- Department of Hand Surgery, Honghui Hospital, Xi’an Jiaotong University, Xi’an, Shaanxi, China
Immunoproteasome is a specialized form of proteasome which plays a crucial role in antigen processing and presentation, and enhances immune responses against malignant cells. This review explores the role of immunoproteasome in the anti-tumor immune responses, including immune surveillance and modulation of the tumor microenvironment, as well as its potential as a target for cancer immunotherapy. Furthermore, we have also discussed the therapeutic potential of immunoproteasome inhibitors, strategies to enhance antigen presentation and combination therapies. The ongoing trials and case studies in urology, melanoma, lung, colorectal, and breast cancers have also been summarized. Finally, the challenges facing clinical translation of immunoproteasome-targeted therapies, such as toxicity and resistance mechanisms, and the future research directions have been addressed. This review underscores the significance of targeting the immunoproteasome in combination with other immunotherapies for solid tumors and its potential broader applications in other diseases.
1 Introduction
The immunoproteasome is a variant of the standard proteasome with distinct catalytic subunits that degrade ubiquitinylated proteins (1, 2). It is activated in both immune and non-immune cells in response to inflammatory cytokines, especially interferon-gamma (IFN-γ), and oxidative stress (3, 4). Nevertheless, the primary function of the immunoproteasome is to cleave intracellular viral or oncogenic proteins into peptides, which are then displayed by the major histocompatibility complex (MHC) class I molecules for CD8+ T cells (1, 5). The immunoproteasome differs from the standard proteasome in terms of both enzymatic activity and subunit composition (6, 7). The β1, β2, and β5 catalytic subunits of the standard proteasome (8, 9) are respectively substituted with β1i (LMP2), β2i (MECL-1), and β5i (LMP7) in the immunoproteasome (1, 10) (Figure 1). These substitutions alter the proteolytic activity of the immunoproteasome, allowing efficient production of peptides with hydrophobic or basic C-terminal residues, which are preferentially bound by the MHC class I molecules for antigen presentation (2, 11, 12). Thus, immunoproteasomes boosts the ability of the immune system to recognize and respond to intracellular infections and tumor cells by increasing the efficacy and specificity of peptide generation.
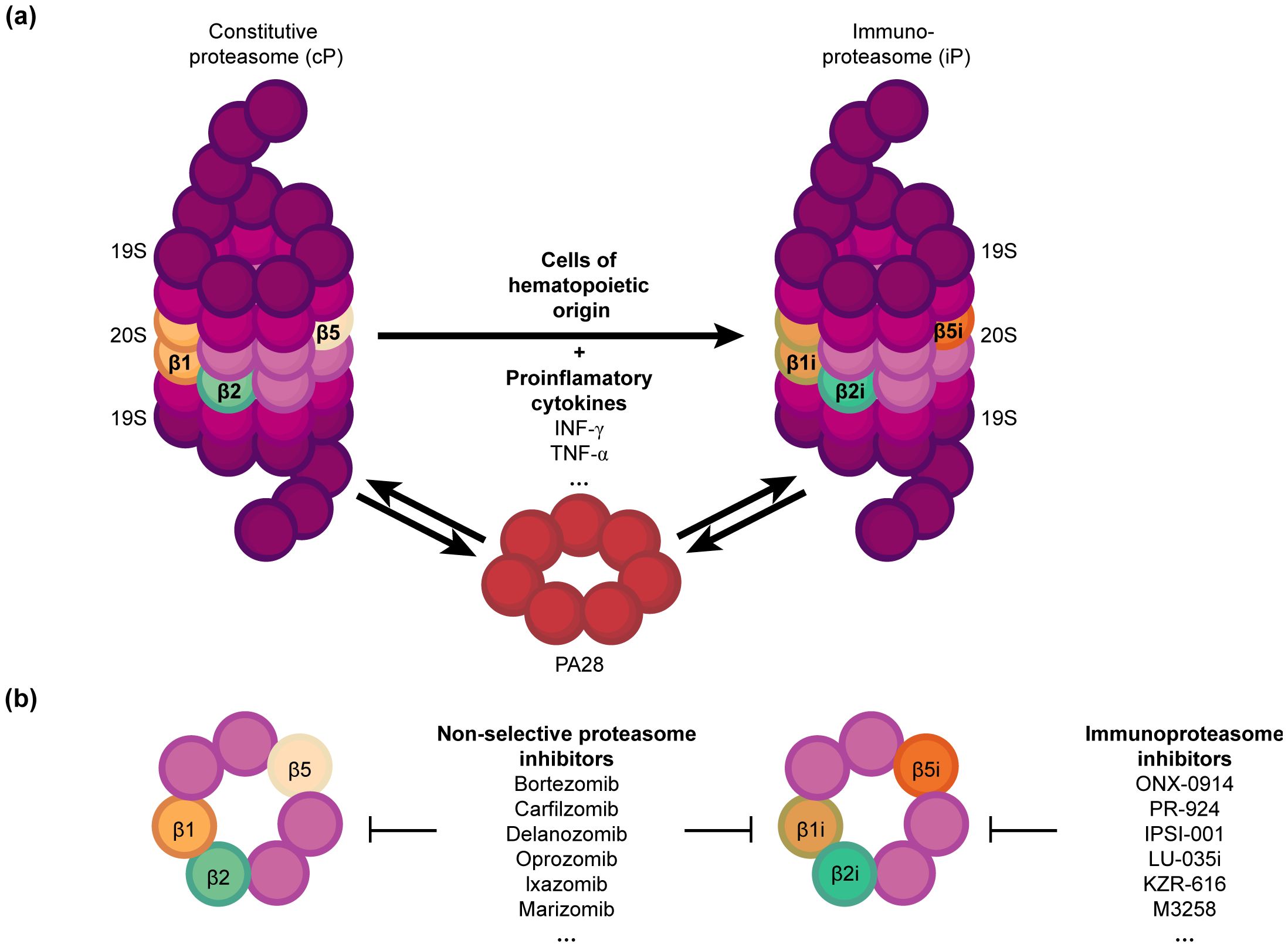
Figure 1. Structure and function of proteasomes (A) Constitutive proteasome (cP) vs. Immunoproteasome (iP): The cP includes the subunits β1, β2 and β5, which are respectively substituted with β1i, β2i, and β5i in the iP upon stimulation by pro-inflammatory cytokines such as IFN-γ. The iP is naturally present in hematopoietic cells. IFN-γ also upregulates the regulatory particle PA28, which can bind to both the cP and iP with equal affinity and enhance their activity. (B) Proteasome inhibitors can selectively target iP, or both cP and iP, offering potential immunomodulatory and therapeutic strategies against cancer.
Solid tumors are abnormal masses of tissue that can be benign (non-cancerous) or malignant (cancerous) (13, 14). Depending on the tissue of origin, solid tumors are broadly classified as carcinomas that originate from epithelial cells (e.g., breast, lung, colorectal cancers), and sarcomas that arise from connective tissues (e.g., bones, muscles). Other specific types include gliomas (brain) and hepatomas (liver) (15–17). Solid tumors are often detected in the advanced stages, which can render the conventional therapies, such as radiation therapy, chemotherapy and surgery, less effective (18–20). In addition, solid tumors frequently develop resistance to these therapies, leading to recurrence and metastasis. The presence of immune cells, blood vessels, and the extracellular matrix in the tumor microenvironment (TME) significantly influences tumor progression and treatment resistance. The heterogeneity of solid tumors further complicates treatment, as different regions of the same tumor can respond differently to the same therapeutic modality. These challenges underscore the need for novel approaches, including immunotherapy.
Immunotherapy harnesses the host immune system to recognize and eliminate cancer cells. For instance, checkpoint inhibitors can augment the anti-tumor immune response by reversing the inhibitory signals on the effector T cells. Likewise, immune cells engineered to effectively recognize and eliminate tumor cells (i.e., adoptive cell transfer) and cytokines have also been shown to enhance immune-based clearance of the malignant cells. Furthermore, cancer vaccines can stimulate the immune system to target specific tumor antigens (21–25). However, tumor cells have developed adaptive mechanisms to escape immune detection, such as decreasing antigen presentation or inducing an immunosuppressive TME. Immunotherapeutic strategies can overcome these challenges by promoting the recognition of tumor-specific antigens. In addition, immunotherapies also offer the potential for long-lasting protection against cancer by establishing immunological memory, as well as a viable alternative for tumors that are resistant to conventional therapy. The therapeutic potential of targeting immune-related pathways has been highlighted by the success of checkpoint inhibitors against melanoma and lung cancer among other malignancies (26–28).
In this review, we have explored the role of immunoproteasome in anti-tumor immune responses and its potential as a therapeutic target for cancer. We have also discussed the current research, clinical applications, and the challenges associated with targeting the immunoproteasome in solid tumors, and identified areas for future research and clinical development.
2 Structure and function of immunoproteasomes
The immunoproteasome differs from the standard proteasome on account of three inducible catalytic subunits. The β1i subunit, also referred to as low molecular weight protein 2 (LMP2), is encoded by a gene located within the MHC class II locus, which emphasizes its significant role in immune function (29, 30). Unlike the β1 subunit, LMP2 primarily generates peptides with hydrophobic C-termini, which are preferred by MHC class I molecules. LMP2 is upregulated by inflammatory cytokines, which ensures that the immunoproteasome is optimized for processing antigens during immune responses (31, 32). The β2 subunit of the standard proteasome is replaced with the β2i subunit, also referred to as multi-catalytic endopeptidase complex-like 1 (MECL-1) (10, 33). MECL-1 enhances cleavage of substrates after basic residues, which generates a broader range of antigenic peptides suitable for MHC class I presentation. The induction of MECL-1 by pro-inflammatory cytokines is an essential step for the activation of CD8+ T cells and the ensuing adaptive immune response (34, 35). Furthermore, the presence of MECL-1 is essential for maintaining the unique substrate specificity of immunoproteasomes. The β5i subunit, also known as LMP7, is the third inducible catalytic subunit of the immunoproteasome, and promotes the generation of hydrophobic peptides or those with basic C-terminal residues. The gene encoding LMP7 is also located in the MHC region, which underscores its role in immune function. It is induced by IFN-γ and other pro-inflammatory cytokines, and is necessary for the effective processing of tumor and viral antigens (36, 37).
IFN-γ is a key effector cytokine involved in the immune responses against viral infections and tumors. Exposure to IFN-γ leads to upregulation of immunoproteasome subunits in the immune cells (38). The tumor necrosis factor-alpha (TNF-α) also contributes to the immunoproteasome induction (39, 40). Both cytokines facilitate the assembly of the β1i, β2i, and β5i subunits into the immunoproteasome during immune responses. Various chaperones are involved in this process to ensure correct incorporation and folding of the subunits (41–43). This regulated assembly ensures that the immunoproteasome is formed efficiently during times of immune activation, providing a tailored response to pathogenic challenges (44–46).
The transporter associated with antigen processing (TAP) cleaves proteins into smaller peptides during protein degradation, which are then transported into the endoplasmic reticulum (47, 48). Within the endoplasmic reticulum, these peptides are loaded onto MHC class I molecules. When MHC class I molecules are present on the surface of malignant or infected cells, CD8+ T cells are able to recognize and respond to these antigens (49, 50).
3 Immunoproteasome in tumor immunology
3.1 Recognition and elimination of cancer cells
Immune cells have the ability to recognize and eliminate cells that express abnormal or cancer-specific antigens, a phenomenon known as immunosurveillance. The immunoproteasome is pivotal to this process, as it generates a wide repertoire of antigenic peptides that are more likely to be recognized by the effector immune cells. The presentation of these peptides by the MHC class I molecules is crucial for the activation of anti-tumor CD8+ cytotoxic T lymphocytes (CTLs) (11, 51). Consequently, the immunoproteasome plays a critical role in the prevention and control of cancer progression by assisting the immune system in detecting and eliminating nascent tumor cells.
Solid tumors have developed several mechanisms to avoid immune detection and clearance, even in the face of effective immune surveillance. For instance, tumor cells can downregulate the expression of MHC class I molecules, which reduces antigen presentation and subsequent recognition by CTLs. Additionally, tumors can create an immunosuppressive microenvironment by secreting factors such as TGF-β, IL-10, and VEGF (52). Likewise, immune checkpoint molecules like PD-L1 that cause T cell exhaustion by triggering inhibitory signals are also typically upregulated on the tumor cells. Finally, mutations or alterations in the immunoproteasome subunits can lower generation of antigenic peptides, and contribute to immune evasion.
3.2 Role of immunoproteasome in modulating the microenvironment
Apart from ensuring effective presentation of tumor-associated antigens (TAAs) on the MHC class I molecules and thus promoting activation of CTLs (53, 54), the immunoproteasome also maintains the balance between immune activation and tolerance, and keeps the immunosuppressive elements of the TME in check (35, 52). It can either promote or inhibit the anti-tumor immune response by modulating the activity of various immune cells and the production of cytokines.
Tumor-infiltrating lymphocytes (TILs) are a subset of immune cells that penetrate the tumor stroma, and are indicative of the immune response against malignant cells. Enhanced antigen processing by the immunoproteasome can boost the responsiveness and cytotoxic function of the TILs against tumor cells (55, 56), which is vital for sustained anti-tumor immune response within the TME. However, tumors often employ immunosuppressive strategies that can hinder TIL function, such as upregulation of checkpoint molecules and the secretion of immunosuppressive cytokines. Targeting the immunoproteasome within the TME can potentially counteract these strategies, and enhance the infiltration, activation, and cytotoxic function of TILs (57, 58).
3.3 Immunoproteasome activity in antigen-presenting cells
Antigen-presenting cells (APCs), including macrophages and dendritic cells (59, 60), process and present antigens to T cells, thereby initiating and modulating immune responses. The immunoproteasome enhances the production of high-quality antigenic peptides, which results in a wide and robust array of tumor-associated antigens being displayed on the surface of APCs. This, in turn, leads to a more effective activation and expansion of CTLs, promoting a stronger and more targeted antitumor immune response. Additionally, by affecting cytokine production and lowering immunosuppressive factors, the immunoproteasome can modify the tumor microenvironment and improve the immune response against tumors as a whole.
4 Immunoproteasome as a therapeutic target
4.1 Enhancing antigen presentation
Enhancing immunoproteasome activity through cytokines, small molecules, or genetic modifications (Figure 2) can boost the ability of the immune system to recognize and destroy cancer cells (61). For instance, administration of IFN-γ and TNF-α, which are potent inducers of immunoproteasome subunits, have been shown to increase the expression and activity of the immunoproteasome (62). Furthermore, small molecules that mimic the effects of these cytokines or directly activate signaling pathways involved in immunoproteasome regulation are also under investigation. Finally, genetic engineering methods like CRISPR-Cas9 have been used to increase the expression of immunoproteasome subunits in tumor or immune cells, and enhance antigen processing and presentation (63–65).
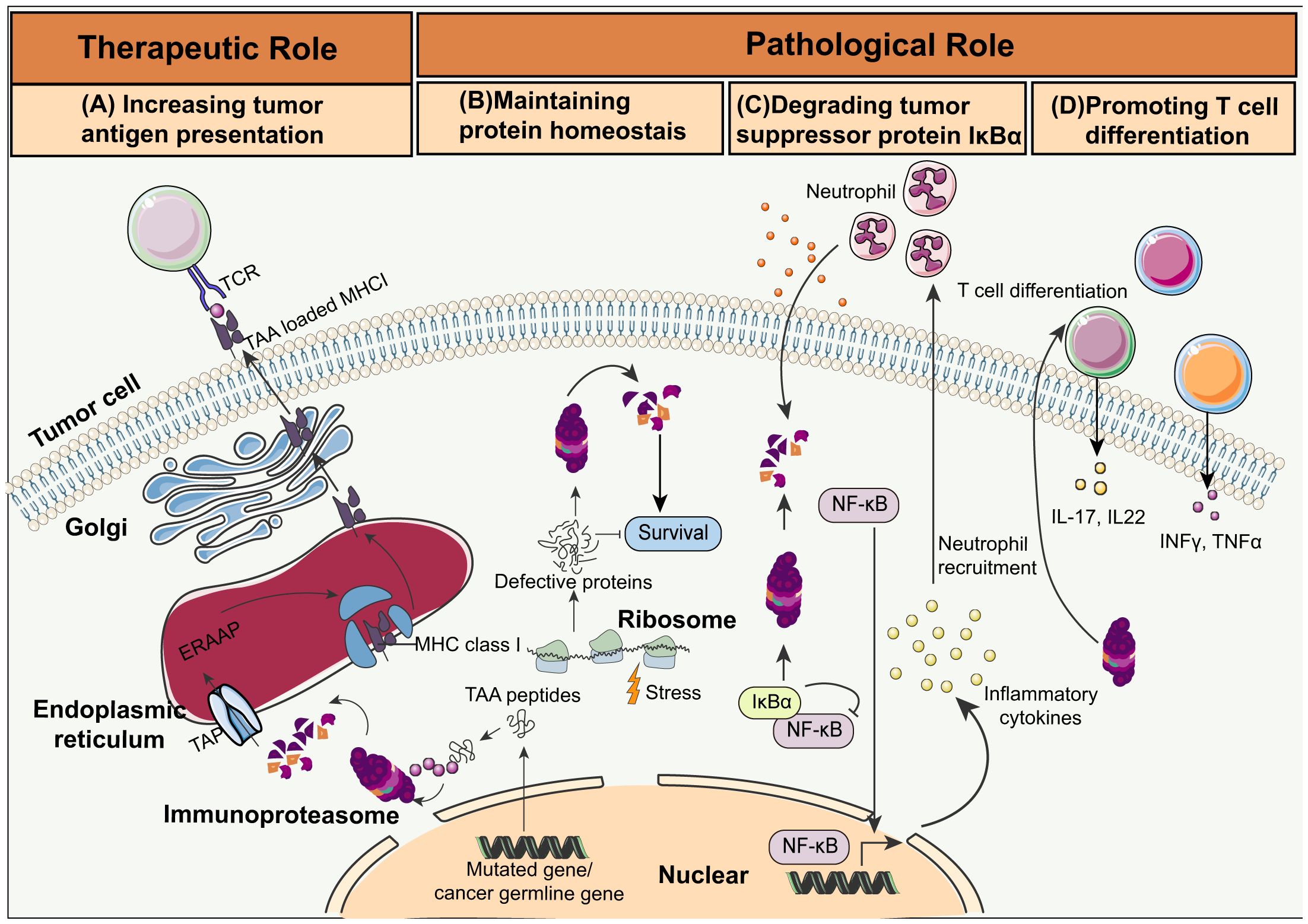
Figure 2. Schematic overview of immunoproteasome activities in cancer. (A) Increasing tumor antigen presentation: Immunoproteasomes degrade tumor-associated antigen (TAA) peptides encoded by mutated or cancer germline genes. The transporter associated with antigen processing (TAP) then delivers these peptides into the endoplasmic reticulum (ER). The TAA peptides are trimmed by the ER-associated aminopeptidase (ERAAP), and loaded on MHC I molecules by a complex of tapasin, calreticulin, and ERP57. The MHC I complex loaded with the TAA peptides migrate to the cell surface for presentation to the CTLs. (B) Maintaining protein homeostasis: Immunoproteasomes maintain protein homeostasis and protect cells from proteotoxic stress by degrading non-functional and misfolded proteins. (C) Degrading tumor suppressor protein IkBa: Immunoproteasome-mediated degradation of IkBa leads to NF-kB activation and cytokine secretion, which in turn recruits neutrophils and initiates colitis-associated cancers (CAC). (D) Promoting T cell differentiation: Immunoproteasomes trigger the differentiation of the pro-inflammatory Th1 and Th17 cells, resulting in increased production of IL-17, IL-22, TNF, and IFN-γ.
4.2 Combination therapies
The combination of immunoproteasome inhibitors with immune checkpoint blockers represents a synergistic approach to cancer treatment. Antibodies targeting checkpoint molecules like PD-1, PD-L1, and CTLA-4 have been shown to enhance T cell-mediated elimination of cancer cells. However, the therapeutic efficacy of checkpoint inhibitors can be limited by the immunosuppressive TME and insufficient antigen presentation. Selective inhibition of the immunoproteasome can relieve the immunosuppression in some solid tumors by enhancing antigen presentation. Preclinical studies using animal models have shown that combining immunoproteasome inhibitors and checkpoint blockers prolonged the survival of tumor-bearing animals through enhanced anti-tumor activity. Furthermore, clinical trials are currently exploring this combination strategy in various cancers to evaluate its safety and therapeutic potential.
Immunoproteasome inhibitors can also augment the efficacy of adoptive cell therapies, such as the CAR-T cell therapy or TCR-engineered T cell therapy, by increasing the availability of target antigens within the TME. Similarly, cancer vaccines, which aim to elicit a strong immune response against tumor-specific antigens, can benefit from immunoproteasome inhibitors due to more effective presentation of vaccine-derived peptides. The activation and proliferation of effector T cells can also be increased by combining immunoproteasome inhibitors and cytokines like IL-2 or IL-15, resulting in a potent anti-tumor response.
4.3 Case studies of specific solid tumors
Bladder cancer is difficult to treat due to its recurrence and resistance to conventional therapies. Cathro et al. showed that immunoproteasome inhibitors synergistically improved the therapeutic effects of checkpoint blockade in an animal model of bladder carcinoma by increasing antigen presentation and infiltration of CTLs (66). Furthermore, several clinical trials are investigating the impact of combining immunoproteasome inhibitors with standard treatments, such as Bacillus Calmette-Guerin (BCG) therapy, on the overall immune response and recurrence rates in bladder cancer patients (67–69).
Bone cancers, including osteosarcoma and Ewing sarcoma, are aggressive malignancies that primarily affect children and young adults, and are difficult to treat due to their ability to evade the immune system. Niewerth et al. showed that immunoproteasome inhibitors sensitized bone cancer cells to immune-mediated destruction in a preclinical model by increasing the repertoire of presented antigens and promoting CTL infiltration. In addition to the direct destruction of tumor cells, this strategy also induced a pro-inflammatory TME with sustained immune surveillance. Current research efforts are focused on combining immunoproteasome-targeted therapies with other immunotherapies, such as CAR-T cell therapy, to achieve more favorable outcomes for bone cancer patients (70).
The immunoproteasome is also a promising therapeutic target for colorectal cancer (CRC). Studies have shown that upregulating immunoproteasome activity in CRC cells can improve antigen presentation and enhance the efficacy of immunotherapies, such as checkpoint blockade (11). Additionally, immunoproteasome inhibitors have been shown to reduce the immunosuppressive environment of CRC tumors by modulating cytokine production and inhibiting the function of regulatory T cells (Tregs) and myeloid-derived suppressor cells (MDSCs) (64, 71). At present, clinical trials are being conducted to assess the safety and efficacy of combining immunoproteasome-targeted therapies with chemotherapy and immunotherapy (72).
Due to its aggressive nature and the absence of targeted therapies, breast cancer, particularly triple-negative breast cancer (TNBC), presents significant treatment challenges. The immunoproteasome is a promising therapeutic target in breast cancer (73, 74). Studies show that immunoproteasome activity is crucial for the effective presentation of breast cancer-associated antigens and the activation of CTLs (75). Furthermore, immunoproteasome inhibitors have been shown to enhance antigen presentation and promote immune cell infiltration in preclinical models of breast cancer. These findings suggest that targeting the immunoproteasome could be an effective strategy to improve the efficacy of existing immunotherapies, such as checkpoint inhibitors, in breast cancer patients. Furthermore, in order to enhance the overall anti-tumor response and raise survival rates for patients with breast cancer, clinical trials are investigating the combination of immunoproteasome-targeted therapies with traditional treatments like radiation therapy and chemotherapy.
Hepatocellular carcinoma (HCC) is one of the main causes of cancer-related death worldwide. Studies in preclinical models have shown that immuneproteasome inhibitors can increase the presentation of antigenic peptides and foster a pro-inflammatory TME, which can improve the effectiveness of immune checkpoint inhibitors, leading to a more effective activation of CTLs against liver cancer cells (76, 77). This combination approach has reduced tumor growth and improved survival rates in liver cancer models. Furthermore, immunoproteasome-targeted therapies in combination with other treatments, such as transarterial chemoembolization (TACE) and radiofrequency ablation (RFA), are also being investigated to enhance the overall anti-tumor response and improve clinical outcomes in liver cancer patients (Figure 3).
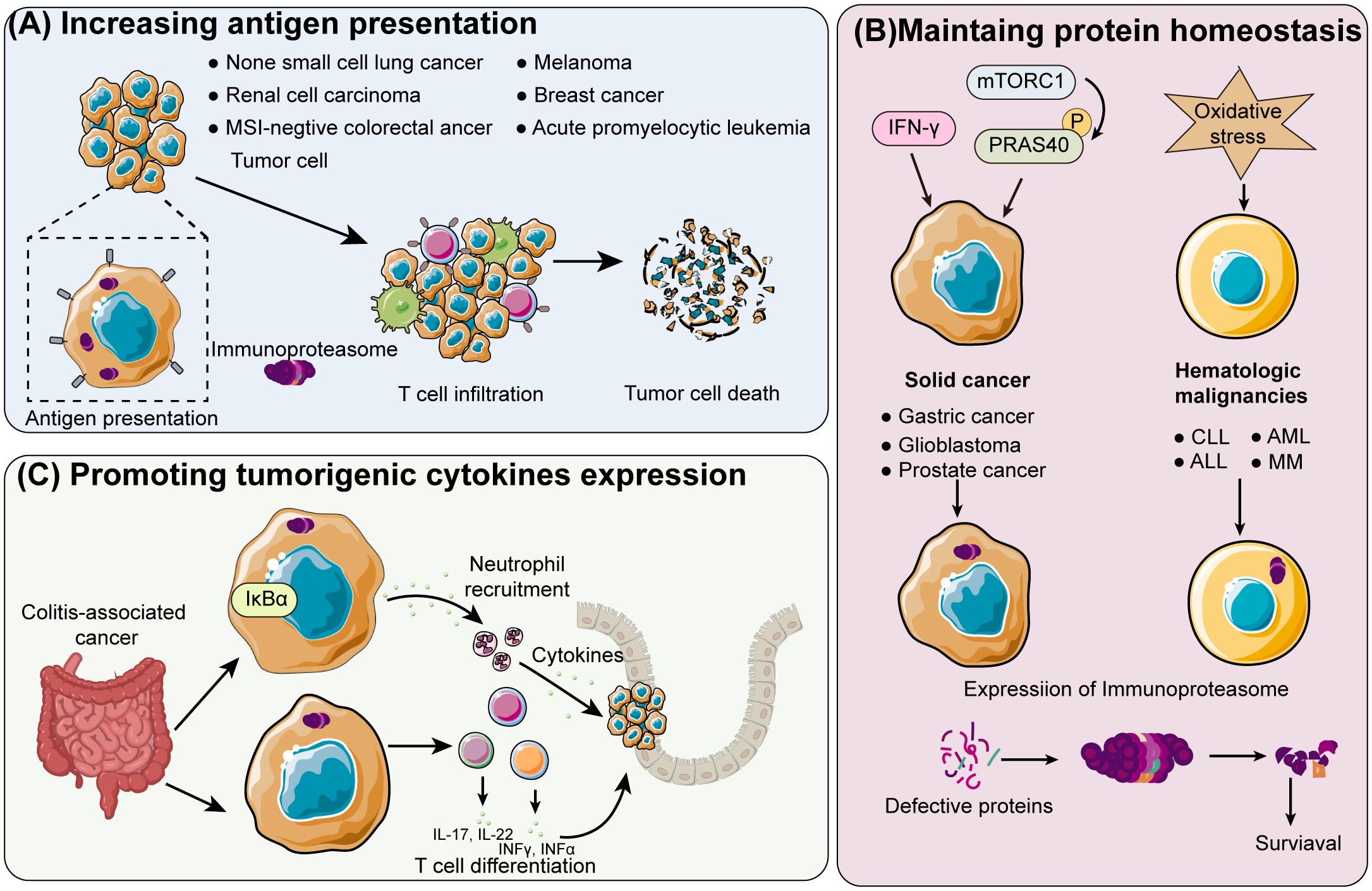
Figure 3. Schematic overview of immunoproteasome mechanisms in different cancer types (A) Enhancing tumor antigen presentation: The immunoproteasome enhances the presentation of tumor antigens in cancers like acute promyelocytic leukemia, breast cancer, non-small cell lung cancer, renal cell carcinoma, melanoma, and colorectal cancer that is negative for microsatellite instability (MSI). This leads to greater T-cell infiltration and subsequent tumor cell death. (B) Shifting proteasome population in solid and hematologic cancers: In solid cancers such as prostate, glioblastoma, and gastric cancer, the proteasome population is shifted towards immunoproteasomes by IFN-γ and phosphorylated proline-rich Akt substrate of 40 kDa (PRAS40), which is induced by hyperactivated mTOR complex 1 (mTORC1). Increased oxidative stress boosts immunoproteasome expression in hematologic malignancies, including multiple myeloma (MM), acute lymphoblastic leukemia (ALL), acute myeloid leukemia (AML), and chronic lymphocytic leukemia (CLL). Increased oxidative stress boosts immunoproteasome expression in hematologic malignancies, including multiple myeloma (MM), acute lymphoblastic leukemia (ALL), acute myeloid leukemia (AML), and chronic lymphocytic leukemia (CLL). Increased cancer cell survival is a result of the overexpressed immunoproteasome maintaining protein homeostasis. (C) Promoting tumorigenesis in colitis-associated cancer: The immunoproteasome degrades IκB in colitis-associated cancer, promoting the differentiation of T cells into the pro-tumorigenic inflammatory T helper cells.
5 Clinical applications
Numerous clinical trials are currently investigating the efficacy, safety, and optimal use of immunoproteasome-targeted therapies, both inhibitors and enhancers, against various types of solid tumors. For example, several early-phase trials are evaluating the efficacy of immunoproteasome inhibitors such as ONX 0914 in combination with checkpoint inhibitors like pembrolizumab and nivolumab (78–80). In addition, preliminary results of some clinical trials show that a combination treatment of immunoproteasome inhibitors and anti-PD-1 antibody achieved better response rates and prolonged progression-free survival in patients with melanoma and lung cancer compared to monotherapy. Similarly, cytokine therapies that upregulate immunoproteasome activity have been shown to enhance the anti-tumor immune response. Therefore, targeting the immunoproteasome can improve the effectiveness of current immunotherapies and offer patients with solid tumors new alternatives. However, further studies are required to validate these results and determine the long-term benefits and safety profiles of these combination therapies.
The identification of reliable biomarkers that can predict patient response is essential for the clinical success of immunoproteasome-targeted therapies. For instance, the expression levels of immunoproteasome subunits (β1i, β2i, and β5i), cytokine profiles, and the presence of particular tumor antigens can predict therapeutic efficacy, as well as help screen patients who will most likely to benefit from these therapies. Furthermore, the immunoproteasome activity in tumor tissues and immune cells can serve as an indicator to guide the selection and optimization of immunoproteasome-targeted personalized therapies as per the unique tumor and immune system characteristics of individual patients. For instance, patients with low baseline immunoproteasome activity may benefit from therapies that upregulate its function. In contrast, those with high activity might respond better to inhibitors that modulate their activity. Thus, personalized treatment strategies can enhance the efficacy and minimize the side effects of immunoproteasome-targeted therapies, leading to more effective and individualized cancer care.
6 Challenges and future directions
Immunoproteasome inhibitors can also target the proteasomes in normal cells, resulting in the accumulation of damaged or misfolded proteins, which trigger cellular stress and apoptosis. In fact, several side effects of immunoproteasome inhibitors have been reported in clinical trials, such as fatigue, gastrointestinal issues, and hematological toxicities. Developing inhibitors with greater specificity for cancer cells or optimizing dosing regimens can minimize these adverse effects. Furthermore, cancer cells can develop resistance to immunoproteasome inhibitors through various mechanisms, such as upregulation of compensatory proteolytic pathways, mutation of target subunits, or altered expression of proteasome-related genes, leading to relapse and treatment failure. Therefore, it is crucial to elucidate these mechanisms and develop novel strategies to overcome resistance to immunoproteasome inhibitors, such as combining other therapeutic agents that target complementary pathways or using sequential treatment approaches.
Future research should also focus on identifying novel targets within the immunoproteasome pathway. High-throughput screening and advanced genomic techniques can facilitate the discovery of new subunits or regulatory proteins. Additionally, understanding the complex interactions between the immunoproteasome and other cellular pathways can reveal new therapeutic opportunities. For instance, targeting regulatory proteins that control immunoproteasome assembly and activity could provide alternative strategies for modulating its function. Furthermore, advances in drug delivery systems can significantly enhance the efficacy and safety of immunoproteasome-targeted therapies. Nanotechnology-based delivery systems, such as liposomes, nanoparticles, and micelles, can improve the selective targeting of immunoproteasome inhibitors to tumor cells while sparing normal tissues. These systems can also provide controlled and sustained release of the therapeutic agents, reducing the frequency of administration and improving patient compliance. Finally, developing targeted delivery systems that exploit tumor-specific markers or the unique microenvironment of tumors can enhance the precision and effectiveness of immunoproteasome inhibitors.
Immunoproteasome-targeted therapies also hold promise for hematological malignancies such as multiple myeloma and lymphoma due to the high immunoproteasome activity that is frequently observed in these cancers. Exploring the efficacy of these therapies in a broader range of cancers can uncover new clinical applications and benefit a larger patient population. Furthermore, the development of more tailored and effective treatments requires a greater understanding of the differential expression and activity of immunoproteasomes across various cancer types. There is also evidence of a regulatory role of the immunoproteasome in autoimmune diseases and persistent infections. Thus, blocking immunoproteasome activity in autoimmune diseases could mitigate the aberrant immune responses, and reduce inflammation and tissue damage. For instance, immunoproteasome inhibitors have shown promising results in preclinical models of rheumatoid arthritis and lupus. Similarly, enhancing immunoproteasome activity could boost the immune response against chronic infections by improving antigen presentation and T-cell activation. Future research should explore these novel therapeutic possibilities for autoimmune diseases and chronic infections.
In conclusion, the clinical translation of immunoproteasome-targeted therapies will rely on addressing toxicity and resistance mechanisms and exploring novel targets and delivery systems, in order to optimize the therapeutic potential of the immunoproteasome.
7 Conclusion
The immunoproteasome is a key determinant of the response to cancer immunotherapy as it ensures the activation of anti-tumor CTLs by promoting generation of antigenic peptides and their presentation on MHC class I molecules, which in turn improves identification and elimination of cancer cells. Goven its role in modulating the TME and anti-tumor immune responses, the immunoproteasome has garnered considerable interest as a therapeutic target for cancer treatment. Recent studies have greatly improved our understanding of its structure, function, and regulation. Furthermore, clinical trials have reported promising results of immunoproteasome inhibitors, such as ONX 0914, against melanoma and lung cancer. Immunoproteasome-targeted therapies have also been shown to enhance the efficacy of checkpoint blockade therapy and improve patient outcomes. Additionally, strategies that upregulate immunoproteasome activity are also being explored to boost immune responses against tumors.
Future studies should focus on comprehending the mechanisms underlying immunoproteasome inhibitor resistance and developing strategies to address these challenges. Additionally, identifying reliable biomarkers for predicting patient response to immunoproteasome-targeted therapies will be crucial for personalizing treatment plans and maximizing therapeutic efficacy. Novel drug delivery systems and integrating immunoproteasome-targeted therapies with existing treatment regimens could offer new hope for patients with solid tumors, particularly those who do not respond well to conventional therapies. Tailoring treatments based on immunoproteasome activity and patient-specific biomarkers can enhance the precision and effectiveness of cancer therapy, leading to better patient outcomes and reduced side effects.
In conclusion, immunoproteasome-targeted therapies can enhance immune responses and overcome tumor-induced immunosuppression, resulting in more effective and durable treatment for cancer patients.
Author contributions
SS: Funding acquisition, Resources, Writing – original draft. XO: Data curation, Investigation, Writing – original draft. CL: Formal analysis, Methodology, Writing – original draft. HW: Methodology, Validation, Writing – original draft. KJ: Writing – original draft.
Funding
The author(s) declare that no financial support was received for the research, authorship, and/or publication of this article.
Conflict of interest
The authors declare that the research was conducted in the absence of any commercial or financial relationships that could be construed as a potential conflict of interest.
Publisher’s note
All claims expressed in this article are solely those of the authors and do not necessarily represent those of their affiliated organizations, or those of the publisher, the editors and the reviewers. Any product that may be evaluated in this article, or claim that may be made by its manufacturer, is not guaranteed or endorsed by the publisher.
References
1. Basler M, Groettrup M. On the role of the immunoproteasome in protein homeostasis. Cells. (2021) 10:3216. doi: 10.3390/cells10113216
2. Wang X, Zhang H, Wang Y, Bramasole L, Guo K, Mourtada F, et al. DNA sensing via the cGAS/STING pathway activates the immunoproteasome and adaptive T-cell immunity. EMBO J. (2023) 42:e110597. doi: 10.15252/embj.2022110597
3. Johnston-Carey HK, Pomatto LC, Davies KJ. The Immunoproteasome in oxidative stress, aging, and disease. Crit Rev Biochem Mol Biol. (2015) 51:268–81. doi: 10.3109/10409238.2016.1172554
4. Basler M, Mundt S, Bitzer A, Schmidt C, Groettrup M. The immunoproteasome: a novel drug target for autoimmune diseases. Clin Exp Rheumatol. (2015) 33:S74–9.
5. Yang Y, Liu W, Hu D, Su R, Ji M, Huang Y, et al. HIV-1 nef interacts with LMP7 to attenuate immunoproteasome formation and major histocompatibility complex class I antigen presentation. mBio. (2020) 11:e02221–19. doi: 10.1128/mBio.02221-19
6. Van den Eynde BJ, Morel S. Differential processing of class-I-restricted epitopes by the standard proteasome and the immunoproteasome. Curr Opin Immunol. (2001) 13:147–53. doi: 10.1016/S0952-7915(00)00197-7
7. Limanaqi F, Biagioni F, Gaglione A, Busceti CL, Fornai F. A sentinel in the crosstalk between the nervous and immune system: the (Immuno)-proteasome. Front Immunol. (2019) 10:628. doi: 10.3389/fimmu.2019.00628
8. Abi Habib J, Lesenfants J, Vigneron N, Van den Eynde BJ. Functional differences between proteasome subtypes. Cells. (2022) 11:421. doi: 10.3390/cells11030421
9. Kim S, Park SH, Choi WH, Lee MJ. Evaluation of immunoproteasome-specific proteolytic activity using fluorogenic peptide substrates. Immune Netw. (2022) 22:e28. doi: 10.4110/in.2022.22.e28
10. French T, Israel N, Düsedau HP, Tersteegen A, Steffen J, Cammann C, et al. The immunoproteasome subunits LMP2, LMP7 and MECL-1 are crucial along the induction of cerebral toxoplasmosis. Front Immunol. (2021) 12:619465. doi: 10.3389/fimmu.2021.619465
11. Xu H, Van der Jeught K, Zhou Z, Zhang L, Yu T, et al. Atractylenolide I enhances responsiveness to immune checkpoint blockade therapy by activating tumor antigen presentation. J Clin Invest. (2021) 131:e146832. doi: 10.1172/JCI146832
12. Canel M, Sławińska AD, Lonergan DW, Kallor AA, Upstill-Goddard R, Davidson C, et al. FAK suppresses antigen processing and presentation to promote immune evasion in pancreatic cancer. Gut. (2023) 73:131–55. doi: 10.1136/gutjnl-2022-327927
13. Maalej KM, Merhi M, Inchakalody VP, Mestiri S, Alam M, Maccalli C, et al. CAR-cell therapy in the era of solid tumor treatment: current challenges and emerging therapeutic advances. Mol Cancer. (2023) 22:20. doi: 10.1186/s12943-023-01723-z
14. Baulu E, Gardet C, Chuvin N, Depil S. TCR-engineered T cell therapy in solid tumors: State of the art and perspectives. Sci Adv. (2023) 9:eadf3700. doi: 10.1126/sciadv.adf3700
15. Xu R, Du S, Zhu J, Meng F, Liu B. Neoantigen-targeted TCR-T cell therapy for solid tumors: How far from clinical application. Cancer Lett. (2022) 546:215840. doi: 10.1016/j.canlet.2022.215840
16. Chen K, Wang S, Qi D, Ma P, Fang Y, Jiang N, et al. Clinical investigations of CAR-T cell therapy for solid tumors. Front Immunol. (2022) 13:896685. doi: 10.3389/fimmu.2022.896685
17. Pocaterra A, Catucci M, Mondino A. Adoptive T cell therapy of solid tumors: time to team up with immunogenic chemo/radiotherapy. Curr Opin Immunol. (2022) 74:53–9. doi: 10.1016/j.coi.2021.10.004
18. Vlashi E, Pajonk F. Cancer stem cells, cancer cell plasticity and radiation therapy. Semin Cancer Biol. (2015) 31:28–35. doi: 10.1016/j.semcancer.2014.07.001
19. Hong JC, Salama JK. The expanding role of stereotactic body radiation therapy in oligometastatic solid tumors: What do we know and where are we going? Cancer Treat Rev. (2017) 52:22–32. doi: 10.1016/j.ctrv.2016.11.003
20. Tinkle CL, Singh C, Lloyd S, Guo Y, Li Y, Pappo AS, et al. Stereotactic body radiation therapy for metastatic and recurrent solid tumors in children and young adults. Int J Radiat Oncol Biol Phys. (2021) 109:1396–405. doi: 10.1016/j.ijrobp.2020.11.054
21. Gibney GT, Weiner LM, Atkins MB. Predictive biomarkers for checkpoint inhibitor-based immunotherapy. Lancet Oncol. (2016) 17:e542–51. doi: 10.1016/S1470-2045(16)30406-5
22. Ribas A, Wolchok JD. Cancer immunotherapy using checkpoint blockade. Science. (2018) 359:1350–5. doi: 10.1126/science.aar4060
23. Restifo NP, Dudley ME, Rosenberg SA. Adoptive immunotherapy for cancer: harnessing the T cell response. Nat Rev Immunol. (2012) 12:269–81. doi: 10.1038/nri3191
24. Propper DJ, Balkwill FR. Harnessing cytokines and chemokines for cancer therapy. Nat Rev Clin Oncol. (2022) 19:237–53. doi: 10.1038/s41571-021-00588-9
25. Liu Y, Adu-Berchie K, Brockman JM, Pezone M, Zhang DKY, Zhou J, et al. Cytokine conjugation to enhance T cell therapy. Proc Natl Acad Sci U.S.A. (2023) 120:e2213222120. doi: 10.1073/pnas.2213222120
26. Ravi A, Hellmann MD, Arniella MB, Holton M, Freeman SS, Naranbhai V, et al. Genomic and transcriptomic analysis of checkpoint blockade response in advanced non-small cell lung cancer. Nat Genet. (2023) 55:807–19. doi: 10.1038/s41588-023-01355-5
27. Kalaora S, Lee JS, Barnea E, Levy R, Greenberg P, Alon M, et al. Immunoproteasome expression is associated with better prognosis and response to checkpoint therapies in melanoma. Nat Commun. (2020) 11:896. doi: 10.1038/s41467-020-14639-9
28. Keshet R, Lee JS, Adler L, Iraqi M, Ariav Y, Lim LQJ, et al. Targeting purine synthesis in ASS1-expressing tumors enhances the response to immune checkpoint inhibitors. Nat Cancer. (2020) 1:894–908. doi: 10.1038/s43018-020-0106-7
29. Du SH, Xiang YJ, Liu L, Nie M, Hou Y, Wang L, et al. Co-inhibition of the immunoproteasome subunits LMP2 and LMP7 ameliorates immune thrombocytopenia. Front Immunol. (2020) 11:603278. doi: 10.3389/fimmu.2020.603278
30. Xiang Y, Tian M, Huang J, Li Y, Li G, Li X, et al. LMP2-mRNA lipid nanoparticle sensitizes EBV-related tumors to anti-PD-1 therapy by reversing T cell exhaustion. J Nanobiotechnology. (2023) 21:324. doi: 10.1186/s12951-023-02069-w
31. Guo Y, Wang S, Li L, Zhang H, Chen X, Huang Z, et al. Immunoproteasome subunit low molecular mass peptide 2 (LMP2) deficiency ameliorates LPS/Aβ(1-42)-induced neuroinflammation. Mol Neurobiol. (2024) 61:28–41. doi: 10.1007/s12035-023-03564-9
32. Chen X, Zhang X, Wang Y, Lei H, Su H, Zeng J, et al. Inhibition of immunoproteasome reduces infarction volume and attenuates inflammatory reaction in a rat model of ischemic stroke. Cell Death Dis. (2015) 6:e1626. doi: 10.1038/cddis.2014.586
33. Opitz E, Koch A, Klingel K, Schmidt F, Prokop S, Rahnefeld A, et al. Impairment of immunoproteasome function by β5i/LMP7 subunit deficiency results in severe enterovirus myocarditis. PloS Pathog. (2011) 7:e1002233. doi: 10.1371/journal.ppat.1002233
34. Caudill CM, Jayarapu K, Elenich L, Monaco JJ, Colbert RA, Griffin TA. T cells lacking immunoproteasome subunits MECL-1 and LMP7 hyperproliferate in response to polyclonal mitogens. J Immunol. (2006) 176:4075–82. doi: 10.4049/jimmunol.176.7.4075
35. Leister H, Luu M, Staudenraus D, Lopez Krol A, Mollenkopf HJ, Sharma A, et al. Pro- and antitumorigenic capacity of immunoproteasomes in shaping the tumor microenvironment. Cancer Immunol Res. (2021) 9:682–92. doi: 10.1158/2326-6066.CIR-20-0492
36. Namiki S, Nakamura T, Oshima S, Yamazaki M, Sekine Y, Tsuchiya K, et al. IRF-1 mediates upregulation of LMP7 by IFN-gamma and concerted expression of immunosubunits of the proteasome. FEBS Lett. (2005) 579:2781–7. doi: 10.1016/j.febslet.2005.04.012
37. Fellerhoff B, Gu S, Laumbacher B, Nerlich AG, Weiss EH, Glas J, et al. The LMP7-K allele of the immunoproteasome exhibits reduced transcript stability and predicts high risk of colon cancer. Cancer Res. (2011) 71:7145–54. doi: 10.1158/0008-5472.CAN-10-1883
38. Rundberg Nilsson AJS, Xian H, Shalapour S, Cammenga J, Karin M. IRF1 regulates self-renewal and stress responsiveness to support hematopoietic stem cell maintenance. Sci Adv. (2023) 9:eadg5391. doi: 10.1126/sciadv.adg5391
39. Narayanan S, Cai CY, Assaraf YG, Guo HQ, Cui Q, Wei L, et al. Targeting the ubiquitin-proteasome pathway to overcome anti-cancer drug resistance. Drug Resist Update. (2020) 48:100663. doi: 10.1016/j.drup.2019.100663
40. Ettari R, Previti S, Bitto A, Grasso S, Zappalà M. Immunoproteasome-selective inhibitors: A promising strategy to treat hematologic Malignancies, autoimmune and inflammatory diseases. Curr Med Chem. (2016) 23:1217–38. doi: 10.2174/0929867323666160318173706
41. Bi M, Du X, Xiao X, Dai Y, Jiao Q, Chen X, et al. Deficient immunoproteasome assembly drives gain of α-synuclein pathology in Parkinson’s disease. Redox Biol. (2021) 47:102167. doi: 10.1016/j.redox.2021.102167
42. Griffin TA, Nandi D, Cruz M, Fehling HJ, Kaer LV, Monaco JJ, et al. Immunoproteasome assembly: cooperative incorporation of interferon gamma (IFN-gamma)-inducible subunits. J Exp Med. (1998) 187:97–104. doi: 10.1084/jem.187.1.97
43. Murata S, Udono H, Tanahashi N, Hamada N, Watanabe K, Adachi K, et al. Immunoproteasome assembly and antigen presentation in mice lacking both PA28alpha and PA28beta. EMBO J. (2001) 20:5898–907. doi: 10.1093/emboj/20.21.5898
44. Preckel T, Fung-Leung WP, Cai Z, Vitiello A, Salter-Cid L, Winqvist O, et al. Impaired immunoproteasome assembly and immune responses in PA28-/- mice. Science. (1999) 286:2162–5. doi: 10.1126/science.286.5447.2162
45. Niewerth D, Kaspers GJ, Assaraf YG, van Meerloo J, Kirk CJ, Anderl J, et al. Interferon-γ-induced upregulation of immunoproteasome subunit assembly overcomes bortezomib resistance in human hematological cell lines. J Hematol Oncol. (2014) 7:7. doi: 10.1186/1756-8722-7-7
46. Prahalad S, Kingsbury DJ, Griffin TA, Cooper BL, Glass DN, Maksymowych WP, et al. Polymorphism in the MHC-encoded LMP7 gene: association with JRA without functional significance for immunoproteasome assembly. J Rheumatol. (2001) 28:2320–5.
47. Lautscham G, Haigh T, Mayrhofer S, Taylor G, Croom-Carter D, Leese A, et al. Identification of a TAP-independent, immunoproteasome-dependent CD8+ T-cell epitope in Epstein-Barr virus latent membrane protein 2. J Virol. (2003) 77:2757–61. doi: 10.1128/JVI.77.4.2757-2761.2003
48. Montserrat V, Galocha B, Marcilla M, Vázquez M, López de Castro JA. HLA-B*2704, an allotype associated with ankylosing spondylitis, is critically dependent on transporter associated with antigen processing and relatively independent of tapasin and immunoproteasome for maturation, surface expression, and T cell recognition: relationship to B*2705 and B*2706. J Immunol. (2006) 177:7015–23. doi: 10.4049/jimmunol.177.10.7015
49. Zhang Y, Yang X, Bi T, Wu X, Wang L, Ren Y, et al. Targeted inhibition of the immunoproteasome blocks endothelial MHC class II antigen presentation to CD4(+) T cells in chronic liver injury. Int Immunopharmacol. (2022) 107:108639. doi: 10.1016/j.intimp.2022.108639
50. Inholz K, Anderl JL, Klawitter M, Goebel H, Maurits E, Kirk CJ, et al. Proteasome composition in immune cells implies special immune‐cell‐specific immunoproteasome function. Eur J Immunol. (2024) 54:e2350613. doi: 10.1002/eji.202350613
51. Schultz ES, Chapiro J, Lurquin C, Claverol S, Burlet-Schiltz O, Warnier G, et al. The production of a new MAGE-3 peptide presented to cytolytic T lymphocytes by HLA-B40 requires the immunoproteasome. J Exp Med. (2002) 195:391–9. doi: 10.1084/jem.20011974
52. Koerner J, Horvath D, Oliveri F, Li J, Basler M. Suppression of prostate cancer and amelioration of the immunosuppressive tumor microenvironment through selective immunoproteasome inhibition. Oncoimmunology. (2023) 12:2156091. doi: 10.1080/2162402X.2022.2156091
53. Di Pilato M, Kfuri-Rubens R, Pruessmann JN, Ozga AJ, Messemaker M, Cadilha BL, et al. CXCR6 positions cytotoxic T cells to receive critical survival signals in the tumor microenvironment. Cell. (2021) 184:4512–4530.e22. doi: 10.1016/j.cell.2021.07.015
54. Xiao M, Xie L, Cao G, Lei S, Wang P, Wei Z, et al. CD4(+) T-cell epitope-based heterologous prime-boost vaccination potentiates anti-tumor immunity and PD-1/PD-L1 immunotherapy. J Immunother Cancer. (2022) 10:e004022corr1. doi: 10.1136/jitc-2021-004022
55. Duong E, Fessenden TB, Lutz E, Dinter T, Yim L, Blatt S, et al. Type I interferon activates MHC class I-dressed CD11b(+) conventional dendritic cells to promote protective anti-tumor CD8(+) T cell immunity. Immunity. (2022) 55:308–323.e9. doi: 10.1016/j.immuni.2021.10.020
56. DhatChinamoorthy K, Colbert JD, Rock KL. Cancer immune evasion through loss of MHC class I antigen presentation. Front Immunol. (2021) 12:636568. doi: 10.3389/fimmu.2021.636568
57. Paijens ST, Vledder A, de Bruyn M, Nijman HW. Tumor-infiltrating lymphocytes in the immunotherapy era. Cell Mol Immunol. (2021) 18:842–59. doi: 10.1038/s41423-020-00565-9
58. Hall M, Liu H, Malafa M, Centeno B, Hodul PJ, Pimiento J, et al. Expansion of tumor-infiltrating lymphocytes (TIL) from human pancreatic tumors. J Immunother Cancer. (2016) 4:61. doi: 10.1186/s40425-016-0164-7
59. Eleftheriadis T, Pissas G, Antoniadi G, Liakopoulos V, Stefanidis I. CD8+ T-cell auto-reactivity is dependent on the expression of the immunoproteasome subunit LMP7 in exposed to lipopolysaccharide antigen presenting cells and epithelial target cells. Autoimmunity. (2013) 46:439–45. doi: 10.3109/08916934.2013.801460
60. Abusarah J, Khodayarian F, El-Hachem N, Salame N, Olivier M, Balood M, et al. Engineering immunoproteasome-expressing mesenchymal stromal cells: A potent cellular vaccine for lymphoma and melanoma in mice. Cell Rep Med. (2021) 2:100455. doi: 10.1016/j.xcrm.2021.100455
61. Besse A, Kraus M, Mendez-Lopez M, Maurits E, Overkleeft HS, Driessen C, et al. Immunoproteasome activity in chronic lymphocytic leukemia as a target of the immunoproteasome-selective inhibitors. Cells. (2022) 11:838. doi: 10.3390/cells11050838
62. Zhang Y, Hu W, Liu Q, Ma Z, Hu S, Zhang Z, et al. Expression of immunoproteasome subunits in the brains of Toxoplasma gondii-infected mice. Exp Mol Pathol. (2021) 123:104684. doi: 10.1016/j.yexmp.2021.104684
63. Dimasuay KG, Schaunaman N, Berg B, Cervantes D, Kruger E, Heppner FL, et al. Airway epithelial immunoproteasome subunit LMP7 protects against rhinovirus infection. Sci Rep. (2022) 12:14507. doi: 10.1038/s41598-022-18807-3
64. Burov A, Funikov S, Vagapova E, Dalina A, Rezvykh A, Shyrokova E, et al. A cell-based platform for the investigation of immunoproteasome subunit β5i expression and biology of β5i-containing proteasomes. Cells. (2021) 10(11):3049. doi: 10.3390/cells10113049
65. Ladi E, Everett C, Stivala CE, Daniels BE, Durk MR, Harris SF, et al. Design and evaluation of highly selective human immunoproteasome inhibitors reveal a compensatory process that preserves immune cell viability. J Med Chem. (2019) 62:7032–41. doi: 10.1021/acs.jmedchem.9b00509
66. Cathro HP, Smolkin ME, Theodorescu D, Jo VY, Ferrone S, Frierson HF Jr. Relationship between HLA class I antigen processing machinery component expression and the clinicopathologic characteristics of bladder carcinomas. Cancer Immunol Immunother. (2010) 59:465–72. doi: 10.1007/s00262-009-0765-9
67. Pérez-Jacoiste Asín MA, Fernández-Ruiz M, López-Medrano F, Lumbreras C, Tejido Á, San Juan R, et al. Bacillus Calmette-Guérin (BCG) infection following intravesical BCG administration as adjunctive therapy for bladder cancer: incidence, risk factors, and outcome in a single-institution series and review of the literature. Med (Baltimore). (2014) 93:236–54. doi: 10.1097/MD.0000000000000119
68. Han J, Gu X, Li Y, Wu Q. Mechanisms of BCG in the treatment of bladder cancer-current understanding and the prospect. BioMed Pharmacother. (2020) 129:110393. doi: 10.1016/j.biopha.2020.110393
69. Rouanne M, Adam J, Radulescu C, Letourneur D, Bredel D, Mouraud S, et al. BCG therapy downregulates HLA-I on Malignant cells to subvert antitumor immune responses in bladder cancer. J Clin Invest. (2022) 132(12):e145666. doi: 10.1172/JCI145666
70. Niewerth D, Jansen G, Assaraf YG, Zweegman S, Kaspers GJ, Cloos J. Molecular basis of resistance to proteasome inhibitors in hematological Malignancies. Drug Resist Update. (2015) 18:18–35. doi: 10.1016/j.drup.2014.12.001
71. Leister H, Krause FF, Mahdavi R, Steinhoff U, Visekruna A. The role of immunoproteasomes in tumor-immune cell interactions in melanoma and colon cancer. Arch Immunol Ther Exp (Warsz). (2022) 70:5. doi: 10.1007/s00005-022-00644-x
72. Yoon JY, Wang JY, Roehrl MHA. An investigation into the prognostic significance of high proteasome PSB7 protein expression in colorectal cancer. Front Med (Lausanne). (2020) 7:401. doi: 10.3389/fmed.2020.00401
73. Lee M, Song IH, Heo SH, Kim YA, Park IA, Bang WS, et al. Expression of immunoproteasome subunit LMP7 in breast cancer and its association with immune-related markers. Cancer Res Treat. (2019) 51:80–9. doi: 10.4143/crt.2017.500
74. Geoffroy K, Araripe Saraiva B, Viens M, Béland D, Bourgeois-Daigneault MC. Increased expression of the immunoproteasome subunits PSMB8 and PSMB9 by cancer cells correlate with better outcomes for triple-negative breast cancers. Sci Rep. (2023) 13:2129. doi: 10.1038/s41598-023-28940-2
75. Anderson AM, Kalimutho M, Harten S, Nanayakkara DM, Khanna KK, Ragan MA. The metastasis suppressor RARRES3 as an endogenous inhibitor of the immunoproteasome expression in breast cancer cells. Sci Rep. (2017) 7:39873. doi: 10.1038/srep39873
76. French BA, Oliva J, Bardag-Gorce F, French SW. The immunoproteasome in steatohepatitis: its role in Mallory-Denk body formation. Exp Mol Pathol. (2011) 90:252–6. doi: 10.1016/j.yexmp.2011.01.004
77. Oliva J, Bardag-Gorce F, Lin A, French BA, French SW. The role of cytokines in UbD promoter regulation and Mallory-Denk body-like aggresomes. Exp Mol Pathol. (2010) 89:1–8. doi: 10.1016/j.yexmp.2010.04.001
78. Wang M, Liu Y, Dai L, Zhong X, Zhang W, Xie Y, et al. ONX0914 inhibition of immunoproteasome subunit LMP7 ameliorates diabetic cardiomyopathy via restraining endothelial-mesenchymal transition. Clin Sci (Lond). (2023) 137:1297–309. doi: 10.1042/CS20230732
79. Waad Sadiq Z, Brioli A, Al-Abdulla R, Çetin G, Schütt J, Murua Escobar H, et al. Immunogenic cell death triggered by impaired deubiquitination in multiple myeloma relies on dysregulated type I interferon signaling. Front Immunol. (2023) 14:982720. doi: 10.3389/fimmu.2023.982720
80. Verbrugge SE, Assaraf YG, Dijkmans BA, Scheffer GL, Al M, den Uyl D, et al. Inactivating PSMB5 mutations and P-glycoprotein (multidrug resistance-associated protein/ATP-binding cassette B1) mediate resistance to proteasome inhibitors: ex vivo efficacy of (immuno)proteasome inhibitors in mononuclear blood cells from patients with rheumatoid arthritis. J Pharmacol Exp Ther. (2012) 341:174–82. doi: 10.1124/jpet.111.187542
Keywords: immunoproteasome, immunotherapy, solid tumors, immune, immune checkpoint inhibitors
Citation: Shi S, Ou X, Liu C, Wen H and Jiang K (2024) Immunoproteasome acted as immunotherapy ‘coffee companion’ in advanced carcinoma therapy. Front. Immunol. 15:1464267. doi: 10.3389/fimmu.2024.1464267
Received: 13 July 2024; Accepted: 14 August 2024;
Published: 30 August 2024.
Edited by:
Pengpeng Zhang, Nanjing Medical University, ChinaReviewed by:
Anshi Wu, Capital Medical University, ChinaJianbin Bi, The First Hospital of China Medical University, China
Copyright © 2024 Shi, Ou, Liu, Wen and Jiang. This is an open-access article distributed under the terms of the Creative Commons Attribution License (CC BY). The use, distribution or reproduction in other forums is permitted, provided the original author(s) and the copyright owner(s) are credited and that the original publication in this journal is cited, in accordance with accepted academic practice. No use, distribution or reproduction is permitted which does not comply with these terms.
*Correspondence: Ke Jiang, amlhbmdrZTA1MDIwNjE1QDE2My5jb20=