- 1Department of Tissue Engineering and Applied Cell Sciences, School of Medicine, Qom University of Medical Sciences, Qom, Iran
- 2Department of Hematology and Blood Banking, Faculty of Allied Medicine, Iran University of Medical Sciences, Tehran, Iran
- 3Department of Biotechnology, School of Paramedical Sciences, Qazvin University of Medical Sciences, Qazvin, Iran
- 4School of Biomedical Engineering, Med-X Research Institute, Shanghai Jiao Tong University, Shanghai, China
- 5Clinical Stem Cell Research Center, Renji Hospital, Shanghai Jiao Tong University School of Medicine, Shanghai, China
- 6Wisconsin National Primate Research Center, University of Wisconsin Graduate School, Madison, WI, United States
- 7Cellular and Molecular Research Center, Qom University of Medical Sciences, Qom, Iran
Acute myeloid leukemia (AML) is a hostile hematological malignancy under great danger of relapse and poor long-term survival rates, despite recent therapeutic advancements. To deal with this unfulfilled clinical necessity, innovative cell-based immunotherapies have surfaced as promising approaches to improve anti-tumor immunity and enhance patient outcomes. In this comprehensive review, we provide a detailed examination of the latest developments in cell-based immunotherapies for AML, including chimeric antigen receptor (CAR) T-cell therapy, T-cell receptor (TCR)-engineered T-cell therapy, and natural killer (NK) cell-based therapies. We critically evaluate the unique mechanisms of action, current challenges, and evolving strategies to improve the efficacy and safety of these modalities. The review emphasizes how promising these cutting-edge immune-based strategies are in overcoming the inherent complexities and heterogeneity of AML. We discuss the identification of optimal target antigens, the importance of mitigating on-target/off-tumor toxicity, and the need to enhance the persistence and functionality of engineered immune effector cells. All things considered, this review offers a thorough overview of the rapidly evolving field of cell-based immunotherapy for AML, underscoring the significant progress made and the ongoing efforts to translate these innovative approaches into more effective and durable treatments for this devastating disease.
1 Introduction
Acute Myeloid Leukemia (AML) is a very assertive and genetically diverse hematologic malignancy that can be life-threatening if not treated (1). Flow cytometry is the primary diagnostic tool for assessing surface antigens on leukemia cells, as simple morphology is insufficient for lineage determination (2). Special histochemical stains are also necessary, while peripheral blood can aid in diagnosis, a bone marrow biopsy is essential for evaluating morphology, cell surface markers, and for cytogenetic and molecular analysis. For a diagnosis, peripheral blood or bone marrow must have a blast count of 20% or above, except in specific cases with certain chromosomal abnormalities (e.g., t(15;17), t(8;21), inv(16), or t(16;16)) (3). The prognosis for AML is unfavorable, with cure rates of 5-15% in patients over 60 years old and 35-40% in those under 60 years old (4). AML genetic changes make cells grow faster, stop leukemic cells from maturing, and slow down programmed cell death (5). This causes cancerous cells to replace healthy erythroid, myeloid, and megakaryocytic progenitors (6). Clinically, AML manifests with low blood cell counts, including anemia, infections, bleeding, and bruising, alongside general symptoms, metabolic irregularities, and various complications (7). Cytogenetic and molecular events significantly impact AML subgroup classification and clinical management (8). AML is classified into two subtypes under the 5th edition of the World Health Organization’s (WHO) classification of hematolymphoid tumors: AML defined by differentiation and AML with defining genetic abnormalities (9). Genetic abnormalities remain essential diagnostic criteria.
Over the past 35 years, several studies have established a therapeutic induction protocol, now deemed the gold standard for patients not participating in clinical trials (10). This “3 + 7” protocol, which marries anthracycline and cytarabine, has emerged as the most potent intervention for AML. The primary objective of this therapeutic approach is eradicating leukemic cells from both the circulatory system and the bone marrow (BM) (11, 12). In certain instances of AML, however, high-dose cytarabine or hematopoietic stem cell transplantation (HSCT) may be effective ways to treat the disease (13). While many patients respond well to first-line therapy and find symptomatic relief, merely a little portion achieve long-term survival due to chemotherapy-resistant relapses (14). Additionally, challenges like drug resistance, limited therapy options for specific patient groups, and the urgent need for more effective targeted therapies present significant obstacles in AML treatment (15). Furthermore, it is possible to have difficulties obtaining and financing medical care and effectively handling the adverse effects of treatment. These deficiencies underscore the urgent need to address these gaps to improve outcomes for individuals battling AML (16). Despite advancements in the treatment of AML, particularly in higher-risk populations, progress in prolonging survival remains slow. Complete remission (CR) rates have increased since targeted treatments were introduced in conjunction with chemotherapy; however, relapse rates have not significantly changed, with over 60% of patients experiencing relapse, leading to a median disease-free survival (DFS) of less than one year (17). This emphasizes the urgent must recognize more effective therapeutic targets and enhance the efficacy of targeted therapies. While consolidation therapy has demonstrated benefits in improving overall survival (OS), its efficacy varies among patients, and maintenance therapy’s place in AML is still debatable (18). Few maintenance therapies, aside from arsenic trioxide and retinoic acid in acute promyelocytic leukemia, have shown adequate effectiveness to be adopted as standard treatment (17). Current induction therapies often fail to completely eliminate leukemic clones, necessitating additional post-remission approaches, especially for patients with adverse biological features (19). Allogeneic hematopoietic cell transplantation (allo-HSCT) is the most effective post-remission therapy but is associated with significant toxicities that may outweigh its benefits in certain patient groups. Furthermore, the detection of minimal residual disease (MRD) is crucial for identifying patients more likely to relapse and may inform maintenance treatment decisions (20). Historical maintenance strategies have often utilized drugs comparable to those employed in consolidation and induction, limiting their effectiveness. However, emerging clinical trials are exploring novel targets and maintenance approaches that incorporate targeted therapies based on specific mutational statuses, potentially offering improved safety and efficacy (21). Addressing these challenges through the identification of better targets and the refinement of targeted therapies is vital for advancing treatment outcomes in AML.
The evolving landscape of cancer treatment is shifting towards targeted therapies that enhance the host’s immune system to mount effective antitumor responses against malignancies (22). Immunotherapy, in particular, has experienced remarkable progress, solidifying its position as a cornerstone of cancer management alongside established methods such as surgery, chemotherapy, and radiotherapy (23). Immunotherapy has become an effective treatment approach against various human cancers, leveraging the immune system’s power to eliminate malignant cells (24). This method is broadly classified into two categories: active and passive immunotherapy. Active immunotherapy primarily involves utilizing dendritic cell vaccines, which stimulate the ability of the immune system to identify and target cancer cells (25). Conversely, passive immunotherapy encircles a range of strategies that directly enhance or modify immune cells to combat cancer (26). These include chimeric antigen receptor T (CAR-T) cell therapy, natural Killer (NK) cell therapy, and T cell receptor-engineered T (TCR-T) cell therapy. Moreover, passive immunotherapy extends to utilizing checkpoint inhibitors, which disengage the immune system’s brakes, enabling it to combat cancer more effectively. Passive immunotherapy also uses oncolytic viruses, which only infect and kill cancer cells, and monoclonal antibodies, which attack specific proteins in cancer cells (27, 28). By harnessing these immunotherapeutic strategies, there is potential for better results for AML patients, particularly in those with relapsed or refractory disease. This study aims to examine the most current developments in AML immune cell treatments, evaluate the current obstacles in this area, and introduce developing approaches that could improve treatment efficacy.
2 Immune cell therapy targeting specific antigens for AML
HSCT has proven highly effective in curing AML, yet it carries risks of transplant-related complications and fatalities. As a result, many patients succumb to disease progression or recurrence as a result of unfavorable side effects or treatment resistance (29). Unfortunately, not all patients qualify for HSCT, and relapse following transplantation continues to be the main reason for treatment failure. Consequently, exploring innovative AML treatments is vital (30). Harnessing the immune system to eradicate leukemic cells shows a great potential therapeutic approach, with successful implementations in HSCT and non-HSCT settings. Immunotherapies that use immune cells, like CAR-T cells, TCR-T cells, and CAR-NK cell therapy, have proven to be very efficient at treating AML invulnerable to chemotherapy (31). This section will explore various cell-based immunotherapies, examining their unique mechanisms and potential applications in AML treatment.
2.1 CAR-T cell therapy
CAR-T cells have revolutionized the therapeutic environment for individuals with lymphoma, acute lymphoblastic leukemia (ALL), and multiple myeloma (MM), demonstrating remarkable clinical success and significantly improving patient survival rates. The US Food and Drug Administration (FDA) has authorized CAR-T cell products for these signs, highlighting their therapeutic potential (32). On the other hand, CAR-T cells need to be used right away to treat AML, where treatment resistance (10–40%) and relapse are still big problems, especially for people who can’t get allogeneic HSCT (33). Since the arrival of cutting-edge technology, CAR-T cell engineering is making significant strides in AML treatment. The focus on enhancing specificity, reducing toxicity, and improving efficacy is set to transform our procedure to AML therapy (34). One of the recent advances in CAR-T cell therapy for AML involves targeting specific antigens like CD33, CD123, FLT3, and CLL-1, among others. Clinical trials are currently in progress to assess the security and performance of CAR-T cells in relation to these targets (35).
Several challenges it has to be addressed to treat AML using CAR-T cells effectively. These include the absence of an appropriate antigen uniformly expressed on leukemic cells, the intricate microenvironment of AML, and the need for suitable cell sources (36). Both preclinical and clinical studies have zeroed in on several antigens, such as CD33, CD123, CLL-1, and CD13, among others, as potential targets (37). Recent research has introduced the development of fourth-generation CAR-T cells, engineered with an immune modulator, which exhibit enhanced effectiveness and durability, potentially overcoming the tumor microenvironment (38). Exploring CAR-T cell therapy in AML treatment has unveiled new targets, notably leukocyte immunoglobulin-like receptor B4 (LILRB4) and Sialic acid-binding Ig-like lectin 6 (Siglec-6). These targets demonstrate a high degree of selectivity and low toxicity compared to normal hematopoietic cells (39). In refractory AML patients, compound CAR (cCAR) T-cells targeting multiple AML antigens, like CLL1 and CD33, have exhibited robust anti-tumor activity. This approach holds the potential to eliminate leukemia progenitor cells, paving the way for complete remission (40). Bi-specific CAR-T cells, intended to reach both CD13 and T cell immunoglobulin and mucin-containing-3 (TIM3), have shown assurance in potentially eradicating AML while minimizing toxic damage to human BM stem cells (41). A study by Sauer et al. underscores the effectiveness of CD70-specific CAR-T cells against AML, preserving normal HSCs and striking a harmony between safety and effectiveness (42).
Over 190 clinical trials are underway to make CAR-T cell biology more efficient and identify new targets, suggesting a promising future for CAR-T cell therapy in treating AML (34). A number of strategies are being investigated to improve the efficacy of CAR-T cell therapy, such as employing nanobodies to target specific antigens like CD13 and TIM3 (43). Studies conducted in vivo and in vitro have demonstrated that CAR-T cells target surface protein., including CD7, CD13, CD25, CD32, CD33, CD38, CD44, CD45RA, CD47, CD70, CD96, CD123, CLL-1, NKG2D ligand, Lewis Y antigen, Folate receptor β, FLT-3, CLEC12A, and TIM3, effectively eliminate AML cells (44) (Table 1).
2.2 TCR-T cell therapy
TCR-T therapy employs T-cell receptors (TCRs) from naive T cells for specificity, as opposed to relying on antibody-based CARs. These T cells are a source of TCRs that target tumor cells and subsequently modified to enhance their expression and functionality (126). The initial step in generating TCR-modified T cells involves isolating TCRs that exhibit precise recognition of leukemia-specific antigen (LSA) or leukemia-associated antigen (LAA) epitopes (127). These TCRs can be identified in T-cell clones that effectively target leukemia cells, sourced from patients’ BM or blood, or derived from healthy donor T cells stimulated with LSA or LAA peptides that restrict major histocompatibility complex (MHC) class I/II (127). Wilms’ Tumor Gene (WT1) is consistently expressed in myeloid leukemia cells, including those affected by myelodysplastic syndrome (MDS), AML, and chronic myeloid leukemia (CML). Notably, cytotoxic T lymphocytes (CTLs) specific to WT1 have been recognized in the blood of leukemia patients. Consequently, WT1 emerges as a desirable objective for CTL stimulation in leukemia immunotherapy (127–129).
The UMIN000011519 trial provided preliminary evidence that WT1-specific TCR-transduced autologous T cells are effective for refractory AML or high-risk MDS in HLA-A*24:02 patients (109, 130). Two of the eight participants exhibited decreased embryonic cells in their BM, suggesting a reversal in leukemia progression. Notably, WT1-specific TCR-T cells persisted in five patients, four surviving beyond 12 months. No toxicity-related adverse effects in healthy tissues were noticed in any participants (130). Numerous tumor-associated antigens (TAAs) and possible objectives have been recognized in preclinical TCR-T treatment investigations for AML (131, 132). Recently, additional leukemia-specific TCRs have been discovered, which bind to Formin-like protein 1 (FMNL1) and are restricted to MHC class I/II (133). Furthermore, cancer-testis antigens (CTAs), minor histocompatibility A (HA)-1, telomerase reverse transcriptase (TERT), and surviving have been recognized as TAAs and are under preclinical investigation (134, 135). HMMR/Rhamm-TCRs have been recognized as well in patients with ALL and AML (136), expanding the repertoire of potential targets for TCR-T therapy in leukemia.
2.3 NK cell therapy
In patients with AML, the NK cell role is often compromised, giving cancer cells the ability to avoid immune detection. NK cell immunotherapy represents a procedure to counteract NK cell inhibition, thereby enhancing their capability to eradicate cancer cells (137). Apart from antibody-mediated cell cytotoxicity (ADCC), NK cells utilize the release of cytolytic granules and cytokines to induce target cell destruction (138, 139). Romee et al. showed that NK cells display memory-like traits, evidenced by increased IFN-γ production after pre-activation with IL-12, IL-15, and IL-18, followed by a 1-3 week resting period before exposure to cytokines or K562 leukemia cells (140). These findings have sparked investigations into diverse cell sources to identify possible contenders for NK cell generation in adoptive cellular therapy. Among the cell sources being explored are cord blood, peripheral blood mononuclear cells (PBMCs), NK-92 cell lines, hematopoietic stem and progenitor cells, and induced pluripotent stem cells (141).
In order to increase the cytotoxicity and selectivity of NK cells, genetic modification through the incorporation of CAR constructs is employed (142). While CAR-T therapy is efficient for B-cell ALL and lymphoma, its use in AML treatment is challenging due to limitations and adverse effects like cytokine release syndrome (CRS) (34). In place of T cells that have been changed, NK cells with a short lifespan present a more cost-effective production option and exhibit fewer harmful side effects (143). The efficiency of CAR-NK cells in different types of cancer models is currently under active investigation. Although they show promise in the preclinical stage of AML treatment, their application is limited (144). Like T cells, NK cells able to be changed to carry identical CAR, enabling CAR-NK cells to target cancer cells for destruction. Regarding the source of CAR-NK cells, preclinical research revealed that primary human donor CD123-CAR-NK cells were less effective as CAR effector cells, while CD123-CAR-NK-92 cell lines demonstrated superior performance (145).
The challenge of identifying leukemia-specific markers that can be effectively targeted by CAR-NK cells arises from the shared phenotypic characteristics between AML and normal HSCs (143, 146). One potential target is the myeloid differentiation marker CD33, which is present in leukemia stem cells (LSCs), and over 85% of AML patient blasts (147, 148). In a preclinical study, the NK cell line YT was validated to target CD33+ AML cell lines via gene transfer of a humanized chimeric T cell receptor (cIgTCR) based on CD33 (149, 150). A subsequent phase I clinical trial confirmed the consideration of safety infusing irradiated CD33 CAR-NK-92 cells in three patients with relapsed or refractory AML, although nothing noteworthy therapeutic efficacy was noted (151). Additional probable targets for CAR-NK-92 cells include CD4 and CD7 antigens found on AML blasts. Preclinical and clinical research has focused on engineering CAR-NK-92 cells to target and eliminate CD4+ and CD7+ AML cells, specifically (152–154). Also, researchers achieved another source on which they could apply CAR structure to employ new cells like NK cells, NKT cells, and macrophage cells (155). For example, The expression level of CD47 is higher in AML stem cells and associated with suboptimal prognosis in adult AML patients (69). Recent advancements in synthetic biology and the expanding comprehension of the CD47/SIRPα axis may offer new possibilities for using engineered macrophages in clinical settings. This axis is a primary pathway that inhibits macrophage phagocytosis and activation. Therefore, CD47-CAR-macrophages hinder the CD47/SIRPα axis and also self-activate to launch an assault the CD47-positive cancer cells (156).
3 Challenges in immune cell therapy for AML
Immunotherapy holds immense potential in the management of AML, yet several challenges must be addressed to optimize its clinical efficacy (157). These challenges can be generally categorized into two groups: disease-specific and treatment-related. Disease-specific challenges include the heterogeneity of antigens in AML, the absence of appropriate antigens, and the BM microenvironment, which is often influenced by the existence of AML blasts (158). On the other hand, treatment-related challenges primarily revolve around ethical concerns, the potential development of cytokine release syndrome, and the continuance of cells post-injection (159) (Figure 1).
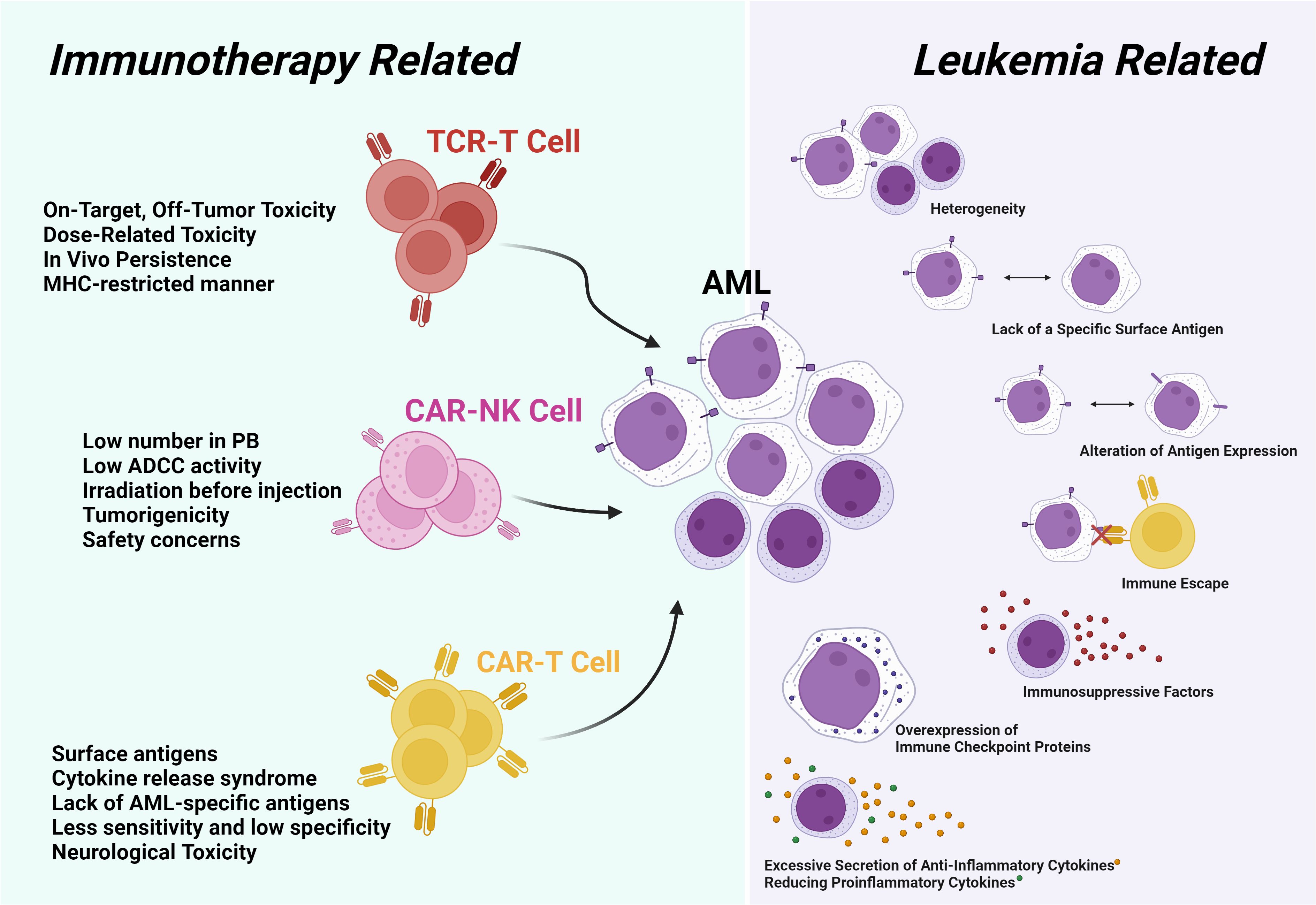
Figure 1. The challenges in AML can be categorized into two main groups. The first group pertains to leukemia-related challenges, encompassing issues of heterogeneity. The second group is associated with challenges related to immunotherapy methods, which may include on-target/off-tumor effects in the type of treatment method, as well as HLA matching in TCR-T cell therapy.
3.1 Leukemia-related challenges
AML offers a substantial challenge due to its tumor heterogeneity, primarily linked to the existence of LSCs. These LSCs perpetuate the disease through self-renewal, quiescence, and treatment-resistance mechanisms (160). Furthermore, the absence of stem cell characteristics in differentiated cells contributes to a negative impact on the surrounding environment of the tumor, consequently influencing tumor biology (161). The dynamic nature of antigen expression, which may diminish or cease during treatment, can lead to treatment insensitivity. AML’s heterogeneity arises from unique chromosomal abnormalities, gene mutations, or gene fusions, further complicating its management and therapy (162).
The challenge in treating AML is its limited efficacy and the lack of distinct surface antigens, crucial for protecting healthy hematopoietic cells. The identification of an antigen target it is essential to the biology of AML and is unique to malignant cells poses a formidable challenge (163). In contrast, CAR-T cell therapy has demonstrated significant progress has been made in In effectively treating diffuse large B-cell lymphoma (DLBCL), MM, and ALL. This success is attributed to its ability to selectively target specific surface antigens (CD19, CD22, and BCMA) (164). However, the intrinsic variability and heterogeneity of tumors present a significant hurdle in predicting patient responses, resulting in a high recurrence rate of 75%. Relapse and treatment resistance, occurring in 10-40% of cases, remain the primary complications post-treatment, thereby emphasizing the urgent need for innovative therapies (165).
Furthermore, the AML bone marrow microenvironment features various cells that suppress T-cell activity, including macrophages, regulatory T-cells (Treg), myeloid-derived suppressor cells (MDSC), and dendritic cells (DC) (166). Notably, Treg cells exhibit abnormally high expression of CD39, and increased CD73 levels have been linked to an unfavorable prognosis. Moreover, MDSC levels in BM may function as a prognostic indicator for AML (3, 167).
The proliferation of AML cells is triggered by reduced CXCL12 expression in bone marrow stromal cells, while WNT ligands from osteoblasts enhance leukemia cell survival (168). In addition to indoleamine 2,3-dioxygenase (IDO), other immunosuppressive substances, like indoleamine 2 and reactive oxygen species (ROS), contribute to immune evasion in AML (169–171). In tumor-bearing mice, the cytotoxicity ability of CD8+ T lymphocytes was discovered to be inhibited due to elevated ROS levels in immature myeloid cells generated from these animals compared to tumor-free animals (172). Similarly, an investigation conducted on human peripheral blood and BM from AML patients indicated that monocytic AML cells activated poly-ADP-ribose polymerase-1-dependent apoptosis to kill T-cells and NK cells by secreting ROS (173). Moreover, patients with AML exhibit higher expression levels of enzymes engaged in producing immunosuppressive products such as IDO. These enzymes possess the capacity to prevent T-cell reactions by causing the high expression of Treg cells (174).
AML blasts can trigger the release of pro-inflammatory cytokines, like tumor necrosis factor-α (TNF-α), IL-1β, and IL-6, in monocytes. Myeloid or lymphoid progenitor cells release two key pro-inflammatory cytokines, including IL-15 and IFN-γ, which play a crucial function in eradicating leukemia cells (175, 176). Low serum IL-15 levels immediately following allogeneic HSCT have been associated with leukemia recurrence (177). As tumors progress, elevated levels of IL-10 display strong immunosuppressive effects, inhibiting T-cell proliferation and the generation of cytokines like IFN-γ and IL-2. In the cancer field, IL-10 has been demonstrated to possess a dual biological effect, either elevating tumor development or inhibiting it (178–180).
In the tumor microenvironment, AML cells enhance the expression of immunomodulatory factors that impede the CTLs activation. These factors include transforming growth factor-β (TGF-β), arginase II, prostaglandin E2 (PGE2), CTL-associated protein 4 (CTLA-4), lymphocyte activation gene 3 (LAG3), and TIM3 on T-cells (35). As an instructional method to avoid immune surveillance systems, AML cells may induce T-cell exhaustion (181). AML relapse can occur when CD8+ and CD4+ T cells express higher levels of programmed cell death protein 1 (PD-1) following allogeneic HSCT, leading to T-cell exhaustion (182). Notably, merging T-cell therapy with drugs targeting the PD-1 immune cell has shown impressive effectiveness in treating leukemia, resulting in enhanced cytolytic activities, the memory of CD8+ T cells, and IFN-γ production (183). Therefore, inhibitory receptor-blocking strategies could be valuable treatment approaches for leukemia, as they augment the immune system’s collaborative ability (109).
Downregulation of activation ligands in addition to high expression of inhibitory receptors for NK on AML cell surface can result in cytotoxic dysregulation of NK cells. NK cells’ antileukemic replies may also be impacted through various AML receptor-ligand interactions or immunosuppressive factors secretion (184). A mouse model revealed that melding NK cells with exogenous IL-15 could boost immune effector cells to eliminate leukemia following allogeneic HSCT (137, 185). In the NCT01885897 phase I trial, leukemia patients relapsing after allogeneic HSCT showed improved CD8+ T cell and NK cell capabilities with ALT-803 (186). Furthermore, leukemia cells capable of avoid the immune system by decreasing levels of IL-1β and granulocyte colony-stimulating factor (G-CSF), which are inflammatory growth factors (187).
Despite significant expansion of infused cells in vivo, substantial therapeutic results are often impeded by inhibitory effects of self-HLA ligands in certain tumors, especially AML (188). Moreover, because autologous NK cells are obtained from heavily pre-treated patients, their growth and operational capabilities may not be as high as expected (189). There is a strong rationale for investigating the potential of NK cells as agents to combat leukemia. Different approaches are under development to get over the current limitations of autologous NK cell lineages. Lirilumab, an anti-KIR antibody, is meant to suppress signals causing inhibition by preventing interactions with MHC class I ligands, resulting in an upregulation of NK cells’ killing capacity (190). Additionally, the application of combination therapy, involving autologous NK cells and various anti-tumor agents, has been demonstrated to augment therapeutic responses against tumors (184). This rationale is supported by evidence from in vitro experiments, animal models, and clinical trials.
3.2 Immunotherapy-related challenges
Immunotherapy presents several challenges and benefits across different modalities, including CAR-T cell therapy, TCR-T cell therapy, NK cell therapy, and other immunotherapies. CAR-T cell therapy can lead to severe side effects like CRS and neurotoxicity, and it has limited efficacy in solid tumors because of antigen escape (191). TCR-T cell therapy confronts problems like the need for suitable TCRs and the risk of autoimmunity, alongside complex manufacturing processes (192). NK cell therapy, while capable of rapid responses, often suffers from a short-lived effect and inhibition by the tumor microenvironment (193). Other immunotherapies, like checkpoint inhibitors, can trigger immune-related unfavorable incidents and encounter resistance mechanisms due to tumor heterogeneity (194). Despite these challenges, these therapies also offer significant benefits. CAR-T cells provide a targeted approach with the potential for durable remissions, while TCR-T cells can recognize a broader range of antigens and be personalized for individual patients. NK cells benefit from a quick innate immune response and a lower risk of graft-versus-host disease (191). Additionally, other immunotherapies can enhance the immune response and be effectively combined with traditional treatments, showcasing their potential in revolutionizing cancer therapy (195). In this part, we discuss the challenges of cell-based immunotherapy.
3.2.1 CAR-T cells challenges
Immunotherapeutic approaches face challenges like high “on-target off-tumor” toxicity, potentially fatal CRS, and neurological issues that hinder their effective use (146, 196). CAR-T cells can attach to substances on the cell surface without requiring antigen processing or HLA expression. A critical aspect of the production process involves choosing the right option surface antigen to target (197). The perfect target would have little to no expression on healthy tissues in order to avoid toxicity and high expression on tumor cells, exceeding CAR-T cell activation thresholds (198). Despite extensive research on the immunopathology of AML, a specific AML target remains difficult to achieve (199, 200). However, the significant danger of on-target/off-tumor activity needs to be taken care of. Prolonged myelosuppression is a concern, as most AML blast surface antigens are co-expressed by mature myeloid cells, HSCs, and other relevant tissues (200, 201). Some phase I trials have reported severe toxicities and fatalities. For instance, patients receiving CAR-T cells targeting CD33, an antigen present in most leukemic blasts and normal myeloid lineages, experienced severe pancytopenia and CRS. Additionally, excellent preclinical outcomes of CD44V6 CAR-T were accompanied by monocytopenia, likely because of the common expression of CD44v6 in circulating monocytes (202, 203). Secondary T cell lymphoma that develops after CAR-T cell therapy is rare but a noteworthy issue. The rising incidence of secondary primary malignancies, particularly myeloid neoplasms, after CAR-T cell therapy requires attention, as several reports indicate the emergence of SMNs, including MDS and AML, following this treatment. These observations suggest that prior chemotherapy and the immunosuppressive environment may elevate this risk (204, 205). The proportion of SMN after CD19 CAR-T cell therapy varies significantly, with rates reported ranging from 0.9% to 12.9%. These differences may have resulted from complex and multifactorial etiologies (205). Therefore, secondary malignancies pose a significant problem, and further research of the mechanisms participant and methods of minimizing these risks is imperative. CAR-T cell therapy has become a ground-breaking medical intervention for various diseases, but the development of secondary T-cell lymphomas and other cancers cannot be ignored.
3.2.2 TCR-T cells challenges
Nevertheless, some TCR-T cell immunotherapies are currently employed to handle AML, these treatments face specific challenges (109). Until a thorough assessment of on-target/off-tumor toxicity, toxicity associated to dose, in vivo durability of TCR-T cells, and potential immune evasion by AML post-TCR-T injection is completed, TCR-T cell therapy usage will stay restricted (192). TCR-T cells eliminate leukemia cells by participating in their engineered TCRs with antigens presented by HLA molecules on the surfaces of these cells. Therefore, identifying neoantigens and matching HLA between donor and patient is a significant obstacle (134). One obstacle in this approach is the restriction of TCR-T cells to HLA, which is often downregulated in AML recurrence (108). However, cytokines like IFN-α, IFN-β, and IFN-γ are essential for enhancing MHC-I expression, and inserting IFN-γ into the C-domain of a TCR could circumvent MHC molecule down-regulation (206). Animal models have demonstrated that modifying TCR-T cells with pro-inflammatory cytokines like IL-15, IL-18, or IL-12 enhances persistence and exhibits a favorable safety profile when used against tumors (207, 208).
The potential of on-target and off-tumor toxicity in treatments employing adoptional swap of antigen-specific TCR-T cells raises concerns. Adoptional swap of autologous TCR-T cells has been associated with neurotoxicity and cardiac toxicity as off-target toxicity side effects in two clinical investigations (209, 210). Another challenge in TCR-T cell therapy is the restricted T cell capacity to strive and proliferate in vivo over extended periods, which reduces therapeutic efficacy (211). To improve T-cell persistence in vivo, several strategies, such as genetic modification of T-cell signaling and cytokine or pharmacological provocation of T cells, can be employed (212). An effective method for enhancing the growth and longevity of TCR-T cells is to incorporate the intracellular domain (ICD) of signaling components (such as CD28 or 4-1BB) onto CD3Ύ, rather than altering the TCR affinity (213, 214). These engineered TCR-T cells have shown improved effectiveness, extended in vivo lifespans, and improved proliferation (215, 216). Studies suggest that administering antigen-specific T-cells with cytokines enhances T-cell persistence and induces T-memory stem cell (TSCM) generation. Consequently, low-dose decitabine treatment of TCR-T cells may also augment phenotypic indicators of TSCM (217).
3.2.3 NK cells challenges
In NK cell-based therapy, both autologous and allogeneic sources face challenges connected to prompt ex vivo expansion, low clinical-grade activation, and an absence of in vivo persistence (218). Combining many cytokines can play a crucial role in the activation (IL-18 and IL-21), proliferation (IL-2 and IL-15), and effector function (IFN-γ and TNF-α) of NK cells (219). The IL-15 super agonist complex, ALT-803, has proved to be a safe agent in the first in-human phase I study, emphasizing senior AML patients who relapsed following HSCT (220). In a research aimed at pre-activating NK cells with IL-2, significant in vitro cytolytic activity and in vivo persistence were observed, but no notable clinical reactions were seen. However, persistent NK cell-mediated ADCC without in vitro cytokine reactivation indicates that combining monoclonal antibodies with autologous adoptive NK cell transfer warrants further assessment and investigation (189, 221). Safety concerns arise from the necessity to irradiate products derived from immortalized NK lymphoma cell lines, which are utilized to cultivate NK cells, before infusion (222). Moreover, even with ADCC, the killing capacity of NK-92 cell lines may be constrained due to the absence of CD16 (FCI) and other activating killer cell immunoglobulin-like receptors (KIRs) (223). Induced pluripotent stem cells are optimal for acquiring NK cells, provided their rapid proliferation. However, they also exhibit lower CD16 levels, which might impair their ability to eliminate cancer cells. This challenge could be addressed through genetic engineering (224). Recently, innovative NK cell-based immunotherapies, like adoptive transfer and CAR-NKs, have been assessed in AML clinical trials (225). The challenges associated with NK cell therapy are depicted in Figure 1.
4 Clinical trial targets of cell-based immunotherapy for AML
AML treatment encompasses conventional chemotherapy, targeted medications, HSCT, and immune-based cell therapies. Each approach has its benefits and drawbacks. Conventional chemotherapy protocols for AML have been well-established and serve as the primary treatment for recently diagnosed cases. However, these agents can cause organ damage and hematopoietic system suppression, especially in elder patients. Emerging targeted drugs such as gilteritinib (FLT3 inhibitor) (226), enasidenib (IDH2 inhibitor) (227), ivosidenib (IDH1 inhibitor) (228), and venetoclax (BCL-2 inhibitor) (229) have demonstrated promising outcomes, but their cost may limit access for some patients. HSCT remains a feasible choice, especially for young patients with suitable donors, but carries risks of severe complications like graft-versus-host disease (GVHD) and infections (230). To develop effective targeted immunotherapies, finding an appropriate target antigen is essential. Cheever et al. listed the characteristics of a perfect antigen for targeting including immunogenicity, clinical effects, and an important part in the differentiation and proliferation of malignant cells. Its expression ought to be limited to cancerous cells; all cancerous cells, including cancerous stem cells, should express it (231). A significant ratio of patients should exhibit positive antigen tests, and malignant cells should have the antigen on their surface, consisting of several antigenic epitopes (232). In Table 1, we discussed the clinical studies of cell-based immunotherapy.
5 New potential targets of AML for cell-based immunotherapy
Based on the challenges mentioned above, various solutions were considered. One crucial solution involves identifying a new target capable of specifically and accurately attacking cancer cells, while also preventing the protection of the stromal cells in the BM niche. The progression of treatments for AML has been hindered by the diversity and high frequency of disease relapse, emphasizing the critical demand for novel therapeutic options. Researchers are investigating diverse strategies for managing AML, and while some are more promising than others, each can cause valuable treatments (233) (Figure 2).
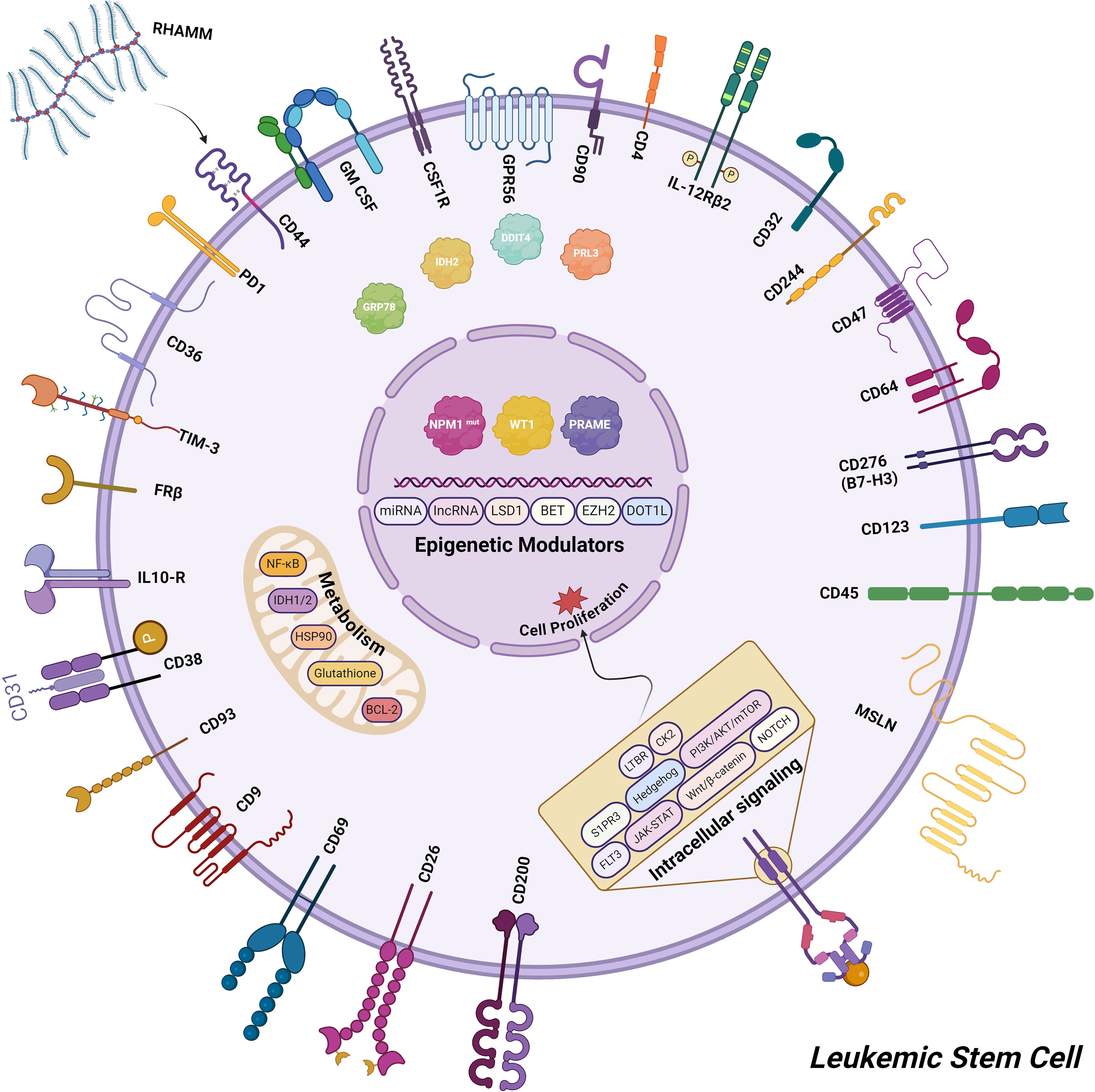
Figure 2. The updated targets for AML cell-based immunotherapy encompass a range of potential candidates, including cell surface antigens, proteins involved in various signaling pathways, key factors in cell metabolism, and epigenetic modulators.
Computational models have recently emerged as useful instruments for the in silico and systematic analysis of significant biological mechanisms and patient remarks in cancer immunotherapy. These models are according to empirical justifications and mathematical simulations with clinical data input (234). However, computational modeling of CAR-T cell therapy remains in its early phases, and there are limited applications for model-informed response prediction. For instance, utilizing information from xenograft mouse models, a multiscale pharmacokinetic-pharmacodynamic model based on physiological principles was created to quantitatively investigate the connection between CAR affinity, antigen abundance, tumor cell depletion, and CAR-T cell expansion (235, 236). Other approaches model factors influencing CAR-T cell dynamics, such as ecological dynamics regulating expansion and exhaustion, signaling variability in cell states, lymphodepletion effects on expansion, and competition between CAR-T and normal T-cells (237, 238). Recently, Liu et al. created a model retrospectively to describe clinical kinetics of CAR-T cells in relation to reaction status, patient populations, and tumor types (236). However, computational models often lack comprehensive analysis of clinical trial data, and a predictive model based on clinical data to forecasts of patient responses to CAR-T cell treatment are mostly lacking (239) (Figure 3).
The significance of the optimized interaction among AML blasts, the hematopoietic niche, and immune cells has been demonstrated to play a critical function in AML expansion and progression in recent years (240). The mechanisms behind the capacity of AML cells to evade the immune system and induce systemic tolerance have been better understood (241). These tolerogens provide an immunosuppressive microenvironment that impairs anti-leukemia immune responses and decreases the effectiveness of both traditional and novel treatments (242). Clinically applicable novel medications, examples include immune checkpoint and macrophage checkpoint inhibitors, have emerged to target these pathways and enhance anti-tumor immunity (243). In the present clinical trials primarily focus on lineage-restricted antigens, but newer approaches, such as split and dual targeting, aim to target leukemia-specific intracellular antigens (244). Bispecific antibodies and adapter CAR-T cells offer temporary exposure, improved safety, and multitargeting capabilities against antigen-escape variants. Bispecific antibodies encompass several types, such as bispecific T-cell engagers (BiTEs), bispecific killer-cell engagers (BiKEs), dual-affinity retargeting antibodies (DARTs), and tandem diabodies (TandAbs). These antibodies feature two antigen recognition sites that help redirect tumor cells toward immune cells (245). To overcome the AML’s molecular variability and the inherent variety of AML blasts, a combined or sequential approach involving immunotherapy, chemotherapy, and molecular therapy is likely to be the most effective (246). This three-pronged strategy aims to control the disease and prevent relapse. To improve AML cure rates, it is also essential to develop new methods for tracking MRD and identifying potential recurring clones at an early stage, in addition to early response biomarkers and genomic profiling (247). Extending the utilization of CAR-T cells to target intracellular antigens could benefit a broader range of cancer patients. Despite this, clinical trials for immunotherapy and chemotherapy combinations are yet in their early years, these treatments hold promise for AML patients. Through complement-dependent cytotoxicity, innate immune system activation, and ADCC, immune agents cause AML cell death (248, 249).
In the last ten years, single-cell technologies have undergone a dramatic evolution, producing a wealth of single-cell expression data that precisely delineates the transcriptomic topography of both cancerous and healthy cells (250). This rich data reservoir, largely unexplored, is capable of creating new treatments, especially in the background of CAR-T cell development and novel antigen predictions (251). These advancements facilitate precise on- and off-tumor antigen predictions, providing unparalleled resolution and distinct perspectives into both malignant and healthy cells. For example, the AML antigens CD33 and CD123 did not meet our stringent overexpression standards for malignant hematopoietic stem and progenitor cells (HSPCs), most likely because of their expression in healthy HSPCs as well (80, 252). Furthermore, endothelial and other lung cell types exhibited high CD123 expression levels, potentially leading to on-target off-tumor toxicity. An investigation was conducted using a group of 15 AML patients to find potential antigens for CAR-T cell treatment. In order to accomplish this, a technique called single-cell RNA sequencing (scRNA-seq) (198, 252). A comprehensive transcriptome atlas was created by utilizing publicly accessible datasets. This atlas includes more than 28,000 malignant and healthy BM cells taken from patients, as well as over 500,000 healthy cells from nine important human tissues (252). The atlas underwent screening to identify cell surface antigens that are expressed on cancerous cells but have low expression on healthy cells, specifically T lymphocytes (253). Through the use of stringent criteria, researchers have successfully identified two CAR-T cell targets in AML that were previously unknown: CD86 and the colony-stimulating factor 1 receptor (CSF1R) (254, 255). CAR-T cells were produced against both targets and assessed for effectiveness using primary AML blasts and other patient-derived models, both in vitro and in vivo (252). For in vitro safety studies, we used advanced primary cell cultures of cell types that express the target. These cultures showed a higher ability to distinguish between different cell types compared to well-known anti-CD33 CAR-T cells (252). To address concerns regarding safety, various in vivo models were employed. These results establish the foundation for the clinical advancement of the CAR candidates and emphasize the potential for practical application of an objective scRNA-seq-based screening technology (252).
Recent innovations in immune-based therapies for AML concentrate on utilizing the immune system to tackle the disease. These approaches focus on targeting intrinsic and surface antigens of cancer cells, additionally modifying the leukemic microenvironment to reduce immune evasion like HLA loss and T-cell exhaustion during cancer progression (256). For example, in study on dual CAR-T, a new combination platform of twofold aiming by an antibody-T cell receptor (AbTCR) and a chimeric costimulatory signaling receptor (CSR) to two different antigens, wherein the cancer cells express both antigens simultaneously, but not together on normal cells. In this study two different antigens Wilm’s tumor 1 protein (WT1) and CD33 were targeted that both are highly expressed on most AML cells. These data suggest that this amalgamation of a AbTCR CAR and CSR might work well as a tactic to lessen toxicity and enhance specificity and clinical results in adoptive T cell therapy in AML (257). So, the search for novel targets and therapies for AML underscores the necessity for innovative strategies. Integrating single-cell technologies and computational modeling presents promising pathways for identifying effective CAR-T cell targets. A multi-faceted approach combining immunotherapy, chemotherapy, and advanced tracking methods is crucial for improving treatment efficacy and minimizing relapse rates.
Advancements in genomics and precision medicine have profoundly shaped the strategic selection of targets for immune therapies, revealing intricate genetic and molecular foundations of diseases, notably cancer. This knowledge permits the precise identification of particular genetic aberrations and molecular routes susceptible to immune therapies, increasing their effectiveness while reducing off-target effects. The employment of high-throughput sequencing technologies enables comprehensive analysis of tumor genomes, which identifies tumor-specific modifications that give rise to neoantigens. These neoantigens serve as optimal targets for immune interventions because of their tumor specificity and recognition by the immune system as foreign entities (258). Furthermore, computational tools leveraging genomic data proficiently predict potential neoantigenic mutations based on the affinity of altered peptides for major histocompatibility complex (MHC) molecules, essential for antigen presentation to T cells (259). A notable correlation can be observed between high tumor mutational burden (TMB) and the proliferation of neoantigens, positioning tumors with elevated TMB as prime candidates for immune checkpoint inhibitors. Genomic sequencing quantifies TMB, thus directing the deployment of these inhibitors. For example, elevated TMB is linked with enhanced responses to checkpoint inhibitors since mutation-rich tumors are more likely to generate recognizable neoantigens (260). Additionally, microsatellite instability (MSI) suggests a heightened mutational load and an abundance of neoantigens, rendering such tumors suitable for immune therapy (261). Genomic assessments can pinpoint mutations essential for the survival of cancer cells (oncogenic drivers). Attacking these drivers with immune therapies through direct targeting or blocking reliant pathways has proven efficacious. For example, although direct drug targeting of KRAS gene mutations has been challenging, immune therapies tailored to attack KRAS-mutant cells are under development. Integrating genomics into precision medicine also aids in unearthing novel immune targets by analyzing the genetic landscape of tumors (262). Identifying mutations in pathways such as the JAK/STAT pathway has catalyzed the development of therapies that enhance immune checkpoint blockade efficacy by thwarting immune evasion mechanisms (263). Advances in transcriptomics have further enabled the exploration of gene expression in the tumor microenvironment, revealing the presence and activity of immune cells such as T cells, macrophages, and regulatory T cells. This information will help with the decision-making of appropriate immune therapies, including checkpoint inhibitors or adoptive cell therapies (227, 264). Single-cell sequencing technology, which analyzes gene expression at the cellular level within the tumor microenvironment, assists in pinpointing specific cell populations that may promote or inhibit an immune response, thereby guiding immune modulator selection (265). Furthermore, genomics has facilitated the creation of biomarkers that guide the selection and fine-tuning of immune therapies. Biomarkers such as MSI and specific gene expression profiles tailor immune therapies to individual patients, ensuring that treatments are optimally matched to their unique genetic profiles (266). Precision medicine employs genomic insights to categorize patients by the molecular attributes of their tumors-this stratification aids in selecting optimal immune therapies that enhance effectiveness and diminish side effects (267). Personalized cancer vaccines, which are designed to target unique neoantigens within a patient’s tumor, provoke specific immune reactions. However, some tumors have evolved resistance to these therapies. Genomic analysis exposes resistance mechanisms, such as mutations that disrupt antigen presentation or elevate immune-suppressive pathways. Comprehending these mechanisms fosters the development of combination therapies that triumph overy such resistance. The integration of genomic information with immune profiling enables the development of multifaceted therapies. These therapies address various elements of tumor biology, thereby improving clinical outcomes and lessening unwanted effects (268, 269). However, despite these advances, significant challenges remain in applying genomics and precision medicine in selecting immune therapy targets (266). The inherent heterogeneity of tumors and the dynamic interactions within the immune system and tumor microenvironment often hinder the consistent identification of stable, effective targets (270).
6 Conclusion
In conclusion, this work highlights the promising potential of various immunotherapeutic strategies in targeting AML while emphasizing the need for a more thorough comprehension of the tumor microenvironment and its interactions with immune cells. In the past three to five years, significant advancements in AML immunology, coupled with technological breakthroughs, have led to innovative therapeutic strategies for AML-targeted T cells. Despite the plethora of continuing investigations, T-cell immunotherapies for myeloid malignancies continue to be available in their nascent stages, poised to evolve and refine AML immunotherapeutic in the coming years. A targeted investigation of biomarkers at various phases-pre-therapy, on-therapy, and relapse—will accelerate clinical advancements, improve immune toxicity management, validate new checkpoints and AML-specific targets, elucidate mechanisms of immune resistance, and identify likely responders. Identifying and implementing these treatments in optimal clinical settings, such as MRD and low-mortality illness, will be essential. Innovative techniques like mass cytometry, single-cell RNA and DNA sequencing, and single-cell cytokine analysis will provide critical insights into non-T-cell compartments in immune responses and tumor microenvironments, guiding sequential or combinatorial immune therapy strategies. Overall, this comprehensive understanding of immunotherapy’s role in AML, alongside biomarker-guided strategies, positions us for an exciting and potentially fruitful decade ahead for AML immunotherapies.
Author contributions
AHK: Writing – original draft. SH: Writing – original draft. MHY: Writing – original draft. SM: Writing – original draft. BM: Writing – review & editing. MS: Writing – review & editing. MK: Conceptualization, Supervision, Writing – review & editing.
Funding
The author(s) declare that no financial support was received for the research, authorship, and/or publication of this article.
Acknowledgments
I want to express my deepest gratitude to my two-year-old son, Ryan, for his patience and understanding during the time it took to complete this research. His joyful presence has been a constant source of inspiration, and I am truly thankful for the moments he allowed me to dedicate to this work. This achievement would not have been possible without his love and support. To ensure the highest quality of writing, this article used Wordtune software to refine the language, improve clarity, enhance readability, and perform spelling and grammar checks. Finally, the entire article was reviewed and approved by the authors.
Conflict of interest
The authors declare that the research was conducted in the absence of any commercial or financial relationships that could be construed as a potential conflict of interest.
Publisher’s note
All claims expressed in this article are solely those of the authors and do not necessarily represent those of their affiliated organizations, or those of the publisher, the editors and the reviewers. Any product that may be evaluated in this article, or claim that may be made by its manufacturer, is not guaranteed or endorsed by the publisher.
Glossary
ADCC: Antibody-dependent cell-mediated cytotoxicity
ALL: Acute lymphoblastic leukemia
AML: Acute myeloid leukemia
BCL-2: B-cell Lymphoma 2
BCMA: B-cell maturation antigen
CAR: Chimeric antigen receptor
cCAR: Compound CAR
CD: Cluster of differentiation
CLEC12A: C-type lectin domain family 12 member A
CLL1: C-type lectin-like molecule-1
CML: Chronic myeloid leukemia
CRS: Cytokine release syndrome
CSF1R: Colony-Stimulating Factor 1 Receptor
CTAs: Cancer-testis antigens
CTL: Cytotoxic T lymphocyte
CTLA-4: Cytotoxic T-lymphocyte-associated protein 4
CXCL12: C-X-C motif chemokine ligand 12
DC: Dendritic cells
DLBCL: Diffuse large B-cell lymphoma
FLT3: Fms-like tyrosine kinase 3
FMNL1: Formin-like protein 1
G-CSF: Granulocyte colony-stimulating factor
GVHD: Graft-versus-Host Disease
HA-1: Minor histocompatibility antigen A-1
HLA: Human leukocyte antigen
HMMR/Rhamm: Hyaluronan-mediated motility receptor
HSC: Hematopoietic stem cell
HSCT: Hematopoietic stem cell transplantation
IDH: Isocitrate Dehydrogenase
IDO: Indoleamine 2,3-dioxygenase
IFN: Interferon
IFN-γ: Interferon-gamma
IL: Interleukin
KIRs: Killer cell immunoglobulin-like receptors
LAA: Leukemia-associated antigen
LAG-3: Lymphocyte-activation gene 3
LILRB4: Leukocyte immunoglobulin-like receptor B4
LSA: Leukemia-specific antigen
LSC: Leukemia stem cell
MDS: Myelodysplastic syndrome
MDSC: Myeloid-derived suppressor cells
MHC: Major histocompatibility complex
MM: Multiple myeloma
MRD: Minimal Residual Disease
NK: Natural Killer
NKG2D: Natural killer group 2, member D
PBMC: Peripheral blood mononuclear cell
PD-1: Programmed cell death protein 1
PD-L1: Programmed death-ligand 1
PGE2: Prostaglandin E2
ROS: Reactive oxygen species
Siglec-6: Sialic acid-binding Ig-like lectin 6
TAA: Tumor-associated antigen
TCR: T-cell receptor
TERT: Telomerase reverse transcriptase
TGF-β: Transforming growth factor-beta
TIM3: T cell immunoglobulin and mucin-domain containing 3
TNF-α: Tumor necrosis factor-alpha
Treg: Regulatory T-cells
TSCM: T-memory stem cells
WT1: Wilms’ Tumor
References
1. Turkalj S, Radtke FA, Vyas P. An overview of targeted therapies in acute myeloid leukemia. Hemasphere. (2023) 7:e914. doi: 10.1097/HS9.0000000000000914
2. Campo E, Swerdlow SH, Harris NL, Pileri S, Stein H, Jaffe ES. The 2008 WHO classification of lymphoid neoplasms and beyond: evolving concepts and practical applications. Blood. (2011) 117:5019–32. doi: 10.1182/blood-2011-01-293050
3. Kavianpour M, Ahmadzadeh A, Shahrabi S, Saki N. Significance of oncogenes and tumor suppressor genes in AML prognosis. Tumor Biol. (2016) 37:10041–52. doi: 10.1007/s13277-016-5067-1
4. Visani G, Chiarucci M, Paolasini S, Loscocco F, Isidori A. Treatment options for acute myeloid leukemia patients aged <60 years. Front Oncol. (2022) 12:897220. doi: 10.3389/fonc.2022.897220
5. Krawiec K, Strzałka P, Czemerska M, Wiśnik A, Zawlik I, Wierzbowska A, et al. Targeting apoptosis in AML: where do we stand? Cancers (Basel). (2022) 14(20):4995. doi: 10.3390/cancers14204995
6. Wang Q, Poole RA, Opyrchal M. Understanding and targeting erythroid progenitor cells for effective cancer therapy. Curr Opin Hematol. (2023) 30:137–43. doi: 10.1097/MOH.0000000000000762
7. Bain BJ, Béné MC. Morphological and immunophenotypic clues to the WHO categories of acute myeloid leukemia. Acta Hematol. (2019) 141:232–44. doi: 10.1159/000496097
8. Moarii M, Papaemmanuil E. Classification and risk assessment in AML: integrating cytogenetics and molecular profiling. Hematol Am Soc Hematol Educ Program. (2017) 2017:37–44. doi: 10.1182/asheducation-2017.1.37
9. Khoury JD, Solary E, Abla O, Akkari Y, Alaggio R, Apperley JF, et al. The 5th edition of the World Health Organization classification of hematolymphoid tumors: myeloid and histiocytic/dendritic neoplasms. Leukemia. (2022) 36(7):1703–19. doi: 10.1038/s41375-022-01613-1
10. Wang M, Rao J, Wen Q, Chen G, Tan X, Ma Y, et al. PB1765: Efficacy of a new induction regimen in the treatment of early t-cell precursor acute lymphoblastic leukemia. HemaSphere. (2022) 6:1646–7. doi: 10.1097/01.HS9.0000849916.02538.70
11. Zhu JF, Dai HP, Zhang QQ, Yin J, Li Z, Cui QY, et al. Efficacy and safety of decitabine combined with HAAG (homoharringtonine, aclarubicin, low-dose cytarabine and G-CSF) for newly diagnosed acute myeloid leukemia. Front Oncol. (2022) 12:998884. doi: 10.3389/fonc.2022.998884
12. Gediga MHE, Middeke JM, Ruhnke L. Novel strategies to treat acute myeloid leukemia. Dtsch Med Wochenschr. (2023) 148:451–8. doi: 10.1055/a-1873-4753
13. Algeri M, et al. The role of allogeneic hematopoietic stem cell transplantation in pediatric leukemia. J Clin Med. (2021) 10:37–90. doi: 10.3390/jcm10173790
14. Anand U, Dey A, Chandel AKS, Sanyal R, Mishra A, Pandey DK, et al. Cancer chemotherapy and beyond: Current status, drug candidates, associated risks and progress in targeted therapeutics. Genes Dis. (2023) 10:1367–401. doi: 10.1016/j.gendis.2022.02.007
15. Huang X, Lin H, Huang F, Xie Y, Wong KH, Chen X, et al. Targeting approaches of nanomedicines in acute myeloid leukemia. Dose Response. (2019) 17:1559325819887048. doi: 10.1177/1559325819887048
16. Vaughn JE, Buckley SA, Walter RB. Outpatient care of patients with acute myeloid leukemia: Benefits, barriers, and future considerations. Leuk Res. (2016) 45:53–8. doi: 10.1016/j.leukres.2016.03.011
17. Molica M, Breccia M, Foa R, Jabbour E, Kadia TM. Maintenance therapy in AML: The past, the present and the future. Am J Hematol. (2019) 94(11):1254–65. doi: 10.1002/ajh.25620
18. Döhner H, Estey E, Grimwade D, Amadori S, Appelbaum FR, Büchner T, et al. Diagnosis and management of AML in adults: 2017 ELN recommendations from an international expert panel. Blood. (2017) 129:424–47. doi: 10.1182/blood-2016-08-733196
19. Terwilliger T, Abdul-Hay M. Acute lymphoblastic leukemia: a comprehensive review and 2017 update. Blood Cancer J. (2017) 7:e577. doi: 10.1038/bcj.2017.53
20. Hirsch P, Tang R, Abermil N, Flandrin P, Moatti H, Favale F, et al. Precision and prognostic value of clone-specific minimal residual disease in acute myeloid leukemia. Hematologica. (2017) 102:1227–37. doi: 10.3324/haematol.2016.159681
21. Rashidi A, Weisdorf DJ, Bejanyan N. Treatment of relapsed/refractory acute myeloid leukemia in adults. Br J Hematol. (2018) 181:27–37. doi: 10.1111/bjh.15077
22. Zhang Y, Zhang Z. The history and advances in cancer immunotherapy: understanding the characteristics of tumor-infiltrating immune cells and their therapeutic implications. Cell Mol Immunol. (2020) 17:807–21. doi: 10.1038/s41423-020-0488-6
23. Kruger S, et al. Advances in cancer immunotherapy 2019 – latest trends. J Exp Clin Cancer Res. (2019) 38:268. doi: 10.1186/s13046-019-1266-0
24. Sahu M, Suryawanshi H. Immunotherapy: The future of cancer treatment. J Oral Maxillofac Pathol. (2021) 25:371. doi: 10.4103/0973-029X.325257
25. Kazemi T, Younesi V, Jadidi-Niaragh F, Yousefi M. Immunotherapeutic approaches for cancer therapy: an updated review. Artif Cells Nanomed Biotechnol. (2016) 44:769–79. doi: 10.3109/21691401.2015.1019669
26. Dong Y, Wan Z, Gao X, Yang G, Liu L. Reprogramming immune cells for enhanced cancer immunotherapy: targets and strategies. Front Immunol. (2021) 12:609762. doi: 10.3389/fimmu.2021.609762
27. Buckley SA, Walter RB. Update on antigen-specific immunotherapy of acute myeloid leukemia. Curr Hematologic Malignancy Rep. (2015) 10:65–75. doi: 10.1007/s11899-015-0250-9
28. Zhang Z, Lu M, Qin Y, Gao W, Tao L, Su W, et al. Neoantigen: A new breakthrough in tumor immunotherapy. Front Immunol. (2021) 12:672356. doi: 10.3389/fimmu.2021.672356
29. Takami A. Hematopoietic stem cell transplantation for acute myeloid leukemia. Int J Hematol. (2018) 107:513–8. doi: 10.1007/s12185-018-2412-8
30. Roshandel E, Tavakoli F, Parkhideh S, Akhlaghi SS, Ardakani MT, Soleimani M. Post-hematopoietic stem cell transplantation relapse: Role of checkpoint inhibitors. Health Sci Rep. (2022) 5:e536. doi: 10.1002/hsr2.536
31. Chergui A, Reagan JL. Immunotherapy in acute leukemias: past success paves the way for future progress. Cancers (Basel). (2023) 15:4137. doi: 10.3390/cancers15164137
32. Haslauer T, Greil R, Zaborsky N, Geisberger R. CAR T-cell therapy in hematological Malignancies. Int J Mol Sci. (2021) 22:8996. doi: 10.3390/ijms22168996
33. Leotta S, Condorelli A, Sciortino R, Milone GA, Bellofiore C, Garibaldi B, et al. Prevention and treatment of acute myeloid leukemia relapse after hematopoietic stem cell transplantation: the state of the art and future perspectives. J Clin Med. (2022) 11:253. doi: 10.3390/jcm11010253
34. Vishwasrao P, Li G, Boucher JC, Smith DL, Hui SK. Emerging CAR T cell strategies for the treatment of AML. Cancers (Basel). (2022) 14:12–41. doi: 10.3390/cancers14051241
35. Atilla E, Benabdellah K. The black hole: CAR T cell therapy in AML. Cancers (Basel). (2023) 15:2713. doi: 10.3390/cancers15102713
36. Vanhooren J, Dobbelaere R, Derpoorter C, Deneweth L, Van Camp L, Uyttebroeck A, et al. CAR-T in the treatment of acute myeloid leukemia: barriers and how to overcome them. Hemasphere. (2023) 7:e937. doi: 10.1097/HS9.0000000000000937
37. Lydeard JR, Lin MI, Ge HG, Halfond A, Wang S, Jones MB, et al. Development of a gene edited next-generation hematopoietic cell transplant to enable acute myeloid leukemia treatment by solving off-tumor toxicity. Mol Ther Methods Clin Dev. (2023) 31:101135. doi: 10.1016/j.omtm.2023.101135
38. Huang Z, Dewanjee S, Chakraborty P, Jha NK, Dey A, Gangopadhyay M, et al. CAR T cells: engineered immune cells to treat brain cancers and beyond. Mol Cancer. (2023) 22:22. doi: 10.1186/s12943-022-01712-8
39. Schorr C, Perna F. Targets for chimeric antigen receptor T-cell therapy of acute myeloid leukemia. Front Immunol. (2022) 13:1085978. doi: 10.3389/fimmu.2022.1085978
40. Wei W, Yang D, Chen X, Liang D, Zou L, Zhao X. Chimeric antigen receptor T-cell therapy for T-ALL and AML. Front Oncol. (2022) 12:967754. doi: 10.3389/fonc.2022.967754
41. He X, Feng Z, Ma J, Ling S, Cao Y, Gurung B, et al. Bispecific and split CAR T cells targeting CD13 and TIM3 eradicate acute myeloid leukemia. Blood. (2020) 135:713–23. doi: 10.1182/blood.2019002779
42. Sauer T, Parikh K, Sharma S, Omer B, Sedloev D, Chen Q, et al. CD70-specific CAR T cells have potent activity against acute myeloid leukemia without HSC toxicity. Blood. (2021) 138:318–30. doi: 10.1182/blood.2020008221
43. Xia B, Lin K, Wang X, Chen F, Zhou M, Li Y, et al. Nanobody-derived bispecific CAR-T cell therapy enhances the anti-tumor efficacy of T cell lymphoma treatment. Mol Ther Oncolytics. (2023) 30:86–102. doi: 10.1016/j.omto.2023.07.007
44. Michelozzi IM, Kirtsios E, Giustacchini A. Driving CAR T stem cell targeting in acute myeloid leukemia: the roads to success. Cancers (Basel). (2021) 13:2816. doi: 10.3390/cancers13112816
45. Gomes-Silva D, Atilla E, Atilla PA, Mo F, Tashiro H, Srinivasan M, et al. CD7 CAR T cells for the therapy of acute myeloid leukemia. Mol Ther. (2019) 27:272–80. doi: 10.1016/j.ymthe.2018.10.001
46. Gomes-Silva D, Srinivasan M, Sharma S, Lee CM, Wagner DL, Davis TH, et al. CD7-edited T cells expressing a CD7-specific CAR for the therapy of T-cell Malignancies. Blood. (2017) 130:285–96. doi: 10.1182/blood-2017-01-761320
47. Francis J, Dharmadhikari AV, Sait SN, Deeb G, Wallace PK, Thompson JE, et al. CD19 expression in acute leukemia is not restricted to the cytogenetically aberrant populations. Leukemia Lymphoma. (2013) 54:1517–20. doi: 10.3109/10428194.2012.754096
48. Wang B, Yang B, Ling Y, Zhang J, Hua X, Gu W, et al. Role of CD19 and specific KIT-D816 on risk stratification refinement in t(8;21) acute myeloid leukemia induced with different cytarabine intensities. Cancer Med. (2021) 10:1091–102. doi: 10.1002/cam4.3705
49. Itoh-Nakadai A, Saito Y, Murasawa-Tomizawa M, Kajita H, Matsumoto T, Matsuda M, et al. CXCR4-expressing anti-CD25 CAR T-cells effectively eliminate human AML cells in vivo. Blood. (2020) 136:35–6. doi: 10.1182/blood-2020-142228
50. Peng Y, Tao Y, Zhang Y, Wang J, Yang J, Wang Y. CD25: A potential tumor therapeutic target. Int J Cancer. (2023) 152:1290–303. doi: 10.1002/ijc.34281
51. Pousse L, Korfi K, Medeiros BC, Berrera M, Kumpesa N, Eckmann J, et al. CD25 targeting with the afucosylated human IgG1 antibody RG6292 eliminates regulatory T cells and CD25+ blasts in acute myeloid leukemia. Front Oncol. (2023) 13:1150149. doi: 10.3389/fonc.2023.1150149
52. Rafiq S, Purdon TJ, Schultz LM, Brentjens RJ. CD33-directed chimeric antigen receptor (CAR) T cells for the treatment of acute myeloid leukemia (AML). Blood. (2016) 128:2825–5. doi: 10.1182/blood.V128.22.2825.2825
53. Kenderian SS, Ruella M, Shestova O, Klichinsky M, Aikawa V, Morrissette JJ, et al. CD33-specific chimeric antigen receptor T cells exhibit potent preclinical activity against human acute myeloid leukemia. Leukemia. (2015) 29:1637–47. doi: 10.1038/leu.2015.52
54. Kügler M, Stein C, Kellner C, Mentz K, Saul D, Schwenkert M, et al. A recombinant trispecific single-chain Fv derivative directed against CD123 and CD33 mediates effective elimination of acute myeloid leukemia cells by dual targeting. Br J Hematol. (2010) 150:574–86. doi: 10.1111/j.1365-2141.2010.08300.x
55. Hauswirth AW, Florian S, Printz D, Sotlar K, Krauth MT, Fritsch G, et al. Expression of the target receptor CD33 in CD34+/CD38–/CD123+ AML stem cells. Eur J Clin Invest. (2007) 37:73–82. doi: 10.1111/j.1365-2362.2007.01746.x
56. Ehninger A, Kramer M, Röllig C, Thiede C, Bornhäuser M, von Bonin M, et al. Distribution and levels of cell surface expression of CD33 and CD123 in acute myeloid leukemia. Blood Cancer J. (2014) 4:e218–8. doi: 10.1038/bcj.2014.39
57. Quek L, Otto GW, Garnett C, Lhermitte L, Karamitros D, Stoilova B, et al. Genetically distinct leukemic stem cells in human CD34– acute myeloid leukemia are arrested at a hemopoietic precursor-like stage. J Exp Med. (2016) 213:1513–35. doi: 10.1084/jem.20151775
58. Engelhardt M, Lübbert M, Guo Y. CD34+ or CD34–: which is the more primitive? Leukemia. (2002) 16:1603–8. doi: 10.1038/sj.leu.2402620
59. Nielsen JS, McNagny KM. Novel functions of the CD34 family. J Cell Sci. (2008) 121:3683–92. doi: 10.1242/jcs.037507
60. Gurney M, Stikvoort A, Nolan E, Kirkham-McCarthy L, Khoruzhenko S, Shivakumar R, et al. CD38 knockout natural killer cells expressing an affinity optimized CD38 chimeric antigen receptor successfully target acute myeloid leukemia with reduced effector cell fratricide. Hematologica. (2022) 107:437–45. doi: 10.3324/haematol.2020.271908
61. Cui Q, Qian C, Xu N, Kang L, Dai H, Cui W, et al. CD38-directed CAR-T cell therapy: a novel immunotherapy strategy for relapsed acute myeloid leukemia after allogeneic hematopoietic stem cell transplantation. J Hematol Oncol. (2021) 14:82. doi: 10.1186/s13045-021-01092-4
62. Yoshida T, Mihara K, Takei Y, Yanagihara K, Kubo T, Bhattacharyya J, et al. All-trans retinoic acid enhances cytotoxic effect of T cells with an anti-CD38 chimeric antigen receptor in acute myeloid leukemia. Clin Trans Immunol. (2016) 5:e116. doi: 10.1038/cti.2016.73
63. Casucci M, Nicolis di Robilant B, Falcone L, Camisa B, Norelli M, Genovese P, et al. CD44v6-targeted T cells mediate potent antitumor effects against acute myeloid leukemia and multiple myeloma. Blood. (2013) 122:3461–72. doi: 10.1182/blood-2013-04-493361
64. Ponta H, Sherman L, Herrlich PA. CD44: From adhesion molecules to signaling regulators. Nat Rev Mol Cell Biol. (2003) 4:33–45. doi: 10.1038/nrm1004
65. Gadhoum Z, Leibovitch MP, Qi J, Dumenil D, Durand L, Leibovitch S, et al. CD44: a new means to inhibit acute myeloid leukemia cell proliferation via p27Kip1. Blood. (2004) 103:1059–68. doi: 10.1182/blood-2003-04-1218
66. Song G, Liao X, Zhou L, Wu L, Feng Y, Han ZC. HI44a, an anti-CD44 monoclonal antibody, induces differentiation and apoptosis of human acute myeloid leukemia cells. Leuk Res. (2004) 28:1089–96. doi: 10.1016/j.leukres.2004.02.005
67. Vey N, Delaunay J, Martinelli G, Fiedler W, Raffoux E, Prebet T, et al. Phase I clinical study of RG7356, an anti-CD44 humanized antibody, in patients with acute myeloid leukemia. Oncotarget. (2016) 7:32532–42. doi: 10.18632/oncotarget.v7i22
68. Kersten B, Valkering M, Wouters R, van Amerongen R, Hanekamp D, Kwidama Z, et al. CD45RA, a specific marker for leukemia stem cell sub-populations in acute myeloid leukemia. Br J Hematol. (2016) 173:219–35. doi: 10.1111/bjh.13941
69. Majeti R, Chao MP, Alizadeh AA, Pang WW, Jaiswal S, Gibbs KD Jr, et al. CD47 is an adverse prognostic factor and therapeutic antibody target on human acute myeloid leukemia stem cells. Cell. (2009) 138:286–99. doi: 10.1016/j.cell.2009.05.045
70. Liu J, Wang L, Zhao F, Tseng S, Narayanan C, Shura L, et al. Pre-clinical development of a humanized anti-CD47 antibody with anti-cancer therapeutic potential. PloS One. (2015) 10:e0137345. doi: 10.1371/journal.pone.0137345
71. Petrova PS, Viller NN, Wong M, Pang X, Lin GH, Dodge K, et al. TTI-621 (SIRPαFc): A CD47-blocking innate immune checkpoint inhibitor with broad antitumor activity and minimal erythrocyte binding. Clin Cancer Res. (2017) 23:1068–79. doi: 10.1158/1078-0432.CCR-16-1700
72. Yan B, Chen Q, Shimada K, Tang M, Li H, Gurumurthy A, et al. Histone deacetylase inhibitor targets CD123/CD47-positive cells and reverse chemoresistance phenotype in acute myeloid leukemia. Leukemia. (2019) 33:931–44. doi: 10.1038/s41375-018-0279-6
73. Theocharides AP, Jin L, Cheng PY, Prasolava TK, Malko AV, Ho JM, et al. Disruption of SIRPα signaling in macrophages eliminates human acute myeloid leukemia stem cells in xenografts. J Exp Med. (2012) 209:1883–99. doi: 10.1084/jem.20120502
74. Ponce LP, Fenn NC, Moritz N, Krupka C, Kozik JH, Lauber K, et al. SIRPα-antibody fusion proteins stimulate phagocytosis and promote elimination of acute myeloid leukemia cells. Oncotarget. (2017) 8:11284–301. doi: 10.18632/oncotarget.v8i7
75. Sallman DA, Asch AS, Al Malki MM, Lee DJ, Donnellan WB, Marcucci G, et al. The first-in-class anti-CD47 antibody magrolimab (5F9) in combination with azacitidine is effective in MDS and AML patients: ongoing phase 1b results. Blood. (2019) 134:569. doi: 10.1182/blood-2019-126271
76. Zhao J, Zhang H-T. Effect of CD56 expression on prognosis of AML patients with AML/ETO mutation. Zhongguo shi yan xue ye xue za zhi. (2020) 28:63–7. doi: 10.19746/j.cnki.issn.1009-2137.2020.01.011
77. Riether C, Pabst T, Höpner S, Bacher U, Hinterbrandner M, Banz Y, et al. Targeting CD70 with cusatuzumab eliminates acute myeloid leukemia stem cells in patients treated with hypomethylating agents. Nat Med. (2020) 26:1459–67. doi: 10.1038/s41591-020-0910-8
78. Leick MB, Silva H, Scarfò I, Larson R, Choi BD, Bouffard AA, et al. Rational chemical and genetic modifications enhance avidity and function of CD70-directed CAR-T-cells for myeloid leukemia. Blood. (2021) 138:405–5. doi: 10.1182/blood-2021-150596
79. Myburgh R, Kiefer JD, Russkamp NF, Magnani CF, Nuñez N, Simonis A, et al. Anti-human CD117 CAR T-cells efficiently eliminate healthy and Malignant CD117-expressing hematopoietic cells. Leukemia. (2020) 34:2688–703. doi: 10.1038/s41375-020-0818-9
80. Boucher JC, Shrestha B, Vishwasrao P, Leick M, Cervantes EV, Ghafoor T, et al. Bispecific CD33/CD123 targeted chimeric antigen receptor T cells for the treatment of acute myeloid leukemia. Mol Ther Oncolytics. (2023) 31:100751. doi: 10.1016/j.omto.2023.100751
81. Lamble AJ, Eidenschink Brodersen L, Alonzo TA, Wang J, Pardo L, Sung L, et al. CD123 expression is associated with high-risk disease characteristics in childhood acute myeloid leukemia: A report from the children’s oncology group. J Clin Oncol. (2022) 40:252–61. doi: 10.1200/JCO.21.01595
82. Boucher JC, Shrestha B, Vishwasrao P, Leick MB, Tu N, Ghafoor T, et al. Bispecific CD33/CD123 targeted chimeric antigen receptor T cells for the treatment of acute myeloid leukemia. Blood. (2022) 140:10275–6. doi: 10.1182/blood-2022-163299
83. El Khawanky N, Hughes A, Yu W, Myburgh R, Matschulla T, Taromi S, et al. Demethylating therapy increases anti-CD123 CAR T cell cytotoxicity against acute myeloid leukemia. Nat Commun. (2021) 12:6436. doi: 10.1038/s41467-021-26683-0
84. Peinert S, Prince HM, Guru PM, Kershaw MH, Smyth MJ, Trapani JA, et al. Gene-modified T cells as immunotherapy for multiple myeloma and acute myeloid leukemia expressing the Lewis Y antigen. Gene Ther. (2010) 17:678–86. doi: 10.1038/gt.2010.21
85. Daver N, Alotaibi AS, Bücklein V, Subklewe M. T-cell-based immunotherapy of acute myeloid leukemia: current concepts and future developments. Leukemia. (2021) 35:1843–63. doi: 10.1038/s41375-021-01253-x
86. Ritchie DS, Neeson PJ, Khot A, Peinert S, Tai T, Tainton K, et al. Persistence and efficacy of second generation CAR T cell against the LeY antigen in acute myeloid leukemia. Mol Ther. (2013) 21:2122–9. doi: 10.1038/mt.2013.154
87. Lichtman EI, Du H, Shou P, Song F, Suzuki K, Ahn S, et al. Preclinical evaluation of B7-H3–specific chimeric antigen receptor T cells for the treatment of acute myeloid leukemia. Clin Cancer Res. (2021) 27:3141–53. doi: 10.1158/1078-0432.CCR-20-2540
88. Ma H, Padmanabhan IS, Parmar S, Gong Y. Targeting CLL-1 for acute myeloid leukemia therapy. J Hematol Oncol. (2019) 12:41. doi: 10.1186/s13045-019-0726-5
89. Tashiro H, Sauer T, Shum T, Parikh K, Mamonkin M, Omer B, et al. Treatment of acute myeloid leukemia with T cells expressing chimeric antigen receptors directed to C-type lectin-like molecule 1. Mol Ther. (2017) 25:2202–13. doi: 10.1016/j.ymthe.2017.05.024
90. Miao X, Shuai Y, Han Y, Zhang N, Liu Y, Yao H, et al. Case report: Donor-derived CLL-1 chimeric antigen receptor T-cell therapy for relapsed/refractory acute myeloid leukemia bridging to allogeneic hematopoietic stem cell transplantation after remission. Front Immunol. (2024) 15:1389227. doi: 10.3389/fimmu.2024.1389227
91. Kottaridis PD, Gale RE, Frew ME, Harrison G, Langabeer SE, Belton AA, et al. The presence of a FLT3 internal tandem duplication in patients with acute myeloid leukemia (AML) adds important prognostic information to cytogenetic risk group and response to the first cycle of chemotherapy: analysis of 854 patients from the United Kingdom Medical Research Council AML 10 and 12 trials. Blood. (2001) 98:1752–9. doi: 10.1182/blood.V98.6.1752
92. Wang Y, Xu Y, Li S, Liu J, Xing Y, Xing H, et al. Targeting FLT3 in acute myeloid leukemia using ligand-based chimeric antigen receptor-engineered T cells. J Hematol Oncol. (2018) 11:60. doi: 10.1186/s13045-018-0603-7
93. Gilliland DG, Griffin JD. The roles of FLT3 in hematopoiesis and leukemia. Blood. (2002) 100:1532–42. doi: 10.1182/blood-2002-02-0492
94. Safarzadeh Kozani P, Safarzadeh Kozani P, Rahbarizadeh F. Novel antigens of CAR T cell therapy: New roads; old destination. Trans Oncol. (2021) 14:101079. doi: 10.1016/j.tranon.2021.101079
95. Knapper S, Burnett AK, Littlewood T, Kell WJ, Agrawal S, Chopra R, et al. A phase 2 trial of the FLT3 inhibitor lestaurtinib (CEP701) as first-line treatment for older patients with acute myeloid leukemia not considered fit for intensive chemotherapy. Blood. (2006) 108:3262–70. doi: 10.1182/blood-2006-04-015560
96. Lam SS, Leung AY. Overcoming resistance to FLT3 inhibitors in the treatment of FLT3-mutated AML. Int J Mol Sci. (2020) 21:1537. doi: 10.3390/ijms21041537
97. Ågerstam H, Karlsson C, Hansen N, Sandén C, Askmyr M, von Palffy S, et al. Antibodies targeting human IL1RAP (IL1R3) show therapeutic effects in xenograft models of acute myeloid leukemia. Proc Natl Acad Sci. (2015) 112:10786–91. doi: 10.1073/pnas.1422749112
98. Shao R, Li Z, Xin H, Jiang S, Zhu Y, Liu J, et al. Biomarkers as targets for CAR-T/NK cell therapy in AML. biomark Res. (2023) 11:65. doi: 10.1186/s40364-023-00501-9
99. Stroopinsky D, Rosenblatt J, Ito K, Mills H, Yin L, Rajabi H, et al. MUC1 is a potential target for the treatment of acute myeloid leukemia stem cells. Cancer Res. (2013) 73:5569–79. doi: 10.1158/0008-5472.CAN-13-0677
100. Deeren D, Maertens JA, Lin T, Beguin Y, Demoulin B, Fontaine M, et al. First results from the dose escalation segment of the phase I clinical study evaluating cyad-02, an optimized non gene-edited engineered NKG2D CAR T-cell product, in relapsed or refractory acute myeloid leukemia and myelodysplastic syndrome patients. Blood. (2020) 136(Suppl 1):36. doi: 10.1182/blood-2020-139667
101. Jetani H, Navarro-Bailón A, Maucher M, Frenz S, Verbruggen C, Yeguas A, et al. Siglec-6 is a novel target for CAR T-cell therapy in acute myeloid leukemia. Blood. (2021) 138:1830–42. doi: 10.1182/blood.2020009192
102. Rafiq S, Purdon TJ, Daniyan AF, Koneru M, Dao T, Liu C, et al. Optimized T-cell receptor-mimic chimeric antigen receptor T cells directed toward the intracellular Wilms Tumor 1 antigen. Leukemia. (2017) 31:1788–97. doi: 10.1038/leu.2016.373
103. Steger B, Floro L, Amberger DC, Kroell T, Tischer J, Kolb HJ, et al. WT1, PRAME, and PR3 mRNA expression in acute myeloid leukemia (AML). J Immunotherapy. (2020) 43:204–15. doi: 10.1097/CJI.0000000000000322
104. Sugiyama H. WT1 (Wilms’ Tumor gene 1): biology and cancer immunotherapy. Japanese J Clin Oncol. (2010) 40:377–87. doi: 10.1093/jjco/hyp194
105. van Balen P, Jedema I, van Loenen MM, de Boer R, van Egmond HM, Hagedoorn RS, et al. HA-1H T-cell receptor gene transfer to redirect virus-specific T cells for treatment of hematological Malignancies after allogeneic stem cell transplantation: A phase 1 clinical study. Front Immunol. (2020) 11. doi: 10.3389/fimmu.2020.01804
106. van Loenen MM, de Boer R, van Liempt E, Meij P, Jedema I, Falkenburg JH, et al. A Good Manufacturing Practice procedure to engineer donor virus-specific T cells into potent anti-leukemic effector cells. Hematologica. (2014) 99:759–68. doi: 10.3324/haematol.2013.093690
107. Straetemans T, Kierkels GJJ, Doorn R, Jansen K, Heijhuurs S, Dos Santos JM, et al. GMP-grade manufacturing of T cells engineered to express a defined γδTCR. Front Immunol. (2018) 9:1062. doi: 10.3389/fimmu.2018.01062
108. Campillo-Davo D, Anguille S, Lion E. Trial watch: adoptive TCR-engineered T-cell immunotherapy for acute myeloid leukemia. Cancers (Basel). (2021) 13:4519. doi: 10.3390/cancers13184519
109. Kang S, Li Y, Qiao J, Meng X, He Z, Gao X, et al. Antigen-specific TCR-T cells for acute myeloid leukemia: state of the art and challenges. Front Oncol. (2022) 12:787108. doi: 10.3389/fonc.2022.787108
110. Klein C, Augsberger C, Xu W, Heitmüller C, Hanisch L, Sam J, et al. Targeting Intracellular WT1 in AML utilizing a T cell bispecific antibody construct: augmenting efficacy through combination with lenalidomide. Blood. (2019) 134:4450. doi: 10.1182/blood-2019-130121
111. Chapuis AG, Egan DN, Bar M, Schmitt TM, McAfee MS, Paulson KG, et al. T cell receptor gene therapy targeting WT1 prevents acute myeloid leukemia relapse post-transplant. Nat Med. (2019) 25:1064–72. doi: 10.1038/s41591-019-0472-9
112. Morris EC, Tendeiro-Rego R, Richardson R, Fox TA, Sillito F, Holler A, et al. A phase I study evaluating the safety and persistence of allorestricted WT1-TCR gene modified autologous T cells in patients with high-risk myeloid Malignancies unsuitable for allogeneic stem cell transplantation. Blood. (2019) 134:1367. doi: 10.1182/blood-2019-128044
113. Tawara I, Kageyama S, Miyahara Y, Fujiwara H, Nishida T, Akatsuka Y, et al. Safety and persistence of WT1-specific T-cell receptor gene–transduced lymphocytes in patients with AML and MDS. Blood. (2017) 130:1985–94. doi: 10.1182/blood-2017-06-791202
114. Chapuis AG, Ragnarsson GB, Nguyen HN, Chaney CN, Pufnock JS, Schmitt TM, et al. Transferred WT1-reactive CD8+ T cells can mediate antileukemic activity and persist in post-transplant patients. Sci Transl Med. (2013) 5:174ra27. doi: 10.1126/scitranslmed.3004916
115. Zhang L, Meng Y, Yao H, Zhan R, Chen S, Miao W, et al. CAR-NK cells for acute myeloid leukemia immunotherapy: past, present and future. Am J Cancer Res. (2023) 13:5559–76. doi: ajcr0152556
116. Zhang H, Gan WT, Hao WG, Wang PF, Li ZY, Chang LJ. Successful anti-CLL1 CAR T-cell therapy in secondary acute myeloid leukemia. Front Oncol. (2020) 10:685. doi: 10.3389/fonc.2020.00685
117. Zeng T, Cao Y, Jin T, Tian Y, Dai C, Xu F. The CD112R/CD112 axis: a breakthrough in cancer immunotherapy. J Exp Clin Cancer Res. (2021) 40:285. doi: 10.1186/s13046-021-02053-y
118. Kaito Y, Sugimoto E, Nakamura F, Tsukune Y, Sasaki M, Yui S, et al. Immune checkpoint molecule DNAM-1/CD112 axis is a novel target for natural killer-cell therapy in acute myeloid leukemia. Hematologica. (2024) 109:1107–20. doi: 10.3324/haematol.2023.282915
119. Zahran AM, Aly SS, Rayan A, El-Badawy O, Fattah MA, Ali AM, et al. Survival outcomes of CD34(+)CD38(-)LSCs and their expression of CD123 in adult AML patients. Oncotarget. (2018) 9:34056–65. doi: 10.18632/oncotarget.v9i75
120. Testa U, Pelosi E, Castelli G. CD123 as a therapeutic target in the treatment of hematological Malignancies. Cancers. (2019) 11:1358. doi: 10.3390/cancers11091358
121. Budde L, Song JY, Kim Y, Blanchard S, Wagner J, Stein AS, et al. Remissions of acute myeloid leukemia and blastic plasmacytoid dendritic cell neoplasm following treatment with CD123-specific CAR T cells: A first-in-human clinical trial. Blood. (2017) 130(Suppl 1):811. doi: 10.1182/blood.V130.Suppl_1.811.811
122. Loff S, Dietrich J, Meyer JE, Riewaldt J, Spehr J, von Bonin M, et al. Rapidly switchable universal CAR-T cells for treatment of CD123-positive leukemia. Mol Ther Oncolytics. (2020) 17:408–20. doi: 10.1016/j.omto.2020.04.009
123. Frazao A, Rethacker L, Messaoudene M, Avril MF, Toubert A, Dulphy N, et al. NKG2D/NKG2-ligand pathway offers new opportunities in cancer treatment. Front Immunol. (2019) 10:661. doi: 10.3389/fimmu.2019.00661
124. Baumeister SH, Murad J, Werner L, Daley H, Trebeden-Negre H, Gicobi JK, et al. Phase I trial of autologous CAR T cells targeting NKG2D ligands in patients with AML/MDS and multiple myeloma. Cancer Immunol Res. (2019) 7:100–12. doi: 10.1158/2326-6066.CIR-18-0307
125. Sallman DA, Kerre T, Poire X, Havelange V, Lewalle P, Davila ML, et al. Remissions in relapse/refractory acute myeloid leukemia patients following treatment with NKG2D CAR-T therapy without a prior preconditioning chemotherapy. Blood. (2018) 132(Suppl 1):902–2. doi: 10.1182/blood-2018-99-111326
126. Wei F, Cheng XX, Xue JZ, Xue SA. Emerging strategies in TCR-engineered T cells. Front Immunol. (2022) 13:850358. doi: 10.3389/fimmu.2022.850358
127. Zhang Y, Li Y. T cell receptor-engineered T cells for leukemia immunotherapy. Cancer Cell Int. (2019) 19:2. doi: 10.1186/s12935-018-0720-y
128. Lazzarotto D, Candoni A. The role of wilms’ Tumor gene (WT1) expression as a marker of minimal residual disease in acute myeloid leukemia. J Clin Med. (2022) 11:3306. doi: 10.3390/jcm11123306
129. Mehralizadeh H, Aliparasti MR, Talebi M, Salekzamani S, Almasi S, et al. WT-1, BAALC, and ERG expressions in Iranian patients with acute myeloid leukemia pre- and post-chemotherapy. Adv Pharm Bull. (2021) 11:197–203. doi: 10.34172/apb.2021.021
130. Tawara I, Kageyama S, Miyahara Y, Fujiwara H, Nishida T, Akatsuka Y, et al. Safety and persistence of WT1-specific T-cell receptor gene-transduced lymphocytes in patients with AML and MDS. Blood. (2017) 130:1985–94. doi: 10.1182/blood-2017-06-791202
131. Kirkey D, Robinson L, Hylkema T, Loeb A, Castro S, Tang T, et al. Preclinical evaluation of targeting PRAME with TCR mimic CAR T cells in AML. J Clin Oncol. (2023) 41:2553–3. doi: 10.1200/JCO.2023.41.16_suppl.2553
132. Al Malki MM, Vasu S, Modi D, Afonso-Smith SD, Kim S, Lu E, et al. An analysis of a first-in-human study of NEXI-001 donor-derived antigen-specific CD8+ T-cell treatment of relapsed AML after allogeneic hematopoietic cell transplantation (HCT). J Clin Oncol. (2023) 41:7043–3. doi: 10.1200/JCO.2023.41.16_suppl.7043
133. Pospiech M, Tamizharasan M, Wei YC, Kumar AMS, Lou M, Milstein J, et al. Features of the TCR repertoire associate with patients’ clinical and molecular characteristics in acute myeloid leukemia. Front Immunol. (2023) 14:1236514. doi: 10.3389/fimmu.2023.1236514
134. Zhou W, Yu J, Li Y, Wang K. Neoantigen-specific TCR-T cell-based immunotherapy for acute myeloid leukemia. Exp Hematol Oncol. (2022) 11:100. doi: 10.1186/s40164-022-00353-3
135. Rafat A, Dizaji Asl K, Mazloumi Z, Movassaghpour AA, Talebi M, Shanehbandi D, et al. Telomerase inhibition on acute myeloid leukemia stem cell induced apoptosis with both intrinsic and extrinsic pathways. Life Sci. (2022) 295:120402. doi: 10.1016/j.lfs.2022.120402
136. Bajwa G, Arber C. Rapid generation of TCR and CD8αβ Transgenic virus specific T cells for immunotherapy of leukemia. Front Immunol. (2022) 13:830021. doi: 10.3389/fimmu.2022.830021
137. Kaweme NM, Zhou F. Optimizing NK cell-based immunotherapy in myeloid leukemia: abrogating an immunosuppressive microenvironment. Front Immunol. (2021) 12:683381. doi: 10.3389/fimmu.2021.683381
138. Paul S, Lal G. The molecular mechanism of natural killer cells function and its importance in cancer immunotherapy. Front Immunol. (2017) 8:1124. doi: 10.3389/fimmu.2017.01124
139. Lowdell MW, Craston R, Samuel D, Wood ME, O'Neill E, Saha V, et al. Evidence that continued remission in patients treated for acute leukemia is dependent upon autologous natural killer cells. Br J Hematol. (2002) 117:821–7. doi: 10.1046/j.1365-2141.2002.03495.x
140. Romee R, Schneider SE, Leong JW, Chase JM, Keppel CR, Sullivan RP, et al. Cytokine activation induces human memory-like NK cells. Blood. (2012) 120:4751–60. doi: 10.1182/blood-2012-04-419283
141. Dahlberg CI, Sarhan D, Chrobok M, Duru AD, Alici E. Natural killer cell-based therapies targeting cancer: possible strategies to gain and sustain anti-tumor activity. Front Immunol. (2015) 6:605. doi: 10.3389/fimmu.2015.00605
142. Carlsten M, Childs RW. Genetic manipulation of NK cells for cancer immunotherapy: techniques and clinical implications. Front Immunol. (2015) 6:266. doi: 10.3389/fimmu.2015.00266
143. Xu J, Niu T. Natural killer cell-based immunotherapy for acute myeloid leukemia. J Hematol Oncol. (2020) 13:167. doi: 10.1186/s13045-020-00996-x
144. Rafei H, Daher M, Rezvani K. Chimeric antigen receptor (CAR) natural killer (NK)-cell therapy: leveraging the power of innate immunity. Br J Hematol. (2021) 193:216–30. doi: 10.1111/bjh.17186
145. Lu H, Zhao X, Li Z, Hu Y, Wang H. From CAR-T cells to CAR-NK cells: A developing immunotherapy method for hematological Malignancies. Front Oncol. (2021) 11:720501. doi: 10.3389/fonc.2021.720501
146. Lim WA, June CH. The principles of engineering immune cells to treat cancer. Cell. (2017) 168:724–40. doi: 10.1016/j.cell.2017.01.016
147. Fathi E, Farahzadi R, Sheervalilou R, Sanaat Z, Vietor I. A general view of CD33(+) leukemic stem cells and CAR-T cells as interesting targets in acute myeloblatsic leukemia therapy. Blood Res. (2020) 55:10–6. doi: 10.5045/br.2020.55.1.10
148. Hauswirth AW, Florian S, Printz D, Sotlar K, Krauth MT, Fritsch G, et al. Expression of the target receptor CD33 in CD34+/CD38-/CD123+ AML stem cells. Eur J Clin Invest. (2007) 37:73–82. doi: 10.1111/j.1365-2362.2007.01746.x
149. Gonzalez A, Hong E, Yucel G, Leitner E, Chinta P, Deng H, et al. Abstract 3195: Preclinical development of SENTI-202, an off-the-shelf logic gated CAR-NK cell therapy, for the treatment of CD33/FLT3+ hematologic Malignancies including AML. Cancer Res. (2023) 83:3195–5. doi: 10.1158/1538-7445.AM2023-3195
150. Schirrmann T, Pecher G. Specific targeting of CD33(+) leukemia cells by a natural killer cell line modified with a chimeric receptor. Leuk Res. (2005) 29:301–6. doi: 10.1016/j.leukres.2004.07.005
151. Tang X, Yang L, Li Z, Nalin AP, Dai H, Xu T, et al. First-in-man clinical trial of CAR NK-92 cells: safety test of CD33-CAR NK-92 cells in patients with relapsed and refractory acute myeloid leukemia. Am J Cancer Res. (2018) 8:1083–9.
152. Garg S, Ni W, Griffin JD, Sattler M. Chimeric antigen receptor T cell therapy in acute myeloid leukemia: trials and tribulations. Hematol Rep. (2023) 15:608–26. doi: 10.3390/hematolrep15040063
153. Salman H, Pinz KG, Wada M, Shuai X, Yan LE, Petrov JC, et al. Preclinical targeting of human acute myeloid leukemia using CD4-specific chimeric antigen receptor (CAR) T cells and NK cells. J Cancer. (2019) 10:4408–19. doi: 10.7150/jca.28952
154. Zhu XY, Liu X, Wang XB, Wang AY, Wang M, Liu NN, et al. Killing effect of A CD7 chimeric antigen receptor-modified NK-92MI cell line on CD7-positive hematological Malignant cells. Zhongguo Shi Yan Xue Ye Xue Za Zhi. (2020) 28:1367–75. doi: 10.19746/j.cnki.issn.1009-2137.2020.04.049
155. Hadiloo K, Taremi S, Heidari M, Esmaeilzadeh A. The CAR macrophage cells, a novel generation of chimeric antigen-based approach against solid tumors. biomark Res. (2023) 11:103. doi: 10.1186/s40364-023-00537-x
156. Yang H, Shao R, Huang H, Wang X, Rong Z, Lin Y. Engineering macrophages to phagocytose cancer cells by blocking the CD47/SIRPα axis. Cancer Med. (2019) 8:4245–53. doi: 10.1002/cam4.2332
157. Mu X, Chen C, Dong L, Kang Z, Sun Z, Chen X, et al. Immunotherapy in leukemia. Acta Biochim Biophys Sin (Shanghai). (2023) 55:974–87. doi: 10.3724/abbs.2023101
158. Genç EE, et al. Diagnostic and treatment obstacles in acute myeloid leukemia: social, operational, and financial. Oncol Ther. (2023) 11:145–52. doi: 10.1007/s40487-023-00229-4
159. Boscaro E, Urbino I, Catania FM, Arrigo G, Secreto C, Olivi M, et al. Modern risk stratification of acute myeloid leukemia in 2023: integrating established and emerging prognostic factors. Cancers (Basel). (2023) 15:3512. doi: 10.3390/cancers15133512
160. Stelmach P, Trumpp A. Leukemic stem cells and therapy resistance in acute myeloid leukemia. Hematologica. (2023) 108:353–66. doi: 10.3324/haematol.2022.280800
161. Aponte PM, Caicedo A. Stemness in cancer: stem cells, cancer stem cells, and their microenvironment. Stem Cells Int. (2017) 2017:5619472. doi: 10.1155/2017/5619472
162. Kumar CC. Genetic abnormalities and challenges in the treatment of acute myeloid leukemia. Genes Cancer. (2011) 2:95–107. doi: 10.1177/1947601911408076
163. Tian C, Chen Z. Immune therapy: a new therapy for acute myeloid leukemia. Blood Sci. (2023) 5:15–24. doi: 10.1097/BS9.0000000000000140
164. Sterner RC, Sterner RM. CAR-T cell therapy: current limitations and potential strategies. Blood Cancer J. (2021) 11:69. doi: 10.1038/s41408-021-00459-7
165. Jamal-Hanjani M, Quezada SA, Larkin J, Swanton C. Translational implications of tumor heterogeneity. Clin Cancer Res. (2015) 21:1258–66. doi: 10.1158/1078-0432.CCR-14-1429
166. Li K, Shi H, Zhang B, Ou X, Ma Q, Chen Y, et al. Myeloid-derived suppressor cells as immunosuppressive regulators and therapeutic targets in cancer. Signal Transduct Target Ther. (2021) 6:362. doi: 10.1038/s41392-021-00670-9
167. Cheng JN, Yuan YX, Zhu B, Jia Q. Myeloid-derived suppressor cells: A multifaceted accomplice in tumor progression. Front Cell Dev Biol. (2021) 9:740827. doi: 10.3389/fcell.2021.740827
168. Kouroukli O, Symeonidis A, Foukas P, Maragkou MK, Kourea EP. Bone marrow immune microenvironment in myelodysplastic syndromes. Cancers. (2022) 14:5656. doi: 10.3390/cancers14225656
169. Leblanc R, Ghossoub R, Goubard A, Castellano R, Fares J, Camoin L, et al. Downregulation of stromal syntenin sustains AML development. EMBO Mol Med. (2023) 15:e17570. doi: 10.15252/emmm.202317570
170. Wang SS, Xu ZJ, Jin Y, Ma JC, Xia PH, Wen X, et al. Clinical and prognostic relevance of CXCL12 expression in acute myeloid leukemia. PeerJ. (2021) 9:e11820. doi: 10.7717/peerj.11820
171. Ponte F, Kim HN, Iyer S, Han L, Almeida M, Manolagas SC. Cxcl12 deletion in mesenchymal cells increases bone turnover and attenuates the loss of cortical bone caused by estrogen deficiency in mice. J Bone Miner Res. (2020) 35:1441–51. doi: 10.1002/jbmr.4002
172. Kusmartsev S, Nefedova Y, Yoder D, Gabrilovich DI. Antigen-specific inhibition of CD8+ T cell response by immature myeloid cells in cancer is mediated by reactive oxygen species. J Immunol. (2004) 172:989–99. doi: 10.4049/jimmunol.172.2.989
173. Aurelius J, Thorén FB, Akhiani AA, Brune M, Palmqvist L, Hansson M, et al. Monocytic AML cells inactivate antileukemic lymphocytes: role of NADPH oxidase/gp91(phox) expression and the PARP-1/PAR pathway of apoptosis. Blood. (2012) 119:5832–7. doi: 10.1182/blood-2011-11-391722
174. Arandi N, Ramzi M, Safaei F, Monabati A. Overexpression of indoleamine 2,3-dioxygenase correlates with regulatory T cell phenotype in acute myeloid leukemia patients with normal karyotype. Blood Res. (2018) 53:294–8. doi: 10.5045/br.2018.53.4.294
175. Ellegast JM, Alexe G, Hamze A, Lin S, Uckelmann HJ, Rauch PJ, et al. Unleashing cell-intrinsic inflammation as a strategy to kill AML blasts. Cancer Discovery. (2022) 12:1760–81. doi: 10.1158/2159-8290.CD-21-0956
176. Holicek P, Truxova I, Rakova J, Salek C, Hensler M, Kovar M, et al. Type I interferon signaling in Malignant blasts contributes to treatment efficacy in AML patients. Cell Death Dis. (2023) 14:209. doi: 10.1038/s41419-023-05728-w
177. Thiant S, Yakoub-Agha I, Magro L, Trauet J, Coiteux V, Jouet JP, et al. Plasma levels of IL-7 and IL-15 in the first month after myeloablative BMT are predictive biomarkers of both acute GVHD and relapse. Bone Marrow Transplant. (2010) 45:1546–52. doi: 10.1038/bmt.2010.13
178. Zamarron BF, Chen W. Dual roles of immune cells and their factors in cancer development and progression. Int J Biol Sci. (2011) 7:651–8. doi: 10.7150/ijbs.7.651
179. Salkeni MA, Naing A. Interleukin-10 in cancer immunotherapy: from bench to bedside. Trends Cancer. (2023) 9:716–25. doi: 10.1016/j.trecan.2023.05.003
180. Chen C, Ma J, Pi C, Huang W, Zhang T, Fu C, et al. PPARδ inhibition blocks the induction and function of tumor-induced IL-10(+) regulatory B cells and enhances cancer immunotherapy. Cell Discovery. (2023) 9:54. doi: 10.1038/s41421-023-00568-6
181. Taghiloo S, Asgarian-Omran H. Immune evasion mechanisms in acute myeloid leukemia: A focus on immune checkpoint pathways. Crit Rev Oncol Hematol. (2021) 157:103164. doi: 10.1016/j.critrevonc.2020.103164
182. Williams P, Basu S, Garcia-Manero G, Hourigan CS, Oetjen KA, Cortes JE, et al. The distribution of T-cell subsets and the expression of immune checkpoint receptors and ligands in patients with newly diagnosed and relapsed acute myeloid leukemia. Cancer. (2019) 125:1470–81. doi: 10.1002/cncr.31896
183. Rivas JR, Liu Y, Alhakeem SS, Eckenrode JM, Marti F, Collard JP, et al. Interleukin-10 suppression enhances T-cell antitumor immunity and responses to checkpoint blockade in chronic lymphocytic leukemia. Leukemia. (2021) 35:3188–200. doi: 10.1038/s41375-021-01217-1
184. Rahmani S, Yazdanpanah N, Rezaei N. Natural killer cells and acute myeloid leukemia: promises and challenges. Cancer Immunol Immunother. (2022) 71:2849–67. doi: 10.1007/s00262-022-03217-1
185. Chen G, Wu D, Wang Y, Cen J, Feng Y, Sun A, et al. Expanded donor natural killer cell and IL-2, IL-15 treatment efficacy in allogeneic hematopoietic stem cell transplantation. Eur J Hematol. (2008) 81:226–35. doi: 10.1111/j.1600-0609.2008.01108.x
186. Cloppenborg T, Stanulla M, Zimmermann M, Schrappe M, Welte K, Klein C. Immunosurveillance of childhood ALL: polymorphic interferon-gamma alleles are associated with age at diagnosis and clinical risk groups. Leukemia. (2005) 19:44–8. doi: 10.1038/sj.leu.2403553
187. Zeiser R, Vago L. Mechanisms of immune escape after allogeneic hematopoietic cell transplantation. Blood. (2019) 133:1290–7. doi: 10.1182/blood-2018-10-846824
188. Veluchamy JP, Kok N, van der Vliet HJ, Verheul HMW, de Gruijl TD, Spanholtz J. The rise of allogeneic natural killer cells as a platform for cancer immunotherapy: recent innovations and future developments. Front Immunol. (2017) 8:631. doi: 10.3389/fimmu.2017.00631
189. Parkhurst MR, Riley JP, Dudley ME, Rosenberg SA. Adoptive transfer of autologous natural killer cells leads to high levels of circulating natural killer cells but does not mediate tumor regression. Clin Cancer Res. (2011) 17:6287–97. doi: 10.1158/1078-0432.CCR-11-1347
190. Rezvani K, Rouce RH. The application of natural killer cell immunotherapy for the treatment of cancer. Front Immunol. (2015) 6:578. doi: 10.3389/fimmu.2015.00578
191. Luo J, Zhang X. Challenges and innovations in CAR-T cell therapy: a comprehensive analysis. Front Oncol. (2024) 14:1399544. doi: 10.3389/fonc.2024.1399544
192. Shafer P, Kelly LM, Hoyos V. Cancer therapy with TCR-engineered T cells: current strategies, challenges, and prospects. Front Immunol. (2022) 13:835762. doi: 10.3389/fimmu.2022.835762
193. Lian G, et al. Challenges and recent advances in NK cell-targeted immunotherapies in solid tumors. Int J Mol Sci. (2021) 23:164. doi: 10.3390/ijms23010164
194. Casagrande S, Sopetto GB, Bertalot G, Bortolotti R, Racanelli V, Caffo O, et al. Immune-related adverse events due to cancer immunotherapy: immune mechanisms and clinical manifestations. Cancers (Basel). (2024) 16:1440. doi: 10.3390/cancers16071440
195. Chen DS, Mellman I. Elements of cancer immunity and the cancer-immune set point. Nature. (2017) 541:321–30. doi: 10.1038/nature21349
196. Taefehshokr S, Parhizkar A, Hayati S, Mousapour M, Mahmoudpour A, Eleid L, et al. Cancer immunotherapy: Challenges and limitations. Pathol Res Pract. (2022) 229:153723. doi: 10.1016/j.prp.2021.153723
197. Stock S, Schmitt M, Sellner L. Optimizing manufacturing protocols of chimeric antigen receptor T cells for improved anticancer immunotherapy. Int J Mol Sci. (2019) 20:6223. doi: 10.3390/ijms20246223
198. Townsend MH, Shrestha G, Robison RA, O'Neill KL. The expansion of targetable biomarkers for CAR T cell therapy. J Exp Clin Cancer Res. (2018) 37:163. doi: 10.1186/s13046-018-0817-0
199. Köhnke T, Liu X, Haubner S, Bücklein V, Hänel G, Krupka C, et al. Integrated multiomic approach for identification of novel immunotherapeutic targets in AML. biomark Res. (2022) 10:43. doi: 10.1186/s40364-022-00390-4
200. Pommert L, Tarlock K. The evolution of targeted therapy in pediatric AML: gemtuzumab ozogamicin, FLT3/IDH/BCL2 inhibitors, and other therapies. Hematol Am Soc Hematol Educ Program. (2022) 2022:603–10. doi: 10.1182/hematology.2022000358
201. Barbosa K, Deshpande AJ. Therapeutic targeting of leukemia stem cells in acute myeloid leukemia. Front Oncol. (2023) 13:1204895. doi: 10.3389/fonc.2023.1204895
202. Rami A, DuBois SG, Campbell K. Reporting and impact of subsequent cycle toxicities in oncology phase I clinical trials. Clin Trials. (2023) p:17407745231210872. doi: 10.1200/JCO.2023.41.16_suppl.3149
203. Epperly R, Giordani VM, Mikkilineni L, Shah NN. Early and late toxicities of chimeric antigen receptor T-cells. Hematol Oncol Clin North Am. (2023) 37:1169–88. doi: 10.1016/j.hoc.2023.05.010
204. Accorsi Buttini E, Farina M, Lorenzi L, Polverelli N, Radici V, Morello E, et al. High risk-myelodysplastic syndrome following CAR T-cell therapy in a patient with relapsed diffuse large B cell lymphoma: A case report and literature review. Front Oncol. (2023) 13:1036455. doi: 10.3389/fonc.2023.1036455
205. Zhao A, Zhao M, Qian W, Liang A, Li P, Liu H. Secondary myeloid neoplasms after CD19 CAR T therapy in patients with refractory/relapsed B-cell lymphoma: case series and review of literature. Front Immunol. (2023) 13:1063986. doi: 10.3389/fimmu.2022.1063986
206. Taylor BC, Balko JM. Mechanisms of MHC-I downregulation and role in immunotherapy response. Front Immunol. (2022) 13:844866. doi: 10.3389/fimmu.2022.844866
207. Olivera I, Bolaños E, Gonzalez-Gomariz J, Hervas-Stubbs S, Mariño KV, Luri-Rey C, et al. mRNAs encoding IL-12 and a decoy-resistant variant of IL-18 synergize to engineer T cells for efficacious intratumoral adoptive immunotherapy. Cell Rep Med. (2023) 4:100978. doi: 10.1016/j.xcrm.2023.100978
208. Wang CQ, Lim PY, Tan AH. Gamma/delta T cells as cellular vehicles for anti-tumor immunity. Front Immunol. (2023) 14:1282758. doi: 10.3389/fimmu.2023.1282758
209. Morgan RA, Chinnasamy N, Abate-Daga D, Gros A, Robbins PF, Zheng Z, et al. Cancer regression and neurological toxicity following anti-MAGE-A3 TCR gene therapy. J Immunother. (2013) 36:133–51. doi: 10.1097/CJI.0b013e3182829903
210. Linette GP, Stadtmauer EA, Maus MV, Rapoport AP, Levine BL, Emery L, et al. Cardiovascular toxicity and titin cross-reactivity of affinity-enhanced T cells in myeloma and melanoma. Blood. (2013) 122:863–71. doi: 10.1182/blood-2013-03-490565
211. Manfredi F, Cianciotti BC, Potenza A, Tassi E, Noviello M, Biondi A, et al. TCR redirected T cells for cancer treatment: achievements, hurdles, and goals. Front Immunol. (2020) 11:1689. doi: 10.3389/fimmu.2020.01689
212. Ghahri-Saremi N, Akbari B, Soltantoyeh T, Hadjati J, Ghassemi S, Mirzaei HR. Genetic modification of cytokine signaling to enhance efficacy of CAR T cell therapy in solid tumors. Front Immunol. (2021) 12:738456. doi: 10.3389/fimmu.2021.738456
213. López-Cantillo G, Urueña C, Camacho BA, Ramírez-Segura C. CAR-T cell performance: how to improve their persistence? Front Immunol. (2022) 13:878209. doi: 10.3389/fimmu.2022.878209
214. Lindner SE, Johnson SM, Brown CE, Wang LD. Chimeric antigen receptor signaling: Functional consequences and design implications. Sci Adv. (2020) 6:eaaz3223. doi: 10.1126/sciadv.aaz3223
215. Miyao K, Terakura S, Okuno S, Julamanee J, Watanabe K, Hamana H, et al. Introduction of genetically modified CD3ζ Improves proliferation and persistence of antigen-specific CTLs. Cancer Immunol Res. (2018) 6:733–44. doi: 10.1158/2326-6066.CIR-17-0538
216. Sakai T, Terakura S, Miyao K, Okuno S, Adachi Y, Umemura K, et al. Artificial T cell adaptor molecule-transduced TCR-T cells demonstrated improved proliferation only when transduced in a higher intensity. Mol Ther Oncolytics. (2020) 18:613–22. doi: 10.1016/j.omto.2020.08.014
217. Chi X, Luo S, Ye P, Hwang WL, Cha JH, Yan X, et al. T-cell exhaustion and stemness in antitumor immunity: Characteristics, mechanisms, and implications. Front Immunol. (2023) 14:1104771. doi: 10.3389/fimmu.2023.1104771
218. Lupo KB, Matosevic S. Natural killer cells as allogeneic effectors in adoptive cancer immunotherapy. Cancers (Basel). (2019) 11:769. doi: 10.3390/cancers11060769
219. Widowati W, Jasaputra DK, Sumitro SB, Widodo MA, Mozef T, Rizal R, et al. Effect of interleukins (IL-2, IL-15, IL-18) on receptors activation and cytotoxic activity of natural killer cells in breast cancer cell. Afr Health Sci. (2020) 20:822–32. doi: 10.4314/ahs.v20i2.36
220. Romee R, Cooley S, Berrien-Elliott MM, Westervelt P, Verneris MR, Wagner JE, et al. First-in-human phase 1 clinical study of the IL-15 superagonist complex ALT-803 to treat relapse after transplantation. Blood. (2018) 131:2515–27. doi: 10.1182/blood-2017-12-823757
221. Hatami Z, Hashemi ZS, Eftekhary M, Amiri A, Karpisheh V, Nasrollahi K, et al. Natural killer cell-derived exosomes for cancer immunotherapy: innovative therapeutics art. Cancer Cell Int. (2023) 23:157. doi: 10.1186/s12935-023-02996-6
222. Klingemann H, Boissel L, Toneguzzo F. Natural killer cells for immunotherapy - advantages of the NK-92 cell line over blood NK cells. Front Immunol. (2016) 7:91. doi: 10.3389/fimmu.2016.00091
223. Zhang J, Zheng H, Diao Y. Natural killer cells and current applications of chimeric antigen receptor-modified NK-92 cells in tumor immunotherapy. Int J Mol Sci. (2019) 20:317. doi: 10.3390/ijms20020317
224. D’Silva SZ, Singh M, Pinto AS. NK cell defects: implication in acute myeloid leukemia. Front Immunol. (2023) 14:1112059. doi: 10.3389/fimmu.2023.1112059
225. Allison M, Mathews J, Gilliland T, Mathew SO. Natural killer cell-mediated immunotherapy for leukemia. Cancers (Basel). (2022) 14:843. doi: 10.3390/cancers14030843
226. Pratz KW, Cherry M, Altman JK, Cooper BW, Podoltsev NA, Cruz JC, et al. Gilteritinib in combination with induction and consolidation chemotherapy and as maintenance therapy: A phase IB study in patients with newly diagnosed AML. J Clin Oncol. (2023) 41:4236–46. doi: 10.1200/JCO.22.02721
227. McBride A, Klink AJ, Ullman AJ, Liassou D, Brown-Bickerstaff C, Gajra A. AML-437 real-world effectiveness of enasidenib (ENA) in older patients with relapsed/refractory (R/R) isocitrate dehydrogenase-2 (IDH2)-mutated acute myeloid leukemia (AML). Clin Lymphoma Myeloma Leukemia. (2022) 22:S250–1. doi: 10.1016/S2152-2650(22)01295-2
228. Watts JM, Patel PA, Choe S, Bai X, Marchione DM, Stein E. Clinical and molecular characteristics of patients with AML with an exceptional response to ivosidenib. Am Soc Clin Oncol. (2023) 7(Suppl):e810173a. doi: 10.1097/01.HS9.0000968672.81017.3a
229. Kristensen DT, Brøndum RF, Ørskov AD, Marcher CW, Schöllkopf C, Sørensen ALT, et al. Venetoclax-based therapy for relapsed or refractory acute myeloid leukemia following intensive induction chemotherapy. Eur J Hematol. (2023) 111:573–82. doi: 10.1111/ejh.14046
230. Liu S, Zhou Z, Zhai WJ, Song XN, Li Q, Jiang EL, et al. The relationship between occurrence of agvhd in patients with acute myeloid leukemia after allogeneic hematopoietic stem cell transplantation and immune cell components in graft. Zhongguo shi yan xue ye xue za zhi. (2023) 31:539–45. doi: 10.19746/j.cnki.issn.1009-2137.2023.02.033
231. Pabon CM, Abbas HA, Konopleva M. Acute myeloid leukemia: therapeutic targeting of stem cells. Expert Opin Ther Targets. (2022) 26:547–56. doi: 10.1080/14728222.2022.2083957
232. Cheever MA, Allison JP, Ferris AS, Finn OJ, Hastings BM, Hecht TT, et al. The prioritization of cancer antigens: a national cancer institute pilot project for the acceleration of translational research. Clin Cancer Res. (2009) 15:5323–37. doi: 10.1158/1078-0432.CCR-09-0737
233. Watts J, Nimer S. Recent advances in the understanding and treatment of acute myeloid leukemia. F1000Res. (2018) 7. doi: 10.12688/f1000research
234. Butner JD, Dogra P, Chung C, Pasqualini R, Arap W, Lowengrub J, et al. Mathematical modeling of cancer immunotherapy for personalized clinical translation. Nat Comput Sci. (2022) 2:785–96. doi: 10.1038/s43588-022-00377-z
235. Sahoo P, Yang X, Abler D, Maestrini D, Adhikarla V, Frankhouser D, et al. Mathematical deconvolution of CAR T-cell proliferation and exhaustion from real-time killing assay data. J R Soc Interface. (2020) 17:20190734. doi: 10.1098/rsif.2019.0734
236. Liu L, Ma C, Zhang Z, Witkowski MT, Aifantis I, Ghassemi S, et al. Computational model of CAR T-cell immunotherapy dissects and predicts leukemia patient responses at remission, resistance, and relapse. J Immunother Cancer. (2022) 10:e005360. doi: 10.1136/jitc-2022-005360
237. Paixão EA, Barros LRC, Fassoni AC, Almeida RC. Modeling patient-specific CAR-T cell dynamics: multiphasic kinetics via phenotypic differentiation. Cancers (Basel). (2022) 14:55–76. doi: 10.3390/cancers14225576
238. Barros LRC, Paixão EA, Valli AMP, Naozuka GT, Fassoni AC, Almeida RC. CARTmath-A mathematical model of CAR-T immunotherapy in preclinical studies of hematological cancers. Cancers (Basel). (2021) 13:29–41. doi: 10.3390/cancers13122941
239. Fogel DB. Factors associated with clinical trials that fail and opportunities for improving the likelihood of success: A review. Contemp Clin Trials Commun. (2018) 11:156–64. doi: 10.1016/j.conctc.2018.08.001
240. Isidori A, Cerchione C, Daver N, DiNardo C, Garcia-Manero G, Konopleva M, et al. Immunotherapy in acute myeloid leukemia: where we stand. Front Oncol. (2021) 11:656218. doi: 10.3389/fonc.2021.656218
241. Jiménez-Morales S, Aranda-Uribe IS, Pérez-Amado CJ, Ramírez-Bello J, Hidalgo-Miranda A. Mechanisms of immunosuppressive tumor evasion: focus on acute lymphoblastic leukemia. Front Immunol. (2021) 12:737340. doi: 10.3389/fimmu.2021.737340
242. Khaldoyanidi S, Nagorsen D, Stein A, Ossenkoppele G, Subklewe M. Immune biology of acute myeloid leukemia: implications for immunotherapy. J Clin Oncol. (2021) 39:419–32. doi: 10.1200/JCO.20.00475
243. Wang Y, Zhang X, Wang Y, Zhao W, Li H, Zhang L, et al. Application of immune checkpoint targets in the anti-tumor novel drugs and traditional Chinese medicine development. Acta Pharm Sin B. (2021) 11:2957–72. doi: 10.1016/j.apsb.2021.03.004
244. Subklewe M, et al. Novel immunotherapies in the treatment of AML: is there hope? Hematology. (2023) 2023:691–701. doi: 10.1182/hematology.2023000455
245. Zhukovsky EA, Morse RJ, Maus MV. Bispecific antibodies and CARs: generalized immunotherapeutics harnessing T cell redirection. Curr Opin Immunol. (2016) 40:24–35. doi: 10.1016/j.coi.2016.02.006
246. Carter JL, Hege K, Yang J, Kalpage HA, Su Y, Edwards H, et al. Targeting multiple signaling pathways: the new approach to acute myeloid leukemia therapy. Signal Transduct Target Ther. (2020) 5:288. doi: 10.1038/s41392-020-00361-x
247. Tiong IS, Loo S. Targeting measurable residual disease (MRD) in acute myeloid leukemia (AML): moving beyond prognostication. Int J Mol Sci. (2023) 24:1–20. doi: 10.3390/ijms24054790
248. Al-Haideri M, Tondok SB, Safa SH, Maleki AH, Rostami S, Jalil AT, et al. CAR-T cell combination therapy: the next revolution in cancer treatment. Cancer Cell Int. (2022) 22:365. doi: 10.1186/s12935-022-02778-6
249. Mitra A, Barua A, Huang L, Ganguly S, Feng Q, He B. From bench to bedside: the history and progress of CAR T cell therapy. Front Immunol. (2023) 14:1188049. doi: 10.3389/fimmu.2023.1188049
250. Huang D, Ma N, Li X, Gou Y, Duan Y, Liu B, et al. Advances in single-cell RNA sequencing and its applications in cancer research. J Hematol Oncol. (2023) 16:98. doi: 10.1186/s13045-023-01494-6
251. De Marco RC, Monzo HJ, Ojala PM. CAR T cell therapy: A versatile living drug. Int J Mol Sci. (2023) 24:1–22. doi: 10.3390/ijms24076300
252. Gottschlich A, Thomas M, Grünmeier R, Lesch S, Rohrbacher L, Igl V, et al. Single-cell transcriptomic atlas-guided development of CAR-T cells for the treatment of acute myeloid leukemia. Nat Biotechnol. (2023) 41:1618–32. doi: 10.1038/s41587-023-01684-0
253. Abbott RC, Cross RS, Jenkins MR. Finding the keys to the CAR: identifying novel target antigens for T cell redirection immunotherapies. Int J Mol Sci. (2020) 21:515. doi: 10.3390/ijms21020515
254. Sletta KY, Castells O, Gjertsen BT. Colony stimulating factor 1 receptor in acute myeloid leukemia. Front Oncol. (2021) 11:654817. doi: 10.3389/fonc.2021.654817
255. Zhang Q, Ma R, Chen H, Guo W, Li Z, Xu K, et al. CD86 is associated with immune infiltration and immunotherapy signatures in AML and promotes its progression. J Oncol. (2023) 2023:9988405. doi: 10.1155/2023/9988405
256. Restelli C, Ruella M, Paruzzo L, Tarella C, Pelicci PG, Colombo E. Recent advances in immune-based therapies for acute myeloid leukemia. Blood Cancer Discov. (2024) 5:234–48. doi: 10.1158/2643-3230.BCD-23-0202
257. Dao T, Xiong G, Mun SS, Meyerberg J, Korontsvit T, Xiang J, et al. A dual-receptor T-cell platform with Ab-TCR and costimulatory receptor achieves specificity and potency against AML. Blood. (2024) 143:507–21. doi: 10.1182/blood.2023021054
258. Tang X, Berger MF, Solit DB. Precision oncology: current and future platforms for treatment selection. Trends Cancer. (2024) 10(9):781–91. doi: 10.1016/j.trecan.2024.06.009
259. Hackl H, Charoentong P, Finotello F, Trajanoski Z. Computational genomics tools for dissecting tumor–immune cell interactions. Nat Rev Genet. (2016) 17(8):441–58. doi: 10.1038/nrg.2016.67
260. Kiyotani K, Toyoshima Y, Nakamura Y. Personalized immunotherapy in cancer precision medicine. Cancer Biol Med. (2021) 18:955. doi: 10.20892/j.issn.2095-3941.2021.0032
261. Geurts BS, Zeverijn LJ, van Berge Henegouwen JM, van der Wijngaart H, Hoes LR, de Wit GF, et al. Characterization of discordance between mismatch repair deficiency and microsatellite instability testing may prevent inappropriate treatment with immunotherapy. J Pathol. (2024) 263(3):288–99. doi: 10.1002/path.6279
262. Kinnersley B, Sud A, Everall A, Cornish AJ, Chubb D, Culliford R, et al. Analysis of 10,478 cancer genomes identifies candidate driver genes and opportunities for precision oncology. Nat Genet. (2024) 56(9):1868–77. doi: 10.1038/s41588-024-01785-9
263. Wang L, Wang H, Li HZ. Real-time customized precision combination therapies based on potential actionability and coalterations to provide therapeutic opportunities for hyperprogressive disease after immune checkpoint inhibitor therapy. JCO. (2023) 41:e14688–e14688. doi: 10.1200/JCO.2023.41.16_suppl.e14688
264. Lee D, Cook S, Jung Y, Lim L, Lee JE, Im H-J, et al. Automated tumor microenvironment analysis for multiple samples by image-based spatial transcriptomics on tissue microarray. Cancer Res. (2024) 84:4948–8. doi: 10.1158/1538-7445.AM2024-4948
265. Zhang S, Fang W, Zhou S, Zhu D, Chen R, Gao X, et al. Single cell transcriptomic analyses implicate an immunosuppressive tumor microenvironment in pancreatic cancer liver metastasis. Nat Commun. (2023) 14:5123. doi: 10.1038/s41467-023-40727-7
266. Drevets WC, Wittenberg GM, Bullmore ET, Manji HK. Immune targets for therapeutic development in depression: towards precision medicine. Nat Rev Drug Discov. (2022) 21:224–44. doi: 10.1038/s41573-021-00368-1
267. Shah RK, Cygan E, Kozlik T, Colina A, Zamora AE. Utilizing immunogenomic approaches to prioritize targetable neoantigens for personalized cancer immunotherapy. Front Immunol. (2023) 14:1301100. doi: 10.3389/fimmu.2023.1301100
268. Savsani K, Dakshanamurthy S. Novel methodology for the design of personalized cancer vaccine targeting neoantigens: application to pancreatic ductal adenocarcinoma. Diseases. (2024) 12:149. doi: 10.3390/diseases12070149
269. Viborg N, Kleine-Kohlbrecher D, Rønø B. Personalized neoantigen DNA cancer vaccines: current status and future perspectives. J Cell Immunol. (2024) 6:15–24. doi: 10.33696/immunology
Keywords: acute myeloid leukemia, cell therapy, immunotherapy, CAR-T cell, TCR-T cell, natural killer cell
Citation: Kheirkhah AH, Habibi S, Yousefi MH, Mehri S, Ma B, Saleh M and Kavianpour M (2024) Finding potential targets in cell-based immunotherapy for handling the challenges of acute myeloid leukemia. Front. Immunol. 15:1460437. doi: 10.3389/fimmu.2024.1460437
Received: 06 July 2024; Accepted: 29 August 2024;
Published: 30 September 2024.
Edited by:
Amirhesam Babajani, Shahid Beheshti University of Medical Sciences, IranReviewed by:
Lipei Shao, National Institutes of Health (NIH), United StatesMohammadreza Kasravi, Children’s Hospital of Los Angeles, United States
Negar Nekooei, University of Southern California, United States
Copyright © 2024 Kheirkhah, Habibi, Yousefi, Mehri, Ma, Saleh and Kavianpour. This is an open-access article distributed under the terms of the Creative Commons Attribution License (CC BY). The use, distribution or reproduction in other forums is permitted, provided the original author(s) and the copyright owner(s) are credited and that the original publication in this journal is cited, in accordance with accepted academic practice. No use, distribution or reproduction is permitted which does not comply with these terms.
*Correspondence: Maria Kavianpour, S2F2aWFucG91ci5tYXJpYUBnbWFpbC5jb20=
†These authors have contributed equally to this work