- 1Department of General Surgery, Clinical Laboratory of Integrative Medicine, The First Affiliated Hospital of Dalian Medical University, Dalian, China
- 2Department of Oncology, First Affiliated Hospital of Dalian Medical University, Dalian, China
- 3Central Laboratory, The First Affiliated Hospital of Dalian Medical University, Dalian, China
- 4Key Laboratory of Clinical Cancer Pharmacology and Toxicology Research of Zhejiang Province, Department of Clinical Pharmacy, Affiliated Hangzhou First People’s Hospital, Westlake University, Hangzhou, China
- 5Institute (College) of Integrative Medicine, Dalian Medical University, Dalian, China
A steady dysfunctional state caused by chronic antigen stimulation in the tumor microenvironment (TME) is known as CD8+ T cell exhaustion. Exhausted-like CD8+ T cells (CD8+ Tex) displayed decreased effector and proliferative capabilities, elevated co-inhibitory receptor generation, decreased cytotoxicity, and changes in metabolism and transcription. TME induces T cell exhaustion through long-term antigen stimulation, upregulation of immune checkpoints, recruitment of immunosuppressive cells, and secretion of immunosuppressive cytokines. CD8+ Tex may be both the reflection of cancer progression and the reason for poor cancer control. The successful outcome of the current cancer immunotherapies, which include immune checkpoint blockade and adoptive cell treatment, depends on CD8+ Tex. In this review, we are interested in the intercellular signaling network of immune cells interacting with CD8+ Tex. These findings provide a unique and detailed perspective, which is helpful in changing this completely unpopular state of hypofunction and intensifying the effect of immunotherapy.
1 Introduction
T cells within the immune system patrol to find pathogens in the human body. When T cells turn into the tumor microenvironment (TME), they will detect and distinguish normal cells and cancer cells according to the tumor antigen information presented by major histocompatibility complex (MHC) molecules on antigen-presenting cells (APC) cells, which induces inflammatory response and antitumor response by secreting cytotoxic secretions (1). However, when antigens chronically and continuously stimulate CD8+ T cells over an extended period, CD8+ T cells will have exhausted key characteristics appearance, including decreased effector function and proliferation, elevated co-inhibitory receptor expression, reduced cytotoxicity, and changes in metabolism and transcription (2). Exhausted-like CD8+ T cells (CD8+ Tex) initially found in mice with chronic infection with the lymphocytic choriomeningitis virus (LCMV) (3). Since then, multiple research efforts have demonstrated that Tex is essential for chronic infection, tumors, and autoimmune diseases (2, 4). CD8+ T cells developing into CD8+ Tex will go through the following four stages: dormant Tex progenitor (Texprog1), proliferating and circulating Tex progenitor (Texprog2), circulating and slightly toxic intermediate exhausted T cells (Texint), and terminally differentiated exhausted T cells (Texterm) (5). T cell exhaustion is triggered by the following four factors in the TME: 1) long-term antigen stimulation and high expression of co-inhibitory receptors/immune checkpoints; 2) soluble cytokines (type I interferon, IL-2, IL-10, and transforming growth factor-β (TGF-β)) in the TME; 3) recruitment of immunosuppressive cells: regulatory T cells (Tregs), Myeloid-Derived Suppressor Cell (MDSC), and tumor-associated macrophages (TAMs); 4) and the lack of oxygen and nutrients in the TME (6).
The successful outcome of cancer immunotherapy, particularly immune checkpoint blockade (ICB) and adoptive T cell treatment (ACT), is diminished by T cell exhaustion (7). Tex is the main reactive cell of ICB, and it is the breakthrough point in improving the efficacy of ICB. One of the aims of ICB therapy is expanding and infiltrating precursor exhausted T cell (Tpex) (8). In addition, TME induces dysfunctional Chimeric Antigen Receptor T (CAR-T) cells and T cell receptor-engineered T (TCR-T) cells, which are major obstacles to ACT in solid tumors. Reducing T cell exhaustion and intensifying the effectuality of ACT not only need to optimize chimeric antigen receptors and downstream signal transduction but also should intervene in transcriptional and metabolic disorders (9). Notably, CAR-Treg therapy, which induces Treg depletion, can also inhibit tumor development (10, 11).
Therefore, this review mainly focuses on the intercellular signaling networks interacting with Tex, the immunosuppressive cells in the TME, and the dendritic cells (DCs) assisting CD8+ T cells in anti-tumor immunity. In the end, we discussed how to deal with the challenges of Tex in ICB therapy and ACT.
2 Immune cells regulate T-cell exhaustion through intercellular communication
When neoplasm antigens are continuously exposed over an extended period, the development of CD8+ Tex integrates other immune cells expressing co-inhibitory or co-stimulatory receptors and cytokines transmitting inhibitory signals in the TME (6). We summarized the intercellular communication between Tregs, MDSCs, TAMs, DCs, and Tex (Figure 1).
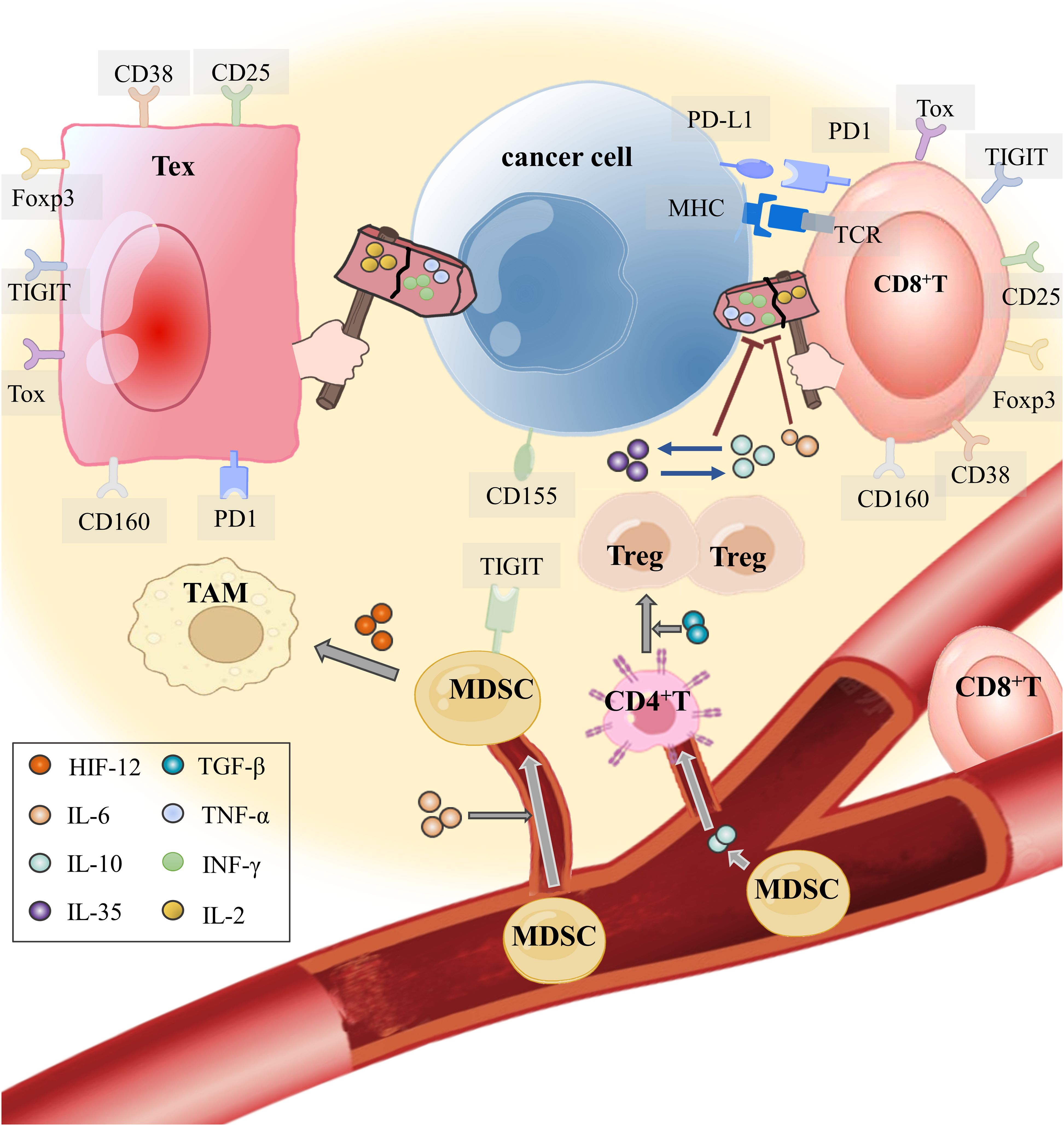
Figure 1. How do tumor cells instigate immune cells into the TME and make them accomplices? T cells in the TME, through their T cell receptor (TCR), recognize the antigen presented by MHC on the APC (including tumor cells) and secrete effector cytokines to exert antitumor immunity. IL-6, which is released by tumor cells in the TME, not only restrains the antitumor immunity of effector T cells but also recruits MDSCs into the TME. Hypoxia in the TME makes HIF-1 α highly expressed. With the mediation of hypoxia-inducible factor-1α (HIF-1α) and IL-6, MDSCs induce monocyte myeloid-derived suppressor cells (m-MDSCs) to differentiate into TAMs for obstructing T cell activation and effector response. In addition, IL-10 produced by MDSCs attracts CD4+ T cells to enter the TME and develop into Tregs with TGF-β. The interaction between IL-35 released by CD177+ Tregs and IL-10 released by PD-1+ Tregs inhibits CD8+ T cells from eliminating tumor cells and mediates T-cell exhaustion.
2.1 Tregs
Tregs can maintain self-tolerance and avoid excessive immune response damage, but Tregs participate in tumor immune evasion mechanisms in the TME. Tregs in the TME can inhibit effector T cells (Teff) and myeloid cells from two aspects. On the one hand, it upregulates the immune checkpoint ligand of tumor cells to avert CD8+ T cell immunological inspection; On the other hand, it secretes cytokines to induce CD8+ Tex. Immunosuppressive cytokines (IL-10, IL-35, and TGF-β) produced by Tregs have the capacity to both drive CD8+ T cells to cease becoming Teffs and deliver immunological escape indications to tumor cells (12).
2.1.1 IL-10 and IL-35
In tumor formation and metastasis, Tregs are the main source of IL-10, a kind of cytokine with durable and versatile anti-inflammatory effects (13). In the TME, Tregs significantly secrete more IL-10, which immune checkpoint molecule TIM-3 plays a non-negligible role. Banerjee H et al. found that Tregs upregulated the secretion of IL-10 promoting CD8+ Tex through T cell immunoglobulin and mucin domain-containing protein 3 (TIM-3) (14). Interestingly, IL-10 also reverses the process of inducing Treg differentiation in the TME, so that the interaction between IL-10 and Treg further acts on T cell exhaustion (15). Furthermore, it was discovered that Salkeni et al. thought IL-10 induced T cell stimulation in the TME. Thus, more investigation is required to learn how IL-10 influences T-cell exhaustion (16). Different Treg subsets produce different cytokines. In the study by Ma et al. of esophageal squamous cell carcinoma, PD-1+ Tregs were the primary producers of IL-10, whereas CD177+ Tregs were the primary producers of IL-35 in the TME (17). As a heterodimer of IL-12, IL-35 derived by Tregs regulates the generation of immune checkpoint molecules: programmed cell death protein 1 (PD-1), TIM-3, and lymphocyte-activation gene 3 (LAG-3), which promote T cell exhaustion by cooperating with IL-10 (12).
2.1.2 TGF-β
An important modulator of both innate and adaptive immunity is TGF-β (18), which is released by tumor cells and immune cells (19, 20). TGF-β prevents immature T cells from differentiating into Th1 cells, promoting Foxp3 to encourage the differentiation of naïve CD4+ T cells into Treg cells and regulating TGF-β signaling to mediate the anti-tumor immune function of Treg inhibiting CD8+ T cells in the TME (21, 22). Knocking down endogenous TGF-β receptor type-2 (TGFBR2), blocking TGF-β signaling, or inhibiting the deubiquitination of Foxp3 from upregulating in the TME can inhibit the differentiation of Tregs in the TME and prevent Tex (23–26). In addition, colonic carcinoma metastasis signal mediated by TGF-β can stimulate the upregulation of Foxp3, CD25, and PD-1, which are markers of CD8+ Tex (27).
2.2 MDSC
In addition to facilitating tumor vascularization, MDSCs in the TME also aid in the tumor cells’ invasion and metastasis. MDSCs inhibit T cell activation and promote the differentiation process of Tregs. In addition, MDSCs can also polarize TAMs (28). In the TME, MDSCs recruitment and infiltration are accompanied by a decrease of Teff-produced cytokines, upregulation of multiple inhibitory receptors (IR), and alterations to T cell subsets, which undermine the long-term maintenance of tumor immunity (29).
2.2.1 IL-6
IL-6, which can be produced by a variety of cells, is a classical cytokine regulating various inflammations. In the inflammatory response, it possesses both opposed- and anti-inflammatory characteristics (30). The aberrant production of IL-6 is the result of TME chronic inflammation (31, 32), so tumor cells will produce and secrete more endogenous IL-6 when proliferating, infiltrating, or metastasizing (33, 34). Tumor patients who showed higher concentrations of IL-6 in their blood in clinical had suppression of CD8+ T cells proliferation and cytokine generation, and they had higher adverse clinical outcomes (35). In the TME, IL-6 can bind its receptor, transmit signals through the downstream JAK/STAT3 pathway, and activate the transcription of tumor-related genes. In addition, IL-6/JAK/STAT3 signal transduction upregulates immune checkpoint ligand PD-L1 expressed and regulates MDSCs to suppress tumor immunity (36). MAPK and IL-6-mediated signaling pathways impact immature MDSCs (i-MDSCs) in the TME, which are activated by IL-6 and attracted to tumor locations where they develop into mature MDSCs and decrease tumor immunity (37). IL-6 recruits MDSCs in the TME, which can inhibit T cell activity and promote partial CD8+ Tex through IFN-γ (38). One important regulator in preserving MDSC differentiation, proliferation, and function is IL-6. When MDSC inhibitors are applied to inhibit the number and function of MDSC, the expression of IL-6 in the TME is also inhibited, and the active immune response is re-established (39). It’s interesting to note that IL-6 released by MDSCs was linked to multidrug resistance via the IL-6/STAT1 axis in the work by Dhar S et al. (40). Within the congenital drug resistance model, combining treatment of Cytotoxic T-Lymphocyte Associated Antigen 4 (CTLA-4) inhibitor and BET inhibitor induced the decrease of MDSCs infiltration and downregulated TIM-3 and LAG-3 in the TME (41). IL-6 in the TME recruits MDSCs to participate in the drug resistance of tumor therapy and may be related to CD8+ Tex, but the clear mechanism needs further exploration.
2.2.2 Immune checkpoint
The reason why acquired resistance happens to ICB therapy is that MDSCs induce CD8+ Tex. According to Koh J et al., the greater the infiltration of MDSCs with the progression of the patients’ non-small cell lung cancer, MDSCs inhibited T cell activity and induced T cell exhaustion in the TME, which contributed to resistance in anti-PD-1 therapy (42). Additionally, Galectin 9 (Gal-9) acts as an immune checkpoint ligand for TIM-3. MDSCs upregulate the expression of Gal-9 in the TME. A rise in Gal-9 causes CD8+ T cells to overexpress TIM-3, which prevents T cells from secreting effector cytokines. Thus, the TIM-3/Gal-9 route may be used by MDSCs to promote CD8+ T cell exhaustion (43). In addition, clinical research on the treatment of breast cancer showed that CDK4/6 inhibitors avoided the T cell exhaustion phenotype by reducing the expression levels of multiple immune checkpoints and the quantity of MDSCs (44).
2.2.3 MDSCs interact with Tregs
As mentioned above, the primary source of IL-10 in the TME is Tregs. It is undeniable that cytokines including IL-10 released by MDSCs can activate Tregs and TAMs in the TME (45). MDSCs and Tregs in the TME share common microRNAs related to immune regulatory pathways, including TNF, TGF-β, FOXO, and Hippo (46, 47). Therefore, MDSCs and Tregs not only have the effect of inhibiting tumor immunity, but also interact and cooperate with each other in TME.
Following the injection of carboplatin, MDSCs stimulated IL-13/IL-33 via the VCAM/RANTES pathway, facilitating CD4+ T cells to convert into Tregs and encouraging Tregs aggregation at tumor locations. The inhibitory receptor TIGIT was upregulated by CD155. The expression of TIGIT was favorably linked with the ratio of T cells to MDSCs in TME. CD8+ T cells upregulated the expression of CTLA-4, TIM-3, and CD160 (48). Blocking TIGIT/CD155 signal transduction alleviates Tex and delays tumor growth (49). MDSCs recruited in the TME, mediate the growth and metastasis of tumors through phosphatidylinositol-3-hydroxylase (PI3Kγ), inhibiting the transcription of down-regulated T cell exhaustion genes in mouse tumors with PI3Kγ, and reducing infiltrated Foxp3+ Tregs in the TME (50).
2.2.4 MDSC with TREM1
The triggering receptor expressed on myeloid cell 1 (TREM1) is a key mediator of innate immunity (51). The high expression of TREM1 in polymeric myeloid-derived suppressor cells (PMN-MDSCs) and M2-like macrophages is associated with the inferior prognosis of lung cancer (52), liver cancer (53), renal cell carcinoma (54), breast cancer (51)and other tumors. Knockdown of TREM1 can enhance the therapeutic effect of anti-PD-1, probably by inhibiting the function of MDSCs and T cell exhaustion in the TME (55). In the DLL3-TREM1/DAP12 CAR-T (DLL3-DT CAR-T) therapy developed by Nie F et al., DLL3-DT CAR-T cells have durable antitumor responses and increased production of memory T cells in the TME (56).
2.2.5 MDSC with exosome
Increasing evidence suggests that exosomes can promote chemoresistance within the TME (57). Tumors regulate the TME by secreting microRNAs from exosomes. Pancreatic cancer-derived exosomes have a macrophage migration inhibitor factor (MIF), and MIF tautomerase regulates the expression of genes required for the differentiation, recruitment, and activation of MDSCs (58). In addition, exosome mir-1298-5p promotes the immunosuppressive effect of MDSCs, promoting the growth of neuroglioma (59). Interestingly, MDSC−derived exosomes (MDSC exo) are hyperactivated inducing CD8+ T cell exhaustion and promoting the production of reactive oxygen species to meet the oxygen supply of tumor cells (60).
2.3 TAMs
Tumor cells in the TME recruit TAMs and induce M2 polarization by secreting cytokines and creating an immunosuppressive environmental state (61). Liu C et al. found that APOE+ macrophages are closely connected to CD8+ Tex (62), which means TAMs inhibit antitumor T‐cell immunity in solid tumors. In the study of Yin C et al., hepatocellular carcinoma (HCC) induced M2 polarization by upregulating the expression of miR-146a-5p in exosomes and activating NF-κB signaling to induce proinflammatory factors for remodeling macrophages. Blocking the interaction between the transcription factor Sal-like protein-4 (SALL4) and mir-146a-5p reduces the expression of inhibitory receptors and reverses the exhaustion of CD8+ T cells (63). In addition, M2-like macrophages secrete extracellular vesicles to promote CD8+ T cells exhaustion of HCC through the mir-21-5p/YOD1/YAP/β-catenin pathway (64).
2.3.1 Hypoxia
TAMs are abundant in TME, tumor blood vessels, and stromal regions, which have immunosuppressive effects and promote Tex (61). Hypoxia is frequently seen in TME, and there is a high expression of hypoxia-inducible factor-1α (HIF-1α). HIF-1α can induce monocyte myeloid-derived suppressor cells (m-MDSCs) to differentiate into TAMs through IL-6/STAT3/p-STAT3 and inhibit T cell activation and effector function (65, 66). Targeted STAT3 tumor immunotherapy reverses the polarization and immunosuppression of TAMs in the TME (67, 68). Through the regulation of XOR-IDH3α, TAMs may impact the function of TAMs and CD8+ T cells. According to Lu Y et al. of hepatocellular carcinoma and xanthine oxidoreductase (XOR), within hypoxia TME, enzymes involved in macrophage polarization interact with IDH3α to mediate TAMs polarization and inhibit anti-tumor immunity of CD8+ T cells (69). The upregulation of CD8+ T cell immune checkpoints (PD-1, CD38, TOX) was positively correlated with the abundance of TAMs in the TME. In addition, Kersten K’s team also found that exhausted T cells would recruit monocytes to the TME and form TAMs to induce CD8+ T cell exhaustion, further inhibiting anti-tumor immunity (70).
HIF-1α can not only induce m-MDSCs to differentiate into TAMs but also upregulate the expression of PD-L1 on the surface of MDSCs by binding to PD-L1 receptor on the surface of T cells, which induces Tex (71, 72). In a therapeutic study of nanomaterials transporting catalase into the TME, ROS were increased by degrading H2O2, significantly reducing MDSCs and Tregs (73).
2.3.2 Chemokines
Chemokines which are a kind of guiding cell migration cytokines expressed in the TME will recruit immune cells into the TME (74–77). There are many different chemokines produced by different cells in the complex TME. In the study of Kamat K et al., CCL23 secreted by TAMs induced T cell exhaustion by mediating GSK3β to upregulate T cell exhaustion markers in ovarian cancer (78). In this part, we focus on the chemokines related to T cells exhaustion and explore their potential research directions in ICB and ACT therapy.
As mentioned above, hypoxia in the TME induces the polarization of TAMs and the exhaustion of T cells (65). Several studies have shown that TAMs play an immunosuppressive role in the TME through the CCR5-dependent signaling axis (79–81), which can block the CCR5-related pathway to inhibit tumor invasion and metastasis (82). In advanced cancer patients, the proportion of exhausted T cells in a hypoxic environment is significantly increased. Analysis of exhausted T cells ligand-receptor interactions revealed that tumor cells would recruit T cells into hypoxic TME through the chemokine CCL3/CCL4/CCL5-CCR5 axis (83). Hernández-Verdin I et al. found that the increased expression of activation-induced cytidine deaminase (AID) caused by mutation induced T cells exhaustion by upregulating the expression of CXCL13/CCR5. The application of ICB therapy can block it, thereby reactivating exhausted T cells (84). Coincidentally, the study of Liu Z et al. found that RUNX3 was the key mediator of decitabine improving anti-PD-1 immunotherapy, and knockdown of RUNX3 significantly reduced the levels of CCR3 and CCR5 (85). In addition, TAMs in the TME will conduct cellular and molecular communication with immature DCs induced by tumor cells through CCR5/CCL5 molecules, which will destroy the antigen-presenting function of DCs (86). Similarly, the study of Horie M et al. also showed that HIF-1α would promote the recruitment of TAMs and DCs in the TME under hypoxia, and create an immunosuppressive microenvironment by upregulating the expression of CCR5/CCL5, inducing T cells exhaustion and peritoneal dissemination of cancer cells (87). TAMs in TME not only inhibit the anti-tumor effect of T cells, but also destroy the auxiliary function of DCs in this process. How DCs become a good helper of CD8+ T cells will be described in the next section.
Hedgehog (Hh) signaling in myeloid cells is essential for M2 polarization of TAMs and tumor growth. Tumor cells secrete an Hh ligand (SHH) to drive TAMs to polarize towards M2 (88). The production of CXCL9 and CXCL10 by polarized TAMs is inhibited, blocking the CXCL9/10/CXCR3 axis and inhibiting the recruitment of CD8+ T cells to the TME (88). Compared with normal renal tissue, the mRNA expression of CXCR3/CXCL9/10/11 in renal cell carcinoma was significantly increased (89), and the upregulation of CXCR3 expression in renal cell carcinoma was related to the hypoxic state in TME (90). In addition, the study of Azuma M et al. demonstrated that Poly (I: C) interacted with DCs, cross-upregulated the expression of CXCR3, and promoted the recruitment of CD8+ T cells to the TME (91). However, CD8+ T cells recruited to the TME will gradually downregulate the expression of CXCR3 under chronic antigen stimulation, while upregulating the exhaustion markers PD-1 and LAG-3. In addition, the knockdown of CXCR3 can enhance the production of IFN-γ, an effector molecule of CD8+ T cells, indicating that CXCR3 promotes the loss of effector function and the process of T cell exhaustion (92). Interestingly, the expression of CXCR3 in tumor mice that did not respond to ICB therapy was significantly reduced (93). Enhancing the CXCR3/CXCL9 axis can promote the interaction between DCs and CD8+ T cells in the TME and improve the sensitivity to PD-1 blockade and clinical therapeutic efficacy (94). The enhancing effect of CXCR3 in PD-1/PD-L1 therapy may be related to its ability to recruit T cells into the TME in an antigen-independent manner and to activate the bystander memory T cells (95). At the same time, previous studies have shown that the CXCR3 ligand helps T cells and B cells recruited to the TME form a cell network directly contacting each other. This network contains TCF1+ PD-1+ CD8+ T cell progenitors, which can be transformed into cytotoxic CD8+ T cells during ICB treatment (96, 97). Therefore, Sullivan PM et al. demonstrated that overexpressed CXCR3 can be applied to ACT therapy to drive the trafficking of CD8+ T cells into the TME and synergize with PD-1 checkpoint blockade immunotherapy (98). In addition, although it played an important role in the entry of CD8+ T cells into the TME, they gave an explanation for the incomprehensible result that CXCR3 expression was downregulated in the TME: it may be attributed to cell-extrinsic variables in the TME, such as inhibitory receptor signal transduction and TGF-β secreted by tumor cells (99). Similarly, Li A et al. demonstrated that a unique anti-human GARP antibody (named PIIO-1) treatment could block CD8+ T cell exhaustion by reducing TGF-β signaling in the TME while enhancing CD8+ T migration into the TME in a CXCR3-dependent manner (25).
2.3.3 Interferon regulatory factor 8 (IRF8)
As mentioned above, tumor cells secrete Shh to drive TAMs to polarize towards M2, and the production of CXCL9 and CXCL10 by polarized TAMs is inhibited (88). In the study of He N et al., it was found that the polarization of TAMs in microwave ablation (MWA) therapy was mediated through the nuclear factor-κB/JAK-STAT1 signaling pathway. MWA significantly upregulated the expression of IFN-γ stimulated transcription factors (especially IRF8) (100). Among the nine IRF members regulating interferon signaling, the expression of IRF8 in HCC was associated with inferior prognosis of HCC patients (101). In addition, MWA combined with αPD-L1 treatment promoted the production of CXCL9 and blocked IFN-γ/CXCL9/CD8+ T axis which could promote tumor progression (100). Tumor cells will induce the expansion of TAMs, which requires TAMs to express IRF8. Specific deletion of IRF8 in TAMs blocks T-cell exhaustion and inhibits tumor growth (102). Therefore, TAMs participate in the process of T cell exhaustion by upregulating IRF8. The study of Wu H et al. demonstrated that IRF8 downregulated the expression of CCL20 by inhibiting c-fos transcription and mediated the immunosuppressive effect of TAMs recruited in the TME (101).
2.4 DCs
In the TME, immunosuppressive cells Tregs, MDSCs, and TAMs cooperate with each other, but CD8+ T cells are not in a helpless situation. DCs will cooperate to participate in the anti-tumor immunity in the TME and help the anti-tumor immunity of CD8+ T cells through antigen presentation (103). The crosstalk between DCs and T cell exhaustion is mainly concentrated on interferons (type I interferons and type II interferons) and IL-2. The dual role of IL-2 still needs further exploration.
2.4.1 Interferon
2.4.1.1 Type I interferon
As antigen-presenting cells, DCs must go through the following processes to achieve effective anti-tumor immunity: professional antigen-presenting cells (pAPCs) absorb tumor neoantigens and promote the maturation and development of immature T cells through cross-presentation. This process is mainly participated by DCs (103). According to the dynamic changes of TME, DC subsets (conventional type 1 dendritic cells (cDC1), conventional type 2 dendritic cells (cDC2), plasmacytoid dendritic cells (pDCs)) affect the progression of tumors through various mechanisms (104). Among them, cDC1 is essential for anti-tumor immunity, which allows tumor antigens to be presented cross-presentatively to activate T cells (105). cDC2 has also been shown to drive protective anti-tumor CD4 T cell immunity (106). pDCs can produce type I interferon in the TME to promote the maturation of cDC1 and type I interferon also enhances the ability of CD8+ T cells (107, 108). However, TME also induces pDCs to express immunosuppressive molecules to encourage the formation of tumors. Chemotherapy carboplatin-resistant tumor cells recruited pDCs and upregulated pDC immune checkpoint ligand inducible costimulator ligand (ICOS-L) expression to maintain MDSCs-dependent immunosuppressive ability and promote Tex in the TME (48). Excessive type I interferons produced by pDCs also drive T-cell exhaustion. According to Wu T et al., the transcription factor TCF-1 induced B-cell lymphoma 6 (BCL6) to antagonize IFN-α/β signaling mediated Tex and maintain the stem cell properties of T cells (109).
2.4.1.2 Type II interferon
After sensing the production of IFN-γ by neighboring T cells, activated DCs produce IL-12 to stimulate anti-tumor immunity (110). The specific recognition of antigen peptides on tumor cells by CD8+ T cells require the presentation of major histocompatibility complex (MHC or HLA) class I molecules. IFN-γ signaling is a key pathway to regulate the expression of HLA-I and HLA-II, and its destruction is one way that tumor immune evasion works (103). HLA-DR is a class II MHC molecule expressed by CD8+ T cells. After being stimulated by tumor antigens, T cells show a multitude of cytokines including IFN-γ, TNF-α, and IL-2 (111). The defect in IFN-γ production means that T cells have entered the exhaustion phase (112).
2.4.2 IL-2
IL-2 is a significant cytokine that regulates Tex. IL-2 in the TME is mainly secreted by CD8+ T cells and activated DCs. IL-2 exerts its immunomodulatory effect mainly by binding to IL-2R. Various conformations of IL-2R mediate the downstream effects of IL-2. Due to the variable conformation of IL-2R, IL-2 shows a dual role of both immunostimulatory and immunosuppressive, which is related to interleukin-18 (IL-18) and Tregs.
2.4.2.1 Immunostimulatory effect of IL-2
Reduced IL-2 production is considered an early sign of CD8+ Tex. DCs and CD8+ T cells secrete IL-2, which can also affect the anti-tumor effect of CD8+ T cells. According to Zhang et al., histamine H1 receptor (H1R1) antagonists can stimulate T cell activation and promote IL-2 secretion, thereby inhibiting T cell exhaustion clinically related to high expression of H1R1 (113). In addition, IL-2 antagonizes TOX by driving STAT5 activity to exert anti-tumor immunity that reverses CD8+ Tex (114). In a study of CAR-T cell therapy combined with infusing aAPC, aAPC infusion promoted the specific recognition of CAR-T cells to tumor cells and released more IFN-γ, TNF-α, and IL-2. The cytokine mixture IL-2-9-21 not only inhibits the upregulation of the immune checkpoints on CAR-T cells but also inhibits the PI3K/AKT signaling pathway and enhances the JAK/STAT3 signaling pathway. This prevents the T cell exhaustion made by the CAR-T cells’ constant binding to tumor antigens in the TME (115).
2.4.2.2 Immunosuppressive effect of IL-2
A high concentration of IL-2 in the TME will activate the initial multiplication of naive T cells. The activated CD8+ T cells transiently express IL-2Rαβγ, a high-affinity receptor for IL-2, and then are driven by IL-2 to differentiate into CD8+ T cells that continuously express the medium affinity receptor IL-2Rβγ (116). Due to the different conformations of IL-2R, IL-2 also exerts immunosuppressive effects. In the study of Feriz AM et al., tumor cells stimulate immature DCs to migrate to the tumor site through inflammatory chemokines. DCs infiltrating the TME upregulate many signaling pathways, such as TNF-α/NF-κB, IL-2/STAT5, and E2F, while the IL-2 autocrine signal in DCs activates the JAK/STAT5 pathway to induce apoptosis of mature DCs (86). IL-2 can also induce the STAT5/TPH1/5-HTP/AhR pathway in CD8+ T cells, leading to synergistic upregulation of inhibitory receptors and downregulation of effector molecules, which induces CD8+ Tex appearance in the TME (117).
2.4.2.3 IL-2 and Interleukin-18 have dual immunomodulatory
In addition, CD8+ Tex can be induced by IL-18 in the TME via the IL-2/STAT5/mTOR pathway. IL-18 may promote the deterioration of pancreatic cancer (118). Conversely, in a study of CAR-T cell therapy targeting Delta-like protein 3 (DLL-3), IL-18 released by CAR-T cells inhibited the upregulation of immune checkpoints PD-1, TIGIT, LAG3, and TIM-3 of DCs (119). IL-18 production increases CAR-T and CD8+ T cells’ activation. We believe that IL-2 may have a role in the dual function of IL-18 in tumor immunity, and further in-depth study is needed.
2.4.2.4 IL-2 and Tregs have dual immunomodulatory
IL-2 high-affinity receptor (IL-2Rαβγ) is continuously expressed on Tregs. Low levels of IL-2 selectively bind to the high-affinity receptor IL-2Rαβγ of Tregs but do not activate T cells in the TME. Foxp3 and CD25 expression of Tregs need to be maintained by IL-2. Because it cannot produce IL-2, Tregs compete with CD8+ T cells to deplete IL-2 in the TME, thus preventing the anti-tumor immunity of CD8+ T cells (120). As mentioned above, the impaired effector function of CD8+ T cells in the TME and the appearance of exhausted phenotype are positively correlated with the accumulation of Tregs. The study of Noyes D et al. demonstrated that Tregs mediated the depletion of IL-2 in the TME and further exacerbated the exhaustion of CD8+ T cells (121). However, in a clinical study of Dasatinib on chronic myelocytic leukemia (CML), patients taking Dasatinib had significantly reduced plasma IL-2 levels, suppressed STAT5 phosphorylation in Tregs cells, and TIM-3-mediated exhaustion of CD8+ T cells (122). How to stimulate CD8+ T cells without inducing TME Tregs has proven a significant obstacle in the development of anticancer treatments targeting IL-2.
3 Immune cell-CD8+ Tex crosstalk in cancer immunotherapy
Recently, although emerging immunotherapy has made indisputable great progress in clinical application, there are also some cases of poor therapeutic effect. T-cell exhaustion directly affects the efficacy of ICB therapy and ACT therapy. We summarize the role of immune cells in the TME-CD8+ Tex in ICB and act to better cope with the challenges of complex TME (Figure 2).
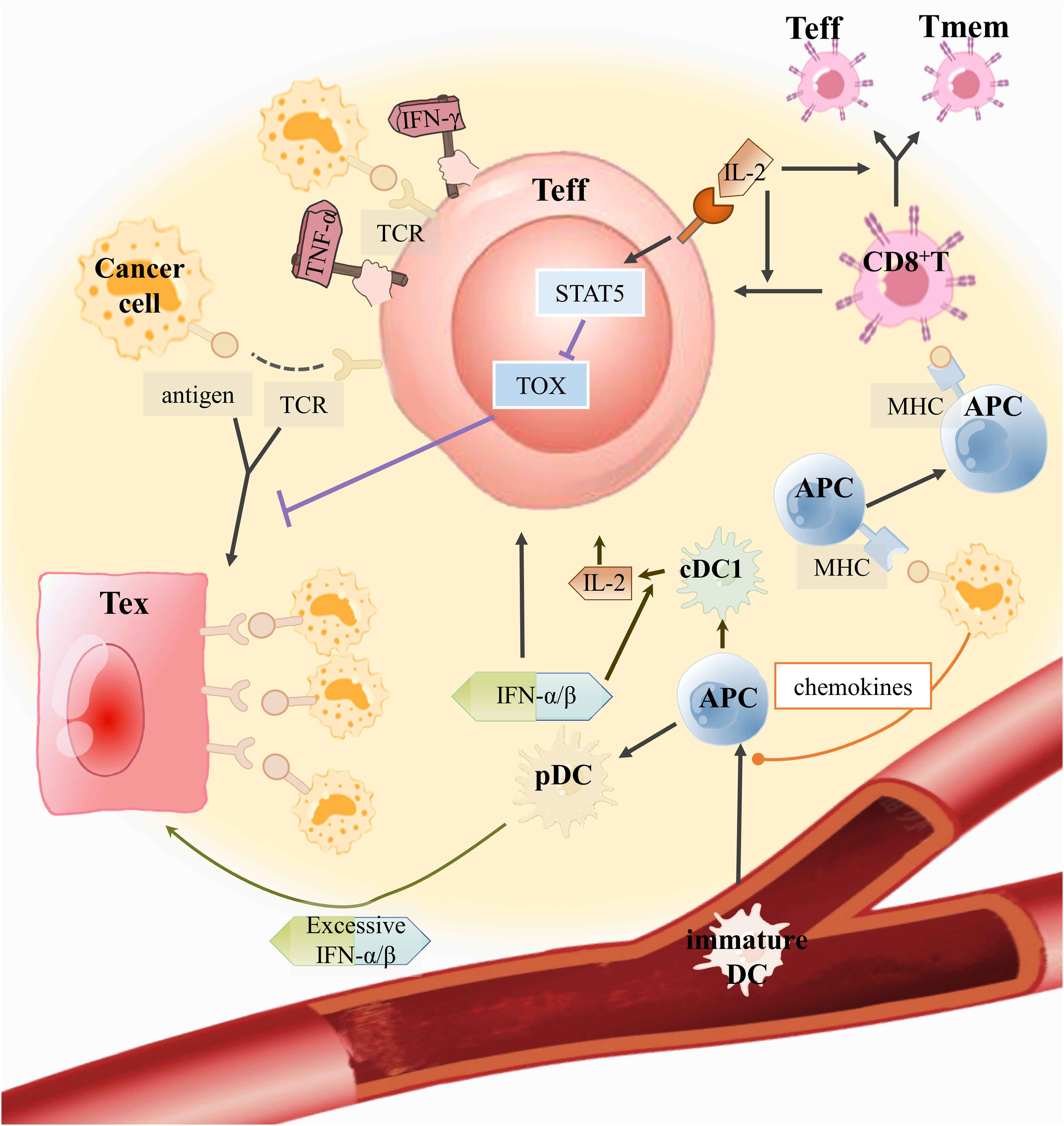
Figure 2. How can good helpers of CD8+ T cells inhibit Tex and assist anti-tumor immunity in the TME? Immature DCs can sense chemokines secreted by tumor cells to develop into APCs and enter the TME to recognize tumor antigens. Tumor antigens are expressed by APC, which further stimulates CD8+ T cells to develop into Teff and memory T cells (Tmem). Plasmacytoid pre-dendritic cells (pDCs) secrete IFN-α/β, which can improve Teff activity and encourage the maturation of APCs into conventional dendritic cell 1(cDC1) to produce IL-2. IL-2 produced by cDC1 will bind to the IL-2R on Teffs to mediate the STAT5/TOX pathway to inhibit excessive antigen-stimulated Teffs toward exhaustion. In addition, IL-2 can also encourage CD8+ T cell growth. However, excessive IFN-α/β production by pDCs would promote Teff toward CD8+ Tex.
3.1 ICB
ICB therapy has made great progress in tumor treatment, but it is only effective for a small number of patients. There are many factors that affect ICB therapy, among which the interference effect of the immunosuppressive state composed of immunosuppressive cells (Tregs, MDSCs, and TAMs) in the tumor microenvironment cannot be ignored. Exhausted CD8+ T cells showed up-expression of co-inhibitory receptors (CTLA-4, PD-1, TIM-3, LAG-3, etc.) and downregulation of co-stimulatory receptors (CD28, 4-1BB) (123–125). The co-stimulatory signal is lacking, and the co-inhibitory signal is too strong. Immune cells, which are also engaged in the process of ICB inhibiting Tex, also exhibit significant expression of these inhibitory receptors. In addition, we also summarized the common CAR-T targets in tumors and the combination therapy with ICB (Table 1).
3.1.1 CTLA-4
CTLA-4 is mainly expressed in activated T cells, but also in Tregs (161). CTLA-4 binds to CD80/CD86 on the tumor surface to prevent T cell activation in the TME (121). As mentioned earlier, the exhaustion-like phenotype and the impairment of effectors of CD8+ T cells are positively correlated with the accumulation of Tregs. Tregs have a high level of CTLA-4 expression, which is essential for developing and maintaining the exhaustive phenotype. In addition, inhibiting CTLA-4 signaling can inhibit the recruitment of Tregs and relieve the immunosuppressive effect of Tregs. Ipilimumab and tremelimumab are FDA-approved CTLA-4 inhibitors for clinical use (162, 163). CTLA-4 inhibitors have the ability to inhibit CTLA-4’s binding to CD80/CD86, promote T-cell proliferation, and have anticancer effects (164).
3.1.2 PD-1
The surface of activated T cells, NK cells, DCs, and other cells expresses PD-1. PD-L1 is highly expressed in tumor cells, and the combination of the two mediates tumor immune escape. PD-1 in TME can not only mediate TCR to decide the activation and differentiation of T cells but also activate Tregs. Therefore, PD-1 plays a non-negligible role in the anti-tumor immunity of remodeling the TME (165, 166). PD-1 is upregulated on T cells of renal angiomyolipoma and pulmonary lymphangioleiomyomatosis. Blocking both PD-1 and CTLA-4 together is more efficient than targeting just one PD-1 inhibitor (167). After the combined blockade of PD-1 and CTLA-4 in the orthotopic hepatocellular carcinoma model, PD-1 was expressed in moderate amounts on Texprog, and Texterm almost disappeared in the TME. Hence, the combined treatment downregulated PD-1 expression and reversed the anti-tumor immunity of Tex (168). These studies confirm that combination therapy is better at reducing CD8+ Tex. Although the efficacy of combination therapy is better, the immune-related adverse reactions (irAEs) related to ICB drugs are more serious (169). Therefore, a significant issue with ICB therapy is how to balance its effects with the emergence of irAEs, and more research is needed. In addition, whether PD-1 inhibitors combined with other immunotherapies can reverse T-cell exhaustion is a hot topic of future research (170–172).
3.1.3 TIM3
TIM3 is expressed in Tregs, TAMs, DCS, natural killer cells (NKs), and mast cells. TIM3 is a key cytokine for Texterm and is at the junction of T-cell exhaustion and rejuvenation (124, 173). The interaction between TIM3 and galectin 9 (Gal9) in CD8+ T cells leads to the reduction of the production of cytokines (IFNγ, IL-2, and TNFα), the inhibition of T cell proliferation, and the inhibition of anti-tumor immunity (174). The progression of tumors can be effectively controlled by simultaneously inhibiting TIM3 and PD1. Datar I et al. indicated that lung cancer patients with TIM3+ CD68+ TAM had shorter survival and worse prognosis than those with TIM3- CD68+ TAM (175, 176). TIM3+ Treg is also more immunosuppressive than TIM3-Treg (177). In addition, the lack of TIM3 on DCs promotes the proliferation of CD8+ T cells in the TME (178).
3.1.4 LAG-3
LAG-3 is an inhibitory receptor protein on activated T cells, NK cells, B cells, plasma cells, and DCs. In the study of Kano Y et al., the soluble recombinant protein LAG-3-Ig, which inhibits the LAG-3 signal, was combined with a tumor vaccine, and it was found that they could down-regulate the expression of LAG-3 and other co-inhibitory receptors on CD8+ T cells, prevent Tex, and boost the tumor vaccine’s therapeutic impact in concert (179). The expression level of LAG-3 increases with the stimulation of tumor antigens. Under long-term stimulation, LAG-3 and other co-inhibitory receptors are continuously expressed on T cells and mediate the exhaustion of T cells (180). Research has demonstrated that LAG3 inhibition can change Tex’s activity. In comparison to single inhibition, dual inhibition of PD-1 and LAG3 produces higher T-cell activation and reduces Tex (181, 182).
3.2 ACT
ACT refers to the transformation, expansion, and quality inspection of immune cells isolated from patients in vitro, and then reinfused into patients to play an anti-tumor role. The ACT includes CAR-T and TCR-T, and we emphasize CAR-T therapy. Tex is a main challenge affecting the therapeutic effect of CAR-T (183). CAR-T cell therapy can be improved by enhancing chimeric antigen receptors and concentrating on how immune cells affect CAR-T cells (184).
3.2.1 CAR-T
Recent years have seen a remarkable development in CAR-T cell therapy. By collecting isolated T cells, and integrating antibodies targeting cells, CAR-T cells are generated and reinfused into patients to kill tumor cells (184). CAR-T can induce the remission of hematological system tumors effectively, but there is still a problem of recurrence in some patients (185). When it comes to treating solid tumors, the therapeutic effect of CAR-T cells is limited, which is characterized by poor penetration, persistence, and low proliferation ability. Therefore, choosing a target has been a hot subject in CAR-T cell therapy research (186–188). CAR-T cells and endogenous CD8+ T cells have similar gene expression and chromatin accessibility, so CAR-T cells will also be stimulated by sustained antigens during anti-tumor immunity, driving CAR-T cell exhaustion (189). Furthermore, the immunosuppressive cells also take part in the CAR-T cells’ exhaustion (23, 190).
3.2.1.1 CD4+/Treg-CAR-T
Tregs may encourage CD8+ T cells’ exhaustion. The immunosuppressive effect mediated by Tregs is often applied to autoimmune diseases (191). Recently, CAR-Treg with antigen specificity has emerged, which is similar to the role of Treg (11). Interestingly, CAR-Tregs that are chronically stimulated by antigens will also have a similar exhaustion state and loss of effector function (10). Tregs as well as CD8+ T cells showed exhaustion-related elevation of immunological checkpoint molecules (165, 192). Luo Y et al. indicated that the neoadjuvant treatment of ovarian cancer with PARP inhibitor (PARPi) can exhaust Tregs in the TME and inhibit tumor growth (193). Treg exhaustion may lead to the development of a novel anti-tumor immune cell therapy, despite the fact that Tregs have the impact of suppressing anti-tumor immunity in the TME. Increased effector T cells and induction of Tregs can be seen in gut‐associated lymphoid tissue, which leads to improved clinical outcomes of cancer immunotherapy with lower incidence of immune‐related adverse events (194).
It is well known that Tregs is one of the cell subsets produced by CD4+ T cell differentiation. Boulch M et al. used the functional intravital image to find that anti-CD19+ CD4+ CAR-T cells mainly derived IFN-γ that diffuses in the TME and directly acts on tumor cells in treatment-responsive B-cell lymphoma (195). At the same time, Kruse B et al. found that TRP-1 CD4+ T cells isolated from ACT mice receiving CD4+ T could reprogram the myeloid cells network in the TME, which means IFN-γ produced by CD4+ T and myeloid cell-derived iNOS produced a tumor-killing phenotype through a synergistic effect (196). In addition, CXCL13+ Th cells, another cell subset differentiated by CD4+ T cells, and DC cells were shown to reactivate exhausted progenitor cells to effective antitumor CD8+ T cells after PD-1 blockade (197). In a recent study, CD4+ T cells combined with effector CD8+ T on the same DC cells to form a three-cell-type cluster to promote the toxicity of CD8+ T cells and eliminate tumor cells (198). The specific combination of this triad in TME will affect the clinical response of patients in ICB treatment (198). Therefore, in-depth understanding and research on the functions and regulatory mechanisms of CD4+ T cells will help to develop new CAR-CD4+ T anti-tumor therapies.
3.2.1.2 MDSC and CAR-T
The recruitment of MDSCs will promote the development of Tex. Cytokines can modify CAR-T cells, which can inhibit MDSCs and improve therapeutic efficacy. CAR-T cells modified by different cytokines can inhibit MDSCs and enhance the therapeutic effect. In the study of Sun et al., Olaparib may inhibit the release of SDF1 α through HIF-1α, further restrict the recruitment of MDSCs to breast cancer tissues, reduce T-cell exhaustion, and raise CAR-T cell effectiveness (199). Next, Sun et al. also discovered that CAR-T cells modified with CXCR4 inhibited the recruitment of MDSCs through the STAT3/NF-kB/SDF-1α axis, enhancing the therapeutic effect in pancreatic cancer (200). Similarly, Liu et al. found that CAR-T targeting fibroblast activating protein (FAP) can also inhibit MDSCs recruitment, increase Tex, and improve pancreatic cancer (152). IL15Rα is preferentially expressed in MDSCs of glioblastoma, and IL15R-modified CAR-T cells can dual target MDSCs and tumor cells (201). Moreover, targeting TREM2 on MDSCs and TAMs can improve anti-PD-1 therapy’s effectiveness. Chen et al. created CAR-T cells secreting PD-1-TREM2 single chain variable fragment (scFv), which has a good effect on lowering the recruitment of MDSCs and TAMs in colorectal cancer (202).
3.2.1.3 TAM and CAR-M
CAR-T cells can mediate the elimination of TAMs and increase CD8+ T cells’ expression. Recently, CAR-modified macrophage (CAR-M) therapy has garnered a lot of interest. Brown BD et al. constructed CAR-T cells targeting pan-macrophage marker F4/80, secreted IFN-γ, promoted the upregulation of MHC molecules on cancer cells and myeloid cells, effectively killed TAMs, and increased the activity and proliferation of CD8+ T cells (203). Rodriguez-Garcia A et al. indicated that CAR-T cells mediated the elimination of TAMs expressing folate receptor β, increased CD8+ T cells, and inhibited ovarian cancer growth (204). Human macrophages are altered by particular CARs to increase their phagocytic activity and ability to deliver antigens to malignancies. Compared with CAR-T cells, CAR-M can penetrate deeper into tumors, promote antigen presentation, and enhance the effect of CD8+ T cells (205).
3.2.1.4 DC and CAR-T
CAR-T will increase DC infiltration and increase CD8+ T cells. Conversely, the DC vaccine will also improve CAR-T cell effectiveness (206). CAR-T promotes the phagocytosis of DCs and triggers the initiation of endogenous CD8+ T cells. During this process, CAR-T secretes IFN-γ, and its metabolism gradually tends to oxidative phosphorylation (207). Sun et al. combined the DC-tumor fusion vaccine with CAR-T cells. DC-tumor fusion vaccine enhanced the number of CD8+ T cells, attenuated T-cell exhaustion, and enhanced the therapeutic effect in solid tumors (208).
3.2.2 TCR-T
TCR-T is to transfer the TCR gene sequence into T cells through genetic engineering technology, specifically recognizes tumor antigens, and inhibits tumors (184). Similar to the problems faced by CAR-T cells in the TME, TCR-T cells lack long-term persistence and are limited by immunosuppressive cells in the TME, resulting in limited therapeutic effect and TCR-T cell exhaustion (209). According to Cianciotti BC et al., knocking down the co-inhibitory receptors TIM-3 and LAG-3 connected to T cell exhaustion can resist the depletion of TCR-T cells and restore its antitumor effect (210).
4 Discussion and conclusion
T cell exhaustion may be an adaptive response to prevent cell death induced by overstimulation and activation in the TME and is regulated by multiple mechanisms during tumorigenesis. In the TME, immune cells (Tregs, MDSCs, and TAMs) regulate intercellular information transmission, inhibit the inflammatory microenvironment and regulate the expression of cell surface-related molecules, maintain the dynamic changes of the TME, and weaken the anti-tumor immune effect, metabolism, transcription and epigenetic regulation of CD8+ T cells. We also described how CD8+ T cells were coerced to exhaustion by Tregs, MDSCs, and TAMs after losing the assistance of DCs.
We summarized the crosstalk between immune cells-CD8+ Tex. Therefore, immunotherapy for tumor patients should not only focus on the anti-tumor immunity of tumor cells themselves, but also on the immunosuppressive cells in the TME that help tumor cells survive and escape. The immunosuppressive cells of the immune system in the body are not naturally the helper of tumor cells but interfere in the TME and stand on the opposite side of CD8+ T cells. However, it is not enough for our review to focus only on the crosstalk of CD8+ Tex with immune cells in the TME. Because complex TME also includes cancer-associated fibroblasts and other cells. How these cells together with immune cells create an immunosuppressive environment can be further explored. In addition, we also hope that the research of ICB therapy and ACT can also pay more attention to the crosstalk between immune cells-CD8+ Tex to create the possibility of improving effect. In conclusion, we believe that the immune system of healthy people is in a process of dynamic balance. The negative effects of immune cells in the TME need to be reversed in anti-tumor treatment, and new treatment methods should be found to make it become a roadblock for CD8+ T cells to become exhausted and a defender of anti-tumor immune effects.
Author contributions
BZ: Conceptualization, Supervision, Writing – original draft, Writing – review & editing. JL: Conceptualization, Writing – original draft, Writing – review & editing. YM: Conceptualization, Writing – original draft, Writing – review & editing. KZ: Writing – original draft. BH: Conceptualization, Writing – original draft, Writing – review & editing. DS: Conceptualization, Supervision, Writing – original draft, Writing – review & editing.
Funding
The author(s) declare that no financial support was received for the research, authorship, and/or publication of this article.
Conflict of interest
The authors declare that the research was conducted in the absence of any commercial or financial relationships that could be construed as a potential conflict of interest.
Publisher’s note
All claims expressed in this article are solely those of the authors and do not necessarily represent those of their affiliated organizations, or those of the publisher, the editors and the reviewers. Any product that may be evaluated in this article, or claim that may be made by its manufacturer, is not guaranteed or endorsed by the publisher.
References
1. Ritter AT, Asano Y, Stinchcombe JC, Dieckmann NM, Chen BC, Gawden-Bone C, et al. Actin depletion initiates events leading to granule secretion at the immunological synapse. Immunity. (2015) 42:864–76. doi: 10.1016/j.immuni.2015.04.013
2. Dolina JS, Van Braeckel-Budimir N, Thomas GD, Salek-Ardakani S. Cd8(+) T cell exhaustion in cancer. Front Immunol. (2021) 12:715234. doi: 10.3389/fimmu.2021.715234
3. Moskophidis D, Lechner F, Pircher H, Zinkernagel RM. Virus persistence in acutely infected immunocompetent mice by exhaustion of antiviral cytotoxic effector T cells. Nature. (1993) 362:758–61. doi: 10.1038/362758a0
4. McLane LM, Abdel-Hakeem MS, Wherry EJ. Cd8 T cell exhaustion during chronic viral infection and cancer. Annu Rev Immunol. (2019) 37:457–95. doi: 10.1146/annurev-immunol-041015-055318
5. Beltra JC, Manne S, Abdel-Hakeem MS, Kurachi M, Giles JR, Chen Z, et al. Developmental relationships of four exhausted Cd8(+) T cell subsets reveals underlying transcriptional and epigenetic landscape control mechanisms. Immunity. (2020) 52:825–41.e8. doi: 10.1016/j.immuni.2020.04.014
6. Wherry EJ, Kurachi M. Molecular and cellular insights into T cell exhaustion. Nat Rev Immunol. (2015) 15:486–99. doi: 10.1038/nri3862
7. Kasakovski D, Xu L, Li Y. T cell senescence and car-T cell exhaustion in hematological Malignancies. J Hematol Oncol. (2018) 11:91. doi: 10.1186/s13045-018-0629-x
8. Guan Q, Han M, Guo Q, Yan F, Wang M, Ning Q, et al. Strategies to reinvigorate exhausted Cd8(+) T cells in tumor microenvironment. Front Immunol. (2023) 14:1204363. doi: 10.3389/fimmu.2023.1204363
9. Chow A, Perica K, Klebanoff CA, Wolchok JD. Clinical implications of T cell exhaustion for cancer immunotherapy. Nat Rev Clin Oncol. (2022) 19:775–90. doi: 10.1038/s41571-022-00689-z
10. Lamarche C, Ward-Hartstonge K, Mi T, Lin DTS, Huang Q, Brown A, et al. Tonic-signaling chimeric antigen receptors drive human regulatory T cell exhaustion. Proc Natl Acad Sci U S A. (2023) 120:e2219086120. doi: 10.1073/pnas.2219086120
11. Arjomandnejad M, Kopec AL, Keeler AM. Car-T regulatory (Car-treg) cells: engineering and applications. Biomedicines. (2022) 10:287. doi: 10.3390/biomedicines10020287
12. Sawant DV, Yano H, Chikina M, Zhang Q, Liao M, Liu C, et al. Adaptive plasticity of Il-10(+) and Il-35(+) T(Reg) cells cooperatively promotes tumor T cell exhaustion. Nat Immunol. (2019) 20:724–35. doi: 10.1038/s41590-019-0346-9
13. Shiri AM, Zhang T, Bedke T, Zazara DE, Zhao L, Lücke J, et al. Il-10 dampens antitumor immunity and promotes liver metastasis via Pd-L1 induction. J Hepatol. (2024) 80:634–44. doi: 10.1016/j.jhep.2023.12.015
14. Banerjee H, Nieves-Rosado H, Kulkarni A, Murter B, McGrath KV, Chandran UR, et al. Expression of Tim-3 drives phenotypic and functional changes in treg cells in secondary lymphoid organs and the tumor microenvironment. Cell Rep. (2021) 36:109699. doi: 10.1016/j.celrep.2021.109699
15. Song J, Lin Z, Liu Q, Huang S, Han L, Fang Y, et al. Mir-192-5p/Rb1/Nf-Kbp65 signaling axis promotes Il-10 secretion during gastric cancer Emt to induce Treg cell differentiation in the tumour microenvironment. Clin Trans Med. (2022) 12:e992. doi: 10.1002/ctm2.992
16. Salkeni MA, Naing A. Interleukin-10 in cancer immunotherapy: from bench to bedside. Trends Cancer. (2023) 9:716–25. doi: 10.1016/j.trecan.2023.05.003
17. Ma M, Li L, Yang SH, Huang C, Zhuang W, Huang S, et al. Lymphatic endothelial cell-mediated accumulation of Cd177(+)Treg cells suppresses antitumor immunity in human esophageal squamous cell carcinoma. Oncoimmunology. (2024) 13:2327692. doi: 10.1080/2162402x.2024.2327692
18. Lin C, Lin CN, Wang YC, Liu FY, Chuang YJ, Lan CY, et al. The role of Tgf-B signaling and apoptosis in innate and adaptive immunity in Zebrafish: A systems biology approach. BMC Syst Biol. (2014) 8:116. doi: 10.1186/s12918-014-0116-0
19. Lu Z, Ding L, Ding H, Hao F, Pu Y, Wang Y, et al. Tumor cell-derived Tgf-B at tumor center independently predicts recurrence and poor survival in oral squamous cell carcinoma. J Oral Pathol Med. (2019) 48:696–704. doi: 10.1111/jop.12888
20. Tritschler I, Gramatzki D, Capper D, Mittelbronn M, Meyermann R, Saharinen J, et al. Modulation of Tgf-Beta activity by latent Tgf-beta-binding protein 1 in human Malignant glioma cells. Int J Cancer. (2009) 125:530–40. doi: 10.1002/ijc.24443
21. Moreau JM, Velegraki M, Bolyard C, Rosenblum MD, Li Z. Transforming growth factor-B1 in regulatory T cell biology. Sci Immunol. (2022) 7:eabi4613. doi: 10.1126/sciimmunol.abi4613
22. Kondo Y, Suzuki S, Takahara T, Ono S, Goto M, Miyabe S, et al. Improving function of cytotoxic T-lymphocytes by transforming growth factor-B Inhibitor in oral squamous cell carcinoma. Cancer Sci. (2021) 112:4037–49. doi: 10.1111/cas.15081
23. Tang N, Cheng C, Zhang X, Qiao M, Li N, Mu W, et al. Tgf-B Inhibition via crispr promotes the long-term efficacy of car T cells against solid tumors. JCI Insight. (2020) 5:e133977. doi: 10.1172/jci.insight.133977
24. Polanczyk MJ, Walker E, Haley D, Guerrouahen BS, Akporiaye ET. Blockade of Tgf-B Signaling to enhance the antitumor response is accompanied by dysregulation of the functional activity of Cd4(+)Cd25(+)Foxp3(+) and Cd4(+)Cd25(-)Foxp3(+) T cells. J Trans Med. (2019) 17:219. doi: 10.1186/s12967-019-1967-3
25. Li A, Chang Y, Song NJ, Wu X, Chung D, Riesenberg BP, et al. Selective targeting of Garp-ltgfβ Axis in the tumor microenvironment augments Pd-1 blockade via enhancing Cd8(+) T cell antitumor immunity. J Immunotherap Cancer. (2022) 10:e005433. doi: 10.1136/jitc-2022-005433
26. Montauti E, Weinberg SE, Chu P, Chaudhuri S, Mani NL, Iyer R, et al. A deubiquitination module essential for T(Reg) fitness in the tumor microenvironment. Sci Adv. (2022) 8:eabo4116. doi: 10.1126/sciadv.abo4116
27. Wiley MB, Bauer J, Mehrotra K, Zessner-Spitzenberg J, Kolics Z, Cheng W, et al. Non-canonical activin a signaling stimulates context-dependent and cellular-specific outcomes in Crc to promote tumor cell migration and immune tolerance. Cancers. (2023) 15:3003. doi: 10.3390/cancers15113003
28. Gabrilovich DI. Myeloid-derived suppressor cells. Cancer Immunol Res. (2017) 5:3–8. doi: 10.1158/2326-6066.Cir-16-0297
29. Feng X, Meng M, Li H, Gao Y, Song W, Di R, et al. T-cell dysfunction in natural killer/T-cell lymphoma. Oncoimmunology. (2023) 12:2212532. doi: 10.1080/2162402x.2023.2212532
30. Scheller J, Chalaris A, Schmidt-Arras D, Rose-John S. The pro- and anti-inflammatory properties of the cytokine interleukin-6. Biochim Biophys Acta. (2011) 1813:878–88. doi: 10.1016/j.bbamcr.2011.01.034
31. Gulubova M, Chonov D, Aleksandrova E, Ivanova K, Ignatova MM, Vlaykova T. Interleukin-6-positive immune cells as a possible new immunologic marker associated with the colorectal cancer prognosis. Appl immunohistochemistry Mol morphology: AIMM. (2024) 32:233–43. doi: 10.1097/pai.0000000000001198
32. Patel SA, Gooderham NJ. Il6 mediates immune and colorectal cancer cell cross-talk via Mir-21 and Mir-29b. Mol Cancer Res: MCR. (2015) 13:1502–8. doi: 10.1158/1541-7786.Mcr-15-0147
33. Chang Q, Bournazou E, Sansone P, Berishaj M, Gao SP, Daly L, et al. The Il-6/Jak/Stat3 feed-forward loop drives tumorigenesis and metastasis. Neoplasia (New York NY). (2013) 15:848–62. doi: 10.1593/neo.13706
34. Fu XL, Duan W, Su CY, Mao FY, Lv YP, Teng YS, et al. Interleukin 6 induces M2 macrophage differentiation by stat3 activation that correlates with gastric cancer progression. Cancer Immunol Immunother: CII. (2017) 66:1597–608. doi: 10.1007/s00262-017-2052-5
35. Yang H, Kang B, Ha Y, Lee SH, Kim I, Kim H, et al. High serum Il-6 correlates with reduced clinical benefit of atezolizumab and bevacizumab in unresectable hepatocellular carcinoma. JHEP reports: Innovation Hepatol. (2023) 5:100672. doi: 10.1016/j.jhepr.2023.100672
36. Johnson DE, O'Keefe RA, Grandis JR. Targeting the Il-6/Jak/Stat3 signalling axis in cancer. Nat Rev Clin Oncol. (2018) 15:234–48. doi: 10.1038/nrclinonc.2018.8
37. Sasidharan Nair V, Saleh R, Toor SM, Taha RZ, Ahmed AA, Kurer MA, et al. Transcriptomic profiling disclosed the role of DNA methylation and histone modifications in tumor-infiltrating myeloid-derived suppressor cell subsets in colorectal cancer. Clin Epigenet. (2020) 12:13. doi: 10.1186/s13148-020-0808-9
38. Zhu H, Gu Y, Xue Y, Yuan M, Cao X, Liu Q. Cxcr2(+) Mdscs promote breast cancer progression by inducing Emt and activated T cell exhaustion. Oncotarget. (2017) 8:114554–67. doi: 10.18632/oncotarget.23020
39. Wang T, Wang J, Jiang H, Ni M, Zou Y, Chen Y, et al. Targeted regulation of tumor microenvironment through the inhibition of mdscs by curcumin loaded self-assembled nano-filaments. Materials Today Bio. (2022) 15:100304. doi: 10.1016/j.mtbio.2022.100304
40. Dhar S, Chakravarti M, Ganguly N, Saha A, Dasgupta S, Bera S, et al. High monocytic mdsc signature predicts multi-drug resistance and cancer relapse in non-Hodgkin lymphoma patients treated with R-chop. Front Immunol. (2023) 14:1303959. doi: 10.3389/fimmu.2023.1303959
41. Tseng HY, Alavi S, Gallagher S, McGuire HM, Hersey P, Al Emran A, et al. Bet inhibition sensitizes innate checkpoint inhibitor resistant melanoma to anti-ctla-4 treatment. Pigm Cell Melanoma R. (2024) . doi: 10.1111/pcmr.13174
42. Koh J, Kim Y, Lee KY, Hur JY, Kim MS, Kim B, et al. Mdsc subtypes and Cd39 expression on Cd8(+) T cells predict the efficacy of anti-Pd-1 immunotherapy in patients with advanced Nsclc. Eur J Immunol. (2020) 50:1810–9. doi: 10.1002/eji.202048534
43. Tao J, Han D, Gao S, Zhang W, Yu H, Liu P, et al. Cd8(+) T cells exhaustion induced by myeloid-derived suppressor cells in myelodysplastic syndromes patients might be through Tim3/Gal-9 pathway. J Cell Mol Med. (2020) 24:1046–58. doi: 10.1111/jcmm.14825
44. Kim CG, Kim MH, Kim JH, Kim SG, Kim GM, Kim TY, et al. On-treatment derived neutrophil-to-lymphocyte ratio and survival with palbociclib and endocrine treatment: analysis of a multicenter retrospective cohort and the paloma-2/3 study with immune correlates. Breast Cancer Res: BCR. (2023) 25:4. doi: 10.1186/s13058-022-01601-4
45. Umansky V, Blattner C, Gebhardt C, Utikal J. The role of myeloid-derived suppressor cells (Mdsc) in cancer progression. Vaccines. (2016) 4:36. doi: 10.3390/vaccines4040036
46. Labib Salem M, Zidan AA, Ezz El-Din El-Naggar R, Attia Saad M, El-Shanshory M, Bakry U, et al. Myeloid-derived suppressor cells and regulatory T cells share common immunoregulatory pathways-related micrornas that are dysregulated by acute lymphoblastic leukemia and chemotherapy. Hum Immunol. (2021) 82:36–45. doi: 10.1016/j.humimm.2020.10.009
47. Zidan M, Zidan AA, Attia Saad M, El-Shanshory M, Bakry U, Sobh A, et al. Altered microrna expression profile is linked to T-cell exhaustion-related pathways in pediatric patients with acute lymphoblastic leukemia. Hum Immunol. (2023) 84:113–22. doi: 10.1016/j.humimm.2022.10.005
48. Anestakis D, Petanidis S, Domvri K, Tsavlis D, Zarogoulidis P, Katopodi T. Carboplatin chemoresistance is associated with Cd11b(+)/Ly6c(+) myeloid release and upregulation of tigit and lag3/cd160 exhausted T cells. Mol Immunol. (2020) 118:99–109. doi: 10.1016/j.molimm.2019.11.008
49. Wu L, Mao L, Liu JF, Chen L, Yu GT, Yang LL, et al. Blockade of Tigit/Cd155 signaling reverses T-cell exhaustion and enhances antitumor capability in head and neck squamous cell carcinoma. Cancer Immunol Res. (2019) 7:1700–13. doi: 10.1158/2326-6066.Cir-18-0725
50. Curcio C, Mucciolo G, Roux C, Brugiapaglia S, Scagliotti A, Guadagnin G, et al. Pi3kγ Inhibition combined with DNA vaccination unleashes a B-cell-dependent antitumor immunity that hampers pancreatic cancer. J Exp Clin Cancer research: CR. (2024) 43:157. doi: 10.1186/s13046-024-03080-1
51. Pullikuth AK, Routh ED, Zimmerman KD, Chifman J, Chou JW, Soike MH, et al. Bulk and single-cell profiling of breast tumors identifies trem-1 as a dominant immune suppressive marker associated with poor outcomes. Front Oncol. (2021) 11:734959. doi: 10.3389/fonc.2021.734959
52. Ho CC, Liao WY, Wang CY, Lu YH, Huang HY, Chen HY, et al. Trem-1 expression in tumor-associated macrophages and clinical outcome in lung cancer. Am J Respir Crit Care Med. (2008) 177:763–70. doi: 10.1164/rccm.200704-641OC
53. Liao R, Sun TW, Yi Y, Wu H, Li YW, Wang JX, et al. Expression of trem-1 in hepatic stellate cells and prognostic value in hepatitis B-related hepatocellular carcinoma. Cancer Sci. (2012) 103:984–92. doi: 10.1111/j.1349-7006.2012.02273.x
54. Ford JW, Gonzalez-Cotto M, MacFarlane A, Peri S, Howard OMZ, Subleski JJ, et al. Tumor-infiltrating myeloid cells co-express trem1 and trem2 and elevated trem-1 associates with disease progression in renal cell carcinoma. Front Oncol. (2021) 11:662723. doi: 10.3389/fonc.2021.662723
55. Ajith A, Mamouni K, Horuzsko DD, Musa A, Dzutsev AK, Fang JR, et al. Targeting trem1 augments antitumor T cell immunity by inhibiting myeloid-derived suppressor cells and restraining anti-Pd-1 resistance. J Clin Invest. (2023) 133:e167951. doi: 10.1172/jci167951
56. Nie F, Chen Y, Hu Y, Huang P, Shi X, Cai J, et al. Trem1/dap12 based novel multiple chain car-T cells targeting dll3 show robust anti-tumour efficacy for small cell lung cancer. Immunology. (2024) 172:362–74. doi: 10.1111/imm.13776
57. Xie J, Zheng Z, Tuo L, Deng X, Tang H, Peng C, et al. Recent advances in exosome-based immunotherapy applied to cancer. Front Immunol. (2023) 14:1296857. doi: 10.3389/fimmu.2023.1296857
58. Jia X, Xi J, Tian B, Zhang Y, Wang Z, Wang F, et al. The tautomerase activity of tumor exosomal Mif promotes pancreatic cancer progression by modulating mdsc differentiation. Cancer Immunol Res. (2024) 12:72–90. doi: 10.1158/2326-6066.Cir-23-0205
59. Qi Y, Jin C, Qiu W, Zhao R, Wang S, Li B, et al. The dual role of glioma exosomal micrornas: glioma eliminates tumor suppressor Mir-1298-5p via exosomes to promote immunosuppressive effects of Mdscs. Cell Death Dis. (2022) 13:426. doi: 10.1038/s41419-022-04872-z
60. Rashid MH, Borin TF, Ara R, Piranlioglu R, Achyut BR, Korkaya H, et al. Critical immunosuppressive effect of mdsc−Derived exosomes in the tumor microenvironment. Oncol Rep. (2021) 45:1171–81. doi: 10.3892/or.2021.7936
61. Boutilier AJ, Elsawa SF. Macrophage polarization states in the tumor microenvironment. Int J Mol Sci. (2021) 22:6995. doi: 10.3390/ijms22136995
62. Liu C, Xie J, Lin B, Tian W, Wu Y, Xin S, et al. Pan-cancer single-cell and spatial-resolved profiling reveals the immunosuppressive role of Apoe+ Macrophages in immune checkpoint inhibitor therapy. Advanced Sci (Weinheim Baden-Wurttemberg Germany). (2024) 11:e2401061. doi: 10.1002/advs.202401061
63. Yin C, Han Q, Xu D, Zheng B, Zhao X, Zhang J. Sall4-mediated upregulation of exosomal Mir-146a-5p drives T-cell exhaustion by M2 tumor-associated macrophages in Hcc. Oncoimmunology. (2019) 8:1601479. doi: 10.1080/2162402x.2019.1601479
64. Pu J, Xu Z, Nian J, Fang Q, Yang M, Huang Y, et al. M2 macrophage-derived extracellular vesicles facilitate Cd8+T cell exhaustion in hepatocellular carcinoma via the Mir-21-5p/Yod1/Yap/B-catenin pathway. Cell Death Discov. (2021) 7:182. doi: 10.1038/s41420-021-00556-3
65. Kumar V, Gabrilovich DI. Hypoxia-inducible factors in regulation of immune responses in tumour microenvironment. Immunology. (2014) 143:512–9. doi: 10.1111/imm.12380
66. Zhu Y, Wang A, Zhang S, Kim J, Xia J, Zhang F, et al. Paclitaxel-loaded ginsenoside Rg3 liposomes for drug-resistant cancer therapy by dual targeting of the tumor microenvironment and cancer cells. J adv Res. (2023) 49:159–73. doi: 10.1016/j.jare.2022.09.007
67. Holtzhausen A, Harris W, Ubil E, Hunter DM, Zhao J, Zhang Y, et al. Tam family receptor kinase inhibition reverses mdsc-mediated suppression and augments anti-Pd-1 therapy in melanoma. Cancer Immunol Res. (2019) 7:1672–86. doi: 10.1158/2326-6066.Cir-19-0008
68. Bu LL, Li YC, Yu GT, Liu JF, Deng WW, Zhang WF, et al. Targeting phosphorylation of stat3 delays tumor growth in Hpv-negative anal squamous cell carcinoma mouse model. Sci Rep. (2017) 7:6629. doi: 10.1038/s41598-017-06643-9
69. Lu Y, Sun Q, Guan Q, Zhang Z, He Q, He J, et al. The Xor-Idh3α Axis controls macrophage polarization in hepatocellular carcinoma. J Hepatol. (2023) 79:1172–84. doi: 10.1016/j.jhep.2023.06.022
70. Kersten K, Hu KH, Combes AJ, Samad B, Harwin T, Ray A, et al. Spatiotemporal co-dependency between macrophages and exhausted Cd8(+) T cells in cancer. Cancer Cell. (2022) 40:624–38.e9. doi: 10.1016/j.ccell.2022.05.004
71. Noman MZ, Desantis G, Janji B, Hasmim M, Karray S, Dessen P, et al. Pd-L1 is a novel direct target of Hif-1α, and its blockade under hypoxia enhanced Mdsc-mediated T cell activation. J Exp Med. (2014) 211:781–90. doi: 10.1084/jem.20131916
72. Lu C, Redd PS, Lee JR, Savage N, Liu K. The expression profiles and regulation of Pd-L1 in tumor-induced myeloid-derived suppressor cells. Oncoimmunology. (2016) 5:e1247135. doi: 10.1080/2162402x.2016.1247135
73. Najafi A, Keykhaee M, Kazemi MH, Karimi MY, Khorramdelazad H, Aghamohamadi N, et al. Catalase-gold nanoaggregates manipulate the tumor microenvironment and enhance the effect of low-dose radiation therapy by reducing hypoxia. Biomedicine pharmacotherapy = Biomedecine pharmacotherapie. (2023) 167:115557. doi: 10.1016/j.biopha.2023.115557
74. Korbecki J, Kojder K, Simińska D, Bohatyrewicz R, Gutowska I, Chlubek D, et al. Cc chemokines in a tumor: A review of pro-cancer and anti-cancer properties of the ligands of receptors Ccr1, Ccr2, Ccr3, and Ccr4. Int J Mol Sci. (2020) 21:8412. doi: 10.3390/ijms21218412
75. Wang Y, Sun Q, Ye Y, Sun X, Xie S, Zhan Y, et al. Fgf-2 signaling in nasopharyngeal carcinoma modulates pericyte-macrophage crosstalk and metastasis. JCI Insight. (2022) 7(10):e157874. doi: 10.1172/jci.insight.157874
76. Heidari A, Sharif PM, Rezaei N. The association between tumor-associated macrophages and glioblastoma: A potential target for therapy. Curr Pharm design. (2021) 27:4650–62. doi: 10.2174/1381612827666210816114003
77. Yue Y, Zhang Q, Sun Z. Cx3cr1 acts as a protective biomarker in the tumor microenvironment of colorectal cancer. Front Immunol. (2021) 12:758040. doi: 10.3389/fimmu.2021.758040
78. Kamat K, Krishnan V, Dorigo O. Macrophage-derived Ccl23 upregulates expression of T-cell exhaustion markers in ovarian cancer. Br J Cancer. (2022) 127:1026–33. doi: 10.1038/s41416-022-01887-3
79. Wang K, Chen X, Lin P, Wu J, Huang Q, Chen ZN, et al. Cd147-K148me2-driven tumor cell-macrophage crosstalk provokes Nsclc immunosuppression via the Ccl5/Ccr5 axis. Advanced Sci (Weinheim Baden-Wurttemberg Germany). (2024) 11:e2400611. doi: 10.1002/advs.202400611
80. Anstee JE, Feehan KT, Opzoomer JW, Dean I, Muller HP, Bahri M, et al. Lyve-1(+) macrophages form a collaborative ccr5-dependent perivascular niche that influences chemotherapy responses in murine breast cancer. Dev Cell. (2023) 58:1548–61.e10. doi: 10.1016/j.devcel.2023.06.006
81. Guan B, Li H, Yao J, Guo J, Yu F, Li G, et al. Ccl3-ccr5 axis promotes cell migration and invasion of colon adenocarcinoma via Akt signaling pathway. Environ Toxicol. (2023) 38:172–84. doi: 10.1002/tox.23675
82. Chen C, Wang S, Wang N, Zheng Y, Zhou J, Hong M, et al. Icariin inhibits prostate cancer bone metastasis and destruction via suppressing Tam/Ccl5-mediated osteoclastogenesis. Phytomedicine: Int J phytotherapy phytopharmacology. (2023) 120:155076. doi: 10.1016/j.phymed.2023.155076
83. Yan M, Wu R, Fu H, Hu C, Hao Y, Zeng J, et al. Integrated analysis of single-cell and bulk Rna sequencing data reveals the association between hypoxic tumor cells and exhausted T cells in predicting immune therapy response. Comput Biol Med. (2024) 171:108179. doi: 10.1016/j.compbiomed.2024.108179
84. Hernández-Verdin I, Akdemir KC, Ramazzotti D, Caravagna G, Labreche K, Mokhtari K, et al. Pan-cancer landscape of aid-related mutations, composite mutations, and their potential role in the Ici response. NPJ Precis Oncol. (2022) 6:89. doi: 10.1038/s41698-022-00331-2
85. Liu Z, Li X, Gao Y, Liu J, Feng Y, Liu Y, et al. Epigenetic reprogramming of runx3 reinforces Cd8 + T-cell function and improves the clinical response to immunotherapy. Mol Cancer. (2023) 22:84. doi: 10.1186/s12943-023-01768-0
86. Feriz AM, Khosrojerdi A, Lotfollahi M, Shamsaki N, GhasemiGol M, HosseiniGol E, et al. Single-cell Rna sequencing uncovers heterogeneous transcriptional signatures in tumor-infiltrated dendritic cells in prostate cancer. Heliyon. (2023) 9:e15694. doi: 10.1016/j.heliyon.2023.e15694
87. Horie M, Takagane K, Itoh G, Kuriyama S, Yanagihara K, Yashiro M, et al. Exosomes secreted by St3gal5(High) cancer cells promote peritoneal dissemination by establishing a premetastatic microenvironment. Mol Oncol. (2024) 18:21–43. doi: 10.1002/1878-0261.13524
88. Petty AJ, Li A, Wang X, Dai R, Heyman B, Hsu D, et al. Hedgehog signaling promotes tumor-associated macrophage polarization to suppress intratumoral Cd8+ T cell recruitment. J Clin Invest. (2019) 129:5151–62. doi: 10.1172/jci128644
89. Pichler R, Siska PJ, Tymoszuk P, Martowicz A, Untergasser G, Mayr R, et al. A chemokine network of T cell exhaustion and metabolic reprogramming in renal cell carcinoma. Front Immunol. (2023) 14:1095195. doi: 10.3389/fimmu.2023.1095195
90. Utsumi T, Suyama T, Imamura Y, Fuse M, Sakamoto S, Nihei N, et al. The association of Cxcr3 and renal cell carcinoma metastasis. J Urol. (2014) 192:567–74. doi: 10.1016/j.juro.2014.01.100
91. Azuma M, Takeda Y, Nakajima H, Sugiyama H, Ebihara T, Oshiumi H, et al. Biphasic function of tlr3 adjuvant on tumor and spleen dendritic cells promotes tumor T cell infiltration and regression in a vaccine therapy. Oncoimmunology. (2016) 5:e1188244. doi: 10.1080/2162402x.2016.1188244
92. Burrack AL, Spartz EJ, Rollins MR, Miller EA, Firulyova M, Cruz E, et al. Cxcr3 constrains pancreatic cancer dissemination through instructing T cell fate. Cancer Immunol Immunother: CII. (2023) 72:1461–78. doi: 10.1007/s00262-022-03338-7
93. Chen IX, Newcomer K, Pauken KE, Juneja VR, Naxerova K, Wu MW, et al. A bilateral tumor model identifies transcriptional programs associated with patient response to immune checkpoint blockade. Proc Natl Acad Sci U S A. (2020) 117:23684–94. doi: 10.1073/pnas.2002806117
94. Chow MT, Ozga AJ, Servis RL, Frederick DT, Lo JA, Fisher DE, et al. Intratumoral activity of the Cxcr3 chemokine system is required for the efficacy of anti-Pd-1 therapy. Immunity. (2019) 50:1498–512.e5. doi: 10.1016/j.immuni.2019.04.010
95. Maurice NJ, McElrath MJ, Andersen-Nissen E, Frahm N, Prlic M. Cxcr3 enables recruitment and site-specific bystander activation of memory Cd8(+) T cells. Nat Commun. (2019) 10:4987. doi: 10.1038/s41467-019-12980-2
96. Silina K. B cell-rich niches support stem-like Cd8(+) T cells in cancer microenvironment. Cancer Cell. (2023) 41:824–5. doi: 10.1016/j.ccell.2023.04.007
97. Gaglia G, Burger ML, Ritch CC, Rammos D, Dai Y, Crossland GE, et al. Lymphocyte networks are dynamic cellular communities in the immunoregulatory landscape of lung adenocarcinoma. Cancer Cell. (2023) 41:871–86.e10. doi: 10.1016/j.ccell.2023.03.015
98. Sullivan PM, Reed SJ, Kalia V, Sarkar S. Solid tumor microenvironment can harbor and support functional properties of memory T cells. Front Immunol. (2021) 12:706150. doi: 10.3389/fimmu.2021.706150
99. Gunderson AJ, Yamazaki T, McCarty K, Fox N, Phillips M, Alice A, et al. Tgfβ Suppresses Cd8(+) T cell expression of Cxcr3 and tumor trafficking. Nat Commun. (2020) 11:1749. doi: 10.1038/s41467-020-15404-8
100. He N, Huang H, Wu S, Ji W, Tai Y, Gao R, et al. Microwave ablation combined with Pd-L1 blockade synergistically promotes Cxcl9-mediated antitumor immunity. Cancer Sci. (2024) 115:2196–208. doi: 10.1111/cas.16182
101. Wu H, Li Y, Shi G, Du S, Wang X, Ye W, et al. Hepatic interferon regulatory factor 8 expression suppresses hepatocellular carcinoma progression and enhances the response to anti-programmed cell death protein-1 therapy. Hepatol (Baltimore Md). (2022) 76:1602–16. doi: 10.1002/hep.32316
102. Nixon BG, Kuo F, Ji L, Liu M, Capistrano K, Do M, et al. Tumor-associated macrophages expressing the transcription factor Irf8 promote T cell exhaustion in cancer. Immunity. (2022) 55:2044–58.e5. doi: 10.1016/j.immuni.2022.10.002
103. Jhunjhunwala S, Hammer C, Delamarre L. Antigen presentation in cancer: insights into tumour immunogenicity and immune evasion. Nat Rev Cancer. (2021) 21:298–312. doi: 10.1038/s41568-021-00339-z
104. Del Prete A, Salvi V, Soriani A, Laffranchi M, Sozio F, Bosisio D, et al. Dendritic cell subsets in cancer immunity and tumor antigen sensing. Cell Mol Immunol. (2023) 20:432–47. doi: 10.1038/s41423-023-00990-6
105. Ferris ST, Durai V, Wu R, Theisen DJ, Ward JP, Bern MD, et al. Cdc1 prime and are licensed by Cd4(+) T cells to induce anti-tumour immunity. Nature. (2020) 584:624–9. doi: 10.1038/s41586-020-2611-3
106. Binnewies M, Mujal AM, Pollack JL, Combes AJ, Hardison EA, Barry KC, et al. Unleashing type-2 dendritic cells to drive protective antitumor Cd4(+) T cell immunity. Cell. (2019) 177:556–71.e16. doi: 10.1016/j.cell.2019.02.005
107. Das A, Chauhan KS, Kumar H, Tailor P. Mutation in Irf8 gene (Irf8(R294c) ) impairs type I Ifn-mediated antiviral immune response by murine Pdcs. Front Immunol. (2021) 12:758190. doi: 10.3389/fimmu.2021.758190
108. Hubo M, Jonuleit H. Plasmacytoid dendritic cells are inefficient in activation of human regulatory T cells. PLoS One. (2012) 7:e44056. doi: 10.1371/journal.pone.0044056
109. Wu T, Ji Y, Moseman EA, Xu HC, Manglani M, Kirby M, et al. The Tcf1-Bcl6 axis counteracts type I interferon to repress exhaustion and maintain T cell stemness. Sci Immunol. (2016) 1:eaai8593. doi: 10.1126/sciimmunol.aai8593
110. Garris CS, Arlauckas SP, Kohler RH, Trefny MP, Garren S, Piot C, et al. Successful anti-Pd-1 cancer immunotherapy requires T cell-dendritic cell crosstalk involving the cytokines Ifn-Γ and Il-12. Immunity. (2018) 49:1148–61.e7. doi: 10.1016/j.immuni.2018.09.024
111. Marius W, Leticia OF, Friedrich KN, Stephan M, Louisa H, Tabea S, et al. Expression of Cd39 is associated with T cell exhaustion in ovarian cancer and its blockade reverts T cell dysfunction. Oncoimmunology. (2024) 13:2346359. doi: 10.1080/2162402x.2024.2346359
112. Wherry EJ, Blattman JN, Murali-Krishna K, van der Most R, Ahmed R. Viral persistence alters Cd8 T-cell immunodominance and tissue distribution and results in distinct stages of functional impairment. J Virol. (2003) 77:4911–27. doi: 10.1128/jvi.77.8.4911-4927.2003
113. Zhang S, Zhao L, Guo M, Liu P, Li S, Xie W, et al. Anticancer effects of ikarugamycin and astemizole identified in a screen for stimulators of cellular immune responses. J. Immunotherap Cancer. (2023) 11:e006785. doi: 10.1136/jitc-2023-006785
114. Beltra JC, Abdel-Hakeem MS, Manne S, Zhang Z, Huang H, Kurachi M, et al. Stat5 Opposes the Transcription Factor Tox and Rewires Exhausted Cd8(+) T cells toward Durable Effector-Like States During Chronic Antigen Exposure. Immunity. (2023) 56:2699–718.e11. doi: 10.1016/j.immuni.2023.11.005
115. Li J, Zhou W, Li D, Huang Y, Yang X, Jiang L, et al. Co-infusion of car T cells with Aapcs expressing chemokines and costimulatory ligands enhances the anti-tumor efficacy in mice. Cancer Lett. (2023) 568:216287. doi: 10.1016/j.canlet.2023.216287
116. Muhammad S, Fan T, Hai Y, Gao Y, He J. Reigniting hope in cancer treatment: the promise and pitfalls of Il-2 and Il-2r targeting strategies. Mol Cancer. (2023) 22:121. doi: 10.1186/s12943-023-01826-7
117. Liu Y, Zhou N, Zhou L, Wang J, Zhou Y, Zhang T, et al. Il-2 regulates tumor-reactive Cd8(+) T cell exhaustion by activating the aryl hydrocarbon receptor. Nat Immunol. (2021) 22:358–69. doi: 10.1038/s41590-020-00850-9
118. Lutz V, Hellmund VM, Picard FSR, Raifer H, Ruckenbrod T, Klein M, et al. Il18 receptor signaling regulates tumor-reactive Cd8+ T-cell exhaustion via activation of the Il2/Stat5/Mtor pathway in a pancreatic cancer model. Cancer Immunol Res. (2023) 11:421–34. doi: 10.1158/2326-6066.Cir-22-0398
119. Jaspers JE, Khan JF, Godfrey WD, Lopez AV, Ciampricotti M, Rudin CM, et al. Il-18-secreting Car T cells targeting dll3 are highly effective in small cell lung cancer models. J Clin Invest. (2023) 133:e166028. doi: 10.1172/jci166028
120. Abbas AK, Trotta E D, Marson A, Bluestone JA. Revisiting Il-2: biology and therapeutic prospects. Sci Immunol. (2018) 3:eaat1482. doi: 10.1126/sciimmunol.aat1482
121. Noyes D, Bag A, Oseni S, Semidey-Hurtado J, Cen L, Sarnaik AA, et al. Tumor-associated Tregs obstruct antitumor immunity by promoting T cell dysfunction and restricting clonal diversity in tumor-infiltrating Cd8+ T cells. J immunotherapy Cancer. (2022) 10:e004605. doi: 10.1136/jitc-2022-004605
122. Harrington P, Dillon R, Radia D, Rousselot P, McLornan DP, Ong M, et al. Differential inhibition of T-cell receptor and Stat5 signaling pathways determines the immunomodulatory effects of dasatinib in chronic phase chronic myeloid leukemia. Haematologica. (2023) 108:1555–66. doi: 10.3324/haematol.2022.282005
123. Philip M, Schietinger A. Cd8(+) T cell differentiation and dysfunction in cancer. Nat Rev Immunol. (2022) 22:209–23. doi: 10.1038/s41577-021-00574-3
124. Miller BC, Sen DR, Al Abosy R, Bi K, Virkud YV, LaFleur MW, et al. Subsets of exhausted Cd8(+) T cells differentially mediate tumor control and respond to checkpoint blockade. Nat Immunol. (2019) 20:326–36. doi: 10.1038/s41590-019-0312-6
125. Zhang Y, Zheng J. Functions of immune checkpoint molecules beyond immune evasion. Adv Exp Med Biol. (2020) 1248:201–26. doi: 10.1007/978-981-15-3266-5_9
126. Li G, Liao G, Xie J, Liu B, Li X, Qiu M. Overexpression of Smad7 improves the function of Egfr-targeted human Car-T cells against non-small-cell lung cancer. Respirology (Carlton Vic). (2023) 28:869–80. doi: 10.1111/resp.14541
127. van de Haar J, Mankor JM, Hummelink K, Monkhorst K, Smit EF, Wessels LFA, et al. Combining genomic biomarkers to guide immunotherapy in non-small cell lung cancer. Clin Cancer Res. (2024) 30:1307–18. doi: 10.1158/1078-0432.Ccr-23-4027
128. Zuo YH, Gao WN, Xie YJ, Yang SY, Zhou JT, Liang HH, et al. Tumor pkcδ Instigates immune exclusion in Egfr-mutated non-small cell lung cancer. BMC Med. (2022) 20:470. doi: 10.1186/s12916-022-02670-0
129. Rubio-Pérez L, Lázaro-Gorines R, Harwood SL, Compte M, Navarro R, Tapia-Galisteo A, et al. A Pd-L1/Egfr bispecific antibody combines immune checkpoint blockade and direct anti-cancer action for an enhanced anti-tumor response. Oncoimmunology. (2023) 12:2205336. doi: 10.1080/2162402x.2023.2205336
130. Zhang H, Liu Z, Wen H, Guo Y, Xu F, Zhu Q, et al. Immunosuppressive Trem2(+) macrophages are associated with undesirable prognosis and responses to anti-Pd-1 immunotherapy in non-small cell lung cancer. Cancer immunology immunotherapy: CII. (2022) 71:2511–22. doi: 10.1007/s00262-022-03173-w
131. Ou Z, Dou X, Tang N, Liu G. Pressure increases Pd-L1 expression in A549 lung adenocarcinoma cells and causes resistance to anti-ror1 car T cell-mediated cytotoxicity. Sci Rep. (2022) 12:6919. doi: 10.1038/s41598-022-10905-6
132. Ma HY, Das J, Prendergast C, De Jong D, Braumuller B, Paily J, et al. Advances in Car T cell therapy for non-small cell lung cancer. Curr Issues Mol Biol. (2023) 45:9019–38. doi: 10.3390/cimb45110566
133. Li N, Liu S, Sun M, Chen W, Xu X, Zeng Z, et al. Chimeric antigen receptor-modified T cells redirected to epha2 for the immunotherapy of non-small cell lung cancer. Trans Oncol. (2018) 11:11–7. doi: 10.1016/j.tranon.2017.10.009
134. Li H, Harrison EB, Li H, Hirabayashi K, Chen J, Li QX, et al. Targeting brain lesions of non-small cell lung cancer by enhancing Ccl2-mediated car-T cell migration. Nat Commun. (2022) 13:2154. doi: 10.1038/s41467-022-29647-0
135. Liu J, Yang S, Cao B, Zhou G, Zhang F, Wang Y, et al. Targeting B7-H3 via chimeric antigen receptor T cells and bispecific killer cell engagers augments antitumor response of cytotoxic lymphocytes. J Hematol Oncol. (2021) 14:21. doi: 10.1186/s13045-020-01024-8
136. Bajor M, Graczyk-Jarzynka A, Marhelava K, Burdzinska A, Muchowicz A, Goral A, et al. Pd-L1 car effector cells induce self-amplifying cytotoxic effects against target cells. J immunotherapy Cancer. (2022) 10:e002500corr1. doi: 10.1136/jitc-2021-002500
137. Padmanabhan R, Kheraldine H, Gupta I, Meskin N, Hamad A, Vranic S, et al. Quantification of the growth suppression of Her2+ Breast cancer colonies under the effect of trastuzumab and Pd-1/Pd-L1 inhibitor. Front Oncol. (2022) 12:977664. doi: 10.3389/fonc.2022.977664
138. Voorwerk L, Sanders J, Keusters MS, Balduzzi S, Cornelissen S, Duijst M, et al. Immune landscape of breast tumors with low and intermediate estrogen receptor expression. NPJ Breast Cancer. (2023) 9:39. doi: 10.1038/s41523-023-00543-0
139. Page DB, Beal K, Linch SN, Spinelli KJ, Rodine M, Halpenny D, et al. Brain radiotherapy, tremelimumab-mediated ctla-4-directed blockade +/- trastuzumab in patients with breast cancer brain metastases. NPJ Breast Cancer. (2022) 8:50. doi: 10.1038/s41523-022-00404-2
140. Corti C, Venetis K, Sajjadi E, Zattoni L, Curigliano G, Fusco N. Car-T cell therapy for triple-negative breast cancer and other solid tumors: preclinical and clinical progress. Expert Opin investigational Drugs. (2022) 31:593–605. doi: 10.1080/13543784.2022.2054326
141. Srivastava S, Furlan SN, Jaeger-Ruckstuhl CA, Sarvothama M, Berger C, Smythe KS, et al. Immunogenic chemotherapy enhances recruitment of car-T cells to lung tumors and improves antitumor efficacy when combined with checkpoint blockade. Cancer Cell. (2021) 39:193–208.e10. doi: 10.1016/j.ccell.2020.11.005
142. Yin L, Chen GL, Xiang Z, Liu YL, Li XY, Bi JW, et al. Current progress in chimeric antigen receptor-modified T cells for the treatment of metastatic breast cancer. Biomedicine pharmacotherapy = Biomedecine pharmacotherapie. (2023) 162:114648. doi: 10.1016/j.biopha.2023.114648
143. Siddiqui RS, Sardar M. A systematic review of the role of chimeric antigen receptor T (Car-T) cell therapy in the treatment of solid tumors. Cureus. (2021) 13:e14494. doi: 10.7759/cureus.14494
144. Lynch E, Duffy AG, Kelly RJ. Brave new world-new and emerging treatments for gastric cancer. Chin Clin Oncol. (2023) 12:54. doi: 10.21037/cco-23-13
145. Lu LL, Xiao SX, Lin ZY, Bai JJ, Li W, Song ZQ, et al. Gpc3-Il7-Ccl19-Car-T primes immune microenvironment reconstitution for hepatocellular carcinoma therapy. Cell Biol Toxicol. (2023) 39:3101–19. doi: 10.1007/s10565-023-09821-w
146. Wang Y, Zhao Y, Li M, Hou H, Jian Z, Li W, et al. Conversion of primary liver cancer after targeted therapy for liver cancer combined with Afp-targeted Car T-cell therapy: A case report. Front Immunol. (2023) 14:1180001. doi: 10.3389/fimmu.2023.1180001
147. Huang SL, Wang YM, Wang QY, Feng GG, Wu FQ, Yang LM, et al. Mechanisms and clinical trials of hepatocellular carcinoma immunotherapy. Front Genet. (2021) 12:691391. doi: 10.3389/fgene.2021.691391
148. Zhang C, Wang Z, Yang Z, Wang M, Li S, Li Y, et al. Phase I escalating-dose trial of Car-T therapy targeting Cea(+) metastatic colorectal cancers. Mol Ther. (2017) 25:1248–58. doi: 10.1016/j.ymthe.2017.03.010
149. Magee MS, Abraham TS, Baybutt TR, Flickinger JC Jr., Ridge NA, Marszalowicz GP, et al. Human Gucy2c-targeted chimeric antigen receptor (Car)-expressing T cells eliminate colorectal cancer metastases. Cancer Immunol Res. (2018) 6:509–16. doi: 10.1158/2326-6066.Cir-16-0362
150. Hagel KR, Arafeh R, Gang S, Arnoff TE, Larson RC, Doench JG, et al. Systematic interrogation of tumor cell resistance to chimeric antigen receptor T-cell therapy in pancreatic cancer. Cancer Res. (2023) 83:613–25. doi: 10.1158/0008-5472.Can-22-2245
151. Zhang E, Yang P, Gu J, Wu H, Chi X, Liu C, et al. Recombination of a dual-car-modified T lymphocyte to accurately eliminate pancreatic Malignancy. J Hematol Oncol. (2018) 11:102. doi: 10.1186/s13045-018-0646-9
152. Liu Y, Sun Y, Wang P, Li S, Dong Y, Zhou M, et al. Fap-targeted Car-T suppresses mdscs recruitment to improve the antitumor efficacy of claudin18.2-targeted car-T against pancreatic cancer. J Trans Med. (2023) 21:255. doi: 10.1186/s12967-023-04080-z
153. Zhang B, Liu J, Li H, Huang B, Zhang B, Song B, et al. Integrated multi-omics identified the novel intratumor microbiome-derived subtypes and signature to predict the outcome, tumor microenvironment heterogeneity, and immunotherapy response for pancreatic cancer patients. Front Pharmacol. (2023) 14:1244752. doi: 10.3389/fphar.2023.1244752
154. Schoutrop E, Poiret T, El-Serafi I, Zhao Y, He R, Moter A, et al. Tuned activation of Msln-Car T cells induces superior antitumor responses in ovarian cancer models. J immunotherapy Cancer. (2023) 11:e005691. doi: 10.1136/jitc-2022-005691
155. Joy JD, Malacrida B, Laforêts F, Kotantaki P, Maniati E, Manchanda R, et al. Human 3d ovarian cancer models reveal Malignant cell-intrinsic and -extrinsic factors that influence car T-cell activity. Cancer Res. (2024) 84:2432–49. doi: 10.1158/0008-5472.Can-23-3007
156. Fang L, Tian W, Zhang C, Wang X, Li W, Zhang Q, et al. Oncolytic adenovirus-mediated expression of Ccl5 and Il12 facilitates Ca9-targeting Car-T therapy against renal cell carcinoma. Pharmacol Res. (2023) 189:106701. doi: 10.1016/j.phrs.2023.106701
157. Alzubi J, Dettmer-Monaco V, Kuehle J, Thorausch N, Seidl M, Taromi S, et al. Psma-directed car T cells combined with low-dose docetaxel treatment induce tumor regression in a prostate cancer xenograft model. Mol Ther oncolytics. (2020) 18:226–35. doi: 10.1016/j.omto.2020.06.014
158. Hong H, Stastny M, Brown C, Chang WC, Ostberg JR, Forman SJ, et al. Diverse solid tumors expressing a restricted epitope of L1-cam can be targeted by chimeric antigen receptor redirected T lymphocytes. J immunotherapy (Hagerstown Md: 1997). (2014) 37:93–104. doi: 10.1097/cji.0000000000000018
159. O'Rourke DM, Nasrallah MP, Desai A, Melenhorst JJ, Mansfield K, Morrissette JJD, et al. A single dose of peripherally infused Egfrviii-directed Car T cells mediates antigen loss and induces adaptive resistance in patients with recurrent glioblastoma. Sci Trans Med. (2017) 9:eaaa0984. doi: 10.1126/scitranslmed.aaa0984
160. Bagley SJ, Binder ZA, Lamrani L, Marinari E, Desai AS, Nasrallah MP, et al. Repeated peripheral infusions of anti-egfrviii car T cells in combination with pembrolizumab show no efficacy in glioblastoma: A phase 1 trial. Nat Cancer. (2024) 5:517–31. doi: 10.1038/s43018-023-00709-6
161. Hossen MM, Ma Y, Yin Z, Xia Y, Du J, Huang JY, et al. Current understanding of ctla-4: from mechanism to autoimmune diseases. Front Immunol. (2023) 14:1198365. doi: 10.3389/fimmu.2023.1198365
162. Cameron F, Whiteside G, Perry C. Ipilimumab: first global approval. Drugs. (2011) 71:1093–104. doi: 10.2165/11594010-000000000-00000
163. Keam SJ. Tremelimumab: first approval. Drugs. (2023) 83:93–102. doi: 10.1007/s40265-022-01827-8
164. Liu Y, Zheng P. Preserving the ctla-4 checkpoint for safer and more effective cancer immunotherapy. Trends Pharmacol Sci. (2020) 41:4–12. doi: 10.1016/j.tips.2019.11.003
165. Kamada T, Togashi Y, Tay C, Ha D, Sasaki A, Nakamura Y, et al. Pd-1(+) regulatory T cells amplified by Pd-1 blockade promote hyperprogression of cancer. Proc Natl Acad Sci United States America. (2019) 116:9999–10008. doi: 10.1073/pnas.1822001116
166. Geels SN, Moshensky A, Sousa RS, Murat C, Bustos MA, Walker BL, et al. Interruption of the intratumor Cd8(+) T cell:Treg crosstalk improves the efficacy of Pd-1 immunotherapy. Cancer Cell. (2024) 42:1051–66.e7. doi: 10.1016/j.ccell.2024.05.013
167. Liu HJ, Lizotte PH, Du H, Speranza MC, Lam HC, Vaughan S, et al. Tsc2-deficient tumors have evidence of T cell exhaustion and respond to anti-Pd-1/anti-ctla-4 immunotherapy. JCI Insight. (2018) 3:e98674. doi: 10.1172/jci.insight.98674
168. Bufe S, Zimmermann A, Ravens S, Prinz I, Buitrago-Molina LE, Geffers R, et al. Pd-1/ctla-4 blockade leads to expansion of Cd8(+)Pd-1(Int) Tils and results in tumor remission in experimental liver cancer. Liver Cancer. (2023) 12:129–44. doi: 10.1159/000526899
169. Lozano AX, Chaudhuri AA, Nene A, Bacchiocchi A, Earland N, Vesely MD, et al. T cell characteristics associated with toxicity to immune checkpoint blockade in patients with melanoma. Nat Med. (2022) 28:353–62. doi: 10.1038/s41591-021-01623-z
170. Ju F, Luo Y, Lin C, Jia X, Xu Z, Tian R, et al. Oncolytic virus expressing Pd-1 inhibitors activates a collaborative intratumoral immune response to control tumor and synergizes with Ctla-4 or Tim-3 blockade. J immunotherapy Cancer. (2022) 10:e004762corr1. doi: 10.1136/jitc-2022-004762
171. Spranger S, Koblish HK, Horton B, Scherle PA, Newton R, Gajewski TF. Mechanism of tumor rejection with doublets of Ctla-4, Pd-1/Pd-L1, or Ido blockade involves restored Il-2 production and proliferation of Cd8(+) T cells directly within the tumor microenvironment. J immunotherapy Cancer. (2014) 2:3. doi: 10.1186/2051-1426-2-3
172. Tawbi HA, SChadendorf D, Lipson EJ, Ascierto PA, Matamala L, Castillo Gutiérrez E, et al. Relatlimab and nivolumab versus nivolumab in untreated advanced melanoma. New Engl J Med. (2022) 386:24–34. doi: 10.1056/NEJMoa2109970
173. Dixon KO, Lahore GF, Kuchroo VK. Beyond T cell exhaustion: Tim-3 regulation of myeloid cells. Sci Immunol. (2024) 9:eadf2223. doi: 10.1126/sciimmunol.adf2223
174. Sakuishi K, Apetoh L, Sullivan JM, Blazar BR, Kuchroo VK, Anderson AC. Targeting Tim-3 and Pd-1 pathways to reverse T cell exhaustion and restore anti-tumor immunity. J Exp Med. (2010) 207:2187–94. doi: 10.1084/jem.20100643
175. Datar I, Sanmamed MF, Wang J, Henick BS, Choi J, Badri T, et al. Expression analysis and significance of Pd-1, Lag-3, and Tim-3 in human non-small cell lung cancer using spatially resolved and multiparametric single-cell analysis. Clin Cancer Res. (2019) 25:4663–73. doi: 10.1158/1078-0432.Ccr-18-4142
176. Hu Q, Wu G, Wang R, Ma H, Zhang Z, Xue Q. Cutting edges and therapeutic opportunities on tumor-associated macrophages in lung cancer. Front Immunol. (2022) 13:1007812. doi: 10.3389/fimmu.2022.1007812
177. Sakuishi K, Ngiow SF, Sullivan JM, Teng MW, Kuchroo VK, Smyth MJ, et al. Tim3(+)Foxp3(+) regulatory T cells are tissue-specific promoters of T-cell dysfunction in cancer. Oncoimmunology. (2013) 2:e23849. doi: 10.4161/onci.23849
178. Dixon KO, Tabaka M, Schramm MA, Xiao S, Tang R, Dionne D, et al. Tim-3 restrains anti-tumour immunity by regulating inflammasome activation. Nature. (2021) 595:101–6. doi: 10.1038/s41586-021-03626-9
179. Kano Y, Iguchi T, Matsui H, Adachi K, Sakoda Y, Miyakawa T, et al. Combined adjuvants of poly(I:C) plus lag-3-ig improve antitumor effects of tumor-specific T cells, preventing their exhaustion. Cancer Sci. (2016) 107:398–406. doi: 10.1111/cas.12861
180. Ruffo E, Wu RC, Bruno TC, Workman CJ, Vignali DAA. Lymphocyte-activation gene 3 (Lag3): the next immune checkpoint receptor. Semin Immunol. (2019) 42:101305. doi: 10.1016/j.smim.2019.101305
181. Harris-Bookman S, Mathios D, Martin AM, Xia Y, Kim E, Xu H, et al. Expression of lag-3 and efficacy of combination treatment with anti-lag-3 and anti-pd-1 monoclonal antibodies in glioblastoma. Int J Cancer. (2018) 143:3201–8. doi: 10.1002/ijc.31661
182. Sung E, Ko M, Won JY, Jo Y, Park E, Kim H, et al. Lag-3xpd-L1 Bispecific Antibody Potentiates Antitumor Responses of T cells through Dendritic Cell Activation. Mol Ther. (2022) 30:2800–16. doi: 10.1016/j.ymthe.2022.05.003
183. Gumber D, Wang LD. Improving car-T immunotherapy: overcoming the challenges of T cell exhaustion. EBioMedicine. (2022) 77:103941. doi: 10.1016/j.ebiom.2022.103941
184. Wang Z, Cao YJ. Adoptive cell therapy targeting neoantigens: A frontier for cancer research. Front Immunol. (2020) 11:176. doi: 10.3389/fimmu.2020.00176
185. Shahzad M, Nguyen A, Hussain A, Ammad-Ud-Din M, Faisal MS, Tariq E, et al. Outcomes with chimeric antigen receptor T-cell therapy in relapsed or refractory acute myeloid leukemia: A systematic review and meta-analysis. Front Immunol. (2023) 14:1152457. doi: 10.3389/fimmu.2023.1152457
186. Hickman TL, Choi E, Whiteman KR, Muralidharan S, Pai T, Johnson T, et al. Boxr1030, an anti-Gpc3 car with exogenous Got2 expression, shows enhanced T cell metabolism and improved anti-cell line derived tumor xenograft activity. PLoS One. (2022) 17:e0266980. doi: 10.1371/journal.pone.0266980
187. Yu T, Nie FQ, Zhang Q, Yu SK, Zhang ML, Wang Q, et al. Effects of methionine deficiency on B7h3-Dap12-Car-T cells in the treatment of lung squamous cell carcinoma. Cell Death Dis. (2024) 15:12. doi: 10.1038/s41419-023-06376-w
188. Liang S, Zheng R, Zuo B, Li J, Wang Y, Han Y, et al. Smad7 expression in car-T cells improves persistence and safety for solid tumors. Cell Mol Immunol. (2024) 21:213–26. doi: 10.1038/s41423-023-01120-y
189. Philip M, Fairchild L, Sun L, Horste EL, Camara S, Shakiba M, et al. Chromatin states define tumour-specific T cell dysfunction and reprogramming. Nature. (2017) 545:452–6. doi: 10.1038/nature22367
190. Agliardi G, Liuzzi AR, Hotblack A, De Feo D, Núñez N, Stowe CL, et al. Intratumoral il-12 delivery empowers car-T cell immunotherapy in a pre-clinical model of glioblastoma. Nat Commun. (2021) 12:444. doi: 10.1038/s41467-020-20599-x
191. Eggenhuizen PJ, Ng BH, Ooi JD. Treg enhancing therapies to treat autoimmune diseases. Int J Mol Sci. (2020) 21:7015. doi: 10.3390/ijms21197015
192. Togashi Y, Shitara K, Nishikawa H. Regulatory T cells in cancer immunosuppression - implications for anticancer therapy. Nat Rev Clin Oncol. (2019) 16:356–71. doi: 10.1038/s41571-019-0175-7
193. Luo Y, Xia Y, Liu D, Li X, Li H, Liu J, et al. Neoadjuvant parpi or chemotherapy in ovarian cancer informs targeting effector treg cells for homologous-recombination-deficient tumors. Cell. (2024) 87(18):4905–4925.e24. doi: 10.1016/j.cell.2024.06.013
194. Xie J, Liu M, Deng X, Tang Y, Zheng S, Ou X, et al. Gut microbiota reshapes cancer immunotherapy efficacy: mechanisms and therapeutic strategies. iMeta. (2024) 3:e156. doi: 10.1002/imt2.156
195. Boulch M, Cazaux M, Cuffel A, Guerin MV, Garcia Z, Alonso R, et al. Tumor-intrinsic sensitivity to the pro-apoptotic effects of Ifn-Γ Is a major determinant of Cd4(+) Car T-cell antitumor activity. Nat Cancer. (2023) 4:968–83. doi: 10.1038/s43018-023-00570-7
196. Kruse B, Buzzai AC, Shridhar N, Braun AD, Gellert S, Knauth K, et al. Cd4(+) T cell-induced inflammatory cell death controls immune-evasive tumours. Nature. (2023) 618:1033–40. doi: 10.1038/s41586-023-06199-x
197. Magen A, Hamon P, Fiaschi N, Soong BY, Park MD, Mattiuz R, et al. Intratumoral dendritic cell-Cd4(+) T helper cell niches enable Cd8(+) T cell differentiation following pd-1 blockade in hepatocellular carcinoma. Nat Med. (2023) 29:1389–99. doi: 10.1038/s41591-023-02345-0
198. Espinosa-Carrasco G, Chiu E, Scrivo A, Zumbo P, Dave A, Betel D, et al. Intratumoral immune triads are required for immunotherapy-mediated elimination of solid tumors. Cancer Cell. (2024) 42:1202–16.e8. doi: 10.1016/j.ccell.2024.05.025
199. Sun R, Luo H, Su J, Di S, Zhou M, Shi B, et al. Olaparib suppresses Mdsc recruitment via Sdf1α/Cxcr4 axis to improve the anti-tumor efficacy of Car-T cells on breast cancer in mice. Mol Ther. (2021) 29:60–74. doi: 10.1016/j.ymthe.2020.09.034
200. Sun R, Sun Y, Wu C, Liu Y, Zhou M, Dong Y, et al. Cxcr4-Modified car-T cells Suppresses Mdscs Recruitment Via Stat3/Nf-Kb/Sdf-1α Axis to Enhance Efficacy against Pancreatic Cancer. Mol Ther. (2023) 31:3193–209. doi: 10.1016/j.ymthe.2023.09.010
201. Zannikou M, Duffy JT, Levine RN, Seblani M, Liu Q, Presser A, et al. Il15 modification enables car T cells to act as a dual targeting agent against tumor cells and myeloid-derived suppressor cells in Gbm. J immunotherapy Cancer. (2023) 11:e006239. doi: 10.1136/jitc-2022-006239
202. Chen J, Zhu T, Jiang G, Zeng Q, Li Z, Huang X. Target delivery of a Pd-1-Trem2 Scfv by Car-T cells enhances anti-tumor efficacy in colorectal cancer. Mol Cancer. (2023) 22:131. doi: 10.1186/s12943-023-01830-x
203. Sánchez-Paulete AR, Mateus-Tique J, Mollaoglu G, Nielsen SR, Marks A, Lakshmi A, et al. Targeting macrophages with car T cells delays solid tumor progression and enhances antitumor immunity. Cancer Immunol Res. (2022) 10:1354–69. doi: 10.1158/2326-6066.Cir-21-1075
204. Rodriguez-Garcia A, Lynn RC, Poussin M, Eiva MA, Shaw LC, O'Connor RS, et al. Car-T cell-mediated depletion of immunosuppressive tumor-associated macrophages promotes endogenous antitumor immunity and augments adoptive immunotherapy. Nat Commun. (2021) 12:877. doi: 10.1038/s41467-021-20893-2
205. Chen Y, Yu Z, Tan X, Jiang H, Xu Z, Fang Y, et al. Car-macrophage: A new immunotherapy candidate against solid tumors. Biomedicine pharmacotherapy = Biomedecine pharmacotherapie. (2021) 139:111605. doi: 10.1016/j.biopha.2021.111605
206. Adachi K, Kano Y, Nagai T, Okuyama N, Sakoda Y, Tamada K. Il-7 and Ccl19 expression in car-T cells improves immune cell infiltration and Car-T cell survival in the tumor. Nat Biotechnol. (2018) 36:346–51. doi: 10.1038/nbt.4086
207. Ma L, Hostetler A, Morgan DM, Maiorino L, Sulkaj I, Whittaker CA, et al. Vaccine-boosted car T crosstalk with host immunity to reject tumors with antigen heterogeneity. Cell. (2023) 186:3148–65.e20. doi: 10.1016/j.cell.2023.06.002
208. Sun S, Ding Z, Gao L, Hammock BD, Huang X, Xu ZP, et al. A dendritic/tumor fusion cell vaccine enhances efficacy of nanobody-based Car-T cells against solid tumor. Theranostics. (2023) 13:5099–113. doi: 10.7150/thno.84946
209. Chen Y, Ouyang D, Wang Y, Pan Q, Zhao J, Chen H, et al. Ebv promotes Tcr-T-cell therapy resistance by inducing Cd163+M2 macrophage polarization and mmp9 secretion. J immunotherapy Cancer. (2024) 12:e008375. doi: 10.1136/jitc-2023-008375
Keywords: CD8 + T cell, T cell exhaustion, tumor microenvironment, immune checkpoint blockade, adoptive T cell treatment
Citation: Zhang B, Liu J, Mo Y, Zhang K, Huang B and Shang D (2024) CD8+ T cell exhaustion and its regulatory mechanisms in the tumor microenvironment: key to the success of immunotherapy. Front. Immunol. 15:1476904. doi: 10.3389/fimmu.2024.1476904
Received: 06 August 2024; Accepted: 04 September 2024;
Published: 20 September 2024.
Edited by:
Xiangyu Chen, Chongqing Medical University, ChinaReviewed by:
Farah Alfatyan, MUSC Health, United StatesJindong Xie, Sun Yat-sen University Cancer Center (SYSUCC), China
Copyright © 2024 Zhang, Liu, Mo, Zhang, Huang and Shang. This is an open-access article distributed under the terms of the Creative Commons Attribution License (CC BY). The use, distribution or reproduction in other forums is permitted, provided the original author(s) and the copyright owner(s) are credited and that the original publication in this journal is cited, in accordance with accepted academic practice. No use, distribution or reproduction is permitted which does not comply with these terms.
*Correspondence: Dong Shang, c2hhbmdkb25nQGRtdS5lZHUuY24=; Bingqian Huang, aGJxcTA4MTFAMTYzLmNvbQ==
†These authors have contributed equally to this work