- 1School of Biotechnology and Biomolecular Sciences, Faculty of Science, University of New South Wales, Sydney, NSW, Australia
- 2Immune Biotherapeutics Program, Garvan Institute of Medical Research, Sydney, NSW, Australia
- 3St Vincent’s School of Medicine, Faculty of Medicine, University of New South Wales, Sydney, NSW, Australia
- 4The Kinghorn Cancer Centre, St Vincent’s Hospital, Sydney, NSW, Australia
Neutrophils rapidly respond to inflammation resulting from infection, injury, and cancer. Intravital microscopy (IVM) has significantly advanced our understanding of neutrophil behavior, enabling real-time visualization of their migration, interactions with pathogens, and coordination of immune responses. This review delves into the insights provided by IVM studies on neutrophil dynamics in various inflammatory contexts. We also examine the dual role of neutrophils in tumor microenvironments, where they can either facilitate or hinder cancer progression. Finally, we highlight how computational modeling techniques, especially agent-based modeling, complement experimental data by elucidating neutrophil kinetics at the level of individual cells as well as their collective behavior. Understanding the role of neutrophils in health and disease is essential for developing new strategies for combating infection, inflammation and cancer.
Introduction
Neutrophils are the most abundant white cells in blood (1, 2) and are rapidly recruited from the bloodstream into inflamed tissues to combat pathogens and facilitate tissue repair (3–6). They wield an array of microbicidal molecules designed to destroy microorganisms through phagocytosis (7), release of neutrophil extracellular traps (NETs) (8), tissue remodeling and production of reactive oxygen species (ROS) (9–11).
Intravital microscopy (IVM) in combination with fluorescent neutrophil reporter mice (12–14) has enabled researchers to visualize neutrophils in real time (15, 16) and revolutionized our understanding of the cellular and molecular processes underpinning neutrophil activity in response to wounds, infections, and cancers (3, 17, 18). Recently, computational modeling has emerged as a valuable way of simulating neutrophil behavior in various environments to predict their collective responses. This integration of imaging and computational techniques provides a new framework for studying neutrophils not only as individual entities but for understanding their collective behavior and how it relates to their functions (19–21). This review highlights recent insights obtained through IVM studies of neutrophils and computational modeling of their movement, offering predictive capabilities that inform both basic research and clinical applications.
Visualizing neutrophils with IVM
Several different imaging modalities have been employed for IVM and are reviewed in (22–24). Although both confocal and multiphoton microscopy are widely used for in vivo imaging, multiphoton microscopy can be advantageous for longitudinal imaging due to its confined volume of excitation (and reduced tissue photodamage and light scattering) (25, 26). Exciting advances in tissue clearing agents suitable for in vivo applications suggest the possibility of even greater depth of tissue penetration that would significantly expand IVM capabilities (27).
Both ex vivo and in vivo approaches can be used to label neutrophils for IVM but while ex vivo labeling offers more flexibility, it may lead to neutrophil activation during labeling. On the other hand, fluorescent reporter mice allow for long-term imaging of unmanipulated neutrophils, but fluorescent labels are not always restricted to neutrophils complicating subsequent analysis and interpretation (Table 1).
Neutrophils in infection and injury
Neutrophils are pivotal to the body’s immune response to infection and injury (39, 40). Within minutes after injury, neutrophils infiltrate the site of inflammation in response to danger-associated molecular patterns (DAMPs) from damaged cells or pathogen-associated molecular patterns (PAMPs) from microorganisms (41, 42). There they release an array of anti-microbial agents as well as NETs containing DNA, histones, and proteases (43, 44). While neutrophils are best known for their essential roles in pathogen clearance, they also play an important role in wound healing after injury (45, 46).
Neutrophil recruitment to the site of inflammation
Neutrophils circulate in a non-activated state until endothelial activation triggers their recruitment to sites of infection through a series of steps including tethering, rolling, adhesion, crawling, and transmigration (47). Their recruitment involves selectins on endothelial cells binding to ligands on neutrophils, such as P-selectin glycoprotein ligand-1 (PSGL-1), initiating tethering and rolling (48). This is followed by the engagement of integrins like lymphocyte function-associated antigen 1 (LFA-1) and Mac-1 with intercellular adhesion molecule-1 (ICAM-1) on endothelial cells, which strengthens adhesion and enables neutrophils to crawl and transmigrate into inflamed tissues (49).
Efficient navigation through capillary networks is crucial for optimal neutrophil recruitment to injury sites. To prevent congestion and ensure smooth passage through narrow vessels, neutrophils can alternate between branches at capillary bifurcation (50). This behavior is regulated by interactions between consecutive neutrophils, with the first neutrophil in one capillary branch altering the migration of the following neutrophil towards the other branch through a mechanism driven by chemoattractant gradient perturbation and hydraulic resistance (50). This alternating migration helps distribute neutrophil traffic uniformly at bifurcations of capillaries and ensures swift arrival at sites of damage without causing congestion in narrow vessels (50).
Subsequent trans-endothelial migration involves temporary spikes in calcium levels inside neutrophils as they squeeze through the vessel walls in live mice (51). These calcium spikes are triggered by a sensor protein called Piezo1. When it is activated, it leads to the production of hypoxic inducible factor 1α (HIF1α), which then turns on NADPH oxidase 4 (NOX4) and helps produce ROS to kill bacteria during infections (51).
Neutrophil recruitment into tissue is a crucial step in immune defense against infections, as evidenced by the findings that impaired extravasation through the basement membrane in neutrophils lacking mammalian sterile 20-like kinase 1 may contribute to the severe immune defect observed in patients with mammalian sterile 20-like kinase 1 (MST1) deficiency (52, 53).
The importance of regulating neutrophil migration in circulation was also underscored in recent studies of severe acute respiratory syndrome coronavirus 2 (SARS-CoV-2) infection. IVM revealed that neutrophils accumulated in the microcirculation of the lungs and brain of SARS-CoV-2 infected mice (54). This accumulation was associated with endothelial activation, local inflammation, and the formation of large platelet aggregates, particularly in the brain. These findings suggest that neutrophils contribute to the localized vascular and inflammatory changes observed in SARS-CoV-2 infection, highlighting their importance in the pathogenesis of coronavirus disease 2019 (COVID-19)-related complications (54).
Neutrophil migration in inflamed tissues
Following transmigration neutrophils enter inflamed tissues and transition to directed migration towards the inflammatory foci called chemotaxis (55). For example, during focal necrosis in the liver, neutrophils were guided by a multistep process involving ATP release from necrotic cells, which activates the inflammasome, triggering neutrophil adhesion (56). Subsequently, their migration toward the injury site was directed by a chemokine gradient, but signals such as formyl peptides could override this gradient, ensuring that neutrophils are precisely guided into necrotic tissue (56). This finely tuned mechanism allows neutrophils to navigate through healthy tissue without causing unnecessary damage, focusing their action on damaged areas. Experiments in zebrafish and mouse neutrophils show that during chemotaxis neutrophils employ a ‘search and run’ strategy, where an initial actin-driven orientation is followed by rapid actin flows, enabling them to navigate tissue gradients effectively (57).
The orchestrated movement of neutrophils toward the attractant results in their focal accumulation and formation of dynamic clusters, termed ‘neutrophil swarms’ (3, 55). Since its discovery in 2008, neutrophil swarming has been observed in more than 30 inflammatory conditions, including sterile inflammation and infections by bacteria, parasites, viruses, and fungi (3, 55, 58, 59). In fact, swarming is a conserved function in tissue inflammation and is an integral part of wound healing.
The initial conceptual framework of swarming emerged from observations of dynamic cooperative behavior among neutrophils during intracellular parasitic Toxoplasma gondii (T. gondii) infection (3). Initially, “pioneer” neutrophils detected damage and then signaled to recruit additional neutrophils from distances greater than 70 μm, forming a swarm (3). These swarms were either transient or persistent, with the latter potentially leading to early granuloma formation and contributing to tissue remodeling by displacing collagen fibers (3).
Further insights into the molecular pathways governing neutrophil swarming have been gained through IVM studies of genetically modified and transgenic neutrophils in mice and zebrafish (57, 60–62). Leukotriene B4 (LTB₄) has emerged as key requirement for initiating neutrophil swarming (60). Upon detecting tissue or cell damage, neutrophils rapidly release LTB₄ in response to elevated intracellular calcium levels (63). This triggers a second wave of neutrophils, amplifying recruitment and forming clusters to isolate the injury or infection from healthy tissue (63). Neutrophil chemoattractant synthesis in response to tissue damage is triggered in response to “calcium alarm” signals propagated through direct contact among pioneer swarming neutrophils via connexins (proteins forming gap junctions in the membranes of adjacent cells) (61). Connexins such as Cx43 enhance the synthesis of chemoattractants, coordinate neutrophil swarming and promote wound sterilization in the damaged area (61). Another trigger for swarm initiation is the release of cellular components (including chromatin, gasdermin, neutrophil elastase and myeloperoxidase) by pioneer neutrophils during NETosis (62). An earlier study differentiated between two types of NETosis: “suicidal” NETosis where neutrophils release NETs and undergo apoptosis, and “vital” where neutrophils remain motile and viable for some time after NETosis (64). However, it appears that, at least in this case, neutrophils undergoing “suicidal” rather than “vital” NETosis served as swarm initiators (62).
Regulation of the later stages of neutrophil swarming is still poorly understood but a recent study used a swarming-on-a-chip platform to demonstrate that inhibiting the Arp2/3 complex (which is crucial for regulating actin cytoskeleton dynamics) leads to cell death in densely clustered neutrophils within swarms (65). The pentose phosphate pathway plays an important role in supporting the viability and functionality of neutrophils during swarming by metabolically regulating the growth of neutrophil clusters and ROS production (65).
Although several studies have demonstrated how neutrophils can amplify swarming, there is considerably less clarity about how this self-amplifying behavior is turned off. In an elegant set of experiments, Lammermann and colleagues showed that neutrophils regulate their swarming behavior by desensitizing themselves to chemoattractants through a mechanism dependent on G protein-coupled receptor (GPCR) kinases (66). Specifically, neutrophils lacking GPCR kinase 2 (GRK2) were unable to desensitize to swarm-specific chemoattractants, although their response to other chemoattractants like LTB₄ and CXCL12 remained unaffected (66). Interestingly, GRK2-deficient neutrophils displayed increased mobility and greater infiltration into lymph nodes infected with Pseudomonas aeruginosa (P. aeruginosa), yet this increased presence paradoxically correlated with a reduced ability to clear the infection, highlighting the complex regulation of neutrophil functions during swarming (66).
Other immune cells can also help to limit the extent of neutrophil swarming. For instance, in a microlesion laser injury model targeting the murine peritoneal serosa (a scenario that simulates single cell death), resident tissue macrophages extended their membrane processes to cloak the injury site, effectively preventing neutrophil swarming (67). In contrast, macrolesion laser injuries (which involve larger areas of damage), triggered neutrophil swarming because the resident macrophages were unable to cloak the injured site (67). This dichotomy in neutrophil response to different types of injury illustrates how neutrophil activity can be finely tuned to the specific context, further emphasizing the importance of balanced regulation to avoid excessive tissue damage.
In contrast to macrophages, degranulating mast cells (MCs) can exploit neutrophil swarming to perpetuate allergic inflammation (68). Upon releasing LTB₄, MCs attract swarming neutrophils and trap them within themselves forming structures called MC intracellular traps (MITs). This process represents a novel twist on neutrophil swarming, where instead of isolating damage or pathogens, neutrophils are retained by MCs, contributing to sustained inflammation in allergic tissues (68). Together these studies reveal that while neutrophil swarming is crucial for an effective immune response, dysregulation of this process can lead to excessive neutrophil activity without necessarily improving infection clearance, indicating the need for a delicate balance in neutrophil function to ensure optimal immune outcomes.
Neutrophils in wound repair
Neutrophils play crucial roles in tissue repair beyond their immediate inflammatory response (39, 69, 70). They clear cellular debris, release growth factors like vascular endothelial growth factor A (VEGF-A) and matrix metalloproteases (e.g., MMP-8, MMP-9) to promote tissue regeneration and vascularization, and recruit macrophages for subsequent stages of repair (39, 71, 72). In a model of sterile liver injury, neutrophils contributed to revascularization and new collagen deposition by expressing the peptidase cathepsin C, which is essential for activating other neutrophil proteases (45).
Neutrophils also facilitate tissue repair by actively transporting extracellular matrix components from surrounding connective tissues to injured organs (73). This repair process is facilitated by the upregulation of collagen-binding integrins and the activation of heat shock factors, which enhanced the capacity of neutrophils to support tissue integrity during the healing phase (73). These studies highlight the critical role of neutrophils not only in initiating tissue repair but also in supporting long-term regeneration through mechanisms that facilitate both vascular and tissue remodeling.
In skin injury models nociceptive sensory neurons have recently emerged as a key regulator of neuro-immune interactions. During the healing process, these neurons communicate with immune cells in the skin through the neuropeptide calcitonin gene-related peptide (CGRP) (74). This neuropeptide regulates neutrophil and macrophage phenotype and turn-over, promoting tissue repair in skin and muscle injuries. Mechanistically, CGRP induces the expression of thrombospondin-1 (TSP-1) in these immune cells, limiting their accumulation and accelerating clearance in response to inflammatory cytokines. It also promotes neutrophil clearance via efferocytosis and shifts macrophages toward an anti-inflammatory, pro-repair phenotype (74).
While neutrophils are crucial for tissue repair, their activities can also lead to unintended tissue damage, particularly when their responses become dysregulated. For instance, during a methicillin-resistant Staphylococcus aureus (MRSA) infection, neutrophils released extracellular traps (NETs) in the liver vasculature, which, while trapping bacteria, also inflicted significant liver damage (75). Although DNase can remove the DNA component of NETs, it was less effective against harmful elements like histones and neutrophil elastase, which continued to damage tissue. Similarly, in a zebrafish tail fin regeneration model, neutrophil removal led to faster healing when CXCL8, a neutrophil recruiting chemokine, was knocked down, underscoring neutrophils’ dual role in both promoting repair and contributing to tissue injury (46). Understanding the factors that regulate their function is crucial for promoting wound healing in pathological and physiological conditions.
Neutrophils in vascular disease
Recent animal studies have linked neutrophils to systemic complications following vascular ischemia, such as strokes and myocardial infarctions (76, 77). One type of complication post-stroke is the increased risk of infections, and in clinical studies, stroke patients have lower serum IgA levels compared to healthy controls (78). Tuz et al., highlight an intriguing link between neutrophils and IgA levels. They noted a reduction in serum IgA in mice following stroke or myocardial infarction (76). This corresponded to reduced volume of Peyer’s patches in these animals (one of the sites of IgA production) as well as increased B cell apoptosis (Figure 1). The loss of IgA-producing plasma cells was linked to circulating DNA released during NETosis by neutrophils (which are activated by systemic inflammation post-stroke). Platelet aggregations induced by NETs may trigger oxygen and nutrient reduction and impact B cell survival in Peyer’s patches. The study suggests that neutrophil depletion or NET disruption could prevent IgA loss in patients and reduce the risk of infection.
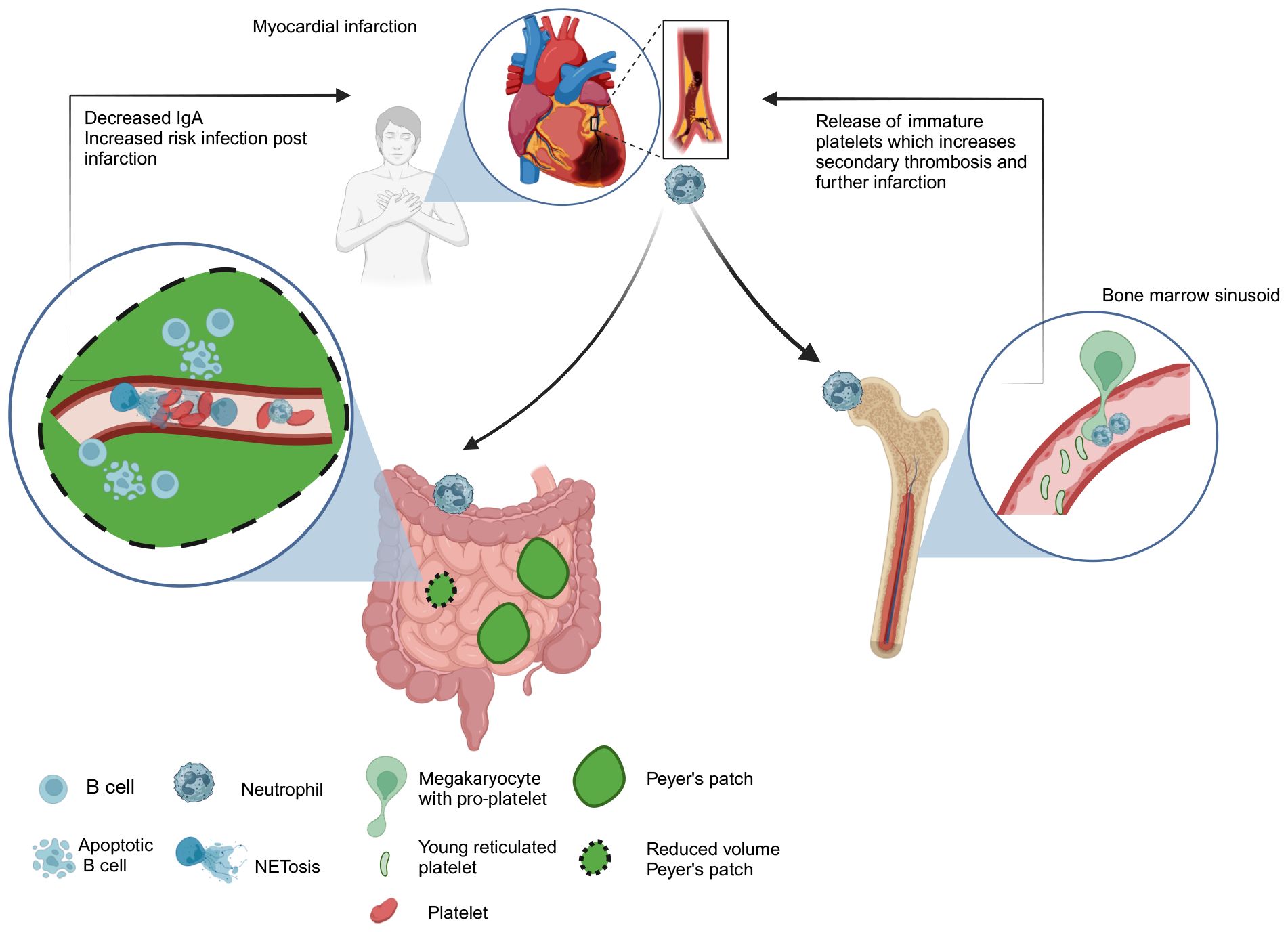
Figure 1. Neutrophil response to myocardial infarction. Systemic inflammation induced by stroke/myocardial infarction triggers NET release by circulating neutrophils. Increased circulating DNA levels lead to platelet aggregation in the vasculature causing microvascular thrombosis of Peyer’s patches. This could deprive them of nutrients and oxygen leading to the loss of IgA producing cells in Peyer’s patches and represents one potential mechanism contributing to increased risk of infection in patients post-infarction (76). Neutrophils may also increase the risk of secondary thrombosis after myocardial infarction by premature release of reticulated platelets from the bone marrow sinusoid. (77). Neutrophils regulate platelet production by “plucking” pro-platelets from megakaryocytes. This process becomes dysregulated following infarction and excessive release of prothrombogenic immature platelets leads to elevated risk of vascular thrombosis events post infarction (77).
In steady state neutrophils facilitate continuous release of platelets by “plucking” on megakaryocytes to drive platelet production but Petzold et al., showed that following myocardial infraction neutrophils can drive excessive release of prothrombogenic immature platelets, which increase risk of vascular thrombosis events post infarction (Figure 1) (77). Considering the critical roles that neutrophils play in mediating systemic effects following stroke and myocardial infarction, manipulating their number or activation state is emerging as a novel therapeutic strategy to reduce thromboischemic events.
Neutrophil fate
Although most neutrophils are thought to undergo apoptosis at the site of inflammation (79), accumulating evidence suggests that tissue neutrophils can have several other fates. For instance, in zebrafish and mouse models, neutrophils can migrate back into the vascular system after completing their function in inflamed tissues in a process called “reverse neutrophil migration” (45, 80–82). In a sterile liver injury model, instead of being phagocytosed neutrophils exited the injured tissue and re-entered the bloodstream via reverse transendothelial migration (rTEM) and then entered the lungs before eventually returning to the bone marrow, where they underwent apoptosis (45).This dynamic process facilitated restoring tissue integrity in the liver by removing activated neutrophils (45). Similarly, in a zebrafish model of spinal cord injury resolution of inflammation was aided by neutrophil transmigration away from the site of injury to various tissues (83).
However, activated neutrophils reentering the vasculature can contribute to systemic inflammation (84). This was observed following ultraviolet B (UVB) light exposure, where fluorescently labeled neutrophils emigrated from the skin (84). A subpopulation of these neutrophils exhibited rTEM, localizing in the kidney and contributing to tissue inflammation and injury (84).
During infections, neutrophils can also use the lymphatic system to egress from inflamed skin and transport antigens to draining lymph nodes in a process dependent on CD11b and CXCR4 (18). This mechanism may facilitate cellular communication between the injury site and lymphoid organs, thereby modulating the adaptive immune response. Another function of neutrophils in lymph nodes is to intercept and neutralize pathogens. For example, in the study looking at Staphylococcus aureus (S. aureus) infection, lymph node neutrophils prevented microbial dissemination to peripheral organs (85). Taken together these studies provide a more nuanced understanding of how tissue neutrophils can perpetuate not only local but also systemic inflammation through reverse transmigration and lymphatic migration extending their capacity to regulate subsequent innate and adaptive responses beyond the initial site of inflammation.
Neutrophils in tumors
Neutrophils infiltrate most solid tumors where they can either contribute to tumor growth and progression or stimulate anti-tumor immunity and tumor growth inhibition (86, 87). Recently, single cell RNA sequencing technology has significantly advanced our understanding of these seemingly contradictory roles by identifying different neutrophil subpopulations with distinct functional phenotypes (88–94). IVM can complement this approach by visualizing the dynamics and cellular interactions of the different intra-tumor neutrophil populations.
Analysis of the very early stages of oncogenesis in zebrafish revealed that neutrophils (as well as macrophages) formed cytoplasmic tethers and phagocytosed the transformed cells (6). As occurs in response to tissue injury, neutrophil recruitment to the tumors was dependent on hydrogen peroxide signaling (95). Inhibiting hydrogen peroxide reduced myeloid cell recruitment and the number of transformed cells suggesting that inflammatory signals from cancer cells recruit myeloid cells to support transformed cell development.
Even outside tumors, neutrophils can contribute to cancer metastasis by creating niches for circulating tumor cells. For instance, neutrophils activated by lipopolysaccharide (LPS) acted as a bridge/linker between circulating tumor cells and the hepatic sinusoid, contributing to metastases in the liver (96). Consistent with this observation, neutrophil depletion decreased the number of metastases (96, 97). Induction of NET formation in murine sepsis models revealed NETs as a potential mechanism whereby neutrophils trap circulating tumor cells in hepatic sinusoids (97, 98). Others have observed that metastatic murine 4T1 breast tumor cells induced NET formation in the lungs even in the absence of infection (99), suggesting that tumor cells can induce NETs thereby building their own metastatic niche. Conversely, NETs’ inhibition with neutrophil elastase inhibitor led to decreased hepatic and pulmonary metastases, while a similar effect was observed in mice deficient in a key enzyme required for NET formation (100), peptidyl-arginine deiminase type 4 (PAD4) (70).
IVM has provided additional clues about the influence of the tumor microenvironment (TME) on neutrophil dynamics and function (17, 101). In the murine oropharyngeal cancer (MOPC) model, intratumor neutrophils were found to move at lower velocity than peri-tumor neutrophils, suggesting that signals within the TME regulated their motility (101). As the tumors developed, neutrophils further decreased their velocity and directionality. Notably, peri-tumor but not tumor neutrophil recruitment was CXCR2-dependent suggesting that several distinct pathways govern neutrophil recruitment to the TME.
Limited motility was also observed in neutrophils infiltrating Lewis lung carcinoma (LLC) tumors (17) (Figures 2A–C). However, tumor neutrophil number and velocity were dramatically increased when an infectious stimulus was introduced into the TME (Figures 2A–C). Within 4 hours of the introduction of S. aureus bioparticles into tumors, neutrophils increased their speed and displacement (Figures 2B, C), but in contrast to what was previously observed in infections, tumor neutrophils did not form dynamic swarms. By twenty-four hours after S. aureus bioparticle injection neutrophil motility was significantly reduced compared to the four-hour time point, suggesting that chronic inflammation in the TME limits their motility. In addition, neutrophils were observed to interact with tumor cells and tumor cells adjacent to neutrophil clusters formed blebs, suggesting that they were undergoing cell death.
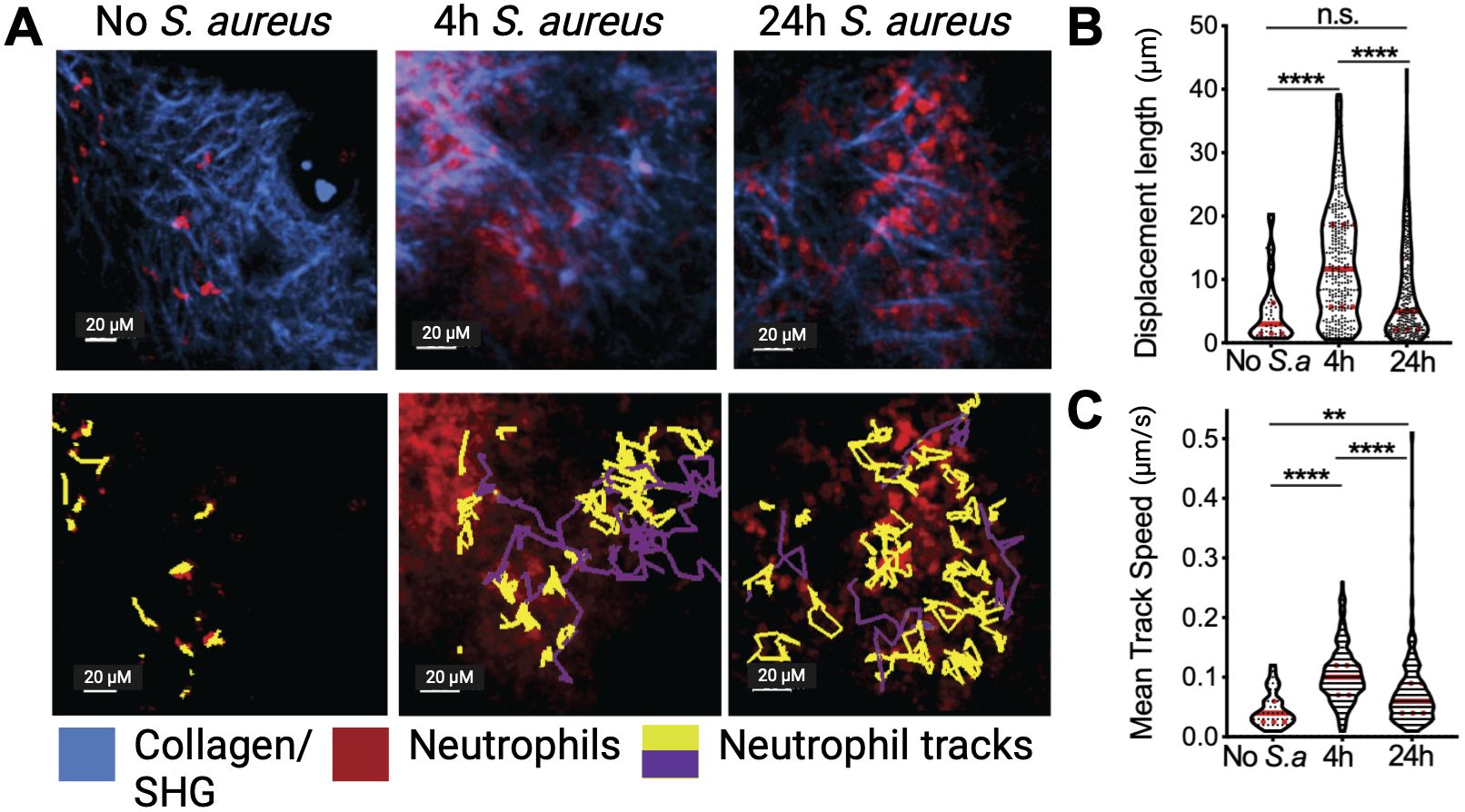
Figure 2. Tumor neutrophil dynamics in vivo. (A) Neutrophils (red) were visualized in a steady state or treated with intratumor injection of S. aureus bioparticles (S.a) for 4 and 24 h in Lewis Lung Cancer (LLC) tumors using intravital two-photon microscopy. Yellow tracks indicate neutrophils with confined motility, and purple tracks indicate migratory neutrophils. Second Harmonic Generation (SHG)/collagen - blue. Bar represents 20 µm. (B) Track displacement length of intratumoral neutrophils. (C) Mean track speed of intratumoral neutrophils. Data from at least 4 independent imaging experiments per time point were analyzed using a one-way ANOVA with Dunn’s correction for multiple comparisons (B, C). Median and quartiles are shown. **P ≤ 0.01, ****P ≤ 0.0001; n.s. not significant. Adapted from Yam et al. (17).
Neutrophils in contact with tumor targets have been found to have a number of tumoricidal mechanisms that include releasing ROS (102–104) or neutrophil elastase (ELANE) (105, 106). They also prime CD8+ T cells with tumor associated antigens and can kill opsonized tumor antigens by antibody-dependent cellular cytotoxicity (ADCC) (107). IVM has shown that antibody-opsonized cancer cells can be removed by neutrophils via trogocytosis, a process in which immune cells acquire a membrane from another cell by an endocytic process (108, 109). Once neutrophils have trogocytosed tumor cells, the latter lose cellular integrity and die. Understanding how neutrophils destroy opsonized tumor cells may improve therapeutic antibody approaches for tumor treatment.
IVM has also contributed to understanding the mechanisms by which neutrophils can modulate cancer therapies. The use of anti-vascular endothelial growth factor (VEGF) was proposed as a possible new form of anti-tumor therapy (110). However, in zebrafish models, anti-VEGF therapy increased neutrophil infiltration, which remodeled tumor collagen to create a metastatic niche (111).
c-MET signaling has been used as an oncogenic target for patients with cancers. Inhibition of c-MET decreased neutrophil recruitment into tumors and their draining lymph nodes and improved T cell activation (112). An IVM-based approach showed that T cell activation was suppressed in the absence of c-MET inhibition by direct contact between T cells and neutrophils. Neutrophil mobility in the vascular space was also impaired in metastatic tumor mouse models (113). This was thought to be due to conformational inactivation of its β2-integrin (Mac-1/CD11b and LFA-1/CD11a), which was recapitulated by treating mice with granulocyte colony stimulating factor (GCSF). Based on these findings, it was proposed that the paraneoplastic effect of GCSF produced endogenously by tumors would cause vascular congestion impairing neutrophil vascular mobility, thereby blocking CD8+ T cell infiltration. In other words, neutrophils, by blocking effective CD8+ T cell infiltration of tumors, could promote resistance to checkpoint inhibitor therapies. In the cancer space, imaging technologies have broadened our understanding of how neutrophils interact with cancer cells and contribute to tumor progression and metastasis, underscoring their significance as potential targets for innovative cancer therapies.
Modeling neutrophil behavior in silico
Mathematical modeling and simulation techniques have significantly advanced our understanding of complex cellular behavior in immune defense (114). Statistical analysis and modeling of cell migratory patterns have been improved through the use of detailed, spatially resolved in vivo data that capture single cells moving through tissue and interacting with each other (115). IVM can record the dynamic movement of fluorescently labeled cells in their native environment (59) and the cell’s x, y, and potentially z coordinates, depending on the dimensions recorded, can be determined for each time point in the imaging sequence (Figures 3A, B). Using statistical analysis, we can then calculate various metrics of the cell’s movements, such as average turning speeds, velocities, and displacements or distances traveled (115, 116) (Figures 2B, C). This can be expanded to include detailed analysis of hundred cells (73, 77) from the same imaging volume to study how collective migration, such as swarming, is coordinated. This approach has shown that neutrophils can exhibit diverse behaviors in inflamed tissues, including a pathogenic sessile state localized near endothelial junctions, which contrasts with their typical migratory role (20). Notably, this study also demonstrated that neutrophil morphology, the center of mass, and the height-to-length ratio were linked to their behavior. Therefore, IVM coupled with statistical analysis can provide additional parameters to classify neutrophils and their associated function and help identify pathogenic and non-pathogenic states.
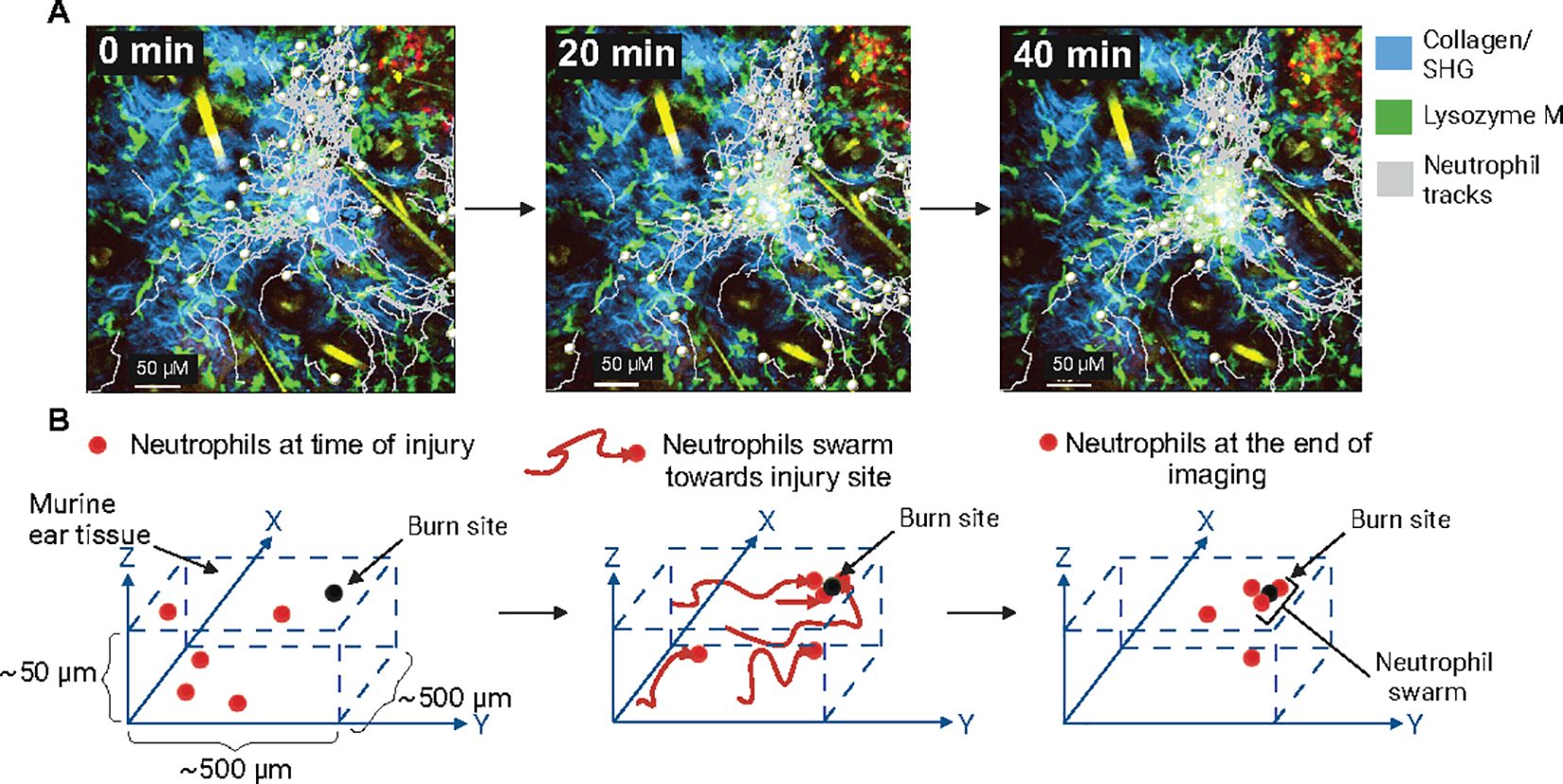
Figure 3. Neutrophil swarming and intravital imaging of neutrophil dynamics (A). Lysozyme M (LysM) positive neutrophils (green) migrating towards the site of sterile laser murine ear injury (track marks grey) to form swarms. Second harmonic generation (blue), tick marks are 50 µM apart, and images were acquired by IVM. (unpublished data). (B) Schematic representation of neutrophil swarming towards a sterile laser injury. Neutrophils are tracked, moving towards the site of inflammation in 3D tissue.
Following the quantitative analysis of IVM data, cell motility can create mathematical representations or ‘models’ to simulate and predict the movement of immune cell populations and their responses to environmental stimuli (117). One commonly used modeling approach to studying immune cell behavior is agent-based models (ABMs) (114). ABMs characterize discrete agents (for example, cells) with their own set of rules or behaviors (for example, velocity) and simulate the interactions of these agents within a defined environment. IVM data have been combined with the ABM approach to help understand leukocyte behavior, migration patterns, and search strategies in various contexts. Read et al. (21) found that the migration of neutrophils under inflammatory conditions was best captured by ABMs that account for differences in speed and direction changes within the population. They found that neutrophil populations are statistically heterogeneous in rotational and translational speeds, and directional persistence. Neutrophils had inversely correlated translational and turn speeds, meaning cells moving quickly do not perform large reorientations and vice versa. This pattern may stem from limitations in cytoskeletal remodeling or reflect the need to navigate environmental obstacles, necessitating slower movement to maneuver effectively around them. Similar findings were observed in the exploration patterns of T cells in larval zebrafish, which showed a broad distribution of speeds within cell populations and that, like neutrophils, fast-moving T cells make shallow turns while slow-moving T cells make bigger turns (118). These observations made from modeling T cell migration suggest that there is an actin-dependent intrinsic component of cells that jointly controls speed and directional persistence. Given the growing use of ABMs and IVM to model leukocyte migration behavior, there is significant potential to apply these models to study neutrophil dynamics in tumors, infections, and injuries.
Currently, IVM data often requires time-consuming manual analysis, which limits the number of parameters that can be analyzed (i.e., type and number of cells tracked). Application of models such as ABM has the potential to increase the throughput of data acquired through IVM. Others have trained computer learning networks with manually labeled images to track neutrophils (19). Refinement of neutrophil modeling and application of machine learning methodologies can provide tools for higher throughput spatial and kinetic subtyping of neutrophils.
Technological challenges
However, developing reliable methods for statistical analysis and modeling immune cells using in vivo imaging data presents several challenges. The choice of quantitative metrics can influence which model best describes a cell’s motility (21, 119). Furthermore, in situ fluorescence microscopy, which is necessary to observe single cells over various spatial scales, faces technical limitations, including the need for micron-scale resolution over millimeter-scale fields of view (118). Long-term imaging can also cause photodamage to cells (120), altering their behavior and, therefore, negatively impacting the model’s validity. These constraints underscore the need for improved methods and complementary metrics in studying cell migration. For example, data from IVM modeling could be used to understand how to create organoid environments that better mimic in vivo physiological and pathological conditions (121). Combining single cell RNA-sequencing approaches with intravital imaging could help correlate in vivo heterogeneity with the diversity of neutrophil populations identified by single-cell sequencing. Although it is technically challenging to link an individual cell’s trajectory and gene expression, in vivo photolabeling could be used to mark cells that are swarming or exhibit specific motility patterns as a prelude to sequencing them.
Discussion
Advances in IVM and fluorescent neutrophil-reporter animal models have provided a highly detailed window into the role of neutrophils in health and disease, which means there is a rich vein of neutrophil spatial-temporal data in response to different stimuli. IVM showed how neutrophils’ functions (therefore, their temporal and spatial kinetics) are shaped by their tissue environment. In some conditions their spatial kinetics are highly orchestrated and conserved, as evidenced by their ability to form swarms in a staged fashion. In other pathophysiological conditions, such as neutrophil responses to cancers, we are yet to identify a signature balletic movement. Detailed insights into neutrophil motility patterns and interactions could enable the design of targeted therapies to modulate their activity, potentially improving outcomes in inflammatory and infectious conditions as well as solid tumors.
Author contributions
AY: Writing – review & editing, Writing – original draft. AJ: Writing – review & editing, Writing – original draft. CG: Writing – review & editing, Writing – original draft. TC: Writing – review & editing, Writing – original draft, Conceptualization.
Funding
The author(s) declare financial support was received for the research, authorship, and/or publication of this article. This research was supported by funding to T.C. from the National Breast Cancer Foundation (IIRS-22-053), Tour De Cure RSP-010-2024 and ARC Discovery Project Grant DP220102278. AOY was supported by the Phil Salter Immuno-Oncology Fellowship, University of New South Wales Triple-I Seed Grant and St Vincent's Clinic Research Foundation Grant. CG was supported by the Australian Government Research Training Program.
Acknowledgments
We thank Prof. Anthony Basten for critical reading of the manuscript.
Conflict of interest
The authors declare that the research was conducted in the absence of any commercial or financial relationships that could be construed as a potential conflict of interest.
Publisher’s note
All claims expressed in this article are solely those of the authors and do not necessarily represent those of their affiliated organizations, or those of the publisher, the editors and the reviewers. Any product that may be evaluated in this article, or claim that may be made by its manufacturer, is not guaranteed or endorsed by the publisher.
References
1. Bain B, Seed M, Godsland I. Normal values for peripheral blood white cell counts in women of four different ethnic origins. J Clin Pathol. (1984) 37:188–93. doi: 10.1136/jcp.37.2.188
2. Doeing DC, Borowicz JL, Crockett ET. Gender dimorphism in differential peripheral blood leukocyte counts in mice using cardiac, tail, foot, and saphenous vein puncture methods. BMC Clin Pathol. (2003) 3:3. doi: 10.1186/1472-6890-3-3
3. Chtanova T, Schaeffer M, Han SJ, van Dooren GG, Nollmann M, Herzmark P, et al. Dynamics of neutrophil migration in lymph nodes during infection. Immunity. (2008) 29:487–96. doi: 10.1016/j.immuni.2008.07.012
4. Niethammer P, Grabher C, Look AT, Mitchison TJ. A tissue-scale gradient of hydrogen peroxide mediates rapid wound detection in zebrafish. Nature. (2009) 459:996–9. doi: 10.1038/nature08119
5. de Oliveira S, Reyes-Aldasoro CC, Candel S, Renshaw SA, Mulero V, Calado A. Cxcl8 (IL-8) mediates neutrophil recruitment and behavior in the zebrafish inflammatory response. J Immunol. (2013) 190:4349–59. doi: 10.4049/jimmunol.1203266
6. Feng Y, Santoriello C, Mione M, Hurlstone A, Martin P. Live imaging of innate immune cell sensing of transformed cells in zebrafish larvae: parallels between tumor initiation and wound inflammation. PLoS Biol. (2010) 8:e1000562. doi: 10.1371/journal.pbio.1000562
7. Segal AW. How neutrophils kill microbes. Annu Rev Immunol. (2005) 23:197–223. doi: 10.1146/annurev.immunol.23.021704.115653
8. Takei H, Araki A, Watanabe H, Ichinose A, Sendo F. Rapid killing of human neutrophils by the potent activator phorbol 12-myristate 13-acetate (PMA) accompanied by changes different from typical apoptosis or necrosis. J Leukoc Biol. (1996) 59:229–40. doi: 10.1002/jlb.59.2.229
9. Babior BM, Curnutte JT, McMurrich BJ. The particulate superoxide-forming system from human neutrophils. Properties of the system and further evidence supporting its participation in the respiratory burst. J Clin Invest. (1976) 58:989–96. doi: 10.1172/JCI108553
10. Babior BM, Kipnes RS, Curnutte JT. Biological defense mechanisms. The production by leukocytes of superoxide, a potential bactericidal agent. J Clin Invest. (1973) 52:741–4. doi: 10.1172/JCI107236
11. Iyer GYN, Quastel JH. Nadph and nadh oxidation by Guinea pig polymorphonuclear leucocytes. Can J Biochem Physiol. (1963) 41:427–34. doi: 10.1139/o63-051
12. Faust N, Varas F, Kelly LM, Heck S, Graf T. Insertion of enhanced green fluorescent protein into the lysozyme gene creates mice with green fluorescent granulocytes and macrophages. Blood. (2000) 96:719–26. doi: 10.1182/blood.V96.2.719.014k29_719_726
13. Clausen BE, Burkhardt C, Reith W, Renkawitz R, Forster I. Conditional gene targeting in macrophages and granulocytes using LysMcre mice. Transgenic Res. (1999) 8:265–77. doi: 10.1023/A:1008942828960
14. Hasenberg A, Hasenberg M, Mann L, Neumann F, Borkenstein L, Stecher M, et al. Catchup: a mouse model for imaging-based tracking and modulation of neutrophil granulocytes. Nat Methods. (2015) 12:445–52. doi: 10.1038/nmeth.3322
15. Mihlan M, Safaiyan S, Stecher M, Paterson N, Lämmermann T. Surprises from intravital imaging of the innate immune response. Annu Rev Cell Dev Biol. (2022) 38:467–89. doi: 10.1146/annurev-cellbio-120420-112849
16. Zaid A. Intravital imaging of skin infections. Cell Immunol. (2020) 350:103913. doi: 10.1016/j.cellimm.2019.04.001
17. Yam AO, Bailey J, Lin F, Jakovija A, Youlten SE, Counoupas C, et al. Neutrophil conversion to a tumor-killing phenotype underpins effective microbial therapy. Cancer Res. (2023) 83:1315–28. doi: 10.1158/0008-5472.CAN-21-4025
18. Hampton HR, Bailey J, Tomura M, Brink R, Chtanova T. Microbe-dependent lymphatic migration of neutrophils modulates lymphocyte proliferation in lymph nodes. Nat Commun. (2015) 6:7139. doi: 10.1038/ncomms8139
19. R Moghadam M, Chen YP. Tracking leukocytes in intravital time lapse images using 3D cell association learning network. Artif Intell Med. (2021) 118:102129. doi: 10.1016/j.artmed.2021.102129
20. Crainiciuc G, Palomino-Segura M, Molina-Moreno M, Sicilia J, Aragones DG, Li JLY, et al. Behavioural immune landscapes of inflammation. Nature. (2022) 601:415–21. doi: 10.1038/s41586-021-04263-y
21. Read MN, Bailey J, Timmis J, Chtanova T. Leukocyte motility models assessed through simulation and multi-objective optimization-based model selection. PLoS Comput Biol. (2016) 12:e1005082. doi: 10.1371/journal.pcbi.1005082
22. Scheele C, Herrmann D, Yamashita E, Celso CL, Jenne CN, Oktay MH, et al. Multiphoton intravital microscopy of rodents. Nat Rev Methods Primers. (2022) 2. doi: 10.1038/s43586-022-00168-w
23. Xu C, Nedergaard M, Fowell DJ, Friedl P, Ji N. Multiphoton fluorescence microscopy for in vivo imaging. Cell. (2024) 187:4458–87. doi: 10.1016/j.cell.2024.07.036
24. Hoover EE, Squier JA. Advances in multiphoton microscopy technology. Nat Photonics. (2013) 7:93–101. doi: 10.1038/nphoton.2012.361
25. Chen IH, Chu SW, Sun CK, Cheng PC, Lin BL. Wavelength dependent damage in biological multi-photon confocal microscopy: A micro-spectroscopic comparison between femtosecond Ti:sapphire and Cr:forsterite laser sources. Optical Quantum Electron. (2002) 34:1251–66. doi: 10.1023/A:1021303426482
26. Helmchen F, Denk W. Deep tissue two-photon microscopy. Nat Methods. (2005) 2:932–40. doi: 10.1038/nmeth818
27. Ou Z, Duh Y-S, Rommelfanger NJ, Keck CHC, Jiang S, Brinson K, et al. Achieving optical transparency in live animals with absorbing molecules. Science. (2024) 385:eadm6869. doi: 10.1126/science.adm6869
28. Daley JM, Thomay AA, Connolly MD, Reichner JS, Albina JE. Use of Ly6G-specific monoclonal antibody to deplete neutrophils in mice. J Leukoc Biol. (2008) 83:64–70. doi: 10.1189/jlb.0407247
29. Fleming TJ, Fleming ML, Malek TR. Selective expression of Ly-6G on myeloid lineage cells in mouse bone marrow. RB6-8C5 mAb to granulocyte-differentiation antigen (Gr-1) detects members of the Ly-6 family. J Immunol. (1993) 151:2399–408. doi: 10.4049/jimmunol.151.5.2399
30. Passegue E, Wagner EF, Weissman IL. JunB deficiency leads to a myeloproliferative disorder arising from hematopoietic stem cells. Cell. (2004) 119:431–43. doi: 10.1016/j.cell.2004.10.010
31. Reber LL, Gillis CM, Starkl P, Jonsson F, Sibilano R, Marichal T, et al. Neutrophil myeloperoxidase diminishes the toxic effects and mortality induced by lipopolysaccharide. J Exp Med. (2017) 214:1249–58. doi: 10.1084/jem.20161238
32. Tomura M, Yoshida N, Tanaka J, Karasawa S, Miwa Y, Miyawaki A, et al. Monitoring cellular movement in vivo with photoconvertible fluorescence protein "Kaede" transgenic mice. Proc Natl Acad Sci U.S.A. (2008) 105:10871–6. doi: 10.1073/pnas.0802278105
33. Ando R, Hama H, Yamamoto-Hino M, Mizuno H, Miyawaki A. An optical marker based on the UV-induced green-to-red photoconversion of a fluorescent protein. Proc Natl Acad Sci U.S.A. (2002) 99:12651–6. doi: 10.1073/pnas.202320599
34. Tsutsui H, Karasawa S, Shimizu H, Nukina N, Miyawaki A. Semi-rational engineering of a coral fluorescent protein into an efficient highlighter. EMBO Rep. (2005) 6:233–8. doi: 10.1038/sj.embor.7400361
35. Patterson GH, Lippincott-Schwartz J. A photoactivatable GFP for selective photolabeling of proteins and cells. Science. (2002) 297:1873–7. doi: 10.1126/science.1074952
36. Naumenko V, Nikitin A, Garanina A, Melnikov P, Vodopyanov S, Kapitanova K, et al. Neutrophil-mediated transport is crucial for delivery of short-circulating magnetic nanoparticles to tumors. Acta Biomater. (2020) 104:176–87. doi: 10.1016/j.actbio.2020.01.011
37. Chu D, Dong X, Zhao Q, Gu J, Wang Z. Photosensitization priming of tumor microenvironments improves delivery of nanotherapeutics via neutrophil infiltration. Adv Mater. (2017) 29. doi: 10.1002/adma.201701021
38. Pellico J, Lechuga-Vieco AV, Almarza E, Hidalgo A, Mesa-Nunez C, Fernandez-Barahona I, et al. In vivo imaging of lung inflammation with neutrophil-specific (68)Ga nano-radiotracer. Sci Rep. (2017) 7:13242. doi: 10.1038/s41598-017-12829-y
39. Siwicki M, Kubes P. Neutrophils in host defense, healing, and hypersensitivity: Dynamic cells within a dynamic host. J Allergy Clin Immunol. (2023) 151:634–55. doi: 10.1016/j.jaci.2022.12.004
40. Chadwick JW, Fine N, Khoury W, Tasevski N, Sun CX, Boroumand P, et al. Tissue-specific murine neutrophil activation states in health and inflammation. J Leukoc Biol. (2021) 110:187–95. doi: 10.1002/JLB.4AB1020-248RRR
41. Tu H, Ren H, Jiang J, Shao C, Shi Y, Li P. Dying to defend: neutrophil death pathways and their implications in immunity. Adv Sci (Weinh). (2024) 11:e2306457. doi: 10.1002/advs.202306457
42. Rosales C. Neutrophil: A cell with many roles in inflammation or several cell types? Front Physiol. (2018) 9:113. doi: 10.3389/fphys.2018.00113
43. Jorch SK, Kubes P. An emerging role for neutrophil extracellular traps in noninfectious disease. Nat Med. (2017) 23:279–87. doi: 10.1038/nm.4294
44. Papayannopoulos V. Neutrophil extracellular traps in immunity and disease. Nat Rev Immunol. (2018) 18:134–47. doi: 10.1038/nri.2017.105
45. Wang J, Hossain M, Thanabalasuriar A, Gunzer M, Meininger C, Kubes P. Visualizing the function and fate of neutrophils in sterile injury and repair. Science. (2017) 358:111–6. doi: 10.1126/science.aam9690
46. Li L, Yan B, Shi YQ, Zhang WQ, Wen ZL. Live imaging reveals differing roles of macrophages and neutrophils during zebrafish tail fin regeneration. J Biol Chem. (2012) 287:25353–60. doi: 10.1074/jbc.M112.349126
47. Filippi MD. Neutrophil transendothelial migration: updates and new perspectives. Blood. (2019) 133:2149–58. doi: 10.1182/blood-2018-12-844605
48. Marki A, Esko JD, Pries AR, Ley K. Role of the endothelial surface layer in neutrophil recruitment. J Leukoc Biol. (2015) 98:503–15. doi: 10.1189/jlb.3MR0115-011R
49. Hentzen ER, Neelamegham S, Kansas GS, Benanti JA, McIntire LV, Smith CW, et al. Sequential binding of CD11a/CD18 and CD11b/CD18 defines neutrophil capture and stable adhesion to intercellular adhesion molecule-1. Blood. (2000) 95:911–20. doi: 10.1182/blood.V95.3.911.003k36_911_920
50. Wang X, Hossain M, Bogoslowski A, Kubes P, Irimia D. Chemotaxing neutrophils enter alternate branches at capillary bifurcations. Nat Commun. (2020) 11:2385. doi: 10.1038/s41467-020-15476-6
51. Mukhopadhyay A, Tsukasaki Y, Chan WC, Le JP, Kwok ML, Zhou J, et al. trans-Endothelial neutrophil migration activates bactericidal function via Piezo1 mechanosensing. Immunity. (2024) 57:52–67.e10. doi: 10.1016/j.immuni.2023.11.007
52. Masgrau-Alsina S, Wackerbarth LM, Lim DS, Sperandio M. MST1 controls murine neutrophil homeostasis via the G-CSFR/STAT3 axis. Front Immunol. (2022) 13:1038936. doi: 10.3389/fimmu.2022.1038936
53. Kurz AR, Pruenster M, Rohwedder I, Ramadass M, Schafer K, Harrison U, et al. MST1-dependent vesicle trafficking regulates neutrophil transmigration through the vascular basement membrane. J Clin Invest. (2016) 126:4125–39. doi: 10.1172/JCI87043
54. Castanheira FVS, Nguyen R, Willson M, Davoli-Ferreira M, David BA, Kelly MM, et al. Intravital imaging of three different microvascular beds in SARS-CoV-2-infected mice. Blood Adv. (2023) 7:4170–81. doi: 10.1182/bloodadvances.2022009430
55. Mihlan M, Glaser KM, Epple MW, Lammermann T. Neutrophils: amoeboid migration and swarming dynamics in tissues. Front Cell Dev Biol. (2022) 10:871789. doi: 10.3389/fcell.2022.871789
56. McDonald B, Pittman K, Menezes GB, Hirota SA, Slaba I, Waterhouse CC, et al. Intravascular danger signals guide neutrophils to sites of sterile inflammation. Science. (2010) 330:362–6. doi: 10.1126/science.1195491
57. Georgantzoglou A, Poplimont H, Walker HA, Lammermann T, Sarris M. A two-step search and run response to gradients shapes leukocyte navigation in vivo. J Cell Biol. (2022) 221. doi: 10.1083/jcb.202103207
58. Lammermann T. In the eye of the neutrophil swarm-navigation signals that bring neutrophils together in inflamed and infected tissues. J Leukoc Biol. (2016) 100:55–63. doi: 10.1189/jlb.1MR0915-403
59. Kienle K, Lammermann T. Neutrophil swarming: an essential process of the neutrophil tissue response. Immunol Rev. (2016) 273:76–93. doi: 10.1111/imr.12458
60. Lammermann T, Afonso PV, Angermann BR, Wang JM, Kastenmuller W, Parent CA, et al. Neutrophil swarms require LTB4 and integrins at sites of cell death in vivo. Nature. (2013) 498:371–5. doi: 10.1038/nature12175
61. Poplimont H, Georgantzoglou A, Boulch M, Walker HA, Coombs C, Papaleonidopoulou F, et al. Neutrophil swarming in damaged tissue is orchestrated by connexins and cooperative calcium alarm signals. Curr Biol. (2020) 30:2761–2776.e7. doi: 10.1016/j.cub.2020.05.030
62. Isles HM, Loynes CA, Alasmari S, Kon FC, Henry KM, Kadochnikova A, et al. Pioneer neutrophils release chromatin within in vivo swarms. Elife. (2021) 10. doi: 10.7554/eLife.68755
63. Glaser KM, Mihlan M, Lammermann T. Positive feedback amplification in swarming immune cell populations. Curr Opin Cell Biol. (2021) 72:156–62. doi: 10.1016/j.ceb.2021.07.009
64. Yipp BG, Petri B, Salina D, Jenne CN, Scott BN, Zbytnuik LD, et al. Infection-induced NETosis is a dynamic process involving neutrophil multitasking in vivo. Nat Med. (2012) 18:1386–93. doi: 10.1038/nm.2847
65. Glaser KM, Doon-Ralls J, Walters N, Rima XY, Rambold AS, Reategui E, et al. Arp2/3 complex and the pentose phosphate pathway regulate late phases of neutrophil swarming. iScience. (2024) 27:108656. doi: 10.1016/j.isci.2023.108656
66. Kienle K, Glaser KM, Eickhoff S, Mihlan M, Knopper K, Reategui E, et al. Neutrophils self-limit swarming to contain bacterial growth in vivo. Science. (2021) 372. doi: 10.1126/science.abe7729
67. Uderhardt S, Martins AJ, Tsang JS, Lammermann T, Germain RN. Resident macrophages cloak tissue microlesions to prevent neutrophil-driven inflammatory damage. Cell. (2019) 177:541–555.e17. doi: 10.1016/j.cell.2019.02.028
68. Mihlan M, Wissmann S, Gavrilov A, Kaltenbach L, Britz M, Franke K, et al. Neutrophil trapping and nexocytosis, mast cell-mediated processes for inflammatory signal relay. Cell. (2024) 187, (19):5316–5335.e28. doi: 10.1016/j.cell.2024.07.014
69. Peiseler M, Kubes P. More friend than foe: the emerging role of neutrophils in tissue repair. J Clin Invest. (2019) 129:2629–39. doi: 10.1172/JCI124616
70. Phillipson M, Kubes P. The healing power of neutrophils. Trends Immunol. (2019) 40:635–47. doi: 10.1016/j.it.2019.05.001
71. Gong Y, Koh DR. Neutrophils promote inflammatory angiogenesis via release of preformed VEGF in an in vivo corneal model. Cell Tissue Res. (2010) 339:437–48. doi: 10.1007/s00441-009-0908-5
72. Wang J. Neutrophils in tissue injury and repair. Cell Tissue Res. (2018) 371:531–9. doi: 10.1007/s00441-017-2785-7
73. Fischer A, Wannemacher J, Christ S, Koopmans T, Kadri S, Zhao J, et al. Neutrophils direct preexisting matrix to initiate repair in damaged tissues. Nat Immunol. (2022) 23:518–31. doi: 10.1038/s41590-022-01166-6
74. Lu Y-Z, Nayer B, Singh SK, Alshoubaki YK, Yuan E, Park AJ, et al. CGRP sensory neurons promote tissue healing via neutrophils and macrophages. Nature. (2024) 628:604–11. doi: 10.1038/s41586-024-07237-y
75. Kolaczkowska E, Jenne CN, Surewaard BG, Thanabalasuriar A, Lee WY, Sanz MJ, et al. Molecular mechanisms of NET formation and degradation revealed by intravital imaging in the liver vasculature. Nat Commun. (2015) 6:6673. doi: 10.1038/ncomms7673
76. Tuz AA, Ghosh S, Karsch L, Ttoouli D, Sata SP, Ulusoy Ö, et al. Stroke and myocardial infarction induce neutrophil extracellular trap release disrupting lymphoid organ structure and immunoglobulin secretion. Nat Cardiovasc Res. (2024) 3:525–40. doi: 10.1038/s44161-024-00462-8
77. Petzold T, Zhang Z, Ballesteros I, Saleh I, Polzin A, Thienel M, et al. Neutrophil "plucking" on megakaryocytes drives platelet production and boosts cardiovascular disease. Immunity. (2022) 55, (12):2285–2299.e7. doi: 10.1016/j.immuni.2022.10.001
78. McCulloch L, Allan SM, Emsley HC, Smith CJ, McColl BW. Interleukin-1 receptor antagonist treatment in acute ischaemic stroke does not alter systemic markers of anti-microbial defence. F1000Res. (2019) 8:1039. doi: 10.12688/f1000research.19308.2
79. de Oliveira S, Rosowski EE, Huttenlocher A. Neutrophil migration in infection and wound repair: going forward in reverse. Nat Rev Immunol. (2016) 16:378–91. doi: 10.1038/nri.2016.49
80. Woodfin A, Voisin MB, Beyrau M, Colom B, Caille D, Diapouli FM, et al. The junctional adhesion molecule JAM-C regulates polarized transendothelial migration of neutrophils in vivo. Nat Immunol. (2011) 12:761–9. doi: 10.1038/ni.2062
81. Mathias JR, Perrin BJ, Liu TX, Kanki J, Look AT, Huttenlocher A. Resolution of inflammation by retrograde chemotaxis of neutrophils in transgenic zebrafish. J Leukoc Biol. (2006) 80:1281–8. doi: 10.1189/jlb.0506346
82. Colom B, Bodkin JV, Beyrau M, Woodfin A, Ody C, Rourke C, et al. Leukotriene B4-neutrophil elastase axis drives neutrophil reverse transendothelial cell migration in vivo. Immunity. (2015) 42:1075–86. doi: 10.1016/j.immuni.2015.05.010
83. de Sena-Tomás C, Lameira LR, Taborda PN, Laborde A, Orger M, de Oliveira S, et al. Neutrophil immune profile controls spinal cord regeneration in zebrafish. bioRxiv. (2024) 2024:1.17.576035. doi: 10.1101/2024.01.17.576035
84. Skopelja-Gardner S, Tai J, Sun X, Tanaka L, Kuchenbecker JA, Snyder JM, et al. Acute skin exposure to ultraviolet light triggers neutrophil-mediated kidney inflammation. Proc Natl Acad Sci U.S.A. (2021) 118. doi: 10.1073/pnas.2019097118
85. Bogoslowski A, Butcher EC, Kubes P. Neutrophils recruited through high endothelial venules of the lymph nodes via PNAd intercept disseminating Staphylococcus aureus. Proc Natl Acad Sci U.S.A. (2018) 115:2449–54. doi: 10.1073/pnas.1715756115
86. Sagiv JY, Michaeli J, Assi S, Mishalian I, Kisos H, Levy L, et al. Phenotypic diversity and plasticity in circulating neutrophil subpopulations in cancer. Cell Rep. (2015) 10:562–73. doi: 10.1016/j.celrep.2014.12.039
87. Fridlender ZG, Sun J, Kim S, Kapoor V, Cheng G, Ling L, et al. Polarization of tumor-associated neutrophil phenotype by TGF-beta: "N1" versus "N2" TAN. Cancer Cell. (2009) 16:183–94. doi: 10.1016/j.ccr.2009.06.017
88. Ng MSF, Kwok I, Tan L, Shi C, Cerezo-Wallis D, Tan Y, et al. Deterministic reprogramming of neutrophils within tumors. Science. (2024) 383:eadf6493. doi: 10.1126/science.adf6493
89. Zilionis R, Engblom C, Pfirschke C, Savova V, Zemmour D, Saatcioglu HD, et al. Single-cell transcriptomics of human and mouse lung cancers reveals conserved myeloid populations across individuals and species. Immunity. (2019) 50:1317–1334.e10. doi: 10.1016/j.immuni.2019.03.009
90. Wang C, Yu Q, Song T, Wang Z, Song L, Yang Y, et al. The heterogeneous immune landscape between lung adenocarcinoma and squamous carcinoma revealed by single-cell RNA sequencing. Signal Transduct Target Ther. (2022) 7:289. doi: 10.1038/s41392-022-01130-8
91. Salcher S, Sturm G, Horvath L, Untergasser G, Kuempers C, Fotakis G, et al. High-resolution single-cell atlas reveals diversity and plasticity of tissue-resident neutrophils in non-small cell lung cancer. Cancer Cell. (2022) 40:1503–1520.e8. doi: 10.1016/j.ccell.2022.10.008
92. Wu Y, Ma J, Yang X, Nan F, Zhang T, Ji S, et al. Neutrophil profiling illuminates anti-tumor antigen-presenting potency. Cell. (2024) 187:1422–1439.e24. doi: 10.1016/j.cell.2024.02.005
93. Fetit R, McLaren AS, White M, Mills ML, Falconer J, Cortes-Lavaud X, et al. Characterizing neutrophil subtypes in cancer using scRNA sequencing demonstrates the importance of IL1beta/CXCR2 axis in generation of metastasis-specific neutrophils. Cancer Res Commun. (2024) 4:588–606. doi: 10.1158/2767-9764.CRC-23-0319
94. Horvath L, Puschmann C, Scheiber A, Martowicz A, Sturm G, Trajanoski Z, et al. Beyond binary: bridging neutrophil diversity to new therapeutic approaches in NSCLC. Trends Cancer. (2024) 10:457–74. doi: 10.1016/j.trecan.2024.01.010
95. Headley MB, Bins A, Nip A, Roberts EW, Looney MR, Gerard A, et al. Visualization of immediate immune responses to pioneer metastatic cells in the lung. Nature. (2016) 531:513–7. doi: 10.1038/nature16985
96. Spicer JD, McDonald B, Cools-Lartigue JJ, Chow SC, Giannias B, Kubes P, et al. Neutrophils promote liver metastasis via Mac-1-mediated interactions with circulating tumor cells. Cancer Res. (2012) 72:3919–27. doi: 10.1158/0008-5472.CAN-11-2393
97. McDonald B, Spicer J, Giannais B, Fallavollita L, Brodt P, Ferri LE. Systemic inflammation increases cancer cell adhesion to hepatic sinusoids by neutrophil mediated mechanisms. Int J Cancer. (2009) 125:1298–305. doi: 10.1002/ijc.24409
98. Cools-Lartigue J, Spicer J, McDonald B, Gowing S, Chow S, Giannias B, et al. Neutrophil extracellular traps sequester circulating tumor cells and promote metastasis. J Clin Invest. (2013) 23(8):3446–58. doi: 10.1172/JCI67484
99. Park J, Wysocki RW, Amoozgar Z, Maiorino L, Fein MR, Jorns J, et al. Cancer cells induce metastasis-supporting neutrophil extracellular DNA traps. Sci Transl Med 8(361) 361ra138. (2016). doi: 10.1126/scitranslmed.aag1711
100. Hemmers S, Teijaro JR, Arandjelovic S, Mowen KA. PAD4-mediated neutrophil extracellular trap formation is not required for immunity against influenza infection. PLoS One. (2011) 6:e22043. doi: 10.1371/journal.pone.0022043
101. Sody S, Uddin M, Gruneboom A, Gorgens A, Giebel B, Gunzer M, et al. Distinct spatio-temporal dynamics of tumor-associated neutrophils in small tumor lesions. Front Immunol. (2019) 10:1419. doi: 10.3389/fimmu.2019.01419
102. Takeshima T, Pop LM, Laine A, Iyengar P, Vitetta ES, Hannan R. Key role for neutrophils in radiation-induced antitumor immune responses: Potentiation with G-CSF. Proc Natl Acad Sci U.S.A. (2016) 113:11300–5. doi: 10.1073/pnas.1613187113
103. Granot Z, Henke E, Comen EA, King TA, Norton L, Benezra R. Tumor entrained neutrophils inhibit seeding in the premetastatic lung. Cancer Cell. (2011) 20:300–14. doi: 10.1016/j.ccr.2011.08.012
104. Gallego-Ortega D, Ledger A, Roden DL, Law AM, Magenau A, Kikhtyak Z, et al. ELF5 drives lung metastasis in luminal breast cancer through recruitment of gr1+ CD11b+ Myeloid-derived suppressor cells. PLoS Biol. (2015) 13:e1002330. doi: 10.1371/journal.pbio.1002330
105. Cui C, Chakraborty K, Tang XA, Zhou G, Schoenfelt KQ, Becker KM, et al. Neutrophil elastase selectively kills cancer cells and attenuates tumorigenesis. Cell. (2021) 184:3163–3177.e21. doi: 10.1016/j.cell.2021.04.016
106. Legrand AJ, Konstantinou M, Goode EF, Meier P. The diversification of cell death and immunity: memento mori. Mol Cell. (2019) 76:232–42. doi: 10.1016/j.molcel.2019.09.006
107. Albanesi M, Mancardi DA, Jönsson F, Iannascoli B, Fiette L, Di Santo JP, et al. Neutrophils mediate antibody-induced antitumor effects in mice. Blood. (2013) 122:3160–4. doi: 10.1182/blood-2013-04-497446
108. Cone RE, Sprent J, Marchalonis JJ. Antigen-binding specificity of isolated cell-surface immunoglobulin from thymus cells activated to histocompatibility antigens. Proc Natl Acad Sci. (1972) 69:2556–60. doi: 10.1073/pnas.69.9.2556
109. Matlung HL, Babes L, Zhao XW, van Houdt M, Treffers LW, van Rees DJ, et al. Neutrophils kill antibody-opsonized cancer cells by trogoptosis. Cell Rep. (2018) 23:3946–3959.e6. doi: 10.1016/j.celrep.2018.05.082
110. Cao Y, Langer R, Ferrara N. Targeting angiogenesis in oncology, ophthalmology and beyond. Nat Rev Drug Discovery. (2023) 22:476–95. doi: 10.1038/s41573-023-00671-z
111. He S, Lamers GE, Beenakker JW, Cui C, Ghotra VP, Danen EH, et al. Neutrophil-mediated experimental metastasis is enhanced by VEGFR inhibition in a zebrafish xenograft model. J Pathol. (2012) 227:431–45. doi: 10.1002/path.4013
112. Glodde N, Bald T, van den Boorn-Konijnenberg D, Nakamura K, O'Donnell JS, Szczepanski S, et al. Reactive neutrophil responses dependent on the receptor tyrosine kinase c-MET limit cancer immunotherapy. Immunity. (2017) 47:789–802.e9. doi: 10.1016/j.immuni.2017.09.012
113. Fercoq F, Cairns GS, Donatis MD, Mackey JBG, Floerchinger A, McFarlane A, et al. Integrin inactivation slows down neutrophils congesting the pre-metastatic lung in a model of breast cancer. bioRxiv. (2024) 2024:3.19.585724. doi: 10.1101/2024.03.19.585724
114. Shinde SB, Kurhekar MP. Review of the systems biology of the immune system using agent-based models. IET Syst Biol. (2018) 12:83–92. doi: 10.1049/iet-syb.2017.0073
115. Beltman JB, Marée AFM, de Boer RJ. Analysing immune cell migration. Nat Rev Immunol. (2009) 9:789–98. doi: 10.1038/nri2638
116. Georgantzoglou A, Matthews J, Sarris M. Neutrophil motion in numbers: How to analyse complex migration patterns. Cells Dev. (2021) 168:203734. doi: 10.1016/j.cdev.2021.203734
117. Butler G, Rudge J, Dash PR. Mathematical modelling of cell migration. Essays Biochem. (2019) 63:631–7. doi: 10.1042/ebc20190020
118. Jerison ER, Quake SR. Heterogeneous T cell motility behaviors emerge from a coupling between speed and turning in vivo. Elife. (2020) 9. doi: 10.7554/eLife.53933
119. Textor J, Peixoto A, Henrickson SE, Sinn M, von Andrian UH, Westermann J. Defining the quantitative limits of intravital two-photon lymphocyte tracking. Proc Natl Acad Sci U.S.A. (2011) 108:12401–6. doi: 10.1073/pnas.1102288108
120. Okada T, Takahashi S, Ishida A, Ishigame H. In vivo multiphoton imaging of immune cell dynamics. Pflügers Archiv - Eur J Physiol. (2016) 468:1793–801. doi: 10.1007/s00424-016-1882-x
Keywords: neutrophil, intravital 2-photon microscopy, imaging, migration, infection, wound healing, cancer
Citation: Yam AO, Jakovija A, Gatt C and Chtanova T (2024) Neutrophils under the microscope: neutrophil dynamics in infection, inflammation, and cancer revealed using intravital imaging. Front. Immunol. 15:1458035. doi: 10.3389/fimmu.2024.1458035
Received: 01 July 2024; Accepted: 13 September 2024;
Published: 08 October 2024.
Edited by:
Craig N. Jenne, University of Calgary, CanadaCopyright © 2024 Yam, Jakovija, Gatt and Chtanova. This is an open-access article distributed under the terms of the Creative Commons Attribution License (CC BY). The use, distribution or reproduction in other forums is permitted, provided the original author(s) and the copyright owner(s) are credited and that the original publication in this journal is cited, in accordance with accepted academic practice. No use, distribution or reproduction is permitted which does not comply with these terms.
*Correspondence: Tatyana Chtanova, dC5jaHRhbm92YUB1bnN3LmVkdS5hdQ==