- 1Department of Otolaryngology Head and Neck Surgery, The Affiliated Hospital of Southwest Medical University, Luzhou, Sichuan, China
- 2Department of Otolaryngology, Biomedical Innovation Center, The Sixth Affiliated Hospital, Sun Yat-sen University, Guangzhou, Guangdong, China
- 3Metabolic Vascular Diseases Key Laboratory of Sichuan Province, Metabolic Vascular Diseases Key Laboratory of Sichuan-Chongqing Cooperation, Department of Endocrinology and Metabolism, Luzhou, Sichuan, China
- 4Department of Otolaryngology, The First Affiliated Hospital, Sun Yat-sen University, Guangzhou, Guangdong, China
Head and neck squamous cell carcinoma (HNSCC) is a prevalent malignant tumor globally. Despite advancements in treatment methods, the overall survival rate remains low due to limitations such as poor targeting and low bioavailability, which result in the limited efficacy of traditional drug therapies. Nanomedicine is considered to be a promising strategy in tumor therapy, offering the potential for maximal anti-tumor effects. Nanocarriers can overcome biological barriers, enhance drug delivery efficiency to targeted sites, and minimize damage to normal tissues. Currently, various nano-carriers for drug delivery have been developed to construct new nanomedicine. This review aims to provide an overview of the current status of HNSCC treatment and the necessity of nanomedicine in improving treatment outcomes. Moreover, it delves into the research progress of nanomedicine in HNSCC treatment, with a focus on enhancing radiation sensitivity, improving the efficacy of tumor immunotherapy, effectively delivering chemotherapy drugs, and utilizing small molecule inhibitors. Finally, this article discussed the challenges and prospects of applying nanomedicine in cancer treatment.
1 Introduction
Head and neck squamous cell carcinoma (HNSCC) is the predominant malignant neoplasm in the head and neck region, originating from the mucosal epithelium of the oral cavity, pharynx, and larynx. Based on data from the GLOBOCAN, in 2020, there were approximately 930,000 new cases of HNSCC documented globally, leading to 467,000 mortalities. Regretfully, the incidence and mortality of HNSCC have persistently escalated (1, 2) (Figure 1A). Given the complex and obscured anatomy of the head and neck region coupled with the paucity of initial symptoms, the majority of HNSCC patients are diagnosed with advanced-stage malignancies when deemed necessary for medical attention. Furthermore, the precise location of head and neck cancer (HNC) can profoundly affect essential sensory functions, leading to impaired emotional health and restricted social functioning. Unfortunately, A comprehensive analysis of HNSCC cases from the Surveillance, Epidemiology, and End Results database, demonstrated that the 5-year and 10-year overall survival (OS) rates for HNSCC were 46% and 31%, respectively (3). Despite extensive improvements in HNSCC treatment, upwards of 65% of patients encounter recurrence or metastasis (4). For locally recurrent malignancies that fail to respond to rescue surgery, radiation therapy, or a combination of both, the prognosis becomes exceedingly dire, akin to distant metastases. Without intervention, survival is confined to only 6-9 months, underscoring the ongoing difficulty of attaining long-term remission and disease control (5).
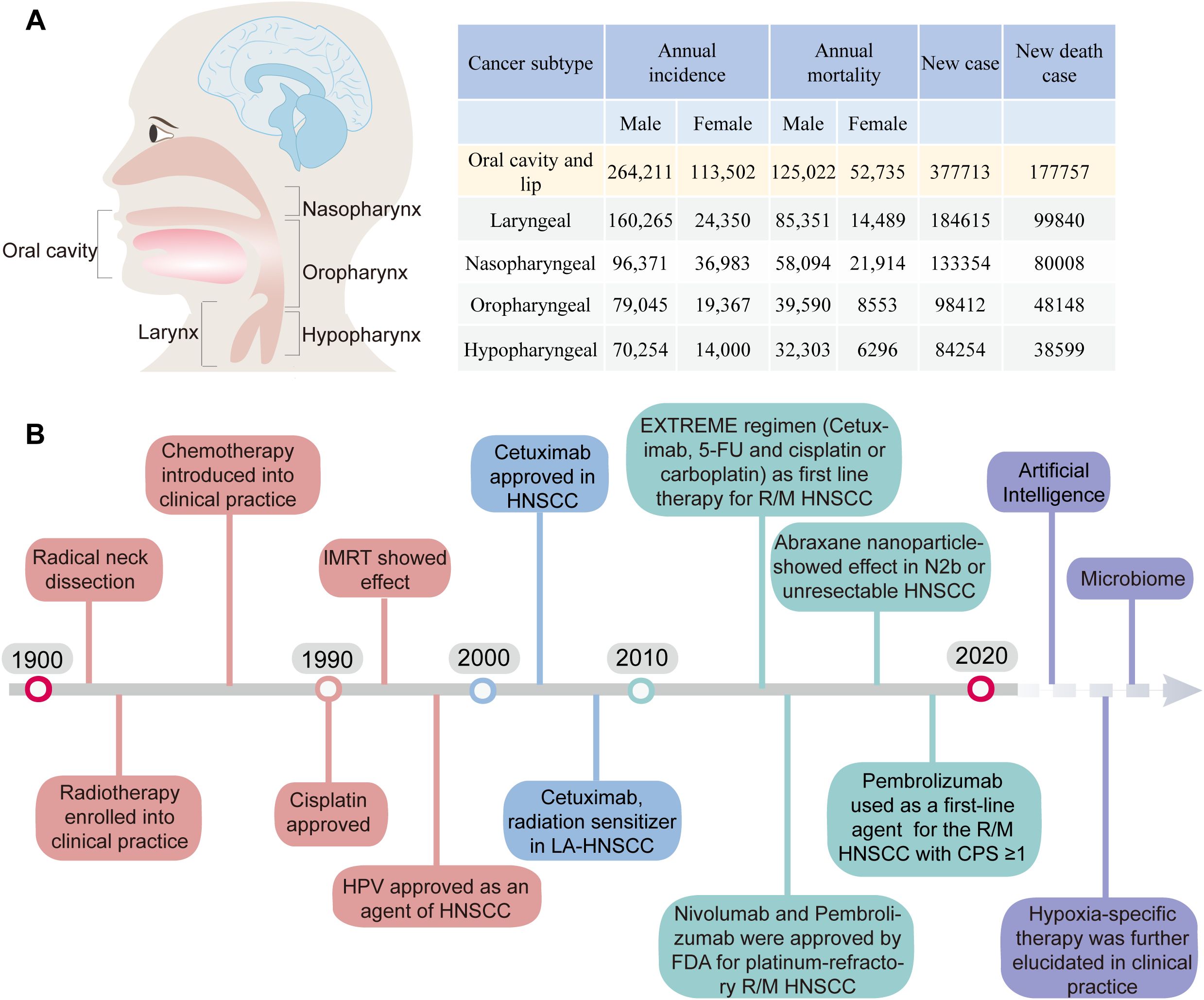
Figure 1. (A) The incidence and mortality rates of HNSCC. (B) Timeline of the Development of Treatment Regimens and Targeted Therapy for HNC and Future Exploration (1).
HPV vaccines have the potential to serve as an effective preventive measure against HPV+ HNSCC (6, 7). Whereas HPV- HNSCC are more heterogeneous in outcome (8). Surgery or radiation therapy can serve as feasible options for early-stage oral and laryngeal malignancies (9). Nevertheless, approximately 70%-80% of HNSCC patients presenting with advanced stage III/IV tumors often experience disease progression associated with extensive local invasion and regional lymph node dissemination. Contemporary treatment strategies for advanced HNSCC encompass surgery, radiotherapy, and systemic therapy (Figure 1B).
In 2006, the FDA approved cetuximab, an EGFR inhibitor, as a first-line treatment for recurrent or metastatic (R/M) HNSCC. Cetuximab has shown significant effectiveness as a radiosensitizer, either alone or in combination with chemotherapy for R/M HNSCC (10). It has unequivocally demonstrated that cetuximab exhibits the ability to downregulate IFN-γ and induce the expression of programmed death ligand 1 (PD-L1) in HNSCC (11). Nevertheless, cetuximab therapy may potentially augment the influx of immunoregulatory regulatory T cells (Tregs) within the tumor microenvironment (TME) (12). Additionally, it may hinder the cytotoxic abilities of activated CD8+ T cells, as indicated by the increased co-expression of programmed death receptor 1 (PD-1) and T-cell immunoglobulin and mucin domain 3 (13). These findings highlight the dual influence of cetuximab therapy.
The emergence of immune checkpoint inhibitors (ICIs) has brought about a revolutionary shift in cancer treatment. Notably, between 2015 and 2019, there was an impressive 8.2% reduction in mortality rates (14). At present, the ICIs have consistently exhibited significant therapeutic effects in melanoma, non-small cell lung cancer (NSCLC), glioblastoma, and advanced skin squamous cell carcinoma (15–20). Pembrolizumab and nivolumab were FDA-approved for the treatment of R/M HNSCC in 2016 and 2019 (21–23). Up to September 30th, 2024, the FDA has approved 196 clinical trials investigating immunotherapy for HNSCC (2, 24, 25). Of these, 74 are currently in active participant recruitment, 32 have been initiated but not yet recruited, and 37 have concluded. However, completed trials have shown a relatively low durable response rate of 15%-20% among patients (26). Furthermore, some patients may experience significant immune-related side effects, such as dermatitis, colitis, hepatitis, and pneumonitis, which may require temporary suspension of ICI treatment or alternative interventions (27, 28).
In recent years, nanomedicine has undergone substantial advancement in oncological treatment (29). It proffers several advantages to cancer therapy, encompassing augmented drug availability, diminished side effects, and precise drug delivery (30–33). A variety of nanoparticle (NP) categories have been leveraged for tumor diagnostics and treatments (34–36). This review provides a synopsis of nanomedicine research progression in HNSCC treatment, emphasizing the extraordinary advancements in nanomedicine-based immunotherapeutic strategies.
2 The advantage of nanomedicines application in HNSCC
Although HNSCC is a solid tumor, due to its special anatomical location, nanomedicines exhibit greater specialized benefits in addressing HNSCC compared with other solid tumors. Primarily, radiotherapy serves as a prevalent treatment option for HNSCC. Consequently, nanomedicine holds potential application possibilities in treating HNSCC via leveraging its radiosensitizing effect. Additionally, the cervical region is abundant in lymphatic tissue, thereby rendering HNSCC susceptible to lymph node (LN) metastasis, resulting in recurrence and metastasis. Through modifications of nanomedicine, the LN targeting of nanomedicine can mitigate tumor LN metastasis and enhance patient survival rates. For instance, Liang et al. engineered a hybrid nano-vaccine (Hy-M-Exo) by amalgamating tumor-derived exosomes (TEX) and dendritic cell membrane vesicles (DCMV), which inherited CCR7, a pivotal lymphatic homing protein of DCMV, and enhanced targeting efficacy against LN. Concurrently, Hy-M-Exo stimulated retained tumor antigens and endogenous danger signals in antigen-presenting cells (APCs), inciting a robust T-cell response (37).
NPs are artificially manufactured particles ranging from 1 to 100 nm (38). They come in various types, including metal NPs, metal oxide NPs, mesoporous NPs, polymer NPs, micelles, liposomes, and others (39). These NPs have special characteristics like large surface area, strong electrical conductivity, optical absorption spectral shift, and unique fluorescence properties. Nanomaterial delivery systems offer several advantages in HNSCC treatment, making them a promising therapeutic approach. These advantages encompass refined targeting, precise drug delivery, multifunctional carriers, enhanced drug stability, photothermal therapy augmentation, and solubility/biocompatibility. These features enable nanomaterials to selectively target HNSCC cells, regulate drug release, deliver multiple therapies, safeguard drugs, facilitate photothermal therapy, and demonstrate favorable solubility and biocompatibility.
Otherwise, the nano-delivery system enters the bloodstream, it undergoes a series of five cascading steps, collectively known as “CAPIR” to achieve successful and efficient delivery of therapeutic drugs to tumor cells. These steps include Circulation within the blood vessels (C), Accumulation at the tumor site (A), Penetration into the deeper regions of the tumor tissue (P), Internalization by tumor cells (I), and Release of therapeutic agents within the intracellular compartment (R) (40). Each step is crucially designed and meticulously constructed to ensure the targeted delivery of nanomedicine to the tumor site (Figure 2).
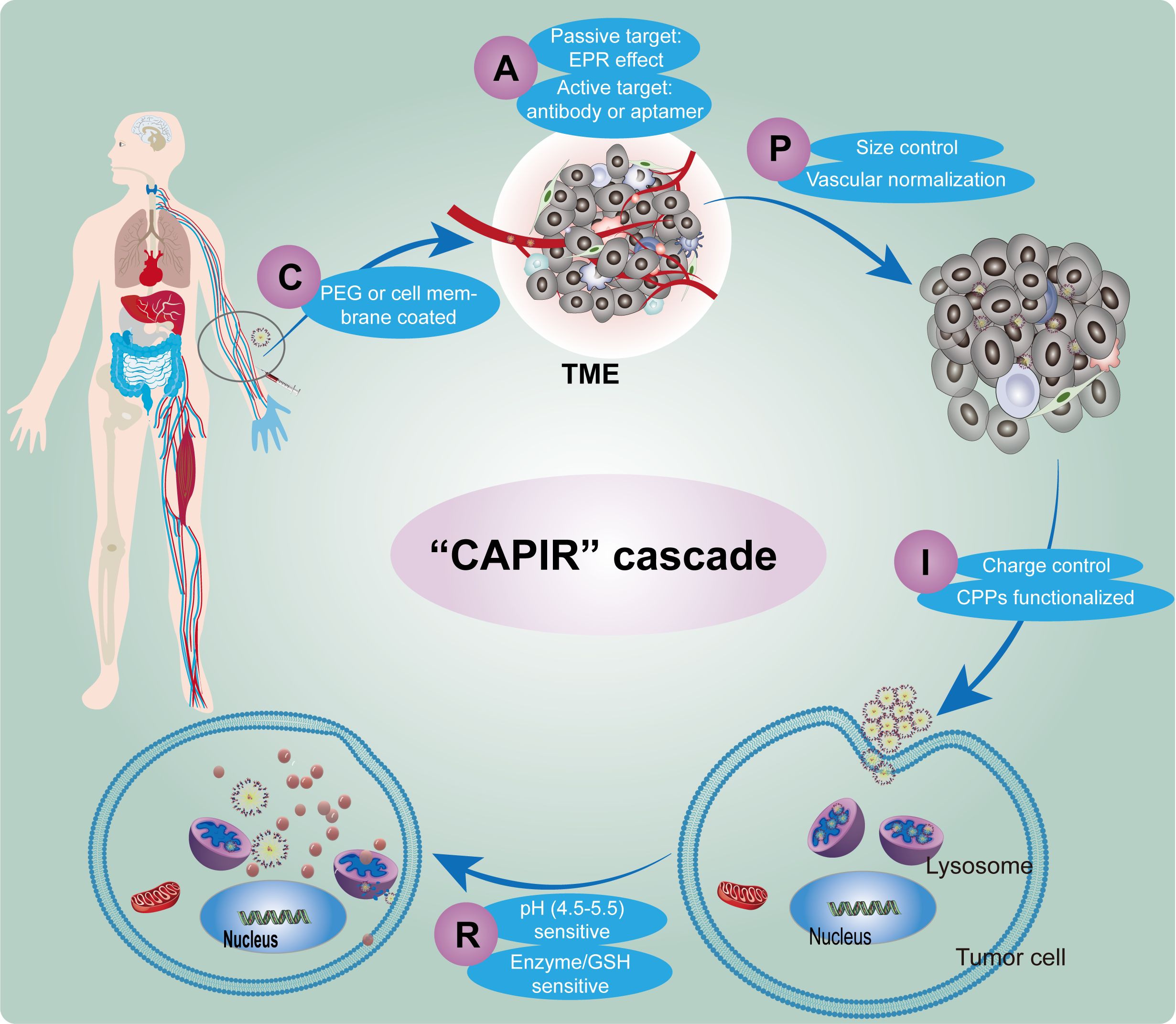
Figure 2. Schematic diagram of the CAPIR cascade of nanomedicine delivering drugs to tumor cell. (C) Circulation within the blood vessels, (A) Accumulation at the tumor site, (P) Penetration into the deeper regions of the tumor tissue, (I) Internalization by tumor cells, (R) Release of therapeutic agents within the intracellular compartment.
A high-throughput nanoparticle assay was employed to assess the delivery of nucleic acids to HNSCC solid tumors in vivo using 94 chemically distinct nanoparticles. DNA barcoding was utilized to identify LNPHNSCC, which demonstrated the ability to inhibit HNSCC tropism while minimizing off-target delivery to the liver (41). Meanwhile, NPs possess the capability to modulate various key elements, thereby disrupting the tumor’s immunosuppressive network (42). The dedicated pursuit of NP-based drug delivery holds immense promise in revolutionizing cancer therapy and fostering improved patient outcomes in the foreseeable future. A variety of nanomedicine has been used for HNSCC treatment and summarized in Table 1.
3 Advancements in nanomedicines for HNSCC treatment
3.1 Nanomedicines enhance radiosensitivity
Over the past few decades, personalized radiotherapy has made remarkable advancements. However, the occurrence of toxic effects frequently impedes the ability to escalate radiation dosage, thus hindering further improvements in treatment outcomes (67). Radioresistance leads to local control failure in about 40% of HNSCC (68). Enhancing the sensitivity of tumor cells to radiation and achieving tumor eradication with a reduced radiation dose hold paramount importance in HNSCC treatment (69). Brown et al. have identified pivotal factors that influence the efficacy of tumor radiotherapy, encompassing: (1) repair of sublethal cell damage; (2) cell regeneration following irradiation; (3) cell redistribution during the cell cycle; (4) reoxygenation of surviving cells; and (5) intrinsic radiosensitivity (70).
In the realm of cancer treatment, nanomaterials containing metals such as gold (Au) and silver (Ag) NPs have demonstrated remarkable capabilities in absorbing, scattering, and emitting radiation energy. These NPs can be readily metabolized and eliminated, rendering them a promising avenue for radiosensitization in cancer therapy (71, 72) (Figure 3). As an example, Zhang’s team has pioneered the development of gold nanoparticles (GNPs)-based system named Au@MC38, which demonstrates the capability to significantly enhance radiation-induced DNA damage and reactive oxygen species (ROS) production when used in conjunction with radiotherapy. This synergistic approach leads to heightened tumor cell apoptosis and necrosis (73). Additionally, an acid-responsive nano-aggregation system incorporating amycin (Dox) has shown notable radiosensitization effects in esophageal cancer (74).
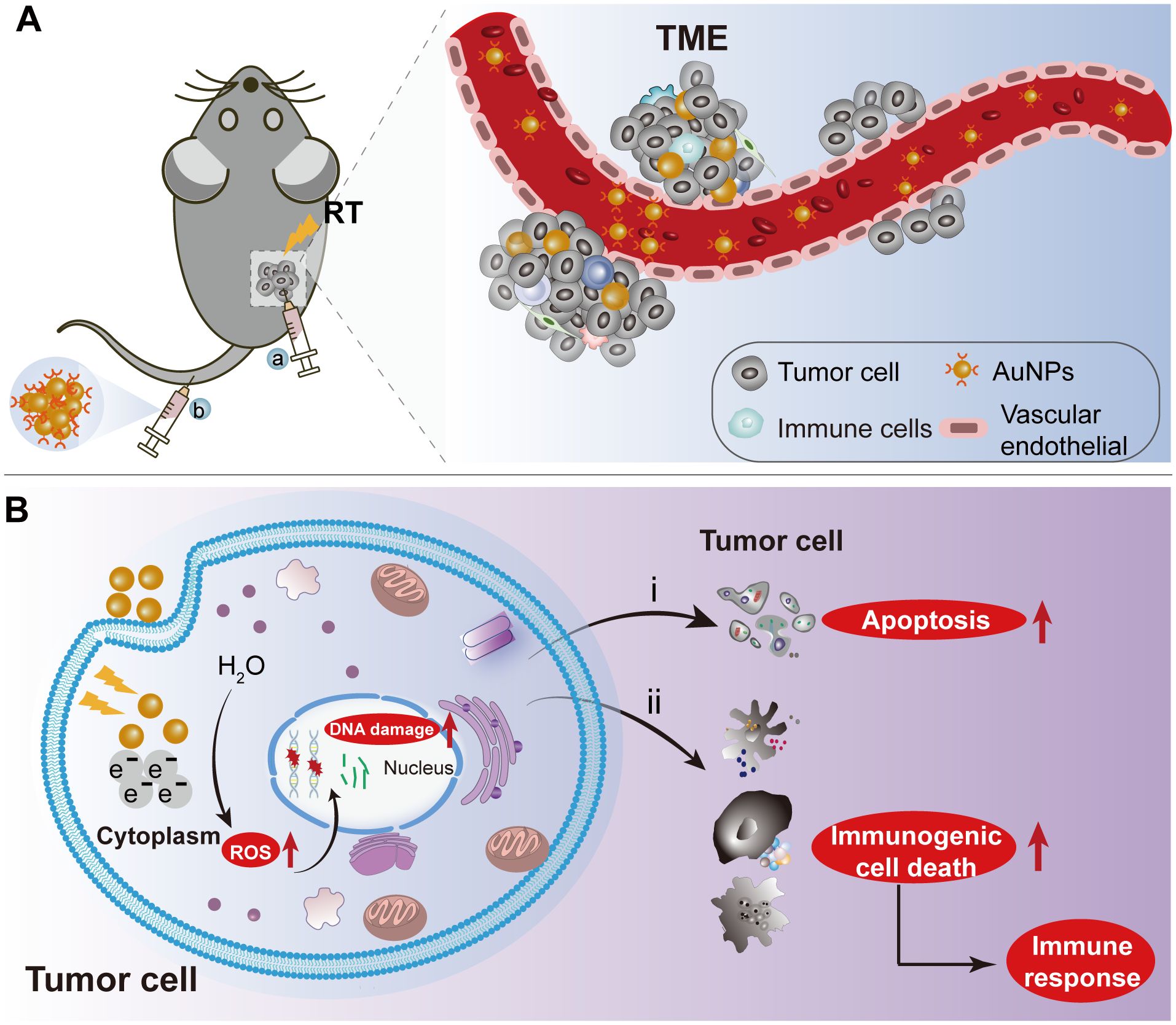
Figure 3. The schematic depiction of AuNPs as radiation sensitizers for HNSCC treatment. (A) Nanoparticles were delivered into the tumor microenvironment by intravenous or intratumoral injection of AuNPs combined with RT to tumor-bearing mice. (B) The internalization of AuNPs into tumor cells enhances radiation-induced DNA damage and reactive oxygen species (ROS) generation, exacerbating tumor cell apoptosis and necrosis during radiotherapy. Additionally, the localized radiation-induced immunogenic cell death elicits a robust immune response. RT: Radiation therapy. AuNPs: gold nanoparticles.
Remarkable advancements have been achieved in harnessing nanomedicines to augment radiosensitivity in HNSCC (43). Regular administration of GNPs through bi-weekly injections has been observed to have a significant therapeutic effect. This approach demonstrates the immense potential of GNPs in enhancing radiation sensitivity and improving the efficacy of radiotherapy in HNSCC (44–46, 75). Nayak et al. developed polyethylene glycol (PEG)-coated gold-silver alloy nanoparticles (BNPs) that show efficient uptake by oral cancer cells. These BNPs exhibit a radiosensitization ratio of 1.5-1.7, demonstrating a robust radiosensitization effect (47). As previously mentioned, cetuximab is known as a radiosensitizing agent. One effective strategy to overcome tumor radioresistance is to use cetuximab-targeted GNPs to increase the absorption of radiation by tumors at clinically relevant energy and radiation doses (68).
In addition to GNPs, mesoporous silica, liposomes, bovine serum albumin, and polymers, have been extensively investigated as carriers to enhance the effectiveness of radiotherapy (76–81). HMG-CoA reductase inhibitors, commonly referred to as statins, have demonstrated potential anti-tumor and radiosensitizing effects (82–84). Nevertheless, the limited bioavailability of orally administered statins poses a significant challenge. Thierry et al. developed high-density lipoprotein NPs for parenteral delivery of statins. This innovative formulation not only enhances the radiobiological response but also exhibits immunoreactive properties in 2D/3D models of HNSCC (48). Biodegradable gelling-and-lipid dual nanocarrier ion-triggered porous adhesive hydrogels have been used to optimize the targeted delivery of clinical radiosensitizers. This advanced nanosystem facilitates the efficient intracellular uptake and cellular retention of radiosensitizers, resulting in a synergistic enhancement of radiation-induced DNA damage and apoptosis. Furthermore, in-gel NPs have demonstrated improved in vivo efficacy of both chemotherapeutic agents by prolonging tumor bioaccumulation and reducing systemic absorption, thereby outperforming systemic commercial agents approved for HNSCC chemoradiotherapy (49). Gadolinium-based NPs (GBNs) have emerged as a highly promising avenue for radiosensitization. Notably, Ardail’s team has achieved remarkable advancements by introducing a novel formulation of GBNs known as AGuIX®. These NPs exhibit the ability to accumulate within lysosomes upon cellular uptake, enabling effective radiosensitization specifically for HNSCC (50).
3.2 Application of nanomedicines in HNSCC immunotherapy
3.2.1 TME and immunotherapy for HNSCC
The TME is an intricate biological system comprising multifaceted components, including tumor cells, tumor-associated fibroblasts, immune cells (including T lymphocytes, tumor-associated macrophages, myeloid-derived suppressor cells, natural killer cells, and tumor-associated neutrophils), and extracellular matrix. The TME serves a pivotal role in the development, progression, and prognosis of tumors (Figure 4) (85, 86). In accordance with Daniel’s classification scheme, the TME may be stratified into three phenotypes: the inflamed phenotype, the immune-excluded phenotype, and the immune-desert phenotype (87). In a research conducted by Jakob et al., detailed histological sections of 965 solid tumors were critically assessed to categorize tumor types based on the distribution of immune cells within the TME. The results indicate that in HNSCC, over 50% of tumors displayed infiltration by immunosuppressive Foxp3+ Tregs. Moreover, HNSCC frequently demonstrated diminished immune cell populations, including tumors with exclusive lymphocytic or myeloid infiltration (88). Huang et al. executed a proteogenomic analysis of 108 cases of HPV-positive HNSCC and discovered significant deletions in immunomodulatory genes, leading to a low immune infiltration state within the tumors (89, 90). Consequently, the TME of HNSCC can be classified as harboring an immuno-excluded phenotype or an immuno-desert phenotype.
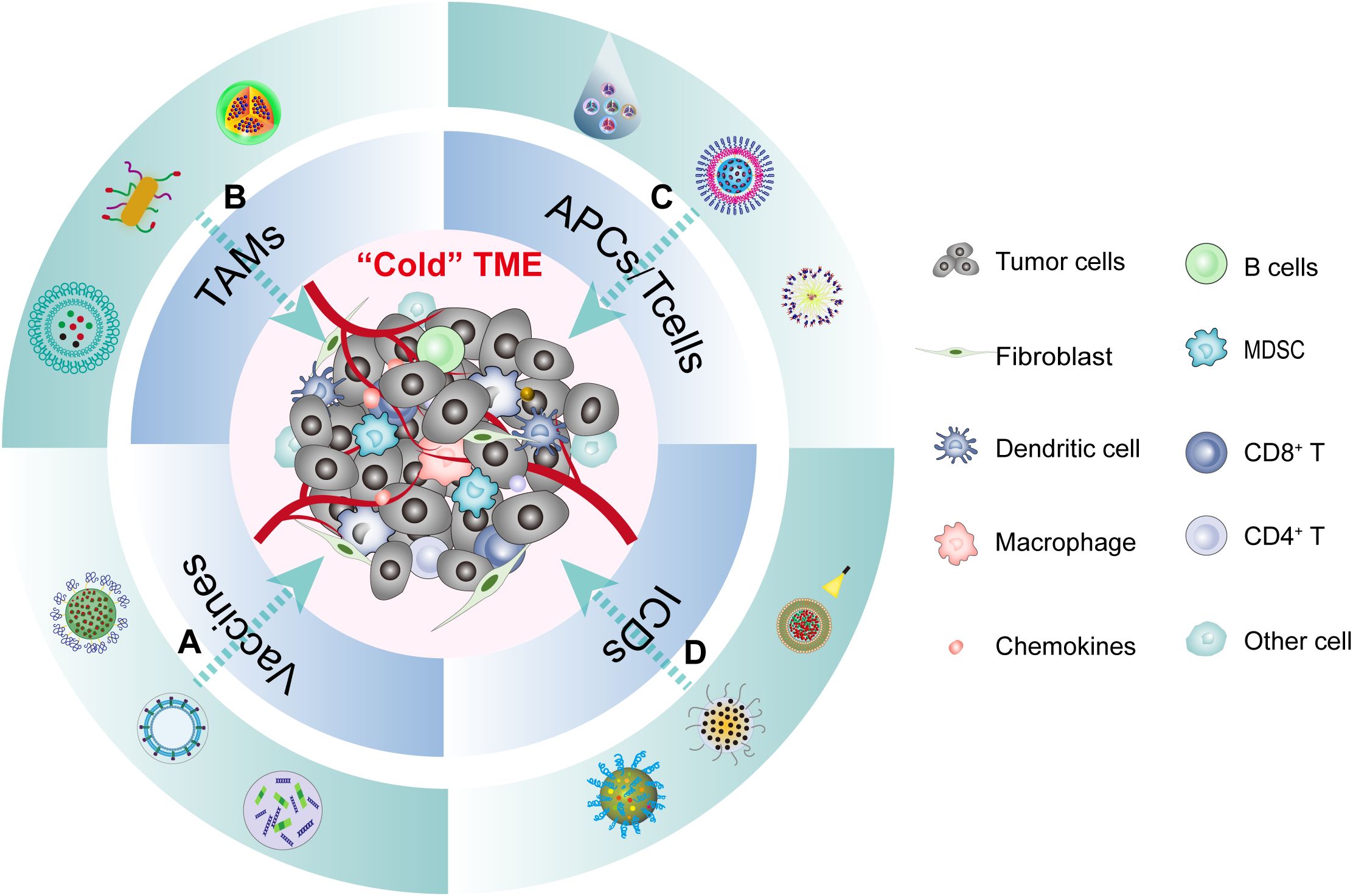
Figure 4. The TME of HNSCC and multifunction of nanomedicine immunotherapy. The cold tumor microenvironment of HNSCC can be reshaped into a hot tumor through various forms of immune microenvironment modulation using different nanoparticles. This process encompasses four main aspects: (A) Nanovaccines regulate the TME by triggering innate immunity. (B) Nanomedicines polarize TAMs into immunocompetent M1 TAMs. (C) Nanomedicines activate tumor-specific immune responses by increasing T-cell infiltration in the TME. (D) Nanomedicines stimulate anti-tumor immune responses by inducing ICD in tumor cells.
A variety of inflammatory factors and immune cells are present within the TME. Furthermore, the tumor can stimulate an immune response, thereby complicating the complex interplay between the tumor and the immune system (91). Nevertheless, solid tumors can reshape the TME by altering the phenotype of immune cells. Such reshaping encompasses modifications in the expression of immune checkpoint proteins, suppression of effector T cell functionality, and promotion of the transition of pro-inflammatory (M1) tumor-associated macrophages (TAMs) towards anti-inflammatory (M2) TAMs (92). The effectiveness of immunotherapy is intertwined with the immune status of the TME (93). The immune system undergoes dynamic alterations throughout the inception and progression of tumors, as well as subsequent treatments such as chemotherapy, radiotherapy, or immunotherapy (94). In the context of HNSCC, the TME displays considerable heterogeneity and employs diverse mechanisms to evade immune surveillance.
The application of noninvasive nanoparticle-based imaging to visualize TAMs can yield valuable insights into the immune cell composition within the TME (95, 96). This data possesses considerable potential as a guiding principle for immunotherapy. Furthermore, by harnessing nanomaterials for precise and targeted delivery of therapeutic agents, nanomedicine offers an opportunity to enhance the efficacy of immunotherapy whilst simultaneously mitigating treatment-associated side effects (97, 98) (Figure 4). This review will explore four facets of nanomedicine in HNSCC immunotherapy (1): Nanovaccines regulate the TME by triggering innate immunity; (2) Nanomedicines polarize TAMs into immunocompetent M1 TAMs; (3) Nanomedicines activate tumor-specific immune responses by augmenting T-cell infiltration in the TME; (4) Nanomedicines stimulate anti-tumor immune responses by inducing ICD.
3.2.2 Nanovaccines for HNSCC
Tumor vaccines are an active form of immune treatment that is garnering significant attention due to their favorable safety profile and minimal side effects (99). Tumor antigens have the capacity to activate immune cells and provoke robust immune responses (100, 101). For instance, vaccination targeting HR-HPV E6 and E7 oncoproteins has demonstrated the ability to induce T-cell responses specific to HPV-16 and even achieve complete histologic responses in certain patients (102, 103). Nevertheless, the presence of immunosuppressive elements within the TME, coupled with its dynamic and diverse attributes, can substantially hinder the clinical efficacy of tumor vaccines in advanced HNSCC (104).
Nanomedicine has achieved considerable advancements, providing a viable methodology to augment the efficacy of vaccines and counteract immunosuppression (105). Initially, nanomaterial-based tumor vaccines can efficiently convey tumor antigens and immune adjuvants to stimulate antigen presentation and amplify the immune response. Secondly, highly immunogenic nano-vaccines can be directly instilled at the tumor site to evoke acquired immunity and eradicate tumor cells. Hydrophilic polyester polymeric nanoparticles constructed of polylactic acid-hydroxymethylglycolic acid (pLHMGA), have been employed in the formulation of therapeutic HR-HPV vaccines. These nanoparticles are laden with a lengthy peptide derived from the HPV16 E7 oncogene and enveloped with toll-like receptor 3 (TLR3) ligand (poly I: C) to augment the immune response. This strategy has demonstrated a significant augmentation in the proportion of HPV-specific CD8+ T cells (106). Furthermore, the implementation of E6/E7-oriented nanosatellite vaccines has shown a remarkable augmentation of more than 12-fold in tumor-specific CD8+ T cells, culminating in diminished tumor burden (51). Similarly, the application of mesoporous silica rods (MSR)-based HPV-16 E7 vaccines has led to extensive infiltration of antigen-specific CD8+ T cells in MOC2-E6E7 tumor cells. This infiltration has been associated with inhibited tumor growth and prolonged survival in MOC2-E6E7 tumor mice, particularly in the HNC models with specific antigen expression (52). Recently, a novel ribonucleic acid lipoplex (RNA-LPX)-based HPV16 vaccine (E7 RNA-LPX) combined with local radiotherapy has been reported to induce a substantial infiltration of E7-specific CD8+ T cells in the tumor. This combination therapy has the potential to convert a “cold” tumor into a “hot” tumor (53). Currently, phase I and II clinical trials (NCT04534205, NCT03418480) are underway to investigate the efficacy of the HPV16 E6/E7 RNA-LPX vaccine against various HPV-driven cancers, including HNSCC. Additionally, there are other clinical trials (NCT04287868, NCT04260126, and NCT05232851) that are exploring the therapeutic potential of nano-vaccines in HNSCC.
Moreover, virus-like NPs (VLPs) derived from plants and bacteria have shown high immunogenicity, such as cowpea mosaic virus (CPMV) or tobacco mosaic virus (TMV) (107–109). CPMV nanoparticles are stable, non-toxic, scalable in production, and can be modified with drugs and antigens. Additionally, novel virus-like hollow mesoporous silica nanoparticles (VH-MSNs) have been developed by mimicking the structural characteristics of viruses using an autophagy perovskite template. The distinctive surface topology of VH-MSNs amplifies cellular internalization and magnifies immune responses. These NPs can accommodate doxorubicin, facilitating the integration of chemotherapy and immunotherapy (110). Similarly, in a preclinical model of HNSCC, orthotopic inoculation of virus-like microparticle-coated toll-like receptor 9 agonist (CMP-001) instigated both local and distal antitumor immune responses (111).
3.2.3 Nanomedicines for HNSCC by polarizing TAMs
M2 TAMs are known to contribute to tumor progression by fostering tumor growth, angiogenesis, and metastasis (Figure 5). They create an immunosuppressive barrier that impedes effective anti-tumor immune responses, thus hampering immune surveillance against the tumor (112). In HNSCC, the predominance of M2 TAMs is notable, and their presence is closely linked to an unsatisfactory overall prognosis (113, 114). Encouragingly, studies have provided compelling evidence showcasing the effectiveness of TAMs-targeting strategies in diverse tumor types (115–118). However, the clinical applicability of current small-molecule drugs targeting TAMs faces several challenges (119).
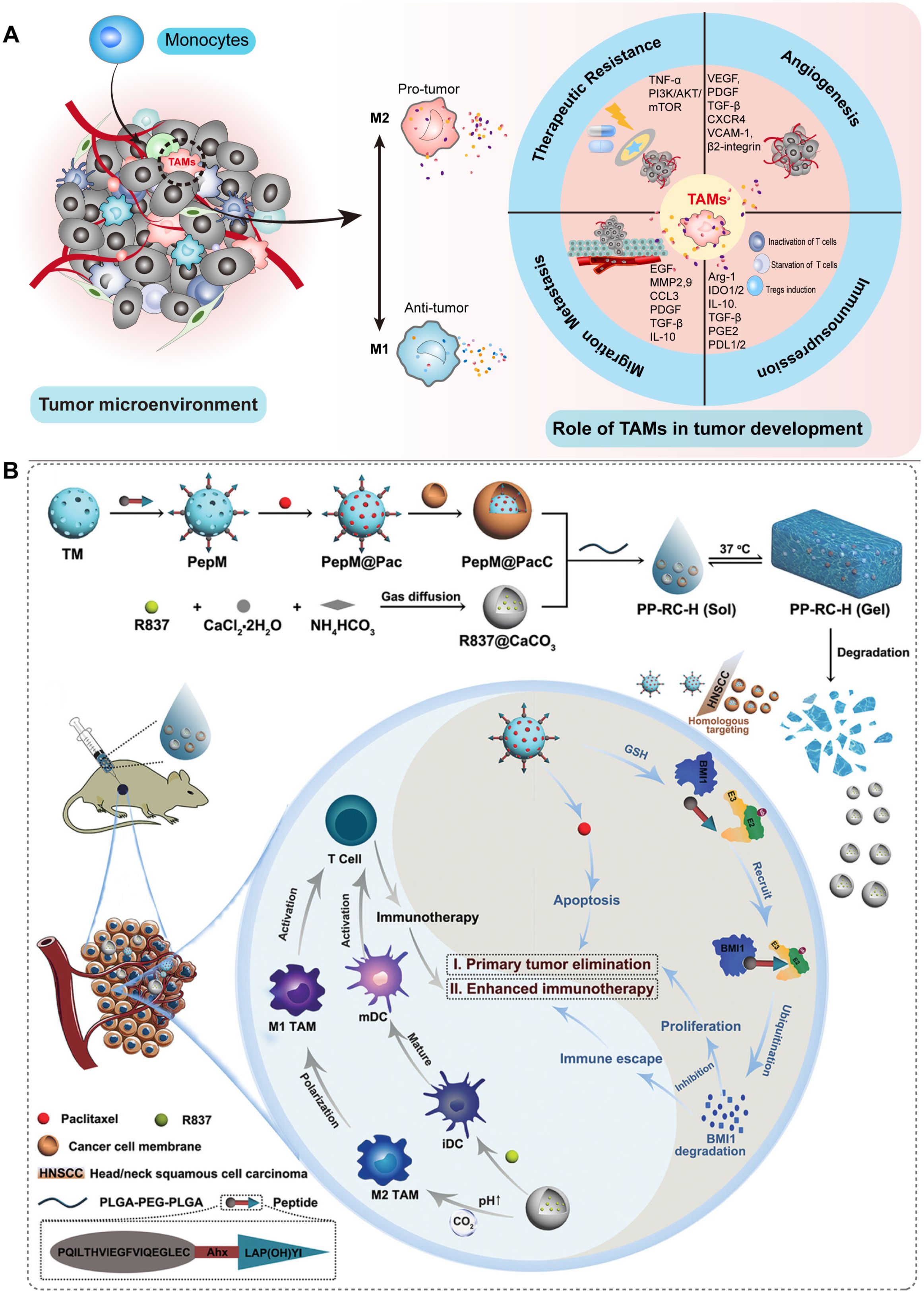
Figure 5. Schematic illustration of the involvement of TAMs in HNSCC and their potential as therapeutic targets for nanomedicine-based treatment of HNSCC. (A) TAMs primarily consist of M1 and M2 phenotypes. They play crucial roles in angiogenesis, resistance to treatment, cellular migration, and metastasis, as well as immunosuppression within the TME. (B) A nanocomposite hydrogel with antitumor properties for the treatment of primary and metastatic HNSCC (54).
Nanomaterial-based drug delivery systems have dramatically revolutionized the field of TAM-related immunotherapy (119–123). Rodell et al. synthesized a nanoparticle called CDP-R848, a potent agonist of TLR7 and TLR8. CDP-R848 has been shown to convert TAMs to the M1 phenotype, leading to suppressed tumor growth and prevention of tumor re-invasion in various tumors. Notably, when combined with anti-PD-1 therapy, CDP-R848 has shown an improved response to immunotherapy, even in tumors that were resistant to anti-PD-1 treatment (124). A prodrug formulation called R848-Toco, coupled with α-tocopherol, has been formulated as a polymeric nanosuspension using tocopherol-modified hyaluronic acid (HA-Toco). This delivery system offers prolonged release kinetics and maintains the activity of R848. In the HNC model, subcutaneous injection of the nanosuspension has been shown to recruit immune cells in the TME and achieve significant anti-tumor effects (125). Furthermore, NPs constructed from mRNA encoding interferon regulatory factor 5 and IKKb kinase have demonstrated the ability to reprogram TAMs from the M2 to M1 phenotype. This reprogramming leads to the induction of tumor immunity and anti-tumor effects in ovarian cancer, melanoma, and glioblastoma (126). Recently, there have been reports on the utilization of a nano-platform called M1mDDTF, which involves disguising the iron supply regeneration system with the membrane of M1-type macrophages. This innovative approach has shown promising results in promoting the gradual polarization of TAMs towards the M1 TAMs (127).
The CD47, a signal found on tumor cells that essentially tells immune cells “don’t eat me”, can interact with signal regulatory protein α (SIRPα) on macrophages. This interaction effectively prevents macrophages from engulfing and eliminating the tumor cells. Additionally, tumor cells release certain factors that push macrophages toward the M2 phenotype, further aiding the growth of the tumor. Therefore, Rao et al. developed hybrid membrane nano-vasomes (hNVs) that address both mechanisms simultaneously. These hNVs operate by inhibiting the CD47-SIRPα signaling pathway, thereby facilitating macrophages in recognizing and eliminating tumor cells. Additionally, the hNVs facilitate the repolarization of the M2 to M1 phenotype. This repolarization process helps to inhibit local tumor recurrence and distant metastasis of melanoma (128). The stimulator of interferon genes (STING) is a crucial molecule involved in transmitting signals within the innate immune response (129). Researchers loaded STING agonists into hollow nanovesicles, which resulted in significant suppression of tumor growth in a model of triple-negative breast cancer with low immunogenicity (128).
The lysosomes of TAMs have elevated cysteine protease activity, which hinders antigen cross-presentation and CD8+ T cell activation. Cui et al. developed a DNA nanoparticle targeting TAMs lysosomes to specifically inhibit cysteine proteases, improve the ability of TAMs to cross-present antigens, and inhibit tumor growth (130). The latest research reports an injectable nanocomposite hydrogel with a polymer framework (PLGA-PEG-PLGA) loaded with imiquimod-coated CaCO3 nanoparticles (RC) and cancer cell membrane (CCM)-coated mesoporous silica nanoparticles PepM@PacC, in which RC not only facilitates the maturation of DCs but also enhances the polarization of TAMs towards the M1 phenotype. Moreover, this nanocomposite hydrogel has demonstrated the ability to improve the TME, suppress tumor growth, and notably impede tumor metastasis to the lungs in a mouse model of HNSCC (54). Despite the use of nanomedicine to target TAMs in HNSCC is still being investigated, it holds significant potential to enhance the therapeutic outcomes of HNSCC. For instance, it has been reported that the combination of intratumoral injection of TLR7 and TLR9 agonists with anti-PD-1 can promote the transformation of TAMs from M2 to M1 and inhibit tumor growth in HNSCC (131). Converting M2 into M1 TAMs using small interfering RNA (siRNA) is a highly promising approach, where the involvement of nanosystems cannot be overlooked (132). Conspicuously, it has been demonstrated that maintaining an appropriate balance between M1 and M2 in the TME (instead of completely polarizing towards M1) can enhance the therapeutic effect mediated by NPs (133).
3.2.4 Nanomedicines treat HNSCC by increasing tumor-infiltrating lymphocytes
The insufficient presence of TILs within the TME significantly hampers the immunotherapy effectiveness. Otherwise, the depletion of T cells is also one of the important factors affecting the effect of immunotherapy (134). Strategies aim to increase tumor-specific TILs in two ways: promoting TIL infiltration in the TME and enhancing APC function to improve T cell activity.
Studies have demonstrated the crucial role of the host STING pathway in the production of type I interferon, activation of DCs, and stimulation of CD8+ T cells in response to tumor-associated antigens (135, 136). As a result, STING agonists are being developed as a novel therapeutic agent for the treatment of cancer (137). Nonetheless, their effectiveness is limited by barriers to drug delivery. Consequently, Cheng et al. encapsulated the STING agonist cGAMP in liposomes (cGAMP NPs), which markedly improved the cellular uptake of cGAMP, stimulated the secretion of IFN-γ, and increased the infiltration of CD4+ and CD8+ T cells into the TME. As a result, cGAMP NPs effectively inhibited the growth of triple-negative breast cancer and B16F10 melanoma (138). Furthermore, nanosatellite vectors loaded with STING agonists demonstrated a remarkable 12-fold increase in the infiltration of tumor-specific CD8+ T cells in the TME (51). As previously described with nanomedicine PepM@PacC, CaCO3 nanoparticles promote DCs maturation and activate T cells, leading to synergistic tumor cell killing and effective inhibition of HNSCC metastasis (54). In certain studies, nanoparticles have been modified by incorporating PD-L1 antibodies to create nanomedicines known as all-trans retinoic acid-polylactic acid-glycolic acid copolymer (PLGA)-PEG-PD-L1. These nanomedicines are designed to specifically target tumor cells. By activating CD8+ T cells surrounding PD-L1+ tumor cells, the anti-tumor activity is enhanced, effectively converting “cold” tumors into “hot” tumors (55). Importantly, our previous study demonstrated that the functionalized two dimensions nanomaterials MoS2 loaded with TLR9 agonist CpG effectively suppressed tumor growth and significantly prolonged mouse survival in the HNSCC model by augmenting TILs within the TME (56) (Figure 6).
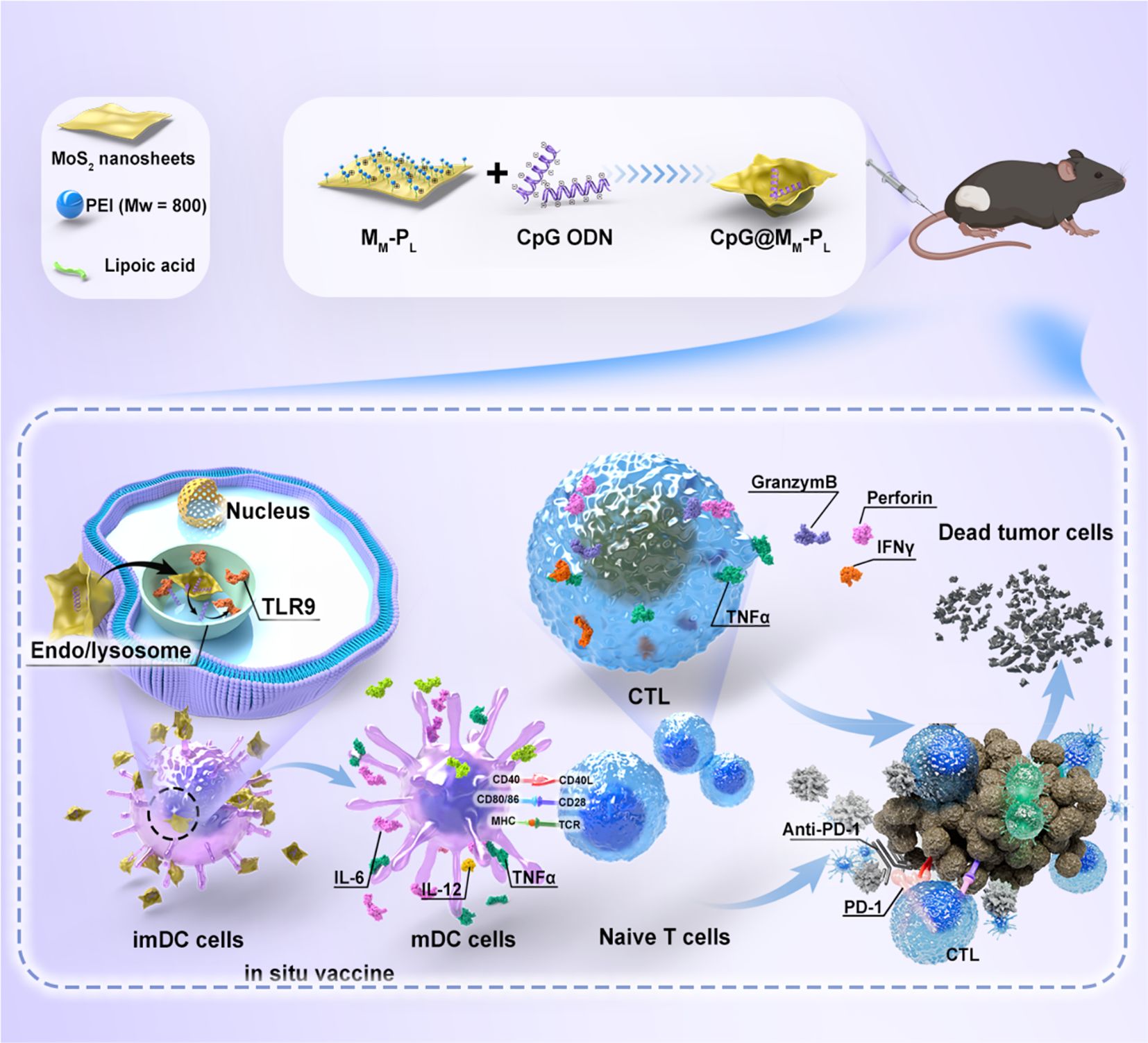
Figure 6. Schematic representation of the antitumor effects of the functionalized nano-platform. The CpG-loaded nanosheets elicit an anti-tumor immune response, enhance the maturation and antigen presentation capacity of BMDCs, and further augment the tumor cytotoxicity of CTLs, thereby ultimately advancing the immunotherapeutic efficacy against HNSCC (56).
As mentioned earlier, NPs carrying tumor vaccines can indirectly promote the infiltration of TILs in the TME by activating APCs (53). In addition to improving DCs targeting, the surface of nanoparticles can be modified with antibodies or ligands that selectively bind to the surface receptors of DCs, including C-type lectin receptors, mannose receptors, DCIR2, and CLEC9A (139). Surface modification of NPs can also augment the antigen transport and cross-presentation capabilities of DCs. For instance, NPs modified with polyethylene imine and aluminum hydroxide are more readily endocytosed by DCs, leading to improved release of antigens into the cytoplasm, enhancing antigen cross-presentation ability, and facilitating infiltration of TILs (140).
Recent research has advanced our understanding of the connection between microorganisms and tumor development. It has revealed the complex relationship between commensal bacteria and the host immune system (141, 142). Studies have revealed that lactobacillus, for example, can inhibit the onset of oral squamous cell carcinoma (OSCC) by enhancing the anti-tumor immune response (143), indicating that modulation of the oral microbiota can potentially boost the immune response. Furthermore, Zheng et al. made an intriguing discovery that tumor tissues in OSCC harbored elevated levels of peptostreptococcus bacteria, which were found to be positively associated with survival rates (57). In response, a hydrogel based on silver NPs was developed to suppress the growth of competing bacteria, including peptostreptococcus. The hydrogel was designed to co-deliver anaerobes (which increase the levels of peptostreptococcus) along with exogenous anaerobes and anti-PD-1 therapy. This combination significantly increased the infiltration of CD8+ T cells, resulting in inhibited tumor growth in subcutaneous xenograft tumors and 4-NQO-induced spontaneous tongue cancer tumor models (57).
3.2.5 Nanomedicines treat HNSCC by inducing ICD
In recent years, several emerging tumor treatment methods have been discovered, including photodynamic therapy (PDT), photothermal therapy (PTT), and sonodynamic therapy (SDT) (144–146). These therapies utilize photodynamic or sonodynamic approaches to induce death in tumor cells, which release a significant amount of damage-associated molecular patterns (DAMPs), including calretinin, adenosine 3-phosphate (ATP), and high mobility group box 1. DAMPs play a crucial role in activating the adaptive immune response of T cells and promoting the formation of long-term immune memory (147). Consequently, the ICD has the potential to reverse the “cold” TME and enhance the effectiveness of immunotherapy.
Nanomedicine offers great potential in enhancing the efficacy of tumor immunotherapy by inducing ICD of tumor cells, through its high component selectivity and functional modification capabilities (147–150). As an illustration, the photosensitizer mTHPP (5,10,15,20-tetra hydroxyphenyl porphyrin) can be effectively incorporated into polymeric micelles, thereby enhancing the efficiency of PDT (58). As mentioned earlier, M1mDDTF not only possesses the ability to directly eliminate tumor cells but also exhibits the potential to stimulate ICD and facilitate DCs development (127). Additionally, Hackenberg et al. synthesized zinc oxide nanoparticles capable of inducing ICD via photocatalysis, highlighting the potential of PDT as a treatment option for HNSCC (59). Notably, certain proteins such as matrix metalloproteinase (MMP)2 and MMP9, which are overexpressed in the tumor, have shown promise in the enrichment of gelatin nanoparticles, further enhancing their therapeutic potential (151, 152). As a result, researchers have developed gelatin nanoparticles (GENPs) that can be degraded by MMP2 and MMP9. These GENPs were loaded with the photosensitizer indocyanine green (ICG) and the STAT3 inhibitor NSC74859 (NSC, N). Upon near-infrared irradiation, the released ICG can effectively utilize the photothermal effect to kill tumor cells, while NSC can stimulate effective anti-tumor immunity, consequently enhancing the efficacy of tumor therapy (60). Furthermore, Zhou et al. developed a novel nanocomposite called TiO2@Ru@siRNA, which involved the modification of TiO2 nanoparticles with a ruthenium-based photosensitizer (Ru) and the loading of hypoxia-inducible factor-1α (HIF-1α) siRNA. When exposed to visible light, TiO2@Ru@siRNA exhibited both type I and II photodynamic effects. In addition to causing lysosomal damage and silencing the HIF-1α, it was also effective in killing OSCC cells. By alleviating hypoxia and inducing pyroptosis, a form of programmed cell death, TiO2@Ru@siRNA can significantly inhibit tumor progression by downregulating key immunosuppressive factors, upregulating immune stimulating factors, and thereby reestablishing the immune microenvironment and enhancing anti-tumor immunity (61). Moreover, TiO2@Ru@siRNA-mediated PDT has demonstrated significant efficacy in both xenograft tumor models and rat oral cancer models (61). As mentioned earlier, Au@MC38 can enhance radiosensitivity, moreover, it also elicits local radiation-ICD, which activates a robust immune response and leads to a notable augmentation of CD8a+ DCs within the TME (73).
However, the irreversible hypoxic conditions in the TME of HNSCC hinder the effectiveness of PDT. To address this challenge, researchers have focused on chemokinetic approaches by exploring iron-dependent cell death mechanisms with ROS cytotoxicity. Ferroptosis, a non-apoptotic programmed cell death process, not only improves the efficiency of PDT by providing oxygen to the hypoxic TME but also stimulates an immune response through ROS production (153). For instance, a 2-in-1 nanoplatform, known as SRF@Hb-Ce6, loaded with the ferroptosis promoter sorafenib was constructed by linking hemoglobin (Hb) to the photosensitizer chlorinated e6 (Ce6) (154). Furthermore, amphiphilic MMP2-responsive peptides are incorporated into the backbone of the nano-platform to ensure drug release specificity and enhance safety. SRF@Hb-Ce6 has the potential to improve the efficacy of both PDT and ferroptosis. Notably, PDT not only recruits immune cells to secrete IFN-γ, thus promoting the process of ferroptosis but also enhances the sensitivity of tumors to ferroptosis. These findings underscore the promising prospects of combining nanoplatforms with PDT and ferroptosis in cancer treatment (154). Zhu et al. developed a novel supramolecular nanomedicine by self-assembling the photosensitizer Ce6 and ferroptosis-inducing agent erastin through hydrogen bonding and π-π stacking. This nanomedicine, known as Ce6-erastin, exhibits low toxicity to normal tissues but can cause excessive accumulation of ROS, increase the oxygen concentration in tumor cells, and inhibit SLC7A11 expression. Consequently, it enhances toxicity to tongue cancer CAL-27 cells and demonstrates significant anti-tumor activity against OTSCC transplanted tumors after radiation treatment (62).
3.3 Other applications of nanomedicines in the treatment of HNSCC
Moreover, nanomaterials are widely utilized as nanocarriers for targeted delivery of chemotherapy drugs, small molecule inhibitors, and nucleic acid agents (155–157). Notably, albumin-bound paclitaxel (NAB-paclitaxel) is an FDA-approved nanomedicine widely used in anti-tumor therapy. Jessica et al. conducted a single-center retrospective analysis, revealing the efficacy of NAB-paclitaxel in the treatment of R/M HNSCC that had shown progression after prior use of other taxanes, such as cremophor-based paclitaxel or docetaxel (158). Furthermore, Wang et al. employed nanomaterial modification techniques to develop NR7 ligand-modified PLGA-PEG/NR7 nanoparticles that could target tumor sites and were simultaneously loaded with cisplatin. In OSCC cells with an overexpression of the receptor, the NR7 peptide could be specifically delivered and rapidly taken up by tumor cells, thereby exerting a potent anti-tumor effect (63).
Targeted delivery of small molecule inhibitors via NPs shown exceptional efficacy in HNSCC treatment. Src, a non-receptor tyrosine kinase, plays a pivotal role in the progression and metastasis of HNSCC. Encapsulating the Src inhibitor saracatinib within NPs has demonstrated significant inhibition of HNSCC metastasis, surpassing the effectiveness of the free drug. Additionally, the co-delivery of the saracatinib (AZD0530) and the AKT inhibitor capivasertib (AZD5363) via cathepsin B-sensitive NPs significantly enhanced the antitumor effect while minimizing side effects. This is primarily attributed to the highly specific and efficient tumor targeting achieved by nanomedicine (64, 65). NAD(P)H: quinone oxidoreductase 1 (NQO1) is an enzyme that is frequently upregulated in squamous cell carcinoma, exerting a significant influence on tumor proliferation and chemotherapy resistance. β-lapachone is a small molecule inhibitor of NQO1. MSN loaded with 5-FU/β-lapachone (FNQ-MSN) can effectively target NQO1, inhibit its expression, and overcome 5-FU resistance, resulting in enhanced cytotoxicity against HNSCC (157). Furthermore, nanoparticles loaded with the novel AKT/PDK1 inhibitor PHT-427 have also demonstrated enhanced anti-tumor effects in HNSCC (66).
siRNA holds great potential as an effective tool for cancer therapy. However, its clinical application faces numerous obstacles, including susceptibility to degradation by ribonucleases, poor stability in physiological conditions, potential to induce inflammatory responses, and lack of site-specificity. Researchers are actively investigating the use of nanoparticles as carriers for efficient siRNA delivery. As an illustration, the use of nanoparticles has demonstrated the ability to suppress the proliferation of HNSCC through the delivery of siRNA targeting ribonucleotide reductase subunit M2. In HNSCC xenografts models, nanoparticles can accumulate in tumors with intravenous injection and the targeted gene knockout effect can persist for 10 days, leading to significant inhibition of tumor progression (159). Additionally, CXCR4 is an attractive target for drug delivery in HNSCC therapy (160, 161). In recent years, DNA tetrahedron-based nanostructures have emerged as promising platforms for siRNA delivery, garnering significant attention (162, 163).
Nanobody (NB) is an innovative immunoglobulin discovered in the serum of Camelidae. It presents the advantages of petite size, potent specificity, elevated stability, facile expression, and the capacity to identify concealed epitopes. It demonstrates a vast scope of application value across various fields and has progressively evolved as a nascent force in the next-generation of therapeutic biomedicals and clinical diagnostic reagents (164). A multiattribute platform consisting of a nanoantibody recognizing the outer membrane domain (EGa1) of EGFR coupled to PEG-liposomes remarkably downregulated EGFR to suppress tumor proliferation both in vitro and in vivo (165). Nanoantibody-directed photodynamic therapy (NB-PDT) is an efficacious tumor-selective treatment methodology utilizing NBs to specifically transport photosensitizers (PSs) to tumor cells, typically targeting EGFR in conjunction with PDT. Since 2014, NB -directed photodynamic therapy (NB-PDT) has matured into a highly precise tumor treatment method (166, 167). For instance, one investigation characterized two EGFR-directed nanobody-photosensitizer conjugates (NB-PSs), the monovalent 7D12-PS and the bivalent 7D12-9G8-PS, which exhibited significant tumor colocalization (168). In feline oral carcinoma, NB-PDT hasachieved a significant anti-tumor effect (169). Furthermore, amalgamating the photosensitizer IRDye700DX with a nanoantibody targeting EGFR, this nanoantibody-PS conjugate resulted in extensive tumor necrosis (approximately 2/3) with minimal toxicity to healthy tissues in a model of HSNCC (167).
3.4 Combination therapy of nanomedicine with other therapeutic approaches
Combining surgery, radiotherapy, chemotherapy, immunotherapy, and targeted therapy is often necessary for effective tumor treatment. However, single-drug therapy has limitations, leading to suboptimal efficacy and side effects. To address this, integrating nanotechnology with various therapeutic approaches shows promise for future research.
Currently, there is increasing research on combining nanomedicine for the treatment of HNSCC. One notable example is that the combination of photocatalytically active zinc oxide subnanoparticles with chemotherapy drugs, cisplatin, and paclitaxel, exerts a significantly stronger effect on the proliferation of HNSCC cells compared to single-drug therapy (170). Furthermore, coordination polymer-based core-shell NPs loaded with cisplatin and photosensitizer pyrolipid (NCP@pyrolipid) have demonstrated the ability to induce apoptosis and necrosis of cancer cells synergistically. In a cisplatin-resistant HNC SQ20B xenograft model, NCP@pyrolipid exhibited remarkable tumor regression (83% reduction in tumor volume) at low doses (171). As mentioned, the ICD in combination with other treatments to stimulate the anti-tumor immune response may be one of the strategies for combination therapy (60, 61). Additionally, numerous studies have explored combination therapy approaches concerning nanotechnology, including nano-vaccine therapy combined with local radiotherapy (53), the delivery of siRNA or gene editing through nanomaterials combined with chemotherapy (172–174), and PTT combined with chemotherapy (175, 176).
4 Summary and prospects
Nanotechnology has significantly advanced therapeutic drug delivery in cancer treatment. Several nanomedicines, encompassing Doxil (1995), Feraheme (2009), and albumin-bound paclitaxel nanoparticles (Abraxane, 2005), have surfaced and are presently employed in clinical applications (177). These modalities have widened the therapeutic efficacy for HNSCC. Numerous clinical studies have explored the conceivable utility of nanomedicine for diagnosing and treating HNSCC (Table 2). For example, the Abraxane combination therapy has exhibited promising outcomes in the context of N2b lymph node invasion or unresectable HNSCC (178). A single-arm, multi-center, phase II trial indicated superior objective response rate and OS with albumin-bound paclitaxel, cetuximab, and carboplatin (CACTUX) as compared to the EXTREME regimen in patients afflicted with R/M HNSCC (179). In 2021, NBTXR3 nanoparticles were employed as a radiosensitizer for the management of locally advanced OSCC patients eligible for radiotherapy. Phase I clinical trials confirmed its safety, paving the way for ongoing phase II trials (180). Notably, phase II/III clinical trials (NCT04892173 and NCT04862455) are presently enlisting participants to evaluate the therapeutic efficacy of NBTXR3 nanoparticles in locally advanced and R/M HNSCC. Furthermore, multi-center, phase II-III randomized controlled trials have validated the efficacy of NBTXR3 in soft tissue sarcomas (181). Additionally, mRNA-2752, a lipid nanoparticle encapsulating mRNAs encoding human OX40L, IL-23, and IL-36γ, is currently undergoing clinical trials for various malignancies, including HNSCC (NCT03739931).
Nevertheless, several obstacles need to be surmounted before translating preliminary nanomedicine research into triumphant clinical applications. A pivotal challenge is achieving equilibrium between the intricacy of nanomedicine constituents, manufacturing expenses, and therapeutic potency (182). The uniformity and stability of nanomedicine batches are equally crucial for enduring clinical advantages. Additionally, apprehensions concerning pharmacokinetics, efficacy, and long-term safety require meticulous scrutiny. Discrepancies between animal models and human physiology may affect the dependability of preliminary study outcomes. Tumor heterogeneity and the necessity for patient stratification further impact the effectiveness of nanomedicines. Identifying credible biomarkers or characteristics for patient selection in HNSCC nanomedicine treatments necessitates further research.
Preserving a positive perspective on the potential potency of nanomedicine in treating HNSCC is paramount. Preclinical studies have exhibited extraordinary effects and hold substantial promise for the discipline. Addressing the obstacles outlined above necessitates collaborative endeavors across disciplines like materials science, nanoscience, chemistry, biology, medicine, and pharmacy. By nurturing interdisciplinary collaborations, we can strive towards the successful clinical implementation of nanomedicines, ultimately improving the prognosis and results for cancer patients.
Author contributions
H-XL: Conceptualization, Data curation, Investigation, Methodology, Software, Writing – original draft, Writing – review & editing. Y-WG: Investigation, Methodology, Software, Writing – review & editing. P-JY: Investigation, Methodology, Writing – review & editing. YX: Formal analysis, Resources, Writing – review & editing. GQ: Project administration, Resources, Supervision, Writing – review & editing. W-PW: Conceptualization, Project administration, Resources, Supervision, Writing – review & editing. F-YT: Funding acquisition, Resources, Writing – original draft, Writing – review & editing.
Funding
The author(s) declare financial support was received for the research, authorship, and/or publication of this article. This work was supported by the Research Start-up Fund of Affiliated Hospital of Southwest Medical University (grant number: 24075), the Natural Science Foundation of China (grant numbers: 82403915, 32201056), Sichuan Province Science and Technology Program Joint Innovation Project (grant number: 2022YFS0612), Sichuan Province Science and Technology Program (application number: 25QNJJ2586), Scientific Research Funding of Luzhou-Southwest Medical University (application number: 24YKDYB0097; grant number: 2021LZXNYD-J12).
Conflict of interest
The authors declare that the research was conducted in the absence of any commercial or financial relationships that could be construed as a potential conflict of interest.
Publisher’s note
All claims expressed in this article are solely those of the authors and do not necessarily represent those of their affiliated organizations, or those of the publisher, the editors and the reviewers. Any product that may be evaluated in this article, or claim that may be made by its manufacturer, is not guaranteed or endorsed by the publisher.
Abbreviations
ATP, adenosine 3-phosphate; Ce6, chlorinated e6; CPMV, cowpea mosaic virus; DAMPs, damage associated molecular patterns; DCs, dendritic cells; GBNs, Gadolinium-based NPs; GENPs, gelatin nanoparticles; GNPs, gold nanoparticles; HA-Toco, tocopherol-modified hyaluronic acid; Hb, hemoglobin; hNVs, hybrid membrane nanovasomes; HIF-1α, hypoxia-inducible factor-1α; HNSCC, head and neck squamous cell carcinoma; HNC, head and neck cancer; ICD, immunogenic cell death; ICG, indocyanine green; ICIs, immune checkpoint inhibitors; MMP, matrix metalloproteinase; LN, lymph node; MSR, mesoporous silica rods; NQO1, NAD(P)H: quinone oxidoreductase 1; NPs, nanoparticles; NSCLC, non-small cell lung cancer; OS, overall survival; OSCC, oral squamous cell carcinoma; PDT, photodynamic therapy; PD-1, programmed death receptor 1; PD-L1, programmed death ligand 1; PEG, polyethylene glycol; PTT, photothermal therapy; R/M, recurrent or metastatic; Tregs, regulatory T cells; ROS, reactive oxygen species; SDT, sonodynamic therapy; SIRPα, signal regulatory protein α; STING, stimulator of interferon genes; TAMs, tumor-associated macrophages; TME, tumor microenvironment; TMV, tobacco mosaic virus; TLR3, toll-like receptor 3; VH-MSNs, virus-like hollow mesoporous silica nanoparticles; VLPs, virus-like NPs.
References
1. Sung H, Ferlay J, Siegel RL, Laversanne M, Soerjomataram I, Jemal A, et al. Global cancer statistics 2020: GLOBOCAN estimates of incidence and mortality worldwide for 36 cancers in 185 countries. CA: Cancer J Clin. (2021) 71:209–49. doi: 10.3322/caac.21660
2. Sacco AG, Chen R, Worden FP, Wong DJL, Adkins D, Swiecicki P, et al. Pembrolizumab plus cetuximab in patients with recurrent or metastatic head and neck squamous cell carcinoma: an open-label, multi-arm, non-randomised, multicentre, phase 2 trial. Lancet Oncol. (2021) 22:883–92. doi: 10.1016/S1470-2045(21)00136-4
3. Bean MB, Liu Y, Jiang R, Steuer CE, Patel M, McDonald MW, et al. Small cell and squamous cell carcinomas of the head and neck: comparing incidence and survival trends based on surveillance, epidemiology, and end results (SEER) data. oncologist. (2019) 24:1562–9. doi: 10.1634/theoncologist.2018-0054
4. Argiris A, Karamouzis MV, Raben D, Ferris RL. Head and neck cancer. Lancet. (2008) 371:1695–709. doi: 10.1016/S0140-6736(08)60728-X
5. Lefebvre JL. Current clinical outcomes demand new treatment options for SCCHN. Ann Oncol. (2005) 16 Suppl 6:vi7–vi12. doi: 10.1093/annonc/mdi452
6. Johnson DE, Burtness B, Leemans CR, Lui VWY, Bauman JE, Grandis JR. Head and neck squamous cell carcinoma. Nat Rev Dis primers. (2020) 6:92. doi: 10.1038/s41572-020-00224-3
7. Cramer JD, Burtness B, Le QT, Ferris RL. The changing therapeutic landscape of head and neck cancer. Nat Rev Clin Oncol. (2019) 16:669–83. doi: 10.1038/s41571-019-0227-z
8. Xie M, Chaudhary R, Slebos RJC, Lee K, Song F, Poole MI, et al. Immune landscape in molecular subtypes of human papillomavirus-negative head and neck cancer. Mol carcinogenesis. (2024) 63:120–35. doi: 10.1002/mc.23640
9. Pfister DG, Spencer S, Brizel DM, Burtness B, Busse PM, Caudell JJ, et al. Head and neck cancers, Version 2. 2014. Clin Pract guidelines Oncol J Natl Compr Cancer Network: JNCCN. (2014) 12:1454–87. doi: 10.6004/jnccn.2014.0142
10. Bonner JA, Harari PM, Giralt J, Azarnia N, Shin DM, Cohen RB, et al. Radiotherapy plus cetuximab for squamous-cell carcinoma of the head and neck. New Engl J Med. (2006) 354:567–78. doi: 10.1056/NEJMoa053422
11. Concha-Benavente F, Srivastava RM, Ferrone S, Ferris RL. EGFR-mediated tumor immunoescape: The imbalance between phosphorylated STAT1 and phosphorylated STAT3. Oncoimmunology. (2013) 2:e27215. doi: 10.4161/onci.27215
12. Jie HB, Schuler PJ, Lee SC, Srivastava RM, Argiris A, Ferrone S, et al. CTLA-4(+) regulatory T cells increased in cetuximab-treated head and neck cancer patients suppress NK cell cytotoxicity and correlate with poor prognosis. Cancer Res. (2015) 75:2200–10. doi: 10.1158/0008-5472.CAN-14-2788
13. Jie HB, Srivastava RM, Argiris A, Bauman JE, Kane LP, Ferris RL. Increased PD-1(+) and TIM-3(+) TILs during cetuximab therapy inversely correlate with response in head and neck cancer patients. Cancer Immunol Res. (2017) 5:408–16. doi: 10.1158/2326-6066.CIR-16-0333
14. All Cancer Sites Combined Recent Trends in SEER Incidence and US Mortality Rates. Available online at: https://seercancergov/statisticsnetwork/explorer/applicationhtml (Accessed May 2022). 2000–2019.
15. Reck M, Rodriguez-Abreu D, Robinson AG, Hui R, Csoszi T, Fulop A, et al. Pembrolizumab versus chemotherapy for PD-L1-positive non-small-cell lung cancer. New Engl J Med. (2016) 375:1823–33. doi: 10.1056/NEJMoa1606774
16. Forde PM, Chaft JE, Smith KN, Anagnostou V, Cottrell TR, Hellmann MD, et al. Neoadjuvant PD-1 blockade in resectable lung cancer. New Engl J Med. (2018) 378:1976–86. doi: 10.1056/NEJMoa1716078
17. Cloughesy TF, Mochizuki AY, Orpilla JR, Hugo W, Lee AH, Davidson TB, et al. Neoadjuvant anti-PD-1 immunotherapy promotes a survival benefit with intratumoral and systemic immune responses in recurrent glioblastoma. Nat Med. (2019) 25:477–86. doi: 10.1038/s41591-018-0337-7
18. Olino K, Park T, Ahuja N. Exposing Hidden Targets: Combining epigenetic and immunotherapy to overcome cancer resistance. Semin Cancer Biol. (2020) 65:114–22. doi: 10.1016/j.semcancer.2020.01.001
19. Pires da Silva I, Ahmed T, Reijers ILM, Weppler AM, Betof Warner A, Patrinely JR, et al. Ipilimumab alone or ipilimumab plus anti-PD-1 therapy in patients with metastatic melanoma resistant to anti-PD-(L)1 monotherapy: a multicentre, retrospective, cohort study. Lancet Oncol. (2021) 22:836–47. doi: 10.1016/S1470-2045(21)00097-8
20. Stratigos AJ, Sekulic A, Peris K, Bechter O, Prey S, Kaatz M, et al. Cemiplimab in locally advanced basal cell carcinoma after hedgehog inhibitor therapy: an open-label, multi-centre, single-arm, phase 2 trial. Lancet Oncol. (2021) 22:848–57. doi: 10.1016/S1470-2045(21)00126-1
21. Ferris RL, Blumenschein G Jr., Fayette J, Guigay J, Colevas AD, Licitra L, et al. Nivolumab for recurrent squamous-cell carcinoma of the head and neck. New Engl J Med. (2016) 375:1856–67. doi: 10.1056/NEJMoa1602252
22. Seiwert TY, Burtness B, Mehra R, Weiss J, Berger R, Eder JP, et al. Safety and clinical activity of pembrolizumab for treatment of recurrent or metastatic squamous cell carcinoma of the head and neck (KEYNOTE-012): an open-label, multicentre, phase 1b trial. Lancet Oncol. (2016) 17:956–65. doi: 10.1016/S1470-2045(16)30066-3
23. Burtness B, Harrington KJ, Greil R, Soulieres D, Tahara M, de Castro G Jr., et al. Pembrolizumab alone or with chemotherapy versus cetuximab with chemotherapy for recurrent or metastatic squamous cell carcinoma of the head and neck (KEYNOTE-048): a randomised, open-label, phase 3 study. Lancet. (2019) 394:1915–28. doi: 10.1016/S0140-6736(19)32591-7
24. Lee NY, Ferris RL, Psyrri A, Haddad RI, Tahara M, Bourhis J, et al. Avelumab plus standard-of-care chemoradiotherapy versus chemoradiotherapy alone in patients with locally advanced squamous cell carcinoma of the head and neck: a randomised, double-blind, placebo-controlled, multicentre, phase 3 trial. Lancet Oncol. (2021) 22:450–62. doi: 10.1016/S1470-2045(20)30737-3
25. Vos JL, Elbers JBW, Krijgsman O, Traets JJH, Qiao X, van der Leun AM, et al. Neoadjuvant immunotherapy with nivolumab and ipilimumab induces major pathological responses in patients with head and neck squamous cell carcinoma. Nat Commun. (2021) 12:7348. doi: 10.1038/s41467-021-26472-9
26. Ferris RL, Licitra L, Fayette J, Even C, Blumenschein G Jr., Harrington KJ, et al. Nivolumab in patients with recurrent or metastatic squamous cell carcinoma of the head and neck: efficacy and safety in checkMate 141 by prior cetuximab use. Clin Cancer Res. (2019) 25:5221–30. doi: 10.1158/1078-0432.CCR-18-3944
27. Friedman CF, Proverbs-Singh TA, Postow MA. Treatment of the immune-related adverse effects of immune checkpoint inhibitors: A review. JAMA Oncol. (2016) 2:1346–53. doi: 10.1001/jamaoncol.2016.1051
28. Postow MA, Hellmann MD. Adverse events associated with immune checkpoint blockade. New Engl J Med. (2018) 378:1163–5. doi: 10.1056/NEJMra1703481
29. Liu Z, Jiang W, Nam J, Moon JJ, Kim BYS. Immunomodulating nanomedicine for cancer therapy. Nano letters. (2018) 18:6655–9. doi: 10.1021/acs.nanolett.8b02340
30. Irvine DJ, Dane EL. Enhancing cancer immunotherapy with nanomedicine. Nat Rev Immunol. (2020) 20:321–34. doi: 10.1038/s41577-019-0269-6
31. Van Herck S, De Geest BG. Nanomedicine-mediated alteration of the pharmacokinetic profile of small molecule cancer immunotherapeutics. Acta pharmacologica Sinica. (2020) 41:881–94. doi: 10.1038/s41401-020-0425-3
32. Wang J, Sui L, Huang J, Miao L, Nie Y, Wang K, et al. MoS(2)-based nanocomposites for cancer diagnosis and therapy. Bioact Mater. (2021) 6:4209–42. doi: 10.1016/j.bioactmat.2021.04.021
33. Dhas N, Kudarha R, Garkal A, Ghate V, Sharma S, Panzade P, et al. Molybdenum-based hetero-nanocomposites for cancer therapy, diagnosis and biosensing application: Current advancement and future breakthroughs. J Control Release. (2021) 330:257–83. doi: 10.1016/j.jconrel.2020.12.015
34. Ruiz-Pulido G, Medina DI, Barani M, Rahdar A, Sargazi G, Baino F, et al. Nanomaterials for the diagnosis and treatment of head and neck cancers: A review. Materials (Basel). (2021) 14:3706. doi: 10.3390/ma14133706
35. Ding Z, Sigdel K, Yang L, Liu Y, Xuan M, Wang X, et al. Nanotechnology-based drug delivery systems for enhanced diagnosis and therapy of oral cancer. J materials Chem B. (2020) 8:8781–93. doi: 10.1039/D0TB00957A
36. Viegas C, Pereira DSM, Fonte P. Insights into nanomedicine for head and neck cancer diagnosis and treatment. Materials (Basel). (2022) 15:2086. doi: 10.3390/ma15062086
37. Xu J, Liu H, Wang T, Wen Z, Chen H, Yang Z, et al. CCR7 mediated mimetic dendritic cell vaccine homing in lymph node for head and neck squamous cell carcinoma therapy. Advanced science. (2023) 10:e2207017. doi: 10.1002/advs.202207017
38. Shi Y, Lammers T. Combining nanomedicine and immunotherapy. Accounts Chem Res. (2019) 52:1543–54. doi: 10.1021/acs.accounts.9b00148
39. Chen J, Cong X. Surface-engineered nanoparticles in cancer immune response and immunotherapy: Current status and future prospects. BioMed Pharmacother. (2023) 157:113998. doi: 10.1016/j.biopha.2022.113998
40. Li Z, Lai X, Fu S, Ren L, Cai H, Zhang H, et al. Immunogenic cell death activates the tumor immune microenvironment to boost the immunotherapy efficiency. Advanced science. (2022) 9:e2201734. doi: 10.1002/advs.202201734
41. Huayamares SG, Lokugamage MP, Rab R, Da Silva Sanchez AJ, Kim H, Radmand A, et al. High-throughput screens identify a lipid nanoparticle that preferentially delivers mRNA to human tumors in vivo. J Control Release. (2023) 357:394–403. doi: 10.1016/j.jconrel.2023.04.005
42. Yan W, Li Y, Zou Y, Zhu R, Wu T, Sun X, et al. Breaking tumor immunosuppressive network by regulating multiple nodes with triadic drug delivery nanoparticles. ACS nano. (2023) 17:17826–44. doi: 10.1021/acsnano.3c03387
43. Dubey P, Sertorio M, Takiar V. Therapeutic advancements in metal and metal oxide nanoparticle-based radiosensitization for head and neck cancer therapy. Cancers. (2022) 14:514. doi: 10.3390/cancers14030514
44. Huynh M, Kempson I, Bezak E, Phillips W. In silico modeling of cellular probabilistic nanoparticle radiosensitization in head and neck cancers. Nanomedicine (Lond). (2020) 15:2837–50. doi: 10.2217/nnm-2020-0301
45. Huynh M, Kempson I, Bezak E, Phillips W. Predictive modeling of hypoxic head and neck cancers during fractionated radiotherapy with gold nanoparticle radiosensitization. Med physics. (2021) 48:3120–33. doi: 10.1002/mp.14872
46. Sato T, Kakei Y, Hasegawa T, Kashin M, Teraoka S, Yamaguchi A, et al. Gold nanoparticles enhance the tumor growth-suppressing effects of cetuximab and radiotherapy in head and neck cancer in vitro and in vivo. Cancers. (2023) 15:5697. doi: 10.3390/cancers15235697
47. Ahmed S, Baijal G, Somashekar R, Iyer S, Nayak V. One pot synthesis of PEGylated bimetallic gold-silver nanoparticles for imaging and radiosensitization of oral cancers. Int J nanomedicine. (2021) 16:7103–21. doi: 10.2147/IJN.S329762
48. Dehghankelishadi P, Maritz MF, Dmochowska N, Badiee P, Cheah E, Kempson I, et al. Formulation of simvastatin within high density lipoprotein enables potent tumour radiosensitisation. J Control Release. (2022) 346:98–109. doi: 10.1016/j.jconrel.2022.04.017
49. Bhardwaj P, Gota V, Vishwakarma K, Pai V, Chaudhari P, Mohanty B, et al. Loco-regional radiosensitizing nanoparticles-in-gel augments head and neck cancer chemoradiotherapy. J Control Release. (2022) 343:288–302. doi: 10.1016/j.jconrel.2022.01.040
50. Simonet S, Rodriguez-Lafrasse C, Beal D, Gerbaud S, Malesys C, Tillement O, et al. Gadolinium-based nanoparticles can overcome the radioresistance of head and neck squamous cell carcinoma through the induction of autophagy. J Biomed nanotechnology. (2020) 16:111–24. doi: 10.1166/jbn.2020.2871
51. Tan YS, Sansanaphongpricha K, Xie Y, Donnelly CR, Luo X, Heath BR, et al. Mitigating SOX2-potentiated immune escape of head and neck squamous cell carcinoma with a STING-inducing nanosatellite vaccine. Clin Cancer Res. (2018) 24:4242–55. doi: 10.1158/1078-0432.CCR-17-2807
52. Dharmaraj N, Piotrowski SL, Huang C, Newton JM, Golfman LS, Hanoteau A, et al. Anti-tumor immunity induced by ectopic expression of viral antigens is transient and limited by immune escape. Oncoimmunology. (2019) 8:e1568809. doi: 10.1080/2162402X.2019.1568809
53. Salomon N, Selmi A, Grunwitz C, Kong A, Stanganello E, Neumaier J, et al. Local radiotherapy and E7 RNA-LPX vaccination show enhanced therapeutic efficacy in preclinical models of HPV16(+) cancer. Cancer immunology immunotherapy: CII. (2022) 71:1975–88. doi: 10.1007/s00262-021-03134-9
54. Wu Y, Chang X, Yang G, Chen L, Wu Q, Gao J, et al. A physiologically responsive nanocomposite hydrogel for treatment of head and neck squamous cell carcinoma via proteolysis-targeting chimeras enhanced immunotherapy. Advanced materials. (2023) 35(12):e2210787. doi: 10.1002/adma.202210787
55. Chen XJ, Zhang XQ, Tang MX, Liu Q, Zhou G. Anti-PD-L1-modified and ATRA-loaded nanoparticles for immuno-treatment of oral dysplasia and oral squamous cell carcinoma. Nanomedicine (Lond). (2020) 15:951–68. doi: 10.2217/nnm-2019-0397
56. Li H, Liu M, Zhang S, Xie X, Zhu Y, Liu T, et al. Construction of cpG delivery nanoplatforms by functionalized moS(2) nanosheets for boosting antitumor immunity in head and neck squamous cell carcinoma. Small. (2023) 1613-6810:e2300380. doi: 10.1002/smll.202300380
57. Zheng DW, Deng WW, Song WF, Wu CC, Liu J, Hong S, et al. Biomaterial-mediated modulation of oral microbiota synergizes with PD-1 blockade in mice with oral squamous cell carcinoma. Nat BioMed Eng. (2022) 6:32–43. doi: 10.1038/s41551-021-00807-9
58. Cohen EM, Ding H, Kessinger CW, Khemtong C, Gao J, Sumer BD. Polymeric micelle nanoparticles for photodynamic treatment of head and neck cancer cells. Otolaryngol Head Neck Surg. (2010) 143:109–15. doi: 10.1016/j.otohns.2010.03.032
59. Hackenberg S, Scherzed A, Kessler M, Froelich K, Ginzkey C, Koehler C, et al. Zinc oxide nanoparticles induce photocatalytic cell death in human head and neck squamous cell carcinoma cell lines in vitro. Int J Oncol. (2010) 37:1583–90. doi: 10.3892/ijo_00000812
60. Bu LL, Wang HQ, Pan Y, Chen L, Wu H, Wu X, et al. Gelatinase-sensitive nanoparticles loaded with photosensitizer and STAT3 inhibitor for cancer photothermal therapy and immunotherapy. J Nanobiotechnology. (2021) 19:379. doi: 10.1186/s12951-021-01125-7
61. Zhou JY, Wang WJ, Zhang CY, Ling YY, Hong XJ, Su Q, et al. Ru(II)-modified TiO(2) nanoparticles for hypoxia-adaptive photo-immunotherapy of oral squamous cell carcinoma. Biomaterials. (2022) 289:121757. doi: 10.1016/j.biomaterials.2022.121757
62. Zhu T, Shi L, Yu C, Dong Y, Qiu F, Shen L, et al. Ferroptosis promotes photodynamic therapy: supramolecular photosensitizer-inducer nanodrug for enhanced cancer treatment. Theranostics. (2019) 9:3293–307. doi: 10.7150/thno.32867
63. Wang ZQ, Liu K, Huo ZJ, Li XC, Wang M, Liu P, et al. A cell-targeted chemotherapeutic nanomedicine strategy for oral squamous cell carcinoma therapy. J Nanobiotechnology. (2015) 13:63. doi: 10.1186/s12951-015-0116-2
64. Lang L, Shay C, Xiong Y, Thakkar P, Chemmalakuzhy R, Wang X, et al. Combating head and neck cancer metastases by targeting Src using multifunctional nanoparticle-based saracatinib. J Hematol Oncol. (2018) 11:85. doi: 10.1186/s13045-018-0623-3
65. Lang L, Shay C, Zhao X, Xiong Y, Wang X, Teng Y. Simultaneously inactivating Src and AKT by saracatinib/capivasertib co-delivery nanoparticles to improve the efficacy of anti-Src therapy in head and neck squamous cell carcinoma. J Hematol Oncol. (2019) 12:132. doi: 10.1186/s13045-019-0827-1
66. Yanes-Diaz J, Palao-Suay R, Aguilar MR, Riestra-Ayora JI, Ferruelo-Alonso A, Rojo Del Olmo L, et al. Antitumor activity of nanoparticles loaded with PHT-427, a novel AKT/PDK1 inhibitor, for the treatment of head and neck squamous cell carcinoma. Pharmaceutics. (2021) 13:1242. doi: 10.3390/pharmaceutics13081242
67. Wang H, Mu X, He H, Zhang XD. Cancer radiosensitizers. Trends Pharmacol Sci. (2018) 39:24–48. doi: 10.1016/j.tips.2017.11.003
68. Popovtzer A, Mizrachi A, Motiei M, Bragilovski D, Lubimov L, Levi M, et al. Actively targeted gold nanoparticles as novel radiosensitizer agents: an in vivo head and neck cancer model. Nanoscale. (2016) 8:2678–85. doi: 10.1039/C5NR07496G
69. Zhao J, Zhou M, Li C. Synthetic nanoparticles for delivery of radioisotopes and radiosensitizers in cancer therapy. Cancer Nanotechnol. (2016) 7:9. doi: 10.1186/s12645-016-0022-9
70. Brown JM, Carlson DJ, Brenner DJ. The tumor radiobiology of SRS and SBRT: are more than the 5 Rs involved? Int J Radiat oncology biology Phys. (2014) 88:254–62. doi: 10.1016/j.ijrobp.2013.07.022
71. Ma N, Wu FG, Zhang X, Jiang YW, Jia HR, Wang HY, et al. Shape-dependent radiosensitization effect of gold nanostructures in cancer radiotherapy: comparison of gold nanoparticles, nanospikes, and nanorods. ACS Appl materials interfaces. (2017) 9:13037–48. doi: 10.1021/acsami.7b01112
72. Chen Y, Yang J, Fu S, Wu J. Gold nanoparticles as radiosensitizers in cancer radiotherapy. Int J nanomedicine. (2020) 15:9407–30. doi: 10.2147/IJN.S272902
73. Qin X, Yang C, Xu H, Zhang R, Zhang D, Tu J, et al. Cell-derived biogenetic gold nanoparticles for sensitizing radiotherapy and boosting immune response against cancer. Small. (2021) 17:e2103984. doi: 10.1002/smll.202103984
74. Luan S, Xie R, Yang Y, Xiao X, Zhou J, Li X, et al. Acid-responsive aggregated gold nanoparticles for radiosensitization and synergistic chemoradiotherapy in the treatment of esophageal cancer. Small. (2022) 18:e2200115. doi: 10.1002/smll.202200115
75. Hainfeld JF, Dilmanian FA, Zhong Z, Slatkin DN, Kalef-Ezra JA, Smilowitz HM. Gold nanoparticles enhance the radiation therapy of a murine squamous cell carcinoma. Phys Med Biol. (2010) 55:3045–59. doi: 10.1088/0031-9155/55/11/004
76. Jiang X, Zhang B, Zhou Z, Meng L, Sun Z, Xu Y, et al. Enhancement of radiotherapy efficacy by pleiotropic liposomes encapsulated paclitaxel and perfluorotributylamine. Drug Deliv. (2017) 24:1419–28. doi: 10.1080/10717544.2017.1378939
77. Song G, Liang C, Yi X, Zhao Q, Cheng L, Yang K, et al. Perfluorocarbon-loaded hollow bi2Se3 nanoparticles for timely supply of oxygen under near-infrared light to enhance the radiotherapy of cancer. Advanced materials. (2016) 28:2716–23. doi: 10.1002/adma.201504617
78. Huang Y, Luo Y, Zheng W, Chen T. Rational design of cancer-targeted BSA protein nanoparticles as radiosensitizer to overcome cancer radioresistance. ACS Appl materials interfaces. (2014) 6:19217–28. doi: 10.1021/am505246w
79. Au KM, Min Y, Tian X, Zhang L, Perello V, Caster JM, et al. Improving cancer chemoradiotherapy treatment by dual controlled release of wortmannin and docetaxel in polymeric nanoparticles. ACS nano. (2015) 9:8976–96. doi: 10.1021/acsnano.5b02913
80. Han J, Yang W, Li Y, Li J, Jiang F, Xie J, et al. Combining doxorubicin-conjugated polymeric nanoparticles and 5-aminolevulinic acid for enhancing radiotherapy against lung cancer. Bioconjug Chem. (2022) 33:654–65. doi: 10.1021/acs.bioconjchem.2c00066
81. Dehghankelishadi P, Badiee P, Maritz MF, Dmochowska N, Thierry B. Bosutinib high density lipoprotein nanoformulation has potent tumour radiosensitisation effects. J Nanobiotechnology. (2023) 21:102. doi: 10.1186/s12951-023-01848-9
82. Ricco N, Flor A, Wolfgeher D, Efimova EV, Ramamurthy A, Appelbe OK, et al. Mevalonate pathway activity as a determinant of radiation sensitivity in head and neck cancer. Mol Oncol. (2019) 13:1927–43. doi: 10.1002/1878-0261.12535
83. Efimova EV, Ricco N, Labay E, Mauceri HJ, Flor AC, Ramamurthy A, et al. HMG-coA reductase inhibition delays DNA repair and promotes senescence after tumor irradiation. Mol Cancer Ther. (2018) 17:407–18. doi: 10.1158/1535-7163.MCT-17-0288
84. Longo J, Hamilton RJ, Masoomian M, Khurram N, Branchard E, Mullen PJ, et al. A pilot window-of-opportunity study of preoperative fluvastatin in localized prostate cancer. Prostate Cancer prostatic diseases. (2020) 23:630–7. doi: 10.1038/s41391-020-0221-7
85. Weed DT, Zilio S, McGee C, Marnissi B, Sargi Z, Franzmann E, et al. The tumor immune microenvironment architecture correlates with risk of recurrence in head and neck squamous cell carcinoma. Cancer Res. (2023) 83:3886–900. doi: 10.1158/0008-5472.CAN-23-0379
86. Rad HS, Shiravand Y, Radfar P, Ladwa R, Perry C, Han X, et al. Understanding the tumor microenvironment in head and neck squamous cell carcinoma. Clin Transl Immunol. (2022) 11:e1397. doi: 10.1002/cti2.v11.6
87. Chen DS, Mellman I. Elements of cancer immunity and the cancer-immune set point. Nature. (2017) 541:321–30. doi: 10.1038/nature21349
88. Kather JN, Suarez-Carmona M, Charoentong P, Weis CA, Hirsch D, Bankhead P, et al. Topography of cancer-associated immune cells in human solid tumors. Elife. (2018) 7:e36967. doi: 10.7554/eLife.36967
89. Huang C, Chen L, Savage SR, Eguez RV, Dou Y, Li Y, et al. Proteogenomic insights into the biology and treatment of HPV-negative head and neck squamous cell carcinoma. Cancer Cell. (2021) 39:361–79.e16. doi: 10.1016/j.ccell.2020.12.007
90. Muntasell A, Ochoa MC, Cordeiro L, Berraondo P, Lopez-Diaz de Cerio A, Cabo M, et al. Targeting NK-cell checkpoints for cancer immunotherapy. Curr Opin Immunol. (2017) 45:73–81. doi: 10.1016/j.coi.2017.01.003
91. Shalapour S, Karin M. Immunity, inflammation, and cancer: an eternal fight between good and evil. J Clin Invest. (2015) 125:3347–55. doi: 10.1172/JCI80007
92. Elmusrati A, Wang J, Wang CY. Tumor microenvironment and immune evasion in head and neck squamous cell carcinoma. Int J Oral Sci. (2021) 13:24. doi: 10.1038/s41368-021-00131-7
93. Wolpaw AJ, Grossmann LD, Dessau JL, Dong MM, Aaron BJ, Brafford PA, et al. Epigenetic state determines inflammatory sensing in neuroblastoma. Proc Natl Acad Sci U.S.A. (2022) 119:e2102358119. doi: 10.1073/pnas.2102358119
94. Jenkins RW, Barbie DA, Flaherty KT. Mechanisms of resistance to immune checkpoint inhibitors. Br J Cancer. (2018) 118:9–16. doi: 10.1038/bjc.2017.434
95. Gu GJ, Chung H, Park JY, Yoo R, Im HJ, Choi H, et al. Mannosylated-serum albumin nanoparticle imaging to monitor tumor-associated macrophages under anti-PD1 treatment. J Nanobiotechnology. (2023) 21:31. doi: 10.1186/s12951-023-01791-9
96. Roudi R, Pisani L, Pisani F, Kiru L, Daldrup-Link HE. Novel clinically translatable iron oxide nanoparticle for monitoring anti-CD47 cancer immunotherapy. Invest Radiol. (2023) 59(5):391–403. doi: 10.1097/RLI.0000000000001030
97. Xu Q, Fang M, Zhu J, Dong H, Cao J, Yan L, et al. Insights into Nanomedicine for Immunotherapeutics in Squamous Cell Carcinoma of the head and neck. Int J Biol Sci. (2020) 16:2506–17. doi: 10.7150/ijbs.47068
98. Chen X, Tang Q, Wang J, Zhou Y, Li F, Xie Y, et al. A DNA/DMXAA/metal-organic framework activator of innate immunity for boosting anticancer immunity. Advanced materials. (2023) 35:e2210440. doi: 10.1002/adma.202210440
99. Saxena M, van der Burg SH, Melief CJM, Bhardwaj N. Therapeutic cancer vaccines. Nat Rev Cancer. (2021) 21:360–78. doi: 10.1038/s41568-021-00346-0
100. Schuler PJ, Harasymczuk M, Visus C, Deleo A, Trivedi S, Lei Y, et al. Phase I dendritic cell p53 peptide vaccine for head and neck cancer. Clin Cancer Res. (2014) 20:2433–44. doi: 10.1158/1078-0432.CCR-13-2617
101. Qian X, Ma C, Nie X, Lu J, Lenarz M, Kaufmann AM, et al. Biology and immunology of cancer stem(-like) cells in head and neck cancer. Crit Rev oncology/hematology. (2015) 95:337–45. doi: 10.1016/j.critrevonc.2015.03.009
102. van Poelgeest MI, Welters MJ, Vermeij R, Stynenbosch LF, Loof NM, Berends-van der Meer DM, et al. Vaccination against oncoproteins of HPV16 for noninvasive vulvar/vaginal lesions: lesion clearance is related to the strength of the T-cell response. Clin Cancer Res. (2016) 22:2342–50. doi: 10.1158/1078-0432.CCR-15-2594
103. Massarelli E, William W, Johnson F, Kies M, Ferrarotto R, Guo M, et al. Combining immune checkpoint blockade and tumor-specific vaccine for patients with incurable human papillomavirus 16-related cancer: A phase 2 clinical trial. JAMA Oncol. (2019) 5:67–73. doi: 10.1001/jamaoncol.2018.4051
104. Beyaert S, Machiels JP, Schmitz S. Vaccine-based immunotherapy for head and neck cancers. Cancers. (2021) 13:6041. doi: 10.3390/cancers13236041
105. Grippin AJ, Sayour EJ, Mitchell DA. Translational nanoparticle engineering for cancer vaccines. Oncoimmunology. (2017) 6:e1290036. doi: 10.1080/2162402X.2017.1290036
106. Rahimian S, Fransen MF, Kleinovink JW, Christensen JR, Amidi M, Hennink WE, et al. Polymeric nanoparticles for co-delivery of synthetic long peptide antigen and poly IC as therapeutic cancer vaccine formulation. J Control Release. (2015) 203:16–22. doi: 10.1016/j.jconrel.2015.02.006
107. Mohsen MO, Augusto G, Bachmann MF. The 3Ds in virus-like particle based-vaccines: "Design, Delivery and Dynamics. Immunol Rev. (2020) 296:155–68. doi: 10.1111/imr.v296.1
108. Mohsen MO, Bachmann MF. Virus-like particle vaccinology, from bench to bedside. Cell Mol Immunol. (2022) 19:993–1011. doi: 10.1038/s41423-022-00897-8
109. Nooraei S, Bahrulolum H, Hoseini ZS, Katalani C, Hajizade A, Easton AJ, et al. Virus-like particles: preparation, immunogenicity and their roles as nanovaccines and drug nanocarriers. J Nanobiotechnology. (2021) 19:59. doi: 10.1186/s12951-021-00806-7
110. Xu D, Song X, Zhou J, Ouyang X, Li J, Deng D. Virus-like hollow mesoporous silica nanoparticles for cancer combination therapy. Colloids Surf B Biointerfaces. (2021) 197:111452. doi: 10.1016/j.colsurfb.2020.111452
111. Cheng Y, Lemke-Miltner CD, Wongpattaraworakul W, Wang Z, Chan CHF, Salem AK, et al. In situ immunization of a TLR9 agonist virus-like particle enhances anti-PD1 therapy. J Immunother Cancer. (2020) 8:e000940. doi: 10.1136/jitc-2020-000940
112. DeNardo DG, Ruffell B. Macrophages as regulators of tumour immunity and immunotherapy. Nat Rev Immunol. (2019) 19:369–82. doi: 10.1038/s41577-019-0127-6
113. Costa NL, Valadares MC, Souza PPC, Mendonça EF, Oliveira JC, Silva TA, et al. Tumor-associated macrophages and the profile of inflammatory cytokines in oral squamous cell carcinoma. Oral Oncol. (2013) 49:216–23. doi: 10.1016/j.oraloncology.2012.09.012
114. Kumar AT, Knops A, Swendseid B, Martinez-Outschoom U, Harshyne L, Philp N, et al. Prognostic significance of tumor-associated macrophage content in head and neck squamous cell carcinoma: A meta-analysis. Front Oncol. (2019) 9:656. doi: 10.3389/fonc.2019.00656
115. Li X, Liu R, Su X, Pan Y, Han X, Shao C, et al. Harnessing tumor-associated macrophages as aids for cancer immunotherapy. Mol Cancer. (2019) 18:177. doi: 10.1186/s12943-019-1102-3
116. Li C, Xu X, Wei S, Jiang P, Xue L, Wang J, et al. Tumor-associated macrophages: potential therapeutic strategies and future prospects in cancer. J Immunother Cancer. (2021) 9:e001341. doi: 10.1136/jitc-2020-001341
117. Lin Y, Xu J, Lan H. Tumor-associated macrophages in tumor metastasis: biological roles and clinical therapeutic applications. J Hematol Oncol. (2019) 12:76. doi: 10.1186/s13045-019-0760-3
118. Duan Z, Luo Y. Targeting macrophages in cancer immunotherapy. Signal Transduct Target Ther. (2021) 6:127. doi: 10.1038/s41392-021-00506-6
119. Ovais M, Guo M, Chen C. Tailoring nanomaterials for targeting tumor-associated macrophages. Advanced materials. (2019) 31:e1808303. doi: 10.1002/adma.201808303
120. Sylvestre M, Crane CA, Pun SH. Progress on modulating tumor-associated macrophages with biomaterials. Advanced materials. (2020) 32:e1902007. doi: 10.1002/adma.201902007
121. Kumari N, Choi SH. Tumor-associated macrophages in cancer: recent advancements in cancer nanoimmunotherapies. J Exp Clin Cancer research: CR. (2022) 41:68. doi: 10.1186/s13046-022-02272-x
122. Kim Y, Lee S, Jon S. Liposomal delivery of an immunostimulatory cpG induces robust antitumor immunity and long-term immune memory by reprogramming tumor-associated macrophages. Adv Healthc Mater. (2023) 13(6):e2300549. doi: 10.1002/adhm.202300549
123. Zheng J, Jiang J, Pu Y, Xu T, Sun J, Zhang Q, et al. Tumor-associated macrophages in nanomaterial-based anti-tumor therapy: as target spots or delivery platforms. Front bioengineering Biotechnol. (2023) 11:1248421. doi: 10.3389/fbioe.2023.1248421
124. Rodell CB, Arlauckas SP, Cuccarese MF, Garris CS, Li R, Ahmed MS, et al. TLR7/8-agonist-loaded nanoparticles promote the polarization of tumour-associated macrophages to enhance cancer immunotherapy. Nat BioMed Eng. (2018) 2:578–88. doi: 10.1038/s41551-018-0236-8
125. Lu R, Groer C, Kleindl PA, Moulder KR, Huang A, Hunt JR, et al. Formulation and preclinical evaluation of a toll-like receptor 7/8 agonist as an anti-tumoral immunomodulator. J Control Release. (2019) 306:165–76. doi: 10.1016/j.jconrel.2019.06.003
126. Zhang F, Parayath NN, Ene CI, Stephan SB, Koehne AL, Coon ME, et al. Genetic programming of macrophages to perform anti-tumor functions using targeted mRNA nanocarriers. Nat Commun. (2019) 10:3974. doi: 10.1038/s41467-019-11911-5
127. Wang Y, Xu H, Wang D, Lu Y, Zhang Y, Cheng J, et al. Synergistic reinforcement of immunogenic cell death and transformation of tumor-associated macrophages via an M1-type macrophage membrane-camouflaged ferrous-supply-regeneration nanoplatform. Acta Biomater. (2024) 174:358–71. doi: 10.1016/j.actbio.2023.11.041
128. Rao L, Wu L, Liu Z, Tian R, Yu G, Zhou Z, et al. Hybrid cellular membrane nanovesicles amplify macrophage immune responses against cancer recurrence and metastasis. Nat Commun. (2020) 11:4909. doi: 10.1038/s41467-020-18626-y
129. Liu Y, Lu X, Qin N, Qiao Y, Xing S, Liu W, et al. STING, a promising target for small molecular immune modulator: A review. Eur J Med Chem. (2021) 211:113113. doi: 10.1016/j.ejmech.2020.113113
130. Cui C, Chakraborty K, Tang XA, Schoenfelt KQ, Hoffman A, Blank A, et al. A lysosome-targeted DNA nanodevice selectively targets macrophages to attenuate tumours. Nat nanotechnology. (2021) 16:1394–402. doi: 10.1038/s41565-021-00988-z
131. Sato-Kaneko F, Yao S, Ahmadi A, Zhang SS, Hosoya T, Kaneda MM, et al. Combination immunotherapy with TLR agonists and checkpoint inhibitors suppresses head and neck cancer. JCI Insight. (2017) 2:e93397. doi: 10.1172/jci.insight.93397
132. Jurgens DC, Winkeljann B, Kolog Gulko M, Jin Y, Moller J, Winkeljann J, et al. Efficient and targeted siRNA delivery to M2 macrophages by smart polymer blends for M1 macrophage repolarization as a promising strategy for future cancer treatment. ACS Biomater Sci Eng. (2023) 10(1):166–77. doi: 10.1021/acsbiomaterials.3c01595
133. Leonard F, Curtis LT, Hamed AR, Zhang C, Chau E, Sieving D, et al. Nonlinear response to cancer nanotherapy due to macrophage interactions revealed by mathematical modeling and evaluated in a murine model via CRISPR-modulated macrophage polarization. Cancer immunology immunotherapy: CII. (2020) 69:731–44. doi: 10.1007/s00262-020-02504-z
134. Giles JR, Globig AM, Kaech SM, Wherry EJ. CD8(+) T cells in the cancer-immunity cycle. Immunity. (2023) 56:2231–53. doi: 10.1016/j.immuni.2023.09.005
135. Amouzegar A, Chelvanambi M, Filderman JN, Storkus WJ, Luke JJ. STING agonists as cancer therapeutics. Cancers. (2021) 13:2695. doi: 10.3390/cancers13112695
136. Gasparri AM, Sacchi A, Basso V, Cortesi F, Freschi M, Rrapaj E, et al. Boosting interleukin-12 antitumor activity and synergism with immunotherapy by targeted delivery with isoDGR-tagged nanogold. Small. (2019) 15:e1903462. doi: 10.1002/smll.201903462
137. Flood BA, Higgs EF, Li S, Luke JJ, Gajewski TF. STING pathway agonism as a cancer therapeutic. Immunol Rev. (2019) 290:24–38. doi: 10.1111/imr.v290.1
138. Cheng N, Watkins-Schulz R, Junkins RD, David CN, Johnson BM, Montgomery SA, et al. A nanoparticle-incorporated STING activator enhances antitumor immunity in PD-L1-insensitive models of triple-negative breast cancer. JCI Insight. (2018) 3:e120638. doi: 10.1172/jci.insight.120638
139. Kreutz M, Tacken PJ, Figdor CG. Targeting dendritic cells–why bother? Blood. (2013) 121:2836–44. doi: 10.1182/blood-2012-09-452078
140. Dong H, Wen ZF, Chen L, Zhou N, Liu H, Dong S, et al. Polyethyleneimine modification of aluminum hydroxide nanoparticle enhances antigen transportation and cross-presentation of dendritic cells. Int J nanomedicine. (2018) 13:3353–65. doi: 10.2147/IJN.S164097
142. Mager LF, Burkhard R, Pett N, Cooke NCA, Brown K, Ramay H, et al. Microbiome-derived inosine modulates response to checkpoint inhibitor immunotherapy. Science. (2020) 369:1481–9. doi: 10.1126/science.abc3421
143. Wang KK, He KY, Yang JY, Liu MJ, Guo JR, Liang JY, et al. Lactobacillus suppresses tumorigenesis of oropharyngeal cancer via enhancing anti-tumor immune response. Front Cell Dev Biol. (2022) 10:842153. doi: 10.3389/fcell.2022.842153
144. Li X, Lovell JF, Yoon J, Chen X. Clinical development and potential of photothermal and photodynamic therapies for cancer. Nat Rev Clin Oncol. (2020) 17:657–74. doi: 10.1038/s41571-020-0410-2
145. Son S, Kim JH, Wang X, Zhang C, Yoon SA, Shin J, et al. Multifunctional sonosensitizers in sonodynamic cancer therapy. Chem Soc Rev. (2020) 49:3244–61. doi: 10.1039/C9CS00648F
146. Zhang L, Chu C, Lin X, Sun R, Li Z, Chen S, et al. Tunable nanoparticles with aggregation-induced emission heater for precise synergistic photothermal and thermodynamic oral cancer therapy of patient-derived tumor xenograft. Advanced science. (2023) 10:e2205780. doi: 10.1002/advs.202205780
147. Alzeibak R, Mishchenko TA, Shilyagina NY, Balalaeva IV, Vedunova MV, Krysko DV. Targeting immunogenic cancer cell death by photodynamic therapy: past, present and future. J Immunother Cancer. (2021) 9:e001926. doi: 10.1136/jitc-2020-001926
148. Zhang S, Wang J, Kong Z, Sun X, He Z, Sun B, et al. Emerging photodynamic nanotherapeutics for inducing immunogenic cell death and potentiating cancer immunotherapy. Biomaterials. (2022) 282:121433. doi: 10.1016/j.biomaterials.2022.121433
149. Ji B, Wei M, Yang B. Recent advances in nanomedicines for photodynamic therapy (PDT)-driven cancer immunotherapy. Theranostics. (2022) 12:434–58. doi: 10.7150/thno.67300
150. Liu Y, Bhattarai P, Dai Z, Chen X. Photothermal therapy and photoacoustic imaging via nanotheranostics in fighting cancer. Chem Soc Rev. (2019) 48:2053–108. doi: 10.1039/C8CS00618K
151. Xu X, Wang Y, Lauer-Fields JL, Fields GB, Steffensen B. Contributions of the MMP-2 collagen binding domain to gelatin cleavage. Substrate binding via the collagen binding domain is required for hydrolysis of gelatin but not short peptides. Matrix biology: J Int Soc Matrix Biol. (2004) 23:171–81. doi: 10.1016/j.matbio.2004.05.002
152. Saito M, Kimoto M, Araki T, Shimada Y, Fujii R, Oofusa K, et al. Proteome analysis of gelatin-bound urinary proteins from patients with bladder cancers. Eur urology. (2005) 48:865–71. doi: 10.1016/j.eururo.2005.04.028
153. Shan X, Li S, Sun B, Chen Q, Sun J, He Z, et al. Ferroptosis-driven nanotherapeutics for cancer treatment. J Control Release. (2020) 319:322–32. doi: 10.1016/j.jconrel.2020.01.008
154. Xu T, Ma Y, Yuan Q, Hu H, Hu X, Qian Z, et al. Enhanced ferroptosis by oxygen-boosted phototherapy based on a 2-in-1 nanoplatform of ferrous hemoglobin for tumor synergistic therapy. ACS nano. (2020) 14:3414–25. doi: 10.1021/acsnano.9b09426
155. Mitchell MJ, Billingsley MM, Haley RM, Wechsler ME, Peppas NA, Langer R. Engineering precision nanoparticles for drug delivery. Nat Rev Drug discovery. (2021) 20:101–24. doi: 10.1038/s41573-020-0090-8
156. Davidi ES, Dreifuss T, Motiei M, Shai E, Bragilovski D, Lubimov L, et al. Cisplatin-conjugated gold nanoparticles as a theranostic agent for head and neck cancer. Head neck. (2018) 40:70–8. doi: 10.1002/hed.24935
157. Chen J, Zhang S, Zhang S, Gao S, Wang J, Lei D, et al. Mesoporous silica nanoparticle-based combination of NQO1 inhibitor and 5-fluorouracil for potent antitumor effect against head and neck squamous cell carcinoma (HNSCC). Nanoscale Res Lett. (2019) 14:387. doi: 10.1186/s11671-019-3224-3
158. Ley J, Wildes TM, Daly K, Oppelt P, Adkins D. Clinical benefit of nanoparticle albumin-bound-paclitaxel in recurrent/metastatic head and neck squamous cell carcinoma resistant to cremophor-based paclitaxel or docetaxel. Med Oncol. (2017) 34:28. doi: 10.1007/s12032-017-0884-7
159. Rahman MA, Amin AR, Wang X, Zuckerman JE, Choi CH, Zhou B, et al. Systemic delivery of siRNA nanoparticles targeting RRM2 suppresses head and neck tumor growth. J Control Release. (2012) 159:384–92. doi: 10.1016/j.jconrel.2012.01.045
160. Rioja-Blanco E, Arroyo-Solera I, Alamo P, Casanova I, Gallardo A, Unzueta U, et al. Self-assembling protein nanocarrier for selective delivery of cytotoxic polypeptides to CXCR4(+) head and neck squamous cell carcinoma tumors. Acta Pharm Sin B. (2022) 12:2578–91. doi: 10.1016/j.apsb.2021.09.030
161. Rioja-Blanco E, Gallardo A, Arroyo-Solera I, Alamo P, Casanova I, Unzueta U, et al. A novel CXCR4-targeted diphtheria toxin nanoparticle inhibits invasion and metastatic dissemination in a head and neck squamous cell carcinoma mouse model. Pharmaceutics. (2022) 14:887. doi: 10.3390/pharmaceutics14040887
162. Xue H, Ding F, Zhang J, Guo Y, Gao X, Feng J, et al. DNA tetrahedron-based nanogels for siRNA delivery and gene silencing. Chem Commun (Camb). (2019) 55:4222–5. doi: 10.1039/C9CC00175A
163. Huang C, You Q, Xu J, Wu D, Chen H, Guo Y, et al. An mTOR siRNA-Loaded Spermidine/DNA Tetrahedron Nanoplatform with a Synergistic Anti-Inflammatory Effect on Acute Lung Injury. Adv Healthc Mater. (2022) 11:e2200008. doi: 10.1002/adhm.202200008
164. Fridy PC, Rout MP, Ketaren NE. Nanobodies: from high-throughput identification to therapeutic development. Mol Cell proteomics: MCP. (2024) 23:100865. doi: 10.1016/j.mcpro.2024.100865
165. Oliveira S, Schiffelers RM, van der Veeken J, van der Meel R, Vongpromek R, van Bergen En Henegouwen PM, et al. Downregulation of EGFR by a novel multivalent nanobody-liposome platform. J Control Release. (2010) 145:165–75. doi: 10.1016/j.jconrel.2010.03.020
166. Heukers R, van Bergen en Henegouwen PM, Oliveira S. Nanobody-photosensitizer conjugates for targeted photodynamic therapy. Nanomedicine: nanotechnology biology Med. (2014) 10:1441–51. doi: 10.1016/j.nano.2013.12.007
167. van Driel P, Boonstra MC, Slooter MD, Heukers R, Stammes MA, Snoeks TJA, et al. EGFR targeted nanobody-photosensitizer conjugates for photodynamic therapy in a pre-clinical model of head and neck cancer. J Control Release. (2016) 229:93–105. doi: 10.1016/j.jconrel.2016.03.014
168. de Bruijn HS, Mashayekhi V, Schreurs TJL, van Driel P, Strijkers GJ, van Diest PJ, et al. Acute cellular and vascular responses to photodynamic therapy using EGFR-targeted nanobody-photosensitizer conjugates studied with intravital optical imaging and magnetic resonance imaging. Theranostics. (2020) 10:2436–52. doi: 10.7150/thno.37949
169. Beltran Hernandez I, Grinwis GCM, Di Maggio A, van Bergen En Henegouwen PMP, Hennink WE, Teske E, et al. Nanobody-targeted photodynamic therapy for the treatment of feline oral carcinoma: a step towards translation to the veterinary clinic. Nanophotonics. (2021) 10:3075–87. doi: 10.1515/nanoph-2021-0195
170. Hackenberg S, Scherzed A, Harnisch W, Froelich K, Ginzkey C, Koehler C, et al. Antitumor activity of photo-stimulated zinc oxide nanoparticles combined with paclitaxel or cisplatin in HNSCC cell lines. J Photochem Photobiol B Biol. (2012) 114:87–93. doi: 10.1016/j.jphotobiol.2012.05.014
171. He C, Liu D, Lin W. Self-assembled core-shell nanoparticles for combined chemotherapy and photodynamic therapy of resistant head and neck cancers. ACS nano. (2015) 9:991–1003. doi: 10.1021/nn506963h
172. Huang Z, Chen Y, Chen R, Zhou B, Wang Y, Hong L, et al. HPV enhances HNSCC chemosensitization by inhibiting SERPINB3 expression to disrupt the fanconi anemia pathway. Advanced science. (2022) 10:e2202437. doi: 10.1002/advs.202202437
173. Wang D, Xu X, Zhang K, Sun B, Wang L, Meng L, et al. Codelivery of doxorubicin and MDR1-siRNA by mesoporous silica nanoparticles-polymerpolyethylenimine to improve oral squamous carcinoma treatment. Int J nanomedicine. (2018) 13:187–98. doi: 10.2147/IJN.S150610
174. Wang CS, Chang CH, Tzeng TY, Lin AM, Lo YL. Gene-editing by CRISPR-Cas9 in combination with anthracycline therapy via tumor microenvironment-switchable, EGFR-targeted, and nucleus-directed nanoparticles for head and neck cancer suppression. Nanoscale Horiz. (2021) 6:729–43. doi: 10.1039/D1NH00254F
175. Mapanao AK, Santi M, Voliani V. Combined chemo-photothermal treatment of three-dimensional head and neck squamous cell carcinomas by gold nano-architectures. J Colloid Interface Sci. (2021) 582:1003–11. doi: 10.1016/j.jcis.2020.08.059
176. Song C, Tang C, Xu W, Ran J, Wei Z, Wang Y, et al. Hypoxia-targeting multifunctional nanoparticles for sensitized chemotherapy and phototherapy in head and neck squamous cell carcinoma. Int J nanomedicine. (2020) 15:347–61. doi: 10.2147/IJN.S233294
177. Trujillo-Alonso V, Pratt EC, Zong H, Lara-Martinez A, Kaittanis C, Rabie MO, et al. FDA-approved ferumoxytol displays anti-leukaemia efficacy against cells with low ferroportin levels. Nat nanotechnology. (2019) 14:616–22. doi: 10.1038/s41565-019-0406-1
178. Weiss J, Gilbert J, Deal AM, Weissler M, Hilliard C, Chera B, et al. Induction chemotherapy with carboplatin, nab-paclitaxel and cetuximab for at least N2b nodal status or surgically unresectable squamous cell carcinoma of the head and neck. Oral Oncol. (2018) 84:46–51. doi: 10.1016/j.oraloncology.2018.06.028
179. Adkins D, Ley J, Atiq O, Powell S, Spanos WC, Gitau M, et al. Nanoparticle albumin-bound paclitaxel with cetuximab and carboplatin as first-line therapy for recurrent or metastatic head and neck cancer: A single-arm, multicenter, phase 2 trial. Oral Oncol. (2021) 115:105173. doi: 10.1016/j.oraloncology.2020.105173
180. Hoffmann C, Calugaru V, Borcoman E, Moreno V, Calvo E, Liem X, et al. Phase I dose-escalation study of NBTXR3 activated by intensity-modulated radiation therapy in elderly patients with locally advanced squamous cell carcinoma of the oral cavity or oropharynx. Eur J cancer. (2021) 146:135–44. doi: 10.1016/j.ejca.2021.01.007
181. Bonvalot S, Rutkowski PL, Thariat J, Carrere S, Ducassou A, Sunyach MP, et al. NBTXR3, a first-in-class radioenhancer hafnium oxide nanoparticle, plus radiotherapy versus radiotherapy alone in patients with locally advanced soft-tissue sarcoma (Act.In.Sarc): a multicentre, phase 2-3, randomised, controlled trial. Lancet Oncol. (2019) 20:1148–59. doi: 10.1016/S1470-2045(19)30326-2
Keywords: head and neck squamous cell carcinoma, nanomedicine, treatment, tumor microenvironment, drug delivery
Citation: Li H-X, Gong Y-W, Yan P-J, Xu Y, Qin G, Wen W-P and Teng F-Y (2024) Revolutionizing head and neck squamous cell carcinoma treatment with nanomedicine in the era of immunotherapy. Front. Immunol. 15:1453753. doi: 10.3389/fimmu.2024.1453753
Received: 24 June 2024; Accepted: 05 November 2024;
Published: 29 November 2024.
Edited by:
Lei Tao, Fudan University, ChinaReviewed by:
Heng Dong, Nanjing University, ChinaBin Zhang, Shenzhen University, China
Sen Wang, Jining Medical University, China
Copyright © 2024 Li, Gong, Yan, Xu, Qin, Wen and Teng. This is an open-access article distributed under the terms of the Creative Commons Attribution License (CC BY). The use, distribution or reproduction in other forums is permitted, provided the original author(s) and the copyright owner(s) are credited and that the original publication in this journal is cited, in accordance with accepted academic practice. No use, distribution or reproduction is permitted which does not comply with these terms.
*Correspondence: Fang-Yuan Teng, dGVuZ2Zhbmd5dWFuMzgzQHN3bXUuZWR1LmNu; Wei-Ping Wen, d2Vud3BAbWFpbC5zeXN1LmVkdS5jbg==; Gang Qin, cWluZ19sem1jQDE2My5jb20=