- 1Department of Orthopedics, The Second Affiliated Hospital of Jilin University, Changchun, Jilin, China
- 2Department of Hematology, The First Hospital of Jilin University, Changchun, China
- 3Department of Radiation Oncology, The Second Affiliated Hospital of Jilin University, Changchun, China
- 4NHC Key Laboratory of Radiobiology, Jilin University, Changchun, China
The tumor microenvironment (TME) is a complex and dynamic ecosystem composed of tumor cells, immune cells, supporting cells, and the extracellular matrix. Typically, the TME is characterized by an immunosuppressive state. To meet the demands of rapid proliferation, cancer cells undergo metabolic reprogramming, which enhances their biosynthesis and bioenergy supply. Immune cells require similar nutrients for activation and proliferation, leading to competition and immunosuppression within the TME. Additionally, tumor metabolites inhibit immune cell activation and function. Consequently, an immunosuppressed and immune-tolerant TME promotes cancer cell proliferation and metastasis. Long non-coding RNAs (lncRNAs), a category of non-coding RNA longer than 200 nucleotides, regulate tumor metabolic reprogramming by interacting with key enzymes, transporters, and related signaling pathways involved in tumor metabolism. Furthermore, lncRNAs can interact with both cellular and non-cellular components in the TME, thereby facilitating tumor growth, metastasis, drug resistance, and inducing immunosuppression. Recent studies have demonstrated that lncRNAs play a crucial role in reshaping the TME by regulating tumor metabolic reprogramming. In this discussion, we explore the potential mechanisms through which lncRNAs regulate tumor metabolic reprogramming to remodel the TME. Additionally, we examine the prospects of lncRNAs as targets for anti-tumor therapy and as biomarkers for tumor prognosis.
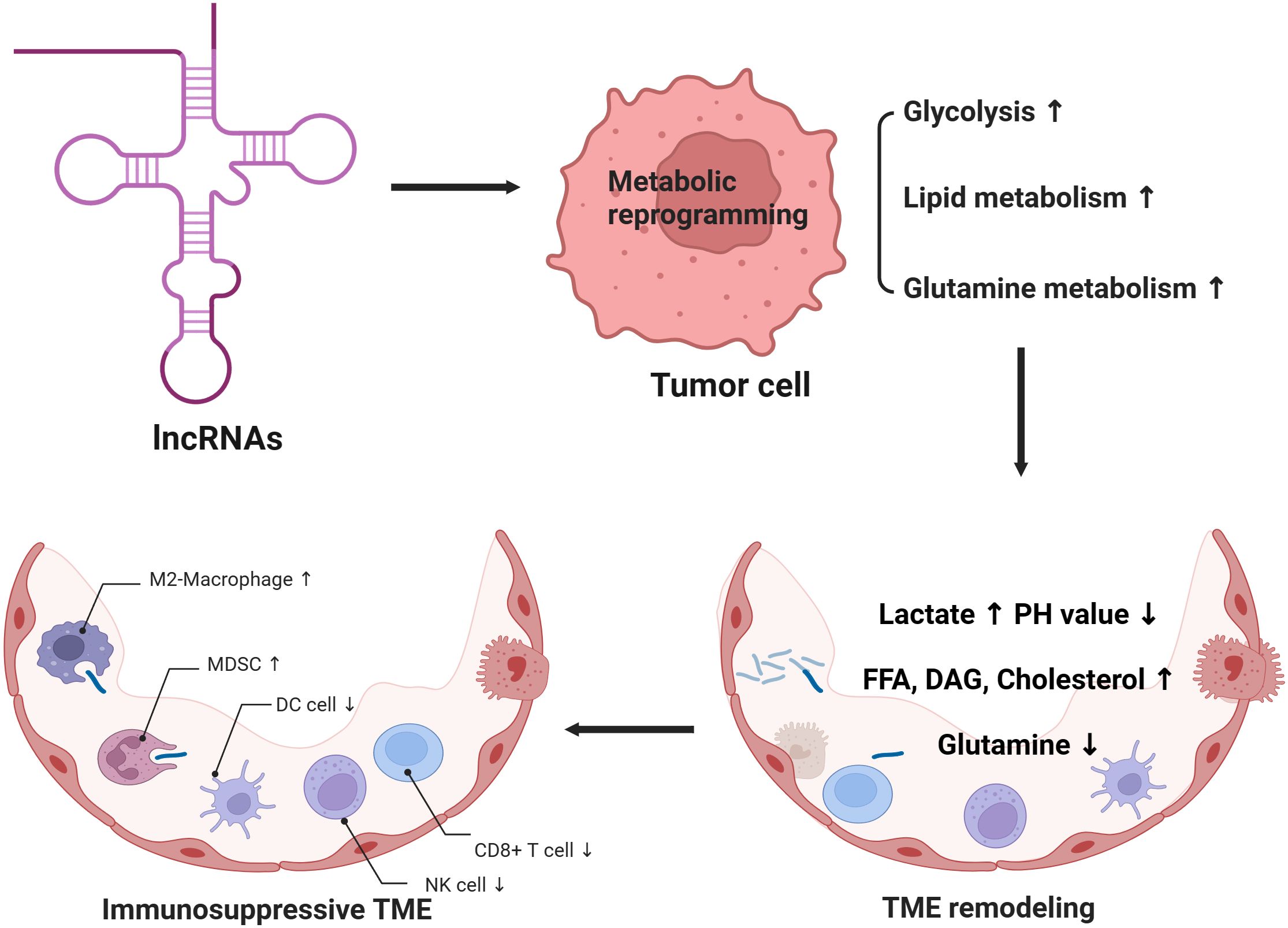
Graphical Abstract. 1. lncRNAs can regulate the metabolic reprogramming process of tumor cells, resulting in increased aerobic glycolysis, lipid metabolism and glutamine metabolism of tumor cells. 2. Tumor metabolic reprogramming can further remodel the tumor microenvironment, resulting in increased lactic acid, decreased PH value, increased cholesterol, free fatty acids and diacylglycerol, as well as decreased glutamine in tumor microenvironment. 3. Tumor microenvironment remodeling induced by tumor metabolic reprogramming can affect immune cell function and form an immunosuppressive microenvironment.
1 Introduction
The mortality rate of malignant tumors ranks second among human diseases, which seriously threaten human health (1). Immunotherapy can activate immune cells and enhance antitumor immune responses, changing the treatment mode of tumors. However, most patients either fail to respond to immune checkpoint inhibitors (ICIs) or develop drug resistance. The tumor immune microenvironment plays a pivotal role in tumor immunity and largely determines the effectiveness of immunotherapy. To maintain malignant growth and rapid proliferation of tumors, cancer cells consume a great amount of nutrients, for instance, oxygen, glucose, glutamine, and amino acids, resulting in nutrient deficiency and hypoxia in the tumor microenvironment (TME). As tumor cells compete with immune cells for nutrients in the TME, the activation and proliferation of immune cells are inhibited (2–4). Furthermore, tumor cells can influence immune and stromal cells within the TME, resulting in an immunosuppressive environment that facilitates tumor immune escape. Therefore, understanding how tumor metabolic reprogramming influences the TME can enhance immunotherapy efficacy and identify new therapeutic targets.
Non-coding RNAs (ncRNAs) occupy for approximately 60% of transcriptional output in human cells (5). Although they do not encode proteins, they play indispensable roles at the transcriptional and post-transcriptional levels by combined with DNA, RNA, and proteins to regulate gene expression and protein function. A diverse array of ncRNAs establishes an intricate network that influences numerous biological functions and signaling pathways (5). The disorder of non-coding RNA is closely related to malignant tumors, nervous system diseases, cardiovascular diseases, and autoimmune diseases. Notably, dysregulation of ncRNAs has been associated with all malignancies studied to date and affects all major tumor biomarkers (6, 7). As the most abundant category of non-coding RNAs, long non-coding RNAs (lncRNAs) can influence tumor metabolic reprogramming by regulating key enzymes, transporters, and related signaling pathways in tumor metabolism. Metabolites in the process of tumor metabolic reprogramming, such as lactic acid and free cholesterol, can impair the activation and function of antitumor immune cells, for example, T and NK cells, and promote the polarization of macrophages to the M2 subtype, eventually forming a tumor immunosuppressive microenvironment. Therefore, lncRNAs affect TME remodeling through tumor metabolic reprogramming (8, 9). In this paper, we introduced the role and relationship of lncRNAs in tumor metabolic reprogramming and tumor microenvironment remodeling and summarized the prospects of lncRNAs as future anti-tumor therapeutic targets and prognostic biomarkers.
2 Overview of lncRNAs
LncRNAs are an important category of non-coding RNA that are greater than 200 nucleotides in length (10). Based on their localization in the genome, lncRNAs can be classified into sense lncRNAs, antisense lncRNAs, intronic lncRNAs, and intergenic lncRNAs. Sense lncRNAs overlap with the positive strand RNA of neighboring genes, and the transcription direction is the same as that of neighboring genes. Antisense lncRNAs are opposite to sense lncRNAs. Antisense lncRNAs overlap with the negative strand RNA of neighboring genes, and its transcription direction is opposite to that of neighboring genes. Intronic lncRNAs are produced in the intron region within genes by different splicing methods. Intergenic lncRNAs, also known as “lincRNAs,” are located between two genes and usually do not overlap with known protein-coding genes (11, 12). In addition, lncRNAs has strong tissue specificity and cell specificity. In one study, the expression of hundreds of novel lncRNAs was shown to be cell type-dependent (13). Previous investigations have suggested that lncRNAs affect the occurrence and progression of tumors via regulation of the epigenome, gene transcription, or protein translation (14, 15).
Some lncRNAs mediate the recruitment of chromatin remodeling complexes or serve as scaffolds for chromatin remodeling complexes (16, 17). LncRNA HOTAIR had been shown to regulate chromatin remodeling, thereby promoting breast cancer metastasis (18). LncRNA DIRC3 in the nucleus affect the local chromatin structure and activate the transcription of tumor suppressor IGFBP5 (19). LncRNA LINC00261 is involved in phosphorylation of ataxia telangiectasia mutated protein (ATM) and DNA damage of lung adenocarcinoma cells, slowing cancer progression (20). LncRNAs can also activate or repress gene expression by binding to or removing transcription factors (21). Some antisense lncRNAs specifically bind to complementary mRNAs to regulate gene splicing, translation, or degradation at the post-transcriptional level (22). LncRNA REG1CP binds the helicase FANCJ to the promoter of the neighboring gene REG3A86, promoting growth of colorectal cancer (23). LncRNA-p21 can bind to heterogeneous ribonucleoproteins, increasing transcription of neighboring gene CDKN1A and synthesis of P21 protein (24). Other lncRNAs can alter protein localization, regulate protein activity, or serve as components of protein complexes. For example, a HIF-1α anti-sense lncRNA, HIFAL introduces the PKM2/PHD3 complex into the nucleus by binding to heterogeneous nuclear ribonucleoprotein F (hnRNPF) to enhance the production of hypoxia-inducible factor-1 (HIF-1) and promote breast cancer. Therefore, HIFAL is also a target for treatment of breast cancer (25, 26). LncRNA LUCAT1 inhibits phosphorylation of annexin A2, further inhibiting the degradation of ANXA2-S100A10 heterotetramer (AIIT) and promoting cancer development (27). In neuroblastoma, lncRNA LINC02525 interacts with ribosomal protein RPL35. They then specifically activated the translation of E2F1, advancing neuroblastoma (28). There are also lncRNAs that can cleave and produce small RNAs precursors or serve as competitive endogenous RNAs that act as “sponges” for miRNAs (29). Several studies have indicated that lncRNAs play indispensable roles in tumor growth, metastasis, angiogenesis, drug resistance, cell metabolic reprogramming, and the induction of immunosuppression through a variety of complex mechanisms (30–32) (Figure 1).
3 Metabolic reprogramming of tumors
Body metabolism includes glucose, lipids, amino acids, and the metabolism of other nutrients. The metabolism of various substances affects each other, and metabolic signaling pathways interact with each other to form a complex metabolic network. The metabolic patterns of cancer cells are quite different from those of normal cells. The environment of the cancers has special characteristics, such as hypoxia, acidity, oxidative stress, and nutrient deficiency. To better adapt to this environment, tumor cells must adjust their metabolic pathways to meet the needs of their various life activities, which is called metabolic reprogramming (33). On the one hand, metabolic reprogramming of cancer promotes tumorigenesis by facilitating rapid proliferation, survival, invasion, and metastasis. On the other hand, as the tumor progresses, tumor cells acquire more mutations and alterations, resulting in enhanced metabolic reprogramming, which in turn accelerates tumor growth, proliferation, and development.
Metabolic and functional changes of tumor cells mainly include: 1) Healthy cells rely on mitochondria to oxidize glucose and release energy, while most tumor cells supply themselves with energy through glycolysis, which has a relatively low energy yield. This metabolic property of tumor cells is also known as the Warburg Effect. Since glycolysis has relatively little capacity, cancer cells must maintain their vital activities by upping their glucose intake. Many cancer cells do this by activating glucose transporters. In addition, tumor cells undergoing metabolic reprogramming use transcription factors such as HIF-1α and c-Myc to up-regulate glycolytic enzyme activity, thereby increasing glycolytic efficiency. At the same time, the conversion of glucose to lactate, rather than to pyruvate increases, which means that less pyruvate enters the mitochondria, leading to inhibition of the tricarboxylic acid cycle (TCA) pathway (34, 35); 2) Since pyruvate is overconsumed in the glycolytic pathway, glutamine is used to supplement important metabolites in TCA. As complementary substrate for TCA, glutamine continuously produces NADH, FADH2, and electrons for adenosine triphosphate (ATP) production through oxidative phosphorylation of the mitochondria. Cancer cells depend on c-Myc transcription factor to increase the expression of glutamine transporter and glutamine lyase, leading to increased cellular uptake and utilization of glutamine (36). Glutamine can produce glutamic acid under the catalysis of glutaminase, and glutamic acid can be converted into alanine, aspartate and other amino acids. At the same time, glutamic acid can be used as a substrate to synthesize α-ketoglutaric acid, continuing to provide fuel for TCA (37); 3) In terms of lipid metabolism, in metabolically reprogrammed cancer cells, most acetyl-CoA used for lipid synthesis comes from citrate produced by the TCA in the mitochondria. In the cytoplasm, citrate can be converted back to acetyl-CoA and lipid synthesis occurs (38); 4) anabolism, including nucleotide synthesis, nonessential amino acid synthesis, lipid synthesis, and hexosamine synthesis, is upregulated, which consumes large amount of energy; 5) The pentose phosphate pathway (PPP) is upregulated, which maintains cellular redox homeostasis and downregulates the generation of reactive oxygen species. As a rate-limiting enzyme in the PPP, glucose-6-phosphate dehydrogenase (G6PD) is frequently elevated in human malignancies, which in turn leads to the production of precursors for nucleotide and lipid synthesis (39–41) (Figure 2).
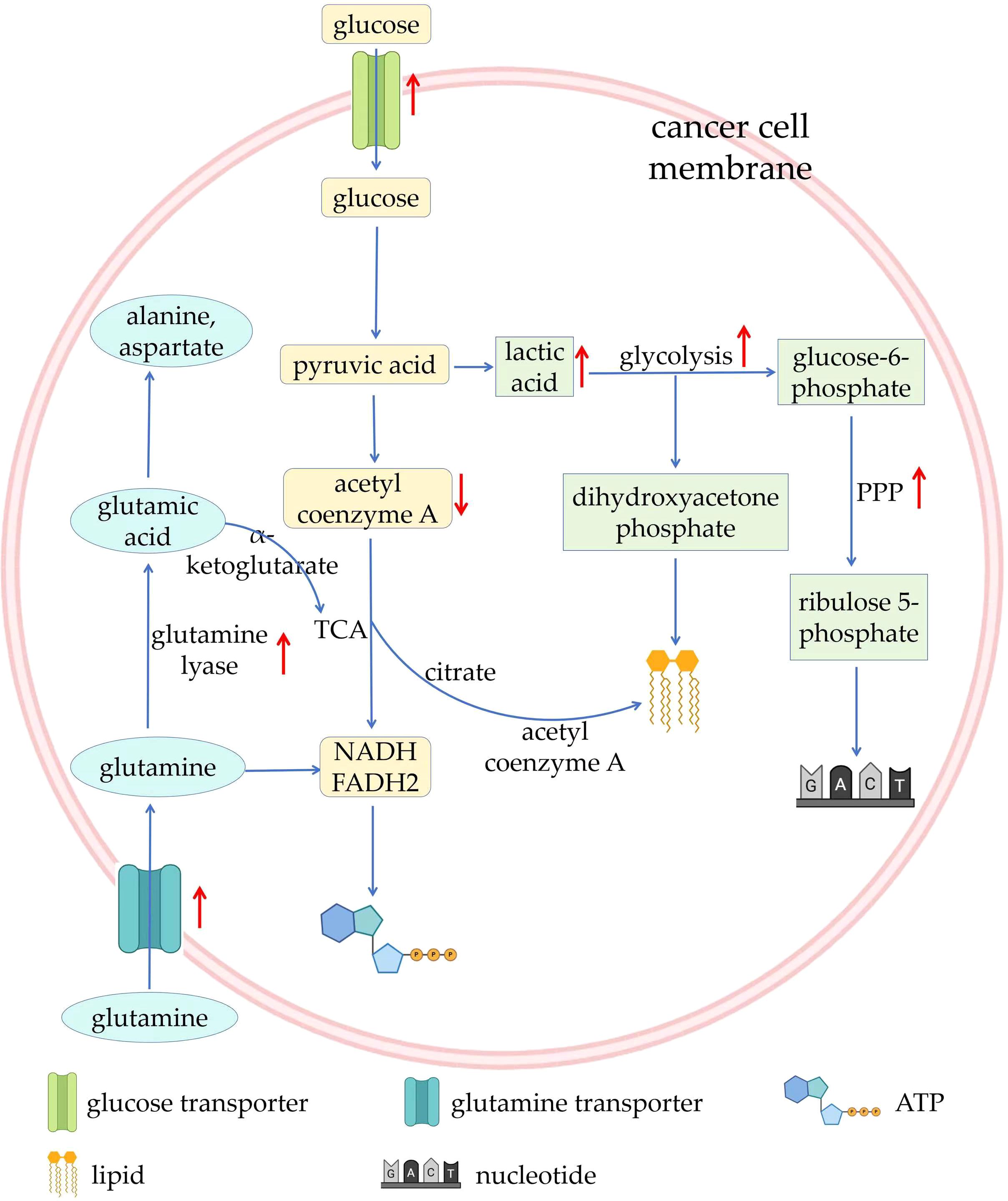
Figure 2. Overview of metabolic reprogramming in tumors. ATP, adenosine triphosphate; PPP, pentose phosphate pathway; TCA, tricarboxylic acid cycle.
4 TME
The TME is a complex microenvironment for tumor cells to survive and is made up of tumor cells, stromal cells, fibroblasts, infiltrating immune cells, and the secreted products and extracellular matrix of the corresponding cells. Immune cells are key players in shaping inhibitory microenvironments. In the TME, immune cells have distinct functions compared to normal tissues. For purpose of satisfying the need of rapid proliferation of tumor cells, they undergo metabolic reprogramming, which causes the TME to show ion homeostasis imbalance, acidity, hypoxia, increased lactate, decreased glucose concentration, nutritional competition, and changes in the secretome. Remodeling of the TME induces the metabolic reprogramming of immune cells, which changes their functions. They exhibit an attenuated inflammatory response or enhanced inhibitory function, assisting immune escape from the tumor. Therefore, TME remodeling caused by tumor metabolic reprogramming is the basis for the functional transformation of immune cells and is particularly critical for the proliferation and metastasis of cancer cells.
The most obvious feature of the TME is the constantly changing in its constituents, particularly in the later stages of cancer development. Tumor-derived cytokines, chemokines, and even metabolic conditions (pH, oxygen levels, and nutrients) affect the function of immune cells in TME (42). In the early stages of oncogenesis, the TME is an immune-activated microenvironment with high proinflammatory signals, and immune cells are prone to show a pro-inflammatory phenotype. In the process of cancer growth and metastasis, the TME changes into an immunosuppressive environment step by step, with low oxygen, low pH, low glucose accumulation, high fatty acid accumulation, low amino acid accumulation, high adenosine accumulation, and high lactic acid accumulation, and immune cells are inclined to show an inhibitory phenotype (43). Unlike other microenvironments in the body, TME changes are mainly dominated by tumor cells rather than by the body itself, which is largely out of the control of the body. In this sophisticated, constantly changing, and uncontrollable microenvironment, the roles of various non-tumor cells are also complex and dynamic. For example, immune cells differentiate into subsets with different phenotypes, metabolic characteristics, and functions, which play anti-tumor or pro-tumor roles, and further change the TME through their own metabolism.
4.1 Immune in the TME
4.1.1 MDSCs in the TME
Myeloid-derived suppressor cells (MDSCs) in TME often exhibit immunosuppressive properties and can promote tumor progression by inhibiting the function of anti-tumor immune cells. The important mechanism of MDSCs-mediated immunosuppression lies in its metabolic activity. Hypoxia and lactate accumulation in TME lead to increased expression of HIF-1α, which activates the expression of CD39/CD73 on MDSCs, promoting differentiation and proliferation of MDSCs (44, 45). Furthermore, HIF-1α can alleviate MDSCs damage by reducing oxidative stress and ROS production through HIF1α-frataxin signaling (46). At the same time, the acidic environment of TME can enhance the inhibitory function of MDSC on T cells through HIF-1α signaling (44).
4.1.2 DCs in the TME
Dendritic cells (DCs) play an important role in the process of presenting tumor antigens and realizing immune regulation. In TME, bioactive substances secreted by tumor cells can achieve immune escape by inhibiting recruitment and maturation of DCs (47). For example, granulocyte colony-stimulating factors (G-CSF) secreted by breast cancer cells lead to directional differentiation of bone marrow precursor cells to MDSCs instead of DCs, and inhibit maturation of DCs by down-regulating CD80 and CD86 (48). GRP78 secreted by breast cancer cells induces differentiation of DCs into regulatory DCs (DCreg), and TGF-β secreted by DCreg significantly inhibits the killing activity of NK cells (49). Cytotoxic T (CD8+T) cells and natural killer (NK) cells secrete toxic substances to kill tumor cells or directly engulf tumor cells, forming a strong defense line in anti-tumor immunity. However, in the immunosuppressive microenvironment, the functions of both CD8+T cells and NK cells are inhibited. For example, exosomes secreted by melanoma induce apoptosis of CD8+T cells in lymph nodes and proliferation of CD8+T cells is significantly inhibited by CXCL1 and CXCL2 secreted by ovarian cancer cells (50, 51). Ovarian cancer cells overexpress the immune checkpoint B7-H3, which promotes glycolysis and results in dysfunction of NK cells (52).
4.1.3 ILCs in the TME
Innate lymphoid cells (ILCs) are also an important component of the tumor microenvironment. ILCs are a group of innate immune cells that do not express antigen-specific receptors. Based on their cytokine production profiles and transcription factor expression, they can be classified into different subgroups (ILC1, ILC2, ILC3). Research has shown that ILCs have both pro-tumor and anti-tumor effects within the tumor microenvironment (53). When performing immune surveillance functions, ILCs can recognize tumor cells and regulate the tumor microenvironment by secreting cytokines, which inhibits tumor growth. However, interactions with other immune cells, such as T cells and macrophages, allow ILCs to promote angiogenesis, tissue remodeling, and immune suppression, thereby supporting tumor growth.
4.1.4 Pericytes in the TME
Pericytes are also an important component of the immune microenvironment, participating in angiogenesis and stabilization. They can regulate vascular permeability, secrete cytokines and chemokines that affect the infiltration of tumor cells and immune cells in the tumor microenvironment, and thus help modulate the immune response within this environment. Their influence may promote or inhibit tumor growth by affecting the migration and function of immune cells (54). Therefore, the metabolic activities of tumor cells and immune cells form a sophisticated and complex interaction network with TME.
4.1.5 Dual-function immune cells in the TME
In addition, there are some dual-function immune cells in TME, such as neutrophils and macrophages. N1 neutrophils and M1 macrophages work against tumors, while N2 neutrophils and M2 macrophages promote tumor (55). Some studies had found that ETS1 exosomes secreted by ovarian cancer cells induce the polarization of M2 macrophages, which overexpress CD163, IL-10, CCL2, CXCL5 and other immunosuppressive factors, and significantly stimulate the migration of ovarian cancer cells (56). The pluripotent factor Lin28B secreted by breast cancer cells induce neutrophils to transform into N2 phenotype, significantly inhibiting proliferation of CD4+ and CD8+T cells (57) (Table 1).
5 LncRNAs bridges metabolic reprogramming and TME remodeling
It is not only tumor cells that require macronutrients and energy to meet their own needs for proliferation and metastasis. For purpose of accomplishing the proliferation, differentiation, and effector functions of various immune cells, the immune system requires a large number of similar nutrients for biosynthesis. In addition, the metabolic modes of immune cells in the activated and effector states are markedly distinguishable from those in the resting state. For example, the metabolism of naïve T cells is usually quiescent, mainly through oxidative phosphorylation to produce energy. Once differentiated into effector T-cells, they lean primarily upon glycolysis for energy production (58). Neutrophils rely mainly on aerobic glycolysis and the pentose phosphate pathway to provide energy. Glycolysis is a primary metabolic pathway in activated B lymphocytes (59). However, the oxidative phosphorylation of fatty acids is a major metabolic pattern in both regulatory T cells and M2 macrophages (60). After the tumor initiates metabolic reprogramming, the function of the immune system is impaired by the competitive uptake of nutrients, for instance, glucose, fatty acids, glutamine, and amino acids. Investigations have shown that cancer cells can compete for glucose uptake and enhance glycolysis to inhibit the function of tumor-infiltrating lymphocytes (TILs), limit glucose consumption by TILs, and ultimately result in T cell exhaustion and tumor immune evasion. Notably, not only changes in tumor metabolic patterns but also metabolites from tumor reprogramming can affect the phenotype and function of immune cells. The accumulation of lactic acid can inhibit the function of T and NK cells. Moreover, lactic acid upregulates the expression of PD-L1 on the surface of tumor cells and mediates T cell exhaustion by binding to the PD-1 receptor on the surface of T cells (61). In addition, reprogramming of tumor lipid metabolism can lead to increased cholesterol levels in the TME, which can upregulate the expression of inhibitory immune checkpoints, such as PD-1 and LAG-3, on the surface of T cells, leading to an increase in the number of exhausted T cells. Similarly, immune cells become less functional and proliferative and are more susceptible to apoptosis (62) (Figure 3).
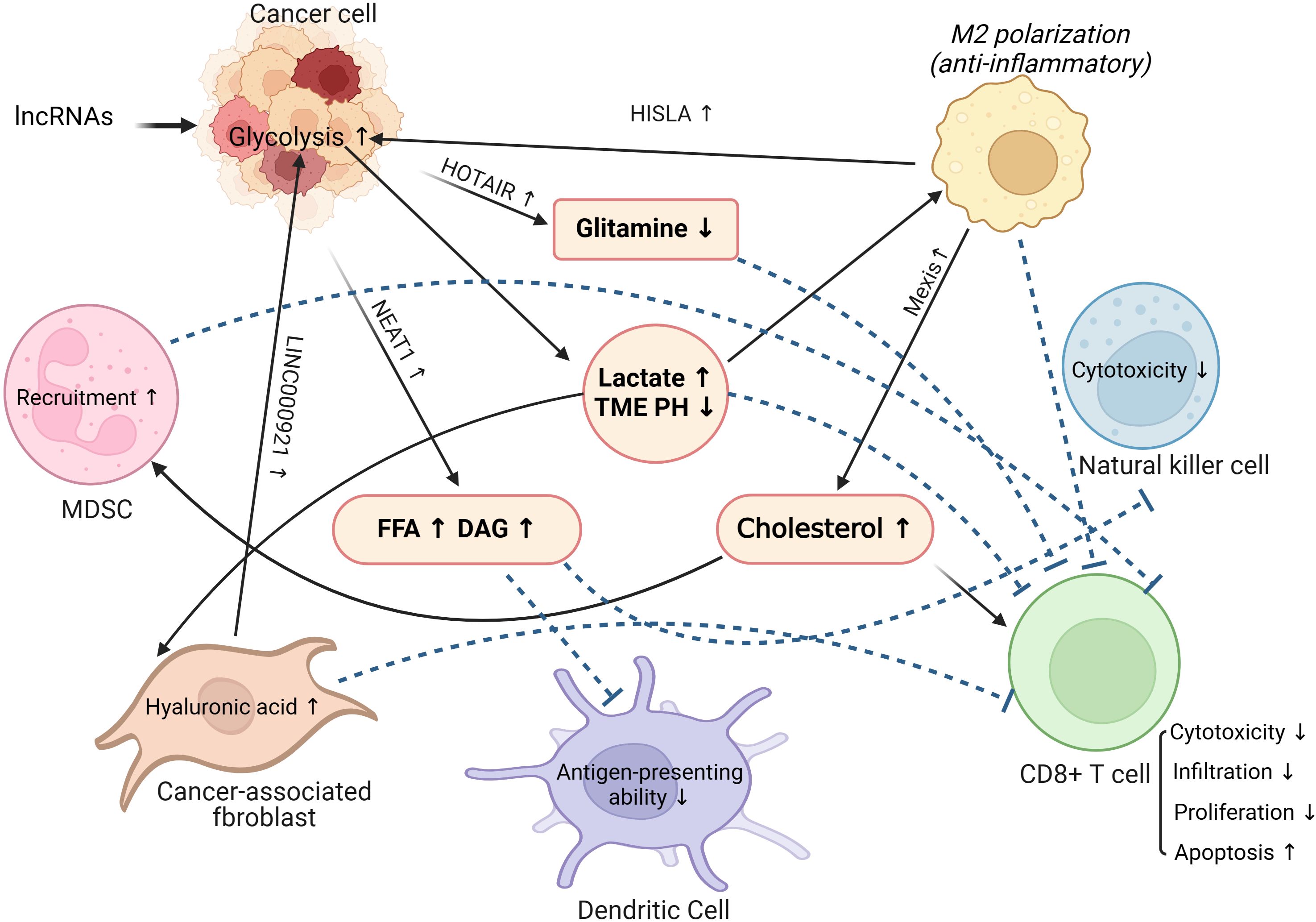
Figure 3. The role of lncRNAs in linking tumor metabolic reprogramming and tumor microenvironment remodeling. MDSC, Myeloid-derived suppressor cells; TME, Tumor microenvironment; FFA, Free fatty acids; DAG, Diacylglycerol.
Furthermore, existing research has confirmed that lncRNAs are closely related to the innate immune system (63). The innate immune system serves as the first line of defense against pathogens and relies on a surveillance system involving neutrophils, macrophages, natural killer cells, and dendritic cells. LncRNAs have been shown to function through modular domains, regulating gene expression and modulating pathogen response pathways by interacting with chromatin, RNA, and proteins (64).
They can engage in T cell development, activation, differentiation, function, and cancer immunology by binding specifically to the epidermal growth factor receptor (EGFR) or inducing the expression of cell surface phenotypes, as well as suppressing Treg-mediated immune evasion in hepatoma carcinoma cells (HCCs) (65, 66). Additionally, lncRNAs play a critical role in macrophage differentiation, recruitment, polarization, and functionality. They regulate macrophage differentiation by modulating ACVR1B (a key factor in macrophage differentiation) to activate the TGF-β pathway (67), or by influencing the calcium-dependent kinase PNCK triggered by Ca2+, which leads to macrophage recruitment and angiogenesis. Similarly, lncRNAs are involved in the reprogramming of normal fibroblasts (NF) to cancer-associated fibroblasts (CAFs). They participate in this process through exosomes delivery, gene knockout, and inhibition of autophagy-lysosomal degradation, which further modulates the metabolism and chemotherapy resistance of CAFs (68, 69).
Latest researches have indicated that lncRNAs upregulate the expression of key enzymes and transporters in tumor metabolic reprogramming through a variety of mechanisms and regulate related signaling pathways, eventually leading to changes in the TME (70–75) (Table 2). Therefore, lncRNAs may reprogram the tumor immune microenvironment by regulating tumor metabolism.
5.1 LncRNA in glucose metabolism and TME
Typically, normal human cells consume glucose and generate an ATP to provide energy to the body through oxidative phosphorylation under aerobic conditions. Interestingly, under aerobic conditions, tumor cells meet their energy requirements through glycolysis, an effect known as the “Warburg Effect” (99). Although aerobic glycolysis produces less ATP than does oxidative phosphorylation, it also produces less reactive oxygen species, which can induce apoptosis in cancer cells (100, 101). LncRNA influences tumor cells by affecting metabolic enzymes and signaling pathways, thus reprogramming their metabolic processes to preferentially convert glucose into lactate, regulating cancer growth, maintenance, and progression (102, 103). In addition, lactate, acetyl-CoA, and ribose generated during glucose metabolism can provide a favorable environment for rapid tumor proliferation and metastasis (104, 105). Five lncRNAs, including MIR4435-2HG, AC078846.1, AL157392.3, AP001273.1 and RAD51-AS1 significantly upregulated in tumors could activate tumor glycolytic metabolic pathways, and were strongly associated with high levels of poor prognosis-related molecules. In particular, the expression of lncRNA MIR4435-2HG was closely related to the high infiltration of M2 macrophages in hepatocellular carcinoma. The finding of these lncRNAs provides new ideas for the establishment of prognostic assessment models for tumor patients and the development of new therapeutic approaches (106–109). Therefore, alterations in glucose metabolism in tumors play a crucial role in their occurrence and development.
Several studies have verified that many lncRNAs affect glucose transporters, various key enzymes, and signaling pathways involved in glucose metabolism to regulate the reprogramming of glucose metabolism in tumors. For instance, the most studied lncRNA involved in genome modification (110), HOTAIR, which is transcribed in the reverse direction of HOXC and represses HOXD transcription by recruiting PRCI in fibroblasts (111), is commonly expressed in HCCs and accelerates cell proliferation by regulating glucose metabolism. Generally, HOTAIR upregulates GLUT1 expression via activating the mTOR signaling pathway and directly binds to HCCs to promote cell proliferation (76). Similarly, ANRIL, as well as UCA1, by activating mTOR signaling pathway and upregulating GLUT1 are involved in glucose metabolism reprogramming in nasopharyngeal carcinoma cells and bladder cancer cells, respectively (70, 83). Additionally, several studies have shown that lnc-p23154, NBR2, MACC1-AS1, LINC00174, and LINC00346 upregulate GLUT1 expression via different mechanisms, which enhance tumor glycolysis (75, 77–80). In contrast, the expression of lncRNA NEF in non-small cell lung cancer (NSCLC) is often decreased, resulting in the downregulation of GLUT1 expression and decreased glycolysis in tumor cells. Thus, NEF negatively regulates tumor progression in NSCLC (93). In addition, CamK-A and CRNDE increase glucose uptake in tumor cells by upregulating the expression of GLUT3 and GLUT4, respectively, to promote aerobic glycolysis (81, 82).
Apart from regulating glucose uptake, lncRNAs can interact with key enzymes and related glycolytic pathways to regulate glucose metabolism reprogramming in tumors. Hexokinase, phosphofructokinase, and pyruvate kinase are the key enzymes in glycolysis (112). UCA1, PVT1, and TUG1 upregulate hexokinase 2 (HK2) expression by interacting with miR-203, miR-497/miR-143, and miR-455-3p, respectively, to boost glycolysis (73, 84, 85, 113–116). LncRNA H19 can upregulate pyruvate kinase M2 (PKM2) expression to promote glycolysis. In addition, lncRNA H19 promotes lactate production through the miR-519D-3p/lactate dehydrogenase A signal axis (86, 117). The lncRNA AC020978 can also directly interact with PKM2 to facilitate glycolysis, proliferation, and invasion of cancer cells (87). Additionally, LINC00092 directly interacts with fructose-2,6-bisphosphatase (PFKFB2) to promote ovarian cancer metastasis by enhancing glycolysis and maintaining CAFs local support functions (71). In contrast, as a negative regulator, GAS5 suppresses glycolysis and tumor cell proliferation by inhibiting glucose 6-phosphate dehydrogenease and phosphoenolpyruvate carboxykinase expression (92). GLCC1, FILNC1, PDIA3P, and other lncRNAs regulate glycolysis by interacting with or regulating the expression of the transcription factor c-Myc (88–90). Besides, LincRNA-P21 and lncRNA AC020978 promote glycolysis by involving the HIF-1α pathway (87, 91).
A great deal of lactate is a significant product of aerobic glycolysis in tumors. Lactate plays an indispensable role in the reprogramming of tumor glucose metabolism and the remodeling of the TME. Elevated lactate concentrations both directly hinder the function of TILs and NK cells, while additionally diminishing their activity, consequently remodeling an immunosuppressive TME (61). Besides, lactate can activate GPR81 receptor on macrophages to lessen inflammasome activation and the generation of pro-inflammatory cytokines, such as IL-6 and TNF-α (118, 119). Moreover, the accumulation of lactate and the decrease in pH value in TME can induce the phenotype of immune cells in TME to be polarized toward immunosuppression and immune tolerance (120). For instance, lactate induces macrophages to polarize to the M2 subtype, sequentially producing the immunosuppressive factor IL-10 (121). Importantly, lncRNAs secreted by extracellular vesicles from tumor-associated macrophages (TAMs), HISLA, can reduce the hydroxylation and degradation of HIF-1α via blocking the interaction between PHD2 and HIF-1α, which promotes glycolysis and lactate accumulation in tumor cells. Conversely, the glycolytic product, lactate upregulates the expression of HISLA in TAMs, constituting a feedforward loop between cancer cells and TAMs (122).
CAFs not only form the matrix components of the TME but are also important regulatory factors in the TME. Large amounts of lactate in the TME would promote the generation of hyaluronic acid in CAFs, which facilitates tumor invasion and metastasis (123). Furthermore, in the presence of high levels of lactate in the TME, CAFs decrease the percentage of Th1 cell subsets via SIRT1-mediated deacetylation/degradation of the T-bet transcription factor. Besides, CAFs can promote the polarization of naive T cells to Treg cells by upregulating the expression of NF-kB and FoxP3 (124). Intriguingly, studies have shown that CAFs upregulate LINC00092 to promote aerobic glycolysis and lactate generation in ovarian cancer, which further reshapes the TME (71). Therefore, tumor metabolic reprogramming and TME remodeling are interlinked and involve complex regulatory networks. LncRNAs can act as upstream regulators and downstream effectors to promote tumor glycolysis and lactate secretion and mediate the remodeling of the immune microenvironment. Finally, the immune system progresses towards immunosuppression and immune tolerance.
5.2 LncRNA in lipid metabolism and TME
Apart from abnormal glucose metabolism, abnormal lipid metabolism is involved in tumor metabolic reprogramming (125). Characteristic changes in lipid metabolism in tumors include de novo lipid synthesis, lipid storage, and the esterification of cholesterol to free cholesterol. Abnormal lipid metabolism in tumor cells provides an important energy source and material for tumor cell proliferation and metastasis. Lipid metabolism plays a vital role in maintaining immune cell development and function in TME (126). Treg cells rely on lipid oxidation for energy rather than glycolysis because they have significantly few glucose transporters on their surfaces. Therefore, the addition of exogenous fatty acids can promote the production of Treg and inhibit the formation of effector T cells (126). T cells synthesize lipids as an energy source through the fatty acid oxidation catabolic pathway and induce lipid biosynthesis through de novo fatty acids (127). Additionally, fatty acids are indispensable for the memory and cytotoxic functions of CD8+ T cells (128). As vital regulators of fatty acid and fat synthesis, phospholipid metabolism, and transport, a large number of lncRNAs form a complex regulatory network through a variety of mechanisms and pathways to induce lipid reprogramming and immune microenvironment remodeling in tumor cells (129).
Studies have shown that HULC can induce the methylation of CpG islands in the promoter region of miR-9, resulting in an increase in peroxisome proliferation activated receptor alpha (PPARα) and acyl CoA synthetase 1 (ACSL1) level, which in turn leads to the accumulation of triglycerides and cholesterol in HCCs. The product of ACSL1 can activate the transcription factor RXRA to augment HULC expression, which leads to an increase in lipids in the TME (94). Similarly, NEAT1 can regulate adipose triglyceride lipase (ATGL) expression by binding to miR-124-3p in HCCs, leading to enhanced lipolysis and increased fatty acids and diglyceride levels (95). Recent research has shown that lncRNAs are enriched in both mitochondria and the cell nucleus, playing a direct or indirect role in significant metabolic processes such as fatty acid metabolism. For instance, circNFIX participates in fatty acid metabolism in breast cancer by regulating the expression of MMP9, while the cis gene ACACB modulates the rate-limiting enzyme in fatty acid oxidation, thereby controlling fatty acid metabolism (130, 131).Notably, lipid accumulation in the TME can further impair the function of a variety of immune cells and induce a tumor-immunosuppressive microenvironment. For example, increased lipid levels decrease the antigen presenting capacity of dendritic cells (132). Likewise, in the presence of PPARα-mediated abnormal fatty acids elevation, the function of NK cell-tumor synaptic transport is inhibited, resulting in poor immune surveillance (133). In addition to reshaping the TME by regulating tumor cell lipid metabolism, NATE1 directly inhibits immune cell function. For instance, NEAT1 inhibits the antitumor immune function of cytotoxic T cells by downregulating the cyclic GMP-AMP stimulatory expression of the interferon gene (72). NEAT1 also regulates dendritic cell and macrophage functions and phenotypes by inducing NLRP3 inflammasomes (74, 134). Low NEAT1 expression levels are associated with high CD8+ T cell infiltration in tumor tissues (72). Moreover, NEAT1 interacts with miR-214 to regulate B7-H3 expression in multiple myeloma, leading to the polarization of macrophages to the M2 subtype, thereby inducing the formation of a tumor-immunosuppressive microenvironment (135).
In the reprogramming of tumor lipid metabolism, cholesterol and its derivatives also play vital roles in inducing a tumor immunosuppressive microenvironment (136). Previous studies have reported that cholesterol derived from the TME increases the expression of CD36 and fatty acid uptake by CD8+ T cells. Superabundant fatty acid intake can induce lipid peroxidation and ferroptosis of CD8+ T cells, which impairs antitumor immune function (137). In addition, the cholesterol hydroxylation products 25-hydroxycholesterol and 27-hydroxycholesterol can increase MDSC infiltration and reduce CD8+T cell numbers in the TME, inducing tumor immunosuppression (138, 139). Under conditions of LXR activation, lncRNA MeXis can enhance ABCA1 expression to facilitate cholesterol efflux (96). Similarly, the LincRNA-DYNLRB2-2 upregulates low density lipoprotein-induced ABCA1 expression, leading to increased cholesterol efflux (97). Cholesterol efflux increases the levels of cholesterol and its derivatives in the TME, thereby inducing TME immunosuppression and immune tolerance.
Sphingolipids, especially ceramides are also critical for TME. By regulating sphingolipids, lncRNAs can further regulate biological behaviors of tumors, such as the occurrence and development. For example, lncRNA ST3Gal6 antisense 1 (ST3Gal6-AS1) promotes metabolism of ceramide in osteosarcoma cells, then promoting the polarization of M2 macrophages, leading to higher level of IL-6 and IL-10 and causing immune escape in osteosarcoma (140, 141). Among sphingolipids-associated lncRNAs, lncRNA ceramide synthase 6 antisense RNA 1 (CERS6-AS1) is the most studied. In May 2022, Zhao et al. pointed out that lncRNA CERS6-AS1 upregulated ceramide and spectrin beta, non-erythrocytic 2 (SPTBN2), thereby promoting the malignant phenotype of colorectal cancer (142). Subsequently, the role of lncRNA CERS6-AS1 in various cancers was studied. LncRNA CERS6-AS1 promotes transcription of ceramide and the proliferation of pancreatic cancer cells, breast cancer cells and cervical cancer cells (143–145).
Overall, lncRNAs and their signaling pathways constitute a complex network that precisely regulates tumor lipid metabolism. The TME, including immune cells, is also affected. An exhaustive understanding of the mechanisms of the entire regulatory network can help us identify new approaches for antitumor therapy.
5.3 LncRNA in amino acid and TME
Amino acids are key nutrients for cancer cells, and the regulation of their metabolism has become a focal point for many studies. These investigations focus on changes in key enzyme genes involved in amino acid metabolism, as well as epigenetic modifications, transcription, translation, and post-translational modifications. Disruption of amino acid metabolism is one of the critical features of cancer metabolism. Amino acid metabolism plays a vital role in cancer cells, including energy production, nucleotide synthesis, and maintaining redox homeostasis. To endure in this nutrient-constrained milieu, cancer cells employ a diverse array of trophic sensing and metabolic mechanisms, dynamically reprogramming their metabolic pathways (146, 147). Accumulating evidence underscores the pivotal role of lncRNAs in these adaptive metabolic responses, particularly in instances where numerous ncRNAs undergo dysregulation under conditions of nutrient scarcity (148). These lncRNAs profoundly influence metabolic alterations and contribute to the malignant transformation of cancer cells (149). Also of interest is the short peptide coding capacity of lncRNAs, despite their limited coding capacity was generally recognized, which has also been confirmed in several recent studies in part (150). Especially the capacity to regulate fatty acid metabolism and redox processes, which can be achieved through peptide-coding lncRNAs, subsequently diminishing the viability and migration of cancer cells (151).
5.3.1 Glutamine metabolism
Glutamine metabolism plays an indispensable role in tumor metabolic reprogramming by providing a carbon source for the tricarboxylic acid cycle and a nitrogen source for amino acid and nucleotide synthesis to meet the demand for rapid tumor cell proliferation. Liu et al. found that lncRNA HOTAIR was abnormally upregulated in glioma, and its content was negatively correlated with miR-126-5p. As a competitive endogenous RNA of miR-126-5p, upregulated HOTAIR led to decreasing expression of glutaminase (GLS), thereby inhibiting glutamine metabolism and enhancing the malignancy of glioma. Understanding the regulatory mechanisms of the HOTAIR/miR-126/GLS axis in gliomas could promote novel treatments for this disease (98). Moreover, HOTAIR also promotes the secretion of CCL2, which recruits TAMs and MDSCs to the TME to induce tumor immunosuppression (152). Upregulation of GLS expression promotes glutamine depletion in tumor cells, leading to HIF-1α activation and IL-23 secretion by TAMs, which can inhibit the tumor-killing effect of cytotoxic lymphocytes (153). Additionally, high glutamine consumption promotes glutamate excretion. Therefore, the metabolism of glutamine can be affected by lncRNA and GLS, thus playing an important role in tumor metabolic reprogramming.
5.3.2 Serine metabolism
Serine is another non-essential amino acid that plays a role in nucleotide synthesis, oxidative stress responses, the TCA cycle, and various other metabolic processes. It can be sourced from extracellular uptake or synthesized through the serine synthesis pathway. The expression of lncRNA MEG8 shows a positive correlation with PSAT1 expression and serine synthesis, with PSAT1 functioning as a competing endogenous RNA (ceRNA) that interacts with miR-15a-5p and miR-15b-5p (154). Interestingly, lncRNAs can influence serine metabolism by modulating the expression of SHMT2 in various types of cancer. In lung cancer, the targets of miR-615-5p include IGF2, SHMT2, and AKT2. LncRNA Gm15290 interacts with miR-615-5p and exhibits a negative correlation with its levels (155). LncRNAs are essential in regulating various enzymes within the serine synthesis pathway and affect tumor progression by modulating serine metabolism. Consequently, further investigation into the regulatory roles of lncRNAs on serine metabolism is of great significance.
5.3.3 Aspartate metabolism
Aspartic acid, one of the amino acids present in the lowest concentrations in the blood, is crucial for protein and nucleotide synthesis, significantly supporting cell growth. The impact of lncRNAs on aspartic acid metabolism primarily involves the regulation of GOT expression. GOT is an essential enzyme linked to both aspartic acid and carbohydrate metabolism, and it also facilitates cancer cell proliferation by helping to maintain redox balance. LncRNA NEAT1 can modulate the expression of GOT1 and the transferrin receptor (TFRC) during ferroptosis (156). Silencing lncRNA-ACOD1 markedly decreases macrophage infections by vesicular stomatitis virus (VSV), vaccinia virus (VACV), and herpes simplex virus type 1 (HSV-1). This indicates a strong association between lncRNAs and aspartic acid metabolism, suggesting that these lncRNAs could be valuable in improving tumor immunotherapy.
Several studies have indicated that Amino acid metabolism is important for tumorigenesis and tumor immunity. However, studies on the regulation of Amino acid metabolism by lncRNAs in tumors are limited. lncRNAs related to Amino acid metabolism are promising metabolic targets for cancer treatment. Future researches should be concentrated on the mechanism by which lncRNAs regulate Amino acid metabolism and immune microenvironment remodeling and identify clinically meaningful targets for anti-tumor molecular targeted therapy.
6 Future clinical applications of lncRNA
It has been shown that TAMs secrete lncRNA HISLA via extracellular vesicles. HISLA can reduce the hydroxylation and degradation of HIF-1α via blocking the interaction between PHD2 and HIF-1α, which promotes glycolysis and lactate accumulation in tumor cells. Accumulated lactic acid can further prompt TAMs to secrete HISLA. Thus, HISLA silencing mediated by RNA interference has great potential to interrupt tumor glucose metabolic reprogramming, which will further weaken TME immunosuppression induced by tumor metabolic reprogramming and help restore immune cell function. The use of aptamer-siRNA chimeras to mediate specific HISLA knockdown in TAMs to abort tumor glucose metabolism remodeling and restore antitumor immune functions warrants further investigation. Similarly, lncRNA HIFAL can promote HIF-1α transactivation complex assembly and glycolytic metabolism. Inhibition of HIFAL can weaken tumor glycolysis and reduce the tumor energy supply and glycolytic products, weakening the inhibitory effect of tumor metabolic reprogramming on immune cells (122). This is a potential new target for antitumor therapy.
In addition to lncRNAs involved in regulating tumor metabolism, the immune checkpoint signaling axis regulates tumor metabolic reprogramming. PD-L1 expression in cancer cells can activate the Akt-mTOR signaling axis, enhance glycolysis, prompt glucose competition between the tumor and T cells, and increase lactate production. Notably, lactate accumulation, glucose stress, and PH value decrease in the TME also upregulate PD-1 expression, which promotes tumor immunosuppression and immune evasion (61). Immune checkpoint inhibitors inhibit glycolysis to a certain extent and weaken immune suppression; however, the overall therapeutic efficiency of immunotherapy remains limited. Whether anti-lncRNA-targeted metabolic therapy combined with immunotherapy can further improve the effects of tumor treatment and enhance antitumor immunity is worth exploring in the future. Theoretically, the inhibition of dysregulated lncRNA-mediated reprogramming of tumor metabolism could restore its suppressive effects on immune cells. However, it is undeniable that, given the physiological situation, these metabolic processes also contribute to the activation of the immune system, and the interruption of these metabolic processes will also influence the activation of immune cells and anti-tumor immunity. Therefore, identifying the best method to accurately target disordered tumor metabolic reprogramming is a direction for our future research.
Targeting lncRNAs operate precise biological functions through various mechanisms, notably as guides, scaffolds, and decoy molecules, among others. A profound understanding of these multifaceted roles is crucial for the development of highly efficient targeting strategies (157). However, the clinical implementation of therapeutic targeting of lncRNAs has been constrained by several unresolved issues. Firstly, the low level of conservation among species for lncRNAs poses a significant hindrance to numerous research models. Furthermore, the subcellular localization of lncRNAs varies significantly between human and mouse embryonic stem cells, and their stability also differs markedly between the two species (158). This implies the potential irrelevance of findings from other organisms to human clinical contexts consequently (159). Moreover, lncRNAs enable targeting multiple genes, which increases the potential probability of off-target effects when attempting to target them. It is imperative that clinical trials meticulously consider both the likelihood and the extent of these effects, aiming to mitigate them in the safest manner possible. Notably, these intricacies may not be fully elucidated through animal studies alone is a formidable challenge in lncRNA-targeting strategies (160). To mitigate the aforementioned issues, incorporating transcriptome-wide association study (TWAS) could serve as an effective complementary approach to genome-wide association studies, enhancing our ability to identify genes linked to traits like diseases and elucidating the intricate regulatory interactions among them (161). For lncRNAs, the genetic association signal about transcript abundance within a particular tissue can be juxtaposed against the signal associated with a specific disease, thereby facilitating the validation of the disease’s causal relationship. Employing these strategies enables the identification of lncRNAs that hold the greatest relevance for experimental investigation. Ideally, these lncRNAs should be tested using humanized models to gain a deeper insight into their underlying mechanisms of action (160, 162).
Existing tumor markers have poor sensitivity and specificity. However, reliable biomarkers for predicting the prognosis of patients with cancer are still lacking. Considering the high stability of lncRNAs in blood and their resistance to nuclease degradation, circulating lncRNAs appear to be reliable and promising prognostic biomarkers. Circulating lncRNAs have been used as reliable diagnostic and prognostic markers in various cancers, including liver, colorectal, gastric, and prostate cancers (163). In particular, the available biomarkers for predicting the response to immunotherapy are limited. Given the role of lncRNAs in mediating the immunosuppressive TME, circulating lncRNAs are expected to be complementary markers for predicting the efficacy of immunotherapy. Further studies are needed to explore the feasibility of using lncRNAs as prognostic markers in clinical practice.
7 Conclusions
Tumors initiate metabolic reprogramming to support their rapid proliferation, altering the TME. This triggers competition for nutrients between tumor and immune cells, leading to metabolic changes in immune cells that promote an immunosuppressive microenvironment and tumor immune evasion. LncRNAs impact TME remodeling via these metabolic shifts. Targeted lncRNA therapy aims to overcome immunotherapy resistance and drug tolerance in tumor patients, presenting a novel anti-tumor strategy.
Author contributions
JJ: Writing – original draft. YZ: Writing – review & editing, Supervision. QL: Writing – original draft, Conceptualization. SJ: Writing – review & editing, Visualization. ZL: Writing – review & editing.
Funding
The author(s) declare that financial support was received for the research, authorship, and/or publication of this article. This work was supported by Department of Science and Technology of Jilin Province (Grant No. YDZJ202301ZYTS086); Department of Health Science and Technology of Jilin Province (Grant No. 2022LC115); Department of Science and Technology of Jilin Province (Grant No. YDZJ202201ZYTS135); Bethune Plan of Jilin University (Grant No. 2023B08).
Conflict of interest
The authors declare that the research was conducted in the absence of any commercial or financial relationships that could be construed as a potential conflict of interest.
Publisher’s note
All claims expressed in this article are solely those of the authors and do not necessarily represent those of their affiliated organizations, or those of the publisher, the editors and the reviewers. Any product that may be evaluated in this article, or claim that may be made by its manufacturer, is not guaranteed or endorsed by the publisher.
References
1. Siegel RL, Miller KD, Jemal A. Cancer statistics, 2019. CA Cancer J Clin. (2019) 69:7–34. doi: 10.3322/caac.21551
2. Guerra L, Bonetti L, Brenner D. Metabolic Modulation of Immunity: A New Concept in Cancer Immunotherapy. Cell Rep. (2020) 32:107848. doi: 10.1016/j.celrep.2020.107848
3. Hurley HJ, Dewald H, Rothkopf ZS, Singh S, Jenkins F, Deb P, et al. Frontline Science: AMPK regulates metabolic reprogramming necessary for interferon production in human plasmacytoid dendritic cells. J Leukoc Biol. (2021) 109:299–308. doi: 10.1002/JLB.3HI0220-130
4. Kolb D, Kolishetti N, Surnar B, Sarkar S, Guin S, Shah AS, et al. Metabolic Modulation of the Tumor Microenvironment Leads to Multiple Checkpoint Inhibition and Immune Cell Infiltration. ACS Nano. (2020) 14:11055–66. doi: 10.1021/acsnano.9b10037
5. Anastasiadou E, Jacob LS, Slack FJ. Non-coding RNA networks in cancer. Nat Rev Cancer. (2018) 18:5–18. doi: 10.1038/nrc.2017.99
6. Gutschner T, Diederichs S. The hallmarks of cancer: a long non-coding RNA point of view. RNA Biol. (2012) 9:703–19. doi: 10.4161/rna.20481
7. Calin GA, Croce CM. MicroRNA-cancer connection: the beginning of a new tale. Cancer Res. (2006) 66:7390–4. doi: 10.1158/0008-5472.CAN-06-0800
8. He XY, Fan X, Qu L, Wang X, Jiang L, Sang LJ, et al. LncRNA modulates Hippo-YAP signaling to reprogram iron metabolism. Nat Commun. (2023) 14:2253. doi: 10.1038/s41467-023-37871-5
9. Yang J, Liu F, Wang Y, Qu L, Lin A. LncRNAs in tumor metabolic reprogramming and immune microenvironment remodeling. Cancer Lett. (2022) 543:215798. doi: 10.1016/j.canlet.2022.215798
10. Huarte M. The emerging role of lncRNAs in cancer. Nat Med. (2015) 21:1253–61. doi: 10.1038/nm.3981
11. Bhan A, Soleimani M, Mandal SS. Long Noncoding RNA and Cancer: A New Paradigm. Cancer Res. (2017) 77:3965–81. doi: 10.1158/0008-5472.CAN-16-2634
12. Latgé G, Poulet C, Bours V, Josse C, Jerusalem G. Natural Antisense Transcripts: Molecular Mechanisms and Implications in Breast Cancers. Int J Mol Sci. (2018) 19(1). doi: 10.3390/ijms19010123
13. Grassi L, Izuogu OG, Jorge NAN, Seyres D, Bustamante M, Burden F, et al. Cell type-specific novel long non-coding RNA and circular RNA in the BLUEPRINT hematopoietic transcriptomes atlas. Haematologica. (2021) 106:2613–23. doi: 10.3324/haematol.2019.238147
14. Engreitz JM, Haines JE, Perez EM, Munson G, Chen J, Kane M, et al. Local regulation of gene expression by lncRNA promoters, transcription and splicing. Nature. (2016) 539:452–5. doi: 10.1038/nature20149
15. Chen CK, Blanco M, Jackson C, Aznauryan E, Ollikainen N, Surka C, et al. Xist recruits the X chromosome to the nuclear lamina to enable chromosome-wide silencing. Science. (2016) 354:468–72. doi: 10.1126/science.aae0047
16. Schertzer MD, Braceros KCA, Starmer J, Cherney RE, Lee DM, Salazar G, et al. lncRNA-Induced Spread of Polycomb Controlled by Genome Architecture, RNA Abundance, and CpG Island DNA. Mol Cell. (2019) 75:523–537.e10. doi: 10.1016/j.molcel.2019.05.028
17. Tsai MC, Manor O, Wan Y, Mosammaparast N, Wang JK, Lan F, et al. Long noncoding RNA as modular scaffold of histone modification complexes. Science. (2010) 329:689–93. doi: 10.1126/science.1192002
18. Gupta RA, Shah N, Wang KC, Kim J, Horlings HM, Wong DJ, et al. Long non-coding RNA HOTAIR reprograms chromatin state to promote cancer metastasis. Nature. (2010) 464:1071–6. doi: 10.1038/nature08975
19. Coe EA, Tan JY, Shapiro M, Louphrasitthiphol P, Bassett AR, Marques AC, et al. The MITF-SOX10 regulated long non-coding RNA DIRC3 is a melanoma tumour suppressor. PloS Genet. (2019) 15:e1008501. doi: 10.1371/journal.pgen.1008501
20. Shahabi S, Kumaran V, Castillo J, Cong Z, Nandagopal G, Mullen DJ, et al. LINC00261 Is an Epigenetically Regulated Tumor Suppressor Essential for Activation of the DNA Damage Response. Cancer Res. (2019) 79:3050–62. doi: 10.1158/0008-5472.CAN-18-2034
21. Long Y, Wang X, Youmans DT, Cech TR. How do lncRNAs regulate transcription? Sci Adv. (2017) 3:eaao2110.
22. Carrieri C, Cimatti L, Biagioli M, Beugnet A, Zucchelli S, Fedele S, et al. Long non-coding antisense RNA controls Uchl1 translation through an embedded SINEB2 repeat. Nature. (2012) 491:454–7. doi: 10.1038/nature11508
23. Yari H, Jin L, Teng L, Wang Y, Wu Y, Liu GZ, et al. LncRNA REG1CP promotes tumorigenesis through an enhancer complex to recruit FANCJ helicase for REG3A transcription. Nat Commun. (2019) 10:5334. doi: 10.1038/s41467-019-13313-z
24. Dimitrova N, Zamudio JR, Jong RM, Soukup D, Resnick R, Sarma K, et al. LincRNA-p21 activates p21 in cis to promote Polycomb target gene expression and to enforce the G1/S checkpoint. Mol Cell. (2014) 54:777–90. doi: 10.1016/j.molcel.2014.04.025
25. Zheng F, Chen J, Zhang X, Wang Z, Chen J, Lin X, et al. The HIF-1α antisense long non-coding RNA drives a positive feedback loop of HIF-1α mediated transactivation and glycolysis. Nat Commun. (2021) 12:1341. doi: 10.1038/s41467-021-21535-3
26. Noh JH, Kim KM, Abdelmohsen K, Yoon JH, Panda AC, Munk R, et al. HuR and GRSF1 modulate the nuclear export and mitochondrial localization of the lncRNA RMRP. Genes Dev. (2016) 30:1224–39. doi: 10.1101/gad.276022.115
27. Bharadwaj A, Bydoun M, Holloway R, Waisman D. Annexin A2 heterotetramer: structure and function. Int J Mol Sci. (2013) 14:6259–305. doi: 10.3390/ijms14036259
28. Liu PY, Tee AE, Milazzo G, Hannan KM, Maag J, Mondal S, et al. The long noncoding RNA lncNB1 promotes tumorigenesis by interacting with ribosomal protein RPL35. Nat Commun. (2019) 10:5026. doi: 10.1038/s41467-019-12971-3
29. Müller V, Oliveira-Ferrer L, Steinbach B, Pantel K, Schwarzenbach H. Interplay of lncRNA H19/miR-675 and lncRNA NEAT1/miR-204 in breast cancer. Mol Oncol. (2019) 13:1137–49.
30. Wang Y, Lu JH, Wu QN, Jin Y, Wang DS, Chen YX, et al. LncRNA LINRIS stabilizes IGF2BP2 and promotes the aerobic glycolysis in colorectal cancer. Mol Cancer. (2019) 18:174. doi: 10.1186/s12943-019-1105-0
31. Shi Q, Li Y, Li S, Jin L, Lai H, Wu Y, et al. LncRNA DILA1 inhibits Cyclin D1 degradation and contributes to tamoxifen resistance in breast cancer. Nat Commun. (2020) 11:5513. doi: 10.1038/s41467-020-19349-w
32. Zhuo W, Liu Y, Li S, Guo D, Sun Q, Jin J, et al. Long Noncoding RNA GMAN, Up-regulated in Gastric Cancer Tissues, Is Associated With Metastasis in Patients and Promotes Translation of Ephrin A1 by Competitively Binding GMAN-AS. Gastroenterology. (2019) 156:676–691.e11. doi: 10.1053/j.gastro.2018.10.054
33. Faubert B, Solmonson A, DeBerardinis RJ. Metabolic reprogramming and cancer progression. Science. (2020) 368(6487). doi: 10.1126/science.aaw5473
34. Jing Z, Liu Q, He X, Jia Z, Xu Z, Yang B, et al. NCAPD3 enhances Warburg effect through c-myc and E2F1 and promotes the occurrence and progression of colorectal cancer. J Exp Clin Cancer Res. (2022) 41:198. doi: 10.1186/s13046-022-02412-3
35. Vaupel P, Schmidberger H, Mayer A. The Warburg effect: essential part of metabolic reprogramming and central contributor to cancer progression. Int J Radiat Biol. (2019) 95:912–9. doi: 10.1080/09553002.2019.1589653
36. Venkateswaran N, Lafita-Navarro MC, Hao YH, Kilgore JA, Perez-Castro L, Braverman J, et al. MYC promotes tryptophan uptake and metabolism by the kynurenine pathway in colon cancer. Genes Dev. (2019) 33:1236–51. doi: 10.1101/gad.327056.119
37. Dorai T, Pinto JT, Denton TT, Krasnikov BF, Cooper AJL. The metabolic importance of the glutaminase II pathway in normal and cancerous cells. Anal Biochem. (2022) 644:114083. doi: 10.1016/j.ab.2020.114083
38. Paul B, Lewinska M, Andersen JB. Lipid alterations in chronic liver disease and liver cancer. JHEP Rep. (2022) 4:100479. doi: 10.1016/j.jhepr.2022.100479
39. Phan LM, Yeung SC, Lee MH. Cancer metabolic reprogramming: importance, main features, and potentials for precise targeted anti-cancer therapies. Cancer Biol Med. (2014) 11:1–19.
41. Vaupel P, Multhoff G. Revisiting the Warburg effect: historical dogma versus current understanding. J Physiol. (2021) 599:1745–57. doi: 10.1113/tjp.v599.6
42. Baginska J, Viry E, Paggetti J, Medves S, Berchem G, Moussay E, et al. The critical role of the tumor microenvironment in shaping natural killer cell-mediated anti-tumor immunity. Front Immunol. (2013) 4:490. doi: 10.3389/fimmu.2013.00490
43. Hinshaw DC, Shevde LA. The Tumor Microenvironment Innately Modulates Cancer Progression. Cancer Res. (2019) 79:4557–66. doi: 10.1158/0008-5472.CAN-18-3962
44. Chang CH, Curtis JD Jr, Maggi LB, Faubert B, Villarino AV, O'Sullivan D, et al. Posttranscriptional control of T cell effector function by aerobic glycolysis. Cell. (2013) 153:1239–51. doi: 10.1016/j.cell.2013.05.016
45. Li L, Wang L, Li J, Fan Z, Yang L, Zhang Z, et al. Metformin-Induced Reduction of CD39 and CD73 Blocks Myeloid-Derived Suppressor Cell Activity in Patients with Ovarian Cancer. Cancer Res. (2018) 78:1779–91. doi: 10.1158/0008-5472.CAN-17-2460
46. Nanayakkara G, Alasmari A, Mouli S, Eldoumani H, Quindry J, McGinnis G, et al. Cardioprotective HIF-1α-frataxin signaling against ischemia-reperfusion injury. Am J Physiol Heart Circ Physiol. (2015) 309:H867–79. doi: 10.1152/ajpheart.00875.2014
47. Jhunjhunwala S, Hammer C, Delamarre L. Antigen presentation in cancer: insights into tumour immunogenicity and immune evasion. Nat Rev Cancer. (2021) 21:298–312. doi: 10.1038/s41568-021-00339-z
48. Schaller J, Agudo J. Metastatic Colonization: Escaping Immune Surveillance. Cancers (Basel). (2020) 12(11). doi: 10.3390/cancers12113385
49. Chen L, Zheng H, Yu X, Liu L, Li H, Zhu H, et al. Tumor-Secreted GRP78 Promotes the Establishment of a Pre-metastatic Niche in the Liver Microenvironment. Front Immunol. (2020) 11:584458. doi: 10.3389/fimmu.2020.584458
50. Leary N, Walser S, He Y, Cousin N, Pereira P, Gallo A, et al. Melanoma-derived extracellular vesicles mediate lymphatic remodelling and impair tumour immunity in draining lymph nodes. J Extracell Vesicles. (2022) 11:e12197. doi: 10.1002/jev2.12197
51. Taki M, Abiko K, Baba T, Hamanishi J, Yamaguchi K, Murakami R, et al. Snail promotes ovarian cancer progression by recruiting myeloid-derived suppressor cells via CXCR2 ligand upregulation. Nat Commun. (2018) 9:1685. doi: 10.1038/s41467-018-03966-7
52. Deng M, Wu D, Zhang Y, Jin Z, Miao J. MiR-29c downregulates tumor-expressed B7-H3 to mediate the antitumor NK-cell functions in ovarian cancer. Gynecol Oncol. (2021) 162:190–9. doi: 10.1016/j.ygyno.2021.04.013
53. Ercolano G, Wyss T, Salome B, Romero P, Trabanelli S, Jandus C. Distinct and shared gene expression for human innate versus adaptive helper lymphoid cells. J Leukocyte Biol. (2020) 108:723–37. doi: 10.1002/JLB.5MA0120-209R
54. Wu Q, Yuan X, Zhang H, Xiu R. Differential expression of lncRNAs in hypertension-induced pericytes. Scandinavian Cardiovasc J. (2021) 55:102–5. doi: 10.1080/14017431.2020.1852306
55. Zhou Z, Wang P, Sun R, Li J, Hu Z, Xin H, et al. Tumor-associated neutrophils and macrophages interaction contributes to intrahepatic cholangiocarcinoma progression by activating STAT3. J Immunother Cancer. (2021) 9(3). doi: 10.1136/jitc-2020-001946
56. Li H, Zeng C, Shu C, Cao Y, Shao W, Zhang M, et al. Laminins in tumor-derived exosomes upregulated by ETS1 reprogram omental macrophages to promote omental metastasis of ovarian cancer. Cell Death Dis. (2022) 13:1028. doi: 10.1038/s41419-022-05472-7
57. Qi M, Xia Y, Wu Y, Zhang Z, Wang X, Lu L, et al. Lin28B-high breast cancer cells promote immune suppression in the lung pre-metastatic niche via exosomes and support cancer progression. Nat Commun. (2022) 13:897. doi: 10.1038/s41467-022-28438-x
58. Sohrabi Y, Lagache SMM, Voges VC, Semo D, Sonntag G, Hanemann I, et al. OxLDL-mediated immunologic memory in endothelial cells. J Mol Cell Cardiol. (2020) 146:121–32. doi: 10.1016/j.yjmcc.2020.07.006
59. Pearce EL, Poffenberger MC, Chang CH, Jones RG. Fueling immunity: insights into metabolism and lymphocyte function. Science. (2013) 342:1242454. doi: 10.1126/science.1242454
60. Gardiner CM. NK cell metabolism. J Leukoc Biol. (2019) 105:1235–42. doi: 10.1002/JLB.MR0718-260R
61. Renner K, Bruss C, Schnell A, Koehl G, Becker HM, Fante M, et al. Restricting Glycolysis Preserves T Cell Effector Functions and Augments Checkpoint Therapy. Cell Rep. (2019) 29:135–150.e9. doi: 10.1016/j.celrep.2019.08.068
62. Perrone F, Minari R, Bersanelli M, Bordi P, Tiseo M, Favari E, et al. The Prognostic Role of High Blood Cholesterol in Advanced Cancer Patients Treated With Immune Checkpoint Inhibitors. J Immunother. (2020) 43:196–203. doi: 10.1097/CJI.0000000000000321
63. Guo YL, Xie YJ, Luo Y. The Role of Long Non-Coding RNAs in the Tumor Immune Microenvironment. Front Immunol. (2022) 13. doi: 10.3389/fimmu.2022.851004
64. Chen YG, Satpathy AT, Chang HY. Gene regulation in the immune system by long noncoding RNAs. Nat Immunol. (2017) 18:962–72. doi: 10.1038/ni.3771
65. Jiang R, Tang J, Chen Y, Deng L, Ji J, Xie Y, et al. The long noncoding RNA lnc-EGFR stimulates T-regulatory cells differentiation thus promoting hepatocellular carcinoma immune evasion. Nat Commun. (2017) 8. doi: 10.1038/ncomms15129
66. Yu Z, Zhao H, Feng X, Li H, Qiu C, Yi X, et al. Long Non-coding RNA FENDRR Acts as a miR-423-5p Sponge to Suppress the Treg-Mediated Immune Escape of Hepatocellular Carcinoma Cells. Mol Therapy-Nucleic Acids. (2019) 17:516–29. doi: 10.1016/j.omtn.2019.05.027
67. Chen MT, Lin HS, Shen C, Ma YN, Wang F, Zhao HL, et al. PU.1-Regulated Long Noncoding RNA lnc-MC Controls Human Monocyte/Macrophage Differentiation through Interaction with MicroRNA 199a-5p. Mol Cell Biol. (2015) 35:3212–24. doi: 10.1128/MCB.00429-15
68. Deng X, Ruan H, Zhang X, Xu X, Zhu Y, Peng H, et al. Long noncoding RNA CCAL transferred from fibroblasts by exosomes promotes chemoresistance of colorectal cancer cells. Int J Cancer. (2020) 146:1700–16. doi: 10.1002/ijc.v146.6
69. Zhao L, Ji G, Le X, Wang C, Xu L, Feng M, et al. Long Noncoding RNA LINC00092 Acts in Cancer-Associated Fibroblasts to Drive Glycolysis and Progression of Ovarian Cancer. Cancer Res. (2017) 77:1369–82. doi: 10.1158/0008-5472.CAN-16-1615
70. Zou ZW, Ma C, Medoro L, Chen L, Wang B, Gupta R, et al. LncRNA ANRIL is up-regulated in nasopharyngeal carcinoma and promotes the cancer progression via increasing proliferation, reprograming cell glucose metabolism and inducing side-population stem-like cancer cells. Oncotarget. (2016) 7:61741–54. doi: 10.18632/oncotarget.11437
71. Zhao L, Ji G, Le X, Wang C, Xu L, Feng M, et al. Long Noncoding RNA LINC00092 Acts in Cancer-Associated Fibroblasts to Drive Glycolysis and Progression of Ovarian Cancer. Cancer Res. (2017) 77:1369–82. doi: 10.1158/0008-5472.CAN-16-1615
72. Ma F, Lei YY, Ding MG, Luo LH, Xie YC, Liu XL. LncRNA NEAT1 Interacted With DNMT1 to Regulate Malignant Phenotype of Cancer Cell and Cytotoxic T Cell Infiltration via Epigenetic Inhibition of p53, cGAS, and STING in Lung Cancer. Front Genet. (2020) 11:250. doi: 10.3389/fgene.2020.00250
73. Zhang Y, Liu Y, Xu X. Knockdown of LncRNA-UCA1 suppresses chemoresistance of pediatric AML by inhibiting glycolysis through the microRNA-125a/hexokinase 2 pathway. J Cell Biochem. (2018) 119:6296–308. doi: 10.1002/jcb.v119.7
74. Zhang M, Zheng Y, Sun Y, Li S, Chen L, Jin X, et al. Knockdown of NEAT1 induces tolerogenic phenotype in dendritic cells by inhibiting activation of NLRP3 inflammasome. Theranostics. (2019) 9:3425–42. doi: 10.7150/thno.33178
75. Wang Y, Zhang X, Wang Z, Hu Q, Wu J, Li Y, et al. LncRNA-p23154 promotes the invasion-metastasis potential of oral squamous cell carcinoma by regulating Glut1-mediated glycolysis. Cancer Lett. (2018) 434:172–83. doi: 10.1016/j.canlet.2018.07.016
76. Wei S, Fan Q, Yang L, Zhang X, Ma Y, Zong Z, et al. Promotion of glycolysis by HOTAIR through GLUT1 upregulation via mTOR signaling. Oncol Rep. (2017) 38:1902–8. doi: 10.3892/or.2017.5840
77. Liu X, Xiao ZD, Han L, Zhang J, Lee SW, Wang W, et al. LncRNA NBR2 engages a metabolic checkpoint by regulating AMPK under energy stress. Nat Cell Biol. (2016) 18:431–42. doi: 10.1038/ncb3328
78. Zhao Y, Liu Y, Lin L, Huang Q, He W, Zhang S, et al. The lncRNA MACC1-AS1 promotes gastric cancer cell metabolic plasticity via AMPK/Lin28 mediated mRNA stability of MACC1. Mol Cancer. (2018) 17:69. doi: 10.1186/s12943-018-0820-2
79. Shi J, Zhang Y, Qin B, Wang Y, Zhu X. Long non-coding RNA LINC00174 promotes glycolysis and tumor progression by regulating miR-152-3p/SLC2A1 axis in glioma. J Exp Clin Cancer Res. (2019) 38:395. doi: 10.1186/s13046-019-1390-x
80. Li Y, Li H, Wang W, Yu X, Xu Q. LINC00346 regulates glycolysis by modulation of glucose transporter 1 in breast cancer cells. Mol Cell Probes. (2020) 54:101667. doi: 10.1016/j.mcp.2020.101667
81. Sang LJ, Ju HQ, Liu GP, Tian T, Ma GL, Lu YX, et al. LncRNA CamK-A Regulates Ca(2+)-Signaling-Mediated Tumor Microenvironment Remodeling. Mol Cell. (2018) 72:71–83.e7. doi: 10.1016/j.molcel.2018.08.014
82. Ellis BC, Graham LD, Molloy PL. CRNDE, a long non-coding RNA responsive to insulin/IGF signaling, regulates genes involved in central metabolism. Biochim Biophys Acta. (2014) 1843:372–86. doi: 10.1016/j.bbamcr.2013.10.016
83. Li Z, Li X, Wu S, Xue M, Chen W. Long non-coding RNA UCA1 promotes glycolysis by upregulating hexokinase 2 through the mTOR-STAT3/microRNA143 pathway. Cancer Sci. (2014) 105:951–5. doi: 10.1111/cas.2014.105.issue-8
84. Chen J, Yu Y, Li H, Hu Q, Chen X, He Y, et al. Long non-coding RNA PVT1 promotes tumor progression by regulating the miR-143/HK2 axis in gallbladder cancer. Mol Cancer. (2019) 18:33. doi: 10.1186/s12943-019-0947-9
85. Lin YH, Wu MH, Huang YH, Yeh CT, Cheng ML, Chi HC, et al. Taurine up-regulated gene 1 functions as a master regulator to coordinate glycolysis and metastasis in hepatocellular carcinoma. Hepatology. (2018) 67:188–203. doi: 10.1002/hep.29462
86. Li H, Li J, Jia S, Wu M, An J, Zheng Q, et al. miR675 upregulates long noncoding RNA H19 through activating EGR1 in human liver cancer. Oncotarget. (2015) 6:31958–84. doi: 10.18632/oncotarget.v6i31
87. Hua Q, Mi B, Xu F, Wen J, Zhao L, Liu J, et al. Hypoxia-induced lncRNA-AC020978 promotes proliferation and glycolytic metabolism of non-small cell lung cancer by regulating PKM2/HIF-1α axis. Theranostics. (2020) 10:4762–78. doi: 10.7150/thno.43839
88. Tang J, Yan T, Bao Y, Shen C, Yu C, Zhu X, et al. LncRNA GLCC1 promotes colorectal carcinogenesis and glucose metabolism by stabilizing c-Myc. Nat Commun. (2019) 10:3499. doi: 10.1038/s41467-019-11447-8
89. Xiao ZD, Han L, Lee H, Zhuang L, Zhang Y, Baddour J, et al. Energy stress-induced lncRNA FILNC1 represses c-Myc-mediated energy metabolism and inhibits renal tumor development. Nat Commun. (2017) 8:783. doi: 10.1038/s41467-017-00902-z
90. Yang X, Ye H, He M, Zhou X, Sun N, Guo W, et al. LncRNA PDIA3P interacts with c-Myc to regulate cell proliferation via induction of pentose phosphate pathway in multiple myeloma. Biochem Biophys Res Commun. (2018) 498:207–13. doi: 10.1016/j.bbrc.2018.02.211
91. Yang F, Zhang H, Mei Y, Wu M. Reciprocal regulation of HIF-1α and lincRNA-p21 modulates the Warburg effect. Mol Cell. (2014) 53:88–100. doi: 10.1016/j.molcel.2013.11.004
92. Kino T, Hurt DE, Ichijo T, Nader N, Chrousos GP. Noncoding RNA gas5 is a growth arrest- and starvation-associated repressor of the glucocorticoid receptor. Sci Signal. (2010) 3:ra8. doi: 10.1126/scisignal.2000568
93. Chang L, Xu W, Zhang Y, Gong F. Long non-coding RNA-NEF targets glucose transportation to inhibit the proliferation of non-small-cell lung cancer cells. Oncol Lett. (2019) 17:2795–801. doi: 10.3892/ol.2019.9919
94. Cui M, Xiao Z, Wang Y, Zheng M, Song T, Cai X, et al. Long noncoding RNA HULC modulates abnormal lipid metabolism in hepatoma cells through an miR-9-mediated RXRA signaling pathway. Cancer Res. (2015) 75:846–57. doi: 10.1158/0008-5472.CAN-14-1192
95. Liu X, Liang Y, Song R, Yang G, Han J, Lan Y, et al. Long non-coding RNA NEAT1-modulated abnormal lipolysis via ATGL drives hepatocellular carcinoma proliferation. Mol Cancer. (2018) 17:90. doi: 10.1186/s12943-018-0838-5
96. Sallam T, Jones M, Thomas BJ, Wu X, Gilliland T, Qian K, et al. Transcriptional regulation of macrophage cholesterol efflux and atherogenesis by a long noncoding RNA. Nat Med. (2018) 24:304–12. doi: 10.1038/nm.4479
97. Hu YW, Yang JY, Ma X, Chen ZP, Hu YR, Zhao JY, et al. A lincRNA-DYNLRB2-2/GPR119/GLP-1R/ABCA1-dependent signal transduction pathway is essential for the regulation of cholesterol homeostasis. J Lipid Res. (2014) 55:681–97. doi: 10.1194/jlr.M044669
98. Liu L, Cui S, Wan T, Li X, Tian W, Zhang R, et al. Long non-coding RNA HOTAIR acts as a competing endogenous RNA to promote glioma progression by sponging miR-126-5p. J Cell Physiol. (2018) 233:6822–31. doi: 10.1002/jcp.v233.9
99. Warburg O, Wind F, Negelein E. THE METABOLISM OF TUMORS IN THE BODY. J Gen Physiol. (1927) 8:519–30. doi: 10.1085/jgp.8.6.519
100. Fan C, Tang Y, Wang J, Xiong F, Guo C, Wang Y, et al. Role of long non-coding RNAs in glucose metabolism in cancer. Mol Cancer. (2017) 16:130. doi: 10.1186/s12943-017-0699-3
101. Wang Y, Wu Y, Wang Y, Fu A, Gong L, Li W, et al. Bacillus amyloliquefaciens SC06 alleviates the oxidative stress of IPEC-1 via modulating Nrf2/Keap1 signaling pathway and decreasing ROS production. Appl Microbiol Biotechnol. (2017) 101:3015–26. doi: 10.1007/s00253-016-8032-4
102. Wen L, Tan C, Ma S, Li X. lncRNAs: Key Regulators of Signaling Pathways in Tumor Glycolysis. Dis Markers. (2022) 2022. doi: 10.1155/2022/2267963
103. Xia M, Wang S, Wang L, Mei Y, Tu Y, Gao L. The role of lactate metabolism-related LncRNAs in the prognosis, mutation, and tumor microenvironment of papillary thyroid cancer. Front Endocrinol. (2023) 14. doi: 10.3389/fendo.2023.1062317
104. Vander Heiden MG, Cantley LC, Thompson CB. Understanding the Warburg effect: the metabolic requirements of cell proliferation. Science. (2009) 324:1029–33. doi: 10.1126/science.1160809
105. DeBerardinis RJ, Mancuso A, Daikhin E, Nissim I, Yudkoff M, Wehrli S, et al. Beyond aerobic glycolysis: transformed cells can engage in glutamine metabolism that exceeds the requirement for protein and nucleotide synthesis. Proc Natl Acad Sci U.S.A. (2007) 104:19345–50.
106. Ho KH, Huang TW, Shih CM, Lee YT, Liu AJ, Chen PH, et al. Glycolysis-associated lncRNAs identify a subgroup of cancer patients with poor prognoses and a high-infiltration immune microenvironment. BMC Med. (2021) 19:59. doi: 10.1186/s12916-021-01925-6
107. Xiao R, Yang M, Tan Y, Ding R, Li D. Identification of Five Immune-Related lncRNAs Predicting Survival and Tumor Microenvironment Characteristics in Breast Cancer. Comput Math Methods Med 2021. (2021) p:6676692. doi: 10.1155/2021/6676692
108. Zhang Y, Zhang L, Xu Y, Wu X, Zhou Y, Mo J. Immune-related long noncoding RNA signature for predicting survival and immune checkpoint blockade in hepatocellular carcinoma. J Cell Physiol. (2020) 235:9304–16. doi: 10.1002/jcp.v235.12
109. Bai Y, Lin H, Chen J, Wu Y, Yu S. Identification of Prognostic Glycolysis-Related lncRNA Signature in Tumor Immune Microenvironment of Hepatocellular Carcinoma. Front Mol Biosci. (2021) 8:645084. doi: 10.3389/fmolb.2021.645084
110. Shi X, Sun M, Liu H, Yao Y, Song Y. Long non-coding RNAs: a new frontier in the study of human diseases. Cancer Lett. (2013) 339:159–66. doi: 10.1016/j.canlet.2013.06.013
111. Rinn JL, Kertesz M, Wang JK, Squazzo SL, Xu X, Brugmann SA, et al. Functional demarcation of active and silent chromatin domains in human HOX loci by noncoding RNAs. Cell. (2007) 129:1311–23. doi: 10.1016/j.cell.2007.05.022
112. Akram M. Mini-review on glycolysis and cancer. J Cancer Educ. (2013) 28:454–7. doi: 10.1007/s13187-013-0486-9
113. Fan L, Huang C, Li J, Gao T, Lin Z, Yao T. Long non−coding RNA urothelial cancer associated 1 regulates radioresistance via the hexokinase 2/glycolytic pathway in cervical cancer. Int J Mol Med. (2018) 42:2247–59. doi: 10.3892/ijmm.2018.3778
114. Robey RB, Hay N. Mitochondrial hexokinases, novel mediators of the antiapoptotic effects of growth factors and Akt. Oncogene. (2006) 25:4683–96. doi: 10.1038/sj.onc.1209595
115. Song J, Wu X, Liu F, Li M, Sun Y, Wang Y, et al. Long non-coding RNA PVT1 promotes glycolysis and tumor progression by regulating miR-497/HK2 axis in osteosarcoma. Biochem Biophys Res Commun. (2017) 490:217–24. doi: 10.1016/j.bbrc.2017.06.024
116. Liu HE, Shi HH, Luo XJ. Upregulated Long Noncoding RNA UCA1 Enhances Warburg Effect via miR-203/HK2 Axis in Esophagal Cancer. J Oncol 2020. (2020) p:8847687. doi: 10.1155/2020/8847687
117. Sun L, Li J, Yan W, Yao Z, Wang R, Zhou X, et al. H19 promotes aerobic glycolysis, proliferation, and immune escape of gastric cancer cells through the microRNA-519d-3p/lactate dehydrogenase A axis. Cancer Sci. (2021) 112:2245–59. doi: 10.1111/cas.v112.6
118. Ranganathan P, Shanmugam A, Swafford D, Suryawanshi A, Bhattacharjee P, Hussein MS, et al. GPR81, a Cell-Surface Receptor for Lactate, Regulates Intestinal Homeostasis and Protects Mice from Experimental Colitis. J Immunol. (2018) 200:1781–9. doi: 10.4049/jimmunol.1700604
119. Hoque R, Farooq A, Ghani A, Gorelick F, Mehal WZ. Lactate reduces liver and pancreatic injury in Toll-like receptor- and inflammasome-mediated inflammation via GPR81-mediated suppression of innate immunity. Gastroenterology. (2014) 146:1763–74. doi: 10.1053/j.gastro.2014.03.014
120. García-Cañaveras JC, Chen L, Rabinowitz JD. The Tumor Metabolic Microenvironment: Lessons from Lactate. Cancer Res. (2019) 79:3155–62. doi: 10.1158/0008-5472.CAN-18-3726
121. Goetze K, Walenta S, Ksiazkiewicz M, Kunz-Schughart LA, Mueller-Klieser W. Lactate enhances motility of tumor cells and inhibits monocyte migration and cytokine release. Int J Oncol. (2011) 39:453–63. doi: 10.3892/ijo.2011.1055
122. Chen F, Chen J, Yang L, Liu J, Zhang X, Zhang Y, et al. Extracellular vesicle-packaged HIF-1α-stabilizing lncRNA from tumour-associated macrophages regulates aerobic glycolysis of breast cancer cells. Nat Cell Biol. (2019) 21:498–510. doi: 10.1038/s41556-019-0299-0
123. Stern R, Shuster S, Neudecker BA, Formby B. Lactate stimulates fibroblast expression of hyaluronan and CD44: the Warburg effect revisited. Exp Cell Res. (2002) 276:24–31. doi: 10.1006/excr.2002.5508
124. Comito G, Iscaro A, Bacci M, Morandi A, Ippolito L, Parri M, et al. Lactate modulates CD4(+) T-cell polarization and induces an immunosuppressive environment, which sustains prostate carcinoma progression via TLR8/miR21 axis. Oncogene. (2019) 38:3681–95. doi: 10.1038/s41388-019-0688-7
125. Li Z, Zhang H. Reprogramming of glucose, fatty acid and amino acid metabolism for cancer progression. Cell Mol Life Sci. (2016) 73:377–92. doi: 10.1007/s00018-015-2070-4
126. Gerriets VA, Rathmell JC. Metabolic pathways in T cell fate and function. Trends Immunol. (2012) 33:168–73. doi: 10.1016/j.it.2012.01.010
127. Berod L, Friedrich C, Nandan A, Freitag J, Hagemann S, Harmrolfs K, et al. De novo fatty acid synthesis controls the fate between regulatory T and T helper 17 cells. Nat Med. (2014) 20:1327–33. doi: 10.1038/nm.3704
128. Chowdhury PS, Chamoto K, Kumar A, Honjo T. PPAR-Induced Fatty Acid Oxidation in T Cells Increases the Number of Tumor-Reactive CD8(+) T Cells and Facilitates Anti-PD-1 Therapy. Cancer Immunol Res. (2018) 6:1375–87. doi: 10.1158/2326-6066.CIR-18-0095
129. Zeng Y, Ren K, Zhu X, Zheng Z, Yi G. Long Noncoding RNAs: Advances in Lipid Metabolism. Adv Clin Chem. (2018) 87:1–36. doi: 10.1016/bs.acc.2018.07.001
130. Chen E, Yi J, Jiang J, Zou Z, Mo Y, Ren Q, et al. Identification and validation of a fatty acid metabolism-related lncRNA signature as a predictor for prognosis and immunotherapy in patients with liver cancer. BMC Cancer. (2022) 22(1). doi: 10.1186/s12885-022-10122-4
131. Li Y, Lei C, Xie Y, Zhang J, Wang N, He W, et al. Differential expression profile of mRNAs, lncRNAs, and circRNAs reveals potential molecular mechanism in breast cancer. Bioscience Rep. (2022) 42(7). doi: 10.1042/BSR20220645
132. Herber DL, Cao W, Nefedova Y, Novitskiy SV, Nagaraj S, Tyurin VA, et al. Lipid accumulation and dendritic cell dysfunction in cancer. Nat Med. (2010) 16:880–6. doi: 10.1038/nm.2172
133. Michelet X, Dyck L, Hogan A, Loftus RM, Duquette D, Wei K, et al. Metabolic reprogramming of natural killer cells in obesity limits antitumor responses. Nat Immunol. (2018) 19:1330–40. doi: 10.1038/s41590-018-0251-7
134. Zhang P, Cao L, Zhou R, Yang X, Wu M. The lncRNA Neat1 promotes activation of inflammasomes in macrophages. Nat Commun. (2019) 10:1495. doi: 10.1038/s41467-019-09482-6
135. Gao Y, Fang P, Li WJ, Zhang J, Wang GP, Jiang DF, et al. LncRNA NEAT1 sponges miR-214 to regulate M2 macrophage polarization by regulation of B7-H3 in multiple myeloma. Mol Immunol. (2020) 117:20–8. doi: 10.1016/j.molimm.2019.10.026
136. Huang B, Song BL, Xu C. Cholesterol metabolism in cancer: mechanisms and therapeutic opportunities. Nat Metab. (2020) 2:132–41. doi: 10.1038/s42255-020-0174-0
137. Ma X, Xiao L, Liu L, Ye L, Su P, Bi E, et al. CD36-mediated ferroptosis dampens intratumoral CD8(+) T cell effector function and impairs their antitumor ability. Cell Metab. (2021) 33:1001–1012.e5. doi: 10.1016/j.cmet.2021.02.015
138. Eibinger G, Fauler G, Bernhart E, Frank S, Hammer A, Wintersperger A, et al. On the role of 25-hydroxycholesterol synthesis by glioblastoma cell lines. Implications for chemotactic monocyte recruitment. Exp Cell Res. (2013) 319:1828–38. doi: 10.1016/j.yexcr.2013.03.025
139. Baek AE, Yu YA, He S, Wardell SE, Chang CY, Kwon S, et al. The cholesterol metabolite 27 hydroxycholesterol facilitates breast cancer metastasis through its actions on immune cells. Nat Commun. (2017) 8:864. doi: 10.1038/s41467-017-00910-z
140. Zou Y, Guo S, Liao Y, Chen W, Chen Z, Chen J, et al. Ceramide metabolism-related prognostic signature and immunosuppressive function of ST3GAL1 in osteosarcoma. Transl Oncol. (2024) 40:101840. doi: 10.1016/j.tranon.2023.101840
141. Hu J, Shan Y, Ma J, Pan Y, Zhou H, Jiang L, et al. LncRNA ST3Gal6-AS1/ST3Gal6 axis mediates colorectal cancer progression by regulating α-2,3 sialylation via PI3K/Akt signaling. Int J Cancer. (2019) 145:450–60. doi: 10.1002/ijc.v145.2
142. Zhao SY, Wang Z, Wu XB, Zhang S, Chen Q, Wang DD, et al. CERS6-AS1 contributes to the malignant phenotypes of colorectal cancer cells by interacting with miR-15b-5p to regulate SPTBN2. Kaohsiung J Med Sci. (2022) 38:403–14. doi: 10.1002/kjm2.12503
143. Gao KF, Zhao YF, Liao WJ, Xu GL, Zhang JD. CERS6-AS1 promotes cell proliferation and represses cell apoptosis in pancreatic cancer via miR-195-5p/WIPI2 axis. Kaohsiung J Med Sci. (2022) 38:542–53. doi: 10.1002/kjm2.12522
144. Pan W, Chen KJ, Huang YC. Ceramide synthase 6 antisense RNA 1 contributes to the progression of breast cancer by sponging miR-16-5p to upregulate ubiquitin-conjugating enzyme E2C. Anticancer Drugs. (2022) 33:913–22. doi: 10.1097/CAD.0000000000001381
145. Yan K, Hu C, Liu C, Chu G, Wang X, Ma S, et al. LncRNA CERS6-AS1, sponging miR-6838-5p, promotes proliferation and invasion in cervical carcinoma cells by upregulating FOXP2. Histol Histopathol. (2023) 38:823–35.
146. Kristensen LS, Jakobsen T, Hager H, Kjems J. The emerging roles of circRNAs in cancer and oncology. Nat Rev Clin Oncol. (2022) 19:188–206. doi: 10.1038/s41571-021-00585-y
147. Lin W, Zhou Q, Wang C-Q, Zhu L, Bi C, Zhang S, et al. LncRNAs regulate metabolism in cancer. Int J Biol Sci. (2020) 16:1194–206. doi: 10.7150/ijbs.40769
148. Li T, Xian H-C, Dai L, Tang Y-L, Liang X-H. Tip of the Iceberg: Roles of CircRNAs in Cancer Glycolysis. OncoTargets Ther. (2021) 14:2379–95. doi: 10.2147/OTT.S297140
149. Lv Y, Lv Y, Wang Z, Yuan K, Zeng Y. Noncoding RNAs as sensors of tumor microenvironmental stress. J Exp Clin Cancer Res. (2022) 41(1). doi: 10.1186/s13046-022-02433-y
150. Xu W, Li H, Wang Z, Kang Y, Zheng L, Liu Y, et al. LINC00152: Potential driver oncogene in pan-cancer. Wiley Interdiscip Reviews-Rna. (2024) 15(3). doi: 10.1002/wrna.v15.3
151. Zhou B, Wu Y, Cheng P, Wu C. Long noncoding RNAs with peptide-encoding potential identified in esophageal squamous cell carcinoma: KDM4A-AS1-encoded peptide weakens cancer cell viability and migratory capacity. Mol Oncol. (2023) 17:1419–36. doi: 10.1002/1878-0261.13424
152. Fujisaka Y, Iwata T, Tamai K, Nakamura M, Mochizuki M, Shibuya R, et al. Long non-coding RNA HOTAIR up-regulates chemokine (C-C motif) ligand 2 and promotes proliferation of macrophages and myeloid-derived suppressor cells in hepatocellular carcinoma cell lines. Oncol Lett. (2018) 15:509–14.
153. Fu Q, Xu L, Wang Y, Jiang Q, Liu Z, Zhang J, et al. Tumor-associated Macrophage-derived Interleukin-23 Interlinks Kidney Cancer Glutamine Addiction with Immune Evasion. Eur Urol. (2019) 75:752–63. doi: 10.1016/j.eururo.2018.09.030
154. Guo K, Qi D, Huang B. LncRNA MEG8 promotes NSCLC progression by modulating the miR-15a-5p-miR-15b-5p/PSAT1 axis. Cancer Cell Int. (2021) 21(1). doi: 10.1186/s12935-021-01772-8
155. Lee GY, Haverty PM, Li L, Kljavin NM, Bourgon R, Lee J, et al. Comparative Oncogenomics Identifies PSMB4 and SHMT2 as Potential Cancer Driver Genes. Cancer Res. (2014) 74:3114–26. doi: 10.1158/0008-5472.CAN-13-2683
156. Liu X, Shen Z. LncRNA TMPO-AS1 Aggravates the Development of Hepatocellular Carcinoma via miR-429/GOT1 Axis. Am J Med Sci. (2020) 360:711–20. doi: 10.1016/j.amjms.2020.08.010
157. Tamblin-Hopper P, Kiss-Toth E, Sudbery I, Young D, Wilkinson JM. The potential therapeutic applications of long non-coding RNAs. J Trans Genet Genomics. (2024) 8:225–43. doi: 10.20517/jtgg.2024.12
158. Boon RA, Jae N, Holdt L, Dimmeler S. Long Noncoding RNAs From Clinical Genetics to Therapeutic Targets? J Am Coll Cardiol. (2016) 67:1214–26.
159. Clark MB, Johnston RL, Inostroza-Ponta M, Fox AH, Fortini E, Moscato P, et al. Genome-wide analysis of long noncoding RNA stability. Genome Res. (2012) 22:885–98. doi: 10.1101/gr.131037.111
160. Ponting CP, Haerty W. Genome-Wide Analysis of Human Long Noncoding RNAs: A Provocative Review. Annu Rev Genomics Hum Genet. (2022) 23:153–72. doi: 10.1146/annurev-genom-112921-123710
161. Mai J, Lu M, Gao Q, Zeng J, Xiao J. Transcriptome-wide association studies: recent advances in methods, applications and available databases. Commun Biol. (2023) 6(1). doi: 10.1038/s42003-023-05279-y
162. Washietl S, Kellis M, Garber M. Evolutionary dynamics and tissue specificity of human long noncoding RNAs in six mammals. Genome Res. (2014) 24:616–28. doi: 10.1101/gr.165035.113
Keywords: lncRNAs, metabolic reprogramming, tumor microenvironment remodeling, tumor immunity, tumor immunotherapy
Citation: Jiao J, Zhao Y, Li Q, Jin S and Liu Z (2024) LncRNAs in tumor metabolic reprogramming and tumor microenvironment remodeling. Front. Immunol. 15:1467151. doi: 10.3389/fimmu.2024.1467151
Received: 19 July 2024; Accepted: 07 October 2024;
Published: 30 October 2024.
Edited by:
Siming Li, Peking University, ChinaReviewed by:
Zhenhua Chen, City of Hope, United StatesGang Xiao, Zhejiang University, China
Sweta Ghosh, University of Louisville, United States
Copyright © 2024 Jiao, Zhao, Li, Jin and Liu. This is an open-access article distributed under the terms of the Creative Commons Attribution License (CC BY). The use, distribution or reproduction in other forums is permitted, provided the original author(s) and the copyright owner(s) are credited and that the original publication in this journal is cited, in accordance with accepted academic practice. No use, distribution or reproduction is permitted which does not comply with these terms.
*Correspondence: Zhongshan Liu, bGl1emhvbmdzaGFuQGpsdS5lZHUuY24=