038/s41580-020-0251-y
41. Villarroya-Beltri C, Gutiérrez-Vázquez C, Sánchez-Cabo F, Pérez-Hernández D, Vázquez J, Martin-Cofreces N, et al. Sumoylated hnRNPA2B1 controls the sorting of miRNAs into exosomes through binding to specific motifs. Nat Commun. (2013) 4:2980. doi: 10.1038/ncomms3980
42. Shurtleff MJ, Temoche-Diaz MM, Karfilis KV, Ri S, Schekman R. Y-box protein 1 is required to sort microRNAs into exosomes in cells and in a cell-free reaction. Elife. (2016) 5:e19276. doi: 10.7554/eLife.19276
43. Zhang J, Li S, Li L, Li M, Guo C, Yao J, et al. Exosome and exosomal microRNA: trafficking, sorting, and function. Genomics Proteomics Bioinf. (2015) 13:17–24. doi: 10.1016/j.gpb.2015.02.001
45. Guiot J, Cambier M, Boeckx A, Henket M, Nivelles O, Gester F, et al. Macrophage-derived exosomes attenuate fibrosis in airway epithelial cells through delivery of antifibrotic miR-142-3p. Thorax. (2020) 75:870–81. doi: 10.1136/thoraxjnl-2019-214077
46. Kishore A, Petrek M. Roles of macrophage polarization and macrophage-derived miRNAs in pulmonary fibrosis. Front Immunol. (2021) 12:678457. doi: 10.3389/fimmu.2021.678457
47. Wang Y, Zhao M, Liu S, Guo J, Lu Y, Cheng J, et al. Macrophage-derived extracellular vesicles: diverse mediators of pathology and therapeutics in multiple diseases. Cell Death Dis. (2020) 11:924. doi: 10.1038/s41419-020-03127-z
48. Chen X, Wan Z, Yang L, Song S, Fu Z, Tang K, et al. Exosomes derived from reparative M2-like macrophages prevent bone loss in murine periodontitis models via IL-10 mRNA. J Nanobiotechnology. (2022) 20:110. doi: 10.1186/s12951-022-01314-y
49. Wang Y, Xu Z, Jiang J, Xu C, Kang J, Xiao L, et al. Endogenous miRNA sponge lincRNA-RoR regulates Oct4, Nanog, and Sox2 in human embryonic stem cell self-renewal. Dev Cell. (2013) 25:69–80. doi: 10.1016/j.devcel.2013.03.002
50. Wang J, Liu X, Wu H, Ni P, Gu Z, Qiao Y, et al. CREB up-regulates long non-coding RNA, HULC expression through interaction with microRNA-372 in liver cancer. Nucleic Acids Res. (2010) 38:5366–83. doi: 10.1093/nar/gkq285
51. Chen B, Luo L, Wei X, Gong D, Li Z, Li S, et al. M1 bone marrow-derived macrophage-derived extracellular vesicles inhibit angiogenesis and myocardial regeneration following myocardial infarction via the MALAT1/microRNA-25-3p/CDC42 axis. Oxid Med Cell Longev 2021. (2021), 9959746. doi: 10.1155/2021/9959746
52. Jiang H, Zhou L, Shen N, Ning X, Wu D, Jiang K, et al. M1 macrophage-derived exosomes and their key molecule lncRNA HOTTIP suppress head and neck squamous cell carcinoma progression by upregulating the TLR5/NF-κB pathway. Cell Death Dis. (2022) 13:183. doi: 10.1038/s41419-022-04640-z
53. Wang YX, Lin C, Cui LJ, Deng TZ, Li QM, Chen FY, et al. Mechanism of M2 macrophage-derived extracellular vesicles carrying lncRNA MEG3 in inflammatory responses in ulcerative colitis. Bioengineered. (2021) 12:12722–39. doi: 10.1080/21655979.2021.2010368
54. Zhang L, Zhang J, Li P, Li T, Zhou Z, Wu H. Exosomal hsa_circ_0004658 derived from RBPJ overexpressed-macrophages inhibits hepatocellular carcinoma progression via miR-499b-5p/JAM3. Cell Death Dis. (2022) 13:32. doi: 10.1038/s41419-021-04345-9
55. Fan L, Xu G, Zeng X. M2 macrophage-derived extracellular vesicles augment immune evasion and development of colorectal cancer via a circRNA_CCDC66/microRNA-342-3p/metadherin axis. Cytotechnology. (2023) 75:293–308. doi: 10.1007/s10616-023-00577-z
56. Gu X, Shi Y, Dong M, Jiang L, Yang J, Liu Z. Exosomal transfer of tumor-associated macrophage-derived hsa_circ_0001610 reduces radiosensitivity in endometrial cancer. Cell Death Dis. (2021) 12:818. doi: 10.1038/s41419-021-04087-8
57. Zheng P, Luo Q, Wang W, Li J, Wang T, Wang P, et al. Tumor-associated macrophages-derived exosomes promote the migration of gastric cancer cells by transfer of functional Apolipoprotein E. Cell Death Dis. (2018) 9:434. doi: 10.1038/s41419-018-0465-5
58. Mulcahy LA, Pink RC, Carter DR. Routes and mechanisms of extracellular vesicle uptake. J Extracell Vesicles. (2014) 3:24641. doi: 10.3402/jev.v3.24641
59. Haimovich G, Ecker CM, Dunagin MC, Eggan E, Raj A, Gerst JE, et al. Intercellular mRNA trafficking via membrane nanotube-like extensions in mammalian cells. Proc Natl Acad Sci U.S.A. (2017) 114:E9873–82. doi: 10.1073/pnas.1706365114
60. Fan J, Watanabe T. Atherosclerosis: known and unknown. Pathol Int. (2022) 72:151–60. doi: 10.1111/pin.13202
61. Fan J, Watanabe T. Inflammatory reactions in the pathogenesis of atherosclerosis. J Atheroscler Thromb. (2003) 10:63–71. doi: 10.5551/jat.10.63
62. Gimbrone MJ, García-Cardeña G. Endothelial cell dysfunction and the pathobiology of atherosclerosis. Circ Res. (2016) 118:620–36. doi: 10.1161/CIRCRESAHA.115.306301
63. Liu P, Wang S, Wang G, Zhao M, Du F, Li K, et al. Macrophage-derived exosomal miR-4532 promotes endothelial cells injury by targeting SP1 and NF-κB P65 signalling activation. J Cell Mol Med. (2022) 26:5165–80. doi: 10.1111/jcmm.v26.20
64. Huang C, Huang Y, Zhou Y, Nie W, Pu X, Xu X, et al. Exosomes derived from oxidized LDL-stimulated macrophages attenuate the growth and tube formation of endothelial cells. Mol Med Rep. (2018) 17:4605–10. doi: 10.3892/mmr.2018.8380
65. Liang W, Chen J, Zheng H, Lin A, Li J, Wu W, et al. MiR-199a-5p-containing macrophage-derived extracellular vesicles inhibit SMARCA4 and alleviate atherosclerosis by reducing endothelial cell pyroptosis. Cell Biol Toxicol. (2022) 39:591–605. doi: 10.1007/s10565-022-09732-2
66. Nguyen MA, Karunakaran D, Geoffrion M, Cheng HS, Tandoc K, Perisic ML, et al. Extracellular vesicles secreted by atherogenic macrophages transfer microRNA to inhibit cell migration. Arterioscler Thromb Vasc Biol. (2018) 38:49–63. doi: 10.1161/ATVBAHA.117.309795
67. Zhang YG, Song Y, Guo XL, Miao RY, Fu YQ, Miao CF, et al. Exosomes derived from oxLDL-stimulated macrophages induce neutrophil extracellular traps to drive atherosclerosis. Cell Cycle. (2019) 18:2674–84. doi: 10.1080/15384101.2019.1654797
68. Lee HD, Kim YH, Kim DS. Exosomes derived from human macrophages suppress endothelial cell migration by controlling integrin trafficking. Eur J Immunol. (2014) 44:1156–69. doi: 10.1002/eji.201343660
69. Li K, Cui M, Zhang K, Wang G, Zhai S. M1 macrophages-derived extracellular vesicles elevate microRNA-185-3p to aggravate the development of atherosclerosis in ApoE(-/-) mice by inhibiting small mothers against decapentaplegic 7. Int Immunopharmacol. (2021) 90:107138. doi: 10.1016/j.intimp.2020.107138
70. Wang Y, Xu Z, Wang X, Zheng J, Peng L, Zhou Y, et al. Extracellular-vesicle containing miRNA-503-5p released by macrophages contributes to atherosclerosis. Aging (Albany NY). (2021) 13:12239–57. doi: 10.18632/aging.103855
71. Cheng X, Zhou H, Zhou Y, Song C. M2 Macrophage-Derived Exosomes Inhibit Apoptosis of HUVEC Cell through Regulating miR-221-3p Expression. BioMed Res Int 2022. (2022) p:1609244. doi: 10.1155/2022/1609244
72. Gangadaran P, Rajendran RL, Oh JM, Hong CM, Jeong SY, Lee SW, et al. Extracellular vesicles derived from macrophage promote angiogenesis in vitro and accelerate new vasculature formation in vivo. Exp Cell Res. (2020) 394:112146. doi: 10.1016/j.yexcr.2020.112146
73. Chen F, Li J, She J, Chen T, Yuan Z. Exosomal microRNA-16-5p from macrophage exacerbates atherosclerosis via modulating mothers against decapentaplegic homolog 7. Microvasc Res. (2022) 142:104368. doi: 10.1016/j.mvr.2022.104368
74. Yaker L, Tebani A, Lesueur C, Dias C, Jung V, Bekri S, et al. Extracellular vesicles from LPS-treated macrophages aggravate smooth muscle cell calcification by propagating inflammation and oxidative stress. Front Cell Dev Biol. (2022) 10:823450. doi: 10.3389/fcell.2022.823450
75. Li Q, Zhang C, Shi J, Yang Y, Xing X, Wang Y, et al. High-Phosphate-Stimulated Macrophage-Derived Exosomes Promote Vascular Calcification via let-7b-5p/TGFBR1 Axis in Chronic Kidney Disease. Cells. (2022) 12:161. doi: 10.3390/cells12010161
76. New SE, Goettsch C, Aikawa M, Marchini JF, Shibasaki M, et al. Macrophage-derived matrix vesicles: an alternative novel mechanism for microcalcification in atherosclerotic plaques. Circ Res. (2013) 113:72–7. doi: 10.1161/CIRCRESAHA.113.301036
77. Niu C, Wang X, Zhao M, Cai T, Liu P, Li J, et al. Macrophage foam cell-derived extracellular vesicles promote vascular smooth muscle cell migration and adhesion. J Am Heart Assoc. (2016) 5. doi: 10.1161/JAHA.116.004099
78. Zhu J, Liu B, Wang Z, Wang D, Ni H, Zhang L, et al. Exosomes from nicotine-stimulated macrophages accelerate atherosclerosis through miR-21-3p/PTEN-mediated VSMC migration and proliferation. Theranostics. (2019) 9:6901–19. doi: 10.7150/thno.37357
79. Bouchareychas L, Duong P, Phu TA, Alsop E, Meechoovet B, Reiman R, et al. High glucose macrophage exosomes enhance atherosclerosis by driving cellular proliferation & hematopoiesis. iScience. (2021) 24:102847. doi: 10.1016/j.isci.2021.102847
80. Bouchareychas L, Duong P, Covarrubias S, Alsop E, Phu TA, Chung A, et al. Macrophage exosomes resolve atherosclerosis by regulating hematopoiesis and inflammation via microRNA cargo. Cell Rep. (2020) 32:107881. doi: 10.1016/j.celrep.2020.107881
81. Qi Y, Zhu T, Zhang T, Wang X, Li W, Chen D, et al. M1 macrophage-derived exosomes transfer miR-222 to induce bone marrow mesenchymal stem cell apoptosis. Lab Invest. (2021) 101:1318–26. doi: 10.1038/s41374-021-00622-5
82. Liu S, Chen J, Shi J, Zhou W, Wang L, Fang W, et al. M1-like macrophage-derived exosomes suppress angiogenesis and exacerbate cardiac dysfunction in a myocardial infarction microenvironment. Basic Res Cardiol. (2020) 115:22. doi: 10.1007/s00395-020-0781-7
83. Wang C, Zhang C, Liu L, A X, Chen B, Li Y, et al. Macrophage-Derived mir-155-Containing Exosomes Suppress Fibroblast Proliferation and Promote Fibroblast Inflammation during Cardiac Injury. Mol Ther. (2017) 25:192–204. doi: 10.1016/j.ymthe.2016.09.001
84. Carroll AP, Tooney PA, Cairns MJ. Context-specific microRNA function in developmental complexity. J Mol Cell Biol. (2013) 5:73–84. doi: 10.1093/jmcb/mjt004
85. Dai Y, Wang S, Chang S, Ren D, Shali S, Li C, et al. M2 macrophage-derived exosomes carry microRNA-148a to alleviate myocardial ischemia/reperfusion injury via inhibiting TXNIP and the TLR4/NF-κB/NLRP3 inflammasome signaling pathway. J Mol Cell Cardiol. (2020) 142:65–79. doi: 10.1016/j.yjmcc.2020.02.007
86. Li L, Cao J, Li S, Cui T, Ni J, Zhang H, et al. M2 macrophage-derived sEV regulate pro-inflammatory CCR2(+) macrophage subpopulations to favor post-AMI cardiac repair. Adv Sci (Weinh). (2023) 10:e2202964. doi: 10.1002/advs.202202964
87. Liu Y, Zhang WL, Gu JJ, Sun YQ, Cui HZ, Bu JQ, et al. Exosome-mediated miR-106a-3p derived from ox-LDL exposed macrophages accelerated cell proliferation and repressed cell apoptosis of human vascular smooth muscle cells. Eur Rev Med Pharmacol Sci. (2020) 24:7039–50. doi: 10.26355/eurrev_202006_21697
88. Wang Y, Li C, Zhao R, Qiu Z, Shen C, Wang Z, et al. CircUbe3a from M2 macrophage-derived small extracellular vesicles mediates myocardial fibrosis after acute myocardial infarction. Theranostics. (2021) 11:6315–33. doi: 10.7150/thno.52843
89. de Luca C, Olefsky JM. Inflammation and insulin resistance. FEBS Lett. (2008) 582:97–105. doi: 10.1016/j.febslet.2007.11.057
90. Ying W, Riopel M, Bandyopadhyay G, Dong Y, Birmingham A, Seo JB, et al. Adipose tissue macrophage-derived exosomal miRNAs can modulate in vivo and in vitro insulin sensitivity. Cell. (2017) 171:372–384.e12. doi: 10.1016/j.cell.2017.08.035
91. Liu T, Sun YC, Cheng P, Shao HG. Adipose tissue macrophage-derived exosomal miR-29a regulates obesity-associated insulin resistance. Biochem Biophys Res Commun. (2019) 515:352–8. doi: 10.1016/j.bbrc.2019.05.113
92. Xiong H, Liu W, Song J, Gu X, Luo S, Lu Z, et al. Adipose tissue macrophage-derived exosomal miR-210-5p in modulating insulin sensitivity in rats born small for gestational age with catch-up growth. Transl Pediatr. (2023) 12:587–99. doi: 10.21037/tp-23-142
93. Li L, Zuo H, Huang X, Shen T, Tang W, Zhang X, et al. Bone marrow macrophage-derived exosomal miR-143-5p contributes to insulin resistance in hepatocytes by repressing MKP5. Cell Prolif. (2021) 54:e13140. doi: 10.1111/cpr.v54.12
94. Ying W, Gao H, Dos RF, Bandyopadhyay G, Ofrecio JM, Luo Z, et al. MiR-690, an exosomal-derived miRNA from M2-polarized macrophages, improves insulin sensitivity in obese mice. Cell Metab. (2021) 33:781–790.e5. doi: 10.1016/j.cmet.2020.12.019
95. Shyu KG, Wang BW, Fang WJ, Pan CM, Lin CM. Exosomal MALAT1 Derived from High Glucose-Treated Macrophages Up-Regulates Resistin Expression via miR-150-5p Downregulation. Int J Mol Sci. (2022) 23:1095. doi: 10.3390/ijms23031095
96. Tian F, Tang P, Sun Z, Zhang R, Zhu D, He J, et al. miR-210 in exosomes derived from macrophages under high glucose promotes mouse diabetic obesity pathogenesis by suppressing NDUFA4 Expression. J Diabetes Res 2020. (2020), 6894684. doi: 10.1155/2020/6894684
97. Phu TA, Ng M, Vu NK, Bouchareychas L, Raffai RL. IL-4 polarized human macrophage exosomes control cardiometabolic inflammation and diabetes in obesity. Mol Ther. (2022) 30:2274–97. doi: 10.1016/j.ymthe.2022.03.008
98. Zhang Y, Shi L, Mei H, Zhang J, Zhu Y, Han X, et al. Inflamed macrophage microvesicles induce insulin resistance in human adipocytes. Nutr Metab (Lond). (2015) 12:21. doi: 10.1186/s12986-015-0016-3
99. Li JM, Li X, Chan L, Hu R, Zheng T, Li H, et al. Lipotoxicity-polarised macrophage-derived exosomes regulate mitochondrial fitness through Miro1-mediated mitophagy inhibition and contribute to type 2 diabetes development in mice. Diabetologia. (2023) 66:2368–86. doi: 10.1007/s00125-023-05992-7
100. Saltiel AR, Olefsky JM. Inflammatory mechanisms linking obesity and metabolic disease. J Clin Invest. (2017) 127:1–4. doi: 10.1172/JCI92035
101. Qian B, Yang Y, Tang N, Wang J, Sun P, Yang N, et al. M1 macrophage-derived exosomes impair beta cell insulin secretion via miR-212-5p by targeting SIRT2 and inhibiting Akt/GSK-3β/β-catenin pathway in mice. Diabetologia. (2021) 64:2037–51. doi: 10.1007/s00125-021-05489-1
102. De Silva N, Samblas M, Martínez JA, Milagro FI. Effects of exosomes from LPS-activated macrophages on adipocyte gene expression, differentiation, and insulin-dependent glucose uptake. J Physiol Biochem. (2018) 74:559–68. doi: 10.1007/s13105-018-0622-4
103. Zhao X, Si L, Bian J, Pan C, Guo W, Qin P, et al. Adipose tissue macrophage-derived exosomes induce ferroptosis via glutathione synthesis inhibition by targeting SLC7A11 in obesity-induced cardiac injury. Free Radic Biol Med. (2022) 182:232–45. doi: 10.1016/j.freeradbiomed.2022.02.033
104. Ding X, Jing N, Shen A, Guo F, Song Y, Pan M, et al. MiR-21-5p in macrophage-derived extracellular vesicles affects podocyte pyroptosis in diabetic nephropathy by regulating A20. J Endocrinol Invest. (2021) 44:1175–84. doi: 10.1007/s40618-020-01401-7
105. Liu Y, Li X, Zhao M, Wu Y, Xu Y, Li X, et al. Macrophage-derived exosomes promote activation of NLRP3 inflammasome and autophagy deficiency of mesangial cells in diabetic nephropathy. Life Sci. (2023) 330:121991. doi: 10.1016/j.lfs.2023.121991
106. Zhu QJ, Zhu M, Xu XX, Meng XM, Wu YG. Exosomes from high glucose-treated macrophages activate glomerular mesangial cells via TGF-β1/Smad3 pathway in vivo and in vitro. FASEB J. (2019) 33:9279–90. doi: 10.1096/fj.201802427RRR
107. Zhao J, Chen J, Zhu W, Qi XM, Wu YG. Exosomal miR-7002-5p derived from highglucose-induced macrophages suppresses autophagy in tubular epithelial cells by targeting Atg9b. FASEB J. (2022) 36:12:e22501. doi: 10.1096/fj.202200550RR
108. Huang H, Liu H, Tang J, Xu W, Gan H, Fan Q, et al. M2 macrophage-derived exosomal miR-25-3p improves high glucose-induced podocytes injury through activation autophagy via inhibiting DUSP1 expression. IUBMB Life. (2020) 72:2651–62. doi: 10.1002/iub.v72.12
109. Wang J, Han Y, Huang F, Tang L, Mu J, Liang Y. Diabetic macrophage small extracellular vesicles-associated miR-503/IGF1R axis regulates endothelial cell function and affects wound healing. Front Immunol. (2023) 14:1104890. doi: 10.3389/fimmu.2023.1104890
110. Zhang D, Wu Y, Li Z, Chen H, Huang S, Jian C, et al. MiR-144-5p, an exosomal miRNA from bone marrow-derived macrophage in type 2 diabetes, impairs bone fracture healing via targeting Smad1. J Nanobiotechnology. (2021) 19:226. doi: 10.1186/s12951-021-00964-8
111. Li M, Wang T, Tian H, Wei G, Zhao L, Shi Y. Macrophage-derived exosomes accelerate wound healing through their anti-inflammation effects in a diabetic rat model. Artif Cells Nanomed Biotechnol. (2019) 47:3793–803. doi: 10.1080/21691401.2019.1669617
112. Xia W, Liu Y, Jiang X, Li M, Zheng S, Zhang Z, et al. Lean adipose tissue macrophage derived exosome confers immunoregulation to improve wound healing in diabetes. J Nanobiotechnology. (2023) 21:128. doi: 10.1186/s12951-023-01869-4
113. Wang Y, Lin Q, Zhang H, Wang S, Cui J, Hu Y, et al. M2 macrophage-derived exosomes promote diabetic fracture healing by acting as an immunomodulator. Bioact Mater. (2023) 28:273–83. doi: 10.1016/j.bioactmat.2023.05.018
114. Lopez-Yrigoyen M, Cassetta L, Pollard JW. Macrophage targeting in cancer. Ann N Y Acad Sci. (2021) 1499:18–41. doi: 10.1111/nyas.v1499.1
115. Wang J, Li D, Cang H, Guo B. Crosstalk between cancer and immune cells: Role of tumor-associated macrophages in the tumor microenvironment. Cancer Med. (2019) 8:4709–21. doi: 10.1002/cam4.v8.10
116. Sousa S, Brion R, Lintunen M, Kronqvist P, Sandholm J, Mönkkönen J, et al. Human breast cancer cells educate macrophages toward the M2 activation status. Breast Cancer Res. (2015) 17:101. doi: 10.1186/s13058-015-0621-0
117. Zhou K, Cheng T, Zhan J, Peng X, Zhang Y, Wen J, et al. Targeting tumor-associated macrophages in the tumor microenvironment. Oncol Lett. (2020) 20:234. doi: 10.3892/ol.2020.12097
118. Chen S, Cao Q, Wen W, Wang H. Targeted therapy for hepatocellular carcinoma: Challenges and opportunities. Cancer Lett. (2019) 460:1–9. doi: 10.1016/j.canlet.2019.114428
119. Li W, Xin X, Li X, Geng J, Sun Y. Exosomes secreted by M2 macrophages promote cancer stemness of hepatocellular carcinoma via the miR-27a-3p/TXNIP pathways. Int Immunopharmacol. (2021) 101:107585. doi: 10.1016/j.intimp.2021.107585
120. Tian B, Zhou L, Wang J, Yang P. miR-660-5p-loaded M2 macrophages-derived exosomes augment hepatocellular carcinoma development through regulating KLF3. Int Immunopharmacol. (2021) 101:108157. doi: 10.1016/j.intimp.2021.108157
121. Pu J, Xu Z, Nian J, Fang Q, Yang M, Huang Y, et al. M2 macrophage-derived extracellular vesicles facilitate CD8+T cell exhaustion in hepatocellular carcinoma via the miR-21-5p/YOD1/YAP/β-catenin pathway. Cell Death Discovery. (2021) 7:182. doi: 10.1038/s41420-021-00556-3
122. Lu Y, Han G, Zhang Y, Zhang L, Li Z, Wang Q, et al. M2 macrophage-secreted exosomes promote metastasis and increase vascular permeability in hepatocellular carcinoma. Cell Commun Signal. (2023) 21:299. doi: 10.1186/s12964-022-00872-w
123. Liu G, Ouyang X, Sun Y, Xiao Y, You B, Gao Y, et al. The miR-92a-2-5p in exosomes from macrophages increases liver cancer cells invasion via altering the AR/PHLPP/p-AKT/β-catenin signaling. Cell Death Differ. (2020) 27:3258–72. doi: 10.1038/s41418-020-0575-3
124. Xu H, Zhu J, Smith S, Foldi J, Zhao B, Chung AY, et al. Notch-RBP-J signaling regulates the transcription factor IRF8 to promote inflammatory macrophage polarization. Nat Immunol. (2012) 13:642–50. doi: 10.1038/ni.2304
125. Oser MG, Niederst MJ, Sequist LV, Engelman JA. Transformation from non-small-cell lung cancer to small-cell lung cancer: molecular drivers and cells of origin. Lancet Oncol. (2015) 16:e165–72. doi: 10.1016/S1470-2045(14)71180-5
126. Siegel RL, Miller KD, Jemal A. Cancer statistics, 2017. CA Cancer J Clin. (2017) 67:7–30. doi: 10.3322/caac.21387
127. Wang X, Huang R, Lu Z, Wang Z, Chen X, Huang D. Exosomes from M1-polarized macrophages promote apoptosis in lung adenocarcinoma via the miR-181a-5p/ETS1/STK16 axis. Cancer Sci. (2022) 113:986–1001. doi: 10.1111/cas.v113.3
128. Chen J, Zhang K, Zhi Y, Wu Y, Chen B, Bai J, et al. Tumor-derived exosomal miR-19b-3p facilitates M2 macrophage polarization and exosomal LINC00273 secretion to promote lung adenocarcinoma metastasis via Hippo pathway. Clin Transl Med. (2021) 11:e478. doi: 10.1002/ctm2.v11.9
129. Lei J, Chen P, Zhang F, Zhang N, Zhu J, Wang X, et al. M2 macrophages-derived exosomal microRNA-501-3p promotes the progression of lung cancer via targeting WD repeat domain 82. Cancer Cell Int. (2021) 21:91. doi: 10.1186/s12935-021-01783-5
130. Wei K, Ma Z, Yang F, Zhao X, Jiang W, Pan C, et al. M2 macrophage-derived exosomes promote lung adenocarcinoma progression by delivering miR-942. Cancer Lett. (2022) 526:205–16. doi: 10.1016/j.canlet.2021.10.045
131. Guan B, Dai X, Zhu Y, Geng Q. M2 macrophage-derived exosomal miR-1911-5p promotes cell migration and invasion in lung adenocarcinoma by down-regulating CELF2 -activated ZBTB4 expression. Anticancer Drugs. (2023) 34:238–47. doi: 10.1097/CAD.0000000000001414
132. Liu C, Liang T, Zhang Z, Chen J, Xue J, Zhan X, et al. Transfer of microRNA-22-3p by M2 macrophage-derived extracellular vesicles facilitates the development of ankylosing spondylitis through the PER2-mediated Wnt/β-catenin axis. Cell Death Discovery. (2022) 8:269. doi: 10.1038/s41420-022-00900-1
133. Song L, Luan B, Xu Q, Shi R, Wang X. microRNA-155-3p delivered by M2 macrophages-derived exosomes enhances the progression of medulloblastoma through regulation of WDR82. J Transl Med. (2022) 20:13. doi: 10.1186/s12967-021-03156-y
134. Zheng N, Wang T, Luo Q, Liu Y, Yang J, Zhou Y, et al. M2 macrophage-derived exosomes suppress tumor intrinsic immunogenicity to confer immunotherapy resistance. Oncoimmunology. (2023) 12:2210959. doi: 10.1080/2162402X.2023.2210959
135. Wang H, Wang L, Pan H, Wang Y, Shi M, Yu H, et al. Exosomes Derived From Macrophages Enhance Aerobic Glycolysis and Chemoresistance in Lung Cancer by Stabilizing c-Myc via the Inhibition of NEDD4L. Front Cell Dev Biol. (2020) 8:620603. doi: 10.3389/fcell.2020.620603
136. Zhang F, Sang Y, Chen D, Wu X, Wang X, Yang W, et al. M2 macrophage-derived exosomal long non-coding RNA AGAP2-AS1 enhances radiotherapy immunity in lung cancer by reducing microRNA-296 and elevating NOTCH2. Cell Death Dis. (2021) 12:467. doi: 10.1038/s41419-021-03700-0
137. Zhang Y. Epidemiology of esophageal cancer. World J Gastroenterol. (2013) 19:5598–606. doi: 10.3748/wjg.v19.i34.5598
138. Smyth EC, Nilsson M, Grabsch HI, van Grieken NC, Lordick F. Gastric cancer. Lancet. (2020) 396:635–48. doi: 10.1016/S0140-6736(20)31288-5
139. Mi X, Xu R, Hong S, Xu T, Zhang W, Liu M. M2 macrophage-derived exosomal lncRNA AFAP1-AS1 and microRNA-26a affect cell migration and metastasis in esophageal cancer. Mol Ther Nucleic Acids. (2020) 22:779–90. doi: 10.1016/j.omtn.2020.09.035
140. Qiao X, Cheng Z, Xue K, Xiong C, Zheng Z, Jin X, et al. Tumor-associated macrophage-derived exosomes LINC01592 induce the immune escape of esophageal cancer by decreasing MHC-I surface expression. J Exp Clin Cancer Res. (2023) 42:289. doi: 10.1186/s13046-023-02871-2
141. Li Z, Suo B, Long G, Gao Y, Song J, Zhang M, et al. Exosomal miRNA-16-5p derived from M1 macrophages enhances T cell-dependent immune response by regulating PD-L1 in gastric cancer. Front Cell Dev Biol. (2020) 8:572689. doi: 10.3389/fcell.2020.572689
142. Wang Y, Shang K, Zhang N, Zhao J, Cao B. Tumor-associated macrophage-derived exosomes promote the progression of gastric cancer by regulating the P38MAPK signaling pathway and the immune checkpoint PD-L1. Cancer Biother Radiopharm. (2021). doi: 10.1089/cbr.2021.0218
143. Yang X, Cai S, Shu Y, Deng X, Zhang Y, He N, et al. Exosomal miR-487a derived from m2 macrophage promotes the progression of gastric cancer. Cell Cycle. (2021) 20:434–44. doi: 10.1080/15384101.2021.1878326
144. Zheng P, Chen L, Yuan X, Luo Q, Liu Y, Xie G, et al. Exosomal transfer of tumor-associated macrophage-derived miR-21 confers cisplatin resistance in gastric cancer cells. J Exp Clin Cancer Res. (2017) 36:53. doi: 10.1186/s13046-017-0528-y
145. Gao H, Ma J, Cheng Y, Zheng P. Exosomal transfer of macrophage-derived miR-223 confers doxorubicin resistance in gastric cancer. Onco Targets Ther. (2020) 13:12169–79. doi: 10.2147/OTT.S283542
146. Murad AM, Santiago FF, Petroianu A, Rocha PR, Rodrigues MA, Rausch M. Modified therapy with 5-fluorouracil, doxorubicin, and methotrexate in advanced gastric cancer. Cancer. (1993) 72:37–41. doi: 10.1002/1097-0142(19930701)72:1<37::AID-CNCR2820720109>3.0.CO;2-P
147. Xu X, Cheng J, Luo S, Gong X, Huang D, Xu J, et al. Deoxycholic acid-stimulated macrophage-derived exosomes promote spasmolytic polypeptide-expressing metaplasia in the stomach. Biochem Biophys Res Commun. (2020) 524:649–55. doi: 10.1016/j.bbrc.2020.01.159
148. Xu X, Cheng J, Luo S, Huang D, Xu J, Qian Y, et al. Deoxycholic acid-stimulated macrophage-derived exosomes promote intestinal metaplasia and suppress proliferation in human gastric epithelial cells. Eur J Pharmacol. (2020) 887:173566. doi: 10.1016/j.ejphar.2020.173566
149. McGuigan A, Kelly P, Turkington RC, Jones C, Coleman HG, McCain RS. Pancreatic cancer: A review of clinical diagnosis, epidemiology, treatment and outcomes. World J Gastroenterol. (2018) 24:4846–61. doi: 10.3748/wjg.v24.i43.4846
150. Hsieh MJ, Chiu TJ, Lin YC, Weng CC, Weng YT, Hsiao CC, et al. Inactivation of APC induces CD34 upregulation to promote epithelial-mesenchymal transition and cancer stem cell traits in pancreatic cancer. Int J Mol Sci. (2020) 21:4473. doi: 10.3390/ijms21124473
151. Yin Z, Zhou Y, Ma T, Chen S, Shi N, Zou Y, et al. Down-regulated lncRNA SBF2-AS1 in M2 macrophage-derived exosomes elevates miR-122-5p to restrict XIAP, thereby limiting pancreatic cancer development. J Cell Mol Med. (2020) 24:5028–38. doi: 10.1111/jcmm.15125
152. Zhang K, Li YJ, Peng LJ, Gao HF, Liu LM, Chen H. M2 macrophage-derived exosomal miR-193b-3p promotes progression and glutamine uptake of pancreatic cancer by targeting TRIM62. Biol Direct. (2023) 18:1. doi: 10.1186/s13062-023-00356-y
153. Yang Y, Guo Z, Chen W, Wang X, Cao M, Han X, et al. M2 macrophage-derived exosomes promote angiogenesis and growth of pancreatic ductal adenocarcinoma by targeting E2F2. Mol Ther. (2021) 29:1226–38. doi: 10.1016/j.ymthe.2020.11.024
154. Binenbaum Y, Fridman E, Yaari Z, Milman N, Schroeder A, Ben DG, et al. Transfer of miRNA in macrophage-derived exosomes induces drug resistance in pancreatic adenocarcinoma. Cancer Res. (2018) 78:5287–99. doi: 10.1158/0008-5472.CAN-18-0124
155. Li X, Xu H, Yi J, Dong C, Zhang H, Wang Z, et al. miR-365 secreted from M2 Macrophage-derived extracellular vesicles promotes pancreatic ductal adenocarcinoma progression through the BTG2/FAK/AKT axis. J Cell Mol Med. (2021) 25:4671–83. doi: 10.1111/jcmm.v25.10
156. Chien CW, Hou PC, Wu HC, Chang YL, Lin SC, Lin SC, et al. Targeting TYRO3 inhibits epithelial-mesenchymal transition and increases drug sensitivity in colon cancer. Oncogene. (2016) 35:5872–81. doi: 10.1038/onc.2016.120
157. Ning J, Hou X, Hao J, Zhang W, Shi Y, Huang Y, et al. METTL3 inhibition induced by M2 macrophage-derived extracellular vesicles drives anti-PD-1 therapy resistance via M6A-CD70-mediated immune suppression in thyroid cancer. Cell Death Differ. (2023) 30:2265–79. doi: 10.1038/s41418-023-01217-x
158. Feng Z, Zhang X, Li L, Wang C, Feng M, Zhao K, et al. Tumor-associated macrophage-derived exosomal microRNA-155-5p stimulates intracranial aneurysm formation and macrophage infiltration. Clin Sci (Lond). (2019) 133:2265–82. doi: 10.1042/CS20190680
159. Lan J, Sun L, Xu F, Liu L, Hu F, Song D, et al. M2 macrophage-derived exosomes promote cell migration and invasion in colon cancer. Cancer Res. (2019) 79:146–58. doi: 10.1158/0008-5472.CAN-18-0014
160. Ma YS, Wu TM, Ling CC, Yu F, Zhang J, Cao PS, et al. M2 macrophage-derived exosomal microRNA-155-5p promotes the immune escape of colon cancer by downregulating ZC3H12B. Mol Ther Oncolytics. (2021) 20:484–98. doi: 10.1016/j.omto.2021.02.005
161. Yin Z, Ma T, Huang B, Lin L, Zhou Y, Yan J, et al. Macrophage-derived exosomal microRNA-501-3p promotes progression of pancreatic ductal adenocarcinoma through the TGFBR3-mediated TGF-β signaling pathway. J Exp Clin Cancer Res. (2019) 38:310. doi: 10.1186/s13046-019-1313-x
162. Ding Y, Zhao H, Niu W, Zhang J, Zheng X, Liu Y, et al. M2 macrophage-derived extracellular vesicles containing microRNA-501-3p promote colon cancer progression through the SETD7/DNMT1/SOCS3 axis. Dis Colon Rectum. (2023) 66:e1234–45. doi: 10.1097/DCR.0000000000002986
163. Guo J, Wang X, Guo Q, Zhu S, Li P, Zhang S, et al. M2 macrophage derived extracellular vesicle-mediated transfer of miR-186-5p promotes colon cancer progression by targeting DLC1. Int J Biol Sci. (2022) 18:1663–76. doi: 10.7150/ijbs.69405
164. Zhang S, Li D, Zhao M, Yang F, Sang C, Yan C, et al. Exosomal miR-183-5p Shuttled by M2 Polarized Tumor-Associated Macrophage Promotes the Development of Colon Cancer via Targeting THEM4 Mediated PI3K/AKT and NF-κB Pathways. Front Oncol. (2021) 11:672684. doi: 10.3389/fonc.2021.672684
165. Zhou W, Li S, Zhang X, Li C, Zhang J. miR-143-3p shuttled by M2 macrophage-derived extracellular vesicles induces progression of colorectal cancer through a ZC3H12A/C/EBPβ axis-dependent mechanism. Int Immunopharmacol. (2023) 119:110137. doi: 10.1016/j.intimp.2023.110137
166. Huang S, Fan P, Zhang C, Xie J, Gu X, Lei S, et al. Exosomal microRNA-503-3p derived from macrophages represses glycolysis and promotes mitochondrial oxidative phosphorylation in breast cancer cells by elevating DACT2. Cell Death Discovery. (2021) 7:119. doi: 10.1038/s41420-021-00492-2
167. Li C, Li R, Hu X, Zhou G, Jiang G. Tumor-promoting mechanisms of macrophage-derived extracellular vesicles-enclosed microRNA-660 in breast cancer progression. Breast Cancer Res Treat. (2022) 192:353–68. doi: 10.1007/s10549-021-06433-y
168. Yang M, Chen J, Su F, Yu B, Su F, Lin L, et al. Microvesicles secreted by macrophages shuttle invasion-potentiating microRNAs into breast cancer cells. Mol Cancer. (2011) 10:117. doi: 10.1186/1476-4598-10-117
169. Zhu X, Shen H, Yin X, Yang M, Wei H, Chen Q, et al. Macrophages derived exosomes deliver miR-223 to epithelial ovarian cancer cells to elicit a chemoresistant phenotype. J Exp Clin Cancer Res. (2019) 38:81. doi: 10.1186/s13046-019-1095-1
170. Li X, Tang M. Exosomes released from M2 macrophages transfer miR-221-3p contributed to EOC progression through targeting CDKN1B. Cancer Med. (2020) 9:5976–88. doi: 10.1002/cam4.v9.16
171. Chen C, Zhang L, Ruan Z. GATA3 encapsulated by tumor-associated macrophage-derived extracellular vesicles promotes immune escape and chemotherapy resistance of ovarian cancer cells by upregulating the CD24/siglec-10 axis. Mol Pharm. (2023) 20:971–86. doi: 10.1021/acs.molpharmaceut.2c00557
172. Lu L, Ling W, Ruan Z. TAM-derived extracellular vesicles containing microRNA-29a-3p explain the deterioration of ovarian cancer. Mol Ther Nucleic Acids. (2021) 25:468–82. doi: 10.1016/j.omtn.2021.05.011
173. Sui HY, Cui XY, Shi CX, Yan ZP, Li HC, Wang F. LRRC75A-AS1 delivered by M2 macrophage exosomes promotes cervical cancer progression via enhancing SIX1 expression. Cancer Sci. (2023) 114:2634–49. doi: 10.1111/cas.v114.6
174. Mehedintu C, Plotogea MN, Ionescu S, Antonovici M. Endometriosis still a challenge. J Med Life. (2014) 7:349–57.
175. Yan P, Wang J, Liu H, Liu X, Fu R, Feng J. M1 macrophage-derived exosomes containing miR-150 inhibit glioma progression by targeting MMP16. Cell Signal. (2023) 108:110731. doi: 10.1016/j.cellsig.2023.110731
176. Yuan Y, Wang Z, Chen M, Jing Y, Shu W, Xie Z, et al. Macrophage-derived exosomal miR-31-5p promotes oral squamous cell carcinoma tumourigenesis through the large tumor suppressor 2-mediated hippo signalling pathway. J BioMed Nanotechnol. (2021) 17:822–37. doi: 10.1166/jbn.2021.3066
177. Tomita R, Sasabe E, Tomomura A, Yamamoto T. Macrophage-derived exosomes attenuate the susceptibility of oral squamous cell carcinoma cells to chemotherapeutic drugs through the AKT/GSK-3β pathway. Oncol Rep. (2020) 44:1905–16. doi: 10.3892/or.2020.7748
178. Zhang Z, Xu J, Chen Z, Wang H, Xue H, Yang C, et al. Transfer of microRNA via macrophage-derived extracellular vesicles promotes proneural-to-mesenchymal transition in glioma stem cells. Cancer Immunol Res. (2020) 8:966–81. doi: 10.1158/2326-6066.CIR-19-0759
179. Yao J, Wang Z, Cheng Y, Ma C, Zhong Y, Xiao Y, et al. M2 macrophage-derived exosomal microRNAs inhibit cell migration and invasion in gliomas through PI3K/AKT/mTOR signaling pathway. J Transl Med. (2021) 19:99. doi: 10.1186/s12967-021-02766-w
180. Fu XH, Li JP, Li XY, Tan Y, Zhao M, Zhang SF, et al. M2-macrophage-derived exosomes promote meningioma progression through TGF-β Signaling pathway. J Immunol Res. (2022) 2022:8326591. doi: 10.1155/2022/8326591
181. Du T, Yang CL, Ge MR, Liu Y, Zhang P, Li H, et al. M1 macrophage derived exosomes aggravate experimental autoimmune neuritis via modulating th1 response. Front Immunol. (2020) 11:1603. doi: 10.3389/fimmu.2020.01603
182. Goulielmaki E, Ioannidou A, Tsekrekou M, Stratigi K, Poutakidou IK, Gkirtzimanaki K, et al. Tissue-infiltrating macrophages mediate an exosome-based metabolic reprogramming upon DNA damage. Nat Commun. (2020) 11:42. doi: 10.1038/s41467-019-13894-9
183. Qu Y, Xu Y, Jiang Y, Yu D, Jiang X, Zhao L. Macrophage-derived extracellular vesicles regulates USP5-mediated HDAC2/NRF2 axis to ameliorate inflammatory pain. FASEB J. (2021) 35:e21332. doi: 10.1096/fj.202001185RR
184. Lu J, Liu D, Tan Y, Deng F, Li R. M1 Macrophage exosomes MiR-21a-5p aggravates inflammatory bowel disease through decreasing E-cadherin and subsequent ILC2 activation. J Cell Mol Med. (2021) 25:3041–50. doi: 10.1111/jcmm.16348
185. Chang X, Song YH, Xia T, He ZX, Zhao SB, Wang ZJ, et al. Macrophage-derived exosomes promote intestinal mucosal barrier dysfunction in inflammatory bowel disease by regulating TMIGD1 via mircroRNA-223. Int Immunopharmacol. (2023) 121:110447. doi: 10.1016/j.intimp.2023.110447
186. Deng F, Yan J, Lu J, Luo M, Xia P, Liu S, et al. M2 Macrophage-Derived Exosomal miR-590-3p Attenuates DSS-Induced Mucosal Damage and Promotes Epithelial Repair via the LATS1/YAP/ β-Catenin Signalling Axis. J Crohns Colitis. (2021) 15:665–77. doi: 10.1093/ecco-jcc/jjaa214
187. Han D, Lu D, Huang S, Pang J, Wu Y, Hu J, et al. Small extracellular vesicles from Ptpn1-deficient macrophages alleviate intestinal inflammation by reprogramming macrophage polarization via lactadherin enrichment. Redox Biol. (2022) 58:102558. doi: 10.1016/j.redox.2022.102558
188. Cai X, Xi X, Li X, Zhang X, Zhang X, Huang Z, et al. Antinociceptive effects of macrophage-derived extracellular vesicles by carrying microRNA-216a. Am J Transl Res. (2021) 13:1971–89.
189. Da-Wa ZX, Jun M, Chao-Zheng L, Sen-Lin Y, Chuan L, De-Chun L, et al. Exosomes derived from M2 macrophages exert a therapeutic effect via inhibition of the PI3K/AKT/mTOR pathway in rats with knee osteoarthritic. BioMed Res Int. (2021) 2021:7218067. doi: 10.1155/2021/7218067
190. Sun K, Luo J, Guo J, Yao X, Jing X, Guo F. The PI3K/AKT/mTOR signaling pathway in osteoarthritis: a narrative review. Osteoarthritis Cartilage. (2020) 28:400–9. doi: 10.1016/j.joca.2020.02.027
191. Zhang B, Lin F, Dong J, Liu J, Ding Z, Xu J. Peripheral Macrophage-derived Exosomes promote repair after Spinal Cord Injury by inducing Local Anti-inflammatory type Microglial Polarization via Increasing Autophagy. Int J Biol Sci. (2021) 17:1339–52. doi: 10.7150/ijbs.54302
192. Wang Y, Jia L, Xie Y, Cai Z, Liu Z, Shen J, et al. Involvement of macrophage-derived exosomes in abdominal aortic aneurysms development. Atherosclerosis. (2019) 289:64–72. doi: 10.1016/j.atherosclerosis.2019.08.016
193. Jiao Y, Li Z, Loughran PA, Fan EK, Scott MJ, Li Y, et al. Frontline Science: Macrophage-derived exosomes promote neutrophil necroptosis following hemorrhagic shock. J Leukoc Biol. (2018) 103:175–83. doi: 10.1189/jlb.3HI0517-173R
194. Holder B, Jones T, Sancho SV, Rice TF, Donaldson B, Bouqueau M, et al. Macrophage exosomes induce placental inflammatory cytokines: A novel mode of maternal-placental messaging. Traffic. (2016) 17:168–78. doi: 10.1111/tra.2016.17.issue-2
195. Rice TF, Donaldson B, Bouqueau M, Kampmann B, Holder B. Macrophage- but not monocyte-derived extracellular vesicles induce placental pro-inflammatory responses. Placenta. (2018) 69:92–5. doi: 10.1016/j.placenta.2018.07.011
196. Li Q, Yuan M, Jiao X, Huang Y, Li J, Li D, et al. M1 macrophage-derived nanovesicles repolarize M2 macrophages for inhibiting the development of endometriosis. Front Immunol. (2021) 12:707784. doi: 10.3389/fimmu.2021.707784
197. Xiao Y, Peng X, Peng Y, Zhang C, Liu W, Yang W, et al. Macrophage-derived extracellular vesicles regulate follicular activation and improve ovarian function in old mice by modulating local environment. Clin Transl Med. (2022) 12:e1071. doi: 10.1002/ctm2.v12.10
198. Pajarinen J, Lin T, Gibon E, Kohno Y, Maruyama M, Nathan K, et al. Mesenchymal stem cell-macrophage crosstalk and bone healing. Biomaterials. (2019) 196:80–9. doi: 10.1016/j.biomaterials.2017.12.025
199. Schlundt C, Fischer H, Bucher CH, Rendenbach C, Duda GN, Schmidt-Bleek K, et al. The multifaceted roles of macrophages in bone regeneration: A story of polarization, activation and time. Acta Biomater. (2021) 133:46–57. doi: 10.1016/j.actbio.2021.04.052
200. Song X, Xue Y, Fan S, Hao J, Deng R. Lipopolysaccharide-activated macrophages regulate the osteogenic differentiation of bone marrow mesenchymal stem cells through exosomes. PeerJ. (2022) 10:e13442. doi: 10.7717/peerj.13442
201. Xia Y, He XT, Xu XY, Tian BM, An Y, Chen FM. Exosomes derived from M0, M1 and M2 macrophages exert distinct influences on the proliferation and differentiation of mesenchymal stem cells. PeerJ. (2020) 8:e8970. doi: 10.7717/peerj.8970
202. Liu K, Luo X, Lv ZY, Zhang YJ, Meng Z, Li J, et al. Macrophage-derived exosomes promote bone mesenchymal stem cells towards osteoblastic fate through microRNA-21a-5p. Front Bioeng Biotechnol. (2021) 9:801432. doi: 10.3389/fbioe.2021.801432
203. Hang R, Tian X, Qu G, Zhao Y, Yao R, Zhang Y, et al. Exosomes derived from magnesium ion-stimulated macrophages inhibit angiogenesis. BioMed Mater. (2022) 17:045008. doi: 10.1088/1748-605X/ac6b03
204. Wang Z, Zhao Y, Zhao Y, Zhang Y, Yao X, Hang R. Exosomes secreted by macrophages upon copper ion stimulation can promote angiogenesis. Mater Sci Eng C Mater Biol Appl. (2021) 123:111981. doi: 10.1016/j.msec.2021.111981
205. Zhang H, Zhao Y, Zhang Y, Hang R, Yao X, Hang R. Exosomes derived from macrophages upon cobalt ion stimulation promote angiogenesis. Colloids Surf B Biointerfaces. (2021) 203:111742. doi: 10.1016/j.colsurfb.2021.111742
206. Yu L, Hu M, Cui X, Bao D, Luo Z, Li D, et al. M1 macrophage-derived exosomes aggravate bone loss in postmenopausal osteoporosis via a microRNA-98/DUSP1/JNK axis. Cell Biol Int. (2021) 45:2452–63. doi: 10.1002/cbin.v45.12
207. Li Z, Wang Y, Li S, Li Y. Exosomes derived from M2 macrophages facilitate osteogenesis and reduce adipogenesis of BMSCs. Front Endocrinol (Lausanne). (2021) 12:680328. doi: 10.3389/fendo.2021.680328
208. Bin-Bin Z, Da-Wa ZX, Chao L, Lan-Tao Z, Tao W, Chuan L, et al. M2 macrophagy-derived exosomal miRNA-26a-5p induces osteogenic differentiation of bone mesenchymal stem cells. J Orthop Surg Res. (2022) 17:137. doi: 10.1186/s13018-022-03029-0
209. Xiong Y, Chen L, Yan C, Zhou W, Yu T, Sun Y, et al. Correction to: M2 Macrophagy-derived exosomal miRNA-5106 induces bone mesenchymal stem cells towards osteoblastic fate by targeting salt-inducible kinase 2 and 3. J Nanobiotechnology. (2021) 19:88. doi: 10.1186/s12951-021-00828-1
210. Zhu Y, Zhao S, Cheng L, Lin Z, Zeng M, Ruan Z, et al. Mg(2+) -mediated autophagy-dependent polarization of macrophages mediates the osteogenesis of bone marrow stromal stem cells by interfering with macrophage-derived exosomes containing miR-381. J Orthop Res. (2022) 40:1563–76. doi: 10.1002/jor.25189
211. Liu A, Jin S, Fu C, Cui S, Zhang T, Zhu L, et al. Macrophage-derived small extracellular vesicles promote biomimetic mineralized collagen-mediated endogenous bone regeneration. Int J Oral Sci. (2020) 12:33. doi: 10.1038/s41368-020-00100-6
212. Liao XM, Guan Z, Yang ZJ, Ma LY, Dai YJ, Liang C, et al. Comprehensive analysis of M2 macrophage-derived exosomes facilitating osteogenic differentiation of human periodontal ligament stem cells. BMC Oral Health. (2022) 22:647. doi: 10.1186/s12903-022-02682-5
213. Zhou M, Li B, Liu C, Hu M, Tang J, Min J, et al. M2 Macrophage-derived exosomal miR-501 contributes to pubococcygeal muscle regeneration. Int Immunopharmacol. (2021) 101:108223. doi: 10.1016/j.intimp.2021.108223
214. Cui H, He Y, Chen S, Zhang D, Yu Y, Fan C. Macrophage-Derived miRNA-Containing Exosomes Induce Peritendinous Fibrosis after Tendon Injury through the miR-21-5p/Smad7 Pathway. Mol Ther Nucleic Acids. (2019) 14:114–30. doi: 10.1016/j.omtn.2018.11.006
215. Ebata T, Terkawi MA, Kitahara K, Yokota S, Shiota J, Nishida Y, et al. Noncanonical pyroptosis triggered by macrophage-derived extracellular vesicles in chondrocytes leading to cartilage catabolism in osteoarthritis. Arthritis Rheumatol. (2023) 75:1358–69. doi: 10.1002/art.42505
216. Haney MJ, Zhao Y, Jin YS, Li SM, Bago JR, Klyachko NL, et al. Macrophage-derived extracellular vesicles as drug delivery systems for triple negative breast cancer (TNBC) therapy. J Neuroimmune Pharmacol. (2020) 15:487–500. doi: 10.1007/s11481-019-09884-9
217. Yuan D, Zhao Y, Banks WA, Bullock KM, Haney M, Batrakova E, et al. Macrophage exosomes as natural nanocarriers for protein delivery to inflamed brain. Biomaterials. (2017) 142:1–12. doi: 10.1016/j.biomaterials.2017.07.011
218. Batrakova EV, Kim MS. Using exosomes, naturally-equipped nanocarriers, for drug delivery. J Control Release. (2015) 219:396–405. doi: 10.1016/j.jconrel.2015.07.030
219. Zhang M, Hu S, Liu L, Dang P, Liu Y, Sun Z, et al. Engineered exosomes from different sources for cancer-targeted therapy. Signal Transduct Target Ther. (2023) 8:124. doi: 10.1038/s41392-023-01382-y
220. Guo L, Zhang Y, Wei R, Zhang X, Wang C, Feng M. Proinflammatory macrophage-derived microvesicles exhibit tumor tropism dependent on CCL2/CCR2 signaling axis and promote drug delivery via SNARE-mediated membrane fusion. Theranostics. (2020) 10:6581–98. doi: 10.7150/thno.45528
221. Li J, Li N, Wang J. M1 macrophage-derived exosome-encapsulated cisplatin can enhance its anti-lung cancer effect. Minerva Med. (2020). doi: 10.23736/S0026-4806.20.06564-7
222. Tang Z, Tang C, Sun C, Ying X, Shen R. M1 macrophage-derived exosomes synergistically enhance the anti- bladder cancer effect of gemcitabine. Aging (Albany NY). (2022) 14:7364–77. doi: 10.18632/aging.204200
223. Wang P, Wang H, Huang Q, Peng C, Yao L, Chen H, et al. Exosomes from M1-polarized macrophages enhance paclitaxel antitumor activity by activating macrophages-mediated inflammation. Theranostics. (2019) 9:1714–27. doi: 10.7150/thno.30716
224. Zhao Y, Zheng Y, Zhu Y, Li H, Zhu H, Liu T. Docetaxel-loaded M1 macrophage-derived exosomes for a safe and efficient chemoimmunotherapy of breast cancer. J Nanobiotechnology. (2022) 20:359. doi: 10.1186/s12951-022-01526-2
225. Zhao Y, Zheng Y, Zhu Y, Zhang Y, Zhu H, Liu T. M1 macrophage-derived exosomes loaded with gemcitabine and deferasirox against chemoresistant pancreatic cancer. Pharmaceutics. (2021) 13:1493. doi: 10.3390/pharmaceutics13091493
226. Kim MS, Haney MJ, Zhao Y, Yuan D, Deygen I, Klyachko NL, et al. Engineering macrophage-derived exosomes for targeted paclitaxel delivery to pulmonary metastases: in vitro and in vivo evaluations. Nanomedicine. (2018) 14:195–204. doi: 10.1016/j.nano.2017.09.011
227. Li S, Wu Y, Ding F, Yang J, Li J, Gao X, et al. Engineering macrophage-derived exosomes for targeted chemotherapy of triple-negative breast cancer. Nanoscale. (2020) 12:10854–62. doi: 10.1039/D0NR00523A
228. Zhang RY, Cheng K, Huang ZY, Zhang XS, Li Y, Sun X, et al. M1 macrophage-derived exosome for reprograming M2 macrophages and combining endogenous NO gas therapy with enhanced photodynamic synergistic therapy in colorectal cancer. J Colloid Interface Sci. (2024) 654:612–25. doi: 10.1016/j.jcis.2023.10.054
229. Gao H, Wang S, Liu Z, Hirvonen JT, A SH. Mycophenolic acid-loaded naïve macrophage-derived extracellular vesicles rescue cardiac myoblast after inflammatory injury. ACS Appl Bio Mater. (2023) 6:4269–76. doi: 10.1021/acsabm.3c00475
230. Cui Y, Hong S, Xia Y, Li X, He X, Hu X, et al. Melatonin engineering M2 macrophage-derived exosomes mediate endoplasmic reticulum stress and immune reprogramming for periodontitis therapy. Adv Sci (Weinh). (2023) 10:e2302029. doi: 10.1002/advs.202302029
231. Wu G, Zhang J, Zhao Q, Zhuang W, Ding J, Zhang C, et al. Molecularly engineered macrophage-derived exosomes with inflammation tropism and intrinsic heme biosynthesis for atherosclerosis treatment. Angew Chem Int Ed Engl. (2020) 59:4068–74. doi: 10.1002/anie.201913700
232. Cheng J, Zhang Y, Ma L, Du W, Zhang Q, Gao R, et al. Macrophage-derived extracellular vesicles-coated palladium nanoformulations modulate inflammatory and immune homeostasis for targeting therapy of ulcerative colitis. Adv Sci (Weinh). (2023) 10:e2304002. doi: 10.1002/advs.202304002
233. Shou J, Li S, Shi W, Zhang S, Zeng Z, Guo Z, et al. 3WJ RNA nanoparticles-aptamer functionalized exosomes from M2 macrophages target BMSCs to promote the healing of bone fractures. Stem Cells Transl Med. (2023) 12:758–74. doi: 10.1093/stcltm/szad052
234. Wang X, Ding H, Li Z, Peng Y, Tan H, Wang C, et al. Exploration and functionalization of M1-macrophage extracellular vesicles for effective accumulation in glioblastoma and strong synergistic therapeutic effects. Signal Transduct Target Ther. (2022) 7:74. doi: 10.1038/s41392-022-00894-3
235. Huang Z, Guo L, Huang L, Shi Y, Liang J, Zhao L. Baicalin-loaded macrophage-derived exosomes ameliorate ischemic brain injury via the antioxidative pathway. Mater Sci Eng C Mater Biol Appl. (2021) 126:112123. doi: 10.1016/j.msec.2021.112123
236. Huo Q, Shi Y, Qi Y, Huang L, Sui H, Zhao L. Biomimetic silibinin-loaded macrophage-derived exosomes induce dual inhibition of Aβ aggregation and astrocyte activation to alleviate cognitive impairment in a model of Alzheimer's disease. Mater Sci Eng C Mater Biol Appl. (2021) 129:112365. doi: 10.1016/j.msec.2021.112365
237. Li F, Zhao L, Shi Y, Liang J. Edaravone-loaded macrophage-derived exosomes enhance neuroprotection in the rat permanent middle cerebral artery occlusion model of stroke. Mol Pharm. (2020) 17:3192–201. doi: 10.1021/acs.molpharmaceut.0c00245
238. Cheng L, Wang Y, Huang L. Exosomes from M1-polarized macrophages potentiate the cancer vaccine by creating a pro-inflammatory microenvironment in the lymph node. Mol Ther. (2017) 25:1665–75. doi: 10.1016/j.ymthe.2017.02.007
239. Long R, et al. M2 macrophage-derived exosomes carry miR-1271-5p to alleviate cardiac injury in acute myocardial infarction through down-regulating SOX6. Mol Immunol. (2021) 136:26–35. doi: 10.1016/j.molimm.2021.05.006
240. Wang Z, et al. miRNA-93-5p in exosomes derived from M2 macrophages improves lipopolysaccharide-induced podocyte apoptosis by targeting Toll-like receptor 4. Bioengineered. (2022) 13:7683–96. doi: 10.1080/21655979.2021.2023794
241. Wang X, et al. Mechanism of M2 type macrophage-derived extracellular vesicles regulating PD-L1 expression via the MISP/IQGAP1 axis in hepatocellular carcinoma immunotherapy resistance. Int Immunopharmacol. (2023) 124:110848. doi: 10.1016/j.intimp.2023.110848
242. Jiang W, et al. Macrophage-derived, LRG1-enriched extracellular vesicles exacerbate aristolochic acid nephropathy in a TGFβR1-dependent manner. Cell Biol Toxicol. (2022) 38:629–48. doi: 10.1007/s10565-021-09666-1
243. Kim H, et al. Extracellular vesicle-guided in situ reprogramming of synovial macrophages for the treatment of rheumatoid arthritis. Biomaterials. (2022) 286:121578. doi: 10.1016/j.biomaterials.2022.121578
244. Li C, et al. MicroRNA-370 carried by M2 macrophage-derived exosomes alleviates asthma progression through inhibiting the FGF1/MAPK/STAT1 axis. Int J Biol Sci. (2021) 17:1795–807. doi: 10.7150/ijbs.59715
245. Li L, et al. Paintable bioactive extracellular vesicle ink for wound healing. ACS Appl Mater Interfaces. (2023) 15:25427–36. doi: 10.1021/acsami.3c03630
246. Wang B, et al. Macrophage-derived exosomal mir-155 regulating cardiomyocyte pyroptosis and hypertrophy in uremic cardiomyopathy. JACC Basic Transl Sci. (2020) 5:148–66. doi: 10.1016/j.jacbts.2019.10.011
Keywords: macrophage-derived extracellular vesicles, exosomes, biological functions, drug delivery systems, chronic non-communicable diseases
Citation: Lin F, Luo H, Wang J, Li Q and Zha L (2025) Macrophage-derived extracellular vesicles as new players in chronic non-communicable diseases. Front. Immunol. 15:1479330. doi: 10.3389/fimmu.2024.1479330
Received: 13 August 2024; Accepted: 23 December 2024;
Published: 17 January 2025.
Edited by:
Yingxin Zhao, University of Texas Medical Branch at Galveston, United StatesReviewed by:
Mario Leonardo Squadrito, Vita-Salute San Raffaele University, ItalyCopyright © 2025 Lin, Luo, Wang, Li and Zha. This is an open-access article distributed under the terms of the Creative Commons Attribution License (CC BY). The use, distribution or reproduction in other forums is permitted, provided the original author(s) and the copyright owner(s) are credited and that the original publication in this journal is cited, in accordance with accepted academic practice. No use, distribution or reproduction is permitted which does not comply with these terms.
*Correspondence: Longying Zha, bHl6aGFAc211LmVkdS5jbg==
†These authors have contributed equally to this work
Disclaimer: All claims expressed in this article are solely those of the authors and do not necessarily represent those of their affiliated organizations, or those of the publisher, the editors and the reviewers. Any product that may be evaluated in this article or claim that may be made by its manufacturer is not guaranteed or endorsed by the publisher.
Research integrity at Frontiers
Learn more about the work of our research integrity team to safeguard the quality of each article we publish.